DOI:
10.1039/D2NH00070A
(Review Article)
Nanoscale Horiz., 2022,
7, 682-714
Engineered extracellular vesicles as intelligent nanosystems for next-generation nanomedicine
Received
10th February 2022
, Accepted 18th May 2022
First published on 19th May 2022
Abstract
Extracellular vesicles (EVs), as natural carriers of bioactive cargo, have a unique micro/nanostructure, bioactive composition, and characteristic morphology, as well as fascinating physical, chemical and biochemical features, which have shown promising application in the treatment of a wide range of diseases. However, native EVs have limitations such as lack of or inefficient cell targeting, on-demand delivery, and therapeutic feedback. Recently, EVs have been engineered to contain an intelligent core, enabling them to (i) actively target sites of disease, (ii) respond to endogenous and/or exogenous signals, and (iii) provide treatment feedback for optimal function in the host. These advances pave the way for next-generation nanomedicine and offer promise for a revolution in drug delivery. Here, we summarise recent research on intelligent EVs and discuss the use of “intelligent core” based EV systems for the treatment of disease. We provide a critique about the construction and properties of intelligent EVs, and challenges in their commercialization. We compare the therapeutic potential of intelligent EVs to traditional nanomedicine and highlight key advantages for their clinical application. Collectively, this review aims to provide a new insight into the design of next-generation EV-based theranostic platforms for disease treatment.
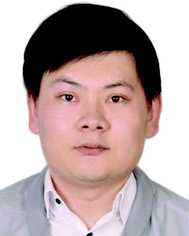
Zhijin Fan
| Zhijin Fan received his BS degree in Biology from Shandong Normal University in 2011. He obtained his master's degree in biophysics under the guidance of Prof. Da Xing from South China Normal University. In 2017, he joined Sun Yat-sen University as a full-time scientific researcher. Now, he is studying as a PhD candidate under the guidance of Prof. Liming Nie and Prof. Changhong Liang in Guangdong Provincial People's Hospital Affiliated to South China University of Technology. His current research interests are the development of multifunctional optical molecular probes and their application in cancer theranostics. |
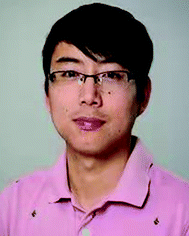
Cheng Jiang
| Dr Cheng Jiang received his PhD degree in 2016 from the University of New South Wales under the supervision of Prof. Justin Gooding. He then started his postdoctoral career in 2017 and currently is a senior research scientist, and co-PI at the Nuffield Department of Clinical Neurosciences, University of Oxford. He won the Early Career Research Award in 2020 at Oxford. His main research interests include fundamental surface chemistry for precise manipulation of mixed molecular layers, electron transfer, biofouling resistance, and also their translational applications for in vitro & in vivo sensing toward exosomal biomarker discovery and personalized theranostics in neurodegenerative disease. |
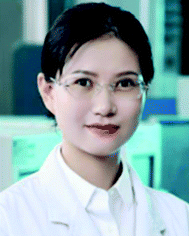
Yichao Wang
| Yichao Wang received her PhD degree in Laboratory Medicine of Tianjin Medical University in 2017, and was a visitor scholar in the Methodist Nanomedicine Institute of US from December 2014 to December 2015. She obtained her master's degree in Laboratory Medicine of Tianjin Medical University in 2013 and her bachelor's degree from The Fourth Military Medical University in 2009. Now, she is studying as a post-doctoral student in the Naval Medical University. Her current research interests are the development of clinical diagnostic assay for biomarkers and its application in theranostics. |
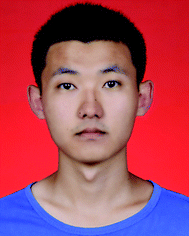
Kaiyuan Wang
| Kaiyuan Wang is a doctoral candidate in Pharmaceutics at Shenyang Pharmaceutical University, and his research focuses on biomimetic drug delivery systems for cancer immunotherapy and deep tumor penetration. |
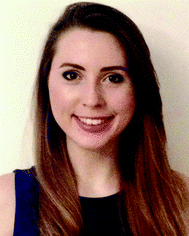
Jade Marsh
| Dr Jade Marsh is a postdoctoral research assistant at the University of Oxford. Her research focuses on molecular neurodegeneration. |
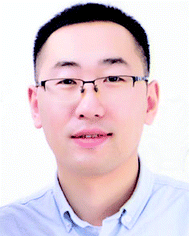
Da Zhang
| Da Zhang received his PhD degree from the College of Chemistry, Fuzhou University, and is a professor at Mengchao Hepatobiliary Hospital of Fujian Medical University. His current research interests are the development of biomimetic nanosystems for targeted cancer therapy, adoptive immunotherapy, and neoantigen-based vaccines for immunotherapy of hepatocellular carcinoma. He has published over 40 papers (10 papers with IFs over 10), and 6 patents as the corresponding author in the fields of biomedicine and materials science, including Angewandte Chemie International Edition, ACS Nano, Advanced Science, iScience, the Chemical Engineering Journal, Theranostics and Small. |
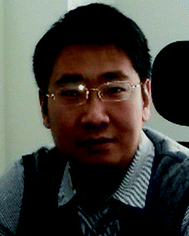
Xin Chen
| Dr Xin Chen is a Professor of Xi’an Jiaotong University (XJTU), P. R. China. He also took honor professor positions at The Fourth Military Medical University, The Second Affiliated Hospital of XJTU and Sichuan University of Science & Engineering. Prof. Chen mainly engaged in the research of synthesis, functionalization and application of stimuli-responsive biomaterials for disease therapy and tissue regeneration. He is hosting 15 national and provincial grants, and has published over 60 papers (18 papers with IFs over 10), 3 book chapters and 10 patents as the corresponding author in the fields of biomedicine and materials science, including Nature Biomedical Engineering, Science Translational Medicine, Science Advances, Advanced Functional Materials, ACS Nano, Biomaterials and the Journal of Materials Chemistry A. Based on his academic reputation, Prof. Chen got the honors of provincial level scholar and national level scholar in 2016 and 2020, respectively. He was also selected as the committee member of the National Society of Tissue Engineering and Regenerative Medicine in 2019 and the National Society of Oral Biomedicine in 2020. |
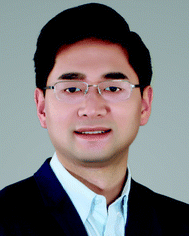
Liming Nie
| Liming Nie is a professor and PhD supervisor at the Research Center of Medical Sciences of Guangdong Provincial People's Hospital, Guangdong Academy of Medical Sciences. His laboratory focuses on optical imaging technology, mainly on photoacoustic microscopy, functional photoacoustic tomography, and other optical imaging modalities. He has published over 80 papers (more than 5613 citations in total) as the corresponding author in the fields of biomedicine and materials science, including Nature Nanotechnology, Nature Biotechnology, Nature Communications, Advanced Materials, Chemical Society Reviews, the Journal of the American Chemical Society, Angewandte Chemie International Edition, ACS Nano, Advanced Science, Biomaterials and Small. One of his aims is to provide effective and low-cost imaging instruments for disease diagnosis and treatment monitoring. |
Introduction
Advances in nanotechnology have made a significant impact in the biomedical field.1–7 For instance, novel nanomaterials have been used to develop therapeutic agents,8–15 diagnostic or contrast agents,16–22 and drug delivery vectors (DDVs),23–27 demonstrating their successful application in biomedicine.28–31 Nanomaterials offer advantageous and editable properties such as considerable loading capacity and sufficient protection for drugs, controllable drug release, and targeting capability. However, nanomaterials namely carbon nanotubes, graphene oxide (GO), noble metal nanoparticles (NMNPs), and mesoporous silicon nanoparticles (MSNs) still suffer from non-specific cytotoxicity, undesirable biocompatibility, and poor delivery efficacy.32–38 Nanoparticles may bind to serum proteins and immune system components in the blood, leading to their recognition and elimination by the immune system and a compromised patient outcome.39–41 In addition, these nanomaterials are either metabolized inefficiently, or not at all, posing risks of accumulation and toxicity. Therefore, there is an urgent need to develop novel DDVs with improved biocompatibility and targeted-delivery.
Recently, researchers have explored using extracellular vesicles (EVs) as natural and alternative DDVs through encapsulation of drugs/nanodrugs.42–45 EVs are membrane-bound vesicular particles with sizes between 30 and 1000 nm.46–49 They can be generated by a wide variety of cell types and exist in most bodily fluids. They serve as an intercellular communication medium by delivering active factors such as nucleic acids and proteins.50–53 The cargo of EVs reflects the physiological state of cells from which they originate and influences the function of recipient cells, enabling them to be used for diagnostic and therapeutic purposes.54–66 As natural DDVs, EVs demonstrate more attractive characteristics than existing nanotheranostic systems, including enhanced biocompatibility,67 high-stability, long-circulation lifespans,68 low-immunogenicity,69 targeting or tropism capability, high-potential to cross the blood–brain barrier (BBB),70–72 and pathogen capture characteristics,73–75 providing a unique therapeutic nanoplatform.76–84 Excitingly, clinical trials for EV-based treatments in humans have been approved and are rapidly increasing.85–87
Although EVs have shown promising performances in preclinical trials, achieving a precise and controllable release of cargo at the target site remains a challenge (Table 1).88–90 To address this issue, researchers have engineered EVs that contain synthetic nanomaterials (intelligent EVs). The nanomaterials are responsive to different environmental stimuli including endogenous stimuli (e.g., pH, redox potential, nucleic acid marker, and enzyme presence) and exogenous stimuli (e.g., temperature, light, magnetic field, and ultrasound) (Scheme 1). These properties give the EVs the ability to release drugs “on-demand”, in response to specific stimuli. In addition, active targeting and therapeutic feedback should also be an essential part of intelligent nanosystems. Although native vesicles bear certain inherent function in terms of targeting, but it is passive targeting and their capabilities of on-demand drug release, and therapeutic feedback are limited, which has been intensively studied in recent years by external engineering strategies. However, a systematic review is still missing to cover this topic. In this review, we aim to critically discuss the recent research on intelligent EVs and their mimetics, with highlights on their advantages and limitations. We also discussed the perspectives of EV-based DDVs in biomedical applications and their challenges in clinical transformation. We aim in this review to offer new insight to help direct the development of next-generation EV-based therapies in the future.
Table 1 Comparison between the advantages and disadvantages of different nanocarriers for disease theranostics
Type |
Advantages |
Disadvantages |
Ref. |
Traditional nanoparticles |
(1) Controllable particle size |
(1) Poor biocompatibility |
91–93
|
(2) Versatile surface modifications |
(2) Potential toxicity |
(3) Diversified morphologies and forms |
(3) Poor biodegradability |
(4) Batch preparation |
(4) High immunogenicity |
(5) Design according to requirements |
|
|
Native EVs |
(1) Good biocompatibility |
(1) Lack of active targeting capability |
94–98
|
(2) Physiological regulatory potential |
(2) Lack of on-demand drug delivery |
(3) Low immunogenicity |
(3) Lack of treatment feedback |
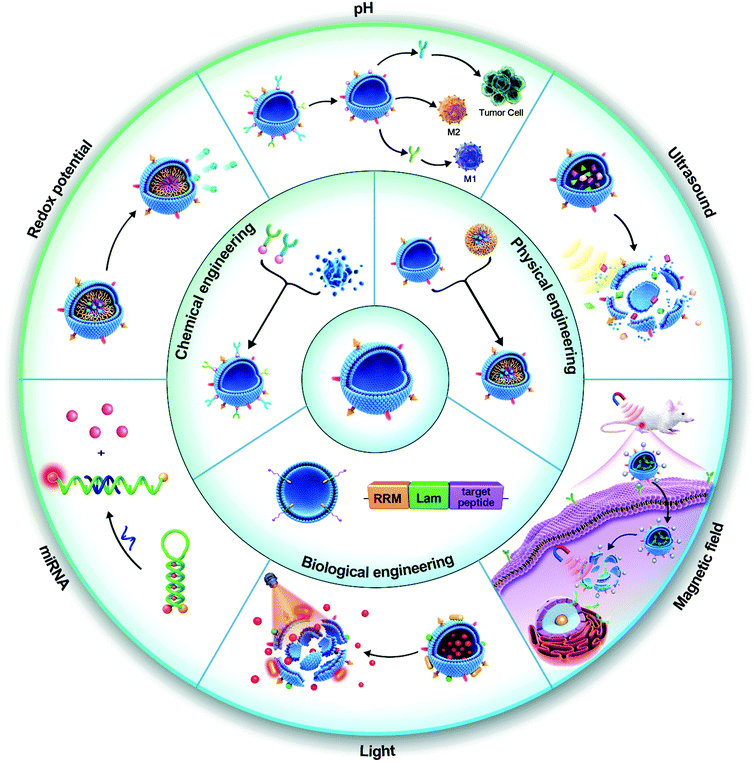 |
| Scheme 1 Construction and application strategies of intelligent EVs. EVs exhibit good physical and chemical properties and can be engineered through various strategies (biological engineering, chemical engineering, and physical engineering). EVs modified with intelligent modules can actively target lesions, respond to stimuli, and provide feedback on the course of treatment. Among them, the most critical is to respond to endogenous and exogenous stimuli (endogenous stimuli: pH, redox potential, biomarkers; exogenous stimuli: light, magnetic field, ultrasound, etc.), to achieve controllable precision treatment. | |
The origin, function, and preparation of EVs
EVs are a group of heterogeneous, membrane-bound vesicles that are secreted by cells. They mediate intercellular communication via the delivery of bioactive cargoes such as nucleic acids and proteins. Due to their biological origin, EVs have good histocompatibility and stability and have unique physiological regulatory functions. EVs have attracted extensive attention in the biomedical field since they were first reported.99 EV mimetics, which were inspired by EVs, are artificial vesicles constructed from cell or organelle membranes.100 EV mimetics are considered to be substitutes for EVs and have also attracted attention due to their similar structures and functions to EVs. In this section, the origin, function, preparation methods, and properties of EVs and their mimetics are briefly described. A more detailed overview of these topics can be found in published reviews.51,101–103
Origin and function of EVs
EVs are typically categorised into subsets by size: exosomes (30–150 nm), microvesicles (50–1000 nm), apoptotic bodies (800–5000 nm), and oncosomes (1–10 μm).49,104–106 In the field of nanomedicine, EVs in the range of 30–200 nm are most commonly used, and are within the scale ranges of exosomes and microvesicles. Given the methods to distinguish EVs within the 30–200 nm scale, exosomes and microvesicles are generally referred to as small EVs.107 EVs mentioned in this paper are mainly small EVs.
EVs are derived from various cell types and have distinct biological functions due to the active components inherited from the cells from which they originate.51 For example, EVs secreted by tumor cells carry PD-L1 and have immunosuppressive functions.108,109 In a recent study, Wang et al. found that the effect of anti-tumor immunotherapy can be improved by suppressing the secretion of exosomes from tumor cells.110 Although this highlights a potential risk in using tumor-derived EVs, other studies have shown that they have beneficial properties. It was reported that the expression of EpCAM in tumor cell-derived EVs can increase their homologous targeting.111 Jing et al. also confirmed that tumor-derived EVs have a higher tumor-targeting ability than adipose stem cell-secreted exosomes in a mouse colon cancer model.112 However, they did not find homologous targeting of tumor-derived EVs at the cellular level. Such studies have promoted the use of tumor-derived EVs in drug delivery. According to the function of original cells, tumor-derived EVs may also promote proliferation, inhibit differentiation and regulate inflammation. However, the function of tumor-derived EVs is not yet fully understood, and the feasibility of their clinical translation needs to be further evaluated.
Recent studies have revealed that EVs derived from immune cells have immune activity. For example, exosomes derived from CART cells were found to kill tumor cells.113 A detailed overview of the immune activity of immune cell-derived EVs can be obtained from other reviews.114,115 These immunoreactive EVs show great potential in the immunotherapy of diseases. Other cell-derived EVs also exhibit unique functions. For example, stem cell-derived EVs can promote tissue regeneration.116–118 In general, the function of EVs comes from the components, which can be divided into proteins, nucleic acids, active lipids, and other active components according to their chemical composition. These components endow EVs with properties such as homologous targeting, immune escape, and physiological regulation.119
Preparation of EVs
EVs can be prepared through a variety of processes, such as differential centrifugation, density gradient ultracentrifugation, immunocapture, ultrafiltration, polymer precipitation, size-exclusion chromatography, and membrane affinity chromatography.41,120–123 Selection of distinct subclasses of EVs can often involve a combination of these separation methods. Polymer precipitation and membrane affinity chromatography exploit the properties of EV outer membranes to isolate them. While these methods can concentrate EVs, they do not become enriched for EVs from distinct cellular origins or EVs of a defined particle size. Differential centrifugation, density gradient ultracentrifugation, and size-exclusion chromatography are used to separate EVs based on their physical properties such as density and particle size, albeit, with limited resolution. These methods poorly separate similarly sized EVs such as exosomes (30–150 nm), microvesicles (50–1000 nm), and lipoproteins (6–1000 nm). Moreover, these methods do not efficiently distinguish EVs derived from specific cell types. In contrast, immunoaffinity chromatography is a highly specific method for the isolation of different EV subgroups. However, it is not suitable for large-scale isolation because of its high cost. The principles, advantages, and limitations of commonly used isolation methods of EVs are summarized in Table 2. Taken together, isolation of subsets of EVs remains a technical challenge, which many teams are working towards overcoming.124 There are extensive reviews detailing the methodologies for EV isolation.41,125–127
Table 2 Comparison of different isolation strategies of EVs
Approach |
Principle |
Yield |
Advantages |
Limitations |
Ref. |
Differential centrifugation |
Density, size, and shape-based sequential spinning separation at low/high centrifugation speed |
Moderate |
Simple operation |
Potential shear damage to EVs |
125, 139 and 140
|
Suitable for large volume preparation |
Low portability |
No additional reagents |
Time-consuming |
|
Specific equipment requirement |
|
Low yield |
Density gradient ultracentrifugation |
Density-based separation under the discontinuous gradient of sucrose or iodixanol |
Moderate |
High purity |
Potential damage to exosomes |
141 and 142
|
Simple operation |
Low portability |
|
Time-consuming |
|
Sensitive to centrifugation time |
|
Low yield |
Immunocapture |
Specific interaction between antigens on the surfaces of EVs and fixed antibodies |
Low |
High purity and selectivity |
Non-specific binding |
60 and 143
|
Specific EV subpopulation isolation |
High cost for antibody input |
|
Low processing volume |
|
Exosome elution steps required |
Ultrafiltration |
Size-based separation by using a filter membrane with a defined molecular weight |
Low |
Simple operation |
Membrane clogging caused EV mass loss |
144 and 145
|
Portability |
Possible damage induced by shear stress |
Fast procedure |
Moderate purity |
Capable of operating with a low amount of sample |
|
Low equipment cost |
|
Size-exclusion chromatography |
Size-based separation |
High |
Relatively high purity |
Time-consuming |
146 and 147
|
Using a column filled with nanoporous beads |
Intact structure of EVs |
Low recovery |
|
Good reproducibility |
Relatively expensive and complex device |
|
|
Low sample volume |
Polymer precipitation methods |
Hydrophilic polymer based “fishing net” to interact with and wrap EVs |
High |
High throughput |
Low purity due to the precipitation of non-EV components |
148 and 149
|
Simple operation |
Polymer and contaminants hinder downstream protein biomarker discovery |
High recovery |
|
Microfluidics-based methods |
Microscale mixing or contact-based isolation using immunoaffinity, size, density, and viscoelasticity |
Low |
Fast, low cost, portable, easy automation, integration, and high portability |
Lack of standardization and large scale tests on clinical samples, lack of method validation, moderate to low sample capacity |
150 and 151
|
Preparation of EV mimetics
The low yield of EVs and the complexity of their purification have contributed to the increase in studies using EV mimetics. EV mimetics are prepared by a variety of methods, including self-assembly, liposome extrusion, and centrifugal extrusion.128–131 They have a similar structure to EVs, such that they have an outer membrane composed of a phospholipid bilayer and they contain components from the cell of their origin. Because they also inherit the active components from the original cells, EV mimetics are expected to perform physiological functions in a cell-free state. These properties make them of great interest in nanomaterial camouflage, tumor homologous targeting, and physiological regulation.132–134 Recent studies have compared the performance of EV mimetics and natural EVs in the treatment of brain glioma.135 EV mimetics can penetrate the blood–brain barrier (BBB) and significantly increase the half-life of drugs in the circulatory system. EV mimetics may therefore be effective substitutes for exosome-based nanomedicine for the treatment of brain tumors. Current methods to prepare EV mimetics take advantage of the dynamic self-assembly properties of phospholipid bilayers. Freeze–thawing, ultrasound, extrusion, filtration, and other methods have been utilised to break and reassemble phospholipid bilayers into nanovesicles. Despite this progress, there is not yet a standardised protocol for the preparation of EV mimetics. Moreover, there is not yet a clear system to identify and classify them. Current classifications of EV mimetics in the literature are based on cell origins and their preparation methods. This has also led to EV mimetics with a variety of names, such as cellular vesicles,136 exosome biomimetics,133 cell membrane nanoparticles,137 and nanovesicles.138 The preparation methods for EV mimetics will be discussed in this review.
Generic engineering strategies for EVs
Native EVs have limitations in their targeting, on-demand delivery, and treatment feedback. Engineered EVs are anticipated to overcome these issues and be a tool for targeted-treatments. For example, modification of the AS1411 aptamer can increase the leukemia cell targeting effect of EVs, leading to obvious cell apoptosis by resisting the function of miR-21 in leukemia cells.152 EV engineering methods can be classified into three categories: biological engineering, chemical engineering, and physical engineering.153 They can also be divided into genetic engineering, membrane modification, encapsulation, and hybridization according to the specific mode of operation (Fig. 1).
 |
| Fig. 1 Generic engineering strategies for EVs. Conventional modification strategies for EVs include membrane modification, encapsulation, and hybridization. Membrane modification is the placement of cargo on the surface of a vesicle utilizing chemical bonding or lipid chimerism. The encapsulation strategies are mainly through freeze–thawing, pH gradient, electroporation, streptolysin O, and ultrasound methods to introduce the drug into the vesicle. A hybrid strategy is the hybridization of different vesicles or vesicles with liposomes, resulting in heterozygotes. | |
Genetic engineering strategies
As derivatives of cells, EVs can incorporate cellular components during their formation. For example, substances from the cytoplasm can be wrapped into exosomes, and substances bound to the membrane are naturally released as cell bubbles. The mechanisms underlying the packaging of EVs can be manipulated by a range of cell engineering techniques to enhance the functionalization of EVs. Genetic engineering is an example of a powerful tool for modifying EVs.
Genetic engineering of EVs can be mediated by the delivery of gene expression vectors into donor cells and their subsequent expression and incorporation into EVs through the natural packaging process. Such engineered EVs can be isolated and purified from the supernatant of cell culture media. Although this method appears to be simple and feasible, many problems may arise. The first aspect to consider is cytotoxicity. The expression of cytotoxic proteins can inhibit the proliferation and even trigger apoptosis of donor cells. Secondly, cells tightly regulate which molecules are packaged into EVs. Donor cells may therefore package the desired expression products into EVs with a low loading efficiency. To achieve improved EV loading, the expressed proteins can be fused with constitutive proteins of EVs. For instance, Wood and colleagues achieved neuro-tissue-specific targeting by expressing exosomal membrane protein Lamp2b from engineered dendritic cells and fusing it with neuron-specific RVG peptides.154 They found that GAPDH siRNA was delivered by RVG-targeted exosomes to the brain network (e.g. neurons, microglia, and oligodendrocytes) after intravenous injection, resulting in tissue-specific knockdown of GAPDH. One other approach to genetically engineer EVs is to fuse nucleic acid sorting proteins with EV structural proteins to achieve specific loading of target sequences.155–157 This method eliminates the subsequent loading process and avoids nucleic acid degradation caused by excessive operation, extending the application prospect to RNA loading and delivery. In conclusion, genetic engineering provides a powerful tool for enhancing the functionality of EVs and enabling precise control of EVs for clinical applications.
Membrane modification strategies
Membrane modification is a common modification method for EVs. Benefiting from the fluidity of the vesicle membrane, fat-soluble components can be easily inserted into the membrane. Distearoyl phosphoethanolamine (DSPE), in particular, can be embedded in the phospholipid bilayer, thereby anchoring the attached components to the EV surface.80 In addition, sulfhydryl groups on the surfaces of EVs are also available for labeling sites. Fan et al. successfully anchored quantum dots to the surfaces of EVs through the addition reaction between maleimide and sulfhydryl groups.158 In recent years, the rise of glucose metabolism chemistry has also expanded the membrane modification strategies of EVs.159–161 The surfaces of EVs can be labeled with azide (N3) through glucose metabolism and substances linked to the outer vesicle membrane through ‘click’ chemistry.159–161 Membrane modification can effectively and nondestructively anchor cargo to the surface of the vesicle, which is ideal for target molecules and other components that need to be exposed to perform their functions. The desired goal for drug delivery is that the drug will work once it reaches the target site. Membrane modification may hinder this goal since it can lead to premature drug leakage, and the drug can have an effect earlier. At the same time, modification of some components with poor histocompatibility on the EV surface will inevitably reduce the overall histocompatibility. Therefore, whether the membrane modification method can be utilised to load the cargo needs to be assessed according to the nature of the cargo and its functioning mechanism.
Encapsulation strategies
EVs with a large internal space can be used as carriers of drugs and contrast agents.162,163 The approach used to wrap these agents into EVs can impact their function as carriers. The properties of the agents to be wrapped also have implications for the packaging methods that can be used (Table 3). Small molecules like paclitaxel can be introduced into EVs through incubation or ultrasound assistance.164 Macromolecules like DNA sequences need to enter EVs by electroporation.154
Table 3 Summary of the methods applied when loading cargo into EVs
Loading methods |
Cargo properties |
Advantages |
Disadvantages |
Efficiency |
Ref. |
Incubation |
Fat-soluble small molecules |
Convenient, small damage to vesicles |
Narrow application range |
PTX 8% |
165–167
|
Curcumin N/A |
Enzyme 4.9% |
Ultrasonic |
Small molecules |
Convenient and fast, relatively small damage |
Only for small molecules, low efficiency |
Erastian N/A |
167–169
|
Imperialine 24.9% |
Enzyme 26.1% |
Freeze–thawing |
Biomacromolecules |
Simple operation |
Destroys the vesicle structure |
Enzyme 14.7% |
167
|
Electroporation |
Biomacromolecules, such as nucleic acids |
High efficiency, less damage |
Higher requirements for conditions such as equipment and buffer |
BAY55-9837 N/A |
170
|
DNA 60% |
Streptolysin O |
Biomacromolecules |
Simple operation |
Costly and disruptive |
DNA N/A |
57
|
Extrusion |
Nanoparticles |
Encapsulates larger particles |
Tedious and time-consuming operation |
NPs N/A |
133 and 167
|
Enzyme 22.2% |
There are also reagents such as streptolysin O that change the structure of the vesicle membrane, allowing the entry of macromolecules.57 For larger nanoparticles, these methods are not effective. In recent years, by exploiting the self-assembly properties of the phospholipid bilayer, a lipid extrusion model has been proposed to effectively solve the coating problem of nanoparticles. However, each loading method has its advantages and limitations. Small membrane-penetrating molecules can enter the vesicle without causing damage, but they can spread out of EVs in the same way and do not provide a good barrier. Cargo that cannot be diffused into the vesicle needs to be introduced by changing the membrane permeability, which can damage the vesicle. The encapsulation method can isolate the cargo from the outside environment and can improve the histocompatibility and targeting of the cargo.
Hybridization strategies
Through genetic modification of parent cells, biomolecules, e.g. non-coding RNA, mRNA, water-soluble proteins, and cell-membrane proteins, can be introduced into EVs.171–173 The surfaces of EVs can also be decorated with proteins of interest using the same technique. However, the industrial application of this method is restricted by the complexity of the process of genetic engineering vesicle modification and the uncertainty of whether the expressed products can enter EVs. Hybridization is an effective and facile engineering strategy for EVs. Drug delivery and EV modification can also be achieved by fusing liposomes with EVs.174 Li et al. constructed exosome–liposome hybrid nanoparticles and implemented the CRISPR/Cas9 system for delivery to MSCs.175 This nanosystem is expected to be used for gene editing in vivo. Recently, Jiang et al. constructed biomimetic EV structures through the hybridization of red blood cell membranes with polymyxin B-modified lipids to facilitate the targeted removal of internal and external toxins from bacteria, providing a new concept for the engineering of EVs.176 On this basis, hybrid EVs with complex functions can be produced by fusing EVs from different sources. For example, hybridization of tumor-derived EVs with natural killer cell-derived EVs yielded hybrid vesicles with tumor homologous targeting and tumor-killing effects. In conclusion, hybridization is an effective EV engineering strategy, which avoids the vesicle damage caused by mechanical methods such as electroporation and extrusion with improved assembly efficiency.
Intelligent upgrade of EVs
To overcome the limitations of traditional nanomaterials, intelligent nanomaterials are being developed and have received more attention in recent years.177 Intelligent nanomaterials are integrated systems that can not only respond to external physical, chemical, or biological stimuli to release cargo, but also have the capabilities of active targeting and therapeutic feedback.178 As a rising star of nanomedicine, intelligent EVs are expected to open a new avenue for modern drug delivery.
Intelligent EVs driven by active targeting
EVs can improve the tissue compatibility and long circulation ability of cargo, and enhance the drug effect through physiological regulation. But for a drug to work, it must first be delivered to its target site. Most nano drugs can passively target neoplastic tissues through the enhanced permeability and retention (EPR) effect. This may also be applicable to EVs. In recent years, there has been increasing scepticism about the universality of the EPR effect,179 especially in human tumors, which have yet to be confirmed.180 Given that passive targeting of nano drugs is inefficient, further research into active targeting strategies is needed.
Nanoparticle surface modification with targeted molecules such as monoclonal antibodies, RGD peptides, folic acid, or aptamers which identify epitopes, ligands, and ECM components of recipient cells based on biological affinity can achieve active tumor targeting.181–183 Through engineering modification with a targeting module, EVs can be endowed with targeting capability.184 A classic approach for targeted modification is to express targeted peptides or donor and recipient proteins on the surfaces of EVs via genetic engineering. Wang and co-workers applied molecular cloning and lentivirus packaging techniques for engineering exosome membrane protein (Lamp2b) fused with ischemic myocardium-targeting peptide CSTSMLKAC (IMTP).185 They found that IMTP labeled exosomes could be more efficiently taken up in hypoxia-injured H9C2 cells than native exosomes. Targeting modification based on the lipid embedding method is a convenient and fast modification method. A recent study showed that AS1411-modified EV mimetics can increase the tumor-targeting efficacy of chemotherapy drugs.186 In another study, the authors used RGD and folic acid dual targeting to achieve precise photothermal therapy for tumors.187 In addition to conventional targeting modifications, the targeting properties of EVs themselves have also been extensively studied. EVs of tumor origin have a tumor homing effect and can accurately deliver drugs to tumor tissues. Qiao et al. reported that tumor exosomes have a homing effect on tumor tissue and can be seen as Trojan horses for delivering anti-cancer drugs.188 A recent study found that low pH treatments lead to increased uptake efficiency and retain cell-type specificity, promising intelligent drug delivery and potential personalized therapy for their homologous tumors.189 Although tumor-derived EVs show the potential for homologous targeting, the potential safety risks of tumor-derived EVs make them controversial for clinical translation. In addition, it is sometimes difficult for the inherent targeting ability of EVs to meet real-world application scenarios. The development of efficient and convenient targeted modification methods is badly needed and has been an emerging hotspot. Overall, active targeting is a significant achievement for intelligent EVs and offers great promise for drug delivery.
Intelligent EVs based on stimuli-response
Although EV-based delivery systems have shown promising results in preclinical trials, a pressing challenge remains, namely, the ability to achieve a precisely controlled release of cargos at the target site. Therefore, the inherent functions of EVs require to be further reshaped or endeavored to ensure that EV-based therapeutics are clinically acceptable. In this regard, integration of responsive modules into EVs for controllable release of drugs in the cellular microenvironment in response to internal (e.g. pH, redox environment, enzyme, and hypoxic) and/or external (e.g. light, thermal, ultrasound, and magnetic field) stimulation is required. As a highly material-dependent process, engineering EVs with responsive modules would endow EVs with intelligent properties, which has stepped into a rapid expansion era of therapeutics. The research progress of intelligent EVs in recent years will be systematically summarized based on the stimulus-response as the mainline.
Intelligent EVs with treatment monitoring functionality
A plethora of research into intelligent EVs has led to significant advances in their therapeutic applications. Importantly, intelligent EVs also have the potential to be utilised for diagnostic and prognostic purposes such as monitoring the effectiveness of treatments and disease progression. While some studies have explored the use of integrated feedback modules in EVs and bioanalysis of EVs to evaluate drug efficacy,190–193 this area of research is still underexplored. Engineering EVs capable of real-time feedback of treatments and disease progression would further extend the utility of intelligent EVs in biomedical applications, highlighting that this is a promising area of research.
Biomedical applications of intelligent EVs
There have been an increasing number of innovative approaches to improve the clinical application of EVs. In this section, we aim to classify intelligent EVs based on the type of stimulus they have been designed to be responsive to, and to summarize recent studies that have engineered such EVs. We focus our discussion on the preparation, intelligent upgrading, diagnosis and therapeutic effect of intelligent EVs, with specific examples (Table 4). Finally, we also briefly describe the progress of treatment feedback.
Table 4 An overview of stimuli-responsive nanomaterials based on exosomes for nanotheranostic application
Stimulus |
EV origin |
Design strategy |
Targeting |
Responsive mechanism |
Disease |
Theranostic performance |
Ref. |
pH (endogenous) |
MDA-MB-231 |
Assembles cargo proteins in pH-responsive ZIF-8 NPs camouflaged with an EV membrane |
Homotypic targeting |
Acid-mediated degradation of ZIF8 |
Breast cancer |
Significantly inhibits the tumor growth in vivo and increases 14-fold the therapeutic efficacy |
213
|
M1 macrophages |
Conjugation of azide-modified M1-exosomes with dibenzocyclooctyne-modified anti-CD47 and anti-SIRPa through benzoic–imine bonds |
Anti-CD47 targets the tumor cell surface |
Acid-cleavable benzoic–imine bond |
Breast cancer |
M1 Exo effectively re-educated pro-tumoral M2 to anti-tumoral M1, while aCD47 and aSIRPa remarkably enhanced the phagocytosis of macrophages by blocking the “don’t eat me” signaling |
43
|
ROS (endogenous) |
MDA-MB-231 |
Incorporation of ROS-responsive thioether-linked paclitaxel–linoleic acid and cucurbitacin B into polymeric micelles, followed by decoration with an exosome membrane (EM) |
Homotypic targeting |
Thioether-linked paclitaxel–linoleic acid |
Breast cancer |
EMPCs amplify prodrug bioactivation, prolong blood circulation, target homotypic tumor cells, enhance tumor penetration and suppress tumor metastasis through CTC clearance and FAK/MMP signaling pathway regulation |
215
|
miRNA (endogenous) |
M1 macrophages |
M1 nanovesicles loaded with functional DNA for the controlled release of miRNA-specific drugs |
Tumor homing effect |
Complementary base pairing |
Breast cancer |
Realize microRNA-responsive delivery systems for visual therapy of tumors, and have biological treatment and chemotherapy functions |
158
|
Thermal (exogenous) |
Fibroblasts |
Engineered exosome-thermosensitive liposome hybrid NPs as gETLs with the loading of GM-CSF and/or docetaxel (DTX) NPs |
EPR effect |
Thermosensitive liposomes |
Metastatic peritoneal cancer |
gETL NPs inhibit tumor development and the efficacy is enhanced about 39% with co-administration of HIPEC agents |
268
|
Photo (exogenous) |
Macrophages |
Biological and synthetic hybrid designer exosomes with photoresponsive functionalities using a donor cell-assisted membrane modification strategy |
Dual ligand-mediated target (folic acid and RGD) |
Gold nanorods achieve photothermal conversion |
HeLa |
The duration for inhibiting tumor relapse in a programmable manner increased to 30 days compared to a single target group (15 days) |
187
|
Magnetic (exogenous) |
Blood |
BAY55-9837-loaded exosomes coupled with SPIONs via electroporation |
External magnetic force (MF) |
Magnetic field interaction |
Type 2 diabetes mellitus |
Enhances pancreas islet targeting and increases insulin secretion, leading to the alleviation of hyperglycemia |
170
|
Ultrasound (exogenous) |
4T1 |
Loading DVDMS onto homotypic tumor cell-derived exosomes via mild incubation |
Homotypic targeting |
Ultrasound/sinoporphyrin sodium |
Breast cancer |
High stability and specificity boost ROS generation and facilitate simultaneous imaging and tumor metastasis inhibition |
329
|
Ultrasound (exogenous) |
Macrophages |
EV membrane bubbles wrapped with a sonosensitizer |
Tumor homing effect |
Ultrasound/Au-BMSNs |
Breast cancer |
Tumor homing and RES evasion abilities to induce cell apoptosis and mitochondrial dysfunction effectively against tumor rechallenge and prevent lung metastasis |
133
|
pH and light (endogenous and exogenous) |
Milk |
Conjugation of milk-exosomes to Dox with a pH-cleavable bond, and endoperoxides and chlorin e6 (Ce6) were also loaded |
EPR effect |
Imine bond and Ce6 |
Oral cancer |
The tumor volume was significantly inhibited to 0.05 cm3 compared to that when the drug alone was used (1.26 cm3) in a mouse OSCC model |
330
|
pH and thermal (endogenous and exogenous) |
AMSCs |
Synthesis of an FHE hydrogel through reversible Schiff base reaction between OHA and EPL, and the thermally-responsive properties of F127 |
None |
Imine bond and thermal hydrogel |
Chronic diabetic wound healing |
Fast self-healing process, shear-thinning injectable ability, efficient antibacterial activity, and long-term pH-responsive bioactive exosome release behavior |
334
|
ROS and light (endogenous and exogenous) |
Erythrocytes |
Exosome-like nanozyme vesicles for in vivo H2O2-responsive PAI of nasopharyngeal carcinoma |
Folate acid |
Graphene quantum dot nanozyme (GQDzyme) effectively converts the ABTS into its oxidized form in the presence of H2O2 |
Tumor |
Excellent biocompatibility and stealth ability |
335
|
Magnetic and pH (endogenous and exogenous) |
Human serum |
Immunosorbent by SPIO-labeled antibodies |
Magnetic |
Magnetic nanoparticles and imine bonds |
Myocardial infarction |
No purification, self-supply, accurate administration, improved left-ventricle ejection fraction, and angiogenesis |
65
|
Endogenous stimuli-responsive EVs
Diseases and disorders in the human body are typically accompanied by distinct patho-physiological changes that can be used to distinguish lesions from healthy tissue. For example, tumor tissue differs from healthy tissue in pH, reduction/oxidation (redox) status, metabolic status, and gene expression status. Such differences have inspired the development of therapeutic strategies for endogenous stimuli-response.194–198 Other diseases such as cardiovascular, inflammatory, neurodegenerative, and infectious diseases are also accompanied by changes in various physiological parameters, providing the possibility of endogenous stimulus–response therapy.199–201
pH stimuli-responsive EVs.
Most tumor cells have reprogrammed their glucose metabolism to enable their rapid proliferation.202,203 This metabolic reprogramming, known as aerobic glycolysis or the Warburg effect, triggers excessive accumulation of lactic acid.204 The pH value (6.5–6.8) of the tumor microenvironment typically changes, and is notably different from that of the normal physiological environment.205,206 In the past few decades, pH stimulation has been considered to be an effective strategy for targeted drug release, and a series of materials such as CaCO3 NPs, ZIF-8, and benzoic–imine bonds conjugated to polymers have been successfully created.207–209
For instance, Li et al. used the process of mineralization to generate doxorubicin (DOX)-loaded calcium carbonate-crosslinked polypeptide nanoparticles.210 The principle behind this technology is founded on the dissolution of minerals when they are exposed to low pH, leading to drug release at a specific tumor site. An interesting study reported that ≥70% and ≤24% of DOX are released from calcium-mineralized polypeptide nanoparticles within 18 h at pH 5.5 and pH 7.4, respectively. Yang et al.211 constructed tumor pH-responsive dissociable albumin–tamoxifen nanocomplexes to achieve effective tumor penetration and hypoxia relief for enhanced cancer photodynamic therapy. In general, most of these designs aim to control drug release through changes in the chemical bonds triggered by pH.
To further optimize the performance of pH-sensitive precursor drugs, EVs were selected as carriers to improve histocompatibility and tumor targeting. Kim et al.212 successfully achieved pH-responsive drug release using I-motif-coated exosomes as anticancer drug carriers. The pH-responsive molecular machinery relies on the intercalated motif (I-motif), a cytosine-rich DNA strand. This study achieved acid-responsive drug release with a 3 h release efficiency of 21%, which provides a new idea for the construction of a pH-responsive anticancer DDV using engineered exosomes. In another study, Cheng et al. constructed self-assembled exosome-like metal–organic framework (MOF) ZIF-8 nanocarriers to protect and deliver pre-loaded biofunctional proteins into target cells (Fig. 2A).213 As a biodegradable material, a MOF is responsive to a variety of biological stimuli and can protect active components such as proteins through biomineralization.214 In this study,213 the self-assembly of exosome-like MOF nanocarriers has an extremely high loading and modification efficiency (>94%). ZIF-8 nanoparticles have good acid response performance and can efficiently release proteins at pH 5 (Fig. 2C). At the same time, the encapsulated material reduced tissue toxicity and achieved protein protection and intracellular release. More importantly, the retained surface proteins (Fig. 2B) can evade immune clearance to increase the long circulation in the blood and achieve homologous targeting (Fig. 2D). Finally, a good anti-tumor effect was achieved in an animal model (Fig. 2E). This study further enriched stimuli-responsive EV materials. Surprisingly, not only traditional chemotherapeutic drugs but also immunotherapeutic drugs were used for pH-sensitive exosome delivery systems. Nie et al.43 successfully constructed a tumor microenvironmental responsive immunotherapy platform by coupling immunotherapy antibodies (anti-CD47 and anti-SIRPα) to the surfaces of M1-type exosomes via pH-sensitive benzoic–imine bonds (Fig. 2F and H). The conjugated antibodies were successfully released under the condition of low pH in the tumor microenvironment (Fig. 2G and I), blocking the tumor-mediated immunosuppression (Fig. 2J). Then, M1 exosome-mediated immune reprogramming induced strong anti-tumor immunity. Finally, the tumor volume was significantly inhibited, and all mice in the experimental group survived at the end of the experiment (the control group mice died by 24 days). Although the surface modification cannot avoid the early exposure to the antibody drug, this unconventional design is worth learning. This study opens up an idea of endogenous stimuli-responsive immunotherapy based on EVs. In general, EVs improve cargo enrichment in the lesion or increase their long circulation ability, increasing the probability for the stimuli-responsive module exposed to the acidic microenvironment.
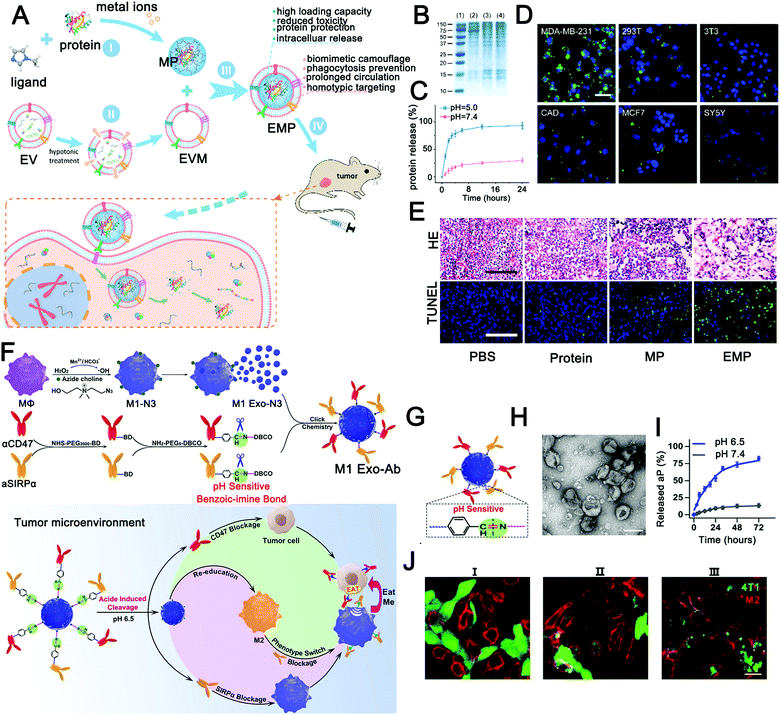 |
| Fig. 2 Engineered extracellular vesicles for pH-responsive delivery of drugs. (A) Schematic diagram of homologous targeting guided protein intracellular delivery with pH-responsive biomimetic EMP NPs. (B–E) Cell and tissue level experiments verified the homologous targeting, pH response, and antitumor effect of biomimetic EMP nanoparticles. (Reprinted with permission from ref. 213, Copyright 2018 American Chemical Society.) (F and G) Schematic of the construction of pH-responsive immunotherapeutic exosomes (M1 Exo-Ab) and the synergistic anticancer effect of M1 Exo-Ab. (H–J) The morphology, pH response, and antitumor effect of M1 Exo-Ab were verified in vitro. (Reprinted with permission from ref. 43, Copyright 2020 Wiley-VCH Verlag GmbH & Co. KGaA, Weinheim.) | |
Redox-responsive EVs.
Oxidative stress is highly related to the occurrence, evolution, and progression of many diseases and can be used as a pathological parameter of diseases. A large number of studies have shown that neoplastic transformation is linked with the increased basal level of oxidant stress.216–218 The redox potential in tumor tissue is elevated by 100- to 1000-fold that in normal tissue.219–222 To avoid the harm caused by reactive oxygen species (ROS), tumor cells can express excess glutathione (GSH), an important antioxidant to inhibit ROS in their cellular components.223–225 It is reported that the concentration of reducing agents such as GSH is two times higher in the cytosol and nucleus of a cell than in the intercellular and extracellular fluids.226 Accordingly, more attention has been drawn to the development of DDVs based on ROS or GSH-responsive assemblies.227 Both ROS and GSH can destroy disulfide bonds in nanoparticles by redox-mediated means. Recent studies have found that nanomaterials such as MOF, black phosphorus quantum dots, and nano-selenium themselves can respond to GSH and achieve conditionally triggered drug release through reduction-mediated degradation.228–230 However, these materials also degrade easily in the blood environment. To avoid premature degradation and missed targets, many studies have armed these materials by wrapping them in a certain shell structure.231,232 EVs are an advantageous membrane–shell structure that can protect cargoes as a barrier against the environment. In a recent study, Wang et al. prepared an exosome-biomimetic sequential-activating prodrug nanosystem (EMPCs) for self-strengthened ROS-triggered prodrug activation (Fig. 3A).215 The ROS-sensitive thioether-linked paclitaxel-linoleic acid prodrug (PTX-S-LA)/cucurbitacin B (CuB) co-loaded polymeric micelles were decorated with an exosome membrane (EM) by squeezing, which assisted EMPCs in avoiding macrophage phagocytosis as well as targeting both circulating tumor cells (CTCs) and primary tumors through the homologous targeting effect of tumor exosomes (Fig. 3D and E). In such a system, the thioether bond of PTX-S-LA could be oxidized to sulphone or sulfoxide groups under oxidative stress conditions, followed by rapid prodrug hydrolysis for PTX release (Fig. 3B and C). However, the effective and rapid release of the parent drug was hindered by inadequate intrinsic ROS levels in heterogeneous tumor cells. This nanoplatform can first release CuB after intercellular uptake to induce ROS cascade amplification and facilitate prodrug bioactivation for boosting PTX chemotherapy. Compared to the control groups, the EMPCs showed a faster release of PTX at the tumor site and potentiated the antitumor activity. Furthermore, the exosome-biomimetic strategy and CuB-mediated FAK/MMP signaling pathway regulation endowed EMPCs with enhanced cancer metastasis inhibition, prolonged blood circulation, superior tumor accumulation, and deeper tumor penetration. This study provides a new paradigm for prodrug development and CTC targeting therapy for cancer. In addition to tumors, redox stress is associated with the development of many diseases, such as acute and chronic inflammation, aging, and metabolic diseases. This undoubtedly broadens the scope of redox responsive therapy.
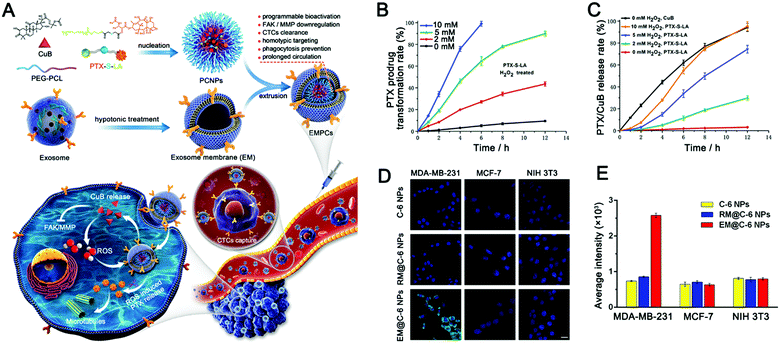 |
| Fig. 3 Exosome-based ROS-responsive paclitaxel prodrug nanoplatforms for enhanced cancer therapy. (A) Schematic diagram of EV-like multilevel activation of paclitaxel prodrug nanoplatforms with redox-responsive chemotherapy. (B) In vitro conversion of the PTX-S-LA prodrug into free PTX in the presence of H2O2. (C) In vitro release of drugs (PTX and CuB) into EMPCs by different concentrations of H2O2. (D) Confocal laser images and (E) flow cytometry analysis were used to analyze the internalization of nanoparticles in tumor cells (MDA-MB-231 and MCF-7) and normal tissue cell (NIH 3T3) incubated with different nanoparticles (C-6 NPs, RM@C-6 NPs, and EM@C-6 NPs). (Reprinted with permission from ref. 215, Copyright 2020 Elsevier.) | |
Enzyme stimuli-responsive EVs.
The tumor microenvironment (TME) is the direct environment for the growth of tumor cells and has been described as the “soil” that supports tumor growth, proliferation, invasion, and metastasis, and helps tumor cells evade immune surveillance.233–236 Significantly, tumor cells grow faster than normal cells and are more likely to invade the surrounding tissues, leading to upregulated levels of enzymes such as matrix metalloproteinases (MMPs), phospholipases, and oxidoreductases in the TME.237–240 Due to the specificity of enzymes to their substrates, a promising anti-tumor strategy is to design enzyme-triggered stimuli-responsive nanomaterials, which can more accurately identify tumor tissue, allowing more drugs to be concentrated at the primary site of the tumor, improving treatment effectiveness and reducing systemic toxicity.241–245
Although the effect of the enzyme stimuli-responsive system in tumor therapy has been demonstrated for several years,247 little attention has been paid to the construction of EV-based enzyme-responsive nanomaterials. Recently, enzyme-responsive nano-platforms with liposomes as carriers were reported. Mallik and colleagues reviewed recent advances in enzyme-responsive liposome nanovesicles for antitumor drug delivery.248 Specifically, they grouped the current bionic vesicles that respond to enzymes into three categories: enzyme-induced depletion of a shielding polymer from the interface, enzyme-induced structural perturbation within the lipid bilayer, and enzyme-induced cleavage of a lipopeptide triggered impairment of the liposome. These methods provide a reference for the design of enzyme-responsive systems based on EVs. In addition to this self-responsive mechanism of EVs, novel EV-loaded hydrogels are also developed in a stimulus-responsive direction. Zhou et al. reported an injectable EV-releasing self-assembled peptide KMP2 nanofiber hydrogel with MMP2-responsive capability by inserting an MMP2-cleavable motif (Fig. 4) as an improved cell-free therapeutic for tissue regeneration.246 Compared with repair peptide KMP2 or EVs alone, KMP2 nanofiber hydrogels exhibited better efficacy in expediting endothelial cell proliferation and angiogenesis and alleviated chronic renal fibrosis in ischemia–reperfusion (I/R) mice. Different from the traditional modification strategy based on EVs, in this study, EVs were used as delivery cargoes to construct enzyme responsive hydrogel skeletons. This makes full use of the physiological regulatory function of EVs and enables intelligent delivery of EVs. In the design stage, it is very important to distinguish the spatiotemporal relationship of enzyme action for designing effective enzyme responsive EVs. For extracellular enzymes, such as matrix metalloproteinases (MMPs) and granular enzymes, response modules should be modified outside the EV. In contrast, for intracellular enzymes, the response modules need to to enter the cell to function. Taken together, enzyme-responsive EV-based materials have many advantages, such as high specificity and concentration-dependent response intensity. This provides wide application prospects for molecular diagnosis and precise treatment.
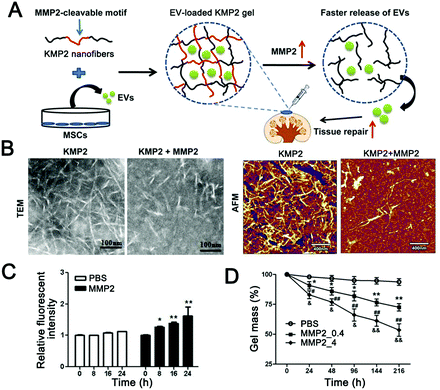 |
| Fig. 4 MMP2-responsive hydrogel for controlled release of extracellular vesicles. (A) Schematic diagram of the construction of an MMP2-sensitive KMP2 hydrogel to enhance tissue repair by delivering MSC-EVs. (B) Transmission electron microscopy and atomic force microscopy were used to observe the morphology of KMP2 nanofibers before and after MMP2 mediated degradation. Scale bar 100 nm in TEM; scale bar 400 nm in AFM. (C) The fluorescence yield of fluorescein reaction with the resulting free N-terminal was used to determine the enzymatic degradation of KMP2 in vitro with or without MMP2. (D) The gel mass loss was used to measure the degradation of the KMP2 hydrogel. n = 3 per group. *p < 0.05, **p < 0.01, PBS group vs. MMP2_0.4 group; #p < 0.05, ##p < 0.01, PBS group vs. MMP2_4 group; &p < 0.05, &&p < 0.01 MMP2_0.4 group vs. MMP2_4 group. (Reprinted with permission from ref. 246, Copyright 2019 Elsevier Ltd.) | |
Nucleic acid stimuli-responsive EVs.
Nucleic acid stimulus-response is a process of specific recognition and conformational change of nucleic acid through base complementary pairing. Compared with normal cells, most tumor cells have differences in terms of nucleic acid structure or expression profile.249–252 Among them, RNA is the most widely studied. In recent years, researchers have developed a large number of RNA-responsive theranostic systems to achieve the integration of tumor diagnosis and treatment.253–257 For instance, Lee and colleagues designed a miR21-responsive drug release system by using a chlorin e6 labeled peptide nucleic acid complex with a complementary sequence to miR21.258 This system was successfully applied for fluorescence imaging and photodynamic therapy (PDT) in vivo, in which strict regulation of the activation of a photosensitizer using oncogenic miRNA as an internal stimulus to minimize the potential risks of traditional PDT was demonstrated. This work paved a new approach for the application of PDT in cancer therapy. However, the metabolic risks of GO nanomaterials have been continuously assessed, which has greatly influenced the clinical transformation prospects of these studies.
EVs have been used to deliver miRNA responsive probes to overcome their poor histocompatibility with traditional nano-carriers. For example, Fan et al. used M1-type macrophage-derived EVs to deliver miR-21-responsive intelligent diagnostic probes, realizing in situ detection of miRNA and chemotherapy induced by target miRNA (Fig. 5).158 In this study, the researchers constructed EV mimetics by extruding M1 macrophages from nanofilters with an aperture of 100 nm to improve the production efficiency of nanocarriers (Fig. 5A and B). Notably, M1 EV mimetics showed anti-tumor activity, which was different from the anti-tumor effect of M1-type EVs reported in other studies. Specifically, in previous studies, M1 macrophage-derived EVs or their mimetics were able to kill tumors by inducing the polarization of tumor-associated macrophages. Fan et al. found that M1 EV mimetics have direct tumor cytotoxicity and can induce tumor cell apoptosis. The authors also demonstrated that M1 EV mimetics can be enriched in tumor tissue through the EPR effect with a long circulation of over 48 hours (Fig. 5C). Finally, an effective anti-tumor effect was achieved (Fig. 5D–F).
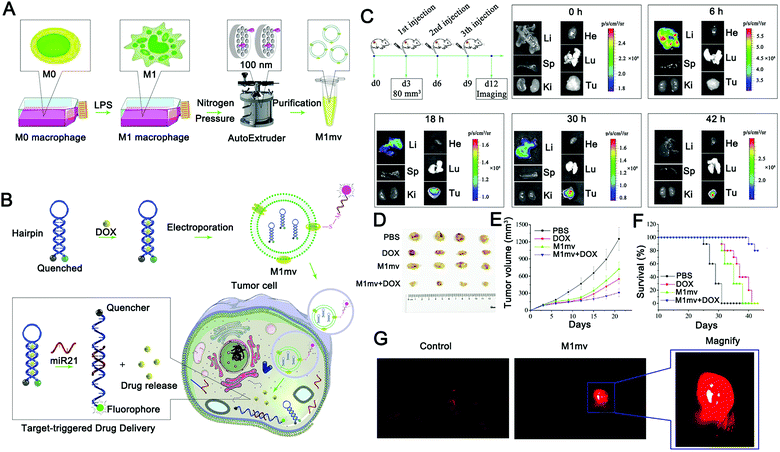 |
| Fig. 5 Principle and verification of a target-triggered visible drug delivery system. (A and B) Schematic diagram of a miRNA-triggered drug delivery system based on M1mv-DNA-QDs. M1-type macrophages were induced and nanovesicles with uniform particle size were prepared. Before being introduced into M1mv, doxorubicin was assembled into the complementary part of the hairpin probe. MiR21 triggers drug release and imaging by opening hairpins when M1mv enters tumor cells via macropinocytosis. (C) Tumor tissue targeting was verified by fluorescence enrichment in organs. (D–F) Effects of M1mv on the tumor volume, body weight, and survival curve of mice. (G) The visualized drug release process was monitored by near-infrared imaging. (Reprinted with permission from ref. 158, Copyright 2019 Wiley-VCH Verlag GmbH & Co. KGaA, Weinheim.) | |
In the absence of miR-21, the probe forms a stem-ring structure, and the fluorescent group and the quenching group realize fluorescence signal closure using fluorescence resonance energy transfer (FRET). When miR21 is present, miR21 competitively binds to the stem-ring and leads to the destruction of the structure, and the fluorophore is separated from the quenching group to enable the fluorescence signal to be switched on. During this process, doxorubicin is released in combination with the complementary region of the probe to achieve chemotherapy for the tumor. This design realizes the real-time monitoring of target miRNA and target miRNA-triggered drug release, and this release process is accompanied by the activation of the fluorescence signal, which can well monitor the drug release process and has excellent research significance (Fig. 5G). However, the diagnosis method based on a single miRNA has great uncertainty, especially for diseases with complex etiology such as a tumor. To this end, it might be worth exploring a multi-miRNA marker collaborative diagnosis and treatment strategy, which can further expand the application prospect of nucleic acid-responsive EVs.
Exogenous stimuli-responsive EVs
Endogenous stimulation differs between normal and pathological tissues, but crosstalk exists in different lesions. For example, tumors have high levels of redox compared to normal tissue, but so do inflammatory sites. It is easy to miss the target of treatment. The exogenous stimuli-responsive system has the potential to overcome the differences between patients.259 Exogenous physical stimuli, such as temperature, light, magnetic fields, and ultrasound, can directly act on the tissues of interest according to the requirements of the operator, triggering the release of drugs, to achieve targeted precise treatment.260–262 The shared merit of most external stimuli-responsive systems is that the drug could be controllably released at the target site with minimum off-target effects on adjacent healthy tissues.263 But, external stimulation cannot distinguish between normal tissue and diseased tissue. In practice, excessive treatment will increase the risk of treatment, while it will be difficult to eradicate the lesion with too mild treatment. To address this issue, the therapeutic agents themselves need to have good histocompatibility and targeting. EVs have good histocompatibility and in vivo stability and achieve specific targets by themselves or through engineering modification.113,264,265 For example, tumor cell-derived EVs have a tumor homing effect and can actively target tumor cells.188,266 There have also been improvements in targeting by preventing EVs from clearance by the immune system. Genetically engineered cell-derived exosomes can overexpress CD47 surface proteins, suppress immune clearance, and increase long circulation.267 At present, a large number of studies have integrated external stimuli-responsive performance into EVs and achieved good results in diagnosis and treatment. We will review recent advances in EV-inspired external stimuli-responsive materials.
Thermostimuli-responsive EVs.
Temperature regulation plays a notable role in the homeostasis of the body.269 Studies have found that the intervention of external stimulation in the regulation of body temperature can change the physiological process of the body and even realize the treatment of diseases.119,270–272 Local hyperthermia has become a powerful tool for treating solid tumors.273–277 During treatment, tumor tissue is heated to over 50 °C, which initiates a strong cytotoxic effect and causes tumor cell necrosis. Nevertheless, the disadvantage of this therapy is that the heating is not uniform, resulting in incomplete tumor destruction, an inability to target deep tumors, and scattered metastasis. The combination of hyperthermia and thermo-responsive materials can improve efficacy by increasing tumor targeting and heat-triggered drug release. Over the past few years, various thermosensitive materials have been developed by 1,2-dipalmitoyl-sn-glycero-3-phosphocholine (DPPC) mediated temperature-based phase transition, such as liposomes, polymersomes, hybrid vesicles, and pillararenes.278–283
EVs have lipid bilayer structures similar to liposomes, which can be easily modified with DPPC.284,285 However, EV-based thermally responsive materials have not received enough attention. This may be due to the functional requirements of EVs in use. In general, EVs need to not only act as nanocarriers but also retain their biological activity.171,286,287 The temperature required for a conventional thermal response may inactivate some active ingredients, such as proteins. Thus, the cost-effectiveness of EVs in adding thermal responsive performance needs to be considered. Conversely, some exosomes are functionally dependent on nucleic acid components,288–290 which are chemically stable and do not become inactivated by thermal stimulation at low temperatures. In such a case, adding a thermal response to exosomes is a good choice. With the deepening of mechanistic research, the functional group of exosomes will be more explicit, which will provide better guidance for the construction of thermally-responsive exosome carriers.
Recently, a thermosensitive exosome–liposome hybrid nanosystem was reported to improve drug delivery in metastatic peritoneal cancer and enhance the efficacy of hyperthermic intraperitoneal chemotherapy (HIPEC).268 In this study, the drug delivery efficiencies of thermosensitive exosome–liposome hybrid nanoparticles and HIPEC were compared, and it was found that exosome hybrid nanoparticles could enhance drug delivery efficiency and release payloads under thermal stimulation (Fig. 6A and B). Finally, the release of colony-stimulating factor (GM-CSF) and docetaxel was shown to be effective in inhibiting tumor development (Fig. 6C and D). This study enriches the exosome-inspired stimuli-responsive system and offers a promising approach to improving the treatment of metastatic peritoneal carcinoma.
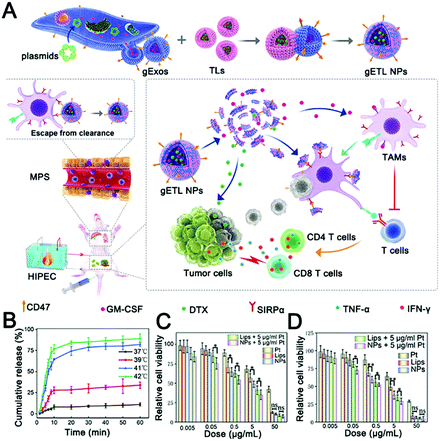 |
| Fig. 6 Thermally responsive hybrid EVs for tumor immunotherapy. (A) Schematic representation of the fabrication and application of thermally responsive hybrid EVs (gETL NPs) for mPC treatment. (B) Cumulative drug release curves of thermally responsive hybrid EVs at the indicated temperatures. (C) The cytotoxicity of gETL NPs at 37 °C on CT26 cells was determined by cell viability assay. (D) The cytotoxicity of gETL NPs at 42 °C on CT26 cells was determined by cell viability assay. *p < 0.05, **p < 0.01, ns: not significant. (Reprinted with permission from ref. 268, Copyright 2020 The Authors.) | |
Photo/light-responsive EVs.
Among all kinds of stimuli, light, as a clean spatial stimulus, has shown unique advantages in realizing local diagnosis,291–294 drug release, and treatment by adjusting various parameters such as the wavelength, polarity, irradiation intensity, and duration of light.295–299 Photo-responsive materials can change their stability or structure in response to specific light sources (such as ultraviolet, visible, and near-infrared) to release cargo at the target site.300–302 Typically, ultraviolet light is destructive to a variety of chemical bonds and is often used for the photolysis of chemical groups.261 For example, coumarin and nitrobenzyl are commonly used as photo-cleavable linkers in response to ultraviolet light. In addition, spiropyran, azobenzene, and azopyrazole undergo photoisomerization under ultraviolet light. This provides the basis for the construction of photo-responsive DDVs. Compared with the very weak tissue penetration using visible and ultraviolet light, which are not appropriate for in vivo therapeutics, near-infrared (NIR) light offers better penetration with negligible tissue damage.303–305 Therefore, the NIR-responsive system is very promising to realize controllable drug release. Currently, there are three different mechanisms of drug release, i.e., photothermal effect, two-photon absorption (TPA), and up-conversion effect. For example, the photothermal effect uses photothermal reagents such as gold nanoparticles and indocyanine green (ICG) to convert light energy into heat energy, so that the liquid can be vaporized and expanded to destroy the carrier structure to achieve controlled drug release. The two-photon effect uses the vibration of a molecule's chemical bond to “resonate” with an infrared laser at the same frequency, activating and eventually breaking the bond to achieve the process of cutting a particular molecule.306 Moreover, the upconversion effect can transform infrared light into short-wavelength visible and even ultraviolet light, and use the cutting effect of ultraviolet light to drive drug release.307 These mechanisms have been widely used in the field of photostimulus response diagnosis and treatment, and have been summarized in relevant reviews.301,308–311 Here, we only criticize the recent progress of EV-based light-responsive materials, aiming to provide a reference for the follow-up research.
Recently, Wang et al. described hybrid biological and synthetic designer exosomes with photo-responsive functionalities using a donor cell-assisted membrane modification methodology (Fig. 7A).187 The engineered exosomes can effectively target and accumulate at tumor sites through folic acid and RGD peptide dual ligand-mediated endocytosis. Then, combined with gold nanorods, local hyperthermia was induced under NIR irradiation, affecting the permeability of the exosome membrane and enhancing drug release from the exosomes, leading to inhibition of tumor recurrence in a programmable mode (Fig. 7B). The hybrid designer exosomes incorporate the advantages of both artificial materials and natural EVs, and not only retain the inherent functions of natural exosomes but also achieve multiple capabilities of highly effective tumor targeting, controllable release, and thermal therapy (Fig. 7C–F). However, this study also has some limitations. First of all, considering the way exosomes are produced, it is controversial whether membrane modification can be effectively integrated into exosomes. Secondly, the use of gold nanorods will undoubtedly increase the metabolic risk, especially the strategy of anchoring gold nanorods to the exosome surface will greatly reduce the histocompatibility of exosomes. For a load of inorganic and even other macromolecules, encapsulation should be adopted to enhance the histocompatibility of exosomes. Overall, this study pioneered the use of photothermal effects as a cargo release strategy, greatly expanding the application prospect of photostimulus-responsive EVs.
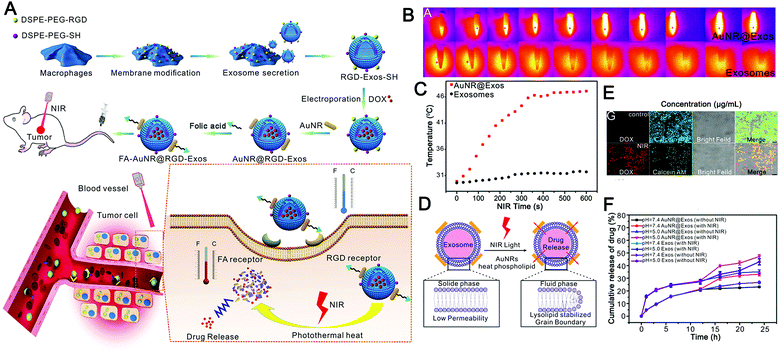 |
| Fig. 7 Engineered exosomes for photo-responsive chemo-photothermal synergistic tumor therapy. (A) Graphical summary of the design of dual-targeted photo-responsive EVs (FA-AuNR@RGD-DOX-Exos) and their chemo-photothermal synergistic antitumor effect. (B) Thermal imaging monitors the photothermal effects of AuNR@Exos with an aqueous dispersion (1.0 mg mL−1) under NIR laser irradiation (808 nm, 1.0 W cm−2). (C) Analysis of the heating curve using the photothermal spectrum of AuNR@Exos (1.0 mg mL−1) under NIR laser irradiation (808 nm, 1.0 W cm−2). (D) The cartoon shows the drug release process of AuNR@Exos induced by NIR laser irradiation. (E) Fluorescence images were used to detect the drug release in HeLa cancer cells treated by incubation with AuNR@DOX-Exos under NIR laser irradiation. (F) In vitro drug release profiles of AuNR@DOX-Exos and native exosomes at different pH values under NIR irradiation. (Reprinted with permission from ref. 187, Copyright 2018 Wiley-VCH Verlag GmbH & Co. KGaA, Weinheim.) | |
Magnetic field-responsive EVs.
Magnetic materials have been widely used in tumor imaging diagnosis, magnetic hyperthermia, magnetic separation, instant detection, cerebral nerve stimulation, controlled drug administration, and other biomedical fields.312–317 Magnetic nanoparticles (MNPs) have the advantages of good biocompatibility, degradability, easy modification, functionalization, etc.318,319 Their applications in the biomedical field are developing rapidly, and they have become one of the hot spots in the nanomedicine field.320,321 In particular, the unique magnetic properties of MNPs can mediate the physical effects of external magnetic fields and produce biological targets. More importantly, MNPs are expected to become potential DDVs with an intellectual response. MNPs can be used as a transducer to transform hysteresis loss and Néel relaxation into thermal energy by using an alternating current magnetic field.322,323 Therefore, MNPs can endow materials with magnetic field-induced thermal effects and magnetic field-guided targeting capability. Like other inorganic materials, the histocompatibility of magnetic nanoparticles has been questioned, greatly limiting their application in vivo.
The combination of magnetic materials and EVs shows good application prospects. Molecular targeting depends on the direct contact between the ligand and the receptor, which requires the ligand to reach the location of the receptor to achieve targeting, which makes the targeting effect greatly reduced. Magnetic material-mediated targeting is expected to solve this problem. In addition, the high tissue penetration and good controllability of the magnetic field provide the possibility for the development of magnetically-responsive exosomal materials. Recently, magnetically-responsive materials based on EVs have been reported. Zhuang et al. demonstrated a method for preparing exosomes loaded with BAY55-9837, a strong and high selective VPAC2 agonist, that binds to SPIONs with an islet targeting ability and enhances response towards blood glucose with the assistance of an external magnetic field (Fig. 8A).170 The authors verified the magnetic responsiveness of BAY55-9837 (Fig. 8B) and characterized BAY55-9837 by transmission electron microscopy and Fourier infrared spectroscopy (Fig. 8C and D). The half-life of BAY55-9837 with the exosome shell in plasma was significantly longer (27-fold) than that of bare BAY55-9837. At the same time, the targeting capability of SIPONs permits the BAY-exosomes to exhibit active targeting behavior, improving the blood GLC-responsive capacity with the aid of an external magnetic field (Fig. 8E and F). Experiments in animal models showed that BAY-exosomes as an effective DDV enhance the targeting of the pancreas islet under the external magnetic field and significantly increase insulin secretion, thereby alleviating hyperglycemia (Fig. 8G). Magnetic materials (e.g. T1 or T2 contrast agents) have the capability of magnetic resonance imaging (MRI), which opens up the possibility of MRI-guided therapy. In conclusion, the combination of magnetic materials and EVs has broad application prospects.
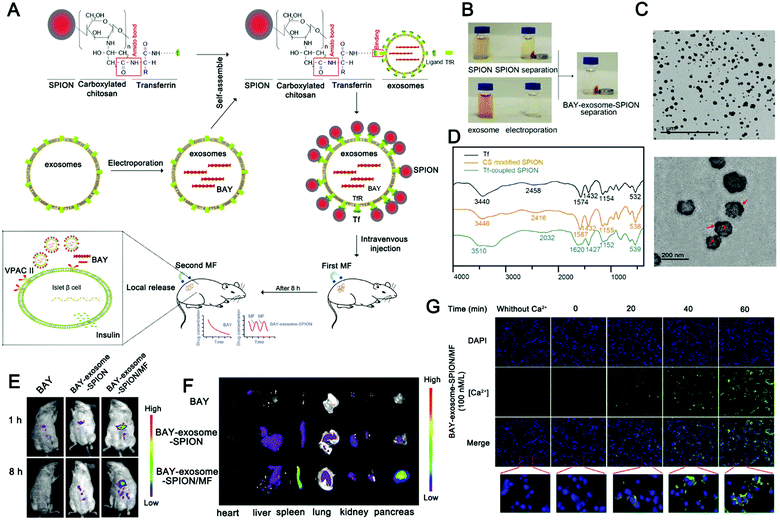 |
| Fig. 8 Engineered extracellular vesicles for magnetically-responsive insulin delivery. (A) Schematic diagram of the construction of BAY-exosome-SPIONs and their antidiabetic application. (B–D) Images (B), TEM image (C), and FT-IR spectra (D) of Tf, CS-SPIONs, and Tf-SPIONs. (E) Near-infrared fluorescence imaging of BAY55-9837 with Cy5.5 and BAY-exosome-SPIONs in mice after 1 or 8 h intravenous injection in the absence or presence of an external MF. (F) In vitro near-infrared fluorescence images were used to observe the fluorescence intensity of major organs after 8 h intravenous injection. (G) Fluorescence imaging was used to monitor the calcium influx in MIN-6 cells after treatment. (Reprinted with permission from ref. 170, Copyright 2019 Wiley-VCH Verlag GmbH & Co. KGaA, Weinheim.) | |
Ultrasound-responsive EVs.
Ultrasound technology has been intensively employed in biomedical areas such as imaging-guided drug administration due to its safety, tissue penetration, non-invasiveness, and better temporal and spatial control by accurately focusing on the target area.324–328 Ultrasonic waves stimulate the release of carrier drugs through thermal or mechanical effects, and the resulting radiative forces. Sonodynamic therapy (SDT) is an emerging treatment method that relies on ultrasound (US) technology, which has attracted extensive attention in recent years due to its simple implementation and intrinsic high tissue penetration.325 SDT further increases the breadth of ultrasound application. However, most sonosensitizers required for acoustic dynamics either have biodegradability and biosecurity problems or are water-soluble and chemically unstable, which greatly limits their clinical use.
To overcome the limitations of conventional delivery systems, more and more attempts are dedicated to exploring autologous cellular or subcellular bioactive structure derived natural carriers. EVs are promising carriers due to their high histocompatibility, high loading efficiency, and low immunogenicity. Liu et al. prepared functionalized intelligent nanoparticles by loading the US responsive agent porphyrin sodium (DVDMS) into exosomes derived from homotypic tumor cells via very mild incubation.329 By camouflaging tumor exosomes, the nanoparticles improve the chemical stability and circulation time of the drugs in the bloodstream and enhance the target delivery of DVDMS to primary and metastatic tumors. The authors improved the therapeutic effect by introducing secondary ultrasound response, where guided ultrasound (US1) was used to promote the local aggregation of DVDMS outside the tumor area and therapeutic ultrasound (US2) for SDT. EXO-DVDMS showed controllable ultrasound-sensitive drug release with enhanced ROS generation, thus improving SDT both in vivo and in vitro.
To increase the long-term efficacy of sonodynamic therapy, a combination of sonodynamic therapy and immunotherapy has been proposed recently. Zhang et al. developed a biomimetic nanosystem that can respond to exogenous stimulation with ultrasound and endogenous stimulation with hydrogen peroxide (H2O2) in the tumor microenvironment, respectively, to provide in situ delivery of singlet oxygen (1O2) and carbon monoxide (CO) to the target tumor (Fig. 9).133 Taking advantage of the RES evasion and tumor homing abilities of the macrophage membrane (Fig. 9B–D), the designed biomimetic nanosystem exhibits outstanding enrichment in the tumor tissue and effective resistance of tumor development via US/H2O2-generated 1O2 and CO to trigger mitochondrial dysfunction and cell apoptosis (Fig. 9E and F). Meanwhile, the nanoparticles can lead to tumor immunogenic cell death (ICD) through 1O2/CO synergistic therapy, permitting strong immune responses with long-term immune memory through the indoleamine 2,3-dioxygenase (IDO) signal blocking that effectively inhibits tumor rechallenge and lung metastasis.
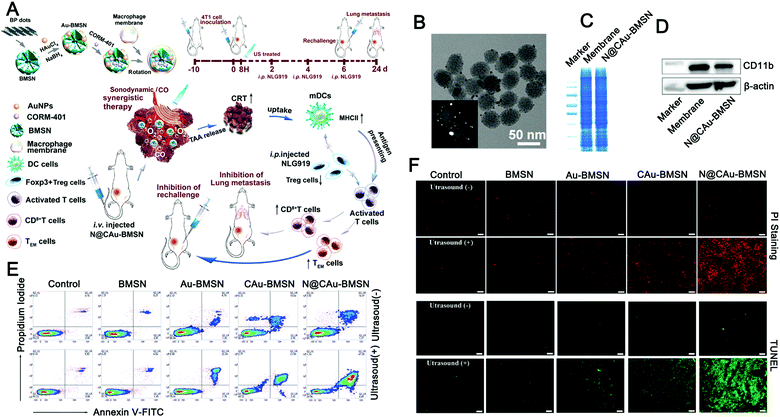 |
| Fig. 9 Ultrasound-driven exosome mimetics suppress tumor growth and metastasis. (A) Graphical summary of the fabrication of biomimetic nanosystems and their anticancer application. (B) TEM was used to observe the morphology of N@CAu-BMSNs. (C and D) SDS-PAGE was used to perform the protein pattern analysis of macrophage cell membranes and N@CAu-BMSNs, and the Western blotting analysis was used to detect the membrane-specific protein markers. (E) Flow cytometry analyses the apoptosis of 4T1 cells treated with different doses of NPs (BMSNs, Au-BMSNs, CAu-BMSNs, and N@CAu-BMSNs) upon US triggering for 60 s (1 MHz, 1 W cm−2). (F) Confocal laser imaging was used to observe cell death after different treatments. (Reprinted with permission from ref. 133, Copyright 2020 American Chemical Society.) | |
Dual and multiple stimuli-responsive EVs
Apart from the single stimulus-responsive EVs mentioned above, combined triggers that use two or more stimuli, such as pH–light, temperature–pH, redox potential–light, and pH–enzyme combinations, are applicable for intelligent EV-based DDVs. The development of these dual or multiple-stimulus responsive exosome-inspired nanosystems would effectively achieve their precise release at target sites.
pH and light dual stimuli-responsive EVs.
Low pH is not unique to tumors, and other lesions or physiological processes may also exhibit tissue acidification.331–333 Therefore, the delivery scheme with pH as the single response condition has the risk of being off-target. Combined with other stimulus responses, it can reduce the risk of being off-target and improve the therapeutic effect. Light irradiation is an effective way to trigger drug release. As a source of energy for photodynamic and photothermal therapy, light is a controlled and promising physical stimulus. The combination of endogenous pH response and exogenous light response is expected to achieve precise and controllable targeted delivery and treatment. Recently, pH and light dual stimuli-responsive systems have been investigated in exosome hybrid materials. Zhang et al. used milk exosomes to construct a pH and photosensitive DDV to enhance the anticancer effect of drugs in oral cancer (Fig. 10A).330 Doxorubicin (DOX) was conjugated to milk-exosomes by a pH-sensitive benzoic–imine bond and can be cracked in acidic microenvironments (Fig. 10C). Chlorin E6 (Ce6) is also loaded to produce singlet oxygen under light to kill cancer cells (Fig. 10B and D). The distribution, antitumor action, and biocompatibility of nanoparticles were also studied (Fig. 10E–G). The new drug release system based on milk exosomes was proven to be effective in controlling drug release, biocompatibility, and OSCC treatment.
 |
| Fig. 10 pH and light dual-responsive EVs for drug release. (A) Graphical summary of the fabrication process and working principle of Exo@DOX-EPT1 (NPs). (B) UV-visible absorption was used to detect the optical properties of EPT1 and 2. (C) In vitro drug release curves of Exo@DOX-EPT1 (NPs) in different pH environments. (D) Fluorescence microscopy was used to compare the internalization of NPs and free DOX by different cell lines (HSC-3, SCC-9, CAL-27, and HCM cells). (E) Tumor volume growth curves of mice receiving different treatments; (F) physical image to observe the tumor volume harvested from each group; and (G) antitumor effects in a mouse model of Exo@DOX-EPT1 under NIR laser irradiation. *P < 0.05. (Reproduced from ref. 330, Copyright 2020 The Authors.) | |
pH and temperature dual stimuli-responsive EVs.
Thermal stimulation is another type of exogenous stimulation commonly used in medicine. Combining the pH response with the thermal response also enables a precise and controlled treatment process. In a recent study, Wang et al. conjugated exosomes to thermo-sensitive hydrogels using a pH-sensitive imine bond to promote chronic diabetic wound healing and skin regeneration (Fig. 11A).334 The hydrogel has a variety of functional properties such as a rapid self-healing process, shear-thinning injectable capability, high antibacterial activity, and long-term pH-responsive exosome release behavior (Fig. 11B and C). The F127/OHA-EPL(FHE)@exo hydrogel significantly improved the healing efficacy of full-thickness diabetic skin trauma, which is characterized by improved wound closure rates, fast angiogenesis, re-epithelialization, and fast collagen deposition in wounds (Fig. 11D–G). This work provides a new approach to completing the repair of chronic injuries through multifunctional hydrogels with controlled exosome release.
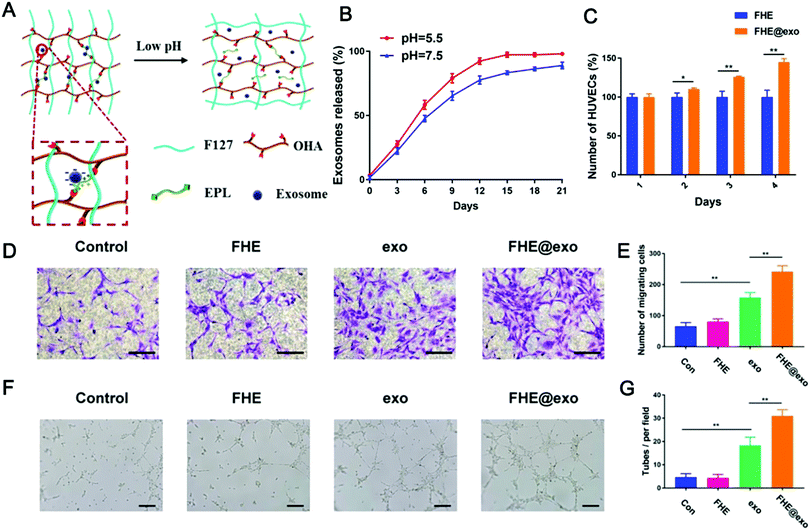 |
| Fig. 11 A pH and temperature dual stimulus-responsive antibacterial exosome hydrogel for promoting wound healing. (A) Schematic diagram of pH and temperature dual-responsive exosome release; (B) exosome release curves under different pH conditions; (C) changes in cell viability of HUVECs treated with FHE and FHE@exo hydrogels; (D and E) experimental results of transwell migration of HUVECs under different treatments (scale bar: 50 μm); and (F and G) effects of different treatments on the tube formation of HUVECs (scale bar: 200 μm). (Reprinted with permission from ref. 334, Copyright © Ivyspring International Publisher.) | |
pH and enzyme dual stimuli-responsive EVs.
In addition to the combination of internal and external stimulus responses, the combination of different internal stimulus responses can also have a synergistic response effect. The physiological changes during the development of the disease are essentially caused by changes in the expression or activity of enzymes in the tissues. Especially in tumors, the expression profiles of enzymes are different from those of normal tissues. The construction of the enzyme and pH dual-responsive system can avoid the off-target brought by a single stimulus, and simultaneously can take into account both intracellular and extracellular stimuli, forming a multilevel response mode, which is expected to realize precisely targeted drug delivery. For example, Zuo et al. achieved drug release in response to acidity/Cath B enzymes by synthesizing the polymer PLga-p-PEI-da and self-assembling it into an exosome-like structure.294 When arriving in tumor tissue, PPP-DA/NPs can deplete the DA shell through pH-cleavable hydrolysis of β-carboxylic amide bonded with PEI and DA. PPP-DA/NPs were further destroyed due to the Cath B enzyme-mediated cleavage of the GFLGF (Gly-Phe-Leu-Gly-Phe) linker. This system has enzyme-dependent selectivity and high efficiency in inhibiting tumor metastasis.
Light and redox potential dual stimuli-responsive EVs.
Light and redox potential dual stimulus-responsive systems have important application value in disease-targeting applications and cancer therapy. As a stimulant, NIR light has attracted extensive attention in the biomedical field. Photothermal therapy uses photothermal agents to convert light energy into heat energy, thereby inhibiting tumor cells by burning the tumor. It should be noted that NIR light-based photothermal therapy can penetrate human tissues without causing invasive damage.257 Ding and co-workers proposed an EV-like nanozyme vector for photoacoustic imaging of nasopharyngeal carcinoma in an H2O2-responsive manner.335 The mechanism is that the inherent peroxidase activity in graphene quantum dots effectively catalyzes the oxidation of the 2,2′-azino-bis(3-ethylbenzothiazoline-6-sulfonic acid) (ABTS) in the presence of H2O2. Significant NIR absorbance can be observed, making it a good contrast agent for photoacoustic imaging. Animal experiments have shown that this EV-like nanozyme vector exhibits good biocompatibility and long blood circulation and effective enrichment in NPC and selectively triggers PAI of NPC through the enzyme catalysis.
Magnetic field and acid dual stimuli-responsive EVs.
The biological distribution of exosomes plays an important role in maintaining tissue homeostasis and pathological processes. Manipulating the biological distribution of exosomes can promote the biological effects of therapy. Liu et al. used magnetic field responsive materials to capture exosomes in vivo and manipulate exosomes to target the heart, altering the distribution of exosomes (Fig. 12A and B).65 At the same time, acid stimulus-responsive groups were introduced into the acidic microenvironment of the lesion to achieve the accurate release of exosomes into the lesion microenvironment. The authors found that the method was efficient in binding exosomes from serum (Fig. 12C–E) and demonstrated in vitro that an acidic microenvironment mediated exosome release (Fig. 12F). Further, they demonstrated the efficacy of vesicular fusions in the treatment of myocardial infarction (MI). This study provides a magnetic field and acid dual stimuli-responsive exosome controlled-release strategy to deliver exosomes from patients to the lesion without isolation, overcoming the current dilemma of exosome purification.
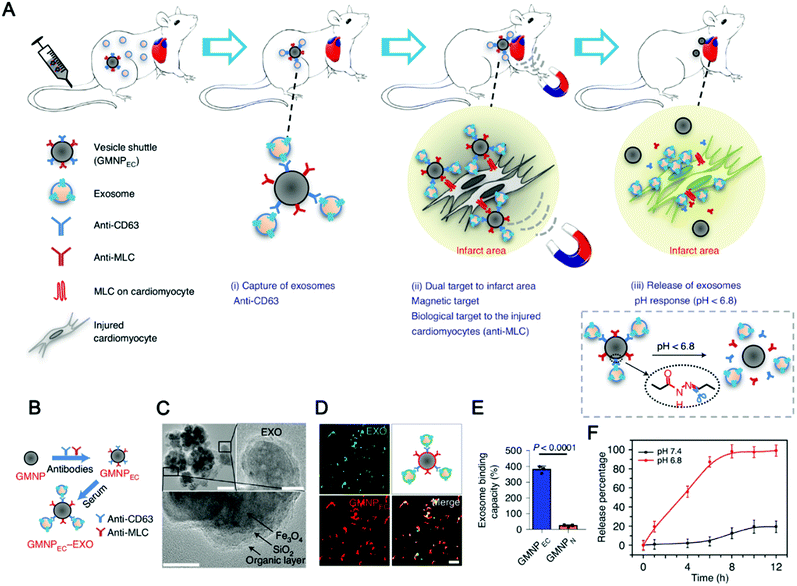 |
| Fig. 12 Exosome capture and delivery platform for magnetic targeting and acid response release. (A and B) Graphical summary of the vesicle-shuttle approach. (C) TEM imaging was used to observe the conjugation between GMNPEC and rat-serum-derived exosomes. Top left scale bar, 100 nm, top right scale bar, 20 nm, and bottom scale bar, 10 nm. (D) Representative confocal microscopy imaging is used for observation of GMNPEC-EXO. (E) The exosome-binding ability of GMNPEC and GMNPN. (F) Exosome release curves were used to study pH-sensitive controlled release. (Reprinted with permission from ref. 65, Copyright the authors.) | |
Intelligent EVs with treatment monitoring and evaluation
Real-time monitoring of targeted therapies in cancer patients can provide important information on the development of resistance and improve treatment outcomes. However, current techniques for measuring drug–target interactions require complex treatments and invasive tissue biopsies, which limit their clinical application in cancer therapeutic monitoring. Treatment monitoring and evaluation using EVs have become a new research hotspot. Liang et al. reported a fast, ultra-sensitive, and inexpensive nanoscale plasmon-enhanced scattering (NPES) platform for direct quantification of oncogenic EVs with only 1 μL plasma.336 NPES analysis can be easily applied to clinical applications and also the monitoring of other disease-specific EV biomarkers. Wang et al. proposed the feasibility of using multiple EV phenotypic analysis chips (EPAC) to monitor patients’ response to treatment based on plasma EV phenotypic evolution.191 They longitudinally monitored the evolution of EV phenotypes in 8 melanoma patients with targeted therapies and identified specific EV profiles associated with drug resistance, reflecting the potential of EV phenotypes in monitoring treatment response. A recent report is encouraging. Pan et al. applied the biological orthogonal reaction to the target site analysis of EVs and realized the precise monitoring of targeted therapy.192 These studies have made significant breakthroughs, but they still leave much to be desired in terms of intelligent feedback to treatment. More intelligent treatment feedback strategies, such as the use of responsive imaging probes to enable non-invasive and in situ treatment monitoring, are still needed.
Challenges and perspectives
EVs integrated with an intelligent core are the dawn of a new generation of nanomedicine, which is expected to realize the integration of active targeting, stimuli-response, and therapeutic feedback, and promote the development of precision diagnosis and treatment. However, many problems still need to be solved before the translation of EVs into clinical practice.337
First, the toxicity and safety of EVs are a huge challenge, which has been widely ignored. Tumor-derived EVs can target tumor tissue through the homologous affinity effect, which has been widely used in preclinical studies of antitumor therapy. However, other reports have shown that tumor-derived EVs can inhibit the proliferation of CD8+ T cells by reducing the level of IL-2 or induce the apoptosis of CD8+ T cells by the FasL-TRAIL pathway or Galectin 9.338–340 Moreover, they can trigger signaling pathways that are conducive to tumor growth, metastasis, and angiogenesis and promote the tumor to escape the recognition of immune vaccines to resist treatment.341 Therefore, finding how to regulate the balance between the antitumor and protumor effects of EVs is a problem that cannot be ignored.342 The pro-inflammatory effect of M1-type macrophage-derived EVs has been used in anti-tumor therapy, but it also has safety risks. For example, M1-type EVs may cause macrophages in the liver and other organs to polarize to a pro-inflammatory phenotype, which may cause organ damage.227,343,344 In other cases, some heterogenous EVs may present immune risks when used to treat diseases. In general, more efforts should be made towards studying the toxicity and side effects of EVs before their clinical transformation.
The heterogeneity of EVs is another challenge in clinical transformation. EVs are a large collection of subsets such as exosomes and microvesicles. Currently, it is difficult to obtain a single subset of EVs using commonly used EV separation methods, such as differential centrifugation, ultrafiltration, and polymer precipitation. This makes standardization of EVs difficult and reduces the reproducibility of the therapeutic effects of EVs. In addition, the purification process is also time- and labor-consuming.345 Size exclusion chromatography uses molecular sieve theory, does not require strong centrifugal force, and can better retain the integrity of EVs, but its low yield and high cost also limit its application, so it still requires improvement or the discovery of a more complete preparation method at present.
The ethic of the use of EVs is another concern. To the authors’ knowledge, there are currently no ethical guidelines for the clinical use of EVs. Is the use of allograft vesicles sufficient to protect both parties’ right to know? Are patients aware of the safety risks associated with using EVs? All these need ethical norms. The establishment and improvement of relevant ethical norms are helpful to protect the rights and interests of patients and promote use of extracellular vesicles correctly.
EV-based drug delivery strategies are playing an increasingly important role in the fields of antitumor therapy and tissue repair. Currently, it is difficult to evaluate the different physiological roles of different EVs produced in vivo. Although the roles and mechanisms of some components of EVs in the occurrence and progression of tumors have been clarified, the roles of more components remain to be further studied due to the complexity of their components. In the future, research on tumor-specific inhibition should be further strengthened to find the most suitable target for reducing toxicity and side effects. In addition, stronger research and modification of EVs are needed to solve the existing problems. EV-based diagnosis and treatment research still has a long way to go, but there is no doubt that it has broad prospects in the field of nanomedicine. Based on the development trend in recent years, we predict that intelligent EVs will develop in the following direction in the future.
From the EV structural dependence to functional dependence
The functions of EVs themselves will be paid more attention in the future, especially the interaction mechanism between vesicles and cells.346 EVs are involved in intercellular communication, immune-modulation, and metabolic regulation, and promote cell proliferation and migration by transporting active molecules such as proteins, nucleic acids, lipid molecules, and other bioactive molecules.347–350 As a natural therapeutic drug, EVs play an important role in the treatment of complex diseases.351–353 However, most of the existing studies take advantage of the good loading efficiency and histocompatibility of EVs but lack the utilization of EVs’ inherent functions. According to clinical needs, EVs or their mimetics with their corresponding biological functions were prepared, and engineered EVs were developed in combination with the advantages of other materials, which had important application prospects.
From single stimuli-responsive to multi-stimuli or multi-mode stimuli-responsive
Stimulus-responsive materials have been developed rapidly in recent years due to their significant advantages in drug delivery. Early stimuli-responsive materials achieve controlled drug release through a single stimulus-response, which often fails to exert ideal effects in the face of a complex physiological environment.354–356 Multi-stimuli-responsive materials can exhibit graded responses to multiple stimuli, and finally achieve controlled drug release when all stimuli are satisfied simultaneously.357 This greatly enhances the targeting and controllability of drugs and is expected to achieve accurate treatment of diseases. Multi-stimuli responsive materials are the future trend of intelligent carriers. Stimuli-responsive EV-materials provide a new idea for the development of intelligent carriers.138 In the future, stimuli-responsive EV-materials will develop from single stimuli-responsive to multi-stimuli-responsive.
The emergence of intelligent EVs in novel therapeutics
Gene therapy is a therapy that carries genetic material or gene-editing equipment into cells to continuously produce therapeutic proteins or to correct faulty genes in cells.307,358–360 However, cell membranes create barriers for nucleic acid molecules to enter the cell. Therefore, the carrier that can carry nucleic acids into the cell is very important for gene therapy. EVs are the natural carriers of cell–cell communication and have been designed to wrap nucleic acids and deliver them to the tissues and cells they need.361,362 However, traditional gene delivery strategies may increase the risk of being off-target.363–365 Stimuli-responsive EV-materials are expected to realize intelligent gene therapy through the controlled release of gene vectors. This will bring about the innovation of vector materials in the gene therapy field and finally promote the clinical application of gene therapy.
Immunotherapy is a treatment method that manipulates the immune system to clear lesions by intervening in the immune process of the body, which is considered to be the most promising treatment strategy in the field of tumor therapy.366–368 As natural information carriers, EVs play a key regulatory function in the body, among which the regulatory function of the immune system has been widely concerned.337 For example, M1 macrophage-derived exosomes have been widely used in anti-tumor research.44,369 However, the tumor immune microenvironment is a state of immune hijacking, and it is very difficult to achieve the therapeutic effect of modulation alone.370–372 Therefore, the development of EV-based multi-mode immunotherapy strategies to increase the infiltration of immune cells and block immunosuppressive immune checkpoints is expected to improve the efficacy of immunotherapy. More importantly, stimuli-responsive EVs are expected to achieve precise immunotherapy and avoid systemic immune risks. In conclusion, stimuli-responsive EVs have great potential in the field of immunotherapy.
An image-guided stimuli-responsive strategy opens a new generation of precision medicine. Chronic diseases such as tumors are complicated diseases and can be originated from many factors with accompanied high heterogeneity. Given this situation, modern medicine has put forward the development direction of precision medicine, aiming at developing new methods and technologies for accurate diagnosis and treatment.373,374 An intelligent nanoplatform has an active targeting strategy and stimuli-responsive strategy, which is consistent with the concept of precision medicine. An image-guided stimuli-responsive strategy is a further upgrade of the stimuli-responsive strategy. It can realize visualization of lesions through imaging methods, and achieve precise treatment control through human judgment and selection. This fully combines human initiatives with intelligent material selectivity organically, avoiding the off-target effect brought about by the complex physiological environment more effectively.
Data-driven and machine learning guided next-generation hybrid design toward intelligent delivery and personalized evaluation
High-throughput experiments supported by small samples are becoming increasingly widespread. The data sets generated by high-throughput experiments are too huge that computational assistance is thus essential.375,376 However, there is a wealth of data about relationships that researchers want to understand. Machine learning (ML) algorithms, and deep learning, in particular, make it possible to analyze big data integration by training models that can be used to classify observed data into discrete groups, understand which features determine performance indicators, or predict the results of new experiments. The application of ML in the analysis and interpretation of biological or genetic information, acceleration of drug discovery, recognition of selective small-molecule modulators or rare molecules, and behavior prediction has aroused increasing interest.377–379 In recent years, ML has been applied to solve nanotechnology problems, including the design of nanoscale systems, nanoscale computing, and nanoscale simulation.380–383 This also provides a new way of thinking for the design of EV materials.
Conclusions
Since their discovery, EVs have attracted extensive attention from researchers,191,384–387 depending on their advantages such as histocompatibility, bioactivity, and low immunogenicity. However, it is difficult for EVs as natural products to meet all the functional needs of clinics. Engineered EVs, that is, EVs are modified with engineering design ideas to meet actual needs, have made great progress in recent years.388,389 In particular, EV-inspired stimuli-responsive systems not only realized functional integration, but also endowed EVs with active targeting, stimulus-response, and treatment feedback properties, which greatly expanded the prospects of EVs. For example, the development of endogenous stimuli-responsive EVs by utilizing the acidity, high redox potential, specific enzymes, or miRNA expression in the tumor microenvironment not only improves the anti-tumor effect of traditional drugs but also reduces their toxic and side effects. In addition, intelligent EVs constructed with exogenous stimuli-responsive modules achieved the passive targeting of tumors by improving the histocompatibility and long circulation of traditional materials,390 and the precise release of drugs was realized under the action of controllable physical stimuli such as light, heat, magnetic fields and ultrasound. These stimuli-responsive characteristics achieve the precise release of drugs or contrast agents, greatly improving the therapeutic effect. However, it must be emphasized that the single stimulus-response strategy is difficult to function well in the complex organism-environment. A combination of two or more stimulus responses can further reduce the risk of a drug being off-target and improve the effect of precise diagnosis and treatment. At the same time, multi-stage response performance is used to develop a coordinated cocktail of different treatment approaches, which is expected to fight the disease to the greatest extent.
Intelligent EVs bring us new hope in drug delivery, but they also have some shortcomings. At first, there is still a lack of standardized methods for the preparation of engineered EVs, which makes their clinical application more difficult. At present, the preparation methods of engineered EVs include self-assembly, extrusion, hybridization, electroporation, and ultrasound. And each method varies greatly from article to article. This is very unfavorable to control the production rate and assembly efficiency. Secondly, although metal or inorganic materials selected for hybrid materials enable enhanced histocompatibility and long circulation, these materials still have metabolic risks. Thirdly, many studies have taken advantage of the structural advantages of EVs, but ignored their physiological functions. EVs act as communication stations in the body,391–394 with a wide range of biological regulatory capabilities that have been largely ignored. These problems have affected the development of EVs as drug carriers. The good news is that researchers are paying attention to these issues.
Intelligent EVs satisfy people's dual needs for natural and engineered carriers, which not only improve histocompatibility but also realize intelligent programmable properties. With the deepening of research, intelligent EVs with active targeting, stimuli-responsive, and treatment monitoring abilities will have broader application prospects in tumor treatment and regenerative medicine. Intelligent EVs have opened the door to a new generation of nanomedicine.
Author contributions
Conceptualization and supervision: D. Z., X. C., and L. N. Investigation, resources, and visualization: Z. F., C. J., and Y. W. Writing – original draft: Z. F., C. J., K. W., and Y. W. Writing – review and editing: J. M., D. Z., X. C., and L. N.
Conflicts of interest
There are no conflicts to declare.
Acknowledgements
This work was supported by grants from the National Natural Science Foundation of China (82002253, 81902138, 81922034, 61805041, and 91859113) and The Project of Public Welfare Technology Application of Zhejiang Province (No. LGF22H200016).
References
- M. Ferrari, Nat. Rev. Cancer, 2005, 5, 161–171 CrossRef CAS PubMed.
- Y. Liu and H. Wang, Nat. Nanotechnol., 2007, 2, 20–21 CrossRef CAS PubMed.
- D. J.-A. Cameron and M. P. Shaver, Chem. Soc. Rev., 2011, 40, 1761–1776 RSC.
- K. Y. Kim, Nat. Biotechnol., 2007, 25, 359–361 CrossRef CAS PubMed.
- G. Liao, F. He, Q. Li, L. Zhong, R. Zhao, H. Che, H. Gao and B. Fang, Prog. Mater. Sci., 2020, 112, 100666 CrossRef CAS.
- Y. J. Li, J. Y. Wu, J. Liu, W. Xu, X. Qiu, S. Huang, X. B. Hu and D. X. Xiang, J Nanobiotechnol., 2021, 19, 242 CrossRef PubMed.
- B. Li, Q. Tan, Z. Fan, K. Xiao and Y. Liao, Adv. Ther., 2020, 3, 1900189 CrossRef CAS.
- F. Zhou, M. Wang, T. Luo, J. Qu and W. R. Chen, Biomaterials, 2021, 265, 120421 CrossRef CAS PubMed.
- H. B. Cheng, Y. Li, B. Z. Tang and J. Yoon, Chem. Soc. Rev., 2020, 49, 21–31 RSC.
- J. Li and K. Pu, Chem. Soc. Rev., 2019, 48, 38–71 RSC.
- K. Ni, G. Lan and W. Lin, ACS Cent. Sci., 2020, 6, 861–868 CrossRef CAS PubMed.
- Y. Wang, J. Yu, Z. Luo, Q. Shi, G. Liu, F. Wu, Z. Wang, Y. Huang and D. Zhou, Adv. Mater., 2021, 33, e2103497 CrossRef PubMed.
- J. Yu, S. Liu, Y. Wang, X. He, Q. Zhang, Y. Qi, D. Zhou, Z. Xie, X. Li and Y. Huang, Bioact. Mater., 2022, 7, 389–400 CrossRef CAS PubMed.
- L. Zhou, W. Pi, M. Hao, Y. Li, H. An, Q. Li, P. Zhang and Y. Wen, Biomater. Sci., 2021, 9, 4904–4921 RSC.
- Y. Wang, Q. Zhao, B. Zhao, Y. Zheng, Q. Zhuang, N. Liao, P. Wang, Z. Cai, D. Zhang, Y. Zeng and X. Liu, Adv. Sci., 2022, 9, e2105631 CrossRef PubMed.
- R. Xu, A. Rai, M. Chen, W. Suwakulsiri, D. W. Greening and R. J. Simpson, Nat. Rev. Clin. Oncol., 2018, 15, 617–638 CrossRef CAS PubMed.
- Y. Fan, P. Wang, Y. Lu, R. Wang, L. Zhou, X. Zheng, X. Li, J. A. Piper and F. Zhang, Nat. Nanotechnol., 2018, 13, 941–946 CrossRef CAS PubMed.
- W. Tang, W. Fan, J. Lau, L. Deng, Z. Shen and X. Chen, Chem. Soc. Rev., 2019, 48, 2967–3014 RSC.
- S. Wang, R. Chen, Q. Yu, W. Huang, P. Lai, J. Tang and L. Nie, ACS Appl. Mater. Interfaces, 2020, 12, 45796–45806 CrossRef CAS PubMed.
- R. Chen, S. Huang, T. Lin, H. Ma, W. Shan, F. Duan, J. Lv, J. Zhang, L. Ren and L. Nie, Nat. Nanotechnol., 2021, 16, 455–465 CrossRef CAS PubMed.
- P. Gao, K. Tang, R. Lou, X. Liu, R. Wei, N. Li and B. Tang, Anal. Chem., 2021, 93, 12096–12102 CrossRef CAS PubMed.
- P. Gao, X. Shen, X. Liu, B. Cui, M. Wang, X. Wan, N. Li and B. Tang, ACS Appl. Mater. Interfaces, 2021, 13, 41498–41506 CrossRef CAS PubMed.
- M. Cully, Nat. Rev. Drug Discovery, 2016, 15, 231 CrossRef CAS PubMed.
- Q. Zhang, G. Kuang, S. He, H. Lu, Y. Cheng, D. Zhou and Y. Huang, Nano Lett., 2020, 20, 3039–3049 CrossRef CAS PubMed.
- X. Duan, C. Chan and W. Lin, Angew. Chem., Int. Ed., 2019, 58, 670–680 CrossRef CAS PubMed.
- T. Luo, K. Ni, A. Culbert, G. Lan, Z. Li, X. Jiang, M. Kaufmann and W. Lin, J. Am. Chem. Soc., 2020, 142, 7334–7339 CrossRef CAS PubMed.
- H. R. Culver, J. R. Clegg and N. A. Peppas, Acc. Chem. Res., 2017, 50, 170–178 CrossRef CAS PubMed.
- Y. Goto, Y. Ogawa, H. Tsumoto, Y. Miura, T. J. Nakamura, K. Ogawa, Y. Akimoto, H. Kawakami, T. Endo, R. Yanoshita and M. Tsujimoto, Biochim. Biophys. Acta, Mol. Cell Res., 2018, 1865, 874–888 CrossRef CAS PubMed.
- Y. Xiao and J. Du, J. Mater. Chem. B, 2020, 8, 354–367 RSC.
- R. Yang, F. Chen, J. Guo, D. Zhou and S. Luan, Bioact. Mater., 2020, 5, 990–1003 CrossRef PubMed.
- H. Liu, W. Lin, L. He and T. Chen, Biomaterials, 2020, 226, 119545 CrossRef CAS PubMed.
- S. Bakand and A. Hayes, Int. J. Mol. Sci., 2016, 17(6), 929 CrossRef PubMed.
- J. G. Croissant, K. S. Butler, J. I. Zink and C. J. Brinker, Nat. Rev. Mater., 2020, 5, 886–909 CrossRef CAS.
- Y. Luo, X. Wang and Y. Cao, Chem.–Biol. Interact., 2021, 333, 109325 CrossRef CAS PubMed.
- A. V. Singh, P. Laux, A. Luch, C. Sudrik, S. Wiehr, A.-M. Wild, G. Santomauro, J. Bill and M. Sitti, Toxicol. Mech. Methods, 2019, 29, 378–387 CrossRef CAS PubMed.
- S. Kheiri, X. Liu and M. Thompson, Colloids Surf., B, 2019, 184, 110550 CrossRef CAS PubMed.
- Y. Cao, J. Appl. Toxicol., 2022, 42, 4–16 CrossRef CAS PubMed.
- S. Li, D. Yan, C. Huang, F. Yang and Y. Cao, J. Hazard. Mater., 2022, 424, 127704 CrossRef CAS PubMed.
- F. Barbero, L. Russo, M. Vitali, J. Piella, I. Salvo, M. L. Borrajo, M. Busquets-Fite, R. Grandori, N. G. Bastus, E. Casals and V. Puntes, Semin. Immunol., 2017, 34, 52–60 CrossRef CAS PubMed.
- C. M. Sayes and D. B. Warheit, Wiley Interdiscip. Rev.: Nanomed. Nanobiotechnol., 2009, 1, 660–670 CAS.
- W. Poon, Y.-N. Zhang, B. Ouyang, B. R. Kingston, J. L.-Y. Wu, S. Wilhelm and W. C.-W. Chan, ACS Nano, 2019, 13, 5785–5798 CrossRef CAS PubMed.
- T. Yong, X. Zhang, N. Bie, H. Zhang, X. Zhang, F. Li, A. Hakeem, J. Hu, L. Gan, H. A. Santos and X. Yang, Nat. Commun., 2019, 10, 3838 CrossRef PubMed.
- W. Nie, G. Wu, J. Zhang, L. L. Huang, J. Ding, A. Jiang, Y. Zhang, Y. Liu, J. Li, K. Pu and H. Y. Xie, Angew. Chem., Int. Ed., 2020, 59, 2018–2022 CrossRef CAS PubMed.
- P. Wang, H. Wang, Q. Huang, C. Peng, L. Yao, H. Chen, Z. Qiu, Y. Wu, L. Wang and W. Chen, Theranostics, 2019, 9, 1714–1727 CrossRef CAS PubMed.
- P. Fu, J. Zhang, H. Li, M. Mak, W. Xu and Z. Tao, Adv. Drug Delivery Rev., 2021, 179, 113910 CrossRef CAS PubMed.
- G. van Niel, G. D'Angelo and G. Raposo, Nat. Rev. Mol. Cell Biol., 2018, 19, 213–228 CrossRef CAS PubMed.
- D. M. Pegtel and S. J. Gould, Annu. Rev. Biochem., 2019, 88, 487–514 CrossRef CAS PubMed.
- A. Thakur, X. Ke, Y. W. Chen, P. Motallebnejad, K. Zhang, Q. Lian and H. J. Chen, Protein Cell, 2021, 1–24 Search PubMed.
- C. Thery, K. W. Witwer, E. Aikawa, M. J. Alcaraz, J. D. Anderson, R. Andriantsitohaina, A. Antoniou, T. Arab, F. Archer, G. K. Atkin-Smith, D. C. Ayre, J. M. Bach, D. Bachurski, H. Baharvand, L. Balaj, S. Baldacchino, N. N. Bauer, A. A. Baxter, M. Bebawy, C. Beckham, A. Bedina Zavec, A. Benmoussa, A. C. Berardi, P. Bergese, E. Bielska, C. Blenkiron, S. Bobis-Wozowicz, E. Boilard, W. Boireau, A. Bongiovanni, F. E. Borras, S. Bosch, C. M. Boulanger, X. Breakefield, A. M. Breglio, M. A. Brennan, D. R. Brigstock, A. Brisson, M. L. Broekman, J. F. Bromberg, P. Bryl-Gorecka, S. Buch, A. H. Buck, D. Burger, S. Busatto, D. Buschmann, B. Bussolati, E. I. Buzas, J. B. Byrd, G. Camussi, D. R. Carter, S. Caruso, L. W. Chamley, Y. T. Chang, C. Chen, S. Chen, L. Cheng, A. R. Chin, A. Clayton, S. P. Clerici, A. Cocks, E. Cocucci, R. J. Coffey, A. Cordeiro-da-Silva, Y. Couch, F. A. Coumans, B. Coyle, R. Crescitelli, M. F. Criado, C. D'Souza-Schorey, S. Das, A. Datta Chaudhuri, P. de Candia, E. F. De Santana, O. De Wever, H. A. Del Portillo, T. Demaret, S. Deville, A. Devitt, B. Dhondt, D. Di Vizio, L. C. Dieterich, V. Dolo, A. P. Dominguez Rubio, M. Dominici, M. R. Dourado, T. A. Driedonks, F. V. Duarte, H. M. Duncan, R. M. Eichenberger, K. Ekstrom, S. El Andaloussi, C. Elie-Caille, U. Erdbrugger, J. M. Falcon-Perez, F. Fatima, J. E. Fish, M. Flores-Bellver, A. Forsonits, A. Frelet-Barrand, F. Fricke, G. Fuhrmann, S. Gabrielsson, A. Gamez-Valero, C. Gardiner, K. Gartner, R. Gaudin, Y. S. Gho, B. Giebel, C. Gilbert, M. Gimona, I. Giusti, D. C. Goberdhan, A. Gorgens, S. M. Gorski, D. W. Greening, J. C. Gross, A. Gualerzi, G. N. Gupta, D. Gustafson, A. Handberg, R. A. Haraszti, P. Harrison, H. Hegyesi, A. Hendrix, A. F. Hill, F. H. Hochberg, K. F. Hoffmann, B. Holder, H. Holthofer, B. Hosseinkhani, G. Hu, Y. Huang, V. Huber, S. Hunt, A. G. Ibrahim, T. Ikezu, J. M. Inal, M. Isin, A. Ivanova, H. K. Jackson, S. Jacobsen, S. M. Jay, M. Jayachandran, G. Jenster, L. Jiang, S. M. Johnson, J. C. Jones, A. Jong, T. Jovanovic-Talisman, S. Jung, R. Kalluri, S. I. Kano, S. Kaur, Y. Kawamura, E. T. Keller, D. Khamari, E. Khomyakova, A. Khvorova, P. Kierulf, K. P. Kim, T. Kislinger, M. Klingeborn, D. J. Klinke, 2nd, M. Kornek, M. M. Kosanovic, A. F. Kovacs, E. M. Kramer-Albers, S. Krasemann, M. Krause, I. V. Kurochkin, G. D. Kusuma, S. Kuypers, S. Laitinen, S. M. Langevin, L. R. Languino, J. Lannigan, C. Lasser, L. C. Laurent, G. Lavieu, E. Lazaro-Ibanez, S. Le Lay, M. S. Lee, Y. X.-F. Lee, D. S. Lemos, M. Lenassi, A. Leszczynska, I. T. Li, K. Liao, S. F. Libregts, E. Ligeti, R. Lim, S. K. Lim, A. Line, K. Linnemannstons, A. Llorente, C. A. Lombard, M. J. Lorenowicz, A. M. Lorincz, J. Lotvall, J. Lovett, M. C. Lowry, X. Loyer, Q. Lu, B. Lukomska, T. R. Lunavat, S. L. Maas, H. Malhi, A. Marcilla, J. Mariani, J. Mariscal, E. S. Martens-Uzunova, L. Martin-Jaular, M. C. Martinez, V. R. Martins, M. Mathieu, S. Mathivanan, M. Maugeri, L. K. McGinnis, M. J. McVey, D. G. Meckes, Jr., K. L. Meehan, I. Mertens, V. R. Minciacchi, A. Moller, M. Moller Jorgensen, A. Morales-Kastresana, J. Morhayim, F. Mullier, M. Muraca, L. Musante, V. Mussack, D. C. Muth, K. H. Myburgh, T. Najrana, M. Nawaz, I. Nazarenko, P. Nejsum, C. Neri, T. Neri, R. Nieuwland, L. Nimrichter, J. P. Nolan, E. N. Nolte-'t Hoen, N. Noren Hooten, L. O'Driscoll, T. O'Grady, A. O'Loghlen, T. Ochiya, M. Olivier, A. Ortiz, L. A. Ortiz, X. Osteikoetxea, O. Ostergaard, M. Ostrowski, J. Park, D. M. Pegtel, H. Peinado, F. Perut, M. W. Pfaffl, D. G. Phinney, B. C. Pieters, R. C. Pink, D. S. Pisetsky, E. Pogge von Strandmann, I. Polakovicova, I. K. Poon, B. H. Powell, I. Prada, L. Pulliam, P. Quesenberry, A. Radeghieri, R. L. Raffai, S. Raimondo, J. Rak, M. I. Ramirez, G. Raposo, M. S. Rayyan, N. Regev-Rudzki, F. L. Ricklefs, P. D. Robbins, D. D. Roberts, S. C. Rodrigues, E. Rohde, S. Rome, K. M. Rouschop, A. Rughetti, A. E. Russell, P. Saa, S. Sahoo, E. Salas-Huenuleo, C. Sanchez, J. A. Saugstad, M. J. Saul, R. M. Schiffelers, R. Schneider, T. H. Schoyen, A. Scott, E. Shahaj, S. Sharma, O. Shatnyeva, F. Shekari, G. V. Shelke, A. K. Shetty, K. Shiba, P. R. Siljander, A. M. Silva, A. Skowronek, O. L. Snyder, 2nd, R. P. Soares, B. W. Sodar, C. Soekmadji, J. Sotillo, P. D. Stahl, W. Stoorvogel, S. L. Stott, E. F. Strasser, S. Swift, H. Tahara, M. Tewari, K. Timms, S. Tiwari, R. Tixeira, M. Tkach, W. S. Toh, R. Tomasini, A. C. Torrecilhas, J. P. Tosar, V. Toxavidis, L. Urbanelli, P. Vader, B. W. van Balkom, S. G. van der Grein, J. Van Deun, M. J. van Herwijnen, K. Van Keuren-Jensen, G. van Niel, M. E. van Royen, A. J. van Wijnen, M. H. Vasconcelos, I. J. Vechetti, Jr., T. D. Veit, L. J. Vella, E. Velot, F. J. Verweij, B. Vestad, J. L. Vinas, T. Visnovitz, K. V. Vukman, J. Wahlgren, D. C. Watson, M. H. Wauben, A. Weaver, J. P. Webber, V. Weber, A. M. Wehman, D. J. Weiss, J. A. Welsh, S. Wendt, A. M. Wheelock, Z. Wiener, L. Witte, J. Wolfram, A. Xagorari, P. Xander, J. Xu, X. Yan, M. Yanez-Mo, H. Yin, Y. Yuana, V. Zappulli, J. Zarubova, V. Zekas, J. Y. Zhang, Z. Zhao, L. Zheng, A. R. Zheutlin, A. M. Zickler, P. Zimmermann, A. M. Zivkovic, D. Zocco and E. K. Zuba-Surma, J. Extracell. Vesicles, 2018, 7, 1535750 CrossRef PubMed.
- M. Mathieu, L. Martin-Jaular, G. Lavieu and C. Thery, Nat. Cell Biol., 2019, 21, 9–17 CrossRef CAS PubMed.
- C. Théry, L. Zitvogel and S. Amigorena, Nat. Rev. Immunol., 2002, 2, 569–579 CrossRef PubMed.
- R. Kalluri and V. S. LeBleu, Science, 2020, 367(6478), eaau6977 CrossRef CAS PubMed.
- D. M. Pegtel and S. J. Gould, Annu. Rev. Biochem., 2019, 88, 487–514 CrossRef CAS PubMed.
- Z. Dong, X. Sun, J. Xu, X. Han, Z. Xing, D. Wang, J. Ge, L. Meng and X. Xu, Med. Sci. Monit., 2019, 25, 7770–7783 CrossRef CAS PubMed.
- H. Wang, K. Wan, Y. Zhou, X. He, D. He, H. Cheng, J. Huang, R. Jia and K. Wang, Chem. Commun., 2020, 56, 12949–12952 RSC.
- X. Zhao, Y. Ren and Z. Lu, Biochim. Biophys. Acta, Rev. Cancer, 2020, 1874, 188414 CrossRef CAS PubMed.
- H. Wang, D. He, K. Wan, X. Sheng, H. Cheng, J. Huang, X. Zhou, X. He and K. Wang, Analyst, 2020, 145, 3289–3296 RSC.
- J. Zhang, C. Song, H. Zhou, J. Jia, Y. Dai, D. Cui, L. Wang and L. Weng, Analyst, 2020, 145, 1219–1226 RSC.
- F. Xie, G. Wen, W. Sun, K. Jiang, T. Chen, S. Chen and J. Wen, Biochem. Biophys. Res. Commun., 2020, 533, 346–353 CrossRef CAS PubMed.
- Z. Fan, J. Yu, J. Lin, Y. Liu and Y. Liao, Analyst, 2019, 144, 5856–5865 RSC.
- Y. Fu, C. Jiang, G. K. Tofaris and J. J. Davis, Anal. Chem., 2020, 92, 13647–13651 CrossRef CAS PubMed.
- P. Wu, B. Zhang, D. K.-W. Ocansey, W. Xu and H. Qian, Biomaterials, 2021, 269, 120467 CrossRef CAS PubMed.
- M. Chinnappan, A. Srivastava, N. Amreddy, M. Razaq, V. Pareek, R. Ahmed, M. Mehta, J. E. Peterson, A. Munshi and R. Ramesh, Cancer Lett., 2020, 486, 18–28 CrossRef CAS PubMed.
- C. Jiang, F. Hopfner, A. Katsikoudi, R. Hein, C. Catli, S. Evetts, Y. Huang, H. Wang, J. W. Ryder, G. Kuhlenbaeumer, G. Deuschl, A. Padovani, D. Berg, B. Borroni, M. T. Hu, J. J. Davis and G. K. Tofaris, J. Neurol Neurosurg., 2020, 91, 720 CrossRef PubMed.
- S. Liu, X. Chen, L. Bao, T. Liu, P. Yuan, X. Yang, X. Qiu, J. J. Gooding, Y. Bai, J. Xiao, F. Pu and Y. Jin, Nat. Biomed. Eng., 2020, 4, 1063–1075 CrossRef CAS PubMed.
- C. Jiang, F. Hopfner, D. Berg, M. T. Hu, A. Pilotto, B. Borroni, J. J. Davis and G. K. Tofaris, Mov. Disord., 2021, 36, 2663–2669 CrossRef CAS PubMed.
- L. Chen, W. Hong, W. Ren, T. Xu, Z. Qian and Z. He, Signal Transduct Target Ther., 2021, 6, 225 CrossRef PubMed.
- W. Liao, Y. Du, C. Zhang, F. Pan, Y. Yao, T. Zhang and Q. Peng, Acta Biomater., 2019, 86, 1–14 CrossRef CAS PubMed.
- S. Samanta, S. Rajasingh, N. Drosos, Z. Zhou, B. Dawn and J. Rajasingh, Acta Pharmacol. Sin., 2018, 39, 501–513 CrossRef CAS PubMed.
- D. Yuan, Y. Zhao, W. A. Banks, K. M. Bullock, M. Haney, E. Batrakova and A. V. Kabanov, Biomaterials, 2017, 142, 1–12 CrossRef CAS PubMed.
- T. Yang, P. Martin, B. Fogarty, A. Brown, K. Schurman, R. Phipps, V. P. Yin, P. Lockman and S. Bai, Pharm. Res., 2015, 32, 2003–2014 CrossRef CAS PubMed.
- M. Qu, Q. Lin, L. Huang, Y. Fu, L. Wang, S. He, Y. Fu, S. Yang, Z. Zhang, L. Zhang and X. Sun, J. Controlled Release, 2018, 287, 156–166 CrossRef CAS PubMed.
- Y. Fu and S. Xiong, J. Controlled Release, 2021, 335, 584–595 CrossRef CAS PubMed.
- V. Sengupta, S. Sengupta, A. Lazo, P. Woods, A. Nolan and N. Bremer, Stem Cells Dev., 2020, 29, 747–754 CrossRef CAS PubMed.
- F. A. Alzahrani, I. M. Saadeldin, A. Ahmad, D. Kumar, E. I. Azhar, A. J. Siddiqui, B. Kurdi, A. Sajini, A. F. Alrefaei and S. Jahan, Stem Cells Int., 2020, 8835986 CAS.
- M. Zhang, X. Zang, M. Wang, Z. Li, M. Qiao, H. Hu and D. Chen, J. Mater. Chem. B, 2019, 7, 2421–2433 RSC.
- G. Lou, Z. Chen, M. Zheng and Y. Liu, Exp. Mol. Med., 2017, 49, e346 CrossRef PubMed.
- I. L. Colao, R. Corteling, D. Bracewell and I. Wall, Trends Mol. Med., 2018, 24, 242–256 CrossRef CAS PubMed.
- W. M. Usman, T. C. Pham, Y. Y. Kwok, L. T. Vu, V. Ma, B. Peng, Y. S. Chan, L. Wei, S. M. Chin, A. Azad, A. B.-L. He, A. Y.-H. Leung, M. Yang, N. Shyh-Chang, W. C. Cho, J. Shi and M. T.-N. Le, Nat. Commun., 2018, 9, 2359 CrossRef PubMed.
- Z. Quinn, W. Mao, Y. Xia, R. John and Y. Wan, Bioact. Mater., 2021, 6, 749–756 CrossRef CAS PubMed.
- C. Feng, Z. Xiong, C. Wang, W. Xiao, H. Xiao, K. Xie, K. Chen, H. Liang, X. Zhang and H. Yang, Bioact. Mater., 2021, 6, 963–974 CrossRef CAS PubMed.
- I. K. Herrmann, M. J.-A. Wood and G. Fuhrmann, Nat. Nanotechnol., 2021, 16, 748–759 CrossRef CAS PubMed.
- S. Lazar, S. Mor, J. Chen, D. Hao and A. Wang, Extracell. Vesicles Circ. Nucl. Acids, 2021, 2, 175–178 Search PubMed.
- C. Liu, D. He, H. Cen, H. Chen, L. Li, G. Nie, Z. Zhong, Q. He, X. Yang, S. Guo, L. Wang and Z. Fan, Extracell. Vesicles Circ. Nucl. Acids, 2022, 3, 14–30 Search PubMed.
- M. Zipkin, Nat. Biotechnol., 2020, 38, 1226–1228 CrossRef CAS PubMed.
- Y. Sun and J. Liu, Clin. Ther., 2014, 36, 863–872 CrossRef PubMed.
- M. Mendt, K. Rezvani and E. Shpall, Bone Marrow Transplant., 2019, 54, 789–792 CrossRef PubMed.
- S. Hossen, M. K. Hossain, M. K. Basher, M. N.-H. Mia, M. T. Rahman and M. J. Uddin, J. Adv. Res., 2019, 15, 1–18 CrossRef CAS PubMed.
- E. V. Batrakova and M. S. Kim, J. Controlled Release, 2015, 219, 396–405 CrossRef CAS PubMed.
- C. von Schirnding, I. Giopanou, A. Hermawan, L. Wehl, G. Ntaliarda, B. Illes, S. Datz, F. Geisslinger, K. Bartel, A.-K. Sommer, M. Lianou, V. Weiß, J. Feckl, A. M. Vollmar, C. Bräuchle, G. T. Stathopoulos, E. Wagner, A. Roidl, T. Bein and H. Engelke, Chem, 2021, 7, 480–494 CAS.
- S. Zhou, Q. Zhong, Y. Wang, P. Hu, W. Zhong, C.-B. Huang, Z.-Q. Yu, C.-D. Ding, H. Liu and J. Fu, Coord. Chem. Rev., 2022, 452, 214309 CrossRef CAS.
- S. Li, L. Tan and X. Meng, Adv. Funct. Mater., 2020, 30, 1908924 CrossRef CAS.
- A. Jin, Y. Wang, K. Lin and L. Jiang, Bioact. Mater., 2020, 5, 522–541 CrossRef PubMed.
- X. Zhang, H. Zhang, J. Gu, J. Zhang, H. Shi, H. Qian, D. Wang, W. Xu, J. Pan and H. A. Santos, Adv. Mater., 2021, 33, e2005709 CrossRef PubMed.
- Y. Zhao, P. Liu, H. Tan, X. Chen, Q. Wang and T. Chen, Front. Oncol., 2021, 11, 743189 CrossRef PubMed.
- O. M. Elsharkasy, J. Z. Nordin, D. W. Hagey, O. G. de Jong, R. M. Schiffelers, S. E. Andaloussi and P. Vader, Adv. Drug Delivery Rev., 2020, 159, 332–343 CrossRef CAS PubMed.
- J. M. Gudbergsson, K. Jonsson, J. B. Simonsen and K. B. Johnsen, J. Controlled Release, 2019, 306, 108–120 CrossRef CAS PubMed.
- L. van der Koog, T. B. Gandek and A. Nagelkerke, Adv. Healthcare Mater., 2021, e2100639, DOI:10.1002/adhm.202100639.
- B. T. Pan and R. M. Johnstone, Cell, 1983, 33, 967–978 CrossRef CAS PubMed.
- S. A. Kooijmans, P. Vader, S. M. van Dommelen, W. W. van Solinge and R. M. Schiffelers, Int. J. Nanomed., 2012, 7, 1525–1541 CAS.
- D. Buschmann, V. Mussack and J. B. Byrd, Adv. Drug Delivery Rev., 2021, 174, 348–368 CrossRef CAS PubMed.
- E. L. Andaloussi, I. Mager, X. O. Breakefield and M. J. Wood, Nat. Rev. Drug Discovery, 2013, 12, 347–357 CrossRef PubMed.
- Y. N. Pi, B. R. Xia, M. Z. Jin, W. L. Jin and G. Lou, Biochem. Pharmacol., 2021, 186, 114487 CrossRef CAS PubMed.
- K. W. Witwer and C. Thery, J. Extracell. Vesicles, 2019, 8, 1648167 CrossRef PubMed.
- L. Bao, G. Dou, R. Tian, Y. Lv, F. Ding, S. Liu, R. Zhao, L. Zhao, J. Zhou, L. Weng, Y. Dong, B. Li, S. Liu, X. Chen and Y. Jin, Bioact. Mater., 2022, 9, 183–197 CrossRef CAS PubMed.
- A. Zijlstra and D. Di Vizio, Nat. Cell Biol., 2018, 20, 228–230 CrossRef CAS PubMed.
- S. Sharma, M. K. Masud, Y. V. Kaneti, P. Rewatkar, A. Koradia, M. S.-A. Hossain, Y. Yamauchi, A. Popat and C. Salomon, Small, 2021, 17, e2102220 CrossRef PubMed.
- Y. Yang, C. W. Li, L. C. Chan, Y. Wei, J. M. Hsu, W. Xia, J. H. Cha, J. Hou, J. L. Hsu, L. Sun and M. C. Hung, Cell Res., 2018, 28, 862–864 CrossRef CAS PubMed.
- G. Chen, A. C. Huang, W. Zhang, G. Zhang, M. Wu, W. Xu, Z. Yu, J. Yang, B. Wang, H. Sun, H. Xia, Q. Man, W. Zhong, L. F. Antelo, B. Wu, X. Xiong, X. Liu, L. Guan, T. Li, S. Liu, R. Yang, Y. Lu, L. Dong, S. McGettigan, R. Somasundaram, R. Radhakrishnan, G. Mills, Y. Lu, J. Kim, Y. H. Chen, H. Dong, Y. Zhao, G. C. Karakousis, T. C. Mitchell, L. M. Schuchter, M. Herlyn, E. J. Wherry, X. Xu and W. Guo, Nature, 2018, 560, 382–386 CrossRef CAS PubMed.
- G. Wang, L. Xie, B. Li, W. Sang, J. Yan, J. Li, H. Tian, W. Li, Z. Zhang, Y. Tian and Y. Dai, Nat. Commun., 2021, 12, 5733 CrossRef CAS PubMed.
- P. H. Tran, D. Xiang, T. N. Nguyen, T. T. Tran, Q. Chen, W. Yin, Y. Zhang, L. Kong, A. Duan, K. Chen, M. Sun, Y. Li, Y. Hou, Y. Zhu, Y. Ma, G. Jiang and W. Duan, Theranostics, 2020, 10, 3849–3866 CrossRef CAS PubMed.
- B. Jing, Y. Gai, R. Qian, Z. Liu, Z. Zhu, Y. Gao, X. Lan and R. An, J. Nanobiotechnol., 2021, 19, 7 CrossRef CAS PubMed.
- W. Fu, C. Lei, S. Liu, Y. Cui, C. Wang, K. Qian, T. Li, Y. Shen, X. Fan, F. Lin, M. Ding, M. Pan, X. Ye, Y. Yang and S. Hu, Nat. Commun., 2019, 10, 4355 CrossRef PubMed.
- R. E. Veerman, G. Gucluler Akpinar, M. Eldh and S. Gabrielsson, Trends Mol. Med., 2019, 25, 382–394 CrossRef CAS PubMed.
- C. Wen, R. C. Seeger, M. Fabbri, L. Wang, A. S. Wayne and A. Y. Jong, J. Extracell. Vesicles, 2017, 6, 1400370 CrossRef PubMed.
- Y. Fang, Y. Zhang, J. Zhou and K. Cao, Cell Tissue Bank, 2019, 20, 153–161 CrossRef PubMed.
- T. Zhao, F. Sun, J. Liu, T. Ding, J. She, F. Mao, W. Xu, H. Qian and Y. Yan, Curr. Stem Cell Res. Ther., 2019, 14, 482–494 CrossRef CAS PubMed.
- C. Liu, N. Bayado, D. He, J. Li, H. Chen, L. Li, J. Li, X. Long, T. Du, J. Tang, Y. Dang, Z. Fan, L. Wang and P. C. Yang, Front. Cardiovasc. Med., 2021, 8, 758050 CrossRef CAS PubMed.
- F. Gang, Q. Zhang, L. Jiang, Y. Xiao, N. Xu, Y. Wang, Y. Xiao, A. Li, Z. Liu, B. Liu, Y. Wu, X. Su, A. G. Perkins, Q. Wu, J. Zhang, J. Lin and X. Sun, Adv. Funct. Mater., 2021, 31, 2104131 CrossRef CAS.
- P. Li, M. Kaslan, S. H. Lee, J. Yao and Z. Gao, Theranostics, 2017, 7, 789–804 CrossRef CAS PubMed.
- H. Bu, D. He, X. He and K. Wang, ChemBioChem, 2019, 20, 451–461 CrossRef CAS PubMed.
- Y. P. Loh, Extracell. Vesicles Circ. Nucl. Acids, 2021, 2, 1–2 Search PubMed.
- Z. Zhao, H. Wijerathne, A. K. Godwin and S. A. Soper, Extracell. Vesicles Circ. Nucl. Acids, 2021, 2, 80–103 Search PubMed.
- M. Tayebi, D. Yang, D. J. Collins and Y. Ai, Nano Lett., 2021, 21, 6835–6842 CrossRef CAS PubMed.
- M. A. Livshits, E. Khomyakova, E. G. Evtushenko, V. N. Lazarev, N. A. Kulemin, S. E. Semina, E. V. Generozov and V. M. Govorun, Sci. Rep., 2015, 5, 17319 CrossRef PubMed.
- N. Ludwig, C. S. Hong, S. Ludwig, J. H. Azambuja, P. Sharma, M. N. Theodoraki and T. L. Whiteside, Curr. Protoc. Immunol., 2019, 127, e91 CAS.
- W. Wang, J. Luo and S. Wang, Adv. Healthcare Mater., 2018, 7, e1800484 CrossRef PubMed.
- Y. Zha, T. Lin, Y. Li, X. Zhang, Z. Wang, Z. Li, Y. Ye, B. Wang, S. Zhang and J. Wang, Biomaterials, 2020, 247, 119985 CrossRef CAS PubMed.
- S. P. Li, Z. X. Lin, X. Y. Jiang and X. Y. Yu, Acta Pharmacol. Sin., 2018, 39, 542–551 CrossRef CAS PubMed.
- A. J. Vazquez-Rios, A. Molina-Crespo, B. L. Bouzo, R. Lopez-Lopez, G. Moreno-Bueno and M. de la Fuente, J. Nanobiotechnol., 2019, 17, 85 CrossRef PubMed.
- S. C. Jang and Y. S. Gho, Nanomedicine, 2014, 9, 177–180 CrossRef CAS PubMed.
- L. Liang, L. Wen, Y. Weng, J. Song, H. Li, Y. Zhang, X. He, W. Zhao, M. Zhan, Y. Li, L. Lu, Y. Xin and C. Lu, Chem.
Eng. J., 2021, 425, 131451 CrossRef CAS.
- Q. Jiang, K. Wang, X. Zhang, B. Ouyang, H. Liu, Z. Pang and W. Yang, Small, 2020, 16, e2001704 CrossRef PubMed.
- S. Li, J. Liu, M. Sun, J. Wang, C. Wang and Y. Sun, Front. Pharmacol., 2020, 11, 24 CrossRef CAS PubMed.
- J. Y. Wu, Y. J. Li, X. B. Hu, S. Huang, S. Luo, T. Tang and D. X. Xiang, J. Controlled Release, 2021, 336, 510–521 CrossRef CAS PubMed.
- X. Zhang, C. Wang, J. Wang, Q. Hu, B. Langworthy, Y. Ye, W. Sun, J. Lin, T. Wang, J. Fine, H. Cheng, G. Dotti, P. Huang and Z. Gu, Adv. Mater., 2018, 30, e1707112 CrossRef PubMed.
- Y. Yang, K. Wang, Y. Pan, L. Rao and G. Luo, Adv. Sci., 2021, 8, e2102330 CrossRef PubMed.
- L. Rao, L. Wu, Z. Liu, R. Tian, G. Yu, Z. Zhou, K. Yang, H. G. Xiong, A. Zhang, G. T. Yu, W. Sun, H. Xu, J. Guo, A. Li, H. Chen, Z. J. Sun, Y. X. Fu and X. Chen, Nat. Commun., 2020, 11, 4909 CrossRef PubMed.
- P. A. Gonzales, H. Zhou, T. Pisitkun, N. S. Wang, R. A. Star, M. A. Knepper and P. S. Yuen, Methods Mol. Biol., 2010, 641, 89–99 CrossRef CAS PubMed.
- D. K. Jeppesen, M. L. Hvam, B. Primdahl-Bengtson, A. T. Boysen, B. Whitehead, L. Dyrskjot, T. F. Orntoft, K. A. Howard and M. S. Ostenfeld, J. Extracell. Vesicles, 2014, 3, 25011 CrossRef PubMed.
- K. Li, D. K. Wong, K. Y. Hong and R. L. Raffai, Methods Mol. Biol., 2018, 1740, 69–83 CrossRef CAS PubMed.
- Z. Zhang, C. Wang, T. Li, Z. Liu and L. Li, Oncol. Lett., 2014, 8, 1701–1706 CrossRef PubMed.
- D. W. Greening, R. Xu, H. Ji, B. J. Tauro and R. J. Simpson, Methods Mol. Biol., 2015, 1295, 179–209 CrossRef CAS PubMed.
- R. Xu, D. W. Greening, A. Rai, H. Ji and R. J. Simpson, Methods, 2015, 87, 11–25 CrossRef CAS PubMed.
- S. K. Channavajjhala, M. Rossato, F. Morandini, A. Castagna, F. Pizzolo, F. Bazzoni and O. Olivieri, Clin. Chem. Lab. Med., 2014, 52, 345–354 CrossRef CAS PubMed.
- M. An, J. Wu, J. Zhu and D. M. Lubman, J. Proteome Res., 2018, 17, 3599–3605 CrossRef CAS PubMed.
- T. Baranyai, K. Herczeg, Z. Onodi, I. Voszka, K. Modos, N. Marton, G. Nagy, I. Mager, M. J. Wood, S. El Andaloussi, Z. Palinkas, V. Kumar, P. Nagy, A. Kittel, E. I. Buzas, P. Ferdinandy and Z. Giricz, PLoS One, 2015, 10, e0145686 CrossRef PubMed.
- M. L. Alvarez, M. Khosroheidari, R. Kanchi Ravi and J. K. DiStefano, Kidney Int., 2012, 82, 1024–1032 CrossRef CAS PubMed.
- M. Lee, T. Liu, W. Im and M. Kim, Eur. J. Neurosci., 2016, 44, 2114–2119 CrossRef PubMed.
- C. Liu, J. Guo, F. Tian, N. Yang, F. Yan, Y. Ding, J. Wei, G. Hu, G. Nie and J. Sun, ACS Nano, 2017, 11, 6968–6976 CrossRef CAS PubMed.
- J. Wang, P. Ma, D. H. Kim, B. F. Liu and U. Demirci, Nano Today, 2021, 37, 101066 CrossRef CAS PubMed.
- L. Huang, N. Gu, X.-E. Zhang and D.-B. Wang, Adv. Funct. Mater., 2019, 29, 1807189 CrossRef.
- H. Liu, Z. Geng and J. Su, Extracell. Vesicles Circ. Nucl. Acids, 2022, 3, 63–86 CrossRef.
- L. Alvarez-Erviti, Y. Seow, H. Yin, C. Betts, S. Lakhal and M. J. Wood, Nat. Biotechnol., 2011, 29, 341–345 CrossRef CAS PubMed.
- C. Villarroya-Beltri, C. Gutierrez-Vazquez, F. Sanchez-Cabo, D. Perez-Hernandez, J. Vazquez, N. Martin-Cofreces, D. J. Martinez-Herrera, A. Pascual-Montano, M. Mittelbrunn and F. Sanchez-Madrid, Nat. Commun., 2013, 4, 2980 CrossRef PubMed.
- S. D. Gurien, M. Aziz, H. Jin, H. Wang, M. He, Y. Al-Abed, J. M. Nicastro, G. F. Coppa and P. Wang, EMBO Rep., 2020, 21, e48075 CrossRef CAS PubMed.
- S. Campagne, T. de Vries, F. Malard, P. Afanasyev, G. Dorn, E. Dedic, J. Kohlbrecher, D. Boehringer, A. Clery and F. H. Allain, Nucleic Acids Res., 2021, 49, e63 CrossRef CAS PubMed.
- Z. Fan, K. Xiao, J. Lin, Y. Liao and X. Huang, Small, 2019, 15, e1903761 CrossRef PubMed.
- X. Ai, S. Wang, Y. Duan, Q. Zhang, M. S. Chen, W. Gao and L. Zhang, Biochemistry, 2021, 60, 941–955 CrossRef CAS PubMed.
- X. Ai, D. Wang, A. Honko, Y. Duan, I. Gavrish, R. H. Fang, A. Griffiths, W. Gao and L. Zhang, J. Am. Chem. Soc., 2021, 143, 17615–17621 CrossRef CAS PubMed.
- X. Yang, B. Xie, H. Peng, G. Shi, B. Sreenivas, J. Guo, C. Wang and Y. He, J. Controlled Release, 2021, 329, 454–467 CrossRef CAS PubMed.
- D. Zhu, Y. Duo, M. Suo, Y. Zhao, L. Xia, Z. Zheng, Y. Li and B. Z. Tang, Angew. Chem., Int. Ed., 2020, 59, 13836–13843 CrossRef CAS PubMed.
- X. Zhang, J. Xiong, K. Wang, H. Yu, B. Sun, H. Ye, Z. Zhao, N. Wang, Y. Wang, S. Zhang, W. Zhao, H. Zhang, Z. He, C. Luo and J. Sun, Bioact. Mater., 2021, 6, 2291–2302 CrossRef CAS PubMed.
- N. Jasiewicz, C. Drabenstott and J. Nguyen, Curr. Opin. Colloid Interface Sci., 2021, 51, 101412 CrossRef CAS.
- A. K. Agrawal, F. Aqil, J. Jeyabalan, W. A. Spencer, J. Beck, B. W. Gachuki, S. S. Alhakeem, K. Oben, R. Munagala, S. Bondada and R. C. Gupta, Nanomedicine, 2017, 13, 1627–1636 CrossRef CAS PubMed.
- D. Sun, X. Zhuang, X. Xiang, Y. Liu, S. Zhang, C. Liu, S. Barnes, W. Grizzle, D. Miller and H. G. Zhang, Mol. Ther., 2010, 18, 1606–1614 CrossRef CAS PubMed.
- M. J. Haney, N. L. Klyachko, Y. Zhao, R. Gupta, E. G. Plotnikova, Z. He, T. Patel, A. Piroyan, M. Sokolsky, A. V. Kabanov and E. V. Batrakova, J. Controlled Release, 2015, 207, 18–30 CrossRef CAS PubMed.
- Q. Lin, M. Qu, B. Zhou, H. K. Patra, Z. Sun, Q. Luo, W. Yang, Y. Wu, Y. Zhang, L. Li, L. Deng, L. Wang, T. Gong, Q. He, L. Zhang, X. Sun and Z. Zhang, J. Controlled Release, 2019, 311–312, 104–116 CrossRef CAS PubMed.
- M. Yu, C. Gai, Z. Li, D. Ding, J. Zheng, W. Zhang, S. Lv and W. Li, Cancer Sci., 2019, 110, 3173–3182 CrossRef CAS PubMed.
- M. Zhuang, D. Du, L. Pu, H. Song, M. Deng, Q. Long, X. Yin, Y. Wang and L. Rao, Small, 2019, 15, 1903135 CrossRef CAS PubMed.
- Z. Yang, J. Shi, J. Xie, Y. Wang, J. Sun, T. Liu, Y. Zhao, X. Zhao, X. Wang, Y. Ma, V. Malkoc, C. Chiang, W. Deng, Y. Chen, Y. Fu, K. J. Kwak, Y. Fan, C. Kang, C. Yin, J. Rhee, P. Bertani, J. Otero, W. Lu, K. Yun, A. S. Lee, W. Jiang, L. Teng, B. Y.-S. Kim and L. J. Lee, Nat. Biomed. Eng., 2020, 4, 69–83 CrossRef CAS PubMed.
- J. Yang, S. Wu, L. Hou, D. Zhu, S. Yin, G. Yang and Y. Wang, Mol. Ther. Nucleic Acids, 2020, 21, 512–522 CrossRef CAS PubMed.
- U. Sterzenbach, U. Putz, L. H. Low, J. Silke, S. S. Tan and J. Howitt, Mol. Ther., 2017, 25, 1269–1278 CrossRef CAS PubMed.
- L. Sun, M. Fan, D. Huang, B. Li, R. Xu, F. Gao and Y. Chen, Biomaterials, 2021, 271, 120761 CrossRef CAS PubMed.
- L. Duan, L. Xu, X. Xu, Z. Qin, X. Zhou, Y. Xiao, Y. Liang and J. Xia, Nanoscale, 2021, 13, 1387–1397 RSC.
- L. Jiang, Y. Zhu, P. Luan, J. Xu, G. Ru, J. G. Fu, N. Sang, Y. Xiong, Y. He, G. Q. Lin, J. Wang, J. Zhang and R. Li, ACS Nano, 2021, 15, 4173–4185 CrossRef CAS PubMed.
- R. Lehner, X. Wang, S. Marsch and P. Hunziker, Nanomedicine, 2013, 9, 742–757 CrossRef CAS PubMed.
-
A. Tiwari, A. K. Mishra, H. Kobayashi and A. P.-F. Turner, Intelligent Nanomaterials, John Wiley & Sons, 2012 Search PubMed.
- S. Sindhwani, A. M. Syed, J. Ngai, B. R. Kingston, L. Maiorino, J. Rothschild, P. MacMillan, Y. Zhang, N. U. Rajesh, T. Hoang, J. L.-Y. Wu, S. Wilhelm, A. Zilman, S. Gadde, A. Sulaiman, B. Ouyang, Z. Lin, L. Wang, M. Egeblad and W. C.-W. Chan, Nat. Mater., 2020, 19, 566–575 CrossRef CAS PubMed.
- F. Danhier, J. Controlled Release, 2016, 244, 108–121 CrossRef CAS PubMed.
- R. Munagala, F. Aqil, J. Jeyabalan and R. C. Gupta, Cancer Lett., 2016, 371, 48–61 CrossRef CAS PubMed.
- D. Lin, H. Zhang, R. Liu, T. Deng, T. Ning, M. Bai, Y. Yang, K. Zhu, J. Wang, J. Duan, S. Ge, B. Sun, G. Ying and Y. Ba, Mol. Oncol., 2021, 15, 3430–3446 CrossRef PubMed.
- F. Hosseini Shamili, M. Alibolandi, H. Rafatpanah, K. Abnous, M. Mahmoudi, M. Kalantari, S. M. Taghdisi and M. Ramezani, J. Controlled Release, 2019, 299, 149–164 CrossRef CAS PubMed.
- C. Liu, W. Zhang, Y. Li, J. Chang, F. Tian, F. Zhao, Y. Ma and J. Sun, Nano Lett., 2019, 19, 7836–7844 CrossRef CAS PubMed.
- X. Wang, Y. Chen, Z. Zhao, Q. Meng, Y. Yu, J. Sun, Z. Yang, Y. Chen, J. Li, T. Ma, H. Liu, Z. Li, J. Yang and Z. Shen, J. Am. Heart Assoc., 2018, 7, e008737 CrossRef CAS PubMed.
- Y. Wan, L. Wang, C. Zhu, Q. Zheng, G. Wang, J. Tong, Y. Fang, Y. Xia, G. Cheng, X. He and S. Y. Zheng, Cancer Res., 2018, 78, 798–808 CrossRef CAS PubMed.
- J. Wang, Y. Dong, Y. Li, W. Li, K. Cheng, Y. Qian, G. Xu, X. Zhang, L. Hu, P. Chen, W. Du, X. Feng, Y.-D. Zhao, Z. Zhang and B.-F. Liu, Adv. Funct. Mater., 2018, 28, 1707360 CrossRef.
- L. Qiao, S. Hu, K. Huang, T. Su, Z. Li, A. Vandergriff, J. Cores, P.-U. Dinh, T. Allen, D. Shen, H. Liang, Y. Li and K. Cheng, Theranostics, 2020, 10, 3474–3487 CrossRef CAS PubMed.
- C. Gong, X. Zhang, M. Shi, F. Li, S. Wang, Y. Wang, Y. Wang, W. Wei and G. Ma, Adv. Sci., 2021, 8, 2002787 CrossRef CAS PubMed.
- F. Tian, S. Zhang, C. Liu, Z. Han, Y. Liu, J. Deng, Y. Li, X. Wu, L. Cai, L. Qin, Q. Chen, Y. Yuan, Y. Liu, Y. Cong, B. Ding, Z. Jiang and J. Sun, Nat. Commun., 2021, 12, 2536 CrossRef CAS PubMed.
- J. Wang, A. Wuethrich, A. A. Sina, R. E. Lane, L. L. Lin, Y. Wang, J. Cebon, A. Behren and M. Trau, Sci. Adv., 2020, 6, eaax3223 CrossRef CAS PubMed.
- S. Pan, Y. Zhang, A. Natalia, C. Z.-J. Lim, N. R.-Y. Ho, B. Chowbay, T. P. Loh, J. K.-C. Tam and H. Shao, Nat. Nanotechnol., 2021, 16, 734–742 CrossRef CAS PubMed.
- S. H. Hilton and I. M. White, Sens. Actuators Rep., 2021, 3, 100052 CrossRef PubMed.
- G. Yang, L. Xu, Y. Chao, J. Xu, X. Sun, Y. Wu, R. Peng and Z. Liu, Nat. Commun., 2017, 8, 902 CrossRef PubMed.
- S. Uthaman, K. M. Huh and I. K. Park, Biomater. Res., 2018, 22, 22 CrossRef PubMed.
- X. Wang, X. Zhong, H. Lei, N. Yang, X. Gao and L. Cheng, J. BioX Res., 2020, 3(04), 144–156 Search PubMed.
- Q. Fu, Z. Li, F. Fu, X. Chen, J. Song and H. Yang, Nano Today, 2021, 36, 101014 CrossRef CAS PubMed.
- J. Wang, W. Tang, M. Yang, Y. Yin, H. Li, F. Hu, L. Tang, X. Ma, Y. Zhang and Y. Wang, Biomaterials, 2021, 273, 120784 CrossRef CAS PubMed.
- C. Liu, Z. Fan, D. He, H. Chen, S. Zhang, S. Guo, B. Zheng, H. Cen, Y. Zhao, H. Liu and L. Wang, Pharmaceutics, 2022, 14(4), 758 CrossRef CAS PubMed.
- C. Tapeinos, H. Gao, T. Bauleth-Ramos and H. A. Santos, Small, 2022, 2200291 CrossRef PubMed.
- Y. Huang, L. Zou, J. Wang, Q. Jin and J. Ji, Wiley Interdiscip. Rev.: Nanomed. Nanobiotechnol., 2022, e1775 Search PubMed.
- N. N. Pavlova and C. B. Thompson, Cell Metab., 2016, 23, 27–47 CrossRef CAS PubMed.
- A. E. Allen and J. W. Locasale, Cancer Cell, 2018, 33, 337–339 CrossRef CAS PubMed.
- D. Hanahan and R. A. Weinberg, Cell, 2011, 144, 646–674 CrossRef CAS PubMed.
- D. Rotin, P. Wan, S. Grinstein and I. Tannock, Cancer Res., 1987, 47, 1497 CAS.
- X. Li, B. Y. Zheng, M. R. Ke, Y. Zhang, J. D. Huang and J. Yoon, Theranostics, 2017, 7, 2746–2756 CrossRef CAS PubMed.
- W. Gao, J. M. Chan and O. C. Farokhzad, Mol. Pharmaceutics, 2010, 7, 1913–1920 CrossRef CAS PubMed.
- P. Gupta, K. Vermani and S. Garg, Drug Discovery Today, 2002, 7, 569–579 CrossRef CAS PubMed.
- S. Zhang, A. M. Bellinger, D. L. Glettig, R. Barman, Y.-A. L. Lee, J. Zhu, C. Cleveland, V. A. Montgomery, L. Gu, L. D. Nash, D. J. Maitland, R. Langer and G. Traverso, Nat. Mater., 2015, 14, 1065–1071 CrossRef CAS PubMed.
- K. Li, D. Li, L. Zhao, Y. Chang, Y. Zhang, Y. Cui and Z. Zhang, Bioact. Mater., 2020, 5, 721–731 CrossRef PubMed.
- Z. Yang, Q. Chen, J. Chen, Z. Dong, R. Zhang, J. Liu and Z. Liu, Small, 2018, 14, e1803262 CrossRef PubMed.
- J. Y. Kim, J. Song, H. Jung and H. Mok, Appl. Biol. Chem., 2018, 61, 599–606 CrossRef CAS.
- G. Cheng, W. Li, L. Ha, X. Han, S. Hao, Y. Wan, Z. Wang, F. Dong, X. Zou, Y. Mao and S. Y. Zheng, J. Am. Chem. Soc., 2018, 140, 7282–7291 CrossRef CAS PubMed.
- K. Liang, R. Ricco, C. M. Doherty, M. J. Styles, S. Bell, N. Kirby, S. Mudie, D. Haylock, A. J. Hill, C. J. Doonan and P. Falcaro, Nat. Commun., 2015, 6, 7240 CrossRef CAS PubMed.
- K. Wang, H. Ye, X. Zhang, X. Wang, B. Yang, C. Luo, Z. Zhao, J. Zhao, Q. Lu, H. Zhang, Q. Kan, Y. Wang, Z. He and J. Sun, Biomaterials, 2020, 257, 120224 CrossRef CAS PubMed.
- F. Weinberg, N. Ramnath and D. Nagrath, Cancers, 2019, 11(8), 1191 CrossRef CAS PubMed.
- K. Omidian, H. Rafiei and B. Bandy, Free Radic. Biol. Med., 2020, 152, 767–775 CrossRef CAS PubMed.
- P. T. Schumacker, Cancer Cell, 2006, 10, 175–176 CrossRef CAS PubMed.
- S. Tan and G. Wang, Drug Des. Dev. Ther., 2017, 11, 3519–3529 CrossRef CAS PubMed.
- J. Wang, X. Sun, W. Mao, W. Sun, J. Tang, M. Sui, Y. Shen and Z. Gu, Adv. Mater., 2013, 25, 3670–3676 CrossRef CAS PubMed.
- J. Zheng, Q. Zeng, R. Zhang, D. Xing and T. Zhang, J. Am. Chem. Soc., 2019, 141, 19226–19230 CrossRef CAS PubMed.
- J. Zheng, Y. Wu, D. Xing and T. Zhang, Nano Res., 2019, 12, 931–938 CrossRef CAS.
- N. Yan, L. Lin, C. Xu, H. Tian and X. Chen, Small, 2019, 15, e1903016 CrossRef PubMed.
- J. A. Cook, D. Gius, D. A. Wink, M. C. Krishna, A. Russo and J. B. Mitchell, Semin. Radiat. Oncol., 2004, 14, 259–266 CrossRef PubMed.
- C. Gorrini, I. S. Harris and T. W. Mak, Nat. Rev. Drug Discovery, 2013, 12, 931–947 CrossRef CAS PubMed.
- A. Russo, W. DeGraff, N. Friedman and J. B. Mitchell, Cancer Res., 1986, 46, 2845–2848 CAS.
- X. Zhong, X. Wang, L. Cheng, Y. A. Tang, G. Zhan, F. Gong, R. Zhang, J. Hu, Z. Liu and X. Yang, Adv. Funct. Mater., 2019, 30, 1907954 CrossRef.
- H. Li, R. Xie, C. Huang, J. He, P. Yang, J. Tao, B. Lin and P. Zhao, Sens. Actuators, B, 2020, 321, 128518 CrossRef CAS.
- Z. Fan, H. Liu, Y. Xue, J. Lin, Y. Fu, Z. Xia, D. Pan, J. Zhang, K. Qiao, Z. Zhang and Y. Liao, Bioact. Mater., 2021, 6, 312–325 CrossRef CAS PubMed.
- B. Hosnedlova, M. Kepinska, S. Skalickova, C. Fernandez, B. Ruttkay-Nedecky, Q. Peng, M. Baron, M. Melcova, R. Opatrilova, J. Zidkova, G. Bjorklund, J. Sochor and R. Kizek, Int. J. Nanomed., 2018, 13, 2107–2128 CrossRef CAS PubMed.
- R. Ghosh Chaudhuri and S. Paria, Chem. Rev., 2012, 112, 2373–2433 CrossRef CAS PubMed.
- H. T. Nguyen, J. H. Byeon, C. D. Phung, L. M. Pham, S. K. Ku, C. S. Yong and J. O. Kim, ACS Appl. Mater. Interfaces, 2019, 11, 24959–24970 CrossRef CAS PubMed.
- N. S. Nicholas, B. Apollonio and A. G. Ramsay, Biochim. Biophys. Acta, 2016, 1863, 471–482 CrossRef CAS PubMed.
- L. Wang, K. Ding, C. Zheng, H. Xiao, X. Liu, L. Sun, R. Omer, Q. Feng and Z. Zhang, Nano Lett., 2020, 20, 6272–6280 CrossRef CAS PubMed.
- M. Wang, J. Zhao, L. Zhang, F. Wei, Y. Lian, Y. Wu, Z. Gong, S. Zhang, J. Zhou, K. Cao, X. Li, W. Xiong, G. Li, Z. Zeng and C. Guo, J. Cancer, 2017, 8, 761–773 CrossRef CAS PubMed.
- D. A. Senthebane, A. Rowe, N. E. Thomford, H. Shipanga, D. Munro, M. Mazeedi, H. A.-M. Almazyadi, K. Kallmeyer, C. Dandara, M. S. Pepper, M. I. Parker and K. Dzobo, Int. J. Mol. Sci., 2017, 18(7), 1586 CrossRef PubMed.
- Q. Yao, L. Kou, Y. Tu and L. Zhu, Trends Pharmacol. Sci., 2018, 39, 766–781 CrossRef CAS PubMed.
-
D. Barisano and M. A. Frohman, in Tumor Microenvironment: Molecular Players – Part A, ed. A. Birbrair, Springer International Publishing, Cham, 2020, pp. 77–87 DOI:10.1007/978-3-030-43093-1_5.
- Z. Peng, Y. Chang, J. Fan, W. Ji and C. Su, Cancer Lett., 2021, 497, 165–177 CrossRef CAS PubMed.
- J. R. Hernandez-Fernaud, E. Ruengeler, A. Casazza, L. J. Neilson, E. Pulleine, A. Santi, S. Ismail, S. Lilla, S. Dhayade, I. R. MacPherson, I. McNeish, D. Ennis, H. Ali, F. G. Kugeratski, H. Al Khamici, M. van den Biggelaar, P. V.-E. van den Berghe, C. Cloix, L. McDonald, D. Millan, A. Hoyle, A. Kuchnio, P. Carmeliet, S. M. Valenzuela, K. Blyth, H. Yin, M. Mazzone, J. C. Norman and S. Zanivan, Nat. Commun., 2017, 8, 14206 CrossRef CAS PubMed.
- A. Barve, A. Jain, H. Liu, Z. Zhao and K. Cheng, Acta Biomater., 2020, 113, 501–511 CrossRef CAS PubMed.
- X. Du, Y. Bi, P. He, C. Wang and W. Guo, Adv. Funct. Mater., 2020, 2006305 CrossRef CAS.
- J. Mu, J. Lin, P. Huang and X. Chen, Chem. Soc. Rev., 2018, 47, 5554–5573 RSC.
- M. Shahriari, M. Zahiri, K. Abnous, S. M. Taghdisi, M. Ramezani and M. Alibolandi, J. Controlled Release, 2019, 308, 172–189 CrossRef CAS PubMed.
- J. Hu, G. Zhang and S. Liu, Chem. Soc. Rev., 2012, 41, 5933–5949 RSC.
- Y. Zhou, S. Liu, M. Zhao, C. Wang, L. Li, Y. Yuan, L. Li, G. Liao, W. Bresette, J. Zhang, Y. Chen, J. Cheng, Y. Lu and J. Liu, J. Controlled Release, 2019, 316, 93–104 CrossRef CAS PubMed.
- Z. T. Zhang, M. Y. Huang-Fu, W. H. Xu and M. Han, Eur. J. Pharm. Biopharm., 2019, 137, 122–130 CrossRef CAS PubMed.
- F. Fouladi, K. J. Steffen and S. Mallik, Bioconjugate Chem., 2017, 28, 857–868 CrossRef CAS PubMed.
- J. Lu, G. Getz, E. A. Miska, E. Alvarez-Saavedra, J. Lamb, D. Peck, A. Sweet-Cordero, B. L. Ebert, R. H. Mak, A. A. Ferrando, J. R. Downing, T. Jacks, H. R. Horvitz and T. R. Golub, Nature, 2005, 435, 834–838 CrossRef CAS PubMed.
- S. K. Penland, T. O. Keku, C. Torrice, X. He, J. Krishnamurthy, K. A. Hoadley, J. T. Woosley, N. E. Thomas, C. M. Perou, R. S. Sandler and N. E. Sharpless, Lab. Invest., 2007, 87, 383–391 CrossRef CAS PubMed.
- X.-N. Li, Z.-J. Wang, C.-X. Ye, B.-C. Zhao, Z.-L. Li and Y. Yang, J. Exp. Clin. Cancer Res., 2018, 37, 325 CrossRef CAS PubMed.
- S. Wu, K. M. Turner, N. Nguyen, R. Raviram, M. Erb, J. Santini, J. Luebeck, U. Rajkumar, Y. Diao, B. Li, W. Zhang, N. Jameson, M. R. Corces, J. M. Granja, X. Chen, C. Coruh, A. Abnousi, J. Houston, Z. Ye, R. Hu, M. Yu, H. Kim, J. A. Law, R. G.-W. Verhaak, M. Hu, F. B. Furnari, H. Y. Chang, B. Ren, V. Bafna and P. S. Mischel, Nature, 2019, 575, 699–703 CrossRef CAS PubMed.
- Q. Hu, H. Li, L. Wang, H. Gu and C. Fan, Chem. Rev., 2019, 119, 6459–6506 CrossRef CAS PubMed.
- G. Qiao, L. Zhuo, Y. Gao, L. Yu, N. Li and B. Tang, Chem. Commun., 2011, 47, 7458–7460 RSC.
- L. M. Kranz, M. Diken, H. Haas, S. Kreiter, C. Loquai, K. C. Reuter, M. Meng, D. Fritz, F. Vascotto, H. Hefesha, C. Grunwitz, M. Vormehr, Y. Hüsemann, A. Selmi, A. N. Kuhn, J. Buck, E. Derhovanessian, R. Rae, S. Attig, J. Diekmann, R. A. Jabulowsky, S. Heesch, J. Hassel, P. Langguth, S. Grabbe, C. Huber, Ö. Türeci and U. Sahin, Nature, 2016, 534, 396–401 CrossRef PubMed.
- Y. Li, X. Teng, K. Zhang, R. Deng and J. Li, Anal. Chem., 2019, 91, 3989–3996 CrossRef CAS PubMed.
- P. Gao, X. Shen, X. Liu, Y. Chen, W. Pan, N. Li and B. Tang, Anal. Chem., 2021, 93, 11751–11757 CrossRef CAS PubMed.
- J. S. Lee, S. Kim, H. K. Na and D. H. Min, Adv. Healthcare Mater., 2016, 5, 2386–2395 CrossRef CAS PubMed.
- S. Mohamed M, S. Veeranarayanan, T. Maekawa and S. Kumar D, Adv. Drug Delivery Rev., 2019, 138, 18–40 CrossRef PubMed.
- G. Bai, P. Yuan, B. Cai, X. Qiu, R. Jin, S. Liu, Y. Li and X. Chen, Adv. Funct. Mater., 2019, 29, 1904401 CrossRef.
- U. Kauscher, M. N. Holme, M. Bjornmalm and M. M. Stevens, Adv. Drug Delivery Rev., 2019, 138, 259–275 CrossRef CAS PubMed.
- S. Mitragotri, Nat. Rev. Drug Discovery, 2005, 4, 255–260 CrossRef CAS PubMed.
- S. H. Pham, Y. Choi and J. Choi, Pharmaceutics, 2020, 12(7), 630 CrossRef CAS PubMed.
- L. Cheng, Y. Wang and L. Huang, Mol. Ther., 2017, 25, 1665–1675 CrossRef CAS PubMed.
- W. S. Toh, R. C. Lai, J. H.-P. Hui and S. K. Lim, Semin. Cell Dev. Biol., 2017, 67, 56–64 CrossRef CAS PubMed.
- J. Park, H. Lee, Y. S. Youn, K. T. Oh and E. S. Lee, Pharmaceutics, 2020, 12(4), 372 CrossRef CAS PubMed.
- S. Kamerkar, V. S. LeBleu, H. Sugimoto, S. Yang, C. F. Ruivo, S. A. Melo, J. J. Lee and R. Kalluri, Nature, 2017, 546, 498–503 CrossRef CAS PubMed.
- Q. Lv, L. Cheng, Y. Lu, X. Zhang, Y. Wang, J. Deng, J. Zhou, B. Liu and J. Liu, Adv. Sci., 2020, 7, 2000515 CrossRef CAS PubMed.
- C. L. Tan and Z. A. Knight, Neuron, 2018, 98, 31–48 CrossRef CAS PubMed.
- M. W. Dewhirst, Z. Vujaskovic, E. Jones and D. Thrall, Int. J. Hypertherm., 2005, 21, 779–790 CrossRef PubMed.
- M. M. Mato, L. M. Casás, J. L. Legido, C. Gómez, L. Mourelle, D. Bessières and F. Plantier, J. Therm. Anal. Calorim., 2017, 130, 479–484 CrossRef CAS.
- E. Franciosi, L. Narduzzi, A. Paradiso, S. Carlin, K. Tuohy, A. Beretta and F. Mattivi, Sci. Rep., 2020, 10, 17931 CrossRef CAS PubMed.
-
S. Mondal, A. Sur and M. Kanoria, Waves in Random and Complex Media, 2020, pp. 1–20 DOI:10.1080/17455030.2020.1779388.
- N. S. Abadeer and C. J. Murphy, J. Phys. Chem. C, 2016, 120, 4691–4716 CrossRef CAS.
- E. A. Kaye, F. H. Cornelis, E. N. Petre, N. Tyagi, W. Shady, W. Shi, Z. Zhang, S. B. Solomon, C. T. Sofocleous and J. C. Durack, Eur. Radiol., 2019, 29, 2698–2705 CrossRef PubMed.
- A. Sanchez, A. S. Feldman and A. A. Hakimi, J. Clin. Oncol., 2018, 36, 3591–3600 CrossRef PubMed.
- N. Yang, W. Li, F. Gong, L. Cheng, Z. Dong, S. Bai, Z. Xiao, C. Ni and Z. Liu, Small Methods, 2020, 4, 2000147 CrossRef CAS.
- Y.-Y. Xiao, X.-L. Gong, Y. Kang, Z.-C. Jiang, S. Zhang and B.-J. Li, Chem. Commun., 2016, 52, 10609–10612 RSC.
- S. Zhang, C. Cao, X. Lv, H. Dai, Z. Zhong, C. Liang, W. Wang, W. Huang, X. Song and X. Dong, Chem. Sci., 2020, 11, 1926–1934 RSC.
- Y. Ke, C. Zhou, Y. Zhou, S. Wang, S. H. Chan and Y. Long, Adv. Funct. Mater., 2018, 28, 1800113 CrossRef.
- H. Yang, W. R. Leow and X. Chen, Adv. Mater., 2018, 30, 1704347 CrossRef PubMed.
- H. S. O'Neill, C. C. Herron, C. L. Hastings, R. Deckers, A. Lopez Noriega, H. M. Kelly, W. E. Hennink, C. O. McDonnell, F. J. O'Brien, E. Ruiz-Hernandez and G. P. Duffy, Acta Biomater., 2017, 48, 110–119 CrossRef PubMed.
- F. Liu, S. Zhang, Y. Meng and B. Tang, Small, 2020, 16, 2002319 CrossRef CAS PubMed.
- Y. T. Sato, K. Umezaki, S. Sawada, S.-A. Mukai, Y. Sasaki, N. Harada, H. Shiku and K. Akiyoshi, Sci. Rep., 2016, 6, 21933 CrossRef CAS PubMed.
- B. You, W. Xu and B. Zhang, Am. J. Cancer Res., 2018, 8, 1332–1342 CAS.
- A. Montecalvo, A. T. Larregina, W. J. Shufesky, D. Beer Stolz, M. L.-G. Sullivan, J. M. Karlsson, C. J. Baty, G. A. Gibson, G. Erdos, Z. Wang, J. Milosevic, O. A. Tkacheva, S. J. Divito, R. Jordan, J. Lyons-Weiler, S. C. Watkins and A. E. Morelli, Blood, 2012, 119, 756–766 CrossRef CAS PubMed.
- F. Beretti, M. Zavatti, F. Casciaro, G. Comitini, F. Franchi, V. Barbieri, G. B. La Sala and T. Maraldi, BioFactors, 2018, 44, 158–167 CrossRef CAS PubMed.
- Z. Sun, K. Shi, S. Yang, J. Liu, Q. Zhou, G. Wang, J. Song, Z. Li, Z. Zhang and W. Yuan, Mol. Cancer, 2018, 17, 147 CrossRef PubMed.
- B. Mead and S. Tomarev, Stem Cells Transl. Med., 2017, 6, 1273–1285 CrossRef CAS PubMed.
- E. Iranifar, B. M. Seresht, F. Momeni, E. Fadaei, M. H. Mehr, Z. Ebrahimi, M. Rahmati, E. Kharazinejad and H. Mirzaei, J. Cell. Physiol., 2019, 234, 2296–2305 CrossRef CAS PubMed.
- J. Lv, S. Li, J. Zhang, F. Duan, Z. Wu, R. Chen, M. Chen, S. Huang, H. Ma and L. Nie, Theranostics, 2020, 10, 816–828 CrossRef CAS PubMed.
- O. Betzer, E. Barnoy, T. Sadan, I. Elbaz, C. Braverman, Z. Liu and R. Popovtzer, Wiley Interdiscip. Rev.: Nanomed. Nanobiotechnol., 2020, 12, e1594 Search PubMed.
- O. Betzer, N. Perets, A. Angel, M. Motiei, T. Sadan, G. Yadid, D. Offen and R. Popovtzer, ACS Nano, 2017, 11, 10883–10893 CrossRef CAS PubMed.
- T. Zuo, J. Li, J. Zhang, L. Sun, X. Liang, J. Yang and Q. Shen, Biomater. Sci., 2019, 7, 5054–5067 RSC.
- Y. Yu, Q. Xu, S. He, H. Xiong, Q. Zhang, W. Xu, V. Ricotta, L. Bai, Q. Zhang, Z. Yu, J. Ding, H. Xiao and D. Zhou, Coord. Chem. Rev., 2019, 387, 154–179 CrossRef CAS.
- C. Lu, Z. Fan and D. Xing, Int. J. Biochem. Cell Biol., 2016, 78, 206–216 CrossRef CAS PubMed.
- J. Lee, K. H. Ku, J. Kim, Y. J. Lee, S. G. Jang and B. J. Kim, J. Am. Chem. Soc., 2019, 141, 15348–15355 CrossRef CAS PubMed.
- H. Chang, Z. Zou, J. Li, Q. Shen, L. Liu, X. An, S. Yang and D. Xing, Cancer Lett., 2021, 523, 57–71 CrossRef CAS PubMed.
- Q. Wang, H. Chang, Q. Shen, Y. Li and D. Xing, J. Thromb. Haemost., 2021, 19, 2029–2043 CrossRef CAS PubMed.
- G. Liu and C.-M. Dong, Biomacromolecules, 2012, 13, 1573–1583 CrossRef CAS PubMed.
- A. Y. Rwei, W. Wang and D. S. Kohane, Nano Today, 2015, 10, 451–467 CrossRef CAS PubMed.
- T. Zhao, L. Chen, Q. Li and X. Li, J. Mater. Chem. B, 2018, 6, 7112–7121 RSC.
- J. Zhao, D. Zhong and S. Zhou, J. Mater. Chem. B, 2018, 6, 349–365 RSC.
- G. Hong, A. L. Antaris and H. Dai, Nat. Biomed. Eng., 2017, 1, 0010 CrossRef CAS.
- F. Ding, Y. Zhan, X. Lu and Y. Sun, Chem. Sci., 2018, 9, 4370–4380 RSC.
- X. M.-M. Weyel, M. A.-H. Fichte and A. Heckel, ACS Chem. Biol., 2017, 12, 2183–2190 CrossRef CAS PubMed.
- Y. Wu, J. Zheng, Q. Zeng, T. Zhang and D. Xing, Nano Res., 2020, 13, 2399–2406 CrossRef CAS.
- Y. Zhou, H. Ye, Y. Chen, R. Zhu and L. Yin, Biomacromolecules, 2018, 19, 1840–1857 CrossRef CAS PubMed.
- M. Mathiyazhakan, C. Wiraja and C. Xu, Nanomicro Lett., 2018, 10, 10 Search PubMed.
- S. Luo, E. Zhang, Y. Su, T. Cheng and C. Shi, Biomaterials, 2011, 32, 7127–7138 CrossRef CAS PubMed.
- A. Haque, M. S.-H. Faizi, J. A. Rather and M. S. Khan, Bioorg. Med. Chem., 2017, 25, 2017–2034 CrossRef CAS PubMed.
- V. F. Cardoso, A. Francesko, C. Ribeiro, M. Banobre-Lopez, P. Martins and S. Lanceros-Mendez, Adv. Healthcare Mater., 2018, 7(5), 1700845 CrossRef PubMed.
- Y. Wan, G. Cheng, X. Liu, S.-J. Hao, M. Nisic, C.-D. Zhu, Y.-Q. Xia, W.-Q. Li, Z.-G. Wang, W.-L. Zhang, S. J. Rice, A. Sebastian, I. Albert, C. P. Belani and S.-Y. Zheng, Nat. Biomed. Eng., 2017, 1, 0058 CrossRef CAS PubMed.
- R. Damadian, Science, 1971, 171, 1151–1153 CrossRef CAS PubMed.
- M. G. Christiansen, C. M. Howe, D. C. Bono, D. J. Perreault and P. Anikeeva, Rev. Sci. Instrum., 2017, 88, 084301 CrossRef PubMed.
- J. N. Pooam, M. El Esawi, R. M. Sherrard and M. Ahmad, PLoS One, 2020, 15, e0243038 CrossRef PubMed.
- Y. Guan, C. Jiang, C. Hu and L. Jia, Talanta, 2010, 83, 337–343 CrossRef CAS PubMed.
- J. L. Corchero and A. Villaverde, Trends Biotechnol., 2009, 27, 468–476 CrossRef CAS PubMed.
- S. Xu, C. Jiang, Y. Lin and L. Jia, Microchim. Acta, 2012, 179, 257–264 CrossRef CAS.
- G. Traini, A. Ruiz-de-Angulo, J. B. Blanco-Canosa, K. Zamacola Bascaran, A. Molinaro, A. Silipo, D. Escors and J. C. Mareque-Rivas, Small, 2019, 15, e1803993 CrossRef PubMed.
- K. Lv, P. Wang, C. Wang, Z. Shen, Z. Lu, H. Zhang, M. Zheng, P. He and H. Zhou, Small, 2020, 16, e2000870 CrossRef PubMed.
- J.-P. Fortin, F. Gazeau and C. Wilhelm, Eur. Biophys. J., 2008, 37, 223–228 CrossRef CAS PubMed.
- R. Hergt, S. Dutz and M. Röder, J. Phys.: Condens. Matter, 2008, 20, 385214 CrossRef PubMed.
- X. Wang, X. Zhong, L. Bai, J. Xu, F. Gong, Z. Dong, Z. Yang, Z. Zeng, Z. Liu and L. Cheng, J. Am. Chem. Soc., 2020, 142, 6527–6537 CrossRef CAS PubMed.
- X. Wang, X. Zhong, F. Gong, Y. Chao and L. Cheng, Mater. Horiz., 2020, 7, 2028–2046 RSC.
- Y. Luo, Y. Song, M. Wang, T. Jian, S. Ding, P. Mu, Z. Liao, Q. Shi, X. Cai, H. Jin, D. Du, W. J. Dong, C. L. Chen and Y. Lin, Small, 2019, 15, e1902485 CrossRef PubMed.
- B. P. Mead, C. T. Curley, N. Kim, K. Negron, W. J. Garrison, J. Song, D. Rao, G. W. Miller, J. W. Mandell, B. W. Purow, J. S. Suk, J. Hanes and R. J. Price, Small, 2019, 15, e1903460 CrossRef PubMed.
- X. Wang, X. Wang, X. Zhong, G. Li, Z. Yang, Y. Gong, Z. Liu and L. Cheng, Appl. Phys. Rev., 2020, 7, 041411 CAS.
- Y. Liu, L. Bai, K. Guo, Y. Jia, K. Zhang, Q. Liu, P. Wang and X. Wang, Theranostics, 2019, 9, 5261–5281 CrossRef CAS PubMed.
- Q. Zhang, Q. Xiao, H. Yin, C. Xia, Y. Pu, Z. He, Q. Hu, J. Wang and Y. Wang, RSC Adv., 2020, 10, 28314–28323 RSC.
- N. S. Ihrcke, W. Parker, K. J. Reissner and J. L. Platt, J. Cell. Physiol., 1998, 175, 255–267 CrossRef CAS PubMed.
- I. Ichimonji, H. Tomura, C. Mogi, K. Sato, H. Aoki, T. Hisada, K. Dobashi, T. Ishizuka, M. Mori and F. Okajima, Am. J. Physiol.: Lung Cell. Mol. Physiol., 2010, 299, L567–L577 CrossRef CAS PubMed.
- S. Grinstein, C. J. Swallow and O. D. Rotstein, Clin. Biochem., 1991, 24, 241–247 CrossRef CAS PubMed.
- C. Wang, M. Wang, T. Xu, X. Zhang, C. Lin, W. Gao, H. Xu, B. Lei and C. Mao, Theranostics, 2019, 9, 65–76 CrossRef CAS PubMed.
- H. Ding, Y. Cai, L. Gao, M. Liang, B. Miao, H. Wu, Y. Liu, N. Xie, A. Tang, K. Fan, X. Yan and G. Nie, Nano Lett., 2019, 19, 203–209 CrossRef CAS PubMed.
- K. Liang, F. Liu, J. Fan, D. Sun, C. Liu, C. J. Lyon, D. W. Bernard, Y. Li, K. Yokoi, M. H. Katz, E. J. Koay, Z. Zhao and Y. Hu, Nat. Biomed. Eng., 2017, 1(4), 1–11 Search PubMed.
- N. L. Syn, L. Wang, E. K. Chow, C. T. Lim and B. C. Goh, Trends Biotechnol., 2017, 35, 665–676 CrossRef CAS PubMed.
- F. Andre, N. E. Schartz, M. Movassagh, C. Flament, P. Pautier, P. Morice, C. Pomel, C. Lhomme, B. Escudier, T. Le Chevalier, T. Tursz, S. Amigorena, G. Raposo, E. Angevin and L. Zitvogel, Lancet, 2002, 360, 295–305 CrossRef CAS.
- G. Andreola, L. Rivoltini, C. Castelli, V. Huber, P. Perego, P. Deho, P. Squarcina, P. Accornero, F. Lozupone, L. Lugini, A. Stringaro, A. Molinari, G. Arancia, M. Gentile, G. Parmiani and S. Fais, J. Exp. Med., 2002, 195, 1303–1316 CrossRef CAS PubMed.
- Y. Shi, J. Zhang, Z. Mao, H. Jiang, W. Liu, H. Shi, R. Ji, W. Xu, H. Qian and X. Zhang, Front. Oncol., 2020, 10, 629 CrossRef PubMed.
- K. K. Jella, T. H. Nasti, Z. Li, S. R. Malla, Z. S. Buchwald and M. K. Khan, Vaccines, 2018, 6(4), 69 CrossRef CAS PubMed.
- Y. Liu, Y. Gu and X. Cao, Oncoimmunology, 2015, 4, e1027472 CrossRef PubMed.
- L. Dou, X. Shi, X. He and Y. Gao, Front. Immunol., 2019, 10, 3112 CrossRef CAS PubMed.
- J. S. Duffield, S. J. Forbes, C. M. Constandinou, S. Clay, M. Partolina, S. Vuthoori, S. Wu, R. Lang and J. P. Iredale, J. Clin. Invest., 2005, 115, 56–65 CrossRef CAS PubMed.
- G. H. Nam, Y. Choi, G. B. Kim, S. Kim, S. A. Kim and I. S. Kim, Adv. Mater., 2020, 32, e2002440 CrossRef PubMed.
- S. A.-A. Kooijmans, O. G. de Jong and R. M. Schiffelers, Adv. Drug Delivery Rev., 2021, 173, 252–278 CrossRef CAS PubMed.
- M. Simons and G. Raposo, Curr. Opin. Cell Biol., 2009, 21, 575–581 CrossRef CAS PubMed.
- M. Dragomir, B. Chen and G. A. Calin, Transl. Cancer Res., 2018, 7, S243–S252 CrossRef CAS PubMed.
- N. Regev-Rudzki, D. W. Wilson, T. G. Carvalho, X. Sisquella, B. M. Coleman, M. Rug, D. Bursac, F. Angrisano, M. Gee, A. F. Hill, J. Baum and A. F. Cowman, Cell, 2013, 153, 1120–1133 CrossRef CAS PubMed.
- T. Umezu, K. Ohyashiki, M. Kuroda and J. H. Ohyashiki, Oncogene, 2013, 32, 2747–2755 CrossRef CAS PubMed.
- I. M. Bjorge, S. Y. Kim, J. F. Mano, B. Kalionis and W. Chrzanowski, Biomater. Sci., 2017, 6, 60–78 RSC.
- W. C. Newton, J. W. Kim, J. Z.-Q. Luo and L. Luo, J. Mol. Endocrinol., 2017, 59, R155–R165 CAS.
- P. Mathiyalagan, Y. Liang, D. Kim, S. Misener, T. Thorne, E. Kamide Christine, E. Klyachko, W. Losordo Douglas, J. Hajjar Roger and S. Sahoo, Circ. Res., 2017, 120, 1466–1476 CrossRef CAS PubMed.
- Y. Dong, J. Wang, X. Guo, S. Yang, M. O. Ozen, P. Chen, X. Liu, W. Du, F. Xiao, U. Demirci and B.-F. Liu, Nat. Commun., 2019, 10, 4087 CrossRef PubMed.
- J. Zhuang, M. R. Gordon, J. Ventura, L. Li and S. Thayumanavan, Chem. Soc. Rev., 2013, 42, 7421–7435 RSC.
- X. Fu, L. Hosta-Rigau, R. Chandrawati and J. Cui, Chem, 2018, 4, 2084–2107 CAS.
- X. Yi, W. Zeng, C. Wang, Y. Chen, L. Zheng, X. Zhu, Y. Ke, X. He, Y. Kuang and Q. Huang, Nano Res., 2021, 15, 1205–1212 CrossRef.
- F. S. Collins and S. Gottlieb, N. Engl. J. Med., 2018, 379, 1393–1395 CrossRef PubMed.
- D. Wang, P. W.-L. Tai and G. Gao, Nat. Rev. Drug Discovery, 2019, 18, 358–378 CrossRef CAS PubMed.
- M. Cavazzana, F. D. Bushman, A. Miccio, I. André-Schmutz and E. Six, Nat. Rev. Drug Discovery, 2019, 18, 447–462 CrossRef CAS PubMed.
- R. Chen, H. Huang, H. Liu, J. Xi, J. Ning, W. Zeng, C. Shen, T. Zhang, G. Yu, Q. Xu, X. Chen, J. Wang and F. Lu, Small, 2019, 15, e1902686 CrossRef PubMed.
- S. M. Kim, Y. Yang, S. J. Oh, Y. Hong, M. Seo and M. Jang, J. Controlled Release, 2017, 266, 8–16 CrossRef CAS PubMed.
- L. M. Wallace, N. Y. Saad, N. K. Pyne, A. M. Fowler, J. O. Eidahl, J. S. Domire, D. A. Griffin, A. C. Herman, Z. Sahenk, L. R. Rodino-Klapac and S. Q. Harper, Mol. Ther. Methods Clin. Dev., 2018, 8, 121–130 CrossRef CAS PubMed.
- D. Sinha, B. Steyer, P. K. Shahi, K. P. Mueller, R. Valiauga, K. L. Edwards, C. Bacig, S. S. Steltzer, S. Srinivasan, A. Abdeen, E. Cory, V. Periyasamy, A. F. Siahpirani, E. M. Stone, B. A. Tucker, S. Roy, B. R. Pattnaik, K. Saha and D. M. Gamm, Am. J. Hum. Genet., 2020, 107, 278–292 CrossRef CAS PubMed.
- P. Akcakaya, M. L. Bobbin, J. A. Guo, J. Malagon-Lopez, K. Clement, S. P. Garcia, M. D. Fellows, M. J. Porritt, M. A. Firth, A. Carreras, T. Baccega, F. Seeliger, M. Bjursell, S. Q. Tsai, N. T. Nguyen, R. Nitsch, L. M. Mayr, L. Pinello, M. Bohlooly-Y, M. J. Aryee, M. Maresca and J. K. Joung, Nature, 2018, 561, 416–419 CrossRef CAS PubMed.
- L. Galluzzi, T. A. Chan, G. Kroemer, J. D. Wolchok and A. Lopez-Soto, Sci. Transl. Med., 2018, 10(459), eaat7807 CrossRef PubMed.
- S. K. Wculek, F. J. Cueto, A. M. Mujal, I. Melero, M. F. Krummel and D. Sancho, Nat. Rev. Immunol., 2020, 20, 7–24 CrossRef CAS PubMed.
- M. S. Goldberg, Nat. Rev. Cancer, 2019, 19, 587–602 CrossRef CAS PubMed.
- Y. W. Choo, M. Kang, H. Y. Kim, J. Han, S. Kang, J.-R. Lee, G.-J. Jeong, S. P. Kwon, S. Y. Song, S. Go, M. Jung, J. Hong and B.-S. Kim, ACS Nano, 2018, 12, 8977–8993 CrossRef CAS PubMed.
- I. Vitale, G. Manic, L. M. Coussens, G. Kroemer and L. Galluzzi, Cell Metab., 2019, 30, 36–50 CrossRef CAS PubMed.
- D. V.-F. Tauriello and E. Batlle, Trends Cancer, 2016, 2, 495–504 CrossRef PubMed.
- D. Gurusamy, D. Clever, R. Eil and N. P. Restifo, Cancer Immunol. Res., 2017, 5, 426 CrossRef PubMed.
- E. A. Ashley, Nat. Rev. Genet., 2016, 17, 507–522 CrossRef CAS PubMed.
- R. Hodson, Nature, 2016, 537, S49 CrossRef CAS PubMed.
- P. Hassanzadeh, F. Atyabi and R. Dinarvand, Adv. Drug Delivery Rev., 2019, 151–152, 169–190 CrossRef CAS PubMed.
- K. A. Brown, S. Brittman, N. Maccaferri, D. Jariwala and U. Celano, Nano Lett., 2020, 20, 2–10 CrossRef CAS PubMed.
- K. T. Butler, D. W. Davies, H. Cartwright, O. Isayev and A. Walsh, Nature, 2018, 559, 547–555 CrossRef CAS PubMed.
- D. S. Char, N. H. Shah and D. Magnus, N. Engl. J. Med., 2018, 378, 981–983 CrossRef PubMed.
- B. J. Erickson, P. Korfiatis, Z. Akkus and T. L. Kline, RadioGraphics, 2017, 37, 505–515 CrossRef PubMed.
- O. Adir, M. Poley, G. Chen, S. Froim, N. Krinsky, J. Shklover, J. Shainsky-Roitman, T. Lammers and A. Schroeder, Adv. Mater., 2020, 32, e1901989 CrossRef PubMed.
- P. R. Wiecha, A. Lecestre, N. Mallet and G. Larrieu, Nat. Nanotechnol., 2019, 14, 237–244 CrossRef CAS PubMed.
- R. Yan, T. Wang, X. Jiang, Q. Zhong, X. Huang, L. Wang and X. Yue, Nanotechnology, 2020, 31, 375202 CrossRef CAS PubMed.
- S. C. Glotzer, P. Nordlander and L. E. Fernandez, ACS Nano, 2017, 11, 6505–6506 CrossRef CAS PubMed.
- C. Soekmadji, B. Li, Y. Huang, H. Wang, T. An, C. Liu, W. Pan, J. Chen, L. Cheung, J. M. Falcon-Perez, Y. S. Gho, H. B. Holthofer, M. T.-N. Le, A. Marcilla, L. O'Driscoll, F. Shekari, T. L. Shen, A. C. Torrecilhas, X. Yan, F. Yang, H. Yin, Y. Xiao, Z. Zhao, X. Zou, Q. Wang and L. Zheng, J. Extracell. Vesicles, 2020, 9, 1809766 CrossRef PubMed.
- S.-C. Tao, S.-C. Guo and C.-Q. Zhang, Adv. Sci., 2018, 5, 1700449 CrossRef PubMed.
- X. Li, C. Li, L. Zhang, M. Wu, K. Cao, F. Jiang, D. Chen, N. Li and W. Li, Mol. Cancer, 2020, 19, 1 CrossRef PubMed.
- Y.-J. Li, J.-Y. Wu, J.-M. Wang and D.-X. Xiang, J. Controlled Release, 2020, 320, 105–111 CrossRef CAS PubMed.
- Z. Tang, D. Li, S. Hou and X. Zhu, Int. J. Cancer, 2020, 146, 2946–2959 CrossRef CAS PubMed.
- P. H.-L. Tran, D. Xiang, T. T.-D. Tran, W. Yin, Y. Zhang, L. Kong, K. Chen, M. Sun, Y. Li, Y. Hou, Y. Zhu and W. Duan, Adv. Mater., 2020, 32, 1904040 CrossRef CAS PubMed.
- C. Jiang, G. Wang, R. Hein, N. Liu, X. Luo and J. J. Davis, Chem. Rev., 2020, 120, 3852–3889 CrossRef CAS PubMed.
- Y. Wang, J. Liu, J. Ma, T. Sun, Q. Zhou, W. Wang, G. Wang, P. Wu, H. Wang, L. Jiang, W. Yuan, Z. Sun and L. Ming, Mol.
Cancer, 2019, 18, 116 CrossRef PubMed.
- Y. Zhao, M.-F. Zhao, S. Jiang, J. Wu, J. Liu, X.-W. Yuan, D. Shen, J.-Z. Zhang, N. Zhou, J. He, L. Fang, X.-T. Sun, B. Xue and C.-J. Li, Nat. Commun., 2020, 11, 719 CrossRef CAS PubMed.
- H. Xu, X. Du, J. Xu, Y. Zhang, Y. Tian, G. Liu, X. Wang, M. Ma, W. Du, Y. Liu, L. Dai, W. Huang, N. Tong, Y. Wei and X. Fu, PLoS Biol., 2020, 18, e3000603 CrossRef CAS PubMed.
- H. Song, X. Li, Z. Zhao, J. Qian, Y. Wang, J. Cui, W. Weng, L. Cao, X. Chen, Y. Hu and J. Su, Nano Lett., 2019, 19, 3040–3048 CrossRef CAS PubMed.
Footnote |
† These authors contributed equally. |
|
This journal is © The Royal Society of Chemistry 2022 |
Click here to see how this site uses Cookies. View our privacy policy here.