A multi-pathway exposure assessment for polycyclic aromatic hydrocarbons among residents in the Athabasca oil sands region, Canada†
Received
22nd December 2022
, Accepted 23rd February 2023
First published on 28th February 2023
Abstract
Due to increasing emissions from ongoing development of the oil sands in Northern Alberta, Canada, there is concern that local residents and organisms are experiencing elevated exposures to hazardous contaminants. We modified an existing human bioaccumulation model (ACC-Human) to represent the local food chain in the Athabasca oil sands region (AOSR), the focus of oil sands development in Alberta. We used the model to assess the potential exposure to three polycyclic aromatic hydrocarbons (PAHs) among local residents that have a high intake of locally sourced traditional foods. To place these estimates into context, we complemented them with estimated PAH intake through market foods and smoking. Our approach was able to produce realistic body burdens of the PAHs in aquatic and terrestrial wildlife and in humans, both in magnitude and with respect to the relative difference between smokers and non-smokers. Over the model simulation period (1967–2009), market food was the dominant dietary exposure route for phenanthrene and pyrene, while local food, and in particular local fish, dominated the intake of benzo[a]pyrene. Exposure to benzo[a]pyrene therefore was also predicted to increase over time in concert with expanding oil sands operations. Those smoking at the average rate of Northern Albertans take in an additional amount of all three PAHs that is at least as large as dietary intake. Estimated daily intake rates are below toxicological reference thresholds for all three PAHs. However, daily intake of BaP in adults is only ∼20 fold below those thresholds and is predicted to increase. Key uncertainties in the assessment included the effect of food preparation on the PAH content in food (e.g., smoking of fish), the limited availability of market food contamination data specific to Canada, and the PAH content of the vapor phase of first-hand cigarette smoke. Considering the satisfactory model evaluation, ACC-Human AOSR should be suited to making predictions of future contaminant exposure based on development scenarios in the AOSR or in response to potential emission reduction efforts. It should also be applicable to other organic contaminants of concern released by oil sands operations.
Environmental significance
The exposure to contaminants and the associated health hazard among human populations living in the vicinity of fossil fuel extraction operations is a considerable concern. This concern is elevated in the Alberta oil sands region, because of the immense scale of those developments, and the reliance of local First Nations populations on locally sourced traditional foods, such as fish, meat and plants. Exposure assessments are complex because of the large number of potentially relevant contaminants, emission rates that have been changing over decadal time scales, and the confounding presence of contaminant exposures that are not related to oil sands operations, such as the intake with market foods and smoking. The model-based assessment strategy introduced and applied here to three polycyclic aromatic hydrocarbons can complement assessments based on empirical measurements, by filling data gaps related to levels below detection limits, dietary items that have not been analyzed, and contaminants that have not been quantified. The approach further provides the opportunity for both retrospective analysis and forecasting of future exposures.
|
1. Introduction
The bitumen reserves in Alberta, Canada constitute the third largest reserve of petroleum in the world.1 The continued exploitation of this resource has led to concerns over increasing local emissions of hazardous contaminants, such as certain polycyclic aromatic hydrocarbons (PAHs).2,3 Elevated concentrations of PAHs in local soil,4 water bodies5 and air6–8 have all been linked to oil sands operations. Industry-derived PAHs have been associated with reproductive, developmental, and immunological changes in local biota.9–11 Concerns have also been raised about the potential health effects on local First Nations populations arising from oil sands development.12–15
Concern regarding chronic and high-dose human exposure to some PAHs is due to their known or suspected carcinogenic, teratogenic, and genotoxic effects, while acute and low-dose effects are relatively poorly characterized.16 Major pathways for non-occupational PAH exposure include inhalation of ambient air, dietary ingestion, and cigarette smoking. The mean excess cancer risk for First Nations populations in Northern Alberta arising from inhalation and inadvertent soil ingestion of PAHs was assessed to be negligible.17 However, contaminated biota may also be an important contributor to PAH body burdens for local residents, especially for First Nations communities for whom locally-sourced, traditional food (TF) have nutritional and cultural significance.18 Oil sands pollutants have been found in First Nations' TF sources in Northern Alberta.19 However, in recent decades market foods that are not locally-produced have comprised an increasing fraction of the diets of various northern Indigenous communities.20 In Alberta, declining TF consumption is due to the decreasing availability of equipment, transportation, and hunters in the household.21 As a result, market foods may also account for a significant fraction of PAH exposure in the Athabasca oil sands region (AOSR). Finally, considering that 59% of First Nations adults in boreal Alberta are smokers,21 and that PAHs are a few of the many constituents of mainstream cigarette smoke,22 smoking is also expected to elevate PAH exposure depending on individual smoking habits.
The relative importance of inhalation, dietary ingestion, and cigarette smoking as PAH exposure pathways is highly dependent on individual behaviors and lifestyle characteristics. Among non-smoking adults, diet typically contributes at least 90% of PAH intake.23,24 A diet rich in foods prepared with high temperature cooking methods (e.g., grilling) further eclipses any PAH contributions from inhalation.25 In areas with significant atmospheric PAH contamination, the dietary contribution may be less, e.g., 70% in Beijing, China.26 For smokers, one pack of cigarettes per day can account for 40 to 63% of an individual's PAH intake.27
Multi-pathway exposure assessments to determine major intake pathways typically use some combination of environmental and biological monitoring, often for one or multiple groups of people with similar age, gender, and occupational and smoking statuses, over a limited span of time. Given sufficient information about chemical and environmental properties and individual lifestyle characteristics, a time-variant mechanistic model presents some advantages over these empirical methods.28 First, developing and applying such a model is much less resource-intensive, particularly in comparison to biological monitoring methods. In addition, this type of model can provide long-term, temporally variable exposure estimates, allowing insight into both key life stages for exposure, and comparisons between individuals born and raised under varying chemical emissions scenarios. A mechanistic approach also facilitates extrapolation to other compounds of potential exposure concern. Finally, a mechanistic model can serve as a management tool for making predictions of future contaminant exposure based on future AOSR development scenarios or in response to potential emission reduction efforts.
In the present study we apply this approach to individuals in the AOSR. Specifically, we modified and parametrized an existing time-variant, mechanistic bioaccumulation model to include food items relevant to residents in the region. Then, we applied the model to calculate the inhalation and dietary exposure of the residents of varying ages to PAHs emitted to the local environment by AOSR operations. Finally, we compared PAH uptake due to local TF, market foods and cigarette smoking, as well as temporal patterns of PAH uptake amongst individuals born at different times relative to development in the AOSR.
2. Methods
2.1 Overview of modeling approach
Fig. 1 depicts a conceptual overview of the model approach. We modified and validated the existing human bioaccumulation model ACC-Human29 by including food items local to Northern Alberta. Using TF intake rates for the region21 and time trends of abiotic environmental contamination obtained previously by modelling,30 we estimated time-variant human PAH uptake (i.e., intake corrected for absorption efficiency in gut or lung) arising from industrial development within the AOSR for different human birth cohorts. Pathways being considered include inhalation of ambient air and ingestion of locally-sourced drinking water, soil and TF. To place those estimates into context, we further used literature data to estimate PAH uptake due to consumption of market foods and smoking. With this approach, we were able to compare the relative importance of sources of human PAH exposure arising from AOSR development (intake of local air, water, soil and TF) with sources not impacted by that development (market food and cigarette smoking). Three PAHs were selected to represent a broad range of environmental partitioning properties: phenanthrene (PHE: most volatile, least hydrophobic), pyrene (PYR: intermediate volatility and hydrophobicity), and benzo[a]pyrene (BAP: least volatile, most hydrophobic). The approach is described in further detail in the following section.
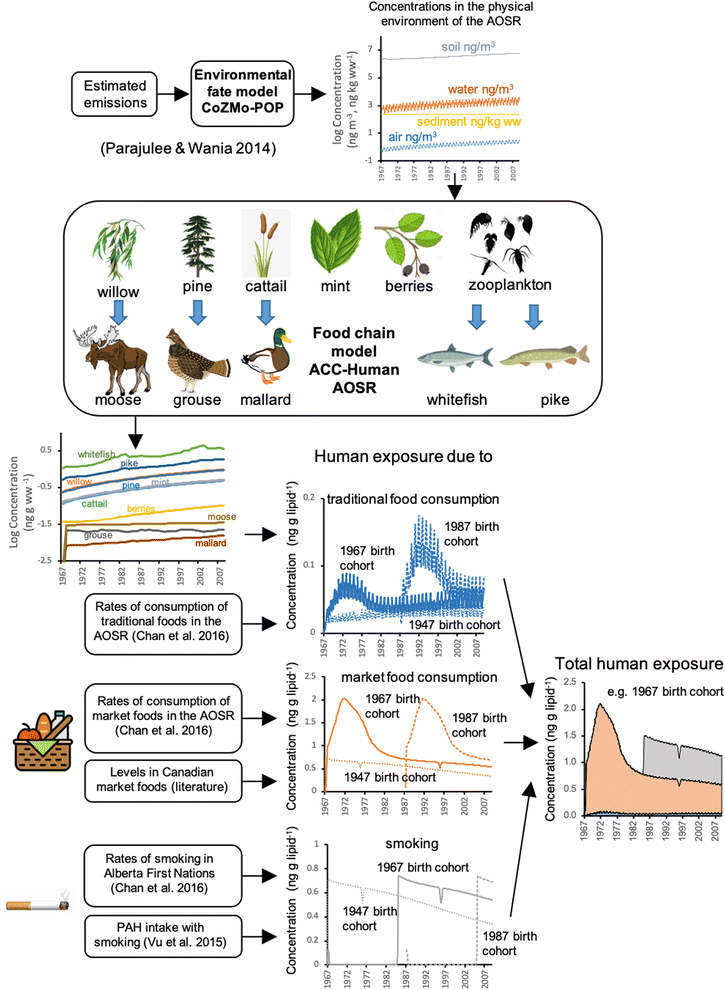 |
| Fig. 1 Schematic representation of the approach to human PAH exposure assessment taken in this study. | |
2.2 Modeling the different exposure pathways
2.2.1 The ACC-Human model.
PAH body burdens in humans and wildlife in the AOSR were estimated using ACC-Human, a mechanistic non-steady state bioaccumulation model.29,31 In ACC-Human, contamination of each organism, represented by a one compartment sub-model, is calculated by balancing inputs (respiration and ingestion) and outputs (urinary or percutaneous excretion, respiration, egestion, biotransformation, and, for females, childbirth and lactation). The concentration of contaminants in air, water, soil, and sediment, which control the inputs, were taken from our previous work estimating time trends of PAH contamination in the AOSR environment from 1967, the start of oil sands operations, to 2009.30
The model calculates exposures of different human birth cohorts throughout their entire life, assumed to last 80 years. When presenting results, we focus on the three cohorts born in 1947 (20 years before oil sands development started), 1967 (start of development), and 1987 (20 years into oil sands development). These human cohorts represent the parts of the population residing in the AOSR, that rely heavily on locally sourced food items, but complements those items with market foods. They also potentially smoke at the average level of the local First Nations population. The rationale for focusing on this part of the population is that they are likely to experience the highest potential exposure to locally emitted PAHs. Importantly, by focusing assessment on the worst case, this analysis is not a probabilistic exposure assessment.
Because we were interested in simulating the situation during the time period of oil sands development, we only present results for 1967 forward. No spin-up was deemed necessary, i.e., we assumed that cohorts born prior to 1967 were not exposed to PAHs until 1967. They were assumed to be living in an environment that was relatively “clean” of PAHs. This implies that we ignore PAHs arriving in the AOSR from elsewhere by long range transport and PAHs from local natural sources, such as bitumen outcrops32 or wildfires,33 not just prior to 1967, but throughout the entire simulation period. We also assume that prior to 1967 intake of PAHs with market food was negligible.
Because pregnant woman and infants are often more vulnerable to contaminant exposure, we modeled primiparous females who give birth at age 29 to one child that is at chemical equilibrium with the mother.34 For the first 6 months of life, infants exclusively consume breastmilk that is also assumed to be at chemical equilibrium with the body tissue of the mother.35 After 6 months, humans consume a regular diet that is scaled for age according to the assumption that 25 year-olds have the highest food intake rate, and is also corrected for gastrointestinal absorption efficiency. Whole-body biotransformation occurs according to first-order kinetics. As detailed in Section S2.2 in the ESI,† biotransformation rate constants for humans were estimated considering values obtained by two prediction methods and by allometrical scaling of biotransformation rate constants measured in fish and rats (specific values listed in ESI Table S1†). These rate constants were assumed to be constant over an individual's lifespan.
2.2.2 Local food and environment.
ACC-Human was modified to represent a food chain reflecting locally-sourced TF consumption in the AOSR. In the new “ACC-Human AOSR”, selected TF were those with the highest reported consumption rates in a recent survey of food consumption habits among First Nations people in northern boreal Alberta.21 In the modified model, humans consume moose (Alces alces), northern pike (Esox lucius), whitefish (Coregonus clupeaformis), mallard duck (Anas platyrhynchos), ruffed grouse (Bonasa umbellus), berries (Vaccinium spp., Amelanchier alnifolia), and mint (Mentha spp.). Prey items were also added for the moose (willow, Salix spp.), ruffed grouse (lodgepole pine, Pinus contorta), and mallard (cattail, Typha latifolia, plankton), while the two fish species were incorporated into existing sub-food chains for fish (northern pike – northern pike, whitefish, plankton, benthos; whitefish – plankton). Biotransformation rate constants for model organisms were estimated using the allometric scaling method described in 2.2.1 for humans, except for plants, which were assumed to metabolize PAHs at the same rate as ryegrass.36
Human consumption rates were daily averages reported for “heavy” TF consumers aged 19 to 50 (Table 1 in ref. 21). Because 73% of survey respondents reported using tap water as drinking water, and 62% of households reported receiving their water from a treatment plant, we assumed that the source of this drinking water was local surface water. Soil ingestion was included in the model using the median soil ingestion rate reported for a rural population in Northern Alberta practicing a wilderness lifestyle.37 Soil ingestion was restricted to those aged 12 to 60. Similar to food, soil and water ingestion were scaled according to age and gender, and also corrected for gastrointestinal absorption efficiency. Further model details, including equations describing model transport pathways, estimation of biotransformation rate constants, and model validation details can be found in the ESI.†
Table 1 Comparison of the daily intakes of three PAHs in ng per day per kg body weight estimated for different cohorts and age groups of Northern Alberta residents with a high intake of TF with tolerable daily intake (TDI) thresholds recommended by Health Canada54 and the Michigan Department of Environmental Quality55
Chemical |
PHE |
PYR |
BaP |
Cohort birth year |
1947 |
1967 |
1987 |
1947 |
1967 |
1987 |
1947 |
1967 |
1987 |
Infancy: 0–1 year old, body weight: 3.5 kg.
Toddler: 1–3 years old, body weight: 12 kg.
Childhood & adolescence: 3–18 years old, body weight: 45 kg.
Adulthood: 20–22 years old, body weight: 60 kg.
Ref. 55.
Ref. 54.
|
Infancya |
|
44 |
46 |
|
8.8 |
9.7 |
|
0.87 |
1.7 |
Toddlerb |
|
31 |
32 |
|
6.1 |
6.8 |
|
0.55 |
1.2 |
Childhood & adolescencec |
|
22 |
23 |
|
4.5 |
5.1 |
|
0.61 |
1.1 |
Adulthoodd |
45 |
46 |
47 |
11 |
11 |
12 |
1.8 |
2.3 |
2.9 |
TDI |
7100e |
30 000f |
67f |
2.2.3 Ingestion of PAHs with market foods.
Intake of PAHs with market food was estimated by multiplying consumption rates, scaled for age as in ACC-Human, with PAH concentrations in market foods taken from the literature. Intake was then converted to uptake using the gut absorption efficiency implemented in ACC-Human. The market foods consumed in the greatest quantities by First Nations adults in northern boreal Alberta are water, coffee, tea, soup, potatoes, other vegetables, pasta/noodles, pork, chicken, and fruit (Table 1 in ref. 21). We assumed the market food intake rates were the same for both males and females. PAH concentrations in these foods were taken from the literature (Table S14†), with an attempt to use only concentrations in foods found in Canadian markets if possible. Detailed information on the sources and methods used to obtain PAH concentrations in all of these food items is provided in the ESI.† We used concentrations in raw foods to facilitate a more valid comparison with the raw TF concentrations calculated by ACC-Human AOSR.
2.2.4 Smoking.
First Nations adults aged 19 to 50 in Alberta were reported to smoke an average of 9 cigarettes per day.21 Intake rates of PHE, PYR and BaP due to smoking were calculated using the smoking rate and mass of PAH consumed per cigarette. The latter is based on Vu et al.,38 who report the average yields of PAHs per cigarette in mainstream smoke across 50 different cigarette brands in the United States and two methods of smoking (varying puff interval, puff duration, etc.) (Table S14†). ACC-Human corrects inhalation rates with an absorption factor (0.7, constant across gender and age) that represents the fraction of inhaled air that is in contact/equilibrium with blood.29 Inhalation is also corrected with a scaling factor that accounts for differences in inhalation rates across age, assuming that 25 year-olds have the greatest inhalation rates. The estimated PAH intake rates due to smoking were also reduced by these same factors. In addition, in consideration of legal age restrictions for the purchase of cigarettes in Alberta, PAH intake via smoking in the model was restricted to those aged 18 and over.
3. Results & discussion
3.1 Model evaluation
We needed to establish confidence in the results of the ACC-Human simulations, before we could use them in an overall exposure assessment. We sought to do this by collecting all of the empirical measurements of PAHs in traditionally consumed food items from Northern Alberta and comparing them with predictions. Sometimes this effort was hampered by data being reported as the sum of PAHs or as “not detected”, with limits of detection not always reported. Of particular value were the studies on PAHs in fishes from the Athabasca and Slave River up- and downstream of the AOSR39 and in fish, moose, mallard, and grouse collected from the Bigstone Cree Nation in Northern Alberta.40 We also used concentration data on pooled plant samples4 and moose scat41 from the AOSR.
These comparisons are presented in Tables S8–S12 in the ESI.† Overall, the level of agreement was better for aquatic species than for organisms in the terrestrial environment. Specifically, the predicted levels in whitefish and northern pike are in excellent agreement with the data reported for these fish species from the Athabasca and Slave Rivers39 and the Wabasca Lakes40 (Table S9†). For terrestrial plants (Table S11†) and the mammalian (Table S10†) and avian (Table S12†) herbivores eating them, the model tended to predict levels that are at the lower end of, or slightly lower than, the range of measured values. However, only in grouse was this discrepancy notable, i.e., the predictions were lower than the measurements by an order of magnitude (Table S12†). A potential reason for this discrepancy could be that grouse are not solely feeding on pine, as is assumed in the model, but also on worms and insects that are potentially more contaminated with PAHs.
To the best of our knowledge no biomonitoring data on PAHs in human samples from the AOSR are available. While the Alberta Biomonitoring Program included PAHs among the compounds targeted in serum samples collected during phase 1, they were not detected above limits of detection.42 To at least confirm the plausibility of the PAH residue concentrations calculated by our approach, we compared the predicted, age-matched exposures in women with measurements in human breastmilk from Turkey,43 the Czech Republic44 and the USA.45 The mean of the predicted PHE and PYR concentrations (0.7 and 0.17 ng per g lipid for non-smokers, and 1.36 and 0.36 for smokers, respectively) are approximately one order magnitude lower than the measurements (PHE: 9.4–20 ng per g lipid, PYR: 0.67–5.4 ng per g lipid for non-smokers; PHE: 24 ng per g lipid, PYR: 8.2 ng per g lipid for smokers). For BaP, the predictions for non-smokers (0.05 to 0.08 ng per g lipid) are about three times lower than the measurements (0.14–0.27 ng per g lipid). However, predicted (0.16 ng per g lipid) and measured (0.27 ng per g lipid) BaP exposures for smokers are similar. More details are provided in Table S13.† Because predictions and measurements apply to very different human populations, it is not possible to conclusively explain why the predictions are lower. However, in addition to dietary contamination differences between human populations, discrepancies may be related to an overestimation of the human biotransformation rate constants of the PAHs. Importantly, predicted PAH concentrations differed between smokers and non-smokers by a factor of ∼2, similar to the differences in average concentrations of PHE, PYR, and BaP in human breastmilk between smokers and non-smokers in Turkey, which ranged from 1.1 to 1.6-fold.43 It is also similar to the average difference of 1.3 to 4.2 times found between breastmilk concentrations of PHE and PYR in smoking versus non-smoking women in Italy.46
3.2 Temporal trends in simulated body burdens
The time trends in lipid-normalized PAH body burdens for the three cohorts of women born in 1947, 1967, and 1987 are presented in Fig. 2. The trends in males were almost identical, except they did not include the decrease in body burdens during nursing.
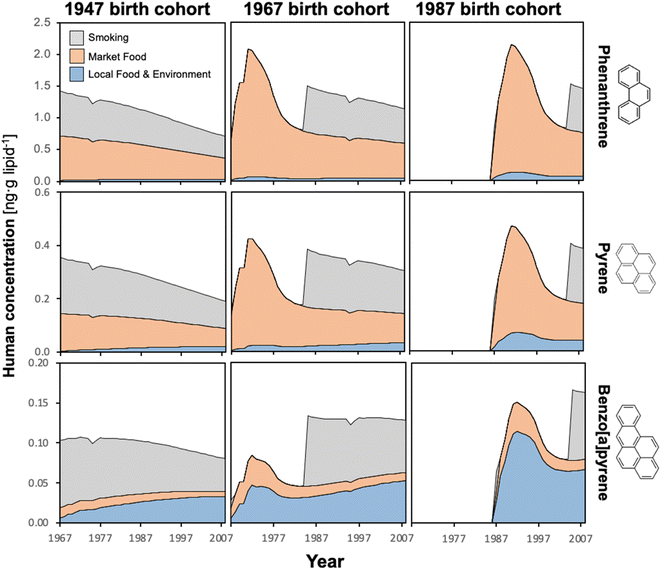 |
| Fig. 2 Time trends of phenanthrene (top), pyrene (middle), and benzo[a]pyrene (bottom) body burdens [ng per g lipid] in various age cohorts (birth year, left: 1947, middle: 1967, right: 1987). Colors represent contributions from the assessed PAH exposure sources: local food and environment (blue), market food (orange), and cigarettes (grey). The data have been smoothened to remove seasonal variability. | |
3.2.1 The age dependence of the simulated body burden arising from dietary intake.
The sum of the blue and orange areas in Fig. 2 represents the body burden of a PAH arising mostly from dietary intake (intake of air, water and soil makes a negligible contribution). This body burden for all three PAHs is calculated to increase in infancy, peak at an age around 3 to 5, then decrease rapidly during adolescence, with minor changes during the remainder of life. Levels of PHE and PYR decline slightly, whereas those of BaP trend upwards during adulthood. This is an age trend predicted to occur for all but the most persistent organic contaminants taken up from the diet.47 This trend arises as a result of the age dependence of two factors. First, changes in the rate of dietary intake are mostly responsible for the increase in body burden in the first few years of life and also explain the slight decrease during adulthood. Second, the rapid increase in the amount of body lipids during adolescence, with a lifetime minimum in body lipid occurring during childhood, is the reason for the rapid decrease in body burden during adolescence, i.e., it is the result of a dilution effect.
3.2.2 The relative contribution of market food and local TF to dietary intake.
Comparing the orange and blue areas in Fig. 2 we can assess the relative contribution of market and TF to human body burdens. The model simulations suggest that market food dominates the dietary intake of PHE and PYR, whereas TF is the dominant source of exposure to BaP. Over time, the relative importance of TF as a source of PAH exposure increases, i.e., the blue areas are slightly larger for the more recent birth cohorts, whereas the orange areas remain the same size. This is a result of the assumption that market food contamination during the entire simulation period stayed constant, whereas the contamination of the local foods increased gradually with the increase in oil sands development. In reality it is likely that the contamination of market foods with PAHs declined during the simulated time period, because of reduction in PAH air concentrations48,49 in response to emission reductions.50 Because market foods dominate dietary intake of PHE and PYR, human dietary exposure to these PAHs did not increase notably over time, despite the increasing contamination of local foodstuffs. However, because of the importance of TF to BaP intake, the model predicts that (i) BaP body burdens slightly increase with age in every birth cohort, and (ii) strongly increase from one birth cohort to another. For example, the model suggests that the 1987 cohort experiences almost triple the childhood exposure to BaP compared to the 1967 cohort. It is important to recall that these simulations assume no change in the relative intake of market and TF between cohorts, because they focus on the part of the First Nations population that continued to have a relatively high reliance on TF. Quantifying the effect of the intergenerational shift from TF to market food occurring at the population level would require a different modelling scenario.51
3.2.3 The PAH exposure added by smoking.
The grey areas in Fig. 2 show the added body burden of PAHs if the human is a smoker. Smoking resulted in an approximate two-fold increase in simulated concentrations of all three PAHs. In other words, someone smoking at the average rate of First Nations in Alberta doubles their PAH exposure compared to dietary intake alone. The impact of age-specific lifestyle behaviors, most notably smoking, on PAH uptake has been suggested by previous observations of concentration differences in urinary PAH metabolites in different age cohorts in Australia.52
While smoking adds to the PAH exposure in adulthood, the model also indicates added exposure during nursing, coming from smoking mothers with elevated PAH body burdens themselves. Incidentally, the loss of PAHs in the nursing mother is evident in a slight decline in the calculated body burden at age 29. This effect was also more pronounced for infants nursing in 1987, which is an artifact of the assumption that individuals born prior to 1967 were assumed to not experience any PAH uptake prior to this date. As a result, mothers giving birth in 1987 had a longer history of PAH exposure, including via smoking, relative to mothers that gave birth in 1967.
3.2.4 The seasonality in PAH body burden.
Following seasonal concentration patterns in abiotic environmental media (greater concentrations in winter relative to summer), seasonal patterns in body burdens were also evident for all three chemicals (not shown in Fig. 2, which displays temporally smoothened data). This variability was most obvious for BaP concentrations, which were most affected by local food concentrations, which were in turn a result of local environmental contamination. On the other hand, the seasonality in PHE and PYR body burdens was much less pronounced. This is because there was no seasonality presumed in the contamination of first-hand cigarette smoke and market foods, which were overwhelmingly the major contributors to PHE and PYR uptake. Seasonality in human PAH body burdens has been previously suggested by observations of greater PAH concentrations in human breastmilk of non-smoking Czech females in winter relative to summer, though this effect was more pronounced for PHE and PYR, and not observed at all for BaP.44
3.3 Items dominating PAH intake
Fig. 2 only distinguishes between three types of PAH intake: market foods, TF combined with intake with air, water and soil, and smoking. Fig. 3 provides more finely resolved information on which food items are more important for PAH intake. Of the local food sources of PAHs, fish were most important for all three chemicals (Fig. 3). This is because residents of Northern Alberta report eating far more fish lipids than lipids derived from plant-eating animals (moose and birds),21 and biotransformation is assumed to be quite fast in plants but non-existent in zooplankton. Importantly, while our model's underestimation of terrestrial food chain contamination with PAHs (see Section 3.1) also results in an underestimation of human PAH intake from plants, birds and moose, the dominance of PAH uptake from fish is so large, that even an order of magnitude underestimation of the contamination of terrestrial foods has a very limited impact on the estimated overall dietary intake.
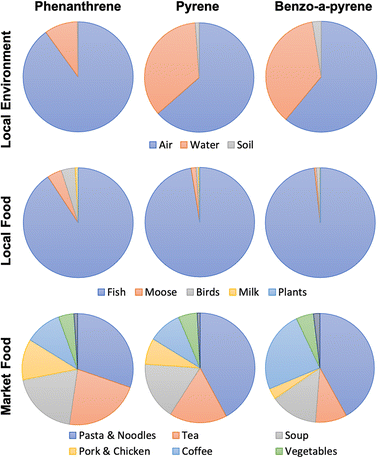 |
| Fig. 3 Average fractional contribution to uptake fluxes from 1967 to 2009 of phenanthrene, pyrene, and benzo[a]pyrene from sources in the local environment, local foods, and market foods for individuals born in 1947, 1967, and 1987. | |
PAH uptake via market foods was comprised mostly of pasta/noodles, followed by coffee and tea (Fig. 3). Amongst all market foods, consumption rates of pasta and noodles were not unusually high. However, PAH contamination of pasta/noodles was at least 2.4 to 5 times greater than all other market foods (Table S14†). The estimated PAH concentrations in pasta/noodles were based on only two studies that differed by a factor of 10 in their measurements of PAH content. In coffee and tea brews, PAH contamination results from environmental uptake followed by processing techniques such as roasting (additional contamination) and drying (concentration of existing contamination), and transfer of a fraction of PAHs into the final brewed liquid.53 Due to the low transfer efficiencies from leaves and beans to brews, there was little difference in PAH contamination of coffee and tea brews relative to other market foods; rather, it was the relatively high intake rates of the brews that resulted in their relatively large contribution to human PAH uptake.
Intake of PAHs associated with inhaling ambient air, and ingestion of local water and soils was calculated to be too small to be visible in Fig. 2. Among these three pathways, inhalation of PAHs is largest, whereas PAH ingestion with soil is very limited (Fig. 3). Overall, however, these pathways make a negligible contribution to human PAH exposure.
3.4 Total estimated intake versus toxicological reference values
In order to assess whether the intake of PAHs estimated with our modelling approach for those with a high consumption of TF reaches levels of toxicological concern, we compared the daily intakes estimated by the model with Tolerable Daily Intake (TDI) levels recommended for risk assessment in Canada54 and in the US55 (Table 1). Because we are not aware whether age-class specific TDI for PAH exist, we used those values for all age classes.
While the estimated daily intakes for PHE and PYR are more than two orders of magnitude below the TDI, the daily intake of BaP estimated for young adults born in 1987 is only ∼23 times lower than the TDI. It is noteworthy that BaP, for which the margin of safety is the smallest, is also the PAH for which we estimated that TF makes the largest contribution and therefore the estimated exposure increases in response to expanding oil sands development. Overall, however, the comparison in Table 1 indicates that intake of the three investigated PAHs does not presently reach levels of concern.
3.5 Model limitations
While the modeling approach used in the current study offers certain advantages over entirely empirical methods, there are also some associated limitations to consider. These limitations stem from elements both internal and external to the model.
The total dietary contribution to PAH uptake was likely underestimated as foods were considered in their raw state. Smoking of fish and meat is a common preparation method among the First Nations of Northern Alberta and likely will lead to an increase in the PAHs levels. Since PAH contamination of food is induced by pyrolysis of fat and fuel sources, the fat content of meat, cooking time, and exposure to smoke affects the final PAH content of prepared meals.56,57 Specifically, high temperature cooking methods result in the production of PAHs, which are subsequently deposited on meat surfaces.58 For example, Golzadeh et al.40 reported that PAHs were higher in smoked moose meat than in non-smoked animal samples. Not only does PAH contamination of raw tissue increase due to cooking, but it can also increase if stored in close proximity to food preparation facilities such as smokehouses.59 It is conceivable that some of the conclusion derived in this study may have to be revisited if the increase in the PAH content during food preparation is taken into account. If we assume that traditional and market foods experience similar changes in PAH concentrations during preparation and that food preparation methods did not change over the investigated time period, many of the model results, such as the relative importance of traditional and market foods and the temporal trends in dietary exposure should not be strongly affected by the failure to account for the effect of food preparation.
PAH uptake due to smoking is likely underestimated, particularly for PHE, as Vu et al.38 only report particle-associated PAH in firsthand smoke. Presumably, the inhaled vapor phase of cigarette smoke also contains PAHs, including a greater fraction of more volatile PAHs such as PHE, relative to BaP. We also do not account for second and third hand smoke exposure, e.g., among infants and children, even though it is known to contribute to PAH exposure.60,61 Another possible reason for underestimating exposure is that we do not take local PAH emissions in the AOSR in account that are not related to the oil sand developments. Examples are wildfires and natural bitumen outcrops.32,33
Considering the 10-fold difference in the only available measurements of pasta, the contamination of popular market foods such as pasta and soup available in Canadian grocery stores is highly uncertain. Uncertainty also stems from potential exposure pathways unaccounted for in the current study. Inhalation in the indoor environment has been identified as potentially important for PHE exposure, less important for PYR exposure, and negligible for BaP exposure62,63 due to indoor sources such as cooking and burning of candles and incense.64 Also, certain types of home heating with solid fuels could influence indoor inhalation exposure to PAHs.65 This was not considered in our study, even though wood stoves may be important for winter heating in Northern Alberta and the prevalence of their use may have changed during the simulation period. In addition, depending on individual habits, certain food preparation practices such as barbecuing66 can increase inhalation and dermal exposure to PAHs, of which the latter pathway was not considered here. While barbecuing likely occurs on a limited and sporadic basis each year, it is uncertain how frequently smokehouse activities occur in First Nations communities in Northern Alberta.
4. Conclusion
Given population-specific food consumption and cigarette smoking rates, our model approach provides insight into the relative importance of various PAH exposure pathways and how these vary across chemicals, age cohorts, and individual lifetimes. In the present study we considered a First Nations population residing in the Athabasca oil sands region that consumed market foods and smoked cigarettes at “average” reported rates and consumed local foods at “heavy” rates. The estimations presented here suggest that oil sands operations are unlikely to contribute notably to human exposure to the lighter PAHs, such as phenanthrene and pyrene. On the other hand, the simulations suggest that local emissions may be responsible for the majority of dietary BaP ingestion by those who rely heavily on local foods. This intake may even match the intake associated with smoking an average of 9 cigarettes a day. The simulations further suggest that BaP exposure is mostly due to fish consumption and much less due to the consumption of terrestrial TF such as moose, grouse, and mallard. The estimated intake from locally sourced food is predicted to increase over the simulation period and is expected to continue doing so with increasing industrial activity in the oil sands. While the estimated BaP intake is currently below TDI reference values, emissions are expected to increase in the future and BaP is only one of many potentially toxic/carcinogenic compounds emitted by oil sands operations.67
Among the advantages of the presented approach are the ability to apply it to other organic contaminants emitted by oil sands operations, as long as emission rates, physical chemical properties and degradation and biotransformation rates can be estimated. For example, Golzadeh et al.40 noted that concentrations of alkylated PAHs exceeded those of unsubstituted PAHs in all TF samples from the Bigstone Cree Nation in Northern Alberta. Other examples of contaminants present in the AOSR are substituted PAHs, such as oxygenated and nitrated PAHs.68 Furthermore, the approach's predictive capabilities could enable the simulation of the contaminant exposures arising from future oil sands development scenarios (e.g., as part of environmental risk assessments) and of the effectiveness of emissions abatement measures aimed at keeping such exposures below toxicological reference values.
The model results also highlight key sources of uncertainty, and thus parameters that require refining with empirical data. The use of raw food concentrations for meat in particular likely leads to the underestimation of the importance of dietary ingestion. Future work could focus on quantifying the role local food preparation techniques play in modifying PAH concentrations in TF. Also, measurements of body burdens of PAHs or their metabolites in AOSR residents would allow for a more robust assessment of the plausibility of modelled exposures. Considering the estimated growth trajectory of oil sands operations and likely concomitant increases in local chemical emissions, it is important to address these key uncertainties in order to produce a multi-pathway exposure assessment that is as informative as possible.
Conflicts of interest
The authors declare no conflict of interest.
Acknowledgements
Thank you to Alessandro Sangion and Moosa Said for assistance with model validation and James M. Armitage for providing comments on the parameterization of the human model. Tom Harner, Ryan Abel and David Spink are acknowledged for providing comments on a draft manuscript. Funding from a Grant and Contribution Agreement of Environment and Climate Change Canada is gratefully acknowledged.
References
-
Canada Energy Regulator, Provincial and Territorial Energy Profiles – Canada, accessed September 25, 2022 at: https://www.cer-rec.gc.ca/en/data-analysis/energy-markets/provincial-territorial-energy-profiles/provincial-territorial-energy-profiles-canada.html Search PubMed.
- J. Kurek, J. L. Kirk, D. C. G. Muir, X. Wang, M. S. Evans and J. P. Smol, Legacy of a half century of Athabasca oil sands development recorded by lake ecosystems, Proc. Natl. Acad. Sci. U. S. A., 2013, 110, 1761–1766 CrossRef CAS PubMed.
- T. Harner, C. Rauert, D. Muir, J. Schuster, Y. M. Hsu, L. Zhang, G. Marson, J. Watson, J. Ahad, S. Cho, N. Jariyasopit, J. Kirk, J. Korosi, M. Landis, J. Martin, Y. Zhang, K. Fernie, G. Wentworth, A. Wnorowski, E. Dabek, J. P. Charland, B. Pauli, F. Wania, E. Galarneau, I. Cheng, P. Makar, C. Whaley, J. Chow and X. Wang, Air synthesis review: polycyclic aromatic compounds in the oil sands region, Environ. Rev., 2018, 26, 430–468 CrossRef CAS.
- C. Boutin and D. J. Carpenter, Assessment of wetland/upland vegetation communities and evaluation of soil-plant contamination by polycyclic aromatic hydrocarbons and trace metals in regions near oil sands mining in Alberta, Sci. Total Environ., 2017, 576, 829–839 CrossRef CAS PubMed.
- E. N. Kelly, J. W. Short, D. W. Schindler, P. V. Hodson, M. Ma, A. K. Kwan and B. L. Fortin, Oil sands development contributes polycyclic aromatic compounds to the Athabasca River and its tributaries, Proc. Natl. Acad. Sci. U. S. A., 2009, 106, 22346–22351 CrossRef CAS PubMed.
- J. K. Schuster, T. Harner, K. Su, C. Mihele and A. Eng, First results from the oil sands passive air monitoring network for polycyclic aromatic compounds, Environ. Sci. Technol., 2015, 49, 2991–2998 CrossRef CAS PubMed.
- J. K. Schuster, T. Harner, K. Su, A. Eng, A. Wnorowski and J. P. Charland, Temporal and spatial trends of polycyclic aromatic compounds in air across the Athabasca Oil Sands Region reflect inputs from open pit mining and forest fires, Environ. Sci. Technol. Lett., 2019, 6, 178–183 CrossRef CAS.
- A. Wnorowski, Y. A. Aklilu, T. Harner, J. K. Schuster and J. P. Charland, Polycyclic aromatic compounds in ambient air in the surface minable area of Athabasca oil sands in Alberta (Canada), Atmos. Environ., 2021, 244, 117897 CrossRef CAS.
- K. J. Fernie, S. C. Marteinson, C. Soos, D. Chen, L. Cruz-Martizen and J. E. G. Smits, Reproductive and developmental changes in tree swallows (Tachycineta bicolor) are influenced by multiple stressors, including polycyclic aromatic compounds, in the Athabasca Oil Sands, Environ. Pollut., 2018, 238, 931–941 CrossRef CAS.
- J. L. Parrott, J. R. Marentette, L. M. Hewitt, M. E. McMaster, P. L. Gillis, W. P. Norwood, J. L. Kirk, K. M. Peru, J. V. Headley, Z. Wang and R. A. Frank, Meltwater from snow contaminated by oil sands emissions is toxic to larval fish, but not spring river water, Sci. Total Environ., 2018, 625, 264–274 CrossRef CAS.
- J. Rodríguez-Estival and J. E. G. Smits, Small mammals as sentinels of oil sands related contaminants and health effects in northeastern Alberta, Canada, Ecotoxicol. Environ. Saf., 2016, 124, 285–295 CrossRef.
- L. Eggertson, High cancer rates among Fort Chipewyan residents, CMAJ, 2009, 181(12), E309, DOI:10.1503/cmaj.090248.
-
Y. Chen, Cancer incidence in Fort Chipewyan, Alberta: 1995-2006, Alberta Health Services report, 2009, p. 91, accessed October 14, 2022 at: https://www.osti.gov/etdeweb/biblio/21221990 Search PubMed.
- D. J. Tenenbaum, Oil sands development: A health risk worth taking?, Environ. Health Perspect., 2009, 117(4), A150–A156 Search PubMed.
-
D. Droitsch and T. Simieritsch, Canadian Aboriginal Concerns with Oils Sands, A compilation of key issues, resolutions and legal activities, Briefing Note, Pembina Institute, Sept. 2010, 10 pages, https://www.pembina.org/reports/briefingnoteosfntoursep10.pdf Search PubMed.
- K.-H. Kim, S. A. Jahan, E. Kabir and R. J. C. Brown, A review of airborne polycyclic aromatic hydrocarbons (PAHs) and their human health effects, Environ. Int., 2013, 60, 71–80 CrossRef CAS PubMed.
- G. M. Irvine, J. M. Blais, J. R. Doyle, L. E. Kimpe and P. A. White, Cancer risk to First Nations' people from exposure to polycyclic aromatic hydrocarbons near in-situ bitumen extraction in Cold Lake, Alberta, Environ. Health, 2014, 13, 7, DOI:10.1186/1476-069X-13-7.
- E. M. Power, Conceptualizing food security for Aboriginal people in Canada, Can. J. Public Health, 2008, 99(2), 95–97 CrossRef PubMed.
- J. Edwards, Oil sands pollutants in traditional foods, CMAJ, 2014, 186(12), E444, DOI:10.1503/cmaj.109-4859.
- H. V. Kuhnlein and O. Receveur, Dietary change and traditional food systems of Indigenous peoples, Annu. Rev. Nutr., 1996, 16, 417–442 CrossRef CAS PubMed.
-
L. Chan, O. Receveur, M. Batal, W. David, H. Schwartz, A. Ing, K. Fediuk and C. Tikhonov, First Nations Food, Nutrition and Environment Study (FNFNES): Results from Alberta 2013, University of Ottawa, Ottawa, 2016 Search PubMed.
- Y. S. Ding, J. S. Trommel, X. J. Yan, D. Ashley and C. H. Watson, Determination of 14 polycyclic aromatic hydrocarbons in mainstream smoke from domestic cigarettes, Environ. Sci. Technol., 2005, 39, 471–478 CrossRef CAS PubMed.
- K. Suzuki and J. Yoshinaga, Inhalation and dietary exposure to polycyclic aromatic hydrocarbons and urinary 1-hydroxypyrene in non-smoking university students, Int. Arch. Occup. Environ. Health, 2007, 81, 115–121 CrossRef CAS PubMed.
- A. Vyskocil, Z. Fiala, V. Chénier, L. Krajak, E. Ettlerova, C. Bukac, C. Viau and S. Emminger, Assessment of multipathway exposure of small children to PAH, Environ. Toxicol. Pharmacol., 2000, 8, 111–118 CrossRef CAS PubMed.
- T. J. Buckley and P. J. Lioy, An examination of the time course from human dietary exposure to polycyclic aromatic hydrocarbons to urinary elimination of 1-hydroxypyrene, Br. J. Ind. Med., 1992, 49, 113–124 CAS.
- Y. Zhang, J. Ding, G. Shen, J. Zhong, C. Wang, S. Wei, C. Chen, Y. Chen, Y. Lu, H. Shen, W. Li, Y. Huang, H. Chen, S. Su, N. Lin, X. Wang, W. Liu and S. Tao, Dietary and inhalation exposure to polycyclic aromatic hydrocarbons and urinary excretion of monohydroxy metabolites – A controlled case study in Beijing, China, Environ. Pollut., 2014, 184, 515–522 CrossRef CAS PubMed.
- C. A. Menzie, B. B. Potocki and J. Santodonato, Exposure to carcinogenic PAHs in the environment, Environ. Sci. Technol., 1992, 26, 1278–1283 CrossRef CAS.
-
F. Wania, L. Li and M. S. McLachlan, Mechanistically modeling human exposure to persistent organic pollutants, in From Concepts to Insights: A New Paradigm of Environmental Chemistry and Toxicology, ed. X. Li and G. Jiang, Springer-Verlag, 2019, pp. 115–128 Search PubMed.
- G. Czub and M. S. McLachlan, A food chain model to predict the levels of lipophilic organic contaminants in humans, Environ. Toxicol. Chem., 2004, 23, 2356–2366 CrossRef CAS PubMed.
- A. Parajulee and F. Wania, Evaluating officially reported polycyclic aromatic hydrocarbon emissions in the Athabasca oil sands region with a multimedia fate model, Proc. Natl. Acad. Sci. U. S. A., 2014, 111, 3344–3349 CrossRef CAS PubMed.
- M. J. Binnington, M. S. Curren, C. L. Quinn, J. M. Armitage, J. A. Arnot, H. M. Chan and F. Wania, Mechanistic polychlorinated biphenyl exposure modeling of mothers in the Canadian Arctic: the challenge of reliably establishing dietary composition, Environ. Int., 2016, 92–93, 256–268 CrossRef CAS PubMed.
- I. G. Droppo, P. di Cenzo, J. Parrott and J. Power, The Alberta oil sands eroded bitumen/sediment transitional journey: Influence on sediment transport dynamics, PAH signatures and toxicological effect, Sci. Total Environ., 2019, 677, 718–731 CrossRef CAS PubMed.
- G. R. Wentworth, Y. A. Aklilu, M. S. Landis and Y. M. Hsu, Impacts of a large boreal wildfire on ground level atmospheric concentrations of PAHs, VOCs and ozone, Atmos. Environ., 2018, 178, 19–30 CrossRef CAS PubMed.
- X. Zhang, X. Li, Y. Jing, F. Fang, X. Zhang, B. Lei and Y. Yu, Transplacental transfer of polycyclic aromatic hydrocarbons in paired samples of maternal serum, umbilical cord serum, and placenta in Shanghai, China, Environ. Pollut., 2017, 222, 267–275 CrossRef CAS PubMed.
- H. L. Tsang, S. Wu, C. K. M. Leung, S. Tao and M. H. Wong, Body burden of POPs of Hong Kong residents, based on human milk, maternal and cord serum, Environ. Int., 2011, 37, 142–151 CrossRef CAS PubMed.
- Y. Gao, W. Ling and M. H. Wong, Plant-accelerated dissipation of phenanthrene and pyrene from water in the presence of a nonionic-surfactant, Chemosphere, 2006, 63, 1560–1567 CrossRef CAS PubMed.
- G. Irvine, J. R. Doyle, P. A. White and J. M. Blais, Soil ingestion rate determination in a rural population of Alberta, Canada practicing a wilderness lifestyle, Sci. Total Environ., 2014, 470–471, 138–146 CrossRef CAS.
- A. T. Vu, K. M. Taylor, M. R. Holman, Y. S. Ding, B. Hearn and C. H. Watson, Polycyclic aromatic hydrocarbons in mainstream smoke of popular US cigarettes, Chem. Res. Toxicol., 2015, 28, 1616–1626 Search PubMed.
- E. Ohiozebau, B. Tendler, G. Codling, E. Kelly, J. P. Giesy and P. D. Jones, Potential health risks posed by polycyclic aromatic hydrocarbons in muscle tissues of fishes from the Athabasca and Slave Rivers, Canada, Environ. Geochem. Health, 2017, 39, 139–160 Search PubMed.
- N. Golzadeh, B. D. Barst, J. M. Baker, J. C. Auger and M. A. McKinney, Alkylated polycyclic aromatic hydrocarbons are the largest contributor to polycyclic aromatic compound concentrations in traditional foods of the Bigstone Cree Nation in Alberta, Canada, Environ. Pollut., 2021, 275, 116625 CrossRef CAS.
- J. I. Lundin, J. A. Riffell and S. K. Wasser, Polycyclic aromatic hydrocarbons in caribou, moose, and wolf scat samples from three areas of the Alberta oil sands, Environ. Pollut., 2015, 206, 527–534 CrossRef CAS.
- A. M. MacDonald, S. Gabos, S. Braakman, L. Cheperdak, B. Lee, S. E. Hrudey, X. C. Le, X.-F. Li, R. Mandal, J. W. Martin, D. Schopflocher, M. E. Lyon, P.-Y. Cheung, F. Ackah, J. A. Graydon, M. Reichert, A. W. Lyon, J. Jarrell, G. Benadé, C. Charlton and D. W. Kinniburgh, Maternal and child biomonitoring strategies and levels of exposure in western Canada during the past seventeen years: The Alberta Biomonitoring Program: 2005–2021, Int. J. Hyg. Environ. Health, 2022, 244, 113990 CrossRef CAS.
- I. Çok, B. Mazmanci, M. A. Mazmanci, C. Turgut, B. Henkelmann and K.-W. Schramm, Analysis of human milk to assess exposure to PAHs, PCBs, and organochlorine pesticides in the vicinity Mediterranean city Mersin, Turkey, Environ. Int., 2012, 40, 63–69 CrossRef PubMed.
- J. Pulkrabova, M. Stupak, A. Svarcova, P. Rossner, A. Rossnerova, A. Ambroz, R. Sram and J. Hajslova, Relationship between atmospheric pollution in the residential area and concentrations of polycyclic aromatic hydrocarbons (PAHs) in human breast milk, Sci. Total Environ., 2016, 562, 640–647 CrossRef CAS PubMed.
- S. R. Kim, R. U. Halden and T. J. Buckley, Polycyclic aromatic hydrocarbons in human milk of nonsmoking U.S. women, Environ. Sci. Technol., 2008, 42, 2663–2667 CrossRef CAS PubMed.
- L. Zanieri, P. Galvan, L. Checchini, A. Cincinelli, L. Lepri, G. P. Donzelli and M. Del Bubba, Polycyclic aromatic hydrocarbons (PAHs) in human milk from Italian women: Influence of cigarette smoking and residential area, Chemosphere, 2007, 67, 1265–1274 CrossRef CAS PubMed.
- C. L. Quinn and F. Wania, Understanding differences in the body burden-age relationships of bioaccumulating contaminants based on population cross-sections versus individuals, Environ. Health Perspect., 2012, 120, 554–559 CrossRef CAS PubMed.
- S. N. Meijer, A. J. Sweetman, C. J. Halsall and K. C. Jones, Temporal trends of polycyclic aromatic hydrocarbons in the U.K. atmosphere: 1991–2005, Environ. Sci. Technol., 2008, 42, 3213–3218 CrossRef CAS PubMed.
- M. Venier and R. A. Hites, Time trend analysis of atmospheric POPs concentrations in the Great Lakes region since 1990, Environ. Sci. Technol., 2010, 44, 8050–8055 CrossRef CAS.
- H. Shen, Y. Huang, R. Wang, D. Zhu, W. Li, G. Shen, B. Wang, Y. Zhang, Y. Chen, Y. Lu, H. Chen, T. Li, K. Sun, B. Li, W. Liu, J. Liu and S. Tao, Global atmospheric emissions of polycyclic aromatic hydrocarbons from 1960 to 2008 and future predictions, Environ. Sci. Technol., 2013, 47, 6415–6424 CrossRef CAS PubMed.
- C. L. Quinn, J. M. Armitage, K. Breivik and F. Wania, A methodology for evaluating the influence of diets and intergenerational dietary transitions on historic and future human exposure to persistent organic pollutants in the Arctic, Environ. Int., 2012, 49, 83–91 CrossRef CAS PubMed.
- P. K. Thai, A. L. Heffernan, L.-M. Toms, Z. Li, A. M. Calafat, P. Hobson, S. Broomhall and J. F. Mueller, Monitoring exposure to polycyclic aromatic hydrocarbons in an Australian population using pooled urine samples, Environ. Int., 2016, 88, 30–35 CrossRef CAS PubMed.
- L. Duedahl-Olesen, M. A. Navaratnam, J. Jewula and A. H. Jensen, PAH in some brands of tea and coffee, Polycyclic Aromat. Compd., 2015, 35, 74–90 CrossRef CAS.
-
Health Canada, Federal Contaminated Site Risk Assessment in Canada: Toxicological Reference Values (TRVs), Version 3.0, 2021, p. 66, accessed September 23, 2022 at: https://publications.gc.ca/collections/collection_2021/sc-hc/H129-108-2021-eng.pdf Search PubMed.
-
Michigan Department of Environmental Quality, Cleanup Criteria Requirements for Response Activity, 2013, available at: https://www.michigan.gov/egle/-/media/Project/Websites/egle/Documents/Programs/RRD/Remediation/Rules---Criteria/Table-4-Toxicological-and-Chemical-Physical-Data.pdf?rev=7b8f80e6cf054566b8541431c9ca8a7f&hash=47DDF71B33A3287A2066EC0AC085642Fb Search PubMed.
- P. Mottier, V. Parisod and R. Turetsky, Quantitative determination of polycyclic aromatic hydrocarbons in barbecued meat sausages by gas chromatography coupled to mass spectrometry, J. Agric. Food Chem., 2000, 48, 1160–1166 CrossRef CAS PubMed.
- E. Ledesma, M. Rendueles and M. Díaz, Contamination of meat products during smoking by polycyclic aromatic hydrocarbons: Processes and prevention, Food Control, 2016, 60, 64–87 CrossRef CAS.
- W. Lijinsky, The formation and occurrence of polynuclear aromatic hydrocarbons associated with food, Mutat. Res., 1991, 259, 252–261 Search PubMed.
- M. J. Binnington, Y. D. Lei, L. Pokiak, J. Pokiak, S. K. Ostertag, L. L. Loseto, H. M. Chan, L. W. Y. Yeung, H. Huang and F. Wania, Effects of preparation on nutrient and environmental contaminant levels in Arctic beluga whale (Delphinapterus leucas) traditional foods, Environ. Sci.: Processes Impacts, 2017, 19, 1000–1015 RSC.
- T. Burkhardt, M. Scherer, G. Scherer, N. Pluym, T. Weber and M. Kolossa-Gehring, Time trend of exposure to secondhand tobacco smoke and polycyclic aromatic hydrocarbons between 1995 and 2019 in Germany – Showcases for successful European legislation, Environ. Res., 2023, 216, 114638 CrossRef CAS PubMed.
- G. Ferrante, M. Simoni, F. Cibella, F. Ferrara, G. Liotta, V. Malizia, G. Corsello, G. Viegi and S. La Grutta, Third-hand smoke exposure and health hazards in children, Monaldi Arch. Chest Dis., 2013, 79, 38–43 CAS.
- H.-M. Shin, T. E. McKone and D. H. Bennett, Evaluating environmental modeling and sampling data with biomarker data to identify sources and routes of exposure, Atmos. Environ., 2013, 69, 148–155 CrossRef CAS.
- E. Nethery, A. J. Wheeler, M. Fisher, A. Sjödin, Z. Li, L. C. Romanoff, W. Foster and T. E. Arbuckle, Urinary polycyclic aromatic hydrocarbons as a biomarker of exposure to PAHs in air: A pilot study among pregnant women, J. Exposure Sci. Environ. Epidemiol., 2012, 22, 70–81 CrossRef CAS PubMed.
- Y. Y. Naumova, S. J. Eisenreich, B. J. Turpin, C. P. Weisel, M. T. Morandi, S. D. Colome, L. A. Totten, T. H. Stock, A. M. Winer, S. Alimokhtari, J. Kwon, D. Shendell, J. Jones, S. Maberti and S. J. Wall, Polycyclic aromatic hydrocarbons in the indoor and outdoor air of three cities in the U.S., Environ. Sci. Technol., 2002, 36, 2552–2559 CrossRef CAS PubMed.
- X. Duan, B. Wang, X. Zhao, G. Shen, Z. Xia, N. Huang, Q. Jiang, B. Lu, D. Xu, J. Fang and S. Tao, Personal inhalation exposure to polycyclic aromatic hydrocarbons in urban and rural residents in a typical northern city in China, Indoor Air, 2014, 24, 464–473 CrossRef CAS PubMed.
- J.-Y. Lao, S.-Y. Xie, C.-C. Wu, L.-J. Bao, S. Tao and E. Y. Zeng, Importance of dermal absorption of polycyclic aromatic hydrocarbons derived from barbecue fumes, Environ. Sci. Technol., 2018, 52, 8330–8338 CrossRef CAS PubMed.
- X. Zhang, X. Wang, X. Zhao, Z. Tang, W. Liang, X. Wu, J. Wang, X. Wang and L. Niu, Important but overlooked potential risks of substituted polycyclic aromatic hydrocarbon: Looking below the tip of the iceberg, Rev. Environ. Contam. Toxicol., 2022, 260, 18 CrossRef.
- T. Vasiljevic, N. Jariyasopit, J. K. Schuster and T. Harner, Insights into sources and occurrence of oxy- and nitro-PAHs in the Alberta oil sands region using a network of passive air samplers, Environ. Pollut., 2021, 286, 117513 CrossRef CAS PubMed.
Footnotes |
† Electronic supplementary information (ESI) available: New organism sub-model details and validation results. See DOI: https://doi.org/10.1039/d2em00526c |
‡ The three first authors contributed equally to this work. |
|
This journal is © The Royal Society of Chemistry 2023 |
Click here to see how this site uses Cookies. View our privacy policy here.