DOI:
10.1039/C5QI00002E
(Chemistry Frontiers)
Inorg. Chem. Front., 2015,
2, 510-524
Functionalization of cyclometalated iridium(III) polypyridine complexes for the design of intracellular sensors, organelle-targeting imaging reagents, and metallodrugs
Received
5th January 2015
, Accepted 7th April 2015
First published on 8th April 2015
Abstract
In the past decade, there has been increasing interest in the applications of cyclometalated iridium(III) polypyridine complexes in different areas. Since many of these complexes exhibit interesting photophysical properties, they have been utilized in the development of luminescent chemosensors, light-emitting devices, and photovoltaic cells. In addition, a number of organometallic iridium(III) polypyridine complexes have been applied in a range of biological studies. In this article, we summarize the applications of a selection of these complexes with a focus on intracellular sensors, organelle-targeting imaging reagents, and metallodrugs.
Introduction
In the past decade, there has been increasing interest in the applications of cyclometalated iridium(III) polypyridine complexes in different areas. Since many of these complexes exhibit interesting photophysical properties such as photoluminescence, they have been utilized in the development of luminescent chemosensors, light-emitting devices, and photovoltaic cells. Similar to many other luminescent transition metal complexes, cyclometalated iridium(III) complexes exhibit favorable photophysical properties, including (1) enhanced photostability, which can avoid photobleaching upon continuous exposure to irradiation; (2) large Stokes’ shifts due to the phosphorescence nature of luminescence, which is very useful in minimizing self-quenching that is commonly observed in fluorescent organic dyes; (3) variable emission energies by altering the structures of ligands and modification of the ligands with substituents; and (4) relatively long emission lifetimes (in the submicrosecond or microsecond timescale), which can eliminate short-lived autofluorescence (in the nanosecond timescale) through time-gated and time-resolved techniques.1 Compared to other luminescent inorganic complexes such as rhenium(I), ruthenium(II), and osmium(II) polypyridines, the emission origins of cyclometalated iridium(III) polypyridine complexes are more diverse, which can range from triplet metal-to-ligand charge transfer (3MLCT), triplet intra-ligand (3IL), triplet ligand-to-ligand CT (3LLCT), and triplet sigma-bond-to-ligand CT (3SBLCT).1c,d This diversity not only enriches the photophysical and photochemical properties of these complexes, but also allows fine tuning of these properties using various ligand systems. In the past few years, there has been a tremendous growth of biological applications using luminescent iridium(III) polypyridine complexes; for example, as biomolecular probes and bioimaging reagents for live cells. The reasons are that many of these complexes, especially those that are cationic and lipophilic, show facile cellular uptake and interesting intracellular localization properties. Similar to other heavy metal-based bioreagents, the cellular uptake of these complexes by live cells can be readily quantitated using atomic absorption spectroscopy (AAS) and inductively-coupled plasma mass spectrometry (ICP-MS).1g All these advantages have facilitated cyclometalated iridium(III) polypyridine complexes to become a new generation of biological reagents with applications including intracellular sensing and organelle-selective staining. In addition, non-luminescent organometallic iridium(III) complexes have been found to be useful in other biological applications; for example, the design of anticancer agents since the iridium(III) metal center is kinetically inert, which allows the complexes to perform specific functions without modification and interference by other intracellular biomolecules such as glutathione, and the octahedral geometry of these complexes can fit in the active sites of many important enzymes.2 In this article, we summarize the applications of a selection of cyclometalated iridium(III) polypyridine complexes with a focus on intracellular sensors, organelle-targeting imaging reagents, and metallodrugs. Readers are referred to a number of excellent review articles for more exhaustive and detailed descriptions of other examples.1
Intracellular sensors
Although the detection of biologically relevant ions and small molecules has been very well developed, the design of luminescent probes that can perform intracellular sensing with high accuracy and reproducibility remains a great challenge. On the basis of their environment-sensitive emission properties, many luminescent cyclometalated iridium(III) polypyridine complexes have recently been developed as intracellular sensors for ions and small molecules.
Zinc is an essential trace metal element involved in many cellular processes such as the synthesis of deoxyribonucleic acid (DNA) and ribonucleic acid (RNA), vascular endothelial repair, and epidermal cell division. Depletion of the biological zinc level may lead to impaired collagen synthesis, weaker wound healing strength, and growth retardation.3 The di-2-picolylamine (DPA) moiety is a commonly used receptor that has been appended to many reporter groups to afford Zn2+ ion sensors. Lo and co-workers have attached a DPA unit to the 5-position of 1,10-phenanthroline (phen) and synthesized the complexes [Ir(N^C)2(phen-DPA)]+ (1).4 Since the amine group suppresses the emission of the complexes through photoinduced electron transfer (PET), these complexes show 1.2- to 5.4-fold emission enhancement upon binding of Zn2+ to the DPA unit. Titration experiments indicate that the dissociation constant (Kd) is of the order of 10−5 M. Confocal images of human cervical adenocarcinoma (HeLa) cells treated with complex 1a reveal that the incorporation of the DPA unit into the iridium(III) complexes does not hamper their cell membrane permeability. Unfortunately, the emission of the complexes inside the cells does not display noticeable changes in the presence of Zn2+. Lippard and co-workers have appended the DPA unit to the 4-position of phen to obtain the complex [Ir(dfppy)2(phen-DPA)]+ (2).5 Unexpectedly, the complex shows dual emission bands in CH3CN solution, which have been assigned to excited states associated with the dfppy (blue emission) and phen (yellow emission) ligands, respectively. Interestingly, a ratiometric phosphorescence response is observed upon coordination of Zn2+ to the DPA unit. In addition, an increase in emission lifetime is detected in complex-stained human lung adenocarcinoma epithelial (A549) cells on treatment with Zn2+ (Fig. 1). These findings indicate that the complex is a promising candidate as a zinc sensor for live cells. A related complex [Ir(ppy)2(btp-DPA)] (3) has also been designed as an intracellular ratiometric Cu2+ ion sensor.6
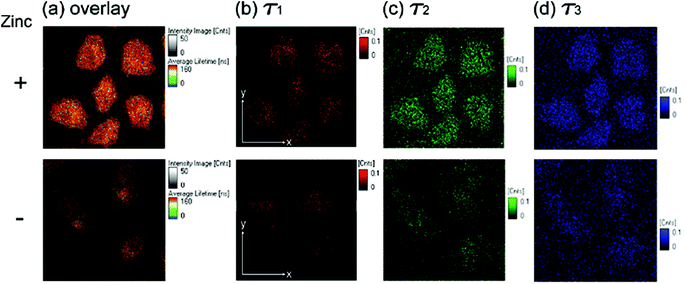 |
| Fig. 1 Fluorescence lifetime microscopy images (80 μm × 80 μm) of fixed A549 cells treated with complex 2 (5 μM, 30 min). Cells in the upper panels were incubated with ZnCl2/NaPT (10 μM, 15 min) prior to complex treatment. (a) Overlay of images (b–d); (b) long-lived component (τ1) image; (c) midrange component (τ2) image; (d) short-lived component (τ3) image. Taken with permission from ref. 5. | |
Thiol-containing amino acids, homocysteine (Hcy) and cysteine (Cys), play an important role in the physiological activities of living systems. The level of Hcy in plasma is related to cardiovascular disorders and Alzheimer's disease, while that of Cys is closely associated with liver metabolism.
7 Similar to many other luminescent transition metal complexes, the emission properties of cyclometalated iridium(
III) complexes depend heavily on the structures of the ligands. Thus, the modification of the coordinated ligands by an analyte usually leads to a change of the luminescence properties of the complexes, which can be utilized to develop new sensors. To illustrate this, a cyclometalated iridium(
III) polypyridine complex [Ir(pba)
2(bpy)]
+ (
4) bearing an aldehyde unit on the pba ligand has been reported by Li and co-workers.
8 This phosphorescent probe responds to Hcy and Cys, through the selective reaction of aldehyde moieties with the β,γ-aminothiols, resulting in a change of emission color from yellow to red, which is visible to the naked eye. The change of emission color has been interpreted as a switch of emissive-state nature from
3IL (π → π*)(pba) to
3MLCT (dπ(Ir) → π*(bpy))/
3LLCT (π(pba) → π*(bpy)). The complex shows negligible cytotoxicity and can monitor the changes of Hcy or Cys within living cells in a ratiometric manner (
Fig. 2).
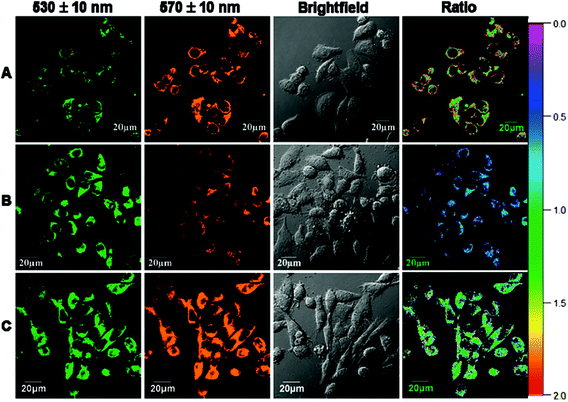 |
| Fig. 2 Ratio phosphorescence images of complex 4 in KB cells. (A) KB cells incubated with complex 4 (20 μM) for 30 min; (B) KB cells incubated with N-ethylmaleimide (200 μM) for 1 h and then further incubated with complex 4 (20 μM) for 30 min; (C) KB cells incubated with N-ethylmaleimide (200 μM) for 1 h and then further incubated with complex 4 (20 μM) for 1 h. Ratio of emission intensity at 570 ± 10 nm to 530 ± 10 nm is shown. Taken with permission from ref. 8. | |
Nitric oxide (NO) is an intracellular signaling molecule and a biological regulator, which is involved in different physiological and pathological processes.9 It also plays important roles in neurotransmission, muscle relaxation, blood pressure, and immune response regulation. There are two major strategies in the design of NO sensors; the first utilizes a paramagnetic copper(II) ion to quench the fluorescence of an organic dye.10 Upon reduction by NO, the copper(I) departs from the dye, resulting in fluorescence turn-on. In the second strategy, an electron-rich o-diaminoaromatic moiety is used to quench the emission of a fluorescent molecule through PET.11 This unit is converted to a triazole derivative by NO under aerobic conditions, leading to the restoration of emission intensity. Lo and co-workers used the second strategy to design iridium(III)-based NO sensors. Unlike other luminescent cyclometalated iridium(III) polypyridine complexes, the phenylenediamine complexes [Ir(N^C)2(bpy-diamine)]+ (5) display very weak emission upon photoexcitation.12 Treatment of complex 5b with an NO-releasing reagent 3-(2-hydroxy-1-methyl-2-nitroso-hydrazino)-N-methyl-1-propanamine (NOC-7) enhances the emission of the solution by 18-fold; the conversion of the diamine moiety to the triazole analogue has been confirmed by 1H NMR and MS. Importantly, the reaction is specific toward NO over a series of reactive oxygen and nitrogen species (RONS) and the emission intensity of complex 5b is pH-insensitive in the biologically relevant pH range (ca. 7.20–8.10), enabling it to function as an NO sensor. HeLa cells loaded with complex 5b exhibit ca. 2-fold enhanced intracellular emission intensity upon incubation with NOC-7 (Fig. 3). Interestingly, the complex can detect endogenous NO in the mouse leukemic monocyte macrophage (RAW264.7) upon treatment with lipopolysaccharides, as a result of the overexpression of inducible nitric oxide synthase (iNOS).13 These findings illustrate that these iridium(III) phenylenediamine complexes are versatile intracellular sensors for NO generated both exogenously and endogenously.
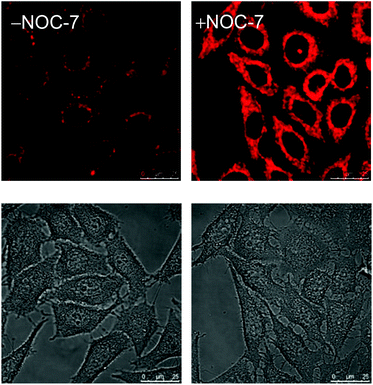 |
| Fig. 3 Luminescence (top) and bright-field (bottom) images of HeLa cells upon incubation with complex 5b (5 μM, 1 h) at 37 °C without (left) and with (right) post-treatment with NOC-7 (100 μM, 1 h). Taken with permission from ref. 12. | |
Organelle-targeting imaging reagents
Visualizing the structures of cellular compartments and tracing the morphological changes of organelles provide important information about the basic properties of cells and intracellular dynamic processes. Selective organelle-targeting probes are therefore valuable biological tools to cell and molecular biology. Although many organic dyes and lanthanide chelates have been developed as selective organelle stains, luminescent transition metal complexes have also emerged as a new generation of bioimaging reagents in recent years.
Endoplasmic reticulum (ER) is a flattened organelle responsible for the initial synthesis and folding of proteins. It protects cells from stress through an unfolded protein response,14 and is a storage for intracellular calcium ions, which is involved in cellular signaling and survival.15 Fei and co-workers have reported the cellular uptake and cytotoxicity of a series of cyclometalated iridium(III) polypyridine complexes [Ir(ppy)2(polypy)]+ (6).16 Interestingly, the diphenylphenanthroline complex [Ir(ppy)2(Ph2phen)]+ (6c) is preferentially localized in the membrane fraction (ca. 60%) of HeLa cells. Co-staining with ER-Tracker Red demonstrates nearly complete overlap between complex 6c and the marker (Fig. 4). Importantly, the selective accumulation of the complex causes ER stress in cells and calcium release into cytoplasm, which trigger mitochondrial dysfunction and apoptosis.
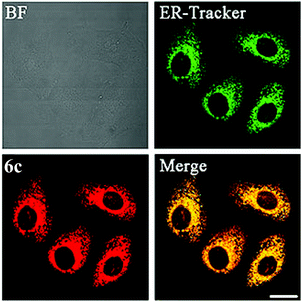 |
| Fig. 4 Confocal microscopy images of HeLa cells pre-stained with ER-Tracker Red (500 nM) for 30 min and then co-incubated with complex 6c (3 μM) for 20 min at 37 °C. Scale bar: 20 μm. Taken with permission from ref. 16. | |
The Golgi apparatus is a stack of membrane-bound vesicles involved in the processing, modifying, and packaging of macromolecules such as proteins and lipids. It refines the protein export from the ER through a multistage process.
17 Thus, it is strategically interposed between the ER and the final destination for protein-sorting processes. Compared to organic dyes such as fluorescent ceramides and sphingolipids, luminescent inorganic complexes for staining the Golgi apparatus of live cells are very rare. One of the examples is a series of dendritic cyclometalated iridium(
III) complexes [{Ir(N^C)
2}
8(bpy-8)]
8+ (
7) reported by Lo and co-workers.
18 Upon internalization by HeLa cells, these highly cationic-charged complexes are localized in the perinuclear region and bound to the Golgi apparatus, as confirmed by co-staining experiments involving antigolgin-97 (human) mouse IgG
1 and fluorescent Alexa 635 goat antimouse IgG (H+L) (
Fig. 5). The selective localization is probably related to the dendritic structure of the complexes. Another iridium-based Golgi stain is the neutral complex [Ir(ppy-NPh
2)
2(ppy-BAr
2)] (
8) designed by Wong and co-workers.
19 This complex displays a strong two-photon absorption cross section (340 GM) upon excitation at 750 nm and intense two-photon induced emission. The complex has negligible cytotoxicity toward HeLa and A549 cells and is localized at the Golgi apparatus after uptake. Notably, the complex shows high stability inside the cells and resides at the organelle for more than 6 hours with no subcellular migration to the cytoplasm and nucleus. The reasons for the selective staining are not fully known but the aryl rings may play a role in this interesting localization property.
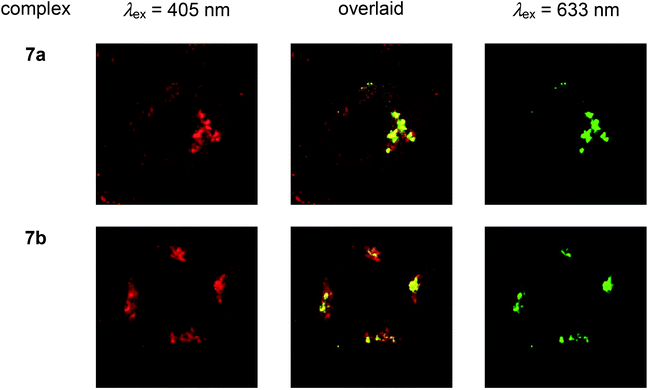 |
| Fig. 5 Laser-scanning confocal microscopy images of HeLa cells treated successively with complexes 7a and 7b ([Ir] = 2 μM) at 37 °C for 2 h, PBS containing 3% paraformaldehyde, antigolgin-97 (human) mouse IgG1 (1 μg mL−1, 1 h), and Alexa 635 goat antimouse IgG (H+L) (10 μg mL−1, 30 min). Taken with permission from ref. 18. | |
Mitochondria are involved in cellular energy production and other cellular activities such as maintenance of redox and calcium balance and controlled cell death.20 They can induce mitophagy (a selective degradation of mitochondria by autophagy) as a result of the production of ROS. Dysfunction in the mitophagy pathway has been discovered to be a probable cause of Parkinson's disease and Alzheimer's disease.21 Many luminescent transition metal complexes are found to be localized at the mitochondria after cellular uptake, which is most likely a result of the cationic charge and lipophilicity of the complexes; for example, the iridium(III) complexes [Ir(N^C)2(phen-Se)]+ (9) have been developed by Chao and co-workers as multicolor probes for mitochondrial imaging.22 The results of the ICP-MS measurements show that the ratio of the iridium content between the mitochondrial and whole cell uptake is >80%. It is noteworthy that these complexes are superiorly photostable and highly resistant to loss of mitochondrial membrane potential and changes in the intracellular environment. In addition, they can be applied to track the mitochondrial morphological changes of cells. In another study, Lo and co-workers have appended a β-D-glucose moiety to a series of luminescent cyclometalated iridium(III) polypyridine complexes to afford [Ir(N^C)2(bpy-glc)]+ (10).23 Various inhibition and competitive uptake experiments conclude that the cellular uptake of the complexes is mediated by glucose transporters (GLUTs), which are overexpressed on the membrane of cancer cells. Thus, the complexes have the capability of differentiating cancerous and normal cells. In addition, co-staining experiments involving MitoTracker Deep Red FM indicate mitochondrial staining of complex 10a (Fig. 6).
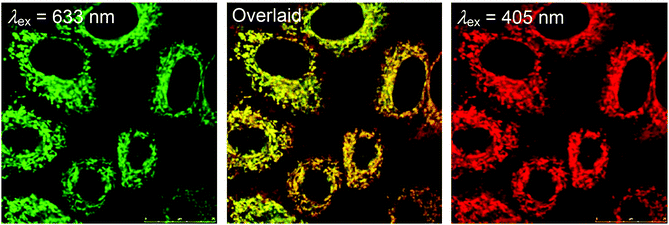 |
| Fig. 6 Laser-scanning confocal microscopy images of HeLa cells upon incubation successively with MitoTracker Deep Red FM (left) (100 nM, 20 min) and complex 10a (right) (50 μM, 5 min) (Pearson's coefficient: 86%) in a glucose-free medium at 37 °C. Taken with permission from ref. 23. | |
The nucleolus is the origin of the main ribosomal RNA (rRNA) and is the largest structure inside the nucleus that assembles ribosomal subunits. Also, it assembles signal recognition particles, modifies the RNAs, participates in messenger RNA (mRNA) splicing and human immunodeficiency virus 1 (HIV-1) replication, and senses cellular stress.24 Staining of nucleoli has been demonstrated by Lo and co-workers using luminescent cyclometalated iridium(III) dipyridoquinoxaline (dpq) complexes [Ir(N^C)2(dpq)]+ (11).25 UV-Vis and emission titrations show that all the complexes bind to double-stranded calf-thymus DNA by intercalation. Madin–Darby canine kidney (MDCK) cells incubated with complex 11a reveal substantial cytoplasmic staining. Upon further incubation, staining of nuclear structures reminiscent of the nucleoli is observed. Cells fixed with MeOH or treated with ribonuclease A show that the complex still stains the nucleoli, which means that the localization is not due to active sequestration by the cells and does not originate from the binding of the complexes to RNA (Fig. 7). Sodium dodecyl sulfate-polyacrylamide gel electrophoresis (SDS-PAGE) experiments involving proteins from various tissues of Medaka, subcellular fractions of human umbilical vein endothelial cells (HUVEC), and total proteins from MDCK cells reveal that the complex stains a wide range of proteins, which conclude that the observed nucleolar staining in live cells results from non-specific binding of the complex to the hydrophobic pockets of proteins in the cells (Fig. 8).
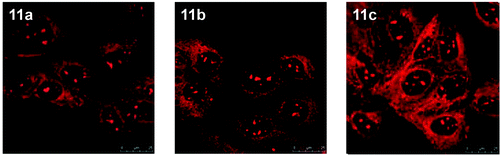 |
| Fig. 7 Fluorescence laser-scanning confocal microscopy images of fixed MDCK cells treated successively with ribonuclease A (50 μg mL−1, 1 h, 37 °C) and complexes 11a (left), 11b (middle), and 11c (right) ([Ir] = 5 μM, 30 min). Taken with permission from ref. 25. | |
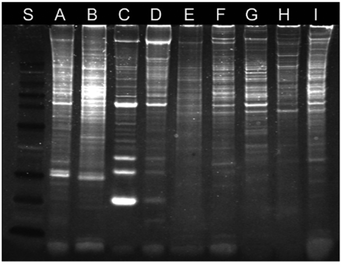 |
| Fig. 8 SDS-PAGE analysis of proteins upon staining with complex 11a under ultraviolet transillumination. Lanes A–D correspond to the proteins from the gill, liver, muscle, and skin of Medaka, respectively. Lane E corresponds to total proteins of HUVEC. Lanes F–I correspond to proteins from the cytoplasm, nucleus, nucleoplasm, and nucleolus of HUVEC, respectively. Lane S corresponds to protein markers. Taken with permission from ref. 25. | |
Lysosomes are primary degradative compartments of the cell that control cell death. They are active in physiological processes and cell signaling pathways such as apoptosis induced by catabolic hydrolases under endogenous or exogenous stress, and autophagy caused by lysosomal hydrolases under physiological conditions.
26 Real-time tracking of lysosomes gives useful insights into cellular events during autophagy, which is a lysosomal degradation pathway important for cellular development and treatment of human diseases.
27 Lysosome dyes derived from the iridium(
III) complexes [Ir(ppy)
2(N^N-carboline)]
+ (
12) have been designed by Mao and co-workers.
28 Large two-photon absorption cross-sections (958 and 972 GM at pH 3.4 for complexes
12a and
12b, respectively) are determined. Interestingly, live A549 cells treated with LysoTracker Green DND-26 (LTG) and complex
12a or
12b display specific lysosomal staining (
Fig. 9). Under two-photon excitation, the Pearson's co-localization coefficients of complexes
12a and
12b with LTG are determined to be 0.85 and 0.91, respectively. Their specific lysosome-targeting properties have been confirmed using human breast adenocarcinoma (MCF-7) cells and HeLa cells as models.
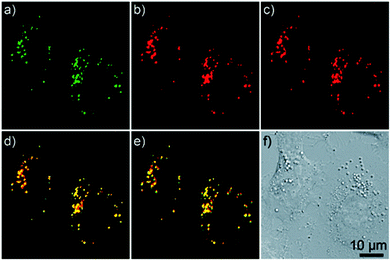 |
| Fig. 9 Confocal microscopy images of A549 cells co-incubated with LTG (150 nm, 0.5 h) and complex 12b (10 μM, 1 h). (a) LTG; (b) one-photon excited complex 12b (10 μM, 1 h); (c) two-photon excited complex 12b; (d) overlay of panels (a) and (b); (e) overlay of panels (a) and (c); (f) brightfield. Taken with permission from ref. 28. | |
The nucleus stores chromosomes that control metabolism, heredity and reproduction
via replication and recombination of DNA, and transcription and processing of RNA.
29 It separates DNA from cytoplasmic processes, while the nuclear pores provide a unique biochemical environment within the nucleus through selective transport.
30 Due to the important roles of the nucleus, studies of its morphology and tracking its activities are required. Although many organic-based nuclear stains are commercially available, inorganic dyes that target this organelle with high selectivity are very rare. Li and co-workers have designed a non-emissive cyclometalated iridium(
III) complex [Ir(ppy)
2(DMSO)
2]
+ (
13), which is the first reaction-based emission turn-on reagent for the nuclei of living cells.
31 This probe undergoes rapid reactions with histidine and histidine-containing proteins to afford strongly emissive adducts. Upon incubation of live HeLa, mouth epidermal carcinoma (KB), fibroblast-like synoviocytes (FLS), and mesenchymal stem cells (MSC) with the complex for as short as 10 minutes, the nuclear regions of the four cell lines display intense emission. The structure–localization relationship has been studied to optimize efficiency of cellular uptake and nuclear accumulation. Modification of the cyclometalating and solvent ligands and the counter anions leads to a class of non-emissive complexes [Ir(ppy-R)
2(solv)
2]
+L
− (R = Me, Et,
i-Pr,
t-Bu; solv = DMSO, H
2O, CH
3CN; L
− = PF
6−, OTf
−) (
14).
32 The results show that variation in the solvent ligands and/or counter anions does not affect the localization behavior. However, changing the length of the side-chain of the cyclometalating ligands has significant effects on the cellular uptake and localization of the complexes in cells; for example, increasing the length of the side carbon chain and branched chains of the cyclometalating ligand translocates the complexes from the nucleus to the cytoplasm (
Fig. 10).
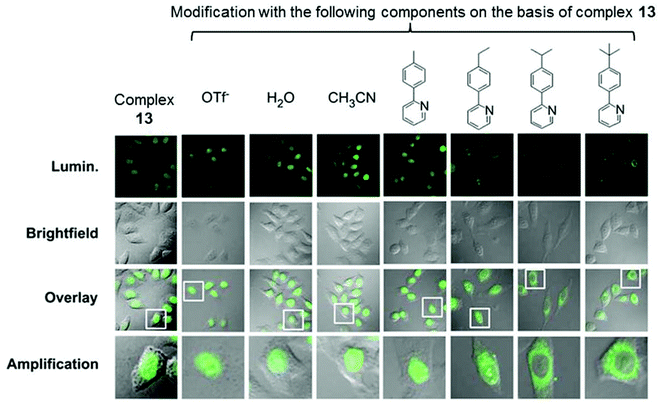 |
| Fig. 10 Confocal luminescence, brightfield and their overlay images of living HeLa cells incubated with complexes 13 and 14 (10 μM) in DMSO/PBS (pH 7.4, 1 : 99, v/v) for 10 min at 37 °C. Taken with permission from ref. 32. | |
Metallodrugs
There has been much interest in the correlation of the structural features of organometallic iridium(III) complexes with their mechanisms of cytotoxic activity, which is expected to lead to the development of potent metallodrugs. Sadler and co-workers have reported a series of iridium(III) half-sandwich pentamethylated-cyclopentadienyl (Cp*) complexes [Ir(Cpx)(L^L)(Z)]n+ (Cpx = Cp*, extended Cp*; L^L = N^C, N^N; Z = Cl, py) (15), which possess high cytotoxic potency, down to the nanomolar concentration, toward a range of cancer cells.33 The mechanism of action of these complexes may involve an attack on DNA and/or a perturbation of the redox status of cells owing to hydrolytic properties. The aqua complexes (formed by substitution of Cl− of the chloro complexes with H2O) react more readily with nucleobases.34 The extended arene in the functionalized Cp* ligand intercalates into DNA base pairs and it also facilitates the cellular uptake of the complex due to increased lipophilicity. In addition, the electron-donating methyl groups on the Cp* ring increases the negative charge on the metal, which favors the substitution of Cl− by H2O, resulting in fast hydrolysis (Fig. 11). The complex [Ir(biphenyl-Cp*)(ppy)(py)]+ (15g), chelated with a negatively-charged N^C ligand and the extended Cp* ligands, shows high cytotoxic activity (IC50 = 120 nM) toward human ovarian carcinoma (A2780) cells.35 The complex passages across cell membranes with the assistance of the N^C and extended Cp* ligands, leading to enhanced cellular uptake efficiency and intercalation into DNA, respectively. The pyridine ligand helps the complex to reach intracellular target sites through slowing down the hydrolysis in order to prevent the reaction with abundant intracellular glutathione. This complex has been screened by the NCI panel of 60 cancer cell lines and shown to have comparable or even higher activity than the clinical drugs oxaliplatin and cisplatin. In addition, complex 15g is found to generate higher levels of ROS in cancer cells than normal cells. Detailed mechanistic studies show that the complex can generate H2O2 by catalytic hydride transfer from the coenzyme NADH to oxygen. These exciting results are highly valuable in the design of a new generation of anticancer drugs for effective oxidant therapy.
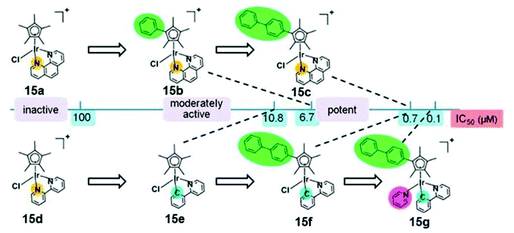 |
| Fig. 11 Comparison of cytotoxicity of complexes 15a–g against A2780 cancer cells. Taken with permission from ref. 33. | |
The coordinative bonds of the iridium(
III) complexes tend to be inert,
36 and their octahedral coordination geometries can fill protein pockets such as active sites of enzymes.
2 Meggers and co-workers have reported iridium(
III) complexes as potent kinase inhibitors; for example, they have designed and synthesized iridium(
III) complexes containing a pyridocarbazole (py-carbazole) pharmacophore bidentate ligand [Ir(cod)(py-carbazole)(X)(Y)] (
16) that target the ATP-binding site of protein kinases.
37 Upon incubation of complex
16c and ATP, only 12 out of 263 kinases, including fms-related tyrosine kinase 4 (Flt4), show activities below 25%. Flt4 is important for the development and maintenance of lymphatic vessels and embryonic cardiovascular systems.
38 Expectedly, ligands X and Y of the complexes have a profound effect on the inhibition properties. Complexes with smaller ligands X and Y demonstrate higher kinase inhibition; for example, the IC
50 value of complex
16b (X = Y = Cl) is determined to be 123 ± 14 μM, which is the most effective inhibitor among its structural analogues. The findings are consistent with previous studies of organometallic protein kinase inhibitors, indicative of the importance of the ligand pointing toward a glycine-rich loop for kinase inhibition.
39 Also, the inhibition properties of complex
16b have been investigated using transgenic zebrafish (
Danio rerio) embryos as an animal model. As revealed by confocal fluorescence microscopy, this complex strongly inhibits vessel formation with 100% of the zebrafish embryos at a low complex concentration (5 μM) (
Fig. 12). Although the antiangiogenicity of complex
16d is independent of light, a
ca. 34-fold increase in its cytotoxicity toward HeLa cells is observed upon exposure to visible light.
40
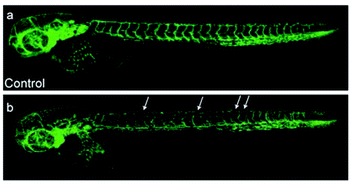 |
| Fig. 12 Laser scanning confocal microscopy images of 3 days post-fertilization of zebrafish embryos treated with (a) DMSO or (b) complex 16b (5 μM). Vessel defects are marked with white arrows. Taken with permission from ref. 37. | |
Photodynamic therapy (PDT) requires administration of a non-cytotoxic photosensitizer and irradiation of a specific wavelength. During the treatment, the photosensitizer generates highly reactive singlet oxygen (
1O
2) upon illumination, which incurs damage to target tissues.
41 Since light is directional, PDT can provide the spatiotemporally-controlled treatment with high precision. Similar to other phosphorescent inorganic complexes, iridium(
III) complexes can be efficient photosensitizers with an appropriate choice of ligands.
42 To design effective photodynamic therapeutics, the reagents should be of minimal toxicity in the dark. Lo and co-workers have designed a class of highly bio-compatible and water-soluble iridium(
III) poly(ethylene glycol) (PEG) complexes [Ir(N^C)
2(bpy-PEG)]
+ (
17).
43 The emission of the complexes is readily quenched by O
2 with the generation of
1O
2. Cell-based assays indicate that the PEG complexes are non-cytotoxic toward HeLa cells in the dark (IC
50 > 300 μM). However, they become significantly cytotoxic (IC
50 = 3.4–23.2 μM) upon photoexcitation (
Fig. 13). Confocal microscopy images reveal that complex
17a is localized in the mitochondrial region of HeLa cells. Thus, photoexcitation of cells stained by this complex leads to oxidative damage of this organelle and subsequent necrotic cell death.
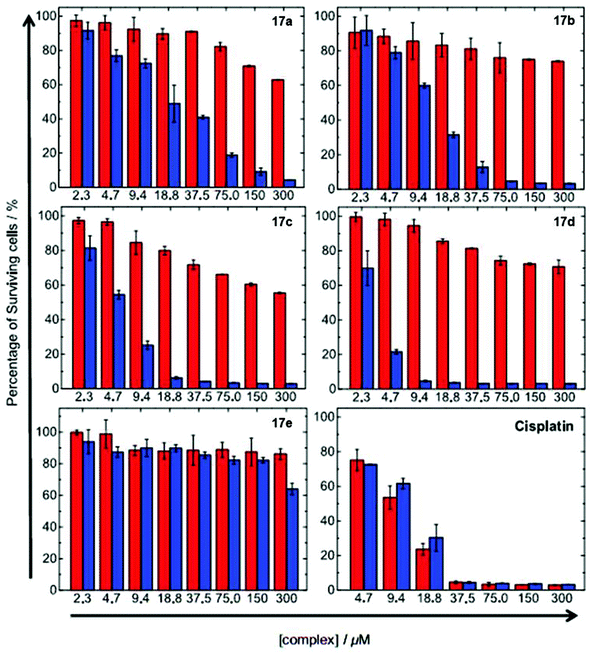 |
| Fig. 13 Cytotoxicity of complexes 17a–e and cisplatin toward HeLa cells upon incubation in the dark for 12 h. The cells were further incubated in the dark (red) or irradiated at 365 nm (blue) for 30 min. Taken with permission from ref. 43. | |
Aoki and co-workers have synthesized and characterized a class of pH-sensitive neutral tris-cyclometalated iridium(
III) complexes that contain free pyridine rings [Ir(Py-ppy)
3] (
18).
44 These complexes exhibit strong green emission in DMSO upon excitation. The emission bands of complexes
18a and
18c display a significant red-shift upon protonation of the free pyridine rings. As a result, they show green emission under neutral and basic conditions, and weak red emission at acidic pH. The generation of
1O
2 by complexes
18a and
18b upon irradiation is evidenced by the decomposition of 1,3-diphenylisobenzofuran, the oxidation of thioanisole, and the oxidation of 2,2,5,5-tetramethyl-3-pyrroline-3-carboxamide. Incubation of HeLa-S3 cells with complex
18a does not significantly reduce the cell viability (survival of cells >95%), as revealed by propidium iodide staining. However, irradiation of the stained cells at 465 nm for 10 and 20 minutes results in 60 and 75% cell death, respectively (
Fig. 14). Morphological examinations of the stained cells upon irradiation suggest that the cell death is necrosis in nature.
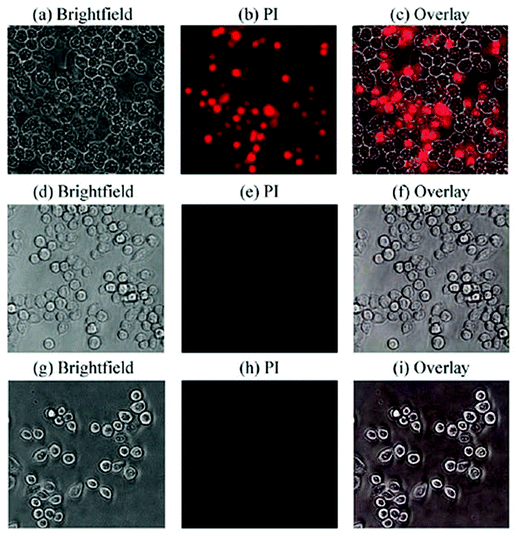 |
| Fig. 14 Fluorescence microscopy images of HeLa-S3 cells (×20) (a)–(c) irradiated at 465 nm for 10 min after pre-incubation for 30 min with complex 18a (10 μM); (d)–(f) irradiated for 10 min after pre-incubation for 30 min with DMSO only; (g)–(i) incubated for 30 min with complex 18a (10 μM) in the dark, then washed and stained with PI (30 μM) for 30 min. Taken with permission from ref. 44. | |
To improve tissue penetration and reduce bio-autofluorescence, near-infrared (NIR) fluorescent probes have been developed.
45 Zhao and co-workers have modified cyclometalated iridium(
III) complexes with a BODIPY pendant through a π-conjugated linker [Ir(ppy)
2(bpy-BODIPY)]
+ (
19).
46 These complexes show strong NIR (644–729 nm) absorption, NIR emission (700–800 nm), and long-lived triplet excited states (92.5–156.5 μs). The
1O
2-photosensitizing ability of the complexes has been investigated with visible light photooxidation using 1,5-dihydroxynaphthalene (DHN) as the
1O
2 scavenger. Trypan blue staining images show that the majority of lung cancer (LLC) cells are dead upon incubation with complex
19b under illumination with a 635 nm LED for 4 hours (
Fig. 15). In addition, the MTT assays reveal a
ca. 2-fold increase in cytotoxicity of complex
19b toward 1121 and LLC cell lines upon photodynamic treatment.
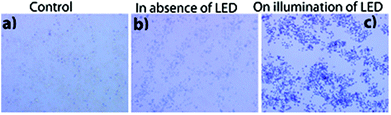 |
| Fig. 15 Trypan blue staining images of LLC cells treated (a) in the dark and complex 19b; (b) in the dark but in the presence of complex 19b (10 μM); (c) upon illumination at 635 nm and complex 19b (10 μM). Taken with permission from ref. 46. | |
Conclusion
In this article, we have introduced the applications of a selection of iridium(III) complexes as intracellular sensors, organelle-targeting imaging reagents, and metallodrugs. Although the photophysical properties of cyclometalated iridium(III) polypyridine complexes [Ir(N^C)2(N^N)]+ have been known for many years, it was only in the last decade that reports on the applications of these complexes as biological probes appeared. The cationic and lipophilic nature of these complexes has promoted the cellular uptake, and the applications in cellular imaging and intracellular sensors have attracted much attention recently. Regarding the formal charge of the complexes, a higher cationic charge is certainly an advantage over neutral complexes in terms of higher water solubility. However, a too high cationic charge will significantly limit the cellular uptake efficiency. Thus, one must strike a balance between water solubility and cellular uptake efficiency when designing new probes and drugs using iridium(III) complexes and transition metal complexes in general. Despite the many attractive advantages of iridium(III) complexes, there are limitations in their applications such as the high cost of the metal. Also, the potential toxicity of these metal complexes, for example due to organelle disruption after uptake and the long-term effects, must not be overlooked if new iridium(III) complexes are to be further developed as bioprobes and biomedicine. We believe that with a judicious choice of ligands, functional pendants, spacer-arms of different properties, the intracellular localization properties of these complexes can be adjusted readily. In addition, the hydrolytic properties and catalytic hydride transfer ability of organoiridium complexes [Ir(Cpx)(L^L)(Z)], and the rigid coordination geometry and photosensitizing properties of other polypyridine complexes have inspired the design and development of interesting therapeutic reagents. On the basis of the recent studies summarized in this article and other excellent reviews, we believe that the structural diversity and the favorable photophysical and photochemical properties of organometallic iridium(III) polypyridine complexes will continue to contribute to the development of new theranostic reagents with high application potential.
Acknowledgements
We thank City University of Hong Kong (Project no. 7004210 and 9667090) for financial support. KKWL thanks the Croucher Foundation for a Croucher Senior Research Fellowship. KKST acknowledges the receipt of a Postgraduate Studentship administered by City University of Hong Kong.
References
-
(a) K. K.-W. Lo, M.-W. Louie and K. Y. Zhang, Coord. Chem. Rev., 2010, 254, 2603 CrossRef CAS PubMed;
(b) V. Fernandez-Moreira, F. L. Thorp-Greenwood and M. P. Coogan, Chem. Commun., 2010, 46, 186 RSC;
(c) K. K.-W. Lo, K. Y. Zhang and S. P.-Y. Li, Pure Appl. Chem., 2011, 83, 823 CrossRef CAS;
(d) K. K.-W. Lo, S. P.-Y. Li and K. Y. Zhang, New J. Chem., 2011, 35, 265 RSC;
(e) Q. Zhao, C. Huang and F. Li, Chem. Soc. Rev., 2011, 40, 2508 RSC;
(f) K. K.-W. Lo, A. W.-T. Choi and W. H.-T. Law, Dalton Trans., 2012, 41, 6021 RSC;
(g) K. K.-W. Lo and K. Y. Zhang, RSC Adv., 2012, 2, 12069 RSC;
(h) Y. Geldmacher, M. Oleszak and W. S. Sheldrick, Inorg. Chim. Acta, 2012, 393, 84 CrossRef CAS PubMed;
(i) C.-H. Leung, H.-J. Zhong, D. S.-H. Chan and D.-L. Ma, Coord. Chem. Rev., 2013, 257, 1764 CrossRef CAS PubMed;
(j) K. K.-W. Lo and S. P.-Y. Li, RSC Adv., 2014, 4, 10560 RSC.
- J. Maksimoska, L. Feng, K. Harms, C. Yi, J. Kissil, R. Marmorstein and E. Meggers, J. Am. Chem. Soc., 2008, 130, 15764 CrossRef CAS PubMed.
-
A. S. Prasad, Zinc Deficiency: Its Characterization and Treatment, in Metal Ions in Biological Systems, 41, Metal Ions and Their Complexes in Medication, ed. A. Sigel and H. Sigel, Marcel Dekker, New York, 2004, pp. 103–134 Search PubMed.
- P.-K. Lee, W. H.-T. Law, H.-W. Liu and K. K.-W. Lo, Inorg. Chem., 2011, 50, 8570 CrossRef CAS PubMed.
- Y. You, S. Lee, T. Kim, K. Ohkubo, W.-S. Chae, S. Fukuzumi, G.-J. Jhon, W. Nam and S. J. Lippard, J. Am. Chem. Soc., 2011, 133, 18328 CrossRef CAS PubMed.
- Y. You, Y. Han, Y.-M. Lee, S. Y. Park, W. Nam and S. J. Lippard, J. Am. Chem. Soc., 2011, 133, 11488 CrossRef CAS PubMed.
-
(a) S. Shahrokhian, Anal. Chem., 2001, 73, 5972 CrossRef CAS;
(b) S. Seshadri, A. Beiser, J. Selhub, P. F. Jacques, I. H. Rosenberg, R. B. D'Agostino, P. W. F. Wilson and P. A. Wolf, N. Engl. J. Med., 2002, 346, 476 CrossRef CAS PubMed.
- L. Xiong, Q. Zhao, H. Chen, Y. Wu, Z. Dong, Z. Zhou and F. Li, Inorg. Chem., 2010, 49, 6402 CrossRef CAS PubMed.
-
(a) S. Moncada, R. M. J. Palmer and E. A. Higgs, Pharmacol. Rev., 1991, 43, 109 CAS;
(b) E. M. Conner and M. B. Grisham, Methods, 1995, 7, 3 CrossRef CAS;
(c) F. Murad, Angew. Chem., Int. Ed., 1999, 38, 1856 CrossRef CAS.
- M. H. Lim, D. Xu and S. J. Lippard, Nat. Chem. Biol., 2006, 2, 375 CrossRef CAS PubMed.
-
(a) H. Kojima, Y. Urano, K. Kikuchi, T. Higuchi, Y. Hirata and T. Nagano, Angew. Chem., Int. Ed., 1999, 38, 3209 CrossRef CAS;
(b) H. Kojima, M. Hirotani, N. Nakatsubo, K. Kikuchi, Y. Urano, T. Higuchi, Y. Hirata and T. Nagano, Anal. Chem., 2001, 73, 1967 CrossRef CAS;
(c) Y. Gabe, Y. Urano, K. Kikuchi, H. Kojima and T. Nagano, J. Am. Chem. Soc., 2004, 126, 3357 CrossRef CAS PubMed;
(d) E. Sasaki, H. Kojima, H. Nishimatsu, Y. Urano, K. Kikuchi, Y. Hirata and T. Nagano, J. Am. Chem. Soc., 2005, 127, 3684 CrossRef CAS PubMed.
- W. H.-T. Law, K.-K. Leung, L. C.-C. Lee, C.-S. Poon, H.-W. Liu and K. K.-W. Lo, ChemMedChem, 2014, 9, 1316 CrossRef CAS PubMed.
- C. Bogdan, Nat. Immunol., 2001, 2, 907 CrossRef CAS PubMed.
- D. Ron and P. Walter, Nat. Rev. Mol. Cell Biol., 2007, 8, 519 CrossRef CAS PubMed.
- S. Orrenius, B. Zhivotovsky and P. Nicotera, Nat. Rev. Mol. Cell Biol., 2003, 4, 552 CrossRef CAS PubMed.
- R. Cao, J. Jia, X. Ma, M. Zhou and H. Fei, J. Med. Chem., 2013, 56, 3636 CrossRef CAS PubMed.
- J. E. Rothman, Science, 1981, 213, 1212 CAS.
- K. Y. Zhang, H.-W. Liu, T. T.-H. Fong, X.-G. Chen and K. K.-W. Lo, Inorg. Chem., 2010, 49, 5432 CrossRef CAS PubMed.
- C.-L. Ho, K.-L. Wong, H.-K. Kong, Y.-M. Ho, C. T.-L. Chan, W.-M. Kwok, K. S.-Y. Leung, H.-L. Tam, M. H.-W. Lam, X.-F. Ren, A.-M. Ren, J.-K. Feng and W.-Y. Wong, Chem. Commun., 2012, 48, 2525 RSC.
-
(a) K. Henze and W. Martin, Nature, 2002, 426, 127 CrossRef PubMed;
(b) H. M. McBride, M. Neuspiel and S. Wasiak, Curr. Biol., 2006, 16, R551 CrossRef CAS PubMed;
(c) D. R. Green, Cell, 1998, 94, 695 CrossRef CAS.
- R. X. Santos, S. C. Correia, X. Wang, G. Perry, M. A. Smith, P. I. Moreira and X. Zhu, J. Alzheimers Dis., 2010, 20, 401 Search PubMed.
- Y. Chen, L. Qiao, L. Ji and H. Chao, Biomaterials, 2014, 35, 2 CrossRef CAS PubMed.
- W. H.-T. Law, L. C.-C. Lee, M.-W. Louie, H.-W. Liu, T. W.-H. Ang and K. K.-W. Lo, Inorg. Chem., 2013, 52, 13029 CrossRef CAS PubMed.
-
M. O. J. Olson, Nucleolus: Structure and Function, in Encyclopedia of Life Sciences (eLS), John Wiley & Sons, Ltd., Chichester, 2010, pp. 1–8 Search PubMed.
- K. Y. Zhang, S. P.-Y. Li, N. Zhu, I. W.-S. Or, M. S.-H. Cheung, Y.-W. Lam and K. K.-W. Lo, Inorg. Chem., 2010, 49, 2530 CrossRef CAS PubMed.
-
(a) P. Saftig and J. Klumperman, Nat. Rev. Mol. Cell Biol., 2009, 10, 623 CrossRef CAS PubMed;
(b) G. Kroemer and M. Jaattela, Nat. Rev. Cancer, 2005, 5, 886 CrossRef CAS PubMed;
(c) M. E. Guicciardi, M. Leist and G. J. Gores, Oncogene, 2004, 23, 2881 CrossRef CAS PubMed.
-
(a) N. Mizushima and B. Levine, Nat. Cell Biol., 2010, 12, 823 CrossRef CAS PubMed;
(b) E. White, Nat. Rev. Cancer, 2012, 12, 401 CrossRef CAS PubMed.
- L. He, C.-P. Tan, R.-R. Ye, Y.-Z. Zhao, Y.-H. Liu, Q. Zhao, L.-N. Ji and Z.-W. Mao, Angew. Chem., Int. Ed., 2014, 53, 1 CrossRef PubMed.
-
D. K. Rao and J. J. Kaur, Living Science Biology 9, Ratna Sagar P. Ltd, Delhi, 2007, pp. 7–30 Search PubMed.
- J. W. Newport and D. J. Forbes, Annu. Rev. Biochem., 1987, 56, 535 CrossRef CAS PubMed.
- C. Li, M. Yu, Y. Sun, Y. Wu, C. Huang and F. Li, J. Am. Chem. Soc., 2011, 133, 11231 CrossRef CAS PubMed.
- C. Li, Y. Liu, Y. Wu, Y. Sun and F. Li, Biomaterials, 2013, 34, 1223 CrossRef CAS PubMed.
- Z. Liu and P. J. Sadler, Acc. Chem. Res., 2014, 47, 1174 CrossRef CAS PubMed.
- Z. Liu, A. Habtemariam, A. M. Pizarro, S. A. Fletcher, A. Kisova, O. Vrana, L. Salassa, P. C. A. Bruijnincx, G. J. Clarkson, V. Brabec and P. J. Sadler, J. Med. Chem., 2011, 54, 3011 CrossRef CAS PubMed.
- Z. Liu, I. Romero-Canelón, B. Qamar, J. M. Hearn, A. Habtemariam, N. P. E. Barry, A. M. Pizarro, G. J. Clarkson and P. J. Sadler, Angew. Chem., Int. Ed., 2014, 53, 3941 CrossRef CAS PubMed.
- J. Burgess, Inorg. React. Mech., 1972, 2, 140 Search PubMed.
- A. Wilbuer, D. H. Vlecken, D. J. Schmitz, K. Kräling, K. Harms, C. P. Bagowski and E. Meggers, Angew. Chem., Int. Ed., 2010, 49, 3839 CrossRef CAS PubMed.
- M. J. Karkkainen and T. V. Petrova, Oncogene, 2000, 19, 5598 CrossRef CAS PubMed.
- H. Bregman, P. J. Carroll and E. Meggers, J. Am. Chem. Soc., 2006, 128, 877 CrossRef CAS PubMed.
- A. Kastl, A. Wilbuer, A. L. Merkel, L. Feng, P. D. Fazio, M. Ockerb and E. Meggers, Chem. Commun., 2012, 48, 1863 RSC.
- T. J. Dougherty, C. J. Gomer, B. W. Henderson, G. Jori, D. Kessel, M. Korbelik, J. Moan and Q. Peng, J. Natl. Cancer Inst., 1998, 90, 889 CrossRef CAS PubMed.
-
(a) R. Gao, D. G. Ho, B. Hernandez, M. Selke, D. Murphy, P. I. Djurovich and M. E. Thompson, J. Am. Chem. Soc., 2002, 124, 14828 CrossRef CAS PubMed;
(b) P. I. Djurovich, D. Murphy, M.E. Thompson, B. Hernandez, R. Gao, P. L. Hunt and M. Selke, Dalton Trans., 2007, 34, 3763 RSC;
(c) S. Takizawa, R. Aboshi and S. Murata, Photochem. Photobiol. Sci., 2011, 10, 895 RSC.
- S. P.-Y. Li, C. T.-S. Lau, M.-W. Louie, Y.-W. Lam, S. H. Cheng and K. K.-W. Lo, Biomaterials, 2013, 34, 7519 CrossRef CAS PubMed.
- A. Nakagawa, Y. Hisamatsu, S. Moromizato, M. Kohno and S. Aoki, Inorg. Chem., 2014, 53, 409 CrossRef CAS PubMed.
-
(a) M. Nurunnabi, Z. Khatun, G. R. Reeck, D. Y. Lee and Y.-K. Lee, Chem. Commun., 2013, 49, 5079 RSC;
(b) T. Myochin, K. Kiyose, K. Hanaoka, H. Kojima, T. Terai and T. Nagano, J. Am. Chem. Soc., 2011, 133, 3401 CrossRef CAS PubMed;
(c) F. Yu, P. Li, G. Li, G. Zhao, T. Chu and K. Han, J. Am. Chem. Soc., 2011, 133, 11030 CrossRef CAS PubMed.
- P. Majumdar, X. Yuan, S. Li, B. L. Guennic, J. Ma, C. Zhang, D. Jacqueminde and J. Zhao, J. Mater. Chem. B, 2014, 2, 2838 RSC.
|
This journal is © the Partner Organisations 2015 |
Click here to see how this site uses Cookies. View our privacy policy here.