DOI:
10.1039/D4EN00780H
(Critical Review)
Environ. Sci.: Nano, 2025,
12, 98-120
Nanozymes as a tool to boost agricultural production: from preparation to application†
Received
27th August 2024
, Accepted 8th October 2024
First published on 18th October 2024
Abstract
Nanozymes, as an emerging class of biomimetic enzymes, not only inherit the unique properties of nanomaterials but also endow them with catalytic functions that are similar to biological enzymes. With high designability of catalytic activity and the ability to mimic the catalytic conditions and mechanisms of biological enzymes, nanozymes progressively attract significant attention in agricultural research. This research aims to provide researchers with a comprehensive overview of this emerging tool, from preparation of nanozymes to their applications in agricultural production systems. Firstly, this review systematically summarized the selection of various elements involved in nanozyme preparation, covering both metal-based and non-metal-based materials. Secondly, it outlined the mainstream chemical and environmentally friendly nanozyme synthesis technologies, critically analyzing their advantages and limitations. Thirdly, it explored the multifaceted contributions of nanozymes within the agricultural field, encompassing enhancements in crop quality and yields, augmentation of nitrogen fixation efficiency, and stimulation of microbial activity in the plant rhizosphere, as well as the improvement of agricultural crops' resilience to environmental stresses. Finally, the research discussed the main challenges faced by nanozyme research and provided forward-looking insights for future agricultural research directions. This work significantly advances understanding of the role of nanozymes in sustainable agricultural production.
Environmental significance
The utilization of nanozymes in agricultural production holds immense potential for promoting sustainable agriculture. By enhancing crop yields, improving stress resistance, and optimizing nutrient utilization, nanozymes offer an innovative approach to address food security challenges. Their ability to modulate plant–rhizobia interactions and facilitate biological nitrogen fixation significantly reduces the need for synthetic fertilizers, thereby mitigating environmental pollution. Furthermore, nanozymes can target specific stresses, such as drought and salinity, and alleviate their impact on crops, enhancing their resilience to climate change. Additionally, the use of eco-friendly green synthesis methods ensures the environmental compatibility of these nanomaterials. Ultimately, the environmental significance of nanozymes lies in their potential to revolutionize sustainable agriculture practices and mitigate the negative impacts of conventional farming techniques on ecosystems.
|
1 Introduction
The term ‘nanozymes’ has gained increasing acceptance in the scientific community as it specifically refers to nanomaterials that possess enzyme-like catalytic activities. These nanozymes exhibit a dual capacity, embodying the distinctive attributes of nanomaterials while also replicating the catalytic conditions and operational mechanisms characteristic of biological enzymes.1 This specificity enables researchers to precisely characterize and optimize the performance of these materials in a wide range of applications, including agriculture. In the past two decades, the field of nanozymes has undergone a rapid developing trend, attracting the interest of hundreds of research teams worldwide and resulting in thousands of research papers. This trend underscores the scholars' keen interest in exploring the use of low-cost, highly controllable nanomaterials as alternatives to natural enzymes. The development of nanozymes can be roughly divided into three stages.
In the early stage (before 2007), although the term “nanozyme” had not yet emerged, related research had just begun. It was in 1997 that Dugan (1997)2 revealed that water-soluble carboxyfullerene—a derivative of C60 through malonic acid—possessed a remarkable ability to scavenge hydroxyl radicals (OH−) and superoxide anions (O2−), ultimately hindering neuronal apoptosis. Advancing a decade later, Manea (2004)3 was the first to discover that immobilizing 1,4,7-triazacyclononane/Zn2+ on gold nanoparticles (AuNPs) could efficiently cleave phosphodiester bonds. Compared to free metal complexes, this immobilized catalyst exhibited higher activity, demonstrating enzyme-like characteristics. This discovery further propelled the field forward, underscoring the temporal progression and growing sophistication in the development of nanozymes. From 2007 to 2013, nanozyme research made significant progress, entering a second phase of development. Yan (2007)4 observed that iron oxide nanoparticles (Fe3O4 NPs) possessed peroxidase-like (POD) activity similar to horseradish peroxidase, which could induce substrate coloration. Although the term “nanozyme” was not directly mentioned in the paper, the authors described these nanoparticles as having “intrinsic POD-like activity,” marking a rapid development stage in nanozyme research. During this period, various inorganic nanomaterials with diverse nanozyme activities were reported. However, the term “nanozyme” was often used loosely in the literature to describe immobilized catalysts, immobilized enzymes, or encapsulated enzymes, where the catalytic activity originated from small molecule catalysts or enzymes. Since 2013, nanozyme research has entered a synthetic design phase, showing a more rapid growth trend. Numerous research papers have reported inorganic nanomaterials with various nanozyme activities that can mimic the catalytic reactions of natural enzymes, with exciting prospects for biosensing and therapeutics. As research progressed, the term “nanozyme” increasingly referred to nanomaterials with specific catalytic functions, gradually diverging from the direct association with the concept of “enzyme.” Thus, in 2013, nanozymes were clearly defined for the first time as nanomaterials with enzyme-like characteristics.1 Although this definition facilitated research on nanomaterials as enzyme alternatives, the specific similarities between enzymes and nanozymes remained vague, and scholars anticipated discovering structural or functional analogues between the two.5 By 2021, the definition of nanozymes was further refined as “nanomaterials that catalyze the conversion of enzyme substrates to products and follow enzymatic kinetics (e.g., Michaelis–Menten) under physiologically relevant conditions, even though the molecular mechanisms of the reactions could be different between nanozymes and the corresponding enzymes”.6 However, some scholars still pointed out that the boundary of the term “nanozyme” remains somewhat fuzzy, and its definition may continue to evolve and improve in the future.7
Nanozyme technology originated in the field of immobilized catalysis. However, its application in agriculture is still in the early stages of exploration, and a unified and clear definition in the agricultural field has not yet been formed. To enhance the in-depth understanding of the latest achievements in agricultural nanozyme research, this paper systematically reviewed the main types of nanozymes, preparation techniques, and their impact on agricultural production. While doing this, this review first summarized the materials involved in nanozyme preparation, including non-metallic, monometallic, bimetallic, and multi-metallic materials, as well as a series of innovative substances. Subsequently, this paper delved into the chemical synthesis pathways and green synthesis strategies of nanozymes. Finally, this review summarized the application prospects of nanozymes in agricultural production, highlighting their potential capabilities and mechanisms in improving crop yields and quality, enhancing crop stress resistance, promoting rhizosphere microbial activity, and improving biological nitrogen fixation efficiency. The overarching aim of this work is to provide a valuable reference for the in-depth research and application of nanozymes in the agricultural field.
2 Types of nanozymes
2.1 Non-metallic nanozymes
Non-metallic nanozymes, identified as a distinct group of nanozymes, are distinguished by their active centers that operate independently of metal ions, thus showcasing unique functions and effects in the catalytic process.8,9 This group mainly consists of carbon-based nanomaterials, including carbon nanotubes, graphene, graphene oxide, carbon quantum dots, and graphene quantum dots.10 With their outstanding physicochemical properties, these materials are found to have broad application prospects in diverse sectors such as agriculture, energy science, environmental science, and catalytic science.11–13 In agriculture, carbon-based nanozymes have attracted significant attention and have been identified to possess antioxidant-like properties, indicating their potential value in mitigating a range of abiotic and biotic stresses affecting agricultural production. It has been reported that concentrations of 20 mg L−1 of single-walled carbon nanotubes (SWNTs) and multi-walled carbon nanotubes (MWNTs) can serve as antioxidant enzymes, regulating the activity of superoxide dismutase (SOD) and POD in tomatoes.14 Similarly, graphene oxide has been found to exhibit enzyme-like activity, specifically in its ability to interact with various reactive oxygen species, such as O2−, H2O2, and OH−. This activity enabled it to reduce excess malonic dialdehyde (MDA) in tomatoes.15 To improve the stability of non-metallic nanozymes, modifications in their electronic structure and catalytic activity are often made by doping with non-metallic atoms such as nitrogen (N), boron (B) and selenium (Se). For example, N-doped carbon quantum dot nanozymes have been prepared using electrochemical methods, exhibiting strong antioxidant properties and increasing the tolerance of Arabidopsis to cadmium.16 Furthermore, N-functionalized carbon nanospheres synthesized through hydrothermal methods have been shown to mimic the activity of catalase (CAT), effectively maintaining mitochondrial membrane potential and preventing lipid peroxidation and DNA damage, thus significantly reducing the toxicity due to oxidative stress in cells.17 These findings not only provide new insights into the preparation of CAT-like nanozymes but also support the development of engineered non-metallic carbon nanozymes with similar enzymatic activities. An innovative approach involving an amine-functionalized fullerene derivative, named the C70-(EDA)8 nanozyme, has demonstrated excellent reactive oxygen species (ROS) scavenging ability and the capacity to modulate immune responses.18 Additionally, Se-doped carbon quantum dots (Se-CQDs) have been found to excel in ROS scavenging, highlighting their potential in protecting biological systems from oxidative stress damage.19 A novel nitrogen/iodine co-doped carbon dot (N/I-CD) synthesized using a one-step hydrothermal method has been shown to exhibit exceptional POD-like activity.20 Moreover, the development of polymer synthesis methods for non-metallic nanozymes has recently gained momentum. For instance, a bio-inspired polydopamine nanozyme was developed and its multiple antioxidant mechanisms were explored, revealing its high catalytic activity against various reactive oxygen and nitrogen species, such as O2−, H2O2, OH−, and NO.21 Collectively, these studies contribute to advancing the field of non-metallic nanozymes and expand the possibilities for their application in areas including biomedicine and environmental protection.22
2.2 Single metal-based nanozymes
Transition metal elements, particularly those based on Fe, are capable of forming coordination bonds with substrate molecules via their empty d or f orbitals, effectively lowering the activation energy of reactions and thus accelerating chemical processes.23,24 Such properties showcase their immense potential in the nanozyme field.25 One of the examples is the POD-like function of Fe3O4 nanozymes in plants. Some studies have demonstrated significant reductions in H2O2 levels in barley (Hordeum vulgare L.) when exposed to high concentrations of these nanozymes, suggesting their POD-like properties.26 Further research confirmed that smaller Fe3O4 nanozymes could effectively scavenge excess ROS in soybean nodules, enhancing nitrogenase activity.27 The structure of nanozymes is known to significantly affect their electronic structure and transport properties, which in turn influences their chemical reactivity and surface energy, ultimately impacting their catalytic activity.28,29 Various morphologies of Fe3O4 nanozymes, such as nanoclusters, nanoflowers, and nanodiamonds, prepared through hydrothermal reactions, have shown that the nanostructure markedly affects the POD-like catalytic activity, with nanoclusters exhibiting the highest performance.30 The surface modification of Fe3O4 nanozymes has also been a significant area of research, illustrated by enhancements in catalytic activity through surface modifications such as the introduction of histidine, which has increased catalytic efficiency by twentyfold.31 Collectively, these studies underscore the need for focused research on surface modification and functionalization strategies to enhance the catalytic activity and selectivity of Fe3O4 nanozymes, aiming for a more efficient simulation of natural enzyme functions in applications across agriculture and biomedicine.
Unlike the field of catalysis where Fe dominates, magnetic elements like cobalt (Co) and nickel (Ni) have received less attention in agriculture. The essential role of Fe in biological processes such as oxygen transport and energy generation, coupled with the strong reactivity of iron oxides, especially under acidic soil conditions, underscores its prevalence.32,33 However, some advancements have been made in the research on Co and Ni-based nanozymes. Initial studies on Co3O4 nanozymes revealed their inherent POD-like and CAT-like activities, attributed to their electron transfer capabilities.34 Recent experiments demonstrated the antioxidant enzyme-like properties of Co3O4 nanozymes in soil pot experiments, though high concentrations also triggered oxidative damage in rapeseed.35 Similarly, NiO nanozymes synthesized using a solid-state microwave radiation method significantly increased antioxidant enzyme activities in soybeans, although they also initiated a stress response, leading to elevated levels of MDA and H2O2.36 These results highlight the need for further research and optimization in the use of Co and Ni-based nanozymes in biological systems.
In addition to the aforementioned magnetic element nanozymes, single-metal oxide nanozymes such as copper (Cu), zinc (Zn), and manganese (Mn) have also garnered significant attention. These nanozymes not only exhibit excellent catalytic efficacy but also enhance plants' antioxidant defense systems and improve crop yields and quality when applied as nanozyme forms of essential micronutrients in agriculture. For instance, ZnO nanozymes applied via foliar spray have proven effective in alleviating oxidative damage in tomatoes caused by iron deficiency and promoting nutrient accumulation.37 CuxO nanoclusters, prepared through a phenylalanine-mediated method, have shown the ability to mimic the functions of several natural enzymes, demonstrating their broad potential across multiple fields.38 Manganese oxide derivatives, including MnO, MnO2, Mn2O3 and Mn3O4, have gained interest due to their high catalytic activity, ease of control, and stability. Recent studies on Mn3O4 nanozymes synthesized via a hydrothermal method revealed their potential to enhance resistance to salt stress in cucumbers by increasing levels of endogenous antioxidant metabolites.39 These findings suggest the broad applicability of Mn3O4 nanozymes as nanoregulatory agents, helping plants withstand oxidative stress.
2.3 Bimetallic nanozymes
Bimetallic nanozymes are increasingly preferred by researchers due to their enhanced selectivity, activity, and stability.40,41 In the field of agriculture, studies focus primarily on the synthesis and application of bimetallic magnetic transition metal elements and their oxides. Research indicated that Co-doped Fe3O4 nanozymes exhibited remarkable POD-like activity, enhancing catalytic efficiency by up to 100 times due to their strong affinity for substrates.42 Additionally, various MnFe2O4 nanozymes have been prepared using a simple co-precipitation method, demonstrating a close correlation between nanozyme catalytic activity and their structure, with the octahedral shape showing superior activity compared to nanosheets and nanowires.43 Numerous studies provide compelling evidence for the catalytic activity of bimetallic magnetic nanozymes.44–47 In practical agricultural applications, either mixing these nanozymes with seeds or applying them as foliar sprays can effectively alleviate abiotic stresses in crops, thereby improving yields and quality. For example, MnFe2O4 nanozymes used as carriers in hydroponics have significantly enhanced POD activity in the leaves and roots of barley, providing a functional role in agriculture.48 Similarly, CoFe2O4 nanozymes have significantly affected ROS levels in tomato leaves and roots.49 Recent studies focused on a composite manganese–iron oxide (MnFe2O4) in tomatoes have shown that foliar application of these nanozymes can significantly improve nutritional quality, yields, and resistance to pests and diseases, highlighting their potential application value in agriculture.50 While this review does not detail the application of nanozymes in pesticide detection and photodegradation, interested readers can refer to specific literature for a deeper understanding.51–55 Moreover, there is significant research on nanozymes composed of other bimetallic elements. Cu0.89Zn0.11O nanozymes synthesized by doping CuO with Zn enhanced the POD-like catalytic activity compared to undoped-CuO.56 The enhanced enzymatic activity of nanozymes containing both precious and non-precious metals was primarily due to the synergistic properties of the metals, which impart superior functionality to the nanozymes.57 As much of this research remains in the experimental phase, there is a need for systematic methods to translate these findings into practical agricultural applications. This involves leveraging the catalytic capabilities of these materials while ensuring their safety and environmental sustainability. Future research should focus on advancing the practical applications of these nanozymes, particularly in agriculture, to provide new solutions for green and efficient agricultural production.
2.4 Multi-metallic nanozymes
For many chemical reactions, single-metal catalysts often face challenges in achieving both high selectivity and high yields simultaneously, which has led researchers to explore multi-metallic catalysts. Multi-metallic nanozymes, characterized by their unique catalytic centers consisting of composite systems of multiple metal ions, exhibit exceptional catalytic activity and stability due to the synergistic effects between the metals, offering new avenues to overcome these challenges.58–60 An innovative approach was demonstrated in 2018 with the proposal of a bionic nanoflower design based on self-assembled nanozymes. In this research, PtCo nanozymes were synthesized and used to promote the growth of MnO2, ultimately forming MnO2@PtCo nanoflowers with a distinctive structure. By finely adjusting the proportion of reactants, the catalytic efficiency of these nanoflowers was enhanced while maintaining their structural integrity and orderliness. During this process, the PtCo component exhibited POD-like activity, while MnO2 showed CAT-like activity.61 Additionally, in 2021, a study reported on a Pt/Pd/Mo nanozyme characterized by redox capabilities and a uniform hollow cubic nanostructure with face-centered cubic features. This nanozyme not only displayed high catalytic activity but also enhanced the selectivity of nanozymes in physiological environments. Further mechanistic studies suggested that lattice deformation and the exposure of highly active sites were key factors in improving catalytic performance.62 However, research on multimetallic nanozymes in the field of agriculture has not progressed as rapidly. The continuous exploration and refinement of fundamental scientific issues and technical preparation methods remain as limiting factors. Moreover, the complexity of interactions between metal ions in multimetallic nanozymes poses challenges to their catalytic activity and stability. Therefore, conducting in-depth research on the composition and structure of multimetallic nanozymes is crucial for optimizing and regulating their catalytic performance.63 Meanwhile, the achievements of single and bi-metallic nanozymes in agricultural applications have influenced the progression of multimetallic nanozymes in this sector to some extent.64–66 Looking ahead, as research on multimetallic nanozymes deepens, more innovations and breakthrough applications are anticipated in the agricultural sector. Table 1 provides a comprehensive overview of the characteristics of various nanozyme types, highlighting their strengths in catalytic activity, stability, and application potential. It also points out the drawbacks such as preparation complexity, cost, and potential environmental and health risks.
Table 1 Comparison of nanozyme types: Non-metallic, single metal-based, bimetallic, and multi-metallic nanozymes
Nanozyme type |
Specific examples |
Mode of action |
Advantages |
Disadvantages |
Non-metallic nanozymes |
Carbon nanotubes, graphene, graphene QDs, etc. |
Interact with ROS to exhibit antioxidant enzyme-like activity |
1. Excellent physicochemical properties |
1. Potential environmental risks |
2. Good biocompatibility |
2. Complex preparation processes |
3. Performance can be enhanced through doping |
Single metal-based nanozymes |
Fe3O4, CuO, ZnO, MnO2, etc. |
Mimic natural enzyme catalytic processes, such as peroxidase-like and catalase-like activities |
1. Relatively simple structures |
1. Potential toxicity to biological systems |
2. High catalytic activity |
2. Potentially high costs |
3. Applicable for various agricultural applications |
Bimetallic nanozymes |
MnFe2O4, CoFe2O4, Cu0.89Zn0.11O, etc. |
Utilize synergistic effects between two metals to enhance catalytic activity and stability |
1. Higher catalytic activity |
1. More complex preparation processes |
2. Better stability |
2. Potentially higher costs |
3. Stronger selectivity |
Multi-metallic nanozymes |
Pt/Pd/Mo, PtCo@MnO2 nanoflowers, etc. |
Achieve high catalytic activity and stability through synergistic effects of multiple metal ions |
1. Extremely high catalytic activity |
1. Extremely complex preparation processes |
2. Unique nanostructures |
2. High costs |
3. Broad application potential |
3. Insufficient mechanistic understanding |
3 Nanozyme synthesis
3.1 Strategies for green synthesis of nanozymes
In the green synthesis of nanozymes, the primary extracts used include those derived from plants and microorganisms. Plant extracts are particularly favored due to their environmental friendliness and sustainability.67 For example, 10–13 nm ZnO nanozymes were successfully synthesized using leaf extracts from tomato (Lycopersicon esculentum), orange (Citrus sinensis), grapefruit (Citrus paradisi), and citron (Citrus aurantifolia) as reducing agents, with Zn(NO3)2 serving as the precursor. These particles exhibited polyhedral structures.68 Microorganisms, such as bacteria and fungi, are also utilized in nanozyme synthesis due to their complex metabolic pathways that facilitate the reduction of metal ions into nanoparticles, offering a diverse synthesis toolkit. This capability has garnered significant attention, exemplified by the preparation of spherical ZnO nanozymes ranging from 20 to 100 nm in diameter using Acinetobacter schindleri (SIZ7) as a reducing agent.69 Additionally, algae extracts are noted for their biocompatibility, suggesting that nanoparticles synthesized from algae exhibit lower toxicity to organisms and the environment. Using extracts from seaweed, variously structured ZnO nanozymes such as spherical, triangular, radial, hexagonal, rod-shaped, and rectangular were green-synthesized, demonstrating an average particle size of 36 nm. Notably, the water-soluble pigments in these extracts play a crucial role in the formation and stabilization of the ZnO nanozymes, maintaining particle dispersion without significant aggregation over six months.70
When selecting plant extracts, fungi, or algae for the green synthesis of nanoparticles, it is essential to consider factors such as the characteristics of the target nanomaterials, synthesis efficiency, extraction and cultivation conditions, cost, sustainability, application areas, stability, storage, and regulatory requirements. This approach ensures the economic feasibility and environmental friendliness of the synthesis process. In addition to ZnO nanozymes, significant progress has also been made in exploring other nanozymes such as CuO,71 Ag,72 Fe2O3,73 and MgO.74 There have been numerous reports on the green synthesis of nanozymes mediated by fungi and yeast,75–78 as well as viruses.79–81
The steps involved in the green synthesis of nanozymes are illustrated in Fig. 1. The current knowledge of mechanisms behind formation of metal oxide nanozymes during green synthesis is still incomplete. Based on existing studies,82,83 it is speculated that certain reducing components, such as polyphenols, alkaloids and biotin, in green synthesis extracts can reduce metal salts to a zero-valent state upon contact.84–86 Subsequently, these zero-valent metals can be converted into metal oxide nanozymes through heating, oxidation, etc., thereby adjusting the surface properties and structure of the particles. During this process, the morphology and size of the particles are influenced by various factors such as the extract type, temperature, pH, and solvent nature. By adjusting these factors, effective control over the morphology and particle size can be achieved. It's worth noting that metal oxide nanozymes may aggregate during the green synthesis process, which can affect their performance. Fortunately, certain organic compounds in the extracts can act as dispersants, effectively preventing aggregation and enhancing the stability of nanozymes.
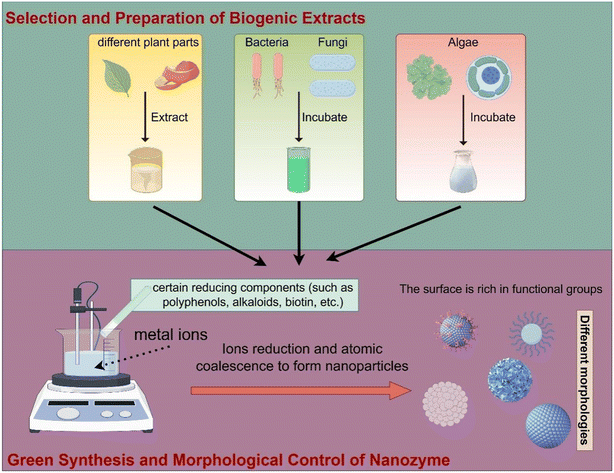 |
| Fig. 1 Green synthesis and morphological control of nanozymes: selection and preparation of biogenic extracts. | |
3.2 Chemical synthesis strategies for nanozymes
Although green synthesis of nanozymes offers advantages in terms of environmental friendliness and sustainability, chemically synthesized nanozymes continue to dominate in various fields due to their high yield, superior performance, and extensive diversity of forms and functionalities. The specific strengths of chemical synthesis are reflected in the following aspects: firstly, it enables precise process control and large-scale production; secondly, the flexible adjustment of reaction conditions can effectively tailor the properties of nanozymes; thirdly, it is suitable for the preparation of multiple types of nanozymes.
Prior to 2010, the focus was on establishing basic synthesis methods. During this phase, researchers systematically investigated and established the foundational synthesis approaches for nanozymes. Basic chemical synthesis methods such as hydrothermal, solvothermal, and vapor deposition methods were extensively used in the preparation of nanozymes.87–89 These methods allowed for precise control over the size, shape, and structure of nanomaterials, significantly boosting the catalytic performance of nanozymes. As chemical synthesis techniques have progressed, researchers have gained even greater mastery over the morphology, dimensions, and architecture of nanozymes, tailoring them to suit a wide range of applications. For instance, ternary ZnO/CuO/Co3O4 nanozymes were synthesized in a one-step process using nitrates and chlorides as raw materials by adjusting the pH (Fig. 2A).90 Another example includes the preparation of Mn3O4 nanozymes using a hydrothermal precipitation method91 (Fig. 2B).
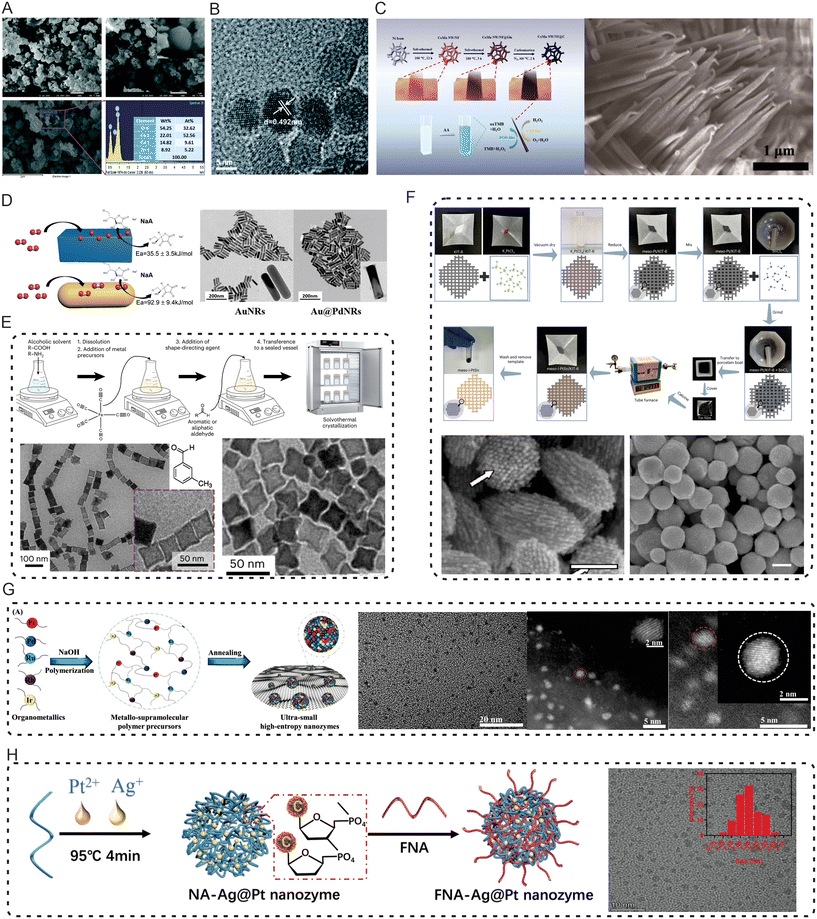 |
| Fig. 2 The chemical synthesis of nanozymes. (A) One-step wet chemical synthesis of ternary ZnO/CuO/Co3O4 nanozymes via pH adjustment. Reproduced with permission from ref. 90. Copyright 2019, The Royal Society of Chemistry. (B) Preparation of Mn3O4 nanozymes via a hydrothermal precipitation method. Reproduced with permission from ref. from ref. 91. Copyright 2018, The Royal Society of Chemistry. (C) Preparation of carbon-shielded three-dimensional Co–Mn nanowire arrays on nickel foam (CoMn NW/NF@C) through solvothermal and thermal annealing processes. Reproduced with permission from ref. 45. Copyright 2019, American Chemical Society. (D) Using Au nanorods (Au NRs) as a strong plasmonic core, a thin layer of Pd is coated to form a rod-shaped core–shell structure to obtain Au@Pd NRs. Reproduced with permission from ref. 92. Copyright 2019, American Chemical Society. (E) Solvothermal-based preparation method for gram-scale production of nanocube and nanoferrite nanozymes. Reproduced with permission from ref. 93. Copyright 2023, Springer Nature. (F) Preparation of ordered mesoporous intermetallic nanomaterials with controllable composition, morphology/structure, and phase through a versatile, synchronous templating strategy. Reproduced with permission from ref. 94. Copyright 2023, Springer Nature. (G) Construction of ultra-small high-entropy alloy nanozymes. Reproduced with permission from ref. 95. Copyright 2023, John Wiley and Sons. (H) Design and synthesis of NA-Ag@Pt nanozymes and FNA-Ag@Pt nanozymes through rapid one-step self-assembly driven by nucleic acid and metal ion coordination. Reproduced with permission from ref. 96. Copyright 2023, John Wiley and Sons. | |
As research has advanced, the refinement of chemical synthesis methods has further enabled meticulous manipulation of nanozyme characteristics to cater to the varying requirements of different application contexts. These advancements have allowed for the creation of highly specific nanozyme structures tailored to enhance catalytic functions. For instance, a solvothermal and annealing process was utilized to fabricate a carbon-shielded three-dimensional Co–Mn nanowire array on nickel foam, which exhibited peroxidase-like and catalase-like activities (Fig. 2C).45 Similarly, peroxidase-active rod-shaped core-shell structured Au@Pd nanorods were developed using gold nanorods as the core, which were then coated with a thin palladium layer to enhance their enzymatic activity.92 These examples demonstrate the potential of chemical synthesis to produce nanozymes with precise functionalities tailored to specific applications (Fig. 2D).
The field of synthetic technology for nanozymes has now reached an advanced stage, marked by the rapid progression of nanoscience and nanotechnology. This development has ushered in more sophisticated synthesis techniques, significantly enhancing the preparation of nanozymes. Techniques such as templating, microwave-assisted synthesis, and ultrasonic treatment are now employed to produce nanozymes with specific morphologies and properties, thus improving their quality and catalytic efficiency. For example, a solvothermal method has been developed for the large-scale production of iron oxide nanoparticles, featuring a variety of morphologies such as nanocubes and nanostars93 (Fig. 2E). Additionally, the fabrication of crystalline mesoporous materials has made notable progress due to their capacity to expose more active sites, facilitate proton and electron transfer, and create a unique mesoporous nano-confined environment. Recent innovations include a universal and synchronous templating strategy to prepare ordered mesoporous metal-intercalated nanomaterials with a controllable composition, morphology, structure, and phase94 (Fig. 2F). Furthermore, the development of ultra-small high-entropy alloys has been explored, with studies focusing on their enzyme-like catalytic activity. This research has led to the synthesis of high-entropy nanozymes using a scalable metal–ligand crosslinking reaction, which demonstrated exceptional peroxidase-like activity by catalyzing the generation of highly toxic hydroxyl radicals from endogenous hydrogen peroxide95 (Fig. 2G). Significant advancements have also been made in the field of self-assembled nanozymes. Innovative bio-inspired nanoflowers were developed, synthesizing PtCo nanozymes to guide the growth of MnO2, thus forming highly catalytically efficient MnO2@PtCo nanoflowers.61 More recently, novel nanozymes with significant peroxidase-like and antibacterial effects were designed and synthesized using a one-step rapid self-assembly process driven by the coordination between nucleic acids and metal ions. These nanozymes showed an enzyme activity approximately eight times greater than those produced by traditional chemical reduction methods.96
Looking ahead, with the rise of artificial intelligence and machine learning technologies, researchers have begun applying these techniques to optimize the synthesis of nanozymes. By establishing a nanozyme database and utilizing machine learning algorithms, researchers can more accurately predict and design nanozymes with excellent catalytic performance. This intelligent synthesis approach provides a new pathway for the efficient preparation and application of nanozymes.
4 Application of nanozymes in agriculture: enhancing crop yields and quality
The application of nanozymes has emerged as a hotspot in agricultural research, paving new avenues for boosting crop yields and quality. As a pivotal technology in this domain, the dual role of nanozymes in augmenting both crop quality and yields has garnered widespread attention. The amalgamation of nanotechnology and agriculture has facilitated precise and controllable fertilizer release, ensuring a sustained and balanced nutrient supply for crops throughout their growth cycle.97–99 This aspect is particularly prominent in horticultural crops.100–103
Studies indicated that various nanozymes based on the use of Cu,104 ZnO,105 SiO2,106 TiO2,107 Fe3O4,27 Mo,108 CeO2,109 and MgO110 effectively enhance photosynthesis and energy conversion efficiency in plants.111–113 This enhancement translates into higher crop yields and improved quality. When applied either to the soil or as a foliar spray, nanozymes outperform traditional fertilizers in several ways. They contribute to better soil conditions. Furthermore, these nanozymes promote the uptake of essential micronutrients and macronutrients by crops, thereby significantly improving nutrient use efficiency, accelerating crop growth, and ultimately resulting in a substantial yield increase.114–116 Upon analysis of the cumulative impacts of nanozyme applications on crop productivity, a discernible trend becomes evident. On an average basis, nanozyme treatments have been observed to enhance crop yields by approximately 10–40% in comparison with untreated controls (Table 2). This increase is contingent upon factors such as the specific nanozyme type, crop species, and the mode of application. As depicted in Table 2, ZnO nanozymes have exhibited exceptional efficacy in augmenting yields, ranging from a modest 2.41% enhancement in tomatoes to a substantial 65.12% improvement in lentils, contingent upon the crop variety and application methodology. Similarly, Fe3O4 nanozymes have demonstrated significant yield advancements, particularly in wheat (with a 10.11% increase) and soybean (exhibiting a 40.12% enhancement). Furthermore, the application of CuO, MgO, and other metal-based nanozymes has resulted in notable yield improvements, underlining the versatility and broad applicability of nanozymes in agricultural practices. The biofortification effects of nanozymes on crops are remarkably significant, especially in improving crop quality by increasing the content of micronutrients.117–119 Scholars have further observed that nanozymes can promote plant growth and development by regulating plant growth hormone levels, comprehensively enhancing crop yields and quality.120–122
Table 2 Effects of nanozymes on increasing yields and improving quality of different crops
Nanoenzyme |
Crop type |
Application method |
Concentration |
Yield increase |
Quality improvement |
Study duration |
Reference |
ZnO |
Rice |
Soil application |
0.3–4.8 Zn per pot |
Panicle number +4.83–13.14% |
Increased amylose content and protein content |
46 days |
123
|
ZnO |
Lentil |
Foliar application |
1 mg L−1 |
Seed yield +65.12% |
Increased seed trace elements |
60 days |
124
|
ZnO |
Dragonhead |
Foliar application |
160 mg L−1 |
Oil yield +80% |
More oil yield |
4 months |
125
|
ZnO |
Tomato |
Foliar application |
100 mg L−1 |
Fruit yield +2.41% |
Increased TSS, fruit firmness, and titratable acidity |
25 days |
126
|
ZnO |
Soybean |
Soil application |
200 mg L−1 |
Pod +36.2% |
Improved seed yield, seed P and K, and oil and protein yield |
120 days |
127
|
ZnO |
Rice |
Soil application |
25, 100 mg L−1 |
Total biomass +6.8–7.6% |
— |
2 years |
128
|
ZnO |
Grapes |
Foliar application |
0–250 mg L−1 |
— |
Increased total and reducing sugars; improved red coloration |
1 year |
129
|
ZnO |
Tomato |
Root dipping |
10–200 mg L−1 |
— |
Increased β-carotene and lycopene content |
60 days |
130
|
ZnO |
Rice |
Foliar application |
0.75–12.00 kg hm−2 |
Grain yield +2.3–4.1% |
Enhanced zinc and aroma content |
2 years |
131
|
ZnO |
Wheat |
Soil application |
5 kg ha −1 |
Dry weight +40% |
Increased protein content |
90 days |
132
|
Fe3O4 |
Wheat |
Foliar application |
1.37 g L−1 |
Grain yield +10.11% |
Increased starch, sugar and protein content |
2 years |
133
|
Fe3O4 |
Cucumber |
Foliar application |
100–300 mg L−1 |
— |
Increased starch, soluble protein, sugar and oil content |
180 days |
134
|
Fe3O4 |
Muskmelon |
Irrigated |
100, 200 mg L−1 |
Weight +11.50% |
Increased soluble proteins and VC |
30 days |
135
|
Fe3O4 |
Soybean |
Foliar application |
100, 200 mg L−1 |
Grain yield +40.12% |
Increased oil content |
120 days |
136
|
Fe3O4 |
Strawberry |
Irrigated |
0.06, 6 mg L−1 |
Yield +77.37% |
Increased starch and soluble proteins |
120 days |
137
|
Fe3O4 |
Broad bean |
Foliar application |
120 mg g−1 |
— |
Increased starch, proteins, and amino acid |
120 days |
138
|
Fe2O3 |
Raphanus sativus
|
Foliar application |
6.5–13 mg plant−1 |
Biomass +30% |
Increased VC |
42 days |
139
|
Fe2O3 |
Tomato |
Irrigated |
0, 50, 100 mg L−1 |
Yield +11% |
— |
70 days |
140
|
Fe2O3 |
Soybean |
Foliar application |
10 mg L−1 |
Yield +13.7% |
Increased amino acid |
100 days |
27
|
Fe |
Washington navel orange |
Foliar application |
0.1% |
Yield +32% |
Increased TSS |
1 year |
141
|
CuO |
Tomato |
Irrigated |
1 mg kg−1 |
Yield +12.2% |
Increased starch, microelements, and VC |
45 days |
104
|
MgO |
Cotton |
Foliar application |
20–100 mg kg−1 |
Yield +42% |
Improved fiber quality |
90 days |
142
|
MgO |
Mustard |
Seed exposure |
10–150 mg kg−1 |
Grain yield +6 times |
Increased starch and proteins |
30 days |
110
|
MgO |
Date palm |
Foliar application |
1 g L−1 |
— |
Increased proteins and TSS |
— |
143
|
MgO |
Carrot |
Molecular docking |
50–100 mg L−1 |
Fresh weight +20.46% |
Increased amino acid |
90 days |
144
|
MgO |
Cowpea |
Foliar application |
200 mg L−1 |
Shoot dry weight +45.09% |
Increased starch and proteins |
42 days |
145
|
MoS2 |
Soybean |
Soil application |
10 mg kg−1 |
Yield +30% |
— |
120 days |
108
|
MoO3 |
Soybean |
Foliar application |
10–40 mg L−1 |
Grain yield +84.1% |
Increased proteins |
2 years |
146
|
CeO2 |
Cabbage |
Soil application |
100 mg L−1 |
Yield +3.68 times |
— |
75 days |
147
|
Ag |
Wheat |
Soil application |
20–2000 mg kg−1 |
Grain yield −23.7% |
Decreased amino acid |
120 dys |
148
|
Carbon |
Corn |
Foliar application |
100–400 mg L−1 |
Shoot dry weight +27.1% |
Increased microelements |
45 days |
117
|
Se |
Corn |
Foliar application |
2, 20 mg L−1 |
— |
Increased starch and amino acid |
— |
149
|
The average increase in yields observed across various crop species and nanozymes indicates that the application of nanozymes can constitute an effective approach for enhancing agricultural productivity. The observed variability in yield enhancements emphasizes the necessity of optimizing the type, concentration, and method of nanozyme application according to specific crop species and growth conditions. Looking ahead, further research is required to refine nanozyme formulations and application strategies, thereby maximizing their effectiveness in a range of agricultural contexts. By achieving this, we can fully exploit the potential of nanozymes to sustainably elevate crop yields and quality, ultimately ensuring global food security amidst growing population demands and environmental challenges.
5 Enhancing crop stress resistance through nanozyme applications
As global climate change and environmental challenges intensify, enhancing crop stress resistance becomes a critical priority. Abiotic stresses such as drought, salinity, or flooding cost agriculture over $180 bln p.a. in lost productivity,150 and are only going to increase, given the current climate trends. The rapid development of nanotechnology, particularly the application of nanozymes, has introduced innovative approaches for boosting crop resilience.151 Recent studies have demonstrated significant advancements in using nanozymes to reduce crop abiotic stress by improving drought and salt tolerance, and mitigating toxic effects of heavy metals.152–157 The initial stimulus plays a crucial role in triggering the crop's resistance mechanism against various abiotic stresses.158 In this regard, ROS are especially critical as key signaling molecules in the crop's response to abiotic stress. For instance, research has shown that under drought conditions, polyacrylic acid-modified Mn3O4 nanozymes can enhance the mitotic ability of maize root tip cells by maintaining ROS balance, thus improving the crop's drought resistance.159 Additionally, these nanozymes can alleviate salt stress in cotton by eliminating excess ROS accumulation in leaf cells and maintaining optimal cellular K+/Na+ balance, enhancing its salt tolerance.160 In addressing heavy metal stress, the primary strategy involves eliminating ROS and activating antioxidant defense mechanisms to reduce oxidative damage. For example, TiO2 nanozymes can minimize DNA damage by reducing total chromium accumulation and decreasing the production of H2O2 and MDA, thereby mitigating the toxicity of chromium(VI).161 Furthermore, the efficient nutrient delivery mechanism of nanozymes enhances nutrient absorption in crops, reduces nutrient loss, and promotes growth and development under stressful conditions. For example, research has highlighted the significant impact of Cd stress on tobacco's metabolism and ion balance, leading to severe impairment of seedling growth. The external application of Fe3O4 and ZnO nanozymes has been found to enhance plant nutrient absorption, reconstruct vital metabolic processes, and stimulate plant growth.162 Similarly, under salt stress conditions, Si nanozymes have been shown to enhance the utilization efficiency of nutrients such as phosphorus and potassium, thus promoting corn growth.163 These findings not only underscore the vast potential of nanotechnology in enhancing crop stress resistance but also provide new perspectives and methodological guidance for future advancements in agricultural science.
Nanozymes may also play an important role in mitigating biotic stresses, particularly in resisting diseases and pests, although this research will not extensively cover their general applications. Nanozymes used as pesticides can be broadly categorized into two types. The first type activates the plant's natural defense systems, induces systemic acquired resistance (SAR), regulates plant hormone signaling, and enhances antioxidant capacity. This effectively alleviates oxidative stress caused by pathogens, including bacteria, fungi, and viruses. Research has shown that SiO2 nanozymes can activate plants' SAR, enhancing their resistance to pathogens.164 Additionally, a water-soluble CMCS-coupled DA nanozyme (DA@CMCS) has been developed, which significantly boosts the activity of plant antioxidant enzymes such as CAT, polyphenol oxidase (PPO), and SOD. DA@CMCS also activates the SAR-related PR1 gene and the disease resistance-related NPR1 gene, while suppressing the expression of the repressor gene JAZ3 in the jasmonic acid pathway.165 On the other hand, some specific nanozymes possess direct antibacterial effects, effectively inhibiting the growth and reproduction of pathogenic bacteria. For instance, it has been found that CuO nanozymes can significantly disrupt the reproductive processes of certain fungi, including inhibiting hyphal growth, spore germination, and sporangium formation.166 Additionally, Ag nanozymes are notable for their broad spectrum of antibacterial mechanisms and are widely utilized as antibacterial agents. This is primarily due to their ability to interact with bacterial DNA, causing chain breaks that disrupt key cellular functions and target various bacterial pathogens.167,168
The second type of nano-pesticide currently dominates research and industrialization primarily due to its notable advantages in controlling drug release, targeting specific locations, and reducing environmental impact. A temperature-responsive copolymer, poly(2-(dimethylamino)ethyl methacrylate)-b-poly(ε-caprolactone) (PDMAEMA-b-PCL), has been employed as a carrier using transient nanoprecipitation technology to successfully prepare a nano-pesticide formulation. This formulation is characterized by its temperature responsiveness, excellent leaf adhesion, and enhanced drug loading capacity.169 Additionally, nano-pesticides with dual-responsiveness to glutathione (GSH) and pH have been developed. For example, a formulation in which chlorfluazuron (CHL) and spinosad (SPI) were dual-loaded demonstrated impressive pesticide loading capacity (up to 52.03%), strong adhesion to corn leaves (reaching 87.63%), and improved pesticide release behavior under GSH and alkaline conditions.170 These attributes significantly enhance the utilization efficiency of dual-stimulus-responsive pesticides.
Furthermore, an innovative nano-platform (NIT@MON@CuS) capable of responding to redox and near-infrared light stimuli for pesticide delivery has been designed. This platform consists of CuS nanoparticles and a metal–organic network (MON), successfully loaded with neonicotinoid insecticides (NIT). With an average size of 190 nm and a loading efficiency of up to 22%, NIT@MON@CuS exhibits significant thermal response characteristics in the near-infrared region, along with excellent photothermal conversion capability and stability.171In vitro release kinetics studies have shown rapid release of imidacloprid under near-infrared light and glutathione conditions, which not only promotes the desired temperature increase but also accelerates the drug release rate. After 96 hours of treatment, the mortality rate in the NIT@MON@CuS treatment group increased significantly compared to the group treated with imidacloprid in its original form (from 62% to 88%).
6 Promotion of nanozymes on rhizosphere microbial activity and nitrogen fixation
Nanozymes offer significant advantages over non-nano formulations in regulating plant-associated microbial communities due to their direct interactions at multiple levels, including stress alleviation, essential nutrient release from minerals, enhancement of plant nutrient absorption from soil, and modulation of root secretions. In the latter context, CuO nanozymes promoted the formation of more specialized microbial communities and reduced the denitrification process in the wheat rhizosphere compared to Cu2+. This alteration is accompanied by an enrichment of functional genes and increased interconnectedness of bacterial genus networks, suggesting an improvement in the health of the wheat rhizosphere microbial community.172 It was also shown that the rhizosphere soil of purple alfalfa treated with Fe nanozymes attracted more beneficial microorganisms, constructing a more complex fungal symbiotic network and enhancing the structure and function of the microbial communities in the rhizosphere.173 Both pristine ZnO nanozymes and their aged counterparts were able to promote the production of specific metabolites in alfalfa, attracting microorganisms related to the decomposition of plant-derived and resilient organic matter to colonize the rhizosphere. Co-occurrence network analysis confirmed that these nanozymes significantly promote the growth and activity of microorganisms involved in the formation and decomposition of organic matter in the rhizosphere soil.174
The activity of rhizosphere microorganisms is crucial in ensuring the normal growth and development of crops under adverse conditions. Research has shown that the biostimulation of Se nanozymes promotes the growth of beneficial microorganisms such as Gamma-proteobacteria, Bacteroidia, Gemmatimonadetes, Delta-proteobacteria, and Anaerolineae in rhizosphere soil. Changes in microbial communities are closely related to environmental indicators, enzymes, soil metabolites, and Se speciation, ultimately reducing the bioavailability and accumulation of Cd in pepper plants.175 A comprehensive overview highlighted the dynamic interactions between nanozymes and plant-associated microbial communities, laying a theoretical foundation for the safe development and application of nano-microbiome engineering.176 Collectively, these studies reveal the potential and application value of nanozymes in regulating plant-associated microbial communities.
Nanozymes also significantly impact the symbiotic relationship between plants and rhizobia, influencing plant growth, the root system structure, and N fixation processes.177 Research indicates that Fe3O4 nanozymes can promote the growth of leguminous plants and increase the colonization rate of rhizobia, thereby enhancing the symbiosis between rhizobia and leguminous plants.178 Additionally, the interaction between Fe3O4 nanozymes and two rhizobia species under in vitro conditions revealed that these nanozymes significantly increase the growth rate and extracellular polysaccharide production, potentially facilitating bacterial attachment to root hairs and strengthening the symbiotic connection.179 Also, plant hormones play an important role in the nodule formation and colonization processes.180 Experiments have demonstrated that small Fe2O3 nanozymes can modulate cytokinin synthesis while reducing levels of ethylene and jasmonic acid during nodulation, promoting nodule development and delaying senescence27 (Fig. 3A). Furthermore, Fe2O3 nanozymes have been shown to enhance the activity of key soybean enzymes (PAL) and upregulate genes (GmPAL, GmC4H, Gm4CL, and GmCHS) related to flavonoid synthesis, leading to the accumulation of flavonoid compounds that enhance rhizobia colonization.181 These changes lead to the accumulation of flavonoid compounds and their release into the rhizosphere, attracting rhizobia (Fig. 3B). However, the effects of nanozymes on plant-rhizobia symbiosis also exhibit significant dose effects and type differences. High concentrations of nanozymes may inhibit symbiosis,182,183 while certain metal-based nanozymes may even hinder plant–rhizobia symbiosis. Ag nanozymes may disrupt rhizobia colonization and symbiotic nodule formation184 (Fig. 3C), and ZnO nanozymes have been reported to negatively impact the pea root length and rhizobia morphology, affecting nodule formation and nitrogen fixation.185 TiO2 nanozymes have been shown to reduce rhizobia growth rates, negatively impacting the growth and symbiotic nodules of red clover.186 Despite these challenges, nanozymes exhibit beneficial effects on microbial nitrogen fixation within the plant rhizosphere. For instance, α-multi-walled carbon nanotubes (α-MWCNTs) have been observed to increase the nodule count and enhance nitrogenase activity in alfalfa (Medicago sativa), significantly boosting plant biomass187 (Fig. 3D). Recent innovations include the development of an Fe–Mn nanozyme tailored to the structural characteristics of soybean nitrogenase, which significantly increased nodule weight and count, nitrogen fixation efficiency, and biomass accumulation188 (Fig. 3E). Moreover, low doses of MoS2 nanozymes have been found to improve soybean nitrogen fixation efficiency substantially, increasing the yield by 30% (ref. 108) (Fig. 3F).
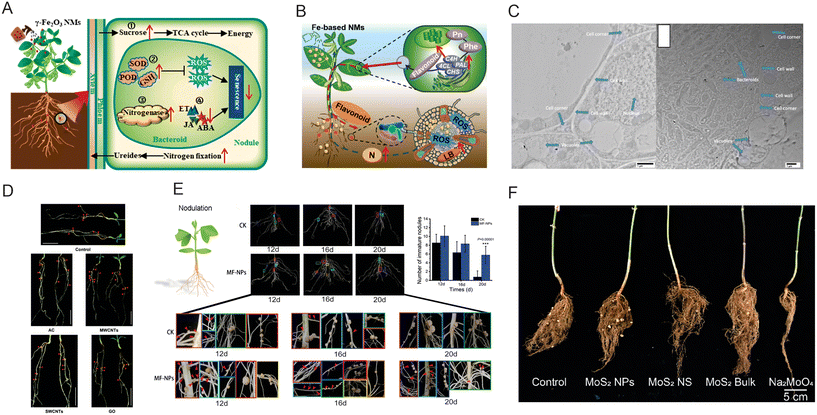 |
| Fig. 3 The impact of nanozymes on symbiotic nitrogen fixation by rhizobia in leguminous crops. (A) Fe2O3 nanozymes promote symbiotic nitrogen fixation in nodules. Reproduced with permission from ref. 27. Copyright 2022, American Chemical Society. (B) Fe2O3 nanozymes induce enhanced PAL activity and flavonoid biosynthesis gene expression, recruiting rhizobia for colonization. Reproduced with permission from ref. 181. Copyright 2022, American Chemical Society. (C) Nano-silver inhibits rhizobia infection and symbiotic nodulation. Reproduced with permission from ref. 184. Copyright 2023, Springer Nature. (D) o-MWCNTs significantly increase the number of nodules and nitrogenase activity during symbiotic nitrogen fixation in the leguminous plant Lotus japonicus. Reproduced with permission from ref. 187. Copyright 2017, The Royal Society of Chemistry. (E) Manganese–iron nanozymes promote nitrogen fixation efficiency in soybeans.188 (F) Low doses of MoS2 nanozymes enhance biological nitrogen fixation efficiency in soybeans.108 | |
Regulation of nitrogen fixation in leguminous plants by nanozymes may be also related to their antioxidant and biocatalyst activities, optimizing the survival conditions of rhizobia and facilitating smooth nitrogen fixation reactions.189 For instance, functional nanozymes have been found to exhibit significant POD activity, which can mitigate the effects of ROS-induced stress on nitrogenase during nitrogen fixation.190 Specifically, CoFe2O4 nanozymes have been shown to not only alleviate oxidative stress but also promote the accumulation of soybean hemoglobin, thereby creating a conducive micro-oxygen environment for rhizobia and ensuring active nitrogenase function.190 This supports the view that nanozymes can enhance the efficiency of the nitrogen fixation process in leguminous crops. Moreover, ROS, which act as signaling molecules in nodule regulation within leguminous plants, can be modulated by nanozymes through the Fenton reaction.188 Further investigations have revealed that Fe2O3 nanozymes enhance gene expression related to plant hormone synthesis, which promotes nodule development and delays senescence processes, underscoring the potential of nanozymes to support plant growth and productivity over longer periods.27 Additionally, recent research indicated that MoS2 nanozymes, known for their strong antioxidant enzyme activity, help clear excess ROS in plant tissues. This action extends the lifespan of nodules, suggesting that such nanozymes can protect nodules from ROS damage, delay nodule aging, and maintain persistent nitrogen fixation functionality.108 These findings collectively underscore the significant regulatory role of nanozymes in the nitrogen fixation mechanisms of leguminous plants and highlight their potential application prospects in enhancing agricultural productivity and sustainability (Fig. 4).
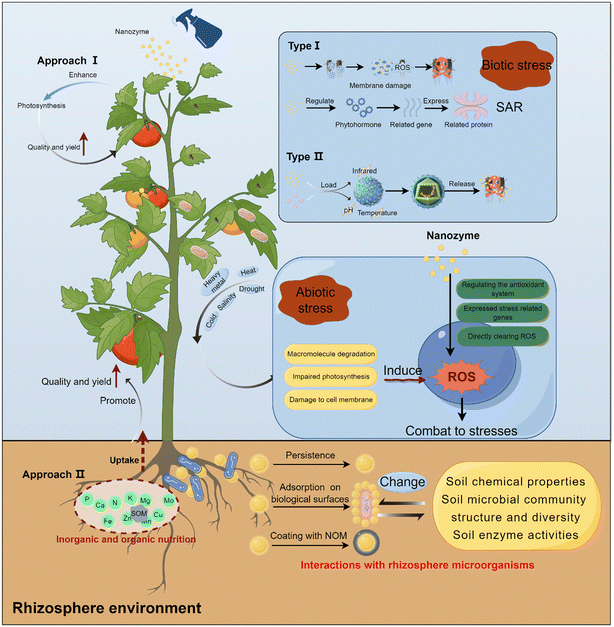 |
| Fig. 4 A schematic diagram of the mechanisms by which nanozymes enhance crop yields and quality, regulate the rhizosphere microbial environment, and alleviate biotic and abiotic stresses. Note: SOM represents soil organic matter and NOM represents natural organic matter. | |
Conclusions and future prospects
In recent years, nanozymes have continuously made new breakthroughs in agriculture. Originating from the field of catalysis, they seem to be tailor-made and perfect for agriculture, paving the way for innovative strategies such as the use of nanozyme-based fertilizers to enhance crop yields and quality, promoting nitrogen fixation through nanozyme catalysis and improving stress resistance by leveraging the exceptional free radical scavenging abilities of nanozymes. Nanozymes can be classified into non-metallic, single-metal, bimetallic, and multi-metallic types based on their composition. Currently, the synthesis methods of nanozymes, though diverse, are not without their drawbacks. Chemical synthesis methods offer high yields and precision but are often energy-intensive and may involve the use of toxic chemicals. On the other hand, green synthesis methods, though environmentally friendly, can be challenging to scale up and may yield nanozymes with lower catalytic activity. To optimize these methods, efforts must be directed towards enhancing synthesis efficiency and scalability by devising green synthesis methods that are not only environmentally benign but also scalable to meet industrial demands. Secondly, the stability and reproducibility of nanozymes need to be reinforced to guarantee that they maintain their catalytic activity and structural integrity under diverse agricultural settings. Lastly, tailoring the physicochemical properties of nanozymes, including particle size, morphology, and surface chemistry, is crucial for optimizing their performance in specific agricultural applications. These strategies, collectively, will contribute to the academic rigor and practical relevance of nanozyme research in agriculture.
It is particularly noteworthy that in the symbiotic nitrogen fixation system of leguminous plants, nanozymes can enhance the interaction with rhizobia, thereby improving the biological nitrogen fixation efficiency of crops. In addition, nanozymes can effectively stimulate the antioxidant defense system in plants when dealing with crop abiotic stresses such as drought, salinity, and heavy metal pollution, thereby improving crop stress resistance and environmental adaptability. At the same time, studies have also found that nanozymes show good application prospects in defending against crop biotic stresses, such as directly inactivating pathogens or stimulating the crop's own immune response, providing a new strategy for reducing the impact of diseases.
Currently, the research progress of nanozymes in agriculture is quite similar to the second stage of nanozyme development in the field of catalysis. Many studies have touted different nanomaterials as nanozymes, but many of them do not meet the strict definition of nanozymes. Also, the main consensus now is that specific nanomaterials can participate in plant stress resistance or nitrogen fixation processes by regulating ROS levels. However, the mechanisms are still unclear, and many studies are mostly limited to in vitro H2O2 catalytic experiments. It remains to be answered whether nanozymes directly catalyze ROS in plants, or the reported effects are indirect. Also, the potential role of nanozymes in scavenging hydroxyl radicals (˙OH) or O2˙− remains to be revealed. Plants can employ a range of enzymatic antioxidants, SOD, GR and APX, to deal with H2O2; however, none of them will be able to handle hydroxyl radicals – the most aggressive and toxic type of ROS.
One promising direction is the application of nanoenzymes for biological nitrogen fixation in both leguminous plants and possibly nonlegumes. Nanozymes have explored new paths to improve nitrogen fixation efficiency by improving rhizosphere microbial activity, maintaining ROS homeostasis, and regulating plant hormone signaling. Nevertheless, research on whether nanozymes have nitrogenase activity still faces many challenges, and there is no conclusive evidence that they truly possess “nitrogenase activity”. The binding and reduction processes of nanozymes with nitrogen need to meet a series of specific conditions and mechanisms, involving complex processes such as interface interaction, energy transfer, and electron transfer. In addition, although ATP hydrolysis is regarded as an important energy source in the nitrogen fixation process, the energy transfer mechanism between nanozymes and nitrogenase remains unclear. Filling these gaps in the knowledge may open a new era for biological nitrogen fixation. For example, the question of whether nanozymes can be transported in nodules and simulate the function of nitrogenase after spraying still needs to be verified through rigorous scientific research. This first requires confirmation of whether nanoparticles can be effectively transported to the key tissue of nodules, and carefully designed experiments to verify whether they have nitrogenase-like functions. Assuming that nanoparticles can function independently on nodules on legumes, this may imply that nanoenzymes may function in biological nitrogen fixation in non-legumes, a highly promising and economically important direction. Meanwhile, future research on the preparation of nanozymes also needs to delve into material selection, structural design, surface modification and stability, synthesis methods, and many other aspects. For key issues such as the influence of different materials on nanozyme performance, structural design, and surface functionalization modification, optimal solutions need to be sought to achieve efficient biocatalysis. In addition, optimizing synthesis methods and improving stability and reproducibility are also the focus of future research. These explorations are expected to promote the in-depth development of nanozyme enzyme simulation research and provide strong support for the understanding and application of biological nitrogen fixation processes and other enzymes.
Targeted delivery of nutrients may be another interesting direction for nanoenzymes in agriculture. As nutrient distribution in plants is determined by their mobility, all essential micronutrients Cu, Zn, Fe, Mo and Ni go to mature leaves (delivered via transpiration flow) but not grains or young leaves (where they are needed). Will nanozymes be able to help? From what we know they can, being more efficient than just nutrient sprays. Understanding the underlying mechanisms may drastically reduce the environmental footprint of modern agricultural practices by replacing traditional fertilizers with nanoenzymes, boosting green agriculture.
Data availability
The original contributions presented in the study are included in the manuscript. Further data will be made available upon request to the corresponding authors.
Conflicts of interest
The authors declare that they have no known competing financial interests or personal relationships that could have appeared to influence the work reported in this paper.
Acknowledgements
This work was supported by the National Natural Science Foundation of China (42077095), the National Natural Science Foundation of China (42477356), and the National Natural Science Foundation of China (42377042).
References
- H. Wei and E. Wang, Nanomaterials with Enzyme-like Characteristics (Nanozymes): Next-Generation Artificial Enzymes, Chem. Soc. Rev., 2013, 42(14), 6060–6093, 10.1039/c3cs35486e.
- L. L. Dugan, J. K. Gabrielsen, S. P. Yu, T. S. Lin and D. W. Choi, Buckminsterfullerenol Free Radical Scavengers Reduce Excitotoxic and Apoptotic Death of Cultured Cortical Neurons, Neurobiol. Dis., 1996, 3(2), 129–135, DOI:10.1006/nbdi.1996.0013.
- F. Manea, F. B. Houillon, L. Pasquato and P. Scrimin, Nanozymes: Gold-Nanoparticle-Based Transphosphorylation Catalysts, Angew. Chem., Int. Ed., 2004, 43(45), 6165–6169, DOI:10.1002/anie.200460649.
- L. Gao, J. Zhuang, L. Nie, J. Zhang, Y. Zhang, N. Gu, T. Wang, J. Feng, D. Yang, S. Perrett and X. Yan, Intrinsic Peroxidase-like Activity of Ferromagnetic Nanoparticles, Nat. Nanotechnol., 2007, 2(9), 577–583, DOI:10.1038/nnano.2007.260.
- S. Scott, H. Zhao, A. Dey and T. B. Gunnoe, Nano-Apples and Orange-Zymes, ACS Catal., 2020, 10(23), 14315–14317, DOI:10.1021/acscatal.0c05047.
- H. Wei, L. Gao, K. Fan, J. Liu, J. He, X. Qu, S. Dong, E. Wang and X. Yan, Nanozymes: A Clear Definition with Fuzzy Edges, Nano Today, 2021, 40, 101269, DOI:10.1016/j.nantod.2021.101269.
- M. Zandieh and J. Liu, Nanozymes: Definition, Activity, and Mechanisms, Adv. Mater., 2024, 36(10), 2211041, DOI:10.1002/adma.202211041.
- R. Zhang, X. Yan and K. Fan, Nanozymes Inspired by Natural Enzymes, Acc. Mater. Res., 2021, 2(7), 534–547, DOI:10.1021/accountsmr.1c00074.
- Y. Huang, J. Ren and X. Qu, Nanozymes: Classification, Catalytic Mechanisms, Activity Regulation, and Applications, Chem. Rev., 2019, 119(6), 4357–4412, DOI:10.1021/acs.chemrev.8b00672.
- J. He, Y. Hou, Z. Zhang, J. Zhang, X. Yan, K. Fan and M. Liang, Carbon-Based Nanozymes: How Structure Affects Performance, Nano Biomed. Eng., 2024, 16(1), 28–47, DOI:10.26599/NBE.2024.9290053.
- A. C. Power, B. Gorey, S. Chandra and J. Chapman, Carbon Nanomaterials and Their Application to Electrochemical Sensors: A Review, Nanotechnol. Rev., 2018, 7(1), 19–41, DOI:10.1515/ntrev-2017-0160.
- K. P. Loh, D. Ho, G. N. C. Chiu, D. T. Leong, G. Pastorin and E. K.-H. Chow, Clinical Applications of Carbon Nanomaterials in Diagnostics and Therapy, Adv. Mater., 2018, 30(47), 1802368, DOI:10.1002/adma.201802368.
- O. Zaytseva and G. Neumann, Carbon Nanomaterials: Production, Impact on Plant Development, Agricultural and Environmental Applications, Chem. Biol. Technol. Agric., 2016, 3(1), 17, DOI:10.1186/s40538-016-0070-8.
- H. Zhang, M. Yue, X. Zheng, C. Xie, H. Zhou and L. Li, Physiological Effects of Single- and Multi-Walled Carbon Nanotubes on Rice Seedlings, IEEE Trans. NanoBiosci., 2017, 16(7), 563–570, DOI:10.1109/TNB.2017.2715359.
- J. Jiao, F. Cheng, X. Zhang, L. Xie, Z. Li, C. Yuan, B. Xu and L. Zhang, Preparation of Graphene Oxide and Its Mechanism in Promoting Tomato Roots Growth, J. Nanosci. Nanotechnol., 2016, 16(4), 4216–4223, DOI:10.1166/jnn.2016.12601.
- Q. Chen, H. Man, L. Zhu, Z. Guo, X. Wang, J. Tu, G. Jin, J. Lou, L. Zhang and L. Ci, Enhanced Plant Antioxidant Capacity and Biodegradation of Phenol by Immobilizing Peroxidase on Amphoteric Nitrogen-Doped Carbon Dots, Catal. Commun., 2020, 134, 105847, DOI:10.1016/j.catcom.2019.105847.
- A. K. Shukla, C. Sharma and A. Acharya, Bioinspired Metal-Free Fluorescent Carbon Nanozyme with Dual Catalytic Activity to Confront Cellular Oxidative Damage, ACS Appl. Mater. Interfaces, 2021, 13(13), 15040–15052, DOI:10.1021/acsami.1c03130.
- J. Zhang, J. Xu, H. Ma, H. Bai, L. Liu, C. Shu, H. Li, S. Wang and C. Wang, Designing an Amino-Fullerene Derivative C70–(EDA)8 to Fight Superbacteria, ACS Appl. Mater. Interfaces, 2019, 11(16), 14597–14607, DOI:10.1021/acsami.9b01483.
- F. Li, T. Li, C. Sun, J. Xia, Y. Jiao and H. Xu, Selenium-Doped Carbon Quantum Dots for Free-Radical Scavenging, Angew. Chem., Int. Ed., 2017, 56(33), 9910–9914, DOI:10.1002/anie.201705989.
- X. Wang, Y. Lu, K. Hua, D. Yang and Y. Yang, Iodine-Doped Carbon Dots with Inherent Peroxidase Catalytic Activity for Photocatalytic Antibacterial and Wound Disinfection, Anal. Bioanal. Chem., 2021, 413(5), 1373–1382, DOI:10.1007/s00216-020-03100-x.
- Y. Liu, K. Ai, X. Ji, D. Askhatova, R. Du, L. Lu and J. Shi, Comprehensive Insights into the Multi-Antioxidative Mechanisms of Melanin Nanoparticles and Their Application To Protect Brain from Injury in Ischemic Stroke, J. Am. Chem. Soc., 2017, 139(2), 856–862, DOI:10.1021/jacs.6b11013.
- S. Liu, J. Xu, Y. Xing, T. Yan, S. Yu, H. Sun and J. Liu, Nanozymes as Efficient Tools for Catalytic Therapeutics, View, 2022, 3(2), 20200147, DOI:10.1002/VIW.20200147.
- X. Wang, Y. Zhang, J. Wu, Z. Zhang, Q. Liao, Z. Kang and Y. Zhang, Single-Atom Engineering to Ignite 2D Transition Metal Dichalcogenide Based Catalysis: Fundamentals, Progress, and Beyond, Chem. Rev., 2022, 122(1), 1273–1348, DOI:10.1021/acs.chemrev.1c00505.
- J. Feng, X. Yang, T. Du, L. Zhang, P. Zhang, J. Zhuo, L. Luo, H. Sun, Y. Han, L. Liu, Y. Shen, J. Wang and W. Zhang, Transition Metal High-Entropy Nanozyme: Multi-Site Orbital Coupling Modulated High-Efficiency Peroxidase Mimics, Adv. Sci., 2023, 10(33), 2303078, DOI:10.1002/advs.202303078.
- J. Kwon, X. Mao and J. Lee, Fe-Based Multifunctional Nanoparticles with Various Physicochemical Properties, Curr. Appl. Phys., 2017, 17(8), 1066–1078, DOI:10.1016/j.cap.2017.04.018.
- H. Tombuloglu, Y. Slimani, G. Tombuloglu, M. Almessiere and A. Baykal, Uptake and Translocation of Magnetite(Fe3O4) Nanoparticles and Its Impact on Photosynthetic Genes in Barley (Hordeum Vulgare L.), Chemosphere, 2019, 226, 110–122, DOI:10.1016/j.chemosphere.2019.03.075.
- X. Cao, L. Yue, C. Wang, X. Luo, C. Zhang, X. Zhao, F. Wu, J. C. White, Z. Wang and B. Xing, Foliar Application with Iron Oxide Nanomaterials Stimulate Nitrogen Fixation, Yield, and Nutritional Quality of Soybean, ACS Nano, 2022, 16(1), 1170–1181, DOI:10.1021/acsnano.1c08977.
- X. Zhang, Z. Shao, X. Zhang, Y. He and J. Jie, Surface Charge Transfer Doping of Low-Dimensional Nanostructures toward High-Performance Nanodevices, Adv. Mater., 2016, 28(47), 10409–10442, DOI:10.1002/adma.201601966.
- A. H. Al-Naggar, N. M. Shinde, J.-S. Kim and R. S. Mane, Water
Splitting Performance of Metal and Non-Metal-Doped Transition Metal Oxide Electrocatalysts, Coord. Chem. Rev., 2023, 474, 214864, DOI:10.1016/j.ccr.2022.214864.
- S. Fu, S. Wang, X. Zhang, A. Qi, Z. Liu, X. Yu, C. Chen and L. Li, Structural Effect of Fe3O4 Nanoparticles on Peroxidase-like Activity for Cancer Therapy, Colloids Surf., B, 2017, 154, 239–245, DOI:10.1016/j.colsurfb.2017.03.038.
- K. Fan, H. Wang, J. Xi, Q. Liu, X. Meng, D. Duan, L. Gao and X. Yan, Optimization of Fe3O4 Nanozyme Activity via Single Amino Acid Modification Mimicking an Enzyme Active Site, Chem. Commun., 2016, 53(2), 424–427, 10.1039/C6CC08542C.
- H. Wu, J.-J. Yin, W. G. Wamer, M. Zeng and Y. M. Lo, Reactive Oxygen Species-Related Activities of Nano-Iron Metal and Nano-Iron Oxides, J. Food Drug Anal., 2014, 22(1), 86–94, DOI:10.1016/j.jfda.2014.01.007.
-
E. Gumienna-Kontecka, M. Rowińska-Żyrek and M. Łuczkowski, The Role of Trace Elements in Living Organisms, in Recent Advances in Trace Elements, John Wiley & Sons, Ltd, 2018, pp. 177–206, DOI:10.1002/9781119133780.ch9.
- J. Mu, Y. Wang, M. Zhao and L. Zhang, Intrinsic Peroxidase-like Activity and Catalase-like Activity of Co3O4 Nanoparticles, Chem. Commun., 2012, 48(19), 2540–2542, 10.1039/C2CC17013B.
- M. Jahani, R. A. Khavari-Nejad, H. Mahmoodzadeh and S. Saadatmand, Effects of Foliar Application of Cobalt Oxide Nanoparticles on Growth, Photosynthetic Pigments, Oxidative Indicators, Non-Enzymatic Antioxidants and Compatible Osmolytes in Canola (Brassica Napus L.), Acta Biol. Cracov., Ser. Bot., 2019, 61(1), 29–42, DOI:10.24425/abcsb.2019.127736.
- Z. Tohidiyan, S. Hashemi and K. P. Boroujeni, Facile Microwave-Assisted Synthesis of NiO Nanoparticles and Its Effect on Soybean (Glycine Max), IET Nanobiotechnol., 2019, 13(2), 101–106, DOI:10.1049/iet-nbt.2018.5003.
- L. Sun, Y. Wang, R. Wang, R. Wang, P. Zhang, Q. Ju and J. Xu, Physiological, Transcriptomic, and Metabolomic Analyses Reveal Zinc Oxide Nanoparticles Modulate Plant Growth in Tomato, Environ. Sci.: Nano, 2020, 7(11), 3587–3604, 10.1039/D0EN00723D.
- C. Hao, A. Qu, L. Xu, M. Sun, H. Zhang, C. Xu and H. Kuang, Chiral Molecule-Mediated Porous CuxO Nanoparticle Clusters with Antioxidation Activity for Ameliorating Parkinson's Disease, J. Am. Chem. Soc., 2019, 141(2), 1091–1099, DOI:10.1021/jacs.8b11856.
- L. Lu, M. Huang, Y. Huang, P. F.-X. Corvini, R. Ji and L. Zhao, Mn3O4 Nanozymes Boost Endogenous Antioxidant Metabolites in Cucumber (Cucumis Sativus) Plant and Enhance Resistance to Salinity Stress, Environ. Sci.: Nano, 2020, 7(6), 1692–1703, 10.1039/D0EN00214C.
- M. Sankar, N. Dimitratos, P. J. Miedziak, P. P. Wells, C. J. Kiely and G. J. Hutchings, Designing Bimetallic Catalysts for a Green and Sustainable Future, Chem. Soc. Rev., 2012, 41(24), 8099–8139, 10.1039/C2CS35296F.
- X. Liu, D. Wang and Y. Li, Synthesis and Catalytic Properties of Bimetallic Nanomaterials with Various Architectures, Nano Today, 2012, 7(5), 448–466, DOI:10.1016/j.nantod.2012.08.003.
- Y. Wang, H. Li, L. Guo, Q. Jiang and F. Liu, A Cobalt-Doped Iron Oxide Nanozyme as a Highly Active Peroxidase for Renal Tumor Catalytic Therapy, RSC Adv., 2019, 9(33), 18815–18822, 10.1039/C8RA05487H.
- A. A. Vernekar, T. Das, S. Ghosh and G. Mugesh, A Remarkably Efficient MnFe2O4 -Based Oxidase Nanozyme, Chem. – Asian J., 2016, 11(1), 72–76, DOI:10.1002/asia.201500942.
- H. Wang, W. Yang, X. Wang, L. Huang, Y. Zhang and S. Yao, A CeO2@MnO2 Core–Shell Hollow Heterojunction as Glucose Oxidase-like Photoenzyme for Photoelectrochemical Sensing of Glucose, Sens. Actuators, B, 2020, 304, 127389, DOI:10.1016/j.snb.2019.127389.
- M. Lu, B. Li, L. Guan, K. Li and Y. Lin, Carbon-Shielded Three-Dimensional Co–Mn Nanowire Array Anchored on Ni Foam with Dual-Enzyme Mimic Performance for Selective Detection of Ascorbic Acid, ACS Sustainable Chem. Eng., 2019, 7(18), 15471–15478, DOI:10.1021/acssuschemeng.9b03095.
- Y. Wang, C. Chen, D. Zhang and J. Wang, Bifunctionalized Novel Co-V MMO Nanowires: Intrinsic Oxidase and Peroxidase like Catalytic Activities for Antibacterial Application, Appl. Catal., A, 2020, 261, 118256, DOI:10.1016/j.apcatb.2019.118256.
- P. K. Boruah and M. R. Das, Dual Responsive Magnetic Fe3O4-TiO2/Graphene Nanocomposite as an Artificial Nanozyme for the Colorimetric Detection and Photodegradation of Pesticide in an Aqueous Medium, J. Hazard. Mater., 2020, 385, 121516, DOI:10.1016/j.jhazmat.2019.121516.
- H. Tombuloglu, G. Tombuloglu, Y. Slimani, I. Ercan, H. Sozeri and A. Baykal, Impact of Manganese Ferrite (MnFe2O4) Nanoparticles on Growth and Magnetic Character of Barley (Hordeum Vulgare L.), Environ. Pollut., 2018, 243, 872–881, DOI:10.1016/j.envpol.2018.08.096.
- M. L. López-Moreno, L. L. Avilés, N. G. Pérez, B. Á. Irizarry, O. Perales, Y. Cedeno-Mattei and F. Román, Effect of Cobalt Ferrite (CoFe2O4) Nanoparticles on the Growth and Development of Lycopersicon Lycopersicum (Tomato Plants), Sci. Total Environ., 2016, 550, 45–52, DOI:10.1016/j.scitotenv.2016.01.063.
- L. Yue, Y. Feng, C. Ma, C. Wang, F. Chen, X. Cao, J. Wang, J. C. White, Z. Wang and B. Xing, Molecular Mechanisms of Early Flowering in Tomatoes Induced by Manganese Ferrite (MnFe2O4) Nanomaterials, ACS Nano, 2022, 16(4), 5636–5646, DOI:10.1021/acsnano.1c10602.
- S. Luo, J. Gao, H. Yuan, J. Yang, Y. Fan, L. Wang, H. Ouyang and Z. Fu, Mn Single-Atom Nanozymes with Superior Loading Capability and Superb Superoxide Dismutase-like Activity for Bioassay, Anal. Chem., 2023, 95(24), 9366–9372, DOI:10.1021/acs.analchem.3c01623.
- Q. Feng, G. Wang, L. Xue, Y. Wang, M. Liu, J. Liu, S. Zhang and W. Hu, Single-Atom Nanozyme Based on Mn-Center with Enhanced Peroxidase-like Activity for Organic Dye Degradation, ACS Appl. Nano Mater., 2023, 6(6), 4844–4853, DOI:10.1021/acsanm.3c00571.
- H. Shang, X. Zhang, M. Ding, A. Zhang and C. Wang, Dual-Mode Paper Biosensing Platform Based on Oxidase-Like CoFeMn Nanozymes for Point-of-Care Detection of Glutathione, ACS Appl. Nano Mater., 2023, 6(10), 8907–8915, DOI:10.1021/acsanm.3c01310.
- J. Li, X. Cai, P. Jiang, H. Wang, S. Zhang, T. Sun, C. Chen and K. Fan, Co-Based Nanozymatic Profiling: Advances Spanning Chemistry, Biomedical, and Environmental Sciences, Adv. Mater., 2024, 36(8), 2307337, DOI:10.1002/adma.202307337.
- S. G. Liu, H. Wang, Q. Zhao, W. Gao, X. Shi and Z. Liu, A Portable Colorimetric Sensing Platform for Rapid and Sensitive Quantification of Dichlorvos Pesticide Based on Fe-Mn Bimetallic Oxide Nanozyme-Participated Highly Efficient Chromogenic Catalysis, Anal. Chim. Acta, 2024, 1292, 342243, DOI:10.1016/j.aca.2024.342243.
- A. P. Nagvenkar and A. Gedanken, Cu0.89Zn0.11O, A New Peroxidase-Mimicking Nanozyme with High Sensitivity for Glucose and Antioxidant Detection, ACS Appl. Mater. Interfaces, 2016, 8(34), 22301–22308, DOI:10.1021/acsami.6b05354.
- Z. Li, X. Yang, Y. Yang, Y. Tan, Y. He, M. Liu, X. Liu and Q. Yuan, Peroxidase-Mimicking Nanozyme with Enhanced Activity and High Stability Based on Metal-Support Interactions, Chemistry, 2018, 24(2), 409–415, DOI:10.1002/chem.201703833.
- D. Li, D. Dai, G. Xiong, S. Lan and C. Zhang, Metal-Based Nanozymes with Multienzyme-Like Activities as Therapeutic Candidates: Applications, Mechanisms, and Optimization Strategy, Small, 2023, 19(7), 2205870, DOI:10.1002/smll.202205870.
- Y. Mao, F. Jia, T. Jing, T. Li, H. Jia and W. He, Enhanced Multiple Enzymelike Activity of PtPdCu Trimetallic Nanostructures for Detection of Fe2+ and Evaluation of Antioxidant Capability, ACS Sustainable Chem. Eng., 2021, 9(1), 569–579, DOI:10.1021/acssuschemeng.0c08230.
- F. Meng, P. Zhu, L. Yang, L. Xia and H. Liu, Nanozymes with Atomically Dispersed Metal Centers: Structure–Activity Relationships and Biomedical Applications, Chem. Eng. J., 2023, 452, 139411, DOI:10.1016/j.cej.2022.139411.
- Z. Wang, Y. Zhang, E. Ju, Z. Liu, F. Cao, Z. Chen, J. Ren and X. Qu, Biomimetic Nanoflowers by Self-Assembly of Nanozymes to Induce Intracellular Oxidative Damage against Hypoxic Tumors, Nat. Commun., 2018, 9(1), 3334, DOI:10.1038/s41467-018-05798-x.
- X. Mu, J. Wang, Y. Li, F. Xu, W. Long, L. Ouyang, H. Liu, Y. Jing, J. Wang, H. Dai, Q. Liu, Y. Sun, C. Liu and X.-D. Zhang, Redox Trimetallic Nanozyme with Neutral Environment Preference for Brain Injury, ACS Nano, 2019, 13(2), 1870–1884, DOI:10.1021/acsnano.8b08045.
- J. Chen, Y. Wang, Y. Yu, J. Wang, J. Liu, H. Ihara and H. Qiu, Composite Materials Based on Covalent Organic Frameworks for Multiple Advanced Applications, Exploration, 2023, 3(3), 20220144, DOI:10.1002/EXP.20220144.
- C. A. S. Ballesteros, L. A. Mercante, A. D. Alvarenga, M. H. M. Facure, R. Schneider and D. S. Correa, Recent Trends in Nanozymes Design: From Materials and Structures to Environmental Applications, Mater. Chem. Front., 2021, 5(20), 7419–7451, 10.1039/D1QM00947H.
-
J. A. Elegbede and A. Lateef, Microbial Enzymes in Nanotechnology and Fabrication of Nanozymes: A Perspective, in Microbial Nanobiotechnology: Principles and Applications, ed. A. Lateef, E. B. Gueguim-Kana, N. Dasgupta and S. Ranjan, Materials Horizons: From Nature to Nanomaterials, Springer, Singapore, 2021, pp. 185–232, DOI:10.1007/978-981-33-4777-9_7.
-
S. Bhagat, J. Shah and S. Singh, Metal-Based Nanozyme: Strategies to Modulate the Catalytic Activity to Realize Environment Application, in Nanozymes for Environmental Engineering,Environmental Chemistry for a Sustainable World, ed. H. K. Daima, P. N. Navya and E. Lichtfouse, Springer International Publishing, Cham, 2021, pp. 177–212, DOI:10.1007/978-3-030-68230-9_7.
- M. Huston, M. DeBella, M. DiBella and A. Gupta, Green Synthesis of Nanomaterials, Nanomaterials, 2021, 11(8), 2130, DOI:10.3390/nano11082130.
- O. J. Nava, C. A. Soto-Robles, C. M. Gómez-Gutiérrez, A. R. Vilchis-Nestor, A. Castro-Beltrán, A. Olivas and P. A. Luque, Fruit Peel Extract Mediated Green Synthesis of Zinc Oxide Nanoparticles, J. Mol. Struct., 2017, 1147, 1–6, DOI:10.1016/j.molstruc.2017.06.078.
- S. Busi, J. Rajkumari, S. Pattnaik, P. Parasuraman and S. Hnamte, Extracellular Synthesis Of Zinc Oxide Nanoparticles Using Acinetobacter Schindleri Siz7 And Its Antimicrobial Property Against Foodborne Pathogens, J. Microbiol., Biotechnol. Food Sci., 2016, 5(5), 407–411, DOI:10.15414/jmbfs.2016.5.5.407-411.
- S. Nagarajan and K. Arumugam Kuppusamy, Extracellular Synthesis of Zinc Oxide Nanoparticle Using Seaweeds of Gulf of Mannar, India, J. Nanobiotechnol., 2013, 11(1), 39, DOI:10.1186/1477-3155-11-39.
- Z. Alhalili, Green Synthesis of Copper Oxide Nanoparticles CuO NPs from Eucalyptus Globoulus Leaf Extract: Adsorption and Design of Experiments, Arabian J. Chem., 2022, 15(5), 103739, DOI:10.1016/j.arabjc.2022.103739.
- S. N. Hawar, H. S. Al-Shmgani, Z. A. Al-Kubaisi, G. M. Sulaiman, Y. H. Dewir and J. J. Rikisahedew, Green Synthesis of Silver Nanoparticles from Alhagi Graecorum Leaf Extract and Evaluation of Their Cytotoxicity and Antifungal Activity, J. Nanomater., 2022, 2022, e1058119, DOI:10.1155/2022/1058119.
- P. Prabhu, M. Rao, G. Murugesan, M. K. Narasimhan, T. Varadavenkatesan, R. Vinayagam, N. T. Lan Chi, A. Pugazhendhi and R. Selvaraj, Synthesis, Characterization and Anticancer Activity of the Green-Synthesized Hematite Nanoparticles, Environ. Res., 2022, 214, 113864, DOI:10.1016/j.envres.2022.113864.
- S. Vijayakumar, V. N. Punitha and N. Parameswari, Phytonanosynthesis of MgO Nanoparticles: Green Synthesis, Characterization and Antimicrobial Evaluation, Arabian J. Sci. Eng., 2022, 47(6), 6729–6734, DOI:10.1007/s13369-021-06107-3.
- A. A. Mohamed, A. Fouda, M. A. Abdel-Rahman, S. E.-D. Hassan, M. S. El-Gamal, S. S. Salem and T. I. Shaheen, Fungal Strain Impacts the Shape, Bioactivity and Multifunctional Properties of Green Synthesized Zinc Oxide Nanoparticles, Biocatal. Agric. Biotechnol., 2019, 19, 101103, DOI:10.1016/j.bcab.2019.101103.
- A. Fouda, H. S. El-Din, S. S. Salem and T. I. Shaheen, In-Vitro Cytotoxicity, Antibacterial, and UV Protection Properties of the Biosynthesized Zinc Oxide Nanoparticles for Medical Textile Applications, Microb. Pathog., 2018, 125, 252–261, DOI:10.1016/j.micpath.2018.09.030.
- G. S. Dhillon, S. K. Brar, S. Kaur and M. Verma, Green Approach for Nanoparticle Biosynthesis by Fungi: Current Trends and Applications, Crit. Rev. Biotechnol., 2012, 32(1), 49–73, DOI:10.3109/07388551.2010.550568.
- F. N. Spagnoletti, C. Spedalieri, F. Kronberg and R. Giacometti, Extracellular Biosynthesis of Bactericidal Ag/AgCl Nanoparticles for Crop Protection Using the Fungus Macrophomina Phaseolina, J. Environ. Manage., 2019, 231, 457–466, DOI:10.1016/j.jenvman.2018.10.081.
- Q. Zeng, H. Wen, Q. Wen, X. Chen, Y. Wang, W. Xuan, J. Liang and S. Wan, Cucumber Mosaic Virus as Drug Delivery Vehicle for Doxorubicin, Biomaterials, 2013, 34(19), 4632–4642, DOI:10.1016/j.biomaterials.2013.03.017.
- C. Mao, C. E. Flynn, A. Hayhurst, R. Sweeney, J. Qi, G. Georgiou, B. Iverson and A. M. Belcher, Viral Assembly of Oriented Quantum Dot Nanowires, Proc. Natl. Acad. Sci. U. S. A., 2003, 100(12), 6946–6951, DOI:10.1073/pnas.0832310100.
- A. J. Love, V. Makarov, I. Yaminsky, N. O. Kalinina and M. E. Taliansky, The Use of Tobacco Mosaic Virus and Cowpea Mosaic Virus for the Production of Novel Metal Nanomaterials, Virology, 2014, 449, 133–139, DOI:10.1016/j.virol.2013.11.002.
- V. P. Aswathi, S. Meera, C. G. A. Maria and M. Nidhin, Green Synthesis of Nanoparticles from Biodegradable Waste Extracts and Their Applications: A Critical Review, Nanotechnol. Environ. Eng., 2023, 8(2), 377–397, DOI:10.1007/s41204-022-00276-8.
- R. V. Bordiwala, Green Synthesis and Applications of Metal Nanoparticles.- A Review Article, Results Chem., 2023, 5, 100832, DOI:10.1016/j.rechem.2023.100832.
- M. Khatami, H. Q. Alijani, M. S. Nejad and R. S. Varma, Core@shell Nanoparticles: Greener Synthesis Using Natural Plant Products, Appl. Sci., 2018, 8(3), 411, DOI:10.3390/app8030411.
- J. A. Kumar, T. Krithiga, S. Manigandan, S. Sathish, A. A. Renita, P. Prakash, B. S. N. Prasad, T. R. P. Kumar, M. Rajasimman, A. Hosseini-Bandegharaei, D. Prabu and S. Crispin, A Focus to Green Synthesis of Metal/Metal Based Oxide Nanoparticles: Various Mechanisms and Applications towards Ecological Approach, J. Cleaner Prod., 2021, 324, 129198, DOI:10.1016/j.jclepro.2021.129198.
- O. V. Kharissova, B. I. Kharisov, C. M. Oliva González, Y. P. Méndez and I. López, Greener Synthesis of Chemical Compounds and Materials, R. Soc. Open Sci., 2019, 6(11), 191378, DOI:10.1098/rsos.191378.
- L. Yang, S. Dong, S. Gai, D. Yang, H. Ding, L. Feng, G. Yang, Z. Rehman and P. Yang, Deep Insight of Design, Mechanism, and Cancer Theranostic Strategy of Nanozymes, Nano-Micro Lett., 2023, 16(1), 28, DOI:10.1007/s40820-023-01224-0.
- N. Saini, R. Choudary, D. S. Chopra, D. Singh and N. Singh, Nanozymes: Classification, Synthesis and Challenges, Appl. Nanosci., 2023, 13(9), 6433–6443, DOI:10.1007/s13204-023-02933-z.
- V. Harish, M. M. Ansari, D. Tewari, A. B. Yadav, N. Sharma, S. Bawarig, M.-L. García-Betancourt, A. Karatutlu, M. Bechelany and A. Barhoum, Cutting-Edge Advances in Tailoring Size, Shape, and Functionality of Nanoparticles and Nanostructures: A Review, J. Taiwan Inst. Chem. Eng., 2023, 149, 105010, DOI:10.1016/j.jtice.2023.105010.
- M. M. Alam, A. M. Asiri, M. T. Uddin, Inamuddin, M. A. Islam, M. R. Awual and M. M. Rahman, One-Step Wet-Chemical Synthesis of Ternary ZnO/CuO/Co3O4 Nanoparticles for Sensitive and Selective Melamine Sensor Development, New J. Chem., 2019, 43(12), 4849–4858, 10.1039/C8NJ06361C.
- J. Yao, Y. Cheng, M. Zhou, S. Zhao, S. Lin, X. Wang, J. Wu, S. Li and H. Wei, ROS Scavenging Mn3O4 Nanozymes for in Vivo Anti-Inflammation, Chem. Sci., 2018, 9(11), 2927–2933, 10.1039/C7SC05476A.
- H. Fan, Y. Li, J. Liu, R. Cai, X. Gao, H. Zhang, Y. Ji, G. Nie and X. Wu, Plasmon-Enhanced Oxidase-Like Activity and Cellular Effect of Pd-Coated Gold Nanorods, ACS Appl. Mater. Interfaces, 2019, 11(49), 45416–45426, DOI:10.1021/acsami.9b16286.
- H. Gavilán, G. M. R. Rizzo, N. Silvestri, B. T. Mai and T. Pellegrino, Scale-up Approach for the Preparation of Magnetic Ferrite Nanocubes and Other Shapes with Benchmark Performance for Magnetic Hyperthermia Applications, Nat. Protoc., 2023, 18(3), 783–809, DOI:10.1038/s41596-022-00779-3.
- H. Lv, Y. Wang, L. Sun, Y. Yamauchi and B. Liu, A General Protocol for Precise Syntheses of Ordered Mesoporous Intermetallic Nanoparticles, Nat. Protoc., 2023, 18(10), 3126–3154, DOI:10.1038/s41596-023-00872-1.
- Y. Ai, M.-Q. He, H. Sun, X. Jia, L. Wu, X. Zhang, H. Sun and Q. Liang, Ultra-Small High-Entropy Alloy Nanoparticles: Efficient Nanozyme for Enhancing Tumor Photothermal Therapy, Adv. Mater., 2023, 35(23), 2302335, DOI:10.1002/adma.202302335.
- Z. Du, L. Zhu, P. Wang, X. Lan, S. Lin and W. Xu, Coordination-Driven One-Step Rapid Self-Assembly Synthesis of Dual-Functional Ag@Pt Nanozyme, Small, 2023, 19(35), 2301048, DOI:10.1002/smll.202301048.
- Z. Cui, Y. Li, H. Zhang, P. Qin, X. Hu, J. Wang, G. Wei and C. Chen, Lighting Up Agricultural Sustainability in the New Era through Nanozymology: An Overview of Classifications and Their Agricultural Applications, J. Agric. Food Chem., 2022, 70(42), 13445–13463, DOI:10.1021/acs.jafc.2c04882.
- Z. Cui, Y. Li, O. V. Tsyusko, J. Wang, J. M. Unrine, G. Wei and C. Chen, Metal–Organic Framework-Enabled Sustainable Agrotechnologies: An Overview of Fundamentals and Agricultural Applications, J. Agric. Food Chem., 2024, 72(16), 8890–8905, DOI:10.1021/acs.jafc.4c00764.
-
S. Padhi and A. Behera, Nano-Enabled Approaches for the Suitable Delivery of Fertilizer and Pesticide for Plant Growth, in Plant Growth-Promoting Microbes for Sustainable Biotic and Abiotic Stress Management, ed. H. I. Mohamed, H. E.-D. S. El-Beltagi and K. A. Abd-Elsalam, Springer International Publishing, Cham, 2021, pp. 355–394, DOI:10.1007/978-3-030-66587-6_13.
- Q. Teng, D. Zhang, X. Niu and C. Jiang, Influences of Application of Slow-Release Nano-Fertilizer on Green Pepper Growth, Soil Nutrients and Enzyme Activity, IOP Conf. Ser.: Mater. Sci. Eng., 2018, 208(1), 012014, DOI:10.1088/1755-1315/208/1/012014.
- P. Min-hui, L. I. Li-xia, D. Shu-qi, L. I. U. Dong-sheng, L. I. Hong-yan and L. Li-na, Research progress on nano-materials application in slow/controlled-release fertilizers, Zhiwu Yingyang Yu Feiliao Xuebao, 2022, 28(9), 1708–1719, DOI:10.11674/zwyf.2022131.
- A. Mirbolook, M. R. Sadaghiani, P. Keshavarz and M. Alikhani, New Slow-Release Urea Fertilizer Fortified with Zinc for Improving Zinc Availability and Nitrogen Use Efficiency in Maize, ACS Omega, 2023, 8(48), 45715–45728, DOI:10.1021/acsomega.3c06013.
-
K. R. Rakhimol, S. Thomas, N. Kalarikkal and K. Jayachandran, Chapter 10 - Nanotechnology in Controlled-Release Fertilizers*, in Controlled Release Fertilizers for Sustainable Agriculture, ed. F. B. Lewu, T. Volova, S. Thomas and K. R. Rakhimol, Academic Press, 2021, pp. 169–181, DOI:10.1016/B978-0-12-819555-0.00010-8.
- C. Wang, X. Liu, J. Li, L. Yue, H. Yang, H. Zou, Z. Wang and B. Xing, Copper Nanoclusters Promote Tomato (Solanum Lycopersicum L.) Yield and Quality through Improving Photosynthesis and Roots Growth, Environ. Pollut., 2021, 289, 117912, DOI:10.1016/j.envpol.2021.117912.
- P. Rai-Kalal and A. Jajoo, Priming with Zinc Oxide Nanoparticles Improve Germination and Photosynthetic Performance in Wheat, Plant Physiol. Biochem., 2021, 160, 341–351, DOI:10.1016/j.plaphy.2021.01.032.
- D. Sun, H. I. Hussain, Z. Yi, J. E. Rookes, L. Kong and D. M. Cahill, Mesoporous Silica Nanoparticles Enhance Seedling Growth and Photosynthesis in Wheat and Lupin, Chemosphere, 2016, 152, 81–91, DOI:10.1016/j.chemosphere.2016.02.096.
- B. Ahmad, A. Shabbir, H. Jaleel, M. M. A. Khan and Y. Sadiq, Efficacy of Titanium Dioxide Nanoparticles in Modulating Photosynthesis, Peltate Glandular Trichomes and Essential Oil Production and Quality in Mentha Piperita L, Curr. Plant Biol., 2018, 13, 6–15, DOI:10.1016/j.cpb.2018.04.002.
- M. Li, P. Zhang, Z. Guo, W. Cao, L. Gao, Y. Li, C. F. Tian, Q. Chen, Y. Shen, F. Ren, Y. Rui, J. C. White and I. Lynch, Molybdenum Nanofertilizer Boosts Biological Nitrogen Fixation and Yield of Soybean through Delaying Nodule Senescence and Nutrition Enhancement, ACS Nano, 2023, 17(15), 14761–14774, DOI:10.1021/acsnano.3c02783.
- H. Zhang, L. Lu, X. Zhao, S. Zhao, X. Gu, W. Du, H. Wei, R. Ji and L. Zhao, Metabolomics Reveals the “Invisible” Responses of Spinach Plants Exposed to CeO2 Nanoparticles, Environ. Sci. Technol., 2019, 53(10), 6007–6017, DOI:10.1021/acs.est.9b00593.
- A. Gautam, P. Sharma, S. Ashokhan, J. S. Yaacob, V. Kumar and P. Guleria, Magnesium Oxide Nanoparticles Improved Vegetative Growth and Enhanced Productivity, Biochemical Potency and Storage Stability of Harvested Mustard Seeds, Environ. Res., 2023, 229, 116023, DOI:10.1016/j.envres.2023.116023.
- C. Mony, P. Kaur, J. E. Rookes, D. L. Callahan, S. V. Eswaran, W. Yang and P. K. Manna, Nanomaterials for Enhancing Photosynthesis: Interaction with Plant Photosystems and Scope of Nanobionics in Agriculture, Environ. Sci.: Nano, 2022, 9(10), 3659–3683, 10.1039/D2EN00451H.
- P. Li, Y. Xia, K. Song and D. Liu, The Impact of Nanomaterials on Photosynthesis and Antioxidant Mechanisms in Gramineae Plants: Research Progress and Future Prospects, Plants, 2024, 13(7), 984, DOI:10.3390/plants13070984.
-
S. Sonkar, P. K. Sarangi, B. Pandey, A. Prakash and A. K. Singh, Response of Plant Photosynthesis to Nanomaterials, in Nanomaterial Interactions with Plant Cellular Mechanisms and Macromolecules and Agricultural Implications, ed. J. M. Al-Khayri, L. M. Alnaddaf and S. M. Jain, Springer International Publishing, Cham, 2023, pp. 49–67, DOI:10.1007/978-3-031-20878-2_3.
-
Q. u. l. Ain, H. A. Hussain, Q. Zhang, A. Rasheed, A. Imran, S. Hussain, N. Ahmad, H. Bibi and K. S. Ali, Chapter Thirteen - Use of Nano-Fertilizers to Improve the Nutrient Use Efficiencies in Plants, in Sustainable Plant Nutrition, ed. T. Aftab and K. R. Hakeem, Academic Press, 2023, pp. 299–321, DOI:10.1016/B978-0-443-18675-2.00013-4.
- M. Yang, C. Dong and Y. Shi, Nano Fertilizer Synergist Effects on Nitrogen Utilization and Related Gene Expression in Wheat, BMC Plant Biol., 2023, 23(1), 26, DOI:10.1186/s12870-023-04046-9.
- S. G. Abdel-Hakim, A. S. A. Shehata, S. A. Moghannem, M. Qadri, M. F. A. El-Ghany, E. A. Abdeldaym and O. S. Darwish, Nanoparticulate Fertilizers Increase Nutrient Absorption Efficiency and Agro-Physiological Properties of Lettuce Plant, Agronomy, 2023, 13(3), 691, DOI:10.3390/agronomy13030691.
- X. Xin, J. Nepal, A. L. Wright, X. Yang and Z. He, Carbon Nanoparticles Improve Corn (Zea Mays L.) Growth and Soil Quality: Comparison of Foliar Spray and Soil Drench Application, J. Cleaner Prod., 2022, 363, 132630, DOI:10.1016/j.jclepro.2022.132630.
- H. Hernández-Hernández, T. Quiterio-Gutiérrez, G. Cadenas-Pliego, H. Ortega-Ortiz, A. D. Hernández-Fuentes, M. Cabrera de la Fuente, J. Valdés-Reyna and A. Juárez-Maldonado, Impact of Selenium and Copper Nanoparticles on Yield, Antioxidant System, and Fruit Quality of Tomato Plants, Plants, 2019, 8(10), 355, DOI:10.3390/plants8100355.
- J. Hu, X. Wu, F. Wu, W. Chen, J. C. White, Y. Yang, B. Wang, B. Xing, S. Tao and X. Wang, Potential Application of Titanium Dioxide Nanoparticles to Improve the Nutritional Quality of Coriander (Coriandrum Sativum L.), J. Hazard. Mater., 2020, 389, 121837, DOI:10.1016/j.jhazmat.2019.121837.
- Y. Li, K. Xi, X. Liu, S. Han, X. Han, G. Li, L. Yang, D. Ma, Z. Fang, S. Gong, J. Yin and Y. Zhu, Silica Nanoparticles Promote Wheat Growth by Mediating Hormones and Sugar Metabolism, J. Nanobiotechnol., 2023, 21(1), 2, DOI:10.1186/s12951-022-01753-7.
- K. Shiono and S. Taira, Imaging of Multiple Plant Hormones in Roots of Rice(Oryza Sativa) Using Nanoparticle-Assisted Laser Desorption/Ionization Mass Spectrometry, J. Agric. Food Chem., 2020, 68(24), 6770–6775, DOI:10.1021/acs.jafc.0c00749.
- G. M. R. El-Shawa, K. Alharbi, M. AlKahtani, L. AlHusnain, K. A. Attia and K. Abdelaal, Improving the Quality and Production of Philodendron Plants Using Nanoparticles and Humic Acid, Horticulturae, 2022, 8(8), 678, DOI:10.3390/horticulturae8080678.
- H. Zhang, R. Wang, Z. Chen, P. Cui, H. Lu, Y. Yang and H. Zhang, The Effect of Zinc Oxide Nanoparticles for Enhancing Rice (Oryza Sativa L.) Yield and Quality, Agriculture, 2021, 11(12), 1247, DOI:10.3390/agriculture11121247.
- M. Kolenčík, D. Ernst, M. Komár, M. Urík, M. Šebesta, Ľ. Ďurišová, M. Bujdoš, I. Černý, J. Chlpík, M. Juriga, R. Illa, Y. Qian, H. Feng, G. Kratošová, K. Č. Barabaszová, L. Ducsay and E. Aydın, Effects of Foliar Application of ZnO Nanoparticles on Lentil Production, Stress Level and Nutritional Seed Quality under Field Conditions, Nanomaterials, 2022, 12(3), 310, DOI:10.3390/nano12030310.
- M. Nekoukhou, S. Fallah, A. Abbasi-Surki, L. R. Pokhrel and A. Rostamnejadi, Improved Efficacy of Foliar Application of Zinc Oxide Nanoparticles on Zinc Biofortification, Primary Productivity and Secondary Metabolite Production in Dragonhead, J. Cleaner Prod., 2022, 379, 134803, DOI:10.1016/j.jclepro.2022.134803.
- R. Ahmed, M. K. Uddin, M. A. Quddus, M. Y. A. Samad, M. A. M. Hossain and A. N. A. Haque, Impact of Foliar Application of Zinc and Zinc Oxide Nanoparticles on Growth, Yield, Nutrient Uptake and Quality of Tomato, Horticulturae, 2023, 9(2), 162, DOI:10.3390/horticulturae9020162.
- E. Yusefi-Tanha, S. Fallah, L. R. Pokhrel and A. Rostamnejadi, Addressing Global Food Insecurity: Soil-Applied Zinc Oxide Nanoparticles Promote Yield Attributes and Seed Nutrient Quality in Glycine Max L, Sci. Total Environ., 2023, 876, 162762, DOI:10.1016/j.scitotenv.2023.162762.
- G. Yang, H. Yuan, H. Ji, H. Liu, Y. Zhang, G. Wang, L. Chen and Z. Guo, Effect of ZnO Nanoparticles on the Productivity, Zn Biofortification, and Nutritional Quality of Rice in a Life Cycle Study, Plant Physiol. Biochem., 2021, 163, 87–94, DOI:10.1016/j.plaphy.2021.03.053.
- M. K. Abou El-Nasr, H. M. El-Hennawy, M. S. F. Samaan, T. A. Salaheldin, A. Abou El-Yazied and A. El-Kereamy, Using Zinc Oxide Nanoparticles to Improve the Color and Berry Quality of Table Grapes Cv. Crimson Seedless, Plants, 2021, 10(7), 1285, DOI:10.3390/plants10071285.
- M. Faizan, A. Faraz and S. Hayat, Effective Use of Zinc Oxide Nanoparticles through Root Dipping on the Performance of Growth, Quality, Photosynthesis and Antioxidant System in Tomato, J. Plant Biochem. Biotechnol., 2020, 29(3), 553–567, DOI:10.1007/s13562-019-00525-z.
- R. Wang, K. Mi, X. Yuan, J. Chen, J. Pu, X. Shi, Y. Yang, H. Zhang and H. Zhang, Zinc Oxide Nanoparticles Foliar Application Effectively Enhanced Zinc and Aroma Content in Rice (Oryza Sativa L.) Grains, Rice, 2023, 16(1), 36, DOI:10.1186/s12284-023-00653-0.
- S. Saleem, A. Malik and S. T. Khan, ZnO Nanoparticles in Combination with Zn Biofertilizer Improve Wheat Plant Growth and Grain Zn Content without Significantly Changing the Rhizospheric Microbiome, Environ. Exp. Bot., 2023, 213, 105446, DOI:10.1016/j.envexpbot.2023.105446.
- Z. Lv, M. Zhong, Q. Zhou, Z. Li, H. Sun, J. Bai, J. Liu and H. Mao, Nutrient Strengthening of Winter Wheat by Foliar ZnO and Fe3O4 NPs: Food Safety, Quality, Elemental Distribution and Effects on Soil Bacteria, Sci. Total Environ., 2023, 893, 164866, DOI:10.1016/j.scitotenv.2023.164866.
- N. Gupta, S. K. Jain, B. S. Tomar, A. Anand, J. Singh, V. Sagar, R. Kumar, V. Singh, T. Chaubey, K. A. Abd-Elsalam and A. K. Singh, Impact of Foliar Application of ZnO and Fe3O4 Nanoparticles on Seed Yield and Physio-Biochemical Parameters of Cucumber (Cucumis Sativus L.) Seed under Open Field and Protected Environment Vis a Vis during Seed Germination, Plants, 2022, 11(23), 3211, DOI:10.3390/plants11233211.
- Y. Wang, S. Wang, M. Xu, L. Xiao, Z. Dai and J. Li, The Impacts of γ-Fe2O3 and Fe3O4 Nanoparticles on the Physiology
and Fruit Quality of Muskmelon (Cucumis Melo) Plants, Environ. Pollut., 2019, 249, 1011–1018, DOI:10.1016/j.envpol.2019.03.119.
- D. B. Dola, M. A. Mannan, U. Sarker, M. A. A. Mamun, T. Islam, S. Ercisli, M. H. Saleem, B. Ali, O. L. Pop and R. A. Marc, Nano-iron oxide accelerates growth, yield, and quality of Glycine max seed in water deficits, Front. Plant Sci., 2022, 13, 992535, DOI:10.3389/fpls.2022.992535.
- S. Azizkhani, T. Javadi, N. Ghaderi and A. Farzinpour, Replacing Conventional Iron with Cysteine-Coated Fe3O4 Nanoparticles in Soilless Culture of Strawberry, Sci. Hortic., 2023, 318, 112098, DOI:10.1016/j.scienta.2023.112098.
- A. W. M. Mahmoud, A. A. Ayad, H. S. M. Abdel-Aziz, L. L. Williams, R. M. El-Shazoly, A. Abdel-Wahab and E. A. Abdeldaym, Foliar Application of Different Iron Sources Improves Morpho-Physiological Traits and Nutritional Quality of Broad Bean Grown in Sandy Soil, Plants, 2022, 11(19), 2599, DOI:10.3390/plants11192599.
- C. A. Garza-Alonso, G. Cadenas-Pliego, A. Juárez-Maldonado, J. A. González-Fuentes, G. Tortella and A. Benavides-Mendoza, Fe2O3 Nanoparticles Can Replace Fe-EDTA Fertilizer and Boost the Productivity and Quality of Raphanus Sativus in a Soilless System, Sci. Hortic., 2023, 321, 112261, DOI:10.1016/j.scienta.2023.112261.
- H. S. El-Desouky, K. R. Islam, B. Bergefurd, G. Gao, T. Harker, H. Abd-El-Dayem, F. Ismail, M. Mady and R. M. Y. Zewail, Nano Iron Fertilization Significantly Increases Tomato Yield by Increasing Plants' Vegetable Growth and Photosynthetic Efficiency, J. Plant Nutr., 2021, 44(11), 1649–1663, DOI:10.1080/01904167.2021.1871749.
- S. F. El-Gioushy, Z. Ding, A. M. E. Bahloul, M. S. Gawish, H. M. Abou El Ghit, A. M. R. A. Abdelaziz, H. S. El-Desouky, R. Sami, E. Khojah, T. A. Hashim, A. M. S. Kheir and R. M. Y. Zewail, Foliar Application of Nano, Chelated, and Conventional Iron Forms Enhanced Growth, Nutritional Status, Fruiting Aspects, and Fruit Quality of Washington Navel Orange Trees (Citrus Sinensis L. Osbeck), Plants, 2021, 10(12), 2577, DOI:10.3390/plants10122577.
- D. Kanjana, Foliar Application of Magnesium Oxide Nanoparticles on Nutrient Element Concentrations, Growth, Physiological, and Yield Parameters of Cotton, J. Plant Nutr., 2020, 43(20), 3035–3049, DOI:10.1080/01904167.2020.1799001.
- H. J. Shareef, R. A. Al-Yahyai, A. E.-D. K. Omar and W. A. Barus, Foliar Nano-Fertilization Enhances Fruit Growth, Maturity, and Biochemical Responses of Date Palm, Can. J. Plant Sci., 2021, 101(3), 299–306, DOI:10.1139/cjps-2020-0081.
- L. Ahamad, A. Ali khan, M. Khan, O. Farid and M. Alam, Exploring the Nano-Fungicidal Efficacy of Green Synthesized Magnesium Oxide Nanoparticles (MgO NPs) on the Development, Physiology, and Infection of Carrot (Daucus Carota L.) with Alternaria Leaf Blight(ALB): Molecular Docking, J. Integr. Agric., 2023, 22(10), 3069–3080, DOI:10.1016/j.jia.2023.02.034.
- N. A. H. Abdelfattah, M. A. Yousef, A. A. Badawy and S. S. Salem, Influence of Biosynthesized Magnesium Oxide Nanoparticles on Growth and Physiological Aspects of Cowpea (Vigna Unguiculata L.) Plant, Cowpea Beetle, and Cytotoxicity, Biotechnol. J., 2023, 18(12), 2300301, DOI:10.1002/biot.202300301.
- S. A. Osman, D. M. Salama, M. E. Abd El-Aziz, E. A. Shaaban and M. S. Abd Elwahed, The Influence of MoO3-NPs on Agro-Morphological Criteria, Genomic Stability of DNA, Biochemical Assay, and Production of Common Dry Bean (Phaseolus Vulgaris L.), Plant Physiol. Biochem., 2020, 151, 77–87, DOI:10.1016/j.plaphy.2020.03.009.
- M. F. Abdulhameed, A. A. Taha and R. A. Ismail, Improvement of Cabbage Growth and Yield by Nanofertilizers and Nanoparticles, Environ. Nanotechnol., Monit. Manage., 2021, 15, 100437, DOI:10.1016/j.enmm.2021.100437.
- J. Yang, F. Jiang, C. Ma, Y. Rui, M. Rui, M. Adeel, W. Cao and B. Xing, Alteration of Crop Yield and Quality of Wheat upon Exposure to Silver Nanoparticles in a Life Cycle Study, J. Agric. Food Chem., 2018, 66(11), 2589–2597, DOI:10.1021/acs.jafc.7b04904.
- M. Wang, Y. Wang, C. Ge, H. Wu, F. Jing, S. Wu, H. Li and D. Zhou, Foliar Selenium Nanoparticles Application Promotes the Growth of Maize (Zea Mays L.) Seedlings by Regulating Carbon, Nitrogen and Oxidative Stress Metabolism, Sci. Hortic., 2023, 311, 111816, DOI:10.1016/j.scienta.2022.111816.
- A. Razzaq, P. Kaur, N. Akhter, S. H. Wani and F. Saleem, Next-Generation Breeding Strategies for Climate-Ready Crops, Front. Plant Sci., 2021, 12, 620420, DOI:10.3389/fpls.2021.620420.
- L. Zhao, T. Bai, H. Wei, J. L. Gardea-Torresdey, A. Keller and J. C. White, Nanobiotechnology-Based Strategies for Enhanced Crop Stress Resilience, Nat. Food, 2022, 3(10), 829–836, DOI:10.1038/s43016-022-00596-7.
- A. D. Hernández-Fuentes, E. R. López-Vargas, J. M. Pinedo-Espinoza, R. G. Campos-Montiel, J. Valdés-Reyna and A. Juárez-Maldonado, Postharvest Behavior of Bioactive Compounds in Tomato Fruits Treated with Cu Nanoparticles and NaCl Stress, Appl. Sci., 2017, 7(10), 980, DOI:10.3390/app7100980.
- L. M. Mahmoud, A. M. Shalan, M. S. El-Boray, C. I. Vincent, M. E. El-Kady, J. W. Grosser and M. Dutt, Application of Silicon Nanoparticles Enhances Oxidative Stress Tolerance in Salt Stressed ‘Valencia’ Sweet Orange Plants, Sci. Hortic., 2022, 295, 110856, DOI:10.1016/j.scienta.2021.110856.
- I. F. Hassan, R. Ajaj, M. S. Gaballah, C. C. Ogbaga, H. M. Kalaji, H. M. Hatterman-Valenti and S. M. Alam-Eldein, Foliar Application of Nano-Silicon Improves the Physiological and Biochemical Characteristics of ‘Kalamata’ Olive Subjected to Deficit Irrigation in a Semi-Arid Climate, Plants, 2022, 11(12), 1561, DOI:10.3390/plants11121561.
- N. G. Mohamed and M. A. Abdel-Hakeem, Chitosan Nanoparticles Enhance Drought Tolerance in Tomatoes (Solanum Lycopersicum L) via Gene Expression Modulation, Plant Gene, 2023, 34, 100406, DOI:10.1016/j.plgene.2023.100406.
- L. Sun, R. Wang, Q. Ju, M. Xing, R. Li, W. Li, W. Li, W. Wang, Y. Deng and J. Xu, Mitigation mechanism of zinc oxide nanoparticles on cadmium toxicity in tomato, Front. Plant Sci., 2023, 14, 1162372, DOI:10.3389/fpls.2023.1162372.
- S. Faiz, N. A. Yasin, W. U. Khan, A. A. Shah, W. Akram, A. Ahmad, A. Ali, N. H. Naveed and L. Riaz, Role of Magnesium Oxide Nanoparticles in the Mitigation of Lead-Induced Stress in Daucus Carota: Modulation in Polyamines and Antioxidant Enzymes, Int. J. Phytorem., 2022, 24(4), 364–372, DOI:10.1080/15226514.2021.1949263.
- F. Hayat, F. Khanum, J. Li, S. Iqbal, U. Khan, H. U. Javed, M. K. Razzaq, M. A. Altaf, Y. Peng, X. Ma, C. Li, P. Tu and J. Chen, Nanoparticles and Their Potential Role in Plant Adaptation to Abiotic Stress in Horticultural Crops: A Review, Sci. Hortic., 2023, 321, 112285, DOI:10.1016/j.scienta.2023.112285.
- G. Sun, Z. Dong, G. Li, H. Yuan, J. Liu, X. Yao, J. Gu, H. Wu and Z. Li, Mn3O4 Nanoparticles Alleviate ROS-Inhibited Root Apex Mitosis Activities to Improve Maize Drought Tolerance, Adv. Biol., 2023, 7(7), 2200317, DOI:10.1002/adbi.202200317.
- J. Liu, J. Gu, J. Hu, H. Ma, Y. Tao, G. Li, L. Yue, Y. Li, L. Chen, F. Cao, H. Wu and Z. Li, Use of Mn3O4 Nanozyme to Improve Cotton Salt Tolerance, Plant Biotechnol. J., 2023, 21(10), 1935–1937, DOI:10.1111/pbi.14145.
- D. Kumar, O. P. Dhankher, R. D. Tripathi and C. S. Seth, Titanium Dioxide Nanoparticles Potentially Regulate the Mechanism(s) for Photosynthetic Attributes, Genotoxicity, Antioxidants Defense Machinery, and Phytochelatins Synthesis in Relation to Hexavalent Chromium Toxicity in Helianthus Annuus L, J. Hazard. Mater., 2023, 454, 131418, DOI:10.1016/j.jhazmat.2023.131418.
- C. Zou, T. Lu, R. Wang, P. Xu, Y. Jing, R. Wang, J. Xu and J. Wan, Comparative Physiological and Metabolomic Analyses Reveal That Fe3O4 and ZnO Nanoparticles Alleviate Cd Toxicity in Tobacco, J. Nanobiotechnol., 2022, 20(1), 302, DOI:10.1186/s12951-022-01509-3.
- A. Rizwan, M. Zia-ur-Rehman, M. Rizwan, M. Usman, S. Anayatullah, Areej, H. F. Alharby, A. A. Bamagoos, B. M. Alharbi and S. Ali, Effects of Silicon Nanoparticles and Conventional Si Amendments on Growth and Nutrient Accumulation by Maize (Zea Mays L.) Grown in Saline-Sodic Soil, Environ. Res., 2023, 227, 115740, DOI:10.1016/j.envres.2023.115740.
- N. Kandhol, V. P. Singh, J. Peralta-Videa, F. J. Corpas and D. K. Tripathi, Silica Nanoparticles: The Rising Star in Plant Disease Protection, Trends Plant Sci., 2022, 27(1), 7–9, DOI:10.1016/j.tplants.2021.10.007.
- Y. Wang, L. Yang, X. Zhou, Y. Wang, Y. Liang, B. Luo, Y. Dai, Z. Wei, S. Li, R. He and W. Ding, Molecular Mechanism of Plant Elicitor Daphnetin-Carboxymethyl Chitosan Nanoparticles against Ralstonia Solanacearum by Activating Plant System Resistance, Int. J. Biol. Macromol., 2023, 241, 124580, DOI:10.1016/j.ijbiomac.2023.124580.
- J. Chen, L. Wu, K. Song, Y. Zhu and W. Ding, Nonphytotoxic Copper Oxide Nanoparticles Are Powerful “Nanoweapons” That Trigger Resistance in Tobacco against the Soil-Borne Fungal Pathogen Phytophthora Nicotianae, J. Integr. Agric., 2022, 21(11), 3245–3262, DOI:10.1016/j.jia.2022.08.086.
- E. O. Mikhailova, Silver Nanoparticles: Mechanism of Action and Probable Bio-Application, J. Funct. Biomater., 2020, 11(4), 84, DOI:10.3390/jfb11040084.
- D.-Y. Kim, S. K. S. Patel, K. Rasool, N. Lone, S. K. Bhatia, C. S. Seth and G. S. Ghodake, Bioinspired Silver Nanoparticle-Based Nanocomposites for Effective Control of Plant Pathogens: A Review, Sci. Total Environ., 2024, 908, 168318, DOI:10.1016/j.scitotenv.2023.168318.
- J. Tang, X. Tong, Y. Chen, Y. Wu, Z. Zheng, A. B. Kayitmazer, A. Ahmad, N. Ramzan, J. Yang, Q. Huang and Y. Xu, Deposition and Water Repelling of Temperature-Responsive Nanopesticides on Leaves, Nat. Commun., 2023, 14(1), 6401, DOI:10.1038/s41467-023-41878-3.
- K. Wang, Y. Wang, Y. Wu, J. Jiang, Y. Zhang, N. Yu and Z. Liu, A Novel Dual Stimuli-Responsive and Double-Loaded Insecticidal Nanoformulation for Efficient Control of Insect Pest, Chem. Eng. J., 2023, 474, 146012, DOI:10.1016/j.cej.2023.146012.
- M. Zong, C. Yu, J. Li, D. Sun, J. Wang, Z. Mo, C. Qin, D. Yang, Z. Zhang, Q. Zeng, C. Li, K. Ma, H. Wan, J. Li and S. He, Redox and Near-Infrared Light-Responsive Nanoplatform for Enhanced Pesticide Delivery and Pest Control in Rice: Construction, Efficacy, and Potential Mechanisms, ACS Appl. Mater. Interfaces, 2023, 15(35), 41351–41361, DOI:10.1021/acsami.3c08413.
- X. Guan, X. Gao, A. Avellan, E. Spielman-Sun, J. Xu, S. Laughton, J. Yun, Y. Zhang, G. D. Bland, Y. Zhang, R. Zhang, X. Wang, E. A. Casman and G. V. Lowry, CuO Nanoparticles Alter the Rhizospheric Bacterial Community and Local Nitrogen Cycling for Wheat Grown in a Calcareous Soil, Environ. Sci. Technol., 2020, 54(14), 8699–8709, DOI:10.1021/acs.est.0c00036.
- M.-X. Zhang, L.-Y. Zhao, Y.-Y. He, J.-P. Hu, G.-W. Hu, Y. Zhu, A. Khan, Y.-C. Xiong and J.-L. Zhang, Potential Roles of Iron Nanomaterials in Enhancing Growth and Nitrogen Fixation and Modulating Rhizomicrobiome in Alfalfa (Medicago Sativa L.), Bioresour. Technol., 2024, 391, 129987, DOI:10.1016/j.biortech.2023.129987.
- L. Liu, O. V. Tsyusko, J. M. Unrine, S. Liu, Y. Liu, L. Guo, G. Wei and C. Chen, Pristine and Sulfidized Zinc Oxide Nanoparticles Promote the Release and Decomposition of Organic Carbon in the Legume Rhizosphere, Environ. Sci. Technol., 2023, 57(24), 8943–8953, DOI:10.1021/acs.est.3c02071.
- D. Li, C. Zhou, Y. Wu, Q. An, J. Zhang, Y. Fang, J.-Q. Li and C. Pan, Nanoselenium Integrates Soil-Pepper Plant Homeostasis by Recruiting Rhizosphere-Beneficial Microbiomes and Allocating Signaling Molecule Levels under Cd Stress, J. Hazard. Mater., 2022, 432, 128763, DOI:10.1016/j.jhazmat.2022.128763.
- T. Ahmed, M. Noman, J. L. Gardea-Torresdey, J. C. White and B. Li, Dynamic Interplay between Nano-Enabled Agrochemicals and the Plant-Associated Microbiome, Trends Plant Sci., 2023, 28(11), 1310–1325, DOI:10.1016/j.tplants.2023.06.001.
- M. Li, L. Gao, J. C. White, C. L. Haynes, T. L. O'Keefe, Y. Rui, S. Ullah, Z. Guo, I. Lynch and P. Zhang, Nano-Enabled Strategies to Enhance Biological Nitrogen Fixation, Nat. Nanotechnol., 2023, 1–4, DOI:10.1038/s41565-023-01392-5.
- A. De Souza-Torres, E. Govea-Alcaide, E. Gómez-Padilla, S. H. Masunaga, F. B. Effenberger, L. M. Rossi, R. López-Sánchez and R. F. Jardim, Fe3O4 Nanoparticles and Rhizobium Inoculation Enhance Nodulation, Nitrogen Fixation and Growth of Common Bean Plants Grown in Soil, Rhizosphere, 2021, 17, 100275, DOI:10.1016/j.rhisph.2020.100275.
- M. D. Groppa, M. S. Zawoznik, M. P. Benavides and M. F. Iannone, Beneficial effects of magnetite nanoparticles on soybean-Bradyrhizobium japonicum and alfalfa-Sinorhizobium meliloti associations, Plant Physiol. Biochem., 2022, 180, 42–49, DOI:10.1016/j.plaphy.2022.03.025.
- K. Velandia, J. B. Reid and E. Foo, Right Time, Right Place: The Dynamic Role of Hormones in Rhizobial Infection and Nodulation of Legumes, Plant Commun., 2022, 3(5), 100327, DOI:10.1016/j.xplc.2022.100327.
- J. Wang, X. Cao, C. Wang, F. Chen, Y. Feng, L. Yue, Z. Wang and B. Xing, Fe-Based Nanomaterial-Induced Root Nodulation Is Modulated by Flavonoids to Improve Soybean (Glycine Max) Growth and Quality, ACS Nano, 2022, 16(12), 21047–21062, DOI:10.1021/acsnano.2c08753.
- H. Tian, M. Kah and K. Kariman, Are Nanoparticles a Threat to Mycorrhizal and Rhizobial Symbioses? A Critical Review, Front. Microbiol., 2019, 10, 1660, DOI:10.3389/fmicb.2019.01660.
- H. Sun, Q. Peng, J. Guo, H. Zhang, J. Bai and H. Mao, Effects of Short-Term Soil Exposure of Different Doses of ZnO Nanoparticles on the Soil Environment and the Growth and Nitrogen Fixation of Alfalfa, Environ. Pollut., 2022, 309, 119817, DOI:10.1016/j.envpol.2022.119817.
- P. J. Boersma, F. Lagugné-Labarthet, T. McDowell and S. M. Macfie, Silver Nanoparticles Inhibit Nitrogen Fixation in Soybean (Glycine Max) Root Nodules, Environ. Sci. Pollut. Res., 2023, 30(11), 32014–32031, DOI:10.1007/s11356-022-24446-y.
- Y. C. Huang, R. Fan, M. A. Grusak, J. D. Sherrier and C. P. Huang, Effects of Nano-ZnO on the Agronomically Relevant Rhizobium–Legume Symbiosis, Sci. Total Environ., 2014, 497–498, 78–90, DOI:10.1016/j.scitotenv.2014.07.100.
- J. Moll, A. Okupnik, A. Gogos, K. Knauer, T. D. Bucheli, M. G. A. van der Heijden and F. Widmer, Effects of Titanium Dioxide Nanoparticles on Red Clover and Its Rhizobial Symbiont, PLoS One, 2016, 11(5), e0155111, DOI:10.1371/journal.pone.0155111.
- Z. Yuan, Z. Zhang, X. Wang, L. Li, K. Cai and H. Han, Novel Impacts of Functionalized Multi-Walled Carbon Nanotubes in Plants: Promotion of Nodulation and Nitrogenase Activity in the Rhizobium-Legume System, Nanoscale, 2017, 9(28), 9921–9937, 10.1039/C7NR01948C.
- J. Ma, Y. Zhou, J. Li, Z. Song and H. Han, Novel Approach to Enhance Bradyrhizobium Diazoefficiens Nodulation through Continuous Induction of ROS by Manganese Ferrite Nanomaterials in Soybean, J. Nanobiotechnol., 2022, 20(1), 168, DOI:10.1186/s12951-022-01372-2.
- D. Jiang, D. Ni, Z. T. Rosenkrans, P. Huang, X. Yan and W. Cai, Nanozyme: New Horizons for Responsive Biomedical Applications, Chem. Soc. Rev., 2019, 48(14), 3683–3704, 10.1039/C8CS00718G.
- J. Ma, Z. Song, J. Yang, Y. Wang and H. Han, Cobalt Ferrite Nanozyme for Efficient Symbiotic Nitrogen Fixation via Regulating Reactive Oxygen Metabolism, Environ. Sci.: Nano, 2021, 8(1), 188–203, 10.1039/D0EN00935K.
|
This journal is © The Royal Society of Chemistry 2025 |
Click here to see how this site uses Cookies. View our privacy policy here.