DOI:
10.1039/D1QM00969A
(Review Article)
Mater. Chem. Front., 2021,
5, 7931-7963
Advanced XPS characterization: XPS-based multi-technique analyses for comprehensive understanding of functional materials
Received
23rd July 2021
, Accepted 22nd September 2021
First published on 23rd September 2021
Abstract
X-ray photoelectron spectroscopy (XPS) has achieved maturity as an analytical technique in that it is a ubiquitous tool in the materials community, however as made apparent by recent reviews highlighting it's misuse as a means of chemical deduction, it is a practice which is greatly misunderstood even in its simplest form. Advanced XPS techniques, or a combination of XPS and a complementary surficial probe may elicit auxiliary information outside of the scope of the standard sphere of appreciation. This review aims to bring to the attention of the general materials audience a landscape of some atypical applications of lab-based XPS and combinatorial approaches of related surface analysis, such as ion scattering, ultraviolet photoelectron, electron energy loss and auger emission spectroscopies found on many lab-based instrument set-ups.
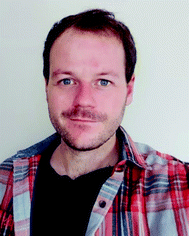
Mark A. Isaacs
| Mark Isaacs graduated with an MChem from Cardiff University in 2010, before undertaking a PhD with Profs Adam Lee and Karen Wilson in the development of silver-based nanocomposites for antibacterial applications. He moved to Aston university as a PDRA in 2014, developing hierarchically porous catalysts for domino or cascade reactions, before joining University College London and HarwellXPS in 2018. His current research interests include developing composite oxide porous nanomaterials and the study of nanomaterial formation by XPS and related surface analysis techniques. He is the current chair for the Society of Chemical Industry's early career materials science committee. |

Josh Davies-Jones
| Josh Davies-Jones graduated from Cardiff University in 2012 with a Master of Chemistry. He pursued his PhD in materials science under the supervision of Professor Phil Davies. Studying surface chemistry of anisotropic nanoparticles used for magneto-optical diagnosis. Josh joined Harwell XPS in 2021 as a postdoctoral researcher exploring novel applications of CRYO-XPS. |
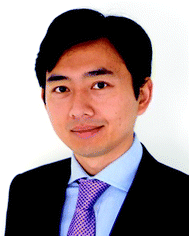
Shaoliang Guan
| Shaoliang Guan is currently a Research Associate and Co-Investigator at the EPSRC National Facility for Photoelectron Spectroscopy (HarwellXPS), UK. He received his BS degree in Applied Chemistry from Beijing University of Chemical Technology (China) and MSc in Chemical Enzymology from Queen's University Belfast (UK). After that, he received his PhD degree in Electrochemistry from Cardiff University (UK) under the supervision of Prof. Gary Attard in 2015. His research interests include investigating selectively catalytic hydrogenation using electrochemical methods and CO oxy-chlorination reactions with various surface characterization techniques such as X-ray photoelectron spectroscopy and Surface enhanced Raman spectroscopy. |
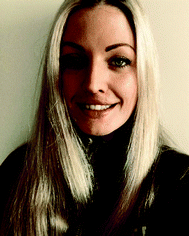
Roxy Lee
| Roxy graduated with an MSci Chemistry from UCL in 2018. In her fourth year project she developed and modified nanostructured TiO2 electrodes and used these to fabricate and analyse their performance in solar cells. She is currently enrolled on an EngD programme (2019–2023), sponsored by Thermo Fisher Scientific, and focuses on photoemission spectroscopy and valence band spectrum analysis. She also uses computational methods to simulate photoemission spectra, and uses a joint experimental and theoretical approach in her research. |
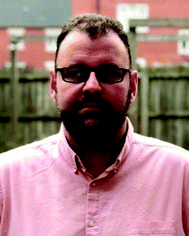
David J. Morgan
| David J. Morgan obtained his PhD in surface science and catalysis in 2002 from Cardiff University under the supervision of Prof. P. R. Davies, where he then undertook postdoctoral research under the supervision of Prof. Graham Hutchings FRS focusing on model catalytic studies using high pressure XPS and oversaw the ESPRC Access to Research Equipment initiative for X-Ray Photoelectron Spectroscopy. Since 2007 he has been the Surface Analysis manager at Cardiff University and the technical manager of HarwellXPS since 2017. He has interests in standards, experimental design, data analysis and multi-technique analysis. Roxy graduated with an MSci Chemistry from UCL in 2018. In her fourth year project she developed and modified nanostructured TiO2 electrodes and used these to fabricate and analyse their performance in solar cells. She is currently enrolled on an EngD programme (2019–2023), sponsored by Thermo Fisher Scientific, and focuses on photoemission spectroscopy and valence band spectrum analysis. She also uses computational methods to simulate photoemission spectra, and uses a joint experimental and theoretical approach in her research. |
Introduction
Functional materials on the nano- to macro-scale provide solutions for some of the most critical scientific problems of the current age, from the use of heterogeneous catalysts to improve the environmental footprint and commercial viability of fine chemical synthesis (e.g. pharmaceuticals, food additives, cosmetics)1 to solid state materials as energy storage materials,2 fuel cells3 or photovoltaics4 to tackle humanity's dependence on dwindling resources such as fossil fuels. Such is the scope in the application of functional materials that examples may be found across the spectrum of the physical sciences; including drug-delivery systems,5 molecular sensors,6 tackling drug resistant pathogens,7 aerospace components,8 electronics9 and many more. In order to design and develop the most effective materials, a thorough understanding of the material properties is often paramount – particularly at the surface of a material which forms the interactive boundary between the functional material and the materials or substances on which they will act.10
X-ray photoelectron spectroscopy (XPS) has become a well-known technique in the field of materials science, one which may be used to probe the elemental, electronic and chemical characteristics of a material surface.11–14 While the interpretation of XPS data via peak deconstruction is commonplace, fundamental understanding of the technique and an appreciation for correct data handling are often missing.15 Recently, a series of invaluable guides have been prepared from a community effort among the leading practitioners in the field of XPS, with the aim of enabling researchers new to XPS to plan experiments and understand their data to a high level. This series is published under the title ‘Practical Guides for X-Ray Photoelectron Spectroscopy’, for example ‘Practical Guides for X-ray Photoelectron Spectroscopy: First Steps in Planning, Conducting, and Reporting XPS Measurements’16 and ‘Practical guide for curve fitting in X-ray photoelectron spectroscopy.’17 Furthermore, there exist a number of advanced techniques with which many materials scientists will be unfamiliar. Furthermore, a current focus of several of the XPS manufacturers is high throughput examination of a single analysis spot using an assembly of surface probes and even non-surface specific techniques such as Raman spectroscopy. As the availability of such systems becomes more widespread, the a wider knowledge of the capabilities, strengths and weaknesses of multi-technique surface analysis is required.
This review aims to highlight the benefits of such a combinatorial approach to the analysis of materials utilising lab-based XPS and related surface techniques. Applications of those experimental probes which may be found most regularly on lab-based systems (ion scattering, ultraviolet photoelectron, auger emission and electron energy loss spectroscopies) are covered in detail here, though many other complementary approaches may provide pathways through which to tackle research problems in materials analysis. As mentioned above, Raman spectroscopy has found much interest recently in unison with XPS, since Raman may afford a probe of structural properties including analysis of defect sites, often of paramount importance when dealing with surfaces and interfaces (the study of which XPS excels at).18–22 Such is the potential of this approach, selected commercial instruments now afford the option of an ‘off-the-shelf’ Raman module upgrade – permitting users to perform tandem experiments with little to no experience of the modification of UHV chambers.
The sheer scope of combinatorial analysis renders a full discussion of all methods impractical, and hence only a selection (of those deemed most readily available) will be presented here, however for a look into other powerful conjunctive techniques the reader may be directed towards some reviews highlighting the use of XPS/Scanning Tunnelling Microscopy (STM),23–25 XPS/Scanning Probe Microscopy (SPM),25,26 XPS/Infrared Reflectance Absorption Spectroscopy (IRRAS),27–29 XPS/Time-of-Flight Secondary Ion Mass Spectroscopy (TOF-SIMS)25,26 and XPS/Atomic Force Microscopy (AFM)25,27 though even this is itself by no means an exhaustive list.
Advanced XPS techniques
As well as providing a method by which to qualitatively and quantitatively describe the chemical nature of the surfaces of materials, XPS can also provide insights into the topology, morphology and homogeneity of a sample as a function of depth. Such techniques may facilitate the understanding of new interfaces (e.g. in semiconductors, electrodes),30,31 solid synthesis pathways,32 catalytic and surface processes33–35 and electronically active materials,36,37 among others.
Tougaard background analysis
Each individual photoemission peak in an XP spectrum is accompanied by a characteristic stepped background (Fig. 1) which is the result of photoelectrons which have undergone an inelastic scattering process.38 In order to correctly analyse XP data, this inelastic scattering process must be properly accounted for in the form of fitted model background.39
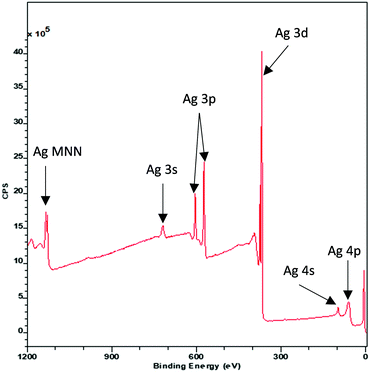 |
| Fig. 1 X-ray photoelectron survey spectrum of a clean silver surface. | |
The most widely used model, known as the Shirley background after its creator D. A. Shirley,40 uses an iterative process to calculate the number of inelastically scattered electrons based upon the integrated peak area either side of the energy E. It has proven to be a reliable and robust method for accounting for inelastic scatter. However, the physical origin of the Shirley background is rather poorly defined.41 Detailed theoretical analysis with comparison to experimental data has led to much improved models of electron inelastic scattering42 and a new method for quantitatively describing the XPS background named after its creator Sven Tougaard has been developed.43 A discussion of the merits of the various background types for standard XPS analysis is beyond the scope of this review but we will highlight how Tougaard's approach provides a non-destructive technique by which depth profile and structural information, including the spatial arrangement of overlayers, islands and sandwich structures can be obtained from the background profiles to complex composite materials.44
Nucleation and growth modes in systematic samples can be obtained with an even greater degree of accuracy and consistency than that provided by ellipsometry or Rutherford backscattering spectroscopy.45,46 Tougaard background analysis has yielded excellent agreement with atomic force microscopy (AFM) measurements in determining Stransky–Krastanov growth of NiO deposition onto highly ordered pyrolytic graphite (HOPG).47 The technique has advanced to the point where analysis may be performed quickly and easily using specialist software (QUASES).48,49
Overlayer thickness analysis
Nanocomposite materials in the form of thin films or core–shell particles often offer unique solutions to critical research problems in areas such as electronics,50 medical devices32 and catalysis.51 Control over the film or shell thickness can be vital to obtain the precise physical and electronic properties required of such composite materials and therefore a robust method is needed to determine said thickness. X-ray reflectivity (XRR)52 and electron microscopy53 are often used for this purpose, however they are not without drawbacks; XRR requires highly ordered samples with almost no surface roughness, whilst electron microscopy requires long analysis times to obtain a statistically significant dataset. XPS can provide an alternative approach to film thickness determination involving a large area analysis with few limitations regarding sample chemistry.
For planar materials, such as semiconductor thin films deposited onto a substrate, there are a number of approaches to using XPS to determine film thickness. For simple metal oxide on metal systems such as Al2O3/Al for example, one may use the Strohmeier equation (eqn (1)) based on a single high resolution region spectrum.54,55
| 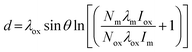 | (1) |
Strohmeier equation;
λ = inelastic mean free path,
N = volume density (density/mass),
I = intensity and
θ = photoelectron take-off angle.
This method assumes a uniform overlayer and that the photoelectrons from both metal and oxide are of similar energy (hence is largely restricted to metal oxide layers of the same element as the underlying metal).
Differences in the inelastic mean free path (IMFP) between oxide and metal permit a facile calculation of path length using only intensity ratios between the two species. An important note for users relatively unfamiliar with XPS, is that between IMFP and the information depth of the sample. IMFP (λ) is defined as the distance between successive inelastic collisions of photoelectrons and the surrounding medium (i.e. atoms in the sample as the photoelectron travels to the surface). The information depth (ID), refers to the depth of analysis from which the majority (>95%) of the signal originates.57 This is dependent on both the material IMFP and the energy of the incoming X-rays and is typically found to be approximate to 3λ.
Since the electron escape path length scales with the angle of emission (while X-ray penetration depth remains constant, Fig. 2), angle resolved XPS (ARXPS) provides a simple, effective and non-destructive system by which to measure film thickness and/or produce relative depth profiles for mapping layer structures.58–61 A major advantage of ARXPS over XRR and electron microscopy is the ability to not only probe film thicknesses but also reveal elemental and chemical environments at differing depths.62–64 This makes ARXPS a particularly useful tool for studying solid interfaces, which play a crucial role in many electronic systems.65–68 Application of ARXPS to the valence orbitals is also extensively used to map electronic band structures.69
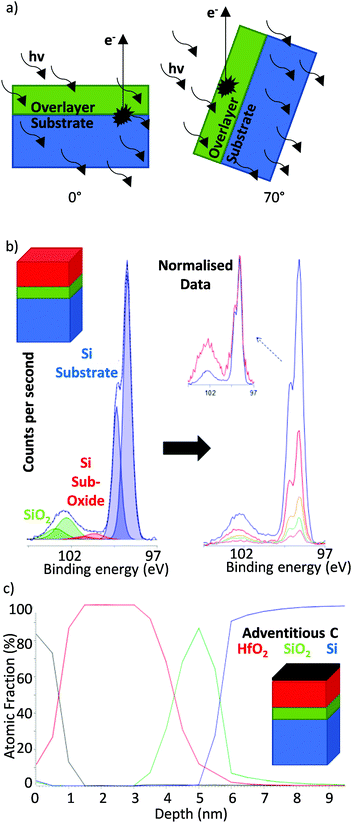 |
| Fig. 2 (a) Angular dependence of the electron path length in ARXPS (vertical black arrows represent photoelectron pathway to the sample surface the escape depth of which, perpendicular to the sample surface, is dependent on IMFP and sample angle), (b) example of ARXPS applied to Hf oxide films on a silicon substrate in which dependence of silicon signal on angle of emission may be clearly observed and (c) relative depth profile calculated from the quantitatively processed spectra in (b). Reprinted (adapted) with permission from ref. 56. Copyright {2019} Kratos Analytical. | |
One method with which ultrathin-film thickness may be determined is by monitoring the intensity of the substrate before and after deposition of an overlayer and using known inelastic mean free path (IMFP) lengths to calculate the path length of the attenuating material (overlayer thickness).70 This method is not without it's restrictions, since it may not account for differences in surface contamination, and surface topography and it also assumes identical experimental conditions and a pure substrate sample – which may not always be possible. It is, however, an excellent probe of uniform flat surfaces such as those prepared via deposition.71 A technique developed by Cumpson known as the “thickogram” facilitates overlayer analysis from a single XPS spectrum via graphical means.72 The method uses minimal calculation, and has the advantages of being independent of contamination layer and instrument factors, and works well over a large range of thicknesses and energies. The ratio of kinetic energies and the ratio of integrated peak areas for a substrate and overlayer peak, are plotted onto a thickogram template. The intersection of a line joining these two points with the third axis on the thickogram provides a value for the coating thickness. Since sample morphology may still influence the analysis when using this method, a series of correction factors (known as topofactors) have been derived by Shard which may permit the determination of non-uniform sample surfaces and even complex structures such as core shell nanoarchitectures.73
The analysis of smaller particles (<10 nm) requires particular consideration since the substrate on which the particles are mounted may also contribute to the XP spectrum and in these cases considerable care must be taken with methods such as Tougaard's background analysis. Angle-resolved measurements are typically unsuitable for such systems, since at such small size ranges we have topological considerations rendering the technique inappropriate. Models have been developed which permit calculations to account for the morphological differences of non-planar samples at a wide range of sample dimensions.74 Employing a twin energy X-ray source (for example Mg and Al) is one method by which to overcome some limitations regarding experimental conditions, since different energy X-rays may analyse the same region of the same sample but result in photoelectrons with different mean free paths and thus provide more information on the overlayer structures.32 This method has been shown to be in good agreement with electron microscopy for Ag@SiO2 core shells over a small size range.32
The overlayer analysis methods described so far have the major advantage of being non-destructive and the superb depth resolution (in the order of Angstroms) of such non-destructive methods makes them ideal for analysing small particles (<20 nm for energy sources below 5 keV) thin films, overlayers or inhomogeneous samples.75 However, all these techniques are limited by the maximum kinetic energy of the chosen X-ray source (typically around the order of 10 nm for a 1486.69 eV Al kα X-ray source). To address this limitation, a range of high energy laboratory X-ray sources such as the liquid gallium jet (9.25 keV),76 are being developed which complement the variable energy photons offered by synchrotrons (up to 18 keV)75,77,78 and facilitate examination of more deeply lying interfaces. An additional limitation when dealing with HAXPES is the decreased sensitivity due to diminishing photoionisation cross-sections at higher excitation energies79–81 (thus also requiring higher powered sources which has the potential to lead to greater X-ray induced sample damage). One result of this is the need for high energy analysers with a large angle of acceptance, creating a further barrier in the development of HAXPES systems.82,83 Nevertheless there will always be an upper limit to the depth that non-destructive XPS methods can probe.
Depth profiling
An alternative method for exploring composition gradients in the Z-dimension by XPS is to use a destructive bombardment technique. This typically involves an ion beam (most commonly Ar+) in which accelerating voltage, current and raster size may be tuned in order to control the removal of surface atoms via collisions with the ions.84,85 Obtaining snapshot spectra at regular intervals during the etching process (at which point the ion beam is turned off) permits the construction of a depth profile in which elemental or chemical state may be charted as a function of total sputter time (Fig. 3); converting the data to a depth profile requires an estimate of the etching rate for the material under study. The major drawbacks in ion bombardment depth profiling are a reduced depth resolution due to a mixture of non-uniform sputtering (e.g. cratering),86 preferential sputtering (e.g. oxygen in metal oxides),87 chemical reduction during sputtering88 and the need to calibrate etching time with depth.84
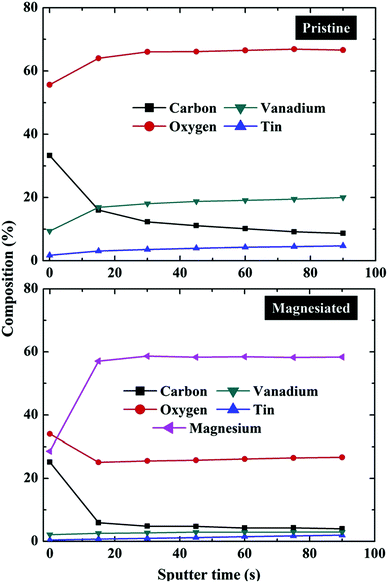 |
| Fig. 3 XPS depth profile composition measurements of pristine and magnesiated V2O5 cathodes indicating the relative contents of carbon, oxygen, vanadium, tin and magnesium as a function of overall ion bombardment time (in itself a function of depth from the surface of the sample down into the bulk).89 | |
Until recently another major issue with ion bombardment sputtering was that it was limited when applied to organic materials, since ion etching of polymers results in significant chemical degradation.90 However, the advent of gas cluster ion sources/beams (GCIS/GCIB) in which ionised multi-atomic clusters are used in place of single ions has led to more stable etch craters due to multiple secondary collision events91 and exhibit minimal damage for organic molecules, enabling z-dimension profiling and 3D imaging for the vast majority of organic materials.92 Depth resolutions as low as 7 nm have been recorded and may be readily improved through the utilisation of a rotating sample stage and a decrease in X-ray flux, since exposure to X-rays can also degrade the steadiness of the etching process.93 Further improvements to depth resolution may come from combining ion bombardment with a technique such as TOF-SIMS.94
Advanced sample handling for lab-based XPS systems
While not necessarily an individual technique, the development of technology designed to overcome the imitations of analysing samples within a UHV system has widened the reach of XPS as a tool for probing materials. The advent of near-ambient pressure (NAP)-XPS95 is one pertinent example of the science adapting to accommodate samples previously difficult or even impossible to analyse – though this has historically required specialist instrumentation often only found at a synchrotron. Recent years have seen a marked rise in the number of lab-based NAP-XPS systems,96 with manufacturers such as Omicron Scienta and SPECS offering bespoke and ‘off-the-shelf’ instruments capable of performing ambient pressure measurements. This review will, however, focus on techniques by which we can use traditional lab-based XPS systems to probe air-sensitive materials or perform in situ experiments. For a more detailed discussion regarding the use and application of NAP-XPS to materials analysis, several reviews and articles are available for the reader to explore.24,95–100
Studying electrochemical systems using XPS
One discipline in which such an approach has had great impact is the field of electrochemistry which is currently of great research significance due to its role in the energy sector where electrocatalysis represents a viable solution to hydrogen production101 and electrode materials to that of energy storage.102 It is generally accepted that an improved understanding of the underlying mechanisms that occur at the interface between the electrolyte and the electrode, and any restructuring of the electrode surface during polarisation, activation and deactivation is extremely important.103Ex situ and in situ XPS studies of electrode materials,104 ionic liquids and electrochemical processes including electrochemical double layer (EDL) studies have been employed to provide insights into such mechanisms.
The surface sensitivity of XPS and its UHV requirements make it quite complicated to apply to the electrode/electrolyte interface; liquid electrolytes are obviously not compatible with vacuum conditions, making true in situ measurements difficult.
Ex situ electrochemical XPS refers to investigations where the material studied is removed from the original electrochemical cell and transferred to the vacuum chamber, usually this would involve disassembly in a glovebox and transfer using a suitable inert transfer device (Fig. 4). Such transfer modules may involve either the use of a vacuum sealed system (Fig. 4a–c), in which samples may be mounted inside a glovebox and the transfer module evacuated within the antechamber, where a check valve permits evacuation inside the cell and holds the vacuum until loading inside the instrument. Alternatively, through the use of a sealed manipulator, gate valve and standard KF flange, samples may be held under vacuum or inert gas, transferred from glovebox to instrument and the KF flange directly mounted onto the vacuum chamber, permitting the sample to be moved into the instrument after the gate valve is opened. Such measurements always introduce the possibility that the surfaces may be different from their original states but valuable electrochemical information can still be obtained following such disassembly as demonstrated by XPS studies of the oxygen evolution reaction (OER) on electrodes.105
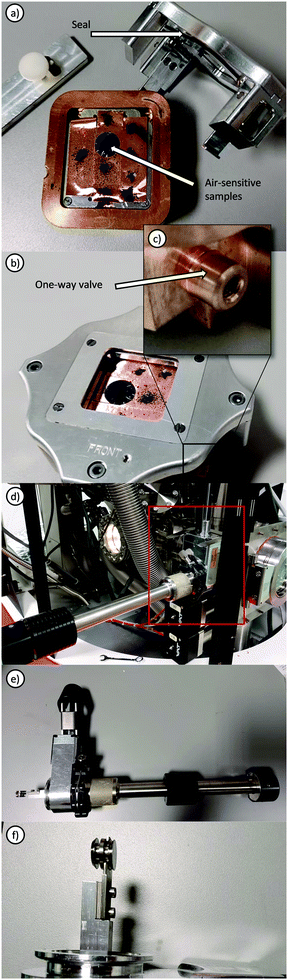 |
| Fig. 4 Typical inert sample handling devices. (a–c) Sample transfer under vacuum from Thermo ScientificTM. (a) Disassembled transfer unit, (b) assembled transfer unit and (c) check valve to hold internal vacuum – (d–f) sample transfer under vacuum or inert gas from Kratos Analytical. (d) Transfer arm attached to UHV chamber, (e) transfer arm removed from UHV chamber and (f) sample stub held on manipulator probe. | |
A variety of methods have been developed to overcome issues with the ex situ approach including elaborate transfer systems to minimise exposure to atmosphere,106 and the electrode immersion technique.107–109 The latter, attempts to retain the original conditions of the samples from the electrochemical cell whilst in the XP spectrometer. Pioneering work by Kolb et al.110 showed that it was possible to remove electrodes from the electrolyte during potential control and keep the electrical double layer (EDL) intact. Gold electrodes were immersed from various electrolytes prior to performing XPS analysis, which was used to determine the surface excesses and adsorption isotherms for a number of ions and metal deposits. Later, Hecht et al.111 examined the EDL on Ag in alkaline NaCl after immersion with various potentials, and studied the concentrations of the cations (Na+) and anions (Cl− and OH−) adsorbed on the silver electrode via quantitative XPS analysis. Their analysis revealed that the anions tend to bind Na+ into the double layer for charge compensation, and that roughly one monolayer of water was found for all potentials studied, in agreement with classical double layer studies.
Ex situ electrochemical measurements
The importance of the surface sensitivity and chemical state information available from XPS is demonstrated well by recent studies carried out by Andreu et al.104 into lithium ion batteries. The research involved modification of the positive electrode material, a mixed transition metal oxide consisting of LiNixMnxCo1−2xO2 where (0.33 ≤ x ≤ 0.5)112–116 to improve its electrochemical performance. It has been shown that coating the electrode with a metal oxide coating such as Al2O3 or ZrO2 can improve the electrochemical performance at high voltages.117–119 Investigations into how the transition metal nature and Al2O3 coating on LiCoO2 and LiNi1/3Mn1/3Co1/3O2 (NMC) surfaces affect performance were carried out by Andreu et al.104 studying the adsorption of SO2 on the surface with XPS. The resulting spectra showed only one adsorption mode present for the LiCoO2 surface, signified by the sulfate species (Fig. 5a and a′) whereas the NMC electrode material exhibited both sulfite and sulfate species (Fig. 5b and b′). In the case of the LiCoO2 electrode, the coating modifies the surface reactivity, indicated by the appearance of both sulfite and sulfate species, and the authors attribute this to Al/Co substitution shifting the adsorption mode from redox to acid–base. In the case of the NMC electrode, a decrease of sulfite species is seen at the surface, a phenomenon that can be related to the cation mixing effect in the NMC.120
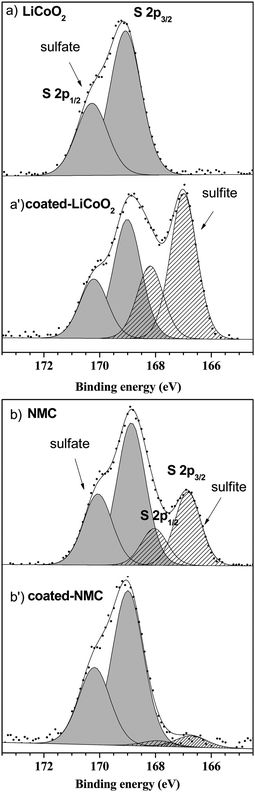 |
| Fig. 5 XPS spectra for the S 2p core peaks after SO2 adsorption on (a) LiCoO2 and (a′) Al2O3 coated LiCoO2 and XPS spectra for the S 2p core peaks after SO2 adsorption on (b) NMC and (b′) Al2O3 coated NMC. Reprinted (adapted) with permission from ref. 104. Copyright {2015} American Chemical Society. | |
XPS depth profiling has also been important in research into alternative energy storage with pseudocapacitors, which are based on redox reactions of electroactive materials, have a longer life than batteries and a higher energy density than conventional capacitors.121
A study of the pseudocapacitive performance of an Mn oxide electrode in the ionic liquid (IL) EMI-SCN was carried out by Chang et al.122 ILs are composed solely of ions and exhibit several desirable properties including high electrical conductivity (up to 100 mS cm−1), a wide electrochemical window (up to 5.8 V), and extended liquid-state temperature range (ca. 173–523 K).123 Furthermore, their negligible vapour pressure overcomes the issue of incompatible liquid electrolytes in UHV systems. In the case of EMI-SCN, the cation (EMI+) and the anion (SCN−) both contain nitrogen atoms and so can be conveniently compared using the N 1s region of the XP spectrum, (Fig. 6). Chang et al. were able to identify the working species in the pseudocapacitor as the SCN− anions by recording sequential N 1s spectra of the Mn oxide electrodes in EMI-SCN during argon ion etching; 10 spectra recorded with the electrode under a potential of −0.3 V are shown superimposed in Fig. 6. The peak at 398.7 eV (due to the EMI+ cation) disappears after only one Ar+ sputtering cycle, indicating that it is only adsorbed on the surface signal whereas the at 401.6 eV (due to the SCN− anion) only gradually decreases with depth, indicating that it has penetrated into the Mn oxide surface.
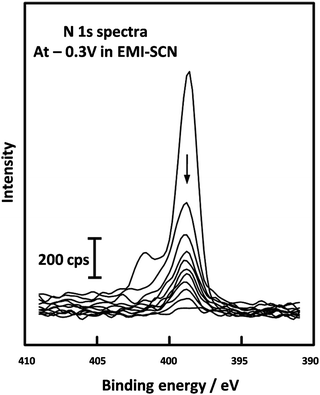 |
| Fig. 6 XPS depth profiling spectra of the N 1s level from Mn oxide electrodes in EMI-SCN, [C2C1Im][SCN] polarised at −0.3 V, reported by Chang et al. Reprinted (adapted) with permission from ref. 122. Copyright {2009} American Chemical Society. | |
In situ electrochemical cells
Despite advances brought by ex situ characterisation, post-analysis can mean that some metastable species are not analysed since they are only present under reaction conditions,124 therefore in situ, or in operando, analysis is of great value. An in situ XPS cell reported by Weingarth et al.125 demonstrated that XPS analysis can be performed simultaneously whilst carrying out electrochemical measurements, to determine the direct electrochemical shift as a function of applied potential. The system studied was a three-electrode set up involving a counter electrode (CE) and reference electrode (RE) prepared of activated carbon, and a Pt working electrode (WE) in contact with the IL electrolyte [EMIM][BF4]. XPS analysis was carried out at the interphase boundary between the IL and Pt WE, with selected associated spectra in Fig. 7. The binding energies (BE) for the electrolyte species core level spectra (B 1s, C 1s, N 1s) exhibited an electrochemical shift of −1.0 eV V−1 under the applied voltage because these atoms are in the outer Helmholtz plane (OHP) or the bulk of the electrolyte, and due to a lack of direct contact with the electrode, subsequently experience the full potential drop. The WE was grounded by the XPS system and therefore the Pt 4f BE and peak shape did not change. N 1s spectra were also recorded at very negative potentials, where the appearance of an extra peak at a potential of −1.9 V indicated a degradation product of an electrochemical reaction. Further examples of in situ UHV XPS cells include those of Wibowo et al.126 and Gokturk et al.127,128
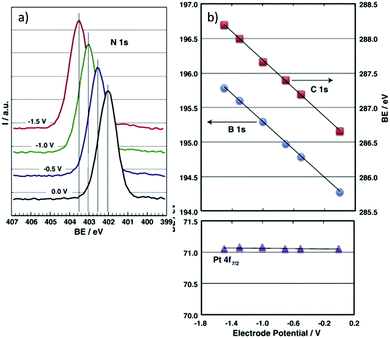 |
| Fig. 7
In situ electrochemical XPS measurements as reported by Weingarth et al.125 for their three electrode in situ XPS cell, performed using a VG ESCALAB 220iXL spectrometer equipped with a monochromatic Al Kα X-ray source. (a) The N 1s binding energy shift under control of the working electrode (polycrystalline platinum foil) potential (as indicated); (b) the dependence of the binding energies of the B 1s, C 1s and Pt 4f7/2 core levels on the applied electrode potential. The system used a [EMIM][BF4] ionic liquid electrolyte. | |
Developments in XPS techniques that use synchrotron-based radiation sources as well as lab-based differentially pumped analysers and advanced electrostatic lenses now mean that it is possible to make in situ electrochemical XPS measurements at near ambient pressure (NAP-XPS), where NAP refers to a working pressure of up to a few millibars.129 For now, these remain on the cusp of lab-based surface analysis, but work from multiple independent groups are bringing such cells closer to widespread use.130–134In situ electrochemical XPS studies offer huge potential in electrochemistry research and further developments are expected to be made as the field progresses.
Cryo-XPS
Cryo-XPS employs a similar approach to the cryo-TEM technique that won Dubochet, Frank and Henderson the 2017 Nobel prize for chemistry, and enables the characterisation of solid–liquid interfaces and volatiles. Samples are prepared as suspensions which are supported on a holder, fast frozen and introduced into the vacuum chamber. Usually, the sample is warmed slightly to a stage where multilayers of frozen volatiles sublime and a virtually unaltered liquid monolayer interface can be studied.
Cryo-XPS provides several clear advantages over conventional preparation by avoiding steps such as freeze drying which can damage the surfaces of more sensitive samples while providing ostensibly in situ measurements.136,137 Furthermore, the presence of a hydrated layer also reduces levels of adventitious carbon because these carbon contaminants tend to be trapped in the first few layers, which are lost during sublimation.138,139 Key considerations are the condensation of volatile species from the laboratory atmosphere onto the surface of the cooled sample and potential issues with sample warming on transfer between cooling, transfer and analysis stages. Ideally then an XPS system suitable for cryogenic analysis would have a glove box attached directly to the load dock allowing for sample preparation in an inert atmosphere140,141 and LN2 cooling in both the load dock and analysis chamber, ensuring the sample is maintained at a low temperature during analysis.
Schukarev et al. have carried out several investigations probing water–mineral interfaces using the cryo-XPS approach, delineating the interaction between hydrated surfaces and the chemical speciation of absorbates.142,143 Pastes of metal oxides were prepared through the centrifugation of particles suspended in different pH environments. The wet pastes are then placed onto precooled transfer rods at 170 °C and 1 × 10−8 torr before introduction to the analysis chamber.144 The zwitterionic nature of N-(phosphonomethyl)glycine (PMG) absorbed on goethite and manganite was studied using this method and provided information that would not have been possible for traditional XPS analysis. Fig. 8a), shows the stark difference between the N 1s regions recorded at cryogenic and room temperatures, with the absence of the ∼402 eV peak due to a protonated nitrogen species at room temperature.135,145Fig. 8b demonstrates the sensitivity of the approach to pH changes in the flash frozen solution with dramatic changes to the relative ratios of NH2+ and NH at 402 eV and 399.8 eV respectively with varying pH. The 402 eV decreases in intensity as pH increases. The experiment clearly demonstrates the potential for direct monitoring of the changes to the chemical speciation as a function of pH, which could not be extracted from a dry surface.
 |
| Fig. 8 (a) N 1s spectrum of PMG recorded on a MnOOH surface at room temperature versus fast frozen, evidencing an addition nitrogen species following preparation via cryo-transfer. (b) N 1s spectrum of PMG on MnOOH at pH 3.9, 4.9, 6.0, 7.9 and 8.6 (top to bottom spectral features at around 402 eV). Here we can see the pH dependence on the amount of protonated PMG species on the surface of MnOOH. Copyright © 2004 John Wiley & Sons, Ltd.135 | |
Similar studies have been carried out on sulfur rich systems such as pyrites and sphalerite, which until recently could not be accurately characterised in a UHV system due to the loss of sulfur species in vacuum.146,147 Ejtemaei et al.148 combined cryo-XPS and depth profiling to study the activation of metal sulfides through the presence of Cu. Polished mineral samples were submerged in a copper sulfate solution for 10 minutes before being precooled to below −160 °C and maintained at that temperature for analysis by XPS. The samples were then etched 1–10 nm by argon ion bombardment. Survey scans of copper activated sphalerite (ZnS) showed copper ions present up to 10 nm deep (Fig. 9). Furthermore, the Cu and S 2p spectra show a greater proportion of Cu+ and S− compared to Cu2+ and S2− species present on the surface following copper oxidation.
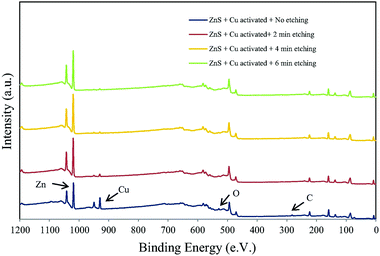 |
| Fig. 9 Survey spectra of copper activated sphalerite after; no etching, 2 minutes, 4 minutes, and 6 minutes of etching corresponding to a depth of 3, 6, 10 nm respectively Reprinted from Ejtemaei et al., Characterisation of sphalerite and pyrite surfaces activated by copper sulfate, Miner. Eng., 2017, 100, 223–232, Copyright (2007), with permission from Elsevier.148 | |
These findings were interpretated as evidence for a 2 step mechanism in the copper activation of sphalerite involving: Step 1: Cu ion exchange, Step 2: oxidation of the surface forming a CuS layer.
The study of soft matter including biological samples such as bacteria, is an emerging field within cryo-XPS. Fast freezing of cell walls can reduce the alterations and degradation caused by dehydration and beam damage, allowing for a more natural environment for analysis.149 The preparation of biological matter is comparable to that of inorganic media with suspensions of cells inoculated and subsequently pelleted onto a sample holder and fast frozen.150–152
Ramstedt et al.149 have used Cryo-XPS to study bacterial cell walls treated in different environments, using a multivariate curve resolution analysis of carbon spectra to analyse the lipid, protein, and sugar content of the bacteria cell surface layers. The work demonstrates the capability to reveal changes in the cell wall chemistry depending on the solution pH,150 with the chemical composition of Bacillus subtilis being radically different at low pH values compared to high pH, with much higher proportions of protein compared to lipids and carbohydrates. Bacillus subtilis tend to survive in environments between 6–9 pH and when exposed to more acidic environments the bacteria form a ‘spore coat’ which is higher in protein and low in sugars and lipids. Recently Shchukarev et al.153 applied this method to successfully characterise the surface layers of both fungi and viruses, demonstrating that the method can be applied to a large range of biological systems, and illuminating mechanisms such as absorption and antimicrobial interactions.
It is interesting to consider the similarities and differences between cryo-XPS and near ambient pressure XPS, which both offer opportunities to examine surfaces in the presence of high concentrations of adsorbates, albeit in very different physical states. Kjærvik et al.154 used the two techniques in a characterisation of the surface of Pseudomonas fluorescens. Fig. 10 shows that both methods give spectra with at least three clearly defined C 1s species at the surface of the bacteria and very little disparity can be immediately discerned between the two sets of data. However, the scattering of photoelectrons by gas phase components in NAP produces much lower signal intensities, and as a result additional S 2p and N 1s species were identified in the cryo-XPS experiments (not shown). Furthermore, there is a slight but significant increase in the 285.0 eV peak of the NAP spectra over that of the cryo-XPS. The authors propose that this difference arises from the presence of adventitious carbon on the surface of the NAP sample which was not present in its cryogenic counterpart because of the sublimation of the condensed water layers.
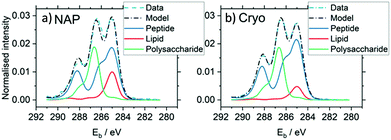 |
| Fig. 10 A comparison of C 1s regions obtained by NAP and cryo-XPS evidencing the benefits cryo-XPS may bring to the analysis of materials in a traditional UHV atmosphere. These graphs show the application of multivariate curve resolution analysis to the spectra.154 | |
Ion scattering spectroscopy
Ion scattering spectroscopy (ISS), also known as low energy ion scattering (LEIS), is a surface technique which involves probing the outermost surface layers of a solid using elastic collisions of ions. While ISS is generally be used to provide surface identities and ratios, it may also be used in a semi-quantitative manner through comparison with standard samples.155 Originally involving high energy (MeV) ions (and often referred to as Rutherford Backscattering Spectroscopy or RBS),156 the ISS technique was refined to utilise lower energy ions to be more readily available in laboratories.157–159 It was the discovery that ion-surface collisions could be described kinematically by a simple two body elastic collision model that allowed for facile determination of surface composition: | 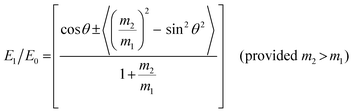 | (2) |
The dependence of peak energy position on atomic mass in ISS. E0 = primary ion Ek, E1 = primary ion Ek following a scattering process at θ° to initial trajectory, m1 = mass of primary ion, m2 = mass of target atom.
The extreme surface sensitivity of ISS allows it to offer additional structural information, complementary to that of pure XPS analysis and it has found use in many fields including catalysis,160–194 thin films,195–202 electronics195,203–206 and sensor materials.191,200–202,207,208 While ISS remains a valuable tool in materials analysis, care needs to be taken over sample choice and preparation. For example, ‘rough’ surfaces such as silicas commonly used in heterogeneous catalysis may exhibit broadened peaks in eventual ISS profiles.209 To overcome this, compacting samples into pellets may improve surface suitability, however, care must be taken so as not to alter the material properties.210 Furthermore, ion scattering may have limitations depending on sample composition, since preferential sputtering can lead to erroneously low concentrations of easily sputtered components such as sodium.211
Morphological analysis
Owing to its enhanced surface sensitivity in comparison to XPS, which has an information depth of as much as 9–10 nm (using a typical Al kα X-ray source),212 ISS has found much use in extracting qualitative information on elemental distributions in the very uppermost atomic layers of a sample. The ability to probe only a single monolayer allows for a more sophisticated analysis of the atomic arrangement at the surface and near surface.
This information is of critical concern in fields such as heterogeneous catalysis, wherein performance and activity are directed by surface interactions of reactants onto active sites. Ceria based mixed oxides for example, possess unique surface properties which render them highly useful in a variety of applications. Surface segregation of either dopant or ceria, determined by ISS, has enabled a deeper understanding of how different thermal treatments or synthesis methods affect the resultant materials properties as catalysts for CO/alkane oxidations164,180,181,213 and dehydrogenation/hydrogen transfer214 as well as for oxygen ion conductors/oxygen storage.181,215 Furthermore, since this is in effect an ion bombardment technique, the correct experimental parameters may induce sputtering of the surface of a material (even at low accelerating voltages), successive ISS spectra may therefore reveal an elemental gradient, affording further information which with knowledge of sputtering yields (or in comparison with alternative methods of probing the depth distribution of the outer surface) may even be used to prepare an elemental depth profile (Fig. 11).215,216
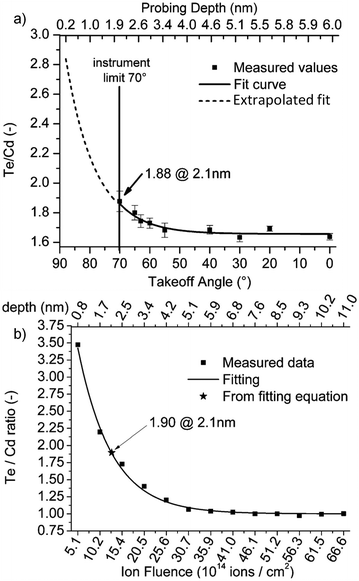 |
| Fig. 11 (a) Te : Cd ratio from a sequence of ARXPS measurements compared with (b) a profile of sequential 5 keV Ne+ ISS measurements as a comparison probe of the two methods efficacy in the analysis of ultra-thin films.216 | |
Thin film development requires methodologies to determine surface coverage and monolayer build-up. ISS is uniquely suited to measuring a relatively large field area to probe full thin film coverage or detect remaining substrate signal.177 For example, TiCrN thin films for drill coatings, developed using DC magnetron sputtering exhibit different properties when manufactured at different pulse frequencies. Analysis by XPS and ISS enabled the link between surface construction (and in particular, surface segregation of different species) and material properties to be determined.198 Surface morphology probed by ISS may be advantageous, compared with purely XPS methodologies such as Tougaard's inelastic background modelling since it is free from any contributions from subsurface species (for example, underlying pores containing materials of competing photoemission). The caveat, however, is that multiple samples/measurements are often required for meaningful representation.
Structural changes following reaction
ISS may also be used to determine changes to the outermost surface following chemical or physical processing and has been used to study systems undergoing many common processes such as thermal treatments,217–219 hydrogen reduction161,220 or exposure to gases or vapours such as O2 and H2O.221
ISS has also been used to study sintering, an important consideration in the field of catalysis, both in terms of the stability and reusability of the catalytic materials but also in the preparation of the materials. Vapour deposition,222 wet impregnation223 and even cluster deposition224 are often followed by a thermal treatment step to nucleate particles or remove organics, counterions or capping agents. Changes to surface coverage may give an insight into sintering processes which are difficult to observe by alternative means such as XRD or STM.170 This principle applies to monitoring synthesis procedures to optimise conditions. For example, determining the content of transition metal sites within a PtCo electrocatalyst/polymer electrolyte membrane fuel cell. Marked performance changes are observed depending on the number of transition metal sites within the surface layer. Thermal processing and ISS/XPS was used to optimise preparation methods and monitor surface properties in combination with catalytic studies.217
ISS is also a viable method for monitoring the surface of a system during exposure to working conditions. For example, alloy oxidation is a key parameter to optimal material properties, ISS/XPS confirmed a Grimley-modified Cabrera–Mott model for the oxidation of a multitude of mixed metal oxides during plasma oxidation, as well as using ISS etching to profile the distribution of elements.205 Similarly, in catalysis, Ag foils exposed to NO/O2 mixtures to model the catalytic process of NOx removal showed structural reorganisation, forming oxidic and nitric sandwich layers.193 Pt based automotive three-way catalysts were studied by ISS to monitor coking under different conditions the enhanced surface sensitivity of ISS in comparison to XPS enabling the analysis of catalysts with active component loadings below the XPS limit of ∼0.5 wt%.167
Temperature dependant ISS (TD-ISS)
ISS has found use in several sectors in conjunction with variable heating stages in order to utilise the unique information available to ISS as a probe for surface changes resulting from an increase in system temperature.
In one example of this approach, size selective Ir clusters deposited onto TiO2 surfaces for model catalytic studies made use of a very simplistic form of TD-ISS in which model surfaces were analysed by XPS and ISS before and after a the temperature programmed desorption (TPD) of CO in order to assess surface restructuring.160 It was observed that following a TPD procedure, the surface Ir intensity (ISS) greatly diminished.
While XP data indicated that Ir desorption was not the likely cause of this attenuation, some attenuation suggested that perhaps the composite was restructuring either via sintering or encapsulation of the Ir centres. A second TPD was performed and revealed markedly differing behaviour, which combined with the ISS and XPS data suggested the Ir centres were undergoing encapsulation by the reducible support (a strong-metal support interaction which has been observed for a number of metal–titania systems).166,225,226
Monitoring dynamic changes to ISS spectra as an in situ method of determining temperature-structure relationships, rather than an ex situ pre- and post-process snapshot, can offer even greater insights. For example, following exposure to CO, small (Pd2) and large (Pd20) clusters on TiO2 were analysed by ISS whilst heating from 100 K to 500 K (Fig. 12).
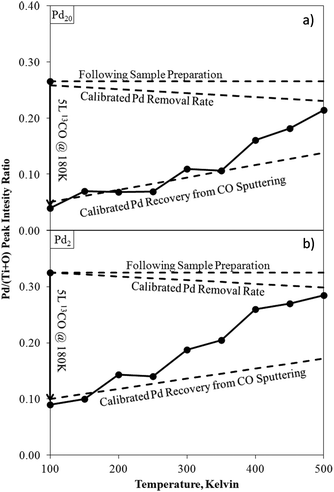 |
| Fig. 12 Pd/(Ti + O) ISS intensity ratios for Pd20/TiO2 (a) and Pd2/TiO2 (b) as-deposited, after exposure to CO at 180 K, and during temperature ramping. Calibrated rates for recovery of Pd from ISS sputtering.171 Calibration lines account for unintended sputtering effects from the ISS experimental conditions selected for this analysis. Deviations from this calibration indicate a changing surface structure (desorption of CO). | |
Comparisons of these changing ISS ratios with CO TPDs led to the conclusion that low temperature CO desorption in the TPD was due to CO adsorbed onto Pd–TiO2 peripheral sites, rather than directly on-top of Pd clusters. A similar experimental procedure has been used to study other Pd cluster/metal oxide systems172,173 as well as the relationships between Pt cluster sizes and ethylene227 and diborane228 surface reactions.
Modelling growth modes
When considering nanoparticle or thin-film growth over a substrate (of non-matching identities), knowledge of nucleation and growth modes permits sophisticated and precise control over nanomaterial synthesis. ISS analysis combined with XPS affords a simple methodology for modelling the growth based on the calculated surface coverage or peak intensity relative ratios.189 It is important to note, that the related higher energy technique, RBS, provides beneficial complementary here in that it may probe film thicknesses up to ∼200 nm due to the deeper probing depth compared with ISS.229 The efficacy of RBS, however, diminishes with smaller overlayer thicknesses (<3 nm) however, and as such ISS is much more suited for applications such as modelling growth modes on the atomic scale.
For example, surface coverages and XPS peak ratios enabled González-Elipe et al. to study a range of metal oxide–metal oxide interfaces, model the growth of the different oxide combinations and relate said models to binding energy and modified Auger parameter effects in the XPS spectra.200–202,230 ISS was also used to shed light on the nucleation and growth of iron oxides on Au(111), in which the rate of change of surface coverage with calculated monolayer coverage provided insights into a change in growth mode at 0.5 ML from layer-by-layer to island growth.196 STM was used alongside the XPS and ISS to confirm these observations.
More complex systems, involving mixed oxide interfacial layers of multiple metal site systems may make use of the combined power of ISS analysis and subsequent modelling, with the chemical information gleaned from XPS (particularly angle resolved XPS) to construct sophisticated models of changing interfacial species and growth modes. When modelling the deposition of titanium onto Si(100) Palacio and Arranz measured the Ti and Si ISS signals at regular time intervals during the deposition process and used them to rule out Volmer–Weber growth during the initial stages.195 Combining ISS with XPS, they were also able to determine that the process involved a period of titanium silicate growth (up to 4 ML) followed by Stranski–Krastanov growth of metallic Ti.
Similarly, Liu et al. analysed platinum deposition on nickel using ISS and XPS (plus the application of overlayer models to the XPS data) to develop a model for the alloying and growth of the adsorbate layer.231
Cu/CeO2 catalysts are widely used for oxidations, water-gas shift and methanol synthesis. Measuring the ISS spectra of a range of depositions of discrete amounts of Cu onto a Ce(111) surface and normalising the signal to that of bulk (>40 ML) Cu/CeO2 resulted in the profiles observed in Fig. 13.169 Theoretical profiles for layer-by-layer (Frank–van der Merwe) and hemispherical cap growth (island growth of uniform hemispherical particles)232 are superimposed onto the measured datapoints to illustrate the correct growth formation. Combination of the surface coverage from ISS with the XPS data and knowledge of the growth mode (and parameters such as IMFP) allows for high sophisticated calculations of particle thickness/radius.232
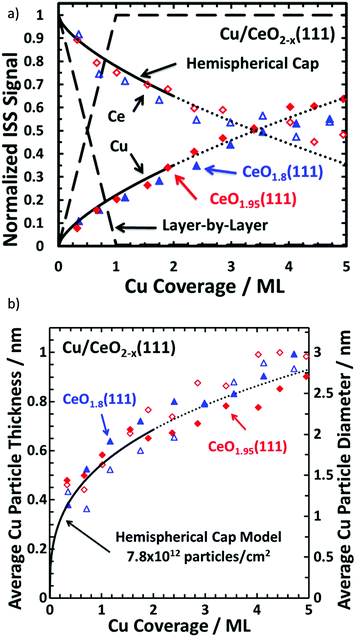 |
| Fig. 13 (a) Normalised ISS intensities for Cu on CeO2(111) with model growth modes for comparison and (b) average Cu thickness versus Cu surface coverage. The dashed line represents the normalised ISS signal to be observed if Cu grew in a layer-by-layer fashion, whereas the solid line represents Cu growing as hemispherical caps with a fixed radius and a fixed particle density of 7.8 × 1012 particles per cm2. This model is only reasonable up to ∼35% surface coverage (above this, potential particulate overlaps render the model unreliable) and the dotted lines are purely for visualisation. Reprinted (adapted) with permission from ref. 169. Copyright {2015} American Chemical Society169 | |
Measuring surface potential
Ion-induced secondary electron (IISE) emission is an important material property to be considered in the production of coatings for scientific equipment, such as non-evaporable getters for distributed pumping at CERN.233 ISS has been used as an effective probe for determining the surface potential of thin films under ion irradiation. Nagatomi et al. derived an experimental approach for the study of MgO films on silica (at the time an important material composite for plasma screen displays) in which irradiation of the surface with 950 eV He+ ions with an electron flood gun was used to determine the resulting surface potential.234,235
Ultraviolet photoelectron spectroscopy (UPS)
The process giving rise to UPS is in essence identical to that of XPS. The only difference is the energy of the incoming photons and thus the source of the photoelectrons that are emitted. Typically, UPS is performed using a He(I) or He(II) lamp emissions (21.22 and 40.8 eV respectively), relating to 1s 2p → 1s2 relaxations from He atoms (He(I)) and ions (He(II)).236 These lower energy sources limits this technique to the analysis of valence shell electrons and molecular orbitals, or density-of-states (DOS) structure, and also results in a shallower sampling depth (due to the reduced kinetic energy of emitted photoelectrons). The technique has the potential to provide powerful spectroscopic representation of molecular orbitals and can reveal information on their bonding or antibonding character, how the bonding is localised in a molecule and also changes to molecular geometry.236–238 It is particularly powerful in the study of molecules in the gas phase where multiple emission states may arise as a result of vibrational lines on top of purely energetic transitions but UPS is also a powerful probe of solid and composite materials, occasionally as a simple fingerprint technique or through more detailed understanding of the emissions. UPS has found use in the analysis of polymers,239,240 self-assembled monolayers (SAMs),241 catalysts242–244 and semiconductors/electronic materials.245,246
Valence band structure
A simple application of UPS is as a tool to fingerprint valence structure to obtain information either not available using X-rays or to glean information from closer to the surface. In the case of metal–semi/non-conductor systems (prevalent in the field of catalysis), emissions near the Fermi energy allow monitoring of the presence of metallic bonding. For example, Roberts et al. used the presence of photoemission intensity near EF to discern the amount of metallic bonding in the deposition of size selective clusters of Pd on TiO2 and correlate high catalytic activity with higher Pd 4d occupancy (vs. Pd 5s).247 Spectral intensity (or lack thereof) around the Fermi energy of MoO3 based catalysts for alkane/ene cracking was used to monitor changes to the molybdenum centres following hydrogen reduction.161 Prior to reduction, no structure near the Fermi level could be observed, however following thermal treatment under H2 at 673 K, π and σ bands from MoO2 could be observed in the DOS as well as a clear emission around the Fermi energy. While the XPS did indeed show a marked reduction from MoO3 to a mixed MoO3–MoO2 system, no metallic Mo could be observed. The UPS however, indicated that the system was largely MoO2 with metallic sites present, suggesting these species may be found predominantly at the surface. UPS and XPS were further used to determine the degree of reduction following doping with Pt and K where it was revealed that K doping enables the full reduction of MoO3 to MoO2. Such utilisation of UPS is often hindered by strong overlapping signals in mixed or complex systems, however small windows in the spectra can provide critical insights in many structures.
Electronic structure
Possibly the most widely used application of UPS is the analysis of electronic states (for example in semiconductors). This requires slightly more detailed analysis and understanding of the photoemission in a system than the simple fingerprint approach.
Since UPS concerns itself with the energy region associated with valence orbitals, it is primarily analysing the electrons concerned when discussing DOS. XPS may also be used to record these of course, but with the penalty of reduced resolution due to the greater line width of the exciting photons sources.248 While not often a requirement for pure metals, the enhanced resolution allows for more detailed analysis of composite materials where determination of orbital characteristics in the DOS may be more complicated.249
There are a number of terms which are vital to understanding molecular electronics including, but not limited to: the vacuum level (EVAC – the energy level at which an electron is at rest a few nm outside of the atom), the Fermi energy (EF – in metals this is the boundary between occupied and unoccupied states in the continuum, in semiconductors this is a statistical state within the band gap) and work function (the energy required to take an electron from EF to EVAC).250 Understanding the work function is key to improving our knowledge of semiconductors and developing improved materials. While it is possible to measure work function using XPS, UPS is traditionally favoured due to its superior line width and flux.251
Sample work function may be determined using the spectral width (this is the energy difference between the secondary electron cut-off and the Fermi energy),252 though while this process may be simple for conductors, it is less straightforward for semiconductors because EF lies within the band gap. Analysis of the high kinetic energy edge permits determination of the Fermi edge of a material, while the low kinetic energy edge provides the position of the secondary electron cut-off. Sample biases may also be applied in order to decouple emissions arising from secondary electrons associated with the spectrometer.253 It should also be noted, that while this review does not intend to dive deeply into (N)AP-XPS, powerful methodologies have been determined for the determination of material work functions through the analysis of gas phase molecules in the vicinity of the material surface.254 While this analysis requires the use of a (N)AP-XPS system – it serves as an additional probe and may be a powerful combinatorial approach with UPS, enabling the more facile determination of the valence band maximum (VBM) of semiconductors and assisting with the interpretation of the UP spectrum.
Using this data in combination with a band gap measurement (e.g. from UV-Vis), one may construct a full band energy diagram – including the positions of the Fermi level, conduction band minimum (CBM) and VBM.255–257
TiO2 represents a major class of semiconducting solids in which the understanding of the electronic structure of the pure material, as well as modified, doped and mixed oxide systems is important for the development of advanced functional materials in fields such as photocatalysis,258 solar cells259 and gas sensors.260
As a result it has been studied extensively using UPS with particular interest in the calculation of absolute values of the CBM and VBM when for example, Tauc-plots.261 generated from UV-Vis spectroscopy permits only relative values. Experimentally, recording UPS spectra from non- or semi-conducting samples represents a challenge due to a more demanding need for sample homogeneity and electrical contact with the system. Maheu et al. compared two preparation methods which have shown some promise as means of acquiring UPS spectra from granular semiconducting materials: electrophoretic deposition (EPD) and drop casting a suspension of TiO2 in alkanes.262 The objective was to study anatase, rutile and P-25 (a mixture of rutile and anatase often used as a benchmark material for photocatalytic applications due to it's beneficial electronic properties) in an effort to understand the nature of the unusually high photoactivity of P-25 when compared with other forms of titania.
While EPD prepared a sample in which electrical contact was sufficient to overcome any potential charging, drop casting required the determination of the absolute position of the CBM and VBM energies using the signal from the Ag foil substrate. Both methods were able to determine the EVB of anatase and rutile from which an energy level diagram and representation of DOS was developed (Fig. 14), correlating well with theoretical predictions.263
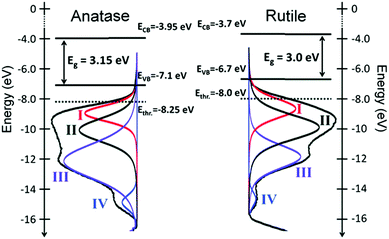 |
| Fig. 14 He(I) UPS profiles, fit with an established empirical model and an overlayed electronic structure of the two TiO2 polymorphs anatase and rutile relative to the vacuum level. Ethr. corresponds to the deepest state that can be excited in a photocatalytic test (Ephotons ≤ 4.3 eV).262 | |
The inclusion of heating stages in many modern spectrometers raises the possibility of combining procedures such as those demonstrated by Maheu et al. with annealing processes capable of producing oxygen defects in TiO2 with the ability to effectively neutralise charge build up and further augment the spectral acquisition process.264 Note this method makes use of a modified background developed by Li et al, designed and optimised for UPS spectra.265
Whilst the ability to calculate the work function of a material is vital to semiconductors and electronics,266 its also very important to chemical performance in many other areas including catalysis,267,268 photochemistry269,270 and chemical sensors.271,272 For example, work function changes were correlated with molecular dipole moment measurements of partially fluorinated alkyl and aromatic phosphonate SAMs on a Zn-terminated ZnO(0001) surface to create a tuneable ZnO work function that could be tailored to improve the efficiency of organic–inorganic electronic devices (Fig. 15).273
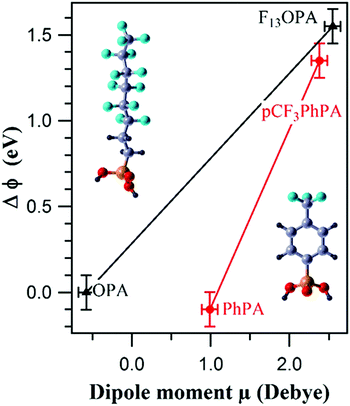 |
| Fig. 15 Correlation between the experimental change in work function (ΔΦ) and the molecular dipole moment μ of the PA molecules for 3,3,4,4,5,5,6,6,7,7,8,8,8-tridecafluorooctyl phosphonic acid-((F13)OPA, left molecule in figure) and p-(trifluoromethyl)phenylphosphonic acid-((pCF3)PhPA, right molecule in figure) modified ZnO surfaces, along with corresponding non-fluorinated analogues.273 | |
In some cases, work function measurements can also reveal insights into growth modes. Monitoring the work function of Ag deposited onto MgO for example, revealed a valley-like gradient which was ascribed to Ag atoms binding to surface defects and forming sites of nucleation for further growth.274
Organic semiconductors have gained popularity in the 21st century, wherein widely conjugated π-systems take the role of the solid system.275 AR-UPS has been able to evidence the electronic structure and charge carrier ability of such systems, though experimental methods are often not so straightforward, requiring organic single crystals which may need extra consideration for charge compensation and supplementary excitation sources.276,277 The result of the utilisation of AR-UPS, however, is that a picture of the electronic structure of the HOMO-derived valence band may be derived, which is fundamental to understanding the nature of positive charge carriers in organic semiconductors.277
Adsorbate analysis
UPS analysis of molecular adsorbates has for a long time been the preserve mainly of the heterogeneous catalysis community, but the advent of hybrid organic–inorganic systems has resulted in a much wider interest in the interaction between substrate and adsorbate as materials designed for electronics, sensors and optics benefit from an increased understanding of the bonding and interfaces involved.278
CO adsorption was an early model for this field, since metal–CO interactions are prevalent among a multitude of chemical catalytic transformations. CO adsorbed onto Pd(111) produced 2 of the three spectral emissions associated with the gas phase spectrum of CO (namely, the 1π and 5σ features).279 The 5σ is primarily situated on the carbon, the 1π on the oxygen and the 4σ an oxygen lone pair. Due to final-state screening even in the absence of any bonding280,281 adsorbate binding energies will decrease by 2–3 eV which led to the proposed model that the 1π and 5σ orbitals are non-bonding and the 4σ orbital increases in energy (due to the interaction with the metal) to the point where it is no longer observable on the UPS spectrum. This was observed to be true for a number of systems282 and led to the explanation that the CO 5σ orbital is responsible for the bonding of this system via the carbon atom, and donates electron density to the vacant d-orbitals of the metal and subsequent back-donation from filled d-orbitals is directed into unoccupied CO 2π* antibonding orbitals, a model which would come to be known as the Dewar–Chatt–Duncanson (DCD) model.
The 5σ peak can actually be found underneath the 1π peak using angle resolved UPS,283 while inverse photoemission spectroscopy was used to identify the partially filled 2π* antibonding orbital.284
UPS was also used to study the effect of potassium and caesium doping of Pt(111) (plus O2 preadsorption) on the adsorption of ethylene.285 Firstly, ethylene was adsorbed (π-bonded – mild disruption to double bond) on the surface of the crystal at 37 K so as to avoid any double-bond dissociation before heating to 57 and 300 K which resulted in the formation of a di-σ species (major disruption to the double bond followed by the formation of an ethylidyne molecule on the Pt surface. Multilayer formation at 37 K was observed in the presence of alkali-dopants, with minimal distortions to the adsorbed molecular orbitals, however the introduction of halides, resulted in a more weakly bound species with UPS revealing that with increasing alkali coverage there were fewer di-σ species formed at higher temperatures, to the point where π-ethylene is the only adsorbate (desorbing at 130 K).286,287
This analysis is not limited to observing changes in the adsorbate itself, however, as electronic changes induced by the process of adsorption may also produce simultaneous spectral features which provide insight into surface reaction mechanics. One example of this is measurement of the work function of a metal surface before and after adsorption.285–290 For a clean Pt(111) surface, ethylene adsorption reduces the work function (φ) by 1.05 eV, consistent with the adsorbate UPS and vibration spectroscopy measurements in observing a large charge transfer from ethylene to Pt(111) upon forming the di-σ species.287 Adsorption of potassium onto Pt(111) reduces φ by 4.8 eV, after which adsorption of ethylene induces very little change in φ, again consistent with the observation from UPS/TPD that alkali doping of Pt(111) inhibits σ-bonding of ethylene to the metal surface. In contrast, CO adsorbed onto Pt(111) increases the work function by 1.5 eV,291 consistent with metal to adsorbate charge transfer in the form of π-back bonding. The combination of UPS spectral features and substrate analysis, particularly when used with complementary techniques such as HREELS and TPD, has been used in this way to provide insight into a bounty of systems, studying the combination and reaction of free molecule and active site and facilitating a greater understanding of catalytic processes.292–297
Monolayer orientation
UPS has been used to reveal information regarding the orientation and organization of self-assembled alkyl chains adsorbed on to metal films, often in conjunction with XPS in order to probe monolayer thickness.298 Chain tilt angles for alkanethiols on metal facets (e.g. Ag(111) vs. Au(111)) differ significantly, and this difference was noted in the UPS spectra.299,300 It was observed that not only can UPS identify adsorbates in different orientations (e.g. standing vs. lying chains), but it can also identify them independently, unlike techniques such as Fourier transformed infrared (FT-IR) and near-edge X-ray absorption (NEXAFS) spectroscopies which report an average tilt-angle.301 Measurements recorded at differing take off angles (TOA) may also yield information regarding the degree of homogeneity in the orientation of the chains,302 where spectral features which do not change as a function of TOA are indicative of randomly oriented chains while TOA dependence suggests a good molecular orientation.303 Furthermore, the intensity of the Fermi edge as a function of TOA may yield additional insights into the order of the adsorbates.304 This technique has found use with more complex surface molecules, such as 2- and 4-chlorobenzylmercaptan, adsorbed on gold films. Identification of spectral features from model gas phase UPS spectra permitted the identification of both the ortho- and para-positions of the benzene ring as well as the orientation of the molecules, although this technique is often performed using a related technique called metastable induced emission spectroscopy (MIES), due to its superior surface sensitivity.305
Auger electron spectroscopy
Auger electron spectroscopy (AES) is a type of secondary electron emission spectroscopy, resulting from the bombardment of a surface by an electron beam. AES complements XPS and ISS through it's highly surface sensitive probing (∼5 atomic layers depending on energy) and is capable of extremely specific elemental identification.306 Furthermore, unlike ISS, it is capable of determining chemical or valence states in certain cases.307 In the case of carbon, for example, analysis of the KLL Auger peak (involving valence electrons) may provide more chemical information than even XPS.307
Auger emission is a widely known process and occurs as an inter-atomic response to the photoemission process caused from the irradiation by X-rays or electrons. Following emission of an electron, the atom is left in an ionised, energetically unfavourable state with a core-hole formed in a low-lying orbital. Relaxation for this state comes as an electron in a higher orbital may drop down to fill the core-hole, releasing excess energy which may then facilitate the emission of a secondary electron, the “Auger” electron, with a kinetic energy that is independent of the exciting radiation/electron.
Analysis of films and overlayers
The enhanced surface sensitivity of AES (when applied to lab-based sources) results in a lower window for film thickness analysis than for XPS for example, with its suitability limited to ultrathin layers only (though it may be possible to analyse even up to 100 Å in some cases depending on the element).308 Much as with XPS, this surface sensitivity may be used to produce depth profiles in combination with a destructive ion bombardment technique – though with highly surface sensitive measurements, care must be taken to avoid topological changes which can affect the resulting profile.309
An example of Auger spectroscopy being used in this way is for the determination of graphene layer thickness. Multilayer graphene thickness is commonly determined by Raman spectroscopy,310 however, there are major drawbacks with using Raman from complications arising from graphene on substrates that negatively affect the Raman signal (e.g. Ni311 and GaAs312). The use of AES avoids these issues. Importantly, it is also possible to determine the thickness or number of graphene layers from a single, non-destructive spectrum – providing an advantage over most XPS methods and may also determine crucial sample properties such as electronic coupling between graphene and substrate, observe structural defects and quantify dopants or chemical impurities.313
For adsorbates, monolayers and multilayers the analysis of AES peak maxima of substrate (IS) and adsorbate (Ia), can readily probe adsorbate growth modes and determine monolayer coverage even if sticking probability changes with surface coverage.314 Such a technique enables the calibration of monolayer coverage and subsequent calculation of a coefficient of attenuation, which may be used to determine the surface coverage of an unknown sample (e.g. carbon monolayer coverage on a spent catalyst).315 Furthermore, a simple graphical model of IS/Ia allows for the facile distinction between Volmer–Weber, Stranski–Krastanov and Frank–van der Merwe growth of films.316
Surface mapping
Since AES utilises an exciting beam of electrons, rather than X-rays, there exists a much finer spatial resolution on the area of illumination than for traditional XPS. This enhancement permits much more detailed surface imaging than XPS imaging, with image resolutions capable of reaching 10 s of nm, rather than 1000 s.317
Modern spectrometers often combine the traditional XPS configuration with a field emission gun and provide both AES map scanning and scanning emission microscopy into one unit.318 This combination facilitates the simultaneous acquisition of a morphological/topological image with that of a chemical surface map, which may be superimposed upon one another (Fig. 16).
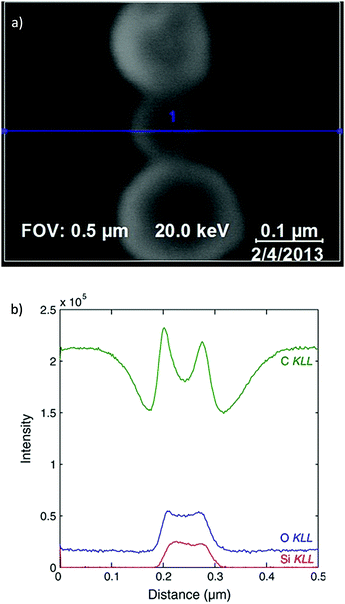 |
| Fig. 16 (a) Scanning electron microscopy (SEM) micrograph indicating region of analysis for corresponding AES spectrum (blue line), (b) resultant AES profiles providing chemical analysis along the aforementioned spatial dimension.326 | |
X-ray generation of auger electrons
The scope of this review has so far focused on AES using an electron gun as the exciting beam, however X-rays are capable of producing augers too and the generation of X-ray induced auger electrons represents an invaluable tool for the chemical analysis of several key systems. Auger peaks often provide a fingerprint method by which to identify chemical compounds by which XPS may not be possible or straightforward (such as some first row transition metal compounds).319 The classic example of the importance of auger electrons in XPS is the speciation of copper. Copper metal and copper(I) are virtually indistinguishable upon analysis of the Cu 2p photoemission, however the Cu LMM auger produces a difference of around 2 eV, facilitating the chemical distinction of the sample.320 This may be taken further with the use of the modified auger parameter (α′),321,322 which is defined as the product of the kinetic energy of the auger emission and the binding energy of the primary photoelectron peak (e.g. Cu LMM Ek + Cu 2p EB). This parameter is advantageous, since it is independent of both exciting energy and any calibration factors applied to non-conductive material data. When working with the modified auger parameter, it is often beneficial to refer to, or produce, a Wagner plot322,323 – a graphical means by which to visualise trends in α′ across a series of compounds.
In the analysis of nanomaterials, α′ may provide even more insight into the surface chemistry and inter-particulate interactions.324,325 Particle size effects and metal–support interactions between supported metal nanoparticles and oxidic supports have been observed to influence the resultant α′. Since α′ depends on initial and final state effects, an estimate of the relaxation energy or degree of screening in the presence of core holes may be extracted from changes to α′.327 An increase to α′ is associated with a higher relaxation energy/screening energy – which may be correlated with increasing particle sizes (and subsequently an increase in the average number of neighbouring atoms capable of screening the core-hole).324 Metal–support interactions may also influence this parameter, with more polarizable supports enhancing the resultant α′.328
EELS & REELS
Electron energy loss spectroscopy (EELS) involves a beam of monoenergetic electrons, (typically with kinetic energies <2 keV), incident with a sample of interest. Inelastic scattering collisions occur from which information about the electronic state of the surface can be deduced.
In reflection electron energy loss spectroscopy (REELS), both elastically and inelastically scattered electrons are collected. The elastically scattered electrons mostly reflect directly from the surface without losing any energy, forming the most intense part of the REELS spectrum which defines the 0 eV position on the energy loss scale. The low-energy loss part of the spectrum (<50 eV) can then be used to determine information about the band structure and dielectric properties of the sample.
The high-energy loss region (generally >50 eV) contains the ionisation edges due to inner shell ionisation, whose features are equivalent of an absorption edge in X-ray absorption spectroscopy (XAS). They are characteristic of the elements present in the sample surface (ca. 15 nm) and their local bonding environment. Therefore, the information from EELS is complementary to XPS and other surface sensitive techniques.
Probing surface valence and conduction band electronic properties
EELS is particularly sensitive to the valence region of a solid surface promoting electrons from the occupied valence band to the unoccupied conduction band and as a result EELS is capable of identifying chemical states that may not be determined through other methods.
For instance, SnO and SnO2 have similar binding energies in XP spectra. Neither XPS nor AES can unambiguously distinguish between the various forms of tin oxides.329–331 However, Hoflund et al. were able to use EELS to distinguish between metallic Sn, SnO, and even suboxides believed to be Sn3O4 and SnO2.332–334 Non-destructive depth profile information may also be obtained by the simple process of tuning the primary electron beam energy. Such information is crucial for understanding the mechanisms of various surface modification processes. In the example of EELS analysis of the oxidation of tin surfaces, it was revealed that the oxidation process underwent a three-dimensional growth mechanism within the subsurface layers, while the more oxidic species were formed at the metal–oxide interface.333,334
A major drawback in the use of EELS is that, for many of the most electronically relevant solids (e.g. mixed oxides/perovskites), the EELS spectra are too complex to interpret. Typically, the intensities and the energy positions of EELS features from interband transitions are determined by the dipole selection rules for single-particle excitations. It also depends on the joint density of filled and unfilled states of the solid.335 However, with the presence of delocalised electrons in a polycrystalline sample, a combination of dipole and non-dipole active transitions in the EELS spectra may occur. For accurate interpretation, EELS then requires a detailed knowledge of the electronic structure within the solid, prior to unravelling the various components of the resultant spectra.
EELS can provide a useful insight into the interaction between probe molecules and a model surface and, like many surface science techniques has found much use, within the field of catalysis as a result. One system to have been investigated thoroughly is that of Ni and NiO which are strongly catalytically active (yet cheap and abundant) materials; EELS studies have been made of the adsorption of various gases, such as O2,336–339 CO,336,340–342 H2,340,341,343 H2O,344 N2345–347 and NO,348 on clean Ni and on Ni alloyed with, or following the deposition of, other metals, such as Al,349,350 Cu,351,352 Fe,353,354 Mo,355 and the alkali metals (Na, and Cs).356,357 In the case of NiO, both EELS studies of single crystalline336,358–364 and polycrystalline NiO365–367 have been carried out and EELS has also been used to monitor changes to other material properties of NiO, such as temperature dependence368,369 and optical behaviours.370,371
However, it becomes apparent from a survey of the available data that the literature lacks consistency of peak assignments and interpretations for the Ni and NiO EELS spectra. Part of this could be due to the lack of detailed systematic investigation but can also be attributed to the need, as mentioned above, for an accurate knowledge of the electronic structure. Hoflund et al. have addressed that issue with an extensive study of the electronic structure of Ni and NiO both experimentally and theoretically.372,373 With the aid of this relevant information, Hoflund et al. were able to carry out the peak assignments of EELS spectra of Ni and NiO obtained from different depths of the samples with systematic alteration of the primary electron energy from 100 to 1150 eV.335 In this approach, the surface and bulk loss peaks can be more clearly distinguished, since an enhancement in the surface sensitivity is more noticeable than the surface loss features, which leads to the improvement of the EELS peak assignments.
Finally, of note is the use of EELS in the probing of plasmons, screened and unscreened final states – of great importance when confronted with the complex multiplet structures associated with transition metal photoemission spectra. As previously mentioned, EELS was used to study the electronic structure of Ni metal, however as well as purely evaluating EELS data of the metal, the combined use of EELS with XPS has resulted in the determination of peaks in the XPS spectrum too.374 Nickel metal, as well as producing a typically asymmetric photoemission, produces two additional broad peaks at +3.7 and +6.0 eV. While there had been some debate on the origin of the major emission at 6.0 eV, Grovesnor et al. used REELS to determine both surface and bulk plasmons as the primary origin of these emissions (though small shake-up features could not be discounted as contributing), and further observed a similar process within Co metal.375 Similar insights are available to more complex systems, such as metal oxides376,377 and semiconductors.378 For example, REELS has been used in combination with XPS and theoretical calculations to probe OsO2 where again, REELS was able to identify the presence and energy of a plasmon. This measurement contributed to the complete understanding of the electronic configuration through theoretical modelling of the density-of-states applied to the valence region of the photoelectron spectrum.376
Analysis of inelastic scattering of electrons
As discussed at the beginning of this review, upon exiting a solid material, photoelectrons may undergo scattering processes which reduce their kinetic energies, contributing to asymmetric peaks, spectral backgrounds and satellite peaks.38 We have covered the importance of the analysis of overlayers and introduced several non-destructive techniques by which we can probe these such as the Tougaard background analysis method.44 One parameter which is of paramount importance in this analysis is an understanding of the inelastic background produced by the overlayer. While the QUASES software provides some elegant solutions to this, one opportunity provided by REELS is the ability to probe the inelastic scattering via electron beam. This may then be modelled to not only build a background model, but also provide additional insights into the surface chemistry. For example, TiO2 overlayer formation on Ti metal was analysed using REELS to produce an inelastic background,379 which was then applied to the Ti 2p region and the resultant background subtraction revealed weak suboxides which may otherwise have not been observed.
Band gap determination for semiconductor materials
Modern device construction often requires the controlled deposition of monolayers with desired properties one of which, that is often crucial for optimised device performance, is the band gap of the materials involved. However, the usual optical means (i.e. a Kubelka–Munk function on UV-Vis spectra)261 often fail when a layer is thin or grown on a different substrate. For samples with a well-defined band gap, the REELS technique provides a convenient method of measurement in these cases since REELS’ electrons must give up at least the energy equivalent to the band gap. Furthermore, while it is already a surface sensitive technique, decreasing the energy of the incoming electron beam can augment the surface sensitivity and hence, the band gap of thin films may be more accurately determined.
Vos et al. have used REELS under controlled conditions to extensively study a series of semiconductors with well-established band gap values.380 They discovered that, without surface preparation, it was possible to get a meaningful value of the band gap if a relatively high incoming energy of ∼5 keV was used. When the energy was below 1 keV however, the intensity of the REELS spectrum inside the gap is insignificant. This usually caused a fading of the onset, and resulted in an inability to record the band gap energy.
Recently, REELS has also been successfully used to investigate ultrathin gate oxide materials applied in complementary metal–oxide–semiconductor (CMOS).381–384 Transition metal oxides such as HfO2, ZrO2, and their compounds deposited on SiO2 or Al2O3 have been exploited as alternative high-k gate dielectrics385–387 to avoid quantum tunnelling388 during further scale reduction process of semiconductors. Kang and Tougaard and their colleagues obtained the band gap of HfZrO4 gate oxide thin film and inelastic mean free path (IMFP) values through a quantitative analysis of REELS spectra.383
Investigation of conjugation or aromaticity in organic materials
For materials with conjugated carbon bonding systems, REELS can provide complementary information to that obtained via XPS. An example is shown in Fig. 17, involving the organic light emitting diode (OLED) material poly(9,9-di-n-octylfluorenyl-2,7-diyl), known as PFO, which was probed using XPS and REELS. The C 1s XPS spectrum shows two very weak peaks at a binding energy position slightly greater than the main peak.
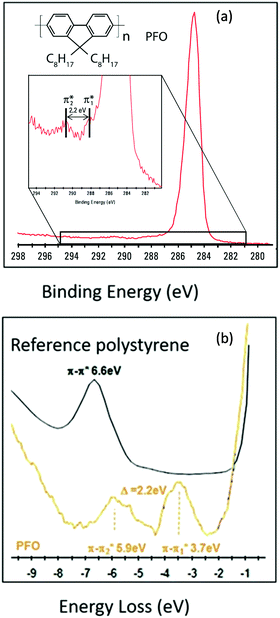 |
| Fig. 17 (a) Photoelectron spectrum of poly (9,9-dioctylfluorene) (PFO), including observation of weak shake-up peaks caused from inelastic collisions between emitted photoelectrons and π/π* HOMO/LUMO (inset) and (b) REELS profile from PFO in which π/π* shake-up satellites may be clearly observed and energies recorded. Polystyrene is included as a reference for position of phenyl π–π* transition. Reprinted (adapted) with permission from ref. 389. Copyright {2013} Thermo Scientific. | |
These are assigned as shake-up satellites promoting an electronic transition from HOMO (π) into LUMO (π*) of PFO. In the REELS spectrum the same two peaks are much more intense and can therefore be more easily identified. Quantification of sp2/sp3 hybridisation of carbons may also be achieved by REELS, in cases where it is not always possible via other techniques such as XPS/AES.390
Comparison of relative hydrogen content in polymer materials
XPS has great difficulty detecting hydrogen (H 1s) because of its very low photoionisation cross section, its only really feasible with a synchrotron source. REELS however has the capability to detect and quantify hydrogen because of a process that resembles that of the ISS phenomenon. When the REELs electron strikes a hydrogen atom, the atom recoils because it is so much lighter than other elements and results in a discrete energy loss for the electron. This is demonstrated in Fig. 18 in which REELS with a 1 keV beam energy was used to analyse a series of polymers. Strong features relating to conjugated carbon bonding are readily observed, however another set of peaks at around 1.8 eV from the primary elastic peak are also present and these are assigned to hydrogen, an assignment that is supported by the REELS spectrum for PTFE (which has no hydrogens), where this peak is not visible.
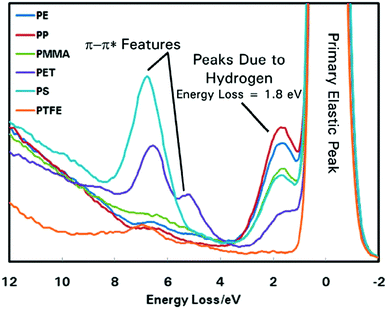 |
| Fig. 18 REELS spectra of polyethylene, polypropylene, poly(methyl methacrylate), polyethylene terephthalate, polystyrene and poly tetrafluoroethylene to evidence the changing peak intensity at ∼1.8 eV with differing H concentration. Reprinted (adapted) with permission from ref. 391. Copyright {2013} Thermo Scientific. | |
The relative intensity of the hydrogen peak to the primary elastic peak is proportional to the amount of hydrogen relative to other atoms (e.g. C, O and N). A simple peak deconvolution of the two peaks can therefore be used to quantify the hydrogen present.
Summary
In this review, we hope to have emphasised the great importance of utilising a multi-technique approach to materials analysis, in order to extract the maximum amount of information and enable a greater understanding of materials properties at play. Surface analysis of materials is of enormous importance in almost every aspect of science and technology and combinations of techniques can provide much more information than is often apparent through the utilisation of a single technique alone. Whilst XPS is arguably the most widely appreciated technique in this area, we have highlighted some of the many ways in which it may be used in conjunction with a broad range of complementary analyses to elucidate additional sample information, far more than simply the chemical nature of the material surface as is often the extent of the information gleaned in the general analysis of materials. Electronic, 2-dimensional, topological and structural information are readily obtained through the combination of multiple techniques associated with photoelectron spectroscopy. Manufacturers have developed multi-technique systems for many years now, largely focused on users in the surface science field and relying on knowledge and experience of working with complex UHV chambers and systems. The advent of current gen systems such as the Kratos Analytical Ltd AXIS Supra+ and Thermo ScientificTM NEXSATM G2 has signalled a pronounced shift towards the development of multifunctional XPS based instruments aimed at a more general user base. Particularly the introduction of automated sample handling, more robust software and data handling and easy switching between experimental modes represents a great opportunity for such techniques to really enter mainstream use across research laboratories of many fields. Whilst the relatively large investment will restrict the uptake somewhat, the reduced requirement for specialised skills and knowledge will certainly broaden the general userbase and increase the number of researchers looking to utilise XPS and multi-technique surface analysis. The field is even starting to see more niche general use systems, such as the SPECS GmbH EnviroESCATM and Scienta Omicron GmbH HAXPES Lab – capable of user friendly NAP-XPS and HAXPES experiments respectively. While some manufacturers will remain focused on one or two applications, others seem to be moving in the direction of adding more and more functionality to individual instruments. As the demand for such systems grows, it is entirely possible we see an increase in the number of available add-ons to standard commercial instruments, for example Diffuse Reflectance UV-Vis (DRUVS), Attenuated Total Reflectance Infrared (ATR) and even traditionally synchrotron exclusive techniques such as Near-edge X-ray Absorption Fine Structure (NEXAFS)392 as improvements to radiation sources and detectors are developed. The combination of these, in particular with the in operando capabilities of systems such as the lab-based NAP-XPS instruments – represents the potential for incredibly powerful analytical investigations to operate within the confines of even a small scale laboratory.
Author contributions
Conceptualisation: MAI. Funding acquisition: PRD and RP. Investigation: JDD, MAI, RL, SG and DJM. Resources: PRD and RP. Writing – original draft: JDD, MAI, RL, SG and DJM. Writing – reviewing and editing: PRD, MAI, DJM, RP.
Conflicts of interest
There are no conflicts to declare.
Acknowledgements
The EPSRC National Facility for XPS (‘HarwellXPS’), operated by Cardiff University and UCL, under contract no. PR16195.
Notes and references
- R. Schlögl, Heterogeneous Catalysis, Angew. Chem., Int. Ed., 2015, 54, 3465–3520 CrossRef PubMed
.
- Y. Li, Z. Y. Fu and B. L. Su, Hierarchically Structured Porous Materials for Energy Conversion and Storage, Adv. Funct. Mater., 2012, 22, 4634–4667 CrossRef CAS
.
- F. S. da Silva and T. M. de Souza, Novel materials for solid oxide fuel cell technologies: a literature review, Int. J. Hydrogen Energy, 2017, 42, 26020–26036 CrossRef CAS
.
- A. Polman, M. Knight, E. C. Garnett, B. Ehrler and W. C. Sinke, Photovoltaic materials: present efficiencies and future challenges, Science, 2016, 352, 307–318 CrossRef CAS PubMed
.
- S. Saghazadeh, C. Rinoldi, M. Schot, S. S. Kashaf, F. Sharifi, E. Jalilian, K. Nuutila, G. Giatsidis, P. Mostafalu, H. Derakhshandeh, K. Yue, W. Swieszkowski, A. Memic, A. Tamayol and A. Khademhosseini, Drug delivery systems and materials for wound healing applications, Adv. Drug Delivery Rev., 2018, 127, 138–166 CrossRef CAS PubMed
.
- Y. Y. Jian, W. W. Hu, Z. H. Zhao, P. F. Cheng, H. Haick, M. S. Yao and W. W. Wu, Gas Sensors Based on Chemi-Resistive Hybrid Functional Nanomaterials, Nanomicro Lett., 2020, 12, 1–43 Search PubMed
.
- Y. C. Yeh, T. H. Huang, S. C. Yang, C. C. Chen and J. Y. Fang, Nano-Based Drug Delivery or Targeting to Eradicate Bacteria for Infection Mitigation: A Review of Recent Advances, Front. Chem., 2020, 8, 1–22 CrossRef PubMed
.
- X. S. Zhang, Y. J. Chen and J. L. Hu, Recent advances in the development of aerospace materials, Prog. Aeronaut. Sci., 2018, 97, 22–34 CrossRef
.
- N. Briggs, S. Subramanian, Z. Lin, X. F. Li, X. T. Zhang, K. H. Zhang, K. Xiao, D. Geohegan, R. Wallace, L. Q. Chen, M. Terrones, A. Ebrahimi, S. Das, J. Redwing, C. Hinkle, K. Momeni, A. van Duin, V. Crespi, S. Kar and J. A. Robinson, A roadmap for electronic grade 2D materials, 2D Mater., 2019, 6, 1–23 Search PubMed
.
-
J. F. Watts and J. Wolstenholme, An introduction to surface analysis by XPS and AES, John Wiley & Sons, 2019 Search PubMed
.
- D. Son, S. Cho, J. Nam, H. Lee and M. Kim, X-ray-Based Spectroscopic Techniques for Characterization of Polymer Nanocomposite Materials at a Molecular Level, Polymers, 2020, 12, 1–33 Search PubMed
.
- C. S. Fadleys, X-ray photoelectron spectroscopy: From origins to future directions, Nucl. Instrum. Methods Phys. Res., Sect. A, 2009, 601, 8–31 CrossRef CAS
.
- D. D. Sarma, P. K. Santra, S. Mukherjee and A. Nag, X-ray Photoelectron Spectroscopy: A Unique Tool To Determine the Internal Heterostructure of Nanoparticles, Chem. Mater., 2013, 25, 1222–1232 CrossRef CAS
.
- S. Thapa, R. Paudel, M. D. Blanchet, P. T. Gemperline and R. B. Comes, Probing surfaces and interfaces in complex oxide films via in situ X-ray photoelectron spectroscopy, J. Mater. Res., 2021, 36, 26–51 CrossRef CAS
.
- M. R. Linford, V. S. Smentkowski, J. T. Grant, C. R. Brundle, P. M. Sherwood, M. C. Biesinger, J. Terry, K. Artyushkova, A. Herrera-Gómez and S. Tougaard, Proliferation of faulty materials data analysis in the literature, Microsc. Microanal., 2020, 26, 1–2 CrossRef CAS PubMed
.
- D. R. Baer, K. Artyushkova, C. Richard Brundle, J. E. Castle, M. H. Engelhard, K. J. Gaskell, J. T. Grant, R. T. Haasch, M. R. Linford and C. J. Powell, Practical guides for X-ray photoelectron spectroscopy: first steps in planning, conducting, and reporting XPS measurements, J. Vac. Sci. Technol., A, 2019, 37, 1–13 CrossRef PubMed
.
- G. H. Major, N. Fairley, P. M. Sherwood, M. R. Linford, J. Terry, V. Fernandez and K. Artyushkova, Practical guide for curve fitting in X-ray photoelectron spectroscopy, J. Vac. Sci. Technol., 2020, 38, 1–22 Search PubMed
.
- E. Vanea and V. Simon, XPS and Raman study of zinc containing silica microparticles loaded with insulin, Appl. Surf. Sci., 2013, 280, 144–150 CrossRef CAS
.
- Y. M. Piao, V. N. Tondare, C. S. Davis, J. M. Gorham, E. J. Petersen, J. W. Gilman, K. Scott, A. E. Vladar and A. R. H. Walker, Comparative study of multiwall carbon nanotube nanocomposites by Raman, SEM, and XPS measurement techniques, Compos. Sci. Technol., 2021, 208, 108753 CrossRef CAS
.
- S. Malvankar, S. Doke, R. Gahlaut, E. Martinez-Teran, A. A. El-Gendy, U. Deshpande and S. Mahamuni, Co-Doped SnO2 Nanocrystals: XPS, Raman, and Magnetic Studies, J. Electron. Mater., 2020, 49, 1872–1880 CrossRef CAS
.
- W. S. Cho, Y. S. Oh, C. S. Kim, M. Osada, M. Kakihana, D. S. Lim and D. S. Cheong, Characterization of Si3N4/SiC nanocomposite by Raman scattering and XPS, J. Alloys Compd., 1999, 285, 255–259 CrossRef CAS
.
- N. Catalin and M. Cernea, Characterization of BaTi4O9 ceramics by Raman spectroscopy and XPS after ion etching, J. Optoelectron. Adv. Mater., 2006, 8, 1879–1883 CAS
.
-
P. Marcus and V. Maurice, X-ray photoelectron spectroscopy and scanning tunneling microscopy studies of thin anodic oxide overlayers on metal and alloy single-crystal surfaces, Solid-liquid Electrochemical Interfaces, 1997, vol. 656, pp. 236–244 Search PubMed
.
- H. Zhang, H. L. Sun, K. C. Shen, J. P. Hu, J. B. Hu, Z. Jiang and F. Song, Recent Progress with In Situ Characterization of Interfacial Structures under a Solid-Gas Atmosphere by HP-STM and AP-XPS, Materials, 2019, 12, 3674 CrossRef CAS PubMed
.
- D. R. Baer, D. J. Gaspar, P. Nachimuthu, S. D. Techane and D. G. Castner, Application of surface chemical analysis tools for characterization of nanoparticles, Anal. Bioanal. Chem., 2010, 396, 983–1002 CrossRef CAS PubMed
.
- K. Durose, S. E. Asher, W. Jaegermann, D. Levi, B. E. McCandless, W. Metzger, H. Moutinho, P. D. Paulson, C. L. Perkins, J. R. Sites, G. Teeter and M. Terheggen, Physical characterization of thin-film solar cells, Prog. Photovolt. Res. Appl., 2004, 12, 177–217 CrossRef CAS
.
- Y. Cho and A. Ivanisevic, TAT peptide immobilization on gold surfaces: a comparison study with a thiolated peptide and alkylthiols using AFM, XPS, and FT-IRRAS, J. Phys. Chem. B, 2005, 109, 6225–6232 CrossRef CAS PubMed
.
- T. A. Jurgens-Kowal and J. W. Rogers, Pyrolysis of tetraethoxysilane on Mo(100) at low temperatures, J. Phys. Chem. B, 1998, 102, 2193–2206 CrossRef CAS
.
- Z. Liu, T. Duchoň, H. Wang, D. C. Grinter, I. Waluyo, J. Zhou, Q. Liu, B. Jeong, E. J. Crumlin and V. Matolín, Ambient pressure XPS and IRRAS investigation of ethanol steam reforming on Ni–CeO2(111) catalysts: an in situ study of C–C and O–H bond scission, Phys. Chem. Chem. Phys., 2016, 18, 16621–16628 RSC
.
- B. T. Young, D. R. Heskett, C. C. Nguyen, M. Nie, J. C. Woicik and B. L. Lucht, Hard X-ray photoelectron spectroscopy (HAXPES) investigation of the silicon solid electrolyte interphase (SEI) in lithium-ion batteries, ACS Appl. Mater. Interfaces, 2015, 7, 20004–20011 CrossRef CAS PubMed
.
- O. Renault, E. Martinez, C. Zborowski, J. Mann, R. Inoue, J. Newman and K. Watanabe, Analysis of buried interfaces in multilayer device structures with hard XPS (HAXPES) using a CrKα source, Surf. Interface Anal., 2018, 50, 1158–1162 CrossRef CAS
.
- M. A. Isaacs, L. J. Durndell, A. C. Hilton, L. Olivi, C. M. Parlett, K. Wilson and A. F. Lee, Tunable Ag@SiO2 core–shell nanocomposites for broad spectrum antibacterial applications, RSC Adv., 2017, 7, 23342–23347 RSC
.
- K. Devriendt, H. Poelman, L. Fiermans, G. Creten and G. Froment, Angular resolved XPS applied to V2O5-based catalysts, Surf. Sci., 1996, 352, 750–754 CrossRef
.
- O. Baschenko, V. Bukhtiyarov and A. Boronin, ARXPS-based concentration profiles restoration applied to adsorbate/metal systems, Surf. Sci., 1992, 271, 493–500 CrossRef
.
- V. Bukhtiyarov, A. Boronin and O. Baschenko, ARXPS-based analysis of different oxygen states adsorbed at silver foil, Surf. Rev. Lett., 1994, 1, 577–579 CrossRef CAS
.
- E. Talik, M. Kruczek, H. Sakowska and W. Szyrski, XPS studies of chemically etched surfaces of (La, Sr)(Al, Ta)O3 single crystals, J. Alloys Compd., 2003, 361, 282–288 CrossRef CAS
.
- Y. Kawabata, J. Taniguchi and I. Miyamoto, XPS studies on damage evaluation of single-crystal diamond chips processed with ion beam etching and reactive ion beam assisted chemical etching, Diamond Relat. Mater., 2004, 13, 93–98 CrossRef CAS
.
- A. Jablonski and C. J. Powell, Relationships between electron inelastic mean free paths, effective attenuation lengths, and mean escape depths, J. Electron Spectrosc. Relat. Phenom., 1999, 100, 137–160 CrossRef CAS
.
- H. Tokutaka, N. Ishihara, K. Nishimori, S. Kishida and K. Isomoto, Background removal in X-ray photoelectron spectroscopy, Surf. Interface Anal., 1992, 18, 697–704 CrossRef CAS
.
- D. A. Shirley, High-resolution X-ray photoemission spectrum of the valence bands of gold, Phys. Rev. B: Condens. Matter Mater. Phys., 1972, 5, 4709 CrossRef
.
- J. Végh, The Shirley background revised, J. Electron Spectrosc. Relat. Phenom., 2006, 151, 159–164 CrossRef
.
- S. Tougaard and P. Sigmund, Influence of elastic and inelastic scattering on energy spectra of electrons emitted from solids, Phys. Rev. B: Condens. Matter Mater. Phys., 1982, 25, 4452 CrossRef CAS
.
- S. Tougaard and B. Jørgensen, Inelastic background intensities in XPS spectra, Surf. Sci., 1984, 143, 482–494 CrossRef CAS
.
- S. Tougaard and H. Hansen, Non-destructive depth profiling through quantitative analysis of surface electron spectra, Surf. Interface Anal., 1989, 14, 730–738 CrossRef CAS
.
- B. Semak, C. Van der Marel and S. Tougaard, Comparison of the Tougaard, ARXPS, RBS and ellipsometry methods to determine the thickness of thin SiO2 layers, Surf. Interface Anal., 2002, 33, 238–244 CrossRef CAS
.
- N. Suzuki, T. Kato and S. Tougaard, Level of consistency in quantification and IMFP determination by the Tougaard method applied to XPS of a Langmuir–Blodgett film taken at widely different emission angles, Surf. Interface Anal., 2001, 31, 862–868 CrossRef CAS
.
- I. Preda, L. Soriano, L. Alvarez, J. Méndez, F. Yubero, A. Gutiérrez and J. Sanz, Study of the morphology of NiO nanostructures grown on highly ordered pyrolytic graphite, by the Tougaard method and atomic force microscopy: a comparative study, Surf. Interface Anal., 2010, 42, 869–873 CrossRef CAS
.
- S. Tougaard, Surface nanostructure determination by X-ray photoemission spectroscopy peak shape analysis, J. Vac. Sci. Technol., 1996, 14, 1415–1423 CrossRef CAS
.
-
S. Tougaard, QUASES-XS-REELS, 1998, http://www.quases.com/products/quases-xs-reels/.
- N. Kaiser, Review of the fundamentals of thin-film growth, Appl. Opt., 2002, 41, 3053–3060 CrossRef CAS PubMed
.
- A. F. Lee, C. V. Ellis, K. Wilson and N. S. Hondow, In situ studies
of titania-supported Au shell–Pd core nanoparticles for the selective aerobic oxidation of crotyl alcohol, Catal. Today, 2010, 157, 243–249 CrossRef CAS
.
- M. F. Toney and S. Brennan, Measurements of carbon thin films using X-ray reflectivity, J. Appl. Phys., 1989, 66, 1861–1863 CrossRef CAS
.
- A. K. Petford-Long and A. N. Chiaramonti, Transmission electron microscopy of multilayer thin films, Annu. Rev. Mater. Res., 2008, 38, 559–584 CrossRef CAS
.
- B. R. Strohmeier, An ESCA method for determining the oxide thickness on aluminum alloys, Surf. Interface Anal., 1990, 15, 51–56 CrossRef CAS
.
- M. C. Biesinger, B. P. Payne, L. W. Lau, A. Gerson and R. S. C. Smart, X-ray photoelectron spectroscopic chemical state quantification of mixed nickel metal, oxide and hydroxide systems, Surf. Interface Anal., 2009, 41, 324–332 CrossRef CAS
.
- K. Analytical, Applications note MO454(A): Reconstructed Concentration Depth Profiles from Angle-Resolved XPS using MEM Software https://www.kratos.com/application-areas/application-downloads/reconstructed-concentration-depth-profiles-angle-resolved, accessed 30/06/2021, 2021.
- A. Jablonski and C. J. Powell, Information depth and the mean escape depth in Auger electron spectroscopy and X-ray photoelectron spectroscopy, J. Vac. Sci. Technol., A, 2003, 21, 274–283 CrossRef CAS
.
- C. Fadley, R. Baird, W. Siekhaus, T. Novakov and S. Bergström, Surface analysis and angular distributions in X-ray photoelectron spectroscopy, J. Electron Spectrosc. Relat. Phenom., 1974, 4, 93–137 CrossRef CAS
.
- C. Fadley and S. Bergström, Angular distribution of photoelectrons from a metal single crystal, Phys. Lett. A, 1971, 35, 375–376 CrossRef CAS
.
- W. Fraser, J. Florio, W. Delgass and W. Robertson, Surface sensitivity and angular dependence of X-ray photoelectron spectra, Surf. Sci., 1973, 36, 661–674 CrossRef CAS
.
- J. E. Fulghum, Determination of overlayer thickness by angle-resolved XPS: a comparison of algorithms, Surf. Interface Anal., 1993, 20, 161–173 CrossRef CAS
.
- V. Schier, H.-J. Michel and J. Halbritter, ARXPS-analysis of sputtered TiC, SiC and Ti0.5Si0.5C layers, Fresenius' J. Anal. Chem., 1993, 346, 227–232 CrossRef CAS
.
- K. L. Parry, A. Shard, R. Short, R. White, J. Whittle and A. Wright, ARXPS characterisation of plasma polymerised surface chemical gradients, Surf. Interface Anal., 2006, 38, 1497–1504 CrossRef CAS
.
- R. G. Palgrave, D. J. Payne and R. G. Egdell, Nitrogen diffusion in doped TiO2(110) single crystals: a combined XPS and SIMS study, J. Mater. Chem., 2009, 19, 8418–8425 RSC
.
- N. Koshizaki, M. Kudo, M. Owari, Y. Nihei and H. Kamada, Structural and Chemical State Analysis of the Heat-Treated Au/GaSb(110) Interface by Means of Angle-Resolved X-Ray Photoelectron Spectroscopy (ARXPS), Jpn. J. Appl. Phys., 1980, 19, L349 CrossRef CAS
.
- J. Halbritter, Model of Si–SiO2 interfaces based on ARXPS measurements, J. Mater. Res., 1988, 3, 506–513 CrossRef CAS
.
- B. Hornetz, H. Michel and J. Halbritter, ARXPS studies of SiO2–SiC interfaces and oxidation of 6H SiC single crystal Si-(001) and C-(001) surfaces, J. Mater. Res., 1994, 9, 3088–3094 CrossRef CAS
.
- M. Zier, S. Oswald, R. Reiche, M. Kozlowska and K. Wetzig, Interface formation and reactions at Ta–Si and Ta–SiO2 interfaces studied by XPS and ARXPS, J. Electron Spectrosc. Relat. Phenom., 2004, 137, 229–233 CrossRef
.
- A. Damascelli, Probing the electronic structure of complex systems by ARPES, Phys. Scr., 2004, 2004, 61 CrossRef
.
- A. Osatiashtiani, A. F. Lee, M. Granollers, D. R. Brown, L. Olivi, G. Morales, J. A. Melero and K. Wilson, Hydrothermally stable, conformal, sulfated zirconia monolayer catalysts for glucose conversion to 5-HMF, ACS Catal., 2015, 5, 4345–4352 CrossRef CAS
.
- J. Walton, M. Alexander, N. Fairley, P. Roach and A. Shard, Film thickness measurement and contamination layer correction for quantitative XPS, Surf. Interface Anal., 2016, 48, 164–172 CrossRef CAS
.
- P. J. Cumpson, The Thickogram: a method for easy film thickness measurement in XPS, Surf. Interface Anal., 2000, 29, 403–406 CrossRef CAS
.
- A. Shard, J. Wang and S. Spencer, XPS topofactors: determining overlayer thickness on particles and fibres, Surf. Interface Anal., 2009, 41, 541–548 CrossRef CAS
.
- A. G. Shard, A straightforward method for interpreting XPS data from core–shell nanoparticles, J. Phys. Chem. C, 2012, 116, 16806–16813 CrossRef CAS
.
- G. Panaccione and K. Kobayashi, Hard X-ray photoemission spectroscopy: variable depth analysis of bulk, surface and interface electronic properties, Surf. Sci., 2012, 606, 125–129 CrossRef CAS
.
-
T. Wiell, C. Liljenberg and P. Palmgren, Hard X-ray photoelectron spectroscopy arrangement and system, US Pat., 11002694, 2021 Search PubMed
.
- G. Panaccione, G. Cautero, M. Cautero, A. Fondacaro, M. Grioni, C. Henriquet, G. Monaco, M. Mulazzi, F. Offi and L. Paolasini, Results and perspectives in hard X-ray photoemission spectroscopy (HAXPES) from solids, Nucl. Instrum. Methods Phys. Res., Sect. B, 2006, 246, 106–111 CrossRef CAS
.
- J. Kahk, C. Poll, F. Oropeza, J. Ablett, D. Céolin, J. Rueff, S. Agrestini, Y. Utsumi, K. Tsuei and Y. Liao, Understanding the Electronic Structure of IrO2 Using Hard-X-ray Photoelectron Spectroscopy and Density-Functional Theory, Phys. Rev. Lett., 2014, 112, 117601 CrossRef CAS PubMed
.
-
J. H. Scofield, Theoretical photoionization cross sections from 1 to 1500 keV, California Univ., Livermore, Lawrence Livermore Lab., 1973 Search PubMed
.
-
J.-J. Yeh, Atomic Calculation of Photoionization Cross-Sections and Asymmetry Parameters, Gordon & Breach Science, Publishers, 1993 Search PubMed
.
- J. Yeh and I. Lindau, Atomic subshell photoionization cross sections and asymmetry parameters: 1⩽ Z⩽ 103, At. Data Nucl. Data Tables, 1985, 32, 1–155 CrossRef CAS
.
- A. Regoutz, M. Mascheck, T. Wiell, S. K. Eriksson, C. Liljenberg, K. Tetzner, B. A. Williamson, D. O. Scanlon and P. Palmgren, A novel laboratory-based hard X-ray photoelectron spectroscopy system, Rev. Sci. Instrum., 2018, 89, 073105 CrossRef PubMed
.
- C. Kalha, N. K. Fernando, P. Bhatt, F. O. Johansson, A. Lindblad, H. Rensmo, L. Z. Medina, R. Lindblad, S. Siol and L. P. Jeurgens, Hard X-ray photoelectron spectroscopy: a snapshot of the state-of-the-art in 2020, World J. Condens. Matter Phys., 2021, 33, 233001 CrossRef CAS PubMed
.
- K. Kim, W. Baitinger, J. Amy and N. Winograd, ESCA studies of metal-oxygen surfaces using argon and oxygen ion-bombardment, J. Electron Spectrosc. Relat. Phenom., 1974, 5, 351–367 CrossRef CAS
.
- R. Holm and S. Storp, ESCA studies on changes in surface composition under ion bombardment, Appl. Phys., 1977, 12, 101–112 CAS
.
- J. W. Coburn and E. Kay, Techniques for elemental composition profiling in thin films, Crit. Rev. Solid State Mater. Sci., 1973, 4, 561–590 CrossRef
.
- J. B. Malherbe, S. Hofmann and J. M. Sanz, Preferential sputtering of oxides: a comparison of model predictions with experimental data, Appl. Surf. Sci., 1986, 27, 355–365 CrossRef CAS
.
- R. Grilli, R. Simpson, C. F. Mallinson and M. A. Baker, Comparison of Ar+ Monoatomic and cluster ion sputtering of Ta2O5 at different ion energies, by XPS: Part 1-Monoatomic Ions, Surf. Sci. Spectra, 2014, 21, 50–67 CrossRef CAS
.
- C. Drosos, C. Jia, S. Mathew, R. G. Palgrave, B. Moss, A. Kafizas and D. Vernardou, Aerosol-assisted chemical vapor deposition of V2O5 cathodes with high rate capabilities for magnesium-ion batteries, J. Power Sources, 2018, 384, 355–359 CrossRef CAS
.
- A. Holländer, M. Haupt and C. Oehr, On depth profiling of polymers by argon ion sputtering, Plasma Process. Polym., 2007, 4, 773–776 CrossRef
.
- I. Yamada, J. Matsuo, Z. Insepov, T. Aoki, T. Seki and N. Toyoda, Nano-processing with gas cluster ion beams, Nucl. Instrum. Methods Phys. Res., Sect. B, 2000, 164, 944–959 CrossRef
.
- I. Yamada, J. Matsuo, N. Toyoda and A. Kirkpatrick, Materials processing by gas cluster ion beams, Mater. Sci. Eng., R, 2001, 34, 231–295 CrossRef
.
- E. F. Smith, J. D. Counsell, J. Bailey, J. S. Sharp, M. R. Alexander, A. G. Shard and D. J. Scurr, Sample rotation improves gas cluster sputter depth profiling of polymers, Surf. Interface Anal., 2017, 49, 953–959 CrossRef CAS
.
- R. E. Galindo, R. Gago, D. Duday and C. Palacio, Towards nanometric resolution in multilayer depth profiling: a comparative study of RBS, SIMS, XPS and GDOES, Anal. Bioanal. Chem., 2010, 396, 2725–2740 CrossRef PubMed
.
- L. Zhong, D. Chen and S. Zafeiratos, A mini review of in situ near-ambient pressure XPS studies on non-noble, late transition metal catalysts, Catal. Sci. Technol., 2019, 9, 3851–3867 RSC
.
- J. Schnadt, J. Knudsen and N. Johansson, Present and new frontiers in materials research by ambient pressure X-ray photoelectron spectroscopy, World J. Condens. Matter Phys., 2020, 32, 413003 CrossRef CAS PubMed
.
- A. Jürgensen, N. Esser and R. Hergenröder, Near ambient pressure XPS with a conventional X-ray source, Surf. Interface Anal., 2012, 44, 1100–1103 CrossRef
.
- C. Arble, M. Jia and J. T. Newberg, Lab-based ambient pressure X-ray photoelectron spectroscopy from past to present, Surf. Sci. Rep., 2018, 73, 37–57 CrossRef CAS
.
- R. M. Palomino, R. Hamlyn, Z. Liu, D. C. Grinter, I. Waluyo, J. A. Rodriguez and S. D. Senanayake, Interfaces in heterogeneous catalytic reactions: ambient pressure XPS as a tool to unravel surface chemistry, J. Electron Spectrosc. Relat. Phenom., 2017, 221, 28–43 CrossRef CAS
.
- Y. Takagi, T. Uruga, M. Tada, Y. Iwasawa and T. Yokoyama, Ambient Pressure Hard X-ray Photoelectron Spectroscopy for Functional Material Systems as Fuel Cells under Working Conditions, Acc. Chem. Res., 2018, 51, 719–727 CrossRef CAS PubMed
.
- J. Theerthagiri, S. J. Lee, A. P. Murthy, J. Madhavan and M. Y. Choi, Fundamental aspects and recent advances in transition metal nitrides as electrocatalysts for hydrogen evolution reaction: A review, Curr. Opin. Solid State Mater. Sci., 2020, 24, 100805 CrossRef CAS
.
- B. L. Ellis and L. F. Nazar, Sodium and sodium-ion energy storage batteries, Curr. Opin. Solid State Mater. Sci., 2012, 16, 168–177 CrossRef CAS
.
-
A. F. Silva, Trends in Interfacial Electrochemistry, Springer, Netherlands, 2012 Search PubMed
.
- N. Andreu, D. Flahaut, R. Dedryvère, M. Minvielle, H. Martinez and D. Gonbeau, XPS Investigation of Surface Reactivity of Electrode Materials: Effect of the Transition Metal, ACS Appl. Mater. Interfaces, 2015, 7, 6629–6636 CrossRef CAS PubMed
.
- R. Kotz, H. Neff and S. Stucki, Anodic iridium oxide-films – XPS-studies of oxidation-state changes and O2-evolution, J. Electrochem. Soc., 1984, 131, 72–77 CrossRef
.
- H. Neff, W. Foditsch and R. Kotz, An Electrochemical Preparation Chamber for the Kratos Es-300 Electron Spectrometer, J. Electron Spectrosc. Relat. Phenom., 1984, 33, 171–174 CrossRef CAS
.
- W. N. Hansen, C. L. Wang and T. W. Humpherys, A study of electrode immersion and emersion, J. Electroanal. Chem., 1978, 93, 87–98 CrossRef CAS
.
- W. N. Hansen, C. L. Wang and T. W. Humphryes, Electrode emersion and the double layer, J. Electroanal. Chem., 1978, 90, 137–141 CrossRef CAS
.
- W. N. Hansen, D. M. Kolb, D. L. Rath and R. Wille, An Esca Study on Emersed Electrodes, J. Electroanal. Chem., 1980, 110, 369–373 CrossRef CAS
.
- D. M. Kolb, D. L. Rath, R. Wille and W. N. Hansen, An Esca Study on the Electrochemical Double-Layer of Emersed Electrodes, Phys. Chem. Chem. Phys., 1983, 87, 1108–1113 CAS
.
- D. Hecht and H. H. Strehblow, XPS investigations of the electrochemical double layer on silver in alkaline chloride solutions, J. Electroanal. Chem., 1997, 440, 211–217 CAS
.
- N. Yabuuchi and T. Ohzuku, Novel lithium insertion material of LiCo1/3Ni1/3Mn1/3O2 for advanced lithium-ion batteries, J. Power Sources, 2003, 119–121, 171–174 CrossRef CAS
.
- S.-Y. Yang, X.-Y. Wang, Z.-L. Liu, Q.-Q. Chen, X.-K. Yang and Q.-L. Wei, Influence of pretreatment process on structure, morphology and electrochemical properties of Li[Ni1/3Co1/3Mn1/3]O2 cathode material, Trans. Nonferrous Met. Soc. China, 2011, 21, 1995–2001 CrossRef CAS
.
- H. Kobayashi, Y. Arachi, S. Emura, H. Kageyama, K. Tatsumi and T. Kamiyama, Investigation on lithium de-intercalation mechanism for Li1−yNi1/3Mn1/3Co1/3O2, J. Power Sources, 2005, 146, 640–644 CrossRef CAS
.
- M. Ma, N. A. Chernova, B. H. Toby, P. Y. Zavalij and M. S. Whittingham, Structural and electrochemical behavior of LiMn0.4Ni0.4Co0.2O2, J. Power Sources, 2007, 165, 517–534 CrossRef CAS
.
- Y. Bentaleb, I. Saadoune, K. Maher, L. Saadi, K. Fujimoto and S. Ito, On the LiNi0.2Mn0.2Co0.6O2 positive electrode material, J. Power Sources, 2010, 195, 1510–1515 CrossRef CAS
.
- S. Oh, J. K. Lee, D. Byun, W. I. Cho and B. Won Cho, Effect of Al2O3 coating on electrochemical performance of LiCoO2 as cathode materials for secondary lithium batteries, J. Power Sources, 2004, 132, 249–255 CrossRef CAS
.
- Y. Oh, D. Ahn, S. Nam and B. Park, The effect of Al2O3-coating coverage on the electrochemical properties in LiCoO2 thin films, J. Solid State Electrochem., 2010, 14, 1235–1240 CrossRef CAS
.
- Y. Huang, J. Chen, F. Cheng, W. Wan, W. Liu, H. Zhou and X. Zhang, A modified Al2O3 coating process to enhance the electrochemical performance of Li(Ni1/3Co1/3Mn1/3)O2 and its comparison with traditional Al2O3 coating process, J. Power Sources, 2010, 195, 8267–8274 CrossRef CAS
.
- J. Van Elp, J. L. Wieland, H. Eskes, P. Kuiper, G. A. Sawatzky, F. M. F. De Groot and T. S. Turner, Electronic structure of CoO, Li-doped CoO, and LiCoO2, Phys. Rev. B: Condens. Matter Mater. Phys., 1991, 44, 6090–6103 CrossRef CAS PubMed
.
-
B. E. Conway, Electrochemical Supercapacitors: Scientific Fundamentals and Technological Applications, Springer, US, 2013 Search PubMed
.
- J. K. Chang, M. T. Lee, W. T. Tsai, M. J. Deng, H. F. Cheng and I. W. Sun, Pseudocapacitive Mechanism of Manganese Oxide in 1-Ethyl-3-methytimidazolium Thiocyanate Ionic Liquid Electrolyte Studied Using X-ray Photoelectron Spectroscopy, Langmuir, 2009, 25, 11955–11960 CrossRef CAS PubMed
.
- T. Torimoto, T. Tsuda, K.-I. Okazaki and S. Kuwabata, New Frontiers in Materials Science Opened by Ionic Liquids, Adv. Mater., 2010, 22, 1196–1221 CrossRef CAS PubMed
.
- V. Pfeifer, T. E. Jones, J. J. Velasco Vélez, R. Arrigo, S. Piccinin, M. Hävecker, A. Knop-Gericke and R. Schlögl,
In situ observation of reactive oxygen species forming on oxygen-evolving iridium surfaces, Chem. Sci., 2017, 8, 2143–2149 RSC
.
- D. Weingarth, A. Foelske-Schmitz, A. Wokaun and R. Kötz, In situ electrochemical XPS study of the Pt/[EMIM][BF4] system, Electrochem. Commun., 2011, 13, 619–622 CrossRef CAS
.
- R. Wibowo, L. Aldous, R. M. J. Jacobs, N. S. A. Manan and R. G. Compton,
In situ electrochemical-X-ray Photoelectron Spectroscopy: Rubidium metal deposition from an ionic liquid in competition with solvent breakdown, Chem. Phys. Lett., 2011, 517, 103–107 CrossRef CAS
.
- P. Gokturk, S. Donmez, B. Ulgut, Y. Turkmen and S. Suzer, Optical and XPS evidence for the electrochemical generation of an N-heterocyclic carbene and its CS2 adduct from the ionic liquid [bmim][PF6], New J. Chem., 2017, 41, 10299–10304 RSC
.
- P. Gokturk, U. Salzner, L. Nyulaszic, B. Ulgut, C. Kocabas and S. Suzer, XPS-evidence for in situ electrochemically-generated carbene formation, Electrochim. Acta, 2017, 234, 37–42 CrossRef
.
- M. Salmeron and R. Schlogl, Ambient pressure photoelectron spectroscopy: a new tool for surface science and nanotechnology, Surf. Sci. Rep., 2008, 63, 169–199 CrossRef CAS
.
- I. N. Dahmke, A. Verch, J. Hermannsdörfer, D. B. Peckys, R. S. Weatherup, S. Hofmann and N. de Jonge, Graphene liquid enclosure for single-molecule analysis of membrane proteins in whole cells using electron microscopy, ACS Nano, 2017, 11, 11108–11117 CrossRef CAS PubMed
.
- J. J. Velasco-Velez, V. Pfeifer, M. Hävecker, R. S. Weatherup, R. Arrigo, C. H. Chuang, E. Stotz, G. Weinberg, M. Salmeron and R. Schlögl, Photoelectron spectroscopy at the graphene–liquid interface reveals the electronic structure of an electrodeposited cobalt/graphene electrocatalyst, Angew. Chem., Int. Ed., 2015, 54, 14554–14558 CrossRef CAS PubMed
.
- R. S. Weatherup, B. Eren, Y. Hao, H. Bluhm and M. B. Salmeron, Graphene membranes for atmospheric pressure photoelectron spectroscopy, J. Phys. Chem. Lett., 2016, 7, 1622–1627 CrossRef CAS PubMed
.
- E. A. Carbonio, J.-J. Velasco-Velez, R. Schlögl and A. Knop-Gericke, Perspective—Outlook on Operando Photoelectron and Absorption Spectroscopy to Probe Catalysts at the Solid–Liquid Electrochemical Interface, J. Electrochem. Soc., 2020, 167, 054509 CrossRef CAS
.
- P. M. Dietrich, L. Gehrlein, J. Maibach and A. Thissen, Probing Lithium-Ion Battery Electrolytes with Laboratory Near-Ambient Pressure XPS, Crystals, 2020, 10, 1056 CrossRef CAS
.
- M. Ramstedt, C. Norgren, J. Sheals, A. Shchukarev and S. Sjöberg, Chemical speciation of N-(phosphonomethyl) glycine in solution and at mineral interfaces, Surf. Interface Anal., 2004, 36, 1074–1077 CrossRef CAS
.
- J. Škvarla, M. Kaňuchová, A. Shchukarev, A. Girová and I. Brezáni, Cryo-XPS–A new technique for the quantitative analysis of the structure of electric double layer at colloidal particles?, Colloids Surf., A, 2020, 586, 124234 CrossRef
.
- A. Shchukarev and M. Ramstedt, Cryo-XPS: probing intact interfaces in nature and life, Surf. Interface Anal., 2017, 49, 349–356 CrossRef CAS
.
- A. Shchukarev, J. Rosenqvist and S. Sjöberg, XPS study of the silica–water interface, J. Electron Spectrosc. Relat. Phenom., 2004, 137, 171–176 CrossRef
.
- Y. Mikhlin, A. Romanchenko and Y. Tomashevich, Surf. Interface Anal. of iron sulfides in aqueous media using X-ray photoelectron spectroscopy of fast-frozen dispersions, Appl. Surf. Sci., 2021, 549, 149261 CrossRef CAS
.
-
A. R. Boccaccini and P. X. Ma, Tissue Eng. Part A, Elsevier, 2014 Search PubMed
.
-
D. R. Baer, D. J. Cant, D. G. Castner, G. Ceccone, M. H. Engelhard, A. S. Karakoti and A. Müller, Characterization of Nanoparticles, Elsevier, 2020, pp. 295–347 Search PubMed
.
- A. Shchukarev and J.-F. Boily, XPS study of the hematite–aqueous solution interface, Surf. Interface Anal., 2008, 40, 349–353 CrossRef CAS
.
- A. Shchukarev, XPS at solid–aqueous solution interface, Adv. Colloid Interface Sci., 2006, 122, 149–157 CrossRef CAS PubMed
.
- M. Ramstedt, B. M. Andersson, A. Shchukarev and S. Sjöberg, Surface properties of hydrous manganite (γ-MnOOH). A potentiometric, electroacoustic, and X-ray photoelectron spectroscopy study, Langmuir, 2004, 20, 8224–8229 CrossRef CAS PubMed
.
- A. Shchukarev, XPS at solid–solution interface: experimental approaches, Surf. Interface Anal., 2006, 38, 682–685 CrossRef CAS
.
- Y. Mikhlin, X-ray photoelectron spectroscopy in mineral processing studies, Appl. Sci., 2020, 10, 5138 CrossRef CAS
.
- Y. Mikhlin, A. Karacharov, Y. Tomashevich and A. Shchukarev, Interaction of sphalerite with potassium n-butyl xanthate and copper sulfate solutions studied by XPS of fast-frozen samples and zeta-potential measurement, Vacuum, 2016, 125, 98–105 CrossRef CAS
.
- M. Ejtemaei and A. V. Nguyen, Characterisation of sphalerite and pyrite surfaces activated by copper sulphate, Miner. Eng., 2017, 100, 223–232 CrossRef CAS
.
- M. Ramstedt, R. Nakao, S. N. Wai, B. E. Uhlin and J.-F. Boily, Monitoring surface chemical changes in the bacterial cell wall: multivariate analysis of cryo-X-ray photoelectron spectroscopy data, J. Biol. Chem., 2011, 286, 12389–12396 CrossRef CAS PubMed
.
- M. Ramstedt, L. Leone, P. Persson and A. Shchukarev, Cell wall composition of Bacillus subtilis changes as a function of pH and Zn2+ exposure: insights from cryo-XPS measurements, Langmuir, 2014, 30, 4367–4374 CrossRef CAS PubMed
.
- A. Hagberg, O. Rzhepishevska, A. Semenets, D. A. Cisneros and M. Ramstedt, Surface analysis of bacterial systems using cryo-X-ray photoelectron spectroscopy, Surf. Interface Anal., 2020, 52, 792–801 CrossRef CAS
.
- A. Shchukarev, Z. Gojkovic, C. Funk and M. Ramstedt, Cryo-XPS analysis reveals surface composition of microalgae, Appl. Surf. Sci., 2020, 526, 146538 CrossRef CAS
.
- A. Shchukarev, E. Backman, S. Watts, S. Salentinig, C. F. Urban and M. Ramstedt, Applying Cryo-X-ray Photoelectron Spectroscopy to Study the Surface Chemical Composition of Fungi and Viruses, Front. Chem., 2021, 9, 666853 CrossRef CAS PubMed
.
- M. Kjærvik, M. Ramstedt, K. Schwibbert, P. M. Dietrich and W. E. Unger, Comparative study of NAP-XPS and cryo-XPS for the investigation of surface chemistry of the bacterial cell-envelope, Front. Chem., 2021, 9, 312 Search PubMed
.
- C. V. Cushman, P. Brüner, J. Zakel, G. H. Major, B. M. Lunt, N. J. Smith, T. Grehl and M. R. Linford, Low energy ion scattering (LEIS). A practical introduction to its theory, instrumentation, and applications, Anal. Methods, 2016, 8, 3419–3439 RSC
.
- S. Brown, Fowler, and Lauritsen, Phys. Rev., 1951, 82, 159 CrossRef
.
- S. Datz and C. Snoek, Large-angle, single-collision scattering of argon ions (40–80 keV) from metals, Phys. Rev., 1964, 134, A347 CrossRef
.
- D. P. Smith, Scattering of Low-Energy Noble Gas Ions from Metal Surfaces, J. Appl. Phys., 1967, 38, 340–347 CrossRef CAS
.
- D. P. Smith, Scattering of Low-Energy Noble Gas Ions from Metal Surfaces, J. Appl. Phys., 1967, 38, 340–347 CrossRef CAS
.
- M. Aizawa, S. Lee and S. L. Anderson, Deposition dynamics and chemical properties of size-selected Ir clusters on TiO2, Surf. Sci., 2003, 542, 253–275 CrossRef CAS
.
- H. Al-Kandari, A. Mohamed, F. Al-Kharafi and A. Katrib, XPS-UPS, ISS characterization studies and the effect of Pt and K addition on the catalytic properties of MoO2−x(OH)y deposited on TiO2, J. Electron Spectrosc. Relat. Phenom., 2011, 184, 472–478 CrossRef CAS
.
- H. Al-Kandari, A. Mohamed, F. Al-Kharafi and A. Katrib, Effect of Pt addition on the isomerization properties of MoO2−x(OH)y deposited on TiO2, Catal. Commun., 2011, 12, 1188–1192 CrossRef CAS
.
- P. Bharali, G. Thrimurthulu, L. Katta and B. M. Reddy, Preparation of highly dispersed and thermally stable nanosized cerium–hafnium solid solutions over silica surface: Structural and catalytic evaluation, J. Ind. Eng. Chem., 2012, 18, 1128–1135 CrossRef CAS
.
- C. T. Campbell, A. W. Grant, D. E. Starr, S. C. Parker and V. A. Bondzie, Model oxide-supported metal catalysts: energetics, particle thicknesses, chemisorption and catalytic properties, Top. Catal., 2000, 14, 43–51 CrossRef CAS
.
- S. Francis, J. Corneille, D. Goodman and M. Bowker, The adsorption and oxidation of methanol on thin palladium films, Surf. Sci., 1996, 364, 30–38 CrossRef CAS
.
- W. Grünert, A. Brückner, H. Hofmeister and P. Claus, Structural properties of Ag/TiO2 catalysts for acrolein hydrogenation, J. Phys. Chem. B, 2004, 108, 5709–5717 CrossRef
.
- J. Harmsen, W. Jansen, J. Hoebink, J. Schouten and H. Brongersma, Coke deposition on automotive three-way catalysts studied with LEIS, Catal. Lett., 2001, 74, 133–137 CrossRef CAS
.
- G. B. Hoflund, Z. Li, T. J. Campbell, W. S. Epling and H. W. Hahn, Catalytic Activity and Surface Characterization Study of Pd Supported on Nanocrystalline and Polycrystalline CeO2, MRS Online Proc. Libr., 1999, 581, 449–459 CrossRef
.
- T. E. James, S. L. Hemmingson, T. Ito and C. T. Campbell, Energetics of Cu adsorption and adhesion onto reduced CeO2(111) surfaces by calorimetry, J. Phys. Chem. C, 2015, 119, 17209–17217 CrossRef CAS
.
- W. Kaden, W. Kunkel and S. L. Anderson, Cluster size effects on sintering, CO adsorption, and implantation in Ir/SiO2, J. Chem. Phys., 2009, 131, 114701 CrossRef CAS PubMed
.
- W. E. Kaden, W. A. Kunkel, F. S. Roberts, M. Kane and S. L. Anderson, CO adsorption and desorption on size-selected Pdn/TiO2(110) model catalysts: size dependence of binding sites and energies, and support-mediated adsorption, J. Chem. Phys., 2012, 136, 204705 CrossRef PubMed
.
- W. E. Kaden, W. A. Kunkel, F. S. Roberts, M. Kane and S. L. Anderson, Thermal and adsorbate effects on the activity and morphology of size-selected Pdn/TiO2 model catalysts, Surf. Sci., 2014, 621, 40–50 CrossRef CAS
.
- M. D. Kane, F. S. Roberts and S. L. Anderson, Mass-selected supported cluster catalysts: Size effects on CO oxidation activity, electronic structure, and thermal stability of Pdn/alumina (n ≤ 30) model catalysts, Int. J. Mass Spectrom., 2014, 370, 1–15 CrossRef CAS
.
- A. Kohl, S. Labich, E. Taglauer and H. Knözinger, Agglomeration of supported rhodium on model catalysts, Surf. Sci., 2000, 454, 974–978 CrossRef
.
- C. Linsmeier and E. Taglauer, Strong metal–support interactions on rhodium model catalysts, Appl. Catal., A, 2011, 391, 175–186 CrossRef CAS
.
- F. Masini, P. Hernández-Fernández, D. Deiana, C. E. Strebel, D. N. McCarthy, A. Bodin, P. Malacrida, I. Stephens and I. Chorkendorff, Exploring the phase space of time of flight mass selected Pt x Y nanoparticles, Phys. Chem. Chem. Phys., 2014, 16, 26506–26513 RSC
.
- T. Matsumoto, M. Batzill, S. Hsieh and B. E. Koel, Fundamental studies of titanium oxide–Pt (1 0 0) interfaces: I. Stable high temperature structures formed by annealing TiOx films on Pt (1 0 0), Surf. Sci., 2004, 572, 127–145 CrossRef CAS
.
- T. Matsumoto, M. Batzill, S. Hsieh and B. E. Koel, Fundamental studies of titanium oxide-Pt (1 0 0) interfaces II. Influence of oxidation and reduction reactions on the surface structure of TiOx films on Pt (1 0 0), Surf. Sci., 2004, 572, 146–161 CrossRef CAS
.
- G. Mestl, C. Linsmeier, R. Gottschall, M. Dieterle, J. Find, D. Herein, J. Jäger, Y. Uchida and R. Schlögl, Molybdenum oxide based partial oxidation catalyst: 1. Thermally induced oxygen deficiency, elemental and structural heterogeneity and the relation to catalytic performance, J. Mol. Catal. A: Chem., 2000, 162, 463–492 CrossRef
.
- B. M. Reddy, P. Bharali, P. Saikia, S.-E. Park, M. W. van den Berg, M. Muhler and W. Grünert, Structural Characterization and Catalytic Activity of Nanosized CexM1−xO2 (M = Zr and Hf) Mixed Oxides, J. Phys. Chem. C, 2008, 112, 11729–11737 CrossRef CAS
.
- B. M. Reddy, L. Katta and G. Thrimurthulu, Novel nanosized CexZr1−xO2, CexHf1−xO2 and CexTb1−xO2−δ solid solutions: structural characteristics and catalytic performance, Catal. Today, 2011, 175, 585–592 CrossRef CAS
.
- B. M. Reddy, L. Katta and G. Thrimurthulu, Novel nanosized CexZr1−xO2, CexHf1−xO2 and CexTb1−xO2−δ solid solutions: Structural characteristics and catalytic performance, Catal. Today, 2011, 175, 585–592 CrossRef CAS
.
- B. M. Reddy, P. Saikia, P. Bharali, S.-E. Park, M. Muhler and W. Grünert, Physicochemical Characteristics and Catalytic Activity of Alumina-Supported Nanosized Ceria–Terbia Solid Solutions, J. Phys. Chem. C, 2009, 113, 2452–2462 CrossRef CAS
.
- J. N. Riedel, M. D. Rötzer, M. Jørgensen, U. G. Vej-Hansen, T. Pedersen, B. Sebok, F. F. Schweinberger, P. C. K. Vesborg, O. Hansen and J. Schiøtz, H2/D2 exchange reaction on mono-disperse Pt clusters: enhanced activity from minute O2 concentrations, Catal. Sci. Technol., 2016, 6, 6893–6900 RSC
.
- F. S. Roberts, M. D. Kane, E. T. Baxter and S. L. Anderson, Oxygen activation and CO oxidation over size-selected Pt n/alumina/Re(0001) model catalysts: correlations with valence electronic structure, physical structure, and binding sites, Phys. Chem. Chem. Phys., 2014, 16, 26443–26457 RSC
.
- S. J. Scierka, M. Houalla, A. Proctor and D. M. Hercules, Dispersion and coverage of Cr/Al2O3 catalysts, J. Phys. Chem., 1995, 99, 1537–1542 CrossRef CAS
.
- M. Shen, Q. Ma, I. Lee and F. Zaera, Oxygen adsorption and oxide formation on V(100) surfaces, J. Phys. Chem. C, 2007, 111, 6033–6040 CrossRef CAS
.
- Z. Song, X. Bao, U. Wild, M. Muhler and G. Ertl, Oxidation of amorphous Ni–Zr alloys studied by XPS, UPS, ISS and XRD, Appl. Surf. Sci., 1998, 134, 31–38 CrossRef CAS
.
- G. H. Vurens, V. Maurice, M. Salmeron and G. A. Somorjai, Growth, structure and chemical properties of FeO overlayers on Pt(100) and Pt(111), Surf. Sci., 1992, 268, 170–178 CrossRef CAS
.
- W. Weiss and W. Ranke, Surface chemistry and catalysis on well-defined epitaxial iron-oxide layers, Prog. Surf. Sci., 2002, 70, 1–151 CrossRef CAS
.
- J. Yoshihara and C. Campbell, Chemisorption of formic acid and CO on Cu particles on the Zn-terminated ZnO(0001) surface, Surf. Sci., 1998, 407, 256–267 CrossRef CAS
.
- F. Zaera, N. R. Gleason, B. Klingenberg and A. H. Ali, Partial oxidation of hydrocarbons on nickel: from surface science mechanistic studies to catalysis, J. Mol. Catal. A: Chem., 1999, 146, 13–23 CrossRef CAS
.
- D. Y. Zemlyanov, A. Nagy and R. Schlögl, The reaction of silver with NO/O2, Appl. Surf. Sci., 1998, 133, 171–183 CrossRef CAS
.
- H. Ziaei-azad, C.-X. Yin, J. Shen, Y. Hu, D. Karpuzov and N. Semagina, Size-and structure-controlled mono-and bimetallic Ir–Pd nanoparticles in selective ring opening of indan, J. Catal., 2013, 300, 113–124 CrossRef CAS
.
- A. Arranz and C. Palacio, The room temperature growth of Ti on sputter-cleaned Si(1 0 0): Composition and nanostructure of the interface, Surf. Sci., 2005, 588, 92–100 CrossRef CAS
.
- N. A. Khan and C. Matranga, Nucleation and growth of Fe and FeO nanoparticles and films on Au(1 1 1), Surf. Sci., 2008, 602, 932–942 CrossRef CAS
.
- C. Palacio and A. Arranz, Spectroscopic characterization of the surface nanostructure of Ti during deposition on polycrystalline aluminium, Surf. Interface Anal., 1999, 27, 871–879 CrossRef CAS
.
- V. A. Thampi, A. Bendavid and B. Subramanian, Nanostructured TiCrN thin films by Pulsed Magnetron Sputtering for cutting tool applications, Ceram. Int., 2016, 42, 9940–9948 CrossRef CAS
.
- S. Thanka Rajan, A. K. Nandakumar, T. Hanawa and B. Subramanian, Materials properties of ion beam sputtered Ti–Cu–Pd–Zr thin film metallic glasses, J. Non-Cryst. Solids, 2017, 461, 104–112 CrossRef CAS
.
- V. Jimenez, J. Espinos and A. Gonzalez-Elipe, Interface effects for metal oxide thin films deposited on another metal oxide III. SnO and SnO2 deposited on MgO(100) and the use of chemical state plots, Surf. Sci., 1996, 366, 556–563 CrossRef CAS
.
- V. Jimenez, A. Fernandez, J. Espinos and A. González-Elipe, Interface effects for metal oxide thin films deposited on another metal oxide I. SnO deposited on SiO2, Surf. Sci., 1996, 350, 123–135 CrossRef CAS
.
- V. Jimenez, J. Mejias, J. Espinos and A. Gonzalez-Elipe, Interface effects for metal oxide thin films deposited on another metal oxide II. SnO2 deposited on SiO2, Surf. Sci., 1996, 366, 545–555 CrossRef CAS
.
- M. W. Herdiech, A. Kakekhani, X. Zhu, S. Ismail-Beigi and E. I. Altman, Growth of ultrathin Ru oxide films on perovskite and corundum substrates, Surf. Sci., 2019, 688, 51–62 CrossRef CAS
.
- S. T. Rajan, A. Nandakumar, T. Hanawa and B. Subramanian, Materials properties of ion beam sputtered Ti–Cu–Pd–Zr thin film metallic glasses, J. Non-Cryst. Solids, 2017, 461, 104–112 CrossRef
.
- R. Schennach, S. Promreuk, D. G. Naugle and D. L. Cocke, Thermal Electrochemical, and Plasma Oxidation of Ti–50Zr, Cu–50Zr, Cu–50Ti, and Cu–33Ti–33Zr Alloys, Oxid. Met., 2001, 55, 523–541 CrossRef CAS
.
- V. A. Thampi, U. Nithiyanantham, A. N. Kumar, P. Martin, A. Bendavid and B. Subramanian, Fabrication of sputtered titanium vanadium nitride (TiVN) thin films for micro-supercapacitors, J. Mater. Sci.: Mater. Electron., 2018, 29, 12457–12465 CrossRef
.
- T. Skala, K. Veltruska, M. Moroseac, I. Matolınová, G. Korotchenkov and V. Matolın, Study of Pd–In interaction during Pd deposition on pyrolytically prepared In2O3, Appl. Surf. Sci., 2003, 205, 196–205 CrossRef CAS
.
- J. Yoshihara, J. Campbell and C. Campbell, Cu films on a Zn-terminated ZnO(0001) surface: structure and electronic properties, Surf. Sci., 1998, 406, 235–245 CrossRef CAS
.
- G. Nelson, Influence of surface roughness on the intensity of elastically scattered low-energy noble-gas ions, J. Appl. Phys., 1976, 47, 1253–1255 CrossRef CAS
.
- W. Jansen, A. Knoester, A. Maas, P. Schmit, A. Kytökivi, A. Denier vd Gon and H. Brongersma, Influence of compaction and surface roughness on low-energy ion scattering signals, Surf. Interface Anal., 2004, 36, 1469–1478 CrossRef CAS
.
- W. Gruenert, R. Schloegl and H. G. Karge, Investigations of zeolites by photoelectron and ion scattering spectroscopy. 1. New applications of surface spectroscopic methods to zeolites by a high-temperature measurement technique, J. Phys. Chem., 1993, 97, 8638–8645 CrossRef CAS
.
- E. Cartier, P. Pfluger, J.-J. Pireaux and M. R. Vilar, Mean-free paths and scattering processes for 0.1–4500 eV electrons in saturated hydrocarbon films, Appl. Phys. A: Mater. Sci. Process., 1987, 44, 43–53 CrossRef
.
- S. Zhao and R. J. Gorte, The effect of oxide dopants in ceria on n-butane oxidation, Appl. Catal., A, 2003, 248, 9–18 CrossRef CAS
.
- B. G. Mishra and G. R. Rao, Promoting effect of ceria on the physicochemical and catalytic properties of CeO2–ZnO composite oxide catalysts, J. Mol. Catal. A: Chem., 2006, 243, 204–213 CrossRef CAS
.
- P. Scanlon, R. Bink, F. Van Berkel, G. Christie, L. Van Ijzendoorn, H. Brongersma and R. Van Welzenis, Surface composition of ceramic CeGd–oxide, Solid State Ion., 1998, 112, 123–130 CrossRef CAS
.
- O. Šik, P. Bábor, J. Polčák, E. Belas, P. Moravec, L. Grmela and J. Staněk, Low energy ion scattering as a depth profiling tool for thin layers – Case of bromine methanol etched CdTe, Vacuum, 2018, 152, 138–144 CrossRef
.
- A. Stassi, I. Gatto, G. Monforte, V. Baglio, E. Passalacqua, V. Antonucci and A. Aricò, The effect of thermal treatment on structure and surface composition of PtCo electro-catalysts for application in PEMFCs operating under automotive conditions, J. Power Sources, 2012, 208, 35–45 CrossRef CAS
.
- M. Wu and D. M. Hercules, Studies of supported nickel catalysts by X-ray photoelectron and ion scattering spectroscopies, J. Phys. Chem., 1979, 83, 2003–2008 CrossRef CAS
.
- P. Chappell, M. Kibel and B. Baker, X-ray photoelectron and ion scattering studies of tungsten oxides and tungsten oxide catalysts, J. Catal., 1988, 110, 139–149 CrossRef CAS
.
- W. Wallace, Q. Zhong, J. Genzer, R. Composto and D. Bonnell, On the use of ion scattering to examine the role of hydrogen in the reduction of TiO2, J. Mater. Res., 1993, 8, 1629–1634 CrossRef CAS
.
- J. M. Pan, B. Maschhoff, U. Diebold and T. Madey, Interaction of water, oxygen, and hydrogen with TiO2(110) surfaces having different defect densities, J. Vac. Sci. Technol., A, 1992, 10, 2470–2476 CrossRef CAS
.
- J. Campos-Delgado, Y. Kim, T. Hayashi, A. Morelos-Gómez, M. Hofmann, H. Muramatsu, M. Endo, H. Terrones, R. Shull and M. Dresselhaus, Thermal stability studies of CVD-grown graphene nanoribbons: defect annealing and loop formation, Chem. Phys. Lett., 2009, 469, 177–182 CrossRef CAS
.
- S. P. Jiang, A review of wet impregnation—an alternative method for the fabrication of high performance and nano-structured electrodes of solid oxide fuel cells, Mater. Sci. Eng., 2006, 418, 199–210 CrossRef
.
- M. J. Taylor, L. J. Durndell, M. A. Isaacs, C. M. Parlett, K. Wilson, A. F. Lee and G. Kyriakou, Highly selective hydrogenation of furfural over supported Pt nanoparticles under mild conditions, Appl. Catal., B, 2016, 180, 580–585 CrossRef CAS
.
- O. Dulub, W. Hebenstreit and U. Diebold, Imaging cluster surfaces with atomic resolution: the strong metal-support interaction state of Pt supported on TiO2(110), Phys. Rev. Lett., 2000, 84, 3646 CrossRef CAS PubMed
.
- H. Tang, Y. Su, B. Zhang, A. F. Lee, M. A. Isaacs, K. Wilson, L. Li, Y. Ren, J. Huang and M. Haruta, Classical strong metal–support interactions between gold nanoparticles and titanium dioxide, Sci. Adv., 2017, 3, e1700231 CrossRef PubMed
.
- E. T. Baxter, M.-A. Ha, A. C. Cass, A. N. Alexandrova and S. L. Anderson, Ethylene dehydrogenation on Pt4, 7, 8 clusters on Al2O3: strong cluster size dependence linked to preferred catalyst morphologies, ACS Catal., 2017, 7, 3322–3335 CrossRef CAS
.
- E. T. Baxter, M.-A. Ha, A. C. Cass, H. Zhai, A. N. Alexandrova and S. L. Anderson, Diborane Interactions with Pt7/Alumina: Preparation of Size-Controlled Borated Pt Model Catalysts, J. Phys. Chem. C, 2018, 122, 1631–1644 CrossRef CAS
.
- O. Meyer, J. Gyulai and J. W. Mayer, Analysis of amorphous
layers on silicon by backscattering and channeling effect measurements, Surf. Sci., 1970, 22, 263–276 CrossRef CAS
.
- V. M. Jimenez, J. Espinos, A. Fernandez and A. R. Gonzalez-Elipe, Surf. Sci., 1996, 350, 123 CrossRef CAS
.
- Y. Liu, C. M. Hangarter, D. Garcia and T. P. Moffat, Self-terminating electrodeposition of ultrathin Pt films on Ni: an active, low-cost electrode for H2 production, Surf. Sci., 2015, 631, 141–154 CrossRef CAS
.
- U. Diebold, J.-M. Pan and T. Madey, Growth mode of ultrathin copper overlayers on TiO2(110), Phys. Rev. B: Condens. Matter Mater. Phys., 1993, 47, 3868 CrossRef CAS PubMed
.
- T. Richard, I. Furno, A. Sublet and M. Taborelli, Influence of ion-induced secondary electron emission parameters in PICMC plasma simulations with experimental validation in DC cylindrical diode and magnetron discharges, Plasma Sources Sci. Technol., 2020, 29, 095003 CrossRef CAS
.
- T. Nagatomi, T. Kuwayama, Y. Takai, K. Yoshino, Y. Morita, M. Kitagawa and M. Nishitani, Application of ion scattering spectroscopy to measurement of surface potential of MgO thin film under ion irradiation, Appl. Phys. Lett., 2008, 92, 084104 CrossRef
.
- T. Nagatomi, T. Kuwayama, K. Yoshino, Y. Takai, Y. Morita, M. Nishitani and M. Kitagawa,
In situ measurement of surface potential developed on MgO thin film surface under ion irradiation using ion scattering spectroscopy, J. Appl. Phys., 2009, 106, 104912 CrossRef
.
-
J. H. D. Eland, Photoelectron spectroscopy: an introduction to ultraviolet photoelectron spectroscopy in the gas phase, Elsevier, 2013 Search PubMed
.
- O. Cheshnovsky, S. Yang, C. Pettiette, M. Craycraft, Y. Liu and R. Smalley, Ultraviolet photoelectron spectroscopy of semiconductor clusters: silicon and germanium, Chem. Phys. Lett., 1987, 138, 119–124 CrossRef
.
- J. Lin, C. Yu, S. Peng, I. Akiyama, K. Li, L. K. Lee and P. LeBreton, Ultraviolet photoelectron studies of the ground-state electronic structure and gas-phase tautomerism of purine and adenine, J. Am. Chem. Soc., 1980, 102, 4627–4631 CrossRef CAS
.
- G. Greczynski, T. Kugler, M. Keil, W. Osikowicz, M. Fahlman and W. R. Salaneck, Photoelectron spectroscopy of thin films of PEDOT–PSS conjugated polymer blend: a mini-review and some new results, J. Electron Spectrosc. Relat. Phenom., 2001, 121, 1–17 CrossRef CAS
.
- W. R. Salaneck and M. Fahlman, Hybrid interfaces of conjugate polymers: Band edge alignment studied by ultraviolet photoelectron spectroscopy, J. Mater. Res., 2004, 19, 1917–1923 CrossRef CAS
.
- A.-S. Duwez, Exploiting electron spectroscopies to probe the structure and organization of self-assembled monolayers: a review, J. Electron Spectrosc. Relat. Phenom., 2004, 134, 97–138 CrossRef CAS
.
- D. W. Goodman, Model studies in catalysis using surface science probes, Chem. Rev., 1995, 95, 523–536 CrossRef CAS
.
- F. Zaera, Probing catalytic reactions at surfaces, Prog. Surf. Sci., 2001, 69, 1–98 CrossRef CAS
.
- P. L. Gunter, J. Niemantsverdriet, F. H. Ribeiro and G. A. Somorjai, Surface science approach to modeling supported catalysts, Catal. Rev., 1997, 39, 77–168 CrossRef CAS
.
- R. Leckey and J. Riley, Semiconductor band structure as determined from angle resolved photoelectron spectroscopy, Crit. Rev. Solid State Mater. Sci., 1992, 17, 307–352 CrossRef CAS
.
- J.-P. Yang, F. Bussolotti, S. Kera and N. Ueno, Origin and role of gap states in organic semiconductor studied by UPS: as the nature of organic molecular crystals, J. Phys. D: Appl. Phys., 2017, 50, 423002 CrossRef
.
- F. S. Roberts, S. L. Anderson, A. C. Reber and S. N. Khanna, Initial and Final State Effects in the Ultraviolet and X-ray Photoelectron Spectroscopy (UPS and XPS) of Size-Selected Pdn Clusters Supported on TiO2(110), J. Phys. Chem. C, 2015, 119, 6033–6046 CrossRef CAS
.
- S. Hüfner, G. Wertheim, N. Smith and M. Traum, XPS density of states of copper, silver, and nickel, Solid State Commun., 1972, 11, 323–326 CrossRef
.
- P. Oelhafen, M. Liard, H.-J. Güntherodt, K. Berresheim and H. Polaschegg, Photoemission (XPS, UPS) studies of Pd-Si metallic glasses, Solid State Commun., 1979, 30, 641–644 CrossRef CAS
.
- A. Kahn, Fermi level, work function and vacuum level, Mater. Horiz., 2016, 3, 7–10 RSC
.
- J. Rowe, S. Christman and E. Chaban, Ultrahigh-vacuum hollow-cathode resonance lamp for He ii (40.8 eV) photoemission, Rev. Sci. Instrum., 1985, 56, 759–761 CrossRef CAS
.
- J. Sitte, Relation between reference levels, work functions and contact potential differences in photoelectron spectroscopy, Chem. Phys. Lett., 1976, 42, 131–132 CrossRef CAS
.
- M. Helander, M. Greiner, Z. Wang and Z. Lu, Pitfalls in measuring work function using photoelectron spectroscopy, Appl. Surf. Sci., 2010, 256, 2602–2605 CrossRef CAS
.
- S. Axnanda, M. Scheele, E. Crumlin, B. Mao, R. Chang, S. Rani, M. Faiz, S. Wang, A. P. Alivisatos and Z. Liu, Direct work function measurement by gas phase photoelectron spectroscopy and its application on PbS nanoparticles, Nano Lett., 2013, 13, 6176–6182 CrossRef CAS PubMed
.
- M. Bär, B.-A. Schubert, B. Marsen, S. Krause, S. Pookpanratana, T. Unold, L. Weinhardt, C. Heske and H.-W. Schock, Impact of KCN etching on the chemical and electronic surface structure of Cu2ZnSnS4 thin-film solar cell absorbers, Appl. Phys. Lett., 2011, 99, 152111 CrossRef
.
- W.-J. Chun, A. Ishikawa, H. Fujisawa, T. Takata, J. N. Kondo, M. Hara, M. Kawai, Y. Matsumoto and K. Domen, Conduction and valence band positions of Ta2O5, TaON, and Ta3N5 by UPS and electrochemical methods, J. Phys. Chem. B, 2003, 107, 1798–1803 CrossRef CAS
.
- R. C. Shallcross, Y. Zheng, S. S. Saavedra and N. R. Armstrong, Determining Band-Edge Energies and Morphology-Dependent Stability of Formamidinium Lead Perovskite Films Using Spectroelectrochemistry and Photoelectron Spectroscopy, J. Am. Chem. Soc., 2017, 139, 4866–4878 CrossRef CAS PubMed
.
- R. Trofimovaite, C. M. Parlett, S. Kumar, L. Frattini, M. A. Isaacs, K. Wilson, L. Olivi, B. Coulson, J. Debgupta and R. E. Douthwaite, Single atom Cu(I) promoted mesoporous titanias for photocatalytic Methyl Orange depollution and H2 production, Appl. Catal., B, 2018, 232, 501–511 CrossRef CAS
.
- M. Grätzel, Dye-sensitized solar cells, J. Photochem. Photobiol., C, 2003, 4, 145–153 CrossRef
.
- G. K. Mor, M. A. Carvalho, O. K. Varghese, M. V. Pishko and C. A. Grimes, A room-temperature TiO2-nanotube hydrogen sensor able to self-clean photoactively from environmental contamination, J. Mater. Res., 2004, 19, 628–634 CrossRef CAS
.
- P. Makuła, M. Pacia and W. Macyk, How to correctly determine the band gap energy of modified semiconductor photocatalysts based on UV-Vis spectra, J. Phys. Chem. Lett., 2018, 9, 6814–6817 CrossRef PubMed
.
- C. Maheu, L. Cardenas, E. Puzenat, P. Afanasiev and C. Geantet, UPS and UV spectroscopies combined to position the energy levels of TiO2 anatase and rutile nanopowders, Phys. Chem. Chem. Phys., 2018, 20, 25629–25637 RSC
.
- D. O. Scanlon, C. W. Dunnill, J. Buckeridge, S. A. Shevlin, A. J. Logsdail, S. M. Woodley, C. R. A. Catlow, M. J. Powell, R. G. Palgrave, I. P. Parkin, G. W. Watson, T. W. Keal, P. Sherwood, A. Walsh and A. A. Sokol, Band alignment of rutile and anatase TiO2, Nat. Mater., 2013, 12, 798–801 CrossRef CAS PubMed
.
- M. Li, W. Hebenstreit, U. Diebold, A. M. Tyryshkin, M. K. Bowman, G. G. Dunham and M. A. Henderson, The Influence of the Bulk Reduction State on the Surface Structure and Morphology of Rutile TiO2(110) Single Crystals, J. Phys. Chem. B, 2000, 104, 4944–4950 CrossRef CAS
.
- X. Li, Z. Zhang and V. E. Henrich, Inelastic electron background function for ultraviolet photoelectron spectra, J. Electron Spectrosc. Relat. Phenom., 1993, 63, 253–265 CrossRef CAS
.
- L. Diederich, O. Küttel, P. Aebi and L. Schlapbach, Electron affinity and work function of differently oriented and doped diamond surfaces determined by photoelectron spectroscopy, Surf. Sci., 1998, 418, 219–239 CrossRef CAS
.
- C. Vayenas, S. Bebelis and S. Ladas, Dependence of catalytic rates on catalyst work function, Nature, 1990, 343, 625–627 CrossRef CAS
.
- W. Sachtler and R. Jongepier, The surface of copper-nickel alloy films: II. Phase equilibrium and distribution and their implications for work function, chemisorption, and catalysis, J. Catal., 1965, 4, 665–671 CrossRef CAS
.
- S. Wang, Y. Guan, L. Wang, W. Zhao, H. He, J. Xiao, S. Yang and C. Sun, Fabrication of a novel bifunctional material of BiOI/Ag3VO4 with high adsorption–photocatalysis for efficient treatment of dye wastewater, Appl. Catal., B, 2015, 168, 448–457 CrossRef
.
- L. Wang, J. Ge, A. Wang, M. Deng, X. Wang, S. Bai, R. Li, J. Jiang, Q. Zhang and Y. Luo, Designing p-type semiconductor–metal hybrid structures for improved photocatalysis, Angew. Chem., 2014, 126, 5207–5211 CrossRef
.
-
G. Gu and L. S. Pan, Nanotube chemical sensor based on work function of electrodes, US Pat., 7052588, 2006 Search PubMed
.
- T. A. Dam, D. Pijanowska, W. Olthuis and P. Bergveld, Highly sensitive glucose sensor based on work function changes measured by an E MOSFET, Analyst, 2003, 128, 1062–1066 RSC
.
- M. Timpel, M. V. Nardi, S. Krause, G. Ligorio, C. Christodoulou, L. Pasquali, A. Giglia, J. Frisch, B. Wegner and P. Moras, Surface modification of ZnO (0001)–Zn with phosphonate-based self-assembled monolayers: Binding modes, orientation, and work function, Chem. Mater., 2014, 26, 5042–5050 CrossRef CAS
.
- P. Stracke, S. Krischok and V. Kempter, Ag-adsorption on MgO: investigations with MIES and UPS, Surf. Sci., 2001, 473, 86–96 CrossRef CAS
.
- Y. Nakayama, S. Kera and N. Ueno, Photoelectron spectroscopy on single crystals of organic semiconductors: Experimental electronic band structure for optoelectronic properties, J. Mater. Chem. C, 2020, 9, 9090–9132 RSC
.
- S.-i. Machida, Y. Nakayama, S. Duhm, Q. Xin, A. Funakoshi, N. Ogawa, S. Kera, N. Ueno and H. Ishii, Highest-occupied-molecular-orbital band dispersion of rubrene single crystals as observed by angle-resolved ultraviolet photoelectron spectroscopy, Phys. Rev. Lett., 2010, 104, 156401 CrossRef PubMed
.
- Y. Nakayama, Y. Mizuno, M. Hikasa, M. Yamamoto, M. Matsunami, S. Ideta, K. Tanaka, H. Ishii and N. Ueno, Single-crystal pentacene valence-band dispersion and its temperature dependence, J. Phys. Chem. Lett., 2017, 8, 1259–1264 CrossRef CAS PubMed
.
- J. Taborski, P. Väterlein, H. Dietz, U. Zimmermann and E. Umbach, NEXAFS investigations on ordered adsorbate layers of large aromatic molecules, J. Electron Spectrosc. Relat. Phenom., 1995, 75, 129–147 CrossRef CAS
.
- T. Gustafsson, E. W. Plummer, D. E. Eastman and J. L. Freeouf, Interpretation of the photoelectron spectra of molecularly adsorbed CO, Solid State Commun., 1975, 17, 391–396 CrossRef CAS
.
- J. Demuth and D. Eastman, Photoemission Observations of π− d Bonding and Surface Reactions of Adsorbed Hydrocarbons on Ni(111), Phys. Rev. Lett., 1974, 32, 1123 CrossRef CAS
.
- E. Plummer, B. Waclawski and T. V. Vorburger, Photoelectron spectra of the decomposition of ethylene on (110) tungsten, Chem. Phys. Lett., 1974, 28, 510–515 CrossRef CAS
.
- E. Plummer, W. Salaneck and J. Miller, Photoelectron spectra of transition-metal carbonyl complexes: comparison with the spectra of adsorbed CO, Phys. Rev. B: Condens. Matter Mater. Phys., 1978, 18, 1673 CrossRef CAS
.
- G. Apai, P. Wehner, R. Williams, J. Stöhr and D. Shirley, Orientation of CO on Pt(111) and Ni(111) surfaces from angle-resolved photoemission, Phys. Rev. Lett., 1976, 37, 1497 CrossRef CAS
.
- F. Himpsel and T. Fauster, Empty orbitals of adsorbates determined by inverse ultraviolet photoemission, Phys. Rev. Lett., 1982, 49, 1583 CrossRef CAS
.
- A. Cassuto, M. Mane, M. Hugenschmidt, P. Dolle and J. Jupille, The effect of K, Cs and O atoms on ethylene adsorption on the Pt(111) surface, Surf. Sci., 1990, 237, 63–71 CrossRef CAS
.
- M. Hugenschmidt, P. Dolle, J. Jupille and A. Cassuto, Ethylene π species on bare and cesiated pt(111) surfaces, J. Vac. Sci. Technol., A, 1989, 7, 3312–3316 CrossRef CAS
.
- R. Windham, M. Bartram and B. E. Koel, Coadsorption of ethylene and potassium on platinum (111). 1. Formation of a. pi.-bonded state of ethylene, J. Phys. Chem., 1988, 92, 2862–2870 CrossRef CAS
.
- H. Luftman, Y.-M. Sun and J. White, Coadsorption of CO and K on Ni(100): II. XPS, UPS, Δφ and ELS studies, Surf. Sci., 1984, 141, 82–100 CrossRef CAS
.
- P. Angevaare, H. Hendrickx and V. Ponec, Coadsorption and promoter effect in the adsorption of CO on palladium catalysts, J. Catal., 1988, 110, 11–17 CrossRef CAS
.
- H. Praliaud, J. Dalmon, C. Mirodatos and G. Martin, Influence of potassium salt addition on the catalytic properties of silica-supported nickel, J. Catal., 1986, 97, 344–356 CrossRef CAS
.
- M. Kiskinova, G. Pirug and H. Bonzel, Coadsorption of potassium and CO on Pt(111), Surf. Sci., 1983, 133, 321–343 CrossRef CAS
.
- M. Kiskinova, G. Pirug and H. Bonzel, NO adsorption on Pt(111), Surf. Sci., 1984, 136, 285–295 CrossRef CAS
.
- C. Brundle, Oxygen adsorption and thin oxide formation at iron surfaces: An XPS/UPS study, Surf. Sci., 1977, 66, 581–595 CrossRef CAS
.
- A. Boronin, V. Bukhityarov, A. Vishnevskii, G. Boreskov and V. Savchenko, XPS and UPS studies of oxygen adsorption over clean and carbon-modified silver surfaces, Surf. Sci., 1988, 201, 195–210 CrossRef CAS
.
- F. Matsui, H. Yeoim, A. Imanishi, K. Isawa, I. Matsuda and T. Ohta, Adsorption of acetylene and ethylene on the Si(001) 2 × 1 surface studied by NEXAFS and UPS, Surf. Sci., 1998, 401, L413–L419 CrossRef CAS
.
- C. Au, W. Hirsch and W. Hirschwald, Adsorption of carbon monoxide and carbon dioxide on annealed and defect zinc oxide (0001) surfaces studied by photoelectron spectroscopy (XPS and UPS), Surf. Sci., 1988, 197, 391–401 CrossRef CAS
.
- Y.-M. Sun, D. Sloan, D. Alberas, M. Kovar, Z.-J. Sun and J. White, SO2 adsorption on Pt(111): HREELS, XPS and UPS study, Surf. Sci., 1994, 319, 34–44 CrossRef CAS
.
- B. Adolphi, E. Jähne, G. Busch and X. Cai, Characterization of the adsorption of ω-(thiophene-3-yl alkyl) phosphonic acid on metal oxides with AR-XPS, Anal. Bioanal. Chem., 2004, 379, 646–652 CrossRef CAS PubMed
.
- M. Brückner, B. Heinz and H. Morgner, Molecular orientation in organic monolayers probed by UPS and MIES (metastable induced electron spectroscopy), Surf. Sci., 1994, 319, 370–380 CrossRef
.
- B. Heinz and H. Morgner, MIES investigation of alkanethiol monolayers self-assembled on Au(111) and Ag(111) surfaces, Surf. Sci., 1997, 372, 100–116 CrossRef CAS
.
- C. Zubrägel, F. Schneider, M. Neumann, G. Hähner, C. Wöll and M. Grunze, Electronic structure of alkane chains. Complete one-dimensional band structures of the valence states, Chem. Phys. Lett., 1994, 219, 127–131 CrossRef
.
- A.-S. Duwez, J. Riga, B.-Y. Han and J. Delhalle, Probing the surface molecular structure in the UPS spectra of octadecanethiol and 1-cyclohexyl-12-dodecanethiol self-assembled on gold, J. Electron Spectrosc. Relat. Phenom., 1996, 81, 55–61 CrossRef CAS
.
- K. Seki and H. Inokuchi, Angular-and light-polarization-dependent valence UV photoelectron spectra of the hexatriacontane (n-C36H74) crystal, Chem. Phys. Lett., 1982, 89, 268–272 CrossRef CAS
.
- A.-S. Duwez, G. Pfister-Guillouzo, J. Delhalle and J. Riga, Probing organization and structural characteristics of alkanethiols adsorbed on gold and of model alkane compounds through their valence electronic structure: an ultraviolet photoelectron spectroscopy study, J. Phys. Chem. B, 2000, 104, 9029–9037 CrossRef CAS
.
- J. Stultz, S. Krischok and D. Goodman, Orientation determination of 2-and 4-chlorobenzylmercaptan self-assembled monolayers using metastable impact electron spectroscopy, Langmuir, 2002, 18, 2962–2963 CrossRef CAS
.
- C. C. Chang, Auger electron spectroscopy, Surf. Sci., 1971, 25, 53–79 CrossRef CAS
.
- T. Haas, J. Grant and G. Dooley Iii, Chemical effects in Auger electron spectroscopy, J. Appl. Phys., 1972, 43, 1853–1860 CrossRef CAS
.
- P. Holloway, Thickness determination of ultrathin films by Auger electron spectroscopy, J. Vac. Sci. Technol., 1975, 12, 1418–1422 CrossRef
.
- J. Ong and L. Lucas, Auger electron spectroscopy and its use for the characterization of titanium and hydroxyapatite surfaces, Biomaterials, 1998, 19, 455–464 CrossRef CAS PubMed
.
- S. Shivaraman, M. Chandrashekhar, J. J. Boeckl and M. G. Spencer, Thickness estimation of epitaxial graphene on SiC using attenuation of substrate Raman intensity, J. Electron. Mater., 2009, 38, 725–730 CrossRef CAS
.
- A. Reina, S. Thiele, X. Jia, S. Bhaviripudi, M. S. Dresselhaus, J. A. Schaefer and J. Kong, Growth of large-area single-and bi-layer graphene by controlled carbon precipitation on polycrystalline Ni surfaces, Nano Res., 2009, 2, 509–516 CrossRef CAS
.
- D. Graf, F. Molitor, K. Ensslin, C. Stampfer, A. Jungen, C. Hierold and L. Wirtz, Spatially resolved Raman spectroscopy of single-and few-layer graphene, Nano Lett., 2007, 7, 238–242 CrossRef CAS PubMed
.
- M. Xu, D. Fujita, J. Gao and N. Hanagata, Auger electron spectroscopy: a rational method for determining thickness of graphene films, ACS Nano, 2010, 4, 2937–2945 CrossRef CAS PubMed
.
- J. Biberian and G. Somorjai, On the determination of monolayer coverage by Auger electron spectroscopy. Application to carbon on platinum, Appl. Surf. Sci., 1979, 2, 352–358 CrossRef CAS
.
- P. Beccat, J. Bertolini, Y. Gauthier, J. Massardier and P. Ruiz, Crotonaldehyde and methylcrotonaldehyde hydrogenation over Pt(111) and Pt80Fe20(111) single crystals, J. Catal., 1990, 126, 451–456 CrossRef CAS
.
- G. Rhead, M.-G. Barthes and C. Argile, Determination of growth modes of ultrathin films from Auger electron spectroscopy: an assessment and commentary, Thin Solid Films, 1981, 82, 201–211 CrossRef CAS
.
- F. Reniers and C. Tewell, New improvements in energy and spatial (x, y, z) resolution in AES and XPS applications, J. Electron Spectrosc. Relat. Phenom., 2005, 142, 1–25 CrossRef CAS
.
- C. V. Cushman, S. Chatterjee, G. H. Major, N. J. Smith, A. Roberts and M. R. Linford, Trends in Advanced XPS Instrumentation, Vac. Technol. Coat., 2017, 9, 25–31 Search PubMed
.
- M. C. Biesinger, L. W. M. Lau, A. R. Gerson and R. S. C. Smart, The role of the Auger parameter in XPS studies of nickel metal, halides and oxides, Phys. Chem. Chem. Phys., 2012, 14, 2434–2442 RSC
.
- M. C. Biesinger, Advanced analysis of copper X-ray photoelectron spectra, Surf. Interface Anal., 2017, 49, 1325–1334 CrossRef CAS
.
- S. Gaarenstroom and N. Winograd, Initial and final state effects in the ESCA spectra of cadmium and silver oxides, J. Chem. Phys., 1977, 67, 3500–3506 CrossRef CAS
.
- C. Wagner, Auger lines in X-ray photoelectron spectrometry, Anal. Chem., 1972, 44, 967–973 CrossRef CAS
.
- C. Wagner, L. Gale and R. Raymond, Two-dimensional chemical state plots: a standardized data set for use in identifying chemical states by X-ray photoelectron spectroscopy, Anal. Chem., 1979, 51, 466–482 CrossRef CAS
.
- J. P. Espinós, J. Morales, A. Barranco, A. Caballero, J. P. Holgado and A. R. González-Elipe, Interface Effects for Cu, CuO, and Cu2O Deposited on SiO2 and ZrO2. XPS Determination of the Valence State of Copper in Cu/SiO2 and Cu/ZrO2 Catalysts, J. Phys. Chem. B, 2002, 106, 6921–6929 CrossRef
.
- O. P. Vaughan, G. Kyriakou, N. Macleod, M. Tikhov and R. M. Lambert, Copper as a selective catalyst for the epoxidation of propene, J. Catal., 2005, 236, 401–404 CrossRef CAS
.
- S. Rades, T. Wirth and W. Unger, Investigation of silica nanoparticles by Auger electron spectroscopy (AES), Surf. Interface Anal., 2014, 46, 952–956 CrossRef CAS
.
- A. Thøgersen, S. Diplas, J. Mayandi, T. Finstad, A. Olsen, J. F. Watts, M. Mitome and Y. Bando, An experimental study of charge distribution in crystalline and amorphous Si nanoclusters in thin silica films, J. Appl. Phys., 2008, 103, 024308 CrossRef
.
- J. Batista, A. Pintar, J. P. Gomilšek, A. Kodre and F. Bornette, On the structural characteristics of γ-alumina-supported Pd–Cu bimetallic catalysts, Appl. Catal., A, 2001, 217, 55–68 CrossRef CAS
.
- C. Lau and G. Wertheim, Oxidation of tin: An ESCA study, J. Vac. Sci. Technol., 1978, 15, 622–624 CrossRef CAS
.
- A. W. Lin, N. R. Armstrong and T. Kuwana, X-ray photoelectron/Auger electron spectroscopic studies of tin and indium metal foils and oxides, Anal. Chem., 1977, 49, 1228–1235 CrossRef CAS
.
- S. Sen, S. Sen and C. Bauer, Determination of the oxidation states of tin by Auger electron spectroscopy, Thin Solid Films, 1981, 82, 157–164 CrossRef CAS
.
- D. F. Cox and G. B. Hoflund, An electronic and structural interpretation of tin oxide ELS spectra, Surf. Sci., 1985, 151, 202–220 CrossRef CAS
.
- G. B. Hoflund and G. R. Corallo, Electron-energy-loss study of the oxidation of polycrystalline tin, Phys. Rev. B: Condens. Matter Mater. Phys., 1992, 46, 7110 CrossRef CAS PubMed
.
- J. F. Weaver, T. J. Campbell, G. B. Hoflund and G. N. Salaita, Oxidation of polycrystalline tin by hyperthermal atomic oxygen: an investigation using electron energy-loss spectroscopy, J. Electron Spectrosc. Relat. Phenom., 2000, 106, 81–91 CrossRef CAS
.
- H. A. Hagelin-Weaver, J. F. Weaver, G. B. Hoflund and G. N. Salaita, Electron energy loss spectroscopic investigation of Ni metal and NiO before and after surface reduction by Ar+ bombardment, J. Electron Spectrosc. Relat. Phenom., 2004, 134, 139–171 CrossRef CAS
.
- K. Akimoto, Y. Sakisaka, M. Nishijima and M. Onchi, Electron energy-loss spectra of co-adsorbed Ni(100) surfaces, Surf. Sci., 1979, 88, 109 CrossRef CAS
.
- A. Gorschlüter and H. Merz, EELS study of single crystalline NiO(100), Int. J. Mod. Phys. B, 1993, 7, 341–344 CrossRef
.
- J. Küppers, Investigation of the influence of adsorbed gases on the electron energy
loss spectra of a Ni(110) surface, Surf. Sci., 1973, 36, 53–73 CrossRef
.
- S. Ohtani, K. Terada and Y. Murata, Electronic-energy-level measurements of chemisorbed oxygen on the (100) plane of nickel by electron energy-loss spectroscopy, Phys. Rev. Lett., 1974, 32, 415 CrossRef CAS
.
- K. Christmann, O. Schober and G. Ertl, Adsorption of CO on a Ni(111) surface, J. Chem. Phys., 1974, 60, 4719–4724 CrossRef CAS
.
- B. Koel, D. Peebles and J. White, Low temperature coadsorption of hydrogen and carbon monoxide on Ni(100): I. TPD, Δ, and UPS studies, Surf. Sci., 1983, 125, 709–738 CrossRef CAS
.
- H. Madden, J. Küppers and G. Ertl, Interaction of carbon monoxide with (110) nickel surfaces, J. Chem. Phys., 1973, 58, 3401–3410 CrossRef CAS
.
- H. Kato, Y. Sakisaka, M. Nishijima and M. Onchi, Electron-energy-loss and secondary-electron emission spectroscopies of clean and hydrogen-covered Ni(100) surfaces, Phys. Rev. B: Condens. Matter Mater. Phys., 1980, 22, 1709 CrossRef CAS
.
- C. Benndorf, C. Nöbl, M. Rusenberg and F. Thieme, H2O interaction with clean and oxygen precovered Ni(110), Surf. Sci., 1981, 111, 87–101 CrossRef CAS
.
- E. Roman and R. Riwan, Ionic implantation of N2+ in Ni(110) at 300 K, Surf. Sci., 1982, 118, 682–696 CrossRef CAS
.
- E. Sickafus and F. Steinrisser, Elastic-Scattering Effects in Characteristic Electron-Energy-Loss Spectra of Ni(110) Adsorbate Surfaces, Phys. Rev. B: Condens. Matter Mater. Phys., 1972, 6, 3714 CrossRef CAS
.
- F. Steinrisser and E. Sickafus, Surface molecular-orbital excitations in electron energy-loss spectra, Phys. Rev. Lett., 1971, 27, 992 CrossRef CAS
.
- Y. Sakisaka, M. Miyamura, J. Tamaki, M. Nishijima and M. Onchi, Interactions of NO with a Ni(100) surface, Surf. Sci., 1980, 93, 327–337 CrossRef CAS
.
- A. Arranz and C. Palacio, Study of Ni–Al interface formation, Thin Solid Films, 1998, 317, 55–58 CrossRef CAS
.
- E. Bakulin, M. Bredov and V. Vasil'ev, Electron energy loss in alloys of aluminum with 3D transition metals, Sov. Phys. Solid-State, 1972, 13, 2536–2540 Search PubMed
.
- M. Abu-Joudeh, B. Davies and P. Montano, Leed, auger, and electron energy loss studies of Ni epitaxially grown on Cu(100), Surf. Sci., 1986, 171, 331–348 CrossRef CAS
.
- P. Montano, P. Vaishnava and E. Boling, Electron energy loss and Auger study of epitaxially grown Cu on Ni(100), Surf. Sci., 1983, 130, 191–202 CrossRef CAS
.
- S. D'Addato, L. Pasquali, G. Gazzadi, R. Verucchi, R. Capelli and S. Nannarone, Growth of Fe ultrathin films on Ni(111): structure and electronic properties, Surf. Sci., 2000, 454, 692–696 CrossRef
.
- Y. Lee, M. Abu-Joudeh and P. Montano, LEED and electron energy loss measurements of iron deposited on Ni(100), Surf. Sci., 1984, 143, 469–481 CrossRef CAS
.
- A. Fedorus, A. Gributa and I. Kotlyarova, Order in nickel films deposited at low temperatures and its effect on their electronic properties, Surf. Sci., 1994, 317, 170–182 CrossRef CAS
.
- M. Kiskinova, L. Surnev and G. Bliznakov, Oxygen adsorption on an alkali metal-covered Ni(100) surface, Surf. Sci., 1981, 104, 240–252 CrossRef CAS
.
- L. Surnev, G. Bliznakov and M. Kiskinova, Els study of alkali metal adsorption on Ni(100), Solid State Commun., 1981, 37, 87–90 CrossRef CAS
.
- K. Akimoto, Y. Sakisaka, M. Nishijima and M. Onchi, Electron energy-loss spectroscopy of UHV-cleaved NiO(100), CoO(100), and UHV-cracked MnO clean surfaces, J. Phys. C: Solid State Phys., 1978, 11, 2535 CrossRef CAS
.
- A. Gorschlüter and H. Merz, Localized d–d excitations in NiO(100) and CoO(100), Phys. Rev. B: Condens. Matter Mater. Phys., 1994, 49, 17293 CrossRef PubMed
.
- A. Gorschlüter and H. Merz, Resonant electron exchange scattering in late transition metal monoxides, J. Electron Spectrosc. Relat. Phenom., 1998, 87, 211–220 CrossRef
.
- J. M. McKay and V. E. Henrich, Structure of valence and conduction levels in NiO, Phys. Rev. Lett., 1984, 53, 2343 CrossRef CAS
.
- J. M. McKay and V. E. Henrich, Surface electronic structure of NiO: defect states, O2 and H2O interactions, Phys. Rev. B: Condens. Matter Mater. Phys., 1985, 32, 6764 CrossRef CAS PubMed
.
- F. Netzer and M. Prutton, LEED and electron spectroscopic observations on NiO(100), J. Phys. C: Solid State Phys., 1975, 8, 2401 CrossRef CAS
.
- Y. Sakisaka, K. Akimoto, M. Nishijima and M. Onchi, Electron energy-loss spectroscopy of UHV-cleaved NiO(100) surfaces, Solid State Commun., 1977, 24, 105–107 CrossRef CAS
.
- F. C. S. Aires, A. Howie and C. Walsh, Valence loss electron spectroscopy of Ni–Al mixed oxides, J. Solid State Chem., 1993, 106, 48–54 CrossRef
.
- E. Bakulin, M. Bredov and V. Vasil'ev, Formation of the electron energy-loss spectra in iron, cobalt, and nickel, Sov. Phys. Solid-State, 1972, 13, 3114–3115 Search PubMed
.
- L. A. B. E. A. Bakulin and V. A. Vasil’ev, Characteristic electron energy losses in NI and NIO, Sov. Phys. Solid State, 1971, 13, 542 Search PubMed
.
- B. Heimann and J. Hölzl, Variation of Characteristic Energy Losses in the Curie-Temperature Region of Ni(111), Phys. Rev. Lett., 1971, 26, 1573 CrossRef CAS
.
- M. Wolf, J. Loboda-Cackovic, K. Kambe, J. Block and M. Grunze, Anomalous electron energy-loss spectra of Ni(430) and a disordering of atomic steps, World J. Condens. Matter Phys., 1989, 1, 3701 CrossRef CAS
.
- L. Feldkamp, M. Stearns and S. Shinozaki, Electronic structure and optical constants of Ni by electron inelastic scattering, Phys. Rev. B: Condens. Matter Mater. Phys., 1979, 20, 1310 CrossRef CAS
.
- J. C. Ingram, K. W. Nebesny and J. E. Pemberton, Optical properties of selected first-row transition metals determined by reflection electron energy loss spectroscopy, Appl. Surf. Sci., 1990, 45, 247–256 CrossRef CAS
.
- D. Adler and J. Feinleib, Electrical and optical properties of narrow-band materials, Phys. Rev. B: Condens. Matter Mater. Phys., 1970, 2, 3112 CrossRef
.
- S. Hüfner, Electronic structure of NiO and related 3d-transition-metal compounds, Adv. Phys., 1994, 43, 183–356 CrossRef
.
- A. P. Grosvenor, M. C. Biesinger, R. S. C. Smart and N. S. McIntyre, New interpretations of XPS spectra of nickel metal and oxides, Surf. Sci., 2006, 600, 1771–1779 CrossRef CAS
.
- A. P. Grosvenor, S. D. Wik, R. G. Cavell and A. Mar, Examination of the Bonding in Binary Transition–Metal Monophosphides MP (M = Cr, Mn, Fe, Co) by X-Ray Photoelectron Spectroscopy, Inorg. Chem., 2005, 44, 8988–8998 CrossRef CAS PubMed
.
- A. Regoutz, A. M. Ganose, L. Blumenthal, C. Schlueter, T.-L. Lee, G. Kieslich, A. K. Cheetham, G. Kerherve, Y.-S. Huang, R.-S. Chen, G. Vinai, T. Pincelli, G. Panaccione, K. H. L. Zhang, R. G. Egdell, J. Lischner, D. O. Scanlon and D. J. Payne, Insights into the electronic structure of OsO2 using soft and hard X-ray photoelectron spectroscopy in combination with density functional theory, Phys. Rev. Mater., 2019, 3, 025001 CrossRef CAS
.
- T. Pillo, R. Zimmermann, P. Steiner and S. Hüfner, The electronic structure of PdO found by photoemission (UPS and XPS) and inverse photoemission (BIS), World J. Condens. Matter Phys., 1997, 9, 3987–3999 CrossRef CAS
.
- Z. J. T. Gray-Grychowski, W. R. Flavell, R. G. Egdell, R. A. Stradling, B. A. Joyce and J. H. Neave, Application of HREELS to MBE-grown III-V materials, Spectrochim. Acta, Part A, 1987, 43, 1503–1506 CrossRef
.
- P. A. Lee, K. F. Stork, B. L. Maschhoff, K. W. Nebesny and N. R. Armstrog, Oxide formation on Fe and Ti thin films and on Fe thin films modified with ultrathin layers of Ti, Surf. Interface Anal., 1991, 17, 48–56 CrossRef CAS
.
- M. Vos, S. W. King and B. L. French, Measurement of the band gap by reflection electron energy loss spectroscopy, J. Electron Spectrosc. Relat. Phenom., 2016, 212, 74–80 CrossRef CAS
.
- H. Jin, S. Oh, H. Kang, S. Lee, Y. Lee and M.-H. Cho, Band alignment in ultrathin Hf–Al–O/Si interfaces, Appl. Phys. Lett., 2005, 87, 212902 CrossRef
.
- H. Jin, S. K. Oh, Y. J. Cho, H. J. Kang and S. Tougaard, Electronic properties of ultrathin (HfO2)x(SiO2)1−x dielectrics on Si(100), J. Appl. Phys., 2007, 102, 053709 CrossRef
.
- H. C. Shin, D. Tahir, S. Seo, Y. R. Denny, S. K. Oh, H. J. Kang, S. Heo, J. G. Chung, J. C. Lee and S. Tougaard, Reflection electron energy loss spectroscopy for ultrathin gate oxide materials, Surf. Interface Anal., 2012, 44, 623–627 CrossRef CAS
.
- D. Tahir, E. K. Lee, S. K. Oh, H. J. Kang, S. Heo, J. G. Chung, J. C. Lee and S. Tougaard, Dielectric and optical properties of Zr silicate thin films grown on Si(100) by atomic layer deposition, J. Appl. Phys., 2009, 106, 084108 CrossRef
.
- M.-H. Cho, H. Chang, Y. Cho, D. Moon, K.-H. Min, R. Sinclair, S. Kang, D.-H. Ko, J. H. Lee and J. Gu, Investigation of the chemical state of ultrathin Hf–Al–O films during high temperature annealing, Surf. Sci., 2004, 554, L75–L80 CrossRef CAS
.
- R. Johnson, J. Hong, C. Hinkle and G. Lucovsky, Electron trapping in noncrystalline remote plasma deposited Hf-aluminate alloys for gate dielectric applications, J. Vac. Sci. Technol., B, 2002, 20, 1126–1131 CrossRef CAS
.
- G. Wilk, R. M. Wallace and J. M. Anthony, High-κ gate dielectrics: Current status and materials properties considerations, J. Appl. Phys., 2001, 89, 5243 CrossRef CAS
.
- G. D. Wilk, R. M. Wallace and J. Anthony, High-κ gate dielectrics: Current status and materials properties considerations, J. Appl. Phys., 2001, 89, 5243–5275 CrossRef CAS
.
-
P. Mack, Multi-technique surface characterization of organic LED material, Thermo Scientific Application Notes.
- A. Ferrari, A. Libassi, B. Tanner, V. Stolojan, J. Yuan, L. Brown, S. Rodil, B. Kleinsorge and J. Robertson, Density, sp3 fraction, and cross-sectional structure of amorphous carbon films determined by X-ray reflectivity and electron energy-loss spectroscopy, Phys. Rev. B: Condens. Matter Mater. Phys., 2000, 62, 11089 CrossRef CAS
.
-
P. Mack, J. Wolstenholme and A. Wright, Hydrogen Quantification Using the ESCALAB 250Xi, Thermo Scientific Application Notes.
- M. Müller, M. Schellhorn and K. Mann, Laboratory-scale near-edge X-ray absorption fine structure spectroscopy with a laser-induced plasma source, J. Anal. At. Spectrom., 2019, 34, 1779–1785 RSC
.
|
This journal is © the Partner Organisations 2021 |
Click here to see how this site uses Cookies. View our privacy policy here.