DOI:
10.1039/D4TA04567J
(Review Article)
J. Mater. Chem. A, 2024,
12, 26439-26456
Engineered diatomic catalyst empowered electro-Fenton processes for advanced water purification
Received
2nd July 2024
, Accepted 12th September 2024
First published on 12th September 2024
Abstract
The heterogeneous electro-Fenton (EF) process has attracted tremendous interest as a promising advanced oxidation reaction to treat refractory organic contaminants in water. However, its practical application has been limited by unsatisfactory catalyst performance. Atomically dispersed metal catalysts are appealing for EF systems due to their high atomic utilization, intrinsic activity, and selectivity. In this review, we introduce the latest advances in metal-based single atom catalysts (SACs) for coordinating multiple oxygen reduction pathways in EF. First, we present a comprehensive summary of rational SAC design for selectively converting O2 to H2O2 and ultimately to ˙OH in successive reactions, as well as the associated mechanisms. Subsequently, we delve into the design and synthesis principles of diatomic catalysts (DACs), highlighting their collaborative functions to direct selective O2 conversion to ˙OH. Finally, we conclude the review by discussing current challenges and further directions for the intelligent design of electrodes and reactors coupled with well-defined DACs for EF-based purification technologies.
 Wenxin Sun | Wenxin Sun is currently a doctoral candidate at Jiangnan University, supervised by Dr Hua Zou. He is also a joint PhD candidate at the University of Adelaide School of Chemical Engineering, under the supervision of Dr Xiaoguang Duan. He obtained his Master's degree from Jiangnan University. His main research focuses are the structural design of single-atom catalysts and advanced oxidation technologies. |
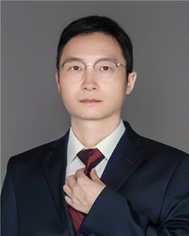 Hua Zou | Hua Zou received his PhD in Environmental Science and Technology from the Chinese Academy of Sciences (2005). He currently works as a professor at Jiangnan University. His current interests include wastewater treatment and resource recovery. |
 Guoshuai Liu | Guoshuai Liu received his PhD in Environmental Science and Technology from the Harbin Institute of Technology (2020). He currently works as an associate professor at the School of Environment and Ecology, Jiangnan University. His current interests include low-carbon electrochemical wastewater purification and resource recovery. |
 Xiaohong Guan | Xiaohong Guan received her PhD in Environmental Engineering from the Department of Civil Engineering at the Hong Kong University of Science and Technology in 2005. She is currently a professor at East China Normal University and a recipient of the National Science Fund for Distinguished Young Scholars. Her primary research focus is on water pollution control chemistry. She was also listed among the top 2% of the world's most highly cited scientists for career-long impact in 2022. |
 Shaobin Wang | Shaobin Wang obtained his PhD in Chemical Engineering from the University of Queensland, Australia. He is now a Professor and ARC Laureate Fellow at the School of Chemical Engineering, The University of Adelaide, Australia. His research interests focus on nanomaterial synthesis and application for adsorption and catalysis, fuel and energy conversion and environmental remediation. |
 Xiaoguang Duan | Xiaoguang Duan obtained his PhD in Chemical Engineering from Curtin University, Australia. He is now an Associate Professor and ARC Future Fellow at the School of Chemical Engineering, The University of Adelaide, Australia. His research areas focus on environmental science, green catalysis, materials engineering, advanced oxidation technologies, and waste upgrading. |
1 Introduction
Advanced oxidation processes (AOPs) are highly attractive technologies capable of degrading and completely mineralizing recalcitrant pollutants, such as pharmaceuticals, endocrine disrupters, and personal-care products.1–4 As environmentally benign AOPs based on hydroxyl radicals, heterogeneous electro-Fenton (EF) processes have attracted extensive attention for the degradation of recalcitrant pollutants from water by virtue of strong oxidization capability.5–10 Generally, two reactions are responsible for the overall EF efficiency: one is the electroreduction of O2 to produce H2O2 as the parent oxide;11–16 the subsequent one is the catalytic activation of H2O2 to produce potent hydroxyl radicals.17–21 In this manner, H2O2 is in situ produced by the cathodic reduction of O2, which avoids the costs and challenges inherent in the H2O2 operating, transportation, and storage processes. However, heterogeneous EF processes still suffer from some limitations, such as insufficient selectivity and activity for the production and activation of H2O2.22–26 Therefore, it is imperative to develop a highly effective “bifunctional catalyst/electrode”, which could synergistically promote H2O2 generation via a 2e− oxygen reduction reaction (ORR) and activate H2O2 to form ˙OH ultimately for water decontamination.19,27 To achieve high efficiency in the EF process, it is essential for the catalyst to possess both the 2e− ORR catalytic site and the H2O2 activation site. This dual-site functionality is critical for optimal performance. Single-atom catalysts (SACs) have emerged as a promising new class of materials, characterized by atomically dispersed metal sites anchored on matched supports, which offer the utmost utilization efficiency of heterogeneous metal atoms and enormously boost their catalytic activity while ensuring chemical stability.28–33 Different from the conventional catalyst paradigm, SACs have received significant attention mainly because of the following remarkable advantages: (1) outstanding activity and selectivity attributed to the unique electronic structures and maneuverable molecular structure;23,34 (2) remarkable reduction in metal consumption and cost caused by high atom-utilization efficiency;35,36 (3) easy identification of the reaction mechanisms due to the well-defined active site;37 and (4) a good platform for understanding structure–activity relationships in catalysis.35 Despite the initial focus on energy conversion, biomedical science, and chemical synthesis, the footprint of SACs has expanded into the realm of electrocatalysis, with demonstrated applications in the hydrogen evolution reaction (HER),38–41 oxygen reduction/evolution reactions (ORR/OER),42–46 and CO2 and N2 reduction reactions (CO2RR and NRR).47–52 Furthermore, SACs could overcome the shortage of EF kinetics (sluggish H2O2 generation or activation) by precise engineering of the catalytic sites. However, most SACs still face the inherent problem of being unable to concurrently synthesize and activate H2O2 effectively, due to the occupation of active sites or the linear relationship constraint on the same “one” active site.49,53 Therefore, introducing a second metal atom to construct “dual-metal-site catalysts” or “dual atom catalysts” (DACs) might be a possible solution to achieve the production and activation of H2O2 simultaneously.54–56 DACs are distinguishable from their SAC counterparts due to the unique adsorption properties of different metal atoms at the active sites, which facilitate the conversion of *OOH and *H2O2 into ˙OH, thereby enabling the transformation of O2 into ˙OH.57 To date, DAC-based EF is still in its infancy, with research primarily focused on designing DAC structures. There is no comprehensive review of the design principles of DACs for EF reactions specifically. Particularly, it is necessary to systematically compare and reveal the functions and synergy of dual catalytic sites and associated mechanisms of DACs in EF systems.
Here, we first highlight the key principles of catalytic O2 to ˙OH transition by SACs, aiming to understand SAC functioning regimes and enlighten smart DAC design. Second, we summarize advanced synthetic techniques of DACs and associated structure features. Third, we propose prospective strategies for controllable and oriented DAC synthesis to realize high efficiency and selectivity in EF, including: (i) manipulating the spatial position of atomic sites; (ii) fine-tuning the elements and electronic structures of atoms. Finally, we discuss the design of specialized EF reactors and engineering approaches for DACs in EF wastewater treatment, aiming to enhance the feasibility of applying DACs in EF processes and potentially paving the way for green and more sustainable remediation systems.
2 Characterization
The identification of the geometric and electronic configuration of atomic sites is the premise for studying the structure–activity relationship of DACs. The geometric structure provides direct evidence to discern the isolated atoms and shows the coordination environments between the single site and neighboring atoms, while the electronic configuration is mainly related to the electronic orbits and oxidation states. Characterization techniques such as aberration-corrected high-angle annular dark-field scanning transmission electron microscopy (AC HAADF-STEM), X-ray absorption spectroscopy (XAS), and Mössbauer spectroscopy have been widely employed to study SACs. Thus, the comprehensive utilization and understanding of the characterization methods are important for accurately identifying the dual-atomic sites of DACs.
2.1 AC HAADF-STEM
When the atomic numbers of metal atoms in SACs are much heavier than those of support atoms, AC HAADF-STEM with a high resolution of 0.1 nm provides a visual observation of the position and distribution of atomic structures on supports. A typical AC HAADF-STEM image of CoN4 is shown in Fig. 1a, confirming that the isolated Co atoms were anchored on the supports.23 Meanwhile, the AC HAADF-STEM image can confirm the density of and distance between metal atoms, further suggesting the formation of metal–metal diatomic structures in DACs (Fig. 1b).58 For DACs, which are composed of two elements with a great difference in molecule weight, AC HAADF-STEM imaging can distinctly display contrasting features of the two atoms, effectively indicating the presence of a dual-atomic-site structure. However, it is difficult to distinguish the DACs containing two different metal elements with similar molecule weights due to their similar brightness in AC HAADF-STEM images. Electron energy loss spectroscopy (EELS) is a powerful technology to distinguish the two elements at the dual-atomic site. For instance, when placing the electron beam on the Fe–Mn DACs, both Fe and Mn signals are present in the EELS, demonstrating the coexistence of Fe and Mn in Fe–Mn dual-atomic sites (Fig. 1c). Meanwhile, the Fe–Mn diatomic distance of two corresponding areas (A1, A2 and B1, B2) in Fig. 1b are 2.4 and 2.5 Å, respectively (Fig. 1d). Additionally, in the EF atomic catalysts, the catalytic atomic centers primarily consist of noble and transition metals,53,59 some of which have atomic (or nuclear) numbers approximating those of the elements in the support. Active sites with atomic numbers close to or less than those of the support elements can also be identified by EELS, providing a reliable technical basis for visualized analysis of DACs.
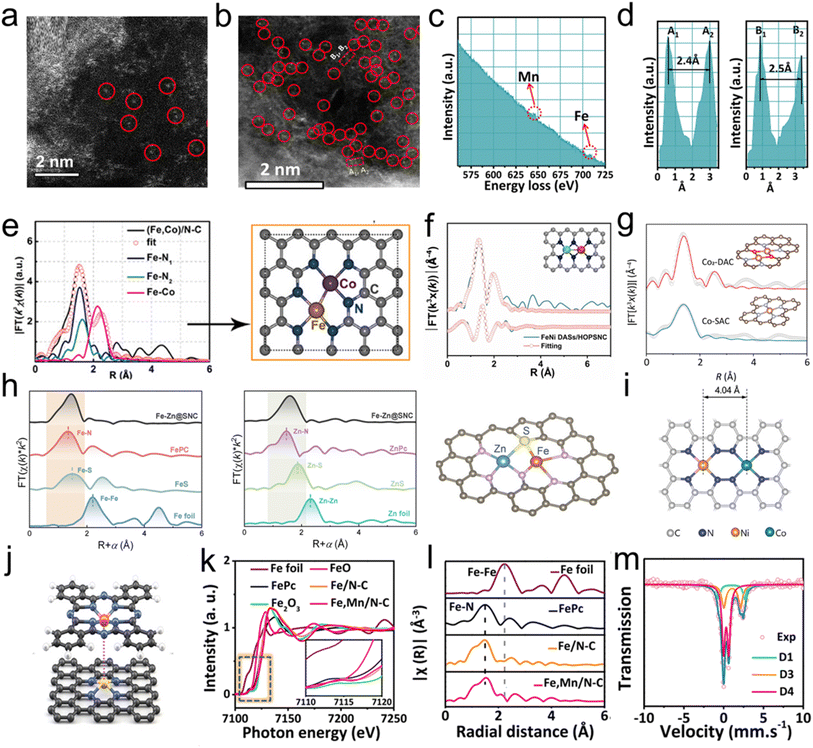 |
| Fig. 1 Characterization of SACs and DACs. (a) AC HAADF-STEM image of CoN4 single atom catalysts. Reprinted with permission from ref. 23, copyright (2022), the American Chemical Society. (b) AC HAADF-STEM image of Fe–Mn dual atom catalysts and (c, d) EELS of the Fe–Mn DACs. Reprinted with permission from ref. 58, copyright (2021), Springer Nature. (e) Corresponding Fe k-edge EXAFS fittings of (Fe, Co)/N–C. Reprinted with permission from ref. 62, copyright (2017), the American Chemical Society. (f) FT-EXAFS fitting curves of the Ni k-edge for FeNi DASs/HOPSNC in R space. Reprinted with permission from ref. 63, copyright (2023), Elsevier. (g) Fitting of the EXAFS spectra of Co2-DAC and Co-SAC in R space. Reprinted with permission from ref. 64, copyright (2024), the American Chemical Society. (h) Fitting of the EXAFS spectra of Fe–Zn@SNC. Reprinted with permission from ref. 65, copyright (2023), Wiley-VCH. (i) Schematic model of neighboring CoNi-DACs. Reprinted with permission from ref. 66, copyright (2024), Wiley-VCH. (j) Schematic model of neighboring CoSAC@FePc. Reprinted with permission from ref. 67, copyright (2024), Wiley-VCH. (k) Fe K-edge XANES spectra. (l) Fourier-transformed (FT) k3-weighted χ(k)-function of the EXAFS spectra for the Fe–Mn DAC. (m) Fe Mössbauer spectra of the Fe–Mn DAC. Reprinted with permission from ref. 58, copyright (2021), Springer Nature. | |
2.2 XAS
XAS, which can be divided into extended X-ray absorption fine structure (EXAFS) and X-ray absorption near edge structure (XANES), is another key technology to characterize SACs and DACs.60,61 The combination of EXAFS and XANES can be employed to provide the average geometric and electronic structure of the DACs. With Fourier transform, the K space in EXAFS is transformed into R space, which is often used to qualitatively assess the coordination environment of target atoms, such as the bond distance and coordination number. SACs are characterised by the absence of metal–metal bonds in the EXAFS spectra. Meanwhile, the positive signature of metal–metal or metal–nonmetal bonding in the EXAFS spectra can provide evidence of the formation of dual-atom structures in DACs in a very closed pack. For instance, both the M1N3–M2N3 and M1N4–M2N4 DACs can exhibit M–N and M–M bonds in EXAFS spectra (Fig. 1e and f).62,63 Similarly, the M1N2O2–M2N2O2 DACs can demonstrate M–O and M–M bonds in EXAFS spectra (Fig. 1g).64 Additionally, M1N3S–M2N3S configurations can manifest M–N and M–S bonds in EXAFS spectra (Fig. 1h).65 Even dual-atom configurations at greater distances can characterize bond types through EXAFS spectra, aiding in determining the structure of DACs through theoretical calculations (Fig. 1i and j).66,67 XANES is the primary technology used to evaluate the electronic orbits and valence states of the target atoms in DACs, which provides direct evidence of the interactions between atomic sites and supports.68 Meanwhile, the XANES absorption edge energy of central atoms is generally located between the corresponding metallic foil and the oxidized reference, indicating the intermediate valence states of target metal atoms. As shown in Fe K-edge XANES spectra, the valence of Fe in the Fe–Mn DAC prefers an oxidation state of Fe2+–Fe3+ rather than the metallic state (Fig. 1k). And the peaks at ≈1.4 in EXAFS data are attributed to the Fe–N DAC (Fig. 1l).58 Utilizing XAS, the coordination numbers and oxidation states of the metal catalytic centers within EF atomic catalysts can be precisely determined. Identifying these centers in an oxidation state reveals their capacity to donate electrons, thereby activating H2O2 effectively.
2.3 Mössbauer spectroscopy
Since the discovery of Ir by Rudolf Mössbauer in 1958, Mössbauer spectroscopy has been carried out to probe the structure and electronic properties of materials. As a rule, Mössbauer spectroscopy can be deconvoluted into singlet, doublet, and sextet components. As to the SACs, doublets, including low (D1), intermediate (D2), and high (D3) spin states, can be detected, which has been the universally accepted evidence for the existence of M–Nx sites (M = Fe, Ni, Ru, Ir, etc.).69 Nevertheless, no consistent analysis of Mössbauer spectroscopy has been performed with respect to DACs. However, in the Fe–Mn DAC, D2 and singlet components of the single Fe site are absent in the Mössbauer spectrum, while a new doublet (D4) appears, which indicates that the Fe species exist in the form of Fe2+ in a low spin state or Fe3+ in a high spin state (Fig. 1m).58 Therefore, Mössbauer spectroscopy can be employed to ascertain the oxidation states of metal catalytic centers in EF systems and to elucidate the coordination environment of the surrounding atoms. This technique, when coupled together with XAS, offers compelling and molecule-level evidence regarding the structural configuration of EF atomic catalysts.
Although the above technologies can elucidate the structural and electronic information of DACs, each one still has its own limitations. For example, AC HAADF-STEM can only represent the local region of the catalyst, while XAS and Mössbauer spectroscopy are bulk analytical techniques, which reflect the comprehensive result of all sites, including the substrate of the materials. Typically, density functional theory (DFT) models, used for the catalytic mechanism investigation of DACs, are based on molecular structures from characterization results. Therefore, reliable analyses of the geometric and electronic structures of DACs are crucial. Multiple technologies should be employed concurrently to characterize DACs precisely and minimize the depressing discrepancy in understanding the structure–activity relationship.
3 Atomic catalysts in EF processes for H2O2 generation and activation
3.1 SACs in the EF system for H2O2 generation
Both noble metal atom (Pd and Pt) catalysts,30,46,70 transition metal atom catalysts (Mn, Co, Ni, Zn, and Mo),3,10,12,33,71–75 and main-group metal catalysts (In and Sb) play important roles in H2O2 electrochemical generation.9,76 There are two steps for H2O2 generation on the cathode. The first step is the migration and interaction of O2 with active sites, followed by its reaction with H+ and receiving e− to generate *OOH.77 In the second step, the obtained *OOH species undergo a reaction with another H+ to accomplish the 2e− pathway, forming H2O2.71 The 2e− pathway is composed of two proton-coupled electron transfer steps, producing only the *OOH intermediate. The single metal atoms immobilized on the support inherit properties similar to those of homogeneous metal ions dissolved in aqueous solution, ensuring that all the metal atoms are exposed to participate in H2O2 generation reactions. Zhao et al. obtained an atomically dispersed Pt atom anchored into CNTs to form Pt–S coordination, which exhibited a higher selectivity of H2O2 production in the EF system compared with the Pt–N based SAC (Fig. 2a).46 Another study conducted by Gao et al. involved theoretical design and experimental investigations on several transition metal SACs (Mn, Fe, Co, Ni, and Cu) anchored in N-doped graphene, aiming to explore their electrochemical ORR performances (Fig. 2b).3 Interestingly, main-group metals have also been introduced into EF SACs recently. Yan et al. reported a unique Sb2S3-templated strategy to fabricate high-density main-group Sb atoms dispersed in N, S co-doped hollow carbon nanofibers that are further assembled into a flower-like architecture.76 Combined experimental and theoretical studies suggest that the superior catalytic performance of Sb-SACs can be attributed to the dense Sb atom population, hierarchical porosity, and the S-doping regulated microenvironments of the Sb–N4 moieties. Meanwhile, Zhang et al. reported that In-SACs possess an optimized electronic structure and enhanced adsorption energy for the key *OOH intermediate, which can be attributed to the presence of the synergistic effects between first-coordinated N, S and second-coordinated B and single In atoms, resulting in accelerated reaction kinetics and boosted catalytic 2e− ORR performance.9 This study opens an attractive avenue to design highly efficient main-group SACs for the 2e− ORR and other electrocatalytic processes. Overall, these SACs demonstrated high selectivity toward the 2e− ORR. The regulation of the local electronic structure of metal centers is significant for O2 adsorption and activation and the subsequent electrochemical transformation of intermediates during the ORR process.
 |
| Fig. 2 Some representative atom catalysts in an electro-Fenton system. (a) DFT calculations of *OOH adsorption on central Pt–S atom sites. Reprinted with permission from ref. 46, copyright (2022), Springer Nature. (b) Schematic of the ORR along the different pathways on various transition metal SACs. Reprinted with permission from ref. 3, copyright (2020), Elsevier. (c) Reaction pathways for H2O2 adsorption and activation at FeN4 sites. Reprinted with permission from ref. 59, copyright (2023), Elsevier. (d) Schematic illustrations of H2O2 activation for ˙OH generation via Cu-SAC sites. Reprinted with permission from ref. 79, copyright (2023), National Academy of Sciences. (e) Activation of H2O2 on Fe–N single sites in the FeCuSA-NPC catalyst. Reprinted with permission from ref. 80, copyright (2021), the American Chemical Society. (f) Schematic illustrations of the 2 + 1e− ORR pathway for ˙OH generation via CoFe-DAC sites. Reprinted with permission from ref. 57, copyright (2023), the American Chemical Society. | |
3.2 SACs in the EF system for H2O2 activation
Fe SACs have been used for the catalytic activation of H2O2 to produce strongly oxidizing ˙OH.8,78 Xia et al. reported a catalyst with single-atom Fe–N4 on a N-doped porous carbon matrix (Fe-SAC/NC) for a heterogeneous EF process, in which the H2O2 formed in situ was activated to form ˙OH on single-site Fe–N4 (Fig. 2c).59 Besides Fe species, incorporated Cu atoms can catalytically activate H2O2 to generate ˙OH with robust stability. Gu et al. reported a slow-release Cu-SAC deposited on a C3N4/montmorillonite interface by facilitating dynamic equilibrium between metal precursor supply and anchoring site formation, exhibiting excellent H2O2 activation performance (Fig. 2d).79 By contrast, single Fe sites are more reactive to activate H2O2 to generate ˙OH than Cu sites. For instance, Zhao et al. reported a catalyst with dual Fe–N4 and Cu–N4 sites on carbon (FeCuSA-NPC) for a hetero-EF reaction, in which pollutant chlorophenol (CP) was dechlorinated on the Cu–N4 site, while the in situ formed H2O2 was activated to form ˙OH on individual Fe–N4 (Fig. 2e).80 Subsequently, the dechlorinated pollutant was oxidized by ˙OH. Benefiting from the synergistic effect, the chlorinated pollutants can be efficiently degraded and mineralized. To summarize, an Fe/Cu single site on different supports could greatly enhance the breakage of the superoxide O–O bond in H2O2 to generate ˙OH, guaranteeing high activity in the heterogeneous EF catalytic process.
3.3 DACs in the EF system for tandem H2O2 generation and activation
To deliver efficient performance in hetero-EF, developing bifunctional atom catalysts with high activity and selectivity to achieve the conversion of O2 to ˙OH is imperative. Qin et al. observed that the atomically dispersed Co sites are assigned to enhance O2 reduction to H2O2 intermediates, while Fe sites are responsible for activating as-generated H2O2 to ˙OH (Fig. 2f).57 This new strategy provides instructive guidance for designing DAC cathodes to independently drive the successive pathways in heterogeneous EF systems.
DACs refer to the combination of two different single-atom sites, which are distributed in pairs or homogeneously all over the supports.81 The distance between the two metal atoms (M and M′) does not have strict restrictions. At the cathode of the EF system, DACs serve to reduce oxygen directly to ˙OH through a 2 + 1e− pathway (2e− ORR + 1e− Fenton) with fast kinetics for higher ˙OH generation efficiency.1,18,82 As shown in Fig. 3, we propose apparent 2 + 1e− O2 reduction mechanisms for ˙OH generation on DACs. First, O2 is preferentially adsorbed on the metal atom site (e.g., Pd, Pt, Mn, Co, Ni, Zn, Mo, In, and Sb). Subsequently, O2 is reduced to *OOH on the metal atom site via an *OOH intermediate with a small Gibbs free energy change value (O2 + * + H+ + e− → *OOH and *OOH + H+ + e− → H2O2 + *, * denoting the reactive sites). *H2O2 might migrate to the neighboring Fenton site (e.g., Fe and Cu) with strong *H2O2 binding energy and decomposed into ˙OH (H2O2 + * + e− → ˙OH + OH−). The coordination atoms in the metal can regulate the electronic structure of the metal atom. Doping with elements such as N, S, and O has electronegativity to change the d-bond center of the metal sites, thereby reasonably controlling electron interactions between the d-orbital (metal sites) and p-orbital (O2 and key intermediate), influencing catalytic efficiency.32 Additionally, it can adjust the energy levels of the d orbitals at the metal center, making it more suitable for reactant adsorption and intermediate stabilization in specific catalytic reactions. By modulating the electronic energy levels of the metal center, the reaction pathway can be optimized, reducing the activation energy of the reaction. DACs are essentially the combination of two SAC sites, achieving tandem catalysis of O2.
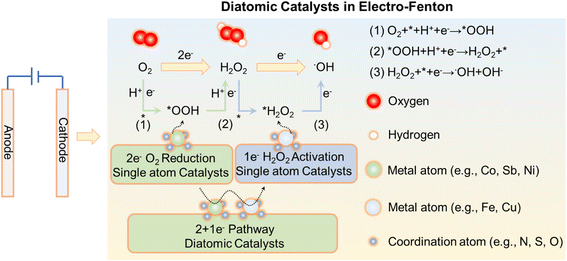 |
| Fig. 3 Schematic illustrations of diatomic catalyst mechanisms for electro-Fenton. | |
4 Preparation strategy for electro-Fenton DACs
4.1 Synthetic technology of DACs
Diverse approaches (e.g., etching method, precursor preselection, template method, single-molecule/atom hybrid, and wet-chemistry) have been developed to create DACs, achieving precise modulation of atom site configurations (Fig. 4a). Based on the distance between the diatomic metal centers, we can categorize DACs into metal-bonded and non-metal-bonded types. Usually, the preparation of metal-bonded catalysts primarily involves the etching method. Zhang et al. reported that the fabricating process of Fe/Cu-HNG catalysts begins by etching graphene oxide (GO) with nitric acid. The strong oxidation capability of nitric acid breaks the C–C bonds in the GO layers, yielding epoxy chains (carboxyl and/or hydroxyl) and other defects. Subsequently, Fe/Cu nanoparticles and GO are mixed and pyrolyzed to form a dimer structure (Fe–Cu bind) inside the holes (Fig. 4b).83
 |
| Fig. 4 Synthesis of DACs with the combination of two kinds of single-atom sites. (a) Synthesis methods of DACs. (b) Synthetic procedures of SiO2@Mo/Fe catalysts. Reprinted with permission from ref. 83, copyright (2023), Springer Nature. (c) Synthetic procedures of M1M2-DAC. Reprinted with permission from ref. 84, copyright (2023), the American Chemical Society. (d) Synthetic procedures of Fe,Mn/N–C. Reprinted with permission from ref. 58, copyright (2021), Springer Nature. (e) Synthetic procedures of CoSAC@FePc. Reprinted with permission from ref. 67, copyright (2024), Wiley-VCH. (f) Synthetic procedures of the CoFe DAC. Reprinted with permission from ref. 80, copyright (2018), the American Chemical Society. | |
As the distance between DAC metal centers increases, the synthesis primarily falls into three categories: precursor preselection, wet chemistry, and single-molecule/atom hybrid methods. The precursor preselection approach is favored for synthesizing DACs due to its simplicity and ease of operation. Preselecting a specific reagent containing a dual-atomic structure as the precursor offers a great opportunity for the successful formation of a dual-atomic configuration by inheriting the dual-atomic coordination from the origin molecule. Zhang et al. reported that a porous material-encapsulated macrocyclic complex mediates the structure of DACs by preserving the main body of the molecular framework during pyrolysis (Fig. 4c).84 Additionally, the synthesis of DACs can also be conducted through a template method. The process of fabricating Fe,Mn/N–C catalysts begins by mixing iron and manganese with a dicyandiamide template, followed by a calcination step at 900 °C under an argon atmosphere for six hours to form Fe,Mn/N–C catalysts (Fig. 4d).58 The single-molecule/atom hybrid methods allow for the blending of two types of single-atom catalysts, thereby enabling the synthesis of bimetallic catalysts without metal bonds. Xie et al. reported the synthesis of CoSAC@FePc was achieved by a simple sonication-assisted coupling interaction between FePc (containing FeN4 moieties) and a single-atom Co catalyst (containing CoN4 sites) (Fig. 4e).67 While this approach allows for the straightforward creation of bimetallic active sites without metal bonds, precise control over the distance between bimetallic active centers is challenging to achieve. In wet chemistry, metal precursors are selected and mixed with carbonaceous materials, followed by high-temperature heat treatment.29,85 Zhao et al. reported the synthesis of CoFe-DACs by one-step pyrolysis of Co and Fe ion doped metal–organic frameworks (MOFs).80 Specifically, Zn-based MOFs were first synthesized and then doped with Co and Fe ions via a wet-chemistry method. Finally, the products were pyrolyzed at 1000 °C under a N2 atmosphere (Fig. 4f). Under such circumstances, Zn ions were reduced and volatilized, while the ligands in MOFs were decomposed and transformed into a porous carbon network, and Co and Fe ions were transformed into atomically dispersed metal sites, respectively.86 Only isolated bright dots are shown in the AC HAADF-STEM image, indicating that the as-prepared Co or Fe single-atom sites in the catalysts are separated.
The distance between the diatomic atoms in DACs is the most crucial factor affecting its synergistic effects. Mo et al. provided an outlook on the inter-atomic distance effect of DACs in EF.87 However, there was no detailed explanation of the synthesis methods for DACs with different inter-atomic distances. Therefore, we have summarized the synthesis methods suitable for different distances between DACs (Fig. 5). Based on these synthetic approaches, a variety of DACs with well-defined atomic center coordination distances have been produced. Two different single-atom sites in the catalysts were homogeneously distributed, providing great opportunities for facilitating catalytic activity with synergetic effects. These DACs prepared from versatile synthetic approaches show different geometric distances and synergistic effects, leading to distinct behaviors in catalytic processes, particularly in the 2 + 1e− DAC EF systems focused on in this review.
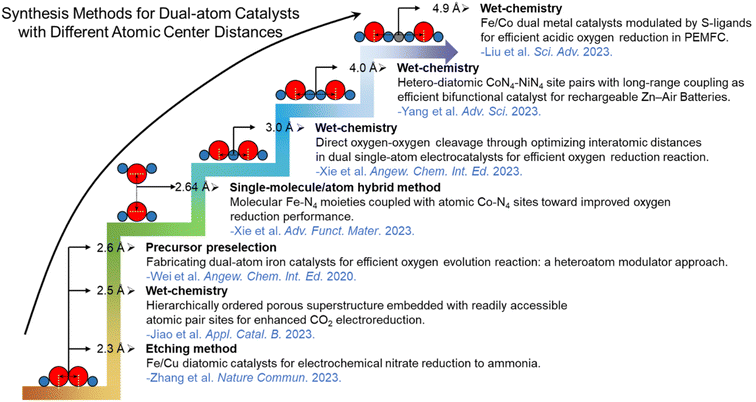 |
| Fig. 5 Summary of a synthesis method for DACs with different atomic center distances. | |
4.2 Structural design of electro-Fenton DACs
The metal atom centers of single-atom/dual-atom catalysts are considered to directly interact with the components and intermediates in the 2 + 1e− ORR. The d-orbital electrons of the central metal atom have a direct interaction with the p-orbital electrons of O2 and intermediate species to accomplish electron transfer, reflecting that the intrinsic properties of the central metal atom are particularly significant.88,89 Therefore, it is widely considered the actual active site of DACs and directly influences the activity and reaction pathway. Sun et al. reviewed single-site heterogeneous catalysts (SSHCs) present in EF, summarizing the 2e− ORR and 2 + 1e− ORR pathways and providing some discussion on SSHCs.89 However, there was no further summary or discussion on the selection of elements for EF catalysts. In fact, element selection is crucial in the design of EF catalysts. In Fig. 6, we present the commonly observed SACs (M–N4) in the EF system, along with homonuclear (MM′–N6)/heteronuclear (M–N4/M′–N4) DACs. The selection of the M (2e− ORR for H2O2 generation) metal center elements is primarily categorized into two groups: noble metals and transition metals. For example, platinum supported on carbon (Pt/C) has been extensively used as an electrocatalyst for the ORR.13 It is worth noting that Pt SACs exhibit a notably different catalytic behavior from traditional Pt/C. The O2 adsorbed onto atomically dispersed Pt followed a 2e− pathway, showing a high yield of H2O2. This phenomenon is because two adjacent Pt atoms in the bulk Pt/C catalyst could bond with both two O atoms of O2 to form a “side-on” adsorption mode, which facilitates the splitting of the O–O bond, while the Pt single atom interacts with only one O atom by forming an “end-on” adsorption mode, which is not beneficial for the breakage of the O–O bond.
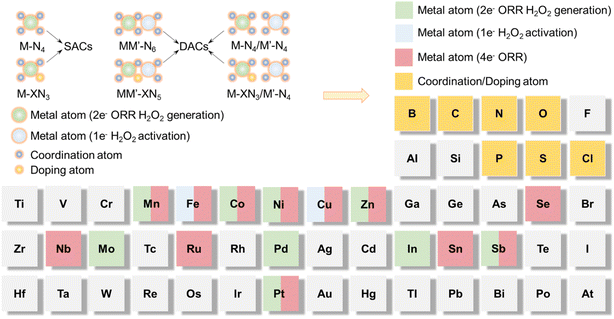 |
| Fig. 6 Schematic illustrations of electro-Fenton DAC and DAC catalytic atomic elements. | |
SACs with a non-precious metal as the central atom have also been applied in the 2e− ORR. Meanwhile, Strasser's group prepared SACs with a M–N4 structure from ZIF-8 using metal acetate to guarantee geometry consistency except with varied central metal sites.14 The ORR selectivity for H2O2 at 0.1 V RHE followed the order of Co-NC (80%) > Ni-NC (52%) > NC (45%) > Mn-NC (43%) > Cu-NC (36%) > Fe-NC (28%). Theoretical calculations suggested that Fe- and Mn-NC displayed strong adsorption for *OOH, leading to more favorable O–O bond cleavage to form H2O, while Cu- and Ni-NC presented unsatisfactory H2O2 selectivity because of the inhibited formation of the *OOH intermediate. The selection of the M′ (1e− H2O2 activation) metal center elements is primarily limited to Fe and Cu (Fig. 6).59,79,90–92Fig. 6 demonstrates that in the summary of metal catalytic centers for EF DACs, most reactive metal centers can coordinate both 2e− and 4e− ORR processes. Two approaches can be adopted to improve the selectivity of metal catalytic centers: (1) by manipulating the coordination chemistry of the metal catalytic centers (MM′–XN5 and M–XN3/M′–N4, where X is a foreign doping atom such as B, O, S, and P) (Fig. 6),46,75,93 to fine-tune the electronic structure of the metal catalytic centers with suitable adsorption binding energy for O2/intermediates; (2) utilizing the tri-phase transfer of solid, liquid, and gas to separate the metal catalytic centers of DACs by hydrophilicity and hydrophobicity engineering.93 For instance, positioning the M catalytic centers at the hydrophobic interface will facilitate O2 adsorption for H2O2 production, while anchoring M′ catalytic centers at the hydrophilic interface will enhance H2O2 activation.94
5 Electro-Fenton DAC engineering design for wastewater treatment
Complementary to research on developing promising EF DACs is the equally important pursuit of engineering the catalyst and other reactor components into an efficient device. The EF reactor design plays a critical role in scaling up electrochemical cells from the laboratory scale to the industrial scale.
5.1 Reactor design of electro-Fenton DAC systems
The design of the cathode electrode structure is crucial for EF reactors. An ideal electrode structure should have sufficient active sites for oxygen adsorption, fast mass migration, allowing rapid oxygen diffusion and H2O2 desorption, electron transfer, and good stability. A typical air-cathode, composed of a hydrophobic gas-diffusion layer (GDL) facing the air and a submerged catalyst layer facing the electrolyte,95,96 maintains an O2 diffusion path from the air and then enables oxygen reduction on the cathodes. Chen et al. reported the fabrication of a solid–liquid–gas three-phase interface using Nafion, which not only inhibited the formation of O2˙− but also promoted its efficient conversion into H2O2 during the 2e− ORR process through iron doping (Fig. 7a).97 Zhao et al. reported a mesostructured catalyst with hydrophilicity/hydrophobicity by adjusting the polytetrafluoroethylene (PTFE) content in a graphite/carbon black/PTFE hybrid catalyst layer, aimed at improving 2e− ORR activity for efficient H2O2 generation (Fig. 7b).98 Geng et al. reported the preparation of a graphite felt electro-Fenton cathode with hydrophobic and hydrophilic properties using PVDF (Fig. 7c).99 These studies revealed that the ideal balance of hydrophilicity and hydrophobicity can result in a steady three-phase interface among the electrolyte solution, O2 and catalytic sites in the catalyst layer for the sustainable electro-generation of H2O2 (Fig. 7d). The principle behind hydrophobic/hydrophilic interfaces lies in the presence of relevant hydrophilic (–OH and –COOH) and hydrophobic (–CF2) groups. These groups can be rapidly introduced into the cathode surface by plasma etching of coatings containing Nafion, PTFE, or PVDF, thereby achieving a solid–liquid–gas three-phase interface. Therefore, three-phase mass transfer remains a critical issue for the engineering application of EF DAC electrodes. Despite the M–Nx and M′–Nx sites exhibiting excellent catalytic activity for converting O2 to H2O2 and H2O2 activation, the production capacity of H2O2 in the EF reactor is also influenced by reaction kinetics. Thus, enhancing mass transfer is key to the development of EF DAC electrodes.
 |
| Fig. 7 (a) Schematic drawing of the Nafion carbon nanotube. Reprinted with permission from ref. 97, copyright (2024), The American Chemical Society. (b) Schematic drawing of the PTFE carbon paper. Reprinted with permission from ref. 98, copyright (2019), The American Chemical Society. (c) Schematic drawing of the PTFE graphite felt. Reprinted with permission from ref. 99, copyright (2022), Elsevier. (d) Schematic drawing method for creating hydrophobic/hydrophilic sites. (e) Schematic illustration of the H-cell device. Reprinted with permission from ref. 100, copyright (2008), Wiley-VCH. (f) Schematic design of the developed 2e− WOR/2e− ORR electrosynthesis cell. Reprinted with permission from ref. 101, copyright (2020), Springer Nature. (g) Schematic illustration of the electrochemical filtration apparatus. Reprinted with permission from ref. 102, copyright (2021), the American Chemical Society. | |
The configuration of the EF reactor is also an essential factor affecting mass transfer. For practical applications, an H-cell setup was designed to evaluate the H2O2 electrosynthesis performance of large-area electrodes (Fig. 7e). The H-cell setup received considerable attention for its easy operation and capacity to generate bulk H2O2. The electrode was submerged in a liquid electrolyte. Yamanaka et al. studied H2O2 production using an H-cell in a one-pot batch reactor. In this system, the reported H2O2 production reached a maximum value of 0.289 mmol cm−2 h−1 at the beginning.100 However, the H2O2 electrosynthesis performance quickly declines, leading to low reaction rates, H2O2 concentrations, and faradaic efficiency. For the dual-chamber reactor, the cathode chamber and the anode chamber were separated with a proton-exchange membrane (PEM). However, the long distance between the cathode and the anode leads to an increase in the ion diffusion path accompanied by increased solution resistance. Moreover, the low solubility of O2 in liquid electrolytes further limits the achievable H2O2 production rate. Although H-cells have been widely used for preliminary catalyst screening, they still cannot accurately evaluate how electrocatalysts and electrodes behave in industrial reactors because H-cells are not continuous and the accumulated H2O2 can be further decomposed by the electrodes. Additionally, Xia et al. manifested a high efficiency of a membrane-free flow cell for H2O2 electrocatalytic generation (Fig. 7f).101 The maximum FE jumped to 200% because the hydrophilic carbon fiber paper could be used as the cathode and anode to simultaneously produce H2O2. This approach provides an excellent strategy for designing DAC EF reactors, as utilizing the anode and cathode simultaneously significantly enhances energy utilization efficiency. Recently, Guo et al. reported an electrochemical filtration apparatus that utilizes an Fe2O3/CNT filter cathode to intercept pollutants in wastewater, enabling the rapid degradation of pollutants by hydroxyl radicals produced on the cathode surface (Fig. 7g).102 This provides an excellent idea for the design of DAC EF cathodes. On this basis, DACs can be constructed into filter cathodes using commercial Whatman polycarbonate filtration. Furthermore, the filter cathode can be replaced with a GDL-type cathode loaded with porous DACs, offering a strategy for the engineering design of DAC cathodes for EF systems.
5.2 Engineering design of the electro-Fenton DAC system
The engineering design of electrochemical systems has always been a hotspot for environmental applications.25 Applying electrochemical reaction systems to engineering involves considering the following two aspects: (1) cost, including the fabrication/construction of catalysts and reactors compared to traditional wastewater treatment systems, which is also the current bottleneck in the engineering application of electrocatalysts; (2) environmental friendliness, as the electrochemical catalysis system needs not only to purify wastewater but also to avoid generating secondary pollution (such as metal leaching and disinfection products). Xu et al. reported that inspired by the respiratory system of fish, they developed a localized O2 concentration (LOC) strategy by in situ constructing an O2 concentration reticular framework (RF) on the cathode to improve O2 utilization efficiency (OUE) (Fig. 8a and b).103 This approach can reduce or even eliminate the need for external oxygen supply, providing a conceptual advance for designing energy-efficient EF processes. Moreover, Xu et al. demonstrated that Cu–N–C could produce H2O2 at a low cost.37 The total cost for producing 10 g L−1 H2O2 in 0.1 M Na2SO4 was only US$2.93 per m3, much lower than the traditional anthraquinone method. Moreover, the produced H2O2 can treat synthetic wastewater (containing 10 ppm triclosan, 17α-ethinyl estradiol, and cefazolin sodium) to be below the detection limit. Zebrafish embryo teratogenicity analysis demonstrated that the effluent was safe for ecosystems (Fig. 8c and d). Huang et al. reviewed the selection of energy inputs for H2O2 reactors, noting that green solar and wind energy can be employed.104 Under the global carbon neutrality goal, sustainable energy is the most ideal energy supply for the future. Wind and solar power generation are suitable energy sources for on-site production of H2O2. Additionally, energy can also be generated through waste incineration, which simultaneously achieves waste resource utilization. Therefore, we attempted to design an EF DAC wastewater treatment system, which is shown in Fig. 8e and includes 4 steps: (1) waste-to-energy, solar energy, and wind energy provide the necessary power for the EF DAC system; (2) wastewater flows into the EF DAC system, where the mineralization of organic pollutants can be achieved without the need for additional H2O2; (3) the solution further flows through an Fe3O4-carbon filter where the residual H2O2 is quenched; and (4) the treated effluent is discharged into the environment.
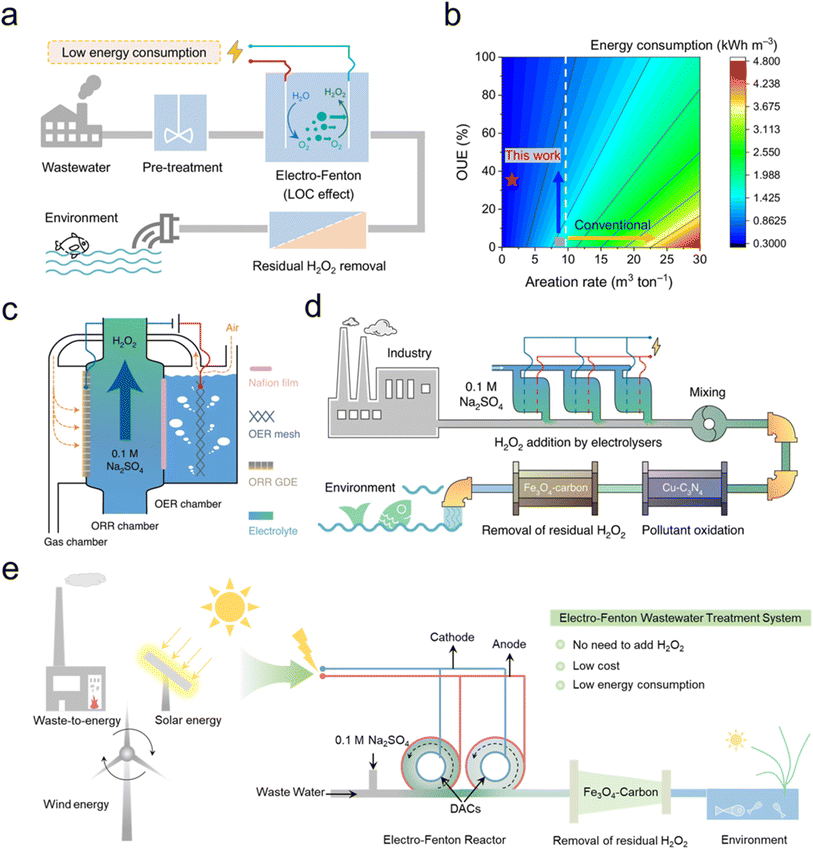 |
| Fig. 8 (a) Schematic drawing of the energy-efficient LOC electro-Fenton process for wastewater treatment. Reprinted with permission from ref. 103, copyright (2022), Proceedings of the National Academy of Sciences of the United States of America. (b) Technoeconomic analysis of the energy consumed by wastewater treatment with the electro-Fenton process. Reprinted with permission from ref. 103, copyright (2022), Proceedings of the National Academy of Sciences of the United States of America. (c) Scheme of the H2O2 electrolyzer. Reprinted with permission from ref. 37, copyright (2021), Springer Nature. (d) Schematic drawing of the wastewater treatment system. Reprinted with permission from ref. 37, copyright (2021), Springer Nature. (e) Schematic drawing of the electro-Fenton wastewater treatment system. | |
6 Conclusions and outlook
EF is a promising process for wastewater treatment applications, exhibiting the advantages of high catalytic activity in in situ formation and oriented decomposition of H2O2, as well as continuous operation and easy separation. The key component of EF is the catalyst materials, which can in situ electrochemically generate H2O2via the 2e− ORR, after which H2O2 spontaneously decomposes to generate ˙OH. Atomic-precision catalysts possess some appealing advantages in various catalytic reactions. Here, we summarize various single atom catalysts, focusing on the electrocatalytic formation of ˙OH via the 2 + 1e− pathway, which is highly correlated with the element type of the metal center as well as coordination/chemical environment. Overall, the synthesis of DACs aims to achieve bifunctional and successive catalysis from O2 toward ˙OH via the 2 + 1e− pathway. This ensures both high H2O2 selectivity and ˙OH yield, thus ultimately high electricity-to-chemical energy conversion efficiency.
Until now, all the research on DAC EF has been performed at the laboratory scale. The DAC EF process is a promising research area, where investigation trials should be implemented to pave the way for scaling up. To advance the engineering application of DAC EF, three core challenges must be addressed: (1) DAC design; (2) construction of hydrophobic/hydrophilic interfaces; (3) selection of power sources (Fig. 9). Additionally, real life wastewater generally involves complicated background constituents such as cations, anions, organics, and microorganisms, which might influence the overall behavior to an extent through consuming ˙OH, surface contamination, or coverage of active sites. Besides, the structure of DACs could be destroyed because of the attack on support substrates by ˙OH, resulting in slight leakage of metals. Therefore, several future research directions for DAC EF wastewater treatment systems are suggested. First, future research should endeavor to refine synthesis methodologies to afford precise control over the atomic configuration of DACs. The deployment of sophisticated characterization techniques will be instrumental in demystifying the atomic-level dynamics underpinning DAC catalytic efficacy, paving the way for the intelligent design of catalysts with enhanced activity and durability. Second, bridging the gap between laboratory success and industrial applicability necessitates addressing practical challenges, including catalyst stability, regeneration, and the economic viability of DAC-mediated EF processes. Finally, innovations in reactor design and optimization of operational parameters are crucial to fully leverage the potential of DACs in scalable wastewater treatment endeavors.
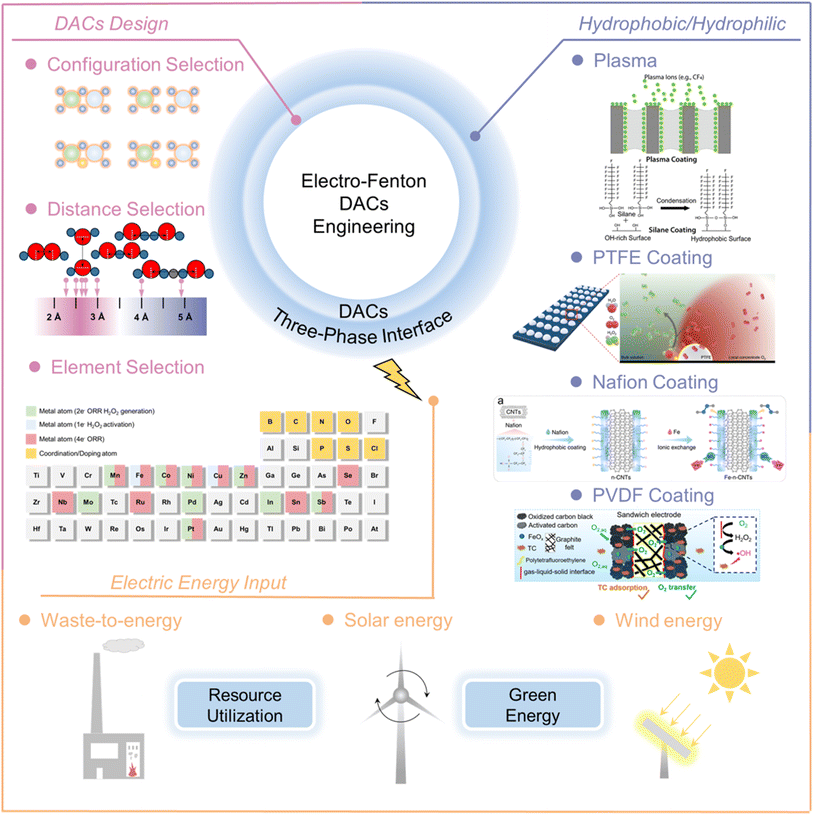 |
| Fig. 9 Illustration of the design in the development of the DAC electro-Fenton engineering. | |
In summary, there is still a long road ahead to achieving precise synthesis and a comprehensive understanding of DACs. The development of DAC-based catalysts represents the promising and state-of-the-art strategy responsible for the application of EF technology. Through the integration of advanced materials manufacturing, catalytic engineering, and system optimization, the development of DAC-based catalysts stands poised to be a transformative strategy, heralding a new paradigm shift in the application of EF technology.
Data availability
No primary research results, software or code have been included and no new data were generated or analyzed as part of this review.
Conflicts of interest
There are no conflicts to declare.
Acknowledgements
The authors acknowledge the financial support from the National Natural Science Foundation of China (Grant No. 52200055) and the Australian Research Council (DP230102406).
References
- F. Xiao, Z. Wang, J. Fan, T. Majima, H. Zhao and G. Zhao, Selective Electrocatalytic Reduction of Oxygen to Hydroxyl Radicals via 3-Electron Pathway with FeCo Alloy Encapsulated Carbon Aerogel for Fast and Complete Removing Pollutants, Angew. Chem., Int. Ed., 2021, 60(18), 10375–10383 CrossRef CAS PubMed
.
- H. Fu, J. Wei, G. Chen, M. Xu, J. Liu, J. Zhang, K. Li, Q. Xu, Y. Zou, W.-x. Zhang, S. Xi, X. Chen, S. Li and L. Ling, Axial Coordination Tuning Fe Single-atom Catalysts for Boosting H2O2 Activation, Appl. Catal., B, 2023, 321, 122012 CrossRef CAS
.
- J. Gao, H. b. Yang, X. Huang, S.-F. Hung, W. Cai, C. Jia, S. Miao, H. M. Chen, X. Yang, Y. Huang, T. Zhang and B. Liu, Enabling Direct H2O2 Production in Acidic Media through Rational Design of Transition Metal Single Atom Catalyst, Chem, 2020, 6(3), 658–674 CAS
.
- J. Hu, S. Wang, J. Yu, W. Nie, J. Sun and S. Wang, Duet Fe3C and FeNx Sites for H2O2 Generation and Activation toward Enhanced Electro-Fenton Performance in Wastewater Treatment, Environ. Sci. Technol., 2021, 55(2), 1260–1269 CrossRef CAS PubMed
.
- S. Yang, A. Verdaguer-Casadevall, L. Arnarson, L. Silvioli, V. Čolić, R. Frydendal, J. Rossmeisl, I. Chorkendorff and I. E. L. Stephens, Toward the Decentralized Electrochemical Production of H2O2: A Focus on the Catalysis, ACS Catal., 2018, 8(5), 4064–4081 CrossRef CAS
.
- Z. Ye, J. A. Padilla, E. Xuriguera, J. L. Beltran, F. Alcaide, E. Brillas and I. Sires, A Highly Stable Metal-Organic Framework-Engineered FeS2/C Nanocatalyst for Heterogeneous Electro-Fenton Treatment: Validation in Wastewater at Mild pH, Environ. Sci. Technol., 2020, 54(7), 4664–4674 CrossRef CAS PubMed
.
- C. Zhang, M. Zhou, G. Ren, X. Yu, L. Ma, J. Yang and F. Yu, Heterogeneous Electro-Fenton Using Modified Iron-carbon as Catalyst for 2,4-Dichlorophenol Degradation: Influence Factors, Mechanism and Degradation Pathway, Water Res., 2015, 70, 414–424 CrossRef CAS PubMed
.
- D. Zhang, K. Yin, Y. Tang, Y. Wei, H. Tang, Y. Du, H. Liu, Y. Chen and C. Liu, Hollow Sea-Urchin-Shaped Carbon-anchored Single-atom Iron as Dual-functional Electro-Fenton Catalysts for Degrading Refractory Thiamphenicol with Fast Reaction Kinetics in a Wide pH Range, Chem. Eng. J., 2022, 427, 130996 CrossRef CAS
.
- E. Zhang, L. Tao, J. An, J. Zhang, L. Meng, X. Zheng, Y. Wang, N. Li, S. Du, J. Zhang, D. Wang and Y. Li, Engineering the Local Atomic Environments of Indium Single-Atom Catalysts for Efficient Electrochemical Production of Hydrogen Peroxide, Angew. Chem., Int. Ed., 2022, 61(12), e202117347 CrossRef CAS PubMed
.
- Q. Zhang, X. Tan, N. M. Bedford, Z. Han, L. Thomsen, S. Smith, R. Amal and X. Lu, Direct Insights into the Role of Epoxy Groups on Cobalt Sites for Acidic H2O2 Production, Nat. Commun., 2020, 11(1), 4181 CrossRef PubMed
.
- S. Siahrostami, A. Verdaguer-Casadevall, M. Karamad, D. Deiana, P. Malacrida, B. Wickman, M. Escudero-Escribano, E. A. Paoli, R. Frydendal, T. W. Hansen, I. Chorkendorff, I. E. Stephens and J. Rossmeisl, Enabling Direct H2O2 Production Through Rational Electrocatalyst Design, Nat. Mater., 2013, 12(12), 1137–1143 CrossRef CAS PubMed
.
- P. Smith, Y. Kim, B. Benke, K. Kim and C. Chang, Supramolecular Tuning Enables Selective Oxygen Reduction Catalyzed by Cobalt Porphyrins for Direct Electrosynthesis of Hydrogen Peroxide, Angew. Chem., Int. Ed., 2020, 59(12), 4902–4907 CrossRef CAS PubMed
.
- Y. Sun, L. Han and P. Strasser, A Comparative Perspective of Electrochemical and Photochemical Approaches for Catalytic H2O2 Production, Chem. Soc. Rev., 2020, 49(18), 6605–6631 RSC
.
- Y. Sun, L. Silvioli, N. Sahraie, W. Ju, J. Li, A. Zitolo, S. Li, A. Bagger, L. Arnarson, X. Wang, T. Moeller, D. Bernsmeier, J. Rossmeisl, F. Jaouen and P. Strasser, Activity-Selectivity Trends in the Electrochemical Production of Hydrogen Peroxide over Single-Site Metal-Nitrogen-Carbon Catalysts, J. Am. Chem. Soc., 2019, 141(31), 12372–12381 CrossRef CAS PubMed
.
- J. Wang, S. Li, Q. Qin and C. Peng, Sustainable and Feasible Reagent-free Electro-Fenton Via Sequential Dual-cathode Electrocatalysis, Proc. Natl. Acad. Sci. U. S. A., 2021, 118(34), e2108573118 CrossRef CAS PubMed
.
- T. Wang, Z. Zeng, L. Cao, Z. Li, X. Hu, B. An, C. Wang and W. Lin, A Dynamically Stabilized Single-Nickel Electrocatalyst for Selective Reduction of Oxygen to Hydrogen Peroxide, Chemistry, 2018, 24(64), 17011–17018 CrossRef CAS PubMed
.
- P. Cao, X. Quan, K. Zhao, S. Chen, H. Yu and Y. Su, High-Efficiency Electrocatalysis of Molecular Oxygen toward Hydroxyl Radicals Enabled by an Atomically Dispersed Iron Catalyst, Environ. Sci. Technol., 2020, 54(19), 12662–12672 CrossRef CAS PubMed
.
- S. Cheng, Y. Liu, H. Zheng, Y. Pan, J. Luo, J. Cao and F. Liu, FeCo-ZIF Derived Carbon-Encapsulated Metal Alloy as Efficient Cathode Material for Heterogeneous Electro-Fenton Reaction in 3-Electron ORR Pathway: Enhanced Performance in Alkaline Condition, Sep. Purif. Technol., 2023, 325, 124545 CrossRef CAS
.
- C. Dong, Z. Wang, C. Yang, X. Hu, P. Wang, X. Gong, L. Lin and X. Li, Dual-Functional Single-atomic Mo/Fe Clusters-decorated C3N5 Via Three Electron-pathway in Oxygen Reduction Reaction for Tandemly Removing Contaminants from Water, Proc. Natl. Acad. Sci. U. S. A., 2023, 120(39), e2305883120 CrossRef CAS PubMed
.
- X. Du, W. Fu, P. Su, J. Cai and M. Zhou, Internal-micro-electrolysis-enhanced Heterogeneous Electro-Fenton Process Catalyzed by Fe/Fe3C@PC Core–shell Hybrid for Sulfamethazine Degradation, Chem. Eng. J., 2020, 398, 125681 CrossRef CAS
.
- Z. Guo, Y. Xie, J. Xiao, Z. Zhao, Y. Wang, Z. Xu, Y. Zhang, L. Yin, H. Cao and J. Gong, Single-Atom Mn-N4 Site-Catalyzed Peroxone Reaction for the Efficient Production of Hydroxyl Radicals in an Acidic Solution, J. Am. Chem. Soc., 2019, 141(30), 12005–12010 CrossRef CAS PubMed
.
- P. Cao, X. Quan, X. Nie, K. Zhao, Y. Liu, S. Chen, H. Yu and J. Chen, Metal Single-site Catalyst Design for Electrocatalytic Production of Hydrogen Peroxide at Industrial-relevant Currents, Nat. Commun., 2023, 14(1), 172 CrossRef CAS PubMed
.
- S. Chen, T. Luo, X. Li, K. Chen, J. Fu, K. Liu, C. Cai, Q. Wang, H. Li, Y. Chen, C. Ma, L. Zhu, Y. R. Lu, T. Chan, M. Zhu, E. Cortes and M. Liu, Identification of the Highly Active Co-N4 Coordination Motif for Selective Oxygen Reduction to Hydrogen Peroxide, J. Am. Chem. Soc., 2022, 144(32), 14505–14516 CrossRef CAS PubMed
.
- M. Deng, D. Wang and Y. Li, General Design Concept of High-Performance Single-Atom-Site Catalysts for H2O2 Electrosynthesis, Adv. Mater., 2024, e2314340 CrossRef PubMed
.
- X. Huang, M. Song, J. Zhang, T. Shen, G. Luo and D. Wang, Recent Advances of Electrocatalyst and Cell Design for Hydrogen Peroxide Production, Nanomicro Lett., 2023, 15(1), 86 CAS
.
- E. Jung, H. Shin, B. Lee, V. Efremov, S. Lee, H. S. Lee, J. Kim, W. Hooch Antink, S. Park, K. Lee, S. Cho, J. Yoo, Y. Sung and T. Hyeon, Atomic-level Tuning of Co-N-C Catalyst for High-performance Electrochemical H2O2 Production, Nat. Mater., 2020, 19(4), 436–442 CrossRef CAS PubMed
.
- W. Zhang, Y. Chao, W. Zhang, J. Zhou, F. Lv, K. Wang, F. Lin, H. Luo, J. Li, M. Tong, E. Wang and S. Guo, Emerging Dual-Atomic-Site Catalysts for Efficient Energy Catalysis, Adv. Mater., 2021, 33(36), e2102576 CrossRef PubMed
.
- K. Jiang, S. Back, A. Akey, C. Xia, Y. Hu, W. Liang, D. Schaak, E. Stavitski, J. Norskov, S. Siahrostami and H. Wang, Highly Selective Oxygen Reduction to Hydrogen Peroxide on Transition Metal Single Atom Coordination, Nat. Commun., 2019, 10(1), 3997 CrossRef PubMed
.
- Z. Jin, D. Jiao, Y. Dong, L. Liu, J. Fan, M. Gong, X. Ma, Y. Wang, W. Zhang, L. Zhang, Y. Gen, D. Voiry, W. Zheng and X. Cui, Boosting Electrocatalytic Carbon Dioxide Reduction via Self-Relaxation of Asymmetric Coordination in Fe-Based Single Atom Catalyst, Angew. Chem., Int. Ed., 2024, 63(6), e202318246 CrossRef CAS PubMed
.
- H. Kim, I. Lee, J. Cho, S. Shin, H. Ham, J. Kim and H. Lee, Palladium Single-Atom Catalysts Supported on C@C3N4 for Electrochemical Reactions, ChemElectroChem, 2019, 6(18), 4757–4764 CrossRef CAS
.
- Y. Li, J. Chen, Y. Ji, Z. Zhao, W. Cui, X. Sang, Y. Cheng, B. Yang, Z. Li, Q. Zhang, L. Lei, Z. Wen, L. Dai and Y. Hou, Single-atom Iron Catalyst with Biomimetic Active Center to Accelerate Proton Spillover for Medical-level Electrosynthesis of H2O2 Disinfectant, Angew. Chem., Int. Ed., 2023, 62(34), e202306491 CrossRef CAS PubMed
.
- H. Shang, X. Zhou, J. Dong, A. Li, X. Zhao, Q. Liu, Y. Lin, J. Pei, Z. Li, Z. Jiang, D. Zhou, L. Zheng, Y. Wang, J. Zhou, Z. Yang, R. Cao, R. Sarangi, T. Sun, X. Yang, X. Zheng, W. Yan, Z. Zhuang, J. Li, W. Chen, D. Wang, J. Zhang and Y. Li, Engineering Unsymmetrically Coordinated Cu-S1N3 Single Atom Sites With Enhanced Oxygen Reduction Activity, Nat. Commun., 2020, 11(1), 3049 CrossRef CAS PubMed
.
- J. Shen, Y. Wen, H. Jiang, S. Yu, C. Dong, Y. Fan, B. Liu and C. Li, Identifying Activity Trends for the Electrochemical Production of H2O2 on M–N–C Single-Atom Catalysts Using Theoretical Kinetic Computations, J. Phys. Chem. C, 2022, 126(25), 10388–10398 CrossRef CAS
.
- J. Li, W. Xia, X. Xu, D. Jiang, Z. Cai, J. Tang, Y. Guo, X. Huang, T. Wang, J. He, B. Han and Y. Yamauchi, Selective Etching of Metal-Organic Frameworks for Open Porous Structures: Mass-Efficient Catalysts with Enhanced Oxygen Reduction Reaction for Fuel Cells, J. Am. Chem. Soc., 2023, 145(50), 27262–27272 CrossRef CAS PubMed
.
- Y. Wang, H. Su, Y. He, L. Li, S. Zhu, H. Shen, P. Xie, X. Fu, G. Zhou, C. Feng, D. Zhao, F. Xiao, X. Zhu, Y. Zeng, M. Shao, S. Chen, G. Wu, J. Zeng and C. Wang, Advanced Electrocatalysts with Single-Metal-Atom Active Sites, Chem. Rev., 2020, 120(21), 12217–12314 CrossRef CAS PubMed
.
- Y. Shang, X. Xu, B. Gao, S. Wang and X. Duan, Single-atom Catalysis in Advanced Oxidation Processes for Environmental Remediation, Chem. Soc. Rev., 2021, 50(8), 5281–5322 RSC
.
- J. Xu, X. Zheng, Z. Feng, Z. Lu, Z. Zhang, W. Huang, Y. Li, D. Vuckovic, Y. Li, S. Dai, G. Chen, K. Wang, H. Wang, J. Chen, W. Mitch and Y. Cui, Organic Wastewater Treatment by a Single-atom Catalyst and Electrolytically Produced H2O2, Nat. Sustain., 2021, 4, 233–241 CrossRef PubMed
.
- Y. Shi, Z. Ma, Y. Xiao, Y. Yin, W. Huang, Z. Huang, Y. Zheng, F. Mu, R. Huang, G. Shi, Y. Sun, X. Xia and W. Chen, Electronic Metal-support Interaction Modulates Single-atom Platinum Catalysis for Hydrogen Evolution Reaction, Nat. Commun., 2021, 12(1), 3021 CrossRef PubMed
.
- S. Fang, X. Zhu, X. Liu, J. Gu, W. Liu, D. Wang, W. Zhang, Y. Lin, J. Lu, S. Wei, Y. Li and T. Yao, Uncovering Near-free Platinum Single-atom Dynamics During Electrochemical Hydrogen Evolution Reaction, Nat. Commun., 2020, 11(1), 1029 CrossRef CAS PubMed
.
- S. Gutić, A. Dobrota, E. Fako, N. Skorodumova, N. López and I. Pašti, Hydrogen Evolution Reaction-from Single Crystal to Single Atom Catalysts, Catalysts, 2020, 10(3), 290 CrossRef
.
- W. Lai, H. Wang, L. Zheng, Q. Jiang, Z. C. Yan, L. Wang, H. Yoshikawa, D. Matsumura, Q. Sun and Y. Wang, General Synthesis of Single-atom Catalysts for Hydrogen Evolution Reactions and Room-temperature Na-S Batteries, Angew. Chem., Int. Ed., 2020, 59(49), 22171–22178 CrossRef CAS PubMed
.
- K. Shah, R. Dai, M. Mateen, Z. Hassan, Z. Zhuang, C. Liu, M. Israr, W. Cheong, B. Hu and R. Tu, Cobalt Single Atom Incorporated in Ruthenium Oxide Sphere: A Robust Bifunctional Electrocatalyst for HER and OER, Angew. Chem., 2022, 134(4), e202114951 CrossRef
.
- S. Talib, Z. Lu, X. Yu, K. Ahmad, B. Bashir, Z. Yang and J. Li, Theoretical Inspection of M1/PMA Single-atom Electrocatalyst: Ultra-high Performance for Water Splitting (HER/OER) and Oxygen Reduction Reactions (OER), ACS Catal., 2021, 11(14), 8929–8941 CrossRef CAS
.
- Y. Ying, K. Fan, X. Luo, J. Qiao and H. Huang, Unravelling the Origin of Bifunctional OER/ORR Activity for Single-atom Catalysts Supported on C2N by DFT and Machine Learning, J. Mater. Chem. A, 2021, 9(31), 16860–16867 RSC
.
- J. An, N. Li, Q. Zhao, Y. Qiao, S. Wang, C. Liao, L. Zhou, T. Li, X. Wang and Y. Feng, Highly Efficient Electro-generation of H2O2 by Adjusting Liquid-gas-solid Three Phase Interfaces of Porous Carbonaceous Cathode During Oxygen Reduction Reaction, Water Res., 2019, 164, 114933 CrossRef CAS PubMed
.
- J. Zhao, C. Fu, K. Ye, Z. Liang, F. Jiang, S. Shen, X. Zhao, L. Ma, Z. Shadike, X. Wang, J. Zhang and K. Jiang, Manipulating the Oxygen Reduction Reaction Pathway on Pt-coordinated Motifs, Nat. Commun., 2022, 13(1), 685 CrossRef CAS PubMed
.
- M. Li, H. Wang, W. Luo, P. Sherrell, J. Chen and J. Yang, Heterogeneous Single-atom Catalysts for Electrochemical CO2 Reduction Reaction, Adv. Mater., 2020, 32(34), 2001848 CrossRef CAS PubMed
.
- L. Gong, D. Zhang, C. Lin, Y. Zhu, Y. Shen, J. Zhang, X. Han, L. Zhang and Z. Xia, Catalytic Mechanisms and Design Principles for Single-atom Catalysts in Highly Efficient CO2 Conversion, Adv. Energy Mater., 2019, 9(44), 1902625 CrossRef CAS
.
- H. Zhang, J. Li, S. Xi, Y. Du, X. Hai, J. Wang, H. Xu, G. Wu, J. Zhang and J. Lu, A Graphene-supported Single-atom FeN5 Catalytic Site for Efficient Electrochemical CO2 Reduction, Angew. Chem., 2019, 131(42), 15013–15018 CrossRef
.
- Z. Geng, Y. Liu, X. Kong, P. Li, K. Li, Z. Liu, J. Du, M. Shu, R. Si and J. Zeng, Achieving a Record-high Yield Rate of 120.9 for N2 Electrochemical Reduction over Ru Single-atom Catalysts, Adv. Mater., 2018, 30(40), 1803498 CrossRef PubMed
.
- C. Choi, S. Back, N. Kim, J. Lim, Y. Kim and Y. Jung, Suppression of Hydrogen Evolution Reaction in Electrochemical N2 Reduction Using Single-atom Catalysts: A Computational Guideline, ACS Catal., 2018, 8(8), 7517–7525 CrossRef CAS
.
- X. Li, P. Shen, Y. Luo, Y. Li, Y. Guo, H. Zhang and K. Chu, PdFe Single-Atom Alloy Metallene for N2 Electroreduction, Angew. Chem., 2022, 134(28), e202205923 CrossRef
.
- J. Liu, Z. Wei, Z. Gong, M. Yan, Y. Hu, S. Zhao, G. Ye and H. Fei, Single-atom CoN4 Sites with Elongated Bonding Induced by Phosphorus Doping for Efficient H2O2 Electrosynthesis, Appl. Catal., B, 2023, 324, 122267 CrossRef CAS
.
- L. Li, K. Yuan and Y. Chen, Breaking the Scaling Relationship Limit: From Single-Atom to Dual-Atom Catalysts, Acc. Mater. Res., 2022, 3(6), 584–596 CrossRef CAS
.
- R. Li and D. Wang, Superiority of Dual-Atom Catalysts in Electrocatalysis: One Step Further Than Single-Atom Catalysts, Adv. Energy Mater., 2022, 12(9), 2103564 CrossRef CAS
.
- W. Yang, Z. Jia, B. Zhou, L. Wei, Z. Gao and H. Li, Surface States of Dual-atom Catalysts Should be Considered for Analysis of Electrocatalytic Activity, Commun. Chem., 2023, 6(1), 6 CrossRef CAS PubMed
.
- X. Qin, P. Cao, X. Quan, K. Zhao, S. Chen, H. Yu and Y. Su, Highly Efficient Hydroxyl Radicals Production Boosted by the Atomically Dispersed Fe and Co Sites for Heterogeneous Electro-Fenton Oxidation, Environ. Sci. Technol., 2023, 57(7), 2907–2917 CrossRef CAS PubMed
.
- G. Yang, J. Zhu, P. Yuan, Y. Hu, G. Qu, B. Lu, X. Xue, H. Yin, W. Cheng, J. Cheng, W. Xu, J. Li, J. Hu, S. Mu and J. N. Zhang, Regulating Fe-spin State by Atomically Dispersed Mn-N in Fe-N-C Catalysts with High Oxygen Reduction Activity, Nat. Commun., 2021, 12(1), 1734 CrossRef CAS PubMed
.
- P. Xia, Z. Ye, L. Zhao, Q. Xue, S. Lanzalaco, Q. He, X. Qi and I. Sirés, Tailoring Single-Atom FeN4 Moieties as a Robust Heterogeneous Catalyst for High-performance Electro-Fenton Treatment of Organic Pollutants, Appl. Catal., B, 2023, 322, 122116 CrossRef CAS
.
- C. van Oversteeg, H. Doan, F. de Groot and T. Cuk, In situ X-ray Absorption Spectroscopy of Transition Metal Based Water Oxidation Catalysts, Chem. Soc. Rev., 2017, 46(1), 102–125 RSC
.
- W. Fan, Z. Duan, W. Liu, R. Mehmood, J. Qu, Y. Cao, X. Guo, J. Zhong and F. Zhang, Rational Design of Heterogenized Molecular Phthalocyanine Hybrid Single-atom Electrocatalyst Towards Two-electron Oxygen Reduction, Nat. Commun., 2023, 14(1), 1426 CrossRef CAS PubMed
.
- J. Wang, Z. Huang, W. Liu, C. Chang, H. Tang, Z. Li, W. Chen, C. Jia, T. Yao, S. Wei, Y. Wu and Y. Li, Design of N-Coordinated Dual-Metal Sites: A Stable and Active Pt-Free Catalyst for Acidic Oxygen Reduction Reaction, J. Am. Chem. Soc., 2017, 139(48), 17281–17284 CrossRef CAS PubMed
.
- L. Jiao, X. Li, W. Wei, S. Zhou, S. Han, D. Ma, Y. Mao, Q. Xu, X. Wu and Q. Zhu, Hierarchically Ordered Porous Superstructure Embedded with Readily Accessible Atomic Pair Sites for Enhanced CO2 Electroreduction, Appl. Catal., B, 2023, 330, 122638 CrossRef CAS
.
- H. Huang, M. Sun, S. Li, S. Zhang, Y. Lee, Z. Li, J. Fang, C. Chen, Y. Zhang, Y. Wu, Y. Che, S. Qian, W. Zhu, C. Tang, Z. Zhuang, L. Zhang and Z. Niu, Enhancing H2O2 Electrosynthesis at Industrial-Relevant Current in Acidic Media on Diatomic Cobalt Sites, J. Am. Chem. Soc., 2024, 146(13), 9434–9443 CrossRef CAS PubMed
.
- Y. Xie, X. Chen, K. Sun, J. Zhang, W. Lai, H. Liu and G. Wang, Direct Oxygen-Oxygen Cleavage through Optimizing Interatomic Distances in Dual Single-atom Electrocatalysts for Efficient Oxygen Reduction Reaction, Angew. Chem., Int. Ed., 2023, 62(17), e202301833 CrossRef CAS PubMed
.
- Y. Yang, B. Li, Y. Liang, W. Ni, X. Li, G. Shen, L. Xu, Z. Chen, C. Zhu, J. Liang and S. Zhang, Hetero-Diatomic CoN4-NiN4 Site Pairs with Long-Range Coupling as Efficient Bifunctional Catalyst for Rechargeable Zn-Air Batteries, Adv. Sci., 2024, e2310231 CrossRef PubMed
.
- P. Xie, H. Zhong, L. Fang, Z. Lyu, W. Yu, T. Li, J. Lee, H. Shin, S. Beckman, Y. Lin, S. Ding, I. Kim and J. Li, Molecular Fe-N4 Moieties Coupled with Atomic Co-N4 Sites Toward Improved Oxygen Reduction Performance, Adv. Funct. Mater., 2024, 2314554 CrossRef CAS
.
- D. Pacile, M. Papagno, A. Rodríguez, M. Grioni, L. Papagno, Ç. Girit, J. Meyer, G. Begtrup and A. Zettl, Near-edge X-ray Absorption Fine-structure Investigation of Graphene, Phys. Rev. Lett., 2008, 101(6), 066806 CrossRef CAS PubMed
.
- Y. Wang, H. Su, Y. He, L. Li, S. Zhu, H. Shen, P. Xie, X. Fu, G. Zhou and C. Feng, Advanced Electrocatalysts with Single-metal-atom Active Sites, Chem. Rev., 2020, 120(21), 12217–12314 CrossRef CAS PubMed
.
- J. Xi, S. Yang, L. Silvioli, S. Cao, P. Liu, Q. Chen, Y. Zhao, H. Sun, J. Hansen, J. Haraldsted, J. Kibsgaard, J. Rossmeisl, S. Bals, S. Wang and I. Chorkendorff, Highly Active, Selective, and Stable Pd Single-atom Catalyst Anchored on N-doped Hollow Carbon Sphere for Electrochemical H2O2 Synthesis under Acidic Conditions, J. Catal., 2021, 393, 313–323 CrossRef CAS
.
- X. Song, N. Li, H. Zhang, L. Wang, Y. Yan, H. Wang, L. Wang and Z. Bian, Graphene-Supported Single Nickel Atom Catalyst for Highly Selective and Efficient Hydrogen Peroxide Production, ACS Appl. Mater. Interfaces, 2020, 12(15), 17519–17527 CrossRef CAS PubMed
.
- H. Wang, Q. Zhu, R. Zou and Q. Xu, Metal-Organic Frameworks for Energy Applications, Chem, 2017, 2(1), 52–80 CAS
.
- X. Zhang, H. Su, P. Cui, Y. Cao, Z. Teng, Q. Zhang, Y. Wang, Y. Feng, R. Feng, J. Hou, X. Zhou, P. Ma, H. Hu, K. Wang, C. Wang, L. Gan, Y. Zhao, Q. Liu, T. Zhang and K. Zheng, Developing Ni Single-atom Sites in Carbon Nitride for Efficient Photocatalytic H2O2 Production, Nat. Commun., 2023, 14(1), 7115 CrossRef CAS PubMed
.
- J. Tang, S. Xu, K. Sun, X. Gao, A. Chen, S. Tian, D. Zhou and X. Sun, Recycling Synthesis of Single-atom Zn-nitrogen-carbon Catalyst for Electrocatalytic Reduction of O2 to H2O2, Sci. China Mater., 2022, 65(12), 3490–3496 CrossRef CAS
.
- C. Tang, Y. Jiao, B. Shi, J. N. Liu, Z. Xie, X. Chen, Q. Zhang and S. Z. Qiao, Coordination Tunes Selectivity: Two-Electron Oxygen Reduction on High-Loading Molybdenum Single-Atom Catalysts, Angew. Chem., Int. Ed., 2020, 59(23), 9171–9176 CrossRef CAS PubMed
.
- M. Yan, Z. Wei, Z. Gong, B. Johannessen, G. Ye, G. He, J. Liu, S. Zhao, C. Cui and H. Fei, Sb2S3-Templated Synthesis of Sulfur-doped Sb-N-C with Hierarchical Architecture and High Metal Loading for H2O2 Electrosynthesis, Nat. Commun., 2023, 14(1), 368 CrossRef CAS PubMed
.
- J. Gao and B. Liu, Progress of Electrochemical Hydrogen Peroxide Synthesis over Single Atom Catalysts, ACS Mater. Lett., 2020, 2(8), 1008–1024 CrossRef CAS
.
- R. Ren, X. Shang, Z. Song, C. Li, Z. Wang, F. Qi, A. Ikhlaq, J. Kumirska, E. Maria Siedlecka and O. Ismailova, Active Electronic Structure Derived by Fe-Cl-C Coordination of Single-atom Cathode Applied in Antibiotics Degradation by Electro-Fenton: Enhanced Transformation of Oxygen to Hydroxyl Radicals Via 3-Electron Pathway, Chem. Eng. J., 2023, 474, 145545 CrossRef CAS
.
- C. Gu, S. Wang, A. Zhang, C. Liu, J. Jiang and H. Yu, Slow-release Synthesis of Cu Single-atom Catalysts with the Optimized Geometric Structure and Density of State Distribution for Fenton-like Catalysis, Proc. Natl. Acad. Sci. U. S. A., 2023, 120(43), e2311585120 CrossRef CAS PubMed
.
- K. Zhao, X. Quan, Y. Su, X. Qin, S. Chen and H. Yu, Enhanced Chlorinated Pollutant Degradation by the Synergistic Effect between Dechlorination and Hydroxyl Radical Oxidation on a Bimetallic Single-Atom Catalyst, Environ. Sci. Technol., 2021, 55(20), 14194–14203 CrossRef CAS PubMed
.
- Y. Hu, Z. Li, B. Li and C. Yu, Recent Progress of Diatomic Catalysts: General Design Fundamentals and Diversified Catalytic Applications, Small, 2022, 18(46), e2203589 CrossRef PubMed
.
- J. Noel, A. Latus, C. Lagrost, E. Volanschi and P. Hapiot, Evidence for ·OH Radical Production During Electrocatalysis of Oxygen Reduction on Pt Surfaces: Consequences and Application, J. Am. Chem. Soc., 2012, 134(5), 2835–2841 CrossRef CAS PubMed
.
- S. Zhang, J. Wu, M. Zheng, X. Jin, Z. Shen, Z. Li, Y. Wang, Q. Wang, X. Wang, H. Wei, J. Zhang, P. Wang, S. Zhang, L. Yu, L. Dong, Q. Zhu, H. Zhang and J. Lu, Fe/Cu Diatomic Catalysts for Electrochemical Nitrate Reduction to Ammonia, Nat. Commun., 2023, 14(1), 3634 CrossRef CAS PubMed
.
- Y. Zhang, S. Zhang, H. Huang, X. Liu, B. Li, Y. Lee, X. Wang, Y. Bai, M. Sun, Y. Wu, S. Gong, X. Liu, Z. Zhuang, T. Tan and Z. Niu, General Synthesis of a Diatomic Catalyst Library via a Macrocyclic Precursor-Mediated Approach, J. Am. Chem. Soc., 2023, 145(8), 4819–4827 CrossRef CAS PubMed
.
- C. Chen, J. Chai, M. Sun, T. Guo, J. Lin, Y. Zhou, Z. Sun, F. Zhang, L. Zhang, W. Chen and Y. Li, An Asymmetrically Coordinated ZnCoFe Hetero-trimetallic Atom Catalyst Enhances the Electrocatalytic Oxygen Reaction, Energy Environ. Sci., 2024, 17(6), 2298–2308 RSC
.
- Y. Zhang, H. Liu, F. Gao, X. Tan, Y. Cai, B. Hu, Q. Huang, M. Fang and X. Wang, Application of MOFs and COFs for photocatalysis in CO2 reduction, H2 generation, and environmental treatment, EnergyChem, 2022, 4(4), 100078 CrossRef CAS
.
- F. Mo, Q. Zhou, C. Li, Z. Tao, Z. Hou, T. Zheng, Q. Wang, S. Ouyang and S. Zhan, Diatomic catalysts for Fenton and Fenton-like reactions: a promising platform for designing/regulating reaction pathways, Chem. Sci., 2023, 14(29), 7818–7827 RSC
.
- X. Li, X. Huang, S. Xi, S. Miao, J. Ding, W. Cai, S. Liu, X. Yang, H. Yang, J. Gao, J. Wang, Y. Huang, T. Zhang and B. Liu, Single Cobalt Atoms Anchored on Porous N-Doped Graphene with Dual Reaction Sites for Efficient Fenton-like Catalysis, J. Am. Chem. Soc., 2018, 140(39), 12469–12475 CrossRef CAS PubMed
.
- Y. Sun, P. Cai, D. Yang and X. Yao, Single-site Catalysis in Heterogeneous Electro-Fenton Reaction for Wastewater Remediation, Chem Catal., 2022, 2(4), 679–692 CrossRef CAS
.
- Y. Yin, L. Shi, W. Li, X. Li, H. Wu, Z. Ao, W. Tian, S. Liu, S. Wang and H. Sun, Boosting Fenton-Like Reactions via Single Atom Fe Catalysis, Environ. Sci. Technol., 2019, 53(19), 11391–11400 CrossRef CAS PubMed
.
- Q. Wu, J. Wang, Z. Wang, Y. Xu, Z. Xing, X. Zhang, Y. Guan, G. Liao and X. Li, High-loaded Single Cu Atoms Decorated on N-doped Graphene for Boosting Fenton-like Catalysis Under Neutral pH, J. Mater. Chem. A, 2020, 8(27), 13685–13693 RSC
.
- H. Yang, Y. Liu, X. Liu, X. Wang, H. Tian, G. Waterhouse, P. Kruger, S. Telfer and S. Ma, Large-scale synthesis of N-doped carbon capsules supporting atomically dispersed iron for efficient oxygen reduction reaction electrocatalysis, eScience, 2022, 2(2), 227–234 CrossRef
.
- X. Cheng, J. Hu, W. Shang, J. Guo, C. Xin, S. Zhang, S. Song, W. Liu and Y. Shi, Asymmetrically Ligated Single Atomic Nickel Sites for Efficient Hydrogen Peroxide Electrosynthesis, Nano Res., 2023, 1–7 Search PubMed
.
- W. Zhou, X. Meng, J. Gao, F. Sun and G. Zhao, Janus Graphite Felt Cathode Dramatically Enhance the H2O2 Yield from O2 Electroreduction by the Hydrophilicity-hydrophobicity Regulation, Chemosphere, 2021, 278, 130382 CrossRef CAS PubMed
.
- L. Cindrella, A. Kannan, J. Lin, K. Saminathan, Y. Ho, C. Lin and J. Wertz, Gas Diffusion Layer for Proton Exchange Membrane Fuel Cells—A review, J. Power Sources, 2009, 194(1), 146–160 CrossRef CAS
.
- S. Park, J. Lee and B. Popov, A Review of Gas Diffusion Layer in PEM Fuel Cells: Materials and Designs, Int. J. Hydrogen Energy, 2012, 37(7), 5850–5865 CrossRef CAS
.
- H. Chen, C. He, H. Niu, C. Xia, F. Li, W. Zhao, F. Song, T. Yao, Y. Chen, Y. Su, W. Guo and B. Xia, Surface Redox Chemistry Regulates the Reaction Microenvironment for Efficient Hydrogen Peroxide Generation, J. Am. Chem. Soc., 2024, 146(22), 15356–15365 CrossRef CAS PubMed
.
- Q. Zhao, J. An, S. Wang, Y. Qiao, C. Liao, C. Wang, X. Wang and N. Li, Superhydrophobic Air-Breathing Cathode for Efficient Hydrogen Peroxide Generation through Two-Electron Pathway Oxygen Reduction Reaction, ACS Appl. Mater. Interfaces, 2019, 11(38), 35410–35419 CrossRef CAS PubMed
.
- J. Geng, H. Zhang, Z. Zhang, J. Gao, S. Wang, X. Hu and J. Li, Enhanced electro-Fenton oxidation by introducing three-phase interface with simultaneous optimization of O2 and pollutant transfer for effective tetracycline hydrochloride removal, Chem. Eng. J., 2022, 450(1), 137891 CrossRef CAS
.
- I. Yamanaka and T. Murayama, Neutral H2O2 Synthesis by Electrolysis of Water and O2, Angew. Chem., 2008, 120(10), 1926–1928 CrossRef
.
- C. Xia, S. Back, S. Ringe, K. Jiang, F. Chen, X. Sun, S. Siahrostami, K. Chan and H. Wang, Confined local oxygen gas promotes electrochemical water oxidation to hydrogen peroxide, Nat. Catal., 2020, 3, 125–134 CrossRef CAS
.
- D. Guo, Y. Liu, H. Ji, C. Wang, B. Chen, C. Shen, F. Li, Y. Wang, P. Lu and W. Liu, Silicate-Enhanced Heterogeneous Flow-Through Electro-Fenton System Using Iron Oxides under Nanoconfinement, Environ. Sci. Technol., 2021, 55(6), 4045–4053 CrossRef CAS PubMed
.
- B. Xu, Z. Lin, F. Li, T. Tao, G. Zhang and Y. Wang, Local O2 concentrating boosts the electro-Fenton process for energy-efficient water remediation, Proc. Natl. Acad. Sci. U. S. A., 2024, 121(11), e2317702121 CrossRef PubMed
.
- X. Huang, M. Song, J. Zhang, T. Shen, G. Luo and D. Wang, Recent Advances of Electrocatalyst and Cell Design for Hydrogen Peroxide Production, Nano-Micro Lett., 2023, 15, 86 CrossRef CAS PubMed
.
|
This journal is © The Royal Society of Chemistry 2024 |
Click here to see how this site uses Cookies. View our privacy policy here.