DOI:
10.1039/D3SU00125C
(Perspective)
RSC Sustainability, 2023,
1, 1704-1721
Chemistry and pathways to net zero for sustainability
Received
25th April 2023
, Accepted 21st September 2023
First published on 26th September 2023
Abstract
Chemistry needs to play a central role in achieving ‘net zero’ emissions of greenhouse gases (GHGs) into the atmosphere to prevent changes to the climate that will have catastrophic impacts for humanity and for many ecosystems on the planet. International action to limit global warming to 1.5 °C has framed as a key goal the reduction of global emissions to as close to zero as possible by 2050, with any remaining emissions re-absorbed from the atmosphere. Chemistry underpins innovative approaches to reducing emission of the key GHGs, comprising CO2, CH4, N2O and fluorinated gases, and to the recapture of gases already in the atmosphere. Rapid progress is needed in the application of green and sustainable chemistry and material circularity principles in developing these approaches worldwide. Of critical importance will be the incorporation of systems thinking, recognition of planetary boundaries that define safe operating spaces for Earth systems, and an overall reorientation of chemistry towards its roles in stewardship of the Earth's material resources and in sustainability for people and the planet.
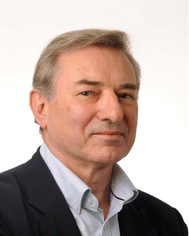 Stephen A. Matlin | Stephen Matlin is an organic chemist who served as Professor of Biological Chemistry in City University, London, and Warwick University. He then worked in international development, as Director of the health and education division of the Commonwealth Secretariat, Chief Education Adviser in the UK Department for International Development and Executive Director of the Global Forum for Health Research in Geneva. He is currently a Visiting Professor in the Institute of Global Health Innovation at Imperial College London. He and the co-authors are all members of the ‘Chemists For Sustainability’ group at the International Organization for Chemical Sciences in Development (IOCD). |
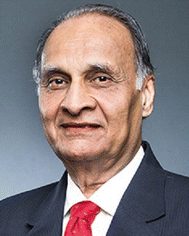 Goverdhan Mehta | Goverdhan Mehta is an organic chemist who has worked for many years at the University of Hyderabad, where he was a Professor, Founder Dean and Vice-Chancellor. He was also Director of the Indian Institute of Science in Bangalore. He is a Fellow of the Indian National Science Academy, serving as President from 1999 to 2001, a Fellow of the Royal Society, London and of numerous other societies, and a former President of the International Council for Science (ICSU). He is currently a University Distinguished Professor in the School of Chemistry at the University of Hyderabad. |
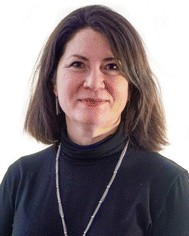 Sarah E. Cornell | Sarah Cornell is a sustainability scientist at the Stockholm Resilience Centre (SRC) at Stockholm University. She holds a PhD in environmental sciences from the University of East Anglia, UK. Her early career research was on the global nitrogen cycle. She has subsequently worked on various aspects of Earth system science and global sustainability. At SRC, she coordinates research and international collaborations on Earth resilience. Associate Professor Cornell leads a transdisciplinary research team working with the planetary boundaries framework, aiming to characterize the global ‘safe operating space for humanity’ in applicable ways. |
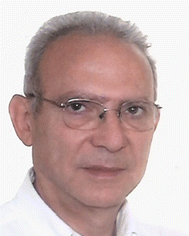 Alain Krief | Alain Krief is widely known for his contributions to organic synthesis, including work on organo-selenium chemistry and the synthesis of pyrethroid derivatives and chrysanthemic acid. He has also worked in chemical informatics. For many years he was Director of the Laboratory of Organic Chemistry at the University Notre Dame de la Paix in Namur, Belgium and subsequently an Emeritus Professor there. A French citizen born in Tunisia, he studied in France, the UK and USA and has been a visiting professor at more than 15 universities worldwide. From 2010 to 2020 he held the position of Executive Director of IOCD. |
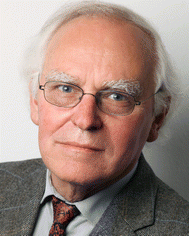 Henning Hopf | Henning Hopf studied chemistry at Göttingen University, Germany and received his PhD from the University of Wisconsin–Madison, USA. He became professor in Würzburg and was then appointed to the Chair of Organic Chemistry of the Technische Universität Braunschweig (TU BS), where he was Managing Director of the Institute of Organic Chemistry until his retirement. He was President of the German Chemical Society and is also a member of the Göttingen, North Rhine-Westphalian and Norwegian Academies of Sciences. His work has been concerned with cyclophanes, highly unsaturated hydrocarbons and aromatic compounds. He is currently an Emeritus Professor in the TU BS. |
Sustainability spotlight
International negotiations to address global warming have set the target of ‘net zero’ – reduction of global emissions of greenhouse gases (principally CO2, CH4, N2O and fluorinated gases) to as close to zero as possible by 2050, with any remaining emissions being reabsorbed from the atmosphere. Essential chemistry contributions to achieving this target must go beyond today's compartmentalised approach to innovation in new materials and processes. Rather, they should be rooted in a reorientation of chemistry towards sustainability, embracing movements, frameworks, and tools embodied in prioritising chemistry's leading role in the material stewardship of planetary resources. This aligns chemistry with the UN Sustainable Development Goals including clean energy, responsible consumption and production, and climate action.
|
1. Introduction
Leaders in governments, industry, academia and wider society are increasingly expressing extreme concern at the polycrises1,2 that are significantly degrading multiple, entangled systems on Earth. Sufficient perturbations in these systems risk leading to tipping points being reached, resulting in long-term changes to the planetary environment and consequently threatening humanity's prospects for survival and wellbeing.3 There is clear evidence4–6 that human activities are causing planetary-scale changes on land, in the ocean, and in the atmosphere, with dramatic and long-lasting impacts, including on the climate system. Four key climate change indicators – greenhouse gas (GHG) concentrations, sea level rise, ocean heat and ocean acidification – set new records7 in 2021 and there is increasing evidence that biogeochemical flows are transgressing planetary boundaries that define safe operating spaces for a number of Earth systems.8–10
As long ago as 1896, Swedish physical chemist Svante Arrhenius predicted that changes in atmospheric CO2 levels could substantially alter the planet's surface temperature through the greenhouse effect and, in 1938, Guy Callendar connected CO2 emissions from industry with global warming.11 With evidence accumulating of the impact of GHG emissions on climate, the establishment of the Intergovernmental Panel on Climate Change (IPCC) in 1988 signposted a new era of interconnection between science and social and political movements aiming to prevent long-lasting changes to the Earth's climate.12 In 1990, the First IPCC Assessment Report played a decisive role in the creation of the United Nations Framework Convention on Climate Change (UNFCCC), a key international treaty to reduce global warming and cope with the consequences of climate change. The Second Assessment Report in 1995 led to the 1997 Kyoto Protocol, which called for reducing the emissions of six greenhouse gases in 41 countries plus the European Union to 5.2% below 1990 levels. However, the impact of the Protocol was considerably weakened by the failure of many of the leading GHG emitters to join or ratify it. The annual meetings of the Conference of the Parties (COP) to the UNFCCC have provided an ongoing mechanism for review of country commitments and a channel for attempts to increase efforts. A major milestone, following the IPCC's Fifth Assessment Report, was the Paris Agreement, a legally binding international treaty on climate change adopted by 196 Parties at COP21 in Paris on 12 December 2015, which entered into force13 on 4 November 2016. This has served as the motive force for the ‘net zero’ approach,14 which is providing a target that helps align societal concern, scientific evidence and innovation and policy-making.
The net zero target is at the forefront of efforts to keep global warming within safe limits,14,15 agreed by countries to be a rise of not more than 1.5 °C above pre-industrial levels by 2050.16,17 Net zero means reducing global emissions of greenhouse gases (GHGs) to as close to zero as possible by 2050, with any remaining emissions re-absorbed from the atmosphere.
Many countries have already pledged national targets to contribute to achieving net zero, although target dates and details of national commitments vary widely.18–20 Moreover, these commitments must be backed by credible action and, to date, few countries have provided climate plans detailing the action they will take. According to the 2022 report21 of the United Nations Environment Program (UNEP), the national commitments so far do not add up to a credible pathway to limiting global warming to 1.5 °C and the problem has been highlighted that some actors engage in ‘net-zero greenwashing’ with empty pledges.22 There is considerable urgency to taking the necessary steps, since global warming has already reached 1.1 °C above pre-industrial levels. A decrease of 45% in GHG emissions compared with 2010 levels is now needed by 2030 to put the world on track to the 1.5 °C limit by 2050. On the present course, however, 2030 emissions will have increased by more than 10% from their 2010 level.23 Many governments,24 private sector companies25,26 and scientists27 agree that pathways to reaching net zero by 2050 are now extremely challenging, requiring governments, businesses, investors and citizens to engage in a total transformation of the energy systems and production/consumption systems that underpin our economies.12,28–30
Essential contributions are required from science and technology (S&T), including chemistry.31–33 Industry in general is responsible for around a quarter of global emissions. As the third-largest industrial emitter of CO2, related both to energy production and material processing, the chemical industry (chemicals & petrochemicals, not including cement) is an important driver of the climate crisis.34 CO2 intensity over recent years has been fairly stable, producing around 1.3 t CO2 per tonne of primary chemicals. The chemical industry therefore must contribute both to reducing its own direct emissions (involving approaches at conceptual and practical implementation levels) and to helping devise ways to assist other sectors to address theirs. Key inputs will include technological innovation, efficiency gains and higher recycling rates, with emphasis on carbon-neutral processing and the requirement that every material component should, as far as practical at the elemental, compound or composite level, have repeated use via recycling to maximise material circularity.
This Perspective considers the connection between net zero and chemistry – the pivotal roles that chemistry, as the science of transformation of matter, can play in achieving it, and the orientations, knowledge and skills that chemists need in order to ensure that the net zero objective translates into sustainability as the outcome. This will bring to the foreground (1) the importance of recognizing and understanding the intimate connections between chemistry processes and Earth systems, highlighting the value of systems thinking as a core competence needed by chemists; (2) the need for the nuanced translation of circular economy ideas into their specific physical adaptation as material circularity,35 through chemistry and other disciplines,36,37 to provide the material/molecular basis of sustainability; (3) the value of adopting the planetary boundaries38 framework to help ensure that anthropogenic activities are contained within the safe operating spaces of Earth systems; and (4) the opportunity for chemistry to develop and display its role in the stewardship of the Earth's material resources, which are embodied in our elemental heritage.
2. Greenhouse gases, chemistry and net zero
Global emissions of GHGs, which in particular include CO2, CH4, N2O and fluorinated gases,39 have risen steeply since the mid-20th Century, doubling from 1970 to a total of about 60 gigatons of CO2-equivalent by 2019 and comprising five main categories (Fig. 1).40 Each of the GHGs has a different global warming potential (GWP) (a measure of how much energy the emissions of 1 ton of a gas will absorb over a given period of time, relative to the emissions of 1 ton of CO2) which results in some relatively low-concentration GHGs having a substantial environmental impact.
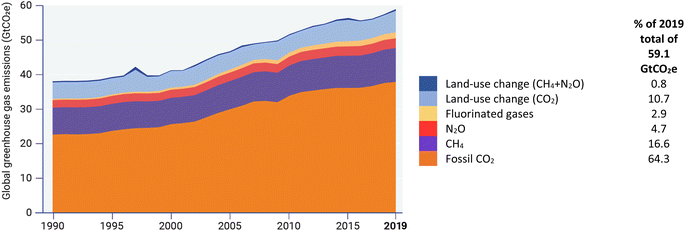 |
| Fig. 1 Global greenhouse gas emissions 1970–2019 (freely reproduced from ref. 40, Fig. 2.1). | |
Several complementary approaches can reduce GHG emissions in the atmosphere. Broadly speaking, these include modifications to existing processes to reduce production of and/or to capture or destroy GHGs prior to emission, substitution of existing processes by new ones that are carbon-neutral and intrinsically produce less GHGs; and sequestration to recapture GHGs that have already been emitted into the atmosphere. Five main categories of emissions (Fig. 1) are discussed below.
2.1 Shifting away from fossil fuel-derived CO2
Carbon dioxide derived from fossil deposits accounted for almost two thirds of the total GHGs emitted in 2019, from applications that generate heat, light and power through combustion and that use carbon and hydrocarbon feedstocks to synthesise a multitude of other useful materials. Innovative options are urgently needed for a ‘clean energy transition’, replacing coal, gas and oil-derived power with primary energy produced from renewable sources such as wind or solar, to dramatically reduce carbon emissions while extending the availability of the non-renewable fossil hydrocarbons as raw materials for synthesis.
However, although the clean energy transition makes economic sense,41 solutions are not simple. The scale of the energy decarbonization issue was already highlighted two decades ago when chemistry Nobel laureate Richard Smalley coined the term ‘The Terawatt Challenge’.42 He noted that in 2004 the vast majority of energy being consumed was from oil, gas, and coal, and that, beyond replacing this with a comparable level of power from sources that did not emit CO2, power production would need to increase fourfold to about 60 terawatts by 2050. Annual global power production has indeed continued to grow rapidly,43,44 almost doubling between 2000 and 2022, and, while renewable energy forms have made an growing contribution, the use of fossil fuels has also risen (Fig. 2) and, with it, global fossil fuel CO2 emissions have continue to increase.45
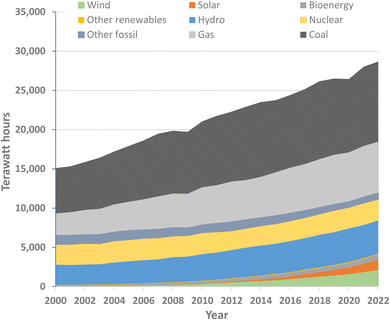 |
| Fig. 2 World electricity generation by source (drawn from data published in ref. 44). | |
It is notable (Fig. 2) that nuclear energy makes a substantial contribution to global electricity production, amounting to 9% in 2022. While the energy-generation step in nuclear fission reactors does not release GHGs, the precursor steps (from mining and refining the uranium ore to producing the concrete for power stations) and the post-reactor storage and further processing of spent fuel, coolant and other irradiated materials at the reactor site have significant environmental impacts. In many of the countries using nuclear power generation, governments have vacillated over policies on sustaining existing nuclear energy stations and investing in new ones. However, in view of the present poor level of global progress towards net zero, it is argued that nuclear energy needs to continue making a substantial contribution at least to mid-century.46–48 Molten salt thorium reactors provide an important alternative to 235U-based reactors.49–51 Their advantages include that Th is more abundant in nature than U and when irradiated with fissile material such as recycled plutonium (generated from fast-breeder reactors), Th fuels can breed fissile 233U in situ.52
Shifts to alternative power sources display complex systemic challenges. Currently used wind and solar technologies have strong dependencies on some elements that have limited practical availability, lack supply-chain resilience and are ‘energy-critical’ elements53 for which competition is increasing. Rare-earth elements, including neodymium, praseodymium, dysprosium and terbium, are extensively used in the permanent magnets for wind turbines, as well as for electric vehicles. A 2022 report54 from the International Energy Agency (IEA) flagged the steeply increasing demand for these elements, which is projected to accelerate. The IEA report emphasised the need for countries to ensure that their energy systems remain resilient and secure as they expand efforts towards the clean energy transition.
For solar power, conventional high-performance optoelectronic devices for capturing sunlight rely on single-crystalline inorganic semiconductors, such as silicon or gallium arsenide. With 173
000 terawatts of solar energy striking the Earth continuously – more than 10
000 times the human world's total energy use – there has been growing interest in the improved performance that can be obtained, for example, with compounds of indium55 and also in applications of other materials such as halide perovskites and organic semiconductors,56 including in single-junction organic solar cells.57 The direct current generated can be stored in a variety of energy carriers, including by H2 production from water splitting using photocatalytic, photoelectrochemical, photovoltaic–electrochemical, solar thermochemical, photothermal catalytic, and photobiological technologies.
Chemistry's contributions include improved efficiencies and cleaner processes in the extraction, refining and recovery of rare earths, as well as in the development of new processes and materials for these important energy production efforts, requiring collaboration with other disciplines.58,59 Recent advances across these technologies have been reviewed, with comparisons using criteria of efficiency, durability, cost and environmental impacts (from lifecycle assessment to completely identify and assess the global warming potential and acidification potential of each process).60 The currently demonstrated approaches of solar H2 production with high efficiency and durability generally show low production cost but high environmental impact, in a trade-off that currently impedes the large-scale implementation of solar H2 production. Photoelectrochemical artificial leaves have potential to lower the costs of sustainable solar fuel production by integrating light harvesting and catalysis within one compact device, but have been limited in their scalability. In one innovation, lightweight artificial leaves have now been fabricated, onto which perovskite-BiVO4 layers have been deposited that show high efficiencies and improved scalability. Moreover, as floating systems they have the potential to be used in open-water applications, thus avoiding competition with land use.61 In another innovation, ultra-thin photovoltaics have been transferred onto light-weight and high-strength composite fabrics, providing portability of power source with the wearer.62
Different approaches are required for mobile energy sources for different modes of transportation. In the case of land transport, options for alternatives to batteries which can be re-charged at intervals include green hydrogen and ammonia as fuels. In the case of shipping, which currently accounts for around 3% of GHG emissions,63–65 ammonia is also being explored as a fuel while the present emphasis for sustainable aviation fuel66 is focused on biofuel production pathways. In each case, there are major challenges in achieving net zero goals. Production of both hydrogen and ammonia currently depends on fossil fuels and generates large amounts of CO2 (see below, Section 4), while aviation biofuels generate CO2 that needs to be offset.
Construction of the hardware and infrastructure required for renewable power sources using currently employed processes and scaled to the projected global levels will require massive additional transformation of materials67 whose production also consumes substantial energy and releases significant amounts of GHGs. For example, about 30 billion tonnes of concrete is used each year, making this the most abundant manufactured material on the planet.68 The cement sector is the third-largest industrial energy consumer and the second-largest industrial CO2 emitter globally, making it essential that this sector moves towards net zero.69 Concrete is an aggregate typically containing sand, crushed stone, and cement. The calcium oxide used as the binder in Portland cement is prepared by decomposition of limestone at c. 900 °C, liberating CO2. More than 4 Gt of cement is produced annually and CO2 emission by the cement industry accounts for 7% of annual GHG production. In recent decades, fly ash (a residue from coal-fired power stations, whose main constituents are oxides of silicon, aluminium, iron and calcium) and other waste materials such as slag from blast furnaces have been used as supplementary cementitious materials. Sustainable alternatives to traditional concrete are being explored,70,71 including options for materials described as ‘C-negative’, for binders (e.g. ferrock containing iron dust from steel production,72 graphenecrete in which graphene partly replaces the cement,73 bio-polymer concrete based on a polyurethane binder74), for aggregates (e.g. hempcrete containing the inner woody core of the hemp plant mixed with lime75), or both (e.g. polymer concrete incorporating calcium silicate as binder and waste polymers as fillers76). Emerging chemistry contributions include use of the reaction CaCO3 + 2NaOH → Ca(OH)2 + Na2CO3 as an alternative that decarbonizes the limestone at ambient temperature and simultaneously captures the CO2 in a product that can be stored or used for regeneration of CO2 as required.77 There has also been demonstration78 of key steps in a carbon-negative process for manufacturing cement from widely abundant seawater-derived Mg2+, involving conversion of Mg(OH)2 into carbonates through reaction with CO2. Recycling of used concrete is also being explored, both for reuse of bulk material and recovery of chemical components.79,80 It should also be noted that concrete absorbs CO2 from the atmosphere, partly offsetting the amount liberated in its production.81
Steel is the most widely used metal in the world, traditionally produced from iron oxide ores by very energy-intensive reduction processes using coal or coke that liberate 1.8 tonnes CO2 per tonne of steel, as a global average. In 2020, world production of 1.86 billion tons of steel contributed 7–9% of annual global anthropogenic carbon emissions.82 As a critical component of the energy transition, including use in wind turbines, grid infrastructure, electric vehicles and solar installations, as well as having many other applications in construction and engineering, global demand for steel is expected to rise by more than a third by 2050. Major reductions in GHG emissions in steel production are therefore essential in moving towards net zero.83 Major contributions to achieving this goal can come from increased recycling of steel and cleaner processes for reducing oxide ores in primary production of iron (e.g., using electrolysis84 or hydrogen85,86). Carbon capture technology has not yet been applied at scale in steelmaking, but is now being explored.87
Copper is the ‘metal of electrification’, and its availability is regarded as essential to all energy transition plans. A market analysis report88 in 2022 suggested that there will be a growing supply-demand gap, as global refined copper consumption is predicted to rise steeply. The report highlighted three main options for increasing supply: new mines or major expansion of existing mines; increasing output as a percentage of a mine's total capacity; and recycling throughs extracting copper from discarded batteries, old wiring, and other equipment. Alternative metals such as aluminium have conduction disadvantages but may be chemically tuneable,89 while new materials such as carbon nanotube wires may offer metal-free options.90
Commentaries on the material requirements for achieving net zero tend to focus mainly on ones discussed above as examples where supply, demand and production factors are critically important. However, there are many other materials that need to be considered in assessing the viability and sustainability of pathways to net zero. These include a range of ‘critical minerals’, such as those required for batteries. A typical electric vehicle requires six times the mineral inputs of a conventional car, with lithium, nickel, cobalt, manganese and graphite being crucial to battery performance, longevity and energy density, while rare earth elements are essential for permanent magnets required for electric vehicle motors.
A current area receiving strong attention is the search for alternatives to lithium. Lithium-ion batteries are presently regarded to be the most effective technology for vehicle electrification. The degree of interest in research on lithium-ion batteries is signalled by a machine-generated summary of research published in 2019, which identified more than 53
000 articles published in the previous three years.91 Current areas receiving strong attention include the search for alternatives to lithium. Ion batteries based on the cheaper and more abundant sodium are being commercialised for some applications, but do not currently provide the same range or speed of charging afforded by lithium. For stationary grid storage, batteries based on iron/air/water systems are also being commercialised.92 The three most commonly used metals in cathode materials for Li-ion batteries (nickel, manganese, cobalt) are expensive and cobalt, in particular, is in limited supply, and alternatives are being examined, including lithium iron phosphate. Under exploration are alternatives to the traditional anode material, graphite, include silicon nanoparticles, which may help increase energy density and speed up charging.93 While the chemistry and physics of batteries themselves are central to power performance, from a perspective of sustainability and net zero goals, it is clear that a host of other factors need to be considered as well, including the total energy and material inputs to manufacturing, the source of electricity for recharging, the fate of battery materials and potential for recovery and reuse, and the wider environmental impacts as assessed by life cycle assessment studies.94 Clean energy transitions will have far-reaching consequences for metals and mining, especially for those metals which have high geographical concentration of production, including lithium, cobalt and rare earths. Security of supply is compounded by a range of factors, including geopolitics, long project development lead times, declining resource quality, growing scrutiny of environmental and social factors and the impact of mining in areas with high water stress levels.43
2.2 Reducing release of fluorinated gases
These first became a cause for alarm in the 1970s to 1980s, with the discovery that chlorofluorocarbons (CFCs) widely used in refrigerants and aerosols were accumulating in the atmosphere and causing depletion of the stratospheric ozone layer. The 1985 Vienna Convention and subsequent 1987 Montreal Protocol banned the use of CFCs, in an example of rapid global action that demonstrated the potential for chemistry, science in diplomacy and international concern to combine on an environmental issue.95 As a result, total column ozone is recovering and is expected to return to 1980 values around 2066 in the Antarctic, around 2045 in the Arctic, and around 2040 for the near-global average.96 This example also demonstrates the potential for incomplete solutions and adverse consequences, however. Unfortunately, the hydrofluorocarbons (HFCs) chosen to replace CFCs turned out to be powerful GHGs, the five most commonly used HFCs being between 150 and 5000 times more potent than CO2.97 HFCs are now being phased out following the agreement of 197 countries to the 2016 Kigali Amendment to the Montreal Protocol.98
What will replace HFCs as refrigerant gases? Apart from possessing suitable physical parameters (including low freezing point, low condensing pressure, high evaporator pressure, high critical pressure, and high vapour density) and chemical properties (including oil solubility, low water solubility, low reactivity) to work efficiently in refrigeration systems, the next-generation refrigerant must meet a host of additional requirements relating to the safety of people (e.g. low toxicity and flammability) and planet (minimal short- and long-term impacts if released into the environment). At present, some hydrofluoroalkenes and hydrocarbons, as well as ammonia (already used in much industrial refrigeration) are being considered as HFC replacements for domestic use, but all have drawbacks.99 This remains an area awaiting further chemical innovation.
Sulphur hexafluoride has been extensively used as a dielectric gas for electrical insulation in high-voltage settings and a considerable proportion tends to leak into the atmosphere, where it acts as an extremely powerful GHG with a GWP of about 23
900 and atmospheric residence of up to 3200 years.100 As a result, its use is regulated and the ongoing search for alternative gases for use in power applications currently focuses on fluorocarbon compounds, including perfluoroketones and perfluoronitriles.101,102 However, in view of the persistence and environmental effects of fluorocarbon compounds,103 other approaches are of increasing interest, include the use of clean air as the insulating medium.104
2.3 Managing emissions of methane and nitrous oxide
Methane in the atmosphere arises from a range of processes,105 with two thirds of the global emissions (estimated at around 590 Mt in 2020)106 attributed to human activities including use of fossil fuels, burning biomass and biofuels, agriculture and landfills and waste (Fig. 3).107
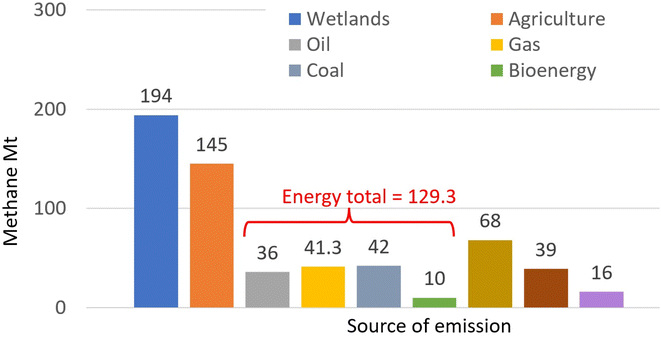 |
| Fig. 3 Global emissions of methane: Mt in 2020 by source. (Drawn from data published in ref. 107: License: CC BY 4.0.) | |
With a shorter lifetime in the atmosphere but a greater capacity to absorb heat, weight-for-weight CH4 has 84–87 times the 20 year GWP of CO2, but 28–36 times when considering its impact over a 100 year time-frame.94 Globally, atmospheric methane concentrations108 rose from 722 ppb in pre-industrial times to 1895 ppb by 2021, the highest value in at least 800
000 years109 and constituting a ‘methane emergency’.110 In the use of fossil fuels, more than 7 MT per year of CH4 escapes from the extraction, transmission and incomplete combustion of hydrocarbon fuels, more than 4 MT per year from coal and 9 MT per year from bioenergy.111 It is estimated that these ‘fugitive emissions’112 could be reduced by 26% using existing technology. Consequently, efficient and cost-effective new processes and technologies are needed,113,114 not only for the fossil fuel-related release115 but also to cut CH4 emissions from the other sources. Attention is also being given to developing ways to remove CH4 from the atmosphere. One method being explored is to release iron-fortified sea water aerosols into the atmosphere, resulting in CH4 oxidation with hydroxyl radicals.116 The wider impacts (e.g. on animal health) of such an approach need to be assessed.
Nitrous oxide, N2O, along with NO and NO2, is generated in internal combustion engines, as well as by burning plant materials, and by the decomposition of nitrogenous fertilizers derived from ammonia. N2O is of particular concern because it is 310 times more powerful than CO2 as a GHG and, in addition, it damages the ozone layer. Human activity is now emitting N2O faster than it is being destroyed in the upper atmosphere (primarily by solar radiation), so it is accumulating at 0.2–0.3% per year.117 Atmospheric concentrations of N2O reached 331 parts per billion in 2018, 22% above levels around the year 1750.118 N2O in the exhausts from internal combustion engines is removed using catalytic converters.119 Some attention has been given to development of methods (e.g., use of metal oxides on activated carbon) for the removal of N2O from industrial exhausts, such as in the adipic acid production process (for nylon production) using HNO3 as oxidizing agent, where the N2O content in tail gas is as high as 38%.120
Shifts in the use of land can release GHGs, particularly CO2, CH4 and N2O, including through deforestation, changes to soil conditions in agriculture and commercial forestry, and conversions to other land uses such as mining and industry. Chemistry contributions are needed across all these areas, including for new knowledge and innovations to promote soil retention of carbon, prevent conversion of nitrogen fertilizers to N2O and capture/recapture GHGs.121–123
3. Carbon capture: from sinks to renewable/reusable carbon sources
The net zero goal requires that global emissions of GHGs are brought as close to zero as possible by 2050. However, the complementary approach of removing or re-absorbing GHGs present in the atmosphere must also play a key role. Sequestration will be vital both in helping bring global efforts on track to limit global warming to 1.5 °C by 2050 and helping sustain the net zero balance beyond this point, given that there will likely remain significant GHG emissions continuing from indispensable sectors such as agriculture.
Three natural processes contribute to the major planetary mechanisms by which CO2 is absorbed from the atmosphere: dissolution in seawater, uptake by plants and especially trees, and retention in soils. However, during the last couple of centuries these mechanisms have become increasingly unable to sustain a steady atmospheric CO2 level within the planetary carbon cycle. Deforestation and changes in land use have led to simultaneous release of CO2 from long-term storage and reduction in the size of these reservoirs, while global warming has itself accelerated the pace of CO2 release (e.g., from permafrost soil) and has reduced the CO2-absorptive capacity of oceans as they have warmed.
3.1 Sequestration
Anthropogenic processes for absorption of atmospheric CO2 are becoming critically important in efforts to rebalance the carbon cycle. While reforestation and improved approaches to land use may play a role, it is also clear that S&T solutions with a core contribution by chemistry are vital.124 According to a McKinsey report,125 achieving CO2 removals, or ‘negative emissions’, will take significant investment to build markets and infrastructure to the scale required for net zero balance. The report highlights three technologies that are currently ready to go to the necessary scale of removing gigatons of carbon: (1) ‘natural’ climate solutions, in which plants are grown to capture CO2 and the plant materials are subsequently transformed into long-term carbon storage forms; (2) bioenergy carbon capture and storage, in which CO2 generated by combustion of bio-materials is captured by technology installed at the site of production;126 and (3) direct air capture (DAC) and storage, in which CO2 is taken out of the atmosphere and stored permanently, safely and securely, deep underground.127
A key input needed from chemistry is the development of affordable, efficient, and sustainable processes to capture CO2 either at the point of production or from the atmosphere. Once sequestered, the CO2 may be pumped directly into geological storage sites deep underground, or mineralised (e.g., as solid carbonates) for underground storage.128 A range of physical, chemical, electrochemical and biochemical methods is being explored.129–131 While CO2 capture from concentrated streams in flue gases is itself challenging in terms of efficiency and cost,132 an additional challenge with DAC approaches is that the ultra-low atmospheric CO2 concentration [c. 400 ppm by volume] is considered a major obstacle due to low CO2 sorption capacities. A hybrid sorbent containing polyamine-Cu(II) complex, Polyam-N-Cu2+, has now been shown to exhibit two to three times greater CO2 capture capacity than most of the DAC sorbents reported to date, at the ultra-dilute concentration of CO2 in the atmosphere. The sorbent is mechanically strong, chemically stable, and amenable to efficient regeneration by salt solutions at an ambient temperature, including seawater. This hybrid sorbent can be regenerated with waste heat or thermal energy at <90 °C, and the captured CO2, sequestered as NaHCO3, can be recovered and stored.133
It is estimated that the oceans are a sink for around 25% of the atmospheric CO2 emitted by human activities each year.134 Concepts for increasing ocean-based carbon removal have been proposed, but most are at early stages of development. Among approaches under exploration are the pumping of ocean acidity from the surface to deep waters,135 and ocean ‘fertilization’ in which iron compounds would be dispersed to stimulate massive blooms of phytoplankton and other photosynthetic algae, which would soak up carbon and sequester it when the algae die and sink.136 Ocean ‘liming’ with calcium oxide or hydroxide is also being investigated.137 Such options illustrate the importance of taking a comprehensive systems approach to the evaluation of possible solutions. For example, the production of lime is currently based on thermal decomposition of calcium carbonate, liberating CO2 (see discussion of concrete in Section 2.1) and in the absence of alternative large-scale, low-carbon manufacturing processes, its use in ocean liming would not lead to a net reduction in atmospheric CO2.
3.2 CO2 reuse
The very large quantities of CO2 generated by anthropogenic processes represent a chemical resource which can used for diverse processes138–141 which, however, should not lead to emission into the atmosphere. Examples of CO2 utilization include its use to make a wide variety of chemicals and plastics, fuel production, concrete enrichment and power generation.142,143 A review of ten pathways for the utilization of CO2, each of which was potentially scalable to over 0.5 gigatonnes of carbon dioxide utilization annually, suggested that the most promising are pathways that involve construction materials, which can both utilize and remove CO2.144
4. Broadening criteria for sustainability: systems perspectives on chemistry for net zero
Historically, large-scale transformations of matter and generation of useful products and processes did not generally require consideration of environmental impacts that were distant in time and space. However, the dramatically increasing extent and pace of transformations of matter have now forced a rethink, a reconceptualization of the parameters to be considered of vital importance to the sustainability of people and planet when the development of any new process or product is being contemplated. A clear signal that this can no longer be delayed came when it was assessed that the production of anthropogenic mass (the global total dry mass of material contained in inanimate solid objects made by humanity) had been doubling every 20 years since 1900 and had reached 30 Gt per year and totalled c. 1.1 trillion tonnes present on the planet in 2020.145 For the first time, in 2020 this accumulated amount equalled the dry weight of total biomass on Earth and, on current trends, total anthropogenic mass will be triple the dry weight of biomass by 2040.
With business-as-usual no longer an option,146,147 radical changes are very urgently needed in how humanity sources, transforms, uses and disposes of the material resources available. The required shifts in approach cut across many spheres, including economics, policy, science, social behaviour and ways of thinking and are highly relevant to chemistry as well to all other sectors.
Particularly important dimensions of the reconceptualization of the transformation of matter at large scale include the adoption of systems perspectives and the combination of these with circular economy and planetary boundary frameworks. For chemistry, these need to be complemented by a reorientation that prioritises the leading role it can play in the stewardship of the Earth's material resources. The interplay among these in relation to production, consumption and disposal is illustrated in Fig. 4.
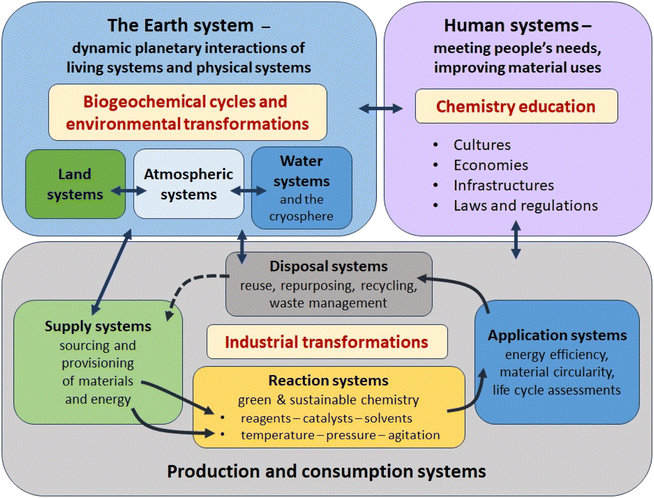 |
| Fig. 4 Production, consumption and disposal in the context of Earth and societal systems. | |
Chemists are used to regarding the reaction vessel and its connected devices, whether at micro-scale in the laboratory or production scale in the chemical plant, as a reaction system. Adding the factor of sustainability through the goal of net zero confers the need to consider the sourcing, extraction, refining, transportation and final preparation of every reagent, catalyst, solvent and piece of equipment, as well as the chain of energy production, transmission and consumption, used in transformations; and the fate of every product, co- and by-product, solvent and waste material, whether these are generated in solid, liquid or gas form. Chemists also need to be aware of different kinds of hazards to the environment and life forms in it; and the impacts of the intended use and of other possible uses and the fate of the product. The systems perspective connects the production/consumption/disposal system with the physical/environmental and biological/ecological systems of the planet and with human systems which mediate the context in which materials are made, used and disposed of.
Many of the components needed to construct such a comprehensive multisystem-based understanding are already available. In particular, extending the ‘chemistry for sustainability’ pyramid presented by Whalen et al.,148 chemistry's contribution to sustainability is seen as being mediated through orientations that prioritise its interactions with Earth and societal systems, using sustainability tools and frameworks (Fig. 5).
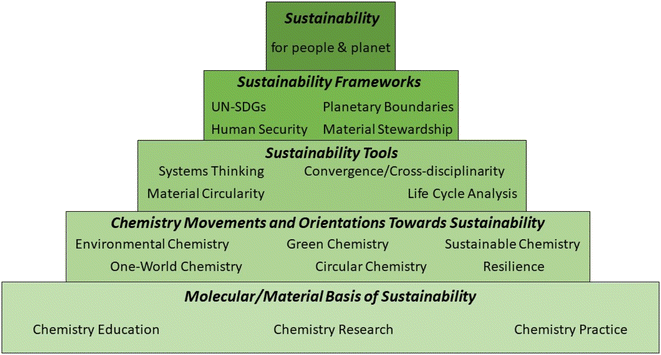 |
| Fig. 5 ‘Chemistry for sustainability’ pyramid. | |
While Fig. 4 develops a comprehensive ‘systems thinking’ picture of actors and factors to be considered, Fig. 5 indicates how the information required to operationalise this approach can be sought, combined and filtered in the overall evaluation. Nevertheless, it is important to emphasise that (1) not all the frameworks (e.g. planetary boundaries,10 human security,149 resilience150) and tools are as yet fully developed with regard to their chemistry dimension; (2) not all the data required (e.g., for the application of material circularity151–153 and life cycle analysis and for the definition of planetary boundaries for all materials produced at large scale9) is available as yet; and (3) the practical integration of frameworks (e.g., planetary boundaries) with sustainability tools (e.g., material circularity) and orientations (e.g. material stewardship) has yet to be achieved.
Importantly, both Fig. 4 and 5 embody the appreciation that sustainability is an emergent property of the whole system – it is not simply a property of individual components of that system. This insight, which requires a process-based, multi-scale and systemic approach,154 provides a valuable perspective from which to evaluate partial solutions to sustainability challenges such as ‘net zero’, when a breakthrough in one aspect (e.g., development of a new catalyst, process, material or policy) is heralded as a ‘green’ solution, in isolation from a comprehensive analysis of the total material and energy balances. Examples that illustrate this in relation to net zero include:
• Much emphasis is placed on the switch from fossil fuels to renewable forms of energy, but it is extremely important to conduct comprehensive assessments of the matter and energy inputs necessary to greatly expand the scale of production of the hardware necessary to achieve the clean energy transition. For example, in the most ambitious climate action scenarios, nearly 2 billion metric tons of steel and 1.3 billion metric tons of cement could be needed for energy infrastructure between now and 2050. Large increases will also be needed in production of rare-earth metals like dysprosium and neodymium used in wind turbine magnets and of high-grade polycrystalline silicon used in solar energy devices. One analysis of 17 of the key materials needed from 2020 to 2050 for the infrastructure to generate low-emissions electricity155 estimated that, while material demands increase, cumulatively they do not exceed the geological reserves. The substantial cumulative CO2 emissions due to the large quantities of materials needing to mobilized during progressive power sector decarbonization would consume only a minor share of global carbon budgets (1–9%), with technological choices strongly influencing the spectrum of future material requirements.
• A major net zero challenge concerns the need for energy carriers that can be used in remote and mobile settings, where fuel/power lines are not practical. The main approaches (see Section 2.1) involve either use of batteries to store and deliver electricity on demand or chemical carriers of energy that can be sourced and used sustainably. For the expansion of use of batteries in critical areas like transport, major constraints include the design of better batteries that can store and deliver energy at practical levels of power and duration, speed of recharging, the sourcing and deliver of sustainable energy for recharging, and the sustainable and resilient sourcing and recycling of battery components.156
• Exploration of chemical carriers of energy to provide viable alternatives to fossil fuels, particularly for transport requirements, include intensive investigations of the potentials for use of H2 and NH3. (a) Hydrogen-based approaches to net zero and sustainable energy have been widely advocated, but since more than 95% of the hydrogen currently produced is derived from fossil fuels, primarily through steam reforming of methane, alternative production methods that use other materials and renewable energy sources are essential.157 Options, including splitting water by heterogeneous catalytic, electrochemical and photochemical methods, need to be evaluated comprehensively for overall sustainability, including in relation to the sourcing of materials and input energies for splitting processes and the fates and potentials for recycling of the hardware employed as well as of the primary products of the splitting reaction itself.158 (b) Ammonia is also being intensively investigated as a potential energy carrier, especially for transport settings. It can be used directly as a fuel in internal combustion engines, or indirectly as a carrier of hydrogen in fuel cells. However, the synthesis of ammonia employed for the last century (Haber–Bosch process) consumes large amounts of hydrogen and energy, both of which are currently derived mainly from fossil fuel sources. Chemistry's major role in developing alternative processes for NH3 synthesis must be guided by the totality of sustainability considerations outlined above.134
5. Stewardship of planetary resources – an essential role for chemistry
The idea of human responsibility to tend the Earth can be found in human cultures throughout history.159 Planetary stewardship in general and especially in an environmental context has been recognized as essential in the face of Anthropocene challenges.160,161 It has been mentioned in relation to specific resources including fossil fuels, phosphorus, metals, and other materials derived from Earth's geological deposits,38 as well as in the context of Earth system processes such as atmospheric and oceanic chemistry and control of air pollution.162 From a commercial perspective, it has been emphasised that product stewardship and sustainable chemistry principles apply across cradle-to-grave product cycles.163 However, only recently have there been preliminary signposts to the overall central role of chemistry in the comprehensive stewardship of the planet's chemical resources,10 recognizing that the planet's elemental resources are finite and, in a growing number of cases, in short supply,164,165 but the implications for chemistry have not yet been elaborated in detail.
The positioning of material stewardship of planetary resource among the overarching frameworks in the highest levels of the sustainability pyramid (Fig. 5) projects the strong contribution it can make to guiding chemistry education, research and practice in their orientations, attention to movements, and adoption of tools aiming at sustainability. In relation to net zero, it importantly expands the scope of attention beyond a focus on keeping materials, products, and components in the market for as long as possible through repair, reuse, re-manufacture, and recycling (technical material circularity). It also requires attention to the scale of global operation (respecting planetary boundaries) and accounting for total balances of material flows (life-cycle analysis) and energy characteristics and the intersections of all these factors with Earth and societal systems (systems thinking and convergence). As well as attending to the implications of physical scale in the transformations of matter, material stewardship incorporates temporal and locational perspectives on the sourcing and redistribution of elements and compounds, with implications for resilience of supplies and the impact of material dispersal on the environment. Net zero must be aligned with broader sustainable development objectives, which implies an equitable net-zero transition, socio-ecological sustainability and the pursuit of broad economic opportunities.
The overarching ambition of material stewardship of planetary resources not only encompasses chemistry's role in achieving net zero, but frames it within a broader landscape of sustainability goals. Among other issues, critiques27,166,167 of net zero argue that, as well as becoming over-focused on aspirational and politically negotiated numerical targets, it can become a distraction from a wider and more comprehensive approach to sustainability, with a result that other critical areas are neglected or even made worse. Material stewardship requires that chemists take a planet-wide view of the stocks and flows of all material resources in the context that sustainability is a property of the whole system.
An example of where this is important concerns the fate of CO2 captured as it is emitted or sequestered from the atmosphere. Net zero necessitates that this CO2 is held long-term in forms from which it is not released to the atmosphere, and much attention has been focused on mineralisation and storage deep under land or oceans. However, principles of material circularity and stewardship point to the very large quantities of CO2 involved being viewed as a chemical resource to be managed. This requires that, in reorienting the carbon reuse economy that has been developing CO2 as a feedstock for fuels, chemicals, materials and food,168,169 two critical criteria are met: (1) ensuring that during transformations to products and their use and recycling, the lock on preventing CO2 release is maintained; and (2) that the transformations and uses are fully analysed using the best knowledge and methods available to ensure that the processes and uses are sustainable from energy, matter and environment perspectives. One area where synergies can be exploited in elaborating the material circularity/stewardship approach is the case of plastics. The damage caused by environmentally persistent plastic wastes has reached such levels of concern that negotiations are now in progress for a UN treaty to address the global plastics crisis.170 Conversion of CO2 into recyclable plastics171via sustainable chemistry/biochemistry and environmentally benign processes offers a convergent way to deal with two major challenges.
6. Conclusion and next steps
The challenge of achieving net zero as a means of driving effective action to limit climate change, and more broadly the challenge of sustainability for people and planet, infiltrate many of the current polycrises. They involve a confluence of factors within the nexus of science, society and policy. Solutions that are purely technological in nature, that only focus on behaviour change or that depend exclusively on setting policies at national and global levels are likely to have limited impact. It is only when all three are able to act in a coordinated manner that decisive shifts take place globally. The strikingly rapid Vienna-Montreal process which ended the use of CFCs that were severely damaging Earth's stratospheric ozone layer (see Section 2.2) demonstrated what is possible when the interests of science, society and policy intersect and result in far-ranging scientific, political, and economic cooperation.95 This contrasts with the slow pace of advance on the more broadly threatening issue of climate change.172 Likewise, the commitment made by countries in 2002, to minimize by 2020 the adverse effects of chemicals and pollutants passing into the environment, was not achieved.173
These lessons from history show that chemistry's role as an innovative source of solutions to problems such as net zero is necessary but not sufficient. Innovation in industrial processes is essential, shifting the frame of reference to a more comprehensive, planetary-scale approach to sustainability. It must originate in well-oriented research that is rooted in an educational foundation creating knowledge and skills that enable professional chemists to apply their creative impulses to seeking pathways to solutions in the context of Earth and societal systems. It must be complemented by chemistry's engagement in the societal, policy and diplomacy processes that lead to systems change, which requires action by individuals, professional bodies and industry associations working together. There is need for greater incentives within all these areas at the nexus of science, society and policy, to accelerate action towards net zero with increased urgency. Incentives along the whole of the S&T pathway are required, including investments in research, development and commercialisation, as well as resources to encourage identification, piloting and large-scale implementation of improved practices. The new process mandated174 by the UN World Environment Assembly and administered through UNEP has established a science-policy panel to contribute further to the sound management of chemicals and waste and to prevent pollution. This represents a major opportunity for the chemistry profession to engage at this critical interface, beginning with participation in the ad hoc open-ended Working Group175 established to develop the process.
Adopting the mission of planetary stewardship of material resources as a human responsibility is vital to ensure that technically sound and sustainable solutions developed are implemented and that trade-offs do not result in ineffective policies or even make global warming worse – especially in the setting of specific targets where competition between net zero and other sustainability goals may arise.27,167,176 The centralization of the stewardship of planetary material resources as a core chemistry mission will require strong leadership from key chemistry bodies, especially those representing the chemistry profession such as the national and international chemistry societies and industry associations.
Author contributions
GM conceived the study. SM wrote the first draft of the manuscript and prepared Fig. 2, 3 and 5. SM SC and AK prepared Fig. 4. All authors reviewed, inputted to and edited the final manuscript. All authors have read and approved the final version of this manuscript for publication.
Conflicts of interest
There are no conflicts to declare.
Acknowledgements
The authors are members of the ‘Chemists for Sustainability’ group of the International Organization for Chemical Sciences in Development. The group has received support from the German Chemical Society and Royal Society of Chemistry.
References
-
M. Lawrence, S. Janzwood and T. Homer-Dixon, What is a global polycrisis?, Discussion Paper 2022-4, Version 2.0, Cascade Institute, Royal Roads University, British Columbia, Canada, available at https://cascadeinstitute.org/technical-paper/what-is-a-global-polycrisis/ Search PubMed.
-
The Global Risks Report 2023, World Economic Forum, Geneva, 18th edn, 2023, available at https://www.weforum.org/reports/global-risks-report-2023/ Search PubMed.
- N. Wunderling, J. F. Donges, J. Kurths and R. Winkelmann, Interacting tipping elements increase risk of climate domino effects under global warming, Earth Syst. Dyn., 2021, 12(2), 601–619, DOI:10.5194/esd-12-601-2021.
-
Climate Change 2023, IPCC AR6 Synthesis Report: Summary for Policymakers, Intergovernmental Panel on Climate Change, Bonn, 2023, available at https://www.ipcc.ch/report/ar6/syr/ Search PubMed.
- M. Prillaman, Are we in the Anthropocene? Geologists could define new epoch for Earth, Nature, 2023, 613, 14–15, DOI:10.1038/d41586-022-04428-3.
- J. Rockström, X. Bai and B. deVries, Global sustainability: the challenge ahead, Glob. Sustain., 2018, 1, E6, DOI:10.1017/sus.2018.8.
-
Five ways to jump-start the renewable energy transition now, United Nations, New York, 2022, available at https://www.un.org/en/climatechange/raising-ambition/renewable-energy-transition Search PubMed.
- V. Tulus, J. Pérez-Ramírez and G. Guillén-Gosálbez, Planetary metrics for the absolute environmental sustainability assessment of chemicals, Green Chem., 2021, 3, 9881–9893, 10.1039/d1gc02623b.
- L. Persson, B. M. C. Almroth, C. D. Collins, S. Cornell, C. A. de Wit, M. L. Diamond, P. Fantke, M. Hassellöv, M. MacLeod, M. W. Ryberg, P. S. Jørgensen, P. Villarrubia-Gómez, Z. Wang and M. Z. Hauschild, Outside the safe operating space of the planetary boundary for novel entities, Environ. Sci. Technol., 2022, 56, 1510–1521, DOI:10.1021/acs.est.1c04158.
- S. A. Matlin, S. E. Cornell, A. Krief, H. Hopf and G. Mehta, Chemistry must respond to the crisis of transgression of planetary boundaries, Chem. Sci., 2022, 13, 11710–11720, 10.1039/d2sc03603g.
-
How Do We Know Climate Change Is Real?, NASA Jet Propulsion Laboratory, California Institute of Technology, Pasadena, CA, 2023, available at: https://climate.nasa.gov/evidence/ Search PubMed.
-
A brief history of climate change discoveries, UK Research and Innovation, 2023, available at: https://www.discover.ukri.org/a-brief-history-of-climate-change-discoveries/index.html Search PubMed.
-
United Nations: Climate Change, The Paris Agreement, UN Framework Convention on Climate Change, New York, 2023, available at: https://unfccc.int/process-and-meetings/the-paris-agreement Search PubMed.
-
Net Zero by 2050, A Roadmap for the Global Energy Sector, International Energy Agency, Paris, 2021, available at https://www.iea.org/reports/net-zero-by-2050 Search PubMed.
-
Net Zero Coalition, For a livable climate: net-zero commitments must be backed by credible action, United Nations, New York, 2023, available at https://www.un.org/en/climatechange/net-zero-coalition Search PubMed.
-
Global Warming of 1.5 °C, an IPCC Special Report on the impacts of global warming of 1.5 °C above pre-industrial levels and related global greenhouse gas emission pathways, in the context of strengthening the global response to the threat of climate change, sustainable development, and efforts to eradicate poverty, ed. V. Masson-Delmotte, P. Zhai, H.-O. Pörtner, D. Roberts, J. Skea, P. R. Shukla, A. Pirani, W. Moufouma-Okia, C. Péan, R. Pidcock, S. Connors, J. B. R. Matthews, Y. Chen, X. Zhou, M. I. Gomis, E. Lonnoy, T. Maycock, M. Tignor, and T. Waterfield, Cambridge University Press, Cambridge, UK and New York, NY, USA, 2018, https://www.ipcc.ch/sr15/ Search PubMed.
-
The Glasgow Climate Pact – Key Outcomes from COP26, UN Framework Convention on Climate Change Secretariat, Bonn, available at https://unfccc.int/process-and-meetings/the-paris-agreement/the-glasgow-climate-pact-key-outcomes-from-cop26 Search PubMed.
-
K. Levin, T. Fransen, C. Schumer, C. Davis and S. Boehm, What Does “Net-Zero Emissions” Mean?, World Resources Institute, Washington DC, 2019, available at https://www.wri.org/insights/net-zero-ghg-emissions-questions-answered Search PubMed.
- D. Yadav, Green hydrogen for a sustainable and ‘aatmanirbhar’ India, Curr. Sci., 2023, 124, 143–144 Search PubMed , https://currentscience.ac.in/Volumes/124/02/0143.pdf.
-
Net-Zero Tracker, Climate Watch, 2023, available at https://www.climatewatchdata.org/net-zero-tracker Search PubMed.
-
Emissions Gap Report 2022, The Closing Window - Climate crisis calls for rapid transformation of societies, United Nations Environment Programme, Nairobi, 2022, available at https://www.unep.org/resources/emissions-gap-report-2022 Search PubMed.
-
Integrity Matters: Net zero commitments by businesses, financial institutions, cities and regions, United Nations' High-Level Expert Group on the Net Zero Emissions Commitments of Non-State Entities, United Nations, New York, 2022, available at https://www.un.org/sites/un2.un.org/files/high-level_expert_group_n7b.pdf Search PubMed.
-
Nationally determined contributions under the Paris Agreement, Synthesis report, UN Framework Convention on Climate Change Secretariat, Bonn, Document FCCC/PA/CMA/2022/4, 2022, available at https://unfccc.int/documents/619180 Search PubMed.
-
R. Watts, ‘Business as usual not an option’: UK energy white paper points direction for North Sea, Upstream, 2020, available at https://www.upstreamonline.com/politics/business-as-usual-not-an-option-uk-energy-white-paper-points-direction-for-north-sea/2-1-931822 Search PubMed.
-
D. Riley, Business as usual is not an option for tackling climate change: time is running short for decarbonising the global economy, BlueBay Asset Management, 2021, available at https://www.bluebay.com/globalassets/documents/bluebay_esg_business_as_usual_sep21.pdf Search PubMed.
-
D. Pacthod and D. Pinner, Time is running out for business leaders who don't have a ‘net zero’ strategy, McKinsey Sustainability, 2021, available at https://www.mckinsey.com/capabilities/sustainability/our-insights/sustainability-blog/time-is-running-out-for-business-leaders-net-zero-strategy Search PubMed.
- S. Fankhauser, S. M. Smith and M. Allen,
et al, The meaning of net zero and how to get it right, Nat. Clim. Change, 2022, 12, 15–21, DOI:10.1038/s41558-021-01245-w.
-
The Journey to Net Zero: Engaging businesses towards a Net Zero economy, PwC, London, 2023, available at https://www.pwc.co.uk/services/sustainability-climate-change/insights/journey-to-net-zero-engaging-businesses.html Search PubMed.
-
The net-zero transition: what it would cost, what it could bring?, McKinsey & Co., New York, 2022, available at https://www.mckinsey.com/capabilities/sustainability/our-insights/the-net-zero-transition-what-it-would-cost-what-it-could-bring Search PubMed.
-
World Trade Report 2022, World Trade Organization, Geneva, 2022, available at https://www.wto.org/english/res_e/booksp_e/wtr22_e/wtr22_e.pdf Search PubMed.
-
SBTi, The Net-Zero Standard, Science-Based Targets Initiative, 2022, available at https://sciencebasedtargets.org/net-zero Search PubMed.
-
C. Sealy, Pathways to Net Zero: The Impact of Clean Energy Research, Elsevier, Amsterdam, 2021, available at https://www.elsevier.com/connect/net-zero-report Search PubMed.
-
The role of materials chemistry in meeting the UK's net zero commitments, RSC Materials Chemistry Division workshop report, Royal Society of Chemistry, London, 2021, available at https://www.rsc.org/globalassets/03-membership-community/connect-with-others/through-interests/divisions/materials-chemistry-division/current-division-activities/documents/rsc-uk-net-zero-commitment-report-final2.pdf Search PubMed.
-
Chemicals, International Energy Agency, Paris, 2022, available at https://www.iea.org/fuels-and-technologies/chemicals Search PubMed.
- B. Megevand, W.-J. Cao, F. Di Maio and P. Rem, Circularity in practice: Review of main current approaches and strategic propositions for an efficient circular economy of materials, Sustainability, 2022, 14, 962, DOI:10.3390/su14020962.
- J. H. Clark, T. J. Farmer, L. Herrero-Davila and J. Sherwood, Circular economy design considerations for research and process development in the chemical sciences, Green Chem., 2016, 18, 3914–3934, 10.1039/C6GC00501B.
- M. Linder, Ripe for disruption: reimagining the role of green chemistry in a circular economy, Green Chem. Lett. Rev., 2017, 10(4), 428–435, DOI:10.1080/17518253.2017.1392618.
- W. Steffen, K. Richardson and J. Rockström,
et al.
, Science, 2015, 347(6223), 736–747, DOI:10.1126/science.1259855.
-
Overview of Greenhouse Gases, United States Environment Protection Agency, 2022, available at https://www.epa.gov/ghgemissions/overview-greenhouse-gases Search PubMed.
-
Emissions Gap Report 2020, United Nations Environment Programme, Nairobi, 2020, available at https://www.unep.org/emissions-gap-report-2020 Search PubMed.
- R. Way, M. C. Ives, P. Mealy and J. D. Farmer, Empirically grounded technology forecasts and the energy transition, Joule, 2022, 6(9), 2057–2082, DOI:10.1016/j.joule.2022.08.009.
- R. E. Smalley, Future global energy prosperity: The terawatt challenge, MRS Bull., 2005, 30, 412–417, DOI:10.1557/mrs2005.124.
-
B. Alvez, Net electricity consumption worldwide in select years from 1980 to 2021, Statista, 2022, https://www.statista.com/statistics/280704/world-power-consumption/ Search PubMed.
-
Electricity Data Explorer, Ember, 2023, available at: https://ember-climate.org/data/data-tools/data-explorer/ Search PubMed.
- P. Friedlingstein, M. O'Sullivan and M. W. Jones, Global Carbon Budget 2022, Earth Syst. Sci. Data, 2022, 14, 4811–4900, DOI:10.5194/essd-14-4811-2022.
-
D. J. C. Mackay, Renewable energy without the hot air, UIT Cambridge Ltd, Cambridge UK, 2009, available at: https://www.withouthotair.com/download.html Search PubMed.
- B. W. Brook, A. Alonso, D. A. Meneley, J. Misak, T. Blees and J. B. van Erp, Why nuclear energy is sustainable and has to be part of the energy mix, Sustainable Mater. Technol., 2014, 1–2, 8–16, DOI:10.1016/j.susmat.2014.11.001.
- V. Knapp, M. Matijević and D. Pevec, The potential of fission nuclear power in resolving global climate change under the constraints of nuclear fuel resources and once-through fuel cycles, Energy Policy, 2010, 38, 6793–6803, DOI:10.1016/j.enpol.2010.06.052.
- V. Knapp, M. Matijević, D. Pevec, B. Crnobrnja and D. Lale, Long term fuel sustainable fission energy perspective relevant for combating climate change, J. Energy Power Eng., 2016, 10, 651–659, DOI:10.17265/1934-8975/2016.11.001.
-
R. Hargraves, Thorium: energy cheaper than coal, CreateSpace Independent Publishing Platform, Scotts Valley, CA, 2012, ISBN 9781478161295 Search PubMed.
- R. A. Sheldon and D. Brady, Green Chemistry, Biocatalysis, and the Chemical Industry of the Future, ChemSusChem, 2022, 15, e202102628, DOI:10.1002/cssc.202102628.
-
Thorium, World Nuclear Association, updated November 2020, available at: https://world-nuclear.org/information-library/current-and-future-generation/thorium.aspx Search PubMed.
- T. Watari, K. Nansai and K. Nakajima, Review of critical metal dynamics to 2050 for 48 elements, Resour., Conserv. Recycl., 2020, 155, 104669, DOI:10.1016/j.resconrec.2019.104669.
-
The Role of Critical Minerals in Clean Energy Transitions, International Energy Agency, Paris, 2022, available at https://www.iea.org/reports/the-role-of-critical-minerals-in-clean-energy-transitions Search PubMed.
- S. Mokkapati and C. Jagadish, III-V compound SC for optoelectronic devices, Mater. Today, 2009, 12(4), 22–32, DOI:10.1016/S1369-7021(09)70110-5.
- J. M. Crow, Unconventional materials that do more with light, Nature, 2022, 608, 838, DOI:10.1038/d41586-022-02288-5.
- H. Yao and J. Hou, Recent Advances in Single-Junction Organic Solar Cells, Angew. Chem., Int. Ed., 2022, 61, e202209021, DOI:10.1002/anie.202209021.
-
A. Durakovic, 15 MW Rare-Earth-Free Offshore Wind Turbine Seeks Path to Market, offshorewind.biz, 2022, https://www.offshorewind.biz/2022/07/28/15-mw-rare-earth-free-offshore-wind-turbine-seeks-path-to-market/ Search PubMed.
- H. Wang, T. N. Lamichhane and M. P. Paranthaman, Review of additive manufacturing of permanent magnets for electrical machines: A prospective on wind turbine, Mater. Today Phys., 2022, 24, 100675, DOI:10.1016/j.mtphys.2022.100675.
- H. Song, S. Luo, H. Huang, B. Deng and J. Ye, Solar-driven hydrogen production: Recent advances, challenges, and future perspectives, ACS Energy Lett., 2022, 7, 1043–1065, DOI:10.1021/acsenergylett.1c02591.
- V. Andrei, G. M. Ucoski, C. Pornrungroj, C. Uswachoke and Q. Wang,
et al, Floating perovskite-BiVO4 devices for scalable solar fuel production, Nature, 2022, 608, 518–524, DOI:10.1038/s41586-022-04978-6.
- M. Saravanapavanantham, J. Mwaura and V. bulović, printed organic photovoltaic modules on transferable ultra-thin substrates as additive power sources, Small Methods, 2023, 7, 2200940, DOI:10.1002/smtd.202200940.
-
D. S. Lee, Update of maritime greenhouse gas emission projections, CE Delft, Delft, 2017, available at https://cedelft.eu/wp-content/uploads/sites/2/2021/03/CE_Delft_7I69_Update_of_Maritime_Greenhouse_Gas_Emission_Projections_DEF.pdf Search PubMed.
-
Reducing emissions from the shipping sector, European Commission Directorate-General for Climate Action, Brussels, 2023, available at https://climate.ec.europa.eu/eu-action/transport-emissions/reducing-emissions-shipping-sector_en Search PubMed.
-
Fourth IMO Greenhouse Gas Study 2020: Executive Summary, International Maritime Organization, London, 2020, available at https://wwwcdn.imo.org/localresources/en/OurWork/Environment/Documents/Fourth%20IMO%20GHG%20Study%202020%20Executive-Summary.pdf Search PubMed.
-
Net zero 2050: sustainable aviation fuels, International Air Transport Association, Montreal, 2022, available at https://www.iata.org/en/iata-repository/pressroom/fact-sheets/fact-sheet---alternative-fuels/ Search PubMed.
- P. C. K. Vesborg and T. F. Jaramillo, Addressing the terawatt challenge: scalability in the supply of chemical elements for renewable energy, RSC Adv., 2012, 2, 7933–7947, 10.1039/C2RA20839C.
- Editorial, Concrete needs to lose its colossal carbon footprint, Nature, 2021, 597, 593–594, DOI:10.1038/d41586-021-02612-5.
-
Technology Roadmap, Low-carbon transition in the cement industry, International Energy Agency, Paris and Cement Sustainability Initiative, Geneva, 2018, available at https://www.iea.org/reports/technology-roadmap-low-carbon-transition-in-the-cement-industry Search PubMed.
- J. W. Phair, Green chemistry for sustainable cement production and use, Green Chem., 2006, 8, 763–780, 10.1039/b603997a.
- J. de Brito and R. Kurda, The past and future of sustainable concrete: A critical review and new strategies on cement-based materials, J. Cleaner Prod., 2021, 281, 123558, DOI:10.1016/j.jclepro.2020.123558.
- D. S. Vijayan, S. Dineshkumar, S. Arvindan and T. S. Janarthanan, Evaluation of ferrock: A greener substitute to cement, Mater. Today: Proc., 2020, 22, 781–787, DOI:10.1016/j.matpr.2019.10.147.
- D. Dimov, I. Amit, O. Gorrie, M. D. Barnes, N. J. Townsend, A. I. S. Neves, F. Withers, S. Russo and M. F. Craciun, Ultrahigh performance nanoengineered graphene–concrete composites for multifunctional applications, Adv. Funct. Mater., 2018, 28, 1705183, DOI:10.1002/adfm.201705183.
- D. H. Murcia, S. Al Shanti, F. Hamidi, J. Rimsza, H. Yoon, B. Gunawan, M. Abdellatef and M. R. Taha, Development and Characterization of a Sustainable Bio-Polymer Concrete with a Low Carbon Footprint, Polymers, 2023, 15(3), 628, DOI:10.3390/polym15030628.
- J. H. Arehart, W. S. Nelson and W. V. Srubar, On the theoretical carbon storage and carbon sequestration potential of hempcrete, J. Cleaner Prod., 2020, 266, 121846, DOI:10.1016/j.jclepro.2020.121846.
- H. M. Naguib, E. G. Zaki, D. E. Abdelsattar, A. S. Dhmees, M. A. Azab, S. M. Elsaeed and U. F. Kandil, Environmentally friendly polymer concrete: polymer treatment, processing, and investigating carbon footprint with climate change, ACS Omega, 2023, 8(9), 8804–8814, DOI:10.1021/acsomega.3c00086.
- T. Hanein, M. Simoni, C. L. Woo, J. L. Provis and H. Kinoshita, Decarbonisation of calcium carbonate at atmospheric temperatures and pressures, with simultaneous CO2 capture, through production of sodium carbonate, Energy Environ. Sci., 2021, 14(12), 6595–6604, 10.1039/d1ee02637b.
- P. Badjatya, A. H. Akca, D. V. Fraga Alvarez, B. Chang, S. Ma, X. Pang, E. Wang, Q. van Hinsberg, D. V. Esposito and S. Kawashimaa, Carbon-negative cement manufacturing from seawater-derived magnesium feedstocks, Proc. Natl. Acad. Sci. U. S. A., 2022, 119(34), e2114680119, DOI:10.1073/pnas.2114680119.
- H.-J. Ho, A. Iizuka and E. Shibata, Chemical recycling and use of various types of concrete waste: a review, J. Cleaner Prod., 2021, 284, 124785, DOI:10.1016/j.jclepro.2020.124785.
- A. Wang and B. Zhu, Influence of nano-SiO2 on the mechanical properties of recycled aggregate concrete with and without polyvinyl alcohol (PVA) fiber, Materials, 2021, 14(6), 1446, DOI:10.3390/ma14061446.
- C. M. Pederneiras, F. C. Brazão and R. Veiga, Carbonation potential of cementitious structures in service and post-demolition: A review, Civ. Eng., 2022, 3(2), 211–223, DOI:10.3390/civileng3020013.
- L. Holappa, A general vision for reduction of energy consumption and CO2 emissions from the steel industry, Metals, 2020, 10, 1117, DOI:10.3390/met10091117.
-
L. Holappa, Special issue “challenges and prospects of steelmaking towards the year 2050”, Metals, 2021, p. 240, DOI:10.3390/books978-3-0365-2776-5.
-
P. Cavaliere, Electrolysis of iron ores: Most efficient technologies for greenhouse emissions abatement, in Clean Ironmaking and Steelmaking Processes, Springer, Cham, 2019, DOI:10.1007/978-3-030-21209-4_10.
- F. Patisson and O. Mirgaux, Hydrogen ironmaking: How it works, Metals, 2020, 10, 922, DOI:10.3390/met10070922.
- J.-P. Birat, F. Patisson and O. Mirgaux, Hydrogen steelmaking, Part 2: Competition with other net-zero steelmaking solutions – geopolitical issues, Mater. Tech., 2021, 109(3–4), 307, DOI:10.1051/mattech/2021023.
-
Carbon capture in the steel industry: ArcelorMittal, Mitsubishi Heavy Industries Engineering, BHP and Mitsubishi Development sign collaboration agreement, ArcelorMittal, 2022, available at https://corporate.arcelormittal.com/media/news-articles/carbon-capture-in-the-steel-industry-arcelormittal-mitsubishi-heavy-industries-engineering-bhp-and-mitsubishi-development-sign-collaboration-agreement Search PubMed.
-
D. Yergin, F. Hoffman, J. Mothersole, K. Rajan, M. Bonakdarpour and O. Beaufils, Growing appetite for copper threatens energy transition and climate goals, S&P Market Intelligence, 2022, available at https://www.spglobal.com/marketintelligence/en/mi/research-analysis/growing-appetite-copper-threatens-energy-transition-climate.html Search PubMed.
- K. N. Subedi, K. Kappagantula, F. Kraft, A. Nittala and D. A. Drabold, Electrical conduction processes in aluminium: Defects and phonons, Phys. Rev. B, 2022, 105, 104114, DOI:10.1103/PhysRevB.105.104114.
- S. G. King, W. G. Buxton, K. Snashall, B. Mirkhaydarov, M. Shkunov, S. R. P. Silva and V. Stolojan, A route towards metal-free electrical cables via carbon nanotube wires, Carbon Trends, 2022, 7, 100159, DOI:10.1016/j.cartre.2022.100159.
-
Beta Writer, Lithium-Ion Batteries: A Machine-Generated Summary of Current Research, Springer, Heidelberg, 2019, DOI:10.1007/978-3-030-16800-1.
-
J. Fialka, Rusty batteries could greatly improve grid energy storage, Sci. American, 2022, available at https://www.scientificamerican.com/article/rusty-batteries-could-greatly-improve-grid-energy-storage/ Search PubMed.
- A. Casimir, H. Zhang, O. Ogoke, J. C. Amine, J. Lu and G. Wu, Silicon-based anodes for lithium-ion batteries: Effectiveness of materials synthesis and electrode preparation, Nano Energy, 2016, 27, 359–376, DOI:10.1016/j.nanoen.2016.07.023.
- Z. Yang, H. Huang and F. Lin, Sustainable electric vehicle batteries for a sustainable world: Perspectives on battery cathodes, environment, supply chain, manufacturing, life cycle, and policy, Adv. Energy Mater., 2022, 12, 2200383, DOI:10.1002/aenm.202200383.
-
R. E. Benedick, Science, diplomacy, and the Montreal Protocol, The Encyclopaedia of Earth, 2007, available at http://www.eoearth.org/view/article/155895/ Search PubMed.
-
Scientific assessment of ozone depletion: executive summary, GAW Report No.
278, World Meteorological Organization, Geneva, 2022, available at https://ozone.unep.org/system/files/documents/Scientific-Assessment-of-Ozone-Depletion-2022-Executive-Summary.pdf Search PubMed.
-
F. Pearce, Ban for gases that saved the ozone layer but now warm the planet, New Scientist, 2016, available at https://www.newscientist.com/article/2109218-ban-for-gases-that-saved-the-ozone-layer-but-now-warm-the-planet/ Search PubMed.
-
Briefing note on ratification of the Kigali amendment, United Nations Environment Programme Ozone Secretariat, 2017, available at https://conf.montreal-protocol.org/meeting/oewg/oewg-39/presession/briefingnotesfr/ratification_kigali_E.pdf Search PubMed.
- A. R. El-Sayed, M. El Morsi and N. A. Mahmoud, A review of the potential replacements of HCFC/HFCs using environment-friendly refrigerants, Int. J. Air-Cond. Refrig., 2018, 26(03), 1830002, DOI:10.1142/S2010132518300021.
-
D. De La Fuente, R. A. Meidl and M. Michot Foss, SF6: the little gas that could… make global warming worse, Forbes, 2021, available at https://www.forbes.com/sites/thebakersinstitute/2021/03/25/sf6-the-little-gas-that-could-make-global-warming-worse/ Search PubMed.
- M. Seeger, R. Smeets, J. Yan, H. Ito, M. Claessens, E. Dullni, L. Falkingham, C. M. Franck, F. Gentils, W. Hartmann, Y. Kieffel, S. Jia, G. Jones, J. Mantilla, S. Pawar, M. Rabie, P. Robin-Jouan, H. Schellekens, J. Spencer, T. Uchii, X. Li and S. Yanabu, Recent trends in development of high voltage circuit breakers with SF6 alternative gases, Plasma Phys. Technol., 2017, 4(1), 1–5, DOI:10.3929/ethz-b-000202543.
- Y. Wang, D. Huang, J. Liu, Y. Zhang and L. Zeng, Alternative environmentally friendly insulating gases for SF6, Processes, 2019, 7, 216, DOI:10.3390/pr7040216.
- D. J. Sheldon and M. R. Crimmin, Repurposing of F-gases: challenges and opportunities in fluorine chemistry, Chem. Soc. Rev., 2022, 51, 4977–4995, 10.1039/d1cs01072g.
-
Siemens Energy and Mitsubishi Electric to develop high-voltage switching solutions with zero GWP, Energy Industry Review, 2021, available at https://energyindustryreview.com/environment/siemens-energy-and-mitsubishi-electric-to-develop-high-voltage-switching-solutions-with-zero-gwp/ Search PubMed.
-
Methane management: the challenge, United Nations Economic Commission for Europe, 2022, available at https://unece.org/challenge Search PubMed.
-
Methane Tracker 2021: methane and climate change, International Energy Agency, Paris, available at https://www.iea.org/reports/methane-tracker-2021 Search PubMed.
-
IEA Methane Tracker 2021, International Energy Agency, Paris, 2021, License: CC BY 4.0, available at: https://www.iea.org/data-and-statistics/charts/sources-of-methane-emissions-3 Search PubMed.
-
Globally averaged marine surface annual mean data, Global Monitoring Division – Global Greenhouse Gas Reference Network, NOAA Earth System Research Laboratories, Boulder, CO, 2023, available at https://gml.noaa.gov/ccgg/trends_ch4/ Search PubMed.
-
IPCC AR5 Working Group 1, Climate Change 2013: The Physical Science Basis – Summary for Policymakers (PDF), Cambridge University Press, 2013, available at https://www.climatechange2013.org/images/report/WG1AR5_SPM_FINAL.pdf Search PubMed.
- E. G. Nisbet, A. E. Jones, J. A. Pyle and U. Skiba, Rising methane: is there a methane emergency?, Philos. Trans. R. Soc., A, 2022, 380, 20210334, DOI:10.1098/rsta.2021.0334.
-
Methane emissions from energy, Methane Tracker 2021, International Energy Agency, Paris, 2021, available at: https://www.iea.org/data-and-statistics/data-tools/methane-tracker Search PubMed.
-
T. Laconde, Fugitive emissions: a blind spot in the fight against climate change, in Global Observatory on Non-State Climate Action, Book 1: Sector-Based Action, ed. T. Laconde, Climate Chance, Paris, 2018, pp. 106–116, available at: https://www.climate-chance.org/wp-content/uploads/2019/03/new-fugitive-emissions-a-blind-spot-in-the-fight-against-climate-change.pdf Search PubMed.
- R. B. Jackson, S. Abernethy, J. G. Canadell, M. Cargnello and S. J. Davis,
et al, Atmospheric methane removal: a research agenda, Philos. Trans. R. Soc., A, 2022, 380, 20200454, DOI:10.1098/rsta.2020.0454.
- P. B. R. Nisbet-Jones, J. M. Fernandez, R. E. Fisher, J. L. France, D. Lowry, D. Waltham, C. A. Woolley Maisch and E. G. Nisbet, Is the destruction or removal of atmospheric methane a worthwhile option?, Philos. Trans. R. Soc., A, 2022, 380, 20210108, DOI:10.1098/rsta.2021.0108.
-
K. C. Michaels, T. de Oliveira Bredariol and K. Konschnik, Driving down methane leaks from the oil and gas industry, International Energy Agency, Paris, 2021, available at https://iea.blob.core.windows.net/assets/465cb813-5bf0-46e5-a267-3be0ccf332c4%2FDriving_Down_Methane_Leaks_from_the_Oil_and_Gas_Industry.pdf Search PubMed.
- F. D. Oeste, R. D. Richter, T. Ming and S. Caillol, Climate engineering by mimicking natural dust climate control: the iron salt aerosol method, Earth Syst. Dyn., 2017, 8(1), 1–54, DOI:10.5194/esd-8-1-2017.
- M. A. Zamudio, S. Bensaid, D. Fino and N. Russo, Influence of the MgCo2O4 preparation method on N2O catalytic decomposition, Ind. Eng. Chem. Res., 2011, 50, 2622–2627, DOI:10.1021/ie100658w.
-
https://phys.org/news/2020-10-nitrous-oxide-emissions-powerful-carbon.html
.
- B. Bozorgi, J. Karimi-Sabet and P. Khadiv-Parsi, The removal of N2O from gas stream by catalytic decomposition over Pt-alkali metal/SiO2, Environ. Technol. Innovation, 2022, 26, 102344, DOI:10.1016/j.eti.2022.102344.
- H. Meng, L. Yuan, J. Gao, N. Ren, Y. Lu and C. Li, Reductive removal of gaseous nitrous oxide by activated carbon with metal oxide catalysts, RSC Adv., 2017, 7, 10407–10414, 10.1039/C6RA26983D.
-
Climate change: introduction to land use, United Nations, New York, 2022, available at https://unfccc.int/topics/introduction-to-land-use Search PubMed.
- H. Tong, A. J. Simpson, E. A. Paul and M. J. Simpson, Land-use change and environmental properties alter the quantity and molecular composition of soil-derived dissolved organic matter, ACS Earth Space Chem., 2021, 5(6), 1395–1406, DOI:10.1021/acsearthspacechem.1c00033.
- C. L. Heald and J. A. Geddes, The impact of historical land use change from 1850 to 2000 on secondary particulate matter and ozone, Atmos. Chem. Phys., 2016, 16(23), 14997–15010 CrossRef CAS , https://acp.copernicus.org/articles/16/14997/2016/.
-
J. Mulligan, G. Ellison, K. Levin, K. Lebling and A. Rudee, 6 ways to remove carbon pollution from the sky, World Resources Institute, 2020, available at https://www.wri.org/insights/6-ways-remove-carbon-pollution-sky Search PubMed.
-
A. Ruddock, J. P. Wolf, K. Smyth, S. Fitzgerald and E. Gibbs, Negative-emissions solutions: how they work and what businesses can gain, McKinsey, London, 2022, available at https://www.mckinsey.com/capabilities/sustainability/our-insights/sustainability-blog/negative-emissions-solutions-how-they-work-and-what-businesses-can-gain Search PubMed.
- M. L. Szulczewski, C. W. MacMinn, H. J. Herzog and R. Juanes, Lifetime of carbon capture and storage as a climate-change mitigation technology, Proc. Natl. Acad. Sci. U. S. A., 2012, 109, 5185–5189, DOI:10.1073/pnas.1115347109.
- C. Beuttler, L. Charles and J. Wurzbacher, The role of negative emission technologies in addressing our climate goals, Front. Clim., 2019, 1, 00010, DOI:10.3389/fclim.2019.00010.
- P. Kelemen, S. M. Benson, H. Pilorgé, P. Psarras and J. Wilcox, An overview of the status and challenges of CO2 storage in minerals and geological formations, Front. Clim., 2019, 1, 00009, DOI:10.3389/fclim.2019.00009.
- P. Panja, B. McPherson and M. Deo, Techno-economic analysis of amine-based CO2 capture technology: Hunter Plant case study, Carbon Capture Sci. Technol., 2022, 3, 100041, DOI:10.1016/j.ccst.2022.100041.
- S. Voskian and T. A. Hatton, Faradaic electro-swing reactive adsorption for CO2 capture, Energy Environ. Sci., 2019, 12, 3530–3547, 10.1039/C9EE02412C.
- H. Demir, G. Onder Aksu, H. C. Gulbalkan and S. Keskin, MOF Membranes for CO2 Capture: Past, present and future, Carbon Capture Sci. Technol., 2022, 2, 100026, DOI:10.1016/j.ccst.2021.100026.
- X. Wang and C. Song, Carbon capture from flue gas and the atmosphere: A perspective, Front. Energy Res., 2020, 8, 560849, DOI:10.3389/fenrg.2020.560849.
- H. Chen, H. Dong, Z. Shi and A. K. SenGupta, Direct air capture (DAC) and sequestration of CO2: Dramatic effect of coordinated Cu(II) onto a chelating weak base ion exchanger, Sci. Adv., 2023, 9, eadg1956 CrossRef CAS PubMed . https://www.science.org/doi/10.1126/sciadv.adg1956.
- A. J. Watson, U. Schuster, J. D. Shutler, T. Holding, I. G. C. Ashton, P. Landschützer, D. K. Woolf and L. Goddijn-Murphy, Revised estimates of ocean-atmosphere CO2 flux are consistent with ocean carbon inventory, Nat. Commun., 2020, 11, 4422, DOI:10.1038/s41467-020-18203-3.
- M. D. Tyka, C. Van Arsdale and J. C. Platt, CO2 capture by pumping surface acidity to the deep ocean, Energy Environ. Sci., 2022, 15, 786–798, 10.1039/D1EE01532J.
- J.-E. Yoon, K.-C. Yoo, A. M. Macdonald, H.-I. Yoon, K.-T. Park, E. J. Yang, H.-C. Kim, J. I. Lee, M. K. Lee, J. Jung, J. Park, J. Lee, S. Kim, S.-S. Kim, K. Kim and I.-N. Kim, Reviews and syntheses: Ocean iron fertilization experiments – past, present, and future looking to a future Korean Iron Fertilization Experiment in the Southern Ocean (KIFES) project, Biogeosci, 2018, 15, 5847–5889, DOI:10.5194/bg-15-5847-2018.
- S. Foteinis, J. Andresen, F. Campo, S. Caserini and P. Renforth, Life cycle assessment of ocean liming for carbon dioxide removal from the atmosphere, J. Cleaner Prod., 2022, 370, 133309, DOI:10.1016/j.jclepro.2022.133309.
-
Putting CO2 to use: creating value from emissions, International Energy Agency, 2019, available at https://iea.blob.core.windows.net/assets/50652405-26db-4c41-82dc-c23657893059/Putting_CO2_to_Use.pdf Search PubMed.
-
CO2 capture and utilisation, International Energy Agency, Technology Report, 2019, available at https://www.iea.org/reports/co2-capture-and-utilisation Search PubMed.
- Y. Warsi, V. Kabanov, P. Zhou and A. Sinha, Novel Carbon Dioxide utilization technologies: A means to an end, Front. Energy Res., 2020, 8, 574147, DOI:10.3389/fenrg.2020.574147.
- P. Gabrielli, M. Gazzani and M. Mazzotti, The role of carbon capture and utilization, Carbon capture and storage, and biomass to enable a net-zero-CO2 emissions chemical industry, Ind. Eng. Chem. Res., 2020, 59(15), 7033–7045, DOI:10.1021/acs.iecr.9b06579.
- Q. Liu, L. Wu, R. Jackstell and M. Beller, Using carbon dioxide as a building block in organic synthesis, Nat. Commun., 2015, 6, 5933, DOI:10.1038/ncomms6933.
-
K. Biniek, R. Davies and K. Henderson, Why commercial use could be the future of carbon capture, McKinsey Sustainablity, 2018, available at https://www.mckinsey.com/capabilities/sustainability/our-insights/why-commercial-use-could-be-the-future-of-carbon-capture Search PubMed.
- C. Hepburn, E. Adlen, J. Beddington, E. A. Carter, S. Fuss, N. M. Dowell, J. C. Minx, P. Smith and C. K. Williams, The technological and economic prospects for CO2 utilization and removal, Nature, 2019, 575, 87–97, DOI:10.1038/s41586-019-1681-6.
- E. Elhacham, L. Ben-Uri, J. Grozovski, Y. M. Bar-On and R. Milo, Global human-made mass exceeds all living biomass, Naure, 2020, 588, 442–444, DOI:10.1038/s41586-020-3010-5.
-
C. Kelly and C. Herweijer, How business can bridge the gap and achieve net zero, PwC, 2021, available at https://www.pwc.com/gx/en/issues/reinventing-the-future/take-on-tomorrow/business-achieving-net-zero.html Search PubMed.
-
J. M. E. Domecq, The new lens that can unlock the net zero economy, World Economic Forum, 2020, available at https://www.weforum.org/agenda/2020/10/new-lens-can-unlock-the-net-zero-economy Search PubMed.
- J. M. Whalen, S. A. Matlin, T. A. Holme, J. J. Stewart and P. G. Mahaffy, A systems approach to chemistry is required to achieve sustainable transformation of matter: The case of ammonia and reactive nitrogen, ACS Sustainable Chem. Eng., 2022, 10, 12933–12947, DOI:10.1021/acssuschemeng.2c03159.
- S. A. Matlin, A. Krief, H. Hopf and G. Mehta, Re-imagining priorities for chemistry: A central science for ‘freedom from fear and want’, Angew. Chem., Int. Ed., 2021, 60(49), 25610–25623, DOI:10.1002/anie.202108067.
- G. Mehta, S. E. Cornell, A. Krief, H. Hopf and S. A. Matlin, A shared future: Chemistry's engagement is essential for resilience of people and planet, R. Soc. Open Sci., 2022, 9, 212004, DOI:10.1098/rsos.212004.
- L. F. Dumée, Circular materials and circular design – review on challenges towards sustainable manufacturing and recycling, Circ. Econ. Sustainability, 2022, 2, 9–23, DOI:10.1007/s43615-021-00085-2.
- V. G. Zuin and K. Kümmerer, Chemistry and materials science for a sustainable circular polymeric economy, Nat. Rev. Mater., 2022, 7, 76–78, DOI:10.1038/s41578-022-00415-2.
- Z. Wang and S. Hellweg, First steps toward sustainable circular uses of chemicals: Advancing the assessment and management paradigm, ACS Sustainable Chem. Eng., 2021, 9, 6939–6951, DOI:10.1021/acssuschemeng.1c00243.
- F. Ceschin and I. Gaziulusoy, Evolution of Design for Sustainability: From product design to design for system innovations and transitions, Des. Stud., 2016, 47, 118–163, DOI:10.1016/j.destud.2016.09.002.
- S. Wang, Z. Hausfather, S. Davis, J. Lloyd, E. B. Olson, L. Liebermann, G. D. Núñez-Mujica and J. McBride, Future demand for electricity generation materials under different climate mitigation scenarios, Joule, 2023, 7, 1–24, DOI:10.1016/j.joule.2023.01.001.
- A. Beaudet, F. Larouche, K. Amouzegar, P. Bouchard and K. Zaghib, Key challenges and opportunities for recycling electric vehicle battery materials, Sustainability, 2020, 12, 5837, DOI:10.3390/su12145837.
- A. J. Vizcaíno, J. A. Calles and A. Carrero, Hydrogen production technologies: From fossil fuels toward renewable sources. A mini review, Energy Fuels, 2021, 35, 16403–16415, DOI:10.1021/acs.energyfuels.1c02501.
- H. Hu and A. C. K. Yip, Grand challenges for heterogeneous catalysis, Front. Catal., 2021, 1, 667675, DOI:10.3389/fctls.2021.667675.
-
Stewardship and the future of the planet: promise and paradox, ed. R. Carnell and C. Mounsey, Routledge, New York NY, 2022, DOI:10.4324/9781003219064.
- W. Steffen, Å. Persson, L. Deutsch, J. Zalasiewicz, M. Williams, K. Richardson, C. Crumley, P. Crutzen, C. Folke, L. Gordon, M. Molina, V. Ramanathan, J. Rockström, M. Scheffer, H. J. Schellnhuber and U. Svedin, The Anthropocene: From global change to planetary stewardship, Ambio, 2011, 40, 739–761, DOI:10.1007/s13280-011-0185-x.
- R. Falkner and B. Buzan, The emergence of environmental stewardship as a primary institution of global international society, Eur. J. Int. Relat., 2017, 25, 131–155, DOI:10.1177/135406611774.
- B. C. Seijo, It's time for better stewardship of our planet's resources, Chem. Eng. News, 2018, 96(19) Search PubMed , available at https://cen.acs.org/articles/96/i19/s-time-better-stewardship-planets.html.
-
Product stewardship/sustainable chemistry, Clariant, Muttenz, GRI Report, 2017, pp. 17–19, https://reports.clariant.com/2017/gri-report/servicepages/downloads/files/gri_report_clariant_gri2017.pdf Search PubMed.
-
D. Cole-Hamilton, Elements in danger, Royal Society of Chemistry, London, 2019, available at https://www.rsc.org/news-events/opinions/2019/jan/elements-in-danger/ Search PubMed.
- S. A. Matlin, G. Mehta, H. Hopf and A. Krief, The periodic table of the chemical elements and sustainable development, Eur. J. Inorg. Chem., 2019, 39–40, 4170–4173, DOI:10.1002/ejic.201801409.
-
L. Steffy, Is ‘net zero’ our best hope for curbing emissions or a ‘dangerous trap’ of self-delusion?, Forbes, Jersey City, NJ, 2022, available at https://www.forbes.com/sites/uhenergy/2022/01/05/is-net-zero-our-best-hope-for-curbing-emissions-or-a-dangerous-trap-of-self-delusion/ Search PubMed.
-
J. Dyke, R. Watson and W. Knorr, Climate scientists: concept of net zero is a dangerous trap, The Conversation, 2021, available at https://theconversation.com/climate-scientists-concept-of-net-zero-is-a-dangerous-trap-157368 Search PubMed.
- E. I. Koytsoumpa, C. Bergins and E. Kakaras, The CO2 economy: Review of CO2 capture and reuse technologies, J. Supercrit. Fluids, 2018, 132, 3–16, DOI:10.1016/j.supflu.2017.07.029.
-
J. Lehtonen, S. Alakurtti, A. Arasto, I. Hannula, A. Harlin, T. Koljonen, R. Lantto, M. Lienemann, K. Onarheim, M. Penttilä, J.-P. Pitkänen and M. Tähtinen, The carbon reuse economy: transforming CO2 from a pollutant into a resource, VTT Technical Research Centre of Finland, 2019, DOI:10.32040/2019.978-951-38-8709-4.
-
Intergovernmental negotiating committee (INC) on plastic pollution, United Nations Environment Programme, Nairobi, 2023, available at https://www.unep.org/about-un-environment/inc-plastic-pollution Search PubMed.
- L. Pires da Mata Costa, D. M. Vaz de Miranda, A. C. Couto de Oliveira, L. Falcon, M. S. Silva Pimenta, I. G. Bessa, S. J. Wouters, M. H. S. Andrade and J. C. Pinto, Capture and reuse of carbon dioxide (CO2) for a plastics circular economy: A review, Processes, 2021, 9, 759, DOI:10.3390/pr9050759.
- Editorial, Don't wait for COP: the end of the fossil-fuel age must start now, Nature, 2023, 613, 216, DOI:10.1038/d41586-023-00039-8.
-
Global Chemicals Outlook II, ed. A. Halpaap and J. Dittkrist, United Nations Environment Programme, Nairobi, 2019, available at https://wedocs.unep.org/bitstream/handle/20.500.11822/28113/GCOII.pdf Search PubMed.
-
Science-policy panel to contribute further to the sound management of chemicals and waste and to prevent pollution, Resolution UNEP/EA.5/Res.8 adopted by the United Nations Environment Assembly on 2 March 2022, available at https://wedocs.unep.org/20.500.11822/40653 Search PubMed.
-
Science-Policy Panel to contribute further to the sound management of chemicals and waste and to prevent pollution, OEWG1.2: Bangkok, Thailand, 30 January - 3 February 2023, United Nations Environment Programme, Nairobi, 2023, available at https://www.unep.org/oewg1.2-ssp-chemicals-waste-pollution Search PubMed.
- T. Searchinger, J. Oliver, P. Dumas, T. Kastner and S. Wirsenius, EU climate plan sacrifices carbon storage and biodiversity for bioenergy, Nature, 2022, 612, 27–30, DOI:10.1038/d41586-022-04133-1.
|
This journal is © The Royal Society of Chemistry 2023 |
Click here to see how this site uses Cookies. View our privacy policy here.