Enhancing maize stress tolerance with nickel ferrite nanoparticles: a sustainable approach to combat abiotic stresses
Received
7th July 2024
, Accepted 1st October 2024
First published on 16th October 2024
Abstract
The use of nanotechnology to pre-treat crop seeds through seed treatments for enhancing their resistance to abiotic stresses is a promising and sustainable approach. This study demonstrates for the first time the potential of nickel ferrite (NiFe2O4) nanoparticles (NPs) in improving the tolerance of maize (Zea mays L.) exposed to drought and salt stress conditions. This study fills the current gap in understanding whether metal ferrite nanoparticles can mitigate abiotic stresses in crops, especially under hydric and saline stress. In this study, NiFe2O4 NPs were used as seed pretreatments to enhance the resistance of maize (Zea mays L.) experiencing drought and salt stress. We conducted a 7 day germination experiment and a 3-week seedling growth experiment to assess the impact of NiFe2O4 NPs on key growth parameters such as seed germination, seedling vigor, root and shoot length, and biomass accumulation. The findings indicated that under drought conditions, 40 mg L−1 NiFe2O4 NPs was the most effective concentration, leading to a substantial increase in the germination rate by 90%. Under salt stress, 20 mg L−1 was the optimal concentration, which resulted in a significant increase in seedling vigor by 521%, shoot length by 177%, and so on. In addition, NiFe2O4 NPs exhibited peroxidase (POD)-like activity, which could increase the antioxidant capacity of maize seedlings, thereby enhancing their stress tolerance. These results offer a theoretical foundation for the use of NiFe2O4 NPs in agricultural practices and highlight their unique potential for promoting plant resistance and sustainable agricultural practices. Although these results are promising, extensive research is needed to comprehensively elucidate the mechanisms through which NiFe2O4 NPs enhance stress tolerance. Future research should explore the prolonged effects of NiFe2O4 NPs on the growth of plants and yield, their potential environmental impacts, and their broader applicability. In addition, there is still a need to explore the interplay between NiFe2O4 NPs and other biotic or abiotic factors to optimize their application in agricultural systems.
Environmental significance
Climate change and its associated stressors, such as drought and soil salinisation, pose a major challenge to global agricultural productivity and food security. Conventional methods of mitigating these stressors usually involve the use of chemical treatments, which may have harmful effects on the environment. In this study, we investigated the use of nickel ferrite (NiFe2O4) nanoparticles as a novel and sustainable pre-treatment for maize (Zea mays L.) seeds and seedlings to enhance their resilience to abiotic stresses. Moreover, it has been shown that NiFe2O4 nanoparticles have antimicrobial activity and are non-toxic. This study highlights the potential of NiFe2O4 nanoparticles as an effective and sustainable agricultural intervention that reduces the reliance on environmentally harmful chemical treatments. By increasing crop resilience to unfavourable environmental conditions, this approach contributes to sustainable agricultural practices and enhances food security in the context of climate change.
|
1. Introduction
Seeds initiate the life cycle of plants, and their germination phase profoundly impacts agricultural quality and production.1,2 Amid global climate change, seeds frequently encounter diverse environmental stresses such as drought, salinity, and heat waves, in addition to biotic stresses from pathogens in soil.3 Numerous studies have documented the adverse effects of these stressors on crop performance; however, innovative solutions remain limited. Salinity stress and drought stress are two common abiotic factors that directly influence crop growth and development, reducing both yield and quality. For example, the accumulation of soil salts reduces the osmotic pressure of underground solution,4 which in turn prevents water uptake by seeds and roots and poisons the embryo with salts and chlorides, thus preventing the production of proteins and thereby hindering plant development and growth.4,5 Conversely, drought leads to inadequate soil moisture, which hinders crop development and ultimately lowers production.6
Maize (Zea mays L.) ranks as the third-largest grain crop grown globally after rice and wheat,7 displays moderate sensitivity to salt stress,5 and can adapt to various environmental conditions. The uncertainties stemming from climate change directly influence global maize yields and quality, posing a critical challenge to global food security. Developing advanced technological methods that expedite seed germination and foster robust seedling growth under challenging settings is crucial for minimizing yield losses and ensuring food security.8,9
Nanotechnology offers new ideas and approaches to solve key problems in agricultural production.10,11 Recent developments in nanotechnology have highlighted the potential of nanoparticles to boost plant tolerance to abiotic stresses. Previous research has demonstrated that metal oxide nanoparticles, including zinc oxide, silica, iron oxide, copper oxide, magnesium oxide, and titanium dioxide, can improve plant growth and stress tolerance by influencing the antioxidant activity and nutrient absorption.12–17 Unlike previous studies focusing on more common nanomaterials such as carbon nanomaterials and metal oxide nanoparticles, our study uniquely investigates NiFe2O4 NPs with low toxicity and biocompatibility in enhancing the stress tolerance of maize.
Ferrite nanoparticles (MFe2O4, where M = Fe, Mn, Co, Ni, etc.)18,19 are highly valued for their superparamagnetic characteristics and extensive applications in medicine,20–22 magnetic energy storage, catalysis,23 electronics, and computing.24 They are especially appealing due to the relatively low cost of raw materials, straightforward synthesis methods (e.g., co-precipitation,25 sol–gel method,26 and hydrothermal synthesis27), and robust magnetic properties compared to other metallic magnetic particles.24 Among these, NiFe2O4 NPs are particularly noteworthy due to their excellent photocatalytic activity, biocompatibility,28,29 chemical stability, and controllability.30–32 It has been shown that NiFe2O4 NPs exhibit low cytotoxicity in a variety of tests. For example, the response of 15–20 mg NiFe2O4 NPs at different pH values was tested in agar overlay (containing tumor cells) and MTT assays, which showed no significant cytotoxicity.33 In addition, Ala Manohar et al. further verified this result. They found that NiFe2O4 NPs were non-toxic to normal human dermal fibroblasts (HDF) and murine melanoma cell lines (B16-F10) at concentrations varying from 0 to 1000 μg mL−1.34 Furthermore, other studies have evaluated the in vitro toxicity of NiFe2O4 NPs on various cancer cell lines, such as HeLa (cervical cancer cells) and PC-3 (prostate cancer cells), and the results showed that NiFe2O4 NPs were virtually non-toxic in the concentration range from 1 to 100 μg mL−1.28 In summary, a large number of studies have demonstrated that NiFe2O4 NPs have good biocompatibility and low toxicity, which can be applied in the fields of thermotherapy, targeted drug delivery, etc.,28,33–36 making them ideal candidates for improving crop resistance and promoting sustainable agriculture.
In conclusion, nanotechnology has become a promising method for enhancing crop resistance to abiotic stress. Previous research reveals that various nanoparticles (NPs) possess the capacity to improve plant stress resistance through mechanisms such as enhanced nutrient uptake and activation of stress-related enzymes. However, there is still a significant gap in understanding the specific effects of magnetic nanoparticles (especially metal ferrites) on crops. This study aims to fill this gap by investigating the effects of NiFe2O4 NPs on maize seedlings under drought and salt stress conditions. The novelty of this study is that it focuses on the dual application of NiFe2O4 NPs to mitigate drought and salt stress, providing a sustainable approach to improve crop resilience under different environmental conditions. In addition, the cost-effectiveness, low toxicity, and biocompatibility of NiFe2O4 NPs greatly reduce environmental risks and are scalable, providing a practical solution for large-scale agricultural applications to boost crop productivity under stress. This research lays the theoretical and practical foundation for the future development of efficient resistant maize varieties and sustainable agricultural production technologies.
2. Materials and methods
2.1 Characterization of nanoparticles
We purchased NiFe2O4 NPs (30 nm) from Bide Pharmatech Ltd. (https://www.bidepharm.com/) in Shanghai, China. The TEM (JEM-1200EX, JEOL), zeta potential, hydrodynamic diameter, and FTIR (VERTEX 70 FT-IR) results of NiFe2O4 NPs are shown in Fig. 1. The spherical shape and 20–30 nm size range of NiFe2O4 NPs were validated.
 |
| Fig. 1 TEM micrographs of NiFe2O4 NPs. (A) Scale bar = 200 nm. (B) Scale bar = 100 nm, (C) scale bar = 50 nm. Zeta potential (D), hydrodynamic diameter (E), and FTIR spectrum (F) of NiFe2O4 NPs. | |
2.2 Experimental design
Maize seeds (Zhengdan 958) were purchased from Hebei Jiafeng Seed Industry Co. To investigate the potential of NiFe2O4 NPs to enhance maize seed resilience under abiotic stress conditions, seeds were soaked in nanopore water or NiFe2O4 NP suspensions before being exposed to salt and drought stress conditions.37 The details are presented in Table 1.
Table 1 Experimental treatments under drought and salt stress conditions
Growth condition |
Priming method |
Treatment |
Abbreviations |
Drought (PEG 10%) |
Hydropriming |
1 |
CK-1 |
20 mg L−1 NiFe2O4 |
2 |
NPs-1 |
40 mg L−1 NiFe2O4 |
3 |
NPs-2 |
80 mg L−1 NiFe2O4 |
4 |
NPs-3 |
Drought (PEG 20%) |
Hydropriming |
5 |
CK-2 |
20 mg L−1 NiFe2O4 |
6 |
NPs-1 |
40 mg L−1 NiFe2O4 |
7 |
NPs-2 |
80 mg L−1 NiFe2O4 |
8 |
NPs-3 |
Salt (50 mM NaCl |
Hydropriming |
9 |
CK-3 |
20 mg L−1 NiFe2O4 |
10 |
NPs-1 |
40 mg L−1 NiFe2O4 |
11 |
NPs-2 |
80 mg L−1 NiFe2O4 |
12 |
NPs-3 |
Salt (100 mM NaCl) |
Hydropriming |
13 |
CK-4 |
20 mg L−1 NiFe2O4 |
14 |
NPs-1 |
40 mg L−1 NiFe2O4 |
15 |
NPs-2 |
80 mg L−1 NiFe2O4 |
16 |
NPs-3 |
1. Surface sterilization: maize seeds were submerged in a 5 percent sodium hypochlorite solution for about 10 minutes and rinsed 7–8 times with ultrapure water.38
2. Preparation of nanoparticle suspensions: NiFe2O4 NPs were suspended in ultrapure water at concentrations: 20 mg L−1, 40 mg L−1, and 80 mg L−1.39 Specifically, 4 mg, 8 mg, and 16 mg of NiFe2O4 NPs were weighed and placed in conical flasks with 200 mL ultrapure water, then sonicated at 45 Hz for 30 minutes.
3. Soaking seeds: conical flasks holding 125 mL of NiFe2O4 NPs suspensions were filled with sterilized seeds.37 In particular, 200 mL of the nanoparticle suspension was used to soak 40 g of seeds, which were then left in the dark at 25 °C for 1 day while being shaken at 100 rpm.37
4. Growth conditions: the treated seeds were positioned in 10 × 10 cm plates coated with filtrate paper in a medium volume of 5 milliliters.2 The growth medium varied depending on the stress condition.
• Non-stress: sterile ultrapure water
• Drought stress: polyethylene glycol (PEG) solutions at 10% and 20% concentrations40
• Salinity stress: NaCl solutions at 50 mM and 100 mM concentrations41
Ten seeds in each Petri dish were used to replicate each treatment four times. The culture was maintained at 25 °C, 70% relative humidity, and kept in the dark. Day 4: as needed, 2–3 mL of the matching growth medium were added to each dish. Measurements were made of biomass, shoot and root length, seed germination, and seedling vigor after 7 days.
2.3 Effects of NiFe2O4 NPs on corn seedling resilience
After one week of incubation, maize seeds were transplanted into 30 g of nutrient soil (Melaleuca, purchased from Taobao Melaleuca flagship store). Stress treatments were applied during the 3rd week of growth (3–4 cotyledon stage) for 1 week. For salt stress, solutions of 100 and 150 mM NaCl were applied to the soil (30 mL each time, every other day for a total of three applications). For drought stress, soil water content was controlled at about 30% using the weighing method.
2.4 Determination of germination rate and seedling vigor index
The germination rate refers to the speed at which seeds germinate over a specific period. The germination percentage refers to the total proportion of seeds that successfully germinate by the end of the experiment. Each day, the number of sprouted seeds was recorded to calculate the germination rate; seeds were deemed germination-positive if the shoot length was greater than half of the seed length.37
Based on Ali et al.,42 the seedling vigor index (VI) is a standard method for assessing seed viability in different environmental settings. VI was calculated through the use of a ruler to measure the seedlings' shoot and root lengths (in centimeters) after they had been incubated for seven days in the artificial climate incubator.43
2.5 Determination of relative water content
To measure the relative water content, the weight of the fresh sample (WF) was first measured. Then, the samples were submerged in distilled water at room temperature for 4 hours, protected from light, and their swelling weight (WT) was measured. The plants were then heated in an oven at 60 °C to a consistent weight medium and the weight after drying (WD) was measured. Finally, RWC was calculated according to the following formula.
Here, WF refers to the fresh weight, WD stands for the dry weight, and WT signifies the swollen weight.
2.6
In vitro nano-enzymatic assay
A type of mimetic enzymes known as nano-enzymes combines the distinctive qualities of nanomaterials with catalytic activities.44 These artificial enzymes offer several advantages, including facile modification, excellent stability, affordability, and variable activity in catalysis.44–46 To assess the enzymatic activity of NiFe2O4 NPs, the oxidation reaction rate of the substrate TMB was measured, expressed as changes in the absorbance or fluorescence intensity.47 Peroxidase (POD) activity was assessed using TMB and H2O2 as substrates.48,49 The POD-like activity of NiFe2O4 NPs was tested by sequentially adding PBS (pH = 3.0), NiFe2O4 NPs, TMB solution, and H2O2 in specific concentrations. UV-visible absorption spectra (500–800 nm) were recorded after a 30 minute reaction at room temperature, protected from light.
2.7 Electron paramagnetic resonance (EPR) determination of hydroxyl radicals
Electron paramagnetic resonance (EPR) is a state-of-the-art technique used to directly identify free radicals through spectral characterization.50 Briefly, 100 μL of 0.5 mM hydrogen peroxide was mixed with 100 μL of a 10 mM acetate buffer at pH 3.6, which contained 50 mM DMPO and 40 mg L−1 of the sample.
After a 5 minute reaction, the solution was collected using a capillary tube and inserted into the EPR chamber for data collection.51 The spectra of DMPO–OH radicals, formed from hydroxyl radicals captured by DMPO, were monitored using EPR spectroscopy (EPR, A300-10/12, Bruker).52
2.8 Determination of antioxidant enzymes
Samples from the drought and salt stress experiments (14 day fertility cycle) were collected, and leaves and roots were taken for antioxidant enzyme assays. Samples were homogenized in 0.05 M Tris-HCl-containing cold phosphate buffer solution (PBS, pH 7.4). For the test, the supernatant was gathered and known concentrations of SOD and MDA standards were prepared according to the sample analysis kit (Cominbio, Suzhou, China). Four replicate samples were tested, and experiments were repeated three times.
Superoxide dismutase (SOD): Weydert and Cullen53 used the xanthine/xanthine oxygen reaction method to quantify SOD activity. The assay was performed following the kit's instructions, with the absorbance measured at 560 nm.
Malondialdehyde (MDA): a red product was produced through the reaction of MDA with thiobarbituric acid (TBA), which allowed for the determination of the MDA level.54 As directed by the kit, absorption was determined at 532 nm and 600 nm.55
2.9 Determination of nickel, iron, and mineral elements in plants
The operational protocol complies with that described by Zhou et al.11 Plant samples underwent drying for 30 minutes at 105 °C and then again for another 30 minutes at 75 °C till their weight was stable. 8 mL of highly pure concentrated nitric acid was applied to about 0.2 g of the dried material that had been put in an ablution tube. After that, the tube was sealed with an open-ended stopper and kept out of the light for the whole night.
The materials were further broken down using a microwave digesting machine (MARS6, CEM, UK) the next day until the digest was reduced to 1 milliliter. After that, the digest was diluted to a final volume of 50 milliliters using ultrapure water. After passing over a 0.25 μm PTFE membrane, the fluid was diluted once again using ultrapure water. Next, using an Elan DRC-e inductively coupled plasma mass spectrometer (ICP-MS, PerkinElmer, USA), the concentrations of nickel, iron, and other mineral elements were ascertained.
2.10 Statistical methods for data analysis
To ensure the reliability of the data presented, we used rigorous statistical methods. We used a two-way analysis of variance (ANOVA) to compare the means of the different treatments with control groups and Tukey's honestly significant difference (HSD) test for pairwise comparisons. Statistical significance was defined as a p-value <0.05 as the critical value. All statistical analyses were performed using IBM SPSS Statistics 27, Origin 2024b, and GraphPad Prism 9 to ensure rigorous and reliable results.
3. Results and discussion
3.1 NiFe2O4 NPs triggering of maize seeds enhances their resistance to abiotic stresses
We conducted seed germination experiments and selected representative seven day-old seeds, as shown in Fig. 2. Under drought conditions induced by PEG at 10% and 20%, corn seeds treated with NiFe2O4 NPs significantly promoted root and shoot germination compared to those treated with pure water. The same trend was detected under conditions of salt stress with NaCl concentrations of 50 mM and 100 mM, where NiFe2O4 NPs-treated maize seeds significantly promoted root germination over the control. These observations align with the outcomes of earlier studies reporting the role of nanoparticles in enhancing seed germination and seedling vigor under abiotic stress conditions. For example, zinc oxide nanoparticles have been shown to improve drought tolerance in wheat by enhancing root development and water uptake.56,57 Similarly, zinc oxide nanoparticles improved salt tolerance in rice by promoting shoot and root growth.58,59
 |
| Fig. 2 Representative images of seeds at 7 days of age, triggered by NiFe2O4 NPs, under different intensities of drought stress and salt stress conditions. | |
Specifically, seeds treated with NiFe2O4 NPs under 10% PEG-induced drought conditions showed a significant increase in germination rate but not in the germination percentage (Fig. 3A and 4B). The germination rate increased from 82.5% to 87% with 40 mg L−1 NiFe2O4 NPs, while treatments with 20 mg L−1 and 80 mg L−1 also showed improvement (Fig. 3A). Under 20% PEG-induced drought conditions, NiFe2O4 NPs significantly promoted seed germination, increasing the germination percentage to 90% and 88% with 40 mg L−1 and 80 mg L−1 treatments, respectively (Fig. 3B).
 |
| Fig. 3 Effect of NiFe2O4 NPs on the maize seed germination rate under different intensities of drought (top) and salinity (bottom) stresses. Values are mean ± standard deviation (n = 4). | |
 |
| Fig. 4 Nanonickel ferrate initiation of maize seeds under PEG-induced drought conditions improves seed vigor, germination rate, and shoot and root length. (A) Seed vigor. (B) Germination rate. (C) Shoot length. (D) Root length. Values are mean ± standard deviation (n = 4). Different asterisks above the bars indicate significant differences between treatment groups, respectively (p < 0.05). | |
Seedling vigor under 10% PEG-induced drought stress significantly increased from 394 cm% to 536 cm% with 40 mg L−1 NiFe2O4 NPs and to 438.68 cm% and 457.1 cm% with 20 mg L−1 and 80 mg L−1 treatments, respectively (Fig. 4A). Under 20% PEG-induced drought conditions, seedling vigor increased significantly from 0 cm% to 370.8 cm% and 245.5 cm% with 40 mg L−1 and 80 mg L−1 treatments, respectively (Fig. 4A). Under 10% PEG-induced drought conditions, root length significantly increased by 20.21%, 37.15%, and 18.64% (Fig. 4C), shoot length by 12%, 42%, and 27% (Fig. 4D), and biomass by 8%, 16%, and 14% in the low- to high-concentration treatment groups (Fig. 7A).
In addition, we found that the 10% PEG treatment caused a noticeable increase in the plant relative water content across the NPs treated groups compared to the control (CK1), with the Ni-2 group exhibiting the highest retention at 90.47%, followed by Ni-1 at 83.43%, and Ni-3 at 78.03% (Fig. 5B). Under the more severe 20% PEG-induced drought stress, the Ni-2 group again demonstrated superior water retention (86.44%), with Ni-1 and Ni-3 groups maintaining similar levels. These findings indicate that NiFe2O4 NPs enhance drought tolerance in maize by promoting seed germination, increasing seedling vigor, and improving water retention, with 40 mg L−1 being the optimal treatment concentration.
 |
| Fig. 5 (A) The biomass of maize plants under drought stress and salt stress conditions. (B) The RWC of three-week-old maize plants under drought stress conditions. Ni-1, Ni-2, and Ni-3 represent the groups treated with different concentrations of NiFe2O4 NPs. Values are mean ± standard deviation (n = 4). Different asterisks above the bars indicate significant differences between the treatment groups (p < 0.05). | |
We observed that salt stress significantly inhibited seed root growth (Fig. 2). However, treatment with 20 mg L−1 and 40 mg L−1 NiFe2O4 NPs significantly improved seed germination to 90% (Fig. 6B), increased seedling vigor from 188.9 cm% to 1174.8 cm% and 1089.2 cm% (Fig. 6A), respectively, enhanced root length by 12.4% and 11.1% (Fig. 6D), and boosted shoot length by 177% and 168% (Fig. 6C), respectively. Biomass also increased by 26.2% and 20.7% (Fig. 7B). Conversely, under the same conditions, 80 mg L−1 NiFe2O4 NPs treatment resulted in significant improvements only in germination (83%) (Fig. 6B), seedling vigor (360.85 cm%) (Fig. 6A), and shoot length (123%) (Fig. 6C). In contrast, increases in root length (28%) (Fig. 6D) and biomass (7.8%) (Fig. 7B) were not significant.
 |
| Fig. 6 NiFe2O4 NPs initiation of maize seeds increases seed germination and vigor under salt stress conditions (A) seed vigor. (B) Germination rate. (C) Shoot length. (D) Root length. Values are mean ± standard deviation (n = 4). Different asterisks above the bars indicate significant differences between treatment groups (p < 0.05). | |
 |
| Fig. 7 Effect of NiFe2O4 NPs on corn seed biomass under different intensities of drought (left) and salinity (right) stresses. Values are mean ± standard deviation (n = 4). Different asterisks above the bars indicate significant differences between treatment groups (p < 0.05). | |
Under 50 mM NaCl-induced salt stress, seedling vigor increased significantly from 406.58 cm% to 1357.7 cm% and 1166.2 cm% (Fig. 6A), root length increased by 738% and 685% (Fig. 6D), respectively, shoot length increased significantly by 44% and 36% (Fig. 6C), and biomass increased by 18.8% and 14.9% (Fig. 7B). However, 80 mg L−1 NiFe2O4 NPs treatment under these conditions significantly increased only seedling vigor (346.9 cm%) (Fig. 6A), with non-significant increases in shoot length (4%) (Fig. 6C) and root length (37%) (Fig. 6D). This is similar to previous findings. For example, a study on the influence of copper oxide nanoparticles (CuO-NPs) on maize under saline conditions found that while some growth parameters improved, some results were not significant, especially at higher nanoparticle concentrations.60 This is similar to our findings that high concentrations of NiFe2O4 NPs had no significant effect on the root length. Another related study reported that higher doses of NPsmay sometimes lead to insignificant or even unfavorable effects due to potential toxicity or inhibitory effects on nutrient uptake.61 Overall, the use of NiFe2O4 NPs therapy, with the 20 mg L−1 concentration being the most effective, enhanced resistance against salt stress in seeds by considerably promoting seed germination and seedling vigor.
3.2 The enzyme activity of NiFe2O4 NPs is similar to POD
To find out if NiFe2O4 NPs may catalyze the creation of ROS, an in vitro nano enzymatic experiment was performed.62 The findings showed that in a mixture of hydrogen peroxide (H2O2) and an acidic environment, NiFe2O4 NPs efficiently catalyzed the oxidization of the target 3,5,3′,5′-tetramethylbenzidine (TMB)63,64 (Fig. 8A), displaying a strong peroxidase-like (POD) activity. The nano-enzymatic activity of NiFe2O4 NPs was assessed via a typical TMB oxidation reaction, which exhibited a characteristic absorption wavelength at 652 nm along with a blue color shift. As shown in Fig. 8C, when either TMB or NiFe2O4 NPs were mixed individually with H2O2, no absorption peaks were observed. In contrast, the mixture of NiFe2O4 NPs, TMB, and H2O2 presented strong absorption peaks, indicating superior POD activity. This phenomenon can be explained by a Fenton-like process2 where H2O is the Fenton's reagent, as also noted by He et al.65 According to Yan et al.,2 the suggested process entails the interaction of NiFe2O4 NPs with H2O2 to provide electrons, which then produce hydroxyl radicals (·OH). This result is consistent with earlier studies that highlight how metal oxide nanoparticles can simulate natural enzyme activities.66–69
 |
| Fig. 8 (A) POD-like enzyme activity of NiFe2O4 NPs. (B) EPR analysis revealed the production of hydroxyl radicals (·OH) by NiFe2O4 NPs. (C) UV-vis absorption spectra for different reaction setups. | |
We further verified this finding using electron paramagnetic resonance (EPR) spectroscopy, which confirmed that NiFe2O4 NPs do indeed generate hydroxyl radicals (·OH) (Fig. 8B), suggesting the existence of a Fenton process.65,70,71 This suggests that NiFe2O4 NPs may play a role in regulating the ROS balance in plants, similar to natural peroxidases,72 thus enhancing plant resistance.
3.3 NiFe2O4 NPs triggered maize seeds to improve the resistance of 3 week-old maize seedlings
Furthermore, we further evaluated the effects of NiFe2O4 NPs on three-week-old maize seedlings subjected to salt and drought stress. Biomass analysis of maize plants (Fig. 5A) underscores the impact of NiFe2O4 NPs in promoting stress tolerance. As shown in Fig. 5A, the biomass of seedlings increased notably under 10% and 20% PEG stress when treated with Ni-2 and Ni-3 NPs, with the Ni-2 group showing the most pronounced effect. At 50 mM salt concentration, biomass accumulation was markedly elevated in the Ni-1 and Ni-2 treatments compared to the control (CK), demonstrating a remarkable increase (*p < 0.05, ***p < 0.001). This analysis of biomass parameters supports the potential of NiFe2O4 NPs to enhance plant resilience.
In addition, we measured malondialdehyde (MDA) and superoxide dismutase (SOD) levels in maize leaves. The results revealed that seeds treated with NiFe2O4 NPs produced maize seedlings that demonstrated a notable increase in SOD activity under different stress conditions (Fig. 9A–D). MDA levels varied, showing a rise during periods of low stress and a fall during periods of high tension. This suggests that NiFe2O4 NPs may enhance the expression of SOD to mitigate the elevated levels of ROS. Moreover, the NiFe2O4 NPs, which are similar to peroxidase (POD) in their action, could aid in scavenging excessive ROS, thereby reducing the MDA content. Based on these findings, we suggest that NiFe2O4 NPs may play a dual role in stress alleviation: enhancing the SOD activity and mimicking POD enzyme function to regulate ROS homeostasis. However, the specific regulatory mechanisms may vary under different abiotic stress conditions, which requires further in-depth studies to clarify their exact mode of action.
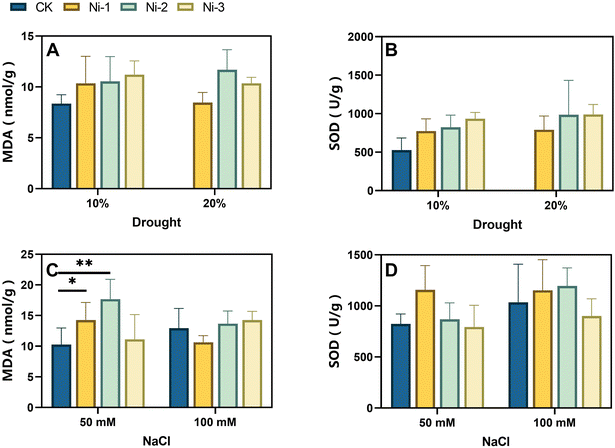 |
| Fig. 9 SOD enzyme and MDA content of three-week-old maize seedlings. Values are mean ± standard deviation (n = 4). Different asterisks above the bars indicate significant differences between treatment groups, respectively (p < 0.05). | |
3.4 Nutrient elemental effects of NiFe2O4 NPs on maize
Abiotic stresses trigger systemic responses, including root-to-shoot signaling. Under drought and salt stress, nutrient uptake, and transport were first regulated by the root system and then transferred to the shoot and leaves. Since leaves are the main site of photosynthesis and metabolic activities, they are important indicators of plant health and stress response.
Thus, we determined the elemental composition of maize leaves using ICP-MS. The results are presented in a heat map in Fig. 10. Under PEG-induced drought stress conditions, the contents of plant leaves including the elements such as calcium (Ca), potassium (K), sulfur (S), and phosphorus (P) treated with 40 mg L−1 of NiFe2O4 NPs (Ni-2 group) were significantly elevated. These elements contributed to enhanced drought tolerance through processes including enhancing the integrity of the cell wall and regulating stomatal dynamics.73–75 Nickel (Ni) and iron (Fe) levels, on the other hand, dropped.
 |
| Fig. 10 Changes in elemental content of maize leaves: Mg, P, S, K, Ca, Mn, Fe, Co, Ni, Cu, and Zn. | |
Under low-intensity salt stress, the group treated with 20 mg L−1 of NiFe2O4 NPs (Ni-1 group) exhibited a notable increase in the amounts of zinc (Zn), calcium (Ca), and iron (Fe). These elements mitigated salt damage by boosting antioxidant enzyme activity and regulating ionic balance.75–77 Under high salt stress, the higher concentration of NiFe2O4 NPs (Ni-2 group) treatment brought about a notable rise in calcium (Ca), manganese (Mn), cobalt (Co), and zinc (Zn). These elements enhance salt tolerance by stabilizing cell membranes, improving antioxidant defense, and maintaining osmotic pressure, which are key factors in the plant's ability to survive and grow under salt stress.75,77
The application of NiFe2O4 oxides under drought and salt stress conditions significantly improved the growth of maize seedlings. These findings align with earlier research showing that nanoparticles play a role in enhancing plant stress tolerance by improving water retention and ionic balance.78,79 In addition, the balanced use of NPs is essential to mitigate ionic stress while avoiding potential toxicity. These results validate the hypothesis that NiFe2O4 NPs can be used as an effective tool to enhance crop resilience to various types of abiotic stresses, providing a new approach to sustainable agriculture.
3.5 Risk analysis
Although nickel compounds are associated with a potential carcinogenic risk, NiFe2O4 NPs are generally considered to have low toxicity compared to other nickel compounds. We know that nickel compounds reach concentrations of less than 1 g Ni2+ per person before causing acute toxicity, which is very rare.80 However, a safety assessment is essential to guarantee their safe application in agriculture. It has been demonstrated that NiFe2O4 NPs (20 mg per 1.5 mL) have good bio-compatibility and low toxicity in vitro experiments with a low rate of ion release under physiological conditions or in different pH environments with good control.28,29 The low toxicity of NiFe2O4 has been demonstrated through experiment.81,82
Magnetite is considered harmless and the use of this material has received approval from the U.S. Food and Drug Administration (FDA) for various biological applications.83 To minimize the potential risks, regulatory agencies typically establish permissible exposure limits and safety standards for elemental concentrations in agricultural soil. Following these guidelines ensures that nanoparticles are used within safe limits and reduces potential health risks.
3.6 Environmental implications and benefits
The application of NiFe2O4 NPs in agriculture offers significant benefits, such as significantly enhancing crop resistance, reducing the need for fertilizers, and boosting agricultural productivity even under challenging conditions. However, the environmental impact of the widespread use of nanoparticles must be carefully assessed. The enduring effects of nanoparticles on soil conditions, microbial communities, and non-target organisms are still being studied intensively. While some studies indicate that NiFe2O4 NPs are less toxic than other metal-based nanoparticles, ongoing research is required to evaluate their long-term bioaccumulation and potential ecotoxicity. Moreover, developing controlled release and targeted application strategies is essential to minimize unintended environmental exposure. Balancing the benefits of nanoparticle-assisted agriculture with potential ecological risks is crucial to promote sustainable and safe agricultural practices.
4. Conclusion
As the global climate is changing rapidly, innovative strategies to enhance crop resilience to extreme weather are essential to ensure food security in the face of a growing population. This study highlights the potential of NiFe2O4 NPs with low toxicity and bio-compatibility to enhance the tolerance of maize (Zea mays L.) under drought and salinity stresses, providing an eco-friendly solution to mitigate the challenges posed by these abiotic stresses in agriculture, which is a key challenge. These nanoparticles effectively mitigate the harmful impact of abiotic stresses and improve seed germination, seedling vigor, and biomass accumulation. The peroxidase-like (POD) activity of nanoparticles plays a crucial role in mitigating oxidative damage and enhancing plant defense mechanisms. These findings are consistent with previous studies and provide additional insight into the deployment of nanotechnology in sustainable agriculture. They not only support the increasing focus on the application of nanotechnology for sustainable agriculture but also open the way for further research. Despite these encouraging outcomes, further studies are necessary to gain a complete understanding of the mechanisms of enhanced resistance of NiFe2O4 oxides, their long-term sustainability, inter-generational transferability, as well as their potential environmental impact and applicability. In addition, the interactions between NiFe2O4 NPs and other biotic or abiotic factors should be further investigated to optimize their application in agricultural systems. The integration of these future perspectives ensures that the conclusions are progressive and consistent with the broader objectives of the study.
Data availability
The data supporting the findings of this study are available within the paper. Should any additional raw data files be needed, they are available from the corresponding author upon reasonable request.
Conflicts of interest
The authors declare no competing financial interest.
Acknowledgements
This work was supported by the National Natural Science Foundation of China (No. 22076199 and No. 22241601) and the National Key R&D Program of China (No. 2018YFC1602305).
References
- Y. Jiang, J. Yang, M. Li, Y. Li, P. Zhou, Q. Wang, Y. Sun, G. Zhu, Q. Wang, P. Zhang, Y. Rui and I. Lynch, Nanomaterials, 2022, 12, 4160 CrossRef CAS PubMed.
- X. Yan, S. Chen, Z. Pan, W. Zhao, Y. Rui and L. Zhao, ACS Nano, 2023, 17, 492–504 CrossRef CAS.
- S. I. Zandalinas, F. B. Fritschi and R. Mittler, Trends Plant Sci., 2021, 26, 588–599 CrossRef CAS.
- M. Farooq, M. Hussain, A. Wakeel and K. H. M. Siddique, Agron. Sustainable Dev., 2015, 35, 461–481 CrossRef CAS.
- M. Farooq, M. Hussain, A. Wakeel and K. H. M. Siddique, Agron. Sustainable Dev., 2015, 35, 461–481 CrossRef CAS.
- W. Wan, Z. Liu, J. Li, J. Xu, H. Wu and Z. Xu, Agric. For. Meteorol., 2022, 315, 108821 CrossRef.
- S. K. Assem, Methods Mol. Biol., 2015, 1223, 119–134 CrossRef CAS.
-
N. Adger, P. Aggarwal, S. Agrawala, J. Alcamo, A. Allali, O. Anisimov, N. Arnell, M. Boko, O. Canziani, T. Carter, G. Casassa, U. Confalonieri, R. V. Cruz, E. D. A. Alcaraz, W. Easterling, C. Field, A. Fischlin, B. Fitzharris, C. G. García, C. Hanson, H. Harasawa, K. Hennessy, M. Lal, R. Lasco, G. Love, X. Lu, G. Magrin, L. J. Mata, R. McLean, B. Menne, G. Midgley, N. Mimura, D. Vaughan, C. Vogel, T. Wilbanks, P. P. Wong, S. Wu and G. Yohe, Contribution of working group II to the fourth assessment report of the intergovernmental panel on climate change, AR4 Climate Change 2007: Impacts, Adaptation, and Vulnerability, 2007 Search PubMed.
- P. Zhou, P. Zhang, M. He, Y. Cao, M. Adeel, N. Shakoor, Y. Jiang, W. Zhao, Y. Li, M. Li, I. Azeem, L. Jia, Y. Rui, X. Ma and I. Lynch, Environ. Pollut., 2023, 320, 121063 CrossRef CAS PubMed.
- M. Rui, C. Ma, Y. Hao, J. Guo, Y. Rui, X. Tang, Q. Zhao, X. Fan, Z. Zhang, T. Hou and S. Zhu, Front. Plant Sci., 2016, 7, 815 Search PubMed.
- P. Zhou, Y. Jiang, M. Adeel, N. Shakoor, W. Zhao, Y. Liu, Y. Li, M. Li, I. Azeem, Y. Rui, Z. Tan, J. C. White, Z. Guo, I. Lynch and P. Zhang, Environ. Sci. Technol., 2023, 57, 7547–7558 CrossRef CAS.
- M. Asgher, A. Rehaman, S. Nazar ul Islam and N. A. Khan, Environ. Pollut., 2024, 341, 122886 CrossRef CAS.
- F. Aghaei, R. S. Sharifi and S. Farzaneh, Silicon, 2024 DOI:10.1007/s12633-024-02917-w.
- J. Qiu, Y. Chen, Z. Liu, H. Wen, N. Jiang, H. Shi and Y. Kou, Environ. Pollut., 2023, 331, 121925 CrossRef CAS.
- S. Kaur, T. Garg, A. Joshi, A. Awasthi, V. Kumar and A. Kumar, Sci. Hortic., 2024, 331, 113146 CrossRef CAS.
- M. Javan, Y. Selahvarzi, P. Sayyad-Amin and S. Rastegar, Sci. Hortic., 2024, 330, 113055 CrossRef CAS.
- S. S. Hojjat, Nanotechnol. Russ., 2020, 15, 204–211 CrossRef CAS.
- A. Gasser, W. Ramadan, Y. Getahun, M. Garcia, M. Karim and A. A. El-Gendy, Mater. Sci. Eng., B, 2023, 297, 116721 CrossRef CAS.
- C. Pereira, A. M. Pereira, C. Fernandes, M. Rocha, R. Mendes, M. P. Fernández-García, A. Guedes, P. B. Tavares, J.-M. Grenèche, J. P. Araújo and C. Freire, Chem. Mater., 2012, 24, 1496–1504 CrossRef CAS.
- P. K. Jain, X. Huang, I. H. El-Sayed and M. A. El-Sayed, Acc. Chem. Res., 2008, 41, 1578–1586 CrossRef CAS.
- J. Mohapatra, A. Mitra, D. Bahadur and M. Aslam, CrystEngComm, 2012, 15, 524–532 RSC.
- A. Gasser, W. Ramadan, Y. Getahun, M. Garcia, M. Karim and A. A. El-Gendy, Mater. Sci. Eng., B, 2023, 297, 116721 CrossRef CAS.
- V. Polshettiwar, R. Luque, A. Fihri, H. Zhu, M. Bouhrara and J.-M. Basset, Chem. Rev., 2011, 111, 3036–3075 CrossRef CAS PubMed.
- B. Parvatheeswara Rao, B. Dhanalakshmi, S. Ramesh and P. S. V. Subba Rao, J. Magn. Magn. Mater., 2018, 456, 444–450 CrossRef CAS.
- D. Zhang, Z. Tong, G. Xu, S. Li and J. Ma, Solid State Sci., 2009, 11, 113–117 CrossRef CAS.
- K. Pubby, S. S. Meena, S. M. Yusuf and S. Bindra Narang, J. Magn. Magn. Mater., 2018, 466, 430–445 CrossRef.
- A. Baykal, N. Kasapoğlu, Y. Köseoğlu, M. S. Toprak and H. Bayrakdar, J. Alloys Compd., 2008, 464, 514–518 CrossRef CAS.
- K. Egizbek, A. L. Kozlovskiy, K. Ludzik, M. V. Zdorovets, I. V. Korolkov, B. Marciniak, J. M. D. Chudoba, A. Nazarova and R. Kontek, Ceram. Int., 2020, 46, 16548–16555 CrossRef CAS.
- L. Andjelković, D. Jeremić, M. R. Milenković, J. Radosavljević, P. Vulić, V. Pavlović, D. Manojlović and A. S. Nikolić, Ceram. Int., 2020, 46, 3528–3533 CrossRef.
- A. Tomitaka, A. Hirukawa, T. Yamada, S. Morishita and Y. Takemura, J. Magn. Magn. Mater., 2009, 321, 1482–1484 CrossRef CAS.
- S. Shokri, N. Shariatifar, E. Molaee-Aghaee, G. J. Khaniki, P. Sadighara, M. A. Faramarzi, M. Mohammadi and A. Rezagholizade-shirvan, Sci. Rep., 2023, 13, 15777 CrossRef CAS PubMed.
- J. Song, J. Zhang, A. Zada, Y. Ma and K. Qi, Ceram. Int., 2023, 49, 12327–12333 CrossRef CAS.
- S. Bae, S. W. Lee and Y. Takemura, Appl. Phys. Lett., 2006, 89, 252503 CrossRef.
- A. Manohar, S. V. Prabhakar Vattikuti, P. Manivasagan, E.-S. Jang, H. Bandi, A. M. Al-Enizi, M. Gupta, M. Ubaidullah and K. H. Kim, Colloids Surf., A, 2024, 682, 132855 CrossRef CAS.
- L. Luo, L. Zhu, Y. Xu, L. Shen, X. Wang, Y. Ding, Q. Li and D. Deng, Microchim. Acta, 2011, 174, 55–61 CrossRef CAS.
- G. Zhu, P. Zhang, W. Zhao, N. Shakoor, Y. Sun, Q. Wang, Q. Wang, M. Li, Y. Jiang, Z. Tan, Y. Rui and I. Lynch, Toxin Rev., 2024, 43, 236–254 CrossRef.
- S. Chen, H. Liu, Z. Yangzong, J. Gardea-Torresdey, J. White and L. Zhao, Environ. Sci. Technol., 2023, 57 DOI:10.1021/acs.est.3c07339.
- A. W. Ajmal, H. Yasmin, M. N. Hassan, N. Khan, B. L. Jan and S. Mumtaz, Front. Microbiol., 2022, 13 DOI:10.3389/fmicb.2022.815704.
-
K. Ben Hamed, A. Dabbous, H. El Shaer and C. Abdely, in Salinity Responses and Tolerance in Plants, Volume 2: Exploring RNAi, Genome Editing and Systems Biology, ed. V. Kumar, S. H. Wani, P. Suprasanna and L.-S. P. Tran, Springer International Publishing, Cham, 2018, pp. 1–19 Search PubMed.
- M. Zheng, Y. Tao, S. Hussain, Q. Jiang, S. Peng, J. Huang, K. Cui and L. Nie, Plant Growth Regul., 2016, 78, 167–178 CrossRef CAS.
- A. T. M. T. Islam, H. Ullah, S. K. Himanshu, R. Tisarum, S. Cha-um and A. Datta, Sci. Hortic., 2022, 304, 111304 CrossRef CAS.
- M. H. Ali, J.-M. Sobze, T. H. Pham, M. Nadeem, C. Liu, L. Galagedara, M. Cheema and R. Thomas, Nanomaterials, 2020, 10, 1852 CrossRef CAS.
- M. Hatami, J. Hadian and M. Ghorbanpour, J. Hazard. Mater., 2017, 324, 306–320 CrossRef CAS PubMed.
- H. Wei and E. Wang, Chem. Soc. Rev., 2013, 42, 6060–6093 RSC.
- T. Lin, L. Zhong, Z. Song, L. Guo, H. Wu, Q. Guo, Y. Chen, F. Fu and G. Chen, Biosens. Bioelectron., 2014, 62, 302–307 CrossRef CAS.
- J. Wu, X. Wang, Q. Wang, Z. Lou, S. Li, Y. Zhu, L. Qin and H. Wei, Chem. Soc. Rev., 2019, 48, 1004–1076 RSC.
- D. Li, C. Lan, B. Chu, L. Meng and N. Xu, J. Hazard. Mater., 2024, 469, 133918 CrossRef CAS.
- S. Mvango and P. Mashazi, Mater. Sci. Eng., C, 2019, 96, 814–823 CrossRef CAS PubMed.
- Y. Wang, Z. Zhang, G. Jia, L. Zheng, J. Zhao and X. Cui, Chem. Commun., 2019, 55, 5271–5274 RSC.
-
F. Gerson and W. Huber, Electron spin resonance spectroscopy of organic radicals, John Wiley & Sons, 2003 Search PubMed.
- M. J. Davies, Methods, 2016, 109, 21–30 CrossRef CAS PubMed.
- G. Fang, C. Zhu, D. D. Dionysiou, J. Gao and D. Zhou, Bioresour. Technol., 2015, 176, 210–217 CrossRef CAS PubMed.
- C. J. Weydert and J. J. Cullen, Nat. Protoc., 2010, 5, 51–66 CrossRef CAS.
-
M. Senthilkumar, N. Amaresan and A. Sankaranarayanan, in Plant-Microbe Interactions: Laboratory Techniques, ed. M. Senthilkumar, N. Amaresan and A. Sankaranarayanan, Springer US, New York, NY, 2021, pp. 103–105 Search PubMed.
- N. Ghezal, I. Rinez, H. Sbai, I. Saad, M. Farooq, A. Rinez, I. Zribi and R. Haouala, S. Afr. J. Bot., 2016, 105, 240–250 CrossRef CAS.
- A. Rukhsar-Ul-Haq, S. Kausar, T. Hussain, S. Javed, S. Zafar, S. Anwar, N. Zahra Hussain and M. Saqib, Plant Physiol. Biochem., 2023, 195, 341–350 CrossRef CAS.
- P. Pandya, S. Kumar, A. A. Sakure, R. Rafaliya and G. B. Patil, Curr. Plant Biol., 2023, 35–36, 100292 CrossRef CAS.
- Y. Mu, Y. Li, Y. Zhang, X. Guo, S. Song, Z. Huang, L. Li, Q. Ma, M. N. Khan and L. Nie, J. Integr. Agric., 2023 DOI:10.1016/j.jia.2023.12.013.
- A. Singh, R. Singh Sengar, R. Singh, U. P. Shahi, M. K. Yadav, V. Shami, L. K. Gangwar and V. D. Rajput, Ecol., Environ. Conserv., 2022, 28, 254–259 Search PubMed.
- H. Shafiq, M. Y. Shani, M. Y. Ashraf, F. De Mastro, C. Cocozza, S. Abbas, N. Ali, Z. Zaib-un-Nisa, A. Tahir, M. Iqbal, Z. Khan, N. Gul and G. Brunetti, Plants, 2024, 13, 1080 CrossRef CAS.
- D. V. Francis, A. K. Abdalla, W. Mahakham, A. K. Sarmah and Z. F. R. Ahmed, Environ. Int., 2024, 190, 108859 CrossRef CAS PubMed.
- M. Ahamed, M. J. Akhtar, M. A. Siddiqui, J. Ahmad, J. Musarrat, A. A. Al-Khedhairy, M. S. AlSalhi and S. A. Alrokayan, Toxicology, 2011, 283, 101–108 CrossRef CAS.
- X. Wang, K. Zheng and P. Ji, Catalysts, 2017, 7, 206 CrossRef.
- D. Wang and Y. Zhao, Chem, 2021, 7, 2635–2671 CAS.
- D. He, C. J. Miller and T. D. Waite, J. Catal., 2014, 317, 198–205 CrossRef CAS.
- G. Xiao, H. Li, Y. Zhao, H. Wei, J. Li and H. Su, ACS Appl. Nano Mater., 2022, 5, 14147–14170 CrossRef CAS.
- M. Liang and X. Yan, Acc. Chem. Res., 2019, 52, 2190–2200 CrossRef CAS.
- Y. Zhang, G. Wei, W. Liu, T. Li, Y. Wang, M. Zhou, Y. Liu, X. Wang and H. Wei, Nat. Rev. Methods Primers, 2024, 4, 1–22 CrossRef.
- H. Wei and E. Wang, Chem. Soc. Rev., 2013, 42, 6060–6093 RSC.
- B. Huang, Z. Wu, H. Zhou, X. Wang, Y. Liu, H. Zhang, Z. Xiong and B. Lai, Appl. Catal., B, 2024, 355, 124157 CrossRef CAS.
- J. Guo, B. Gao, Q. Li, S. Wang, Y. Shang, X. Duan and X. Xu, Adv. Mater., 2024, 36, 2403965 CrossRef CAS.
- X. Xiao, X. Hu, Q. Liu, Y. Zhang, G.-J. Zhang and S. Chen, Inorg. Chem. Front., 2023, 10, 4289–4312 RSC.
- S. Rasheed, K. Bashir, A. Matsui, M. Tanaka and M. Seki, Front. Plant Sci., 2016, 7 DOI:10.3389/fpls.2016.00180.
- S. Hong-Bo, C. Li-Ye and S. Ming-An, BioEssays, 2008, 30 DOI:10.1002/bies.20770.
- T. Sun, M. Li, Y. Shao, L. Yu and F. Ma, Front. Plant Sci., 2017, 8 DOI:10.3389/fpls.2017.00426.
- J. N. Kunz, D. V. Voronine, H. W. H. Lee, A. V. Sokolov and M. O. Scully, Opt. Express, 2017, 25, 7251–7262 CrossRef CAS PubMed.
- D. Ma, D. Sun, C. Wang, H. Ding, H. Qin, J. Hou, X. Huang, Y. Xie and T. Guo, Front. Plant Sci., 2017, 8 DOI:10.3389/fpls.2017.00860.
- A. Singh, S. Agrawal, V. D. Rajput, K. Ghazaryan, A. Yesayan, T. Minkina, Y. Zhao, D. Petropoulos, A. Kriemadis, M. Papadakis and A. Alexiou, Discover Appl. Sci., 2024, 6, 317 CrossRef.
- C. Fu, M. N. Khan, J. Yan, X. Hong, F. Zhao, L. Chen, H. Ma, Y. Li, J. Li and H. Wu, Crop Environ., 2023, 2, 92–99 CrossRef.
-
K. S. Kasprzak and K. Salnikow, Nickel Toxicity and Carcinogenesis, Nickel and Its Surprising Impact in Nature, 2007, vol. 2, DOI:10.1002/9780470028131.ch17.
- N. Hadrup, A. T. Saber, Z. O. Kyjovska, N. R. Jacobsen, M. Vippola, E. Sarlin, Y. Ding, O. Schmid, H. Wallin, K. A. Jensen and U. Vogel, Environ. Toxicol. Pharmacol., 2020, 74, 103303 CrossRef CAS PubMed.
- N. L. Martínez-Rodríguez, S. Tavárez and Z. I. González-Sánchez, Toxicol. In Vitro, 2019, 57, 54–61 CrossRef.
- A. Shah and M. A. Dobrovolskaia, Nanomed.: Nanotechnol., Biol. Med., 2018, 14, 977–990 CrossRef CAS PubMed.
Footnote |
† These authors contributed equally to this work. |
|
This journal is © The Royal Society of Chemistry 2025 |
Click here to see how this site uses Cookies. View our privacy policy here.