Exploring the applications and carbon reduction of multi-technology-coupled membrane biofilm reactors for sustainable wastewater treatment: a review
Received
16th December 2024
, Accepted 7th February 2025
First published on 21st February 2025
Abstract
Membrane biofilm reactors (MBfRs), which efficiently remove pollutants and reduce carbon emissions, hold great promise for wastewater treatment. However, the lack of a cheap local supply of hydrogen, uncontrolled substrate competition, and other issues pose challenges on the long-term stability of these reactors. At the same time, membrane bioreactors have strong bonding capabilities and can be coupled with a variety of processes. Therefore, these reactors are coupled with metal catalysts, electrochemistry, or anaerobic ammonia oxidation (anammox) technology to overcome these challenges. Metal catalysts reduces the replacement cycle and improves the operation stability of MBfRs, while electrochemistry removes pollutants in situ along with providing sufficient hydrogen. When coupled with anammox, the performance of the reactor improves and the energy consumption reduces. In this review, coupling of the hydrogen-based membrane biofilm reactor with the above technologies is discussed in view of their practical applications. Furthermore, their working principles, carbon emission reduction and applications are analyzed. Based on these, practical application and carbon emission reduction of membrane biofilm reactors are discussed along with providing ideas on overcoming their limitations.
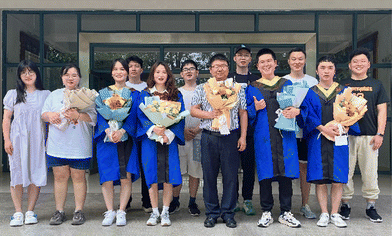 From left to right are Meng Zhang, Yu Han, Yurong Fan, Yukun Tang, Wanying Ding, Yi Xie, Haixiang Li, Qinzhen Wei, Yi Zhang, Ruize Sun, Xing Hu, Chang Mei | Professor Li Haixiang is a Doctor and CPC member born in Jingzhou, Hubei Province in 1984. He is a Doctoral Supervisor, Senior member of Water Environment and Water Ecology Branch of China Urban Science Research Association, Member of Water Supply and Drainage Professional Committee of Guangxi Civil Architecture Society, Expert of Guangxi Zhuang Autonomous Region Environmental Protection Industry Association, Member of International Water Association (IWA), Database Expert of Urban Water Resources Development and Utilization (South) National Engineering Research Center, and the Secretary of China Engineering Education Certification Field Inspection Expert Group. He has completed 11 national and provincial scientific research projects, including 1 surface project and 1 Jieqing project in Guangxi. He has published more than 50 scientific research papers in high-level academic journals, such as Water Research, Science of the Total Environment and Journal of Hazardous Materials, among which 30 have been included by SCI/EI. He has published 2 monographs and participated in the preparation of 3 standards, and he also has 7 authorized patents. He has won second prize in the Guangxi Science and Technology Progress Award, first prize in the Innovation Award of the China Invention Association, first prize in the Environmental Protection Science and Technology Award, and 3 first prizes in the Autonomous Region teaching achievements. |
Water impact
MBfR coupled with palladium nanoparticles, MEC and H2-PDA forms new processes. It improves the efficiency of wastewater treatment and reduces carbon emissions. It has shown remarkable results in reducing energy consumption and pollution.
|
1. Introduction
Growth in industrialization has led to a significant rise in the discharge of industrial wastewater,1 which contains harmful substances that pose a threat to both the environment and human health.2 Additionally, the rapidly growing population in China has resulted in a higher volume of urban sewage, putting more pressure on urban sewage treatment systems.3 Consequently, sewage discharge standards in cities are becoming more stringent.4 Therefore, there is an urgent need to develop cost-effective and efficient sewage treatment technologies to address these issues.
In response to these challenges, a modified version of MBR (membrane bio-reactor), which is called as MBfR, was developed. MBfR features an internal microporous hollow fiber membrane module fixed at both ends of the reactor, with a pressurized clean energy source (H2) passing through the inside.5 Unlike in traditional MBR, in MBfR, the biofilm is attached to the outer surface of the membrane module, and the hollow fiber membrane serves as a microporous aeration system.6,7 Hydrogen is diffused from the inner surface of the membrane to the outer surface by applying pressure, thereby allowing the bacteria to utilize it in its molecular form with a utilization rate of up to 100%.8 Notably, the structure of MBfR enables uniform diffusion of hydrogen through the membrane pores, enriching the biofilm on the membrane module with hydrogen autotrophs that help in reducing and removing pollutants.9,10 This process is highly efficient, clean, non-toxic, and does not cause secondary pollution.9 By enhancing the utilization rate and safety of hydrogen, MBfR significantly decreases the cost of treatment compared to other electron donors.6 The unique mass transfer mode of MBfR results in excellent biofilm structure and properties, with a large number of hydrogen autotrophic microorganisms enriched on the membrane component,11 improving the pollutant removal efficiency.
Even though significant academic research has been conducted in this area, MBfR is still in an early stage of development.12 This is because of the problems such as matrix competition and fluctuation of biofilm treatment efficiency. Hence, this review explores the application and development of the reactor coupled with different technologies.
To further enhance the performance of MBfR, we have taken several innovative approaches.13 First, we introduced biological palladium nanoparticles to optimize the original biofilm. These nanoparticles not only provide a new route for electron transfer but also act as highly efficient conductive materials, significantly promoting the rapid transfer of electrons within the biofilm, thereby increasing the reaction rate and efficiency.14
Secondly, we combined the MEC (microbial electrolytic cell) technology with MBfR to form a new coupling process. In this process, MEC efficiently converts organic waste into carbon dioxide and hydrogen through electrode–microbial interactions.15 Subsequently, these gases are introduced into the MBfR to participate in the deep nitrogen removal process as electron acceptors, further improving the efficiency and quality of wastewater treatment.
In addition, we introduced H2-PDA, a hydrogen-based microbial reaction process, to further optimize the performance of the MBfR. In the H2-PDA process, the nitric acid generated by the anaerobic ammoxidation reaction is converted to nitrite through denitrification, and then the anaerobic ammoxidation reaction with ammonia and nitrogen in the wastewater forms an efficient nitrogen cycle process.16 This process not only reduces matrix competition but also promotes the enrichment and growth of microorganisms, thus further improving the efficiency of wastewater treatment.17
These coupled process technologies not only significantly improve the performance of MBfR but also show great potential to reduce energy consumption, reduce environmental pollution and meet carbon reduction targets. By promoting microbial enrichment, reducing substrate competition and promoting energy recombination, the application scope of MBfR has been broadened, making important contributions to environmental protection and sustainable development.
2. The principle and characteristic of coupling technology
2.1 Membrane biofilm reactors (MBfR)
The MBfR technology, through the metabolic activities of microorganisms in a biofilm, effectively removes a wide range of pollutants from wastewater. It can effectively remove pollutants such as organic matter, inorganic matter, and heavy metals from wastewater.18 This is achieved through the metabolic activities of microorganisms on the biofilm,19 which can convert pollutants into harmless or low-toxic substances (Fig. 1).20 As a result, water quality is improved, and the discharged water meets or exceeds national standards. This technology also helps to reduce treatment costs and improve efficiency.21 By reducing the direct pollution of wastewater and promoting water recycling and reuse, MBfR contributes to environmental protection and sustainable development in China by addressing water scarcity issues.
 |
| Fig. 1 Core of the hydrogen matrix reactor lies in the inner membrane and the attached biofilm, in which micro-microorganisms, hydro autotrophs, sulfate-reducing bacteria, and methanogens are present. Due to the abundance of microorganisms, various pollutants in sewage, such as 1,1,1-trichloroethane, nitrate, dichloromethane, sulfate, and tetracycline, can be treated. | |
There are extremely rich microbial populations in MBfR, and different microbial strains have degradation functions for one or more pollutants.22 Therefore, MBfR can remove numerous types of pollutants; see Table 1 for details.
Table 1 List of pollutants treated by MBfR and their environmental benefits
Pollutant type |
Removal mechanism |
Representative microbial |
Removal effect |
Treatment efficiency |
Environmental impact |
Ref. |
Treatment efficiency: processing efficiency of more than 90% is considered high; a treatment effect of more than 60% and less than 90% is considered medium. |
Chlorinated organic |
Metabolic transformation |
Lysobacter sp., Acetobacterium, Dechloromonas, Geothrix, Pseudomonas |
Significantly reduced biological toxicity |
High |
Reduces environmental risks |
23
|
Chlorinated hydrocarbon |
Remove |
Xanthobacter, Thauera, Hydrogenophaga |
Significant removal |
Medium |
Reduces ecotoxicity |
10
|
Chlorinated phenol |
Remove |
Dechloromonas
|
Effective removal |
High |
Reduces ecological risks |
10
|
Chlorite (NO2−) |
Oxidation |
Dechloromonas
|
Complete removal |
High |
Reduces water pollution |
24
|
Nitrate (NO3−) |
Oxidation |
Dechloromonas
|
Effective removal |
Medium |
Improves water quality |
25–30
|
Bromate (BrO3−) |
Oxidation |
Bacteroidota, Thauera, Brachymonas |
Significant removal |
High |
Reduces health risks |
31, 32 |
Sulfate (SO42−) |
Oxidation |
Sulfur-oxidizing bacteria |
Effective removal |
Medium |
Improves water quality |
28, 33, 34 |
Selenate (SeO2−) |
Oxidation |
Unclassified_f_Rhodo-cyclaceae g_norank_f_Pleomorphomonadacea
|
Complete removal |
High |
Reduces ecological risks |
22, 26, 28, 35–38 |
Antimonate (SbO3−) |
Oxidation |
Pseudomonas chloritidismutans
|
Effective removal |
High |
Reduces environmental risks |
39
|
Arsenate (AsO43−) |
Oxidation |
SMP
|
Complete removal |
High |
Reduces health risks |
40
|
Chromate (Cr6+) |
Oxidation |
Methylosinus, Meiothermus |
Significant removal |
High |
Reduces environmental risks |
30, 41 |
Antibiotic |
Biodegradation |
Betaproteobacteria, Acidovorax caeni, Alicycliphilus denitrificans, etc. |
Effective removal |
High |
Reduces ecological risks |
30, 41 |
The data in Table 1 show that MBfR can efficiently remove various pollutants; even pollutants that are hard to remove can be removed efficiently, and it has various removal effects and environmental impacts.
From the relevant literature, this review aims to identify possible solutions to the following issues and challenges.
Challenge 1: membrane contamination and microbial control.
One of the main challenges for the long-term stable operation of membrane biofilm reactors (MBfRs) is membrane fouling. The excessive growth of harmful microorganisms like sulfate-reducing bacteria, methanogens, and homoacetogen bacteria6,42 can worsen membrane contamination and create unnecessary diffusion resistance layers on the membrane. Therefore, it is crucial to control the growth of these harmful microbial species to reduce membrane contamination. This can be achieved by optimizing the operating conditions, introducing inhibitors, or implementing biological competition strategies to effectively manage the propagation of harmful microorganisms43 and extend the service life of MBfR.
Challenge 2: optimization of hydrogen supply and reverse diffusion.
Efficient gas transport is essential for a high-performance microbial biofilm reactor (MBfR).44 However, challenges arise with the supply of hydrogen and reverse diffusion. The lack of a cost-effective local hydrogen supply increases operating costs, while reverse diffusion in biofilms can reduce H2 concentration and electron donors, impacting the pollutant removal efficiency.6 To address these challenges, optimizing the gas utilization efficiency, improving hydrogen supply, and reducing reverse diffusion are crucial.12,45 By adjusting the biofilm structure and thickness and incorporating technologies like metal catalysts and electrochemical techniques for hydrogen supply optimization, the need for external hydrogen sources can be minimized and the effects of reverse diffusion mitigated.
Challenge 3: substrate competition and membrane structure regulation.
The competition for substrates among the diverse microbial species in membrane biofilm reactors (MBfRs) can lead to challenges in maintaining dominant strains and may result in biofilm thinning and increased reverse diffusion. To address these issues, new strategies and technologies are needed. One approach is to introduce anaerobic ammonia oxidation (anammox) technology, which can alleviate substrate competition and improve system efficiency.46 Additionally, molecular biology techniques, such as pyrophosphate sequencing, clone library and phylogenetic analysis, denaturing gradient gel electrophoresis, and real-time quantitative PCR,10 can be used to analyze and optimize the microbial community structure in biofilms. By promoting the growth and metabolic activities of dominant strains, these techniques can enhance biofilm stability and reduce reverse diffusion occurrences.
Therefore, in order to solve the above problems, the reactor-coupled metal catalyst, electrochemistry and anaerobic ammonia oxidation (anammox) are used to deal with these challenges. The MBCR utilizes biological palladium nanoparticles to optimize the biofilm and enhance microbial activity. The MEC–MBfR converts organic waste into gases for deep nitrogen removal by MBfR; the anammox-MBfR efficiently removes ammonia nitrogen through the H2-PDA reaction. To deal with these challenges, according to its working principle, the effect, reduction of carbon emissions and application prospects are analyzed (Fig. 2).
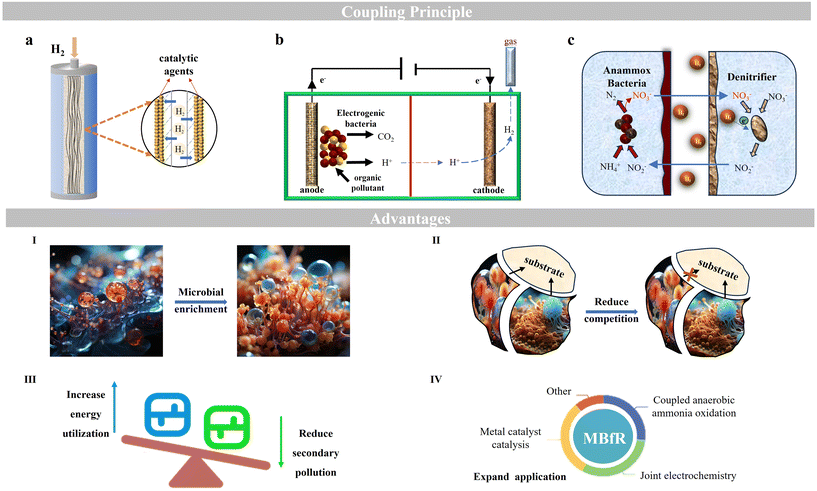 |
| Fig. 2 Coupling principle and four advantages (I–IV) of MBCR (a), MEC–MBfR (b) and H2-PDA (c) for the efficient removal of ammonia nitrogen. | |
2.2 Metal catalyst catalysis
2.2.1 Principle and characteristics.
Nanomaterials47 with small sizes and large specific surfaces have high intrinsic activity due to the exposure of more available surface atoms. Catalysts, which consist of nanoparticles and carriers such as activated carbon or graphene, provide active sites for electrocatalytic reactions involving the adsorption, conversion, and release of reactant molecules.48 Surface modification of catalysts can optimize binding conditions with specific molecules49 to enhance catalytic performance. The activity of electrocatalysts is influenced by the intrinsic activity of the active components and the number of exposed active atoms.50 Nanostructured materials serve as carrier materials for non-homogeneous catalytic hydrogenation catalysts, addressing the limitations of homogeneous catalysts. These carriers possess a large specific surface area, sufficient rigidity, excellent stability, and electrical conductivity to improve the interaction between the metal and carrier, as well as the catalytic properties of the catalysts.51 The combination of these factors ensures that the catalyst promotes rapid reactions.
The utilization of Pd-MBfR resulted in an increase in dechlorinating Aeromonas, Azospirillum, Pseudomonas, and Stenomonas denitrifying bacteria within the microbial community.52 This led to a heightened presence of the NO3−-N reductase-related genes, indicating that denitrifying bacteria were able to redirect their respiratory electron flow towards the NO3− reduction to NO2−. Furthermore, the Pd0NP immobilized by the MBfR within the biofilm matrix spontaneously generated palladium nanoparticles in situ,53 further enhancing the biofilm's capacity to reduce NO2−. The biofilm matrix played a crucial role in stabilizing and dispersing the Pd0NP, aiding in electron transfer by offering alternative pathways or acting as a conductive material.53 The distinct advantages of the Pd0NP, such as gentle synthesis conditions, high biocompatibility, excellent catalyst stability, and biofilm dispersion, provide it with a competitive advantage in NO3− reduction compared to non-precious metal catalysts or other commercial options.54–56
The MBfR utilizes a non-biological catalyst membrane instead of a microbial biofilm. This membrane is unique in that it can offer multiple functions due to its strong catalyst-anchored substrate and controlled delivery of H2 gas. Unlike other water treatment technologies that use membranes loaded with noble metal catalysts, the MBfR's nonporous hollow fiber membranes allow for the efficient and precise diffusion of H2 gas without the formation of bubbles.57 This method of delivery is based on the demand determined by the back diffusion of pollutants to the catalyst.14 By using this bubble-free delivery method, the MBfR is able to avoid the limitations of mass transfer associated with gaseous H2 diffusion, reduce H2 emissions into the liquid, and prevent the gas lift of volatile compounds.
Previous research has indicated that Pd nanoparticles can be recovered using continuous membranes, which serve as active catalysts for nitrite reduction and dechlorination.58 Studies have demonstrated that the catalytic activity is significantly enhanced when H2 is transported through the membrane at the same pressure rather than in the headspace.59 Palladium (Pd) is commonly chosen as the catalyst of choice due to its superior efficiency and activity compared to other noble metals.60 Solid-state characterization of Pd nanoparticles in Pd-MBfR has shown that the nanoparticles are evenly distributed on the outer surface of the bacteria within the biofilm.52
Furthermore, a comprehensive approach may be necessary for optimal performance, as indicated by Realpe et al.61 through their in-depth analysis, suggesting that optimizing a single factor, such as a catalyst, may not always yield the desired results in overall coupling efficiency.
2.2.2 Pd-MBfR issues and prospects.
Overall, the Pd-MBfR plays a crucial role in enhancing the efficiency and effectiveness of the NO3− reduction process, leading to a more environmentally friendly wastewater treatment. It has emerged as a superior deposition method and can be utilized to optimize catalyst attachment, nitrate reduction, and N2 selectivity in future studies involving more complex water matrices, longer treatment cycles, and larger reactors.14
However, in practice, there are still barriers to the application and development of the technology. The cost of stabilizing the catalyst using commonly used methods, which require energy-consuming or environmentally risky steps,62 including high-temperature calcination, organic solvents, and/or the additional cost of stabilizers such as poly(N-vinylpyrrolidone) (PVP) or hydrogels, is a significant barrier. Another barrier is the low lifetime of the catalyst, as the leaching of precious metal catalysts increases operating costs and leads to secondary contamination of metals released into the environment.63 Continuous exploration is still needed to regulate the Pd-MBfR dual-acting system and find suitable working conditions that will ensure the long-term stability of the reaction. The dual-action Pd-MBfR system accelerates the reduction of NO3− through the simultaneous interaction of enzymatic and catalytic reduction while also enhancing the selectivity for ammonium (NH4+).
Including the catalyst in the system significantly improves microbial function and reduces carbon emissions. The addition of the catalyst changes the competitive nature of the substrate in the reactor, directing the microbial action more effectively. This leads to the enrichment and uniform distribution of dominant microorganisms on the membrane surface, allowing for more efficient energy use. Additionally, it enhances the ability of the system to selectively remove ammonia nitrogen, reducing sludge production and subsequent biogas pollution compared to other biological methods.
In general, the coupling effect of the technology can solve the problem of membrane contamination. However, the high cost of precious metal materials is contrary to the low-cost MBfR operation, and the frequent replacement of catalytic materials makes the overall reactor difficult to operate stably. Therefore, instead of replacing the film with precious metals, it is advantageous to investigate the original plastic film, for example, using films of different materials (PP, PE, PVC, PVDF, etc.) for research on modifying the film properties.
2.3 Joint electrochemistry
2.3.1 Principles and characteristics.
Biological hydrogen production from biomass can be carbon-neutral and sustainable using the microbial electrolysis hydrogen production technology.64 This technology utilizes microbial electrolysis cells (MEC), in which electrogenic microorganisms, mainly electrogenic bacteria, grow on the anode and oxidize organic matter to produce electrons, protons, and CO2.65 The anode collects the electrons that travel through an external circuit to the cathode, where they combine with protons to produce hydrogen.15
A hybrid system combining MEC and a membrane biofilm reactor (MBfR) has been developed to improve the hydrogen production process. This system offers a novel approach to producing hydrogen from various organic wastes found in wastewater and solid waste, with low energy consumption and minimal environmental impact compared to traditional industrial methods like steam reforming.66 Previous studies have shown the effectiveness of this hybrid system in enhancing hydrogen production.67
Moreover, the hydrogen precipitation process in microbial electrolysis cells (MEC) can be easily adjusted as the rate of hydrogen production (rH2) in MEC is directly proportional to the applied voltage within a specific range.68 This feature has led to increased interest in using MEC as effective hydrogen-driven denitrification devices for groundwater remediation.46
While direct cathodic denitrification using the external circuit electrons is a commonly reported method, it is limited by the electrode current density in large-scale systems.69 On the other hand, electron compensation through hydrogen offers a solution for long-distance separation between the electron donor (anode) and electron acceptor (cathode) sites in bioelectrochemical systems (BES). This overcomes the challenges of narrow electrode distances in BES, allowing for more efficient denitrification applications.70
In addition, bioelectrochemical systems (BES) require very narrow electrode distances, which limits the operating distance between the organic waste conversion site (anode) and the denitrification site (cathode).71,72 Electron compensation in the form of hydrogen allows for a long-distance separation of the electron donor and the electron acceptor parts, overcoming the above-mentioned limitations for the application of denitrification within a BES.15
In their study, Han et al.15 developed an MEC-assisted membrane biofilm reactor (MEC–MBfR) that used hydrogen to remove nitrogen from nitrate-contaminated groundwater. They utilized single-compartment MECs as high-efficiency devices for organic wastewater degradation and hydrogen generation in the MBfR. By applying negative pressure to the MEC for active pumping and converting it to positive pressure for gas supply, they achieved hydrogen autotrophic denitrification of the contaminated groundwater.73
The MEC–MBfR is expected to effectively address the challenge of insufficient clean electron donors for nitrogen removal in groundwater.74 A key advantage of the microbial electrochemical system (MEC) is its ability to separate a single redox reaction into two electrode reactions, allowing for the separation of electron acceptors and donors.75 This separation enables the anodic respiration reaction of organic waste to be distinct from the target reduction reaction produced by the cathodic biogas in the MEC. Additionally, the liquid–gas phase transition helps prevent potential contamination of the produced gas by the influent wastewater.
Therefore, the single-chamber microbial electrolysis cells (MEC) are more effective than the dual-chamber MEC in facilitating reduction reactions using biogas transport, even in the presence of electrolyte contamination.76 The utilization of single-chamber MEC for producing hydrogen-rich biogas as an electron donor for the on-site removal of groundwater nitrate, in combination with membrane biofilm reactors (MBfRs) to improve hydrogen efficiency through bubble-free membrane gas diffusion and on-site denitrification biofilm, further highlights its efficacy. The MEC is capable of removing various organic pollutants, and the applied voltage boosts the response of hydrogen-producing bacteria to the hydrogen-rich ethanol, leading to increased energy recovery and addressing the limitations of denitrification in bioelectrochemical systems (BES). Furthermore, the hydrogen substrate reactor can efficiently utilize in situ produced H2 and CO2, eliminating the need for transportation and associated safety risks.77
2.3.2 MEC–MBfR issues and prospects.
Compared to conventional treatment methods, the MEC–MBfR has lower energy consumption and shorter hydraulic retention time to meet different groundwater quality standards. Hydrogen autotrophic denitrification has energy consumption similar to heterotrophic denitrification and sulfur autotrophic denitrification. Still, it does not require organic matter and sulfur agents, reducing the operating costs and avoiding secondary contamination.
The MEC–MBfR process is an effective technology for the remediation of low-nutrient water, as it simultaneously removes organic pollutants and facilitates denitrification. This is achieved by the synergistic treatment of the MEC and MBfR, which also prevents contamination of the generated gas by the influent wastewater in the MEC. This process can achieve reduction reactions via biogas delivery without concerns for MEC electrolyte contamination while also addressing the issue of low nutrient levels in subsequent MBfRs. The MEC–MBfR technology has a higher energy consumption than the cathodic nitrate reduction-based BES, which is expected to generate net energy for carbon reduction.
There are still certain limitations in the coupling of this technology. When the MEC technology is used to treat underground sewage, other pollutants could use a large amount of energy to react with other pollutants, and H+ can be generated after the first reaction. Secondly, the subsequent gas coupling has the problem of gas supply and pressure, whether the gas production of MEC can correspond to the demand of MBfR, and whether the gas pressure generated can meet the minimum pressure standard required by the MBfR. If these issues are not solved, coupling the two reactors could be a complex process. Researchers have suggested the direct conversion of underground polluted water into pure water and using water electrolysis technology for tight coupling at a low cost.78
Chen79 modified the original MBfR into a methane-driven membrane biofilm reactor by changing the inlet air. This modification opens up further possibilities for coupling. Since the prevalent anaerobic sludge digestion (AD) can be unpredictable, Park et al.80 proposed using MEC to provide a low voltage (0.2–0.8 V) to accelerate CH4 production and stabilization through rapid degradation of organic waste. This results in the novel AD–MEC–MBfR process, which achieves simultaneous removal of high concentrations of organic pollutants and synergistic operation of the MBfR, providing a suitable treatment process for the complexity of difficult sludge digestion and non-compliance of nitrogen in wastewater plants.
2.4 Coupled anaerobic ammonia oxidation
2.4.1 Principles and characteristics.
Anaerobic ammonium oxidation (anammox) is a process where anammox microorganisms convert ammonia nitrogen (NH4+-N) into nitrogen gas (N2) and some nitrate nitrogen (NO3−-N) under anaerobic or anoxic conditions, using nitrite nitrogen (NO2−-N) as an electron acceptor.81 This process, carried out by autotrophic bacteria,16 does not require additional organic carbon sources or aeration, leading to minimal sludge production.
Anammox helps reduce CH4 and CO2 emissions during sludge digestion, contributing to carbon reduction.82 However, challenges arise from the presence of residual NO3−-N and the availability of NO2−-N sources in wastewater, hindering the progress and practical application of anaerobic ammonia oxidation.83 Partial denitrification (NO3–-N → NO2−-N) offers a potential solution by providing a stable and sustainable source of NO2−-N while removing residual NO3−-N from the Anammox reaction. This process also reduces the need for organic matter and oxygen without affecting the nitrite-oxidizing bacteria.46
Anaerobic ammonia oxidation and partial denitrification offer significant advantages in nitrogen removal.84,85 However, the use of carbon sources like methanol in partial denitrification can lead to secondary organic pollution and hinder the coexistence of autotrophic and heterotrophic microorganisms in a single reactor.86 The MBfR can reduce NO3−-N without organic compounds and has been successfully used to achieve autotrophic partial denitrification.
Previous research has shown that a gas conditioner can achieve the effective transfer of the electron donor H2 through hollow fiber membranes. H2 is non-toxic and more cost-effective than other electron donors, leaving no residues in the bioreactor with a low biomass yield.87 A hydrogen-based partial denitrification system coupled with anaerobic ammonia oxidation (H2-PDA) was implemented in the MBfR to reduce hydrogen demand significantly.88 During the PDA, NO3−-N first accepts electrons from the electron acceptor and is converted by nitrate reductase to NO2−-N via partial denitrification. Anaerobic ammonia-oxidizing bacteria then convert ammonia from the influent water to gaseous nitrogen through oxidation along with NO2−-N.89
Denitrifying bacteria play a crucial role in reducing NO3−-N produced by anaerobic ammonia oxidation to NO2−-N, allowing anaerobic ammonia-oxidizing bacteria to continue utilizing it until it is completely depleted. This process relies on maintaining the proper balance between COD and N, particularly the COD/N ratio.88 This indicates that the H2-PDA process can effectively achieve deep nitrogen removal without relying on an organic carbon source. The H2-PDA process can also help avoid the unbalanced growth of heterotrophic denitrifying bacteria and autotrophic anaerobic ammonia-oxidizing bacteria.90
2.4.2 H2-PDA issues and prospects.
According to the study, it was shown that the introduction of H2-based partial denitrification-anaerobic ammonia oxidation (H2-PDA) in the MBfR provides a new and highly efficient means of denitrification in MBfR, which on the one hand, strengthens the biofilm resistance to shock and the reactor changes from a low concentration of carbon and nitrogen to a relatively high concentration, and on the other hand, removes nitrate and ammonium nitrogen at the same time.91
Since anammox uses NO2− as an electron donor,92 thus reducing the electron donor required for the overall nitrogen removal, and at the same time, hydrogen is not only non-toxic but also less costly than other electron donors,87 and leaves no residues in the bioreactor and has a low biomass yield, its operation and subsequent treatment costs are lower. The H2-PDA process reduces the amount of hydrogen needed for the operation, making it safer. This process is energy-efficient for treating nitrogenous wastewater and expands the practical application of MBfR. These findings suggest that implementing a low-cost H2-PDA process in MBfRs offers a promising direction for advanced wastewater treatment. The process reduces demand and optimizes energy structure, providing a new carbon reduction process for wastewater treatment plants.
A one-stage continuous-flow membrane-hydrogel reactor was designed to integrate the partial nitritation-anammox (PN-anammox) and anaerobic digestion (AD) for simultaneous autotrophic nitrogen and anaerobic carbon removal from the mainstream municipal wastewater.17 We propose a model that combines PN/anammox/DAMO to achieve complete denitrification through a multi-step process. The process involves PN occurring in the aerobic layer to provide nitrite nitrogen for subsequent reactions, followed by the coupling of anammox and DAMO in the anaerobic layer. This coupling of multiple nitrogen removal processes promotes synergistic interactions, enhancing nitrogen removal and reducing treatment costs. The process utilizes the CH4 produced on-site at the wastewater treatment plant for complete nitrogen removal, generating biogas for the treatment and meeting denitrification requirements for excess sludge disposal and energy recovery (Fig. 3).
 |
| Fig. 3 Membrane reaction interface can be divided into aerobic, parthenogenetic, and anaerobic layers depending on the oxygen concentration. First, the PN reaction occurs in the aerobic layer, and then the nitrite produced by the PN reaction is transferred to the anaerobic layer. The anaerobic layer can control the anaerobic and parthenogenetic layers until nitrate is formed. Methane gas transfers mass to the anaerobic layer, and the DAMO reaction occurs. | |
3. Carbon emission assessment and environmental benefits
Reducing carbon emissions is crucial for combating global climate change,93 protecting ecosystems, promoting sustainable development and improving the quality of human life. By reducing greenhouse gas emissions, we can slow climate warming and reduce the risk of extreme weather while preserving biodiversity and maintaining the ecological balance.94 Furthermore, carbon emission reduction is a vital strategy for promoting the transition to a green economy, achieving sustainable development, enhancing air quality, and improving the quality of life.95
3.1 The basic concept and method of life cycle assessment
Various methods can be used to calculate carbon emissions,96 including the mass balance, carbon footprint, emission factor, and model methods. In this study, the carbon footprint method based on the life cycle assessment and emission factor method are combined to calculate carbon emissions, and the main sources of carbon emissions are identified. Life cycle assessment (LCA) can be applied to evaluate the environmental impact of a certain product, process or nature of the process and identify and quantify the resources, energy and environmental emissions used in these processes to obtain their environmental impact,97 and seek opportunities to improve these environmental impacts.
By systematically analyzing the whole process of wastewater treatment technology from raw material acquisition, manufacturing, operation and the use to final disposal, life cycle assessment can fully reveal its potential impact on the environment.98 In the life cycle of wastewater treatment technology, each stage may have different environmental impacts, such as energy consumption, greenhouse gas emissions, water consumption and solid waste generation. Therefore, adopting the life cycle assessment method can realize the comprehensive quantification and analysis of the environmental impact of wastewater treatment technology.99 In terms of specific applications, life cycle assessment first requires a detailed inventory analysis of the full life cycle of the wastewater treatment technology. This step includes the collection and processing of various input and output data related to the technology, such as raw material consumption, energy consumption, pollutant emissions generated during wastewater treatment, etc.;100 using this information, the whole life cycle list of wastewater treatment technologies can be constructed to provide basic data support for the subsequent environmental impact assessment.
3.2 LCA evaluation of MBfR carbon emissions
A common issue in domestic sewage treatment projects is the lack of a carbon source during the denitrification stage, particularly due to low organic matter in the influent sewage.101 Therefore, external carbon sources (such as methanol) are often added in the denitrification stage to facilitate nitrogen removal. However, methanol is considered a fossil carbon as it is ultimately oxidized into CO2 and emitted into the atmosphere.102 This part of CO2 is the direct emission of actual fossil sources.
MCO2 equivalent,Denitrification = 1.375MCH3OH/Q |
M
CH3OH – the total weight of methanol added in the denitrification stage of the sewage treatment, kg d−1, Q – the average daily water intake of sewage treatment, m3 d−1.
There are three kinds of greenhouse gases directly emitted by sewage treatment, namely CO2, CH4 and N2O.103 Among them, the warming potential of CH4 and N2O emissions is about 21 and 310, respectively.104 Therefore, the reduction of carbon emissions in sewage plants needs to be analyzed.93
When calculating carbon emissions from sewage plants, it is crucial to consider the N2O emission coefficient, which can be determined through direct measurement for more accurate calculations.105 However, the measurement method is more complicated and is not easy to operate and popularize in practical applications. Therefore, in the calculation of carbon emissions, the empirical emission coefficient of Foley et al. was adopted as the default value for most of the traditional nitrification and denitrification processes, namely 0.035 kg N2O-N per kg N removal (Fig. 5).106
Compared to traditional denitrification processes (Fig. 6), hydrogen matrix reactors offer a carbon reduction effect through three key mechanisms:
1. Hydrogen utilization: hydrogen is considered to be a high-quality electron donor for autotrophic nitrogen removal because it does not produce harmful substances and greenhouse gases while being cheaper than organic electron donors.87 In the MBfR, hydrogen is diffused into the biofilm in a bubble-free manner through the membrane pores and utilized by the denitrifying bacteria, thus achieving nitrate reduction.19 This approach reduces the use of organic electron donors, carbon source consumption and carbon emissions.
2. Efficient nitrogen removal: biofilm in the MBfR creates numerous microbial habitats, enhancing the efficiency of nitrogen removal by promoting microbial degradation.19 The denitrifying bacteria that grow on the biofilm utilize hydrogen to convert nitrate into nitrogen, resulting in a more effective nitrogen removal process.107 This process helps decrease the release of nitrogen pollutants and minimizes the environmental impact.
3. Low carbon–nitrogen ratio adaptability: the MBfR demonstrates adaptability to low carbon–nitrogen ratios by effectively removing nitrogen, making it a superior option for treating wastewater with such conditions. Unlike traditional biological nitrogen removal processes, which struggle with a low carbon–nitrogen ratio wastewater, the MBfR excels in overcoming this challenge, enhancing treatment efficiency and reducing carbon levels.108
3.3 Carbon emissions in the life cycle of coupling technologies
3.3.1 Carbon reduction analysis of the Pd-MBCR technology.
MBCR technology is an efficient biological wastewater treatment technology, in which the organic matter in wastewater is degraded by microorganisms in a moving-bed biofilm reactor. The technology uses a palladium material as a biofilm carrier, and the palladium material can significantly improve the activity and stability of the biofilm due to its good catalytic performance and stability, thus enhancing the treatment efficiency.
1. Raw material acquisition stage: palladium is a precious metal, and its mining and processing process will produce a certain amount of carbon emissions. However, due to the relatively small amount of palladium used in biofilm carriers and the fact that it can often be recycled, this portion of carbon emissions is relatively limited. In addition, with the advancement of technology and the improvement of recycling processes, the recovery rate of palladium materials continues to increase, further reducing carbon emissions at the raw material acquisition stage. The production process of the supporting equipment (such as pumps, pipes, etc.) also produces carbon emissions. In order to reduce this part of the carbon emissions, environmentally friendly materials and production processes can be chosen, as well as optimize the equipment design, and reduce energy and material consumptions.
2. Operation and use: MBCR technology shows significant advantages in terms of carbon reduction with its efficient processing capacity and low energy consumption. The efficient catalysis of the palladium biofilm carrier enables the MBCR to degrade organic matter more quickly in wastewater treatment, reducing the treatment time and energy consumption. In addition, the MBCR technology also has the advantages of a small footprint, easy operation and low maintenance costs, further improving its carbon reduction effect. According to the network data and the latest research results, the MBCR technology can reduce carbon emissions by about 20% to 30% when treating municipal sewage compared to the traditional activated sludge method (the specific value may vary depending on the scale of treatment, water quality and other factors). This reduction is mainly due to the efficient processing capacity and lower energy consumption of the MBCR technology.
Even though Pd is expensive, it removes conventional pollutants, heavy metal ions, organic pollutants and other difficult-to-deal-with pollutants. This ability allows palladium to play an important role in improving water quality, safety and reliability.
3.3.2 Carbon reduction analysis of the MEC–MBfR technology.
The MEC–MBfR technology combines the advantages of microbial electrolysis cells and membrane bioreactors to convert organic waste into carbon dioxide and hydrogen through electrode–microbial interactions. This technology not only realizes the resource utilization of organic waste but also reduces the carbon emissions in the treatment process.
1. Raw material acquisition stage: carbon emissions are generated during the production of electrode materials (such as carbon cloth, titanium mesh, etc.), membrane components (such as polytetrafluoroethylene film, ceramic film, etc.) and supporting equipment. In order to reduce this part of the carbon emissions, you can choose environmentally friendly materials and production processes, as well as optimize equipment design, and reduce energy and material consumptions.
In addition, considering the renewability and recyclability of the electrode material, carbon emissions can be further reduced at the raw material acquisition stage.
2. Operation and use stage: MEC–MBfR technology converts organic waste into clean energy (hydrogen and carbon dioxide) through the electrolysis of microorganisms and achieves deep nitrogen removal. This conversion process not only reduces the emission of organic waste but also reduces energy consumption and carbon emissions during the treatment process.
According to calculations, the MEC–MBfR technology can reduce carbon emissions by about 30% to 40% when treating organic wastewater compared to the traditional anaerobic digestion technology (the specific value may vary depending on the scale of treatment, water quality and other factors). This reduction is mainly due to the clean energy conversion capability and deep nitrogen removal effect of the MEC–MBfR technology.
The MEC–MBfR technology has the ability to convert organic waste into clean energy, but its electrolytic phase can consume considerable electricity. Electrolysis is the most important consumption, so optimizing the electrolytic conditions is the key. By adjusting the composition, concentration, temperature and pH value of the electrolyte, the charge transfer and reaction kinetics in the electrolysis process can be improved to improve the electrolysis efficiency and reduce power consumption. For example, choosing the right supporting electrolyte can improve the electrochemical stability and efficiency. The optimum reaction temperature can be determined by exploring the influence of temperature on the reaction rate and performance. By adjusting the pH value to change the proton concentration, the reaction mechanism can be optimized.
3.3.3 Carbon reduction analysis of the H2-PDA technology.
The H2-PDA technology is an efficient wastewater nitrogen removal technology that uses anaerobic ammoxidation to convert ammonia nitrogen in wastewater into nitrogen. This technology not only has an efficient nitrogen removal effect but also has low energy consumption and carbon emissions.
1. Raw material acquisition stage: carbon emissions are generated during the production of biofilm carriers, aeration units and supporting equipment. In order to reduce this part of the carbon emissions, you can choose environmentally friendly materials and production processes, as well as optimize equipment design, reduce energy consumption and material consumption. In addition, taking into account the renewability and recycling of biofilm carriers, carbon emissions at the raw material acquisition stage can be further reduced.
2. Operation and use stage: the H2-PDA technology converts ammonia nitrogen from wastewater to nitrogen through an anaerobic ammoxidation reaction, while producing a small amount of nitric acid. This nitric acid can be converted to nitrogen through subsequent denitrification, thus achieving complete nitrogen removal and carbon reduction.
According to calculations, the H2-PDA technology can reduce carbon emissions by about 40% to 50% compared to the traditional nitrifying and denitrification processes when treating wastewater with high ammonia nitrogen (the specific value may vary depending on the scale of treatment, water quality and other factors). This reduction is mainly due to the anammox-MBfR technology's efficient nitrogen removal capacity and lower energy consumption.
3.4 General views and prospects
In general, each coupled process has its advantages and disadvantages. While these processes have the potential to save energy and reduce carbon emissions, they still face challenges in terms of cost, microbiology, safety, and practical applications.
Laboratory operations are often well-controlled and refined, but practical applications may find it challenging to meet the same standards. Variations in conditions can lead to inconsistencies in performance, making it difficult to ensure long-term stability. For example, the Pd-MBfR process shows promise in overcoming membrane issues and expanding application possibilities, but the high cost and difficulty of replacement present significant barriers to real-world implementation.
It is important for coupled processes to prioritize sustainability, considering not only treatment effectiveness but also environmental impact at every stage. For instance, while the MEC–MBfR shows excellent performance in treating wastewater, the electricity consumption of the MEC stage contradicts the goal of energy conservation and emission reduction. This discrepancy hinders widespread adoption and application of the technology.
To tackle these challenges, the following upgrades should be considered.
1. To optimize the process design, one can reduce energy consumption by enhancing the design at various stages. This could involve utilizing more efficient power conversion systems, optimizing reaction conditions, or developing new reaction materials.
2. Conduct a life cycle assessment: perform a comprehensive life cycle assessment (as shown in Fig. 4) of the entire process, analyzing energy consumption, pollutant emissions, resource usage, and other factors. This will help identify any bottlenecks in the process and allow for targeted improvement measures to be proposed.
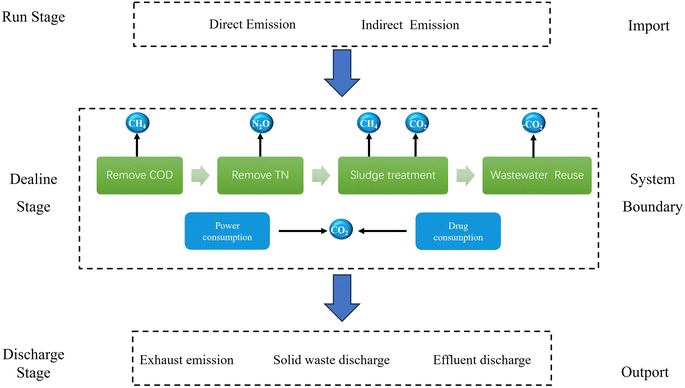 |
| Fig. 4 Life cycle assessment system boundary: the boundary range of the system is defined from the beginning of the sewage treatment system to the end of the tailwater discharge and sludge disposal. | |
 |
| Fig. 5 Difference in carbon emissions from traditional wastewater treatment methods. | |
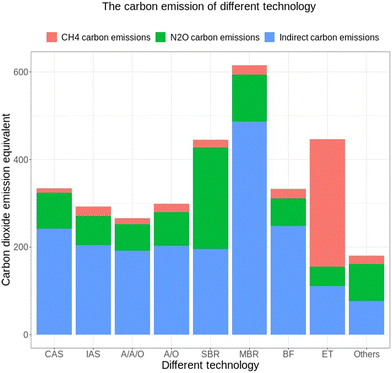 |
| Fig. 6 Carbon emissions in different technologies; data from ref. 109. | |
3. When implementing the technology in real-world scenarios, it is often necessary to integrate it with other systems or equipment to enhance the overall performance. This may involve making modifications and adjustments to the technology, using intelligent computer simulations and automated operations to ensure compatibility and seamless operation with other components. Furthermore, detailed operating specifications and maintenance processes must be established to guarantee the technology's long-term stability.
4. Conclusion
The MBfR process has numerous advantages in wastewater treatment, such as reduced carbon emissions and improved nitrogen removal, leading to a significant amount of related research being conducted. While there has been in-depth research on the influence of MBfR reactors, conditioning factors, and microbial species, many areas still require further exploration and study. Additionally, we propose the PN/anammox/DAMO multi-step coupled MBfR process and the AD–MEC–MBfR process as potential new methods for wastewater treatment.
Despite the progress made, further research on adapting and improving the MBfR reactors is needed to enable the practical application of MBfR-based technologies in sustainable wastewater treatment. Future studies will mainly focus on establishing comprehensive optimization parameters for the MBfR process, biological improvement to cultivate dominant bacteria, improving gas supply to reduce concentration gradients and ensuring the even distribution of bacterial strains within the biofilm. At the same time, more and more people now use automated facilities for multi-technology coupling, further opening a new era of coupling with the intelligence of computer simulation. In exploring the potential of MBfR technology, we should not be limited to the field of urban wastewater treatment. In fact, the MBfR has also shown great application prospects in other fields or scenarios. For example, in agricultural wastewater treatment, MBfR technology can effectively remove organic matter, nitrogen, phosphorus and other pollutants in wastewater and realize the recycling of water resources. In terms of industrial wastewater treatment, MBfR technology can efficiently degrade various toxic and harmful substances, providing a strong guarantee for the green development of industrial production.
To summarize, MBfR technology has application potential in different fields and could significantly contribute to environmental protection and sustainable development owing to its unique technical advantages.
Data availability
No primary research results, software or code have been included and no new data were generated or analysed as part of this review.
Author contributions
Chang Mei: conceptualization, methodology, software, investigation, formal analysis, writing – original draft; Meng Zhang: data curation, writing – original draft; YuChao Chen: visualization, investigation; Kun Dong: software, validation; RuiZe Sun: data curation, supervision; XueHong Zhang: writing – review & editing; HaiXiang Li: conceptualization, funding acquisition, resources, supervision, writing – review & editing.
Conflicts of interest
The authors declare no competing conflicts of interest.
Acknowledgements
The authors gratefully acknowledge the financial support of this work by the National Natural Science Foundation of China (52470029), Guangxi Bagui Youth Top Talent Program, Guangxi Natural Science Foundation (2022GXNSFFA035033), and Research Funds of the Guangxi Key Laboratory of Theory and Technology for Environmental Pollution Control (2301Z003).
References
- D. Dutta, S. Arya and S. Kumar, Industrial wastewater treatment: Current trends, bottlenecks, and best practices, Chemosphere, 2021, 285, 131245 CrossRef CAS PubMed.
- B. J. Singh, A. Chakraborty and R. Sehgal, A systematic review of industrial wastewater management: Evaluating challenges and enablers, J. Environ. Manage., 2023, 348, 119230 CrossRef PubMed.
- Z. Nie, M. Huo, F. Wang, S. Ai, X. Sun, S. Zhu, Q. Li and D. Bian, Pilot study on urban sewage treatment with micro pressure swirl reactor, Bioresour. Technol., 2021, 320, 124305 CrossRef CAS PubMed.
- C. Zhang, G. Zhao, Y. Jiao, B. Quan, W. Lu, P. Su, Y. Tang, J. Wang, M. Wu, N. Xiao, Y. Zhang and J. Tong, Critical analysis on the transformation and upgrading strategy of Chinese municipal wastewater treatment plants: Towards sustainable water remediation and zero carbon emissions, Sci. Total Environ., 2023, 896, 165201 CrossRef CAS PubMed.
- A. T. Mortensen, E. M. Goonesekera, A. Dechesne, T. Elad, K. Tang, H. R. Andersen, B. F. Smets and B. Valverde-Pérez, Methanotrophic oxidation of organic micropollutants and nitrogen upcycling in a hybrid membrane biofilm reactor (hMBfR) for simultaneous O2 and CH4 supply, Water Res., 2023, 242, 120104 CrossRef CAS PubMed.
- Y. Jang, S.-H. Lee, N.-K. Kim, C. H. Ahn, B. E. Rittmann and H.-D. Park, Biofilm characteristics for providing resilient denitrification in a hydrogen-based membrane biofilm reactor, Water Res., 2023, 231, 119654 CrossRef CAS PubMed.
- K. J. Martin and R. Nerenberg, The membrane biofilm reactor (MBfR) for water and wastewater treatment: principles, applications, and recent developments, Bioresour. Technol., 2012, 122, 83–94 CrossRef CAS PubMed.
- B. E. Rittmann, The membrane biofilm reactor: the natural partnership of membranes and biofilm, Water Sci. Technol., 2006, 53, 219–225 CrossRef CAS PubMed.
- C. Zhou, A. Ontiveros-Valencia, R. Nerenberg, Y. Tang, D. Friese, R. Krajmalnik-Brown and B. E. Rittmann, Hydrogenotrophic Microbial Reduction of Oxyanions With the Membrane Biofilm Reactor, Front. Microbiol., 2019, 9, 3248 CrossRef PubMed.
- Y. S. Lai, A. Ontiveros-Valencia, T. Coskun, C. Zhou and B. E. Rittmann, Electron-acceptor loadings affect chloroform dechlorination in a hydrogen-based membrane biofilm reactor, Biotechnol. Bioeng., 2019, 116, 1439–1448 CrossRef CAS PubMed.
- A. Penesyan, I. T. Paulsen, S. Kjelleberg and M. R. Gillings, Three faces of biofilms: a microbial lifestyle, a nascent multicellular organism, and an incubator for diversity, npj Biofilms Microbiomes, 2021, 7, 80 CrossRef CAS PubMed.
- Y. Zhang, M. Jiang, J. Ma, Y. Wang, X. Zhang, Q. Wei, X. Wang, X. Zhang and J. Zheng, Development of a novel periodic venting-controlled membrane biofilm reactor for hydrogenotrophic denitrification: Process performance and microbial mechanism, Chem. Eng. J., 2023, 463, 142529 CrossRef CAS.
- Y. H. Zhang, K. Dong, M. Zhang, X. F. Wang, X. H. Zhang, D. Q. Wang, R. Z. Sun, H. X. Li and W. J. Zhang, Effect of the main properties of membrane materials on denitrification of hydrogen-based membrane biofilm reactors (H2-MBfRs), J. Water Process Eng., 2024, 66, 105975 CrossRef.
- J. Levi, S. Guo, S. Kavadiya, Y. Luo, C. S. Lee, H. P. Jacobs, Z. Holman, M. S. Wong, S. Garcia-Segura, C. Zhou, B. E. Rittmann and P. Westerhoff, Comparing methods to deposit Pd-In catalysts on hydrogen-permeable hollow-fiber membranes for nitrate reduction, Water Res., 2023, 235, 119877 CrossRef CAS PubMed.
- Y. Han, P. Yang, Y. Feng, N. Wang, X. Yuan, J. An, J. Liu, N. Li and W. He, Liquid-gas phase transition enables microbial electrolysis and H2-based membrane biofilm hybrid system to degrade organic pollution and achieve effective hydrogenotrophic denitrification of groundwater, Chemosphere, 2023, 331, 138819 CrossRef CAS PubMed.
- D. Parde, M. Behera, R. R. Dash and P. Bhunia, A review on anammox processes: Strategies for enhancing bacterial growth and performance in wastewater treatment, Int. Biodeterior. Biodegrad., 2024, 191, 105812 CrossRef CAS.
- B. Li, B. J. Godfrey, R. RedCorn, Z. Wang, R. Goel and M.-K. H. Winkler, Simultaneous anaerobic carbon and nitrogen removal from primary municipal wastewater with hydrogel encapsulated anaerobic digestion sludge and AOA-anammox coated hollow fiber membrane, Sci. Total Environ., 2023, 883, 163696 CrossRef CAS PubMed.
- A. Maurya, R. Kumar and A. Raj, Biofilm-based technology for industrial wastewater treatment: current technology, applications and future perspectives, World J. Microbiol. Biotechnol., 2023, 39, 112 CrossRef CAS PubMed.
- C.-Y. Yuan, W.-J. Yan, F.-Y. Sun, H.-H. Tu, J.-J. Lu, L. Feng and W.-Y. Dong, Management of biofilm by an innovative layer-structured membrane for membrane biofilm reactor (MBfR) to efficient methane oxidation coupled to denitrification (AME-D), Water Res., 2024, 251, 121107 CrossRef CAS PubMed.
- W. Zhang, Y. Wu, Y. Chen and X. Zheng, Hydrogen-driven autotrophic degradation of halogenated organic pollutants in a membrane biofilm reactor: Advances and challenges, Chem. Eng. J., 2024, 479, 147754 CrossRef CAS.
- W. Alrashed, R. Chandra, T. Abbott and H.-S. Lee, Nitrite reduction using a membrane biofilm reactor (MBfR) in a hypoxic environment with dilute methane under low pressures, Sci. Total Environ., 2022, 841, 156757 CrossRef CAS PubMed.
- B. Chen, K. Dong, Y. Xu, M. Jiang, J. Zheng, H. Zeng, X. Zhang, Y. Chen and H. Li, Biodegradation of nitrate and p-bromophenol using hydrogen-based membrane biofilm reactors in parallel, Environ. Technol., 2023, 45, 4550–4564 CrossRef PubMed.
- S. Xia, Z. Zhang, F. Zhong and J. Zhang, High efficiency removal of 2-chlorophenol from drinking water by a hydrogen-based polyvinyl chloride membrane biofilm reactor, J. Hazard. Mater., 2011, 186, 1367–1373 CrossRef CAS PubMed.
- J. Chung and B. E. Rittmann, Bio-reductive dechlorination of 1,1,1-trichloroethane and chloroform using a hydrogen-based membrane biofilm reactor, Biotechnol. Bioeng., 2007, 97, 52–60 CrossRef CAS PubMed.
- J. Chung, H. Ryu, M. Abbaszadegan and B. E. Rittmann, Community structure and function in a H2-based membrane biofilm reactor capable of bioreduction of selenate and chromate, Appl. Microbiol. Biotechnol., 2006, 72, 1330–1339 CrossRef CAS PubMed.
- L. Zhou, X. Xu and S. Xia, Effects of sulfate on simultaneous nitrate and selenate removal in a hydrogen-based membrane biofilm reactor for groundwater treatment: Performance and biofilm microbial ecology, Chemosphere, 2018, 211, 254–260 CrossRef CAS PubMed.
- S. W. Van Ginkel, Y. Tang and B. E. Rittmann, Impact of precipitation on the treatment of real ion-exchange brine using the H2-based membrane biofilm reactor, Water Sci. Technol., 2011, 63, 1453–1458 CrossRef CAS PubMed.
- S. Xia, X. Xu and L. Zhou, Insights into selenate removal mechanism of hydrogen-based membrane biofilm reactor for nitrate-polluted groundwater treatment based on anaerobic biofilm analysis, Ecotoxicol. Environ. Saf., 2019, 178, 123–129 CrossRef CAS PubMed.
- J. Chung, B. E. Rittmann, N. Her, S.-H. Lee and Y. Yoon, Integration of H2-Based Membrane Biofilm Reactor with RO and NF Membranes for Removal of Chromate and Selenate, Water, Air, Soil Pollut., 2010, 207, 29–37 CrossRef CAS.
- J. Chung, B. E. Rittmann, W. F. Wright and R. H. Bowman, Simultaneous bio-reduction of nitrate, perchlorate, selenate, chromate, arsenate, and dibromochloropropane using a hydrogen-based membrane biofilm reactor, Biodegradation, 2007, 18, 199–209 CrossRef CAS PubMed.
- L. S. Downing and R. Nerenberg, Kinetics of microbial bromate reduction in a hydrogen-oxidizing, denitrifying biofilm reactor, Biotechnol. Bioeng., 2007, 98, 543–550 CrossRef CAS PubMed.
- Z. Q. Zuo, C. K. Niu, X. Y. Zhao, C. Y. Lai, M. Zheng, J. H. Guo, S. H. Hu and T. Liu, Biological bromate reduction coupled with in situ gas fermentation in H2/CO2-based membrane biofilm reactor, Water Res., 2024, 254, 121402 CrossRef CAS PubMed.
- L. M. Nevatalo, M. F. M. Bijmans, P. N. L. Lens, A. H. Kaksonen and J. A. Puhakka, The effect of sub-optimal temperature on specific sulfidogenic activity of mesophilic SRB in an H2-fed membrane bioreactor, Process Biochem., 2010, 45, 363–368 CrossRef CAS.
- J. I. Suárez, M. Aybar, I. Nancucheo, B. Poch, P. Martínez, B. E. Rittmann and A. Schwarz, Influence of operating conditions on sulfate reduction from real mining process water by membrane biofilm reactors, Chemosphere, 2020, 244, 125508 CrossRef PubMed.
- S. Xia, F. Zhong, Y. Zhang, H. Li and X. Yang, Bio-reduction of nitrate from groundwater using a hydrogen-based membrane biofilm reactor, J. Environ. Sci., 2010, 22, 257–262 CrossRef CAS PubMed.
- S. Xia, C. Wang, X. Xu, Y. Tang, Z. Wang, Z. Gu and Y. Zhou, Bioreduction of nitrate in a hydrogen-based membrane biofilm reactor using CO2 for pH control and as carbon source, Chem. Eng. J., 2015, 276, 59–64 CrossRef CAS.
- J. Zhou, C. Wu, S. Pang, L. Yang, M. Yao, X. Li, S. Xia and B. E. Rittmann, The interplay of sulfate and nitrate triggers abiotic reduction in a hydrogen-based membrane biofilm reactor for antimonate removal, Chem. Eng. J., 2023, 474, 145798 CrossRef CAS.
- X. Chen, C.-Y. Lai, F. Fang, H.-P. Zhao, X. Dai and B.-J. Ni, Model-based evaluation of selenate and nitrate reduction in hydrogen-based membrane biofilm reactor, Chem. Eng. Sci., 2019, 195, 262–270 CrossRef CAS.
- C.-Y. Lai, L.-L. Wen, Y. Zhang, S.-S. Luo, Q.-Y. Wang, Y.-H. Luo, R. Chen, X. Yang, B. E. Rittmann and H.-P. Zhao, Autotrophic antimonate bio-reduction using hydrogen as the electron donor, Water Res., 2016, 88, 467–474 CrossRef CAS PubMed.
- H. Hasar, Simultaneous removal of organic matter and nitrogen compounds by combining a membrane bioreactor and a membrane biofilm reactor, Bioresour. Technol., 2009, 100, 2699–2705 CrossRef CAS PubMed.
- J. Chung, R. Nerenberg and B. E. Rittmann, Bio-reduction of soluble chromate using a hydrogen-based membrane biofilm reactor, Water Res., 2006, 40, 1634–1642 CrossRef CAS PubMed.
- C. Lu, P. Gu, P. He, G. Zhang and C. Song, Characteristics of hydrogenotrophic denitrification in a combined system of gas-permeable membrane and a biofilm reactor, J. Hazard. Mater., 2009, 168, 1581–1589 CrossRef CAS PubMed.
- B. Lories, T. E. R. Belpaire, B. Smeets and H. P. Steenackers, Competition quenching strategies reduce antibiotic tolerance in polymicrobial biofilms, npj Biofilms Microbiomes, 2024, 10, 23 CrossRef CAS PubMed.
- J.-J. Lu, H. Zhang, W. Li, J.-B. Yi, F.-Y. Sun, Y.-W. Zhao, L. Feng, Z. Li and W.-Y. Dong, Biofilm stratification in counter-diffused membrane biofilm bioreactors (MBfRs) for aerobic methane oxidation coupled to aerobic/anoxic denitrification: Effect of oxygen pressure, Water Res., 2022, 226, 119243 CrossRef CAS PubMed.
- S. Xia, X. Xu, C. Zhou, C. Wang, L. Zhou and B. E. Rittmann, Direct delivery of CO 2 into a hydrogen-based membrane biofilm reactor and model development, Chem. Eng. J., 2016, 290, 154–160 CrossRef CAS.
- L. Zhang, Q. Zhang, X. Li, T. Jia, S. Wang and Y. Peng, Enhanced nitrogen removal from municipal wastewater via a novel combined process driven by partial nitrification/anammox (PN/A) and partial denitrification/anammox (PD/A) with an ultra-low hydraulic retention time (HRT), Bioresour. Technol., 2022, 363, 127950 CrossRef CAS PubMed.
- S. Kumar, Z. Wang, W. Zhang, X. C. Liu, M. Y. Li, G. R. Li, B. Y. Zhang and R. Singh, Optically Active Nanomaterials and Its Biosensing Applications-A Review, Biosensors, 2023, 13, 38 Search PubMed.
- C. Vogt and B. M. Weckhuysen, The concept of active site in heterogeneous catalysis, Nat. Rev. Chem., 2022, 6, 89–111 CrossRef PubMed.
- B. C. Gates, From catalyst preparation toward catalyst synthesis, J. Catal., 2015, 328, 72–74 CrossRef CAS.
- N. Govindarajan, G. Kastlunger, H. H. Heenen and K. Chan, Improving the intrinsic activity of electrocatalysts for sustainable energy conversion: where are we and where can we go?, Chem. Sci., 2021, 13, 14–26 RSC.
- S. Mitchell, R. X. Qin, N. F. Zheng and J. Pérez-Ramírez, Nanoscale engineering of catalytic materials for sustainable technologies, Nat. Nanotechnol., 2021, 16, 129–139 CrossRef CAS PubMed.
- J. Cheng, M. Long, C. Zhou, Z.-E. Ilhan, D. C. Calvo and B. E. Rittmann, Long-Term Continuous Test of H2-Induced Denitrification Catalyzed by Palladium Nanoparticles in a Biofilm Matrix, Environ. Sci. Technol., 2023, 57, 11948–11957 CrossRef CAS PubMed.
- M. Vadai, D. K. Angell, F. Hayee, K. Sytwu and J. A. Dionne, In-situ observation of plasmon-controlled photocatalytic dehydrogenation of individual palladium nanoparticles, Nat. Commun., 2018, 9, 4658 CrossRef PubMed.
- Y. Jia, D. Liu, Y. Chen and Y. Hu, Evidence for the feasibility of transmembrane proton gradient regulating oxytetracycline extracellular biodegradation mediated by biosynthesized palladium nanoparticles, J. Hazard. Mater., 2023, 455, 131544 CrossRef CAS PubMed.
- X. Zhang, Y. Wang, C. Liu, Y. Yu, S. Lu and B. Zhang, Recent advances in non-noble metal electrocatalysts for nitrate reduction, Chem. Eng. J., 2021, 403, 126269 CrossRef CAS.
- Y. Choi and S. Y. Lee, Biosynthesis of inorganic nanomaterials using microbial cells and bacteriophages, Nat. Rev. Chem., 2020, 4, 638–656 CrossRef CAS PubMed.
- H. C. Yan, F. Q. Liu, J. N. Zhang and Y. B. A. Liu, Facile Synthesis and Environmental Applications of Noble Metal-Based Catalytic Membrane Reactors, Catalysts, 2022, 12, 13 Search PubMed.
- S. De Marchi, S. Núñez-Sánchez, G. Bodelón, J. Pérez-Juste and I. Pastoriza-Santos, Pd nanoparticles as a plasmonic material: synthesis, optical properties and applications, Nanoscale, 2020, 12, 23424–23443 RSC.
- L. Hou, G. Y. Jiang, Y. Sun, X. H. Zhang, J. J. Huang, S. D. Liu, T. R. Lin, F. G. Ye and S. L. Zhao, Progress and Trend on the Regulation Methods for Nanozyme Activity and Its Application, Catalysts, 2019, 9, 17 CrossRef.
- S. McCarthy, D. C. Braddock and J. Wilton-Ely, Strategies for sustainable palladium catalysis, Coord. Chem. Rev., 2021, 442, 26 CrossRef.
- N. Realpe, S. R. Kulkarni, J. L. Cerrillo, N. Morlanés, G. Lezcano, S. P. Katikaneni, S. N. Paglieri, M. Rakib, B. Solami, J. Gascon and P. Castaño, Modeling-aided coupling of catalysts, conditions, membranes, and reactors for efficient hydrogen production from ammonia, React. Chem. Eng., 2023, 8, 989–1004 RSC.
- W. X. Li, Z. H. Guo, J. Yang, Y. Li, X. L. Sun, H. Y. He, S. Li and J. J. Zhang, Advanced Strategies for Stabilizing Single-Atom Catalysts for Energy Storage and Conversion, Electrochem. Energy Rev., 2022, 5, 41 Search PubMed.
- Y. Tong, H. Ma, F. Xiao, S. Bohm, H. Fu and Y. Luo, Precision engineering of precious metal catalysts for enhanced hydrogen production efficiency, Process Saf. Environ. Prot., 2023, 178, 559–579 CrossRef CAS.
- S. F. Ahmed, N. Rafa, M. Mofijur, I. A. Badruddin, A. Inayat, M. S. Ali, O. Farrok and T. M. Yunus Khan, Biohydrogen Production From Biomass Sources: Metabolic Pathways and Economic Analysis, Front. Energy Res., 2021, 9, 753878 CrossRef.
- B. Kilinc, D. Akagunduz, M. Ozdemir, A. Kul and T. Catal, Hydrogen production using cocaine metabolite in microbial electrolysis cells, 3 Biotech, 2023, 13, 8 CrossRef PubMed.
- A. Boretti and B. K. Banik, Advances in Hydrogen Production from Natural Gas Reforming, Adv. Energy Sustainability Res., 2021, 2, 163–168 Search PubMed.
- K. He, W. Li, L. Tang, W. Li, S. Lv and D. Xing, Suppressing Methane Production to Boost High-Purity Hydrogen Production in Microbial Electrolysis Cells, Environ. Sci. Technol., 2022, 56, 11931–11951 CrossRef CAS PubMed.
- A. Sharma, S. E. Hussain Mehdi, S. Pandit, S. Eun-Oh and V. Natarajan, Factors affecting hydrogen production in microbial electrolysis cell (MEC): A review, Int. J. Hydrogen Energy, 2024, 61, 1473–1484 CrossRef CAS.
- S. Xu, Y. Zhang, Y. Duan, C. Wang and H. Liu, Simultaneous removal of nitrate/nitrite and ammonia in a circular microbial electrolysis cell at low C/N ratios, J. Water Process Eng., 2021, 40, 101938 CrossRef.
- D. Liang, L. Zhang, W. He, C. Li, J. Liu, S. Liu, H.-S. Lee and Y. Feng, Efficient hydrogen recovery with CoP-NF as cathode in microbial electrolysis cells, Appl. Energy, 2020, 264, 114700 CrossRef CAS.
- P. T. Kelly and Z. He, Nutrients removal and recovery in bioelectrochemical systems: a review, Bioresour. Technol., 2014, 153, 351–360 CrossRef CAS PubMed.
- D. Bhowmik, S. Chetri, K. E. Enerijiofi, A. Naha, T. D. Kanungo, M. P. Shah and S. Nath, Multitudinous approaches, challenges and opportunities of bioelectrochemical systems in conversion of waste to energy from wastewater treatment plants, Cleaner and Circular Bioeconomy, 2023, 4, 100040 CrossRef.
- M. Cruces, J. Suarez, I. Nancucheo and A. Schwarz, Optimization of the chemolithotrophic denitrification of ion exchange concentrate using hydrogen-based membrane biofilm reactors, J. Environ. Manage., 2023, 348, 119283 CrossRef CAS PubMed.
- K. Hu, W. Li, Y. Wang, B. Wang, H. Mu, S. Ren, K. Zeng, H. Zhu, J. Liang, Y. e. Wang and J. Xiao, Novel biological nitrogen removal process for the treatment of wastewater with low carbon to nitrogen ratio: A review, J. Water Process Eng., 2023, 53, 103673 CrossRef.
- E. Yang, H. Omar Mohamed, S. G. Park, M. Obaid, S. Y. Al-Qaradawi, P. Castano, K. Chon and K. J. Chae, A review on self-sustainable microbial electrolysis cells for electro-biohydrogen production via coupling with carbon-neutral renewable energy technologies, Bioresour. Technol., 2021, 320, 124363 CrossRef CAS PubMed.
- W. Cui, Y. Lu, C. Zeng, J. Yao, G. Liu, H. Luo and R. Zhang, Hydrogen production in single-chamber microbial electrolysis cell under high applied voltages, Sci. Total Environ., 2021, 780, 146597 CrossRef CAS PubMed.
- N. S. Muhammed, A. O. Gbadamosi, E. I. Epelle, A. A. Abdulrasheed, B. Haq, S. Patil, D. Al-Shehri and M. S. Kamal, Hydrogen production, transportation, utilization, and storage: Recent advances towards sustainable energy, J. Energy Storage, 2023, 73, 109207 CrossRef.
- M. El-Shafie, Hydrogen production by water electrolysis technologies: A review, Results Eng., 2023, 20, 101426 CrossRef CAS.
- R. Chen, Y.-H. Luo, J.-X. Chen, Y. Zhang, L.-L. Wen, L.-D. Shi, Y. Tang, B. E. Rittmann, P. Zheng and H.-P. Zhao, Evolution of the microbial community of the biofilm in a methane-based membrane biofilm reactor reducing multiple electron acceptors, Environ. Sci. Pollut. Res., 2016, 23, 9540–9548 CrossRef CAS PubMed.
- J. Park, B. Lee, D. Tian and H. Jun, Bioelectrochemical enhancement of methane production from highly concentrated food waste in a combined anaerobic digester and microbial electrolysis cell, Bioresour. Technol., 2018, 247, 226–233 CrossRef CAS PubMed.
- Z. Gu, Y. Li, Y. Yang, S. Xia, S. W. Hermanowicz and L. Alvarez-Cohen, Inhibition of anammox by sludge thermal hydrolysis and metagenomic insights, Bioresour. Technol., 2018, 270, 46–54 CrossRef CAS PubMed.
- D. Li, Y. Dong, S. Li, P. Jiang and J. Zhang, Biological carbon promotes the recovery of anammox granular sludge after starvation, Bioresour. Technol., 2023, 384, 129305 CrossRef CAS PubMed.
- H. Li, L. Zhou, H. Lin, X. Xu, R. Jia and S. Xia, Dynamic response of biofilm microbial ecology to para-chloronitrobenzene biodegradation in a hydrogen-based, denitrifying and sulfate-reducing membrane biofilm reactor, Sci. Total Environ., 2018, 643, 842–849 CrossRef CAS PubMed.
- Z.-Q. Ren, H. Wang, L.-G. Zhang, X.-N. Du, B.-C. Huang and R.-C. Jin, A review of anammox-based nitrogen removal technology: From microbial diversity to engineering applications, Bioresour. Technol., 2022, 363, 127896 CrossRef CAS PubMed.
- P. L. Eng Nkonogumo, Z. Zhu, N. Emmanuel, X. Zhang, L. Zhou and P. Wu, Novel and innovative approaches to partial denitrification coupled with anammox: A critical review, Chemosphere, 2024, 358, 142066 CrossRef CAS PubMed.
- S. W. Van Ginkel, R. Lamendella, W. P. Kovacik Jr., J. W. Santo Domingo and B. E. Rittmann, Microbial community structure during nitrate and perchlorate reduction in ion-exchange brine using the hydrogen-based membrane biofilm reactor (MBfR), Bioresour. Technol., 2010, 101, 3747–3750 CrossRef CAS PubMed.
- F. Di Capua, F. Pirozzi, P. N. L. Lens and G. Esposito, Electron donors for autotrophic denitrification, Chem. Eng. J., 2019, 362, 922–937 CrossRef CAS.
- A. Thongsai, S. Krishnan, P. Noophan, D. Gabriel, D. Gonzalez and S. Chaiprapat, Performance and microbial analysis of a fluidized bed membrane bioreactor operated in the partial nitrification and anammox (PN/A) mode for polishing anaerobically treated effluent to industrial discharge standard, J. Environ. Chem. Eng., 2023, 11, 109808 CrossRef CAS.
- C. Zhou, A. Ontiveros-Valencia, L. Cornette de Saint Cyr, A. S. Zevin, S. E. Carey, R. Krajmalnik-Brown and B. E. Rittmann, Uranium removal and microbial community in a H2-based membrane biofilm reactor, Water Res., 2014, 64, 255–264 CrossRef CAS PubMed.
- X. Huang, C. Duan, J. Yu and W. Dong, Transforming heterotrophic to autotrophic denitrification process: Insights into microbial community, interspecific interaction and nitrogen metabolism, Bioresour. Technol., 2022, 345, 126471 CrossRef CAS PubMed.
- M. Yang, J. Li, Z. Li, Y. Peng and L. Zhang, Enhancing anammox bacteria enrichment in integrated fixed-film activated sludge partial nitritation/anammox process via floc retention control, Bioresour. Technol., 2024, 391, 129938 CrossRef CAS PubMed.
- D. R. Shaw, M. Ali, K. P. Katuri, J. A. Gralnick, J. Reimann, R. Mesman, L. van Niftrik, M. S. M. Jetten and P. E. Saikaly, Extracellular electron transfer-dependent anaerobic oxidation of ammonium by anammox bacteria, Nat. Commun., 2020, 11, 12 CrossRef PubMed.
- M. W. Jones, G. P. Peters, T. Gasser, R. M. Andrew, C. Schwingshackl, J. Gütschow, R. A. Houghton, P. Friedlingstein, J. Pongratz and C. Le Quéré, National contributions to climate change due to historical emissions of carbon dioxide, methane, and nitrous oxide since 1850, Sci. Data, 2023, 10, 155 CrossRef CAS PubMed.
- S. M. S. U. Eskander and S. Fankhauser, Reduction in greenhouse gas emissions from national climate legislation, Nat. Clim. Change, 2020, 10, 750–756 CrossRef CAS.
- Y. Shang, S. A. Raza, Z. Huo, U. Shahzad and X. Zhao, Does enterprise digital transformation contribute to the carbon emission reduction? Micro-level evidence from China, Int. Rev. Econ. Finance, 2023, 86, 1–13 CrossRef.
- J. A. Conesa, N. Ortuno and D. Palmer, Estimation of Industrial Emissions during Pyrolysis and Combustion of Different Wastes Using Laboratory Data, Sci. Rep., 2020, 10, 6750 CrossRef CAS PubMed.
- S. Barbhuiya and B. B. Das, Life Cycle Assessment of construction materials: Methodologies, applications and future directions for sustainable decision-making, Case Stud. Constr. Mater., 2023, 19, e02326 Search PubMed.
- R. Parra-Saldivar, M. Bilal and H. M. N. Iqbal, Life cycle assessment in wastewater treatment technology, Curr. Opin. Environ. Sci. Health., 2020, 13, 80–84 CrossRef.
- L. Corominas, D. M. Byrne, J. S. Guest, A. Hospido, P. Roux, A. Shaw and M. D. Short, The application of life cycle assessment (LCA) to wastewater treatment: A best practice guide and critical review, Water Res., 2020, 184, 116058 CrossRef CAS PubMed.
- K. Saavedra-Rubio, N. Thonemann, E. Crenna, B. Lemoine, P. Caliandro and A. Laurent, Stepwise guidance for data collection in the life cycle inventory (LCI) phase: Building technology-related LCI blocks, J. Cleaner Prod., 2022, 366, 132903 CrossRef.
- X. Fu, R. Hou, P. Yang, S. Qian, Z. Feng, Z. Chen, F. Wang, R. Yuan, H. Chen and B. Zhou, Application of external carbon source in heterotrophic denitrification of domestic sewage: A review, Sci. Total Environ., 2022, 817, 153061 CrossRef CAS PubMed.
- Y. Pan, R. Z. Sun, Y. Wang, G. L. Chen, Y. Y. Fu and H. Q. Yu, Carbon source shaped microbial ecology, metabolism and performance in denitrification systems, Water Res., 2023, 243, 120330 CrossRef CAS PubMed.
- D. Wang, W. Ye, G. Wu, R. Li, Y. Guan, W. Zhang, J. Wang, Y. Shan and K. Hubacek, Greenhouse gas emissions from municipal wastewater treatment facilities in China from 2006 to 2019, Sci. Data, 2022, 9, 317 CrossRef CAS PubMed.
- G. Chataut, B. Bhatta, D. Joshi, K. Subedi and K. Kafle, Greenhouse gases emission from agricultural soil: A review, J. Agric. Food Res., 2023, 11, 100533 CAS.
- M. Maktabifard, K. Blomberg, E. Zaborowska, A. Mikola and J. Mąkinia, Model-based identification of the dominant N2O emission pathway in a full-scale activated sludge system, J. Cleaner Prod., 2022, 336, 130347 CrossRef CAS.
- J. Foley, D. de Haas, Z. Yuan and P. Lant, Nitrous oxide generation in full-scale biological nutrient removal wastewater treatment plants, Water Res., 2010, 44, 831–844 CrossRef CAS PubMed.
- S. Li, L. Duan, Y. Zhao, F. Gao and S. W. W. Hermanowicz, Analysis of Microbial Communities in Membrane Biofilm Reactors Using a High-Density Microarray, Membranes, 2023, 13, 324 CrossRef CAS PubMed.
- K. Hu, W. Li, Y. Wang, B. Wang, H. Mu, S. Ren, K. Zeng, H. Zhu, J. Liang, Y. e. Wang and J. Xiao, Novel biological nitrogen removal process for the treatment of wastewater with low carbon to nitrogen ratio: A review, J. Water Process Eng., 2023, 53, 103673 CrossRef.
- H. Hua, S. Jiang, Z. Yuan, X. Liu, Y. Zhang and Z. Cai, Advancing greenhouse gas emission factors for municipal wastewater treatment plants in China, Environ. Pollut., 2022, 295, 118648 CrossRef CAS PubMed.
|
This journal is © The Royal Society of Chemistry 2025 |
Click here to see how this site uses Cookies. View our privacy policy here.