DOI:
10.1039/D1TC06086D
(Paper)
J. Mater. Chem. C, 2022,
10, 13844-13850
Three-dimensional all-inorganic dual halogen emitter Cs2Cd2BrCl5 exhibiting broadband white-light emission†
Received
23rd December 2021
, Accepted 28th January 2022
First published on 2nd February 2022
Abstract
Single-component white-light-emitting materials have received increasing attention due to their excellent luminescence properties. Herein, a novel all-inorganic dual halogen emitter Cs2Cd2BrCl5 was successfully synthesized, which features a three-dimensional (3D) network structure. A series of photophysical characterizations show that Cs2Cd2BrCl5 exhibits broadband white-light emission at room temperature with a correlated color temperature (CCT) of 4286 K, a color rendering index (CRI) of 84.6 and a high photoluminescence quantum yield (PLQY) of 20.49%. Temperature-dependent emission spectra, structural distortion analysis of the CdCl3(Br/Cl)3 octahedron, and DFT calculation reveal that the broadband white-light emission of Cs2Cd2BrCl5 originates from self-trapped excitons (STEs). Importantly, Cs2Cd2BrCl5 shows splendid structural and optical stability, which makes it a potential candidate for light-emitting diodes (LEDs). To our knowledge, it is the first example of white-light emission in 3D Cd-based all-inorganic halide materials, which could provide new insight for the further design and synthesis of all-inorganic single-component white-light emitters.
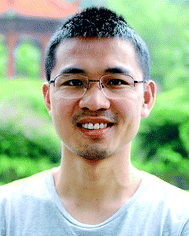
Guohong Zou
| Professor Guohong Zou earned B.S. degree from Central South University in 2008, and got his PhD degree in 2013 from Fujian Institute of Research on the Structure of Matter, Chinese Academy of Sciences, China, under the direction of Professor Ning Ye on discovering new nonlinear optical materials. He performed postdoctoral research in the group of Prof. Kang Min Ok at Chung-Ang University, Keroa, on the UV nonlinear optical crystals. Currently, he is a Professor at Sichuan University. His research includes discovering novel inorganic optical materials. |
Introduction
In the past few years, solid-state lighting technology (SSL) has greatly affected our lives due to its application in light-emitting diodes (LEDs), sensors and displays.1–8 Achieving white-light emission is considered a key step in the effective use of SSL. Generally, there are three ways to achieve white-light emission: one is via a three-component emitter with a mixture of red, blue, and green light sources;9–11 another is via a two-component emitter that combines yellow and blue light sources; and the third is via single-component white-light-emitting materials. Multi-component white-light-emitting materials have a high quantum efficiency and excellent color rendering properties. However, the serious re-absorption between the multiple components and the discontinuity of the emission spectrum in the visible range hinder their application.12,13 By contrast, single-component white-light-emitting materials overcome the above disadvantages, and have attracted increasing attention due to their simple preparation process, lower cost, and good color rendering.14,15
Single-component white-light emission has made remarkable achievements in organic molecules, inorganic nanocrystals and metal–organic frameworks.16,17 These materials exhibit excellent optical properties, but their synthesis involves complicated processing.18 Recently, organic–inorganic hybrid halide-based white-light-emitting materials have received extensive attention due to their high color purity and adjustable structural dimensions. For example, Kanatzidis et al. reported a series of lead bromide perovskites including α-(DMEN)PbBr4, (DMAPA)PbBr4 and (DMABA)PbBr4. They possess a 2D layered structure and exhibit inherent white-light emission.19 In addition, Karundasa et al. synthesized (EDBE)[PbX4] (X = Cl, Br, I) perovskites, which also exhibit broadband white-light emission.20 The broadband emissions of such compounds are ascribed to self-trapped excitons (STEs), which are caused by strong electron–phonon coupling in the distorted structure. In addition to Pb-based halide white-light-emitting materials, some Cd-based organic–inorganic hybrid halide white-light emitters have also been reported recently, including (2cepiH)CdCl3 and (C6H11NH3)2CdBr4, which possess one-dimensional (1D) chain and two-dimensional (2D) layered structures, respectively, and all of them exhibit white-light emission.21,22 Compared with organic–inorganic hybrid halide white-light-emitting materials, all-inorganic halide emitters may have more research value due to their relatively excellent thermal stability. At present, reports on Cd-based all-inorganic halide white-light-emitting materials are very limited. Although Luo et al. reported the white-light emitter Rb2CdCl2I2, it possesses a 2D layered structure.23 3D Cd-based all-inorganic white-light halide materials have not been reported so far.
In this work, we reported a new 3D all-inorganic dual halogen emitter Cs2Cd2BrCl5, which exhibits broadband white-light emission at room temperature. The white-light emission of Cs2Cd2BrCl5 is attributed to STEs as revealed by the analysis of octahedral structure distortion, temperature-dependent emission spectra and DFT theoretical calculations. Moreover, Cs2Cd2BrCl5 possesses good structural thermal stability and an excellent optical performance compared with the organic–inorganic hybrid materials. As far as we know, this emitter is the first example of white-light emission in Cd-based 3D all-inorganic halides. Our studies not only enrich the research on Cd-based light-emitting materials but also provide insight into the design and synthesis of all-inorganic dual-halogen white-light emitters.
Experimental section
Synthesis
Analytical grade reagents including CdBr2·4H2O (AR, 98%) and CsCl (AR, 99%) were used directly without further purification. The Cs2Cd2BrCl5 single crystals were synthesized via a hydrothermal method from the reactions of a mixture of CdBr2·4H2O (0.344 g, 1 mmol) and CsCl (0.337 g, 2 mmol) dissolved in 3 mL deionized water. The mixture was sealed into a 23 mL Teflon autoclave and heated at 180 °C for 3 days before cooling to room temperature. The target product was washed with alcohol and then dried in an oven at 40 °C. Transparent colorless rod crystals were obtained.
Characterization
Single-crystal X-ray diffraction was measured at 293.6(8) K using a Rigaku XtaLAB Synergy R diffractometer with graphite monochromatic Cu Kα (λ = 1.54184 Å) radiation (Table 1). Powder X-ray diffraction (XRD) data were obtained using a Rigaku D/MAX-rA diffractometer with Cu-Kα radiation (λ = 1.5418 Å). Thermogravimetric (TG) analyses were recorded in a flow of N2 with a heating rate of 10 °C min−1 using a Netzsch STA 449c analyzer. Scanning electron microscope (SEM)/energy-dispersive (EDS) analyses were performed using a Hitachi S-3400N/Horiba Energy EX-250 instruments. The UV-vis absorption spectra were obtained at room temperature using a PerkinElmer Lambda-950 UV/VIS spectrophotometer. The band structure, density of states (DOS)/partial density of states (PDOS), and electron density difference (EDD) diagrams were calculated through first principles. Gradient correction functional (GGA) and Perdew–Burke–Ernzerhof (PBE) were used for all calculations. The excitation spectra, emission spectra and photoluminescence quantum yield (PLQY) were measured using an Edinburgh FS-5 fluorescence spectrometer with a calibrated integrating sphere system. The temperature-dependent emission spectra and time-resolved PL decay curves were measured using a Fluoromax-3 fluorescence spectrometer.
Table 1 Crystal data and structure refinement for Cs2Cd2BrCl5
Formula |
Cs2Cd2BrCl5 |
R
1(F) = ∑||Fo| − |Fc||/∑|Fo|; wR2(Fo2) = [∑w(Fo2 − Fc2)2/∑w(Fo2)2]1/2.
|
Formula weight |
747.80 |
Crystal system |
Hexagonal |
Space group |
P63/mmc |
a (Å) |
7.4571(2) |
b (Å) |
7.4571(2) |
c (Å) |
12.5222(6) |
V (Å3) |
603.05(4) |
Z
|
2 |
D
calcd (g cm−3) |
4.118 |
Temperature (K) |
293(2) |
λ (Å) |
0.71073 |
F (000) |
652.0 |
μ (mm−1) |
13.833 |
GOF on F2 |
1.267 |
R
1, wR2 (I > 2σ(I))a |
0.0285/0.0758 |
Result and discussions
Crystal structure, octahedral distortion, phase purity and thermal analysis
Single-crystal X-ray diffraction (SCXRD) analysis demonstrates that Cs2Cd2BrCl5 crystallizes in the hexagonal space group of P63/mmc (no. 194). There are one independent Cd atom, one Cl atom, one Br/Cl co-occupation atom and two Cs atoms in the crystallographic asymmetric unit of Cs2Cd2BrCl5. The Cd atom is coordinated with three Cl atoms and three co-occupied Br/Cl atoms forming a CdCl3(Br/Cl)3 octahedron. Two CdCl3(Br/Cl)3 octahedra are connected forming a Cd2Cl6(Br/Cl)3 dimer via sharing of the three co-occupied Br/Cl atoms (Fig. 1a). The Cd–Cl and Cd–Br bond lengths range from 2.604 to 2.691 Å (Fig. 1a). The adjacent Cd2Cl6(Br/Cl)3 dimers are inter-linked, forming a 2D layer via sharing Cl atoms along the ac plane (Fig. 1b), and this corrugated 2D layer is further linked via sharing Cl atoms along the b axis, resulting in the 3D structure (Fig. 1c and d). The Cs ions are regularly inserted in the 3D anion framework along the c axis and play a role in maintaining the charge balance (Fig. 1c and d). It is worth noting that the Cd–Cl bond lengths are shorter than those of the co-occupied Cd–Br/Cl bonds, which indicates the CdCl3(Br/Cl)3 octahedron is distorted. The distortion degree of the CdCl3(Br/Cl)3 octahedron can be evaluated using the following formula:24,25
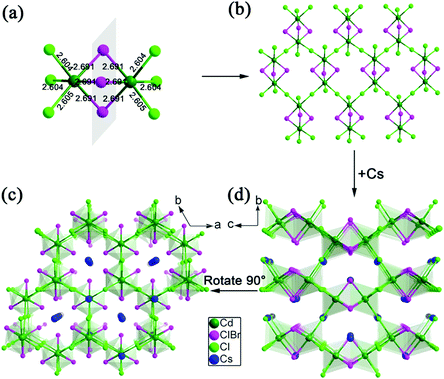 |
| Fig. 1 (a) Coordination of Cd2Cl6(Br/Cl)3 dimers and their bond lengths; (b) 2D layer structure built from adjacent Cd2Cl6(Br/Cl)3 dimers that are inter-linked by sharing the Cl atoms along the ac plane; (c) crystal structure of Cs2Cd2BrCl5 viewed along the ab plane; and (d) crystal structure of Cs2Cd2BrCl5 viewed along the bc plane. | |
where dn represents the length of six independent Cd–Cl or Cd–Br/Cl bonds, and d is their average bond length. The value of Δd is calculated as 2.68 × 10−4, which is comparable to the reported lead-based halide materials of (BA)2PbI4 (Δd = 3.5 × 10−4), ((DMAPA)PbBr4) (Δd = 1.1 × 10−4) and (DMABA)PbBr4 (Δd = 4.3 × 10−4), indicating that the CdCl3(Br/Cl)3 octahedron shows structural distortion.17,26 The distortion of the Cd–Cl/Br framework facilitates the formation of STEs, resulting in broadband emission. The powder XRD data of Cs2Cd2BrCl5 were measured to verify the phase purity, and the results showed that the experimental data are in accordance with the calculated XRD pattern (Fig. 2a). In addition, EDS analysis of Cs2Cd2BrCl5 showed that the elemental content matches well with the theoretical values (Fig. S1, ESI†). Moreover, the TGA and DSC curves of Cs2Cd2BrCl5 were collected at a heating rate of 10 °C min−1 in the temperature range from RT to 850 °C (Fig. 2b). The TGA curve shows that there is almost no heat loss until the heating temperature exceeds 500 °C, and that Cs2Cd2BrCl5 did not undergo any phase change before decomposition can be seen in the DSC curve. The above results indicate that Cs2Cd2BrCl5 has a high thermal stability and is an ideal candidate for single-component white-light-emitting materials in SSL applications.
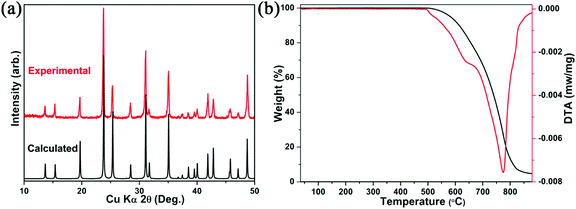 |
| Fig. 2 (a) XRD pattern of Cs2Cd2BrCl5 obtained via a hydrothermal method, and (b) TG–DSC curves of Cs2Cd2BrCl5. | |
PL properties and optical stability analysis
The photoluminescence (PL) properties of Cs2Cd2BrCl5 at room temperature were fully explored. Fig. 3a shows that the Cs2Cd2BrCl5 bulk crystals are colorless and transparent under ambient light and emit bright white light upon 254 nm UV-light irradiation. As shown in the purple line of Fig. 3b, the absorption edge of 291 nm was observed and the corresponding experimental band gap is 4.25 eV. Upon 285 nm excitation, Cs2Cd2BrCl5 exhibits broadband emission covering the entire visible-light range with the strongest emission peak at 557 nm and a FWHM of 206 nm (the black line of Fig. 3b). The large Stokes shift of 272 nm is attributed to the strong electron–phonon coupling in the distorted CdCl3(Br/Cl)3 octahedron.27 As illustrated in Fig. 3c, the CIE chromaticity coordinates of Cs2Cd2BrCl5 are (0.3827, 0.4367) and the correlated color temperature (CCT) is 4286 K, which corresponds to white-light emission. In addition, the CRI reaches up to 84.6, which is higher than most commercial light-emitting diodes (with a CRI of 80), making it a candidate for advanced color-critical applications. Moreover, the PLQY of Cs2Cd2BrCl5 was measured to be 20.49%, which is relatively high for single-component white-light-emitting materials, whose PLQY values are usually <10%. For example, the PLQY values of (C5H9NH3)4CdBr4, (2meptH2)4PbCl4 and (EDBE)4PbBr4 are 1%, 1.05% and 9%, respectively.28–30 The time-resolved PL decay curve shows that the average lifetime of Cs2Cd2BrCl5 is 8.98 ms, which was obtained via triple-exponential fitting (Fig. 3d). As previously reported, the emission of many semiconductor materials originates from surface defects, and the emission will be quenched as the particles aggregate.31–33 Therefore, the emission spectra of bulk crystals and ground powder samples were measured to determine the emission origin. Fig. 4a reflects that there is no difference in the emission spectra of bulk crystals and ground powder, suggesting that the emission of Cs2Cd2BrCl5 is not caused by surface defects but is inherent emission. Considering the large Stokes shift and wide FWHM, we speculate that the white-light emission of Cs2Cd2BrCl5 can be ascribed to STEs.34–37 Generally considered, halide luminescent materials exhibit poor structural and photophysical stability. Therefore, the emission spectra of fresh crystals, bulk crystals placed in the air for 120 d and after irradiation for 12 h with 254 nm UV light (300 W cm−2 Xe lamp) were collected. As shown in Fig. 4b, the emission spectra present similar profiles, and the emission intensities of the crystals after treatment are only slightly lower than that of the fresh crystals, indicating that Cs2Cd2BrCl5 shows excellent structural and photophysical stability.
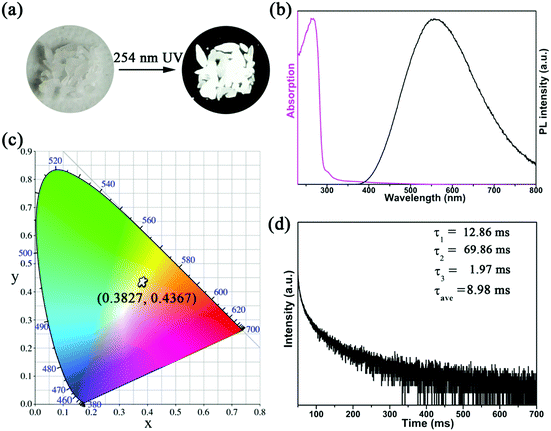 |
| Fig. 3 PL properties of compound Cs2Cd2BrCl5 at room temperature: (a) images of Cs2Cd2BrCl5 crystals under ambient light and 254 nm UV-light excitation, (b) UV-vis absorption spectrum (purple line) and emission spectrum (black line), (c) CIE chromaticity coordinates, and (d) lifetime decay curve. | |
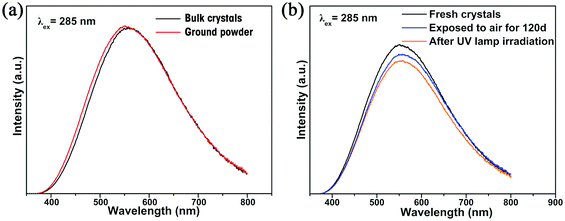 |
| Fig. 4 (a) PL spectra of bulk crystals and ground powder for Cs2Cd2BrCl5, and (b) emission spectra of fresh Cs2Cd2BrCl5 crystals, crystals exposed to air for 120 d, and after 12 h of UV-lamp irradiation. | |
Mechanism study via temperature-dependent PL spectra
To explore the thermal quenching behavior and emission mechanism of compound Cs2Cd2BrCl5, we collected the temperature-dependent PL spectra from 287 to 77 K at identical intervals of 30 K. As shown in Fig. 5a, it can be observed that as the temperature decreases, the intensity of the emission peak is gradually enhanced. The above phenomenon can be explained as lowering the temperature helps to reduce the non-radiative transitions, resulting in more energy being presented in the form of light rather than thermal loss. The activation energy (ΔEa) of Cs2Cd2BrCl5 for thermal quenching can be expressed clearly using the Arrhenius equation:38–41
where I0 and IT represent the PL intensity at the initial temperature and the test temperature T, respectively. The parameters c and ΔEa represent the frequency factor and activation energy, respectively, k is the Boltzmann constant (8.617 × 10−5 eV K−1), and the fitting result is displayed in Fig. 5b. The activation energy (ΔEa) of Cs2Cd2BrCl5 is fitted as 41.17 meV, which is equivalent to previously reported halide luminescent materials.42,43 In particular, the emission spectra of Cs2Cd2BrCl5 at 287 K and 77 K are revealed in Fig. 5c. It can be clearly seen that when the test temperature is reduced from 287 K to 77 K, in addition to the broadband emission at room temperature, a high-energy narrowband emission at 480 nm appears. The corresponding CIE chromaticity coordinates changes from (0.3827, 0.4367) for white light to (0.2807, 0.3926) for green light (Fig. S2, ESI†). Moreover, the time-resolved decay curve of Cs2Cd2BrCl5 at 77 K was also measured (Fig. S3, ESI†). Under excitation at 285 nm, with monitoring at 480 nm, the lifetime is 2.2 ns, and while monitoring at 557 nm, the lifetime is 9.13 ms.
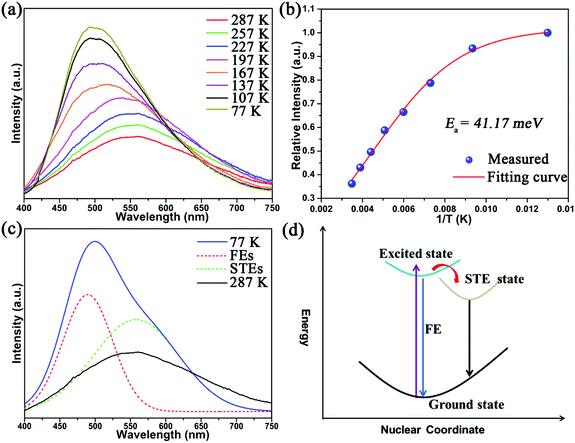 |
| Fig. 5 (a) Temperature-dependent emission spectra; (b) functional relationship between 1/T and the integrated PL intensity; (c) emission spectrum at 77 K (solid blue line) and 287 K (solid black line), with the emission spectra of FEs (red dashed line) and STEs (green dashed line) obtained via Gaussian fitting to split the emission spectrum at 77 K; and (d) corresponding emission mechanism configuration diagram of Cs2Cd2BrCl5. | |
We attribute the narrowband emission at 480 nm to the emission of free excitons (FEs), while the broadband emission at 557 nm can be ascribed to the emission of self-trapped excitons (STEs).44,45 The FE and STE emission can be well represented using Gaussian fitting to split the emission spectrum at 77 K (green and red dashed lines in Fig. 5c). Moreover, the emission mechanism of Cs2Cd2BrCl5 can be further explained using a configuration diagram (Fig. 5d).46,47 Under UV-light excitation, the electrons in the ground state are first excited to the FE excited state, and then they transit to the STE excited state through the relaxation process. Finally, the excitons return to the ground state from the STE excited state. The STEs dominate at high temperature (287 K) because there is sufficient energy to overcome the energy barrier from FEs to STEs, resulting in a strong broadband emission. However, when the temperature drops to 77 K, the thermal energy is not sufficient to overcome the barrier between the two energy levels, meaning that only a small proportion of the excitons can cross the barrier to enter the STE excited state, and most of them directly return to the ground state from the FE excited state. Therefore, in addition to the broadband emission, a strong high-energy narrowband emission peak also appears at 77 K, and the intensity of the narrowband emission is stronger than that of broadband emission.
Electronic structure calculations
In order to evaluate the electronic structure and further confirm the PL mechanism of Cs2Cd2BrCl5, the band structure, partial density of states and electron-density difference map were calculated using the first principles density functional theory method. As shown in Fig. 6a, the conduction band minimum (CBM) and the valence band maximum (VBM) lie at different locations (in the areas of GA and AH, respectively), indicating that Cs2Cd2BrCl5 is an indirect band gap compound. The calculated band gap is 3.7 eV, which is smaller than experimental band gap (4.25 eV). As is well known, DFT–GGA methods will bring down the conduction band levels and therefore underestimate the calculated band gap.48–51 The total densities of states (TDOS) and partial densities of states (PDOS) of Cs2Cd2BrCl5 are calculated and plotted in Fig. 6b. It can be seen that the top of the valence band and the bottom of the conduction band are mainly composed of Cl-3p, Br-4p and Cd-5s orbitals, indicating that there are relatively strong bonds between Cd and Cl, and between Cd and Br. Since the optical properties of materials are mainly determined by the state near the forbidden band, the photoluminescence of Cs2Cd2BrCl5 is contributed by the CdCl3(Br/Cl)3 octahedra. The electron density difference (EDD) map (Fig. 6c) shows that the electron cloud of Cd atoms has significant steric activity and the CdCl3(Br/Cl)3 octahedra are distorted. The distorted structure facilitates electron–phonon coupling to form STEs, resulting in broadband emission.
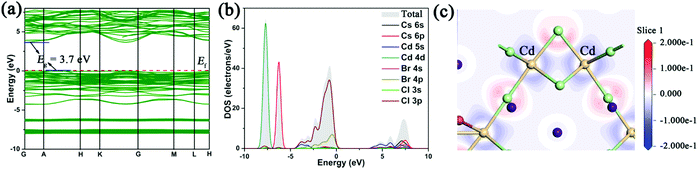 |
| Fig. 6 (a) Cs2Cd2BrCl5 band structure; (b) TDOS and PDOS, where the Fermi level (Ef) is normalized to 0 eV; and (c) EDD map for Cs2Cd2BrCl5. | |
Conclusions
In summary, the 3D all-inorganic dual halogen emitter Cs2Cd2BrCl5 was obtained through a hydrothermal method. Cs2Cd2BrCl5 exhibits broadband white-light emission at room temperature with a CCT of 4286 K, a CRI of 84.6 and a high PLQY of 20.49%. In addition, Cs2Cd2BrCl5 possesses excellent structural and thermal stability, indicating that Cs2Cd2BrCl5 is a promising single-component white-light-emitting material. Photophysical studies show that the broadband emission of Cs2Cd2BrCl5 is attributed to the emission of STEs that originate from the strong electron–phonon coupling in the octahedral distortion, which was further confirmed via DFT calculations. Our studies will provide new insight for the further exploration of novel all-inorganic white-light emitters.
Conflicts of interest
There are no conflicts to declare.
Acknowledgements
This work was supported by the National Natural Science Foundation of China (no. 21875146, 22071158, and 22122106).
Notes and references
- C. K. Zhou, Y. Tian, Z. Yuan, H. R. Lin, B. H. Chen, R. J. Clark, T. Dilbeck, Y. Zhou, J. Hurley, J. Neu, T. Besara, T. Siegrist, P. I. Djurovich and B. W. Ma, ACS Appl. Mater. Interfaces, 2017, 9, 44579–44583 CrossRef CAS PubMed.
- X. Y. Huang, Qi Sun and B. Devakumar, Mater. Today Chem., 2020, 17, 100288 CrossRef CAS.
- M. D. Smith, B. A. Connor and H. I. Karunadasa, Chem. Rev., 2019, 119, 3104–3139 CrossRef CAS PubMed.
- S. Reineke, F. Lindner, G. Schwartz, N. Seidler, K. Walzer, B. Lüssem and K. Leo, Nature, 2009, 459, 234–238 CrossRef CAS PubMed.
- W. Ki and J. Li, J. Am. Chem. Soc., 2008, 130, 8114–8115 CrossRef CAS PubMed.
- M. S. Wang, S. P. Guo, Y. Li, L. Z. Cai, J. P. Zou, G. Xu, W. W. Zhou, F. K. Zheng and G. C. Guo, J. Am. Chem. Soc., 2009, 131, 13572–13573 CrossRef CAS PubMed.
- X. D. Wang, O. S. Wolfbeis and R. J. Meier, Chem. Soc. Rev., 2013, 42, 7834 RSC.
- Y. L. Deng, L. Huang, X. H. Dong, L. Wang, K. M. Ok, H. M. Zeng, Z. E. Lin and G. H. Zou, Angew. Chem., Int. Ed., 2020, 59, 21151–21156 CrossRef CAS PubMed.
- X. Bai, G. Caputo, Z. D. Hao, V. T. Freitas, J. H. Zhang, R. L. Longo, O. L. Malta, R. A. S. Ferreira and N. Pinna, Nat. Commun., 2014, 5, 5702 CrossRef CAS PubMed.
- Y. Q. Li, A. Rizzo, R. Cingolani and G. Gigli, Adv. Mater., 2006, 18, 2545–2548 CrossRef CAS.
- W. B. Im, N. George, J. Kurzman, S. Brinkley, A. Mikhailovsky, J. Hu, B. F. Chmelka, S. P. DenBaars and R. Seshadri, Adv. Mater., 2011, 23, 2300–2305 CrossRef CAS PubMed.
- M. J. Bowers, J. R. McBride and S. J. Rosenthal, J. Am. Chem. Soc., 2005, 127, 15378–15379 CrossRef CAS PubMed.
- Z. W. Zhuang, C. D. Peng, G. Y. Zhang, H. M. Yang, J. L. Yin and H. H. Fei, Angew. Chem., Int. Ed., 2017, 56, 14411–14416 CrossRef CAS PubMed.
- Y. Liu, M. Nishiura, Y. Wang and Z. M. Hou, J. Am. Chem. Soc., 2006, 128, 5592–5593 CrossRef CAS PubMed.
- G. M. Song, M. Z. Li, S. Z. Zhang, N. Z. Wang, P. F. Gong, Z. G. Xia and Z. S. Lin, Adv. Funct. Mater., 2020, 30, 2002468 CrossRef CAS.
- J. R. McBride, A. D. Dukes, M. A. Schreuder and S. J. Rosenthal, Chem. Phys. Lett., 2010, 498, 1–9 CrossRef CAS PubMed.
- S. K. Panda, S. G. Hickey, H. V. Demir and A. Eychmller, Angew. Chem., Int. Ed., 2011, 50, 4432–4436 CrossRef CAS PubMed.
- V. Bachmann, C. Ronda and A. Meijerink, Chem. Mater., 2009, 21, 2077–2084 CrossRef CAS.
- L. L. Mao, Y. L. Wu, C. C. Stoumpos, M. R. Wasielewski and M. G. Kanatzidis, J. Am. Chem. Soc., 2017, 139, 5210–5215 CrossRef CAS PubMed.
- E. R. Dohner, A. Jaffe, L. R. Bradshaw and H. I. Karunadasa, J. Am. Chem. Soc., 2014, 136, 13154–13157 CrossRef CAS PubMed.
- Z. K. Qi, Y. L. Chen, Y. Guo, X. L. Yang, F. Q. Zhang, G. J. Zhou and X. M. Zhang, J. Mater. Chem. C, 2021, 9, 88–94 RSC.
- A. Yangui, S. Pillet, E. E. Bendeif, A. Lusson, S. Triki, Y. Abid and K. Boukheddaden, ACS Photonics, 2018, 5, 1599–1611 CrossRef CAS.
- X. F. Li, S. S. Wang, S. G. Zhao, L. N. Li, Y. Q. Li, B. Q. Zhao, Y. G. Shen, Z. Y. Wu, P. Shan and J. H. Luo, Chem. – Eur. J., 2018, 24, 9243–9246 CrossRef CAS PubMed.
- C. Sun, Y. D. Yue, W. F. Zhang, X. Y. Sun, Y. Du, H. M. Pan, Y. Y. Ma, Y. C. He, M. T. Li and Z. H. Jing, CrystEngComm, 2020, 22, 1480–1486 RSC.
- G. J. Zhou, X. X. Jiang, M. Molokeev, Z. S. Lin, J. Zhao, J. Wang and Z. G. Xia, Chem. Mater., 2019, 31, 5788–5795 CrossRef CAS.
- C. C. Stoumpos, D. H. Cao, D. J. Clark, J. Young, J. M. Rondinelli, J. I. Jang, J. T. Hupp and M. G. Kanatzidis, Chem. Mater., 2016, 28, 2852–2867 CrossRef CAS.
- Y. Y. Jing, Y. Liu, M. Z. Li and Z. G. Xia, Adv. Opt. Mater., 2021, 9, 2002213 CrossRef CAS.
- S. S. Wang, L. N. Li, Z. H. Sun, C. M. Ji, S. J. Liu, Z. Y. Wu, S. G. Zhao, M. C. Hong and J. H. Luo, J. Mater. Chem. C, 2017, 5, 4731–4735 RSC.
- S. S. Wang, Y. P. Yao, Z. Y. Wu, Y. Peng, L. N. Li and J. H. Luo, J. Mater. Chem. C, 2018, 6, 12267–12272 RSC.
- E. R. Dohner, A. Jaffe, L. R. Bradshaw and H. I. Karunadasa, J. Am. Chem. Soc., 2014, 136, 13154–13157 CrossRef CAS PubMed.
- M. Noh, T. Kim, H. Lee, C. K. Kim, S. W. Joo and K. Lee, Colloids Surf., A, 2010, 359, 39–44 CrossRef CAS.
- T. E. Rosson, S. M. Claiborne, J. R. McBride, B. S. Stratton and S. J. Rosenthal, J. Am. Chem. Soc., 2012, 134, 8006–8009 CrossRef CAS PubMed.
- Z. Y. Wu, C. Ji, Z. H. Sun, S. S. Wang, S. G. Zhao, W. C. Zhang, L. N. Li and J. H. Luo, J. Mater. Chem. C, 2018, 6, 1171–1175 RSC.
- Y. W. Ge, Q. Wang, F. Yang, L. Huang, D. J. Gao, J. Bi and G. H. Zou, Inorg. Chem., 2021, 60, 8322–8330 CrossRef CAS PubMed.
- J. L. Huang, B. B. Su, E. H. Song, M. S. Molokeev and Z. G. Xia, Chem. Mater., 2021, 33, 4382–4389 CrossRef CAS.
- Y. Zhou, Z. J. Yong, K. C. Zhang, B. M. Liu, Z. W. Wang, J. S. Hou, Y. Z. Fang, Y. Zhou, H. T. Sun and B. Song, J. Phys. Chem. Lett., 2016, 7, 2735–2741 CrossRef CAS PubMed.
- M. Z. Li, Y. W. Li, M. S. Molokeev, J. Zhao, G. R. Na, L. J. Zhang and Z. G. Xia, Adv. Opt. Mater., 2020, 8, 2000418 CrossRef CAS.
- G. J. Zhou, Z. Y. Liu, M. S. Molokeev, Z. W. Xiao, Z. G. Xia and X. M. Zhang, J. Mater. Chem. C, 2021, 9, 2047–2053 RSC.
- F. Hong, H. M. Cheng, G. X. Liu, X. T. Dong, W. S. Yu and J. X. Wang, Inorg. Chem., 2018, 57, 9892–9901 CrossRef CAS PubMed.
- F. Hong, H. P. Xu, L. Yang, G. X. Liu, C. Song, X. T. Dong and W. S. Yu, New J. Chem., 2019, 43, 14859–14871 RSC.
- F. Hong, G. Pang, L. J. Diao, Z. D. Fu, G. X. Liu, X. T. Dong, W. S. Yu and J. X. Wang, Dalton Trans., 2020, 49, 13805–13817 RSC.
- C. Sun, W. L. He, M. J. Liu, W. J. Pan, L. F. Dong, G. Chen, G. D. Liu and X. W. Lei, Chem. – Asian J., 2020, 15, 3050–3058 CrossRef CAS PubMed.
- C. Y. Yue, C. Sun, D. Y. Li, Y. H. Dong, C. L. Wang, H. F. Zhao, H. Jiang, Z. H. Jing and X. W. Lei, Inorg. Chem., 2019, 58, 10304–10312 CrossRef CAS PubMed.
- X. T. Li, P. J. Guo, M. Kepenekian, I. Hadar, C. Katan, J. Even, C. C. Stoumpos, R. D. Schaller and M. G. Kanatzidis, Chem. Mater., 2019, 31, 3582–3590 CrossRef CAS.
- C. Sun, Y. H. Guo, S. S. Han, J. Z. Li, K. Jiang, L. F. Dong, Q. L. Liu, C. Y. Yue and X. W. Lei, Angew. Chem., Int. Ed., 2020, 59, 16465–16469 CrossRef CAS PubMed.
- J. H. Wei, J. F. Liao, X. D. Wang, L. Zhou, Y. Jiang and D. B. Kuang, Matter, 2020, 3, 892–903 CrossRef.
- L. Zhou, J. F. Liao, Z. G. Huang, J. H. Wei, X. D. Wang, H. Y. Chen and D. B. Kuang, Angew. Chem., Int. Ed., 2019, 58, 15435–15440 CrossRef CAS PubMed.
- Q. Jing, X. Y. Dong, X. L. Chen, Z. H. Yang, S. L. Pan and C. Lei, Chem. Phys., 2015, 453, 42–46 CrossRef.
- A. J. Cohen, P. Mori-Sánchez and W. T. Yang, Phys. Rev. B: Condens. Matter Mater. Phys., 2008, 77, 115123 CrossRef.
- G. H. Zou, C. S. Lin, H. Jo, G. Nam, T. S. You and K. M. Ok, Angew. Chem., Int. Ed., 2016, 128, 12257–12261 CrossRef.
- X. H. Dong, L. Huang, C. F. Hu, H. M. Zeng, Z. E. Lin, X. Wang, K. M. Ok and G. H. Zou, Angew. Chem., Int. Ed., 2019, 58, 6528–6534 CrossRef CAS PubMed.
Footnotes |
† Electronic supplementary information (ESI) available: Additional crystallographic data, EDS, CIE chromaticity coordinates, and time-resolved decay curves. CCDC 2129940. For ESI and crystallographic data in CIF or other electronic format see DOI: 10.1039/d1tc06086d |
‡ These authors contributed equally to this work. |
|
This journal is © The Royal Society of Chemistry 2022 |
Click here to see how this site uses Cookies. View our privacy policy here.