DOI:
10.1039/D4TB01737D
(Paper)
J. Mater. Chem. B, 2025,
13, 1302-1315
A controlled co-assembly approach to tune temperature responsiveness of biomimetic proteins†
Received
5th August 2024
, Accepted 19th November 2024
First published on 21st November 2024
Abstract
The controlled co-assembly of biomacromolecules through tuneable interactions offers a simple and fascinating opportunity to assemble multiple molecules into a single entity with enhanced complexity and unique properties. Herein, our study presents a dynamic co-assembled system using the multistimuli responsive intrinsically disordered protein Rec1-resilin and the adhesive hydrophilic protein silk sericin (SS). We utilized advanced characterization techniques including circular dichroism (CD) spectroscopy, dynamic light scattering (DLS), small-angle X-ray scattering (SAXS), and small/ultra-small angle neutron scattering (SANS/USANS) to elucidate the detailed co-assembly behavior of the system and its evolution over time and temperature. To achieve sufficient neutron contrast, we successfully biosynthesised deuterium-labeled Rec1-resilin (D-Rec1). Our research demonstrates that this co-assembly allows the formation of a robust entity with dynamic conformational assembly and disassembly, exhibiting both the upper critical solution temperature (UCST) and lower critical solution temperature (LCST) with reversibility. The assembly and disassembly dynamics of the co-assembled entity at UCST are very fast, while the process is kinetically controlled at LCST. This study provides significant new insights into the interplay of a hydrophilic, multi-responsive IDP and a highly hydrophilic protein, shaping the thermoresponsive and stable properties of the co-assembled entity.
Introduction
A vast array of complex structures with spectacular characteristics and functions such as hierarchical order, environmental responsiveness, adaptability, self-healing and bioactivity are created by nature using mild conditions through the process of self-assembly and supramolecular co-assembly from a broad range of raw materials, particularly various biomacromolecules, most specifically proteins.1 Inspired by the biological process, many investigators explored the programmed self-assembly and supramolecular assembly process of well-designed polymers, biopolymers and biomimetic polymers on nanometer to micrometer scales to develop bioinspired functional materials, with desired structures and unique functions. The mechanisms and control of the assembly process and polymorphism have also been explored.2 Proteins and peptides have been crucial for this advancement because they not only provide inspiration for assembly mechanisms and establish design guidelines for engineering intelligent materials3 but also serve as abundant sources of adaptable building blocks.4,5 The direct execution of numerous biological activities including signal transduction, cell growth, and immunology is attributed to protein self-assembly and supramolecular co-assemblies.6 Protein self-assemblies and supramolecular co-assemblies play a major role in the formation of highly ordered protein structures with subtle architectures, dynamic interactions and versatile functionalities, which are widely found in connective tissues and structural support components of cells such as collagens, elastin, silk, keratin, amyloid beta, and prions.7,8 Nature utilizes protein assemblies to achieve complex biological phenomena such as signal transduction, cell growth, and immune response.
The co-assembly of two different proteins forms a new frontier in material properties, where ‘protein A’ would co-assemble with ‘protein B’ to form a supramolecular architecture and conformation or dynamic conformational ensemble with enhanced complexity, dynamic properties and functionality.4,9–14 Controlled co-assembly is a powerful technique and has been successfully employed to create new biotechnologies for a variety of uses, such as drug delivery, sensors, and assisted charge transport.15–19 This multicomponent co-assembly is a spontaneous intermolecular non-covalent interaction (e.g., hydrogen bonding, electrostatic attraction, hydrophobic and hydrophilic interactions and van der Waals interactions) derived organization under near thermodynamic equilibrium conditions into stable arrangements. Due to the structural complexity of proteins and the nature of the self-assembly process, it is difficult to control and predict the size and morphology of the self-assembled nanomaterials.20 Surely, some impressive reviews have discussed the effectiveness of the different techniques used for the modelling and prediction of multicomponent assembly.21,22 Thus, to understand these systems completely, it is of paramount importance to co-assemble rationally designed peptides/proteins strategically and simultaneously characterise the multicomponent assemblies across multiple length-scales, from molecular interactions to bulk properties.
Herein, we investigate the co-assembly of two environmentally responsive biomimetic proteins, Rec1-resilin, a multi-stimuli-responsive intrinsically disordered protein (IDP); and silk sericin (SS), a responsive globular glycoprotein that functions as the glue. We investigate in detail how their co-assembly affects the conformational ensembles, aggregation, size, phase behaviour and conformational dynamics; and its impact on thermo-responsiveness using state-of-the-art experimental tools including dynamic light scattering and small/ultrasmall-angle neutron scattering (SANS/USANS). Our goal is to investigate the effect of the co-assembly on the conformational ensemble of the co-assembled system and its impact on the thermo-responsiveness. We biosynthesised deuterium-labelled Rec1-resilin (D-Rec1) to obtain adequate neutron contrast in the two proteins during the small/ultrasmall-angle neutron scattering (SANS/USANS) experiment.
Native resilin is an exoskeletal structural protein found in insects and other arthropods, and is characterized by low stiffness, high extensibility and extraordinary resilience; and professed to be the most efficient elastomeric protein known.23,24 Resilin-mimetic proteins (RMPs), specifically, Rec1-resilin, have attracted great interest due to their unusual structural characteristics, dynamics of conformational ensembles, multi-stimuli responsiveness and outstanding elasticity; and potential for use in a diverse range of applications in nanotechnology, drug delivery and medicine.23 Rec1-resilin, a recombinant version of resilin was first successfully cloned and expressed in 2005 by Chris et al.24 from the first exon of the Drosophila CG15920 gene. The consensus sequence of Rec1-resilin consists of 18 copies of a 15-residue repeat sequence: GGRPSDSYGAPGGGN, exhibiting high content of glycine (Gly) and proline (Pro), transpiring a profoundly hydrophilic IDP with unusual environmental responsiveness;24–26 contrary to conventional wisdom. The structural characteristics of Rec1-resilin at the amino acid sequence level and the resulting unique property attributes have led researchers to investigate diverse RMPs.23 These RMPs stand out as a unique class of IDP, with an unusual structure–function relationship; where they fail to form a stable structure, yet they exhibit unusual environmental responsiveness and biological activities. They are poorly understood and are attractive targets for modern protein research.27,28
Kindred to various extracellular matrix (ECM) environments of tissues, RMPs can also react to diverse parameters due to their multi-stimuli responsive behaviour; demonstrating responsiveness to pH, ion concentration, light and temperature in aqueous solution; including dual-phase behaviour (DPB) – displaying both upper critical temperature (UCST) and lower critical solution temperature (LCST).25,29–31 However, both the UCST (6 °C) and LCST (70 °C) of Rec1-resilin25 falls outside of the physiological temperature range, hindering the possibility of showcasing its stimuli-responsiveness for biological applications. Indeed, using RMPs as a biomaterial for tissue engineering applications would be beneficial where they have shown structural similarity to the ECM of tissues and are responsive to temperature relevant to physiological conditions.32
It has been demonstrated that for self-assembling proteins, the tunability of the LCST can be achieved through modulation of the hydrophobicity of the protein/polypeptide through relevant changes in amino acid residues at the sequence level.33,34 Additionally, the predictive knowledge of the relationship between amino acid residues at the sequence level and protein behaviour provides new avenues for the logical engineering of novel protein activities through the design of amino acid sequences.35 Nevertheless, the process of expressing and purifying a recombinant protein from a designed complementary DNA (cDNA) library is still a grand challenge36 and the potential to design a protein's distinct structure at the sequence level to produce dynamic, hierarchical functional materials is still a distant goal. Conversely, the co-assembly of carefully chosen individual proteins with the appropriate amino acid composition can result in supramolecular complexes whose constituent parts work together harmoniously to perform complicated functions.37 Comparable strategies have been explored for co-assembled protein systems, wherein hydrophobic peptides, proteins and polymers were introduced to modulate LCST.10,38 In a previous work10 we have shown that the recombinant resilin protein Rec1-resilin may be easily co-assembled into a rigid model polypeptide (poly-L-proline) or a hydrophobic rigid protein (Bombyx mori silk fibroin) to adjust its thermo-responsiveness. However, as noted by Liu et al.39 and Wu et al.,40 many variables – both compositional and environmental can dictate the properties and functions of these co-assemblies. Protein co-assembly is still in its infancy, and to regulate protein co-assembly by design and obtain desired functionality, new approaches, cutting-edge instruments, and advanced experimental methodologies must be used.19,41
SS is an abundant polymer, which is widely discarded during the degumming of silk, despite being a biocompatible material with a vast range of biomedical applications such as antioxidant and antibacterial activity.42 SS is structurally a globular protein that possesses β-sheet and random coil structural motifs that can interconvert depending on the environment (responsive to temperature, moisture and mechanical stretching).43–45 In addition to its adhesiveness, SS is chemically and biologically distinctive due to its low immunogenicity, pH responsiveness, antioxidant activity, biocompatibility, and capacity to stimulate cell division, antimicrobial properties, UV resistance, moisturising effect on skin and lowering cholesterol. Due to its unique qualities, such as its good cytocompatibility and ability to promote wound healing, SS films have garnered significant interest from researchers in the biomedical and cosmetic industries. These characteristics make SS valuable in a variety of industries, including food, drug delivery and biomedicine. Additionally, it can be easily transformed into biomaterials by copolymerization, cross-linking, and combining with other polymers.46
Experimental
Materials
SS from Bombyx mori (silkworm) and phosphate-buffered saline (PBS, pH 7.4) were obtained from Sigma-Aldrich, Australia. SS is a water-soluble globular glycoprotein, derived from the silkworm Bombyx mori, which is rich in hydrophilic amino acid residues serine, aspartic acid, and glycine.
Synthesis and purification of Rec1-resilin and D-Rec1
The resilin-mimetic polypeptide Rec1-resilin, was bio-synthesised and purified both as hydrogenated (unlabelled)47 and deuterated48 forms following previously reported strategies. Succinctly, the first exon of the Drosophila melanogaster CG15920 gene was cloned and over-expressed using recombinant DNA technology in a BL21*(DE3) pLysS Escherichia coli strain. Cells from a single colonies were then adapted to a ‘Mod C1’ media49 in H2O (for unlabelled) or 90% D2O (for D-Rec1) with kanamycin where glycerol was used as the carbon source. A Super Optimal Broth (S.O.C.) medium was used to culture the cells where a starter culture of the medium was used once an OD600 between 0.9 and 1.1 had been reached. Cells were then inoculated in a 2 L Minifors 2 bench-top bioreactor at 37 °C with pre-programmed parameters set to automatically regulate the pH, stirring speed and dissolved oxygen. The OD600 of the culture was noted at regular intervals to predict the growth and induction point, where the latter was achieved at an OD600 of 18. At the induction point, 1 mM isopropyl β-D-1-thiogalactopyranoside was added to induce the culture and express the desired protein, where the temperature was lowered to 30 °C. After 18 hours, the biomass was harvested and stored at −80 °C until purification. Purification of the protein was undertaken in three sequential non-chromatographic steps, specifically; salting-out (precipitation using 20% ammonium sulphate), followed by re-dissolution by overnight dialysis in PBS at 4 °C, and heat-precipitation of impurities at 80 °C for 10 min. Briefly, to elaborate, the harvested cell paste was resuspended in homogenization buffer containing 1.7% sodium chloride, 0.6% tris base, 6.2 mM benzamidine, 10.6 mM β-mercaptoethanol, 0.01% Triton X-100, 0.04% deoxyribonuclease I (10 mg mL−1), and 0.016% lysozyme. The suspension was then allowed to stir at 4 °C until lysis occurred. The lysed suspension was then sonicated on ice and subsequently clarified by centrifugation. Saturated ammonium sulfate was slowly added to the supernatant to a final concentration of 20% and stirred at 4 °C overnight. The suspension was centrifuged to pellet the protein. The protein pellet was then resuspended in chilled PBS and left to stir overnight to dissolve. The dialysate was clarified, and the supernatant was heated at 80 °C for 10 min. Subsequently, the denatured proteins were removed by centrifugation at 12
000 g for 15 min at 20 °C. The resulting supernatant obtained was identified as electrophoretically pure Rec1-resilin and D-Rec1 with molecular weights of 28.5 and 29.2 kDa respectively, shown in Fig. S1 (ESI†). The protein samples were stored at −20 °C before use.
Hydrophobicity determined by the Kyte–Doolittle plot
The Kyte–Doolittle plot was used to assess the hydrophobicity and hydrophilicity of proteins through analysis of their own unique amino acid sequence. Utilizing the sequences of the Kyte–Doolittle hydropathy plot enables a theoretical prediction of the hydrophobic value of the proteins through their web database (https://web.expasy.org/protscale/). The full protein sequences were obtained from the UniProt database (https://www.uniprot.org/).
Secondary structure analysis by circular dichroism (CD) spectroscopy
Rec1-resilin and SS co-assembled systems were prepared at a 50
:
50 volumetric ratio with a total concentration of 0.1 mg mL−1. Pure Rec1-resilin and SS systems were also prepared to a final concentration of 0.1 mg mL−1. All solutions were prepared in 1 mM PBS buffer to reduce the chances of a noisy spectrum and maintain a reliable HT value. All systems were aged over time up until 6 days to observe any structural changes, with incubation at 4 °C, 20 °C and 70 °C, respectively. CD spectra of the proteins were obtained using a JASCO spectropolarimeter (Jasco J-150, Jasco, Japan). Systems were measured at the incubation temperature, where heating and cooling were maintained using the Jasco MCB-100 Mini Circulation Bath. Prior to each scan, 15 min of equilibrium was given for each protein solution at their relevant incubation temperature. The experimental spectra were measured in the range of 190–260 nm. The data pitch was set at 0.2 nm, at a scanning speed of 50 nm min−1, a bandwidth of 1.0 nm, DIT of 1 s, and an accumulation of 10 scans. Dichroweb, an online fitting program, was used to extract the secondary structure information from the CD spectra for fitting.50
Size analysis by dynamic light scattering (DLS)
The hydrodynamic diameter (Dh) of the Rec1-resilin/SS co-assembled system was studied in quartz micro cuvettes from room temperature with increasing temperature using a Zetasizer (NanoZS, Malvern Panalytical Ltd Malvern, England). For DLS investigation a final solution of 10 mg mL−1 Rec1-resilin and SS was prepared in 5 mM PBS. The combined solutions were prepared at a 50
:
50 volumetric ratio, followed by stirring and sonication for homogeneous distribution of particles. The system was studied over a period of 0–6 days, incubated at 4 °C, where each temperature increment change was given a 5 min equilibration time. For size determination of temperature cycling measurements, the solution was heated from 4 °C to 70 °C in the same incremental steps as previously described, before being cooled back down to 4 °C in the same manner. The equilibration time was consistent throughout this process. Visual observation of phase transitions was photographed to distinguish the appearance of the system as the transitions occurred. The measured Dh values were averaged from a cumulative analysis of five cycles.
Hierarchical structure analysis using small-angle neutron scattering (SANS)
The hierarchical structure and organisation of the Rec1-resilin/SS co-assembled system in different length-scale at both UCST (5 °C) and LCST (75 °C) were investigated using the Quokka SANS instrument at ANSTO (20 MW OPAL research reactor, Sydney, Australia).51 A 70% deuterated Rec1-resilin (D-Rec1) with neutron scattering length density (SLD) of 4.64 × 10−6 Å−2 was synthesised (as reported in our previous publication48) and used in this study to provide sufficient contrast against hydrogenous SS (SLD of 2.21 × 10−6 Å−2) and water (SLD of −0.55 × 10−6 Å−2). Approximately 30 mg mL−1 co-assembled protein solutions prepared with D-Rec1
:
SS weight ratios of 1
:
1, 1
:
2 and 2
:
1 were loaded into Hellma cells (20 mm diameter and 1 mm path length). The sample to detector distances of 20, 12 and 1.3 m were used with an incident neutron wavelength of 5.0 Å and 8.1 Å (for lens optics configuration). The SANS data were measured in the scattering vector (q) range of 0.0007 to 0.7 Å, and the obtained scattering data were reduced, combined, and background subtracted using the IgorPro software package.52 Structural parameters of the samples were acquired using the SasView software package.
Structural analysis by small-angle X-ray scattering (SAXS)
The nanostructure of the Rec1
:
SS (1
:
1) co-assembled system along with pristine Rec1-resilin and SS was investigated (after 1 day and 6 days of equilibration at 4 and 20 °C) using a bench top Xeuss3.0 SAXS instrument (Xenocs Grenoble). The instrument was equipped with a rotating anode Cu Kα radiation source (1.42 Å) and a 2D detector. The samples (0.1 mg mL−1) were loaded in disposable quartz capillaries, and the SAXS data were collected at 20 ± 0.1 °C in the scattering vector (q) range of 0.01 to 0.5 Å−1. The background data were subtracted from the sample data using ATSAS software package for analysis.
Results and discussion
Hydrophobicity/hydrophilicity of the protein systems
The Kyte–Doolittle hydrophobicity plot53 of Rec1-resilin and SS is displayed in Fig. 1. Fig. 1a shows that the hydropathy score of Rec1-resilin is below zero in every amino acid segment level, with an average hydropathy score of about −1.24, demonstrating the hydrophilicity of the protein surface. Rec1-resilin protein's amino acid residues are primarily hydrophilic, with ∼33% (Ser ∼14.2%, Thr-1.6%, Asn-6.45%, Gln-3.87%, Tyr-6.45%) of them being uncharged and ∼11.5% (Asp ∼ 4% and Arg ∼5%) of them being charged polar (Fig. S2 and Table S1 in the ESI†) and hydrophilicity is reflected throughout the structure.
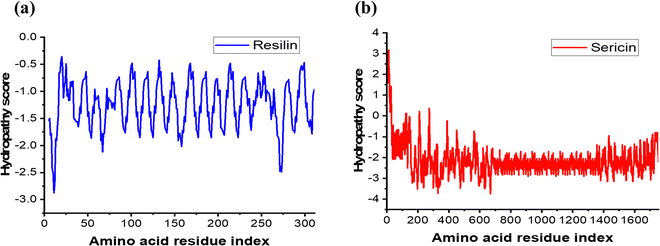 |
| Fig. 1 Kyte–Doolittle hydropathy plot of (a) Rec1-resilin and (b) B. mori silk sericin. The average hydropathy of a moving segment of a fixed window size of 7 is shown in the figure. | |
Fig. 1b shows the Kyte–Doolittle hydrophobicity plot of SS, which has an average hydropathy score of −2.16 reflecting hydrophilicity. SS is a globular hydrophilic protein composed of 18 amino acid residues the majority of which have polar side chains that primarily contain polar side chains made of hydroxyl, carboxyl, and amino groups (Fig. S3 and Table S2 in the ESI†).42,54 Collectively, hydroxy amino acid residues make up 45.8% of the SS structure, polar amino acids account for 42.3%, and nonpolar amino acids make up 12.2%. Compositionally, serine is the most abundant amino acid in SS (∼39 mol%), followed by glycine (∼15 mol%), and aspartic acid containing asparagine (∼15 mol%), which makes them hydrophilic. This is to note that native SS is a protein of complex composition. Based on its solubility, the composition of native-SS has been divided into three groups, respectively SS-A, SS-B, and SS-C.43 SS-A, which is insoluble in hot water forms the outer layer of the SS layer enveloping the fibroin core. The amino acid residues serine, threonine, aspartic acid, and glycine are the primary component observed in this layer with 17.2% of nitrogen. SS-B, the middle layer, has 16.8% nitrogen content and contains a set of amino acids resembling those in SS-A but more soluble in hot water. The last internal layer, known as SS-C, located close to the fibroin core contains 16.6% nitrogen sulphur and is strongly bound to the fibroin core. The SS used in this work is a water-soluble component and is essentially composed of SS-B and the amino acid sequence of SS-B is shown in Fig. S3 in the ESI.† The negative hydropathy score for both the polypeptides, Rec1-resilin and SS reflects that they are completely hydrophilic, however, there are significant differences in their amino acid composition, which is reflected in their secondary structures and properties. Conformationally, SS is constituted by a repeated amino acid sequence capable of forming the β-sheet structure, whereas Rec1-resilin is an IDP. Characteristically, Re1-resilin demonstrates temperature dependent dual phase behaviour – exhibiting both UCST and LCST;25 whereas SS displays thermo-responsive sol–gel transition due to the interconversion of random coil into the β-sheet structure.45 Understanding how co-assembly modifies the secondary structure of the co-assembled entity and its consequences on resilin's temperature phase transition is fascinating from a scientific standpoint and has never been attempted before. This knowledge also has significant practical importance in the application in biology, targeted delivery, and nanotechnology. To reveal the hierarchical organisational features of the co-assembled system in different length scales, we employed scattering (both light scattering and neutron scattering) techniques as follows.
Thermo-responsive characteristics of the Rec1-resilin/SS co-assembled entity
Dynamic light scattering (DLS) techniques were employed to examine the behaviour of the Rec1/SS co-assembled systems (1
:
1 ratio) at the micron level and above. Initially, the hydrodynamic diameter (Dh) of the two individual proteins was measured and shown in Fig. S4 in the ESI.† The Dh distribution plots display Dh values for Rec1-resilin (∼10 nm) and SS (∼10 nm), respectively, that corroborate with the previous literature reported values.25,55Fig. 2a displays the effect of temperature on the variation of Dh of the Rec1-resilin/SS co-assembled entity during the full thermal cycling step between 4 and 70 °C. The curve precisely illustrates the dynamics of the co-assembly pathways and occurrences of phase transitions (pH 7.4) as a function of temperature-both during heating and followed by successive cooling. It is evident from the figure that upon heating the co-assembled system from 4 °C (Fig. 2a), there appears to be a minute fluctuation in Dh, followed by a steep inflection point at 10 °C (UCST). Subsequently, the Dh value drastically decreases with a progressive rise in temperature until it reaches about 22 °C, at which point it adopts a steady size that is independent of temperate up to ∼52 °C. Notably, the hydrodynamic diameter (Dh) of the co-assembled entity composed of Rec1-resilin and SS is roughly 55 nm, in contrast to around 10 nm for the size of the native proteins. This observation implies that there are intermolecular interactions taking place between Rec1-resilin and SS. The pattern suggests the formation of a stable co-assembled individual entity occurs within the temperature range of 22 °C to 52 °C. A second transition during the first heating cycle (Fig. 2a) at ∼52 °C is characterised by a sharp increase in Dh with temperature, which is indicative of LCST behaviour.
 |
| Fig. 2 The change in hydrodynamic size (Dh) of Rec1-resilin/SS co-assembled systems measured using dynamic light scattering (DLS): (a) full thermal cycling between 4 and 70 °C, (b) UCST cycling between 4 °C and 30 °C and (c) LCST time-dependent recovery (75 °C to immediate cooling to 25 °C), and (d) visualization of the system during LCST cooling cycle. | |
Subsequently, the reversibility of the co-assembled entity's UCST behaviour was investigated by measuring Dh as a function of temperature covering the UCST (between 4 and 30 °C) zone in multiple cooling and heating cycles (Fig. 2b). In the cooling cycle at the UCST the dissociated chains initiate association and exhibit a sharp change in the Dh with an inflection point at ∼22 °C followed by a peak at ∼10.0 °C due to the formation of a swollen gel. Experiments involving repeated heating and cooling covering the UCST region verify that the transition is robust and fully reversible, with no hysteresis. Conversely, as seen in the behaviour at LCST (Fig. 2a), the pathways during the cooling phase did not align with those from the heating phase, and the Dh value exhibited considerable hysteresis. We further investigated the time-dependent recovery of post-LCST associated entity at room temperature (Fig. 2c). The figure clarifies that the dissociation process from an associated system post-LCST is kinetically controlled, and the co-assembled entity follows an exponential model, similar to that observed in the pristine Rec1-resilin system.25
This observation could additionally be seen visually (Fig. 2d), where the cooling cycle did not attain a uniform singular unit system at expected temperatures. Interestingly, this Rec1-resilin/SS co-assembled entity could remarkably retain the reversible dual phase behaviour equivalent to Rec1-resilin at both the UCST and LCST,25 though with some noticeable differences. Firstly, both the UCST and the LCST of the co-assembled entity shifted to ∼15 °C and ∼57 °C, respectively, relative to Rec1-resilin, which was reported to have UCST at ∼6 °C and LCST at ∼70 °C, respectively.25 Secondly, the size of the co-assembled entity post-UCST is much higher than pristine Rec1-resilin (∼10 nm). Thirdly, the aggregate size of the co-assembled entity at UCST is lower (<1000 nm) than Rec1-resilin (≫1000 nm). The kinetics of the dissociation process of the co-assembled entity post-LCST appears to be slower than Rec1-resilin.25
To examine the stability of the co-assembled entity, the Rec1-resilin/SS co-assembled systems were aged for a long period (0–6 days). The aliquots would be taken for particle size distribution measurements at a daily interval. The temperature range for the DLS measurement used covered both the UCST and LCST. Fig. S5 in the ESI,† shows the Rec1-resilin/SS co-assembled system Dh size measurements over an incubation period of 6 days heating from 4 °C to 70 °C. Overall, the particle size distribution illustrated a similar trend replicating over the incubation period and the temperature range, notably with the distinctive and identical dual phase transition behaviour, suggesting that co-assembled systems are highly stable under the storage conditions. Fig. 3 presents the visual observation of the temperature phase behaviour of the co-assembled Rec1-resilin/SS system at varying temperatures and incubation periods.
 |
| Fig. 3 Visual observation of the temperature phase behaviour of the co-assembled Rec1-resilin/SS system at varying temperatures and incubation periods. | |
It was compelling to note the temperatures at which the phase shifts (both LCST and UCST) of the co-assembled system occurred compared to the neat Rec1-resilin. The shift is more pronounced in the highly hydrophilic Rec1-resilin/SS co-assembled system than in the previously reported co-assembly of Rec1-resilin with hydrophobic–hydrophilic interactions.10 Intuitively, this is unanticipated, as studies have predominantly suggested that the phase transition behaviour is largely directed by the presence of a hydrophobic block and hydrophobic interaction.56 Although highly hydrophilic proteins have shown phase transition behaviours that undergo self-assembly driven by complex coacervation, these systems follow the principality of polyelectrolytes.57,58 Hence, nullified in the Rec1-resilin/SS co-assembled system studied; as both proteins display a net negative surface charge at the experimental pH.25,59 A prior study45 revealed the thermo-reversible characteristics of SS, where the protein showed rapid gelation with increasing concentrations (10–50 mg mL−1) and at higher temperatures (40 °C). The structural composition of SS shows that it is rich in hydrophilic amino acids, including serine, tyrosine, glutamic acid, aspartic acid, and lysine, which bestow SS with favourable water solubility. The amount of charged residues (Glu + Asp = 13% and Lys + Arg = 15%) confers to some regions of SS a higher degree of solvent accessibility and hydrophilicity. Approximately 45 mol% of SS is made up of amino acids having hydroxyl side chains, such as threonine and serine. Part of the consensus sequence of SS is [GSVSSTGSSSNTDSST]60,61 where G, S, T, V, N and D denote glycine, alanine, serine, threonine, valine, asparagine and aspartic acid, respectively. This part of the sequence of SS has the hydroxyl groups regularly on one side of the peptide backbone allowing SS to approach each other to form hydrogen bonds. A polar zipper interactions, driven by hydrogen bonds among various polar amino acids in the Ser-rich repeating motif, likely play a critical role in forming the β-sheet structure.62,63 Additionally, both Rec1-resilin and SS contain a large proportion –OH containing amino acid residues, serine; ∼15 mol% in Rec1-resilin (Table S1 in the ESI†) and ∼35 mol% in SS and (Table S2 in the ESI†) that have the potential to form inter and intramolecular hydrogen bonding. Given that SS's phase transition occurs at a notably lower temperature than that of Rec1-resilin (∼70 °C) the incorporation of SS results in a negative shift in the LCST of the co-assembled systems and the potential molecular level interactions are discussed later.
Hierarchical structure analysis of temperature phase behaviour by SANS
SANS is a versatile technique for investigating the structure and organisation of biological macromolecules (in the size range of 1 to few 100 nm64) in solution. In this work, SANS was used to study the hierarchical structure and organisation of Rec1-resilin/SS co-assembled systems at UCST and LCST. Fig. 4 compares the SANS intensity profile of deuterated-Rec1-resilin/SS (D-Rec1:SS) co-assembled systems with different D-Rec1
:
SS weight ratios of 2
:
1, 1
:
1, and 1
:
2 at 5 and 75 °C, respectively, in water. The scattering profile of the co-assembled systems (all three ratios) exhibited three distinct regions at the UCST (Fig. 4a): a high-q Porod region (0.06 < q < 0.1 Å−1), a mid-q Guinier-like region (0.02 < q < 0.06 Å−1), and a low-q Porod-like region (0.0007 < q < 0.02 Å−1). On the other hand, the scattering profile of co-assembled systems (all three ratios) exhibited two distinct regions at the LCST (Fig. 4b): a mid-q to high-q Porod region (0.06 < q < 0.1 Å−1) and a low-q to mid-q Porod-like region (0.0007 < q < 0.02 Å−1). The lack of the Guinier region at low-q (i.e. the observed increase in the scattering intensity with a decrease in the q value) indicates the structures formed at both UCST and LCST are beyond the size range of SANS measurement, which is in good agreement with the DLS results. SANS intensity profiles of D-Rec1 in water and a fit of the data with model function to estimate structural parameters are presented in Fig. S6a (ESI†). The radius of gyration (Rg) of D-Rec1 has been established as 6.2 ± 0.1 nm.48 The Rg of SS was estimated in this work from the respective SANS data measured at 25 °C (Fig. S6b in the ESI†). The scattering profile of SS in water (10 mM PBS) exhibited two distinct regions: a high-q Porod region (0.07 < q < 0.3 Å−1), and a mid-q Guinier-like region (0.006 < q < 0.07 Å−1), and a low-q Porod-like region (0.0007 < q < 0.02 Å−1). Therefore, a shape-independent Poly_Gauss_Coil model function (which describes the scattering from polydisperse polymer chains in theta solvents65) was fitted to the data to obtain the Rg (Fig. S6 in the ESI†). The Poly Gauss_Coil fit returned a Rg of 1.8 ± 0.1 nm, which when compared with the measured (using DLS) hydrodynamic diameter of ∼10 nm indicates that the SS molecules stabilise as a multimer through intermolecular interaction in water. The measured (using DLS) hydrodynamic diameter of ∼55 nm for Rec1-resilin/SS (1
:
1) co-assembly at 25 °C indicates intermolecular interaction between Rec1-resilin and SS to form stable nano-aggregates.
 |
| Fig. 4 SANS intensity profile of the D-Rec1 : SS co-assembled system (at 2 : 1, 1 : 1, and 1 : 2 weight ratios) compared at (a) UCST and (b) LCST. | |
To estimate the structural parameters of the D-Rec1:SS co-assembled system at both UCST and LCST, a shape-independent Guinier–Porod model function (which describes scattering from a generalised Guinier/power law object66) was fitted to the mid-q and high-q data to obtain the Rg, whereas a power law function fit to low-q data to obtain the fractal dimension of aggregates (Fig. 5). The structural parameters estimated from the fits are provided in Table 1. In general, the co-assemblies exhibited Gaussian coil intrinsic structure and relatively smaller Rg at UCST compared to the fully swollen coil intrinsic structure and relatively larger Rg at LCST. Moreover, the co-assemblies exhibited surface fractal dimension (Porod slope between 3 and 4; i.e., relatively packed aggregates) at UCST, whereas mass-fractal dimension (Porod slope between 2 to 3; i.e., relatively loose network aggregates) at the LCST. While the Rg was observed to decrease with an increase in the SS content of co-assemblies, no significant difference in the fractal dimension of aggregates was observed.
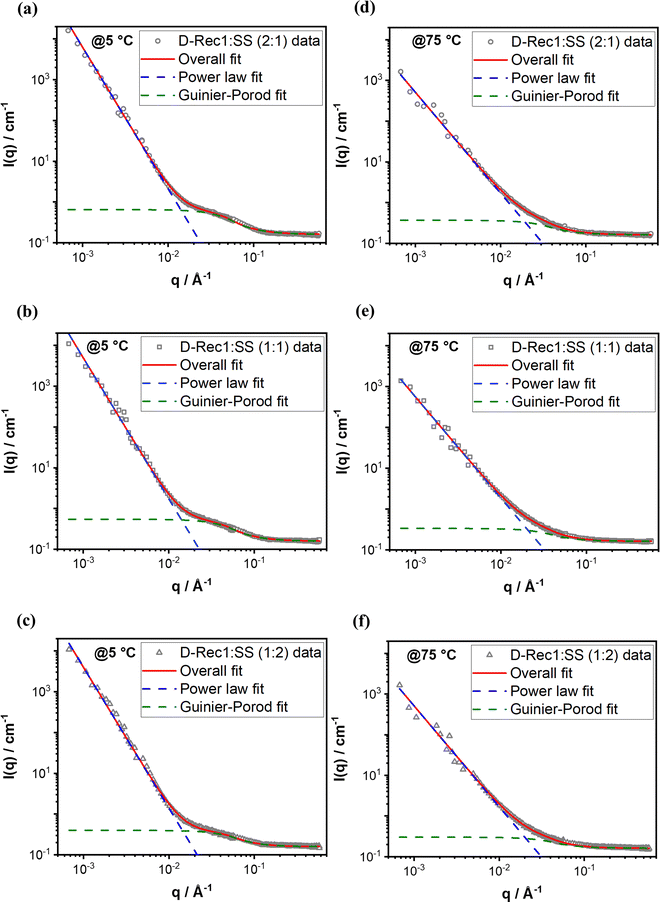 |
| Fig. 5 Shape-independent model functions fit to SANS data of D-Rec1:SS co-assembled system at UCST and LCST: (a) D-Rec1 : SS (2 : 1) at 5 °C, (b) D-Rec1 : SS (1 : 1) at 5 °C, (c) D-Rec1 : SS (1 : 2) at 5 °C, (d) D-Rec1 : SS (2 : 1) at 75 °C, (e) D-Rec1 : SS (1 : 1) at 75 °C, and (f) D-Rec1 : SS (1 : 2) at 75 °C. | |
Table 1 Structural parameters estimated from shape-independent model function fits applied to SANS data of aqueous D-Rec1:SS co-assembled systems at upper and lower critical solution temperatures
Sample |
Temperature (°C) |
Low-q (power law fit) |
Mid-q & high-q (Guinier–Porod fit) |
Exponent/slope |
R
g (nm) |
Exponent/slope |
D-Rec1 : SS (2 : 1) |
5 |
3.47 ± 0.01 |
3.05 ± 0.03 |
1.99 ± 0.06 |
75 |
2.48 ± 0.02 |
4.28 ± 0.14 |
1.70 ± 0.01 |
D-Rec1 : SS (1 : 1) |
5 |
3.46 ± 0.01 |
2.97 ± 0.04 |
1.99 ± 0.07 |
75 |
2.48 ± 0.02 |
4.10 ± 0.16 |
1.70 ± 0.01 |
D-Rec1 : SS (1 : 2) |
5 |
3.45 ± 0.02 |
2.71 ± 0.06 |
1.98 ± 0.09 |
75 |
2.48 ± 0.03 |
3.57 ± 0.15 |
1.70 ± 0.01 |
Structural changes of Rec1-resilin/SS after co-assembly
To examine the influence of SS on the co-assembly of the Rec1-resilin system, CD spectroscopy was employed to understand secondary structural changes as a function of temperature and incubation. Rec1-resilin and SS were prepared individually and as co-assembled solutions, incubated at 4 °C, 20 °C, and 70 °C and subjected to examination at their respective temperatures at day 1 and day 6 of incubation. Fig. 6 illustrates the CD spectra of all three solutions at different experimental temperatures and time points, while Tables S3 and S4 (ESI†) provide a quantitative estimation of the predicted secondary structures derived from deconvolution fits obtained using the CONTIN67 algorithm under the specified conditions. A large negative band at ∼195–200 nm was observed for Rec1-resilin, indicating an unordered structure arising from its known IDP characteristic.29 Similarly, SS also displayed a random-coiled structure, however, with lesser intensity, demonstrating a marginally greater presence of β-sheet and α-helix structures (Table S3 in the ESI†).68 When compared to the two native proteins, the co-assembled system at all three incubation temperatures on day 1 exhibited a trend similar to that of the individual proteins and followed the principle of additivity. The large negative intensity band of the co-assembled system appears to be approximately equidistant in the random-coiled region between Rec1-resilin and SS. This is to note that β-sheet organisation in SS due to moisture absorption and mechanical elongation has been previously reported.69,70 The experimental observation demonstrates that the two proteins have undoubtedly co-assembled in a manner that conserved the most prominent secondary structure, which is associated with the conformational flexibility of the proteins.26,29,68 However, on the final day (6th day) of incubation, it could be noted that the Rec1-resilin protein presented signs of denaturation, where the primarily unordered structure transformed into an ordered secondary form, with a significant increase in both α-helix and β-sheet structures (Fig. 6e).
 |
| Fig. 6 CD spectra of protein systems after 1 day incubation at (a) 4 °C, (b) 20 °C, and (c) 70 °C and 6 day incubation at (d) 4 °C, (e) 20 °C and (f) 70 °C. | |
To gain a deeper understanding of how the secondary structure transitions with varying incubation periods, we conducted a comprehensive study over a span of 7 days at 20 °C across all protein systems. The observation demonstrated that this change for Rec1-resilin began at day 4 of incubation (Fig. 7a), where it would gradually lose its inherent secondary structure. Unlike Rec1-resilin, the SS solution displayed a stable form (Fig. 7b), which in turn aided the co-assembled system in extending its shelf-life stability to retain its secondary structure (Fig. 7c). Compellingly, at both low temperatures, below UCST (Fig. 6d), and high temperatures, above LCST (Fig. 6f), during the incubation periods, there was no indication of any significant secondary structural changes to any of the proteins and their co-assembled entity. Though, previous reports suggested the sol–gel properties of sericin upon heating, which led the random-coil structure to subsequently become a β-sheet.42,69 Accordingly, we postulate that the proteins can maintain their secondary structures at the extreme temperatures of incubation and analysis, or they may have influenced the resulting structures exhibited due to the presence of aggregated units, reflecting what was found in DLS measurements.
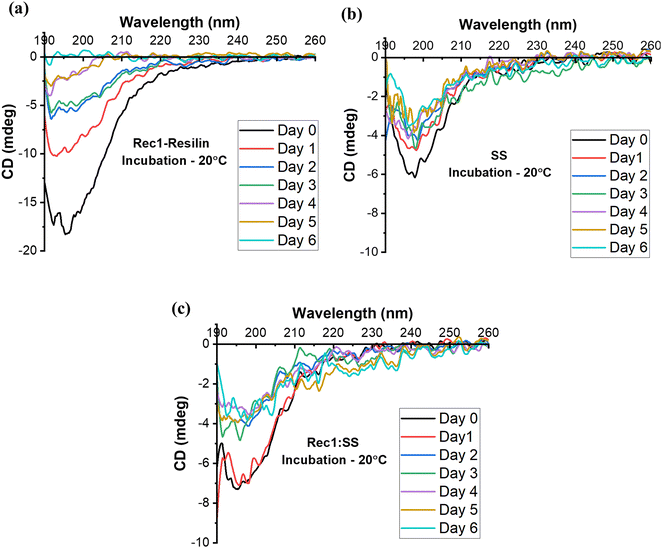 |
| Fig. 7 CD spectra of protein systems (a) Rec1-resilin, (b) SS, and (c) Rec1-resilin and SS co-assembled system at 20 °C, at incubation periods ranging from 0–6 days. | |
Fig. S7 in the ESI,† shows the SAXS intensity profile of the Rec1-resilin/SS co-assembled system with a 1
:
1 ratio compared with pristine Rec1-resilin and SS after 1 and 6 days of equilibration at UCST (4 °C) and room temperature (20 °C). It can be observed that pristine SS and Rec1
:
SS (1
:
1) co-assembled system showed no significant difference in scattering when equilibrated at UCST and room temperature. The pristine Rec1-resilin exhibited no significant difference in scattering when equilibrated at UCST, however, it formed aggregates (increased low-q scattering intensity, Fig. S7d in the ESI†) after 6 days at room temperature. Therefore, the SAXS results are in general agreement with CD spectroscopy results.
Primary amino acid sequence and co-assembly
As observed from Fig. 2–4 the size and solubility of co-assembled Rec1-resilin/SS is a complex phenomenon, and the complexity presumably arises due to the presence of the ionizable amino acid residues. We have established and reported with detailed experimental evidence that Rec1-resilin is an IDP and displays unusual multi-stimuli responsiveness including a dual phase transition-displaying both an UCST and an LCST.25 Noticeably, many native low-complexity proteins (LCPs) and proteins with low-complexity regions (LCRs) are known for their sensitivity to environmental changes. When exposed to variations in temperature, pH, and ion concentration, these proteins can exhibit phase separation in vitro.71 Evidently, such LCPs/LCRs play significant roles in facilitating the compartmentalization of cells via the creation of biomolecular condensates72–75 and are important players in signaling.76 A loose framework for understanding the phase behavior of LCPs has been advanced71,77–79 using the principles of polymer physics and the thermodynamics of mixing and demixing. However, how this behavior is encoded in the amino acid primary composition and sequence remains unclear. Based on the available experimental data, it has been suggested that the solubility of an LCP/LCR under typical solution conditions, as well as its tendency to demix with either UCST and/or LCST behavior depends on the balance between hydrophobic and polar or charged amino acids and their distribution in the sequence. Polar LCPs/LCRs containing a high fraction of serine, glutamine, asparagine and glycine (properties are dominated by its polar backbone), have been reported to phase separate homotypically in vitro when the sequence is punctuated by aromatic and charged amino acids.71,80,81
Fig. 8 shows the consensus primary amino acid repeat sequence and amino acid fraction in Rec1-resilin and SS. Rec1-resilin is an intrinsically disordered protein and its primary structural composition is mainly dominated by 18 copies of a 15-residue repeat sequence: GGRPSDSYGAPGGGN (Fig. 8a and b). It contains a very high content of Gly (∼34 mol%) and the amino acid residues in this recombinant protein are dominated by polar residues including both uncharged, ∼23% (Ser ∼14.2%, Thr-1.6%, Asn-6.45%, Gln-3.87%) and charged polar, ∼11.5% (with Asp ∼4% and Arg ∼5%) hydrophilic residues punctuated with aromatic amino acid residue Tyr (∼6.5%) (Fig. 8c). Due to this unique primary structure of Rec1-resilin liquid/liquid demixing and aggregation at UCST is driven by the electrostatic interactions between positively and negatively charged amino acid repeats. These interactions are amplified by localized alterations in protein dynamics influenced by the water dynamics in the first hydration shell. A reduction in temperature facilitates the ordering of the hydration water molecules, ultimately resulting in protein aggregation through phase separation at ∼6 °C. In a manner similar to Rec1-resilin, the SS sequence contains a high proportion of charged and polar residues (Fig. 8d–f) that impart certain regions of SS with greater solvent accessibility and hydrophilicity. As noted from SANS (Fig. S6 in ESI†) and DLS, in solution SS stabilizes as a multimeric protein. When Rec1-resilin and SS are uniformly mixed in a solution (LCST〈T〉UCST) they form a stable, co-assembled nano-aggregate ∼55 nm in size (Fig. 2a) due to intermolecular interactions without major secondary structural change (Fig. 6a). This co-assembled structure shows a marginal increase in the UCST to ∼15 °C due to the rise in the fraction of overall charged polar amino acids.
 |
| Fig. 8 The primary amino acid repeat sequence and consensus, and amino acid fraction in Rec1-resilin and silk sericin. Single-letter code is used to display the consensus sequences of (a) Rec1-resilin and (d) silk sericin (part of the consensus sequence). Distribution of glycine, polar, aromatic, positively charged negatively charged, hydrophobic in Rec1-resilin (c) and silk sericin (e) in the primary structural sequence, residues coloured orange, golden yellow, light green, purple, black, and forest green, respectively. The overall amino acid fractions for each protein are displayed as a pie chart with similar colouring: (c) Rec1-resilin and (f) silk sericin. | |
The LCST behaviour exhibited by Rec1-resilin at ∼70 °C is attributed to the reduction in the hydration layer around the hydrophilic segment and the intermolecular hydrophobic interaction of the exposed hydrophobic amino acid segments.25 Due to the low fraction of hydrophobic segments present, at LCST, Rec1-resilin forms micro-gel particles rather than a dense gel network.25 The observed decrease in the LCST of the co-assembled nano-aggregate entity of Rec1-resilin/SS from 70 °C to ∼57 °C (Fig. 2a) is counterintuitive. Nonetheless, as elaborated in Fig. 8, both Rec1-resilin and SS comprise a significant percentage of amino acid residues with –OH groups (Ser, Thr), which are predominantly placed on one side of the peptide backbone. Therefore, in Rec1-resilin/SS co-assembled system it is postulated that, while LCST is seeded by hydrophobic interactions, the hydrogen bonds formed by several polar amino acids in the Ser-rich repeating sequence (polar zipper interactions), contribute to the negative shift in LCST. This intermolecular interaction could potentially alter the molecular dynamics of the co-assembled structure from being independently exclusive to mutually cooperative, thus reducing LCST, although there are no notable changes in secondary structure observed.
Our investigation revealed that, despite the inherent challenges associated with a hydrophilic-hydrophilic co-assembled system, the Rec1-resilin/SS system exhibited promising results that enhanced the tunability of the Rec1-resilin protein. This study illustrated that the engineering of stimuli responses can be achieved through the co-assembly of proteins, enabling the straightforward design of materials of interest for various biological and non-biological applications. This innovative possibility also presents several challenges in comprehensively gaining molecular insights into alterations in the local molecular environment and long-range order during the transitions of LCST and UCST. Molecular dynamics simulations at both the atomistic and mesoscale levels can be instrumental in gaining a deeper level understanding of the structural and dynamic characteristics of these conjugates within a temperature range associated with their UCST/LCST-like phase behavior; though this aspect falls outside the focus of this work.
Conclusions
In summary, this study demonstrated that the co-assembly between a multistimuli responsive intrinsically disordered protein, Rec1-resilin and a hydrophilic SS protein, enabled the formation of a robust system with dynamic conformational assembly and disassembly with a tuneable and reversible DPB. The co-assembled system was able to shift Rec1-resilin's DPB from ∼6 to ∼15 °C at its UCST and from ∼70 to ∼57 °C at its LCST. However, the co-assembled system demonstrated slower kinetic reversibility (exponential model) at its LCST compared to UCST. Although, it was proposed that despite SS being hydrophilic, such a decrease in the co-assembled systems at its LCST could arise from intermolecular interaction changing the molecular dynamics of the entity. The ornate structural analysis evaluated through SANS and SAXS confirmed such intermolecular interaction, where different structures were observed at the UCST (relatively packed aggregates) and LCST (relatively loose network aggregates). CD analyses confirm that the addition of the SS protein further prolonged the shelf-life of Rec1-resilin creating a more stable structure, without any conformational change. Therefore, such tuneability in the phase behaviour of the co-assembled system presents valuable insights into how a hydrophilic multi-responsive IDP interacts with another hydrophilic protein in a facile manner that has the potential to be exploited for biomedical applications such as drug delivery or injectable biomaterials.
Author contributions
The manuscript was written through contributions of all authors. All authors have given approval to the final version of the manuscript.
Data availability
The data supporting this article have been included as part of the ESI.†
Conflicts of interest
There are no conflicts to declare.
Acknowledgements
The authors would like to acknowledge the financial support from the Australia India Strategic Research Fund (AISRF) AIRXIV000048. The authors also acknowledge the School of Graduate Research (SGR), RMIT University, for providing the PhD scholarship and AINSE Ltd. for the Postgraduate Research Award (PGRA) for Nisal Wanasingha. The authors acknowledge the facilities and the scientific and technical assistance of the RMIT Micro Nano Research Facility (MNRF). We are thankful to Robert Knott for his support in performing all the SAXS experiments. Access to the National Deuteration Facility (NDF) at ANSTO and the SANS facility at the Australian Centre for Neutron Scattering was supported through the ANSTO beam time award (P9853). NDF is partially supported by the National Collaborative Research Infrastructure Strategy (NCRIS), which is the Federal Government's initiative. This work benefited from the use of the free SasView application (https://www.sasview.org/).
References
- C. Gao and G. Chen, Acc. Chem. Res., 2020, 53, 740–751 CrossRef CAS PubMed.
- L. Li, R. Sun and R. Zheng, Mater. Des., 2021, 197, 109209 CrossRef CAS.
- M. I. Jacobs, P. Bansal, D. Shukla and C. M. Schroeder, ACS Cent. Sci., 2022, 8, 1350–1361 CrossRef CAS PubMed.
- B. O. Okesola and A. Mata, Chem. Soc. Rev., 2018, 47, 3721–3736 RSC.
- Y. Li, J. Liu, H. Zhang, X. Shi, S. Li, M. Yang, T. Zhang, H. Xiao and Z. Du, J. Agric. Food Chem., 2023, 71, 11304–11319 CrossRef CAS PubMed.
- Y. Bai, Q. Luo and J. Liu, Chem. Soc. Rev., 2016, 45, 2756–2767 RSC.
- Y. Sasai, Nature, 2013, 493, 318–326 CrossRef CAS PubMed.
- A. Solomonov, A. Kozell and U. Shimanovich, Angew. Chem., Int. Ed., 2024, 63, e202318365 CrossRef CAS PubMed.
- M. Abdelghani, J. Shao, D. H. Le, H. Wu and J. C. van Hest, Macromol. Biosci., 2021, 21, 2100081 CrossRef CAS PubMed.
- J. L. Whittaker, N. K. Dutta, R. Knott, G. McPhee, N. H. Voelcker, C. Elvin, A. Hill and N. R. Choudhury, Langmuir, 2015, 31, 8882–8891 CrossRef CAS PubMed.
- D. M. Raymond and B. L. Nilsson, Chem. Soc. Rev., 2018, 47, 3659–3720 RSC.
- B. J. Pieters, M. B. Van Eldijk, R. J. Nolte and J. Mecinović, Chem. Soc. Rev., 2016, 45, 24–39 RSC.
- H. Garcia-Seisdedos, C. Empereur-Mot, N. Elad and E. D. Levy, Nature, 2017, 548, 244–247 CrossRef CAS PubMed.
- J. A. Marsh and S. A. Teichmann, Annu. Rev. Biochem., 2015, 84, 551–575 CrossRef CAS PubMed.
- X. Liu, T. Fu, J. Ward, H. Gao, B. Yin, T. Woodard, D. R. Lovley and J. Yao, Adv. Electron. Mater., 2020, 6, 2000721 CrossRef CAS.
- T. Fu, X. Liu, H. Gao, J. Ward, X. Liu, B. Yin, Z. Wang, Y. Zhuo, D. Walker and J. J. Yang, Nat. Commun., 2020, 11, 1861 CrossRef CAS PubMed.
- M. Wang, J. A. Zuris, F. Meng, H. Rees, S. Sun, P. Deng, Y. Han, X. Gao, D. Pouli and Q. Wu, Proc. Natl. Acad. Sci. U. S. A., 2016, 113, 2868–2873 CrossRef CAS PubMed.
- C. A. Stevens, K. Kaur and H.-A. Klok, Adv. Drug Delivery Rev., 2021, 174, 447–460 CrossRef CAS PubMed.
- J. Zhu, N. Avakyan, A. Kakkis, A. M. Hoffnagle, K. Han, Y. Li, Z. Zhang, T. S. Choi, Y. Na and C.-J. Yu, Chem. Rev., 2021, 121, 13701–13796 CrossRef CAS PubMed.
- X. Wang, P. Gao, Y. Yang, H. Guo and D. Wu, Nat. Commun., 2018, 9, 2772 CrossRef PubMed.
- P. Makam and E. Gazit, Chem. Soc. Rev., 2018, 47, 3406–3420 RSC.
- N. H. Kim, H. Choi, Z. M. Shahzad, H. Ki, J. Lee, H. Chae and Y. H. Kim, Nano Convergence, 2022, 9, 1–17 CrossRef PubMed.
- R. Balu, N. K. Dutta, A. K. Dutta and N. R. Choudhury, Nat. Commun., 2021, 12, 149 CrossRef CAS PubMed.
- C. M. Elvin, A. G. Carr, M. G. Huson, J. M. Maxwell, R. D. Pearson, T. Vuocolo, N. E. Liyou, D. C. Wong, D. J. Merritt and N. E. Dixon, Nature, 2005, 437, 999–1002 CrossRef CAS PubMed.
- N. K. Dutta, M. Y. Truong, S. Mayavan, N. Roy Choudhury, C. M. Elvin, M. Kim, R. Knott, K. M. Nairn and A. J. Hill, Angew. Chem., Int. Ed., 2011, 50, 4428–4431 CrossRef CAS PubMed.
- G. Qin, X. Hu, P. Cebe and D. L. Kaplan, Nat. Commun., 2012, 3, 1003 CrossRef PubMed.
- V. N. Uversky, Chem. Rev., 2014, 114, 6557–6560 CrossRef CAS PubMed.
- C. J. Oldfield and A. K. Dunker, Annu. Rev. Biochem., 2014, 83, 553–584 CrossRef CAS PubMed.
- R. Balu, R. Knott, N. P. Cowieson, C. M. Elvin, A. Hill, N. R. Choudhury and N. K. Dutta, Sci. Rep., 2015, 5, 10896 CrossRef CAS PubMed.
- R. Balu, N. K. Dutta, N. R. Choudhury, C. M. Elvin, R. E. Lyons, R. Knott and A. J. Hill, Acta Biomater., 2014, 10, 4768–4777 CrossRef CAS PubMed.
- R. Balu, J. P. Mata, R. Knott, C. M. Elvin, A. J. Hill, N. R. Choudhury and N. K. Dutta, J. Phys. Chem. B, 2016, 120, 6490–6503 CrossRef CAS PubMed.
- L. Li, A. Mahara, Z. Tong, E. A. Levenson, C. L. McGann, X. Jia, T. Yamaoka and K. L. Kiick, Adv. Healthcare Mater., 2016, 5, 266–275 CrossRef CAS PubMed.
- F. G. Quiroz and A. Chilkoti, Nat. Mater., 2015, 14, 1164–1171 CrossRef CAS PubMed.
- J. A. MacKay, D. J. Callahan, K. N. FitzGerald and A. Chilkoti, Biomacromolecules, 2010, 11, 2873–2879 CrossRef CAS PubMed.
- B. Kuhlman and P. Bradley, Nat. Rev. Mol. Cell Biol., 2019, 20, 681–697 CrossRef CAS PubMed.
- B. Jia and C. O. Jeon, Open Biol., 2016, 6, 160196 CrossRef PubMed.
- S. A. Farhadi, A. Restuccia, A. Sorrentino, A. Cruz-Sánchez and G. A. Hudalla, Mol. Syst. Des. Eng., 2022, 7, 44–57 RSC.
- A. García-Peñas, C. S. Biswas, W. Liang, Y. Wang, P. Yang and F. J. Stadler, Polymers, 2019, 11, 991 CrossRef PubMed.
- J. Liu, H. He, Z. Yu, A. Suryawanshi, Y. Li, X. Lin and Z. Sun, Text. Res. J., 2020, 90, 2622–2638 CrossRef CAS.
- W. Wu, W. Li, L. Q. Wang, K. Tu and W. Sun, Polym. Int., 2006, 55, 513–519 CrossRef CAS.
- V. P. Gray, C. D. Amelung, I. J. Duti, E. G. Laudermilch, R. A. Letteri and K. J. Lampe, Acta Biomater., 2022, 140, 43–75 CrossRef CAS PubMed.
- R. I. Kunz, R. M. C. Brancalhão, L. D. F. C. Ribeiro and M. R. M. Natali, BioMed Res. Int., 2016, 2016, 8175701–8175719 Search PubMed.
- Y. Takasu, H. Yamada and K. Tsubouchi, Biosci., Biotechnol., Biochem., 2002, 66, 2715–2718 CrossRef CAS PubMed.
- P. Aramwit, S. Kanokpanont, T. Nakpheng and T. Srichana, Int. J. Mol. Sci., 2010, 11, 2200–2211 CrossRef CAS PubMed.
- L. J. Zhu, M. Arai and K. Hirabayashi, J. Sericult. Sci. Jpn., 1995, 64, 415–419 CAS.
- C. Yang, L. Yao and L. Zhang, Smart Mater. Med., 2023, 4, 447–459 CrossRef.
- R. E. Lyons, E. Lesieur, M. Kim, D. C. Wong, M. G. Huson, K. M. Nairn, A. G. Brownlee, R. D. Pearson and C. M. Elvin, Protein Eng., Des. Sel., 2007, 20, 25–32 CrossRef CAS PubMed.
- R. Balu, N. Wanasingha, J. P. Mata, A. Rekas, S. Barrett, G. Dumsday, A. W. Thornton, A. J. Hill, N. Roy Choudhury and N. K. Dutta, Sci. Adv., 2022, 8, eabq2202 CrossRef CAS PubMed.
-
A. P. Duff, K. L. Wilde, A. Rekas, V. Lake and P. J. Holden, Methods in enzymology, Elsevier, 2015, vol. 565, pp. 3–25 Search PubMed.
- A. J. Miles, S. G. Ramalli and B. A. Wallace, Protein Sci., 2021, 31, 37–46 CrossRef PubMed.
- K. Wood, J. P. Mata, C. J. Garvey, C.-M. Wu, W. A. Hamilton, P. Abbeywick, D. Bartlett, F. Bartsch, P. Baxter and N. Booth, J. Appl. Crystallogr., 2018, 51, 294–314 CrossRef CAS.
- S. R. Kline, J. Appl. Crystallogr., 2006, 39, 895–900 CrossRef CAS.
- J. Kyte and R. F. Doolittle, J. Mol. Biol., 1982, 157, 105–132 CrossRef CAS PubMed.
- M. Saad, L. M. El-Samad, R. A. Gomaa, M. Augustyniak and M. A. Hassan, Int. J. Biol. Macromol., 2023, 250, 126067 CrossRef CAS PubMed.
- M. Yang, Y. Shuai, G. Zhou, N. Mandal, L. Zhu and C. Mao, ACS Appl. Mater. Interfaces, 2014, 6, 13782–13789 CrossRef CAS PubMed.
-
R. Balu, N. K. Dutta and N. R. Choudhury, in Biomimetic Protein Based Elastomers: Emerging Materials for the Future, ed. N. R. Choudhury, J. C. Liu and N. K. Dutta, The Royal Society of Chemistry, 2022 10.1039/9781788012720-00108.
- B. Jing, M. Ferreira, Y. Gao, C. Wood, R. Li, M. Fukuto, T. Liu and Y. Zhu, Macromolecules, 2019, 52, 8275–8284 CrossRef CAS.
- D. Priftis, L. Leon, Z. Song, S. L. Perry, K. O. Margossian, A. Tropnikova, J. Cheng and M. Tirrell, Angew. Chem., Int. Ed., 2015, 54, 11128–11132 CrossRef CAS PubMed.
- Y. Zhang, Y. Zhao, X. He, A. Fang, R. Jiang, T. Wu, H. Chen, X. Cao, P. Liang and D. Xia, Polym. Test., 2019, 80, 106016 CrossRef CAS.
- K. H. Lee, Macromol. Rapid Commun., 2004, 25, 1792–1796 CrossRef CAS.
- A. Garel, G. Deleage and J.-C. Prudhomme, Insect Biochem. Mol. Biol., 1997, 27, 469–477 CrossRef CAS PubMed.
- J. Huang, R. Valluzzi, E. Bini, B. Vernaglia and D. L. Kaplan, J. Biol. Chem., 2003, 278, 46117–46123 CrossRef CAS PubMed.
- S. C. Kundu, B. C. Dash, R. Dash and D. L. Kaplan, Prog. Polym. Sci., 2008, 33, 998–1012 CrossRef CAS.
- D. I. Svergun and M. H. Koch, Rep. Prog. Phys., 2003, 66, 1735 CrossRef CAS.
-
O. Glatter and O. Kratky, Small angle X-ray scattering, Academic Press, 1982, p. 404 Search PubMed.
- B. Hammouda, J. Appl. Crystallogr., 2010, 43, 716–719 CrossRef CAS.
- I. H. van Stokkum, H. J. Spoelder, M. Bloemendal, R. Van Grondelle and F. C. Groen, Anal. Biochem., 1990, 191, 110–118 CrossRef CAS PubMed.
- A. Omar, Y. Gao, A. Wubulikasimu, A. Arken, H. A. Aisa and A. Yili, RSC Adv., 2021, 11, 25431–25440 RSC.
- L. Chen, J. Hu, J. Ran, X. Shen and H. Tong, RSC Adv., 2015, 5, 56410–56422 RSC.
- M. A. Hassan, A. A. Basha, M. Eraky, E. Abbas and L. M. El-Samad, Int. J. Pharm., 2024, 124494 CrossRef CAS PubMed.
- E. W. Martin and T. Mittag, Biochemistry, 2018, 57, 2478–2487 CrossRef CAS PubMed.
- V. N. Uversky, Annu. Rev. Biophys., 2021, 50, 135–156 CrossRef CAS PubMed.
- Y. Shin, J. Berry, N. Pannucci, M. P. Haataja, J. E. Toettcher and C. P. Brangwynne, Cell, 2017, 168(159–171), e114 Search PubMed.
- S. Elbaum-Garfinkle, Y. Kim, K. Szczepaniak, C. C. Chen, C. R. Eckmann, S. Myong and C. P. Brangwynne, Proc. Natl. Acad. Sci. U. S. A., 2015, 112, 7189–7194 CrossRef CAS PubMed.
- C. P. Brangwynne, C. R. Eckmann, D. S. Courson, A. Rybarska, C. Hoege, J. Gharakhani, F. Julicher and A. A. Hyman, Science, 2009, 324, 1729–1732 CrossRef CAS PubMed.
- P. Li, S. Banjade, H. C. Cheng, S. Kim, B. Chen, L. Guo, M. Llaguno, J. V. Hollingsworth, D. S. King, S. F. Banani, P. S. Russo, Q. X. Jiang, B. T. Nixon and M. K. Rosen, Nature, 2012, 483, 336–340 CrossRef CAS PubMed.
- C. P. Brangwynne, P. Tompa and R. V. Pappu, Nat. Phys., 2015, 11, 899 Search PubMed.
- S. C. Weber, Curr. Opin. Cell Biol., 2017, 46, 62–71 CrossRef CAS PubMed.
- S. Chong and M. Mir, J. Mol. Biol., 2021, 433, 166724 CrossRef CAS PubMed.
- J. A. Riback, C. D. Katanski, J. L. Kear-Scott, E. V. Pilipenko, A. E. Rojek, T. R. Sosnick and D. A. Drummond, Cell, 2017, 168, 1028–1040 e1019 CrossRef CAS PubMed.
- A. Molliex, J. Temirov, J. Lee, M. Coughlin, A. P. Kanagaraj, H. J. Kim, T. Mittag and J. P. Taylor, Cell, 2015, 163, 123–133 CrossRef CAS PubMed.
|
This journal is © The Royal Society of Chemistry 2025 |
Click here to see how this site uses Cookies. View our privacy policy here.