DOI:
10.1039/D4TB01957A
(Review Article)
J. Mater. Chem. B, 2025,
13, 15-36
Recent advances of versatile fluorophores for multifunctional biomedical imaging in the NIR-II region
Received
30th August 2024
, Accepted 29th October 2024
First published on 30th October 2024
Abstract
Fluorescence imaging in the second near-infrared region (NIR-II, 1000–1700 nm) enables high-resolution visualization of deep-tissue biological architecture and physiopathological events, due to the reduced light absorption, scattering and tissue autofluorescence. Numerous versatile NIR-II fluorescent probes have been reported over the past decades. In this review, we first provide a detailed account of the advantages of fluorescence imaging in the NIR-II region. Following this, the classification, design and performance optimization strategies of NIR-II fluorescent probes are systematically discussed, along with a broad range of biomedical applications in vivo. Finally, the discussion extends to the next generation of fluorescent probes for in vivo imaging and the challenges and perspectives for the clinical translation of fluorescence imaging technology in the NIR-II region.
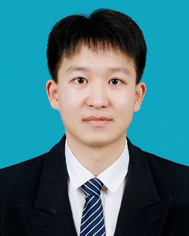
Kaiming Ma
| Kaiming Ma is pursuing his master's degree in the College of Energy Materials and Chemistry, at Inner Mongolia University under the direction of Prof. Fan Zhang. His research interests focus on NIR-II luminescent materials for bioimaging and biosensing. |
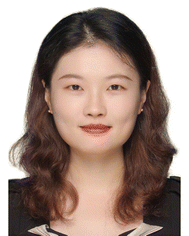
Qunying Jiang
| Qunying Jiang acquired her bachelor's degree (2016) from Lanzhou University and her PhD degree from Wuhan University (2021). She is now joining the College of Energy Materials and Chemistry, at Inner Mongolia University. Her research interests focus on fluorescent probes for bioimaging and biosensing. |
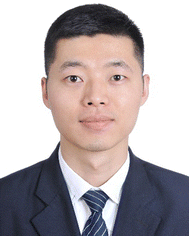
Yang Yang
| Yang Yang received his PhD from Lanzhou University in 2019, and then conducted postdoctoral research at Fudan University under the direction of Prof. Fan Zhang. Currently, he is a professor at Inner Mongolia University. His research interests focus on the design and synthesis of NIR-I/II fluorescent probes for bioimaging and biosensing. |
1. Introduction
In vivo imaging technology has emerged as a pivotal technique in both fundamental scientific research and clinical diagnostics. Traditional in vivo imaging modalities, such as computed tomography (CT),1 magnetic resonance imaging (MRI),2 ultrasound imaging (USI),3 positron emission tomography (PET),4 and single-photon emission computed tomography (SPECT),5 have been extensively used for preoperative examinations, disease diagnosis, monitoring, and prognostic evaluations over the past few decades. In contrast, fluorescence imaging has gained increasing attention due to its high sensitivity, non-ionizing radiation, non-invasive real-time detection, and cost-effectiveness, making it a compelling choice in biomedical research.6 However, fluorescence imaging still faces challenges. The light signals emitted by the fluorescent probes used in traditional fluorescence imaging typically fall within the visible (VIS, 400–700 nm) or first near-infrared (NIR-I, 700–1000 nm) regions, which are prone to tissue absorption, scattering, and background autofluorescence. This susceptibility limits imaging penetration depth, resolution, and signal-to-noise ratio (SNR). As a result, traditional fluorescence imaging techniques are often suitable only for superficial tissues or specially prepared ex vivo samples (such as tissue slices, chemical clearing, or tissue expansion),7,8 or they require invasive operations (such as cranial windows) to image deep tissues in vivo.9
In 2009, Smith and colleagues first proposed the second “tissue transparency window” ranging from 1000 to 1700 nm, which was later defined as the second near-infrared window (NIR-II).10 The NIR-II window was further subdivided into NIR-IIa (1300–1400 nm) and NIR-IIb (1500–1700 nm) subregions due to a water overtone absorption peak at 1450 nm (Fig. 1A).11 Recent studies have highlighted the relationship between the penetration depth of light in biological tissues and scattering. The reduced scattering coefficient
, which varies inversely with the wavelength λ (
, where α = 0.2–4, depending on tissue types such as skin, muscle, fat, bone, brain, etc.), indicates that scattering decreases as wavelength increases (Fig. 1A). Moreover, the autofluorescence of biological tissues also diminishes with increasing wavelength (Fig. 1B).12 Therefore, compared to traditional fluorescence imaging in the VIS and NIR-I regions, fluorescence imaging in the NIR-II region offers greater tissue penetration depth, higher resolution, and an improved SNR. Over the past decade, advancements in the manufacturing of a NIR indium gallium arsenide (InGaAs) detector have accelerated the development of fluorescence imaging in the NIR-II region. Initially applied in the wide-field whole-body imaging of small animals, the NIR-II fluorescence imaging technology has progressed to applications in three-dimensional confocal and light-sheet microscopy.13 Researchers have continually innovated NIR-II fluorescent probes, including lanthanide nanoparticles (LnNPs), quantum dots (QDs), single-wall carbon nanotubes (SWCNTs), gold nanoclusters (AuNCs), organic small molecules, and semiconductor polymer nanoparticles (SPNPs). These advances have enabled non-invasive, high-resolution imaging of cranial brain vasculature and tumor vascular networks, showcasing the significant potential of NIR-II fluorescence imaging technology in biomedical research.
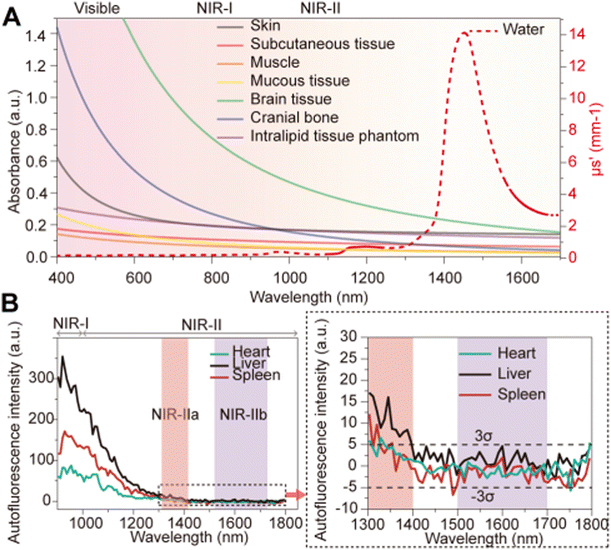 |
| Fig. 1 (A) Reduced scattering of different biological tissues and intralipid solution in the range of 400–1700 nm. Reproduced with permission.11 Copyright 2015, American Chemical Society. (B) Autofluorescence spectra of ex vivo mouse liver (black), spleen (red), and heart tissue (blue) under 808 nm excitation. Reproduced with permission.12 Copyright 2017, Nature Publishing Group. | |
This retrospective review aims to summarize recent advancements in NIR-II fluorophores for biomedical imaging. Firstly, we elaborate in detail on the advantages of fluorescence imaging in the NIR-II region. Next, we systematically introduce the versatile characteristics of NIR-II fluorophores and highlight several representative fluorescent probes. Subsequently, the review comprehensively explores the applications of NIR-II fluorophores in biomedical imaging, including anatomical imaging, pathophysiological imaging, molecular imaging, and surgical navigation. Finally, we envisage potential future applications of fluorescence imaging in the NIR-II region in biomedicine and analyze the challenges it faces in clinical translation.
2. Advantages of fluorescence imaging in the NIR-II region
During fluorescence imaging, both incident excitation light and emitted light from contrast agents interact with biological tissues (Fig. 2A),14 potentially compromising penetration depth, SNR, and resolution. In 2013, Jacques elaborated on the optical properties of biological tissues and summarized the photophysical parameters involved in photon–tissue interactions, providing essential theoretical underpinnings for fluorescence imaging.15 When excitation light enters biological tissues, it initially undergoes reflection at the tissue interface (Fig. 2A). In 2006, Ding et al.16 provided the refractive indices of human skin tissues for different wavelengths, revealing significantly lower indices in the dermis and epidermis for light in the NIR-II region compared to that in the VIS and NIR-I region (Fig. 2B). Furthermore, the absorption coefficient (μa) of biological tissues in the VIS region is generally related to endogenous molecules, while in the NIR-II region, it is closely associated with water absorption. Various biological tissues generally exhibit markedly lower μa in the NIR-II region (Fig. 2C). Additionally, Jacques and Hong et al.14,15 summarized the
curves of different biological tissues at various wavelengths (Fig. 1A), demonstrating that the
values in the NIR-II region are primarily influenced by Mie scattering, resulting in lower values that are inversely proportional to the wavelength. The significantly reduced interaction of light with biological tissues implies that NIR-II light offers enhanced potential for achieving high-quality fluorescence imaging in deep tissues in vivo.
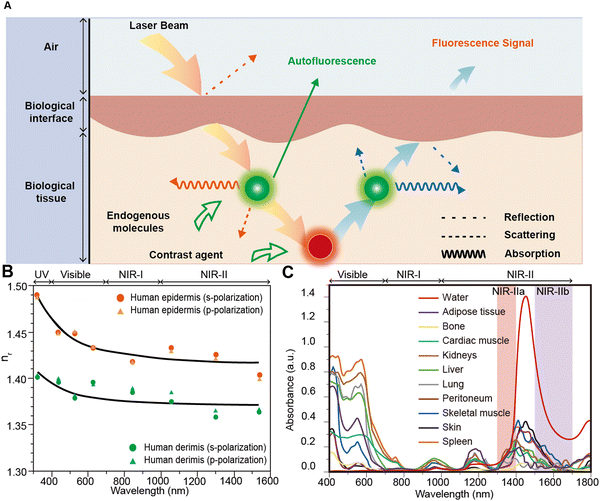 |
| Fig. 2 (A) Schematic diagram of the interactions between the photons and tissue when executing fluorescence imaging. Reproduced with permission.14 Copyright 2017, Springer Nature. (B) The refractive indices of human epidermis and dermis at different wavelengths. UV, ultraviolet; NIR-I, first near-infrared; and NIR-II, second near-infrared. (C) Absorption curves of various biological tissues in the 400–1800 nm region (from top to bottom, water, adipose tissue, myocardium, kidneys, liver, lung tissue, peritoneum, skeletal muscle, skin, and spleen, respectively). Reproduced with permission.16 Copyright 1956, IOP Publishing. | |
2.1 Deep penetration depth
Deep penetration depth offers more opportunities and details for observing deep tissues in vivo using fluorescence imaging. Penetration depth is closely related to the transmittance of light through tissues. Over the past two decades, scientists have extensively studied the transmittance of various biological tissues, laying the foundation for further modeling the penetration depth of light in biological tissues (Fig. 3A).17 Visible light is heavily absorbed and scattered within biological tissues, limiting penetration depth to the skin surface or superficial tissues. In contrast, due to the lower μa and
, NIR-II light can achieve a penetration depth of approximately 1 cm into biological tissues. This capability is crucial for visualizing deep organs and tissues in medical imaging, facilitating more precise assessments of internal conditions by physicians.
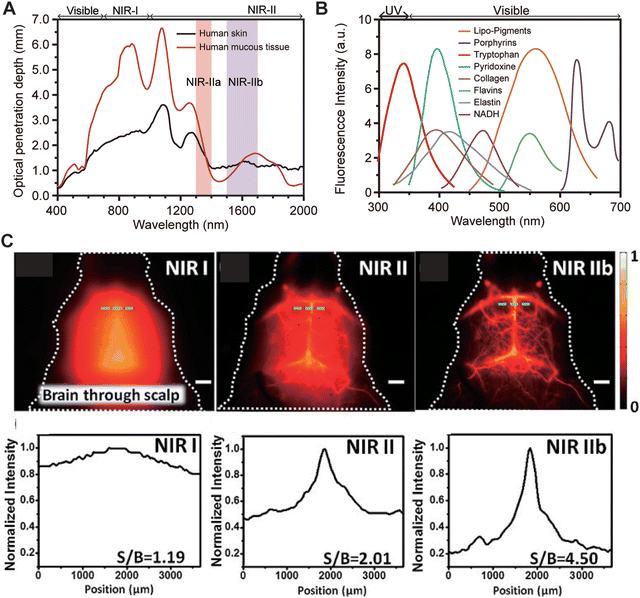 |
| Fig. 3 (A) Penetration depth of diffuse photons in the wavelength range of 400–2000 nm in human skin and mucus tissues. Reproduced with permission.17 Copyright 1970, IOP Publishing. (B) Emission spectra of common endogenous chromogenic groups in living organisms (notes from top to bottom: lipid pigments, porphyrins, tryptophan, pyridoxine, collagen, flavins, elastin, and nicotinamide adenine dinucleotide). Reproduced with permission.12 Copyright 2015, Springer Nature. (C) Fluorescence images of the cerebrovascular system of mice without craniotomy in the NIR-I, NIR-II, and NIR-IIb regions, with the corresponding signal-to-background ratio (SBR) analysis. Reproduced with permission.18 Copyright 2015, Wiley-VCH GmbH. | |
2.2 High signal-to-noise ratio
A high SNR indicates greater clarity of useful information in an image, with minimal interference from noise, directly influencing imaging resolution. Therefore, maintaining a robust SNR is critical for ensuring image clarity, accuracy, and clinical reliability in vivo. However, background autofluorescence poses a significant challenge in in vivo fluorescence imaging, severely impacting both imaging resolution and SNR. In 2005, Monici et al.19 summarized the autofluorescence characteristics of cells and biological tissues, highlighting common endogenous chromophores such as lipo-pigments, porphyrins, tryptophan, pyridoxine, collagen, flavins, elastin, and nicotinamide adenine dinucleotide (NADH), whose emission peaks predominantly lie in the VIS region (Fig. 3B). This results in substantial background fluorescence interference during imaging within this wavelength range. In stark contrast, background autofluorescence in the NIR-II region is notably reduced, enabling NIR-II fluorescence imaging to achieve a high signal-to-background ratio (SBR) in vivo (Fig. 1B). In 2013, Dai et al.18 demonstrated non-invasive brain vascular imaging in mice at a depth of 3 mm using SWCNTs (Fig. 3C). Due to severe scattering and background autofluorescence, the brain vascular imaging in the NIR-I region was very blurry, with an SNR of only 1.19. Extending the imaging wavelength to the NIR-II region significantly improved the SNR to 2.01, while further extension to the NIR-IIb region remarkably clarified the details of brain vasculature, achieving an SNR of 4.50, surpassing values obtained in the NIR-I and NIR-II regions.
2.3 High spatial resolution
In biomedical imaging, achieving high spatial resolution is crucial for obtaining clear images of tissues and cells, enabling early disease diagnosis and precise detection, thereby improving treatment outcomes. Moreover, high spatial resolution enhances imaging accuracy, promoting a deeper understanding of disease mechanisms and biological processes. When detecting optical signals, emitted light undergoes scattering, and the
of biological tissues determines the spatial resolution of these signals (Fig. 4A).17 The scattering of light by biological tissue exhibits significant wavelength dependence, with notably lower
in the NIR-II region, creating an optimal window for low-scattering optical signals and significantly enhancing spatial resolution. In 2022, Dai et al.20 reported biocompatible core–shell lead sulfide/cadmium sulfide QDs with an emission around 1880 nm and achieved NIR-II fluorescence imaging under 1319 nm, 1540 nm, and 1650 nm laser excitation (Fig. 4B–D). Increasing the excitation wavelength progressively improved the spatial resolution of vascular imaging. Notably, high-resolution imaging at depths exceeding 1100 μm in the mouse head was achieved only with a 1650 nm excitation wavelength.
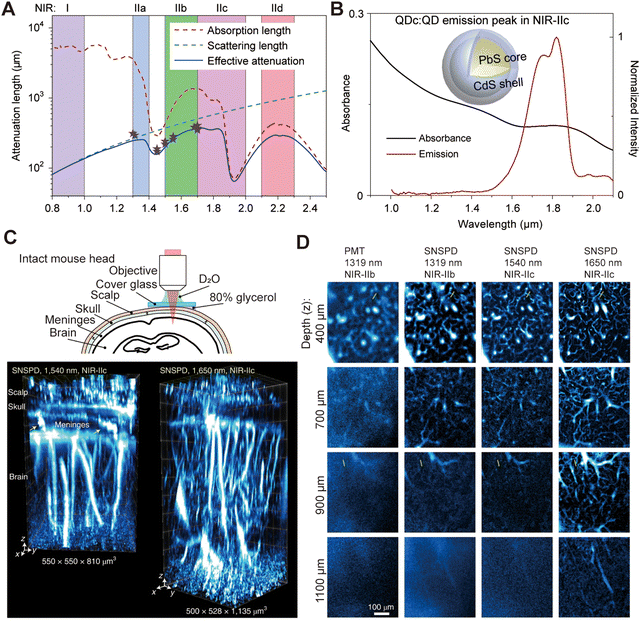 |
| Fig. 4 (A) Wavelength-dependent attenuation length of brain tissue. Reproduced with permission.17 Copyright 1970, IOP Publishing. (B) Emission spectrum and schematic design of NIR-II core–shell PbS/CdS quantum dots. (C) Schematic of intact mouse head imaging in the NIR-IIc (1700–2000 nm) window. Three-dimensional volumetric images of blood vessels in an intact mouse head are visualized through the scalp, skull, meninges, and brain cortex. Arrows indicate vessel-like channels connecting the skull to the brain cortex in the meninges. (D) High-resolution confocal images of blood vessels at various depths in an intact mouse head, captured using a PMT or SNSPD in the NIR-IIb or NIR-IIc window. (B)–(D) Reproduced with permission.20 Copyright 2022, Springer Nature. | |
3. NIR-II fluorophores
With the widespread application of NIR-II fluorescence imaging in the biomedical field, researchers have developed versatile NIR-II fluorophores, including LnNPs, QDs, SWCNTs, AuNCs, organic small molecules, and SPNPs. An ideal NIR-II fluorophore should possess a long wavelength, high brightness, excellent biocompatibility, long lifetime, and either targeting capability or activatability. These properties enable the fluorophore to emit bright fluorescence at specific sites with low doses, thereby achieving high-contrast in vivo fluorescence imaging.
3.1 Lanthanide nanoparticles
LnNPs typically consist of an inorganic crystal matrix with embedded rare-earth ions within the lattice. Renowned for their high stability, narrow emission bands, tunable wavelengths, and long lifetimes, they have gained widespread attention in NIR-II fluorescence imaging.21 Their luminescence primarily arises from the unique electronic transitions of rare-earth ions within the 4f orbitals. Due to the shielding effect of the outer 5s and 5p electrons, the influence of external environments on these energy levels is minimal, resulting in consistent absorption and emission wavelengths. By adjusting the composition of rare-earth elements, NIR-II upconversion or downconversion luminescence can be easily achieved (Fig. 5A). In 2019, Zhang et al.22 synthesized Tm3+/Er3+ co-doped LnNPs:NaYF4/Er3+@NaYF4, achieving fluorescence emission at 1525 nm under 1208 nm excitation. Chen et al.10 explored Ce3+ as a sensitizing ion, enhancing the energy transfer efficiency (85.1% for Nd3+ and 82.6% for Er3+) to achieve NIR-II emission, overcoming the inefficiencies of 4f–4f transitions (Fig. 5B and C). The low molar extinction coefficient (ε) of rare-earth ions as sensitizers limits the brightness of LnNPs. To address this limitation, Zhang et al.23 utilized the transition metal element Cr3+ as a sensitizer, developing a new class of Cr3+-sensitized lanthanide-doped nanoparticles (CLNPs). In CLNPs, Cr3+ within the shell sensitizes the lanthanide activator ions in the core through interface energy transfer. This sensitization enhances the efficiency by 20-fold and increases brightness by 280-fold compared to traditional lanthanide-sensitized downshifting nanoparticles (DSNPs). Dai et al.24 improved NIR-II fluorescence by applying inert or active shells on the surface of LnNPs, thereby reducing the quenching effects of solvent molecules on the luminescent centers and increasing the capture capability of excitation light. They also demonstrated that Ce3+ doping and cross-relaxation with Er3+ inhibited the upconversion processes of Er3+ at the 4I11/2 energy level, boosting the fluorescence intensity of Er3+ at 1550 nm (Fig. 5D and E). Surface modification of LnNPs with organic molecules of higher absorption cross-sections significantly enhances brightness. N. Prasad et al.25 enhanced the brightness of NaYF4/X3+@NaYbF4@NaYF4 (X = Er, Ho, Tm, or Pr) core/shell/shell nanocrystals by fourfold through surface modification with indocyanine green (ICG), utilizing the energy transfer pathway of ICG → Nd3+ (outer shell) → Yb3+ (inner shell) → Yb3+/X3+ (core). Despite the substantial potential of LnNPs for NIR-II bioimaging, the poor water solubility and susceptibility to concentration quenching of rare-earth ions remain pressing issues to be addressed.
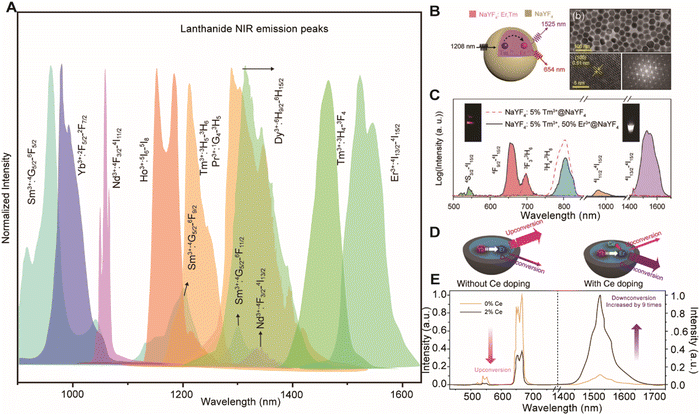 |
| Fig. 5 (A) NIR luminescence spectra of Sm3+, Yb3+, Nd3+, Ho3+, Tm3+, Pr3+, Dy3+, and Er3+ in crystal hosts. Reproduced with permission.26 Copyright 2018, Springer Nature. (B) Schematic design and luminescence spectrum of NaYF4:5%Tm3+,50%Er3+@NaYF4 nanocrystals. Reproduced with permission.22 Copyright 2019, Wiley-VCH GmbH. (C) Schematic design of a NaYbF4:Er,Ce@NaYF4 core–shell nanocrystal, and luminescence spectra of the Er-RENPs with 0 and 2% Ce3+ doping. Reproduced with permission. (D) Schematic illustration of the proposed energy-transfer mechanisms in Er-RENPs with and without Ce3+ doping. (E) Upconversion and downconversion luminescence spectra of the Er-RENPs with 0 and 2% Ce3+ doping.24 Copyright 2018, Springer Nature. | |
3.2 Quantum dots
QDs are semiconductor nanocrystals typically ranging from 1 to 10 nm in diameter. Their unique optical properties stem from the quantum confinement effect, where reducing the particle size increases the bandgap of nanocrystals.27 Therefore, by controlling the size and composition of QDs, emission wavelengths in the NIR-II region can be easily achieved (Fig. 6A). Early NIR-II QDs, however, often contained toxic elements such as Cd, Pb, Hg, and As, found in CdTe, PbSe, HgTe, PbS and InAs QDs, limiting their in vivo imaging applications despite the potential for emitting wavelengths into the mid-infrared region. To address toxicity concerns, there has been a shift towards developing non-toxic or low-toxicity NIR-II QDs. In 2013, Wang et al.28 synthesized Ag2Se QDs using a solvothermal method. These QDs, functionalized with amphiphilic C18-PMH-PEG polymers on their surface, exhibited a narrower bandgap (∼0.15 eV) compared to Ag2S QDs, enabling emission up to 1300 nm without heavy metal elements. This formulation demonstrated excellent biocompatibility, promising applications in in vivo imaging. Additionally, Bawendi et al.29 developed core–shell structured QDs by coating InAs QDs with CdS, CdSe, and ZnSe, achieving up to 30% quantum yield (QY) in aqueous solution with tunable emission wavelengths. Beyond core–shell structures, biomolecule encapsulation, such as coating QDs with bovine serum albumin or ribonuclease A, has emerged as another strategy to mitigate toxicity risks by preventing direct interactions of QDs with biological interfaces (Fig. 6B).30
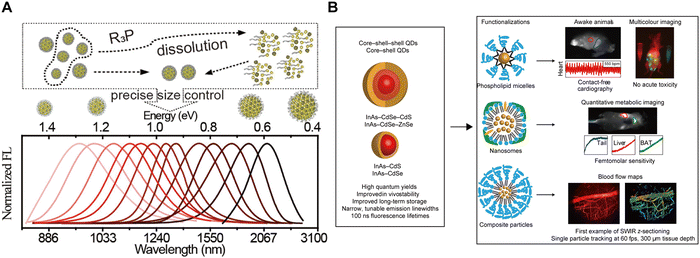 |
| Fig. 6 (A) InAs core QDs were coated with various shell materials to enable fine-tuning of the emission spectrum. Reproduced with permission.28 Copyright 2013, American Chemical Society. (B) The synthesized core–shell and core–shell–shell QDs were functionalized with three distinct surface coatings to tailor their physiological properties for specific NIR-II imaging applications. Reproduced with permission.29 Copyright 2017, Springer Nature. | |
3.3 Single-walled carbon nanotubes
SWCNTs are one-dimensional carbon nanomaterials composed of a single layer of graphene, arranged in a cylindrical structure with diameters ranging from sub-nanometers to several nanometers.31 SWCNTs exhibit exceptional physical properties, including high thermal and electrical conductivity, as well as outstanding photostability, capable of enduring prolonged laser irradiation.32 Moreover, SWCNTs demonstrate low biotoxicity, ensuring minimal interference with cellular processes such as division and growth.33 Due to their Van Hove singularity transitions across the bandgap, SWCNTs emit wavelengths from 800 to 2000 nm, making them promising candidates for in vivo fluorescence imaging in the NIR-II region. However, early SWCNTs were prone to aggregation during synthesis, resulting in reduced fluorescence brightness. To overcome this issue, Smalley et al.34 introduced large quantities of surfactants during ultrasonic treatment of SWCNT aggregates in 2002, leading to the production of surfactant-coated monodisperse SWCNTs (Fig. 7A). Despite a modest QY of 0.1%, these SWCNTs provided valuable insights and paved the way for the development of brighter variants. In 2007, Hertel et al.35 achieved a QY of up to 11% by dispersing SWCNTs in iodixanol and employing gradient centrifugation. In 2009, Dai et al.10 pioneered the use of phospholipid-functionalized SWCNTs as NIR-II fluorescent probes for in vivo imaging, marking a significant milestone in NIR-II fluorescence imaging (Fig. 7B and C).
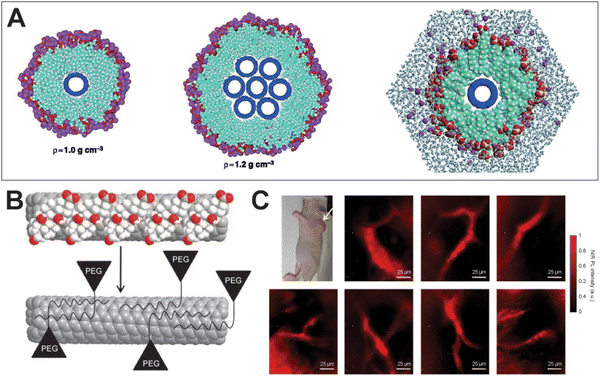 |
| Fig. 7 (A) A single fullerene nanotube in a cylindrical sodium dodecyl sulfate (SDS) micelle (left) and a seven-tube bundle of fullerene nanotubes coated by a layer of SDS (middle). A molecular dynamics simulation of water and the SDS micelle around a single (8,8) nanotube (left). Reproduced with permission.34 Copyright 2002, The American Association for the Advancement of Science. (B) Cholate (red and white balls) on SWNTs (grey) is dialyzed and eventually replaced by phospholipid–polyethylene glycol to form biocompatible nanotubes without damaging the integrity of the nanotube sidewall. (C) Optical image of a LS174T tumor-bearing mouse using exchange-SWNTs. Reproduced with permission.10 Copyright 2009, Springer Nature. | |
3.4 Gold nanoclusters
AuNCs are highly biocompatible due to their small size, minimizing accumulation in organs and reducing the risk of immune response or toxicity, making them safe for in vivo fluorescence imaging applications.36 Furthermore, the size, shape, and surface chemistry of AuNCs can be precisely controlled through synthesis methods to optimize their optical properties and biocompatibility for various biological applications. For instance, in 2019, Liu et al.37 developed Au25 clusters with 25 gold atoms and 18 peptide ligands, emitting wavelengths from 1100 to 1350 nm. Intravenous injection (i.v.) of these AuNCs showed that 86.6% were excreted via urine and 2.2% via feces within 48 h, with kidneys primarily involved in metabolism. In 2023, Huang et al.38 designed bright and stable NIR-II Au24Cd1 clusters to mitigate oxidative stress and acute neuroinflammation from acute kidney injury (AKI) (Fig. 8A and B). Seven days post-injection, Au24Cd1 was nearly negligible in major organs, demonstrating rapid clearance. Optimizing AuNCs with ligands such as acetylcholine and β-cyclodextrin can minimize interactions with biological tissues, enhance renal clearance, and biocompatibility, thus addressing potential biosafety concerns. Dai et al.39 developed AuNC probes functionalized with phosphatidylcholine (PS) ligands, exhibiting “super stealth” properties with minimal interaction with serum proteins, cells, and tissues. ICP-MS analysis showed that approximately 81% of AuNCs were excreted in urine within 1 h post-intravenous injection, increasing to about 93% within 24 h. Similarly, Song et al.40 prepared CD-AuNCs by introducing cyclodextrin onto the surfaces of AuNCs. The CD-AuNCs showed peak fluorescence in the bladder 3 h after intravenous injection, with NIR-II signals diminishing after 24 h, indicating nearly complete urinary excretion (Fig. 8C–E). In conclusion, rational structural design and renal clearance control of AuNCs enable effective applications in drug delivery, renal disease diagnostics, and other biomedical fields.
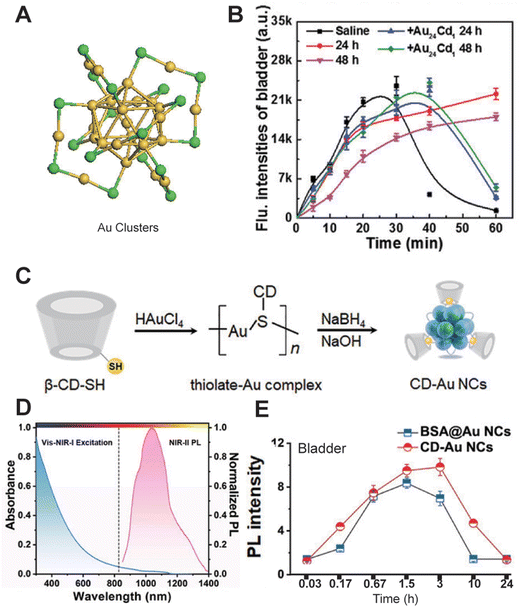 |
| Fig. 8 (A) Structural characterization of Au24Cd1 clusters. (B) NIR-II fluorescence intensities of the bladder after intravenous injection of Au24Cd1. Reproduced with permission.38 Copyright 2023, Wiley-VCH GmbH. (C) Schematic illustration of the synthesis process of CD-Au NCs. (D) Absorption spectrum in the visible/NIR-I region and NIR-II PL spectrum (excitation: 808 nm) of CD-Au NCs. (E) Corresponding NIR-II PL signal changes in the bladder. (C)–(E) Reproduced with permission.40 Copyright 2020, Wiley-VCH GmbH. | |
3.5 Organic small molecules
Organic small molecule fluorophores, prized for their compact structure, excellent biocompatibility, reproducible synthesis, and ease of derivatization, have become pivotal in advancing in vivo imaging technologies.41 Recently, a variety of versatile NIR-II small molecule fluorophores have been developed for NIR-II fluorescence imaging, including polymethine cyanines, benzobisthiadiazole (BBTD) fluorophores, boron dipyrromethene (BODIPY) difluoride, and rhodamine derivatives (Fig. 9A).42–46 Polymethine cyanines, typically containing a central conjugated methine chain and two heterocyclic terminal moieties, have straightforward synthesis processes, exceptionally strong ε, and longer absorption/emission maxima (λmax,abs/λem,max), making them advantageous in NIR-II fluorescence imaging. In 2013, Dai et al.47 utilized commercially available heptamethine cyanine IR-1061, co-assembled with polyacrylic acid (PAA) and DSPE-mPEG (a polyethylene glycol-conjugated phospholipid), to create nanoprobe IR-PEG for NIR-II fluorescence imaging of mouse organs and systemic vasculature (Fig. 9B and C). In 2015, Dai et al.48 reported the first donor–acceptor–acceptor (D–A–D) NIR-II organic small molecule fluorophore, CH1055, incorporating BBTD as the electron acceptor and triphenylamine as the electron donor. Modified with polyethylene glycol, CH1055 demonstrated superior stability and renal clearance, with approximately 90% excreted via urine within 24 h. It enabled precise imaging of sentinel lymph nodes and lymphatic vessels, showing a SNR twice that of FDA-approved ICG (Fig. 9D–F). BODIPY fluorophores, renowned for their stability and high QY, have also gained considerable attention in NIR-II fluorescence imaging. Zhang et al.49 developed FBP912 in 2021, a BODIPY-based NIR-II fluorophore modified with a brush-like polymer (OEGMA) for renal clearance. FBP912 exhibited a high QY of 0.086%, significantly outperforming traditional PEGylated FBPEG912 with 0.027%. Notably, FBP912 demonstrated an extended circulation time of 6.1 h (Fig. 9G–I). Li et al.50 developed NIR-II aza-BODIPY dyes with significant Stokes shifts, exceptional photostability, and strong fluorescence brightness by modulating the electron-donating ability of the donor group. This advancement enables high-contrast in vivo imaging. Rhodamine dyes, known for their high ε, excellent QY, and photostability, have been widely applied in NIR-II fluorescence imaging. In 2024, Zhang et al.51 developed 2X-rhodamine, a novel dye with adjusted heteroatoms and a rigid ring structure, exhibiting robust fluorescence beyond 1000 nm (Fig. 9J–M). Operating through a rigid π-framework and a dimeric bridge structure, 2X-rhodamine surpasses traditional rhodamine dyes with its longer wavelength and higher QY, promising expanded applications in biomedical imaging and offering new insights for future fluorophore development.
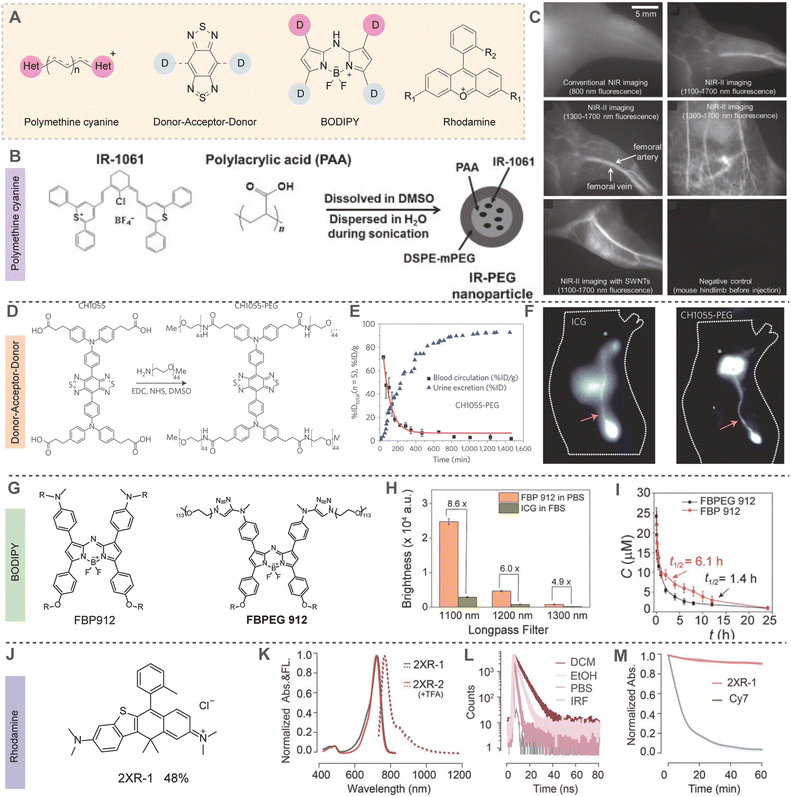 |
| Fig. 9 (A) Chemical structures of several representative NIR-II organic small molecules. (B) Synthesis of biocompatible IR-PEG nanoparticles with fluorescence emission >1000 nm. (C) In vivo NIR imaging of the hindlimb and abdomen of nude mice with fluorophores circulating in the blood streams after intravenous injection. (A)–(C) Reproduced with permission.47 Copyright 2013, Wiley-VCH GmbH. (D) Chemical structure of CH1055 and the one-step synthesis of CH1055-PEG. (E) CH1055-PEG agglomerated cumulative urine excretion curve for five mice (%ID) as well as blood circulation (%ID per g) time points fitted with an exponential decay obtained during the 24 h post-injection (error bars correspond to standard deviation). (F) NIR-I fluorescence image (100 ms) after an ICG injection and NIR-II fluorescence image (300 ms) after a CH1055-PEG injection, showing the inguinal lymph node and lymphatic vasculature in each imaging window in two different mice (n = 3). (D)–(F) Reproduced with permission.48 Copyright 2015, Springer Nature. (G) The chemical structures of FBP912 and FBPEG912. (H) Brightness comparison of FBP912 in PBS and ICG in FBS under 808 nm laser excitation with various long-pass filters. (I) Blood concentration (μM) decay of FBP912 and FBPEG912 after intravenous injection into ICR mice. (G)–(I) Reproduced with permission.49 Copyright 2021, Wiley-VCH GmbH. (J) The chemical structures of the 2XR-1. (K) Normalized absorption and emission spectra of 2XR-1 and 2XR-2 (with 0.1% v/v trifluoroacetic acid) in ethanol. (L) Fluorescence lifetime curves of 2XR-1 in dichloromethane, ethanol, and phosphate buffer (10 mM, pH = 7.4), respectively. (M) Photobleaching profiles of 2XR-1 and Cy7 in phosphate buffer (10 mM, pH = 7.4). (J)–(M) Reproduced with permission.51 Copyright 2024, American Chemical Society. | |
3.6 Semiconductor polymer nanoparticles
SPNPs, utilizing a donor–acceptor (D–A) structure as the molecular unit, have found diverse applications in electronic devices such as organic light-emitting diodes and organic solar cells.3 The photophysical properties of SPNPs can be finely tuned by increasing the number of molecular units or modulating the structure of donor and acceptor segments. In 2014, Dai et al.52 pioneered the synthesis of a series of NIR-II-emitting SPNPs, pDA, through Stille coupling reactions (Fig. 10A). To enhance biocompatibility, they prepared uniform-sized pDA-PEG nanoparticles dispersible in water via nanoprecipitation. pDA-PEG exhibited absorption and emission peaks at 654 nm and 1047 nm, respectively (Fig. 10B). By changing the receptor units of SPNPs, the λem,max can be red-shifted. Huang et al.53 achieved a red shift of the λem,max of PBQx from 1050 nm to 1348 nm by increasing the number of repeating units of the SPNPs. After intravenous injection, the accumulation of PBQ45 nanoparticles in the liver and spleen decreased after 48 h. Additionally, 96 h post-injection, the excretion rate of PBQ45 nanoparticles from the body reached 31.7% (Fig. 10C–F). Subsequently, significant contributions were made by the Pu and Tang groups, who developed NIR-II SPNPs such as SPN2, SP3, and pNIR-3.54–58 Due to their relatively large molecular weight, the metabolic rate of SPNPs in biological organisms is relatively slow, which somewhat limits their application. In 2019, Pu et al.59 reported CDIR2, a renal-clearable SPNP with a QY of 2.2%. Following intravenous injection, 90% of CDIR2 was metabolized within 24 h, exclusively excreted via glomerular filtration into urine without renal tubular reabsorption or secretion. This unidirectional renal clearance pathway of CDIR2 enables real-time monitoring of renal function impairment in live mice exposed to nephrotoxic agents, offering valuable insights for improving the renal clearance efficiency of NIR-II fluorophores.
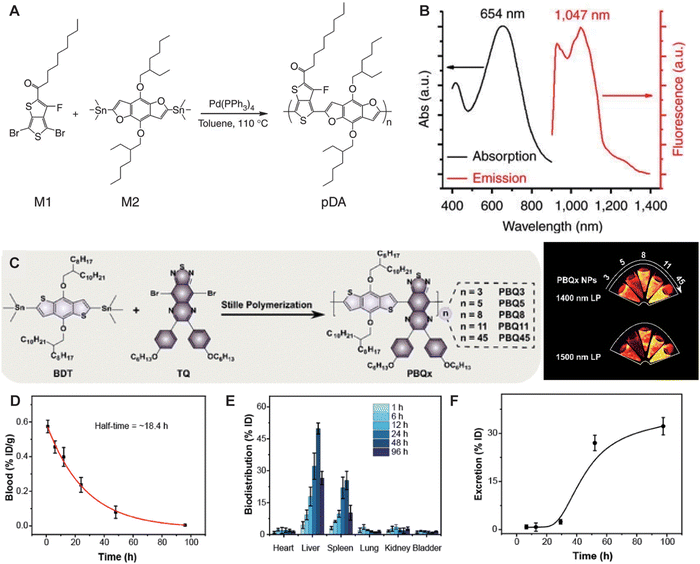 |
| Fig. 10 (A) This scheme shows the chemical structures of the two monomers M1 and M2, along with the structure of the pDA polymer, poly(benzo[1,2-b:3,4-b′]difuran-alt-fluorothieno-[3,4-b]thiophene). (B) Absorption and emission spectra of pDA-PEG, featuring a large Stokes shift of ∼400 nm. Reproduced with permission.52 Copyright 2014, Springer Nature. (C) Synthetic route to PBQx. (D) The concentration of PBQ45 NPs in blood samples. (E) The distribution of PBQ45 NPs in different organs. (F) The excretion behavior of PBQ45 NPs over a period of 96 h (bottom). (C)–(F) Reproduced with permission.53 Copyright 2022, American Chemical Society. | |
4. Multifunctional NIR-II biomedical imaging
With the advent of versatile NIR-II fluorophores, NIR-II fluorescence imaging has emerged as a powerful tool in both fundamental biological sciences and clinical medical research.60 This technology is now extensively utilized in anatomical imaging, pathophysiological imaging, molecular imaging, and surgical navigation.
4.1 Anatomical imaging
Anatomical imaging aids physicians and researchers in visualizing and analyzing the internal structures of living organisms, enhancing the understanding of the functions and abnormalities of tissues, organs, and systems, and providing insights into disease development and diagnostic processes. NIR-II fluorescence imaging, with its advantages of deep penetration, high resolution, and high SNR, facilitates the imaging of visceral organs, vascular systems, lymphatic systems, bones, and pathological sites such as tumors. In 2022, Lee et al.61 successfully developed a high-brightness semiconductor oligomer (IDSe-IC2F) with NIR-IIb emission through precise atomic substitution strategies (Fig. 11A). Using long-pass filters at different wavelengths (1000 nm, 1300 nm, and 1500 nm), IDSe-IC2F achieved high-contrast vascular imaging with SBRs of 1.16, 1.55, and 25.90, respectively. Additionally, four-minute capillaries could be distinctly identified in vivo, with SNRs of 7.48, 16.11, 20.96, and 22.04 (Fig. 11B). In 2023, Sun et al.62 designed and synthesized a series of heptamethine cyanines with tunable emission wavelengths, high brightness, and large Stokes shifts (Fig. 11C). Among them, YH-1c allowed visualization of microvessels approximately 10 μm in diameter, even in deeper regions of mouse limbs and abdomen (Fig. 11D). Notably, the circulation time of YH-1c in blood is 15 times longer than ICG, offering new possibilities for prolonged in vivo imaging. Modification of the xanthene core by substituting oxygen with silicon is known to induce bathochromic shifts of the emission wavelength by ∼90 nm. In 2024, Meador et al.63 investigated the combination of a silicon-substituted xanthene core with DMA-decorated indolizine donors to access longer wavelengths, thereby enabling high-resolution imaging of vessels such as the femoral artery, abdominal cavity, and carotid artery in mice (Fig. 11E–G).
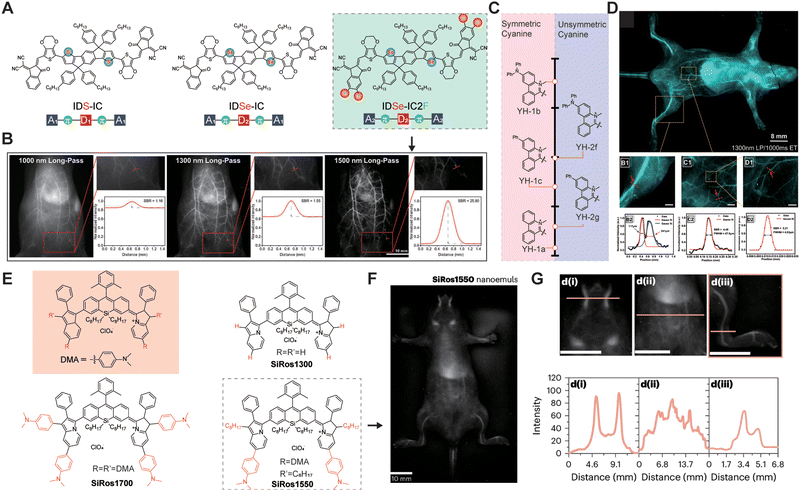 |
| Fig. 11 (A) Chemical structure of NIR-II molecules. (B) Whole-body imaging of IDSe-IC2F NP labeled blood vessels using filters of different long-pass wavelengths with the corresponding SBR analysis. Reproduced with permission.61 Copyright 2022, Wiley-VCH GmbH. (C) Heterocyclic structure and their maximum emission wavelength indicated by arrows. (D) In vivo vascular imaging of BALB/c nude mice with YH-1c NPs. (B1) and (B2) The femoral vascular imaging and fitting of the line intensity of the blood vessel highlighted in panels (B1) to calculate the SBR and diameter. (C1) and (C2) The lower abdominal vascular imaging and the corresponding fitted curve of fluorescence intensity in the delineated region. (D1) The highlighted area of panel (C1) with 5-fold magnification. (D2) Normalized fluorescence intensity of the highlighted area with red line (D1) and Gaussian fitting curve. Reproduced with permission.62 Copyright 2022, American Chemical Society. (E) Emission maxima of xanthene and silicon-substituted xanthene-based fluorophores. (F) In vivo fluorescence images of SiRos1550 nanoemulsion. (G) SiRos1550 nanoemulsion following tail vein injection along with cross-sectional intensities depicted in d(i)–(iii) below each figure. (E)–(G) Reproduced with permission.63 Copyright 2024, Springer Nature. | |
Bones, tendons, and muscles collectively form the body's dynamic system, playing an irreplaceable role in maintaining mobility, immune protection, and metabolism. The integrity of bones is crucial for overall health, as damage or abnormalities can lead to conditions such as skeletal deformities, osteoporosis, rheumatoid arthritis, and osteoarthritis. Therefore, achieving in vivo bone imaging is of paramount importance for understanding and treating bone-related diseases. In 2020, Liu et al.64 designed gold nanoclusters (Au25(SG)18) that specifically bind to hydroxyapatite in bones. These nanoclusters can be rapidly cleared by the kidneys and enable high-resolution NIR-II imaging of ribs and thoracic vertebrae, with SBRs of 2.39 and 2.33, respectively. Guided by NIR-II fluorescence imaging, the entry points and directions for vertebral screw implantation can be determined more safely. This application reveals the potential of AuNCs for targeted drug delivery to bones and for guiding spinal surgeries. Mi et al.65 utilized rare earth nanoparticles (ErNCs) with an emission wavelength of 1550 nm to diagnose bone defects at a depth of 1 mm (Fig. 12A–C). Traditional X-ray imaging confirmed the defect's diameter to be approximately 1 mm, while ErNCs measured the diameter of the left hind limb defect to be 1.2 mm. This study demonstrated that NIR-II imaging can effectively monitor bone defects over an 11-day period. Additionally, water-soluble organic small molecules have been designed for bone imaging. Sletten et al.66 reported a high-brightness NIR-II fluorophore, PhosphoChrom7, with four phosphate groups. These phosphate groups enable PhosphoChrom7 to bind to calcium minerals in bones, facilitating high-resolution imaging of the ventral mandible, sternum, tibia, and phalanges (Fig. 12D–G).
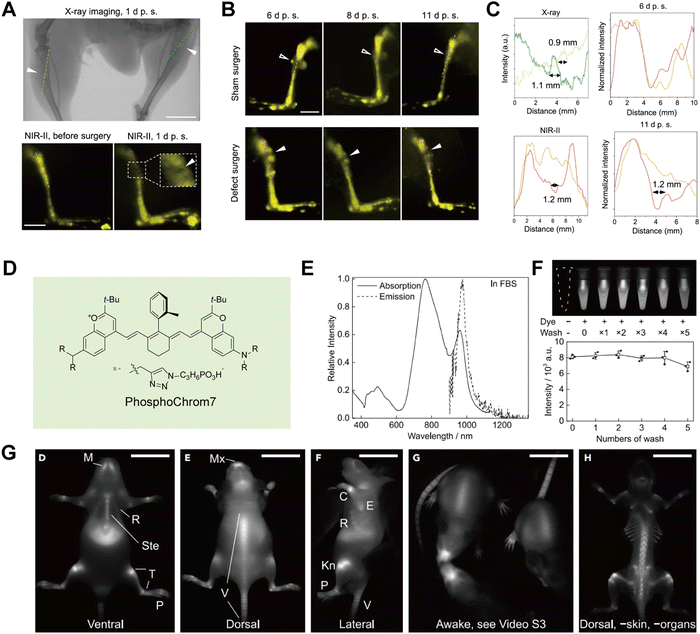 |
| Fig. 12 (A) Comparison between X-ray and NIR-II in vivo imaging on the tibial defects (solid arrowheads). (B) The NIR-II in vivo imaging on the right (sham surgery as control) and left hindlimb (defect surgery) of a mouse on days 6, 8, and 11 after surgery. (C) The intensity profiles along the cross sections are indicated by the corresponding-colored dashed lines in a. The measured sizes of the tibia defects are given. The intensity profiles along the cross sections are represented by the dashed lines in both the sham surgery group (orange) and bone defect surgery group (red) in b. (A)–(C) Reproduced with permission.65 Copyright 2023, Springer Nature. (D) Molecular structure of PhosphoChrom7. (E) Absorption and emission spectra of PhosphoChrom7 in FBS. (F) Fluorescence image of calcium hydroxyapatite suspension in bovine serum treated with PhosphoChrom7, and its subsequent washes with bovine serum. (G) Representative single frame shows fluorescence image of awake mice 48 h after injection and fluorescence images of a mouse after removal of abdominal organs and most of the skin on the dorsal view. (D)–(G) Reproduced with permission.66 Copyright 2023, Elsevier. | |
Barium meal and endoscopic techniques are the primary methods for gastrointestinal (GI) imaging. However, the barium meal is an X-ray-based diagnostic technique with limited spatial resolution and significant radiation risks. Endoscopy, being an invasive diagnostic procedure, is contraindicated for patients with suspected intestinal strictures or obstructions. Thus, there is a strong need for non-invasive GI imaging techniques. In 2022, Li et al.67 reported gold nanoclusters (RNase-A@AuNCs) grafted with ribonuclease A on their surface. RNase-A@AuNCs exhibit a maximum emission wavelength of 1050 nm and a QY of 1.9% (Fig. 13A). These nanoclusters enable real-time detection of GI peristalsis and can be used to examine intestinal tumor nodules with diameters as small as 2.5 mm, presenting a promising approach for diagnosing intestinal tumors (Fig. 13B).
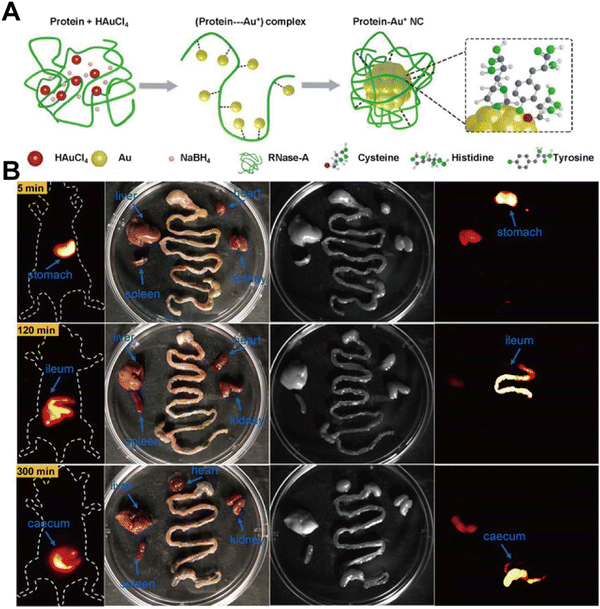 |
| Fig. 13 (A) Schematic representation of the reaction path for the synthesis of RNase-A@AuNCs. (B) NIR-II imaging of gastrointestinal peristalsis by RNase-A@AuNCs. Reproduced with permission.67 Copyright 2023, Wiley-VCH GmbH. | |
Lymph nodes are small, bean-shaped structures in the immune system located along lymphatic vessels, responsible for filtering lymph fluid and capturing foreign microorganisms and cancer cells. Zhu et al.68 developed an NIR-II biomimetic fluorescent protein system. Compared to the unmodified fluorophore CO-1080, the protein-modified HSA@CO-1080 fluorophore offers enhanced lymphatic imaging capabilities, successfully achieving precise localization of tumor sentinel lymph nodes and guiding tumor resection during surgery (Fig. 14A and B). Wang et al.39 constructed NIR-II fluorescent gold nanoclusters (Au-PC) with a “stealth” coating through phosphorylcholine modification. Au-PC can clearly identify lymph nodes with a SNR of up to 22. Unlike ICG dye, Au-PC does not diffuse or nonspecifically bind to biomolecules, resulting in lower background signals and enabling clearer, more targeted lymph node imaging (Fig. 14C and D).
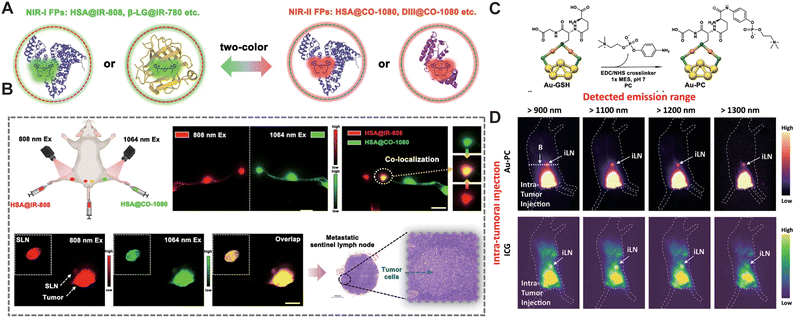 |
| Fig. 14 (A) Schematic of the biomimetic NIR-I FPs and NIR-II FPs constructed via a biomimetic strategy. (B) Dual-color lymph node imaging and colocalization using HSA@IR-808 FPs and HSA@CO-1080 FPs. Co-localization imaging of tumor-associated sentinel lymph nodes using HSA@IR-808 FPs and HSA@CO-1080 FPs. Pathological images of the tumor-draining sentinel lymph node. Reproduced with permission.68 Copyright 2024, Springer Nature. (C) Crystallographic representation of the Au25 cluster structure. Color codes of the elements: Au(0) in the core: yellow, Au(I) in the staple motif: orange, S in the staple motif: green. Post functionalization of the Au-GSH cluster and schematic representation of the Au-PC conjugate structure. (D) Wide-field fluorescence images of the intra-tumoral injected 1× Au-PC conjugate and ICG probes into a mouse bearing 4T1 tumor. (C) and (D) Reproduced with permission.39 Copyright 2022, Springer Nature. | |
4.2 Pathophysiological imaging
Pathophysiological information allows doctors to better understand disease progression and the physiological state of tissues, providing essential data for personalized treatment and medical advancement. NIR-II fluorescence imaging has already played a critical role in the research of diseases affecting vital organs such as the cardiovascular system, liver, intestines, lungs, and kidneys. In 2020, Mateos et al.69 utilized angiotensin II (AngII)-functionalized NIR-II Ag2S QDs to image ischemic myocardial tissue in myocardial infarction (Fig. 15A and B). Ex vivo spectral imaging confirmed that AngII-functionalized Ag2S QDs specifically bind to ischemic myocardial tissue, enabling real-time visualization of the heart following acute infarction. Hepatic ischemia–reperfusion injury (HIRI) is a common clinical condition that can lead to early organ failure in 10% of liver transplants. HIRI is closely associated with oxidative stress, which impairs lysosomal degradation functions and significantly alters lysosomal viscosity. Therefore, lysosomal viscosity is a potential biomarker for accurately targeting HIRI. Tang et al.70 developed a viscosity-specific NIR-II fluorescent probe, NP-V, for detecting lysosomal viscosity in hepatocytes and mice during HIRI. The fluorescence intensity of NP-V in the livers of HIRI mice was 2.1 times higher than that in control mice, with a SNR of 3.7, surpassing the control group. Due to the high SNR of NP-V in NIR-II in vivo imaging, it can clearly delineate the contours and boundaries of liver lesions, facilitating precise resection of diseased areas during surgery (Fig. 15C–F).
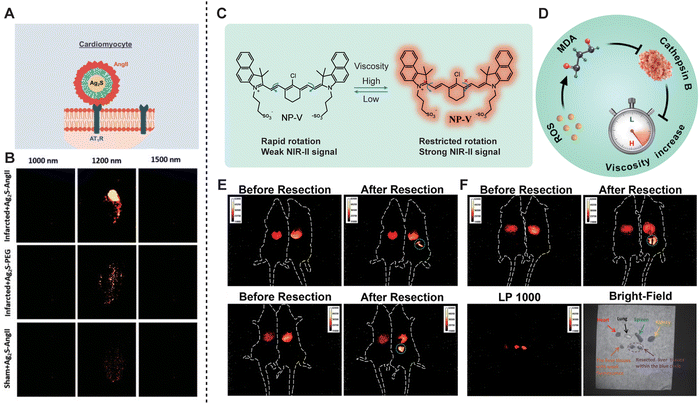 |
| Fig. 15 (A) Schematic representation of the Ag2S experimental procedure followed in this work for in vivo imaging of acute infarct. (B) Ex vivo fluorescence images at three different emission wavelengths (1000, 1200, and 1500 nm) as obtained for a heart subjected to an acute infarct and an intravenous injection of AngII-functionalized Ag2S NDs, a heart subjected to an acute infarct and intravenous injection of PEGylated Ag2S NDs, and a healthy heart from a sham-operated mouse subjected to an intravenous injection of AngII-functionalized Ag2S NDs. Reproduced with permission.69 Copyright 2020, Wiley-VCH Verlag GmbH. (C) Structure and fluorescence properties of viscosity-responsive NP-V. (D) Schematic of lysosomal ROS-MDA-cathepsin B cascade signaling pathway-mediated viscosity change during HIRI. (E) Representative NIR-II fluorescence images for precise navigation and surgical resection of liver lesions in HIRI mice. Control mice are on the left and HIRI mice are on the right. (F) NIR-II fluorescence and bright-field images of the main organs (heart, spleen, lungs, kidneys, and resected liver tissues). (C)–(F) Reproduced with permission.70 Copyright 2022, American Chemical Society. | |
Acute lung injury (ALI) is a severe disease characterized by a dysregulated inflammatory immune response in the lungs. Li et al.71 encapsulated the organic dye TT-F in cerium dioxide nanoparticles and then coated them with macrophage membranes to create HCe-TTF@M (Fig. 16A and B). One hour after aerosolizing HCe-TTF@M, the NIR-II fluorescence signal permeated the entire lung tissue. This study pioneered the combination of high-performance NIR-II fluorescent probes with nano-catalytic immunoregulation for real-time diagnosis and intervention of ALI. Ischemia/reperfusion (I/R)-induced AKI is a serious renal condition known for its high incidence and mortality rates. Monitoring kidney injury is crucial for the early treatment of renal diseases, which are often asymptomatic in the early stages but can progress to severe renal failure. In 2021, Zhang et al.49 developed a series of renal-clearable, high-brightness za-BODIPY brush-like macromolecular fluorophores, FBP-BODIPY, for early monitoring and diagnosis of renal ischemia–reperfusion injury (Fig. 16C and D). Compared to traditional polyethylene glycol-functionalized fluorophores, FBP-BODIPY exhibits a longer blood circulation time of up to 6.1 h. Furthermore, Pu et al.59 developed a molecular semiconductor, CDIR2, with renal clearance capabilities by coupling cyclodextrin with a NIR-II fluorophore through a click reaction. Compared to ICG, CDIR2 exhibits a higher SNR and achieves a renal clearance rate of up to 90% 24 hours post-injection. This remarkable clearance rate is due to CDIR2 being neither reabsorbed nor secreted by renal tubules. The unidirectional renal clearance pathway makes CDIR2 the first NIR-II molecular probe capable of real-time, non-invasive monitoring of cisplatin-induced renal injury.
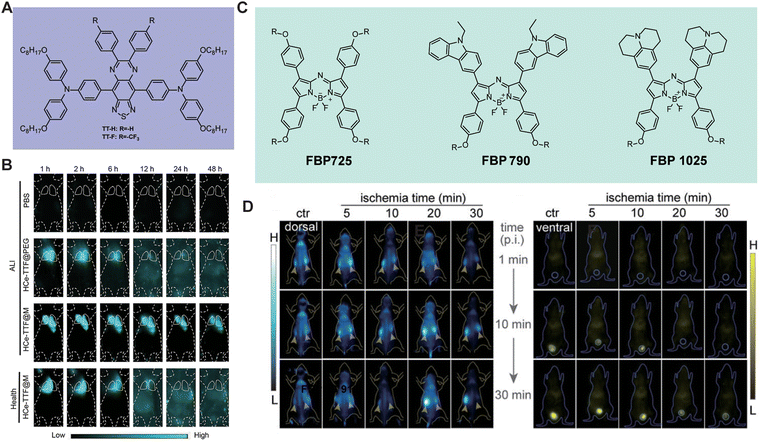 |
| Fig. 16 (A) The molecular structures of TT-H and TT-F. (B) Representative NIR-II fluorescence images of ALI mice. Reproduced with permission.71 Copyright 2024, Wiley-VCH GmbH. (C) The chemical structures of FBP725, FBP790, and FBP1025. (D) Representative in vivo NIR-II images at different time intervals of RIR mice from the dorsal or ventral position after different ischemia time treatment. The yellow arrows and circles in (D) indicate the kidneys and bladder, respectively. (C) and (D) Reproduced with permission.49 Copyright 2021, Wiley-VCH GmbH. | |
4.3 Molecular imaging
The detection and imaging of in vivo biomarkers, such as reactive oxygen species (ROS), reactive nitrogen species (RNS), reactive sulfur species (RSS), temperature, gases, pH, and enzymes, can facilitate the understanding and monitoring of the physiological state, pathological changes, and disease progression within cells and tissues.72–75 Through precise imaging and quantitative analysis, researchers and clinicians are able to diagnose diseases early, evaluate therapeutic efficacy, and optimize treatment regimens. In recent years, a diverse array of NIR-II fluorescent probes has been developed to detect various biomarkers.
RNS play a pivotal role in physiological processes such as cell signaling, immune defense, gene expression regulation, and antioxidant defense.76 Among these, the production of peroxynitrite (ONOO−) is considered an early indicator of drug-induced hepatotoxicity, making its early detection particularly crucial. In 2019, Zhang et al.77 developed an ONOO−-activated NIR-II probe, IRBTP-O, to monitor drug-induced hepatotoxicity (Fig. 17A and B). IRBTP-O exhibited a strong response to ONOO−. Compared to the commercial probe D63, IRBTP-O demonstrated a higher SNR and relative fluorescence intensity in in vivo imaging. This study highlighted the upregulation of ONOO− in a preclinical model of drug-induced liver injury and the reparative effects of N-acetylcysteine (NAC). Nitric oxide (NO) also plays a critical role in various physiological and pathological processes. Liu et al.78 designed an NIR-II ratiometric fluorescent probe, SPANO2, which converts to SPANO2-NOC in the presence of NO. SPANO2 can quantitatively detect NO concentrations in the tumor microenvironment, liver, and kidneys, and perform ratiometric imaging of NO during tumor progression with micron-level resolution. Liu et al.79 reported a NIR-II fluorescent molecular nanoprobe, OSNP, which reacts with NO and then shifts its emission from the NIR-I to the NIR-II window. The application of OSNP imaging revealed that the fluorescence intensity of the lungs treated with SARS-CoV-2 SP was 7.98 times greater than that of healthy lungs, as demonstrated by the results of NIR-II fluorescence imaging (Fig. 17C and D).
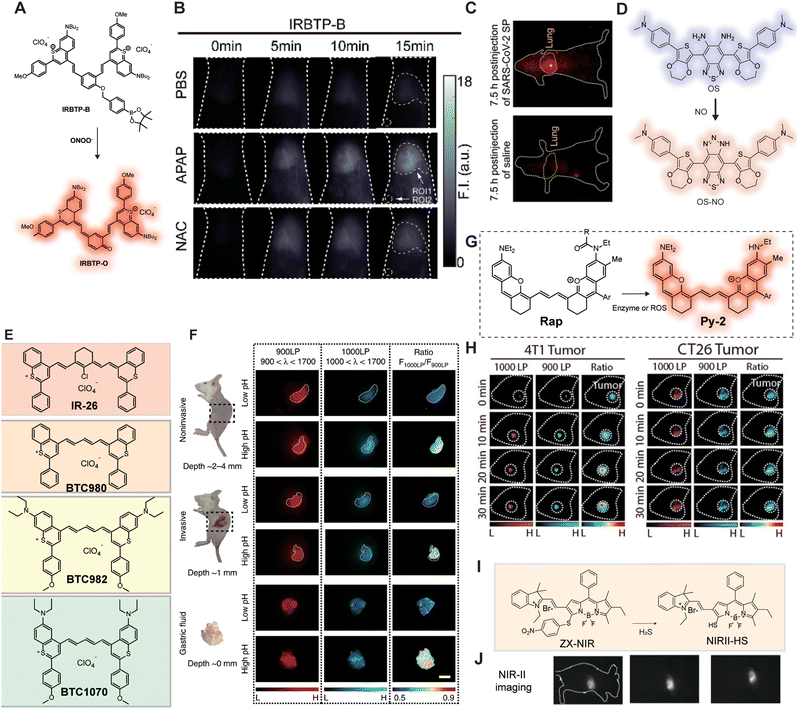 |
| Fig. 17 (A) Design and structure of an ONOO− activatable molecular probe with NIR-II fluorescence. (B) In vivo imaging of livers of mice from IRBTP-B treated with various substances: PBS, APAP, and NAC + APAP. Reproduced with permission.77 Copyright 2019, American Chemical Society. (C) NIR-II fluorescence images at 7.5 h after injection of SARS-CoV-2 SP and OSNP into living mice. (D) The structure of OSNP for in situ noninvasive observation of nitric oxide (NO) fluctuation in SARS-Cov-2 infection in vivo. Reproduced with permission.79 Copyright 2023, American Chemical Society. (E) Chemical structures of IR26, BTC980, BTC982, and BTC1070. (F) In vivo ratiometric fluorescence imaging of gastric pH noninvasive imaging at ∼2–4 mm tissue depth; invasive imaging of gastric fluid covered by ∼1 mm thickness of gastric wall; imaging of exposed gastric fluid. Right: Fluorescence images and corresponding ratiometric fluorescence images of mice stomach at two different pH environments. Reproduced with permission.80 Copyright 2019, Springer Nature. (G) Proposed biosensing mechanism of the ratiometric NIR-II probes for ROS detection. (H) Time-course NIR-II fluorescence images of 4T1 and CT-26 tumor. Reproduced with permission.81 Copyright 2022, American Chemical Society. (I) Schematic of the construction of multi-wavelength nanoprobes with H2S activatable emission in the second near-infrared window. (J) Visualization and differentiation of cancers based on H2S activation of NIR-II@Si. Reproduced with permission.82 Copyright 2018, Wiley-VCH Verlag GmbH. | |
In vivo pH is a critical physiological parameter, and pH imbalance can lead to various diseases. For instance, an acidic environment promotes tumor cell growth and metastasis, while an alkaline environment may result in osteoporosis. Zhang et al.80 designed a series of anti-quenching pentamethine cyanine dyes, including BTC980, BTC982, and BTC1070. BTC1070 exhibited ratiometric fluorescence changes from 1065 nm to 980 nm (Fig. 17E and F). Non-invasive ratiometric imaging can not only identify the gastric profile about 2–4 mm deep on the left side of the abdomen but also distinguish between two pH environments with clear pseudo-color contrast. Compared to standard pH meters, BTC1070 demonstrated reliable pH detection accuracy even in tissues up to 4 mm deep.
Hydrogen sulfide (H2S), as an important gaseous signaling molecule, is closely associated with various diseases when its levels are abnormal. Xu et al.82 designed activatable nanoprobes, ZIX-NIR, which transform into NIR-II-HS upon activation by H2S, exhibiting specific and ratiometric fluorescence reactivity (Fig. 17I and J). ZIX-NIR can selectively identify H2S-rich colon cancer cells in a multicolor imaging mode and distinguish live cell types based on H2S content. In recent years, a series of enzyme-responsive NIR-II fluorescent probes have been successfully developed. In 2022, Lei et al.81 developed an NIR-II molecular platform, Py-2, by integrating rhodamine and a polymethine chain (Fig. 17G and H). Based on Py-2, they constructed an NIR-II ratiometric probe, Rap-N, to detect nitroreductase (NTR). After in situ injection of Rap-N, a significant NIR-II fluorescence signal was observed in the 4T1 and CT-26 tumor regions, confirming that Rap-N is a reliable enzyme detection probe.
4.4 Surgical navigation
The potential clinical applications of NIR-II fluorescence imaging in intraoperative navigation are significant. This technology can provide real-time, high-resolution tissue imaging, aiding surgeons in precisely locating target tissues during surgery.83,84 This guidance improves the accuracy and safety of surgical procedures, avoiding inadvertent damage to critical tissues and reducing the risk of surgical complications. Thus, NIR-II fluorescence imaging offers a reliable real-time imaging technique for surgeries, enhancing surgical quality and patient outcomes.
In 2022, Wang et al.85 developed biocompatible erbium-based rare-earth nanoparticles (ErNPs) that emit bright down-conversion fluorescence in the NIR-IIb window. By modifying the surface of ErNPs with TRC105 antibodies, they constructed nanoprobes (ErNPs-TRC105) that target tumor vasculature. Using ErNPs-TRC105, high-resolution NIR-IIb fluorescence imaging of 4T1 mouse breast tumors was achieved, with a tumor-to-muscle SNR of 300, enabling precise tumor resection (Fig. 18A–C). Fan et al.86 used AIE nanoprobes to completely resect severe colitis under NIR-II fluorescence imaging guidance, ensuring surgical safety.
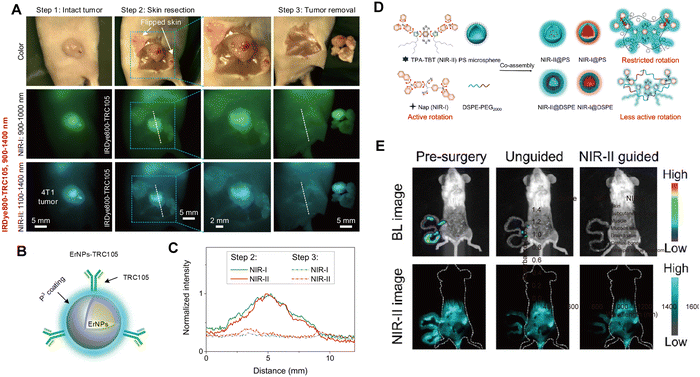 |
| Fig. 18 (A) Color/visible imaging recorded during surgery (upper). NIR-I fluorescence imaging (middle) and NIR-II fluorescence imaging (lower) of 4T1 tumor removal 24 h after i.v. injection of IRDye800-TRC105. (B) Schematic design of NIR-IIb contrast agents by conjugating core–shell ErNPs with the TRC105 antibody. (C) T/M ratios of IRDye800-TRC105 in NIR-I and NIR-II windows. (A)–(C) Reproduced with permission.85 Copyright 2022, PNAS. (D) The fabrication process of NPs involves loading AIEgens into carrier materials to create NIR fluorescent probes for surgical applications. (E) Bioluminescence and NIR-II fluorescence images of the intraperitoneal tumor-bearing mice before surgery, after unguided surgery, and after NIR-II@PS FIG.S. Reproduced with permission.87 Copyright 2024, American Chemical Society. | |
Detection and removal of micrometastases are crucial in the treatment of metastatic cancer. In 2023, Pu et al.88 obtained polymer nanoparticles (NIR-II NPs) with bright NIR-II fluorescence through nanoprecipitation. By constructing early-stage orthotopic tumor models and advanced cancer models, NIR-II NPs could accurately identify orthotopic tumors, tumor-associated lymph nodes, and disseminated peritoneal micrometastases smaller than 1 mm, all with SNR greater than 5. Under the guidance of fluorescence imaging in the NIR-II region, they achieved complete tumor resection comparable to clinical practice. Glioblastoma is a clinically challenging brain tumor. In 2024, Lyu et al.87 developed an NIR-IIa organic fluorescent probe, IR-32p, targeting αvβ3 (Fig. 18D and E). Under 1064 nm excitation, IR-32p enabled high-resolution fluorescence imaging of orthotopic glioblastoma in mice, leading to the precise resection of tumors smaller than 1 mm. IR-32p holds promise for future clinical applications in the detection and resection of orthotopic glioblastomas. In malignant tumors, the saturation state of capillary oxygenated hemoglobin (sO2) is closely linked to tumor metabolism. The dynamic visualization of sO2 in tumor vasculature can provide critical information for cancer prognosis and treatment. Zhong et al.89 developed a NIR-IIb NaErF4@NaYF4-based nanoparticle (pEr) that, after intravenous injection, can monitor sO2 in mouse skin at depths below 1.6 mm with a frame rate of ≥30 Hz, visualizing capillaries with a diameter of approximately 9.7 μm in the tumor region. This non-invasive dynamic monitoring of tumor-associated vascular oxygenated hemoglobin (TAV-sO2) levels enables a more accurate prediction of immunotherapy outcomes.
5. Conclusions and outlook
In recent decades, in vivo fluorescence imaging has emerged as a powerful tool in both basic scientific research and clinical applications. However, challenges such as absorption, scattering of light in the VIS and NIR-I regions, and background autofluorescence in biological tissues limit deep tissue penetration, high resolution and SNR in traditional fluorescence imaging. To overcome these limitations, fluorescence imaging technology in the NIR-II region has been developed. NIR-II fluorescence imaging benefits from reduced interaction between NIR-II light and biological tissues compared to the fluorescence imaging in the VIS and NIR-I regions, enabling deep tissue penetration and high-contrast in vivo imaging. This capability provides detailed insights into biological structures and pathophysiological events within live organisms. Moreover, the advancement of versatile NIR-II fluorophores has driven the progression of in vivo fluorescence imaging technology. This review begins by outlining the advantages of fluorescence imaging in the NIR-II region. It then systematically summarizes various classifications, designs, and strategies employed to optimize the performance of NIR-II fluorophores. Finally, the review comprehensively discusses the diverse applications of NIR-II fluorophores in biomedical imaging.
NIR-II fluorescence imaging has demonstrated tremendous potential in preclinical research, yet its clinical application remains limited, primarily due to the limitations associated with fluorescent probes. An ideal NIR-II fluorescent probe should feature a long wavelength, high brightness, excellent biocompatibility, prolonged lifetime, and either targeting or activatable capabilities. However, current NIR-II fluorophores exhibit various shortcomings that necessitate further optimization of their photophysical properties to facilitate clinical translation. For instance, LnNPs can achieve NIR-II emission through luminescence ion substitution, but they are hampered by the limited characteristic absorption of sensitization ions and weak emission capabilities of partially activated ions, thereby restricting their clinical application. QDs, known for their high QY, can now achieve emission wavelengths surpassing 1500 nm with optimized fabrication techniques. However, instability in aqueous media leading to fluorescence quenching and the presence of toxic elements pose significant barriers to clinical translation. NIR-II organic fluorophores, offering good biocompatibility and tunable wavelengths, are constrained by the energy gap law, where non-radiative decay dominates in narrow-bandgap variants, resulting in lower QYs. The lower brightness often necessitates extended exposure times during imaging, challenging detectors and compromising imaging resolution and SNR. Moreover, the biological safety of NIR-II fluorophores, particularly inorganic fluorophores containing heavy metals, requires thorough investigation for clinical translation. Currently reported NIR-II fluorophores typically exhibit λmax,abs/λem,max below 1300 nm, with few extending beyond 1500 nm. Indeed, there is a need to further emphasize the value of the NIR-IIb window in vivo fluorescence imaging, as this window minimizes interaction with biological tissues and exhibits nearly zero background fluorescence. Furthermore, other imaging modalities in the NIR-II region, such as photoacoustic imaging, deserve attention. Photoacoustic imaging is an emerging hybrid technology that combines optical excitation with ultrasonic detection.90,91 Unlike fluorescence imaging, which is limited by shallow penetration due to photon–tissue interactions, the depth of photoacoustic imaging is primarily determined by the penetration capability of the exciting photons. Meanwhile, ultrasound signals experience minimal scattering and attenuation in biological tissues. As a result, photoacoustic imaging overcomes the limitations of photon imaging depth, enabling tissue penetration of several centimeters and showing tremendous potential for clinical applications.
While NIR-II fluorescence imaging offers advantages such as deep tissue penetration, high resolution, and a high SNR, achieving clinical translation requires advancements beyond fluorophore optimization. Enhancements in detectors and imaging systems are crucial. Currently, the most commonly used cameras for detecting NIR-II optical signals are based on InGaAs, which responds well to light in the 900–1700 nm range. However, as NIR-II fluorophores with λmax,abs/λem,max above 1700 nm continue to be developed, there is an urgent need for new-generation cameras with broader spectral coverage. Mercury cadmium telluride (HgCdTe) and quantum dot infrared photodetectors (QDIPs) are promising candidates for expanding spectral sensitivity. Furthermore, there is a demand for advanced optical imaging systems that can integrate seamlessly with these new detectors and fluorophores, such as integrated systems with multimodal imaging capabilities.
Author contributions
The manuscript was written through contributions of all authors.
Data availability
Data availability is not applicable to this article as no new data were created or analyzed in this study.
Conflicts of interest
There are no conflicts to declare.
Acknowledgements
Y. Y. acknowledges the financial support from the National Key R&D Program of China (2023YFB3507100), the Natural Science Foundation of Inner Mongolia (2023QN02015), and the start-up funding of Inner Mongolia University (10000-23112101/044). Q. J. acknowledges the start-up funding of Inner Mongolia University (10000-23112101/033).
References
- F. Hallouard, N. Anton, P. Choquet, A. Constantinesco and T. Vandamme, Biomaterials, 2010, 31, 6249–6268 CrossRef CAS
.
- H. Kobayashi, M. Ogawa, R. Alford, P. L. Choyke and Y. Urano, Chem. Rev., 2010, 110, 2620–2640 CrossRef CAS PubMed
.
- Y. Tang, Y. Li, X. Lu, X. Hu, H. Zhao, W. Hu, F. Lu, Q. Fan and W. Huang, Adv. Funct. Mater., 2019, 29, 1807376 CrossRef
.
- Y. Sun, M. Yu, S. Liang, Y. Zhang, C. Li, T. Mou, W. Yang, X. Zhang, B. Li, C. Huang and F. Li, Biomaterials, 2011, 32, 2999–3007 CrossRef CAS PubMed
.
- Y. Yang, Y. Sun, T. Cao, J. Peng, Y. Liu, Y. Wu, W. Feng, Y. Zhang and F. Li, Biomaterials, 2013, 34, 774–783 CrossRef CAS PubMed
.
- E. L. Schmidt, Z. Ou, E. Ximendes, H. Cui, C. H. C. Keck, D. Jaque and G. Hong, Nat. Rev. Methods Primers, 2024, 4, 23 CrossRef CAS
.
- R. Gao, S. M. Asano, S. Upadhyayula, I. Pisarev, D. E. Milkie, T.-L. Liu, V. Singh, A. Graves, G. H. Huynh, Y. Zhao, J. Bogovic, J. Colonell, C. M. Ott, C. Zugates, S. Tappan, A. Rodriguez, K. R. Mosaliganti, S.-H. Sheu, H. A. Pasolli, S. Pang, C. S. Xu, S. G. Megason, H. Hess, J. Lippincott-Schwartz, A. Hantman, G. M. Rubin, T. Kirchhausen, S. Saalfeld, Y. Aso, E. S. Boyden and E. Betzig, Science, 2019, 363, eaau8302 CrossRef CAS PubMed
.
- C. Pan, O. Schoppe, A. Parra-Damas, R. Cai, M. I. Todorov, G. Gondi, B. von Neubeck, N. Böğürcü-Seidel, S. Seidel, K. Sleiman, C. Veltkamp, B. Förstera, H. Mai, Z. Rong, O. Trompak, A. Ghasemigharagoz, M. A. Reimer, A. M. Cuesta, J. Coronel, I. Jeremias, D. Saur, A. Acker-Palmer, T. Acker, B. K. Garvalov, B. Menze, R. Zeidler and A. Ertürk, Cell, 2019, 179, 1661–1676.e19 CrossRef CAS PubMed
.
- N. G. Horton, K. Wang, D. Kobat, C. G. Clark, F. W. Wise, C. B. Schaffer and C. Xu, Nat. Photonics, 2013, 7, 205–209 CrossRef CAS
.
- K. Welsher, Z. Liu, S. P. Sherlock, J. T. Robinson, Z. Chen, D. Daranciang and H. Dai, Nat. Nanotechnol., 2009, 4, 773–780 CrossRef CAS PubMed
.
- G. Hong, S. Diao, A. L. Antaris and H. Dai, Chem. Rev., 2015, 115, 10816–10906 CrossRef CAS PubMed
.
- S. Diao, G. Hong, A. L. Antaris, J. L. Blackburn, K. Cheng, Z. Cheng and H. Dai, Nano Res., 2015, 8, 3027–3034 CrossRef CAS
.
- D. Ma, H. Bian, M. Gu, L. Wang, X. Chen and X. Peng, Coord. Chem. Rev., 2024, 505, 215677 CrossRef CAS
.
- G. Hong, A. L. Antaris and H. Dai, Nat. Biomed. Eng., 2017, 1, 0010 CrossRef CAS
.
- W. F. Cheong, S. A. Prahl and A. J. Welch, IEEE J. Quantum Electron., 1990, 26, 2166–2185 CrossRef
.
- H. Ding, J. Q. Lu, W. A. Wooden, P. J. Kragel and X.-H. Hu, Phys. Med. Biol., 2006, 51, 1479 CrossRef PubMed
.
- A. N. Bashkatov, E. A. Genina, V. I. Kochubey and V. V. Tuchin, J. Phys. D: Appl. Phys., 2005, 38, 2543 CrossRef CAS
.
- S. Diao, J. L. Blackburn, G. Hong, A. L. Antaris, J. Chang, J. Z. Wu, B. Zhang, K. Cheng, C. J. Kuo and H. Dai, Angew. Chem., Int. Ed., 2015, 54, 14758–14762 CrossRef CAS PubMed
.
- M. Monici, Annu. Rev., 2005, 11, 227–256 CAS
.
- F. Wang, F. Ren, Z. Ma, L. Qu, R. Gourgues, C. Xu, A. Baghdasaryan, J. Li, I. E. Zadeh, J. W. N. Los, A. Fognini, J. Qin-Dregely and H. Dai, Nat. Nanotechnol., 2022, 17, 653–660 CrossRef CAS PubMed
.
- X. Luo, C. Zhang, Z. Yu, S. Wen and Y. Xian, TrAC, Trends Anal. Chem., 2023, 169, 117368 CrossRef CAS
.
- H. Zhang, Y. Fan, P. Pei, C. Sun, L. Lu and F. Zhang, Angew. Chem., Int. Ed., 2019, 58, 10153–10157 CrossRef CAS PubMed
.
- J. Ming, Y. Chen, H. Miao, Y. Fan, S. Wang, Z. Chen, Z. Guo, Z. Guo, L. Qi, X. Wang, B. Yun, P. Pei, H. He, H. Zhang, Y. Tang, D. Zhao, G. K.-L. Wong, J.-C. G. Bünzli and F. Zhang, Nat. Photonics, 2024 DOI:10.1038/s41566-024-01517-9
.
- Y. Zhong, Z. Ma, S. Zhu, J. Yue, M. Zhang, A. L. Antaris, J. Yuan, R. Cui, H. Wan, Y. Zhou, W. Wang, N. F. Huang, J. Luo, Z. Hu and H. Dai, Nat. Commun., 2017, 8, 737 CrossRef PubMed
.
- W. Shao, G. Chen, A. Kuzmin, H. L. Kutscher, A. Pliss, T. Y. Ohulchanskyy and P. N. Prasad, J. Am. Chem. Soc., 2016, 138, 16192–16195 CrossRef CAS PubMed
.
- Y. Fan, P. Wang, Y. Lu, R. Wang, L. Zhou, X. Zheng, X. Li, J. A. Piper and F. Zhang, Nat. Nanotechnol., 2018, 13, 941–946 CrossRef CAS PubMed
.
- F. P. García de Arquer, D. V. Talapin, V. I. Klimov, Y. Arakawa, M. Bayer and E. H. Sargent, Science, 2021, 373, 640–653 CrossRef PubMed
.
- B. Dong, C. Li, G. Chen, Y. Zhang, Y. Zhang, M. Deng and Q. Wang, Chem. Mater., 2013, 25, 2503–2509 CrossRef CAS
.
- O. T. Bruns, T. S. Bischof, D. K. Harris, D. Franke, Y. Shi, L. Riedemann, A. Bartelt, F. B. Jaworski, J. A. Carr, C. J. Rowlands, M. W. B. Wilson, O. Chen, H. Wei, G. W. Hwang, D. M. Montana, I. Coropceanu, O. B. Achorn, J. Kloepper, J. Heeren, P. T. C. So, D. Fukumura, K. F. Jensen, R. K. Jain and M. G. Bawendi, Nat. Biomed. Eng., 2017, 1, 0056 CrossRef CAS PubMed
.
- Y. Kong, J. Chen, H. Fang, G. Heath, Y. Wo, W. Wang, Y. Li, Y. Guo, S. D. Evans, S. Chen and D. Zhou, Chem. Mater., 2016, 28, 3041–3050 CrossRef CAS
.
- J. Zou and Q. Zhang, Adv. Sci., 2021, 8, 2102860 CrossRef
.
- S. Bhattacharya, X. Gong, E. Wang, S. K. Dutta, J. R. Caplette, M. Son, F. T. Nguyen, M. S. Strano and D. Mukhopadhyay, Cancer Res., 2019, 79, 4515–4523 CrossRef CAS PubMed
.
- M. Kim, C. Chen, Z. Yaari, R. Frederiksen, E. Randall, J. Wollowitz, C. Cupo, X. Wu, J. Shah, D. Worroll, R. E. Lagenbacher, D. Goerzen, Y.-M. Li, H. An, Y. Wang and D. A. Heller, Nat. Chem. Biol., 2023, 19, 1448–1457 CrossRef CAS PubMed
.
- M. J. O’Connell, S. M. Bachilo, C. B. Huffman, V. C. Moore, M. S. Strano, E. H. Haroz, K. L. Rialon, P. J. Boul, W. H. Noon, C. Kittrell, J. Ma, R. H. Hauge, R. B. Weisman and R. E. Smalley, Science, 2002, 297, 593–596 CrossRef
.
- J. Crochet, M. Clemens and T. Hertel, J. Am. Chem. Soc., 2007, 129, 8058–8059 CrossRef CAS PubMed
.
- Z. Zhao, K. He, B. Liu, W. Nie, X. Luo and J. Liu, Angew. Chem., Int. Ed., 2024, 63, e202406016 CrossRef CAS PubMed
.
- H. Liu, G. Hong, Z. Luo, J. Chen, J. Chang, M. Gong, H. He, J. Yang, X. Yuan, L. Li, X. Mu, J. Wang, W. Mi, J. Luo, J. Xie and X.-D. Zhang, Adv. Mater., 2019, 31, 1901015 CrossRef CAS PubMed
.
- Y. Huang, K. Chen, L. Liu, H. Ma, X. Zhang, K. Tan, Y. Li, Y. Liu, C. Liu, H. Wang and X.-D. Zhang, Small, 2023, 19, 2300145 CrossRef CAS
.
- A. Baghdasaryan, F. Wang, F. Ren, Z. Ma, J. Li, X. Zhou, L. Grigoryan, C. Xu and H. Dai, Nat. Commun., 2022, 13, 5613 CrossRef CAS PubMed
.
- X. Song, W. Zhu, X. Ge, R. Li, S. Li, X. Chen, J. Song, J. Xie, X. Chen and H. Yang, Angew. Chem., Int. Ed., 2021, 60, 1306–1312 CrossRef CAS PubMed
.
- X. Liu, B. Yu, Y. Shen and H. Cong, Coord. Chem. Rev., 2022, 468, 214609 CrossRef CAS
.
- K. Shou, C. Qu, Y. Sun, H. Chen, S. Chen, L. Zhang, H. Xu, X. Hong, A. Yu and Z. Cheng, Adv. Funct. Mater., 2017, 27, 1700995 CrossRef PubMed
.
- C. Sun, B. Li, M. Zhao, S. Wang, Z. Lei, L. Lu, H. Zhang, L. Feng, C. Dou, D. Yin, H. Xu, Y. Cheng and F. Zhang, J. Am. Chem. Soc., 2019, 141, 19221–19225 CrossRef CAS
.
- A. L. Antaris, H. Chen, S. Diao, Z. Ma, Z. Zhang, S. Zhu, J. Wang, A. X. Lozano, Q. Fan, L. Chew, M. Zhu, K. Cheng, X. Hong, H. Dai and Z. Cheng, Nat. Commun., 2017, 8, 15269 CrossRef CAS
.
- Y. Sun, X. Zeng, Y. Xiao, C. Liu, H. Zhu, H. Zhou, Z. Chen, F. Xu, J. Wang, M. Zhu, J. Wu, M. Tian, H. Zhang, Z. Deng, Z. Cheng and X. Hong, Chem. Sci., 2018, 9, 2092–2097 RSC
.
- X.-D. Zhang, H. Wang, A. L. Antaris, L. Li, S. Diao, R. Ma, A. Nguyen, G. Hong, Z. Ma, J. Wang, S. Zhu, J. M. Castellano, T. Wyss-Coray, Y. Liang, J. Luo and H. Dai, Adv. Mater., 2016, 28, 6872–6879 CrossRef CAS PubMed
.
- Z. Tao, G. Hong, C. Shinji, C. Chen, S. Diao, A. L. Antaris, B. Zhang, Y. Zou and H. Dai, Angew. Chem., Int. Ed., 2013, 52, 13002–13006 CrossRef CAS
.
- A. L. Antaris, H. Chen, K. Cheng, Y. Sun, G. Hong, C. Qu, S. Diao, Z. Deng, X. Hu, B. Zhang, X. Zhang, O. K. Yaghi, Z. R. Alamparambil, X. Hong, Z. Cheng and H. Dai, Nat. Mater., 2016, 15, 235–242 CrossRef CAS
.
- C. Yao, Y. Chen, M. Zhao, S. Wang, B. Wu, Y. Yang, D. Yin, P. Yu, H. Zhang and F. Zhang, Angew. Chem., Int. Ed., 2022, 61, e202114273 CrossRef CAS PubMed
.
- L. Bai, P. Sun, Y. Liu, H. Zhang, W. Hu, W. Zhang, Z. Liu, Q. Fan, L. Li and W. Huang, Chem. Commun., 2019, 55, 10920–10923 RSC
.
- W. Wu, K. Yan, Z. He, L. Zhang, Y. Dong, B. Wu, H. Liu, S. Wang and F. Zhang, J. Am. Chem. Soc., 2024, 146, 11570–11576 CAS
.
- G. Hong, Y. Zou, A. L. Antaris, S. Diao, D. Wu, K. Cheng, X. Zhang, C. Chen, B. Liu, Y. He, J. Z. Wu, J. Yuan, B. Zhang, Z. Tao, C. Fukunaga and H. Dai, Nat. Commun., 2014, 5, 4206 CrossRef CAS PubMed
.
- H. Zhou, Z. Lu, Y. Zhang, M. Li, D. Xue, D. Zhang, J. Liu, L. Li, J. Qian and W. Huang, ACS Appl. Mater. Interfaces, 2022, 14, 8705–8717 CrossRef CAS PubMed
.
- J. Li and K. Pu, Acc. Chem. Res., 2020, 53, 752–762 CrossRef CAS
.
- S. Liu, H. Ou, Y. Li, H. Zhang, J. Liu, X. Lu, R. T. K. Kwok, J. W. Y. Lam, D. Ding and B. Z. Tang, J. Am. Chem. Soc., 2020, 142, 15146–15156 CrossRef CAS PubMed
.
- Y. Jiang and K. Pu, Adv. Biosyst., 2018, 2, 1700262 CrossRef
.
- Q. Miao and K. Pu, Adv. Mater., 2018, 30, 1801778 CrossRef
.
- Y. Jiang and K. Pu, Acc. Chem. Res., 2018, 51, 1840–1849 CrossRef CAS PubMed
.
- J. Huang, C. Xie, X. Zhang, Y. Jiang, J. Li, Q. Fan and K. Pu, Angew. Chem., Int. Ed., 2019, 58, 15120–15127 CrossRef CAS
.
- Z. Zhang, Y. Du, X. Shi, K. Wang, Q. Qu, Q. Liang, X. Ma, K. He, C. Chi, J. Tang, B. Liu, J. Ji, J. Wang, J. Dong, Z. Hu and J. Tian, Nat. Rev. Clin. Oncol., 2024, 21, 449–467 CrossRef PubMed
.
- Y. Yuan, Z. Feng, S. Li, Z. Huang, Y. Wan, C. Cao, S. Lin, L. Wu, J. Zhou, L.-S. Liao, J. Qian and C.-S. Lee, Adv. Mater., 2022, 34, 2201263 CrossRef CAS
.
- X. He, J. Yu, J. Hu, W. Wei, K. Sun, J. Chen, Z. Cai, M. Liu, Y. Xu and B. Sun, ACS Mater. Lett., 2023, 5, 3192–3202 CrossRef CAS
.
- W. E. Meador, E. Y. Lin, I. Lim, H. C. Friedman, D. Ndaleh, A. K. Shaik, N. I. Hammer, B. Yang, J. R. Caram, E. M. Sletten and J. H. Delcamp, Nat. Chem., 2024, 16, 970–978 CrossRef CAS PubMed
.
- D. Li, Q. Liu, Q. Qi, H. Shi, E.-C. Hsu, W. Chen, W. Yuan, Y. Wu, S. Lin, Y. Zeng, Z. Xiao, L. Xu, Y. Zhang, T. Stoyanova, W. Jia and Z. Cheng, Small, 2020, 16, 2003851 CrossRef CAS
.
- C. Mi, X. Zhang, C. Yang, J. Wu, X. Chen, C. Ma, S. Wu, Z. Yang, P. Qiao, Y. Liu, W. Wu, Z. Guo, J. Liao, J. Zhou, M. Guan, C. Liang, C. Liu and D. Jin, Nat. Commun., 2023, 14, 6287 CrossRef CAS PubMed
.
- S. Jia, E. Y. Lin, E. B. Mobley, I. Lim, L. Guo, S. Kallepu, P. S. Low and E. M. Sletten, Chem, 2023, 9, 3648–3665 CAS
.
- W. Wang, Y. Kong, J. Jiang, Q. Xie, Y. Huang, G. Li, D. Wu, H. Zheng, M. Gao, S. Xu, Y. Pan, W. Li, R. Ma, M. X. Wu, X. Li, H. Zuilhof, X. Cai and R. Li, Angew. Chem., Int. Ed., 2020, 59, 22431–22435 CrossRef CAS
.
- J. Xu, N. Zhu, Y. Du, T. Han, X. Zheng, J. Li and S. Zhu, Nat. Commun., 2024, 15, 2845 CrossRef CAS PubMed
.
- S. Mateos, J. Lifante, C. Li, E. C. Ximendes, T. Muñoz-Ortiz, J. Yao, M. de la Fuente-Fernández, Á. L. García Villalón, M. Granado, I. Zabala Gutierrez, J. Rubio-Retama, D. Jaque, D. H. Ortgies and N. Fernández, Small, 2020, 16, 1907171 CrossRef CAS PubMed
.
- J. Liu, W. Zhang, C. Zhou, M. Li, X. Wang, W. Zhang, Z. Liu, L. Wu, T. D. James, P. Li and B. Tang, J. Am. Chem. Soc., 2022, 144, 13586–13599 CrossRef CAS
.
- Y. Li, D. Liu, T. Chen, J. Song, X. Yu, Q. Liu, J. Qi and W. Li, Adv. Funct. Mater., 2024, 34, 2403183 CrossRef CAS
.
- T. Li, Y. Zhang, F. Wu, G. Chen, C. Li and Q. Wang, Small Methods, 2024, 25, 2400132 CrossRef
.
- Y. Tang, F. Pei, X. Lu, Q. Fan and W. Huang, Adv. Opt. Mater., 2019, 7, 1900917 CrossRef CAS
.
- Z. Lou, P. Li and K. Han, Acc. Chem. Res., 2015, 48, 1358–1368 CrossRef CAS
.
- Y. Tang, Y. Li, Z. Wang, F. Pei, X. Hu, Y. Ji, X. Li, H. Zhao, W. Hu, X. Lu, Q. Fan and W. Huang, Chem. Commun., 2019, 55, 27–30 RSC
.
- Z. Xu, Q. Luo, Y. He, Y. He, X. Zhang, J. Wang, S. Ni, D. Gao and D. Wang, Adv. Funct. Mater., 2024, 34, 2314536 CrossRef CAS
.
- D. Li, S. Wang, Z. Lei, C. Sun, A. M. El-Toni, M. S. Alhoshan, Y. Fan and F. Zhang, Anal. Chem., 2019, 91, 4771–4779 CrossRef CAS PubMed
.
- Z. Jiang, C. Zhang, Q. Sun, X. Wang, Y. Chen, W. He, Z. Guo and Z. Liu, Angew. Chem., Int. Ed., 2024, 63, e202320072 CrossRef CAS PubMed
.
- Y. Tang, Y. Li, Z. Wang, W. Huang, Q. Fan and B. Liu, ACS Nano, 2023, 17, 18299–18307 CrossRef CAS
.
- S. Wang, Y. Fan, D. Li, C. Sun, Z. Lei, L. Lu, T. Wang and F. Zhang, Nat. Commun., 2019, 10, 1058 CrossRef
.
- Q. Lan, P. Yu, K. Yan, X. Li, F. Zhang and Z. Lei, J. Am. Chem. Soc., 2022, 144, 21010–21015 CrossRef CAS PubMed
.
- G. Xu, Q. Yan, X. Lv, Y. Zhu, K. Xin, B. Shi, R. Wang, J. Chen, W. Gao, P. Shi, C. Fan, C. Zhao and H. Tian, Angew. Chem., Int. Ed., 2018, 57, 3626–3630 CrossRef CAS PubMed
.
- C. Li, J. Du, G. Jiang, J. Gong, Y. Zhang, M. Yao, J. Wang, L. Wu and B. Z. Tang, Nat. Commun., 2024, 15, 5832 CrossRef CAS
.
- H. Ren, X.-Z. Zeng, X.-X. Zhao, D.-Y. Hou, H. Yao, M. Yaseen, L. Zhao, W.-H. Xu, H. Wang and L.-L. Li, Nat. Commun., 2022, 13, 418 CrossRef CAS
.
- F. Wang, L. Qu, F. Ren, A. Baghdasaryan, Y. Jiang, R. Hsu, P. Liang, J. Li, G. Zhu, Z. Ma and H. Dai, Proc. Natl. Acad. Sci. U. S. A., 2022, 119, e2123111119 CrossRef CAS
.
- X. Fan, Q. Xia, Y. Zhang, Y. Li, Z. Feng, J. Zhou, J. Qi, B. Z. Tang, J. Qian and H. Lin, Adv. Healthcare Mater., 2021, 10, 2101043 CrossRef CAS PubMed
.
- S. Lyu, S. Lu, C. Gui, C. Guo, J. Han, Y. Xiao, R. Zhang and X. Hong, J. Med. Chem., 2024, 67, 1861–1871 CrossRef CAS
.
- T. Pu, Y. Liu, Y. Pei, J. Peng, Z. Wang, M. Du, Q. Liu, F. Zhong, M. Zhang, F. Li, C. Xu and X. Zhang, ACS Appl. Mater. Interfaces, 2023, 15, 32226–32239 CrossRef CAS PubMed
.
- Z. Fang, C. Wang, J. Yang, Z. Song, C. Xie, Y. Ji, Z. Wang, X. Du, Q. Zheng, C. Chen, Z. Hu and Y. Zhong, Nat. Nanotechnol., 2024, 19, 124–130 CrossRef CAS
.
- Y. Jiang, P. K. Upputuri, C. Xie, Y. Lyu, L. Zhang, Q. Xiong, M. Pramanik and K. Pu, Nano Lett., 2017, 17, 4964–4969 CrossRef CAS PubMed
.
- Y. Jiang, P. K. Upputuri, C. Xie, Z. Zeng, A. Sharma, X. Zhen, J. Li, J. Huang, M. Pramanik and K. Pu, Adv. Mater., 2019, 31, 1808166 CrossRef
.
Footnote |
† Co-first authors who made equal contributions to the article. |
|
This journal is © The Royal Society of Chemistry 2025 |
Click here to see how this site uses Cookies. View our privacy policy here.