DOI:
10.1039/D3NA00093A
(Review Article)
Nanoscale Adv., 2023,
5, 3575-3588
Plant-derived exosomal nanoparticles: potential therapeutic for inflammatory bowel disease
Received
12th February 2023
, Accepted 21st June 2023
First published on 23rd June 2023
Abstract
Inflammatory bowel disease (IBD), encompassing Crohn's disease and ulcerative colitis, is a chronic autoimmune disorder characterized by inflammation. However, currently available disease-modifying anti-IBD drugs exhibit limited efficacy in IBD therapy. Furthermore, existing therapeutic approaches provide only partial relief from IBD symptoms and are associated with certain side effects. In recent years, a novel category of nanoscale membrane vesicles, known as plant-derived exosome-like nanoparticles (PDENs), has been identified in edible plants. These PDENs are abundant in bioactive lipids, proteins, microRNAs, and other pharmacologically active compounds. Notably, PDENs possess immunomodulatory, antitumor, regenerative, and anti-inflammatory properties, making them particularly promising for the treatment of intestinal diseases. Moreover, PDENs can be engineered as targeted delivery systems for the efficient transport of chemical or nucleic acid drugs to the site of intestinal inflammation. In the present study, we provided an overview of PDENs, including their biogenesis, extraction, purification, and construction strategies, and elucidated their physiological functions and therapeutic effects on IBD. Additionally, we summarized the applications and potential of PDENs in IBD treatment while highlighting the future directions and challenges in the field of emerging nanotherapeutics for IBD therapy.
1. Introduction
Inflammatory bowel disease (IBD) is a chronic and recurrent disorder affecting the gastrointestinal (GI) tract. It encompasses two main types: ulcerative colitis (UC) and Crohn's disease (CD).1,2 UC is characterized by continuous inflammation of the mucosa and submucosa of the colon, primarily affecting the distal bowel and rectum, while CD can affect any part of the digestive tract.1–3 Symptoms of IBD typically include abdominal pain, bloody stool, and bowel obstruction, leading to a diminished quality of life.1,2 While the prevalence of IBD remains highest in Europe and the United States, there has been a sharp increase in its incidence in Asia and Latin America.4 The exact etiology and pathogenesis of IBD are not fully understood, although current evidence suggests a combination of genetic and environmental factors.1,5
Current treatment strategies for IBD mainly consist of untargeted therapies, such as aminosalicylates, glucocorticoids, and immunomodulators, as well as targeted biologic therapies, including antitumor necrosis factor-alpha (TNF-α) agents (such as infliximab, adalimumab, certolizumab, and golimumab), anti-integrin agents (vedolizumab and natalizumab), anti-interleukin (IL)-12/IL-23 agents (such as ustekinumab), and selective Janus kinase (JAK) inhibitors (such as tofacitinib).6 Despite the availability of a wide range of medications, it is important to acknowledge that there are still significant rates of primary non-response and loss of response in the treatment of IBD.7 Moreover, these medications are associated with adverse events, including opportunistic infections, cancers, and heart failure, which can compromise their therapeutic benefits.7 Consequently, there is an urgent need for novel alternative treatment options to be explored.
Recently, there has been increasing interest in edible plant-derived exosome-like nanoparticles (PDENs) released by plant cells. PDENs possess certain advantages over animal cell-derived exosomal nanoparticles, such as improved oral bioavailability and resistance to degradation in acidic conditions.8 Unlike animal cell-derived exosomes, PDENs can be administered orally, making them more accessible for therapeutic purposes. PDENs contain various bioactive molecules that play a crucial role in plant cell–cell communication and inter-kingdom signaling.9 Moreover, emerging evidence suggests that PDENs exhibit significant therapeutic potential, including anti-cancer effects, anti-inflammatory responses, and modulation of intestinal dysbiosis.10 Consequently, PDENs have garnered global attention for their potential application in IBD treatment.11,12 Notably, PDENs possess several advantageous characteristics, such as non-toxicity, low immunogenicity, and excellent biocompatibility.11,12
Numerous studies have reported the oral administration of PDENs derived from various sources, such as tea leaves, grapes, and broccoli, demonstrating their ability to alleviate intestinal inflammation and maintain intestinal immune homeostasis in IBD therapy.13–16 Furthermore, PDENs have gained considerable attention as oral colon-targeted drug delivery systems (DDSs) for IBD treatment.17,18 Encapsulating anti-inflammatory agents within PDENs has been shown to restore disrupted intestinal barriers and alleviate experimental mouse colitis.19–21 As a result, PDENs not only enhance therapeutic efficacy but also minimize systemic complications associated with IBD therapy.
This review aimed to provide an overview of PDENs by discussing their biogenesis, characteristics, and biological composition. Additionally, we explored the potential clinical applications of PDENs in IBD treatment, highlighting their therapeutic advantages and challenges. Furthermore, we examined the utility of PDENs as colon-targeted DDSs for IBD therapy. Finally, we addressed current limitations and research gaps in the field and presented our perspectives on future directions.
2. Biogenesis and character of PDENs
The existence of PDENs was first observed in carrot cells using electron microscopy as early as the 1960s.22 While numerous studies have successfully isolated and identified PDENs, the precise mechanisms underlying their biogenesis remain elusive.23 There are three proposed pathways involved in PDEN release, namely the multivesicular body (MVB) pathway, exocyst-positive organelle (EXPO) pathway, and vacuolar pathway (Fig. 1).11,24 Among these pathways, the MVB pathway appears to play a crucial role in PDEN formation. In brief, the plasma membrane undergoes inward sprouting, leading to the formation of an early-sorting endosome, which subsequently matures into a late-sorting endosome. The late-sorting endosome then communicates with the trans-Golgi network and develops into MVBs. Eventually, the MVBs merge with the plasma membrane, facilitating the release of PDENs into the extracellular space.25 In plant cells, the EXPO pathway involves the presence of spherical double-membrane structures that expel PDENs into the extracellular space through fusion with the plasma membrane.26 On the other hand, vacuoles contain hydrolytic enzymes and defense proteins, which can fuse with the plasma membrane and release defensive substances into the extracellular space in response to bacterial pathogen infection.27 Additionally, the endosomal sorting complex required for transport (ESCRT), which binds and sequesters ubiquitinated proteins, is believed to be involved in the maturation process of PDENs.28
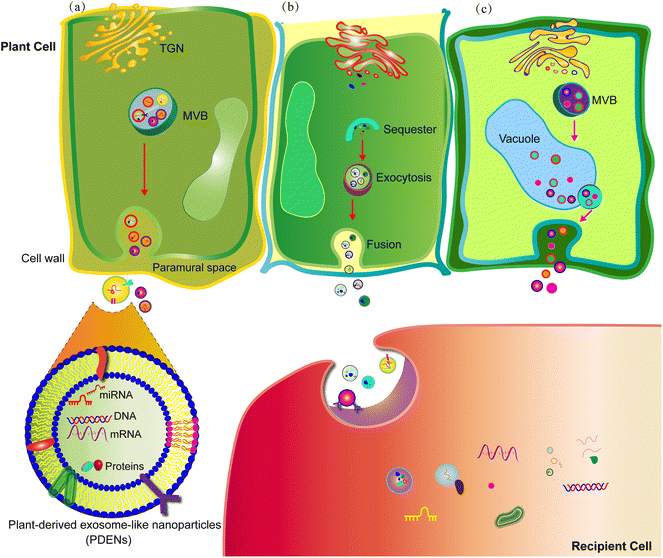 |
| Fig. 1 Biogenesis, secretion and composition of PDENs. The pathways for the biosynthesis of plant EVs can be through (a) multivesicular body (MVB) endosome pathway; (b) exocyst-positive organelle (EXPO) pathway; (c) vacuolar pathway. PDENs are membrane-bound phospholipid nanovesicles that packed with mRNA, miRNA, and various defense proteins and membrane proteins. TGN, trans golgi network; MVB, multivesicular bodies; PDENs, plant-derived exosome-like nanoparticles. | |
PDENs exhibit a diverse range of average particle sizes, ranging from 30 nm to nearly 400 nm. They also display various morphologies, such as spherical, oval, and cup-shaped structures. These specific characteristics of PDENs are dependent on the source of the nanoparticles and the methods used for their extraction.29,30 The surface marker proteins that identify PDENs should possess conserved structural attributes inherent to different plant species.31 Based on current literature, potential candidate surface marker proteins of PDENs may include the syntaxin PEN1 and the tetraspanin-8 (TET8).32 PEN1 has been consistently found to be enriched in PDENs and has been confirmed through western blotting analysis.33 TET8, a mammalian homolog of the exosome surface membrane protein CD63, is widely expressed in PDENs.23 Other marker proteins, such as the ABC transporter protein PEN3, heat shock proteins (HSP70 and HSP90), and pattelin-1 and -2 (PATL-1 and PATL-2), require further investigation to ascertain their role in identifying PDENs.31,32 However, to the best of our knowledge, specific target surface markers for PDENs have not yet been extensively studied.
PDENs exhibit enrichment of specific lipids in their exosomal membranes, including phosphatidic acid (PA), phosphatidylcholines (PC), digalactosyldiacylglycerol (DGDG), and monogalactosyldiacylglycerol (MGDG).34 To evaluate the characteristics of PDENs, various ultrasensitive microscopic methods, such as transmission electron microscopy (TEM), scanning electron microscopy (SEM), and atomic force microscopy (AFM), can be employed to capture ultrastructural information. Dynamic light scattering (DLS) is used to analyze the size and surface potential of PDENs.35,36 With the advancements in nanotechnology, it has become possible to isolate, identify, and characterize PDENs with satisfactory quantity and purity. However, the ultrastructural properties of PDENs are still largely unknown.37
3. Biological composition and function of PDENs
PDENs contain multiple cargoes, including lipids, proteins, and nucleic acids, which play crucial roles in plant cell crosstalk and inter-kingdom communication (Fig. 1).
Lipids are fundamental components of the lipid bilayer structure of PDENs. Lipid profiling of PDENs has identified three distinct groups of lipids: PA, PC, and phosphatidylethanolamine (PE).19,34,38 PA serves as an important lipid messenger in intercellular communication and is involved in several processes, such as mitogenesis, membrane fusion, and fission.18,38 In addition, PA plays a critical role in mammalian cell growth and proliferation by modulating the mammalian target of rapamycin (mTOR) and mitogen-activated protein kinase (MAPK) pathways.39 PC is known to participate in various life activities of organisms through membrane-mediated cell signaling.40,41 Both PC and PE exhibit antioxidant, anti-inflammatory, and anti-colitis activities.42,43 Moreover, these lipids also play a significant role in the uptake by gut microbiota, modification of gut microbiota composition and localization, and maintenance of gut microbiota homeostasis.10
Proteins play a crucial role in the function of PDENs.44 PDENs exhibit a specific protein profile that varies among different plant sources, resulting in a highly diverse range of proteins.25 Therefore, analyzing the proteomic profile of PDENs is an effective approach to confirm their presence and evaluate their potential functions. Various proteins have been identified in PDENs, including actin, proteolytic enzymes, aquaporins, chloride channels, and heat shock proteins, which can be broadly categorized into transmembrane proteins and other plasmalemma-associated proteins.45,46 PDENs utilize these proteins to carry out diverse cellular functions and facilitate intercellular communication by transferring their cargo to recipient cells, resulting in antioxidant stress reduction and anti-inflammatory activation.25,47,48 However, further research is needed to comprehensively identify the wide range of PDEN proteins involved in their biological and pharmacological activities.
PDENs contain a diverse array of nucleic acids, including mRNA, non-coding RNA (ncRNA), microRNA (miRNA), and small RNA (sRNA), which contribute to their role in inter-kingdom communication.37,49 ncRNA and mRNA found in PDENs have the ability to regulate RNA and protein levels in recipient cells, thereby influencing cellular processes.50 Moreover, miRNAs, a specific type of small ncRNA, play a crucial role in the post-transcriptional regulation of protein levels in recipient cells and are involved in various physiological and pathological processes, including cell proliferation, metabolism, and immune responses.46,51 Furthermore, miRNAs have the potential to regulate the expression of inflammatory cytokines, suggesting their involvement in modulating inflammatory responses.37 Moreover, mRNAs and sRNAs present in PDENs have been shown to modulate gut microbiota composition and alleviate colitis.52 However, the precise functions and underlying mechanisms of nucleic acids in PDENs remain largely unknown and require further investigation.
4. Isolation of PDENs
The isolation of PDENs relies primarily on a combination of differential centrifugation and density gradient techniques (Fig. 2). PDENs can be extracted from various plant parts, such as fruits, roots, stems, leaves, and seeds. The plant matrices are typically homogenized using a high-speed grinder, followed by the extraction of juice using a mixer and straining the juice through a colander.19,40,53 The obtained juice is then subjected to a series of centrifugation steps for isolation.12 Initially, a low-speed centrifugation step (500–3000 g for 10–15 minutes) is employed to remove plant fibers and large particles. Subsequently, a medium-speed centrifugation step (3000–10,000 g for 20–40 minutes) is utilized to eliminate large debris and intact organelles. Finally, a high-speed centrifugation step (10
000–150
000 g for 1.5–2 hours) is performed to pellet the PDENs. However, it is important to note that the isolated PDEN pellet often contains contaminants, such as other vesicles, proteins, and nucleic acid aggregates. Therefore, a subsequent purification step using sucrose density gradient ultracentrifugation is employed to enhance the purity of PDENs, albeit with a compromise in yield.54–56
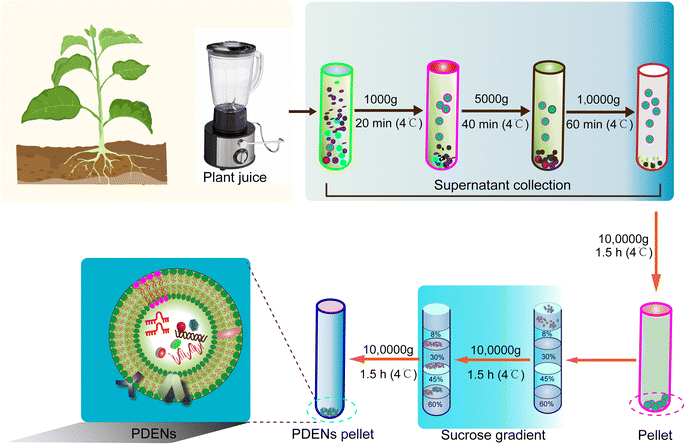 |
| Fig. 2 Graphical representation of the isolation and purification of PDENs. The basic procedure for PDENs isolation is based on differential ultracentrifugation, followed by an additional step of sucrose gradient purification to obtain PDENs. | |
In addition to differential centrifugation and density gradient techniques, there are other methods used for the isolation of PDENs. One such method is precipitation-based isolation, where polyethylene glycol (PEG) is commonly employed to decrease the solubility of PDENs, resulting in their precipitation. This method is favored for its simplicity and high production yield.57 Co-precipitation methods using positively-charged molecules or negatively-charged free proteins have also been utilized to extract PDENs.58 However, these co-precipitation methods generally suffer from low purity and high cost, limiting their widespread use. Ultrafiltration, which employs a polymeric membrane to separate molecules based on their size, is another effective method for PDEN isolation.59 There are two main approaches: direct flow filtration (DFF) and tangential flow filtration (TFF). However, ultrafiltration is more commonly used for the isolation of animal-derived exosomes and is not extensively applied for PDENs due to cost considerations and lower purification efficiency.59 Immunoaffinity enrichment, based on the binding of specific antibodies to antigens present on extracellular vesicles (EVs), can also be employed to isolate PDENs.60 This method offers advantages such as rapid isolation, simplicity, and high specificity. However, its application for PDEN extraction is limited by the current understanding of their membrane compositions.61 It is important to note that the yield of PDENs obtained can vary depending on the source material and the specific isolation method used. Additionally, the lack of a “gold standard” for the purity and uniformity of PDENs hinders their clinical applications.
5. Therapeutic effects of PDENs on IBD
Recent studies have provided compelling evidence that PDENs can be regarded as potent oral therapeutics for treating IBD.8,14,62,63 Notably, PDENs exhibit unique properties and biological functions that make them highly suitable for IBD treatment. The lipid bilayer structures of PDENs, for instance, play a crucial role in their resistance to degradation in the GI tract and contribute to their intrinsic anti-inflammatory effects.19,64 Furthermore, PDENs derived from edible plants represent a particularly promising approach due to their non-toxicity, non-immunogenicity, wide availability, biocompatibility, and biodegradability.17,24 In light of these factors, we presented a comprehensive overview of the emerging applications of PDENs in IBD therapy, as outlined in Table 1 and illustrated in Fig. 3.
Table 1 PDENs for IBD therapya
Sources |
Loaded drug or contain |
Study model |
Function |
Ref. |
HO-1, heme oxygenase-1; DSS, dextran sulphate sodium; MPO, myeloperoxidase; IEC intestinal epithelial cell.
|
Grape |
NA |
Macrophages, DSS-induced mouse colitis |
Promote HO-1 and IL-10 expression in colonic macrophage; protect mouse from DSS-induced colitis |
19 and 54 |
Grapefruit |
Methotrexate (MTX) |
DSS-induced mouse colitis |
Immunomodulators and maintain intestinal macrophage homeostasis; ameliorate DSS-induced mouse colitis |
66
|
Ginger |
Mdo-miR7267-3p |
DSS-induced mouse colitis |
Preferentially uptake by Lactobacillaceae attenuates mouse colitis via IL-22-dependent mechanisms |
38
|
Ginger |
siRNA-CD98 |
RAW 264.7 cells, Colon-26 cells, Mouse colon |
Ameliorate DSS-induced mouse model of colitis and colitis-associated cancer |
21
|
Ginger |
6-Shogaol |
DSS-induced mouse colitis model |
Targets colitis tissue, relieves symptoms of colitis, and promotes wound repair in colitis |
75
|
Ginger |
NA |
Mouse colitis models |
Increases IEC activity, inhibits pro-inflammatory cytokines and increases anti-inflammatory cytokines |
63
|
Broccoli |
Sulforaphane |
DSS-induced mouse acute colitis model, Rag1−/− mice (T cell dependent) |
Protects intestinal inflammation |
14
|
Citrus sinensis |
NA |
Human colonic epithelial cell line |
Maintain intestinal epithelium |
15
|
Mulberry bark |
NA |
DSS-induced colitis |
Activates heat shock protein family A (Hsp70) member 8 (HSPA8) mediated AhR signaling pathway and prevents DSS-induced colitis |
62
|
Tea leaves |
NA |
DSS-induced colitis |
Uptake by macrophages; restores the colonic barrier function barrier function, boosts gut microbial populations, thereby alleviating inflammatory bowel disease |
20
|
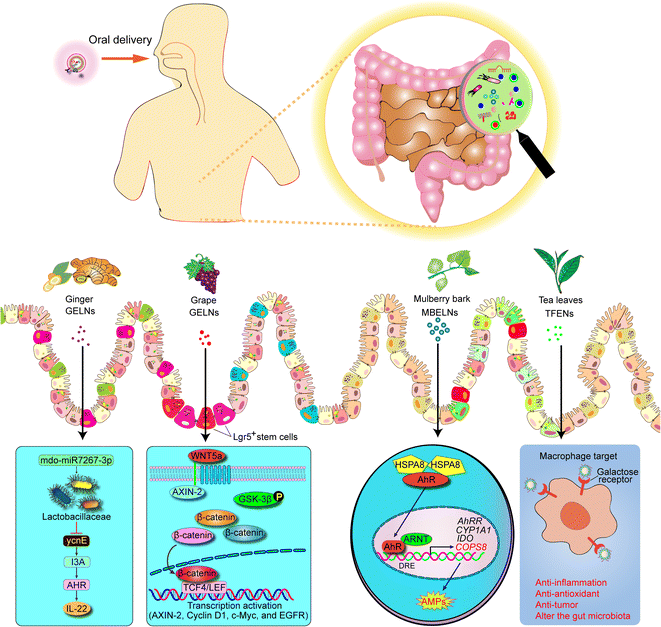 |
| Fig. 3 Schematic representation of mechanism of PDENs that protects against colitis. AMP, anti-microbial peptides. | |
5.1. Grape-derived exosome-like nanoparticles (GDENs)
GDENs have been shown to contain a rich array of proteins, including heat shock protein 70 (HSP70), aquaporin, and lipids with inherent anti-inflammatory properties.19 Remarkably, GDENs possess the ability to penetrate the intestinal mucus barrier and be internalized by mouse intestinal stem cells, thereby stimulating intestinal stem cell proliferation and facilitating the self-renewal of the intestinal epithelium through activation of the Wnt/β-catenin signaling pathway.19 Furthermore, GDENs have been demonstrated to significantly enhance the proliferation of intestinal stem cells and effectively promote the regeneration of the mucosal epithelium, leading to the prevention of dextran sulfate sodium (DSS)-induced colitis in mice and rapid restoration of the intestinal architecture along the entire length of the intestine.19 Additionally, oral administration of GDENs has been found to withstand the harsh conditions of the GI tract, reach the intestinal crypts, and penetrate the epithelial mucosa.54 Subsequently, GDENs are taken up by intestinal stem cells, promoting their proliferation and offering promising therapeutic potential in the treatment of IBD.54
5.2. Grapefruit-derived exosome-like nanoparticles (GFDENs)
Grapefruit is a highly nutritious fruit that contains a wide range of beneficial nutrients and phytochemicals, making it a valuable addition to a healthy diet. Studies have shown that GFDENs have the ability to promote the nuclear translocation of nuclear factor (erythroid-derived 2)-like 2 (Nrf2) and induce the expression of heme oxygenase-1 (HO-1) and IL-10 in RAW 264.7 macrophages and mice with experimental colitis.65 Furthermore, Wang et al. have discovered that orally administered GFDENs, loaded with the medication methotrexate (MTX), can be specifically taken up by intestinal macrophages. This uptake leads to the upregulation of HO-1 levels and inhibition of the expression of IL-1β and TNF-α in intestinal macrophages.66 As a result, GFDENs loaded with MTX exhibit significant efficacy in attenuating DSS-induced colitis in mice.66
5.3. Ginger-derived exosome-like nanoparticles (GELNs)
Ginger, a commonly used traditional Chinese ingredient, has been the subject of increasing research due to its anti-inflammatory effects and its potential to regulate intestinal microbiota.67–69 GELNs are composed of various lipids, a small number of proteins, miRNAs, and abundant bioactive constituents, such as 6-gingerol and 6-shogaol.68–74 In models of DSS-induced colitis, orally administered GELNs are primarily taken up by intestinal epithelial cells (IECs) and macrophages. This uptake not only promotes the survival and proliferation of IECs but also inhibits the expression of pro-inflammatory cytokines (TNF-α, IL-6, and IL-1β) while increasing the levels of anti-inflammatory cytokines (IL-10 and IL-22).63 As a result, GELNs have emerged as promising candidate for ameliorating colitis and promoting healing. Additionally, GELNs have shown preferential uptake by Lactobacillaceae and target specific genes of Lactobacillus rhamnosus (LGG), which contributes to the alteration of gut microbiota composition and induces the expression of IL-22, thereby restoring intestinal barrier function and attenuating colitis.38 Furthermore, Zhou et al. have demonstrated that ginger extract can suppress the expression of pro-inflammatory cytokines, such as TNF-α, IL-1β, and IL-6, while increasing the level of the anti-inflammatory factor IL-10.75 Ginger extract has also been shown to modulate dysbiosis in the intestinal microbiota, improve intestinal barrier function, and inhibit the inflammatory response in DSS-induced mouse colitis.75
5.4. Tea leaf-derived exosome-like nanoparticles (TLDENs)
Tea, a widely consumed beverage worldwide, is known for its content of polyphenols, flavones, lipids, and polysaccharides, which provide various benefits, such as antioxidant, antitumor, and hypolipidemic properties.76,77 Numerous studies have demonstrated that extracts from tea leaves exhibit anti-inflammatory effects, help maintain gut microbiota balance during colitis, and offer protection against colitis-associated colon cancer (CAC) by reducing inflammation.78–80 TLDENs are rich in lipids, functional proteins, and small bioactive molecules.20 In the context of mouse colitis, orally administered TLDENs are selectively internalized by macrophages. This internalization leads to a reduction in reactive oxygen species (ROS) production, inhibition of pro-inflammatory cytokines, such as TNF-α, IL-6, and IL-12, and an increase in the secretion of the anti-inflammatory cytokine IL-10 by macrophages.20 Furthermore, oral administration of TLDENs effectively suppresses inflammatory bowel responses, restores disrupted colonic barriers, and promotes diversity and overall abundance of the gut microbiota in mouse colitis.20
5.5. Broccoli-derived exosome-like nanoparticles (BDENs)
Adenosine monophosphate-activated protein kinase (AMPK) is a vital enzyme and signaling pathway that plays a crucial role in regulating immune homeostatic networks.81 It is widely expressed in various immune cell types, including colonic macrophages and dendritic cells (DCs), and is involved in the modulation of cytokine production, apoptosis, and proliferation, thereby contributing to the pathogenesis of IBD.82–84 Studies have shown that BDENs can inhibit the expression of pro-inflammatory cytokines, such as TNF-α, IL-17A, and INF-γ, in experimental mouse colitis.14 Additionally, BDENs are involved in the prevention of DC activation and induction of tolerant DCs by activating AMPK in mouse colitis.14 Therefore, BDENs play a critical role in maintaining intestinal immune homeostasis and protecting against mouse colitis.14
5.6. Mulberry bark-derived exosome-like nanoparticles (MBDENs)
Mulberry, a deciduous plant, serves various beneficial purposes, such as fruit production and leaf cultivation for silkworm feeding. It also holds significant importance in clinical therapeutic applications.85–87 In the context of IBD, MBDENs have been shown to exert protective effects against DSS-induced mouse colitis. They achieve this by promoting the expression of heat shock protein family A (HSP70) and activating the aryl hydrocarbon receptor (AhR) and constitutive photomorphogenic homolog subunit 8 (COPS8) signaling pathway. As a result, MBDENs help prevent weight loss, colon shortening, and inflammation associated with colitis in mice.62
5.7. Turmeric-derived exosome-like nanoparticles (TDENs)
Turmeric, a perennial plant belonging to the ginger family (Curcuma longa), has a long-standing history in traditional Chinese medicine and is known for its various beneficial properties, including hypolipidemic, antineoplastic, and anti-inflammatory effects.88–90 Studies conducted by Liu C. et al. have shown that TDENs contain abundant lipids and proteins, which contribute to their remarkable anti-inflammatory and antioxidant properties.91 In experimental mouse colitis, oral administration of TDENs has been found to suppress the expression of pro-inflammatory cytokines, such as TNF-α, IL-6, and IL-1β, while promoting the levels of antioxidant genes, particularly HO-1.91 Importantly, TDENs have demonstrated the ability to effectively alleviate experimental mouse colitis and significantly expedite the resolution of colitis symptoms.91
5.8. Carrot-derived exosome-like nanoparticles (CDENs)
The carrot is considered one of the 10 most economically significant vegetable crops globally. Additionally, CDENs have shown an enhanced capacity to promote the nuclear translocation of nuclear factor erythroid-2 related factor 2 (Nrf2) and induce the expression of IL-10 in RAW 264.7 macrophages.65
6. PDENs as a delivery platform in IBD treatment
PDENs have demonstrated the ability to facilitate inter-kingdom communication by delivering payloads to other species.25 Consequently, PDENs have emerged as a promising platform for drug delivery due to their numerous advantageous properties, including non-toxicity, low immunogenicity, and excellent biocompatibility.24,25,28,92 Furthermore, oral administration of PDENs has proven to withstand the harsh environment of the GI tract and effectively target specific inflammatory sites in the colon for the treatment of IBD.66 Consequently, there has been a growing body of research focused on utilizing PDENs as DDSs in the treatment of IBD.21,66 Thus, in this section, we aimed to illuminate the application of PDEN-based nanoplatforms for colon-targeted DDSs in the treatment of IBD, as summarized in Table 1.
6.1. GFDEN platform
In a study conducted by Wang et al., it has been discovered that oral administration of GFDENs encapsulating the anti-inflammatory drug MTX can selectively target lamina propria macrophages. This targeted approach results in a significant decrease in the levels of pro-inflammatory cytokines, such as TNF-α and IL-1β. Furthermore, in a DSS-induced mouse model of colitis, GFDENs encapsulating MTX exhibit a dramatic ability to prevent body weight loss and colon length shortening when compared to the administration of MTX alone.66 Moreover, the delivery of MTX through GFDENs demonstrates a substantial reduction in MTX toxicity compared to free MTX, thereby significantly enhancing its therapeutic effects.66
6.2. Ginger-derived exosome-like nanoparticles (GELNs) platform
It has been reported that elevated colonic expression of CD98 plays a crucial role in the development of colitis and colitis-associated cancer.93–95 Consequently, reducing the colonic levels of CD98 has been shown to mitigate colitis and its associated cancer. In a study by Zhang et al., si-CD98-loaded GELNs exhibit specific uptake by RAW 264.7 and colon-26 cells, effectively leading to a decrease in CD98 expression in vitro.21 Additionally, in a mouse model of UC, oral administration of si-CD98-loaded GELNs labeled with a near-infrared fluorescent dye successfully targets colonic inflammatory sites, resulting in a reduction of CD98 expression and improvement in the colonic inflammatory response.21
6.3. Broccoli-derived exosome-like nanoparticles (BDENs) platform
Edible cruciferous vegetables contain abundant amounts of sulforaphane (SF), which offers several advantages, such as the suppression of cytochrome P450 enzymes, induction of apoptotic pathways, inhibition of cell cycle progression, and anti-inflammatory activity.96–98 Studies have reported that SF-loaded BDENs can specifically target colonic DCs and alleviate symptoms of experimental mouse colitis.14 This therapeutic effect is attributed to the induction of colonic tolerant DCs, inhibition of pro-inflammatory cytokines, and maintenance of intestinal immune homeostasis.14
7. Discussion and perspectives
In this review, we provided a comprehensive discussion of the biogenesis and characteristics of PDENs. We also summarized the composition and functions of PDENs, highlighting their potential as therapeutic agents. Additionally, we briefly introduced the isolation methods for PDENs. Importantly, we reviewed published research studies that demonstrate the protective effects of various PDENs in the treatment of IBD. Furthermore, we highlighted the potential of PDENs as oral colon-targeted DDSs, enabling the specific delivery of drug molecules to the inflamed sites in IBD treatment. Overall, PDENs possess several advantages, including non-toxicity, low immunogenicity, excellent biocompatibility, high efficacy, and limited systemic complications, making them promising candidates for the next generation of therapeutics in IBD treatment.
Mahmood B. et al. have examined the use of various engineered nanoparticles, such as gold nanoparticles (Au nanoparticles), graphene, quantum dots, inorganic nanoparticles, and PDENs, in the detection, imaging, and treatment of IBD.99 They have briefly summarized that PDENs exhibit broad biological functions and therapeutic potential for IBD. In our present review, we provided a comprehensive overview of the biogenesis, isolation, composition, characteristics, and functions of PDENs. We thoroughly classified the diverse types of PDENs and extensively discussed their role as oral weapons and DDSs in the treatment of IBD.
Another review by Hua S. et al. has focused on the diverse physiological changes and variability within the GI tract, including transit time, microbial considerations, colonic pH, intestinal volume, and mucosal integrity.100 These factors are particularly relevant to the biodistribution, accumulation, and efficacy of traditional IBD treatments. Importantly, they have further discussed the use of nanotechnology as a DDS, which has been developed and designed to overcome the aforementioned physiological changes and enhance therapeutic outcomes in IBD.100 Furthermore, in the treatment of IBD, the pharmaceutical routes for targeted drug delivery to the desired colon have been characterized for available nanotechnology drug vehicles. Several parameters, such as size, surface charge, poly (ethylene glycol) coating, and pH-dependent nano-delivery systems, play significant roles in achieving targeted drug delivery.100 Consequently, it is crucial to acknowledge and address these challenges when applying PDENs in the administration of IBD. Therefore, further attention and research should be devoted to this area in the future.
In a review by Shah B. M. et al., they have explored biomacromolecules derived from various sources, such as plants, animals, bacteria, fungi, or algae. Specifically, they have highlighted the protective effects of nanohybrid vehicles composed of a combination of proteins and polysaccharides for loaded bioactive compounds.101 Furthermore, they have discussed the numerous advantages of nanohybrids, including their safety, biodegradability, and biocompatibility, which make them highly appealing for colon-targeted DDSs in the treatment of IBD.101 In our comprehensive review, we primarily focused on PDENs as promising therapeutic strategies and drug carriers for IBD.
Another review has summarized that nanoparticles can be generated from natural or synthetic polymers during the development of nanotechnology.102 These nanoparticles have the capability to encapsulate therapeutic drugs, such as 5-aminosalicylic acid (5-ASA), corticosteroids, and immunomodulators, thereby enabling targeted delivery to the desired colon and reducing adverse events in IBD therapy.102 Therefore, nanoparticles offer a viable approach for targeted drug delivery to the diseased colon in the treatment of IBD.102 Within our review, we extensively discussed the potential of natural nanoparticles, specifically PDENs, as promising therapeutic candidates and interesting DDSs for IBD.
Studies have indicated that plant-based diets low in fats and meats have the ability to alleviate intestinal inflammation, in contrast to diets high in fats and meats commonly associated with western diets.103,104 Additionally, a study has reviewed the association between plant-based dietary components, including proteins, fatty acids, phytochemicals, vitamins, and trace elements, and intestinal inflammation and relapse in IBD.105 Although plant-based dietary components show significant potential in mitigating and preventing intestinal inflammation in IBD, further clinical trials are needed to confirm these effects.105 In the present study, we thoroughly elucidated the pivotal role of PDENs in the treatment of IBD, highlighting their potential as effective therapeutic agents and emphasizing the need for further research and clinical validation.
Despite the therapeutic benefits associated with PDENs, there are several research gaps that need to be addressed in this field. Firstly, one of the main challenges is the standardization of the isolation and purification methods for PDENs. Currently, various techniques, such as ultracentrifugation, ultrafiltration, immune isolation, and PEG-based precipitation, are employed for PDEN isolation. However, the accurate characterization of PDENs, including their surface markers, densities, and sizes, lacks standardized guidelines. It is crucial to establish protocols that enable consistent and reliable characterization of PDENs. Furthermore, the long-term storage strategies for PDENs need to be carefully considered to ensure the maintenance of their structural integrity and bioactivity over time. Developing suitable preservation methods is crucial for their practical application.
Additionally, in-depth characterization studies should be conducted to unravel the distinct biomolecular profiles of different classes of PDENs. Utilizing intensive integrated omics analysis can provide valuable insights into their unique pharmaceutical properties. Understanding the specific characteristics of different PDENs can aid in optimizing their therapeutic efficacy. Addressing these research gaps and achieving breakthroughs in these areas will contribute to the advancement of PDENs as promising therapeutic strategies in the treatment of IBD.
It is important to acknowledge that the therapeutic potential of PDENs in IBD is still in its early stage of development. While several pioneering reports have demonstrated their efficacy in experimental colitis, it is crucial to recognize that these findings may not directly translate to clinical applications in human IBD. Further research and clinical studies are required to validate the effectiveness and safety of PDENs in treating IBD in humans.
The commonly used colitis models, such as the DSS and trinitrobenzene sulfonic acid (TNBS)-induced models, have limitations in accurately replicating the complexity of human IBD. These models do not fully capture the long-term administration, recurrence, and adverse events that occur in IBD patients when PDENs are administered. Therefore, there is a pressing need for the development of more precise colitis models that better mimic the characteristics of human IBD. These models will provide a more reliable platform for studying the therapeutic effects and potential adverse events associated with PDENs in IBD treatment.
Simultaneously, it is crucial to conduct clinical studies to validate the safety, stability, and efficacy of PDENs in the treatment of IBD. Unfortunately, there is currently a scarcity of clinical trials investigating the use of PDENs in IBD administration.
To date, only GELNs have been included in human clinical trials for IBD treatment (NCT04879810). This study aims to test the hypothesis that GELNs will have clinically significant anti-inflammatory effects on IBD patients. Furthermore, it will assess the safety and tolerability of GELNs with and without curcumin in IBD patients and evaluate the effects of GELNs or curcumin alone, as well as their combination, on symptoms and disease scores in patients with refractory IBD.
Therefore, additional efforts are needed to prioritize PDENs in clinical studies and uncover their pharmacological activities in the treatment of IBD. These studies will provide valuable insights into the potential of PDENs as therapeutic agents for IBD patients.
Orally administered PDENs, as promising colon-targeted DDSs, have been rapidly and effectively developed, with some of them even being investigated in experimental colitis.106 However, there are still inevitable challenges that need to be addressed before their widespread application in IBD treatment. One such challenge is how to monitor the PDENs as they traverse the harsh GI tract. Another important consideration is the selection of suitable PDENs as effective drug carriers for IBD treatment.
PDENs can also be surface-functionalized, similar to exosome-like nanoparticles, to enable target-specific delivery, as demonstrated in studies involving mammalian cells.107–112 Various types of PDENs have been identified in plants, including TET8-expressing PDENs,52 PEN1-expressing PDENs,113 and EXPO-origin PDENs.22 TET8 and PEN1 are known to be expressed in different types of PDENs. Consequently, the surface protein of PDENs can be genetically modified to incorporate target peptides. To achieve this, the model plant Arabidopsis thaliana can be chosen as the cell source. Plant cells will be cultured in large quantities and transfected with a plasmid expressing engineered TET8, which displays the targeted peptide on the surface of PDENs. Additionally, a colon-targeted sequence will be inserted at the EC1 of TET8 (Fig. 4a). Alternatively, chemical methods can be employed to modify PDENs. In this approach, a lipid molecule called DSPE will be utilized to introduce the target peptide on the surface of PDENs. A lipid peptide called DSPE-PEG-TL will be chemically synthesized, and incubating the lipid peptide with PDENs will result in the spontaneous insertion of the molecule into the lipid bilayer of the PDENs, thereby displaying the colon-targeted peptide on the surface (Fig. 4b).
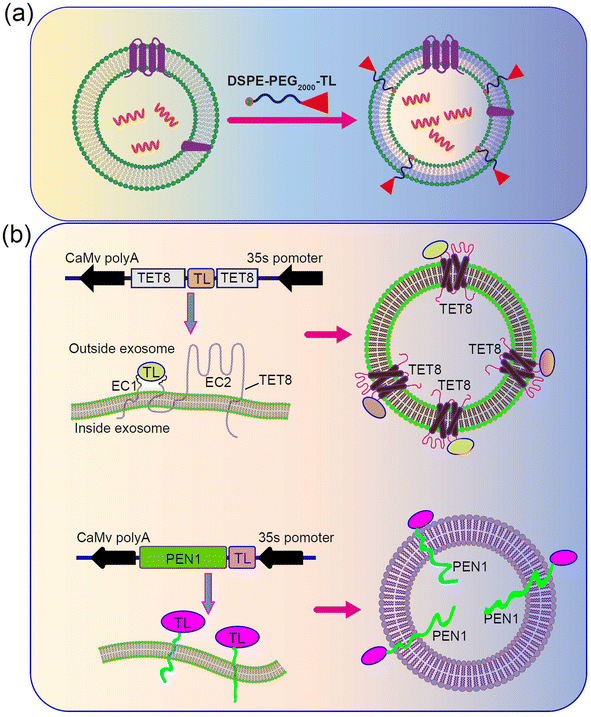 |
| Fig. 4 Schematic illustration of the functionalization of PDENs by target peptide/antibody for drug delivery. (a) Chemical functionalization with a lipid peptide. (b) Genetic engineering of the TET8 or PEN1 protein. TL, targeting ligand (peptide, protein or antibody et al.). | |
Furthermore, additional clinical studies and trials are necessary to advance the understanding and application of PDENs. It is important to note that these limitations present opportunities for future research directions. Overall, there will be several challenges to overcome in implementing PDENs in therapeutics and drug delivery for IBD treatment. However, we are confident that these obstacles will be addressed through the development of nanotechnology. We anticipate that PDEN-based therapeutics and delivery nanoplatforms will emerge as the next-generation strategies in the treatment of IBD.
Abbreviations
IBD | inflammatory bowel disease; |
DMAIDs | disease-modifying anti-IBD drugs; |
PDENs | plant-derived exosome-like nanoparticles |
UC | ulcerative colitis |
CD | Crohn's disease |
DDSs | drug delivery systems |
EXPO | exocyst-positive organelle |
MVBs | multivesicular bodies |
ESCRT | endosomal sorting complex required for transport |
PA | phosphatidic acid; |
PC | phosphatidylcholines |
DGDG | digalactosyldiacylglycerol |
MGDG | monogalactosyldiacylglycerol |
TEM | transmission electron microscopy |
SEM | scanning electron microscopy |
AFM | atomic force microscopy |
DLS | dynamic light scattering |
PE | phosphatidylethanolamine |
mTOR | mammalian target of rapamycin |
MAPK | mitogen-activated protein kinase |
mRNA | microRNA |
sRNA | small RNA |
PEG | polyethylene glycol |
GDENs | grape-derived exosome-like nanoparticles |
DSS | dextran sulphate sodium |
GFDENs | grapefruit-derived exosome-like nanoparticles |
HO-1 | heme oxygenase-1 |
GELNs | ginger-derived exosome-like nanoparticles |
IECs | intestinal epithelial cells |
LGG |
Lactobacillus rhamnosus
|
TLDENs | tea leaves-derived exosome-like nanoparticles |
BDENs | broccoli-derived exosome-like nanoparticles |
AMPK | adenosine monophosphate activated protein kinase |
MBDENs | mulberry bark-derived exosome-like nanoparticles |
AhR | aryl hydrocarbon receptor |
COPS8 | constitute photomorphogenic homolog subunit 8 |
CDENs | carrots-derived exosome-like nanoparticles |
TET8 | tetraspanin-8 |
PEN1 | penetration 1 |
EC1 | extracellular domains 1 |
TL | targeting ligand |
DSPE-PEG | 1,2-distearoyl-sn-glycero-3-phosphoethanolamine-poly(ethylene glycol) |
Author contributions
Y. L. contributed towards the conceiving and illustration of this manuscript. D. L., M. Y. was responsible for writing of this review. Q. T., H. X. J. F. and M. Z. was responsible for assisting in collecting the literature and revising this review. Y. Z., C.T., Y. N. and J. W. provided some suggestions. J. Y. and L. W contributed to discussion. All authors read and approved the final manuscript.
Conflicts of interest
The authors declare no conflict of interest.
Acknowledgements
This work was supported by Technical Research and Development Project of Shenzhen (No. JCYJ20150403101028164, No. JCYC20170307100911479, No. JCYJ20190807145617113, JCYJ20210324113802006, and JCYJ2022053015180024), Shenzhen Fund for Guangdong Provincial High Level Clinical Key Specialties (No. SZGSP013), Shenzhen Key Medical Discipline Construction Fund (No. SZXK042), and Guangdong High-level Hospital Construction Fund (No. ynkt2021-zz07).
References
- J. T. Chang, Pathophysiology of Inflammatory Bowel Diseases, N. Engl. J. Med., 2020, 383, 2652–2664 CrossRef CAS PubMed.
- C. J. Chen, H. Hu and W. T. Liao, Pathophysiology of Inflammatory Bowel Diseases, N. Engl. J. Med., 2021, 384, 1376–1377 CrossRef PubMed.
- H. M. Xu, J. Xu and M. F. Yang,
et al., Epigenetic DNA methylation of Zbtb7b regulates the population of double-positive CD4(+)CD8(+) T cells in ulcerative colitis, J. Transl. Med., 2022, 20, 289 CrossRef CAS PubMed.
- S. C. Ng, H. Y. Shi and N. Hamidi,
et al., Worldwide incidence and prevalence of inflammatory bowel disease in the 21st century: a systematic review of population-based studies, Lancet, 2017, 390, 2769–2778 CrossRef PubMed.
- J. Xu, H. M. Xu and M. F. Yang,
et al., New Insights Into the Epigenetic Regulation of Inflammatory Bowel Disease, Front. Pharmacol., 2022, 13, 813659 CrossRef CAS PubMed.
- Q. Lu, M. F. Yang and Y. J. Liang,
et al., Immunology of Inflammatory Bowel Disease: Molecular Mechanisms and Therapeutics, J. Inflammation Res., 2022, 15, 1825–1844 CrossRef CAS PubMed.
- D. C. Baumgart and C. Le Berre, Newer Biologic and Small-Molecule Therapies for Inflammatory Bowel Disease, N. Engl. J. Med., 2021, 385, 1302–1315 CrossRef CAS PubMed.
- D. K. W. Ocansey, L. Zhang and Y. Wang,
et al., Exosome-mediated effects and applications in inflammatory bowel disease, Biol. Rev. Cambridge Philos. Soc., 2020, 95, 1287–1307 CrossRef PubMed.
- M. Nemati, B. Singh and R. A. Mir,
et al., Plant-derived extracellular vesicles: a novel nanomedicine approach with advantages and challenges, Cell Commun. Signaling, 2022, 20, 69 CrossRef CAS PubMed.
- S. Rome, Biological properties of plant-derived extracellular vesicles, Food Funct., 2019, 10, 529–538 RSC.
- M. Cong, S. Tan and S. Li,
et al., Technology insight: Plant-derived vesicles-How far from the clinical biotherapeutics and therapeutic drug carriers?, Adv. Drug Delivery Rev., 2022, 182, 114108 CrossRef CAS PubMed.
- O. Urzi, S. Raimondo and R. Alessandro, Extracellular Vesicles from Plants: Current Knowledge and Open Questions, Int. J. Mol. Sci., 2021, 22, 5366 CrossRef CAS PubMed.
- Y. Mao, M. Han and C. Chen,
et al., A biomimetic nanocomposite made of a ginger-derived exosome and an inorganic framework for high-performance delivery of oral antibodies, Nanoscale, 2021, 13, 20157–20169 RSC.
- Z. Deng, Y. Rong and Y. Teng,
et al., Broccoli-Derived Nanoparticle Inhibits Mouse Colitis by Activating Dendritic Cell AMP-Activated Protein Kinase, Mol. Ther., 2017, 25, 1641–1654 CrossRef CAS PubMed.
- S. P. Bruno, A. Paolini and V. D'Oria,
et al., Extracellular Vesicles Derived From Citrus sinensis Modulate Inflammatory Genes and Tight Junctions in a Human Model of Intestinal Epithelium, Front. Nutr., 2021, 8, 778998 CrossRef PubMed.
- C. Lei, Y. Teng and L. He,
et al., Lemon exosome-like nanoparticles enhance stress survival of gut bacteria by RNase P-mediated specific tRNA decay, iScience, 2021, 24, 102511 CrossRef CAS PubMed.
- H. A. Dad, T. W. Gu and A. Q. Zhu,
et al., Plant Exosome-like Nanovesicles: Emerging Therapeutics and Drug Delivery Nanoplatforms, Mol. Ther., 2021, 29, 13–31 CrossRef CAS PubMed.
- P. Sarvarian, P. Samadi and E. Gholipour,
et al., Application of Emerging Plant-Derived Nanoparticles as a Novel Approach for Nano-Drug Delivery Systems, Immunol. Invest., 2022, 51, 1039–1059 CrossRef CAS PubMed.
- S. Ju, J. Mu and T. Dokland,
et al., Grape exosome-like nanoparticles induce intestinal stem cells and protect mice from DSS-induced colitis, Mol. Ther., 2013, 21, 1345–1357 CrossRef CAS PubMed.
- M. Zu, D. Xie and B. S. B. Canup,
et al., 'Green' nanotherapeutics from tea leaves for orally targeted prevention and alleviation of colon diseases, Biomaterials, 2021, 279, 121178 CrossRef CAS PubMed.
- M. Zhang, X. Wang and M. K. Han,
et al., Oral administration of ginger-derived nanolipids loaded with siRNA as a novel approach for efficient siRNA drug delivery to treat ulcerative colitis, Nanomedicine, 2017, 12, 1927–1943 CrossRef CAS PubMed.
- W. Halperin and W. A. Jensen, Ultrastructural changes during growth and embryogenesis in carrot cell cultures, J. Ultrastruct. Res., 1967, 18, 428–443 CrossRef CAS PubMed.
- Y. Cui, J. Gao and Y. He,
et al., Plant extracellular vesicles, Protoplasma, 2020, 257, 3–12 CrossRef CAS PubMed.
- J. Kim, S. Li and S. Zhang,
et al., Plant-derived exosome-like nanoparticles and their therapeutic activities, Asian J. Pharm. Life Sci., 2022, 17, 53–69 Search PubMed.
- T. Karamanidou and A. Tsouknidas, Plant-Derived Extracellular Vesicles as Therapeutic Nanocarriers, Int. J. Mol. Sci., 2021, 23, 191 CrossRef PubMed.
- J. Wang, Y. Ding and J. Wang,
et al., EXPO, an exocyst-positive organelle distinct from multivesicular endosomes and autophagosomes, mediates cytosol to cell wall exocytosis in Arabidopsis and tobacco cells, Plant Cell, 2010, 22, 4009–4030 CrossRef CAS PubMed.
- B. He, R. Hamby and H. Jin, Plant extracellular vesicles: Trojan horses of cross-kingdom warfare, FASEB BioAdv., 2021, 3, 657–664 CrossRef PubMed.
- M. Alfieri, A. Leone and A. Ambrosone, Plant-Derived Nano and Microvesicles for Human Health and Therapeutic Potential in Nanomedicine, Pharmaceutics, 2021, 13, 498 CrossRef CAS PubMed.
- R. Kalluri and V. S. LeBleu, The biology, function, and biomedical applications of exosomes, Science, 2020, 367, eaau6977 CrossRef CAS PubMed.
- C. Xie, N. Ji and Z. Tang,
et al., The role of extracellular vesicles from different origin in the microenvironment of head and neck cancers, Mol. Cancer, 2019, 18, 83 CrossRef PubMed.
- M. Pinedo, L. de la Canal and C. de Marcos Lousa, A call for Rigor and standardization in plant extracellular vesicle research, J. Extracell. Vesicles, 2021, 10, e12048 Search PubMed.
- B. D. Rutter and R. W. Innes, Extracellular Vesicles Isolated from the Leaf Apoplast Carry Stress-Response Proteins, Plant Physiol., 2017, 173, 728–741 CrossRef CAS PubMed.
- J. Zhang, Y. Qiu and K. Xu, Characterization of GFP-AtPEN1 as a marker protein for extracellular vesicles isolated from Nicotiana benthamiana leaves, Plant Signaling Behav., 2020, 15, 1791519 CrossRef PubMed.
- M. Zhang, E. Viennois and C. Xu,
et al., Plant derived edible nanoparticles as a new therapeutic approach against diseases, Tissue Barriers, 2016, 4, e1134415 CrossRef PubMed.
- P. D. Subudhi, C. Bihari and S. K. Sarin,
et al., Emerging Role of Edible Exosomes-Like Nanoparticles (ELNs) as Hepatoprotective Agents, Nanotheranostics, 2022, 6, 365–375 CrossRef PubMed.
- F. Man, J. Wang and R. Lu, Techniques and Applications of Animal- and Plant-Derived Exosome-Based Drug Delivery System, J. Biomed. Nanotechnol., 2020, 16, 1543–1569 CrossRef CAS PubMed.
- N. Chen, J. Sun and Z. Zhu,
et al., Edible plant-derived nanotherapeutics and nanocarriers: recent progress and future directions, Expert Opin. Drug Delivery, 2022, 19, 409–419 CrossRef CAS PubMed.
- Y. Teng, Y. Ren and M. Sayed,
et al., Plant-Derived Exosomal MicroRNAs Shape the Gut Microbiota, Cell Host Microbe, 2018, 24, 637–652.e8 CrossRef CAS PubMed.
- X. Wang, S. P. Devaiah and W. Zhang,
et al., Signaling functions of phosphatidic acid, Prog. Lipid Res., 2006, 45, 250–278 CrossRef CAS PubMed.
- X. Chen, Y. Zhou and J. Yu, Exosome-like Nanoparticles from Ginger Rhizomes Inhibited NLRP3 Inflammasome Activation, Mol. Pharm., 2019, 16, 2690–2699 CrossRef CAS PubMed.
- P. Putta, J. Rankenberg and R. A. Korver,
et al., Phosphatidic acid binding proteins display differential binding as a function of membrane curvature stress and chemical properties, Biochim. Biophys. Acta, 2016, 1858, 2709–2716 CrossRef CAS PubMed.
- J. Y. Cho, S. G. Chi and H. S. Chun, Oral administration of docosahexaenoic acid attenuates colitis induced by dextran sulfate sodium in mice, Mol. Nutr. Food Res., 2011, 55, 239–246 CrossRef CAS PubMed.
- W. Stremmel, U. Merle and A. Zahn,
et al., Retarded release phosphatidylcholine benefits patients with chronic active ulcerative colitis, Gut, 2005, 54, 966–971 CrossRef CAS PubMed.
- C. Stanly, M. Moubarak and I. Fiume,
et al., Membrane Transporters in Citrus clementina Fruit Juice-Derived Nanovesicles, Int. J. Mol. Sci., 2019, 20, 6205 CrossRef CAS PubMed.
- M. D. C. Martinez-Ballesta, P. Garcia-Gomez and L. Yepes-Molina,
et al., Plasma membrane aquaporins mediates vesicle stability in broccoli, PLoS One, 2018, 13, e0192422 CrossRef PubMed.
- M. Arpin, D. Chirivino and A. Naba,
et al., Emerging role for ERM proteins in cell adhesion and migration, Cell Adhes. Migr., 2011, 5, 199–206 CrossRef PubMed.
- L. Garaeva, R. Kamyshinsky and Y. Kil,
et al., Delivery of functional exogenous proteins by plant-derived vesicles to human cells in vitro, Sci. Rep., 2021, 11, 6489 CrossRef CAS PubMed.
- E. Woith, G. Fuhrmann and M. F. Melzig, Extracellular Vesicles-Connecting Kingdoms, Int. J. Mol. Sci., 2019, 20, 5695 CrossRef CAS PubMed.
- J. Kowal, G. Arras and M. Colombo,
et al., Proteomic comparison defines novel markers to characterize heterogeneous populations of extracellular vesicle subtypes, Proc. Natl. Acad. Sci. U. S. A., 2016, 113, E968–E977 CrossRef CAS PubMed.
- L. Zhang, D. Hou and X. Chen,
et al., Exogenous plant MIR168a specifically targets mammalian LDLRAP1: evidence of cross-kingdom regulation by microRNA, Cell Res., 2012, 22, 107–126 CrossRef CAS PubMed.
- L. Del Pozo-Acebo, M. C. Lopez de Las Hazas and A. Margolles,
et al., Eating microRNAs: pharmacological opportunities for cross-kingdom regulation and implications in host gene and gut microbiota modulation, Br. J. Pharmacol., 2021, 178, 2218–2245 CrossRef CAS PubMed.
- Q. Cai, L. Qiao and M. Wang,
et al., Plants send small RNAs in extracellular vesicles to fungal pathogen to silence virulence genes, Science, 2018, 360, 1126–1129 CrossRef CAS PubMed.
- J. Xiao, S. Feng and X. Wang,
et al., Identification of exosome-like nanoparticle-derived microRNAs from 11 edible fruits and vegetables, PeerJ, 2018, 6, e5186 CrossRef PubMed.
- M. Rahimi Ghiasi, E. Rahimi and Z. Amirkhani,
et al., Leucine-rich Repeat-containing G-protein Coupled Receptor 5 Gene Overexpression of the Rat Small Intestinal Progenitor Cells in Response to Orally Administered Grape Exosome-like Nanovesicles, Adv. Biomed. Res., 2018, 7, 125 CrossRef PubMed.
- K. Sundaram, D. P. Miller and A. Kumar,
et al., Plant-Derived Exosomal Nanoparticles Inhibit Pathogenicity of Porphyromonas gingivalis, iScience, 2019, 21, 308–327 CrossRef CAS PubMed.
- M. Cao, H. Yan and X. Han,
et al., Ginseng-derived nanoparticles alter macrophage polarization to inhibit melanoma growth, J. Immunother. Cancer., 2019, 7, 326 CrossRef PubMed.
- M. A. Rider, S. N. Hurwitz and D. G. Meckes Jr, ExtraPEG: A Polyethylene Glycol-Based Method for Enrichment of Extracellular Vesicles, Sci. Rep., 2016, 6, 23978 CrossRef CAS PubMed.
- M. C. Deregibus, F. Figliolini and S. D'Antico,
et al., Charge-based precipitation of extracellular vesicles, Int. J. Mol. Med., 2016, 38, 1359–1366 CrossRef CAS PubMed.
- P. Li, M. Kaslan and S. H. Lee,
et al., Progress in Exosome Isolation Techniques, Theranostics, 2017, 7, 789–804 CrossRef CAS PubMed.
- P. Sharma, S. Ludwig and L. Muller,
et al., Immunoaffinity-based isolation of melanoma cell-derived exosomes from plasma of patients with melanoma, J. Extracell. Vesicles, 2018, 7, 1435138 CrossRef PubMed.
- S. Suharta, A. Barlian and A. C. Hidajah,
et al., Plant-derived exosome-like nanoparticles: A concise review on its extraction methods, content, bioactivities, and potential as functional food ingredient, J. Food Sci., 2021, 86, 2838–2850 CrossRef CAS PubMed.
- M. K. Sriwastva, Z. B. Deng and B. Wang,
et al., Exosome-like nanoparticles from Mulberry bark prevent DSS-induced colitis via the AhR/COPS8 pathway, EMBO Rep., 2022, e53365 CrossRef CAS PubMed.
- M. Zhang, E. Viennois and M. Prasad,
et al., Edible ginger-derived nanoparticles: A novel therapeutic approach for the prevention and treatment of inflammatory bowel disease and colitis-associated cancer, Biomaterials, 2016, 101, 321–340 CrossRef CAS PubMed.
- T. Khare, S. S. Palakurthi and B. M. Shah,
et al., Natural Product-Based Nanomedicine in Treatment of Inflammatory Bowel Disease, Int. J. Mol. Sci., 2020, 21, 3956 CrossRef CAS PubMed.
- J. Mu, X. Zhuang and Q. Wang,
et al., Interspecies communication between plant and mouse gut host cells through edible plant derived exosome-like nanoparticles, Mol. Nutr. Food Res., 2014, 58, 1561–1573 CrossRef CAS PubMed.
- B. Wang, X. Zhuang and Z. B. Deng,
et al., Targeted drug delivery to intestinal macrophages by bioactive nanovesicles released from grapefruit, Mol. Ther., 2014, 22, 522–534 CrossRef CAS PubMed.
- S. Guo, W. Geng and S. Chen,
et al., Ginger Alleviates DSS-Induced Ulcerative Colitis Severity by Improving the Diversity and Function of Gut Microbiota, Front. Pharmacol., 2021, 12, 632569 CrossRef CAS PubMed.
- F. Zhang, N. Ma and Y. F. Gao,
et al., Therapeutic Effects of 6-Gingerol, 8-Gingerol, and 10-Gingerol on Dextran Sulfate Sodium-Induced Acute Ulcerative Colitis in Rats, Phytother. Res., 2017, 31, 1427–1432 CrossRef CAS PubMed.
- N. A. Lashgari, N. Momeni Roudsari and D. Khayatan,
et al., Ginger and its constituents: Role in treatment of inflammatory bowel disease, Biofactors, 2022, 48, 7–21 CrossRef CAS PubMed.
- A. C. Brown, C. Shah and J. Liu,
et al., Ginger's (Zingiber officinale Roscoe) inhibition of rat colonic adenocarcinoma cells proliferation and angiogenesis in vitro, Phytother. Res., 2009, 23, 640–645 CrossRef PubMed.
- R. Grzanna, L. Lindmark and C. G. Frondoza, Ginger--an herbal medicinal product with broad anti-inflammatory actions, J. Med. Food, 2005, 8, 125–132 CrossRef CAS PubMed.
- E. A. Al-Suhaimi, N. A. Al-Riziza and R. A. Al-Essa, Physiological and therapeutical roles of ginger and turmeric on endocrine functions, Am. J. Chin. Med., 2011, 39, 215–231 CrossRef CAS PubMed.
- A. P. Suresh, S. P. Kalarikkal and B. Pullareddy,
et al., Low pH-Based Method to Increase the Yield of Plant-Derived Nanoparticles from Fresh Ginger Rhizomes, ACS Omega, 2021, 6, 17635–17641 CrossRef CAS PubMed.
- M. Zhang, C. Xu and D. Liu,
et al., Oral Delivery of Nanoparticles Loaded With Ginger Active Compound, 6-Shogaol, Attenuates Ulcerative Colitis and Promotes Wound Healing in a Murine Model of Ulcerative Colitis, J Crohns Colitis, 2018, 12, 217–229 CrossRef PubMed.
- X. Zhou, X. Liu and Q. He,
et al., Ginger Extract Decreases Susceptibility to Dextran Sulfate Sodium-Induced Colitis in Mice Following Early Antibiotic Exposure, Front. Med., 2021, 8, 755969 CrossRef PubMed.
- D. Chen, G. Chen and C. Chen,
et al., Prebiotics effects in vitro of polysaccharides from tea flowers on gut microbiota of healthy persons and patients with inflammatory bowel disease, Int. J. Biol. Macromol., 2020, 158, 968–976 CrossRef CAS PubMed.
- L. Q. Liu, S. P. Nie and M. Y. Shen,
et al., Tea Polysaccharides Inhibit Colitis-Associated Colorectal Cancer via Interleukin-6/STAT3 Pathway, J. Agric. Food Chem., 2018, 66, 4384–4393 CrossRef CAS PubMed.
- C. Kurbitz, D. Heise and T. Redmer,
et al., Epicatechin gallate and catechin gallate are superior to epigallocatechin gallate in growth suppression and anti-inflammatory activities in pancreatic tumor cells, Cancer Sci., 2011, 102, 728–734 CrossRef PubMed.
- Y. S. Chiou, Q. Huang and C. T. Ho,
et al., Directly interact with Keap1 and LPS is involved in the anti-inflammatory mechanisms of (-)-epicatechin-3-gallate in LPS-induced macrophages and endotoxemia, Free Radical Biol. Med., 2016, 94, 1–16 CrossRef CAS PubMed.
- F. Y. Fan, L. X. Sang and M. Jiang, Catechins and Their Therapeutic Benefits to Inflammatory Bowel Disease, Molecules, 2017, 22, 3956 Search PubMed.
- L. Antonioli, R. Colucci and C. Pellegrini,
et al., The AMPK enzyme-complex: from the regulation of cellular energy homeostasis to a possible new molecular target in the management of chronic inflammatory disorders, Expert Opin. Ther. Targets, 2016, 20, 179–191 CrossRef CAS PubMed.
- A. Hasanvand, The role of AMPK-dependent pathways in cellular and molecular mechanisms of metformin: a new perspective for treatment and prevention of diseases, Inflammopharmacology, 2022, 30, 775–788 CrossRef CAS PubMed.
- S. Banskota, H. Wang and Y. H. Kwon,
et al., Salicylates Ameliorate Intestinal Inflammation by Activating Macrophage AMPK, Inflammatory Bowel Dis., 2021, 27, 914–926 CrossRef PubMed.
- H. Park, W. Kim and D. Kim,
et al., Mesalazine Activates Adenosine Monophosphate-activated Protein Kinase: Implication in the Anti-inflammatory Activity of this Anti-colitic Drug, Curr. Mol. Pharmacol., 2019, 12, 272–280 CrossRef CAS PubMed.
- A. Asai, K. Nakagawa and O. Higuchi,
et al., Effect of mulberry leaf extract with enriched 1-deoxynojirimycin content on postprandial glycemic control in subjects with impaired glucose metabolism, J. Diabetes Invest., 2011, 2, 318–323 CrossRef CAS PubMed.
- P. Aramwit, O. Supasyndh and T. Siritienthong,
et al., Mulberry leaf reduces oxidation and C-reactive protein level in patients with mild dyslipidemia, BioMed Res. Int., 2013, 2013, 787981 Search PubMed.
- N. He, C. Zhang and X. Qi,
et al., Draft genome sequence of the mulberry tree Morus notabilis, Nat. Commun., 2013, 4, 2445 CrossRef PubMed.
- R. Sreedhar, S. Arumugam and R. A. Thandavarayan,
et al., Curcumin as a therapeutic agent in the chemoprevention of inflammatory bowel disease, Drug Discovery Today, 2016, 21, 843–849 CrossRef CAS PubMed.
- Y. He, Y. Yue and X. Zheng,
et al., Curcumin, inflammation, and chronic diseases: how are they linked?, Molecules, 2015, 20, 9183–9213 CrossRef CAS PubMed.
- L. Vecchi Brumatti, A. Marcuzzi and P. M. Tricarico,
et al., Curcumin and inflammatory bowel disease: potential and limits of innovative treatments, Molecules, 2014, 19, 21127–21153 CrossRef PubMed.
- C. Liu, X. Yan and Y. Zhang,
et al., Oral administration of turmeric-derived exosome-like nanovesicles with anti-inflammatory and pro-resolving bioactions for murine colitis therapy, J. Nanobiotechnol., 2022, 20, 206 CrossRef CAS PubMed.
- Y. Cai, L. Zhang and Y. Zhang,
et al., Plant-Derived Exosomes as a Drug-Delivery Approach for the Treatment of Inflammatory Bowel Disease and Colitis-Associated Cancer, Pharmaceutics, 2022, 14, 822 CrossRef CAS PubMed.
- H. T. Nguyen, G. Dalmasso and L. Torkvist,
et al., CD98 expression modulates intestinal homeostasis, inflammation, and colitis-associated cancer in mice, J. Clin. Invest., 2011, 121, 1733–1747 CrossRef CAS PubMed.
- T. Kucharzik, A. Lugering and Y. Yan,
et al., Activation of epithelial CD98 glycoprotein perpetuates colonic inflammation, Lab. Invest., 2005, 85, 932–941 CrossRef CAS PubMed.
- D. A. Sussman, R. Santaolalla and S. Strobel,
et al., Cancer in inflammatory bowel disease: lessons from animal models, Curr. Opin. Gastroenterol., 2012, 28, 327–333 CrossRef CAS PubMed.
- Y. W. An, K. A. Jhang and S. Y. Woo,
et al., Sulforaphane exerts its anti-inflammatory effect against amyloid-beta peptide via STAT-1 dephosphorylation and activation of Nrf2/HO-1 cascade in human THP-1 macrophages, Neurobiol. Aging, 2016, 38, 1–10 CrossRef CAS PubMed.
- N. Juge, R. F. Mithen and M. Traka, Molecular basis for chemoprevention by sulforaphane: a comprehensive review, Cell. Mol. Life Sci., 2007, 64, 1105–1127 CrossRef CAS PubMed.
- C. C. Sun, S. J. Li and C. L. Yang,
et al., Sulforaphane Attenuates Muscle Inflammation in Dystrophin-deficient mdx Mice via NF-E2-related Factor 2 (Nrf2)-mediated Inhibition of NF-kappaB Signaling Pathway, J. Biol. Chem., 2015, 290, 17784–17795 CrossRef CAS PubMed.
- B. Mahmood, R. Abbas and S. Saman,
et al., Nanotechnology for inflammatory bowel disease management: Detection, imaging and treatment, Sens. Bio-Sens. Res., 2021, 32, 100417 CrossRef.
- S. Hua, E. Marks and J. J. Schneider,
et al., Advances in oral nano-delivery systems for colon targeted drug delivery in inflammatory bowel disease: selective targeting to diseased versus healthy tissue, Nanomedicine, 2015, 11, 1117–1132 CrossRef CAS PubMed.
- B. M. Shah, S. S. Palakurthi and T. Khare,
et al., Natural proteins and polysaccharides in the development of micro/nano delivery systems for the treatment of inflammatory bowel disease, Int. J. Biol. Macromol., 2020, 165, 722–737 CrossRef CAS PubMed.
- F. Giron, A. Pasto and E. Tasciotti,
et al., Nanotechnology in the Treatment of Inflammatory Bowel Disease, Inflammatory Bowel Dis., 2019, 25, 1871–1880 CrossRef PubMed.
- J. K. Hou, B. Abraham and H. El-Serag, Dietary intake and risk of developing inflammatory bowel disease: a systematic review of the literature, Am. J. Gastroenterol., 2011, 106, 563–573 CrossRef CAS PubMed.
- C. E. Spooren, M. J. Pierik and M. P. Zeegers,
et al., Review article: the association of diet with onset and relapse in patients with inflammatory bowel disease, Aliment. Pharmacol. Ther., 2013, 38, 1172–1187 CrossRef CAS PubMed.
- C. S. Antoniussen, H. H. Rasmussen and M. Holst,
et al., Reducing Disease Activity of Inflammatory Bowel Disease by Consumption of Plant-Based Foods and Nutrients, Front. Nutr., 2021, 8, 733433 CrossRef PubMed.
- D. F. Li, M. F. Yang and H. M. Xu,
et al., Nanoparticles for oral delivery: targeted therapy for inflammatory bowel disease, J. Mater. Chem. B, 2022, 10, 5853–5872 RSC.
- Y. Liang, L. Duan and J. Lu,
et al., Engineering exosomes for targeted drug delivery, Theranostics, 2021, 11, 3183–3195 CrossRef CAS PubMed.
- Y. Liang, X. Xu and X. Li,
et al., Chondrocyte-Targeted MicroRNA Delivery by Engineered Exosomes toward a Cell-Free Osteoarthritis Therapy, ACS Appl. Mater. Interfaces, 2020, 12, 36938–36947 CrossRef CAS PubMed.
- X. Xu, Y. Liang and X. Li,
et al., Exosome-mediated delivery of kartogenin for chondrogenesis of synovial fluid-derived mesenchymal stem cells and cartilage regeneration, Biomaterials, 2021, 269, 120539 CrossRef CAS PubMed.
- L. Duan, K. Ouyang and J. Wang,
et al., Exosomes as Targeted Delivery Platform of CRISPR/Cas9 for Therapeutic Genome Editing, Chembiochem, 2021, 22, 3360–3368 CrossRef CAS PubMed.
- Y. Liang, X. Xu and L. Xu,
et al., Chondrocyte-specific genomic editing enabled by hybrid exosomes for osteoarthritis treatment, Theranostics, 2022, 12, 4866–4878 CrossRef CAS PubMed.
- Y. Liang, Z. Iqbal and J. Wang,
et al., Cell-derived extracellular vesicles for CRISPR/Cas9 delivery: engineering strategies for cargo packaging and loading, Biomater. Sci., 2022, 10, 4095–4106 RSC.
- Y. Liu, S. Wu and Y. Koo,
et al., Characterization of and isolation methods for plant leaf nanovesicles and small extracellular vesicles, Nanomedicine, 2020, 29, 102271 CrossRef CAS PubMed.
Footnote |
† These authors contributed equally to the manuscript. |
|
This journal is © The Royal Society of Chemistry 2023 |
Click here to see how this site uses Cookies. View our privacy policy here.