DOI:
10.1039/D3SC02549G
(Edge Article)
Chem. Sci., 2023,
14, 8109-8118
Polymer up-cycling by mangana-electrocatalytic C(sp3)–H azidation without directing groups†
Received
19th May 2023
, Accepted 4th July 2023
First published on 5th July 2023
Abstract
The chemical up-cycling of polymers into value-added materials offers a unique opportunity to place plastic waste in a new value chain towards a circular economy. Herein, we report the selective up-cycling of polystyrenes and polyolefins to C(sp3)–H azidated materials under electrocatalytic conditions. The functionalized polymers were obtained with high retention of mass average molecular mass and high functionalization through chemo-selective mangana-electrocatalysis. Our strategy proved to be broadly applicable to a variety of homo- and copolymers. Polyethylene, polypropylene as well as post-consumer polystyrene materials were functionalized by this approach, thereby avoiding the use of hypervalent-iodine reagents in stoichiometric quantities by means of electrocatalysis. This study, hence, represents a chemical oxidant-free polymer functionalization by electro-oxidation. The electrocatalysis proved to be scalable, which highlights its unique feature for a green hydrogen economy by means of the hydrogen evolution reaction (HER).
Introduction
Commodity polymers are indispensable in modern society, considering inter alia their broad applications in food packaging, construction materials, and electronics.1 However, the end-of-life fate of post-consumer plastics is a growing concern for modern society as the global production scale is continuously increasing (Fig. 1A).2 Indeed, in 2018 on average less than one fourth of the overall collected polymer waste was recycled (Fig. 1A). The process-related requirement of near-pristine polymer sorts as well as degradation of the polymer chain during the reprocessing yields low grade recycling material in conventional melt and reform strategies (Fig. 1B).3 Chemical recycling can circumvent these issues, yielding monomers for the de novo synthesis of commodity polymers.4 However, energy-intensive protocols are largely required, jeopardizing the practicality of these methods, particularly with respect to the societal demand for a transition to green energy usage. Thus, sustainable alternatives continue to be in high demand to add value to reprocessed polymer waste, and thereby increase the economic incentive for closed loop polymer recycling.
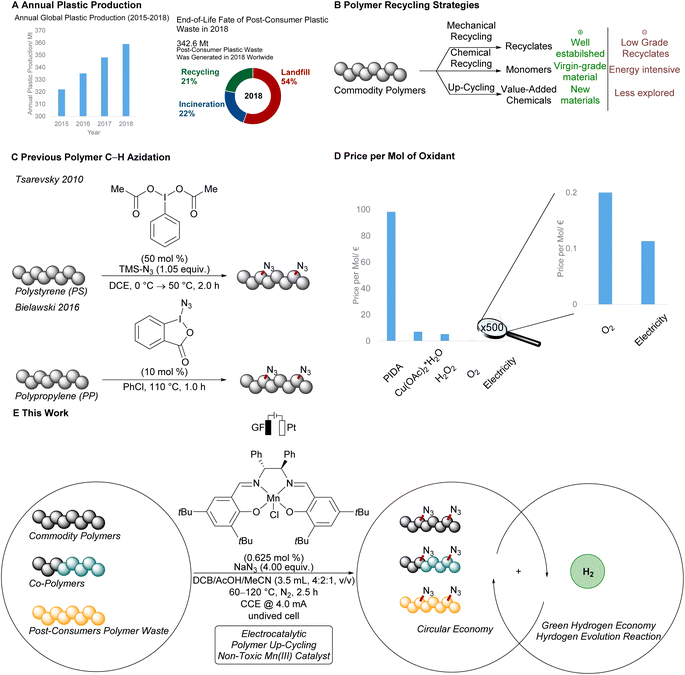 |
| Fig. 1 (A) Annual global plastic production and the waste treatment in the EU in 2018, (B) different approaches to deal with post-consumer polymer waste, (C) previous polymer C–H azidation mediated by hypervalent iodine(III), (D) price per mol in € for various oxidants and (E) this work using electricity as the terminal oxidant. | |
Thus far, up-cycling5 strategies typically rely on either scission of the polymer chain6–9 or modification of the polymer by introduction of functional groups.10–13 Here, the merger of C–H functionalization14–19 and polymer science has witnessed considerable advances by leveraging polymer waste to up-cycled materials.13,20 For instance, Hartwig and Hillmyer reported on the borylation of polypropylene using a rhodium catalyst in a polymer melt.12 Likewise, the oxyfunctionalization of polyethylene,21,22 and polyisobutene10 was accomplished. Under photochemical conditions, Leibfarth and Alexanian devised elegant C–H xanthylation of polyolefins,23,24 and C–H functionalizations via amidyl radicals.25
The azido moiety plays a key role in among others the Staudinger ligation or the 1,3-dipolar cycloaddition for bioorthogonal click reactions.26 In this context, Tsarevsky reported a C–H azidation of polystyrene using stoichiometrically hypervalent iodine (Fig. 1C),27 while Bielawski and Liu used stoichiometric amounts of hypervalent iodine reagents for the mediated azidation of polypropylene.28 Despite this indispensable progress, the use of superstoichiometric amounts of hypervalent iodine reagents significantly reduces the overall sustainability of this approach.
In stark contrast, electrochemistry29–32 has surfaced as an increasingly viable tool to perform C–H functionalizations in a sustainable and cost-effective manner (Fig. 1D).33–45 However, thus far electrosynthetic approaches have proven to be limited to chlorination and cyanation of π-conjugated polymers in the solid film state.46–54 Consequently, we wondered whether metallaelectro-catalyzed C(sp3)–H azidation55 would enable selective electrooxidation for the up-cycling of polymer waste.
As a result, we report on the hypervalent iodine-free mangana-electrocatalyzed post-synthetic azidation on a variety of polymers (Fig. 1E). Notably, the electrocatalytic C–H nitrogenation proved scalable and is operative via cathodic hydrogen evolution reaction (HER), hence featuring a unique potential for scalable anodic oxidation for a green future hydrogen economy.
Results and discussion
Optimization of the reaction conditions
We commenced our studies by evaluating the choice of the metal catalyst under electrochemical conditions using a platinum (Pt) cathode, graphite felt (GF) anode, and polystyrene 1 as the substrate, and sodium azide 2 as being a more readily accessible azide source than previously used TMS-N3 (Table 1). To evaluate the efficiency of the C(sp3)–H azidation two parameters were identified as being of primary relevance. First, the retention of the mass average molar mass (Mw-retention) was considered. Second, the level of functionalization was determined. Thus, a high Mw-retention and high level of functionalization were focused on during the optimization study. The variation of different 3d metal catalysts showed the superior role of manganese complexes as compared to its congeners. The C–H azidation using [Mn1] furnished a modified polystyrene material with a 48% retained mass average molar mass and 1.6 mol% azidation (entry 1–4), the catalytic efficiency being slightly compromised as compared to the C(sp3)–H functionalization of small molecules. Although the level of functionalization was rather promising, the mass average molar mass retention was significantly lower compared to the other 3d metal complexes indicating an accompanied β-scission pathway of the radical intermediate.56
Table 1 Electrochemical azidation of polystyrene 1a
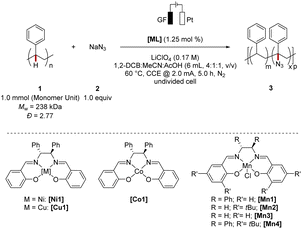
|
Entry |
[ML]
|
Level of functionalizationb/(mol%) |
M
w-retentionc/% |
Đ
|
Catalyst efficiencyd |
Reaction conditions: 1 (1.0 mmol monomer unit), 2 (1.0 equiv.), [ML] (1.25 mol%), LiClO4 (0.17 M), 1,2-DCB (4.0 mL), MeCN (1.0 mL), AcOH (1.0 mL), CCE @ 2.0 mA at 60 °C under N2 for 5.0 h. 1,2-DCB = 1,2-dichlorobenzene, CCE = Constant Current Electrolysis.
Determined by an IR-1H NMR correlation curve (m = 2.7051 mol%, R2 = 0.9942). Relative IR intensities were determined by the ratio of the N3-band (2106 cm−1) to the benzylic C–H band (3026 cm−1).
The mass average molar mass and dispersity (Đ) were determined by analytical GPC against narrow-disperse polystyrene standards using THF as eluent at 30 °C and are reported as mass average molar mass retention of the parent polymer.
Catalyst efficiency = [level of functionalization (mol%)/catalyst loading (mol%)].
|
1 |
[Co1]
|
0.8 |
77 |
2.79 |
0.64 |
2 |
[Ni1]
|
0.8 |
66 |
2.88 |
0.64 |
3 |
[Cu1]
|
1.1 |
66 |
3.11 |
0.88 |
4 |
[Mn1]
|
1.6 |
48 |
2.37 |
1.28 |
5 |
[Mn2]
|
0.8 |
45 |
2.41 |
0.64 |
6 |
[Mn3]
|
1.9 |
65 |
2.65 |
1.52 |
7 |
[Mn4]
|
1.4 |
69 |
2.68 |
1.12 |
We hypothesized whether the deleterious side reaction could be prevented by varying the ligand motif, to thereby achieve a higher Mw-retention, while preserving the remarkable C(sp3)–H functionalization efficiency. Indeed, the substitution pattern exhibited a considerable influence on the Mw-retention (entries 4–7). Notably, high catalytic efficiency in the electrochemical azidation was observed for sterically demanding complex [Mn4] and manganese salen [Mn3]. While the structural simplicity of [Mn3] represents an advantage, we selected [Mn4] for further studies as the chemo-selectivity in terms of suppression of polymer degradation was improved. Furthermore, various metal salts have been probed in the electrochemical up-cycling and confirmed the superior nature of the manganese catalysts (see ESI Table S6†).
With [Mn4] identified as being the optimal catalyst, we probed further reaction parameters (Table S3 and S4†). An excess of sodium azide proved essential to obtain a high Mw-retention (Table S4†). We were delighted to find reaction conditions for high Mw-retention along with a level of functionalization of 1.9 mol% (Table 2, entry 1). Other solvents, such as 1,2-dichloroethane (DCE) or tetrahydrofuran (THF), did not improve the outcome as the increased polymer dispersity Đ indicated masked degradation (entry 2 and 3). Control experiments reflected the essential role of the electricity (entry 4). Thus, the azidation was completely shut down without electricity. Notably, complex [Co1] proved less efficient in the electrochemical C(sp3)–H azidation. Submitting polystyrene to reported electrophotocatalytic small molecule azidation,57 resulted in a high azide incorporation accompanied by significant polymer degradation. Thus, in contrast to the mangana-electrocatalyzed azidation, a very low mass average molar mass retention was observed (entry 7).
Table 2 Optimized reaction conditions for the mangana-electrocatalyzed polystyrene 1 azidationa
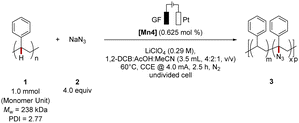
|
Entry |
Deviation from above |
Level of functionalizationb/(mol%) |
M
w-retentionc/% |
Đ
|
Catalyst efficiencyd |
Reaction conditions: 1 (1.0 mmol monomer unit), 2 (4.0 equiv.), [Mn4] (0.625 mol%), LiClO4 (0.17 M), 1,2-DCB (2.0 mL), AcOH (1.0 mL), MeCN (0.5 mL), CCE @ 4.0 mA at 60 °C under N2 for 2.5 h. 1,2-DCB = 1,2-dichlorobenzene, CCE = Constant Current Electrolysis.
Determined by an IR-1H NMR correlation curve (m = 2.7051 mol%, R2 = 0.9942). Relative IR intensities were determined by the ratio of the N3-band (2106 cm−1) to the benzylic C–H band (3026 cm−1).
The mass average molar mass and dispersity (Đ) were determined by analytical GPC against narrow-disperse polystyrene standards using THF as eluent at 30 °C and are reported as mass average molar mass retention of the parent polymer.
Catalyst efficiency = [level of functionalization (mol%)/catalyst loading (mol%)].
See ESI for details, n.o. = not observed.
|
1 |
None |
1.9 |
84 |
2.72 |
3.04 |
2 |
DCE instead of DCB |
2.3 |
81 |
3.37 |
3.68 |
3 |
THF instead of DCB |
0.3 |
96 |
3.34 |
0.48 |
4 |
No electricity |
n.o. |
100 |
2.34 |
— |
5 |
[Co1] instead of [Mn4] |
0.7 |
95 |
2.42 |
1.12 |
6e |
Electrophoto-catalytic conditions |
11.6 |
15 |
4.44 |
1.16 |
Electrode material
Next, we probed the impact of the electrode on the polymer modification (Fig. 2), by examining the optimized mangana-electrocatalyzed polymer up-grading with various carbon-based electrode materials. Reducing the surface area diminished the level of functionalization (Fig. 2). Reticulated vitreous carbon (RVC) – a porous electrode material58 – gave slightly lower levels of functionalization and comparable Mw-retention. We attribute this trend of comparable functionalization to the high specific surface area of the RVC anode. For graphite (G), a lower level of functionalization was observed, mirroring the requirement of a large surface area for efficient C–H azidation. The geometry of the electrode did not significantly alter the outcome, as was demonstrated when using a graphite rod (GR) as anode material. Also, glassy carbon (GC) as well as boron-doped-diamond (BDD) fell short in improving the mangana-electrocatalyzed C(sp3)–H azidation on polystyrene. Control experiments using a glassy carbon (GC) anode at prolonged electrolysis time gave a comparable level of functionalization of 1.5 mol% and Mw-retention of 81% (Table S5, entry 6†). Based on orienting studies and previous findings on mangana-electrocatalyzed small molecule C(sp3)–H azidation,55 we propose the formation of a manganese(IV) diazido species (Fig. 3) through anodic oxidation, along with subsequent hydrogen atom transfer (HAT) and azide transfer, thereby also rationalizing the preferential functionalization at benzylic positions of polystyrene (vide supra). At the cathode, molecular hydrogen is formed through HER.
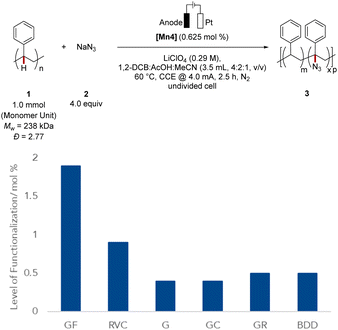 |
| Fig. 2 Variation of carbon-based anode material. Level of functionalization for Graphite Felt (GF), Reticulated Vitreous Carbon (RVC), Glassy Carbon (GC), Graphite (G), Graphite Rod (GR), Boron Doped Diamond (BDD). | |
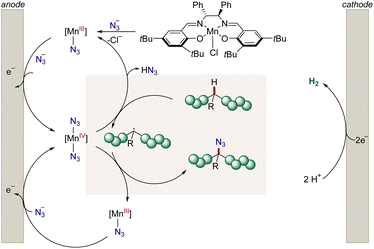 |
| Fig. 3 Proposed mechanism for mangana-electrocatalyzed C–H azidation of commodity polymers. | |
Electrocatalysis robustness
With the optimized reaction conditions in hand, we examined the versatility of the mangana-electrocatalyzed C–H azidation (Scheme 1). First, we probed whether chain scission was hidden by the large polymer dispersity. Thus, we performed the C–H azidation on a narrow-disperse polystyrene (4). Here, we could observe complete Mw-retention (100%) with virtually no change in Đ and comparable high functionalization of 2.1 mol%.
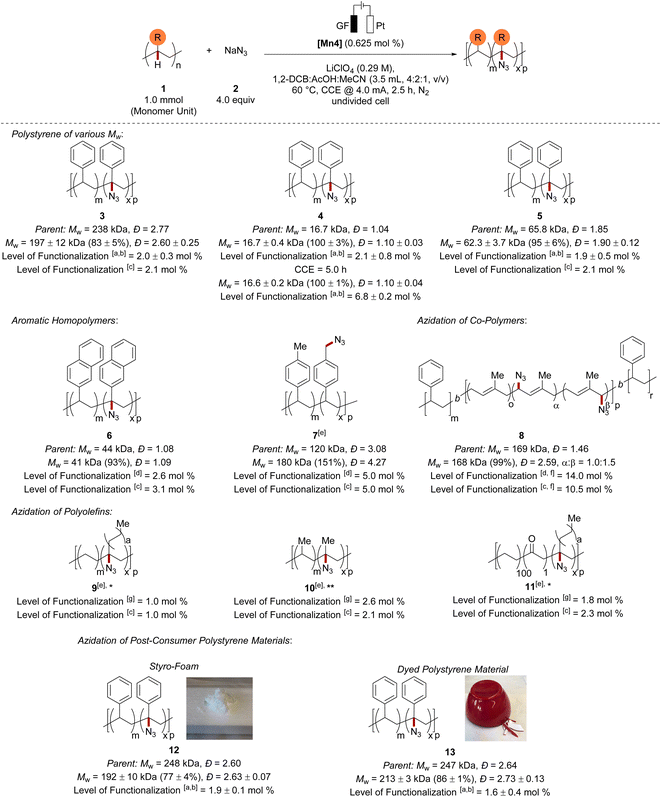 |
| Scheme 1 Scope of the mangana-electrocatalyzed azidation. Standard reaction conditions: 1 (1.0 mmol, 1.0 equiv.), 2 (4.0 mmol, 4.0 equiv.), [Mn4] (6.25 μmol), LiClO4 (0.29 M), 1,2-DCB (2.0 mL), AcOH (1.0 mL), MeCN (0.5 mL), CCE @ 4.0 mA at 60 °C under N2 for 2.5 h. The mass average molar mass and dispersity (Đ) were determined by GPC against narrow-disperse polystyrene standards using THF as eluent at 30 °C and were reported as the molar weight retention of the parent polymer. *Reaction performed at 80 °C. **Reaction performed at 120 °C. aThe level of functionalization was estimated using an IR-1H NMR correlation curve (m = 2.7051 mol%, R2 = 0.9942) and the relative IR intensity of the N3-band (2106 cm−1) to the benzylic C–H band (3026 cm−1) of the polystyrene sample. bResults are reported as average over three runs. cThe level of functionalization was determined by elemental analysis. dThe level of functionalization was determined by 1H NMR analysis of the 1,3-cycloaddition product with methyl propiolate. eFor details on selectivity, see the ESI.†fThe level of functionalization refers to α and β (1.0 : 1.5) in total. gThe level of functionalization was estimated by 1H NMR analysis of the 1,3-cycloaddition product with dimethyl acetylenedicarboxylate. | |
Prolonging the electrolysis time furnished polystyrene material with an increased level of functionalization of 6.8 mol% and a Mw-retention of 100%. However, extending the electrolysis further led to a significant increase of Đ through a competing chain–chain coupling pathway (see Fig. S29 in ESI†). A polystyrene with medium Mw (5) was also efficiently up-cycled and gave a very high Mw-retention of 95% and a similar level of functionalization. To our delight, the electrochemical polymer up-cycling proceeded with a higher efficiency and at the same time a comparable retention of the mass average molar mass in comparison to previously reported hypervalent iodine-mediated azidation on polystyrene27 with stoichiometric chemical oxidants (see Fig. S18 in ESI†). When we subjected a derivative of polystyrene, namely poly(2-vinylnaphthalene), to the mangana-electro C–H azidation, the azidation took site-selectively place at the benzylic C–H bond and furnished 6 with a very high Mw-retention (93%) and high azidation of 2.6 mol%. To our delight, also poly(4-methylstyrene) proved compatible with our strategy, furnishing material 7 with a remarkably high level of functionalization (5.0 mol%).
Here, chain–chain coupling led to an increased mass average molar mass, and Đ being indicative of the formation of cross-linked material. The C–H azidation occurred preferentially on the more readily accessible benzylic methyl moiety of the pendant group rather than at the benzylic C–H of the polymer backbone.59 A styrene-containing copolymer was tolerated in the electrocatalyzed C(sp3)–H functionalization likewise. The mangana-electrocatalyzed C–H azidation of polystyrene-b-polyisoprene-b-polystyrene triblock copolymer delivered the modified polymer 8 with a very high level of functionalization of 14.0 mol%. The azidation of the triblock copolymer preferentially took place on the allylic position of the unsaturated moieties at the polyisoprene block.60 Although the Mw was efficiently retained, an increased Đ indicated accompanied radical–radical recombination. It is particularly noteworthy that our strategy was not limited to polystyrenes, but also challenging polyethylene and polypropylene were functionalized with high efficiency. We were delighted to observe no loss in reactivity furnishing azidated polyethylene 9 (ref. 61) and polypropylene 10.61 The challenging nature of polyethylene25 and polypropylene28 azidation is mirrored in thus far reported catalyst efficiencies of 0.40 and 0.38, respectively. In stark contrast, the mangana-electrocatalyzed C–H nitrogenation proved remarkably efficient for polyethylene and polypropylene azidation with a catalyst efficiency of 1.60 and 4.16, respectively. Moreover, ketone functionalized polyethylene was also suitable in this transformation providing access to the azide functionalized polyethylene 11 with a total functionalization of 2.3 mol% (3°:2° = 3.6
:
1.0) (Scheme 1).61
With the scope of well-defined polymer sources being established, we investigated challenging post-consumer polystyrene materials containing unknown additives and pigments. Here, additives in styrofoam as well as pigments of a polystyrene-based bowl did not suppress the reactivity and gave functionalized polystyrene materials 12 and 13, highlighting the applicability for polymer up-cycling.
Thermal and mechanical properties
To determine the impact of the C(sp3)–H functionalization on the physical properties of the thus obtained polymeric material, we performed differential scanning calorimetry (DSC) and thermogravimetric analysis (TGA) (Table 3). While only minor changes of the glass transition temperature (Tg) was observed for azidated polymers (Table 3, entries 1 to 3), the incorporation of a triazole moiety increased the Tg (entry 4), thus, highlighting the potential of the azide moiety as synthetic handle for late-stage diversification. Interestingly, an initial weight loss at 250 °C, accounting for a loss of the azide was observed for polymer 4 (see ESI† for details). The mechanical properties were not significantly altered through azidation as was shown in a static material testing, with a modulus of 1014 MPa for 12 compared to 1122 MPa of the parent polymer. Overall, the azide functionalized polymers showed comparable mechanical stability as the parent material, enabling late-stage diversification with azides as synthetically useful handle. Furthermore, the lower degradation temperature (Tdeg) of the azidated polymer will allow easier degradation after the end use of this material.
Table 3
T
g and Tdeg of polymer 4 and 15
Entry |
M
w
/kDa |
Level of functionalizationb/(mol%) |
T
g
/°C |
T
deg
/°C |
Determined by GPC against narrow-disperse polystyrene standards.
Determined by IR-1H NMR correlation curve.
Determined by DSC.
Determined by TGA.
Parent polymer.
Results for polymer 15.
Determined by 1H NMR analysis.
|
1e |
16.7 |
0.0 |
101.1 |
410.2 |
2 |
16.9 |
2.4 |
98.6 |
382.2 |
3 |
16.7 |
6.8 |
104.1 |
339.7 |
4f |
16.8 |
6.9g |
120.3 |
346.5 |
Scale-up and click-diversification
Next, we became intrigued by scaling-up the electrocatalytic polymer C–H functionalization to highlight the unique potential of polymer up-grading for a HER-based green hydrogen economy (Fig. 4). Here, we obtained the modified polymer within 2.5 h in high Mw-retention (87%) and a level of functionalization of 1.1 mol%. The trace metal impurity analysis by ICP-MS of the obtained material showed only a minor contamination of 8.56 ppm manganese in the thus obtained material. Notably, the scaling-up was successfully achieved likewise with styrofoam as the polymer source. Thus, the desired functionalized polymer was obtained in high Mw-retention (82%) and a level of functionalization of 0.8 mol%. It is noteworthy, that in situ formed catalysts proved active as well, with MnBr2 and 1,10-phenanthroline, which gave comparable results as the well-defined catalyst [Mn4] (see ESI Table S6†).
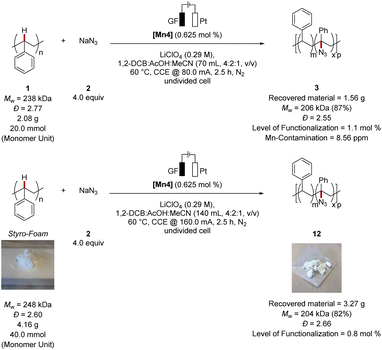 |
| Fig. 4 Scale-up of the C–H azidation on polystyrene. See ESI† for details. | |
Finally, we directly used sunlight as a renewable energy source, highlighting the potential of our mangana-electrocatalyzed C(sp3)–H nitrogenation for a future decentralized sustainable hydrogen economy. Thus, applying a commercially available photovoltaic panel, furnished the desired functionalized polymer in high Mw-retention and high level of functionalization (see ESI† for details).
To further demonstrate the applicability of this method, we exploited the azide moiety as synthetic handle for the copper(I)-catalyzed azide–alkyne cycloaddition (Fig. 5). Fluorescence labeling was performed on polystyrene exploiting ethynyl pyrene, demonstrating the power of the azidation for polymer sorting. Indeed, adding 3 wt% of the fluorescent tagged polymer 22 to a solution of styrofoam provided a fluorescent film. Fluorescence was still observable in a mixture of only 1 wt% 22 in styrofoam. Thus, the up-cycled polymers could reenter the in-use cycle as an additives to facilitate polymer sorting in a tracer-based sorting approach.62
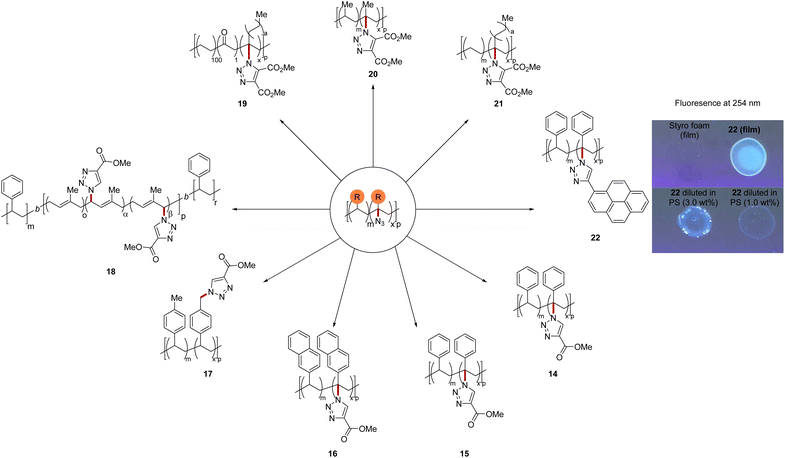 |
| Fig. 5 Product derivatization and fluorescence study. See ESI† for details. | |
Conclusions
In summary, we have reported on an efficient mangana-electrocatalysis strategy for the up-grading of polymers by undirected C(sp3)–H alkane nitrogenation. A wide array of polystyrene-derived polymers as well as challenging polyethylene and polypropylene polymers were altered by synthetically useful azido groups in a sustainable manner. The modification proceeded without significant polymer chain degradation. The electrocatalytic oxidation avoided the use of stoichiometric chemical oxidants, such as iodine(III) reagents. The mangana-electrocatalysis proved broadly applicable and featured ease of scale-up. The functionalized azido-modifications proved to be useful linchpin diversification points for further late-stage click-transformations. On a conceptual level, the merger of this synthetically useful anodic scalable polymer up-grading with the cathodic hydrogen evolution reaction (HER) highlights an outstanding potential for a decentralized green hydrogen economy.
Data availability
The data supporting this article have been uploaded as part of the ESI.†
Author contributions
Conceptualization, L. A.; methodology, R. C. S. and I. M.; investigation, L. A., I. M., R. C. S., S. W., Y. Z., K. Z.; writing – original draft, L. A., R. C. S. and I. M.; writing – reviewing & editing, L. A., R. C. S., S. W., Y. Z., K. Z., I. M.; funding acquisition, L. A.; resources, L. A.; supervision, L. A.
Conflicts of interest
There are no conflicts to declare.
Acknowledgements
The authors gratefully acknowledge support from the ERC Advanced Grant No. 101021358, the DFG (Gottfried Wilhelm Leibniz award to L. A.), and the Alexander von Humboldt Foundation (fellowships to R. C. S.).
Notes and references
- R. Geyer, J. R. Jambeck and K. L. Law, Production, use, and fate of all plastics ever made, Sci. Adv., 2017, 3, e1700782 CrossRef PubMed.
-
Basel Covention, Distribution of plastic waste treatment worldwide in 2018, by method, https://www.statista.com/statistics/1271029/global-plastic-waste-treatment-by-sector/, accessed 05.05.2023 Search PubMed.
- A. Rahimi and J. M. Garcia, Chemical recycling of waste plastics for new materials production, Nat. Rev. Chem., 2017, 1, 0046 CrossRef.
- C. Jehanno, J. W. Alty, M. Roosen, S. De Meester, A. P. Dove, E. Y. Chen, F. A. Leibfarth and H. Sardon, Critical advances and future opportunities in upcycling commodity polymers, Nature, 2022, 603, 803–814 CrossRef CAS PubMed.
- C. W. S. Yeung, J. Y. Q. Teo, X. J. Loh and J. Y. C. Lim, Polyolefins and Polystyrene as Chemical Resources for a Sustainable Future: Challenges, Advances, and Prospects, ACS Mater. Lett., 2021, 3, 1660–1676 CrossRef CAS.
- S. Oh and E. E. Stache, Chemical Upcycling of Commercial Polystyrene via Catalyst-Controlled Photooxidation, J. Am. Chem. Soc., 2022, 144, 5745–5749 CrossRef CAS PubMed.
- Z. Huang, M. Shanmugam, Z. Liu, A. Brookfield, E. L. Bennett, R. Guan, D. E. Vega Herrera, J. A. Lopez-Sanchez, A. G. Slater, E. J. L. McInnes, X. Qi and J. Xiao, Chemical Recycling of Polystyrene to Valuable Chemicals via Selective Acid-Catalyzed Aerobic Oxidation under Visible Light, J. Am. Chem. Soc., 2022, 144, 6532–6542 CrossRef CAS PubMed.
- Y. M. Qin, T. Zhang, H. Y. V. Ching, G. S. Raman and S. Das, Integrated strategy for the synthesis of aromatic building blocks via upcycling of real-life plastic wastes, Chem, 2022, 8, 2472–2484 CAS.
- T. Li, A. Vijeta, C. Casadevall, A. S. Gentleman, T. Euser and E. Reisner, Bridging Plastic Recycling and Organic Catalysis: Photocatalytic Deconstruction of Polystyrene via a C-H Oxidation Pathway, ACS Catal., 2022, 12, 8155–8163 CrossRef CAS PubMed.
- L. Chen, K. G. Malollari, A. Uliana and J. F. Hartwig, Ruthenium-Catalyzed, Chemoselective and Regioselective Oxidation of Polyisobutene, J. Am. Chem. Soc., 2021, 143, 4531–4535 CrossRef CAS PubMed.
- E. R. King, S. B. Hunt, L. J. Hamernik, L. E. Gonce, J. S. Wiggins and J. D. Azoulay, Gold-Catalyzed Post-Polymerization Modification of Commodity Aromatic Polymers, JACS Au, 2021, 1, 1342–1347 CrossRef CAS PubMed.
- C. Bae, J. F. Hartwig, N. K. Boaen Harris, R. O. Long, K. S. Anderson and M. A. Hillmyer, Catalytic hydroxylation of polypropylenes, J. Am. Chem. Soc., 2005, 127, 767–776 CrossRef CAS PubMed.
- J. B. Williamson, S. E. Lewis, R. R. Johnson 3rd, I. M. Manning and F. A. Leibfarth, C-H Functionalization of Commodity Polymers, Angew. Chem., Int. Ed., 2019, 58, 8654–8668 CrossRef CAS PubMed.
- L. Zhang and T. Ritter, A Perspective on Late-Stage Aromatic C–H Bond Functionalization, J. Am. Chem. Soc., 2022, 144, 2399–2414 CrossRef CAS PubMed.
- T. Rogge, N. Kaplaneris, N. Chatani, J. Kim, S. Chang, B. Punji, L. L. Schafer, D. G. Musaev, J. Wencel-Delord, C. A. Roberts, R. Sarpong, Z. E. Wilson, M. A. Brimble, M. J. Johansson and L. Ackermann, C–H activation, Nat. Rev. Methods Primers, 2021, 1, 43 CrossRef CAS.
- S. Rej, Y. Ano and N. Chatani, Bidentate Directing Groups: An Efficient Tool in C–H Bond Functionalization Chemistry for the Expedient Construction of C–C Bonds, Chem. Rev., 2020, 120, 1788–1887 CrossRef CAS PubMed.
- Ł. Woźniak, J.-F. Tan, Q.-H. Nguyen, A. Madron du Vigné, V. Smal, Y.-X. Cao and N. Cramer, Catalytic Enantioselective Functionalizations of C–H Bonds by Chiral Iridium Complexes, Chem. Rev., 2020, 120, 10516–10543 CrossRef PubMed.
- J. Wencel-Delord and F. Glorius, C–H bond activation enables the rapid construction and late-stage diversification of functional molecules, Nat. Chem., 2013, 5, 369 CrossRef CAS PubMed.
- Y. Nakao, Transition-Metal-Catalyzed C-H Functionalization for the Synthesis of Substituted Pyridines, Synthesis, 2011, 2011, 3209–3219 CrossRef.
- For a recent example, see: S. R. Gitter, W. P. Teh, X. Yang, A. F. Dohoda, F. E. Michael and A. J. Boydston, C−H Functionalization and Allylic Amination for Post-Polymerization Modification of Polynorbornenes, Angew. Chem., Int. Ed., 2023, 62, e202303174 CrossRef CAS PubMed.
- A. Bunescu, S. Lee, Q. Li and J. F. Hartwig, Catalytic Hydroxylation of Polyethylenes, ACS Cent. Sci., 2017, 3, 895–903 CrossRef CAS PubMed.
- N. K. Boaen and M. A. Hillmyer, Selective and mild oxyfunctionalization of model polyolefins, Macromolecules, 2003, 36, 7027–7034 CrossRef CAS.
- J. B. Williamson, C. G. Na, R. R. Johnson 3rd, W. F. M. Daniel, E. J. Alexanian and F. A. Leibfarth, Chemo- and Regioselective Functionalization of Isotactic Polypropylene: A Mechanistic and Structure-Property Study, J. Am. Chem. Soc., 2019, 141, 12815–12823 CrossRef CAS PubMed.
- J. B. Williamson, W. L. Czaplyski, E. J. Alexanian and F. A. Leibfarth, Regioselective C-H Xanthylation as a Platform for Polyolefin Functionalization, Angew. Chem., Int. Ed., 2018, 57, 6261–6265 CrossRef CAS PubMed.
- T. J. Fazekas, J. W. Alty, E. K. Neidhart, A. S. Miller, F. A. Leibfarth and E. J. Alexanian, Diversification of aliphatic C-H bonds in small molecules and polyolefins through radical chain transfer, Science, 2022, 375, 545–550 CrossRef CAS PubMed.
- S. Brase, C. Gil, K. Knepper and V. Zimmermann, Organic azides: an exploding diversity of a unique class of compounds, Angew. Chem., Int. Ed., 2005, 44, 5188–5240 CrossRef CAS PubMed.
- N. V. Tsarevsky, Hypervalent Iodine-Mediated Direct Azidation of Polystyrene and Consecutive Click-Type Functionalization, J. Polym. Sci., Part A: Polym. Chem., 2010, 48, 966–974 CrossRef CAS.
- D. Liu and C. W. Bielawski, Direct azidation of isotactic polypropylene and synthesis of ‘grafted to’ derivatives thereof using azide-alkyne cycloaddition chemistry, Polym. Int., 2017, 66, 70–76 CrossRef CAS.
- Z. Siddiqi and D. Sarlah, Electrochemical Dearomatization of Commodity Polymers, J. Am. Chem. Soc., 2021, 143, 21264–21269 CrossRef CAS PubMed.
- R. C. Samanta and L. Ackermann, Evolution of Earth-Abundant 3 d-Metallaelectro-Catalyzed C-H Activation: From Chelation-Assistance to C-H Functionalization without Directing Groups, Chem. Rec., 2021, 21, 2430–2441 CrossRef CAS PubMed.
- P. Gandeepan, L. H. Finger, T. H. Meyer and L. Ackermann, 3d metallaelectrocatalysis for resource economical syntheses, Chem. Soc. Rev., 2020, 49, 4254–4272 RSC.
- L. Ackermann, Metalla-electrocatalyzed C-H Activation by Earth-Abundant 3d Metals and Beyond, Acc. Chem. Res., 2020, 53, 84–104 CrossRef CAS PubMed.
- A. D. Fried, B. J. Wilson, N. J. Galan and J. N. Brantley, Electroediting of Soft Polymer Backbones, J. Am. Chem. Soc., 2022, 144, 8885–8891 CrossRef CAS PubMed.
- S. C. Guo, M. J. Kim, J. C. Siu, N. von Windheim, K. Gall, S. Lin and B. J. Wiley, Eight-Fold Intensification of Electrochemical Azidooxygenation with a Flow-Through Electrode, ACS Sustainable Chem. Eng., 2022, 10, 7648–7657 CrossRef CAS.
- J. I. M. Alvarado, J. M. Meinhardt and S. Lin, Working at the interfaces of data science and synthetic electrochemistry, Tetrahedron Chem, 2022, 1, 100012 CrossRef PubMed.
- Z. M. Li, B. Shuai, C. Ma, P. Fang and T. S. Mei, Nickel-Catalyzed Electroreductive Syntheses of Triphenylenes Using ortho-Dihalobenzene-Derived Benzynes, Chin. J. Chem., 2022, 40, 2335–2344 CrossRef CAS.
- W. Zhang, L. Lu, W. Zhang, Y. Wang, S. D. Ware, J. Mondragon, J. Rein, N. Strotman, D. Lehnherr, K. A. See and S. Lin, Electrochemically driven cross-electrophile coupling of alkyl halides, Nature, 2022, 604, 292–297 CrossRef CAS PubMed.
- Z. H. Wang, L. Wei, K. J. Jiao, C. Ma and T. S. Mei, Nickel-catalyzed decarboxylative cross-coupling of indole-3-acetic acids with aryl bromides by convergent paired electrolysis, Chem. Commun., 2022, 58, 8202–8205 RSC.
- L. F. T. Novaes, J. S. K. Ho, K. Mao, K. Liu, M. Tanwar, M. Neurock, E. Villemure, J. A. Terrett and S. Lin, Exploring Electrochemical C(sp(3))-H Oxidation for the Late-Stage Methylation of Complex Molecules, J. Am. Chem. Soc., 2022, 144, 1187–1197 CrossRef CAS PubMed.
- M. Chen, Z. J. Wu, J. Song and H. C. Xu, Electrocatalytic Allylic C-H Alkylation Enabled by a Dual-Function Cobalt Catalyst, Angew. Chem., Int. Ed., 2022, 61, e202115954 CAS.
- H. Long, T. S. Chen, J. Song, S. Zhu and H. C. Xu, Electrochemical aromatic C-H hydroxylation in continuous flow, Nat. Commun., 2022, 13, 3945 CrossRef CAS PubMed.
- C. Y. Cai, Y. T. Zheng, J. F. Li and H. C. Xu, Cu-Electrocatalytic Diazidation of Alkenes at ppm Catalyst Loading, J. Am. Chem. Soc., 2022, 144, 11980–11985 CrossRef CAS PubMed.
- Z. H. Wang, P. S. Gao, X. Wang, J. Q. Gao, X. T. Xu, Z. He, C. Ma and T. S. Mei, TEMPO-Enabled Electrochemical Enantioselective Oxidative Coupling of Secondary Acyclic Amines with Ketones, J. Am. Chem. Soc., 2021, 143, 15599–15605 CrossRef CAS PubMed.
- K. J. Jiao, Y. K. Xing, Q. L. Yang, H. Qiu and T. S. Mei, Site-Selective C-H Functionalization via Synergistic Use of Electrochemistry and Transition Metal Catalysis, Acc. Chem. Res., 2020, 53, 300–310 CrossRef CAS PubMed.
- P. Xiong and H. C. Xu, Chemistry with Electrochemically Generated N-Centered Radicals, Acc. Chem. Res., 2019, 52, 3339–3350 CrossRef CAS PubMed.
- T. Kurioka, N. Shida, I. Tomita and S. Inagi, Post-Functionalization of Aromatic C–H Bonds at the Main Chains of π-Conjugated Polymers via Anodic Chlorination Facilitated by Lewis Acids, Macromolecules, 2021, 54, 1539–1547 CrossRef CAS.
- T. Kurioka, H. Nishiyama, I. Tomita and S. Inagi, Improvement of Current Efficiency in Anodic Chlorination of Poly(3-hexylthiophene) by using a Boron Trifluoride-Diethyl Ether Complex, ChemElectroChem, 2018, 5, 753–755 CrossRef CAS.
- N. Shida, D. Okazaki, T. Kurioka, H. Nishiyama, D. S. Seferos, I. Tomita and S. Inagi, Anodic Chlorination of Selenophene-Containing Polymers: Reaction Efficiency and Selective Reaction of Single Segment in Rod–Rod Diblockcopolymer, ChemElectroChem, 2017, 4, 1824–1827 CrossRef CAS.
- S. Inagi and T. Fuchigami, Electrochemical Post-Functionalization of Conducting Polymers, Macromol. Rapid Commun., 2014, 35, 854–867 CrossRef CAS PubMed.
- S. Hayashi, S. Inagi and T. Fuchigami, Efficient electrochemical polymer halogenation using a thin-layered cell, Polym. Chem., 2011, 2, 1632–1637 RSC.
- S. Inagi, S. Hayashi, K. Hosaka and T. Fuchigami, Facile Functionalization of a Thiophene−Fluorene Alternating Copolymer via Electrochemical Polymer Reaction, Macromolecules, 2009, 42, 3881–3883 CrossRef CAS.
- S. Hayashi, S. Inagi, K. Hosaka and T. Fuchigami, Post-functionalization of poly(3-hexylthiophene) via anodic chlorination, Synth. Met., 2009, 159, 1792–1795 CrossRef CAS.
- B. Fabre and J. Simonet, Post-polymerization electrochemical functionalization of a conducting polymer: anodic cyanation of poly(p-dimethoxybenzene), J. Electroanal. Chem., 1996, 416, 187–189 CrossRef CAS.
- T. Kurioka and S. Inagi, Electricity-Driven Post-Functionalization of Conducting Polymers, Chem. Rec., 2021, 21, 2107–2119 CrossRef CAS PubMed.
- T. H. Meyer, R. C. Samanta, A. Del Vecchio and L. Ackermann, Mangana(iii/iv)electro-catalyzed C(sp(3))–H azidation, Chem. Sci., 2021, 12, 2890–2897 RSC.
- O. Watanabe, M. Tabata, J. Sohma and A. Lund, Chemical Degradation of Polystyrene Induced by Chlorinated Nitrosobenzene, Polym. Degrad. Stab., 1984, 7, 13–24 CrossRef CAS.
- L. Niu, C. Jiang, Y. Liang, D. Liu, F. Bu, R. Shi, H. Chen, A. D. Chowdhury and A. Lei, Manganese-Catalyzed Oxidative Azidation of C(sp(3))-H Bonds under Electrophotocatalytic Conditions, J. Am. Chem. Soc., 2020, 142, 17693–17702 CrossRef CAS PubMed.
- J. M. Friedrich, C. Ponce-De-Leon, G. W. Reade and F. C. Walsh, Reticulated vitreous carbon as an electrode material, J. Electroanal. Chem., 2004, 561, 203–217 CrossRef CAS.
-
(a) Competing partial oxidation of the pendant methyl group resulted in minor amounts of aldehyde formation with an overall selectivity of 5
:
1 in favor of the azidation: Q. Sheng and H. D. H. Stöver, Macromolecules, 1997, 30, 6451–6457 CrossRef CAS;
(b) The electro-oxygenation has been described for primary and secondary benzylic C–H oxidation: M. R. Barone and A. M. Jones, Org. Biomol. Chem., 2017, 15, 10010–10015 RSC.
- The functionalization gave an α to β ratio of 1.0
:
1.5, which was confirmed by 1H NMR analysis.
- For detailed information see ESI†.
- R. R. Larder and F. L. Hatton, Enabling the Polymer Circular Economy: Innovations in Photoluminescent Labeling of Plastic Waste for Enhanced Sorting, ACS Polym. Au, 2023, 3, 182–201 CrossRef CAS PubMed.
|
This journal is © The Royal Society of Chemistry 2023 |
Click here to see how this site uses Cookies. View our privacy policy here.