An electron-donating system composed of a CIDS@CFO@F0C0 catalyst for the sustainable generation of free radicals to inactivate pathogens†
Received
16th August 2023
, Accepted 14th November 2023
First published on 6th December 2023
Abstract
Transition-metal-catalyzed reactive oxygen species (ROS) play an important role in existing bactericidal systems, but there are some problems, such as poor stability, ease of dissolution, and difficulty in reusing. To address the issue of stability, we have developed a sufficient electron-donating (SED) system. In the presence of the two most active transition metals with multiple valences, several as-formed redox pairs can provide sufficient electrons, and then effectively trigger the reduction of H2O/O2 to produce 1O2, ·OH and ·O2− without any assistance from extra energy or other active oxidants. Consequently, the SED system showed 100% removal efficiency for Escherichia coli in 2 h, and also showed remarkable stability and reusability with 85% inactivation after 11 repetitions. It is worth noting that ·OH and 1O2 play a major role in the bactericidal process. While ·O2− mainly plays a role in promoting the formation of 1O2 and ·OH.
Environmental significance
From the beginning of human society, microbial contamination has always been a great challenge. Waterborne pathogens have led to the death of tens of thousands of people around the world and the non-fatal infection of more than 2 billion people each year. Pathogenic microorganisms in water mainly come from human and animal feces, and human and animal feces contain a large number of Escherichia coli (E. coli). E. coli can cause a variety of infections, such as peritonitis, cholecystitis, cystitis and diarrhea.2,3 Serious E. coli infections can be life-threatening and result in the death of 2 million people in the world each year.4,5 Thus, exploring the development of methods that can effectively inactivate E. coli has been a significant hotspot issue.
|
1. Introduction
From the beginning of human society, microbial contamination has always been a great challenge.1 Waterborne pathogens have led to the death of tens of thousands of people around the world and the non-fatal infection of more than 2 billion people each year. Pathogenic microorganisms in water mainly come from human and animal feces, and human and animal feces contain a large number of Escherichia coli (E. coli). E. coli can cause a variety of infections, such as peritonitis, cholecystitis, cystitis and diarrhea.2,3 Serious E. coli infections can be life-threatening and result in the death of 2 million people in the world each year.4,5 Thus, exploring the development of methods that can effectively inactivate E. coli has been a significant hotspot issue.
Some studies have shown that reactive oxygen species (ROS) are active substances found throughout cells and are used to support the life activities of microorganisms.6 Yet, excessive ROS could attack cell membranes, proteins and DNA, leading to the destruction of microorganisms.7 Due to this feature, stimulating the generation of large amounts of ROS through catalytic methods has shown promising bactericidal feasibility.8 So far, catalytic methods for generating ROS include photocatalysis,9,10 electric catalysis11 and Fenton oxidation.12 However, some of these require extra energy such as light or electricity to excite electrons to trigger the oxidation–reduction reactions; while others need the assistance of highly active oxidants to accelerate the reactions and ease the formation of radicals.13,14 Consequently, the cost will be greatly increased, which will significantly impede their practical application.15 In recent years, catalyzing O2/H2O by strong reductants to obtain ROS has attracted extensive attention.16,17 Among many methods, they have the characteristics of fast reaction speed and low energy consumption, which will be a promising strategy for bacterial inactivation.18,19 Transition metal ions, as excellent electron donors and transporters, are considered to be one of the strongest reductants.20 But a single-metal catalytic system, which lacks sustainability because of rapid oxidation, shows great limitation in the production of large amounts of ROS.21 Moreover, due to the poor stability of the metal, it is easy to cause secondary pollution, which poses a threat to human health.22,23 It is very necessary to explore a sustainable metallic catalyzation system for efficient ROS generation, which is the main focus of this study.
To solve this problem, Jia et al. discovered that the formation of a redox pair between two reductive substances can improve both catalytic efficiency and system stability.24 It is very possible that a redox pair can be formed in the co-presence of different metals with multiple valences.25 CoFe2O4 and Fe–Co alloys are typical materials containing both Fe and Co at the same time, and their excellent proton-donor properties and catalytic performance have been widely clarified.26 Combined with the advantages of high efficiency, environmental friendliness and abundant resources, Fe0/Fe2+/Fe3+ and Co0/Co2+/Co3+ might construct a theoretical sufficient electron-donating system to achieve the purpose of effective sustainable ROS generation.27 Nevertheless, the large-scale application of transition metals and their composites still faces some technical challenges. The most significant one is that low-valent transition metals, especially zero-valence ones, are very active materials that easily agglomerate during synthesis.28 This will result in reduced active sites and catalytic performance in the system.29 Also, they cannot be automatically formed without a reductant, and usually need tedious preparation methods with two or more steps.30 Providing an appropriate reductive carrier, such as porous carbon, is a promising way to address the above issues.31,32 In addition, fringe benefits can be offered and the catalytic performance of metals can be enhanced since carbon-based materials usually possess good electric conductivities and adsorption affinities.33
Our previous study found that using a chelating agent as the raw material can promote the degree of dispersion of metal particles through one-step thermal decomposition preparation.34 Co-incorporated N-doped porous carbon (CFO/Fe@C) was successfully prepared using EDTA, Fe salt and Co salt as the starting materials. EDTA took on multiple significant parts, such as carbon source, metal capturer and nitrogen supplier, in the course of the reaction.35 Iminodisuccinate (IDS) usually shows better chelating forces to typical transition metals such as iron than EDTA.36 In this study, IDS was selected to be the raw material. Besides the above-mentioned roles of EDTA, IDS can further benefit the formation of a porous structure for the derived carbon resulting from the transformation of carboxyl functional groups to CO2 during treatment at elevated temperature. A hybrid catalyst, CIDS@CFO@F0C0, constituting a sufficient electron-donating (SED) system, was obtained through one-step pyrolysis of IDS, Co salt and Fe salt. Detailed surveys of the structure and surface morphology of CIDS@CFO@F0C0 were conducted. The E. coli inactivation performance of the SED system and the effects of key parameters are demonstrated. The stability and reusability of the SED system were also investigated. By combining ROS quenching experiments with the bactericidal results, the ROS that play a key role in the bactericidal process are inferred. Moreover, a reasonable mechanism is also proposed to explore the efficient inactivation capability of the SED system.
2. Materials and methods
2.1. Chemicals and reagents
Cobalt(II) nitrate hexahydrate (Co(NO3)2·6H2O), iron(III) nitrate nonahydrate (Fe(NO3)3·9H2O), tetrasodium iminodisuccinate (30%, IDS), tryptone, yeast extract and sodium chloride (NaCl) were purchased from Aladdin. All drugs were of analytical purity.
2.2. Preparation of CIDS@CFO@F0C0
Firstly, 2.02 g of Fe(NO3)3·9H2O, 0.365 g of Co(NO3)2·6H2O and 10 mL of IDS were dispersed evenly in 10 mL of distilled water through magnetic stirring (15 rpm min−1, 90 °C). A homogeneous slurry was formed and then dried in an oven at 80 °C for 8 h. The mixed substance was passed into a tubular furnace (HF-KEJING, OTF-1200X) for calcination at 500 °C for 2 h at a heating rate of 10 °C min−1. Then, the as-prepared material was washed with distilled water to remove excess substances. Finally, the sample was dried at 80 °C for 1 d and the final material CIDS@CFO@F0C0 was obtained.
2.3. Preparation of contrast samples
Porous carbon was compounded in the same way under identical conditions, using IDS as the raw material. The carbon was named CIDS for convenience. Similarly, C@Fe3O4 and C@Co0 were prepared using the above method except that Co(NO)2 or Fe(NO)3 were not added, respectively.
2.4. Antimicrobial evaluation
2.4.1. Bacterial culture and preparation.
E. coli cells were selected as typical contaminating microbes and expanded in a shaking incubator at 37 °C with a speed of 150 rpm in Luria–Bertani (LB) medium for 18 h. Until the optical density OD600 of the colony grew beyond 0.4, the suspension was centrifuged (at 5000 rpm) for 10 min. Phosphate buffered saline (PBS) was used to remove the nutrients of the suspension and further dilute the E. coli cells to 105 cfu mL−1.
2.4.2. Inactivation experiments.
To explore the inactivation effect on E. coli in the SED system, CIDS@CFO@F0C0 with different masses (0, 0.01, 0.02, 0.03, 0.04, 0.05, 0.06, 0.07, 0.08, 0.09, 0.1 g) was added into 250 mL conical flasks. Then, 100 mL of a solution containing 1 mL of 105 cfu mL−1 bacterial suspension and 99 mL of distilled water after inactivation was put into the same conical flask. The solution without CIDS@CFO@F0C0 served as the control group. The conical flasks were placed in a shaker (200 rpm, 37 °C) for 24 h. After being diluted and smeared, the E. coli cells were incubated at 37 °C for 24 h. The efficiency of killing E. coli was calculated via the plate counting method. In each cycle, CIDS@CFO@F0C0 was collected without washing, and directly added into another 1 mL of bacterial solution. The process described above was repeated six times. Different materials (C@Fe3O4, C@Co0 and CIDS) were also investigated through the above operations.
2.4.3. Exploring the influencing factors.
The factors influencing the antibacterial process were explored by changing the light, oxygen and water environment. The effect of light on bactericidal activity was explored by killing bacteria with or without light. By comparing aerobic and anaerobic conditions, it is clear whether oxygen affects the inactivation performance of the SED system. The effect of the water environment on disinfection by the SED system was studied in different water bodies (distilled water, tap water, river water). The river water was collected from Liuyang River, Changsha, Hunan, P.R. China (112°58′42″E; 28°11′49″N).
2.5. ROS determination
2.5.1. Detection of ROS generation.
An EPR spectrometer was used to detect the generation and kinds of ROS in the FRQB system. After that, the relationship between the generation of ROS and oxygen in the system was tested using EPR under vacuum conditions.
2.5.2. Formation and content determination of hydrogen peroxide.
0.1 g of the composite was added to 10 mL of ultrapure water. After mixing and standing, the H2O2 content (at 3, 6, 10, and 20 min) was determined using potassium permanganate titration.
2.5.3. ·OH and ·O2− content detection.
Based on the electron transport process, the corresponding chromogenic agent was added to discolor the reaction system and the absorbance was tested with a microplate reader. The absorbance is proportional to the amount of free radicals. Detailed procedures are provided in ESI.†
2.5.4. Quenching experiments for ROS.
Different ROS were quenched by adding different quencher agents. 50 mmol L−1 methanol was added to quench ·OH in the system. 5 mmol L−1 benzoquinone was used to quench ·O2−. 5 mmol L−1 furfuryl alcohol was used to quench 1O2. A quenching agent without the catalyst was set as a blank control.
3. Results and discussion
3.1. Characterization of CIDS@CFO@F0C0
XRD analysis of CIDS@CFO@F0C0 is shown in Fig. S1a† (green line). The major characteristic peaks located at 2θ = 30.1°, 35.4°, 43.1°, 57.0° and 62.6° were respectively attributed to the (220), (311), (400), (511) and (440) crystal planes belonging to spinel-type CoFe2O4 (JCPDS no. 22-1086).37 The other peaks found at 2θ = 44.9° and 65.3° corresponded to the main (110) and (200) bands of Fe0Co0 (JCPDS no. 49-1568).38 The above data confirm that crystals of Fe0Co0 and CoFe2O4 were well formed in CIDS@CFO@F0C0. Interestingly, Co0 was found when using IDS and cobalt salt as the feedstock (orange line) but only carbon-supported Fe3O4 was obtained from a mixture of IDS and iron salt (yellow line), indicating that Fe0 could not be obtained without the co-presence of cobalt. A probable reason might be provided by Table 1 and the following equations. | 4Fe(NO3)3·9H2O = 2Fe2O3 + 12NO2 + 3O2 + 36H2O | (1) |
| 2Co(NO3)2·6H2O = 2CoO + 4NO2 + O2 + 12H2O | (2) |
| 2Fe2O3 + 3C = 4Fe + 3CO2 | (3) |
| ΔxGθm = ΔrHθm − TΔrSθm | (5) |
| 2C + Fe2O3 + CoO = 2CO2 + 2Fe + Co | (6) |
Table 1 The standard Gibbs free energy
|
ΔfHθm (kJ mol−1) |
ΔfGθm (kJ mol−1) |
S
θm (J mol−1 K−1) |
C |
0 |
0 |
5.740 |
CO |
−110.525 |
−137.168 |
197.674 |
CO2 |
−393.509 |
−394.359 |
213.74 |
Co |
0 |
0 |
30.0 |
CoO |
−237.9 |
−214.2 |
53.0 |
Fe |
0 |
0 |
27.28 |
Fe2O3 |
−824.2 |
−742.2 |
87.40 |
Typically, Fe(NO3)3 and Co(NO3)2 would degrade into ferric oxide and cobalt oxide under heat.35 In the presence of carbon, the metal oxides may be further reduced into metal elements (eqn (3) and (4)), depending on the reaction conditions where temperature plays a key role.39 The direction and possibility of a chemical reaction can usually be estimated from the changes in Gibbs free energy (ΔxGθm), which can be calculated based on parameters including enthalpy change (ΔrHθm), entropy change (ΔrSθm), and reaction temperature (eqn (5)). According to the ΔxGθm formula, the iron reduction reaction can only be triggered when the pyrolysis temperature is above 838.00 K (564.85 °C). While the cobalt reduction reaction needs much less energy and a 753.99 K (480.84 °C) calcination temperature is high enough. Since the preparation temperature of CIDS@CFO@F0C0 was set to 500 °C, it is highly possible that the ferric oxide could not be reduced to form Fe0 while Co0 could be obtained from the reduction of CoO by carbon. For the formation of zero-valent iron in the composite, it is speculated that a possible reason is that Fe0Co0 can be prepared by carbon thermal reduction at 763.77 K (490.62 °C) in the presence of cobalt (eqn (6)).
For further investigation, various proportions of iron and cobalt were set and three different counterparts were prepared, with the same synthesis process. Fig. S1b† shows the XRD patterns of those three samples, which all contained the typical diffraction peaks corresponding to Fe0Co0 and CoFe2O4. It can be concluded that Fe0Co0 alloys and CoFe2O4 crystals could be obtained using the selected starting materials, despite the difference in Fe
:
Co ratio. By extending the XRD pattern range to 35–36° in Fig. 1b, the changes in lattice parameters of CoFe2O4 could be analyzed in detail. With an increase in cobalt content, the diffraction peak 2θ = 35.4° gradually shifted to a higher direction, suggesting that the crystal structure of CFO might be distorted by doping with Co. It has been reported that metal ions in the occupied lattice position could be substituted by doping ions with similar valence and smaller radii.40 The ion radius of Fe (r(Fe2+) = 0.078 nm; r(Fe3+) = 0.0645 nm) is larger than that of Co (r(Co2+) = 0.0745 nm; r(Co3+) = 0.061 nm) with the same valence state. Consequently, Co ions were possibly inserted into the octahedral position, resulting in cell contraction and lattice reduction. As reported, crystal cell distortion can lead to an increase in surface oxygen species.41 Moreover, such structural disorder might also constitute the active center of the catalyst and provide more active sites for the reaction.42 All of which will enhance the catalytic performance of the SED system.
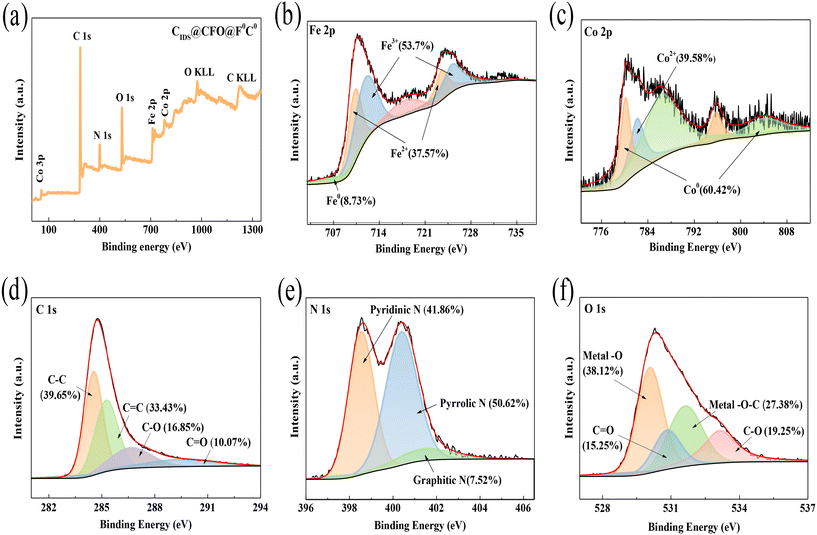 |
| Fig. 1 (a) XPS survey spectrum, (b) Fe 2p, (c) Co 2p, (d) C 1s, (e) N 1s and (f) O 1s spectra of XPS of CIDS@CFO@F0C0. | |
Detection of the morphology of CIDS and CIDS@CFO@F0C0 was carried out by SEM. Fig. S2a† (left) shows that the carbon material derived from IDS had a loose and porous structure. The highly porous structure was conducive to the adhesion of metal particles, avoiding leakage and maintaining the stability. After the addition of iron and cobalt, the loose porous structure of the carbon basically disappeared. For CIDS@CFO@F0C0 (Fig. S2a† (right)), it can be observed that there were many tiny nano-spherical particles that were evenly distributed on the surface of the carbon carrier. The EDS diagram (Fig. S2b†), which can be used to analyze the elemental distribution, shows that those small particles were rich in iron and cobalt elements, further proving the formation of CoFe2O4 and Co0Fe0 composites. In addition, analysis of the TEM image (Fig. S2c†) shows that uniform particles are loaded on the material, which is the same result as in the SEM image. There are two kinds of lattice fringes observed in Fig. S2d,† corresponding to CoFe2O4 and Fe0Co0, respectively. BET analysis of the two materials found that the specific surface area and aperture of CIDS@CFO@F0C0 (Fig. S3b†) were both smaller than those of CIDS (Fig. S3a†), which should be attributed to embedding of the metal particles. Combined with the above characterization results, it can be concluded that the composites of Fe0Co0 and CoFe2O4 were successfully loaded onto the carbon substrate, and distributed uniformly without agglomeration.
To better determine the chemical state of the elements, XPS analysis of CIDS@CFO@F0C0 was conducted (Fig. 1). By observing the characteristic peaks, it can be proved that the composite material was composed of five elements: C, N, O, Co and Fe (Fig. 1a). There were four Gaussian peaks in the C 1s high-resolution XPS spectrum at 284.66 eV, 285.56 eV, 286.74 eV and 289.56 eV, corresponding to C–C, C
C, C–O and C
O bonds, respectively (Fig. 1d). The N 1s energy spectrum can be split into pyridinic-N (398.54 eV), pyrrolic-N (400.40 eV) and graphitic-N (401.41 eV) (Fig. 1e). Studies have shown that nitrogen doping can enhance the binding energy between the active substance and the carrier,43 and nitrogen-doped carbon here might also benefit the redox ability of metals.44 The O 1s spectrum for CIDS@CFO@F0C0 can be divided into four peaks assigned to metal–O, C
O, metal–O–C and C–O (Fig. 1f). Those detected peaks proved that the metal and carbon substrate were firmly bonded in the form of chemical force, which would improve the stability of the metals.45,46 For the Co 2p spectrum (Fig. 1c), Co0 at 780.23 eV and 795.87 eV, and Co2+ at 782.22 eV were observed. The presence of Co2+ in CIDS@CFO@F0C0 was mainly ascribed to the presence of Co2+ in CoFe2O4 and the partial oxidation of Co0 on the surface of the catalyst. The coexistence of Co0 and Co2+ could greatly increase the catalytic effect of CIDS@CFO@F0C0 due to the electron transfer caused by the change in valence between Co0/Co2+, which would also promote the formation of ROS. In a similar manner, the XPS spectrum of Fe 2p (Fig. 1b) shows three chemical species. The presence of Fe0, which can accelerate electron transfer on the surface of CIDS@CFO@F0C0, was confirmed by observing the peak at 707.81 eV. The peaks located at 711.95 eV and 725.24 eV represent 2p2/3 and 2p1/2 of Fe3+, respectively, while the peaks at 710.34 eV and 723.62 eV illustrate the existence of Fe2+. The results of XPS analysis further confirmed that both CoFe2O4 and Fe0Co0 particles existed in the hybrid catalyst.
3.2. The bactericidal effect of the SED system
The effects of different samples, CIDS, C@Fe3O4, C@Co0 and CIDS@CFO@F0C0, on the removal of E. coli were studied and results were obtained from three parallel specimens. Typically, the bactericidal effect of the four catalysts increased with an increase in the quantity of material and gradually reached equilibrium. As summarized in Fig. 2a, after 24 hours of inactivation, CIDS@CFO@F0C0 exhibited a much higher bacterial removal efficiency than its three counterparts, presenting a sequence of CIDS@CFO@F0C0 (100%) > C@Co0 (61.13%) > C@Fe3O4 (42.06%) > CIDS (32.02%). It is worth noting that an outstanding bactericidal effect of up to 92.89% could be obtained with a low addition of CIDS@CFO@F0C0 of 0.05 g, while the highest inactivated efficiency that could be shown by the other three catalysts was only 61.13%, even though twice the mass (0.1 g) was used. Several studies have reported the good catalytic performance of Co0 and Fe3O4. However, the above results suggest that single-metal catalysts could not be effectively activated to form enough ROS and kill the bacteria. This phenomenon indicates that sufficient active radicals could only be stimulated with the co-presence of composite metals, due to the formation of redox pairs, introducing an enhancement in electron transfer and an acceleration of redox reactions. This signified that the SED system shows superior performance. To further clarify the impact of reaction time on the removal rate, time gradient experiments were carried out with 0.1 g of CIDS@CFO@F0C0 (SED-0.1 system), and the results are shown in Fig. 2b. At the early stage, the removal rate of E. coli rose quickly with time and reached 93% in a 30 min reaction. The existing E. coli could be totally removed within two hours, which confirms the promise of the SED system in practical utilization.
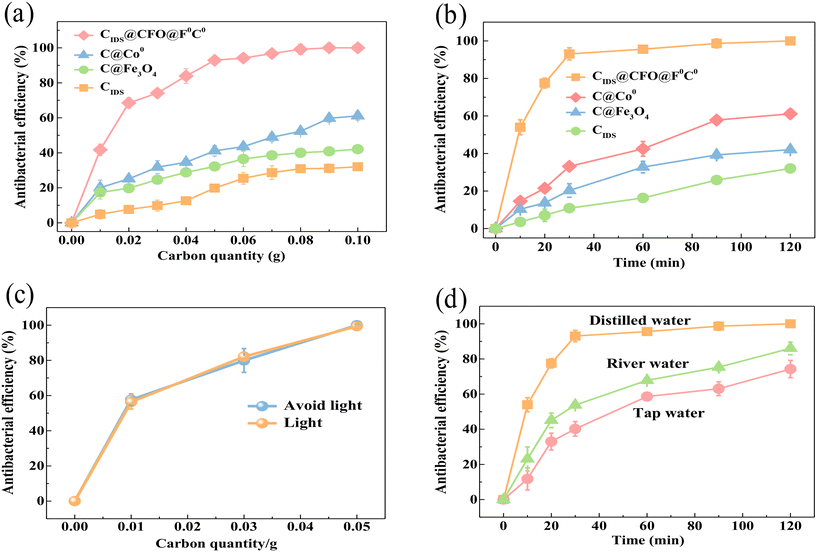 |
| Fig. 2 (a) Influence of different dosages on bactericidal effect, (b) influence of bactericidal agent on bactericidal effect, and comparison of bactericidal effects of the SED-0.1 system under (c) light and dark conditions and (d) different water environments. | |
Furthermore, the electron transfer capability of the SED system was further tested by electrochemical impedance spectroscopy (EIS). The arc radius of the EIS spectrum (Fig. S4†) reflects the magnitude of charge mass transfer resistance, while the linear relation in the spectrum reflects the ion diffusion rate and diffusion resistance of the SED system. The order of current of CIDS@CFO@F0C0 > C@Co0 > C@Fe3O4 suggested that the electron transfer rate of CIDS@CFO@F0C0 was higher than that of C@Co0 or C@Fe3O4. This result demonstrates the excellent electron transport capability of the SED system, which facilitates the efficient generation of ROS. To visually detect the bactericidal effect of the SED system, colony counting evaluation on an agar plate as well as integrity measurement through fluorescein diacetate (FDA) staining of E. coli were performed. For comparison, an E. coli group lacking the antiseptic catalyst was used as a control. In Fig. S5,† the E. coli group grew well without the presence of the catalyst and gave a remarkable FDA staining signal, indicating the strong living and reproductive ability of this kind of bacteria. After the addition of the catalyst, the colony numbers sharply decreased and the FDA signal significantly weakened, clearly presenting the inactivation capability of the SED system and that E. coli will be killed by destroying the integrity of the cell. In addition to E. coli, which represents Gram-negative bacteria, bactericidal experiments with Gram-positive bacteria were also carried out. Staphylococcus aureus, as a common foodborne pathogenic microorganism, is a typical Gram-positive bacterium. According to the results of Fig. S6,† it was found that 100% inactivation of Staphylococcus aureus could be achieved within 2 h under the SED system. This proved that the SED system shows broad spectrum bactericidal activity.
Some studies had reported that the removal of bacteria by transition metals acted through photocatalysis to generate ROS.47 Thus, inactivation experiments were performed with and without light to examine whether the aseptic efficiency of the SED system was associated with light. The bactericidal efficiencies were 56.32% and 57.64% under light (30 W, 450–460 nm) and lightless conditions, respectively, with the SED-0.1 system. When the dosage of the catalyst increased to 0.05 g, the inactivation efficiencies of this material with or without light were both close to 100% (Fig. 2c). No obvious removal discrepancy was detected in the two different conditions, suggesting that there were no photocatalytic reactions, so the use of the SED system was not restricted by light. Experiments were conducted in different water environments (distilled water, tap water and river water) to explore the effect of water. Compared with distilled water (Fig. 2d), the inactivation effects in tap water and river water were affected but remained around 80%. The reason for this should be that those types of water usually contain a lot of impurities that affect the formation of ROS and inactivation.
Ferrite and cobalt materials usually possess magnetic properties, which would benefit their recollection and reusability. A VSM test of CIDS@CFO@F0C0 exhibited an obvious magnetic hysteresis curve at 300 K, suggesting typical superparamagnetic behavior of the SED system at room temperature. The magnetism of CIDS@CFO@F0C0 was close to 34.8 emu g−1 (Fig. S7,† orange line), which is strong enough to guarantee its collection with magnets. It is worth mentioning that the killed bacteria did not weaken the magnetic property of the SED system (Fig. S7,† blue line), indicating the integrity and thus the stability of the material during the inactivation process. Cyclic experiment results confirmed this conclusion. As shown in Fig. 3a, after 11 cycles of inactivation, the inactivation effect of the SED system on E. coli did not obviously change. At the same time, the amounts of iron and cobalt ions dissolved in the system were investigated (Fig. 3b), which were much lower than the international standard (South African Water Quality Guidelines volume 4: Agricultural Water Use: Irrigation second edition, 1996). The crystal structure of the recycled CIDS@CFO@F0C0 was also explored. No significant difference was found either in peak position or in intensity in the XRD pattern after inactivation (blue line) in Fig. S1a.† The above circumstances prove that Fe0Co0 and CoFe2O4 particles were still present in the hybrid catalyst after removal of bacteria, and the SED system was quite stable and could be reused several times. On account of its high ROS catalytic performance, remarkable stability and reusability, along with excellent ambient safety, the SED system has outstanding application potential in practical water treatment.
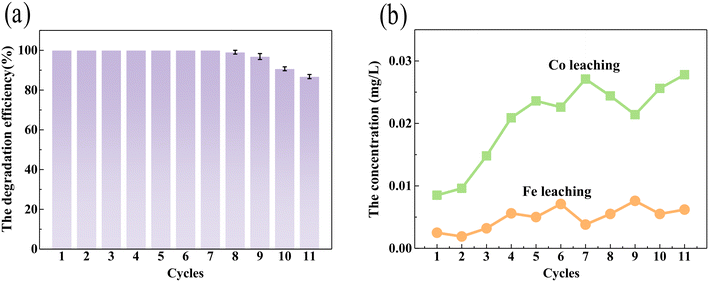 |
| Fig. 3 (a) The SED-0.1 system repeated 11 times for the inactivation effect, and (b) Fe and Co leaching from the SED system over 11 cycles. | |
3.3. The bactericidal mechanism of the SED system
The species of ROS produced with the SED system were then determined by EPR analysis. As shown in Fig. 4a and c, EPR spectra with DMPO as a spin-trapping agent gave strong signals of ·OH, ·O2− and 1O2, which gradually strengthened over time. This detection indicates that these three kinds of ROS were continuously generated in the system. Obviously, the signal strength of DMPO–·OH was higher than that of DMPO–·O2− or TEMP–1O2, suggesting that more ·OH was produced in the SED system. It has been reported that oxygen can accept another electron and then transform into ·O2− (eqn (7)), which is very energetic and can react with water to generate H2O2 and 1O2 (eqn (8)). Hydrogen peroxide is a typical oxidant that can capture electrons and release hydroxyl radicals (eqn (9)). Therefore, O2 should be the critical raw reactant for the formation of the two radicals. To clarify this speculation, oxygen-isolating EPR was further carried out. As shown in Fig. 4b, the peak intensities of ·O2− and ·OH decreased significantly compared to the oxygen-unsequestered EPR, which confirmed the necessity of O2 for the catalyzation and generation of both ·O2− and ·OH free radicals. An inactivation experiment under anaerobic conditions was then carried out to verify the effect of oxygen. As shown in Fig. S8,† there was no inactivation effect in this situation, which also confirmed the significance of oxygen for the formation of free radicals in the system. A potassium permanganate titration experiment was also performed to see whether hydrogen peroxide had been formed during the catalyzation procedure in the SED system (Fig. 4d). During the first 4 minutes, there was no obvious detection of hydrogen peroxide, which should be attributed to the low content of ·O2− within such a short reaction time (DMPO–·O2− 3 min, Fig. 4a). However, the concentration of H2O2 increased rapidly at the 5th minute and reached 25 mmol L−1 within 20 min. Accordingly, the peak intensities of both ·O2−, ·OH and 1O2 increased after 6 min of reaction, as observed in the EPR spectra. This result confirms that the SED system could oxidize O2 and H2O for the generation of ·O2−, ·OH and 1O2. | 2·O2− + 2H2O → H2O2 + 1O2 + 2OH− | (8) |
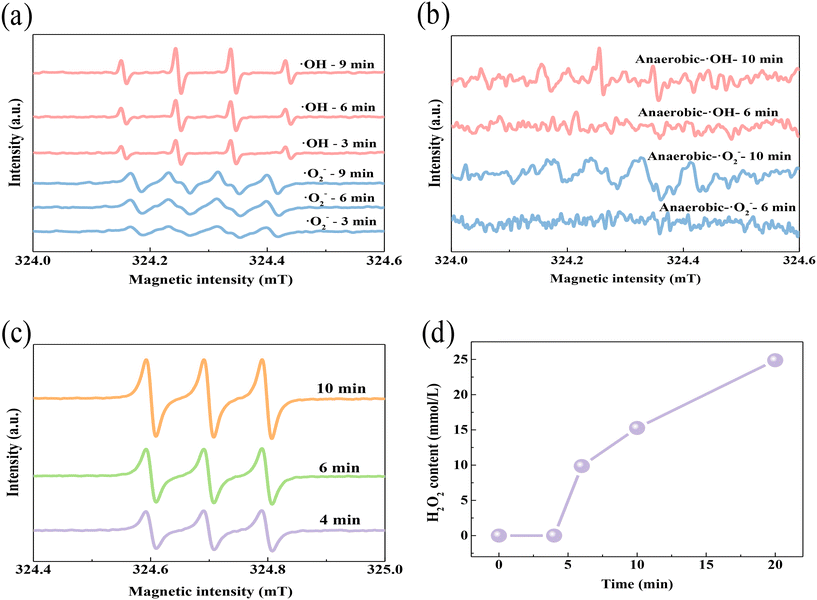 |
| Fig. 4 EPR spectra of ·OH and ·O2− in the SED system under (a) aerobic conditions and (b) anaerobic conditions, (c) EPR spectra of 1O2 and (d) detection of H2O2 generation in the SED system. | |
To visually compare the difference in ROS generation between single-metal catalysis and bimetal catalysis, ROS generation was examined for CIDS, C@Co0 and C@Fe3O4 (Fig. S9, S10 and S11,† respectively). The results of EPR showed that the single-metal catalyst could catalyze the generation of ROS, but the catalytic effect was inferior to the bimetal catalyst. While CIDS had no ability to catalyze hyperoxygen ROS and the catalytic generation of hydroxyl radicals was also less. This signifies that the SED system showed better performance for ROS stimulation, and the main catalytically active components in the SED system will be low-valence metals (Fe0Co0 and CoFe2O4).
By analyzing the bactericidal effect of different amounts of catalyst (Fig. 5a), it was found that SED systems with 0.003 g, 0.005 g and 0.01 g (SED-0.003 system, SED-0.005 system and SED-0.01 system) showed a trend of promoting the growth of E. coli in the early stage, and then had a bactericidal effect. The promotion trend also followed the sequence SED-0.003 system > SED-0.005 system > SED-0.01 system. Since the quantity of free radicals might be regulated by the amount of electrons, in order to find out whether the phenomenon is related to the content of ROS, the concentration of ·O2− and ·OH free radicals during the procedure was determined with a specific hydroxyl radical kit and a superoxide radical kit, respectively (Fig. 5b and c). For the SED-0.003 system and SED-0.005 system with lower material weight, the activity of the bacteria was promoted within 20 min and 30 min, respectively, which is consistent with the conclusion that certain amounts of free radicals are needed for the microorganisms to live. As time went on, the density of the free radicals kept increasing, causing fatal effects to the bacteria. As shown in Fig. 5b and c, the results showed that the concentration of ·O2− was larger in the SED-0.003 system than that of the SED-0.005 system, while the concentration of ·OH showed the totally opposite situation. It can be easily found that the concentration trend of hydroxyl radicals was consistent with the trend of inactivation, suggesting the key contribution of this radical. To better clarify the role of various ROS in sterilization, quenching experiments were performed (Fig. 5d). Quenching ·OH and 1O2 affected part of the bactericidal performance, while quenching three radicals resulted in no killing effect of the E. coli. It was inferred that ·OH accounted for 59.94% and 1O2 accounted for 33.94% of the sterilization. This result indicates that ·OH and 1O2 were the main inactivating ROS in the SED system, and ·O2− mainly played a role in the formation of ·OH and 1O2 (eqn (7)–(9)).
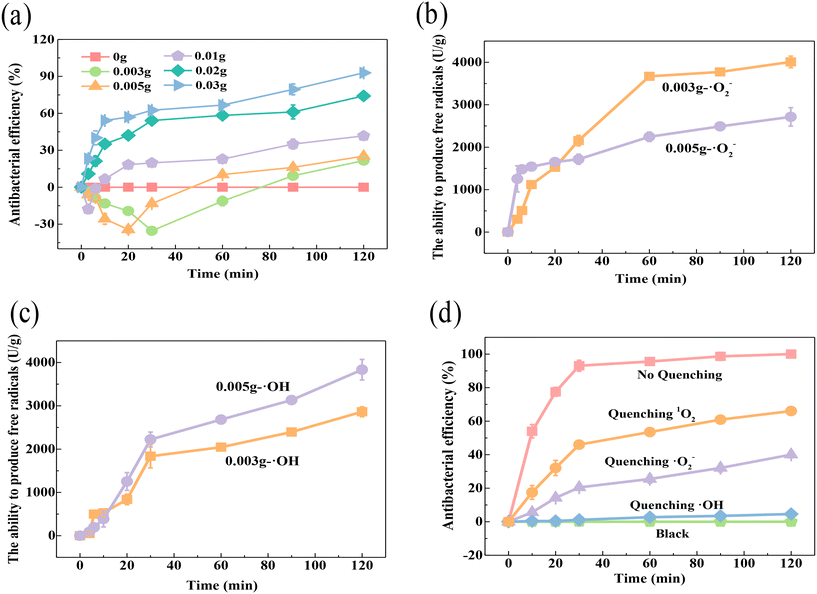 |
| Fig. 5 (a) Effect of ROS content on bactericidal activity, determination of (b) ·O2− and (c) ·OH in SED systems with 0.003 g and 0.005 g catalysts, and (d) quenching experiments for different ROS. | |
In order to gain insight into the reasons for the inactivation performance of the SED system, some microbial detection experiments including Na+K+-ATPase, superoxide dismutase (SOD), malondialdehyde (MDA) and catalase (CAT) were carried out (Fig. 6a–d). The activity of Na+K+-ATPase can embody the integrality of the microbial cell membrane. The level of SOD is able to present the ability of microorganisms to scavenge oxygen ROS. And the main role of CAT is to catalyze the decomposition of H2O2, the product of SOD, into H2O and O2. Fig. 6a, b and d demonstrate that the activity of Na+K+-ATPase, SOD and CAT all dropped significantly after inactivation, and showed a downward trend with an increase in catalyst dosage in the SED system. This phenomenon indicates that the membrane of the cell was gradually destroyed by the SED system and its repellence to the oxygen ROS was degraded. MDA content can tell the rate as well as the extent of lipid peroxidation, and can also be used to image the degree of peroxidative damage. An obvious rise in MDA content was observed in the presence of the catalyst, and it showed gradual growth along with an increase in catalyst quantity (Fig. 6c). The above testing results suggest that oxygen ROS were produced in the SED system, which could peroxidize the lipids inside the cells, resulting in oxidative damage and killing E. coli.
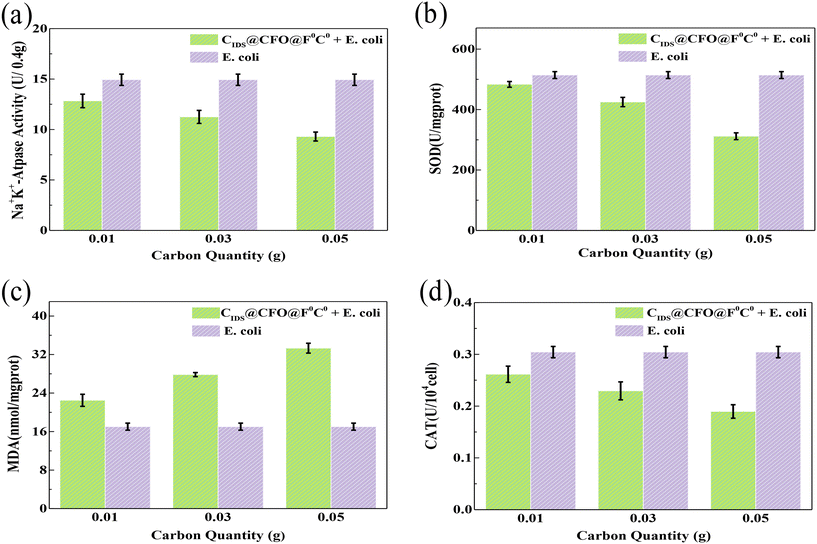 |
| Fig. 6 (a) Na+K+-ATPase activity, (b) the content of MDA, (c) SOD activity and (d) CAT activity of the SED system. | |
To better determine the role of transition metals during the inactivation process, XPS spectra of CIDS@CFO@F0C0 after inactivation were examined. After one cycle of inactivation, on the basis of Co 2p signal deconvolution (Fig. 2c and 7c), a decrease of 40.72% in Co0 content and increases of 21.87% in Co2+ and 18.85% in Co3+ were detected. In a similar manner, the XPS spectra of Fe 2p (Fig. 2b and 7b) indicate the changes in Fe0, Fe2+ and Fe3+ content from 8.73%, 37.57% and 53.70% to 2.40%, 39.99% and 57.61%, respectively. In the process of ROS formation, the lower state Fe0/Co0 and Fe2+/Co2+ tended to switch to higher state Fe3+/Co3+ to provide electrons. Meanwhile, Fe3+/Co3+ would be reduced to lower valence ions Fe2+/Co2+ due to the electron contribution of zero-valence metal elements Fe0/Co0. The XPS results of the system after 6 cycles of inactivation also provide further proof of the above conclusion. As the bactericidal reaction continued, the iron and cobalt changed from zero-valent and bivalent to trivalent (Fig. 7d–f). Thus, the proportions of Fe2+/Co2+ and Fe3+/Co3+ increased, while the proportion of Fe0/Co0 decreased. Notably, the redox reactions could occur not only between the same kinds of metal, such as Fe0/Fe3+ and Co0/Co3+ pairs, but also between different metals like Fe0/Co3+ and Co0/Fe3+ pairs. This formation of various redox couples, thanks to the CoFe2O4 combined Fe–Co alloy structure, could significantly accelerate the redox reactions and result in the generation of a quantity of ROS. And the above results imply that the inactivation of E. coli by the SED system was accomplished through the bicatalyzed performance of Fe and Co.
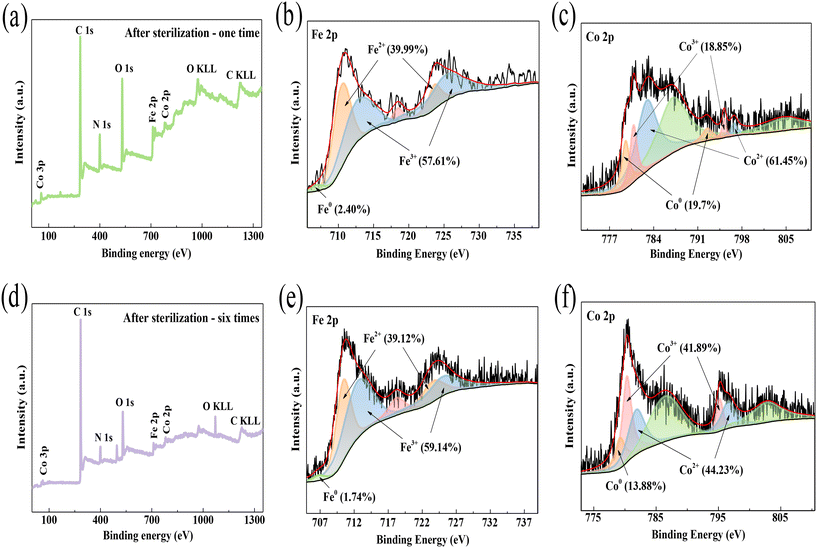 |
| Fig. 7 XPS of CIDS@CFO@F0C0 after (a–c) one and (d–f) six cycles of inactivation. | |
According to the above test results, it is speculated that the inactivation mechanism of the SED system should be as follows (Fig. 8): being prominent electronic donors, the existing Fe0/Co0 and Fe2+/Co2+ are able to participate in single-electron transfer reactions (eqn (10) and (11)), which can oxidize H2O and O2 to form ·OH, ·O2− and 1O2 (eqn (7)–(9)). Then, Co3+ and Fe3+ can be reduced to Co2+ and Fe2+ by the electrons generated between Fe0/Fe2+/Fe3+ and Co0/Co2+/Co3+, since various redox pairs can be generated between the same and different metals (eqn (12)–(16)). The as-obtained Co2+ and Fe2+ can be used to continuously generate ROS by catalytic performance. The attack of ROS on the cells starts from the cell membrane structure. More importantly, the ROS can damage the structure of the proteins and produce aberrations that throw the entire microbial system into disarray. In addition, ROS attack on cells is nonspecific, so the SED system can exhibit broad spectrum activity.
| Fe0/Co0 → Fe2+/Co2+ + 2e− | (10) |
| Fe2+/Co2+ → Fe3+/Co3+ + e− | (11) |
| Fe0 + Co3+ → Fe2+ + Co2+ | (12) |
| Fe2+ + Co3+ → Fe3+ + Co2+ | (13) |
| 2Fe3+ + Co0 → 2Fe2+ + Co2+ | (14) |
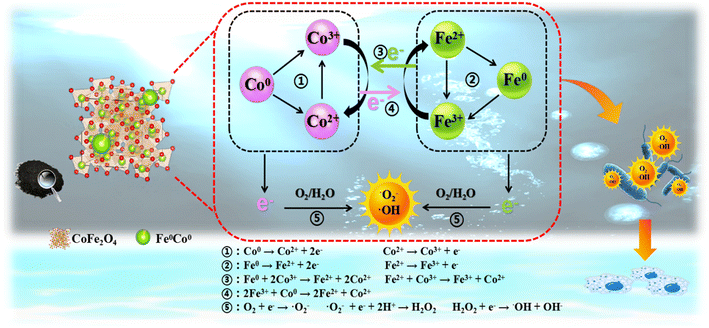 |
| Fig. 8 Schematic diagram of mechanism of killing E. coli by the SED system. | |
4. Conclusions
In summary, SED systems containing a stable Fe–Co alloy and CoFe2O4 have been prepared by a one-step method for inactivating E. coli. The research data showed that the formation of Fe0/Fe2+/Fe3+ and Co0/Co2+/Co3+ in the SED system is of great significance: firstly, it helps the oxidization of O2/H2O to release ·OH, ·O2− and 1O2 through a bicatalytic effect; secondly, redox pairs are formed between iron and cobalt to complete the construction of a sufficient electron-donating system. The system can provide enough electrons to effectively trigger the reduction of O2 to produce ·O2− without any additional energy or the help of other active oxidants. Then, 1O2, H2O2 and ·OH are generated due to the formation of ·O2−. Through analysis of the EPR spectra, ROS concentration and quenching experiments, it was found that ·OH and 1O2 played a dominant role in the inactivation process, and ·O2− mainly plays a role in promoting the formation of ·OH and 1O2. By exploring the influencing factors, CIDS@CFO@F0C0 dosage and aquatic environment in the SED system had a great effect on killing E. coli. In contrast, light conditions had no effect on the reaction. Optimization of reaction parameters demonstrated that the SED system could completely remove E. coli in 2 hours. With outstanding stability and environmental friendliness, the system can maintain more than 85% inactivation efficiency after 11 cycles of sterilization, and the amount of metal dissolution is small. Overall, the findings here construct a sufficient electron-donating system for the sustainable generation of ROS, providing new ideas for the development of bacteria removal in the future.
Conflicts of interest
The authors declare no competing financial interest.
Acknowledgements
The authors would like to show great acknowledgements for the financial support from Hunan Engineering Research Center for Biochar, Hunan High Level Talent Gathering Project (2020RC3051 & 2021RC5006 & 2020RC5007), Key R & D projects in Hunan Province (2020SK2032 & 2021SK2047 & 2020WK2016), Hunan Optical Agriculture Engineering Technology Research Center (2018TP2003), National Natural Science Foundation of China (51974123), The Distinguished Youth Foundation of Hunan Province (2020JJ2018 & 2022JJ10030), The Scientific Research Fund of Hunan Provincial Education Department (19C0903), Hunan Provincial Key Laboratory of Crop Germplasm Innovation and Resource Utilization Science Foundation (19KFXM12), and Hunan Provincial Natural Science Foundation Youth Fund (2021JJ40261).
References
- M. Song, Y. Liu, X. Huang, S. Ding and K. Zhu, A broad-spectrum antibiotic adjuvant reverses multidrug-resistant Gram-negative pathogens, Nat. Microbiol., 2020, 5, 1–11 CrossRef PubMed.
- N. Channa, T. A. Gadhi, R. B. Mahar, A. Chiadò and A. Tagliaferro, Combined photocatalytic degradation of pollutants and inactivation of waterborne pathogens using Solar Light Active α/β-Bi2O3, Colloids Surf., A, 2021, 615, 126214 CrossRef CAS.
- H. Shi, C. Wang, Y. Zhao, E. Liu and Z. Ji, Highly efficient visible light driven photocatalytic inactivation of E. coli with Ag QDs decorated Z-scheme Bi2S3/SnIn4S8 composite, Appl. Catal., B, 2019, 254, 403–413 CrossRef CAS.
- L. Feng, H. Wu, H. Yue, Y. Chu, J. Zhang, X. Huang, S. Pang, L. Zhang, Y. Li and W. Wang, Multiplexed and Rapid AST for Escherichia coli Infection by Simultaneously Pyrosequencing Multiple Barcodes Each Specific to an Antibiotic Exposed to a Sample, Anal. Chem., 2022, 94, 8633–8641 CrossRef CAS PubMed.
- I. Adeniyi, Factor effects and interactions in steam reforming of biomass bio-oil, Chem. Zvesti, 2020, 74, 1459–1470 CrossRef.
- P. L. Lam, S. M. Wong, K. H. Lam, L. K. Hung, M. M. Wong, L. H. Yung, Y. W. Ho, W. Y. Wong, K. P. Hau and R. Gambari, The role of reactive oxygen species in the biological activity of antimicrobial agents: An updated mini review, Chem.-Biol. Interact., 2020, 320, 109023 CrossRef CAS PubMed.
- J. Xu, Z. Liu and J. Dai, Environmental and economic trade-off-based approaches towards urban household waste and crop straw disposal for biogas power generation project -a case study from China, J. Cleaner Prod., 2021, 319, 128620 CrossRef.
- Q. Gao, Z. Wang, Y. Rao, Y. Zhao, J. Cao, K. F. Ho, Y. Zhai, M. Xiong, J. Li and Y. Huang, Oxygen vacancy mediated alpha-MoO(3) bactericidal nanocatalyst in the dark: Surface structure dependent superoxide generation and antibacterial mechanisms, J. Hazard. Mater., 2023, 443, 130275 CrossRef CAS PubMed.
- Y. Ahmed, J. Zhong, Z. Yuan and J. Guo, Simultaneous removal of antibiotic resistant bacteria, antibiotic resistance genes, and micropollutants by a modified photo-Fenton process, Water Res., 2021, 197, 117075 CrossRef CAS PubMed.
- M. H. Guerra, I. O. Alberola, S. M. Rodriguez, A. A. Lopez, A. A. Merino, E. C. Lopera and J. Q. Alonso, Oxidation mechanisms of amoxicillin and paracetamol in the photo-Fenton solar process, Water Res., 2019, 156, 232–240 CrossRef PubMed.
- M. Sun, Y. Zhang, S. Y. Kong, L. F. Zhai and S. Wang, Excellent performance of electro-assisted catalytic wet air oxidation of refractory organic pollutants, Water Res., 2019, 158, 313–321 CrossRef CAS PubMed.
- P. Hu, T. Wu, W. Fan, L. Chen, Y. Liu, D. Ni, W. Bu and J. Shi, Near infrared-assisted Fenton reaction for tumor-specific and mitochondrial DNA-targeted photochemotherapy, Biomaterials, 2017, 141, 86–95 CrossRef CAS PubMed.
- Q. Dong, J. Wang, X. Duan, X. Tan, S. Liu and S. Wang, Self-assembly of 3D MnO2/N-doped graphene hybrid aerogel for catalytic degradation of water pollutants: Structure-dependent activity, Chem. Eng. J., 2019, 369, 1049–1058 CrossRef CAS.
- B. Nsa, B. Cra, A. Xp, B. Pkjra, C. Mcab and C. Mcb, Photocatalytic radical species: An overview of how they are generated, detected, and measured, Nanostruct. Photocatal., 2021, 85–118 Search PubMed.
- Y. Liu, Q. Wang, Y. Fu, X. Lu and X. Bai, The self-catalysis of ferrate (VI) by its reactive byproducts or reductive substances for the degradation of diclofenac: Kinetics, mechanism and transformation products, Sep. Purif. Technol., 2018, 192, 412–418 CrossRef.
- M. Tian, G. Chen and J. Xu, Epigallocatechin gallate-based nanoparticles with reactive oxygen species scavenging property for effective chronic periodontitis treatment, Chem. Eng. J., 2022, 433, 132197 CrossRef CAS.
- N. Zheng, X. He, R. Hu, R. Wang, Q. Zhou, Y. Lian and Z. Hu, In-situ production of singlet oxygen by dioxygen activation on iron phosphide for advanced oxidation processes, Appl. Catal., B, 2022, 307, 121157 CrossRef CAS.
- D. Zhu, W. Chen, W. Lin, Y. Li and X. Liu, Reactive oxygen species-responsive nanoplatforms for nucleic acid-based gene therapy of cancer and inflammatory diseases, Biomed. Mater., 2021, 16(4), 042015 CrossRef CAS PubMed.
- S. Peng, R. Li and Y. Rao, Tuning the unsaturated iron sites in MIL-101(Fe) nanoparticles for reactive oxygen species-mediated bacterial inactivation in the dark, Appl. Catal., B, 2022, 316, 121693 CrossRef CAS.
- H. Wang, J. Cai, Z. Liao, A. Jawad, J. Ifthikar, Z. Chen and Z. Chen, Black liquor as biomass feedstock to prepare zero-valent iron embedded biochar with red mud for Cr(VI) removal: Mechanisms insights and engineering practicality, Sens. Actuators, B, 2020, 311, 123553 CAS.
- Y. Tan, S. Zheng, Y. Di, C. Li, R. Bian and Z. Sun, Diatomite supported nano zero valent iron with 3D network for peroxymonosulfate activation in efficient degradation of bisphenol A, J. Mater. Sci. Technol., 2021, 95, 57–69 CrossRef CAS.
- R. Zhou, X. R. You and C. G. Zhang, Co(II) metal–organic framework for photocatalytic degradation of organic dyes and application values on neonatal gastrointestinal malformations by blocking cholinergic receptor combined with dexmedetomidine, Chem. Zvesti, 2021, 75, 2173–2179 CrossRef CAS.
- D. A. Widyaningrum, A. Rinanti and R. Hadisoebroto, The kinetics of Fe2+ heavy metal adsorption by microalgae Desmodesmus sp. beads, IOP Conf. Ser. Earth Environ. Sci., 2021, 894, 012040 CrossRef.
- Z. Jia, Y. Zeng, P. Tang, D. Gan, W. Xing, Y. Hou, K. Wang, C. Xie and X. Lu, Conductive, Tough, Transparent, and Self-Healing Hydrogels Based on Catechol–Metal Ion Dual Self-Catalysis, Chem. Mater., 2019, 31, 5625–5632 CrossRef CAS.
- M. Gimferrer, S. Danes, D. M. Andrada and P. Salvador, Unveiling the Electronic Structure of the Bi(+1)/Bi(+3) Redox Couple on NCN and NNN Pincer Complexes, Inorg. Chem., 2021, 60(23), 17657–17668 CrossRef CAS PubMed.
- H. Sun, X. Yang, L. Zhao, T. Xu and J. Lian, One-pot hydrothermal synthesis of octahedral CoFe/CoFe2O4 submicron composite as heterogeneous catalysts with enhanced peroxymonosulfate activity, J. Mater. Chem. A, 2016, 4, 9455–9465 RSC.
- Y. Zhou, Y. Zhang and X. Hu, Synergistic coupling Co3Fe7 alloy and CoFe2O4 spinel for highly efficient removal of 2,4-dichlorophenol by activating peroxymonosulfate, Chemosphere, 2020, 242, 125244 CrossRef CAS PubMed.
- H. Niu, D. He, Y. Yang, H. Lv and Y. Liang, Long-lasting activity of Fe0-C internal microelectrolysis-Fenton system assisted by Fe@C-montmorillonites nanocomposites, Appl. Catal., B, 2019, 256, 117820 CrossRef CAS.
- C. Cai, S. Kang, X. Xie, C. Liao and D. D. Dionysiou, Efficient degradation of bisphenol A in water by heterogeneous activation of peroxymonosulfate using highly active cobalt ferrite nanoparticles, J. Hazard. Mater., 2020, 399, 122979 CrossRef CAS PubMed.
- A. Rmb, A. Ii, A. Gg, C. Xtbb and D. Ap, Cobalt ferrite nanoparticles and peroxymonosulfate system for the removal of ampicillin from aqueous solution, J. Water Process. Eng., 2020, 40, 101823 Search PubMed.
- J. Zeng, Z. Guo, Y. Wang, Z. Qin, Y. Ma, H. Jiang, Y. Weizmann and X. Wang, Intelligent bio-assembly imaging-guided platform for real-time bacteria sterilizing and infectious therapy, Nano Res., 2022, 15, 4164–4174 CrossRef CAS.
- Z. Liu, B. Jia, Y. Zhang and M. Haneda, Engineering the Metal-Support Interaction on Pt/TiO2 Catalyst to Boost the H-2-SCR of NOx, Ind. Eng. Chem. Res., 2020, 59(31), 13916–13922 CrossRef CAS.
- Y. Lin, J. Li, Z. Xiong, K. Shi and Q. Liu, Carbon-Based Conductive Frameworks and Metal Catalytic Sites Derived from Cross-Linked Porous Porphyrin-Based Polyimides for Enhanced Conversion of Lithium Polysulfides in Li–S Batteries, ACS Appl. Energy Mater., 2021, 4(12), 14497–14507 CrossRef CAS.
- G. J. Kontoghiorghes, Advances on Chelation and Chelator Metal Complexes in Medicine, Int. J. Mol. Sci., 2020, 21(7), 2499 CrossRef PubMed.
- L. Su, L. Ou, Y. Wen, Y. Wang, W. Zhao, Z. Zhou, M.-e. Zhong, Y. Zhu and N. Zhou, High-efficiency degradation of quinclorac via peroxymonosulfate activated by N-doped CoFe2O4/Fe0@CEDTA hybrid catalyst, J. Ind. Eng. Chem., 2021, 102, 177–185 CrossRef CAS.
- X. Wang, L. Kong, Y. Gan, T. Liang and P. Wang, Microfluidic-based fluorescent electronic eye with CdTe/CdS core-shell quantum dots for trace detection of cadmium ions, Anal. Chim. Acta, 2020, 1131, 126–135 CrossRef CAS PubMed.
- F. Machado, J. M. Soares, O. L. A. Conceicao, E. S. Choi and L. Balicas, Magnetic properties of the nanocomposite CoFe2O4/FeCo-FeO at a high H/T regime, J. Magn. Magn. Mater., 2017, 424, 323–326 CrossRef CAS.
- B. Ntha, C. Nxt, C. Vtko, D. Pvh, E. Nxc, F. Htv and C. Nvv, Preparation of exchange coupled CoFe 2 O 4/CoFe 2 nanopowders, J. Magn. Magn. Mater., 2020, 511, 166984 CrossRef.
- Y. Fujishiro, M. Kawahara, T. Samura, T. Tachiki and T. Uchida, Investigation of fabrication conditions for VO2 thin films by MOD using carbon thermal reduction, Electron. Commun. Jpn., 2021, 104(2), e12306 CrossRef.
- T. H. Lim, S. J. Cho, H. S. Yang, M. H. Engelhard and D. H. Kim, Effect of Co/Ni ratios in cobalt nickel mixed oxide catalysts on methane combustion, Appl. Catal., A, 2015, 505, 62–69 CrossRef CAS.
- J. Xiong, J. Yang, X. Chi, K. Wu, L. Song, T. Li, Y. Zhao, H. Huang, P. Chen, J. Wu, L. Chen, M. Fu and D. Ye, Pd-Promoted Co2NiO4 with lattice Co O Ni and interfacial Pd O activation for highly efficient methane oxidation, Appl. Catal., B, 2021, 292, 120201 CrossRef CAS.
- Z. Duan, N. Wang, H. Xu and P. Wu, Structural Transformation-Involved Synthesis of Nanosized ERI-Type Zeolite and Its Catalytic Property in the MTO Reaction, Inorg. Chem., 2022, 61(20), 8066–8075 CrossRef CAS PubMed.
- Y. Niu, X. Huang, L. Zhao, W. Hu and C. M. Li, One-Pot Synthesis of Co/CoFe2O4 Nanoparticles Supported on N-Doped Graphene for Efficient Bifunctional Oxygen Electrocatalysis, ACS Sustainable Chem. Eng., 2018, 6(3), 3556–3564 CrossRef CAS.
- X. Jia, Y. Zhang, L. Zhang, L. Wang and L. Zhou, Fabrication and bifunctional electrocatalytic performance of FeNi 3/MnFe 2 O 4/nitrogen-doping reduced graphene oxide nanocomposite for oxygen electrocatalytic reactions, Ionics, 2020, 26, 991–1001 CrossRef.
- F. Xiao, W. Li, L. Fang and D. Wang, Synthesis of akageneite (beta-FeOOH)/reduced graphene oxide nanocomposites for oxidative decomposition of 2-chlorophenol by Fenton-like reaction, J. Hazard. Mater., 2016, 308, 11–20 CrossRef CAS PubMed.
- J. Zhou, H. Song, L. Ma and X. Chen, Magnetite/graphene nanosheet composites: interfacial interaction and its impact on the durable high-rate performance in lithium-ion batteries, RSC Adv., 2011, 1, 782–791 RSC.
- H. Yan, R. Wang, R. X. Liu, T. Xu and J. Wang, Recyclable and Reusable Direct Z-scheme Heterojunction CeO2/TiO2 Nanotube Arrays for Photocatalytic Water Disinfection, Appl. Catal., B, 2021, 291, 120096 CrossRef CAS.
|
This journal is © The Royal Society of Chemistry 2024 |
Click here to see how this site uses Cookies. View our privacy policy here.