Human health trade-offs in the disinfection of wastewater for landscape irrigation: microplasma ozonation vs. chlorination†
Received
5th September 2016
, Accepted 31st October 2016
First published on 31st October 2016
Abstract
Wastewater reuse is becoming increasingly common, and there is a need for decentralized and small-scale systems to support the safe recovery of water resources. In this study, an integrated life cycle assessment (LCA) and quantitative microbial risk assessment (QMRA) were used to compare microplasma ozonation (an emerging technology) to chlorination (an established technology) for the disinfection of wastewater for landscape irrigational reuse. Three waterborne pathogens, Legionella pneumophila, Giardia, and Cryptosporidium parvum, were selected to include bacteria and protozoans covering the transmission routes of inhalation and ingestion. Inactivation data from the literature were coupled with bench-scale experiments (to establish inactivation parameters for L. pneumophila by ozone in wastewater) for the design and simulation of disinfection processes. Microplasma-based ozonation reduced more life cycle human health impacts as compared to chlorination for five of the six impact categories, because of the high susceptibility of the pathogens to ozone and the lower impacts stemming from electricity (required in ozonation) vs. chemical production (required in chlorination). These results were consistent across the electricity-fuel mixes of all fifty U.S. states. These results indicate that from the point of view of reducing human health impact, the emerging microplasma ozonation technology is superior to chlorination for wastewater reuse disinfection. To reduce the overall human health impact, future design efforts should focus on reducing process consumables (i.e., chemical and electricity consumption) through longer hydraulic residence times (HRTs), while maintaining adequate disinfectant dosing to provide reliable disinfection efficacy despite influent variability in compounds that may quench or interfere with the disinfectant.
Water impact
Microplasma ozonation (MPO) is a promising technology for decentralized water reuse disinfection. We used an integrated life cycle assessment and quantitative microbial risk assessment to benchmark this technology against chlorination to identify the disinfection process of lesser human health impacts. The results show that for irrigational reuse, the MPO reduced more human health impacts than chlorination across fifty U.S. states.
|
1. Introduction
Wastewater reuse alleviates pressure on freshwater resources. In 2010, the total water reuse in the United States was estimated to be 2400 million gallons per day (MGD),1 an increase of 42% compared to that in 2004.2 However, successful reuse requires that the finished water be disinfected to prevent the spread of pathogens, especially for reuse applications – such as landscape irrigation – that could result in human contact. Currently, chlorination using sodium hypochlorite is a well-established disinfection method for wastewater. Pathogens are inactivated mainly through free chlorine or chloramines (depending on the ammonia content of the treated wastewater), which are effective against a wide range of waterborne pathogens. Due to its efficacy and affordability, chlorination is the most widely used technique for wastewater disinfection.3 However, when dechlorination is required (which is typical, especially if reclaimed water is used for irrigation), the economic benefits of chlorination over alternative disinfection techniques are not always guaranteed.4 Beyond cost, chlorination may also encourage the formation of harmful disinfection byproducts (DBPs)5–7 and is ineffective at low doses against a sub-set of pathogens of concern, such as Cryptosporidium parvum.8
Ozone is a powerful disinfectant for a number of critical microorganisms such as the Norwalk virus,9 poliovirus,10Escherichia coli,11,12 and even chlorine resistant C. parvum,13–17 despite the potential to produce DBPs such as the bromate in bromide-rich waters.3 Lowered water toxicity after ozonation has also been reported.18 Recently, an emerging method to produce ozone – microplasma technology – was invented and brought to market.19 Because the building block of microplasma ozone generators (known as a “chip”) is modular and produces 2–3 grams of ozone per hour, virtually any ozone production rate can be generated by stacking individual chips. As such, ozone may become a more feasible disinfection alternative for small-scale treatment systems. In addition to increased modularity, microplasma generators using oxygen as the feedstock gas have achieved energy efficiencies more than twice that of conventional dielectric barrier discharge reactors (e.g. 120 g (kW h)−1 (ref. 19) vs. 50 g (kW h)−1 (ref. 20)), stemming from the combination of a much smaller plasma impedance (∼41 kΩ) and a much lower driving voltage.19 Finally, microplasma ozone generators do not need water cooling units, owing to their large surface area to volume ratio and the low temperature of the plasmas.21 This additional benefit further reduces the complexity and size of the system without compromising functionality.
Both chlorination and ozonation technologies have the shared goal of reducing pathogen concentrations in reclaimed water to protect human health. However, technologies that consume energy, chemicals, and other resources can result in a variety of environmental emissions across space and time that can be detrimental to human health, creating a tension between direct benefits (reduced pathogen exposure) and negative health consequences stemming from the indirect release of environmental stressors (e.g., PM2.5 released during the transport of materials to the treatment plant via truck). Although direct human health risks from pathogen exposure can be evaluated by Quantitative Microbial Risk Assessment (QMRA, which assesses the risks associated with exposure to specific pathogens of concern22), the implications of indirect impacts of a given technology are better assessed via Life Cycle Assessment (LCA).23 To better understand the health implications of disinfection system design and operational decisions, a hybridized LCA and QMRA methodology can be established by leveraging the common unit of Disability Adjusted Life Years (DALYs).24 This approach was recently used to quantify the human health impacts of different flow regimes at several water reclamation plants24 and could offer insights into the selection and development of disinfection technologies for water reuse.
To date, LCA studies have compared ozonation or chlorination to other treatment alternatives.25–27 For example, ozonation vs. sand filtration or MBRs,28 ozonation vs. chlorination plus ultraviolet treatment,29 ozonation vs. ozonation plus hydrogen peroxide,29,30 chlorination vs. ultraviolet,31 and chlorination vs. ozonation plus hydrogen peroxide.29 Existing relevant QMRA studies focused primarily on a single disinfection technology, such as chlorination,32,33 or a combination of technologies in series, such as ozonation followed by chlorination.34,35 No study thus far has adopted the hybridized LCA and QMRA approach to determine the human health impact of the disinfection unit.
Given the potential of microplasma ozonation technology, the objective of this study is to evaluate the human health impact of microplasma ozonation benchmarked against chlorination. A combination of LCA and QMRA was conducted to determine the factors governing their performance and uncertainty in a wastewater reuse setting. To achieve this objective, two full-scale disinfection treatment trains were designed in parallel: (i) chlorination followed by dechlorination, and (ii) microplasma ozonation. Pathogens explicitly modeled included Giardia, C. parvum, and Legionella pneumophila. This choice of pathogens encompasses protozoans and bacteria covering the transmission routes of both ingestion and inhalation that are likely to occur in landscape irrigation.36Giardia and C. parvum were chosen because they are two of the most frequently documented waterborne enteric pathogens in the United States.2L. pneumophila was included due to its high health care cost of over $33
000 per episode of sickness due to infection,37 as well as the recent outbreak of Legionellosis in New York City (USA) in the summer of 2015.38 Data from the literature (for Giardia and C. parvum inactivation) and data from bench-scale experiments conducted in this study (for L. pneumophila inactivation) were used. Given the anticipated importance of electricity consumption to indirect health impacts,24,39 the comparative assessment was conducted across all 50 U.S. states with a focus on three states with the largest reclaimed water use (Florida, California, and Texas),2 using their respective fuel mixes for the consumed electricity and the quantification of environmental impacts. Finally, the LCA-QMRA modeling tool was integrated into a Monte Carlo framework, and sensitivity analyses were conducted to determine the relative sensitivity of results to input parameters, as well as design and operational decisions. The findings of this study will guide the development and future implementation of microplasma ozonation for wastewater disinfection.
2. Method
2.1. Pathogen inactivation via chlorination or ozonation
2.1.1.
Giardia and C. parvum inactivation parameters.
Literature data were used to design both the chlorination and ozonation disinfection systems for Giardia and C. parvum inactivation. The inactivation kinetics were assumed to follow the Chick–Watson inactivation model with inactivation rate constants of 2.5 × 10−2 (at 18 °C) and 8.36 × 10−4 (at 20 °C) L mg−1 min−1 for Giardia and C. parvum inactivation by chlorine, respectively.40,41 The inactivation rate constants for Giardia and C. parvum using ozone were 27.1 (at 25 °C) and 0.8 (at 20 °C) L mg−1 min−1, respectively.40,42 The effect of temperature on the inactivation rate constants was addressed using the Arrhenius equation. The impact of the water matrix on the residual disinfectant concentration was accounted for by mathematical simulation. Detailed equations, values, and steps are provided in sections 1 and 2 of the ESI.†
2.1.2. Determination of L. pneumophila inactivation parameters.
The chlorination inactivation parameters for L. pneumophila were obtained from the literature, with a second-order inactivation rate constant at 30 °C of 0.307 L mg−1 min−1.43 To obtain a representative inactivation rate constant for L. pneumophila by ozone in secondary wastewater, a set of bench-scale experiments were performed (described below).
L. pneumophila strain ATCC 33152 was cultivated following ATCC instructions. After harvesting, L. pneumophila cells were rinsed three times with sterile 0.01 M phosphate buffer, followed by centrifugation at 5000 rpm. L. pneumophila cells were enumerated by plating onto charcoal-containing CYE agar plates following ATCC protocols. Semi-batch reactors containing a final solution volume of 700 mL were submerged in a water bath for temperature control. Mixing was accomplished by a magnetic stirrer operating at 700 rpm. Ozone gas concentration was adjusted with a transformer in series with the generator to achieve a gas phase ozone concentration of 1.3 to 4.9 g m−3. The generated ozone gas from the microplasma ozonator was directed into the reactor through a ceramic diffuser, and the flow rate of ozone was controlled by a Swagelok® valve and monitored with a rotameter. The off-gas was passed through a 0.25% potassium iodide solution, a 10% bleach solution, and finally a HEPA-CAP filter to prevent the release of any aerosols containing L. pneumophila. Luerlok syringes were used to take samples via PTFE tubing attached through the cap of the reactor. The dissolved ozone concentration was determined by the Indigo colorimetric method44 read by a portable spectrophotometer (HACH, model DR2800; Loveland, CO).
Two types of experiments to determine the inactivation parameters were conducted using solutions with and without wastewater organic matter (WOM). The organic matter-free solution was buffered by phosphate at pH 6.8–7.0, because previous research has demonstrated that ozone disinfection kinetics of L. pneumophila are insensitive to pH at environmentally relevant conditions (pH 7–9).45 The solutions containing organic matter were a mixture of phosphate buffer and wastewater filtered through a 0.22 μm filter. Treated wastewater was collected from the effluent of a secondary clarifier at the Urbana (Illinois, USA) Northeast Wastewater Treatment Plant and kept at 4 °C in the dark until used. Prior to the inactivation experiments, the ozone generator was warmed up for 5 min with ozone passed directly into the ozone destruction units made from glass bubblers filled with KI solution. Subsequently, ozone was pumped through a solution of 0.01 M phosphate buffer at a high flow rate (40 mL min−1) for a period of 0.3 to 3 min, until the desired initial dissolved ozone concentration was achieved. The flow rate was then reduced to a level that maintained a dissolved ozone concentration of 15 to 25 μg L−1 for the organic-free solution experiments, and 33 to 281 μg L−1 for the experiments with WOM. This required an adjustment of the gas phase ozone concentration by changing the voltage applied to the ozone generator (50 V to deliver an initial dissolved ozone concentration of 33 μg L−1, and 120 V to deliver any initial concentrations higher than 33 μg L−1). For experiments with WOM, a mixture of undiluted, filtered secondary effluent and L. pneumophila cells was injected into the reactor through a PTFE tube, while only L. pneumophila in a buffered solution was injected for experiments with organic-free solutions. The temperature of the reactor was maintained by a water bath at 7 or 22 °C, in order to represent winter and summer conditions. A final L. pneumophila cell concentration of 105–106 cells mL−1 in the reactor was used for all experiments. Samples were subsequently obtained at different time points through additional PTFE tubing with Luerlok syringes and immediately transferred into a 0.1% sterilized sodium thiosulfate solution. This solution was then subjected to serial dilutions and plating on charcoal-containing CYE agar plates. At least three separate experiments using different batches of L. pneumophila were conducted. L. pneumophila inactivation did not occur in the control experiments.
To obtain the Chick–Watson inactivation rate constant that is crucial for the design of the ozonation disinfection system, the dissolved ozone concentration must be known. However, residual ozone at low doses during wastewater treatment can be difficult to measure due to turbidity46 and ozone consumption by WOM.47–49 These issues were noted in prior research11,50,51 and were also observed in this study. Therefore, a mass balance model was constructed as a tool to predict the dissolved ozone concentration in wastewater during L. pneumophila inactivation. To do so, a separate set of experiments were conducted to determine the ozone decomposition and transfer rate in solutions with and without WOM. At both 7 and 22 °C, the decomposition kinetics of dissolved ozone in solutions containing various concentrations of initial WOM loadings and initial dissolved ozone concentrations were individually assessed. This approach was proven successful in that it eliminated the interference that was caused by the simultaneous presence of both the L. pneumophila biomass turbidity and WOM on the Indigo colorimetric method for ozone detection at low ozone concentrations (see more in section 3.1). The transfer rate of ozone into water at both 7 and 22 °C was determined by purging ozone into organic-free buffered water at different ozonator driving voltages, during which the increase in dissolved ozone concentration was recorded as a function of time. The dissolved ozone concentrations were then used to fit a series of mass balance equations and develop a model using OriginPro 9.1 (Northampton, MA) that predicts the dissolved ozone concentration. The final function predicts dissolved ozone concentration based on input parameters, such as the initial ozone concentration and Henry's constant at different temperatures. Examples of the parameter fitting are provided in the ESI† of this manuscript (Fig. S1 and S2).
2.2. Life cycle assessment (LCA)
2.2.1. System description.
Two alternative disinfection technologies for secondary effluent were considered in this study: (i) chlorination followed by dechlorination, and (ii) microplasma ozonation. The former was used as a benchmark because it is a well-established and widely accepted technology for water reclamation disinfection. Both systems were designed using design manuals and specifications provided by the manufacturers. Design details can be found in section 2 of the ESI.†
The chlorination-based disinfection system consists primarily of a sodium hypochlorite storage tank and dosing system, a contact tank, a sodium bisulfite storage tank and dosing system, and two inline static mixers (one for each chemical; Fig. S5†). Secondary effluent is mixed with sodium hypochlorite at the entrance of the serpentine contact tank. The inactivation kinetic data were obtained from the literature, as described in section 2.1.1. The chlorine contact tank was designed to have a large length to width ratio (L
:
W = 40
:
1) to minimize dispersion. The chlorinated wastewater continues flowing towards the end of the contact tank, where it is then mixed with sodium bisulfite for dechlorination. The dose of sodium bisulfite was designed to dynamically match the hypochlorite residual. The final discharge is subsequently used for immediate downstream landscape irrigation.
The microplasma ozonation disinfection system consists of a microplasma ozone generator, a venturi ozone injection nozzle, an ozone contact tank, and an ozone destruction unit (Fig. S5†). Air is compressed into the microplasma ozone generator, which then generates plasmas to produce ozone. Ozone gas is injected into the secondary effluent using a venturi injector. The ozonated wastewater flows into an ozone contact tank, which also has a large length to width ratio (L
:
W = 40
:
1) to minimize dispersion. The inactivation in the ozone contact tank was modeled based on kinetic data obtained from this study as well as the literature.40,42 The escaped ozone from the ozonated wastewater is collected at the top of the contact tank and passed through a thermal catalytic ozone destruction device. The final discharge is used for immediate downstream landscape irrigation.
2.2.2. Goal and system boundary.
The goal of this LCA was to compare the environmental impacts on human health stemming from two alternative disinfection technologies. The system boundary included the construction and operation of both systems and did not consider processes that were common to both technologies (e.g., physical and biological processes prior to disinfection) (Fig. S5†). The functional unit for this study was the disinfection (more than 1
log10 inactivation) of 4 MGD of secondary effluent (with pathogen distributions defined in Table S6†) with a project lifetime of ten years.
2.2.3. Inventory analysis.
The quantity of materials and energy consumption for the construction and operation of both the chlorination and ozonation systems were generated from the detailed design (section 2 of the ESI†) and assumptions (Table S1†). The ozone generator, in particular, was inventoried using design documents for a microplasma ozone generator (2 g h−1 production per “chip”; EP Purification, Inc.). To account for differences in environmental impacts stemming from locality specific electricity fuel sources, fuel mixes for three separate states in the U.S. – the states with the highest total annual quantity of reclaimed water usage (Florida, California, and Texas) – were each individually used to quantify the sensitivity of LCA results to the location of the disinfection system. To explore the generalizability of the data, results from the three states were also compared against the rest of the U.S. states in terms of the human health intensity consumed energy (explained more in section 2.2.4). Data on the annual state net electricity consumption profile by fuel sources were obtained from the US Energy Information Administration for 2013 and 2014 (Table S3†).52 Inventory data were obtained from the ecoinvent database (v3, accessed through SimaPro v8.0.5.13) encompassing raw material extraction, processing, manufacturing, and transportation. A detailed summary of the design equations (sections 2.2 and 2.3 in the ESI†), a breakdown list of materials and their quantities, and the inventory materials/processes from ecoinvent 3 are provided in Tables S4 and S5 in the ESI.†
2.2.4. Impact assessment.
Because the impact on human health is the basis of comparison for this study, six out of seventeen impact categories in the ReCiPe method relevant to human health were evaluated, which included climate change, ozone depletion, human toxicity, photochemical oxidant formation, particulate matter formation, and ionizing radiation. The impact assessment was conducted using the ReCiPe endpoint method to express results in DALYs. Although endpoint methods introduce greater uncertainty to the system due to additional embedded assumptions, the use of endpoint impact categories is often more accessible and relevant for stakeholders.53 The hierarchist cultural perspective was set as the base case cultural perspective, which was required for the ReCiPe method. A similar approach was used in a previous study using DALYs as a measurement of the human health effects.24 The final results were normalized by functional unit and expressed in DALYs per year per log10 pathogen inactivation.
2.2.5. Uncertainty and sensitivity analysis.
Uncertainty analysis of the human health impacts of both systems were performed with Monte Carlo calculations and 1500 simulations in Microsoft Excel 2013. Input variables were assigned uniform distributions unless compelling evidence suggested otherwise. Temperature followed a normal distribution54 and the input parameter k – which is the identical survival probability for each organism – was assigned a log-normal distribution for each pathogen according to the literature.55–57 The uncertainty surrounding input parameters with limited data (i.e., point estimations) in the literature were addressed using the pedigree matrix approach.58,59 Briefly, a matrix consisting of five data quality indicators – reliability, completeness, temporal correlation, geographical correlation, and further technological correlation – were used to select indicator scores corresponding to each input parameter and data quality indicator combination. The indicator scores for these input parameters were then calculated to yield a standard deviation that log-normal data would follow. These standard deviation values were converted and are reported as coefficients of variation provided together with the inventory data (Tables S4 and S5 in the ESI†).
A sensitivity analysis was performed by adjusting each input value individually from its median to the 10th and 90th percentiles. The corresponding change of the output metric (human health impact) was recorded to characterize the relative importance of individual sources of uncertainty. Additionally, the percentage change in the output metric was also normalized to the percentage change in the input value to offer additional insight to the system through a relative response (Fig. S4a and b;† the larger the ratio, the more sensitive human health impacts were to relative changes in a given input value).
2.3. Quantitative microbial risk assessment
2.3.1. Hazard identification.
Giardia, C. parvum, and L. pneumophila were identified as the pathogens of concern that could be exposed to people during landscape irrigation with reclaimed water. Giardia and C. parvum represent the chlorine resistant pathogens that the state of Florida regulates as one of the reclaimed water quality monitoring parameters.1 Accidental ingestion during landscape irrigation makes these pathogens public health concerns.60L. pneumophila was selected because it was identified in reclaimed wastewater61,62 and it poses a potential hazard to human health when such water is aerosolized (e.g., during landscape irrigation).63
2.3.2. Exposure assessment.
The most common landscape irrigation system is the sprinkler irrigation system,64 which creates aerosols consisting of large water droplets as well as fine mist that could be accidentally ingested and/or inhaled by humans. As discussed above, the primary route of exposure was identified as ingestion for both Giardia and C. parvum, and inhalation for L. pneumophila. To calculate the final dose, a population size of 40
000 was assumed along with 100 gallons of wastewater produced per capita per day.65 It was assumed that the reclaimed water would be used to irrigate the public landscape, and that the full population would share access to this space. Additionally, a park visit frequency of once a week per person was assumed. For ingestion, an estimated 1 mL ingestion of municipal irrigation water per person per visit to the public lawn was used.60 For inhalation, 10−2 CFU m air−3 (CFU mL water−1)−1 of partitioning coefficient,66 0.72 m3 h−1 inhalation rate,66 0.5 h of exposure duration per visit, and 50% retention fraction of L. pneumophila in lungs were used as the default case.57
2.3.3. Dose response assessment.
Dose response data for the three chosen pathogens were all obtained from the literature. The exponential model (eqn (1)) was the best fit across dose response data for all three pathogens. | Risk probability = 1 − e−k×dose | (1) |
For the exponential model, it is assumed that the pathogens have independent and identical probability of survival to reach and infect at an appropriate site in the host's body, during which one pathogen is capable of producing an infection.22 For Giardia, a dose response model using adult males was used, with infection being the response measured and ingestion being the exposure route.56 The log-normal maximum likelihood estimate (MLE) k value was 1.99 × 10−2 with a 95% percentile of 2.92 × 10−2.56 For C. parvum, a dose response model using human volunteers as hosts and infected with Iowa strain was adopted, with infection being the response measured. The log-normal MLE k value was 4.19 × 10−3 with a 95% percentile value of 7.52 × 10−3.55 A dose response model for L. pneumophila using guinea pigs as hosts was chosen, with infection being the response measured and inhalation being the exposure route. The log-normal MLE k value was 5.99 × 10−2 with a 95% percentile value of 0.111.57
2.3.4. Risk calculation in DALYs.
The probabilities of infection calculated in section 2.3.3 were converted to DALYs incurred per year by multiplying the probability value (in the unit of symptomatic cases per year) by the severity factor (also known as the characterization factor in DALYs per symptomatic case for each pathogen). The severity factors for both Giardia and C. parvum were taken from the literature (1.6 × 10−3 and 1.47 × 10−3 DALYs per symptomatic case for Giardia and C. parvum, respectively).67,68 The severity factor for L. pneumophila was estimated to follow a uniform distribution between 1.05 × 10−3 and 4.37 × 10−2 DALYs per symptomatic case due to a lack of direct estimate from the literature. Detailed calculations for this estimation are provided in section 3 in the ESI.† The final results were normalized by functional unit and expressed in DALYs per year per log10 pathogens inactivated.
3. Results and discussion
3.1. Determination of parameters for L. pneumophila inactivation by ozone
LCA and QMRA require the knowledge of L. pneumophila inactivation kinetics and related disinfection parameters. For this reason, we conducted inactivation experiments and analyzed the data based on a mass balance model that predicted the ozone concentration as a function of disinfection time. Details of the mass balance are presented in section 1 of the ESI.† Based on this mass balance model, the calculated profiles of the dissolved ozone were subsequently used in the Chick–Watson equation to calculate the inactivation rate constant for L. pneumophila in wastewater. Following the log10 scale version of the Chick–Watson law,
values (log10 of the L. pneumophila survival ratio) from all experiments were plotted vs.
, where CL is the instantaneous dissolved ozone concentration at time t. Although a temperature dependence of L. pneumophila inactivation by ozone would be expected,12,42,69 the complex wastewater matrix used for parameter calibration did not result in significantly different inactivation rate constants at 7 and 22 °C at a confidence level of 95% (p > 0.05). We therefore assumed an identical L. pneumophila inactivation rate constant using ozone, with the fitting equation and coefficient of determination being
= −0.063Ct and 0.94, respectively (Fig. 1). Within the TOC range of 0.8 to 3.1 mg C L−1 and at both 7 and 22 °C, a good fit (R2 = 0.94) was observed for L. pneumophila inactivation. The agreement of the Chick–Watson model with the experimental data obtained over a range of wastewater containing 0.8 to 3.1 mg C L−1 WOM indicates the validity of the dissolved ozone concentration predicted by the established mass balance model. The obtained inactivation rate constant for L. pneumophila inactivation, together with the modeling framework for the ozonation decay kinetics in wastewater, were subsequently used for L. pneumophila inactivation predictions in both the LCA and QMRA analysis.
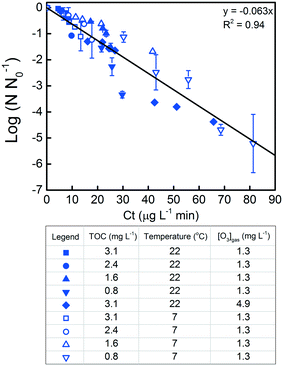 |
| Fig. 1 Summary of L. pneumophila inactivation at 7 and 22 °C with various initial TOC loadings and initial ozone concentrations generated when the ozone generator was driven at 50 V (Cgi = 1.3 g m−3) and 120 V (Cgi = 4.9 g m−3). Data plotted are average values and standard deviations of at least three replicates. | |
3.2. Life cycle human health impacts of disinfection systems
Both disinfection technologies are capable of reducing the overall human health implications of landscape irrigation with secondary effluent, as reflected by the net negative total DALY values in all but one scenario (chlorination with egalitarian weighting; Fig. 2). Regardless of the assumed cultural perspective (individualists, hierarchists, and egalitarians), the DALYs incurred by the treatment are consistently lower for microplasma ozonation than chlorination, and the DALYs avoided because of pathogen inactivation are consistently greater for microplasma ozonation than chlorination. As a result, microplasma ozonation resulted in fewer human health impacts than chlorination when providing the same level of pathogen inactivation. The averted human health impacts were in general more substantial than the life cycle impacts incurred by the construction and operation of the treatment system for both technologies across cultural approaches, exclusive of the egalitarian approach for chlorination (Fig. 2). The microplasma ozonation resulted in net negative DALYs values across all cultural perspectives, and no statistical difference was observed between the human health impacts obtained using the hierarchist or individualist approach (p > 0.05).
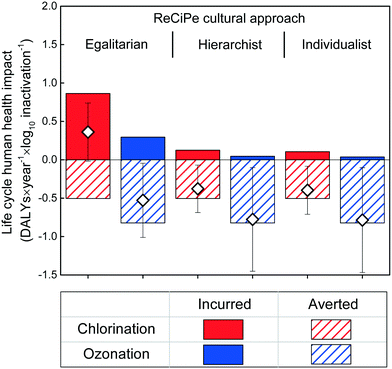 |
| Fig. 2 The impact of cultural perspectives of egalitarians, hierarchists, and individualists on DALYs caused or averted by chlorination and ozonation, together with the total net DALYs. | |
3.3. Sensitivity to scenario and technology assumptions
The microplasma ozonation process incurred fewer human health impacts than chlorination across the majority of the impact categories, such as climate change, human toxicity, and particulate matter emissions (Fig. 3). The impact categories of ozone depletion (OD), photochemical oxidant formation (PO), and ionizing radiation (IR) are much less significant than the other impact categories, being responsible for less than 0.5% of human health impacts for both technologies. For climate change (CC), in particular, chlorination caused more than double the DALYs of microplasma ozonation to achieve the same level of disinfection. The main contributor to the impacts of chlorination were, consistently, consumables and their associated transportation (Fig. 3), accounting for 67–89% and 10–22% of the total incurred DALYs, respectively. The largest source of impacts for the microplasma ozonation were predominantly from electricity consumption (70–99% across all impact categories), with the required materials for the ozone generator and piping assembly being responsible for roughly 1–20% and 1–13% of the total incurred DALYs, respectively. In short, microplasma ozonation provided more human health protection than chlorination across the most impactful environmental categories under the designed conditions and simultaneously incurred fewer human health impacts. These results also point out several directions to reduce the disinfection technology-induced human health impacts. Specifically, the greatest reductions may be achieved through reductions in consumables (chemicals and energy), a finding that is consistent with centralized drinking water treatment systems as well.70
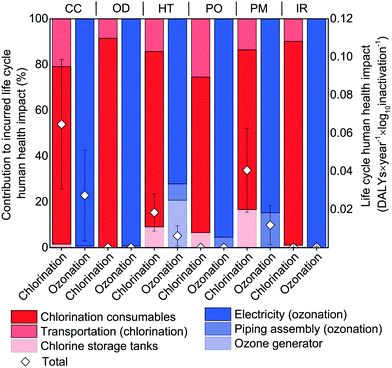 |
| Fig. 3 DALYs incurred by treatment stages and disinfection strategy options (chlorine vs. ozone). White diamonds represent the total normalized DALYs values to be read off the secondary y axis to the right. This figure excludes averted impacts stemming from reduced human exposure to pathogens. | |
Given the importance of electricity to the ozonation system, the sensitivity of these results to the electricity fuel mix was also evaluated (Fig. 4). Despite nearly an order-of-magnitude variability in the human health impact intensity of the non-petroleum energy consumption profiles for the U.S. states (9.8 × 10−8 to 1.4 × 10−6 DALYs kW h−1; used as a surrogate for fuel sources for consumed electricity in a given state), the human health impacts incurred by ozonation were consistently lower than chlorination across all U.S. states. This holds true for the three states with the largest usage of reclaimed wastewater: Florida, California, and Texas (Fig. 4, Fig. S3, Table S3†). Additionally, both chlorination and microplasma ozonation technologies produced negative net DALYs values across all states, suggesting that these technologies are capable of reducing the human health impacts of secondary wastewater effluent reuse for landscape irrigation.
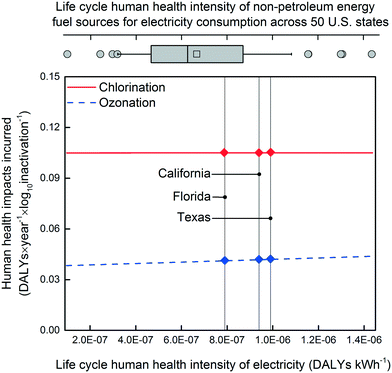 |
| Fig. 4 The relationship between the incurred human health impacts and the life cycle human health intensity (in the unit of DALYs kW h−1) of non-petroleum energy fuel sources for the consumed electricity across 50 U.S. states. Three states with the heaviest reliance on reclaimed water, California, Florida, and Texas, are marked. | |
For both chlorination and ozonation, the inactivation rate constant for L. pneumophila strongly influenced the net human health impacts, underscoring the importance of inactivating such bacteria in reclaimed water for landscape irrigation (Fig. 5a and b). Although C. parvum and Giardia are more resistant to chlorination and ozonation as compared to L. pneumophila, the contribution of the L. pneumophila inactivation rate constants to the human health impacts was consistently higher than those of either C. parvum or Giardia (Fig. 5a and b), due to the higher likelihood of infection caused by the higher identical survival probability for L. pneumophila (MLE estimate of 5.99 × 10−2, compared to 4.19 × 10−3 for C. parvum, and 1.99 × 10−2 for Giardia). For the chlorination system, the human health impacts are most sensitive to the applied sodium hypochlorite dose and the hydraulic residence time, followed by the L. pneumophila inactivation rate constant and water temperature (Fig. 5a). Future improvements to chlorination, therefore, should focus on navigating trade-offs between the chlorine dose and HRT. For the microplasma ozonation system, the reaction of ozone with wastewater organic matter (WOM) plays the most important role as reflected in the sensitivity of human health impacts to COD concentration, to the fraction of ozone demand per carbon of WOM, to the transferred ozone dose, and to the ozone reaction rate constant with WOM (Fig. 5b). Although the need for pre-treatment (e.g., WOM removal) will be locality specific (dependent on the wastewater, preceding processes, etc.), significant reductions in indirect health impacts from microplasma ozonation can also be achieved by increasing the ozone mass transfer efficiency, which increases the transferred ozone dose given a certain applied ozone dose.
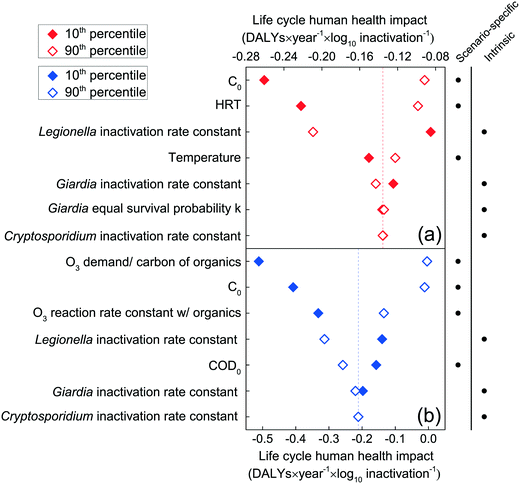 |
| Fig. 5 The top seven input parameters to which human health impacts are most sensitive, for chlorination (a) and microplasma ozonation (b), expressed in DALYs × year−1 × log10 pathogen inactivated−1. Sensitivity analysis for the rest of the parameters can be found in Fig. S4a and b in the ESI.† | |
3.4. Implications for disinfection technologies
To better understand how individual design decisions impact technology performance, a sub-set of parameters that strongly influenced results (initial sodium hypochlorite concentration, C0|Cl2; the transferred ozone dose, C0|O3; hydraulic residence time, HRT) were further analyzed. For chlorination, the life cycle human health impacts were more sensitive to chlorine consumption than reactor sizing (Fig. 3 and Fig. S4a†). As a result, for a fixed level of inactivation, increasing HRT at a given C0|Cl2 (which can achieve the same log10 inactivation as a combination of lower HRT and a higher C0|Cl2) decreases the total DALYs incurred (Fig. 6a and b). To achieve a much higher inactivation level, a higher C0|Cl2 and HRT combination must be used, leading to an increase in the yielded total DALYs normalized by the level of treatment. In other words, the additional human health impacts incurred by the excess levels of treatment cannot be overcome by the additional health impacts averted from more thorough disinfection. For chlorination, therefore, the limited flexibility to reduce life cycle environmental impacts will stem from increasing HRT in order to reduce chlorine consumption. As an example, given the identical level of treatment performance, the change in total net DALYs could be more than 0.2 DALYs per year of operation per log10 inactivation, between operating at C0|Cl2 = 6 mg L−1 and HRT = 20 min, vs. C0|Cl2 = 3 mg L−1 and HRT = 40 min (Fig. 6a and b).
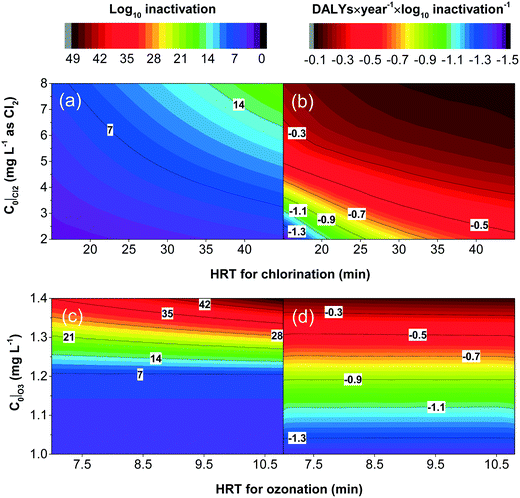 |
| Fig. 6 The relationship between C0|Cl2, HRT, and the net DALYs for different levels of total pathogen inactivation by chlorination (a and b), and the relationship between C0|O3, HRT, and the net DALYs for different levels of total pathogen inactivation by microplasma ozonation (c and d). The left panel (a and c) demonstrates the relationship between log10 pathogens inactivation vs. HRT at various levels of disinfectants; the right panel (b and d) demonstrates the relationship between the normalized net DALYs values and HRT at various levels of disinfectants. Total pathogen inactivation represents the summation of log10 inactivation of three pathogens. For instance, at 12.7 °C, a 27 log10 total inactivation corresponds to approximately 5.2, 0.3, and 21.5 log10 inactivation of Giardia, C. parvum, and L. pneumophila, respectively. | |
For microplasma ozonation, the transferred ozone dose and HRT are critical for life cycle human health impacts. During operation, the level of inactivation by ozone depends on the value of the transferred ozone dose C0|O3 and HRT. When the transferred ozone dose C0|O3 is too small, ozone is quickly consumed, and the inactivation would appear independent of HRT as represented by a horizontal trend in Fig. 6c at lower C0|O3. This observation is consistent with previous findings that the transferred ozone dose rather than HRT could sometimes be the determining factor in controlling inactivation.11 At this low level of transferred ozone dose, for a given level of inactivation, the normalized DALYs values did not increase significantly with HRT since contact tank materials were not a significant driver for life cycle environmental impacts (as identified in Fig. S4b†).
To increase the pathogen inactivation, the transferred ozone dose must increase. At a higher C0|O3, an increase in HRT will also increase inactivation. This influence of HRT on total pathogen inactivation is only observable at a higher C0|O3. This result is consistent with the results from the L. pneumophila inactivation by microplasma ozonation (Fig. 7). At a dissolved ozone concentration of 50 μg L−1 at 0.2 min (Fig. 7b), the log10 inactivation of L. pneumophila was approximately 3 (Fig. 7a), which increased as the dissolved ozone was consumed gradually as the contact time increased. In other words, as long as the residual ozone was available, the HRT would have an effect on overall inactivation. Therefore, the transferred ozone dose must be greater than the wastewater-specific thresholds to achieve residual ozone and increase disinfection efficacy by increasing HRT. A further increase in C0|O3 will enhance the inactivation, but the DALYs incurred because of additional life cycle impacts will be larger than the DALYs averted due to reduced pathogen exposure locally (Fig. 6c and d).
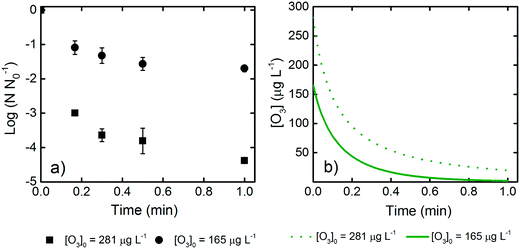 |
| Fig. 7 At 22 °C (a) the effect of HRT on L. pneumophila inactivation, at two initial ozone concentrations and a WOM loading of 3.1 mg C L−1 in a semi-batch reactor; (b) the profile of two initial dissolved ozone concentrations as a function of HRT. The data plotted in (a) are average values and standard deviations of three replicates. The dashed and solid curves in (b) are the results of calculations. | |
For the same mass of ozone produced, given the linear relationship between the incurred DALY values and electric power consumption, less dependence on electricity directly corresponds to fewer incurred DALYs. The microchannel plasma ozone generator requires less power (efficiency of 90 g kW h−1 if fed with air, more than 120 g kW h−1 if fed with oxygen) than a conventional dielectric barrier discharge reactor (e.g. 50 g kW h−1) per mass of ozone produced. Therefore, from the point of view of lowering the life cycle human health impacts, one aspect that future ozone technology development could focus on is reducing the energy consumption per mass of ozone produced.
3.5. Limitations of the study
Before applying the model framework presented in this work to predict dissolved ozone concentration, parameters in the developed model, including the ozone self-decay rate constant k, the volumetric mass transfer coefficient kLa, and the reaction rate constant between WOM and ozone k2 must be fitted to wastewater from specific sources to account for the variations in wastewater characteristics. Additionally, since the actual reactions involved between ozone and various components in the wastewater are likely to be very complex and numerous, models incorporating more details in specific reactions (e.g., additional ozone consuming reactions, such as between ozone and nitrite ions) will likely improve the modeling results. To factor in the effect of temperature, Arrhenius law was employed for all reaction rate constants, except for ozone inactivation of L. pneumophila, since the wastewater matrix led to an insignificant difference in inactivation rate constants at 7 and 22 °C (p > 0.05). Therefore, we assumed an identical L. pneumophila inactivation rate constant using ozone throughout the simulation. Future efforts should validate such observations in wastewater matrices that are equally complex as, if not more complex than, the secondary effluent used in this study.
Additionally, more robust modeling of direct human health implications (both from pathogens and chemicals in reclaimed water) would also improve model accuracy. For example, DBPs generated during disinfection may pose risks to human health, but they were not addressed in this study due to the lack of characterization factors. Although more data related to pathogens of concern would also improve modeling accuracy and enable real-time optimization of disinfection systems, a more routinely monitored microbial parameter to evaluate wastewater disinfection is the total coliform concentration (which is regulated based on different permissible levels depending on the downstream purposes of the wastewater1). As reflected by the sensitivity of the results to pathogen selection (Fig. 5a and b), if future management strategies incorporate more pathogens for routine monitoring, the inclusion of ones that are prevalent in wastewater, such as viruses, may further enhance the comprehensiveness of the current results. Although uncertainties of various sources were taken into account throughout the study, the lack of information on numerous occasions, such as the influent concentration of pathogens, may have caused unwanted uncertainties in the results.
Moreover, other operational practices that could impact disinfection efficacies, such as pH adjustment during chlorination, were not considered in this study. As discussed, the influent COD has a significant impact on the human health performance of the microplasma ozonation system (Fig. 5b), and, therefore, pre-treatments that effectively remove organic matter could potentially improve the human health protection. As a result, further effort could incorporate more operational conditions to offer insights into the relative contribution of each.
To approximate the comparison between conventional ozonation and chlorination, if we assume a hierarchist approach, a typical ozone production efficiency of 40 g kW h−1 at low input voltage (120 V), the human health impacts incurred by ozonation operation would be 0.08 ± 0.09 DALYs × year−1 × log10 pathogen inactivated−1, which is not statistically significantly different from that caused by chlorination (0.13 ± 0.07 DALYs × year−1 × log10 pathogen inactivated−1, p > 0.05).
4. Conclusions
The inactivation parameters for ozone inactivation of L. pneumophila in secondary wastewater and two alternative technologies – chlorination followed by dechlorination, and microplasma ozonation – for wastewater reuse disinfection based on the human health impacts were determined and compared. LCA results revealed that the operation of the microplasma ozonation system has lower impact on human health than the chlorination system in five out of six impact categories. These results were robust and were consistent across electricity/fuel mixes in 50 U.S. states, as well as the three cultural perspectives used in impact assessment – in all cases, the overall human health impacts caused by the microplasma ozonation was consistently lower than that of the chlorination approach.
For the chlorination system, life cycle DALYs were most sensitive to chlorine consumption, enabling minor reductions in impacts by increasing HRT and reducing chemical dosing to achieve the same level of disinfection. For the microplasma ozonation system, depending on the desired level of pathogen inactivation, design criteria such as using a higher transferred ozone dose (just enough to produce residual ozone throughout the ideal part of HRT) combined with a longer HRT should be considered to optimize the system's human health impact performance for a given inactivation goal. Nevertheless, other important factors such as costs also need to be considered to produce a feasible design plan.
Acknowledgements
This project was partially supported by grant RD83582201-0 from the U.S. Environmental Protection Agency (EPA) and an EPA/USDA-NIFA grant. Its contents are solely the responsibility of the grantee and do not necessarily represent the official views of the EPA. Further, the EPA does not endorse the purchase of any commercial products or services mentioned in the publication.
References
-
US Environmental Protection Agency, Guidelines for water reuse, US Agency for International Development, Washington DC, 2012 Search PubMed.
-
National Research Council Committee, Water Reuse: Potential for Expanding the Nation's Water Supply Through Reuse of Municipal Wastewater, National Academies Press, 2012 Search PubMed.
-
J. C. Crittenden, R. R. Trussell, D. W. Hand, K. J. Howe and G. Tchobanoglous, MWH's Water Treatment: Principles and Design, John Wiley & Sons, 2012 Search PubMed.
-
US Environmental Protection Agency, Wastewater Technology Fact Sheet: Chlorine Disinfection, 1999 Search PubMed.
-
E. A. Bryant, G. P. Fulton and G. C. Budd, Disinfection alternatives for safe drinking water, Van Nostrand Reinhold, 1992 Search PubMed.
- X. Yang, C. Shang and J.-C. Huang, DBP formation in breakpoint chlorination of wastewater, Water Res., 2005, 39(19), 4755–4767 CrossRef CAS PubMed.
- G. Hua and D. A. Reckhow, DBP formation during chlorination and chloramination: effect of reaction time, pH, dosage, and temperature, J. - Am. Water Works Assoc., 2008, 100(8), 82–95 CAS.
- D. Korich, J. Mead, M. Madore, N. Sinclair and C. R. Sterling, Effects of ozone, chlorine dioxide, chlorine, and monochloramine on Cryptosporidium parvum oocyst viability, Appl. Environ. Microbiol., 1990, 56(5), 1423–1428 CAS.
- G.-A. Shin and M. D. Sobsey, Reduction of Norwalk virus, poliovirus 1, and bacteriophage MS2 by ozone disinfection of water, Appl. Environ. Microbiol., 2003, 69(7), 3975–3978 CrossRef CAS PubMed.
- S. B. Majumdar, W. H. Ceckler and O. J. Sproul, Inactivation of poliovirus in water by ozonation, J. - Water Pollut. Control Fed., 1973, 2433–2443 CAS.
- P. Xu, M.-L. Janex, P. Savoye, A. Cockx and V. Lazarova, Wastewater disinfection by ozone: main parameters for process design, Water Res., 2002, 36(4), 1043–1055 CrossRef CAS PubMed.
- N. K. Hunt and B. J. Mariñas, Kinetics of Escherichia coli inactivation with ozone, Water Res., 1997, 31(6), 1355–1362 CrossRef.
- M. Cho and J. Yoon, Quantitative evaluation and application of Cryptosporidium parvum inactivation with ozone treatment, Water Sci. Technol., 2007, 55(1–2), 241–250 CrossRef CAS PubMed.
- G. Tang, K. Adu-Sarkodie, D. Kim, J.-H. Kim, S. Teefy, H. M. Shukairy and B. J. Mariñas, Modeling Cryptosporidium parvum oocyst inactivation and bromate formation in a full-scale ozone contactor, Environ. Sci. Technol., 2005, 39(23), 9343–9350 CrossRef CAS PubMed.
- J.-H. Kim, M. S. Elovitz, U. Von Gunten, H. M. Shukairy and B. J. Mariñas, Modeling Cryptosporidium parvum oocyst inactivation and bromate in a flow-through ozone contactor treating natural water, Water Res., 2007, 41(2), 467–475 CrossRef CAS PubMed.
- J.-H. Kim, U. von Gunten and B. J. Mariñas, Simultaneous prediction of Cryptosporidium parvum oocyst inactivation and bromate formation during ozonation of synthetic waters, Environ. Sci. Technol., 2004, 38(7), 2232–2241 CrossRef CAS PubMed.
- B. Corona-Vasquez, A. Samuelson, J. L. Rennecker and B. J. Mariñas, Inactivation of Cryptosporidium parvum oocysts with ozone and free chlorine, Water Res., 2002, 36(16), 4053–4063 CrossRef CAS PubMed.
- S. Dong, J. Lu, M. J. Plewa and T. H. Nguyen, Comparative Mammalian Cell Cytotoxicity of Wastewaters for Agricultural Reuse after Ozonation, Environ. Sci. Technol., 2016, 50(21), 11752–11759 CrossRef CAS PubMed.
- M. Kim, J. Cho, S. Ban, R. Choi, E. Kwon, S. Park and J. Eden, Efficient generation of ozone in arrays of microchannel plasmas, J. Phys. D: Appl. Phys., 2013, 46(30), 305201 CrossRef.
-
Ozone solutions Inc., WIS-600: Ozone Injection System product specification sheet, 2015 Search PubMed.
- J. Cho, S.-J. Park and J. Eden, Propagation and decay of low temperature
plasma packets in arrays of dielectric microchannels, Appl. Phys. Lett., 2012, 101(25), 253508 CrossRef.
-
C. N. Haas, J. B. Rose and C. P. Gerba, Quantitative microbial risk assessment, John Wiley & Sons, 1999 Search PubMed.
-
M. A. Curran, Life cycle assessment, National Institute of Standards and Technology, Gaithersburg, MD (United States), 1994 Search PubMed.
- Y. Kobayashi, G. M. Peters, N. J. Ashbolt, S. Heimersson, M. Svanström and S. J. Khan, Global and local health burden trade-off through the hybridisation of quantitative microbial risk assessment and life cycle assessment to aid water management, Water Res., 2015, 79, 26–38 CrossRef CAS PubMed.
- N. Tangsubkul, P. Beavis, S. Moore, S. Lundie and T. Waite, Life cycle assessment of water recycling technology, Water Resour. Manage., 2005, 19(5), 521–537 CrossRef.
- L. Hoibye, J. Clauson-Kaas, H. Wenzel, H. F. Larsen, B. N. Jacobsen and O. Dalgaard, Sustainability assessment of advanced wastewater treatment technologies, Water Sci. Technol., 2008, 58(5), 963 CrossRef CAS PubMed.
- P. Beavis and S. Lundie, Integrated environmental assessment of tertiary and residuals treatment-LCA in the wastewater industry, Water Sci. Technol., 2003, 47(7–8), 109–116 CAS.
- H. Wenzel, H. F. Larsen, J. Clauson-Kaas, L. Høibye and B. N. Jacobsen, Weighing environmental advantages and disadvantages of advanced wastewater treatment of micro-pollutants using environmental life cycle assessment, Water Sci. Technol., 2008, 57(1), 27–32 CrossRef CAS PubMed.
- M. Meneses, J. C. Pasqualino and F. Castells, Environmental assessment of urban wastewater reuse: treatment alternatives and applications, Chemosphere, 2010, 81(2), 266–272 CrossRef CAS PubMed.
- I. Munoz, A. Rodriguez, R. Rosal and A. R. Fernandez-Alba, Life cycle assessment of urban wastewater reuse with ozonation as tertiary treatment: a focus on toxicity-related impacts, Sci. Total Environ., 2009, 407(4), 1245–1256 CrossRef CAS PubMed.
- T. K. Das, Evaluating the life cycle environmental performance of chlorine disinfection and ultraviolet technologies, Clean Technol. Environ., 2002, 4(1), 32–43 CrossRef CAS.
- P. Nilsson, D. Roser, R. Thorwaldsdotter, S. Petterson, C. Davies, R. Signor, O. Bergstedt and N. Ashbolt, SCADA data and the quantification of hazardous events for QMRA, J. Water Health, 2007, 5(S1), 99–105 CrossRef PubMed.
- S. Petterson and T. Stenström, Quantification of pathogen inactivation efficacy by free chlorine disinfection of drinking water for QMRA, J. Water Health, 2015, 13(3), 625–644 CrossRef CAS PubMed.
- L. Zhou, S. Echigo, Y. Ohkouchi and S. Itoh, Quantitative microbial risk assessment of drinking water treated with advanced water treatment process, J. Water Supply: Res. Technol.--AQUA, 2014, 63(2), 114–123 CrossRef.
- K. Jaidi, B. Barbeau, A. Carrière, R. Desjardins and M. Prévost, Including operational data in QMRA model: development and impact of model inputs, J. Water Health, 2009, 7(1), 77–95 CrossRef PubMed.
- J. M. Thomas, T. Thomas, R. M. Stuetz and N. J. Ashbolt, Your garden hose: a potential health risk due to Legionella spp. growth facilitated by free-living amoebae, Environ. Sci. Technol., 2014, 48(17), 10456–10464 CrossRef CAS PubMed.
- S. Collier, L. Stockman, L. Hicks, L. Garrison, F. Zhou and M. Beach, Direct healthcare costs of selected diseases primarily or partially transmitted by water, Epidemiol. Infect., 2012, 140(11), 2003–2013 CrossRef CAS PubMed.
-
New York City Department of Health and Mental Hygiene, Legionellosis Cluster in the South Bronx and Morris Park from 07/08/2015 to 10/06/2015 based on onset date, 2015.
- D. L. Johnsen, H. Emamipour, J. S. Guest and M. J. Rood, Environmental and Economic Assessment of Electrothermal Swing Adsorption of Air Emissions from Sheet-Foam Production Compared to Conventional Abatement Techniques, Environ. Sci. Technol., 2016, 50(3), 1465–1472 CrossRef CAS PubMed.
- E. L. Jarroll and J. C. Hoff, Effect of disinfectants on Giardia cysts, Crit. Rev. Environ. Sci. Technol., 1988, 18(1), 1–28 CAS.
- A. M. Driedger, J. L. Rennecker and B. J. Mariñas, Inactivation of Cryptosporidium parvum oocysts with ozone and monochloramine at low temperature, Water Res., 2001, 35(1), 41–48 CrossRef CAS PubMed.
- J. L. Rennecker, B. J. Mariñas, J. H. Owens and E. W. Rice, Inactivation of Cryptosporidium parvum oocysts with ozone, Water Res., 1999, 33(11), 2481–2488 CrossRef CAS.
- D. Cunliffe, Inactivation of Legionella pneumophila by monochloramine, J. Appl. Bacteriol., 1990, 68(5), 453–459 CrossRef CAS PubMed.
- H. Bader and J. Hoigné, Determination of ozone in water by the indigo method, Water Res., 1981, 15(4), 449–456 CrossRef CAS.
- E. L. Domingue, R. Tyndall, W. Mayberry and O. Pancorbo, Effects of three oxidizing biocides on Legionella pneumophila serogroup 1, Appl. Environ. Microbiol., 1988, 54(3), 741–747 CAS.
- M. E. Williams and J. L. Darby, Measuring ozone by indigo method: interference of suspended material, J. Environ. Eng., 1992, 118(6), 988–993 CrossRef CAS.
- M. C. Dodd, M.-O. Buffle and U. Von Gunten, Oxidation of antibacterial molecules by aqueous ozone: moiety-specific reaction kinetics and application to ozone-based wastewater treatment, Environ. Sci. Technol., 2006, 40(6), 1969–1977 CrossRef CAS PubMed.
- M. C. Dodd, H.-P. E. Kohler and U. Von Gunten, Oxidation of antibacterial compounds by ozone and hydroxyl radical: elimination of biological activity during aqueous ozonation processes, Environ. Sci. Technol., 2009, 43(7), 2498–2504 CrossRef CAS PubMed.
- S. A. Snyder, E. C. Wert, D. J. Rexing, R. E. Zegers and D. D. Drury, Ozone oxidation of endocrine disruptors and pharmaceuticals in surface water and wastewater, Ozone: Sci. Eng., 2006, 28(6), 445–460 CrossRef CAS.
- K. L. Rakness, K. M. Corsaro, G. Hale and B. D. Blank, Wastewater Disinfection With Ozone-Process Control And Operating Results, Ozone: Sci. Eng., 1993, 15(6), 497–513 CrossRef CAS.
- C. M. Robson and R. G. Rice, Wastewater Ozonation in the USA–History and Current Status-1989, Ozone: Sci. Eng., 1991, 13(1), 23–40 CrossRef CAS.
-
US Energy Information Administration, Annual state net electricity profile by source 2014, 2014 Search PubMed.
- L. Corominas, J. Foley, J. Guest, A. Hospido, H. Larsen, S. Morera and A. Shaw, Life cycle assessment applied to wastewater treatment: state of the art, Water Res., 2013, 47(15), 5480–5492 CrossRef CAS PubMed.
-
G. Tchobanoglous, F. Burton and D. Stensel, Wastewater Engineering (Treatment, Disposal and Reuse), Metcalf and Eddy, New York, 1991, vol. 1334 Search PubMed.
- H. L. DuPont, C. L. Chappell, C. R. Sterling, P. C. Okhuysen, J. B. Rose and W. Jakubowski, The infectivity of Cryptosporidium parvum in healthy volunteers, N. Engl. J. Med., 1995, 332(13), 855–859 CrossRef CAS PubMed.
- R. C. Rendtorff, The experimental transmission of human intestinal protozoan parasites. II. Giardia lamblia cysts given in capsules, Am. J. Hyg., 1954, 59(2), 209–220 CAS.
- D. Muller, M. L. Edwards and D. W. Smith, Changes in Iron and Transferrin Levels and Body Temperature in Experimental Airborne Legionellosis, J. Infect. Dis., 1983, 147(2), 302–307 CrossRef CAS PubMed.
- B. P. Weidema and M. S. Wesnæs, Data quality management for life cycle inventories—an example of using data quality indicators, J. Cleaner Prod., 1996, 4(3), 167–174 CrossRef.
- B. P. Weidema, Multi-user test of the data quality matrix for product life cycle inventory data, Int. J. Life Cycle Assess., 1998, 3(5), 259–265 CrossRef.
-
NRMMC, E., AHMC, Australian
Guidelines for Water REcycling: Managing Health and Environmental Risks (Phase 1), Natural Resource Ministerial Management Council, Environment Protection and Heritage Council and Australian Health Ministers, 2006 Search PubMed.
- H. Wang, M. Edwards, J. O. Falkinham and A. Pruden, Molecular Survey of the Occurrence of Legionella spp., Mycobacterium spp., Pseudomonas aeruginosa, and Amoeba Hosts in Two Chloraminated Drinking Water Distribution Systems, Appl. Environ. Microbiol., 2012, 78(17), 6285–6294 CrossRef CAS PubMed.
- C. J. Palmer, G. F. Bonilla, B. Roll, C. Paszko-Kolva, L. R. Sangermano and R. S. Fujioka, Detection of Legionella species in reclaimed water and air with the EnviroAmp Legionella PCR kit and direct fluorescent antibody staining, Appl. Environ. Microbiol., 1995, 61(2), 407–412 CAS.
- G. Medema, B. Wullings, P. Roeleveld and D. Van Der Kooij, Risk assessment of Legionella and enteric pathogens in sewage treatment works, Water Sci. Technol.: Water Supply, 2004, 4(2), 125–132 Search PubMed.
- Texas Commission on Environmental Quality, Landscape Irrigation, A Take Care of Texas Guide, 2015.
- Maryland Department of the Environment Engineering and Capital Projects Program, Design Guidelines for Wastewater Facilities, 2012.
- M. E. Schoen and N. J. Ashbolt, An in-premise model for Legionella exposure during showering events, Water Res., 2011, 45(18), 5826–5836 CrossRef CAS PubMed.
- K. B. Gibney, J. O'Toole, M. Sinclair and K. Leder, Disease burden of selected gastrointestinal pathogens in Australia, 2010, Int. J. Infect. Dis., 2014, 28, 176–185 CrossRef PubMed.
-
A. Havelaar and J. Melse, Quantifying public health risk in the WHO Guidelines for drinking-water quality: A burden of disease approach, 2003 Search PubMed.
- N. K. Hunt and B. J. Mariñas, Inactivation of Escherichia coli with ozone: chemical and inactivation kinetics, Water Res., 1999, 33(11), 2633–2641 CrossRef CAS.
- J. K. Choe, M. H. Mehnert, J. S. Guest, T. J. Strathmann and C. J. Werth, Comparative assessment of the environmental sustainability of existing and emerging perchlorate treatment technologies for drinking water, Environ. Sci. Technol., 2013, 47(9), 4644–4652 CrossRef CAS PubMed.
Footnote |
† Electronic supplementary information (ESI) available. See DOI: 10.1039/c6ew00235h |
|
This journal is © The Royal Society of Chemistry 2017 |
Click here to see how this site uses Cookies. View our privacy policy here.