DOI:
10.1039/D4NR04108A
(Review Article)
Nanoscale, 2025,
17, 2386-2407
Recent advances in designable nanomaterial-based electrochemical sensors for environmental heavy-metal detection
Received
6th October 2024
, Accepted 25th December 2024
First published on 23rd January 2025
Abstract
The detection of heavy metals serves as a defence measure to safeguard the well-being of the human body and the ecological environment. Electrochemical sensors (ECS) offer significant benefits such as exceptional sensitivity, excellent selectivity, affordability, and portability. This review begins by elucidating the ECS principles and delves into recent advancements in the field of heavy metal detection, including the use of metal nanoparticles, carbon-based nanomaterials, and organic framework materials. Advanced materials enhance the sensitivity and selectivity of ECS, allowing it to efficiently and rapidly identify metallic contaminants in food and the environment. Finally, the future development of ECS and challenges encountered in the development process are discussed, and testing materials for the detection of heavy-metal ions for human health and environmental safety are comprehensively considered. This study is likely to attract the interest of environmentalists and those who prioritise human health.
1. Introduction
Owing to rapid economic growth, significant concerns have arisen regarding societal, environmental, and health safety issues.1 The problem of heavy metal contamination stemming from industrialisation has accentuated,2 along with its adverse impacts on the environment and human well-being.3 Industrialisation has resulted in the emission of toxic metallic elements that pose a substantial threat to both the natural environment and human health into surrounding ecosystems.4 These heavy metals persist in the environment and are dispersed through water, air, and soil.5 Over time, they infiltrate essential resources, such as food, plants, and water, which are vital for sustaining life (Fig. 1). Heavy metal ions in the human body can have detrimental effects,6 leading to a range of health complications. Heavy metals remain in the environment for extended periods because of their non-degradable, stable, and mobile nature.7 Furthermore, heavy metal ions have long half-lives that contribute to their capacity to cause substantial and enduring harm to ecosystems and living organisms.8 The 10th Conference on Prevention Technology and Risk Assessment for Heavy Metal Pollution in 2020 emphasised the urgent need for effective strategies to avert and manage heavy metal contamination.
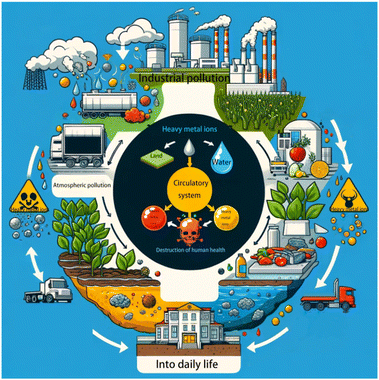 |
| Fig. 1 The cycle of Heavy metals in the environment. | |
The accumulation of heavy metals such as Cr, Co, Mn, Fe, Cu, Zn, Pb, Ni, Cd, Hg, Ag, and Pt in the human body beyond a certain threshold can cause chronic poisoning.9 Notably, metals with a density exceeding 4.5 g cm−3, such as Hg, Cd, Pb, Cr, and AS, have significant environmental impacts.10 Exposure to Cd(II) can result in renal insufficiency and metabolic imbalance, whereas exposure to Pb(II) can harm the nervous system and liver.11 The toxicities of heavy metals in equivalent amounts follow the order of Co < Al < Cr < Pb < Ni < Zn < Cu < Cd < Hg.12 The presence of heavy metal ions in the surroundings can be attributed to various sources, including industrial wastewater, exhaust emissions,13 waste residue, household waste, agricultural pesticides, and fertilisers. Certain specialised industries such as printing and electroplating inevitably expose individuals to heavy metal ions, posing significant risks to human health.14 The fifth general dietary survey revealed the presence of Cd, As, and Cr in vegetables and grains, whereas tap water and meat products were the primary sources of Pb.15 Although trace amounts of metals are essential for human metabolism, their excessive intake can cause severe harm to human health.
China, as a densely populated nation, supports 22% of the global population while occupying just 7% of the world's land. This underscores the enormous amount of environmental pollution in China, which has had a profound impact.16 These findings highlighted the importance of environmental protection in China. The intricate chemical equilibrium of the environment is maintained by the interplay among plants, air, water, and soil,17 and heavy metal ions are an integral part of this cycle. Consequently, heavy-metal pollution has been investigated since the 20th century. A World Health Organization report revealed a staggering global toll of 3–4 million deaths annually attributable to waterborne diseases resulting from pollution. In China, the consumption of drinking water containing excessive levels of heavy metals leads to an alarming 1.6 million cases of cancer each year. This trend is further highlighted by expert forecasts projecting a doubling of cancer-related fatalities in China within 20 years.18 The concentration of heavy metals in soil increases significantly, leading to crop contamination and the subsequent absorption of these heavy metals by the human body.19 To safeguard human health and the environment, it is critical to detect and remediate heavy metal pollution promptly and efficiently. Establishing robust defences against these contaminants is a fundamental step towards mitigating their adverse effects.
2. Overview of heavy metal detection methods: advantages and limitations
The quest to monitor harmful heavy metals has led to the development of several detection methods, each with its unique strengths and constraints: (1) atomic absorption spectrometry is celebrated for its precision, reliability, and discriminatory power, and is a cornerstone in heavy metal detection. However, its application is hindered by laborious sample preparation and sensitivity to matrix interference, which can complicate the analytical processes;20 (2) atomic fluorescence spectrometry is known for its user-friendliness. It has high sensitivity and selectivity, making it a favoured technique. However, its inherently weak fluorescent signal often necessitates the use of intense laser excitation to achieve optimal detection levels;21 (3) inductively coupled plasma emission spectrometry exhibits high accuracy and reduced matrix interference. The tradeoffs include significant capital investment in equipment, high operational costs, and the prerequisite of transforming solid samples into a liquid form for analysis;22 (4) X-ray fluorescence spectrometry provides a high analytical accuracy and streamlined sample preparation. Nevertheless, its relative sensitivity leaves something to be desired and analysis can be confounded by the presence of interfering elements;23 (5) electrochemical sensor technology. Compared to the above technologies, electrochemical sensors (ECS) are ideal for heavy metal detection due to their outstanding detection speed, cost-effectiveness, and operational simplicity.24 The ECS is able to convert the redox reaction signals of heavy metal ions directly into quantifiable electrical signals through a three-electrode system, without the need for complex sample preparation or expensive equipment.25 Other than that, ECS has unique advantages when dealing with complex matrix samples (e.g., sewage, food matrices), which can effectively reduce the impact of matrix interference on detection results and ensure its sensitivity and reliability.26 Its portability and low cost make it particularly suitable for on-site real-time monitoring and resource-constrained applications, such as rapid detection in environmental emergencies.27 Optimization of electrochemical detection techniques also includes the use of advanced electrochemical methods such as differential pulsed voltammetry (DPV) and square-wave anode dissolution voltammetry (SWASV). These technologies improve the ability to capture weak signals by optimizing the potential scanning mode,28 enabling sensitive detection of ultra-low concentrations of heavy metals. ECS offers significant advantages over traditional spectroscopic analysis techniques in terms of detection speed,29 cost-effectiveness, and ease of operation, especially in complex matrix samples.30 The combination of electrochemical devices and optimization techniques makes the ECS an important tool in the field of environmental monitoring and food safety, providing a reliable and efficient solution for heavy metal contamination detection.
This paper discusses the development trajectory of electrochemical sensor (ECS) materials for heavy metal detection. By summarising the current research and future development prospects, the authors conclude that ECS will play a key role in advancing the field of heavy metal detection, offering broad prospects for environmental safety and protection of human health.31 Moreover, owing to recent rapid developments, ECS can be tailored using various materials to enhance their sensitivity and detection limits when identifying heavy metals.32 ECS technologies have become increasingly prominent in medical diagnostics, food safety, and environmental monitoring, and are recognised for their versatility and effectiveness as multipurpose instruments.33 This paper sheds light on recent advancements in ECS for detecting heavy metals and delves into the use of metal nanomaterials, carbon-based nanomaterials, and organic frameworks in sensing applications. It also reviews research advancements and presents a future outlook for the use of ECS in heavy metal detection.
3. Fundamentals of electrochemical detection for heavy metals
3.1 How electrochemical sensors work
ECS are structured in a three-electrode configuration, including a reference electrode (RE), working electrode (WE), and counter electrode (CE),34 as depicted in Fig. 2.
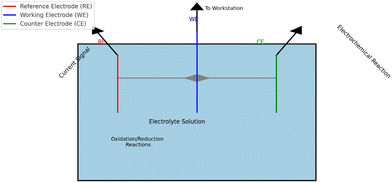 |
| Fig. 2 Schematic represenation of the electrochemical sensor system. | |
Fig. 2 The specific process of the electrochemical sensor detecting heavy metals, with the reference electrode (RE) in red, providing a stable reference potential;35
The blue electrode (WE) is the main area of the electrochemical reaction; the green color is the counter electrode (CE), which assists the working electrode to complete the electrochemical reaction.36
The three-electrode system is the core component of the ECS, which enables efficient detection of target metal ions through electrochemical reactions.37 The reference electrode (RE) provides a stable reference potential, and commonly used reference electrodes include silver/silver chloride electrode (Ag/AgCl) and saturated calomel electrode (SCE) electrode to ensure precise control of the potential during detection.38 The working electrode (WE) is the main region of the electrochemical reaction, which is directly involved in the redox reaction of the target metal ion, and its performance plays a decisive role in the sensitivity and reliability of the sensor.39 The counter electrode (CE) acts as an auxiliary electrode and forms a complete current path with the working electrode, supporting the smooth progress of the reaction.40
The basic principle of ECS is to convert chemical signals into measurable electrical signals for the sensing and quantitative detection of heavy metal ions.41 During the procedure, the sample is simply pre-treated and injected into the cell.42 The target heavy metal ions are captured by physical adsorption or chemical bonding on the surface of the working electrode, followed by a redox reaction driven by an applied electric potential.43 For example, lead (Pb2+) ions are reduced to metallic lead (Pb) on the surface of the working electrode, and the current signal generated in this process is proportional to the ion concentration.44 The potentiostat collects and analyzes these signals, and finally realizes the quantitative detection of heavy metals according to the linear relationship between the current and the concentration of the target.
3.2 Electrochemical detection of the relationship with nanomaterials
As the core component of the electrochemical sensor, the surface modification of the working electrode directly determines the capture capacity and electrochemical reaction efficiency of heavy metals.45 By combining with nanomaterials, the physical and chemical properties of the electrode are significantly optimized,46 which provides ideal conditions for the adsorption, electron transfer and chemical reaction of the target.
Carbon-based materials (e.g., graphene, carbon nanotubes) provide efficient electron transport paths for working electrodes and create more active sites due to their high conductivity and large specific surface area.47 The physical adsorption or chemical bonding between the two-dimensional layered structure of graphene and heavy metal ions significantly enhances the detection sensitivity.48 In addition, metal nanoparticles (such as gold, silver, and platinum) can effectively promote redox reactions due to their unique electronic properties and catalytic activities, while optimizing electron transfer efficiency by adjusting particle morphology and size.49 For example, the high surface energy and chemical stability of gold nanoparticles make them exhibit outstanding performance in highly sensitive sensors.50
Non-metallic nanomaterials (such as oxide nanoparticles, graphitic carbonitrides) are functionalized (such as the introduction of carboxyl, amino and other functional groups) to enhance the specific binding ability of the target to the electrode surface. This functionalization not only improves the selectivity of the electrodes,51 but also enables the sensor to exhibit excellent immunity to interference in complex matrices. In order to further optimize the performance of the working electrode, the design of porous surfaces or nanoarrays provides a higher specific surface area and more reactive sites,52 and the application of porous structures can help enhance the adsorption efficiency of heavy metal ions and accelerate the electrochemical reaction rate by shortening the diffusion path.53
The introduction of nanomaterials has significantly improved the sensitivity and selectivity of electrochemical detection.54 The optimized sensor structure and function show important technical value in environmental monitoring,55 food safety and public health protection. The following chapters of this study will discuss in detail the specific applications of metal nano, carbon-based nano, and organic framework materials in electrochemical sensors,56 and analyze their mechanism of action and technical potential in improving the performance of heavy metal detection.
4. Mechanism and scope of nanomaterial selection for electrochemical sensors
In the process of detecting heavy metals by electrochemical sensors, the selection and application of nanomaterials are crucial,57 and their performance directly determines the sensitivity and selectivity of electrochemical sensors. By analyzing the adsorption, diffusion, and electron transfer processes of metal ions on the surface of nanomaterials,58 as well as the electronic properties of materials (such as conductivity and electron migration rate), we can deeply understand the influence of different nanomaterials on the detection performance and provide a scientific basis for material selection.
4.1 Mechanism of selection of nanomaterials
The selection of nanomaterials requires a comprehensive consideration of several factors:
1. Adsorption performance: the adsorption performance of metal ions on the surface of nanomaterials directly affects the sensitivity of detection. The specific surface area and surface chemical structure of the material (e.g., active site, functional groups) determine its adsorption capacity. For example, porous structures and functionalization can significantly enhance a material's ability to capture ions of interest.59
2. Electron transfer efficiency: an efficient electron transfer process is the key to achieving sensitive detection. The conductivity and electron migration rate of the material determine the transmission efficiency of the signal. Metal nanoparticles are often used as sensor modification materials due to their excellent electrical conductivity, while carbon nanomaterials and organic framework materials further optimize the electron transfer ability through structural design.60
3. Chemical stability and anti-interference ability: heavy metal detection in complex matrices requires materials with high chemical stability and anti-interference ability to ensure the accuracy of the signal. Functionalized nanomaterials can selectively bind to target metal ions through specific functional groups, thereby enhancing the selectivity of detection.61,62
4. Flexibility and adaptability: different heavy metal detection needs require different material properties, and the structural adjustability and easy functionalization of materials make them suitable for a variety of application scenarios.63
4.2 Selection range of nanomaterials
In the field of heavy metal detection, metal nanomaterials, carbon-based nanomaterials and organic framework materials have become hot spots in research and application due to their excellent physical and chemical properties.64 Metal nanomaterials are known for their high electrical conductivity and strong catalytic properties, which can effectively promote electrochemical reactions and enhance the sensitivity of sensors.65 Carbon-based nanomaterials exhibit excellent adaptability and anti-interference ability in complex matrices due to their excellent electron transport ability, structural tunability and stability.66 Organic framework materials, such as MOFs and COFs, offer innovative solutions for the selective capture and detection of heavy metals through their highly ordered porous structures and rich chemical functionality.67 Each of these three types of materials has its own advantages, such as adsorption, electron transfer, and selective detection, so it was selected as the focus of this study.
Metal nanomaterials play an important role in heavy metal detection due to their excellent conductivity and surface chemical activity. Gold nanoparticles (AuNPs) are widely used in sensor designs with high sensitivity requirements due to their high conductivity, chemical stability and oxidation resistance.68 Iron oxide nanoparticles (Fe3O4 NPs) not only have excellent adsorption capacity, but also facilitate separation and recovery due to their magnetic properties, which are suitable for the analysis of samples in complex environments.69 Cobalt oxide (Co3O4) exhibited good catalytic activity and electron transport properties, and had high selectivity in the detection of polymetallic systems.70
Carbon-based nanomaterials have become an important choice for electrochemical sensors due to their unique electronic properties and structural advantages. The one-dimensional structure of carbon nanofibers (CNFs) provides an efficient electron transport channel, and its strong mechanical properties and stability make it suitable for reusable sensors;71 Single-walled carbon nanotubes (SWCNTs) exhibit significant detection sensitivity due to their high specific surface area and excellent electrical conductivity.72 Multi-walled carbon nanotubes (MWCNTs) are easy to functionalize and are suitable for the development of multifunctional composite materials. Graphene materials exhibit excellent adaptability in complex detection environments due to their ultra-high conductivity and two-dimensional structure.73 Graphitic carbonitride (g-C3N4) enhances the adsorption capacity and detection selectivity of target ions through its porous structure and abundant surface functional components.74
Organic frame materials provide a new idea for heavy metal detection due to their highly adjustable structure and functionality. Metal–organic frameworks (MOFs) are outstanding in adsorption and selective detection due to their high specific surface area, regular pore channels and diverse surface functionality.75 Covalent organic frameworks (COFs) provide a reliable solution for heavy metal detection in complex systems due to their excellent chemical stability and electrical conductivity.76 In addition, these materials offer great flexibility in terms of functional design, enabling specific detection optimizations for different metal ions.
In the following sections, we will detail the properties of these nanomaterials and their latest research progress in heavy metal detection, demonstrating their practical applications in improving the performance of electrochemical sensors. These analyses not only provide a new direction for materials science research, but also lay the foundation for the design of high-performance heavy metal sensors.
4.3 Key parameters and research progress affecting detection performance
In electrochemical sensors, the performance of nanomaterials determines the sensitivity and selectivity of heavy metal detection. The key to designing an efficient sensor is to optimize the following parameters: specific surface area, conductivity, topography, detection sensitivity, and detection limit.77 These parameters directly affect the material's adsorption efficiency of heavy metal ions, electron transfer capacity, stability and anti-interference performance in complex environments.78
The specific surface area determines the number of active sites, and a larger specific surface area can significantly improve the adsorption capacity of heavy metal ions, such as porous MOFs and two-dimensional graphene,79 which excel in signal enhancement. Conductivity affects the efficiency of electron transport, and metal nanoparticles (such as AuNPs) and single-walled carbon nanotubes (SWCNTs) greatly improve the signal intensity due to their excellent conductivity. Topography Ion diffusion and electron transport pathways are improved by optimizing the nanostructure of the material, such as one-dimensional fibers, two-dimensional planar, and three-dimensional porous crystals, to further enhance detection performance.80 Functionalized modifications and material structure optimization also enhance detection sensitivity and selectivity, especially at low concentrations in complex matrices (Table 1).
Table 1 Performance of different nanomaterials on key parameters and latest research progress
Key parameters |
Metal nanomaterials |
Carbon-based nanomaterials |
Organic framework materials |
Specific surface area |
20–100 m2 g−1 |
50–300 m2 g−1 |
500–2000 m2 g−1 |
Electrical conductivity |
High |
Medium–high |
Medium–Low |
Appearance |
Spherical, porous |
Fiber, two-dimensional plane |
Porous crystals |
Detection sensitivity |
5.0–20.0 μA ppm−1 |
10.0–30.0 μA ppm−1 |
2.0–8.0 μA ppm−1 |
Limit of detection |
0.05–0.2 ppb |
0.1–0.5 ppb |
0.02–0.1 ppb |
The following table summarizes the performance of different nanomaterials on key parameters and the latest research progress:
These key parameters not only reveal the performance characteristics of different materials, but also provide an optimal direction for sensor design. The following chapters will discuss the latest research progress of metal nanomaterials, carbon-based nanomaterials, and organic framework materials in heavy metal detection, and provide specific guidance for the construction of high-performance electrochemical sensors.
5. Progress in the research of metal nanomaterials
Significant advancements have been made in the research on metal NPs for the electrochemical detection of heavy metals. Noble metal NPs such as Au, Ag, and Pt, as well as transition metals such as Fe, Ni, and Cu, have been extensively utilised in sensor development because of their excellent electrical conductivity, high surface area, and robust catalytic activity.81 These materials enhance the signal response of the sensor to heavy metal ions, thus lowering the detection limits and increasing sensitivity and selectivity.82 Researchers have successfully designed specific sensing platforms for several heavy metal ions by manipulating the shape, size,83 and surface functionalization of NPs. However, the practical application of metal NPs still faces challenges related to cost, stability, and interference in complex samples. Future research is required to improve the practicality and commercialisation of these sensors.
5.1 Introduction of gold nanomaterials
Gold NPs (AuNPs) are nanosized particles or structures composed of Au atoms.84 They stand out because of their remarkable chemical inertness and resistance to surface oxidation, which make them particularly favourable for heavy metal detection. In addition, AuNPs exhibit outstanding electrical and thermal conductivity,85 substantial specific surface areas, biocompatibility, and chemical stability. AuNPs are commonly used for environmental monitoring to detect heavy metal ions and noxious chemicals in water.86 AuNPs belong to a versatile class of nanomaterials and their unique properties make them crucial for various applications.
5.2 Application of gold nanomaterials in heavy metal sensing field
Wang et al.87 prepared L-cysteine functionalized MOF composites with GO and AuNPs, exhibiting a porous structure and good dispersion (Fig. 3(a)). This material showed significantly higher electrochemical signals compared to other materials. Using DPV, they detected Cd2+ and Pb2+ with detection limits of 20.8 μg L−1 and 17.3 μg L−1, respectively, demonstrating a good linear range (Fig. 3(g–i)). Similarly, Yu et al.88 synthesized COF@AuNPs@GR/GCE composites with spherical structures and highly dispersed nanoparticles through reduction reactions and ultrasonic treatments (Fig. 3(b)). They employed DPASV to detect Cd2+, Pb2+, and Cu2+, achieving detection limits of 3.025 μg L−1, 5.849 μg L−1, and 9.993 μg L−1, respectively, also with good linear ranges (Fig. 3(k–n)).
 |
| Fig. 3 (a) Assembly diagram of AuNPs/APTES-ITO and mechanism diagram for detecting heavy metal ions. (c–d) SEM images of MOFs and L-Au-MOFs-GO. (g) Comparison of DPV curves of five kinds of electrodes. (h–i) Standard curves for the L-Au-MOFs-GO sensor in the separate detection of Cd2+ and Pb2+. (j) Standard curves for the simultaneous detection of Cd2+ and Pb2+ by the L-Au-MOFs-GO sensor.87 (b) The synthesis process of COFDPTB@AuNP@GR and the preparation procedure of electrochemical sensors. (e and f) SEM image of COF DPTB and TEM image of COFDPTB@AuNPs. (k–m) Relative standard curves for the separate detection of Cd2+, Pb2+, and Cu2+. (n) Relative standard curves for the simultaneous detection of Cd2+, Pb2+, and Cu2+ using COFDPTB@AuNPs@GR/GCE. Reproduced with permission from ref. 88,89. | |
Both materials were synthesized through complex processes, with their morphologies confirmed by SEM and TEM images. The first material (Fig. 3(c–f)) displayed a porous structure, increasing the specific surface area, while the second material (Fig. 3(e–f)) exhibited a regular spherical structure, enhancing dispersion and stability. Both materials performed exceptionally well in electrochemical detection, with DPV and DPASV results indicating high sensitivity and selectivity for heavy metal ions such as Cd2+, Pb2+, and Cu2+. The first material provided more comparative data across different modified electrodes (e.g., GO/GCE, AuNPs/GO/GCE), whereas the second material presented a simpler yet equally effective detection outcome.
These materials show great potential in heavy metal ion detection, particularly in environmental monitoring and food safety. Their porous structures and high dispersion can significantly improve detection limits and response speeds, paving the way for the development of high-performance, portable electrochemical sensors in the future.
5.3 Introduction of iron oxide nanomaterials
Iron oxide has stable chemical properties that make it resistant to self-chemical reactions or dissolution,89 enabling sustained stability over prolonged use in heavy metal detection. Moreover, it is relatively nontoxic, cost-effective, and easy to prepare, making it environmentally friendly and safe for various applications, such as water quality testing,90 without adversely affecting ecosystems. Manipulation of the shape, size, and crystal structure of iron oxide NPs allows for the tuning of their properties, enabling them to meet diverse heavy metal detection requirements. Additionally, they possess a high adsorption capacity,91 and effectively capture and enrich heavy metal ions, thereby enhancing detection sensitivity.92 Iron oxide NPs can be integrated with various detection techniques,93 such as ECS and adsorptive voltammetry, demonstrating their potential for use in diverse heavy-metal detection methods.
5.4 Application of iron oxide nanomaterials in heavy metal sensing field
Zhang and Guo utilized the sol–gel method, with TEOS as the silicon source, to form a SiO2 layer on Fe3O4 magnetic nanoparticles.94 These particles were then exposed to NaOH solution to create a porous structure, facilitating heavy metal ion adsorption (Fig. 4(a)) Once modified onto the electrode, DPASV was used to detect heavy metal ions, achieving detection limits between 56.7 ppb and 79.4 ppb, and demonstrating good selectivity (Fig. 4(b and c)) his method is cost-effective, requires minimal sample pretreatment, and is simple to operate, making it suitable for routine rapid detection. However, its detection limits may not be ideal for applications requiring ultra-low detection limits.
 |
| Fig. 4 (a) Schematic of the synthesis process and application of the Fe3O4@SiO2 sensor. (b) Differential pulse and adsorptive stripping graphs of the Fe3O4@SiO2 sensor for the detection of four different heavy metal ions. (c) The stability or current variation trend of four ions over multiple cycles.94 (d and e) Schematic of the synthesis process and application of the trGNO/Fc-NH2-UiO-66 sensor. (f–i) DPASV curves and calibration plots for the detection of heavy metal ions using the trGNO/Fc-NH2-UiO-66 sensor.95 Reproduced with permission from ref. 95,96 | |
Wang et al.,95 synthesized materials using a solvothermal method with terephthalic acid (H2BDC) and ZrCl4 as precursors. Through an EDC/NHS coupling reaction, Fc (ferrocene) was linked to NH2-UiO-66, forming Fc-NH2-UiO-66 (Fig. 4(d and e)) This method enhanced detection sensitivity, with detection limits as low as 8.5, 0.6, and 0.8 ppb, respectively (Fig. 4(f–i)), By retaining the straightforward operational steps from Zhang and Guo's method, reducing sample pretreatment time, and ensuring a fast detection process, Wang et al.'s method can be simplified for practical applications. Combining the selectivity and anti-interference capabilities of both methods allows for accurate detection of specific heavy metal ions in complex matrices. Developing a comprehensive detection method that is low-cost, simple to operate, highly sensitive, and highly selective will significantly enhance detection efficiency and broaden application scope.
5.5 Introduction of cobalt oxide nanomaterials
Because of their excellent chemical properties,96 cobalt oxides (CoO and Co3O4) have been used for the detection of heavy metals.97 CoO and Co3O4 have been employed for the electrochemical detection of heavy metal ions in water.98 They are frequently used as electrode materials in sensors because of their outstanding electrical conductivities and advantageous catalytic performance.99 This demonstrates the outstanding sensitivity of the sensor for the detection of heavy metals. Cobalt oxide sensors are widely used for environmental monitoring because of their stability and sensitivity.100 These sensors help monitor heavy metal pollution in various water sources, assess industrial emission controls,101 and improve wastewater treatment. CoO and Co3O4 are crucial for heavy metal detection, ensuring reliable, precise, and selective ion detection in water, and contributing to protecting the environment and promoting human health.102
5.6 Application of cobalt oxide nanomaterials in heavy metal sensing field
Zhu et al.103 and Wang et al.104 combined metal oxides with other nanomaterials to design high-sensitivity electrodes for trace-level heavy metal detection in water. Given the significant health risks posed by water and heavy metal contamination to humans and the environment, such advancements are critical. A primary advantage of these materials is their low detection limits and high sensitivities, which ensure accurate measurements even at minute concentrations (Fig. 5(b–g)). Specifically, the use of the CoFe2O4–CoFe microsphere structures and the semiconductor and binary transition metal oxide blend in the pg-C3N4/CoMn2O4 remarkable progress has been made in the field of heavy metal ion detection using Co and its oxides (Fig. 5(a)). Nanocomposite demonstrates the potential of integrating diverse materials to enhance the electrode performance. However, practical application of these sensors in real-world scenarios is vital. Although laboratory results are promising, evaluating the performance of a sensor under complex environmental conditions is crucial for ensuring reliability and consistency. Additionally, with the improvements and enhancements to these sensors, researchers should also consider factors such as the ease of production, cost efficiency, and long-term stability of the electrodes. As water pollution is a global issue, developing scalable and affordable solutions will ensure that these innovations can be widely implemented, particularly in regions that lack access to sophisticated monitoring tools. In conclusion, the development of advanced nanomaterial-based electrodes is a significant step towards ensuring clean drinking water. Through continuous research and innovation, we look forward to a future in which heavy metal water contamination can be effectively monitored and controlled.
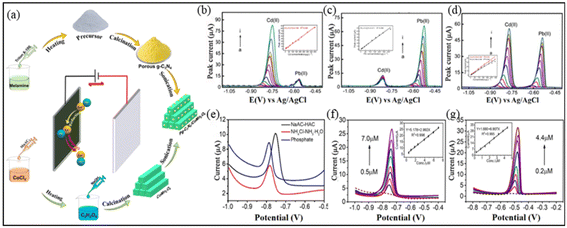 |
| Fig. 5 (a) Synthesis process of g-C3N4/CoMn2O4. (e) Effect of electrolyte on g-C3N4/CoMn2O4 sensor. (f and g) Relative standard SWASV curves of g-C3N4/CoMn2O4 sensor for the detection of Cd2+ and Pb2+.103 (b–d) Relative standard DPASV curves of CFO–CF/GCE sensor for the detection of Cd2+ and Pb2+.104 Reproduced with permission from ref. 104,105. | |
5.7 Introduction to other metal nanoparticles
In addition to the three types of metal NPs mentioned above, AgNPs, CuNPs, PtNPs, NiNPs, and PdNPs have also been used in the detection of heavy metals. AgNPs have excellent electrical conductivity and antimicrobial properties; however, they are prone to oxidation and have less stability compared with AuNPs. CuNPs exhibit good catalytic effects in certain electrochemical reactions and are generally used in conjunction with other nanomaterials for the detection of heavy metals. The remaining concentrated NPs have their own advantages and drawbacks, and continuous improvements and combinations with other materials are necessary to enhance the performance of the sensors. By adjusting the size, shape, composition, and surface modifications of the nanomaterials, sensors can be optimised for sensitivity and selectivity towards specific heavy metal ions. Table 2 displays the performance characteristics of the three most commonly used metal ions.
Table 2 Properties of modified materials for electrochemical heavy metal sensing
Electrode materials |
Heavy metal ions |
Technique |
LOD |
Linear range |
Samples |
Ref |
Abbreviations: glassy carbon electrodes (GCE), screenprinted electrode (SPCE) Three-dimensional gold nanowire (AuNW), carbon paste screen-printed electrodes (SPEs), L-cysteine (Lcyst), reduced lipoic acid (rLA), graphene/gold nanoparticles/modified L-cysteine nanocomposite (PrGO/AuNPs/Sal-Cys), Chitosan (CS), graphene modified glassy (GR), gold nanotextured electrode (Au/GNE), fluorinated multi-walled carbon nanotubes (F-MWNTs), biomass-derived porous carbon (BPC), nanoscale zero-valent iron (nZVI), second-generation polyamidoamine dendrimer (G2-PAD), ion-imprinted polymer (IIP), nanocubes (NC), [Co2(TMC4R)(1,4-BDC)2(μ2H2O)]·3DMF·CH3OH·5CH3CN·H2O (Co-TMC4R-BDC), ntrogen-doped reduced graphene oxide (N-RGO), nanopolyhedron (NP). |
AuNWs/SPE/GCE |
Pb |
SWASV |
4.1 (mg L−1) |
10–300 (mg L−1) |
Tap water |
105
|
|
As |
SWASV |
4.9 (mg L−1) |
|
Hg |
SWASV |
2.7 (mg L−1) |
AuNP-rLA-Lcyst |
As |
SWASV |
3.33 ppb |
3–25ppb |
Tap water |
106
|
AuNPs/MWC/GCE |
Cd |
CV |
0.02 ppm |
10−13–10−4 M |
River |
107
|
PrGO/AuNPs/Sal-Cys/GCE |
Cd |
SWASV |
2.2 μA nM−1 |
0.01–0.1 μg L−1 |
Groudwater, Tap water, Sewage water |
108
|
|
Pb |
|
3.2 μA nM−1 |
CS/AuNPs/GR/GCE |
Cd |
CV |
1.62 × 10−4 μM |
0.1–0.9 μm |
Pure milk River water Tap water |
109
|
AuNPs/mesoporous NiO/nickel foam/GCE |
Pb |
SWV |
0.020 mg L−1 |
2.0–16.0 mg L−1 |
Groundwater |
84
|
|
Cu |
SWV |
0.013 mg L−1 |
0.4–12.8 mg L−1 |
Groundwater |
Au/GNE |
As |
ASV |
0.083 ppb |
0.1 ppb–9 ppb |
Tap water |
110
|
Fe3O4/F-MWCNTs/GCE |
Cd |
DPV |
0.05 nM |
0.5–30.0 μm |
Soybean River water |
111
|
|
Pb |
|
0.08 nM |
0.5–30.0 μm |
|
Cu |
|
0.02 nM |
0.5–30.0 μm |
|
Hg |
|
0.05 nM |
0.5–20.0 μm |
BPC/nZVI/GCE |
Cd |
SWSV |
0.1926 μg L−1 |
2.0–50 μg L−1 |
Tap water |
112
|
|
Pb |
|
0.2082 μg L |
|
Fe3O4@G2-PAD/GCE |
Pb |
SWASV |
0.17 ng mL−1 |
0.5–80 ng mL−1 |
Waster |
113
|
|
Cd |
|
0.21 ng mL−1 |
|
Bi2O3/Fe2O3@GO/GCE |
Cd |
SWV |
1.85 ng L−1 |
6.2–1160.2 ng L−1 |
Water, fruits, soil |
114
|
Fe-Chitosan-coated carbon electrode sensor/GCE |
As |
SWASV |
1.01 ppb |
1.12 ppb–1.01 ppb |
Wastewater |
115
|
Fe3O4@SiO2@IIP |
Pb |
CV |
0.05 ng mL−1 |
0.1–80 ng mL−1 |
Fruit juice Rain water |
116
|
BiNPs@CoFe2O4/GCE |
Pb |
SWASV |
7.3 nM |
0.08–0.6 mM |
Tap water |
117
|
|
Cd |
|
8.2 nM |
|
Co3O4 nanocrystals/rGO/GCE |
Cd |
SWASV |
0.062 ppb |
0.1–450 ppb |
Pool water Well water |
118
|
|
|
|
0.034 ppb |
|
Co3O4-NC/SPCE |
Pb |
DPV |
4.8 μM |
0.01–8.38 μM |
Tap water, Pond water |
119
|
|
Cu |
|
0.5 μM |
0.002–20.22 μM |
|
Hg |
|
0.9 μM |
0.001–69.38 μM |
Co3O4/GO |
Cd |
DPASV |
57 nM |
1.4–6.4 μ m |
Tap water |
120
|
|
Pb |
|
29.4 nM |
0.3–6.4 μm |
Co-TMC4R-BDC/GCE |
Pb |
SWASV |
13 nM |
0.05–12.0 μm |
Tap water |
32
|
|
Cu |
|
11 nM |
0.05–13.0 μm |
|
Cd |
|
26 nM |
0.1–17.0 μm |
|
Hg |
|
18 nM |
0.75–18.0 μm |
Co3O4/GCE |
As |
SWASV |
0.79 ppb |
1–20 ppb |
Tap water |
121
|
AuNPs/Co3O4/SPCE NP/N-RGO |
Hg |
SWASV |
30.00 μM |
0.10–1.00 μM |
Natural water Serum |
122
|
Co3O4/ZnO |
Cd |
CV |
0.49 ng ml−1 |
0.2–015 ng mL−1 |
Tap water, River water |
123
|
6. Progress in the research of carbon nanomaterials
Carbon nanomaterials have achieved significant research progress in the electrochemical detection of heavy metals.124 These materials, including carbon nanotubes (CNTs), graphene,125 and activated carbon, have become popular choices for enhancing the performance of ECS owing to their unique conductivity, high surface area, and excellent chemical stability.126 They improve the sensitivity of the sensors, have lower detection limits, and possess good selectivity, making the detection process quicker and more accurate. In particular, by functionalizing or combining them with other materials, the recognition and affinity of carbon nanomaterials can be tailored for specific heavy metal ions. Additionally, the application of carbon nanomaterials in sensor fabrication helps to reduce costs and promote environmental monitoring and public health protection. Nonetheless, achieving long-term stable detection performance in actual complex samples as well as conducting large-scale production and commercial applications remains a challenge that current research needs to focus on.
6.1 Introduction to carbon nanofiber nanomaterials
Carbon nanofibers (CNFs) are one-dimensional carbon nanomaterials,127 characterized by excellent crystalline orientation, high thermal conductivity, and superior electrical conductivity. These attributes make them exceptionally performant in various domains, particularly in applications requiring high strength and thermal resistance. Beyond the fundamental advantages of ordinary carbon fibers,128 such as lightweight, high strength, and chemical stability, CNFs exhibit even more remarkable properties. For instance, they demonstrate enhanced stability under high-temperature conditions and high efficiency in electronics and energy storage devices.129,130 However, the application of CNFs also faces certain challenges and limitations. For example, their production costs are relatively high, which may limit their widespread adoption in commercial and industrial applications. Moreover, the processing and utilization of carbon nanofibers require specialized techniques and equipment, potentially increasing their overall usage cost. In terms of environmental and health considerations, CNFs, like other nanomaterials, need to be handled with care to prevent potential issues related to the release of nanoparticles. Despite these challenges, their unique advantages in the field of material science make them a focal point of research and development.
6.2 Application of carbon nanofibers in the heavy metal sensing field
Electrospinning is frequently employed in the preparation of CNFs in materials science because of its rapidity and superior performance. Zhang et al. further validated the practicality of this method.131 The fusion of CNFs produced via electrospinning with PtAu introduces an innovative film material for electrochemical sensors. This composite not only amplifies the electrical conductivity of the electrode, but also offers high sensitivity and a broad linear range for detecting heavy metal ions. Moreover, the sensor exhibits exceptional sensitivity and linear range for detecting Cd(II), Pb(II), and Cu(II). Such characteristics are pivotal in several real-world applications to ensure the accurate and rapid detection of heavy metals at varying concentrations. In conclusion, the work of Zhang et al. plays a significant role in environmental pollution and health risk monitoring. They successfully demonstrated that, through electrospinning and composite material fabrication methods, more efficient and sensitive sensors can be designed to address current and future challenges.
6.3 Introduction to carbon nanotube nanomaterials
CNTs are porous carbon nanomaterial that possess a unique hexagonal arrangement in the form of concentric circular tubes.132 Owing to their large specific surface areas and excellent mechanical structures, CNTs are frequently combined with other metallic materials for heavy metal detection.133 These composite nanomaterials exhibit excellent stability and sensitivity.
6.4 Application of single-walled carbon nanotubes in heavy metal sensing field
Recent advancements in the use of single-walled carbon nanotubes (SWCNTs) for the electrochemical detection of heavy metal ions have shown significant innovation and potential for wide-ranging applications. Functionalizing SWCNTs with various groups (e.g., carboxyl, amino) has greatly enhanced sensor selectivity and sensitivity by improving metal ion adsorption and electron transport properties.134 Additionally, combining SWCNTs with other materials such as metal–organic frameworks (MOFs), nanoparticles, and polymers has led to the creation of multifunctional composites capable of efficiently detecting multiple heavy metal ions.
Flexible and wearable sensors incorporating SWCNTs with substrates like PDMS and PET have been developed, providing bendable and stretchable sensors that are portable and suitable for real-time monitoring of environmental heavy metal contamination.135 Enhanced electrochemical detection methods, such as differential pulse voltammetry (DPV) and square wave voltammetry (SWV), when combined with the superior properties of SWCNTs, have significantly increased detection sensitivity and accuracy, allowing for the detection of very low concentrations of heavy metal ions.
SWCNT-based electrochemical sensors have demonstrated excellent performance in real-world samples, such as river water, soil, and food, highlighting their broad application potential in environmental monitoring and food safety.136 These advancements indicate that future research on SWCNTs in electrochemical sensors will continue to drive improvements in heavy metal pollution detection technologies (Fig. 6).
 |
| Fig. 6 Progress of single-walled carbon nanotube electrochemical sensors in the field of heavy metal detection. | |
6.5 Introduction of multi-walled carbon nanotube nanomaterials
MWCNTs can be regarded as multilayer, single-walled tubes arranged concentrically.137 They are synthesised by chemical vapour deposition at 900 °C.138 and exhibit good mechanical properties, unique electrical conductivity, good adsorption, and excellent electron-transfer abilities.139 Consequently, they are excellent nanomaterials for heavy-metal detection.
6.6 Application of multi-walled carbon nanotubes in heavy metal sensing field
Dong et al.140 developed a flexible sensor using carbon cloth (CC) decorated with MWCNTs and CeMOF, forming a CeMOF@MWCNTs/CC composite (Fig. 7(a)). This sensor demonstrated high sensitivity, with detection limits of 2.2 ppb for Cd2+ and 0.64 ppb for Pb2+, and was successfully applied for on-site detection in food and water samples (Fig. 7(c–e)). Zhao et al.,141 created a sensor using an MOF-derived carbon composite linked with MWCNTs Fig. 7(b), which also exhibited excellent linearity and low detection limits, achieving high accuracy and reliability in real sample tests (Fig. 7(f–h)). Both studies introduced significant innovations in material composition and sensor performance. Dong et al.'s one-pot hydrothermal synthesis simplifies the preparation process and improves reproducibility, emphasizing practical convenience and cost-effectiveness. In contrast, Zhao et al. enhanced sensor conductivity and stability using MOF-derived carbon composites, focusing on performance optimization and detection accuracy.
 |
| Fig. 7 (a) Schematic of the preparation process for the CeMOF@MWCNTs/CC sensor and the principle of heavy metal ion detection and identification. (c–e) DPASV curves and corresponding calibration curves for the detection of heavy metals by the CeMOF@MWCNTs/CC sensor.140 (b) Construction of the Co@NC/MWCNT sensor and the principle of heavy metal ion detection and identification. (f–h) DPASV curves and corresponding calibration curves for the detection of heavy metals by the Co@NC/MWCNT sensor.141 Reproduced with permission from ref. 141,142. | |
Future research should tailor these approaches to specific applications, leveraging the unique strengths of each method. This targeted development will further advance the detection and management of heavy metal pollution, maximizing the impact of these innovative sensor technologies.
6.7 Introduction of graphene nanomaterials
Graphene is a carbon material with sp2 hybrid connections and a two-dimensional honeycomb lattice structure.142 It has extremely high electrical conductivity, excellent mechanical strength, and stable chemical properties.143 It was originally synthesised via SIC epitaxy by British University professors Andre Geim and Konstantin Novolov in 2004. Over the years, various graphene preparation methods and forms have emerged. Different forms of GO, including rGO and methylene carbon nitride,144 possess distinct chemical properties. These graphene materials exhibit outstanding detection capabilities for heavy metal substances.
6.8 Application of graphene in heavy metal sensing field
Graphene nanosheets, paired with sulfur-doped C3N4, have proven their efficiency in detecting trace amounts of heavy metals, as showcased by Wang et al.145 In another study conducted by Pang et al.,146 a multifunctional ECS capable of detecting heavy metal ions and facilitating the hydrogen evolution reaction was designed. They prepared NiCo2O4-modified N,S-rGO-doped reduced GO composites via a hydrothermal calcination method. The ECS developed from this material exhibited sensitivities of 2.38 μA μm−1 for Cd(II), 10.90 μA μm−1 for Cu(II), and 5.41 μA μm−1 for Hg(II). The detection limits for Cd(II), Cu(II), and Hg(II) were 123, 14.4, and 67 nM, respectively. The sensor possessed robust interference resistance and could effectively detect heavy metal ions in river water, demonstrating exceptional performance in real-world applications.
6.9 Introduction of graphite carbon nitride nanomaterials
Graphitic carbon nitride (g-C3N4) holds significant promise in the field of electrochemistry, particularly in the detection of heavy metals,147 food safety, and health monitoring. g-C3N4 can serve as a sensor material owing to its surface rich in functional groups that can form specific interactions with heavy metal ions.148 This characteristic enables it to detect trace heavy metal ions, such as Pb, Cd, and Hg.149 g-C3N4 provides an efficient method for monitoring and removing heavy metal pollution in heavy metal detection.150 This has a substantial impact on ensuring environmental safety.
6.10 Application of graphite carbon nitride in heavy metal sensing field
Wang et al.151 developed a sensor based on a composite of polyaniline (PANI) and g-C3N4, optimized for high-sensitivity detection of cadmium(II) ions in water. The sensor demonstrated a detection limit of 0.05 μg L−1 and a sensitivity of 169.36 μA μM−1 cm−2, with a linear range from 0.1 to 140 μg L−1. The multilayer design, incorporating rGO and CNT layers, significantly enhanced detection performance and electron transport, indicating its potential for environmental water quality monitoring (Fig. 8(h–l)).
 |
| Fig. 8 (a) Preparation and detection principle of PANI@g-C3N4 Sensor. (d) DPASV Curves for Cd2+ detection by PANI@g-C3N4 Sensor. (e) Calibration Curve for Cd2+ detection by PANI@g-C3N4 sensor (f) stability test of PANI@g-C3N4 sensor over 15 days.151 (c) Synthesis and detection principle of the V2O5/gCN Sensor. (g) DPASV curves for Hg2+ detection by V2O5/gCN sensor(h) calibration curve for Hg2+ detection by V2O5/gCN sensor. (i) Reproducibility test of the V2O5/gCN sensor across different electrodes.152 (b) Preparation and detection principle of S-g-C3N4/GCE sensor. (j) DPASV curves for Hg2+ detection at low concentrations by S-g-C3N4/GCE sensor. (k) DPASV curves for Hg2+ detection at high concentrations by S-g-C3N4/GCE sensor. (l) Response time test for S-g-C3N4/GCE sensor.153 Reproduced with permission from ref. 152–154. | |
In contrast, Vasu et al.152 developed an electrochemical sensor using doped V2O5 and g-C3N4, primarily for mercury ion detection (Fig. 8(a)). This sensor exhibited excellent performance in food testing, with a detection limit of 9.2 nM and effective voltammetric response across concentrations from 0.2 μM to 100 μM (Fig. 8(d and e)). The design emphasized a large surface area and high electrochemical activity, enhancing sensitivity and detection accuracy (Fig. 8(f)), making it particularly suitable for food safety applications.
In contrast, Zou et al.,153 developed a sulfur-doped g-C3N4 nanoflake-modified glassy carbon electrode (S-g-C3N4/GCE) for lead ion (Pb2+) detection (Fig. 8(b)). This sensor achieved a detection limit of 3.0 × 10−9 mol L−1, demonstrating high selectivity and sensitivity (Fig. 8(g–i)). The strong S–Pb bond formed between S-g-C3N4 and Pb2+ ions enhanced electrochemical response (Fig. 8(c)), allowing stable detection across concentration ranges of 7.5 × 10−8 to 2.5 × 10−6 mol L−1 and 2.5 × 10−6 to 1 × 10−3 mol L−1, proving effective in complex environmental sample analysis (Fig. 8(j–l)). Overall, each study presents distinct innovations in heavy metal ion detection: Wang et al.'s sensor excels in sensitivity and detection limits, suitable for water quality monitoring; Vasu et al.'s sensor offers high sensitivity and a large surface area, ideal for food safety testing; Zou et al.'s sensor provides exceptional selectivity and low detection limits, suitable for complex environmental samples. These studies highlight the substantial potential of nanomaterials in sensor design, providing new methods and insights for developing efficient, precise environmental monitoring tools.
In my opinion, the outstanding performance of these sensors in various applications validates the advantages of nanocomposite materials in enhancing sensor capabilities. Future research should focus on further optimizing the synthesis and integration of these materials to expand their applicability and address more complex detection needs. The development of these innovative sensors undoubtedly offers crucial support for environmental monitoring and public safety (Table 3).
Table 3 Properties of modified materials for electrochemical heavy metal sensing
Electrode materials |
Heavy metal ions |
Technique |
LOD |
Linear range |
Samples |
Ref. |
Abbreviations: bismuth particles (BiPs),1-ethyl-3-methylimidazolium bis (trifluoromethylsulfonyl) imide [EMIM][NTf2], carbon fiber paper (CFP), glutathione as the conductive substrate (GSH), antimony oxide (Sb2O3), cerium oxide catalyzed 1-D carbon nanofibers (Ce-CNFs), highly densified carbon nanotube fiber (HD-CNTf), polyaniline (PANi), graphene aerogel (GA), laser-induced graphene (LIG), furfural (FF), cellulose nanowhiskers (CNW) and polyamide 6 (PA6), graphene/gold nanoparticle-hybrid (AuNPs/ERGO). |
BiPs-CNFs/[EMIM][NTf2]/CPE |
Pb |
SWASV |
0.12 μg L−1 |
2–120 μg L−1 |
Well water |
154
|
|
Cd |
|
0.25 μg L−1 |
CFP/CoMOF/AuNPs/GSH |
Cd |
SWV |
1 nM |
0.001–1 μm |
Yangtse River, Mingzhu Lake |
155
|
Sb2O3/MWCNTs |
Cd |
LSASV |
11.23 ppb |
80–150 ppb |
Tap water |
156
|
|
Pb |
|
2.68 ppb |
5–35 ppb |
Ce-CNFs/GCE |
Pb |
DPV |
0.6 ppb |
0.9–2.1 ppb |
Tap water |
157
|
|
Cu |
|
0.3 ppb |
0.6–1.8 ppb |
HD-CNTf/GCE |
Cu |
SWASV |
6.0 nM |
0.016–7.86 mM |
Tap water |
158
|
|
Pb |
|
0.45 nM |
|
Cd |
|
0.24 nM |
PANi-Bi NPs@GO-MWCNT |
Hg |
DPV |
0.01 nmol L−1 |
0.01–5 × 106 |
Tap water |
159
|
CoMn2O4/CNT |
Pb |
SWASV |
0.004 μm |
0.01 μm–0.85 μm |
Tap-water drinking water |
160
|
N-CNTs/GCE |
Pb |
SWV |
0.001 mM |
0.01–70 μm |
Tap water ground water |
|
Cd |
|
0.005 mM |
0.1–100 μm |
|
Hg/CMWCNTs@ZIF-8/GCE |
Zn |
DPSV |
5.23 × 10−3 mg L−1 |
0.05–1 mg L−1 |
Apple juice Grape juice Kiwi juice |
161
|
|
Cu |
|
6.52 × 10−3 mg L−1 |
AuNPs-SWCNTs |
As |
CV |
1.35 × 105 μm |
1.35 × 10−5–1.35 μm |
— |
162
|
GA-UiO-66-NH2/GCE. |
Pb |
DPSV |
1 nM |
0.01–1.6 μM |
River water soil vegetable |
163
|
|
Cu |
|
8 nM |
0.001–2 μM |
|
Hg |
|
0.9 nM |
0.001–2.2 μM |
|
Cd |
|
9 nM |
0.001–2 μM |
GR/GO/GCE |
Cd |
DPV |
0.087 μM |
0–10 μM |
Tap Water |
164
|
Ni-Fe/LIG |
Pb |
SWASV |
0.771 μg L−1 |
20–1200 μg L−1 |
Tap Water |
165
|
FF/RGO |
Cd |
SWASV |
2 ng ml−1 |
0.01–8.5 μg mL−1 |
Lake water vegetable |
166
|
|
Pb |
LSV |
12 ng ml−1 |
0.05–10 μg mL−1 |
|
Cu |
|
3 ng ml−1 |
0.01–8 μg mL−1 |
|
Hg |
|
20 ng ml−1 |
0.07–10 μg mL−1 |
PA6/CNW : rGO |
Hg |
DPV |
5.2 nM |
2.5–200 μM |
Tap Water |
167
|
GrapheneBrominated White PANi Flakes |
Cd |
DPV |
0.0065 μM |
0.01–0.23 μM |
Tap Water Mineral water Industrial wastewater Plasma |
168
|
|
Pb |
|
0.0073 μM |
0.01–0.18 μM |
GC/rGO-SbNPs |
Cd |
SWASV |
70.03 nmol L−1 |
0.1–3.0 μmol L−1 |
chamomile tea |
169
|
|
Pb |
|
45.50 nmol L−1 |
|
AuNPs/ERGO/GCE |
Hg |
DPASV |
0.06 μg L−1 |
0.5–20 mg L−1 |
Tap water |
170
|
PEDOT/10 wt% gC3N4/GCE |
Cd |
DPV |
0.0014 μM |
0.06–12 μM |
Wastewater |
171
|
|
Pb |
|
0.00421 μM |
0.04–11.6 μM |
g-C3N4/MnWO4/GCE |
Hg |
DPV |
1.03 μM |
0.004–0.071 μM |
Milk Human blood serum Sewage samples River samples |
172
|
g-C3N4/GCE |
Mg |
DPV |
0.05 μM |
2–1208 μM |
River water |
173
|
7. Advancements in organic framework materials
Organic framework materials typically have crystalline structures with highly ordered arrangements, including micropores, cavities, or channels.174 These materials have widespread applications in chemistry, physics, and materials science and play crucial roles in adsorption,175 separation, energy storage, and catalysis. The research and development of organic framework materials are continually expanding,176 with scientists continuously designing new molecular structures to meet the demands of various applications.177 The tunability and multifunctionality of these materials endow them with significant potential across various domains.178
7.1 Progress in metal–organic framework research
Metal–organic frameworks (MOFs) are gaining traction in electrochemical heavy-metal detection owing to their customisable porous structures and chemical features. The high surface area and versatility of MOFs make them ideal for capturing and catalysing heavy metal ions, with selective detection achievable through tailored functional groups. Research has highlighted the effectiveness of MOFs for adsorbing heavy metals from water, enabling rapid and accurate environmental monitoring using electrochemical methods. Despite challenges such as stability, cost, and reusability, ongoing studies aim to refine MOFs for better performance and affordability in heavy-metal assays. Advancements in synthesis methods and nanotechnology have expanded the use of MOFs for the detection of environmental pollutants, indicating a promising evolution of these materials in ecological monitoring applications.
7.2 Introduction of MOF materials nanomaterials
As a novel class of porous crystalline materials, MOFs have been extensively applied in various fields.179 These applications include, but are not limited to, energy storage, catalysis, drug delivery, and sensing.180 The synthesis of MOFs is accomplished through a bottom-up approach, wherein metal and organic molecules are synthesised by a range of techniques, such as hydrothermal, electrochemical, and mechanical techniques.181 The synthesis of MOF requires controlled directional growth. Depending on the synthesis conditions, MOF materials exhibit various crystal structures that can be broadly categorised into hexagonal, cubic, tetragonal, monoclinic, and triangular types.182 These crystals can form one-, two-, or three-dimensional network structures. MOFs display a rich diversity in their crystal structures, resulting in distinct properties. These materials possess uniform pore structures, substantial porosities, adjustable structures, chemical versatility, and large specific surface areas.183 Furthermore, MOFs with distinct crystal structures exhibit excellent adsorption properties owing to their unique morphologies and specialised crystal structures. This diversity and versatility make MOFs highly promising for application in the detection of heavy metal ions.
7.3 Application of MOFs in heavy metal sensing
Several researchers have employed MOFs to monitor and mitigate heavy-metal contamination. However, the chemical stability of MOFs in water is poor. Nevertheless, scientists continue to conduct research to enhance their performance, thereby paving the way for future applications in the prevention and control of heavy metals. Owing to its simple operation, high sensitivity, and rapid response, this material has significant potential.184
In 2023, Huang et al.185,186 developed two zeolitic imidazolate framework-8 (ZIF-8)-based materials for detecting heavy metal ions in food. The BNSP-C material, doped with non-metal atoms (B, N, S, and P) (Fig. 9(a and b)), exhibited high sensitivity for Cd(II) and Pb(II) (1.290 and 0.6611 μA μm−1 cm−2, respectively) and a large surface area (755.58 m2 g−1), providing ample active sites for adsorption and detection (Fig. 9(c–e)). It also demonstrated excellent stability and repeatability. However, its complex preparation process may increase cost and time. In contrast, the BCN material, with a lower detection limit (0.41 and 0.93 μg L−1 for Cd(II) and Pb(II), respectively) (Fig. 9(f-h)), excelled in detecting ultra-low concentrations of heavy metals and showed good electrochemical reactivity and high repeatability, despite having lower sensitivity and surface area. Overall, BCN material is advantageous for ultra-low concentration detection, while BNSP-C material is preferable for high sensitivity and higher concentration ranges. Material selection should be based on specific detection needs, considering preparation costs and complexity. Future research should focus on optimizing the performance and preparation processes of these materials and exploring combinations with other dopants or nanomaterials to enhance sensor capabilities.
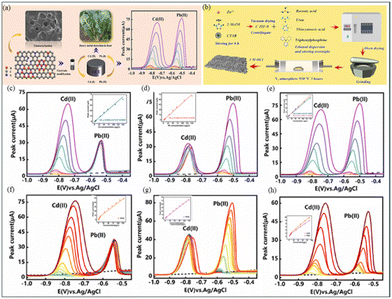 |
| Fig. 9 (a) SEM image of the BCN-Nafion, electrode modification diagram, and schematic of heavy metal ion detection. (c–e) Square wave anodic stripping voltammetry (SWASV) response curves of BCN-Nafion/GCE.185 (b) Synthesis diagram of BNSP-C. (f–h) SWASV curves of BNSP-C-Nafion sensor for the individual and simultaneous detection of heavy metal ions and their corresponding calibration curves.186 Reproduced with permission from ref. 186,187. | |
7.4 Covalent organic framework composites
Covalent organic frameworks (COFs) are highly regarded because of their strong covalent interactions and adjustable porosity,187 and are based on the geometric space topology material design theory. Compared with traditional inorganic materials, COF structures exhibit good functional adjustability.188 COFs and diverse range of COFs materials primarily include imines, triazines,189 among others. Owing to their stable structure and covalent bonding, COFs have large specific surface areas, thermochemical stabilities, high porosities, low skeleton densities, controllable chemical and physical properties, and numerous contact sites.190 COF materials are widely used in fields such as gas separation and storage, environmental, and energy, and are mainly composed of light elements (C, H, O, N, and B).191 In 2005, Yaghi192 reported the discovery of COFs and provided evidence of their adsorption properties. At the molecular level, COFs are connected by (B–O) O, (C
N), (C–N), and (C
C) bonds. COFs materials can be broadly classified into two categories: two-dimensional and three-dimensional structures. The structures of these materials are comparable to those of graphite and diamond. From this, we can understand that COF materials have regular one-dimensional pores,193 which pore sizes of nanometres, allowing small molecules to pass through their pores. The selectivity of COFs is very high, which is beneficial for designing COF materials tailored to specific application goals.
7.5 Design and synthesis of COFs
The synthesis of COFs primarily considers two aspects: (1) the reversibility of the reaction and the thermodynamic control involved in achieving reversibility, and (2) reaction conditions and choice of reaction media for synthesising COFs.194 The bonding mode of COFs is contingent on the presence of dynamic covalent bonds that exhibit favourable reversibility. During the condensation reaction, products with disordered structures are formed.195 Consequently, dynamic chemical bonds with good reversibility are required for the self-repair of disordered structures into ordered structures in a thermodynamic state.
7.6 Synthesis of COFs
The main synthetic methods for COFs are as follows: (1) solvothermal method, (2) mechanical grinding, (3) microwave-assisted method, (4) ultrasonic-assisted method, (5) ion thermal synthesis method,196 and (6) catalysis. The stability of organic chemical bonds is crucial in the synthesis of COFs because the occurrence of dislocations and self-repair processes during their synthesis contributes to their stabilisation.197 Researchers have continuously investigated and attempted to stabilise them. The method adopted by researchers depends on the experimental conditions and study objectives. COFs, with their extensive specific surface areas and tuneable structures, have created a unique niche in the field of materials science. Their adsorptive properties, combined with a rich and modifiable precursor base, underscore their pivotal role in the field of electrochemical heavy-metal detection. The multifunctionality of COFs goes beyond mere detection; they act as guardians of the environment and effectively adsorb harmful heavy-metal ions.198
7.7 Application of covalent organic framework materials in heavy metal detection
A groundbreaking research by Pei et al.,199 developed a two-dimensional crystalline nanosheet, COF MELE-BTDD (Fig. 10(a)), demonstrating exceptional sensitivity for heavy metal detection. It achieved detection limits of 0.00474, 0.00123, 0.00114, and 0.00107 μM and sensitivities of 105.0, 405.5, 439.0, and 465.1 μA μM−1 cm−2 for Hg(II), Pb(II), Cd(II), and Cu(II), respectively (Fig. 11(a–e)). These results highlight COF MELE-BTDD's significant potential in electrochemical detection, particularly for high-precision applications. However, its long-term stability requires further validation.
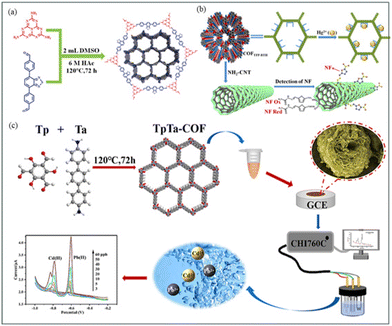 |
| Fig. 10 (a) Preparation process of COF-BTDD and schematic showing the detection of heavy metal ions.199 (b) Synthesis process of COFTFP-BTH as well as the detection of NF and the adsorption principle of heavy metal ions.200 (c) Preparation and detection process of heavy metal ions using the TpTa-COF sensor.201 Reproduced with permission from ref. 200–202. | |
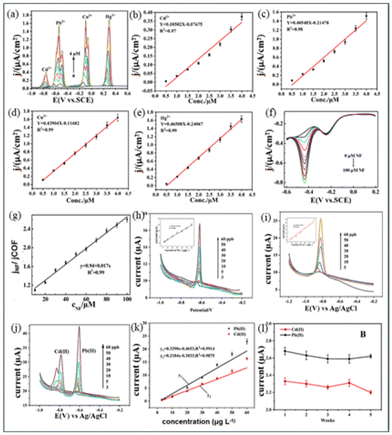 |
| Fig. 11 (a–e) SWASV curves and corresponding calibration curves for heavy metal ion detection using the COF-BTDD sensor.199 (f and g) Detection process of NF and heavy metal ion adsorption using the COFTFP-BTH sensor.200 (h–l) SWASV curves and corresponding calibration curves for heavy metal ion detection using the TpTa-COF sensor.201 Reproduced with permission from ref. 200–202. | |
Wang et al.202 synthesized a novel β-ketoenamine COF combined with NH2-CNTs, significantly enhancing its surface area and stability (Fig. 10(b)). This material exhibited an Hg(II) adsorption capacity of 909 mg g−1 and the ability to detect nitrofurans (NF). Despite its relatively complex synthesis process (Fig. 11(h–l)), its multifunctionality and high adsorption capacity make it outstanding for various environmental detections. Electrochemical analysis confirmed the material's good responsiveness and stability in detecting Hg(II) and NF.
Zhang et al.200 synthesized TpTa-COF via solvothermal methods for detecting trace amounts of Pb(II) and Cd(II) in water(Fig. 10(c)). The sensor showed high sensitivity for Pb(II) and Cd(II) with detection limits of 0.16 μg L−1 and 0.29 μg L−1, respectively, demonstrating good linear response and stability (Fig. 11(f–h)). Its high sensitivity and stability make it suitable for environmental monitoring, though its detection capability for other heavy metals needs improvement.
Overall, Pei et al.'s COF MELE-BTDD excels in heavy metal detection sensitivity, especially in high-precision scenarios, but its long-term stability in practical applications needs verification. In contrast, Wang et al.'s β-ketoenamine COF, despite its complex synthesis, offers excellent multifunctionality and high adsorption capacity, making it effective for various environmental detections. Zhang et al.'s TpTa-COF exhibits high sensitivity and stability for Pb(II) and Cd(II) detection, suitable for environmental monitoring, but requires enhancement in detecting other heavy metals.
Future research should focus on improving COF MELE-BTDD's long-term stability to ensure reliability in practical applications, simplifying the synthesis process of the β-ketoenamine COF to reduce costs and improve production efficiency, and expanding TpTa-COF's application range in multi-metal detection to enhance overall performance. These improvements will enable COF materials to better meet the needs of environmental monitoring and food safety testing, providing more effective solutions for heavy metal pollution.
8. Conclusions and outlook
8.1 Conclusions
Heavy metal ion pollution poses a serious threat to global ecosystems and public health. Not only can these metal ions easily penetrate groundwater systems and soils, but they can also enter the human body through various environmental pathways, causing cumulative toxic effects and triggering a series of ecological and health crises. Therefore, the development of sensitive, selective, and cost-effective detection technologies is an urgent need in the field of environmental science and public health.
Among various detection methods, electrochemical detection technology has become the focus of research in the field of heavy metal detection due to its advantages of high efficiency, portability and significant cost-effectiveness. However, this technology still faces several challenges, such as the signal being greatly affected by complex matrix interference, the need to further improve sensitivity and selectivity, and the fact that the potential for industrial application and commercialization has not yet been fully realized. In this study, we reviewed the latest progress of nanomaterial-based electrochemical sensors in the detection of heavy metals, focusing on the mechanisms of metal nanomaterials, carbon-based nanomaterials, and organic framework materials in improving sensor performance.
It is pointed out that metal nanoparticles significantly improve the efficiency of electrochemical reactions by providing efficient electron transport pathways and catalytic properties due to their excellent conductivity and surface chemical activity. Due to their structural stability and electron migration properties, carbon-based nanomaterials exhibit excellent anti-interference ability in complex environmental matrices. With their rich functionality and highly tunable porous structure, organic framework materials provide an innovative solution for the selective capture and detection of heavy metal ions. By systematically analyzing the basic principles of heavy metal detection and the selection mechanism of nanomaterials, this study clarified the importance of key parameters such as specific surface area, conductivity and electron migration efficiency to improve the performance of the sensor, and revealed the key role of surface modification and structure optimization of nanomaterials in improving the adsorption capacity and redox reaction efficiency of heavy metals.
In summary, because of the characteristics of low cost, easy operation and fast detection, electrochemical sensors have become an important research direction of heavy metal detection technology. Combined with the latest nanomaterial technology, electrochemical sensors have broad application prospects in heavy metal detection, which not only provides strong support for environmental monitoring and food safety, but also provides practical solutions to solve the global problem of heavy metal pollution. The research results and development trends summarized in this review provide an important reference for the design and practical application of electrochemical sensors in the future, and lay a foundation for coping with current challenges and exploring future development directions.
8.2 Shortcomings of electrochemical detection technology and improvement directions
Although electrochemical detection technology has significant advantages in the detection of heavy metal ions, such as fast detection speed, low cost and high sensitivity, its application still has some limitations and needs to be further optimized and improved.
Firstly, the signal is greatly affected by the interference of complex substrates. Impurities in real-world environmental samples, such as soil, water, and food matrices, can interfere with the electrochemical signal and lead to reduced accuracy of test results. To solve this problem, functionalized nanomaterials or separation pretreatment techniques can be introduced to enhance the selectivity of the sensor for the target substance. For example, surface modification of specific functional groups (e.g., carboxyl groups, amino groups) can significantly improve the specific binding capacity of target metal ions, thereby reducing background interference.
Secondly, the detection sensitivity and selectivity still need to be further improved. The performance of existing electrochemical sensors in the detection of ultra-low concentrations of heavy metals is still not completely satisfactory, especially in complex environments where multiple metals coexist. To solve this problem, the efficiency and signal response strength of electrochemical reactions can be improved by developing multifunctional composite materials (e.g., metal–organic framework materials and graphene composites) or optimizing sensor structure design, such as constructing nanoarrays or porous surfaces.
Thirdly, the standardization of electrochemical detection technology is insufficient, and industrial production and large-scale application are facing challenges. Due to the complex preparation process of nanomaterials and the need to improve their stability, it is difficult to ensure the repeatability and batch consistency of the sensor. In the future, it is necessary to develop more economical, environmentally friendly, and efficient nanomaterial fabrication technologies, and optimize the sensor design process to meet the needs of commercialization.
Fourthly, the long-term stability and durability of the sensor need to be improved. In practical applications, long-term exposure of sensors to complex environments may cause problems such as decreased sensitivity or electrode inactivation. This can be done by introducing anti-fouling coatings, improving the chemical stability of the materials, and optimizing the packaging technology of the sensors to extend their service life.
In summary, the future improvement direction of electrochemical detection technology should focus on improving signal anti-interference ability, optimizing sensitivity and selectivity, strengthening the consistency and repeatability of sensors, and focusing on solving technical bottlenecks in industrial applications. These improvements will further promote the widespread application of electrochemical detection technology in environmental monitoring and food safety (Table 4).
Table 4 Advantages and disadvantages of electrode modification materials for ECS Advantages and disadvantages of electrode modification materials for electrochemical sensors
Materials |
Advantages |
Disadvantages |
Ref. |
Metal nanomaterials |
High specific surface area, adjustability, good electrical and thermal conductivity, good biocompatibility and environmental friendliness |
Oxidation may occur in air or water, and some metal nanomaterials may be toxic to organisms, which is complicated to prepare, may aggregate and reunite during use, and is difficult to recover and treat |
201, 203 and 204 |
Carbon nanomaterials |
High specific surface area, good electrical conductivity, high mechanical strength, good chemical stability, environmental protection and sustainable use |
The preparation cost is high, the process is complex, and aggregation and agglomeration are easy to occurs |
205–207
|
Organic framework material |
High surface area, adjustable structure and performance, high porosity and environmental protection |
Complex preparation, poor stability and special requirements |
208–210
|
8.3 Future developments and challenges of electrochemical testing
The future development potential of electrochemical sensors in the field of heavy metal detection is huge, but there are also several key challenges and development directions.
On the one hand, basic research needs to be further deepened. In electrochemical detection, the mechanism of action of nanomaterials needs to be explored more systematically, especially the binding kinetics between metal ions and the surface of materials and the nature of the electron transfer process. Future research should incorporate advanced characterization techniques (e.g., in situ spectroscopy, electrochemical scanning) to uncover the microscopic mechanisms by which different materials improve sensitivity and selectivity. In addition, theoretical simulation and experimental verification of the structural design of materials and sensors will help to develop a more efficient detection platform.
On the other hand, the technical bottleneck in practical application needs to be broken through urgently. The matrix interference and the coexistence effect of heavy metal ions in samples in complex environments are the two major problems faced by current detection technologies. Future research can solve this problem through multifunctional sensor design, such as the development of sensors that can detect multiple heavy metal ions at the same time and ensure the accuracy of detection. In addition, the mobility and integration of sensors are also a focus for future development, and the miniaturized and portable design will make it more suitable for rapid detection in the field.
In terms of industrialization and commercialization, current electrochemical sensors still face the challenges of large-scale production and cost control. In the future, it is necessary to build a greener and more economical material preparation route, and improve the consistency and stability of equipment by optimizing the production process. It is necessary to combine with actual needs to develop special sensor products suitable for different scenarios (such as water quality monitoring, food testing), and realize the transformation from laboratory research to commercial production.
At the same time, it is important to improve regulations and standards. The wide range of applications of electrochemical sensors requires clear technical specifications and testing standards to ensure that their performance can meet the needs of different scenarios. Policy support and coordinated development of the industry will provide a strong guarantee for the promotion of sensor technology.
The future development of electrochemical sensors will focus on the deepening of basic research, the breakthrough of technical bottlenecks, the advancement of commercialization, and the improvement of relevant regulations and standards. These efforts will not only help solve the current problem of heavy metal pollution, but also promote the application of electrochemical detection technology in a wider range of fields, providing new solutions for environmental protection and public health.
8.4 Overview and commercialization potential of industrial-grade sensors
Electrochemical sensors have made significant progress in the field of industrialization and commercialization, especially in water quality monitoring, food safety, and environmental pollution detection. A wide range of industrial-grade sensors are already on the market, including ion-selective electrodes (ISEs), dissolution voltammetry sensors (SWASV and DPV), and nanomaterial-based electrochemical sensors. Due to their high sensitivity, fast detection and easy operation, these sensors are important for specific applications. For example, sensors based on SWASV technology are used to monitor heavy metals (e.g., Pb2+, Hg2+, Cd2+) in drinking water and industrial wastewater due to their excellent detection limits and sensitivity. Through the introduction of metal nanoparticles (e.g., gold and silver) and carbon-based materials (e.g., graphene and carbon nanotubes), the detection performance of the sensor has been significantly improved. Nevertheless, there is still room for improvement in sensitivity, selectivity, anti-interference capability, and cost control of existing sensors.
In terms of marketization, some commercial sensors, such as AquaSensor and MetalGuard, have achieved online real-time monitoring capabilities through modular and portable design. These sensors play an important role in industrial wastewater treatment, drinking water quality monitoring, and more. However, their high cost, short service life, and lack of adaptability to simultaneous detection of multiple heavy metals limit their popularity in a wider range of fields. In addition, existing sensors still face challenges in detecting heavy metals in complex matrices, with signals being susceptible to interference and limited support for multi-target detection.
In order to further tap the commercialization potential of these sensors, it is essential to develop sensors with multi-target detection capabilities. At present, most industrial-grade sensors are mainly aimed at the detection of a single metal ion, and the actual environmental samples often contain the coexistence of multiple heavy metals. By introducing a multi-electrode configuration and a composite functionalized material design, multi-target selective capture and detection can be achieved, which will significantly improve the applicability of the sensor. In addition, controlling costs and optimizing production processes are key to driving the large-scale adoption of sensors. The use of green and economical nanomaterial preparation methods (such as mechanical grinding) and simplified modification technologies can not only reduce production costs, but also improve material utilization and market competitiveness of equipment. At the same time, the optimization of long life and high stability is also a core requirement in industrial applications, and the long-term performance of the sensor can be significantly improved by developing corrosion-resistant electrode coatings, improving material chemical stability, and improving signal consistency.
The following table summarizes the differences in performance between sensors in the academic research stage and industrial-grade sensors, and further clarifies the direction in which the current technology needs to be improved (Table 5).
Table 5 Performance differences between sensors in the academic research stage and industrial-grade sensors, and directions for improvement
Parameter |
Academic research sensors |
Industrial-grade sensors |
Potential areas for improvement |
Limit of detection (LOD) |
As low as 0.01 ppb |
0.1–1 ppb |
Optimize the preparation and surface modification of nanomaterials |
Sensitivity |
High (>30 μA ppm−1) |
Medium (5–15 μA ppm−1) |
Introduction of functionalized composite materials |
Stability |
Susceptible to environmental disturbances |
High, adaptable to a variety of scenarios |
Corrosion-resistant design |
Multi-metal detection capabilities |
Partially implemented |
Weak, often a single metal detection |
Development of multifunctional electrodes and composite materials |
Cost |
Higher, complex material |
Lower, mass production has advantages |
Optimize the production process and reduce material costs |
As can be seen from the table, sensors in academic research usually have higher detection limits and sensitivity, but are lacking in stability and multi-target detection capabilities, while industrial-grade sensors focus more on stability and operability. In the future, it is expected that a balance between high performance and low cost will be achieved by integrating the high-performance nanomaterials developed in academic research with the actual needs of industrial applications.
Overall, the market demand for industrial-grade electrochemical sensors in environmental monitoring is expanding, but its commercialization potential has not yet been fully released. The industrialization of these sensors can be further promoted through advances in nanomaterial technology, the design of multifunctional integrated sensors, and the optimization of production processes. Combined with policy support and market promotion, the wide application of electrochemical sensors in environmental pollution monitoring, food safety testing and other fields in the future will provide strong technical support for solving the global heavy metal pollution problem (Fig. 12).
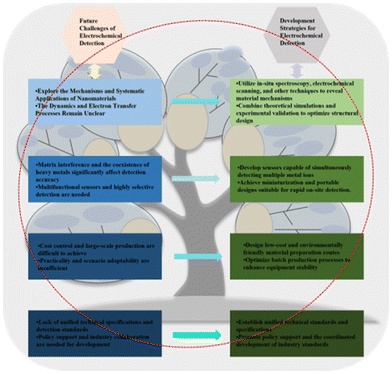 |
| Fig. 12 Future prospects and challenges diagram. | |
Data availability
The data that supports the findings of this study are available upon request.
Conflicts of interest
There are no conflicts to declare.
Acknowledgements
This work was supported by the Major Science and Technology Program of Yunnan Branch of China Tobacco Corporation (2023530000241029), Qilu Lake Field Observation and Research Station for Plateau Shallow Lake in Yunnan Province (202305AM070001), Tianshan Innovation Team Plan of Xinjiang Uygur Autonomous Region (2023D14002), Double Thousand Plan of Jiangxi Province, and Double First University Plan (C176220100042).
References
- M. Li, Q. Shi, N. Song, Y. Xiao, L. Wang, Z. Chen and T. D. James, Chem. Soc. Rev., 2023, 52, 5827–5860 RSC
.
- I. Haider, M. A. Ali, M. Sanaullah, N. Ahmed, S. Hussain, M. T. Shakeel, S. A. H. Naqvi, J. S. Dar, M. Moustafa and M. O. Alshaharni, Chemosphere, 2023, 342, 140193 CrossRef PubMed
.
- K. Zheng, Z. Zeng, Q. Tian, J. Huang, Q. Zhong and X. Huo, Sci. Total Environ., 2023, 868, 161691 CrossRef PubMed
.
- Z. Liu, Y. Fei, H. Shi, L. Mo and J. Qi, Sci. Total Environ., 2022, 808, 151874 CrossRef CAS PubMed
.
- Y. Cui, L. Bai, C. Li, Z. He and X. Liu, Sustain. Cities Soc., 2022, 80, 103796 CrossRef
.
- R. Meng, Q. Zhu, T. Long, X. He, Z. Luo, R. Gu, W. Wang and P. Xiang, Food Control, 2023, 150, 109743 CrossRef CAS
.
- Z. Lin, H. Chen and H. Yang, Environ. Sci. Pollut. Res., 2020, 27, 22795–22805 CrossRef CAS PubMed
.
- G.-R. Xu, Z.-H. An, K. Xu, Q. Liu, R. Das and H.-L. Zhao, Coord. Chem. Rev., 2021, 427, 213554 CrossRef CAS
.
- L. A. Malik, A. Bashir, A. Qureashi and A. H. Pandith, Environ. Chem. Lett., 2019, 17, 1495–1521 CrossRef CAS
.
- F.-J. Zhao, Z. Tang, J.-J. Song, X.-Y. Huang and P. Wang, Mol. Plant, 2022, 15, 27–44 CrossRef CAS PubMed
.
- A. Navarro-Sempere, P. Martínez-Peinado, A. S. Rodrigues, P. V. Garcia, R. Camarinho, G. Grindlay, L. Gras, M. García and Y. Segovia, Environ. Geochem. Health, 2023, 45, 8257–8269 Search PubMed
.
- K. Velusamy, S. Periyasamy, P. S. Kumar, G. Rangasamy, J. M. Nisha Pauline, P. Ramaraju, S. Mohanasundaram and D.-V. Nguyen Vo, Food Chem. Toxicol., 2022, 168, 113307 Search PubMed
.
- H. W. Haso, A. A. Dubale, M. A. Chimdesa and M. Atlabachew, Front. Mater., 2022, 9, 840806 Search PubMed
.
- C. Venkateswara Raju, C. Hwan Cho, G. Mohana Rani, V. Manju, R. Umapathi, Y. Suk Huh and J. Pil Park, Coord. Chem. Rev., 2023, 476, 214920 CrossRef CAS
.
- E. F. Hasan Alzaimoor and E. Khan, Crit. Rev. Anal. Chem., 2023, 1–22 Search PubMed
.
- G. Qin, Z. Niu, J. Yu, Z. Li, J. Ma and P. Xiang, Chemosphere, 2021, 267, 129205 CrossRef CAS PubMed
.
- M. Gavrilescu, Water, 2021, 13, 2746 CrossRef CAS
.
- G. A. Lamas, F. Ujueta and A. Navas-Acien, J. Am. Heart Assoc., 2021, 10, e018692 CrossRef CAS
.
- R. M. Hlihor, M. Roşca, L. Hagiu-Zaleschi, I. M. Simion, G. M. Daraban and V. Stoleru, Toxics, 2022, 10, 499 Search PubMed
.
- K. Li, H. Yang, X. Yuan and M. Zhang, Microchem. J., 2021, 160, 105726 Search PubMed
.
- N. Yadav and A. K. Singh, J. Electrochem. Soc., 2019, 166, B644–B653 Search PubMed
.
- O. V. Lundovskaya, A. R. Tsygankova, N. I. Petrova and A. I. Saprykin, J. Anal. Chem., 2018, 73, 877–883 Search PubMed
.
- B. B. S. Jaswal, P. K. Rai, T. Singh, V. Zorba and V. K. Singh, X-Ray Spectrom., 2019, 48, 178–187 Search PubMed
.
- R. Singh, R. Gupta, D. Bansal, R. Bhateria and M. Sharma, ACS Omega, 2024, 9, 7336–7356 CAS
.
- G. Manasa and C. S. Rout, Mater. Adv., 2024, 5, 83–122 RSC
.
- M. Zahran, Mater. Adv., 2024, 5, 68–82 RSC
.
- H. Chai, Y. Ma, Z. Yuan, Y. Li, G. Liu, L. Chen, Y. Tian, W. Tan, J. Ma and G. Zhang, Microchem. J., 2023, 185, 108288 CrossRef CAS
.
- E. J. Nixon, R. Sakthivel, L.-Y. Lin, Y.-F. Duann, U. Dhawan, X. Liu, Y.-C. Lin, J.-C. Chen, C.-W. Tung and R.-J. Chung, Microchem. J., 2024, 200, 110336 CrossRef CAS
.
- B. Zhang, G. Ren, L. Ran, M. Liu, P. Geng and W. Yi, J. Environ. Chem. Eng., 2024, 12, 113903 Search PubMed
.
- A. Ghazal, A. Shaheen, S. AlBlooshi, M. Almehrzi, R. A. Ismail, N. AlAmoodi, S. Hong and F. AlMarzooqi, Chem. Eng. J., 2024, 484, 149594 CrossRef CAS
.
- Q. Liang, W. Xiao, C. Zhang, D. Zhu, S.-L. Wang, S.-Y.U. Tian, T. Long, E.-L. Yue, J.-J. Wang and X.-Y. Hou, Talanta, 2023, 259, 124491 Search PubMed
.
- F.-F. Wang, C. Liu, J. Yang, H.-L. Xu, W.-Y. Pei and J.-F. Ma, Chem. Eng. J., 2022, 438, 135639 CrossRef CAS
.
- H. Hu, Y. Zhang, Y. Hu, L. Xia and G. Li, Talanta, 2024, 271, 125653 CrossRef CAS PubMed
.
- J.-N. Hengsbach, M. Engel, M. Cwienczek, J. Stiefelmaier, N. Tippkötter, D. Holtmann and R. Ulber, ChemElectroChem, 2023, 10, e202300440 CrossRef CAS
.
- C. Mejia, N. Christensen, R. R. Ceron and D. Rappleye, Electrochim. Acta, 2025, 512, 145496 CrossRef CAS
.
- L. Reuter, L. J. Reinschlüssel and H. A. Gasteiger, J. Electrochem. Soc., 2024, 171, 100524 Search PubMed
.
- Y. Firouzi Jahantigh, M. Mehrpooya, R. Askari Moghadam and M. R. Ganjali, Mater. Chem. Phys., 2025, 331, 130173 CrossRef CAS
.
- L. Keeble, A. Jaccottet, D. Ma, J. Rodriguez-Manzano and P. Georgiou, Electrochim. Acta, 2024, 477, 143780 Search PubMed
.
- P.-A. Gross, T. Jaramillo and B. Pruitt, Anal. Chem., 2018, 90, 6102–6108 CrossRef CAS PubMed
.
- J. S. Dondapati, A. R. Thiruppathi, A. Salverda and A. Chen, Electrochem. Commun., 2021, 124, 106946 CrossRef CAS
.
- A. A. Ali, A.
B. Altemimi, N. Alhelfi and S. A. Ibrahim, Biosensors, 2020, 10, 58 CrossRef CAS PubMed
.
- H. Wang, D. Zhu, A. Paul, L. Cai, A. Enejder, F. Yang and S. C. Heilshorn, Adv. Funct. Mater., 2017, 27, 1605609 CrossRef PubMed
.
- I. Atlas and M. E. Suss, Electrochim. Acta, 2019, 319, 813–821 CrossRef
.
- A. Ehsani, Z. Rezaei, A. Agah, H. M. Shiri and A. A. Heidari, Microchem. J., 2021, 165, 106074 CrossRef
.
- K. Takemura, T. Motomura, W. Iwasaki and N. Morita, ACS Appl. Nano Mater., 2023, 6, 15879–15886 CrossRef
.
- H. Hammad, M. Khalid, M. Yasin, K. Naz, M. G. B. Ashiq, M. Younas, M. Z. Yar, M. M. Al-Anazy, K. B. Hassan, B. Ahmed and B. A. Ahmed, Mater. Chem. Phys., 2024, 315, 128959 CrossRef
.
- C. Cheng, Z. Sun, B. Li, Y. Li, C. Jin, T. Xiang, W. Wang, Z. Wu, H. Xue, Y. Cao and J. Yang, Appl. Surf. Sci., 2023, 614, 156096 CrossRef
.
- S. Zeng, Y. Yu, X. Wen, J. Ni, Z. Song and W. Qiu, Surf. Interfaces, 2024, 51, 104668 CrossRef
.
- Y. Fan, C. Salzemann, B. Tremblay, A. Girard and A. Courty, ACS Appl. Nano Mater., 2024, 7, 6130–6138 CrossRef
.
- Y. Li, H. Han, Y. Fei, D. Pan and H. Wang, Microchem. J., 2024, 197, 109857 CrossRef
.
- H. Zhao, Y. Liu, F. Li, G. Zhu, M. Guo, J. Han, M. Zhao, Z. Wang, F. Nie and Q. Ran, Ceram. Int., 2023, 49, 26289–26301 CrossRef
.
- F. Zhang and L. Qi, Adv. Sci., 2016, 3, 1600049 CrossRef PubMed
.
- H. Zhan, Y. Qiao, Z. Qian, B. Lv, Z. Wu and Z. Liu, Chem. Eng. J., 2024, 497, 154859 CrossRef
.
- B. Han, W. Li, Y. Shen, R. Li, M. Wang, Z. Zhuang, Y. Zhou and T. Jing, Sci. Total Environ., 2023, 864, 161173 CrossRef PubMed
.
- L. Huang, S. Wang, K. Zhang, Y. Li, H. Sui, X. Bu, Y. Jiang, X. Huang and P. Zhang, Sens. Actuators, A, 2023, 360, 114500 CrossRef
.
- M. Soylak, O. Ozalp and F. Uzcan, Trends Environ. Anal. Chem., 2021, 29, e00109 CrossRef
.
- Z. Chen, M. Xie, F. Zhao and S. Han, Foods, 2022, 11, 1404 CrossRef PubMed
.
- X. Zhang, D. An, Z. Bi, W. Shan, B. Zhu, L. Zhou, L. Yu, H. Zhang, S. Xia and M. Qiu, J. Electroanal. Chem., 2022, 911, 116239 CrossRef
.
- L. Gurusamy, L. Karuppasamy, S. Anandan, S. C. Barton, Y.-H. Chuang, C.-H. Liu and J. J. Wu, Coord. Chem. Rev., 2023, 484, 215102 CrossRef
.
- M. Chu, Z. Bai, D. Zhu, W. Chen, G. Yang, J. Xin, H. Ma, H. Pang, L. Tan and X. Wang, J. Electroanal. Chem., 2022, 907, 116083 CrossRef
.
- W. Meng, X. Han, R. Han, X. Zhang, X. Zeng, J. Duan and X. Luo, Anal. Chim. Acta, 2024, 1309, 342685 CrossRef PubMed
.
- B. Li, X. Xie, T. Meng, X. Guo, Q. Li, Y. Yang, H. Jin, C. Jin, X. Meng and H. Pang, Food Chem., 2024, 440, 138213 CrossRef
.
- L. Zhang, Y. Pu, W. Xu, J. Peng, Y. Liu and H. Du, Microchem. J., 2024, 201, 110542 CrossRef
.
- A. M. Díez-Pascual, Int. J. Mol. Sci., 2024, 25, 3032 CrossRef
.
- Z. Ali, R. Ullah, M. Tuzen, S. Ullah, A. Rahim and T. A. Saleh, Trends Environ. Anal. Chem., 2023, 37, e00187 CrossRef
.
- J. Jose, P. Prakash, B. Jeyaprabha, R. Abraham, R. M. Mathew, E. S. Zacharia, V. Thomas and J. Thomas, J. Iran. Chem. Soc., 2023, 20, 775–791 CrossRef
.
- F. Hernández-García, G. A. Álvarez-Romero, R. Colorado-Peralta, J. A. Cruz-Navarro and D. Morales-Morales, J. Electrochem. Soc., 2024, 171, 077521 CrossRef
.
- R. Wu, Q. Du, H. Zhang, P. Zhang, X. Lei and F. Zhang, J. Saudi Chem. Soc., 2024, 28, 101824 CrossRef
.
- D. S. Chaudhari, R. P. Upadhyay, G. Y. Shinde, M. B. Gawande, J. Filip, R. S. Varma and R. Zbořil, Green Chem., 2024, 26, 7579–7655 RSC
.
- J. Mei, T. Liao, G. A. Ayoko, J. Bell and Z. Sun, Prog. Mater. Sci., 2019, 103, 596–677 CrossRef
.
- A. Malara, A. Fotia, E. Paone and G. Serrano, Materials, 2021, 14, 3000 CrossRef PubMed
.
- G. A. Bodkhe, M. S. More, A. Umar, A. A. Ibrahim, S. Siva, M. A. Deshmukh, N. N. Ingle, D. K. Gaikwad, M.-L. Tsai, T. Hianik, M. Kim and M. D. Shirsat, J. Environ. Chem. Eng., 2024, 12, 113024 CrossRef
.
- Y. Jiang, Y. Sima, T. S. Alomar, N. AlMasoud, Z. M. El-Bahy, J. Feng, K. Wan, Z. Toktarbay, Q. He, J. Ding, J. Wang and W. Wang, Adv. Compos. Hybrid Mater., 2024, 7, 223 CrossRef
.
- C. Hu, L.-L. Chang, Y.-H. Lin, T.-J. Lin, Z.-J. Lin, A. Vinu and K.-L. Tung, Surf. Interfaces, 2024, 49, 104419 CrossRef
.
- N. Kajal, V. Singh, R. Gupta and S. Gautam, Environ. Res., 2022, 204, 112320 CrossRef PubMed
.
- J. Liu, X. Zhong, Q. Zhang, L. Deng, L. Hou, J. Zou, S. Liu, Y. Gao, L. Wang and L. Lu, Microchem. J., 2024, 201, 110603 CrossRef
.
- H. Abd El-Raheem, R. Helim, R. Y. A. Hassan, A. F. A. Youssef, H. Korri-Youssoufi and C. Kraiya, Microchem. J., 2024, 207, 112086 CrossRef
.
- M. B. Elamin, A. Chrouda, S. M. A. Ali, L. M. Alhaidari, M. Jabli, R. M. Alrouqi and N. J. Renault, Heliyon, 2024, 10, e26364 CrossRef PubMed
.
- L. Zhang, Y. Pu, W. Xu, J. Peng, Y. Liu and H. Du, Microchem. J., 2024, 201, 110542 CrossRef
.
- P. Mahajan, V. Khanna, A. Singh and K. Singh, J. Electrochem. Soc., 2024, 171, 117527 CrossRef
.
- H. A. Potes-Lesoinne, F. Ramirez-Alvarez, V. H. Perez-Gonzalez, S. O. Martinez-Chapa and R. C. Gallo-Villanueva, Electrophoresis, 2022, 43, 249–262 CrossRef PubMed
.
- T. De Andrade Silva, F. Arcadio, L. Zeni, R. Martins, J. P. De Oliveira, C. Marques and N. Cennamo, Talanta, 2024, 271, 125648 CrossRef PubMed
.
- Z. Chen, B. Chen, M. He, H. Wang and B. Hu, Microchim. Acta, 2019, 186, 107 CrossRef PubMed
.
- B. Xue, Q. Yang, K. Xia, Z. Li, G. Y. Chen, D. Zhang and X. Zhou, Engineering, 2022, S2095809922004428 Search PubMed
.
- Z. Zhang and H. Karimi-Maleh, Adv. Compos. Hybrid Mater., 2023, 6, 68 CrossRef
.
- A. Hyder, J. A. Buledi, M. Nawaz, D. B. Rajpar, Z.-H. Shah, Y. Orooji, M. L. Yola, H. Karimi-Maleh, H. Lin and A. R. Solangi, Environ. Res., 2022, 205, 112475 CrossRef PubMed
.
- B. Wang, J. Chen, H. Tong, Y. Huang, B. Liu, X. Yang, Z. Su, X. Tu and X. Qin, J. Electroanal. Chem., 2023, 943, 117573 CrossRef
.
- L. Yu, X. Chen, L. Sun, Q. Zhang, B. Yang, M. Huang, B. Xu and Q. Xu, React. Funct. Polym., 2024, 194, 105770 CrossRef
.
- A. Kundu, L. Mallick, A. Rajput, Y. Kumar and B. Chakraborty, Mater. Today Chem., 2022, 25, 100927 CrossRef
.
- M. Akhtar, M. Sohail, M. Farooq Warsi, M. M. Ibrahim, M. A. Amin and M. Shahid, FlatChem, 2023, 41, 100537 CrossRef
.
- V. Myndrul, A. Yanovska, N. Babayevska, V. Korniienko, K. Diedkova, M. Jancelewicz, M. Pogorielov and I. Iatsunskyi, Talanta, 2024, 271, 125641 CrossRef PubMed
.
- Y. Gao, S. Xu, Z. Liu, K. Yu, C. Wang, S. Wu, J. Wang and X. Pan, J. Lumin., 2021, 238, 118276 CrossRef
.
- N. Ajinkya, X. Yu, P. Kaithal, H. Luo, P. Somani and S. Ramakrishna, Materials, 2020, 13, 4644 CrossRef PubMed
.
- M. Zhang and W. Guo, Food Chem., 2023, 406, 135034 CrossRef PubMed
.
- X. Wang, Y. Qi, Y. Shen, Y. Yuan, L. Zhang, C. Zhang and Y. Sun, Sens. Actuators, B, 2020, 310, 127756 CrossRef
.
- W. Zou, Y. Tang, H. Zeng, C. Wang and Y. Wu, J. Hazard. Mater., 2021, 417, 125994 CrossRef CAS PubMed
.
- M. Chatterjee, S. Sain, A. Roy, S. Das and S. K. Pradhan, J. Phys. Chem. Solids, 2021, 148, 109733 CrossRef CAS
.
- S.-S. Li, Q.-Q. Xu, J.-T. Xu, G. Yan, Y.-X. Zhang, S.-W. Li and L.-C. Yin, Sens. Actuators, B, 2022, 354, 131095 CrossRef CAS
.
- A. Irshad, F. Farooq, M. Farooq Warsi, N. Shaheen, A. Y. Elnaggar, E. E. Hussein, Z. M. El-Bahy and M. Shahid, FlatChem, 2022, 31, 100325 CrossRef
.
- S. Cheng, D. Tang, Y. Zhang, L. Xu, K. Liu, K. Huang and Z. Yin, Biosensors, 2022, 12, 326 CrossRef PubMed
.
- R. Ranjith, S. Ravikumar, V. Pandiyan, U. Rajaji, K. M. Abualnaja, T. S. Alomar, N. AlMasoud and M. Ouladsmne, J. Mater. Sci.: Mater. Electron., 2022, 33, 9438–9447 CrossRef
.
- S. Anuma, P. Mishra and B. R. Bhat, J. Hazard. Mater., 2021, 416, 125929 CrossRef PubMed
.
- Y. Zhu, D. Zhang, F. Chang, J. Zhu, P. Wang, F. Tan, X. Li, L. Li and G. Hu, Surf. Interfaces, 2021, 25, 101266 CrossRef
.
- Y. Wang, Z. Nie, X. Li, Y. Zhao and H. Wang, Sens. Actuators, B, 2021, 346, 130539 CrossRef
.
- T. Wu, Y. Zhu, L. Song, Y. Chen, Y. Huang, J. Tang, X. Ma, H. Wang, J. Zhang, D. Lin and G. Chen, Anal. Methods, 2022, 14, 859–868 RSC
.
- A. N. Jijana, N. Mphuthi, P. Shumbula, S. Vilakazi and L. Sikhwivhilu, Electrocatalysis, 2021, 12, 310–325 CrossRef
.
- S. Rabai, A. Teniou, G. Catanante, M. Benounis, J.-L. Marty and A. Rhouati, Sensors, 2021, 22, 105 CrossRef PubMed
.
- T. Priya, N. Dhanalakshmi, S. Thennarasu, V. Karthikeyan and N. Thinakaran, Chem. Phys. Lett., 2019, 731, 136621 CrossRef
.
- S. Wu, K. Li, X. Dai, Z. Zhang, F. Ding and S. Li, Microchem. J., 2020, 155, 104710 CrossRef
.
- N.-U.-A. Babar, K. S. Joya, M. A. Tayyab, M. N. Ashiq and M. Sohail, ACS Omega, 2019, 4, 13645–13657 CrossRef PubMed
.
- W. Wu, M. Jia, Z. Zhang, X. Chen, Q. Zhang, W. Zhang, P. Li and L. Chen, Ecotoxicol. Environ. Saf., 2019, 175, 243–250 CrossRef PubMed
.
- M. A. Djebbi, L. Allagui, M. S. El Ayachi, S. Boubakri, N. Jaffrezic-Renault, P. Namour and A. Ben Haj Amara, ACS Appl. Nano Mater., 2022, 5, 546–558 CrossRef
.
- B. Maleki, M. Baghayeri, M. Ghanei-Motlagh, F. Mohammadi Zonoz, A. Amiri, F. Hajizadeh, A. Hosseinifar and E. Esmaeilnezhad, Measurement, 2019, 140, 81–88 CrossRef
.
- T. R. Das and P. K. Sharma, Microchem. J., 2019, 147, 1203–1214 CrossRef
.
- J.-H. Hwang, P. Pathak, X. Wang, K. L. Rodriguez, J. Park, H. J. Cho and W. H. Lee, Sens. Actuators, B, 2019, 294, 89–97 CrossRef
.
- Z. Dahaghin, P. A. Kilmartin and H. Z. Mousavi, Food Chem., 2020, 303, 125374 CrossRef PubMed
.
- Y. He, Z. Wang, L. Ma, L. Zhou, Y. Jiang and J. Gao, RSC Adv., 2020, 10, 27697–27705 RSC
.
- J. You, J. Li, Z. Wang, M. Baghayeri and H. Zhang, Chemosphere, 2023, 335, 139133 CrossRef PubMed
.
- A. Yamuna, C.-Y. Hong, S.-M. Chen, T.-W. Chen, E. A. Alabdullkarem, M. Soylak, M. Mana Al-Anazy, M. Ajmal Ali and X. Liu, J. Electroanal. Chem., 2021, 887, 115159 CrossRef
.
- Y. Hao, C. Zhang, W. Wang, J. Wang, S. Chen, H. Xu and S. Zhuiykov, J. Electrochem. Soc., 2021, 168, 083503 CrossRef
.
- C.-Y. Li, Y.-Y. Wei, W. Shen, X. Dong, M. Yang and J. Wei, Electrochim. Acta, 2021, 368, 137605 CrossRef
.
- F. Xie, Z.-Y. Song, M. Yang, W.-C. Duan, Y.-N. Quan, X.-J. Huang, W.-Q. Liu and P.-H. Xie, Anal. Chim. Acta, 2022, 1200, 339607 CrossRef PubMed
.
- Y. Liu, D. Zhang, J. Ding, K. Hayat, X. Yang, X. Zhan, D. Zhang, Y. Lu and P. Zhou, Biosensors, 2020, 10, 195 CrossRef PubMed
.
- Q. Sun, X. Xu, M. Wu, N. Niu and L. Chen, Talanta, 2024, 271, 125657 CrossRef PubMed
.
- Z. Peng, X. Liu, W. Zhang, Z. Zeng, Z. Liu, C. Zhang, Y. Liu, B. Shao, Q. Liang, W. Tang and X. Yuan, Environ. Int., 2020, 134, 105298 CrossRef PubMed
.
- S. Bilge, L. Karadurmus, A. Sınağ and S. A. Ozkan, TrAC, Trends Anal. Chem., 2021, 145, 116473 CrossRef
.
- L. Yue, H. Zhao, Z. Wu, J. Liang, S. Lu, G. Chen, S. Gao, B. Zhong, X. Guo and X. Sun, J. Mater. Chem. A, 2020, 8, 11493–11510 RSC
.
- X. Ming, A. Wei, Y. Liu, L. Peng, P. Li, J. Wang, S. Liu, W. Fang, Z. Wang, H. Peng, J. Lin, H. Huang, Z. Han, S. Luo, M. Cao, B. Wang, Z. Liu, F. Guo, Z. Xu and C. Gao, Adv. Mater., 2022, 34, 2201867 CrossRef PubMed
.
- A. Wang, S. Xie, R. Zhang, Y. She, C. Chen, M. K. H. Leung, C. Niu and H. Wang, Nanoscale Adv., 2019, 1, 656–663 RSC
.
- H.-F. Li, C. Wang, L.-F. Liu, N. Xie, M. Pan, P. Wu, X.-D. Wang, Z. Zeng, S. Deng and G.-P. Dai, J. Nanopart. Res., 2020, 22, 10 CrossRef
.
- S. Zhang, H. Zhu, P. Ma, F. Duan, W. Dong and M. Du, Anal. Methods, 2017, 9, 6801–6807 RSC
.
- S. Rathinavel, K. Priyadharshini and D. Panda, Mater. Sci. Eng., C, 2021, 268, 115095 CrossRef
.
- R. B. Onyancha, U. O. Aigbe, K. E. Ukhurebor and P. W. Muchiri, J. Mol. Struct., 2021, 1238, 130462 CrossRef
.
- A. K. Wanekaya, Analyst, 2011, 136, 4383 RSC
.
- G. Zhao, H. Wang, G. Liu and Z. Wang, Sensors, 2016, 16, 1540 CrossRef
.
- D. Deng, W. Zhang, X. Chen, F. Liu, J. Zhang, Y. Gu and J. Hong, Eur. J. Inorg. Chem., 2009, 2009, 3440–3446 CrossRef
.
- X. Su, R. Wang, X. Li, S. Araby, H.-C. Kuan, M. Naeem and J. Ma, Nano Mater. Sci., 2022, 4, 185–204 CrossRef
.
- F. Alhashmi Alamer and G. A. Almalki, Polymers, 2022, 14, 5376 CrossRef PubMed
.
- N. A. Aschemacher, C. M. Teglia, Á. S. Siano, F. A. Gutierrez and H. C. Goicoechea, Talanta, 2024, 268, 125313 CrossRef PubMed
.
- J. Dong, L. Wen, D. Zhao, H. Yang, J. Zhao, Z. Hu, Y. Ma, C. Hou and D. Huo, Food Chem., 2023, 418, 135869 CrossRef PubMed
.
- J. Zhao, Y. Long, C. He, H. Yang, S. Zhao, X. Luo, D. Huo and C. Hou, ACS Sustainable Chem. Eng., 2023, 11, 2160–2171 CrossRef
.
- A. Z. Moghaddam, M. Goharjoo, E. Ghiamati and K. Khodaei Tabani, Talanta, 2021, 222, 121557 CrossRef PubMed
.
- H. Yang, H. Zheng, Y. Duan, T. Xu, H. Xie, H. Du and C. Si, Int. J. Biol. Macromol., 2023, 253, 126903 CrossRef PubMed
.
- E. Prabakaran, T. Velempini, M. Molefe and K. Pillay, J. Mater. Res. Technol., 2021, 15, 6340–6355 CrossRef
.
- J. Wang, P. Yu, K. Kan, H. Lv, Z. Liu, B. Sun, X. Bai, J. Chen, Y. Zhang and K. Shi, Chem. Eng. J., 2021, 420, 130317 CrossRef
.
- J. Pang, H. Fu, W. Kong, R. Jiang, J. Ye, Z. Zhao, J. Hou, K. Sun, Y. Zheng and L. Chen, Chem. Eng. J., 2022, 433, 133854 CrossRef
.
- M. A. Qamar, M. Javed, S. Shahid, M. Shariq, M. M. Fadhali, S. K. Ali and M. S. Khan, Heliyon, 2023, 9, e12685 CrossRef PubMed
.
- M. Singh, S. R. Bhardiya, D. Patel, B. Khuntey, S. Yadav, A. Rai and V. K. Rai, Talanta, 2024, 269, 125400 CrossRef PubMed
.
- D. Ayodhya, Mater. Chem. Front., 2022, 6, 2610–2650 RSC
.
- H. Wang, Y. Xu, D. Xu, L. Chen, X. Qiu and Y. Zhu, ACS ES&T Eng., 2022, 2, 140–157 Search PubMed
.
- X. Wang, R. Wang, Q. Xue, Z. Liu, Y. Liu, J. Wang and C. Zhu, Diamond Relat. Mater., 2023, 140, 110427 CrossRef
.
- D. Vasu, A. K. Keyan, P. Moorthi, S. Sakthinathan, Y. You and T.-W. Chiu, Mater. Chem. Phys., 2023, 295, 127065 CrossRef
.
- J. Zou, D. Mao, Arramel, L. Neng Li and J. Jiang, Appl. Surf. Sci., 2020, 506, 144672 CrossRef
.
- L. Oularbi, M. Turmine, F. E. Salih and M. El Rhazi, J. Environ., 2020, 8, 103774 Search PubMed
.
- Y. Qi, X. Chen, S. Liu, P. Yang, S. Zhang, C. Hou and D. Huo, J. Electrochem. Soc., 2021, 168, 067526 CrossRef
.
- T. L. Hai, L. C. Hung, T. T. B. Phuong, B. T. T. Ha, B.-S. Nguyen, T. D. Hai and V.-H. Nguyen, Microchem. J., 2020, 153, 104456 CrossRef
.
- S. Singh, A. Pankaj, S. Mishra, K. Tewari and S. Pratap Singh, J. Environ., 2019, 7, 103250 Search PubMed
.
- P. Gupta, C. E. Rahm, D. Jiang, V. K. Gupta, W. R. Heineman, G. Justin and N. T. Alvarez, Anal. Chim. Acta, 2021, 1155, 338353 CrossRef PubMed
.
- Q. Bao, G. Li, Z. Yang, P. Pan, J. Liu, J. Chang, J. Wei and L. Lin, Chin. Chem. Lett., 2020, 31, 2752–2756 CrossRef
.
- N. Bashir, M. Akhtar, H. Z. R. Nawaz, M. F. Warsi, I. Shakir, P. O. Agboola and S. Zulfiqar, Chemistry Select, 2020, 5, 7909–7918 Search PubMed
.
- J. Qin, W. Li, K. Cai, D. Wang, C. Peng, L. Luo, S. Song, Y. Mei and Y. Wang, Microporous Mesoporous Mater., 2023, 360, 112721 CrossRef
.
- G. Zhao, Y. Shen, T. Pham, Y. Chen and M. Ashok, Anal. Chim. Acta, 2022, 1235, 340553 CrossRef PubMed
.
- M. Lu, Y. Deng, Y. Luo, J. Lv, T. Li, J. Xu, S.-W. Chen and J. Wang, Anal. Chem., 2019, 91, 888–895 CrossRef PubMed
.
- Q. Shan, J. Tian, Q. Ding and W. Wu, Mater. Chem. Phys., 2022, 284, 126064 CrossRef
.
- J. Gao, H. Fu, C. Liu, Y. Zhu and X. Fu, Metals, 2023, 13, 1253 CrossRef
.
- X. Xu, X. Lv, F. Tan, Y. Li, C. Geng, B. Cui and Y. Fang, J. Electrochem. Soc., 2021, 168, 126516 CrossRef
.
- K. B. R. Teodoro, F. L. Migliorini, M. H. M. Facure and D. S. Correa, Carbohydr. Polym., 2019, 207, 747–754 CrossRef PubMed
.
- S. A. Hashemi, S. Bahrani, S. M. Mousavi, N. Omidifar, M. Arjmand, K. B. Lankarani and S. Ramakrishna, Eur. Polym. J., 2022, 162, 110926 CrossRef
.
- E. W. Nunes, M. K. L. Silva and I. Cesarino, Chemosensors, 2020, 8, 53 CrossRef
.
- D. Shi, W. Wu and X. Li, Anal. Methods, 2022, 14, 2161–2167 RSC
.
- W. Wu, A. Ali, R. Jamal, M. Abdulla, T. Bakri and T. Abdiryim, RSC Adv., 2019, 9, 34691–34698 RSC
.
- A. T. E. Vilian, S. Y. Oh, M. Rethinasabapathy, R. Umapathi, S.-K. Hwang, C. W. Oh, B. Park, Y. S. Huh and Y.-K. Han, J. Hazard. Mater., 2020, 399, 122868 CrossRef PubMed
.
- G. Kesavan and S.-M. Chen, Diamond Relat. Mater., 2020, 108, 107975 CrossRef
.
- G. Cai, P. Yan, L. Zhang, H.-C. Zhou and H.-L. Jiang, Chem. Rev., 2021, 121, 12278–12326 CrossRef PubMed
.
- S. Liu, M. Wang, Y. He, Q. Cheng, T. Qian and C. Yan, Coord. Chem. Rev., 2023, 475, 214882 CrossRef
.
- H. B. Wu and X. W. Lou, Sci. Adv., 2017, 3, eaap9252 CrossRef PubMed
.
- Y. Zhou, R. Abazari, J. Chen, M. Tahir, A. Kumar, R. R. Ikreedeegh, E. Rani, H. Singh and A. M. Kirillov, Coord. Chem. Rev., 2022, 451, 214264 CrossRef
.
- Y. Zhu, D. Zhu, Y. Chen, Q. Yan, C.-Y. Liu, K. Ling, Y. Liu, D. Lee, X. Wu, T. P. Senftle and R. Verduzco, Chem. Sci., 2021, 12, 16092–16099 RSC
.
- R. Huang, K. Zhang, H. Sun, D. Zhang, J. Zhu, S. Zhou, W. Li, Y. Li, C. Wang, X. Jia, T. Wågberg and G. Hu, Anal. Chim. Acta, 2022, 1228, 340309 CrossRef CAS PubMed
.
- G. Zhang, L. Jin, R. Zhang, Y. Bai, R. Zhu and H. Pang, Coord. Chem. Rev., 2021, 439, 213915 CrossRef
.
- J. Annamalai, P. Murugan, D. Ganapathy, D. Nallaswamy, R. Atchudan, S. Arya, A. Khosla, S. Barathi and A. K. Sundramoorthy, Chemosphere, 2022, 298, 134184 CrossRef PubMed
.
- N. Shi, Y. Song, X. Xing and J. Chen, Coord. Chem. Rev., 2021, 449, 214204 CrossRef
.
- W. Shi, W. Li, W. Nguyen, W. Chen, J. Wang and M. Chen, Mater.Today, 2022, 15, 100273 CrossRef
.
- Z. Zeng, X. Fang, W. Miao, Y. Liu, T. Maiyalagan and S. Mao, ACS Sens., 2019, 4, 1934–1941 CrossRef PubMed
.
- R. Huang, J. Lv, J. Chen, Y. Zhu, J. Zhu, T. Wågberg and G. Hu, J. Hazard. Mater., 2023, 442, 130020 CrossRef PubMed
.
- R. Huang, H. Zhang, X. Li, A. Shi, J. Zhu, X. Deng, B. Wang, Z. Zuo and G. Hu, Chem. Eng. J., 2023, 471, 144651 CrossRef
.
- L. Peng, W.-F. Zhou, W.-F. Xu, Y. Liu, C.-S. Zhou, J. Xie and K.-W. Tang, New J. Chem., 2023, 47, 6095–6101 RSC
.
- S. Jin, H. Chen, K. Pan, R. Li, X. Ma, R. Yuan, X. Meng and H. He, Talanta, 2024, 270, 125557 CrossRef PubMed
.
- J. Shi, W. Tang, B. Xiong, F. Gao and Q. Lu, Chem. Eng. J., 2023, 453, 139607 CrossRef
.
- Y. Feng, Y. Xu, S. Liu, D. Wu, Z. Su, G. Chen, J. Liu and G. Li, Coord. Chem. Rev., 2022, 459, 214414 CrossRef
.
- R. Yuan, Z. Fu, Y. He, Y. Deng, J. Xi, X. Xing and H. He, Talanta, 2023, 265, 124834 CrossRef PubMed
.
- A. P. Cote, A. I. Benin, N. W. Ockwig, M. O’Keeffe, A. J. Matzger and O. M. Yaghi, Nature, 2005, 310, 1166–1170 CAS
.
- P. W. Fritz and A. Coskun, Chem, 2020, 6, 1046–1048 CAS
.
- L. Cusin, H. Peng, A. Ciesielski and P. Samorì, Angew. Chem., Int. Ed., 2021, 60, 14236–14250 CrossRef CAS
.
- S. Xu, M. Richter and X. Feng, Acc. Mater. Res., 2021, 2, 252–265 CrossRef CAS
.
- R. K. Sharma, P. Yadav, M. Yadav, R. Gupta, P. Rana, A. Srivastava, R. Zbořil, R. S. Varma, M. Antonietti and M. B. Gawande, Mater. Horiz., 2020, 7, 411–454 RSC
.
- C. Yin, S. Fang, X. Shi, Z. Zhang and Y. Wang, J. Membr. Sci., 2021, 618, 118727 CrossRef CAS
.
- R. Qiu, J. Dai, L. Meng, H. Gao, M. Wu, F. Qi, J. Feng and H. Pan, Appl. Biochem. Biotechnol., 2022, 194, 3044–3065 CrossRef CAS PubMed
.
- L. Pei, Microporous Mesoporous Mater., 2022, 111742 CrossRef CAS
.
- R. Zhang, J. Yu, H. Chen, Y. Zhou, X. Yang, S. Wang and H. Bai, Microchem. J., 2023, 193, 109165 CrossRef CAS
.
- M. Gorbunova, V. Apyari, S. Dmitrienko and Y. Zolotov, TrAC, Trends Anal. Chem., 2020, 130, 115974 CrossRef CAS
.
- L. Wang, Y. Song, Y. Luo and L. Wang, J. Ind. Eng. Chem., 2022, 106, 374–381 CrossRef CAS
.
- A. Dreyer, A. Feld, A. Kornowski, E. D. Yilmaz, H. Noei, A. Meyer, T. Krekeler, C. Jiao, A. Stierle, V. Abetz, H. Weller and G. A. Schneider, Nat. Mater., 2016, 15, 522–528 CrossRef CAS PubMed
.
- A. El Mouden, N. El Messaoudi, A. El Guerraf, A. Bouich, V. Mehmeti, A. Lacherai, A. Jada and F. Sher, Chemosphere, 2023, 317, 137922 CrossRef PubMed
.
- M. Ghorbani, O. Seyedin and M. Aghamohammadhassan, J. Environ. Manage., 2020, 254, 109814 CrossRef PubMed
.
- Y. Gu, Z. Yang, J. Zhou, Q. Fang, X. Tan and Q. Long, Sep. Purif. Technol., 2024, 328, 125042 CrossRef
.
- X. Wei, S. Li, W. Wang, X. Zhang, W. Zhou, S. Xie and H. Liu, Adv. Sci., 2022, 9, 2200054 CrossRef
.
- M. Preeyanghaa, R. Dhanalakshmi, A. Aishwarya, M. Anpo, B. Neppolian and V. Sivamurugan, Catal. Rev. Sci. Eng., 2023, 1–71 Search PubMed
.
- D. Perl, S. J. Lee, A. Ferguson, G. B. Jameson and S. G. Telfer, Nat. Chem., 2023, 15, 1358–1364 CrossRef PubMed
.
- Y. Li, M. Chen, Y. Han, Y. Feng, Z. Zhang and B. Zhang, Chem. Mater., 2020, 32, 2532–2540 CrossRef
.
|
This journal is © The Royal Society of Chemistry 2025 |
Click here to see how this site uses Cookies. View our privacy policy here.