DOI:
10.1039/C5RA01068C
(Paper)
RSC Adv., 2015,
5, 27075-27081
Selective synthesis of different ZnO/Ag nanocomposites as surface enhanced Raman scattering substrates and highly efficient photocatalytic catalysts
Received
19th January 2015
, Accepted 10th March 2015
First published on 10th March 2015
Abstract
ZnO/Ag nanocomposites with adjustable surface-enhanced Raman scattering performance and photocatalytic activity were synthesized by a photochemical method. The as-prepared samples were investigated using various spectroscopic and microscopic techniques in detail. Solvents play a key role in controlling the morphologies and properties of these nanocomposites. Detection and degradation of organic dyes is studied. It has been found that suitable experimental parameters are crucial to the detection and degradation of organic dyes molecules. The work is of great importance in practical applications based on the surface-enhanced Raman scattering and photocatalytic performance of noble metal/oxide metal nanocomposites, which could be used for detection and degradation of organic dyes and pollutants.
1. Introduction
Owing to the urgent need for a clean and safe environment, accurate and reliable techniques for the detection of organic dyes need to be developed. Unfortunately, most of the current techniques require time-consuming and expensive sample pretreatment and complex steps.1–3 Therefore, it is necessary to develop time-saving and cost-effective methods for the detection of organic dyes. Surface-enhanced Raman spectroscopy (SERS), as an extremely sensitive and selective technique, which not only overcomes those restrictions mentioned above, but also provides trace detection of various organic dyes, pesticides and biomolecules down to the single-molecule level.4–11 Various noble metal SERS substrates have been designed to achieve high surface-enhanced Raman scattering performance.5–7,12–19 However, from the viewpoint of practical application, preparing composites with both SERS and other additional functionalities is of interest.
Loading noble metal nanoparticles onto semiconductor surface is a good way to design the multifunctional platform. Various noble metal nanoparticles/semiconductor composites have been reported by many groups.20–41 For example, Hou et al. have reported a simple and convenient approach for the synthesis of high-yield ZnO/Ag nanocomposites by applying one-pot nonaqueous route.23 Dou et al. have prepared Ag/ZnO/graphene (Ag/ZnO/G) nanocomposites by a facile low temperature microwave-assisted solution method.24 Liu et al. have synthesized Ag/ZnO heterostructures by photoreduction in various reducing mediums.25 Compared with other deposition techniques, photoreduction has the advantages of environment protection, energy saving and high reproducibility. ZnO/Ag composites have been demonstrated as one of the most suitable materials for detecting and degrading organic dyes.23–41 It not only has high surface enhanced Raman scattering (SERS) activity resulted from the localized surface plasmon resonance (LSPR) of Ag, but also shows enhanced photocatalytic efficiency due to the increase of electron-transfer between ZnO and Ag. Though various methods have been used to successfully synthesize the ZnO/Ag nanocrystal,23–41 the design and synthesis of ZnO/Ag with specific morphologies and applications still needs extra exploration.
In this article, ZnO flowers were prepared by a hydrothermal method. Afterwards, Ag nanoparticles with different morphologies and size were deposited on the surface of ZnO by a photochemical method. The as-synthesized ZnO/Ag nanocomposites were characterized using X-ray diffraction (XRD), field-emission scanning electron microscope (FESEM), energy dispersive spectroscopy (EDS), X-ray photoelectron spectroscopy (XPS), UV-vis absorption spectroscopy (UV-vis), photoluminescence (PL), Raman spectrometer (Raman) and transmission electron microscope (TEM). ZnO/Ag nanocomposites obtained in different solvents exhibited distinct surface-enhanced Raman scattering (SERS) performance and photocatalytic activity. The synthesis process is straightforward, simple, reproducible, cost effective and robust. Moreover, the materials are useful for application in the detection and degradation of harmful organic dyes. In the future, the present process may be helpful for the synthesis of other types of nanomaterials in a small time scale with higher yields.
2. Experimental section
2.1. Material
All the chemical reagents used in this work, including silver nitrate (AgNO3), zinc nitrate hexahydrate (Zn(NO3)2·6H2O), potassium biphthalate (C8H5KO4), sodium hydroxide (NaOH), Rhodamine B (RB, C28H31ClN2O3), crystal violet (CV, C25H30N3Cl·9H2O), methylene blue (MB, C16H18ClN3S), glycol (C2H6O2) and ethanol (C2H5OH). All chemicals were of analytical grade. Deionized water was used throughout the experiment.
2.2. Methods
Synthesis of ZnO flowers: 20 mL potassium biphthalate aqueous (0.3 M) was added into 20 mL zinc nitrate hexahydrate aqueous (0.3 M) with magnetic stirring. After 10 min, 10 mL sodium hydroxide aqueous (2.4 M) was added with stirring. Then the mixed solution was transferred into a Teflon-lined autoclave of 80 mL capacity, filled up to 90% of the total volume with deionized water. After being sealed and heated at 120 °C for 12 h, the autoclave was cooled to room temperature naturally. The resulting products were collected by centrifugation, washed with distilled water and ethanol for several times, and finally dried in vacuum at 60 °C for 6 h.
Synthesis of ZnO/Ag nanocomposites: firstly, 100 mg ZnO flowers were dispersed in 40 mL deionized water with vigorous stirring. Then 5 mL AgNO3 aqueous (0.1 M) was poured into the above suspension. Finally, the mixed solution was placed in a double-wall quartz bottle with stirring (magnetic stirrer, Model 78-1, 400 rpm) and irradiated under two 125 W high-pressure Hg lamp (λmax = 365 nm) for 120 min in a home-made stainless steel photoreaction (Model DW-01). The double-wall quartz bottle loaded with circulating distilled water was used to filtrate the concomitant heat during irradiation. The distance between quartz bottle and light source is 10 cm. The obtained products were collected by centrifugation and washed with deionized water and ethanol for several times. The final products were dried at 60 °C for 6 h and denoted as Sw. Similar procedure was performed under the same reaction condition except using the mixed aqueous (35 mL deionized water, 5 mL ethanol or 35 mL deionized water, 5 mL glycol) instead of 40 mL deionized water and the resulting products were denoted as SE and SG, respectively.
2.3. Characterization
The phase purity of the products was characterized by X-ray diffraction (XRD, German Bruker AXSD8 ADVANCE X-ray diffractometer) using a X-ray diffractometer with Cu Kα radiation (λ = 1.5418 Å). Scanning electron microscope (SEM) images and energy dispersive spectra (EDS) were obtained on a Japan Hitachi S-4800 field emission scanning electron microscopy. Transmission electron microscope (TEM) images, high angle annular dark field (HAADF) images, EDS line scan images and elemental mapping images were obtained on an American FEI Tecnai G2 F30 S-TWIN field-emission transmission electron microscopy (operated at 300 kV). The ultraviolet-visible (UV-vis) diffuse reflectance spectra were obtained on an America Varian Cary 5000 spectrophotometer. Photoluminescence spectra were measured using a Britain Renishaw Invia Raman spectrometer with a solid-state laser (excitation at 325 nm) at room temperature. X-ray photoelectron spectra (XPS) were recorded on an ESCALAB 250Xi system (Thermo Scientific). Prior to the analysis in the XPS system, all investigated materials, in the form of powder, were pasted onto the surface of the sample stage. All XPS measurements were performed using the ESCALAB 250Xi system without external illumination. A monochromated Al Kα X-ray source (1486.6 eV) was used and operated at a voltage of 15 kV. A micro focus X-ray monochromator was used with pass energy of 20 eV, which gives an instrumental resolution of 0.1 eV. The chosen pass energy was found to provide a reasonable compromise between the energy resolution and transmission of the analyzer, in other words good quality spectra with a satisfactory signal to noise ratio could be recorded within acceptably short acquisition times. In order to neutralize the charge build-up on the investigated surfaces, the XPS tool is equipped with a standard dual flood gun, which provides simultaneously a beam of low energy electrons and a beam of low energy Ar-ions. The base pressure of the ultra high vacuum (UHV) analysis chamber was held in the low range of 10−9 mbar prior to the analysis and – due to applied charge neutralization – in the low 10−7 mbar range during the data acquisition. Peak fitting was performed using the Thermo Scientific Avantage software. XPS spectra were carried out with the binding energies calibrated using C 1s (284.8 eV).
3. Results and discussion
3.1. Characterization of as-prepared samples
The XRD patterns of the as-prepared samples are shown in Fig. 1. Peaks at 31.7°, 34.4°, 36.3°, 47.5°, 56.6°, 62.3°, 67.9°, 69.1°, and 77.4° were observed in all patterns, which are representing (1 0 0), (0 0 2), (1 0 1), (1 0 2), (1 1 0), (1 0 3), (1 1 2), (2 0 1) and (2 0 2) crystal planes of wurtzite ZnO (JCPDS 36-1451 card) respectively. While those of 38.1°, 44.3° and 64.4° could be found and indexed to fcc metallic Ag (marked with *, JCPDS 04-0783) after photoirradiation (Fig. 1b–d). There is no notable shift of any diffraction peak, suggesting that Ag did not change the bulk intrinsic property of ZnO nanocrystals or incorporate into the lattice of ZnO to form Ag related solid solution. The lattice expansion or shrinkage of the Ag/ZnO heterostructures can be negligible. Additionally, no trace of impurities and other phases such as Zn(OH)2 and Ag2O are observed, confirming that the additional nanostructures are metallic Ag.
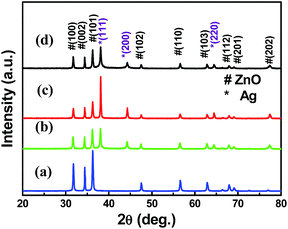 |
| Fig. 1 XRD patterns of (a) ZnO flowers and ZnO/Ag nanocomposites (b) SW (c) SE (d) SG. | |
In order to describe the morphologies of the as-prepared samples, SEM was carried out. Fig. 2a and b show the SEM images of pure ZnO flowers. It can be found that well dispersed samples are composed of flower-like structures with the diameters in the range of 2–3 μm, which is assembled by a large amount of interconnected nanoplates. From the magnified SEM image in Fig. 2b, it reveals that the thickness of these plates is about 30 nm and there are no any particles or sheets on the surface of these nanoplates. For SG in Fig. 2c and d, it can be seen that ZnO flowers are surrounded by a great amount of Ag sheets after UV photoirradiation. Similarly, there are many Ag nanoparticles with about 100 nm in size decorating on the surface of ZnO flowers for SE (Fig. 2e and f). However, some tiny Ag nanoparticles and lathy sheets are found on the surface of ZnO flowers for Sw (Fig. 2g and h). It can be conclude that the microstructure of ZnO flower is almost maintained after photoirradiation in different solvents. The morphologies and sizes of Ag nanoparticles are determined by sorts of solvents, which may be due to different polarity and reduction of solvents.
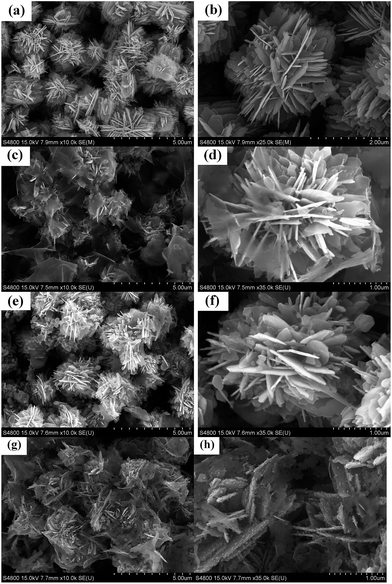 |
| Fig. 2 SEM images of (a and b) ZnO flowers and ZnO/Ag nanocomposites (c and d) SG (e and f) SE (g and h) SW. | |
The elemental composition of the Ag/ZnO composites were also analyzed by EDS equipped with SEM. Fig. 3 gives the elemental spectra of SW, SE and SG. The main elements detected for all samples include O, Zn and Ag from the composites. For SW, SE and SG, the weight percentages (wt%) of Ag were 27.56%, 23.62% and 35.03% respectively. It can be also noticed that the content of Ag on the ZnO increases from SE to SW, and to SG.
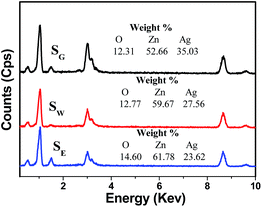 |
| Fig. 3 EDS spectra of ZnO/Ag nanocomposites. | |
To further characterize the as-prepared samples, TEM, STEM and EDS mapping images were also obtained on an American FEI Tecnai G2 F30 S-TWIN field-emission transmission electron microscopy, which is shown in Fig. 4. Different colors indicate different elements, where yellow and blue refer to the presence of Zn and Ag respectively. Obviously, ZnO flowers were coated by a great amount of Ag sheets or particles (Fig. 4a–c). For SE, Ag nanoparticles with about 100 nm were found on the surface of ZnO (Fig. 4b). For Sw (Fig. 4c) and SG (Fig. 4a), though it is different to distinguish the size distribution differences of Ag due to the overlap between Ag nanosheets and ZnO nanoplates, the Ag particles with about 10 nm in SW were observed from the corresponding TEM and STEM images (Fig. 4c).
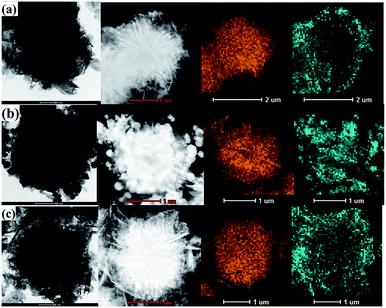 |
| Fig. 4 TEM, the corresponding STEM and elemental mapping images of ZnO/Ag nanocomposites (a) SG (b) SE (c) SW. | |
The surface structures of the as-prepared samples were investigated using XPS analysis with the binding energies calibrated using C 1s (284.8 eV), as shown in Fig. 5. Only Zn, O, C elements were found in the pure ZnO in Fig. 5a, curve a. After photoirradiation, four elements including Zn, O, C, and Ag can be found in Fig. 5a, curve b–d. The presence of C is mainly from pump oil due to vacuum treatment before the XPS test.41 The results are in good agreement with XRD as described above. Fig. 5b depicts the high resolution spectra for Ag species of as-prepared samples respectively. It can be found that compared to ZnO flowers, the binding energies of Ag 3d5/2 and Ag 3d3/2 for ZnO/Ag nanocomposites are found at about 367 and 373 eV, which shifts remarkably to lower binding energy relative to the corresponding values of the synthesized pure metallic Ag (the standard binding energies of Ag 3d5/2 and Ag 3d3/2 for bulk Ag are about 368.2 and 374.2 eV respectively).42,43 The binding energy of Ag for the ZnO/Ag composites is lower than zerovalent Ag, indicating the transfer of electrons from Ag nanoparticles to ZnO nanoplates, which is in good agreement with the fabrication of ZnO/Ag heterojunctions in the previous literatures.28,42,43 Different relative peak intensities and asymmetric shapeline of the photoemission spectra were observed for different nanocomposites, which may be due to their different morphology, size and content of Ag nanoparticles.44–46 Therefore, the XPS result further confirms the formation of nanocomposites between ZnO and Ag.
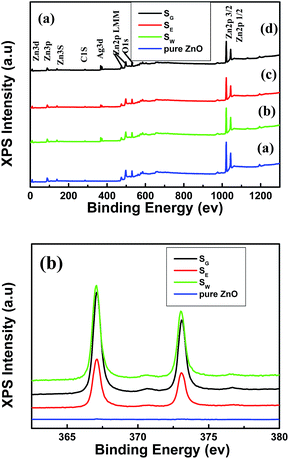 |
| Fig. 5 XPS spectra of the ZnO flowers and ZnO/Ag nanocomposites (a) the scan (b) Ag3d. | |
Fig. 6 shows the representative PL spectra of the as-prepared samples with the excitation wavelength of 325 nm at room temperature. It can be seen that ZnO and ZnO/Ag samples display similar emission bands. There are two peaks: one is the UV near-band-edge (NBE) emission centered at 375 nm, which is generally attributed to the free exaction recombination and quantum confinement effect; the other is a broad green region centered at 580 nm, which may be due to the recombination of photo-generated holes with the electrons in intrinsic or extrinsic defects.47 Compared with that of pure ZnO, the green emission of ZnO/Ag nanocomposites is dramatically reduced, indicating the decreased recombination of photo-generated holes with the electrons in intrinsic or extrinsic defects after photoirradiation process, which will enhance the photocatalytic efficiency.
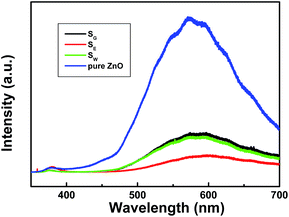 |
| Fig. 6 PL spectra of pure ZnO flowers and ZnO/Ag nanocomposites. | |
The diffuse reflectance spectra of the as-prepared samples were demonstrated in Fig. 7. The absorption edge at about 375 nm is assigned to the absorption of ZnO semiconductor. For SG, a broad absorption covers the range of 400–800 nm, which should be attributed to the surface plasmon resonance effect of Ag nanosheets. And the absorption peaks at 434 nm and 452 nm are the characteristic plasmon absorption of silver nanoparticles with different size in SE and Sw respectively. There is a red shift compared to that of pure Ag nanoparticles.48,49 It is evident that the red shift is due to the formation of ZnO/Ag nanocomposites. The plasmon absorption of silver λp is represented by the following equation:50
|
λp = [(4π2c2meffε0/Ne2)]1/2
| (1) |
Where
meff is the effective mass of the free electron of metal,
N is the electron density of the metal silver and others including
c and
ε0 are constant. For ZnO/Ag nanocomposites, the electron density (
N) of the metallic Ag decreases due to the work function differences between Ag and ZnO nanocrystals. From
eqn (1), it can be seen that the position of plasmon absorption (
λp) of Ag nanoparticles will increase accordingly (
i.e., the red shift of the plasmon absorption) when the electron density of the metallic Ag decreases (
N).
28
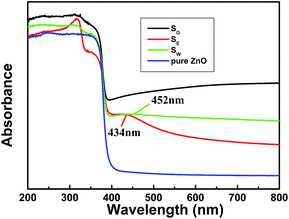 |
| Fig. 7 DRS spectra of the ZnO flowers and ZnO/Ag nanocomposites. | |
3.2. Surface-enhanced Raman scattering performance and photocatalytic activity
3.2.1. Surface-enhanced Raman scattering (SERS) performance. As we all know, the SERS performance of noble metals depends on their sizes and morphologies. To further evaluate the importance of the size and the morphology of AgNPs, the SERS performance of the as-prepared samples was systematically investigated. In Fig. 8a, all peaks at 1194 cm−1 (ν-CH) and 1359, 1506, 1563 and 1649 cm−1 (ν-CC) are attributed to Rhodamine B.51 It can be seen that the order of the SERS signal intensity is as follows: SG (Fig. 8a, curve a) > SE (Fig. 8a, curve b) > SW (Fig. 8a, curve c) > pure ZnO (Fig. 8a, curve d). It is clearly concluded that SERS substrate SG shows the best performance, which may be due to the high content and uniform morphology of Ag on ZnO. The average enhancement factors (EF) were estimated using solid Rhodamine B power in Fig. 8b according to the following formula: |
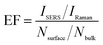 | (2) |
Where ISERS stands for the intensity of the vibrational mode in the SERS spectra and IRaman stands for the same vibrational mode in the normal Raman spectra. These data can be directly obtained from the experiment (Fig. 8a and b). Nbulk and Nsurface are the number of RB molecules illuminated by the laser focus spot under normal Raman and SERS conditions respectively. Assuming the density of 1 g cm−3 for the solid RB, the enhancement factors of SG, SE and SW can be estimated as 5.04 × 107, 3.36 × 107 and 7.35 × 105 respectively.8 Obviously, the size, morphology and content of silver nanoparticles on ZnO flowers determine the SERS performance. The different SERS response behaviors of ZnO/Ag nanocomposites may be due to different electromagnetic enhancement of AgNPs with different morphologies and content.
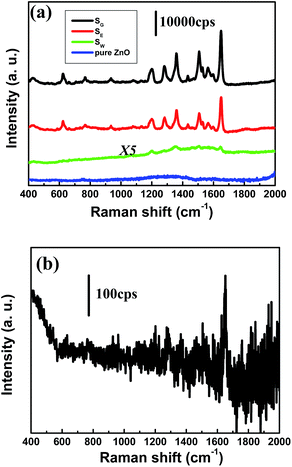 |
| Fig. 8 (a) SERS spectra of RB (10−5 mol L−1) absorbed on the ZnO flowers and ZnO/Ag nanocomposites (b) Raman spectrum of RB power. | |
Organic dyes are a very important class of dyes that are widely used in our daily life. However, some of these dyes pose a potential risk to human health. Different concentrations of organic dyes (RB, CV and MB) can be detected by SERS. Fig. 9a–c show the SERS spectra using SG substrate for different concentrations of dyes (RB, CV and MB). As the concentration of organic dyes (RB, CV and MB) decreased, the spectral intensities became quite weakened. Clearly, the detection concentration for organic dyes on the substrates was as low as 10−8 M. It can be concluded that SG SERS substrate is sufficient enough to directly observe trace amount of organic dyes.
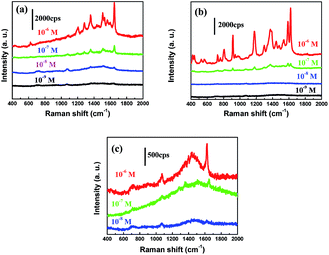 |
| Fig. 9 SERS spectra of different concentration of organic dyes absorbed on the as-prepared SG (a) RB (b) CV (c) MB. | |
3.2.2. Photocatalytic performance. Metal-decorated semiconductor nanoparticles are well known for their photocatalytic activity. For photocatalytic measurement, a mixture of 60 mL 1 × 10−5 M organic dyes (RB, CV and MB) aqueous solution and 10 mg ZnO/Ag composites were put in bottle with a capacity of 200 mL and stirred in the dark for 30 min. Then the mixture was continuously agitated throughout the experiment and irradiated by two 125 W high-pressure Hg-lamp (λmax = 365 nm). After a given irradiation time, about 3 mL the mixture was withdrawn and immediately centrifuged. The photocatalytic degradation process of organic dyes was monitored by measuring its absorption with a UV-vis spectrophotometer. The absorption profiles of organic dyes (RB, CV and MB) during the photocatalytic process are presented in Fig. 10. Fig. 10a plots the RhB concentration ratio (C/C0) as a function of irradiation time over SG, SE, SW and pure ZnO flowers under the same condition. Compared with photocatalytic activities of other three samples, SG shows the best performance. RB conversion over SG, SE, SW and pure ZnO was 100%, 93.8%, 89.5% and 71.4% respectively after 25 min of UV light irradiation. The changes of UV-vis absorption spectra of RB over SG are also shown in Fig. 10b. The reaction under UV light irradiation resulted in a transparent solution after 25 min, which is due to the destruction of the chromophoric structures of RB. The other two dyes CV (Fig. 10c) and MB (Fig. 10d) demonstrated similar patterns with RB. CV and MB disappeared from the solution after 25 min and 15 min under UV irradiation.
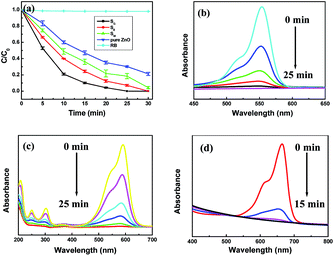 |
| Fig. 10 (a) Photocatalytic degradation of RB with various samples under UV irradiation in 25 min (b and c) The UV-visible spectrum of RB and CV dyes under UV irradiation using SG in 25 min (d) The UV-visible spectrum of MB dye under UV irradiation using SG in 15 min. | |
For SG, the uniform deposition of Ag sheets on the as-synthesized ZnO/Ag composites enlarged the interface between the Ag and ZnO and protected ZnO against photocorrosion, which can be confirmed by the corresponding EDS line scan image in Fig. 11.52 It showed the highest concentration for Zn in the middle of the particle and a high concentration of Ag on the outside edges, which is consistent with the result of EDS mapping of SG. In fact, photocatalysis is a complicated process, which is associated with not only the structure-related physical properties such as crystal plane energy and charge separation, transportation and recombination of catalysts, but also structure-related catalytic processes regarding light harvesting and molecule diffusion kinetics and adsorption thermodynamics.
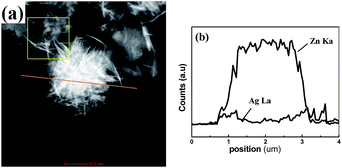 |
| Fig. 11 EDS line scan image of single SG (a) STEM image of single SG (b) EDS line scan. | |
4. Conclusions
In summary, a facile and effective photochemical method was introduced to synthesis of ZnO/Ag nanocomposites. The products were characterized by XRD, FESEM, EDS, HRTEM, XPS, PL and UV-vis. Morphological control of the ZnO/Ag nanocomposites was achieved only adjusting the reaction solvents. The ZnO/Ag nanocomposites obtained in glycol were shown to have superior SERS performance and photocatalytic activity over the other, which demonstrates their potential application as multifunctional platform. The simplicity and generality of our method for the growth selectivity offer great advantages and motivate further research on its application to other metal–metal oxide systems.
References
- X. H. Cheng, Y. Zhou, J. G. Qin and Z. Li, ACS Appl. Mater. Interfaces, 2012, 4, 2133–2138 CAS.
- A. M. López-Montes, A.-L. Dupont, B. Desmazières and B. Lavédrine, Talanta, 2013, 114, 217–226 CrossRef PubMed.
- K. S. Minioti, C. F. Sakellariou and N. S. Thomaidis, Anal. Chim. Acta, 2007, 583, 103–110 CrossRef CAS PubMed.
- S. Sebastian, Angew. Chem., Int. Ed., 2014, 53, 4756–4795 CrossRef PubMed.
- Y. M. Ma, Q. Q. Ding, L. B. Yang, L. Zhang and Y. H. Shen, Appl. Surf. Sci., 2013, 265, 346–351 CrossRef CAS PubMed.
- Y. Y. Zhang, Y. Q. Huang, F. L. Zhai, R. Du, Y. D. Liu and K. Q. Lai, Food Chem., 2012, 135, 845–850 CrossRef CAS PubMed.
- V. Jitraporn, G. R. Evan and M. Don, J. Raman Spectrosc., 2010, 41, 1137–1148 CrossRef.
- D. K. Singh, E. O. Ganbold, E. M. Cho, K. H. Cho, D. Kim, J. Choo, S. Kim, C. M. Lee, S. I. Yang and S. W. Joo, J. Hazard. Mater., 2014, 265, 89–95 CrossRef CAS PubMed.
- Z. Y. Bao, X. Liu, Y. Chen, Y. C. Wu, H. L. W. Chan, J. Y. Dai and D. Y. Lei, J. Hazard. Mater., 2014, 280, 706–712 CrossRef CAS PubMed.
- Y. Q. Wang, B. Yan and L. X. Chen, Chem. Rev., 2013, 113, 1391–1428 CrossRef CAS PubMed.
- S.-C. Luo, K. Sivashanmugan, J.-D. Liao, C.-K. Yao and H.-C. Peng, Biosens. Bioelectron., 2014, 61, 232–240 CrossRef CAS PubMed.
- Q. Zhou, Y. Yang, J. Ni, Z. C. Li and Z. J. Zhang, Nano. Res., 2010, 3, 423–425 CrossRef CAS PubMed.
- S. J. Lee, A. R. Morrill and M. Moskovits, J. Am. Chem. Soc., 2006, 128, 2200–2201 CrossRef CAS PubMed.
- M. L. Yan, Y. C. Xiang, L. L. Liu, L. Y. Chai, X. B. Lia and T. Feng, RSC Adv., 2014, 4, 98–104 RSC.
- C. Zhu, G. Meng, Q. Huang and Z. Huang, J. Hazard. Mater., 2012, 211–212, 389–390 CrossRef CAS PubMed.
- G. Liu, W. Cai, L. Kong, G. Duan, Y. Li, J. Wang and Z. Cheng, J. Hazard. Mater., 2013, 248–249, 435–440 CrossRef CAS PubMed.
- L. Wang, H. L. Li, J. Q. Tian and X. P. Sun, ACS Appl. Mater. Interfaces, 2010, 2, 2987–2991 CAS.
- B. K. Jena, B. K. Mishra and S. Bohidar, J. Phys. Chem. C, 2009, 113, 14753–14758 CAS.
- Y. Y. Xia and J. M. Wang, Mater. Chem. Phys., 2011, 125, 267–270 CrossRef CAS PubMed.
- Q. Cai, S. K. Lu, F. Liao, Y. Q. Li, S. Z. Ma and M. W. Shao, Nanoscale, 2014, 6, 8117–8123 RSC.
- C. E. Bonancea, G. M. do Nascimento, M. L. de Souza, M. L. A. Temperini and P. Corio, Appl. Catal., B, 2006, 69, 34–42 CrossRef CAS PubMed.
- Y. Q. Wang, K. Wang, B. F. Zou, T. Gao, X. L. Zhang, Z. L. Du and S. M. Zhou, J. Mater. Chem. C, 2013, 1, 2441–2447 RSC.
- X. M. Hou, Mater. Lett., 2015, 139, 201–204 CrossRef CAS PubMed.
- P. T. Dou, F. T. Tan, W. Wang, A. Sarreshteh, X. L. Qiao, X. L. Qiu and J. G. Chen, J. Photochem. Photobiol., A, 2015, 302, 17–22 CrossRef CAS PubMed.
- Y. S. Liu, S. H. Wei and W. Gao, J. Hazard. Mater., 2015, 287, 59–68 CrossRef CAS PubMed.
- M.-H. Hsu and C.-J. Chang, J. Hazard. Mater., 2014, 278, 444–453 CrossRef CAS PubMed.
- B. J. Niu, L. L. Wu, W. Tang, X. T. Zhang and Q. G. Meng, CrystEngComm, 2012, 2, 719–725 Search PubMed.
- C. Q. Chen, Y. H. Zheng, Y. Y. Zhan, X. Y. Lin, Q. Zheng and K. M. Wei, Dalton Trans., 2011, 40, 9566–9570 RSC.
- Q. Deng, X. W. Duan, D. H. L. Ng, H. B. Tang, Y. Yang, M. G. Kong, Z. K. Wu, W. P. Cai and G. Z. Wang, ACS Appl. Mater. Interfaces., 2012, 4, 6030–60377 CAS.
- S. C. Motshekga, S. S. Ray, M. S. Onyango and M. N. B. Momba, J. Hazard. Mater., 2013, 262, 439–446 CrossRef CAS PubMed.
- G. Y. Shan, S. J. Zheng, S. P. Chen, Y. W. Chen and Y. C. Liu, Colloids Surf., B, 2012, 94, 157–162 CrossRef CAS PubMed.
- H. B. Hu, Z. H. Wang, S. F. Wang, F. W. Zhang, S. P. Zhao and S. Y. Zhu, J. Alloys Compd., 2011, 509, 2016–2020 CrossRef CAS PubMed.
- P. Fageria, S. Gangopadhyay and S. Pande, RSC Adv., 2014, 4, 24962–24972 RSC.
- W. L. Ong, S. Natarajan, B. Kloostra and G. W. Ho, Nanoscale, 2013, 5, 5568–5575 RSC.
- Z. Z. Han, L. L. Ren, Z. H. Cui, C. Q. Chen, H. B. Pan and J. Z. Chen, Appl. Catal., B, 2012, 126, 298–305 CrossRef CAS PubMed.
- Q. Tao, S. Li, Q. Y. Zhang, D. W. Kang, J. S. Yang, W. W. Qiu and K. Liu, Mater. Res. Bull., 2014, 54, 6–12 CrossRef CAS PubMed.
- A. E. Kandjani, M. Mohammadtaheri, A. Thakkar, S. K. Bhargava and V. Bansal, J. Colloid Interface Sci., 2014, 436, 251–257 CrossRef CAS PubMed.
- R. Li, C. Han and Q. W. Chen, RSC Adv., 2013, 3, 11715–11722 RSC.
- X. He, H. Wang, Z. B. Li, D. Chen and Q. Zhang, Phys. Chem. Chem. Phys., 2014, 16, 14706–14712 RSC.
- Y. S. Zang, J. Yin, X. He, C. Yue, Z. M. Wu, J. Li and J. Y. Kang, J. Mater. Chem. A, 2014, 2, 7747–7753 CAS.
- Y. H. Zheng, L. R. Zheng, Y. Y. Zhan, X. Y. Lin, Q. Zheng and K. M. Wei, Inorg. Chem., 2007, 46, 6980–6986 CrossRef CAS PubMed.
- J. F. Moudler, W. F. Stickle, P. E. Sobol and K. D. Bomben, Handbook of X-ray photoelectron spectroscopy, Perkin-Elmer, Eden Prairie, MN, 1992 Search PubMed.
- D. D. Lin, H. Wu, R. Zhang and W. Pan, Chem. Mater., 2009, 21, 3479–3484 CrossRef CAS.
- D. C. Lim, I. Lopez-Salido and Y. D. Kim, Appl. Surf. Sci., 2006, 253, 959–965 CrossRef CAS PubMed.
- R. J. Zhu, X. A. Zhang, J. W. Zhao, R. P. Li and W. F. Zhang, J. Alloys Compd., 2015, 631, 125–128 CrossRef CAS PubMed.
- C. D. Gu, C. Cheng, H. Y. Huang, T. L. Wong, N. Wang and T.-Y. Zhang, Cryst. Growth Des., 2009, 9, 3278–3285 CAS.
- F. Xu, Y. T. Shen, L. T. Sun, H. B. Zeng and Y. N. Lu, Nanoscale, 2011, 3, 5020–5025 RSC.
- T. C. Deivaraj, L. L. NeetaL and J. Y. Lee, J. Colloid Interface Sci., 2005, 289, 402–409 CrossRef CAS PubMed.
- J. Roh, H. N. Umh, H. K. Sung, B. Lee and Y. Kim, Colloids Surf., A, 2012, 415, 449–453 CrossRef CAS PubMed.
- T. Hirakawa and P. V. Kamat, J. Am. Chem. Soc., 2005, 127, 3928–3934 CrossRef CAS PubMed.
- G.-B. Junga, J.-H. Kim, J. S. Burm and H.-K. Park, Appl. Surf. Sci., 2013, 273, 179–183 CrossRef PubMed.
- Z. Chen, N. Zhang and Y. J. Xu, CrystEngComm, 2013, 15, 3022–3030 RSC.
|
This journal is © The Royal Society of Chemistry 2015 |
Click here to see how this site uses Cookies. View our privacy policy here.