DOI:
10.1039/C9SC02151E
(Edge Article)
Chem. Sci., 2019,
10, 6770-6776
Living supramolecular polymerization based on reversible deactivation of a monomer by using a ‘dummy’ monomer†
Received
2nd May 2019
, Accepted 21st June 2019
First published on 1st July 2019
Abstract
Although living supramolecular polymerization (LSP) has recently been realized, the scope of the monomer structures applicable to the existing methods is still limited. For instance, a monomer that spontaneously nucleates itself cannot be processed in a manner consistent with LSP. Herein, we report a new method for such a “reactive” monomer. We use a ‘dummy’ monomer which has a similar structure to the reactive monomer but is incapable of one-dimensional supramolecular polymerization. We show that in the presence of the dummy monomer, the reactive monomer is kinetically trapped in the dormant state. In this way, spontaneous nucleation of the reactive monomer is retarded; yet, addition of seeds of a supramolecular polymer can initiate the supramolecular polymerization in a chain growth manner. As a result, we obtain the supramolecular polymer of the reactive monomer with a controlled length, which is otherwise thermodynamically inaccessible. We believe that this concept will expand the scope of LSP for the synthesis of other functional supramolecular polymers, and thus lead to a variety of applications.
Introduction
Most supramolecular polymerizations occur spontaneously with a negligible activation barrier for initiation. In other words, a monomer is highly “reactive” toward polymerization. Thus, controlling supramolecular polymerization has remained a significant challenge.1 On the other hand, a similar issue for covalent bond-based polymerization has been successfully resolved even for radical species, by taming radical polymerization into living radical polymerization (LRP). Though there are several approaches for LRP, almost all the current methods are based on a common principle, in which a reversible and dynamic equilibrium generating a so-called dormant state is coupled with the polymerization reaction (Fig. 1a).2,3 The coupled equilibrium decreases the effective concentration of the reactive species (i.e., the propagation sites), thereby allowing for kinetic control of the concurrent polymerization.
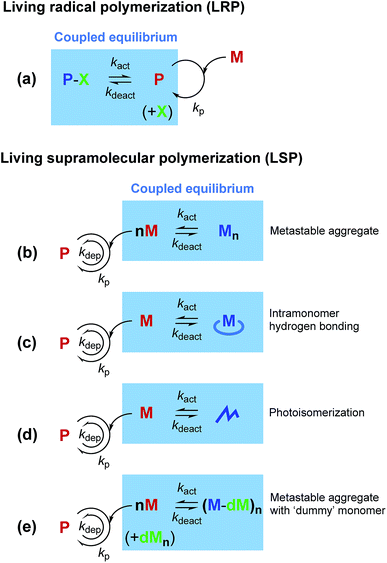 |
| Fig. 1 (a) Mechanism of living radical polymerization (LRP). Existing mechanisms of living supramolecular polymerization (LSP) based on the (b) formation of metastable aggregates, (c) formation of intramonomer hydrogen bonds, and (d) photoisomerization. (e) Proposed mechanism of LSP using a ‘dummy’ monomer (dM) to entrap the active monomer (M). Species shown in red are reactive, while those shown in blue are dormant. | |
In the last decade, mechanistic insights into supramolecular polymerization have been significantly enriched,1,4–11 and have recently led to the development of living supramolecular polymerization (LSP).12–30 It is intriguing that both LRP and LSP rely on reversible and dynamic equilibrium coupled with the polymerization pathway. So far, the formation of metastable aggregates (Fig. 1b)12–17 and intramonomer hydrogen bonds (Fig. 1c),18–23 and photoisomerization (Fig. 1d)24 have been shown to be effective in generating the dormant states in LSP. In this context, the difference between LRP and LSP is also noteworthy. In LRP, the reactive polymer terminus is deactivated (or “capped”) in the dormant state, while in LSP, the spontaneous nucleation of monomers is kinetically suppressed, because of which the monomer itself needs to be deactivated. Inevitably, the monomer design is crucial for achieving LSP, which has limited the scope of the existing methods of LSP for synthesis of other functional supramolecular polymers. In fact, it has been shown that a subtle structural modification in the monomer influences the energy landscape of the self-assembly and often disables the monomer for participating in the LSP.13,20,31 Herein, we show a new concept that would be more tolerant in terms of the monomer design, in comparison with the other methods reported so far. In the present approach, we use a ‘dummy’ monomer that is incapable of one-dimensional (1D) supramolecular polymerization but can entrap a “reactive” monomer into the dormant state owing to the structural similarity (Fig. 1e).
Results and discussion
Impact of monomer design on supramolecular polymerization
The structures of the porphyrin monomers used in this study are shown in Fig. 2. As reported previously, 3Me can be processed in a manner consistent with LSP via the formation of off-pathway aggregates as the dormant species (Fig. 1b).123Me initially forms a metastable off-pathway aggregate but transforms into a thermodynamically stable supramolecular polymer after a lag time (details are given in Fig. S2†). These two aggregates have different porphyrin stacking modes: the former is the J-aggregate while the latter is the H-aggregate. This time-dependent transformation allowed for kinetic control of supramolecular polymerization. It should be noted that in practice, a lag time of about an hour is required to perform LSP conveniently.
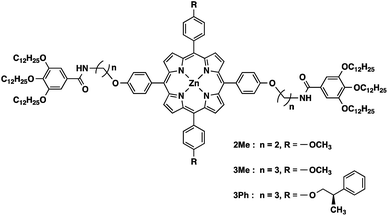 |
| Fig. 2 Structures of porphyrin monomers used in this study. 3Ph is a ‘dummy’ monomer which has a similar structure to the monomers (2Me and 3Me) but is incapable of one-dimensional supramolecular polymerization.31 | |
Despite the structural similarity, the self-assembly of 2Me was distinct from that of 3Me. Cooling a hot methylcyclohexane (MCH) solution of 2Me did not form the metastable J-aggregate but immediately resulted in heavily bundled supramolecular polymers as precipitates (Fig. 3a–c). The difference between the self-assembly of 2Me and 3Me is attributable to the even–odd effect of the alkylene spacers.32,33 We note that Würthner and co-workers20 have previously found the length of the alkylene spacers to influence the LSP in a different mechanism (Fig. 1c). These results clearly exemplify the criticality of the monomer design in achieving LSP.
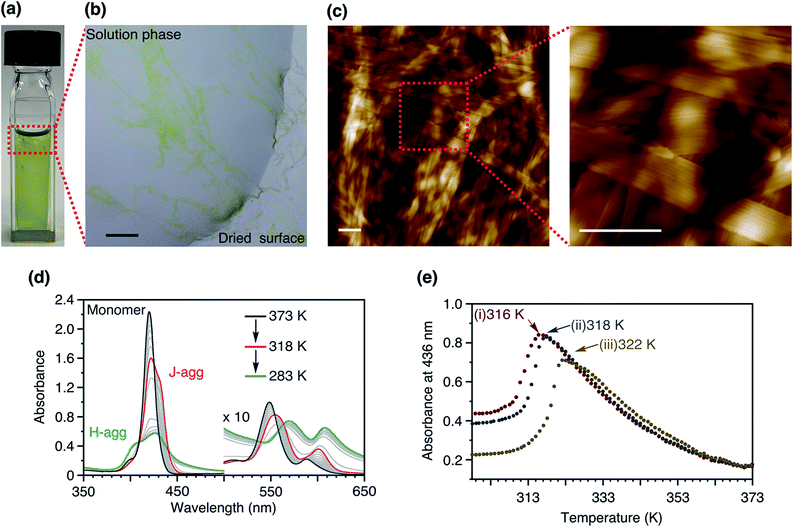 |
| Fig. 3 (a) Photograph of the precipitate of 2Me in MCH. (b) Optical microscopy image (scale bar = 20 μm) and (c) atomic force microscopy (AFM) images (scale bar = 200 nm) of the heavily bundled supramolecular polymer of 2Me. (d) Temperature-dependent changes in the absorption spectra of 2Me in MCH. The temperature was changed from 373 to 283 K at a rate of −1.0 K min−1; [2Me] = 50 μM. Path length of cuvette = 1 mm. (e) Plots of the change in absorbance of 2Me at 436 nm as a function of temperature obtained with different cooling rates. The temperature was changed from 373 to 283 K at a rate of (i) −10 K min−1, (ii) −1 K min−1, and (iii) −0.1 K min−1. | |
To further gain insights into the supramolecular polymerization of 2Me, we studied the temperature-dependent changes in its absorption spectra (Fig. 3d). Upon cooling (−1.0 K min−1), the Soret band underwent a red-shift with the concomitant appearance of isosbestic points (373 to 318 K), thus suggesting the formation of J-aggregates.12 This process was analyzed by the isodesmic model,1 which yielded thermodynamic parameters comparable with those of the J-aggregate formation of 3Me (Fig. S3†). However, further cooling of the solution below 318 K resulted in the appearance of a blue-shifted Soret band, suggesting the formation of H-aggregates.12,13 This process was accompanied by a concomitant increase in the base line due to light scattering by the precipitates, thereby leading to a deviation of the isosbestic points. The complex aggregation behavior as well as the precipitation hindered further mechanistic studies to estimate the thermodynamic parameters.
The turnaround temperature at which the J-aggregates began converting to H-aggregates was dependent on the cooling rate (Fig. 3e). This behavior indicates that the formation of the H-aggregate of 2Me is under kinetic control to a certain extent. However, even when a hot solution of 2Me was quenched in an ice bath, spontaneous nucleation of the H-aggregate could not be prevented. Due to the absence of a lag time, the LSP of 2Me was not feasible (Fig. S4†).
Kinetic trapping of 2Me by using a dummy monomer, 3Ph
To address this issue, we conceived a solution based on our previous findings regarding a mixture of 3Me and 3Ph.313Ph has a similar structure to 3Me, and likewise, forms a J-aggregate; however, the steric hindrance of the side chains prevents the face-to-face stacking of the porphyrin plane (i.e., H-aggregate supramolecular polymerization). When a hot solution of 3Me and 3Ph in MCH was cooled down, 3Me and 3Ph co-assembled into a J-aggregate. We found that this co-assembled J-aggregate was metastable, and the system showed time-dependent narcissistic self-sorting to the H-aggregates of 3Me and J-aggregates of 3Ph after a lag time.31,34–36 Interestingly, this lag time was dependent on the ratio of 3Me to 3Ph; the smaller the ratio, the longer was the lag time. Considering this, we envisaged that if 2Me and 3Ph could co-assemble to form a J-aggregate owing to the structural similarity, the spontaneous nucleation of the 2Me H-aggregate would be retarded, thus enabling kinetic control of the supramolecular polymerization of 2Me.
To verify this concept, we studied the temperature-dependent changes in the absorption spectra of 2Me in the presence of 3Ph (Fig. 4a, molar ratio of 2Me
:
3Ph = 50
:
50). As shown in Fig. 4b, the formation of J-aggregates followed a sigmoidal cooling curve down to room temperature. As such, 2Me was trapped in the J-aggregate in the presence of the ‘dummy’ monomer, 3Ph (Fig. S5†). We infer that the J-aggregate is not crystalline and is tolerant to co-assembly with monomers having different structures.35
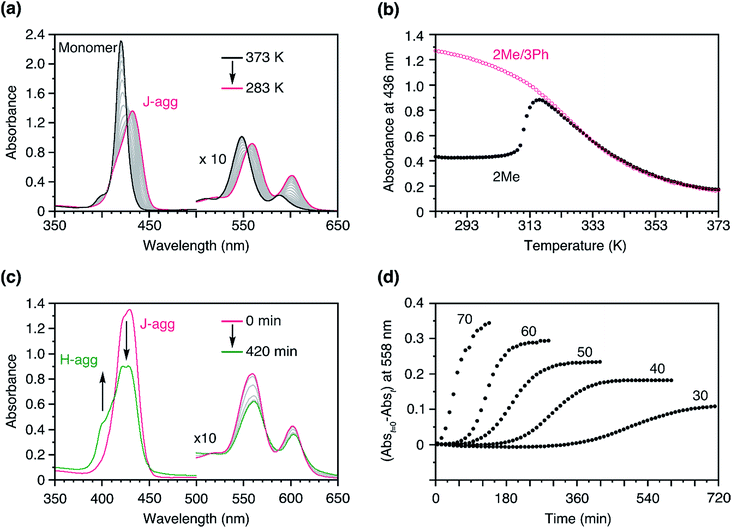 |
| Fig. 4 (a) Temperature-dependent changes in the absorption spectra of 2Me in the presence of 3Me in MCH. The temperature was changed from 373 to 283 K at a rate of −1.0 K min−1; [2Me + 3Ph] = 50 μM, 2Me : 3Ph (molar ratio) = 50 : 50. Path length of cuvette = 1 mm. (b) Plots of the changes in absorbance at 436 nm as a function of temperature, measured in the presence (pink: a) and absence (black: Fig. 3d) of 3Ph. The temperature was changed from 373 to 283 K at a rate of −1.0 K min−1; [2Me + 3Ph] = 50 μM. (c) Time-dependent changes in the absorption spectra of a mixture of 2Me and 3Ph obtained after cooling a hot solution to 308 K from 373 K; [2Me + 3Ph] = 50 μM, molar ratio of 2Me : 3Ph = 50 : 50. (d) Plots of the changes in absorbance of a mixture of 2Me and 3Ph in MCH at 558 nm as a function of time, measured at 308 K; [2Me + 3Ph] = 50 μM. The numerical figures indicate the percentage of 2Me in the co-assembled J-aggregate. Path length of cuvette = 10 mm. The solutions were stirred at a rate of 400 rpm. | |
As in the case of the 3Me/3Ph system,31 the J-aggregate consisting of 2Me and 3Ph was also metastable and showed time-dependent spectral changes (Fig. 4c). When we fixed the total concentration of the porphyrins but changed their mixing ratio, the net changes in absorbance with time (Abst=0 − Abst) were dependent on the proportion of 2Me (Fig. 4d). This result suggests that 3Ph was not involved in the transformation and remained as a J-aggregate, namely, time-dependent narcissistic self-sorting (Fig. S6†). Furthermore, a larger proportion of 2Me resulted in a shorter lag time. These results are consistent with those of our previous study.31 The resultant lag time (49 min for 2Me
:
3Ph = 70
:
30) was shorter than that of the 3Me/3Ph mixture (555 min for 3Me
:
3Ph = 70
:
30), because of the higher nucleation propensity of 2Me as compared with 3Me; nonetheless, it was long enough for the LSP to proceed further.
Living supramolecular polymerization of 2Me
As an initiator for LSP, we prepared seeds of the supramolecular polymer (i.e., H-aggregate) of 2Me by sonication, with a number average length (Ln) of 134 nm (details are given in the ESI, see Fig. S7†). The addition of the seeds to a solution of co-assembled 2Me/3Ph J-aggregates (50
:
50 molar ratio) eliminated the lag time and initiated the supramolecular polymerization (Fig. 5a). The logarithm of the apparent polymerization rate, log(−d(Abs558)/dt), and the amount of the added seed were proportional with a slope of 0.97, thus indicating a first-order polymerization with respect to the concentration of the seed (Fig. 5b).12
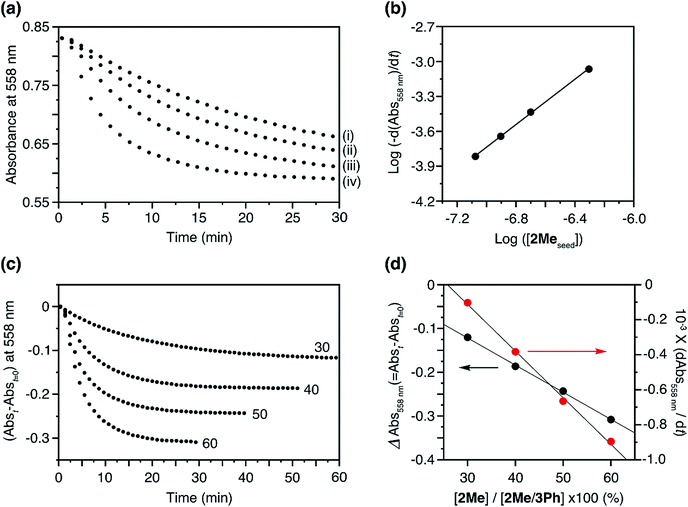 |
| Fig. 5 (a) Plots of the changes in absorbance at 558 nm upon the addition of 2Me H-aggregate seeds to the solution of 2Me/3Ph co-assembled J-aggregates; [2Me in the seed] = (i) 0.084, (ii) 0.125, (iii) 0.200, and (iv) 0.495 μM, [2Me + 3Me in the co-assembled J-aggregate] = 50 μM, molar ratio of 2Me : 3Ph in the co-assembled J-aggregate was 50 : 50. Path length of cuvette = 10 mm. (b) log–log plot of the rate of decrease of absorbance at 558 nm as a function of the concentration of 2Me seeds. (c) Plots of the changes in absorbance at 558 nm upon the addition of 2Me seeds to the solution of 2Me/3Ph co-assembled J-aggregates; [2Me in the seed] = 0.495 μM, [2Me + 3Me in the co-assembled J-aggregate] = 50 μM, molar ratios of 2Me : 3Ph in the J-aggregate = 60 : 40, 50 : 50, 40 : 60, and 30 : 70. The numerical figures indicate the percentage of 2Me in the co-assembled J-aggregate. Path length of cuvette = 10 mm. (d) Plots of the net changes in absorbance with time (Abst=0 − Abst: t = 30, 40, 50, and 60 min for the ratios (2Me : 3Ph) of 60 : 40, 50 : 50, 40 : 60, and 30 : 70, respectively) (black circle) and polymerization rates (red circle) as a function of the proportion of 2Me in the 2Me/3Ph co-assembled J-aggregates. The polymerization rates were determined by linear analysis for the initial polymerization process (a few minutes after the addition of the seed) in Fig. 5c. | |
With a fixed amount of the seeds, the net changes in absorbance with time (Abst=0 − Abst) were proportional to the proportion of 2Me in the co-assembled 2Me/3Ph J-aggregates, which indicated that only 2Me was involved in the LSP (Fig. 5c and d). Correspondingly, the polymerization rate (−d(Abs558)/dt) was also dependent on the proportion of 2Me (Fig. 5d, red circle). In this way, the supramolecular polymerization of 2Me, which was otherwise spontaneous, could be kinetically controlled in the presence of the ‘dummy’ monomer, 3Ph. Importantly, this seeded supramolecular polymerization process was repeatable as in the case of our previous studies (Fig. S8†).12,13
After the LSP, we obtained a clear solution without any precipitate (Fig. S9†). This was spin-coated on a highly oriented pyrolytic graphite (HOPG). Observation under atomic force microscopy (AFM) revealed the presence of both 1D supramolecular polymers and J-aggregate nanoparticles, consisting of 2Me and 3Ph, respectively (Fig. 6a). Statistical analysis for over 100 objects revealed that the number average length (Ln) and weight average length (Lw) of the obtained supramolecular polymers were proportional to the ratio of the concentrations of 2Me in the seed and 2Me in the co-assembled J-aggregate (Fig. 6b and c, and S10 and S11†). The polydispersity index (PDI: Lw/Ln), the distribution of the length, of the thus-obtained supramolecular polymer of 2Me was 1.36, which was much smaller than the value predicted based on a thermodynamic equilibrium (2.0).1,12 Remarkably, supramolecular polymers obtained in this way did not bundle, which clearly contrasts the structures obtained under thermodynamic control (Fig. 3c). This observation would imply that the strong propensity of precipitation of 2Me (Fig. 3a–c) originates from heterogeneous secondary nucleation through lateral interactions among the one-dimensional supramolecular polymers.37 Thus, LSP allows control over the nucleation–elongation process, leading to control over structures and properties (such as crystallinity) of supramolecular polymers at higher hierarchy.
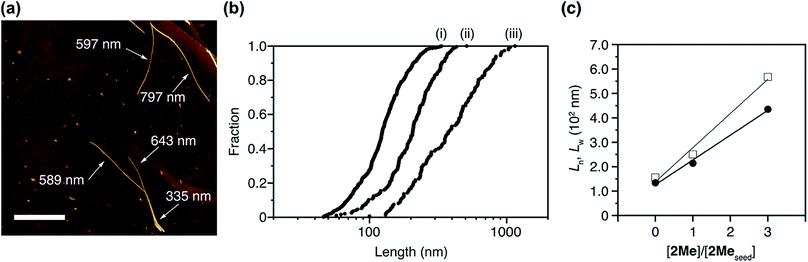 |
| Fig. 6 (a) AFM image (scale bar = 400 nm) of the supramolecular polymer of 2Me obtained through LSP using 3Ph as a ‘dummy’ monomer; [2Me in the seed] : [2Me in the co-assembled J-aggregate] = 1 : 3; on a HOPG substrate. (b) Histogram of the length distribution of (i) seed and supramolecular polymer of 2Me obtained under the conditions of [2Me in the seed] : [2Me in the co-assembled J-aggregate] = (ii) 1 : 1 and (iii) 1 : 3. (c) Number average length (filled circle) and weight average length (open square) as a function of the ratio of concentrations of 2Me in the seed and in the co-assembled J-aggregate. | |
Conclusions
In conclusion, we demonstrated a new LSP method for a monomer that spontaneously nucleated without a lag time. We used a ‘dummy’ monomer that was incapable of 1D supramolecular polymerization but could entrap an active monomer into the dormant state. We note that 2Me is still a unique monomer in that it undergoes biphasic self-assembly (Fig. 3d).10,11,31 Nevertheless, we believe that the present study shows a new possibility of creating a dormant state based on coupled equilibria, which is the key to expanding the scope of LSP and its further applications.
Conflicts of interest
There are no conflicts to declare.
Acknowledgements
This work was supported by KAKENHI (JP15H05483 for K. S.), Scientific Research on Innovative Areas “Dynamical ordering of biomolecular systems for creation of integrated functions (JP16H00787 for K. S.)”, “π-System figuration: control of electron and structural dynamism for innovative functions (JP26102009 for M. T.)”, and the Nanotechnology Network Project from the Ministry of Education, Culture, Sports, Science and Technology, Japan. T. F. thanks the Japan Society for the Promotion of Science (JSPS) for a research fellowship for young scientists (16J02156) and a postdoctoral fellowship for research abroad.
Notes and references
- T. F. A. De Greef, M. M. J. Smulders, M. Wolffs, A. P. H. J. Schenning, R. P. Sijbesma and E. W. Meijer, Chem. Rev., 2009, 109, 5687–5754 CrossRef CAS PubMed.
- M. Ouchi, T. Terashima and M. Sawamoto, Chem. Rev., 2009, 109, 4963–5050 CrossRef CAS PubMed.
- K. Matyjaszewski and J. Xia, Chem. Rev., 2001, 101, 2921–2990 CrossRef CAS PubMed.
- Z. Chen, A. Lohr, C. R. Saha-Möller and F. Würthner, Chem. Soc. Rev., 2009, 38, 564–584 RSC.
- C. Rest, R. Kandanelli and G. Fernández, Chem. Soc. Rev., 2015, 44, 2543–2572 RSC.
- D. Zhao and J. S. Moore, Org. Biomol. Chem., 2003, 1, 3471–3491 RSC.
- P. Jonkheijm, P. van der Schoot, A. P. H. J. Schenning and E. W. Meijer, Science, 2006, 313, 80–83 CrossRef CAS PubMed.
- M. M. J. Smulders, M. M. L. Nieuwenhuizen, T. F. A. De Greef, P. Schoot, A. P. H. J. Schenning and E. W. Meijer, Chem.–Eur. J., 2010, 16, 362–367 CrossRef CAS PubMed.
- P. A. Korevaar, S. J. George, A. J. Markvoort, M. M. J. Smulders, P. A. J. Hilbers, A. P. H. J. Schenning, T. F. A. De Greef and E. W. Meijer, Nature, 2012, 481, 492–496 CrossRef CAS PubMed.
- F. Fennel, S. Wolter, Z. Xie, P.-A. Plöz, O. Kürn, F. Würthner and S. Lochbrunner, J. Am. Chem. Soc., 2013, 135, 18722–18725 CrossRef CAS PubMed.
- M. F. J. Mabesoone, A. J. Markvoort, M. Banno, T. Yamaguchi, F. Helmich, Y. Naito, E. Yashima, A. R. A. Palmans and E. W. Meijer, J. Am. Chem. Soc., 2018, 140, 7810–7819 CrossRef CAS PubMed.
- S. Ogi, K. Sugiyasu, S. Manna, S. Samitsu and M. Takeuchi, Nat. Chem., 2014, 6, 188–195 CrossRef CAS PubMed.
- T. Fukui, S. Kawai, S. Fujinuma, Y. Matsushita, T. Yasuda, T. Sakurai, S. Seki, M. Takeuchi and K. Sugiyasu, Nat. Chem., 2017, 9, 493–499 CrossRef CAS PubMed.
- T. Fukui, T. Uchihashi, N. Sasaki, H. Watanabe, M. Takeuchi and K. Sugiyasu, Angew. Chem., Int. Ed., 2018, 57, 15465–15470 CrossRef CAS PubMed.
- A. T. Haedler, S. C. J. Meskers, R. H. Zha, M. Kivala, H.-W. Schmidt and E. W. Meijer, J. Am. Chem. Soc., 2016, 138, 10539–10545 CrossRef CAS PubMed.
- X. Ma, Y. Zhang, Y. Zhang, Y. Liu, Y. Che and J. Zhao, Angew. Chem., Int. Ed., 2016, 55, 9539–9543 CrossRef CAS PubMed.
- G. Ghosh and S. Ghosh, Chem. Commun., 2018, 54, 5720–5723 RSC.
- J. Kang, D. Miyajima, T. Mori, Y. Inoue, Y. Itoh and T. Aida, Science, 2015, 347, 646–651 CrossRef CAS PubMed.
- S. Ogi, V. Stepanenko, K. Sugiyasu, M. Takeuchi and F. Würthner, J. Am. Chem. Soc., 2015, 137, 3300–3307 CrossRef CAS PubMed.
- S. Ogi, V. Stepanenko, J. Thein and F. Würthner, J. Am. Chem. Soc., 2016, 138, 670–678 CrossRef CAS PubMed.
- W. Wagner, M. Wehner, V. Stepanenko, S. Ogi and F. Würthner, Angew. Chem., Int. Ed., 2017, 56, 16008–16012 CrossRef CAS PubMed.
- S. Ogi, K. Matsumoto and S. Yamaguchi, Angew. Chem., Int. Ed., 2018, 57, 2339–2343 CrossRef CAS PubMed.
- E. E. Greciano, B. Matarranz and L. Sanchez, Angew. Chem., Int. Ed., 2018, 57, 4697–4701 CrossRef CAS PubMed.
- M. Endo, T. Fukui, S. H. Jung, S. Yagai, M. Takeuchi and K. Sugiyasu, J. Am. Chem. Soc., 2016, 138, 14347–14353 CrossRef CAS PubMed.
- A. Pal, M. Malakoutikhah, G. Leonetti, M. Tezcan, M. Colomb-Delsuc, V. D. Nguyen, J. van der Gucht and S. Otto, Angew. Chem., Int. Ed., 2015, 54, 7852–7856 CrossRef CAS PubMed.
- E. M. Robinson, D. J. Lunn, A. Nazemi, G. R. Whittell, L. De Cola and I. Manners, Chem. Commun., 2015, 51, 15921–15924 RSC.
- M. E. Robinson, A. Nazemi, D. J. Lunn, D. W. Hayward, C. E. Boott, M.-S. Hsiao, R. L. Harniman, S. A. Davis, G. R. Whittell, R. M. Richardson, L. De Cola and I. Manners, ACS Nano, 2017, 11, 9162–9175 CrossRef CAS PubMed.
- S. H. Jung, D. Bochicchio, G. M. Pavan, M. Takeuchi and K. Sugiyasu, J. Am. Chem. Soc., 2018, 140, 10570–10577 CrossRef CAS PubMed.
- A. Mishra, D. B. Korlepara, M. Kumar, A. Jain, N. Jonnalagadda, K. K. K. Bejagam, S. Balasubramanian and S. J. George, Nat. Commun., 2018, 9, 1295 CrossRef PubMed.
- A. Jain, S. Dhiman, A. Dhayani, P. K. Vemula and S. J. George, Nat. Commun., 2019, 10, 450 CrossRef PubMed.
- S. Ogi, T. Fukui, M. L. Jue, M. Takeuchi and K. Sugiyasu, Angew. Chem., Int. Ed., 2014, 53, 14363–14367 CrossRef CAS PubMed.
- T. Shimizu and M. Masuda, J. Am. Chem. Soc., 1997, 119, 2812–2818 CrossRef CAS.
- N. Fujita, Y. Sakamoto, M. Shirakawa, M. Ojima, A. Fujii, M. Ozaki and S. Shinkai, J. Am. Chem. Soc., 2007, 129, 4134–4135 CrossRef CAS PubMed.
- M. M. Safont-Sempere, G. Fernández and F. Würthner, Chem. Rev., 2011, 111, 5784–5814 CrossRef CAS PubMed.
- T. Fukui, M. Takeuchi and K. Sugiyasu, Sci. Rep., 2017, 7, 2425 CrossRef PubMed.
- K. Aratsu, D. D. Prabhu, H. Iwawaki, X. Lin, M. Yamauchi, T. Karatsu and S. Yagai, Chem. Commun., 2016, 52, 8211–8214 RSC.
- J. Chen, E. Zhu, J. Liu, S. Zhang, Z. Lin, X. Duan, H. Heinz, Y. Huang and J. J. De Yoreo, Science, 2018, 362, 1135–1139 CrossRef CAS PubMed.
Footnotes |
† Electronic supplementary information (ESI) available. See DOI: 10.1039/c9sc02151e |
‡ Present address: Department of Chemistry, University of Victoria, 3800 Finnerty Road, Victoria, B.C. V8P 5C2, Canada. |
|
This journal is © The Royal Society of Chemistry 2019 |
Click here to see how this site uses Cookies. View our privacy policy here.