DOI:
10.1039/C6CS00746E
(Review Article)
Chem. Soc. Rev., 2018,
47, 357-403
Calcium-based biomaterials for diagnosis, treatment, and theranostics
Received
16th October 2016
First published on 20th December 2017
Abstract
Calcium-based (CaXs) biomaterials including calcium phosphates, calcium carbonates, calcium silicate and calcium fluoride have been widely utilized in the biomedical field owing to their excellent biocompatibility and biodegradability. In recent years, CaXs biomaterials have been strategically integrated with imaging contrast agents and therapeutic agents for various molecular imaging modalities including fluorescence imaging, magnetic resonance imaging, ultrasound imaging or multimodal imaging, as well as for various therapeutic approaches including chemotherapy, gene therapy, hyperthermia therapy, photodynamic therapy, radiation therapy, or combination therapy, even imaging-guided therapy. Compared with other inorganic biomaterials such as silica-, carbon-, and gold-based biomaterials, CaXs biomaterials can dissolve into nontoxic ions and participate in the normal metabolism of organisms. Thus, they offer safer clinical solutions for disease theranostics. This review focuses on the state-of-the-art progress in CaXs biomaterials, which covers from their categories, characteristics and preparation methods to their bioapplications including diagnosis, treatment, and theranostics. Moreover, the current trends and key problems as well as the future prospects and challenges of CaXs biomaterials are also discussed at the end.
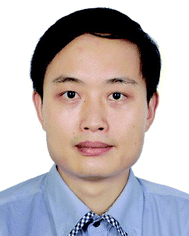
Chao Qi
| Chao Qi received his PhD in Materials Physics and Chemistry from Shanghai Institute of Ceramics, Chinese Academy of Sciences in 2016. Then he joined the Laboratory of Evolutionary Theranostics (LET) at Shenzhen University (SZU) as a postdoctoral fellow under the supervision of Prof. Peng Huang. His research interest focuses on the biomimetic synthesis of nanostructured biomaterials for biomedical applications including diagnosis, treatment, and theranostics. |
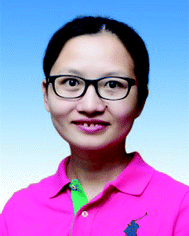
Jing Lin
| Jing Lin received her PhD in Organic Chemistry from the Donghua University and Shanghai Institute of Organic Chemistry, Chinese Academy of Sciences in 2010. Then she joined the PharmaResources (Shanghai) Co., Ltd as a group leader. After two years, she moved to the United States of America and spent 4 years as a postdoc at the University of Maryland and the National Institutes of Health (NIH). In 2016, she joined Shenzhen University (SZU) as an Associate Professor. Her research focuses on the self-assembly of functional nanomaterials for diagnosis, treatment, and theranostics of diseases. |
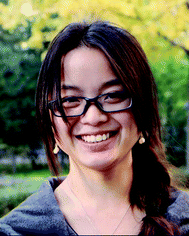
Lian-Hua Fu
| Lian-Hua Fu received her PhD in Chemical Processing Engineering of Forest Products from Beijing Forestry University in 2017. Then she joined the Laboratory of Evolutionary Theranostics (LET) at Shenzhen University (SZU) as a postdoctoral fellow under the supervision of Prof. Peng Huang and Prof. Jing Lin. Her research interest focuses on the design, synthesis, and biomedical applications of natural polymer hydrogel drug delivery systems for cancer therapy. |
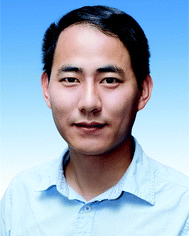
Peng Huang
| Peng Huang received his PhD in Biomedical Engineering from the Shanghai Jiao Tong University in 2012. Then he joined the Laboratory of Molecular Imaging and Nanomedicine (LOMIN) at the National Institutes of Health (NIH) as a postdoctoral fellow under the supervision of Prof. Xiaoyuan (Shawn) Chen. In 2015, he moved to Shenzhen University (SZU) as a Distinguished Professor and Chief of Laboratory of Evolutionary Theranostics (LET). His research focuses on the design, synthesis, and biomedical applications of molecular imaging contrast agents, stimuli-responsive programmed targeting drug delivery systems, and activatable theranostics. |
1. Introduction
Calcium, as the fifth-most-abundant element by mass in the crust of the Earth, is an essential mineral nutrient of living organisms, which plays important roles in a broad variety of cellular and physiological processes.1–4 Most of the calcium exists in the form of biominerals, including calcium phosphates (CaPs), calcium carbonates (CaCs), calcium silicate (CaSi) and calcium fluoride (CaF2), in biological hard tissues such as bones and teeth. Due to their excellent biocompatibility, bioactivity and biodegradability, synthetic calcium-based (CaXs) biomaterials including CaPs, CaCs, CaSi and CaF2 have been extensively investigated for a wide variety of biomedical applications.5–10 Generally, CaXs biomaterials are used to fabricate bioceramics,11,12 bioglass,13 bone cement14,15 and porous scaffolds16,17 for tissue engineering and bone repair, which can simulate the chemical composition and microstructure of natural bone tissues. Recently, thanks to the development of nanotechnology, nanostructured CaXs biomaterials have attracted increasing interest in biomedical fields such as diagnosis, treatment, and theranostics. CaXs biomaterials can dissolve into nontoxic ions (e.g. Ca2+, PO43−, CO32−, SiO32− and F−) and participate in the normal metabolism of organisms, which could overcome the dilemmas including poor biodegradability and potential long-term toxicity of traditional inorganic biomaterials such as silica-, carbon-, and gold-based biomaterials.
By combination of CaXs with imaging contrast agents (CAs) such as organic fluorophores (e.g. Cy3,18–20 indocyanine green (ICG),21–24etc.), inorganic nanomaterials (e.g. Au,25–27 Fe3O4,28–32 quantum dots (QDs),33–36etc.), or elements (e.g. Eu,37–41 Mn,42 Gd,43–45etc.), various molecular imaging modalities including fluorescence imaging (FLI), magnetic resonance imaging (MRI), ultrasound imaging (USI) and multimodal imaging can be achieved for diagnosis (Fig. 1). By combination of CaXs with therapeutic agents, various therapeutic approaches including chemotherapy, gene therapy, hyperthermia therapy (HTT), photodynamic therapy (PDT) and radiation therapy (RT) can be realized (Fig. 1). Therefore, the development of CaXs biomaterials will offer safer clinical solutions for diagnosis, monitoring, and prognosis of diseases by enhancing contrast in various imaging modalities followed by tailored therapy.
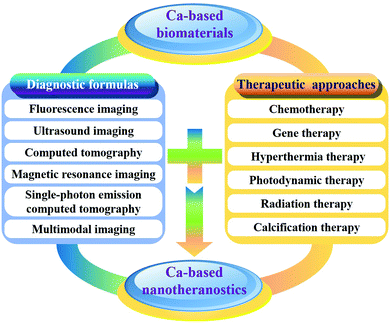 |
| Fig. 1 The main bioapplications of CaXs biomaterials. | |
The excellent biocompatibility and biodegradability together with other advantages of CaXs biomaterials such as easy preparation and functionalization, as well as high drug/gene loading capability and pH-dependent solubility, render them promising for the substitution of traditional inorganic biomaterials. Although much progress in CaXs biomaterials has been achieved in the fields of diagnosis, treatment, and theranostics, their colloidal stability, controllable preparation, and multi-functionalization should be carefully addressed before clinical translation. In this review, we will summarize the recent advances in CaXs biomaterials for diagnosis, treatment, and theranostics. We first discuss their categories, characteristics and preparation methods. Then, we highlight their bioapplications in detail. Finally, we discuss the current trends and key problems, as well as the future prospects and challenges of CaXs biomaterials.
2. Characteristics of CaXs biomaterials
Understanding the characteristics of CaXs biomaterials will be helpful for their design, synthesis and applications. The main characteristics and the corresponding bioapplications of CaXs biomaterials are summarized in Table 1 and further discussed in detail in the following.
Table 1 A summary of the major characteristics and applications of nanostructured CaXs biomaterials
Category |
Characteristics |
Biomedical applications |
Ref. |
CaPs |
Easy functionalization |
Bioimaging: |
|
pH-responsive drug release |
Fluorescence imaging |
18–22, 33, 37–41 and 46–65
|
Alterable structure and composition |
Magnetic resonance imaging |
42 and 43
|
Easy doping with other ions |
Multimodal imaging |
23, 24, 32, 44 and 66–68
|
Easy hybridization with organic phases |
Treatment: |
|
Easy coating on the surface of other nanomaterials |
Drug delivery |
69–153
|
High drug loading capability and gene transfection efficiency |
Protein adsorption |
150–192
|
Excellent biocompatibility and biodegradability |
Gene delivery |
69 and 193–253
|
|
Other treatments (e.g. HTT, PDT, etc.) |
26, 27 and 254–270
|
|
Theranostics |
20, 25, 30, 31, 45 and 271–305
|
|
CaCs |
CO2 gas-generating |
Ultrasound imaging |
306–308
|
pH-responsive drug release |
Treatment: |
|
High drug/gene loading capability |
Drug delivery |
309–347
|
Easy hybridization with organic phases |
Gene delivery |
346–358
|
Removable template for the synthesis of other biomaterials |
Protein/enzyme delivery |
359–367
|
Excellent biocompatibility and biodegradability |
Theranostics |
368–373
|
|
CaSi |
High surface area |
Treatment: |
|
Mesoporous/hollow structure |
Drug delivery |
374–382
|
High drug loading capability |
Protein adsorption |
380–384
|
pH-responsive drug release |
Gene delivery |
385
|
Excellent biocompatibility and biodegradability |
|
|
|
CaF2 |
Unique phosphor host |
Fluorescence imaging |
386–402
|
Excellent biocompatibility |
Protein adsorption |
403 and 404
|
Adjustable fluorescent properties |
Theranostics |
405–412
|
Easy doping with lanthanide ions |
|
|
Easy coating on the surface of upconversion nanoparticles |
|
|
2.1 Calcium phosphates (CaPs)
CaPs are the main inorganic constituents of biological hard tissues such as bones and teeth.413–415 A variety of CaPs with different properties can be prepared from aqueous solution by controlling the reaction conditions (Table 2). Among them, nanostructured amorphous calcium phosphate (ACP), hydroxyapatite (HAP) and calcium-deficient hydroxyapatite (CDHA) are prepared for bioapplications because they are stable in physiological conditions and are easy to be obtained from aqueous solution through a simple coprecipitation method.
Table 2 A summary of the major CaPs that are prepared from aqueous solutions and their properties. Reprinted with permission from ref. 413 and 415
Name |
Chemical formula |
Ca/P ratio |
Solubility at 25 °C, −log(Ks) |
Solubility at 25 °C (g L−1) |
pH stability range at 25 °C |
Monocalcium phosphate monohydrate (MCPM) |
Ca(H2PO4)2·H2O |
0.5 |
1.14 |
∼18 |
0.0–2.0 |
Dicalcium phosphate dihydrate (DCPD, brushite) |
CaHPO4·2H2O |
1.0 |
6.59 |
∼0.088 |
2.0–6.0 |
Octacalcium phosphate (OCP) |
Ca8(HPO4)2(PO4)4·5H2O |
1.33 |
96.6 |
∼0.0081 |
5.5–7.0 |
Amorphous calcium phosphates (ACP) |
CaxHy(PO4)z·nH2O, n = 3–4.5; 15–20% H2O |
1.2–2.2 |
Cannot be measured precisely, always metastable |
∼5–12 |
Calcium-deficient hydroxyapatite (CDHA) |
Ca10−x(HPO4)x(PO4)6−x(OH)2 (0 < x < 1) |
1.5–1.67 |
∼85 |
∼0.0094 |
6.5–9.5 |
Hydroxyapatite (HAP) |
Ca10(PO4)6(OH)2 |
1.67 |
116.8 |
∼0.0003 |
9.5–12 |
Fluorapatite (FAP) |
Ca10(PO4)6F2 |
1.67 |
120.0 |
∼0.0002 |
7–12 |
Nanostructured CaPs biomaterials are ideal carriers for drug/gene delivery because their surface is rich in –OH groups and Ca2+ cations which can effectively adsorb organic molecules with acid groups such as carboxylic (–COO−) and phosphoric groups.154–157 The unique surface characteristics of CaPs allow easy surface functionalization, and they can be designed as targeting nanocarriers for efficient drug/gene delivery to tumours.100,101,206–209,416 Moreover, the solubility of CaPs, especially ACP, increases with the decrease of the pH value of solution, and they can dissolve into ions at a low pH value.417–420 Thus, CaPs are widely designed as pH-responsive nanocarriers for drug/gene delivery.70,74,102–105
Inspired by biomineralization, CaPs are often mineralized with organic templates such as lipidosomes and block copolymers to form organic/inorganic hybrid biomaterials, which not only improve the stability of the organic phase in physiological conditions, but also act as a drug depot and render the drug release in a pH-responsive manner.97–99,200–205 Moreover, the hybridization of CaPs with mesoporous silica significantly increases their biodegradability by the escape of Ca2+ ions from the skeleton in an acid environment.137 Besides, CaPs coating on the surface of other inorganic nanomaterials can enhance their biocompatibility markedly.91–94,96,421 Specifically, CaPs coating on viruses promises to be an efficient strategy to develop viral materials with the characteristics of functional shells, which can overcome the limitations of the native viruses and open up the multiple applications of viruses with therapeutic potential.422,423 In addition, CaPs are usually designed as a gate-keeper system that not only protects payloads from nonspecific triggers but also realizes the pH-responsive release.128,134,424
Another important characteristic of CaPs materials is their structural capacity to accept many ionic substitutions, which not only regulates their composition, structures and solubility, but also renders them unexpected new properties. For example, as shown in Table 3, lanthanide-doped CaPs have excellent luminescent properties which can be used for FLI. By integrating with magnetic ions (e.g. Fe2+/Fe3+, Mn2+, Gd3+, etc.), the obtained magnetic CaPs are promising in MRI, HTT, and magnetic targeting cancer treatment. By labeling with radionuclides (e.g.99mTc, 153Sm, 111In, etc.), the radiolabelled CaPs can be utilized to realize nuclear imaging including single photon emission computer tomography (SPECT) and positron emission tomography (PET).24,44,67 More importantly, ion-doping can modulate the internalization and the payload release of CaPs-based biomaterials.115,162,425
Table 3 Commonly used doping ions in CaPs nanomaterials
2.2 Calcium carbonates (CaCs)
CaCs are ubiquitous and important biominerals in biological systems, and are widely used as a model system to study biomimetic processes.426–428 CaCs occur naturally in an unstable amorphous calcium carbonate (ACC) phase, two hydrated metastable forms (monohydrocalcite and calcium carbonate hexahydrate), and three anhydrous crystalline polymorphs including vaterite, aragonite and calcite in the order of increasing stability.429–431 Among these phases, ACC shows the highest solubility and is the precursor of the crystalline polymorphs, and thus it is very easy to crystallize in aqueous solution to form a more stable crystalline phase with large size (up to the microscale).432,433 The cell uptake of CaCs nanoparticles (NPs) depends on their aspect ratio, whereby the elongated NPs are internalized with a higher frequency than spherical NPs.434
CaCs NPs are stable under neutral pH, but they will decompose into Ca2+ ions and CO2 gas within an acidic solution. The dissolution of CaCs NPs in tumors induces the pH to change asymptotically to 7.4, which can intentionally modulate the acidic environment of tumors.435 Together with their outstanding biocompatibility and biodegradability, nanostructured CaCs biomaterials are proposed as ideal pH-responsive nanocarriers for drug/gene delivery.342–349 Moreover, the generated CO2 gas by CaCs biomaterials at tumoral acidic pH can significantly enhance the USI signal because the continuously generated CO2 nanobubbles coalesce into microbubbles which contribute to the persistence of strong echogenic USI. Therefore, CaCs biomaterials are ideal USI CAs with gas-generating properties in response to tumoral environments.306–308,369,370
For bioapplications, all kinds of additives including biomolecules328,363,436–438 and polymers439,440 are used as stabilizers to obtain stable and uniform CaCs NPs. Similar to CaPs nanomaterials, CaCs are also widely mineralized with organic phase to form organic/inorganic hybrid biomaterials,310–315,347 or used as the gate-keeper144,309 of mesoporous materials to realize pH-responsive payload release. After combination with QDs, CaCs are promising for biosensing and bioimaging.34–36 Besides, CaCs are widely used as self-sacrificing templates for the synthesis of porous hollow inorganic biomaterials,441–445 as well as for the preparation of various organic carriers446–451 due to their mild template elimination conditions. CaCs can recrystallize into CaPs biomaterials in phosphate solution owing to their higher thermodynamic solubility product (Ksp) than that of CaPs, so they can be used as templates or precursors for the synthesis of CaPs biomaterials via an ionic exchange reaction.438,452–455 Besides, CaCs are also utilized as a phase stabilizer of poorly water-soluble drugs to increase their bioavailability.456
2.3 Calcium silicate (CaSi)
CaSi biomaterials are widely used as bioceramics, bioglass and bone cement in bone tissue engineering.457–460 CaSi can entirely transform into CaPs after soaking in phosphate solution because their Ksp is much higher than that of CaPs.379,461 Nanostructured CaSi biomaterials have a high specific surface area and a large pore volume, and the Ca2+ cations on their surface can serve as the inherent active sites to effectively adsorb drug molecules, and thus CaSi nanomaterials are ideal drug delivery systems (DDSs) with a high drug loading capacity and pH-responsive drug release behavior.9,10,376–380 Moreover, CaSi can be coated on the surface of mesoporous inorganic biomaterials to enhance their biocompatibility and realize pH-responsive payload release.382,385,462
2.4 Calcium fluoride (CaF2)
CaF2 is an endogenous component in living organisms, and it is widely used as a promoting agent for bone/tooth reconstruction owing to its good biocompatibility.463 CaF2 has a well-known fluorite structure, a low refractive index and a wide band gap (Eg = 12.1 eV).386,405 CaF2 is a unique phosphor host with fascinating up/down-conversion luminescent properties, which make it a promising host material to generate upconversion luminescence for FLI.386 By doping different lanthanide ions into the host lattices, the luminescence properties of CaF2 can be tuned and enhanced.386–390 By doping with magnetic ions such as Gd3+ ions, it can be used for MRI.391,392 CaF2 is often chosen as the epitaxial shell material for upconversion nanoparticles (UCNPs) to enhance their luminescence intensity and biocompatibility.393,395–399 Moreover, CaF2 can adsorb calmodulin, a calcium sensor protein, on its surface for the detection of calcium concentration.403,404 Nanostructured CaF2 can also be used as a nanocarrier for imaging-guided chemotherapy.405–409 By conjugating with photosensitizers (PS), CaF2 NPs can be designed as a PDT platform for cancer treatment.410,411
3. Preparation of CaXs biomaterials
Different preparation methods have significant effects on the crystal phases and structures of CaXs biomaterials.464–466 For instance, nanostructured CaXs biomaterials with poor crystallinity are generally prepared through a room temperature coprecipitation method under mild conditions. In order to improve the crystallinity and control the structures of CaXs biomaterials, hydrothermal/solvothermal as well as microwave-assisted synthesis and sonochemical methods are developed. The comparison of different preparation methods for the synthesis of nanostructured CaXs biomaterials is summarized in Table 4 and further discussed in detail in the following.
Table 4 A comparison of different preparation methods for the synthesis of nanostructured CaXs biomaterials
Preparation methods |
Advantages |
Disadvantages |
Applications |
Room temperature coprecipitation method |
Simple, cheap, quick and biomolecule friendly |
– Poor crystallinity |
Widely utilized for the synthesis of CaPs, CaCs and CaSi at room temperature, as well as for drug/gene loading in situ |
Convenient doping with other ions |
– Unsuitable for CaF2 |
Convenient hybridization with organic phases |
– Hard to form hierarchical structures |
Easy coating on the surface of other nanomaterials |
|
Small size and uniform structures of the products |
|
In situ loading of drug/gene/protein |
|
|
Hydrothermal and solvothermal methods |
Good crystallinity of the products |
– Energy consumption |
Widely utilized to synthesize CaPs and CaF2 with high crystallinity and hierarchical structures |
Convenient doping with other ions |
– Cannot load drug/gene in situ |
Hierarchical structure of the products |
– Unsuitable for CaCs and CaSi |
Easy to control via heating temperature and time |
– Cannot hybridize with bioactive molecules |
|
Microwave-assisted synthesis method |
Fast and even heating |
– Energy consumption |
Widely utilized to synthesize CaPs and CaF2 with high crystallinity and hierarchical structures |
High reaction rate and efficiency |
– Cannot load drug/gene in situ |
Good crystallinity of the products |
– Unsuitable for CaCs and CaSi |
Convenient doping with other ions |
– Cannot hybridize with bioactive molecules |
Easy to control via heating temperature and time |
|
|
Sonochemical synthesis method |
Local high temperature and pressure |
– Energy consumption |
Widely utilized to synthesize CaPs, CaCs and CaSi nanomaterials |
Extremely rapid cooling rate |
– Unsuitable for CaF2 |
Ultrasonic dispersion in the preparation process |
– Cannot hybridize with bioactive molecules |
Convenient doping with other ions |
|
3.1 Room temperature coprecipitation method
Inspired by biomineralization, CaXs biomaterials can be directly prepared from the supersaturated solution at room temperature through the coprecipitation method. The experimental parameters including solution pH, ion concentration, solvent species, reaction time and additives are often used to control the size, phases and structures of CaXs.157,380,467–470 Due to the convenient, flexible and simple operation, the coprecipitation method is the main preparation method of CaPs, CaCs and CaSi NPs. By coprecipitation with other ions, ion-doped CaXs biomaterials can be easily obtained.37–40,471,472 Doping ions into CaXs nanostructures during the synthesis process not only regulates the crystal phase and morphology of the products, but also endows them with some new properties such as luminescent47,48 and magnetic254,255 properties. By controlling the nucleation and growth in a two phase system,473 or using various hard443,474 and soft475–481 templates during the preparation process, hollow structured CaXs biomaterials can be obtained. In addition, CaXs biomaterials can be directly deposited on the surface of other materials to form a coating layer through the coprecipitation method.28,96,271
However, the coprecipitation strategy of CaXs biomaterials at room temperature faces the challenge of uncontrolled growth, which results in larger products with inevitable aggregation. In order to prevent the uncontrolled growth and inevitable aggregation of CaXs biomaterials, a wide variety of organic additives including polymers98,200,482,483 and biomolecules363,436,484,485 are used to synthesize organic/inorganic hybrid nanomaterials by in situ precipitation of inorganic salts. Another strategy to obtain stable and uniform colloids of CaXs biomaterials is surface coating using organic layers.212,235,486
Thanks to mild synthesis conditions, many functional molecules including drugs,114,132 genes,213,215 proteins160,359 and enzymes487 can be directly encapsulated into CaXs biomaterials at room temperature during the precipitation process. The in situ loading method not only reduces the extra loading process, but also significantly improves the loading efficiency and maintains the bioactivity of functional molecules.
As an extension of the room temperature coprecipitation method, microemulsion or reverse emulsion approaches are widely used to prepare CaXs biomaterials with uniform size and narrow size distribution.155,168,202,231 Because the nucleation and growth of CaXs biomaterials are strictly restricted in microreactors of the microemulsion system, the agglomeration of the products can be effectively inhibited.466 Additionally, these approaches are very convenient to load hydrophobic drugs or efficiently encapsulate genes into CaXs biomaterials during their preparation process.79,97,216,233
3.2 Hydrothermal and solvothermal methods
Generally, hydrothermal and solvothermal methods are widely utilized to prepare CaPs and CaF2 biomaterials with high crystallinity and hierarchical structures. The crystallinity and structures of the products can be easily controlled by adjusting the solution pH, ion concentration, solvent species, reaction temperature and time, as well as organic additives.150,488–497 Similar to the coprecipitation method, ion-doped CaPs and CaF2 with high crystallinity can be obtained by adding other ions at the beginning of the reaction.90,407,498,499 Moreover, the hydrothermal method can accelerate the phase transformation of CaCs146–148 or calcium oleate175,500 precursors into CaPs by controlling the reaction temperature and time.
To further control the growth rate of CaPs nanostructures, biocompatible phosphorus-containing biomolecules (e.g. adenosine triphosphate (ATP), creatine phosphate, etc.) are used as organic phosphorus sources, which can regulate the morphologies, sizes, and structures of the products by controlling the hydrolysis rate of biomolecules.151,501–504 Compared to inorganic phosphorus sources, the strategy of using biocompatible phosphorus-containing biomolecules has many advantages (Fig. 2): firstly, phosphorus-containing biomolecules are essentially non-toxic with high biocompatibility. Secondly, the phosphorus source exists in the form of phosphate groups in organic biomolecules instead of free phosphate ions in the reaction solution, thus avoiding the fast nucleation and disordered growth of the products. Thirdly, phosphorus-containing biomolecules generally require certain conditions to hydrolyze for the formation of free phosphate ions, so the hydrolysis parameters can be used to control the hydrolysis rate of biomolecules, which determines the morphology, size, and structure of the products. Finally, the hydrolysis of phosphorus-containing biomolecules can regulate the crystal growth of the products and facilitate the formation of hierarchical nanostructures. Thus, this strategy of using phosphorus-containing biomolecules as organic phosphorus sources is considered to be a promising “green” synthesis method of nanostructured CaPs biomaterials.
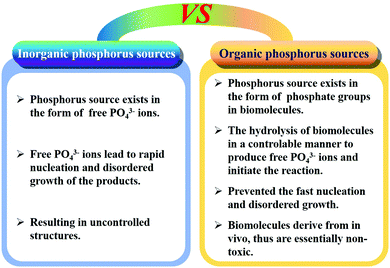 |
| Fig. 2 Comparison of inorganic phosphorus source and organic phosphorus source. | |
3.3 Microwave-assisted synthesis method
Compared to conventional hydrothermal/solvothermal methods, the microwave-assisted synthesis method has a higher reaction rate and efficiency due to the rapid volumetric heating, resulting in time saving and reduced energy consumption (Fig. 3).505 This method can control the crystallinity and structures of CaPs by adjusting the solution pH, ion concentration, solvent species, surfactants, as well as reaction temperature and time.88,89,180,183,506–508 So far, various nanostructured CaPs including nanorods (NRs) and nanowires,184,509 porous nanospheres,86 porous microspheres,85 porous hollow microspheres,153 yolk–shell porous microspheres,152 and so on87 have been prepared by this method using biocompatible phosphorus-containing biomolecules as phosphorus sources. In addition, ion-doped CaPs or CaF2 can be easily prepared by this method by adding the doped ions in the reaction system before microwave heating.149,389
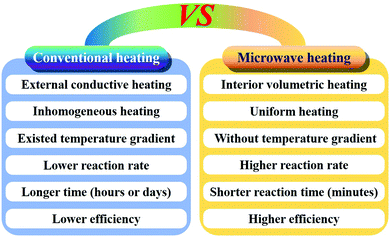 |
| Fig. 3 Comparison of conventional heating and microwave heating. | |
3.4 Sonochemical synthesis method
The sonochemical synthesis method is based on the acoustic cavitation resulting from the continuous formation, growth and implosive collapse of the bubbles in a liquid. The acoustic cavitation process leads to intense local heating, high pressure and extremely rapid cooling rate, which can drive chemical reactions.510,511 This method has been widely used to prepare CaXs biomaterials, such as ACP vesicle-like nanospheres,83 HAP NPs,512 HAP NRs,82,513 HAP nanoflowers,186 magnetic HAP,514 CaP hollow nanospheres,84 nanostructured CaCs microparticles,515 and nanostructured CaSi mesoporous microspheres.374,379,516
3.5 Other synthesis methods
Besides the above mentioned methods, there are some other synthesis methods of nanostructured CaXs biomaterials including sol–gel,517 high-temperature pyrolysis,175,390 spray pyrolysis,518,519 solution combustion,520 mechanochemical,521 precursor transformation,522 and high gravity methods.523
4. CaXs biomaterials for diagnosis
CaXs biomaterials are widely designed as CAs for bioimaging (Table 5). By combination of CaPs-based biomaterials with organic fluorophores (such as Cy3, ICG, etc.), inorganic nanomaterials (such as Au, Fe3O4, QDs, etc.), or elements (such as Mn2+, Eu3+, Gd3+, etc.), FLI, MRI, computed tomography (CT) and SPECT can be realized. By using CaCs biomaterials to generate CO2 gas bubbles at tumoral acidic pH, the USI signal can be significantly enhanced. Additionally, the luminescence properties of CaF2 can be tuned and enhanced by doping different rare earth (RE) ions into the host lattice. Therefore, CaXs biomaterials have great potential in bioimaging.
Table 5 CaXs biomaterials for bioimaging
Imaging modalities |
Host materials |
Applied methods |
Ref. |
Notes: FLI: fluorescence imaging; USI: ultrasound imaging; MRI: magnetic resonance imaging; CT: computed tomography; SPECT: single-photon emission computed tomography. |
FLI |
CaPs |
Self-activated luminescence by introducing defects or impurities |
45, 64 and 271–275
|
Rare-earth ion doping (e.g. Eu3+, Tb3+, Nd3+, Sm3+) |
37–41, 46–56, 68 and 276–286
|
Encapsulation/conjugation of fluorophores (e.g. Cy3, ICG, FITC, etc.) |
18–24, 57–60, 65, 66 and 287–292
|
Coating on UCNPs |
293 and 294
|
Conjugation with QDs |
33 and 61–63
|
Encapsulation of Au nanoclusters |
25
|
CaCs |
Encapsulation/conjugation of fluorophores |
368 and 371
|
Conjugation with QDs |
34–36
|
CaF2 |
Ion doping |
386–391, 402, 405–409 and 412
|
Coating on UCNPs |
306–308, 369, 370, 392–401 and 410
|
|
USI |
CaCs |
CO2 gas generation |
299–301, 362 and 363
|
|
MRI |
CaP |
Conjugation with iron oxide |
30–32, 301 and 302
|
Ion doping (e.g. Gd3+, Mn2+, Ho3+, Dy3+) |
23, 24, 42–45, 66, 68, 281–283 and 298
|
Coating on Gd3+-based UCNPs |
293 and 294
|
CaCs |
Ion doping (e.g. Mn2+) |
371
|
CaF2 |
Coating on Gd3+- or Dy3+-based UCNPs |
401 and 410
|
Ion doping (e.g. Mn2+, Gd3+) |
392 and 407
|
|
CT |
CaPs |
Ion doping (e.g. Eu3+/Gd3+) |
282
|
Encapsulation of Au nanoclusters |
25
|
CaF2 |
Ion doped or coating on UCNPs |
401, 407 and 408
|
|
SPECT |
CaPs |
Radio-labeling (e.g.153Sm, 111In, 177Lu, 99mTc) |
24, 44, 67 and 295–297
|
4.1 Fluorescence imaging (FLI)
FLI, as a low cost and non-invasive optical imaging, has been widely used in biomedical diagnosis. CaXs-Based FLI can be obtained by using self-activated luminescent CaPs and lanthanide doped CaPs as fluorescent agents. Moreover, nanostructured CaPs can also be utilized for FLI by combination with organic fluorophores or QDs. Besides, CaF2 as a unique phosphor host material for generating desirable upconversion luminescence has evoked considerable interest in FLI.
4.1.1 CaPs-based FLI.
Self-activated luminescent CaPs are promising fluorescent agents with good optical properties, high sensitivity and nontoxicity.524 For example, Deshmukh et al. synthesized a new class of nontoxic and self-activated fluorescent HAP NPs with a broad emission spectrum ranging from 350 to 750 nm and a maximum at 502 nm, which could be attributed to the electronic transition in the asymmetric structure of NPs.64 Owing to their excellent biocompatibility and biodegradability, this class of self-activated fluorescent CaPs nanomaterials provided a safe and potential alternative to currently used fluorescent materials for bioimaging.
Lanthanide doped CaPs biomaterials have excellent fluorescence properties for bioimaging.37,38,53 For instance, Eu-doped CaPs NPs with stable luminescence can be used as a luminescent probe to observe the internalization of NPs and the traffic of grafted biomolecules in cells.49 The trace amount of HAP NPs in cells and tissues can be quantitatively detected on the basis of the quantitative fluorescence determination of Eu3+ ions in the HAP crystal lattice.55 The Wei group prepared water-dispersible hydrophilic fluoridated HAP:Ln3+ (Ln = Eu or Tb) NPs via hydrophobic/hydrophilic transformation with surfactants (Pluronic F127) or via hydrophobic interactions between oleic acid and amphiphilic copolymers.39,40 The enhanced colloidal stability together with the unique luminescent properties and excellent biocompatibility endowed the fluoridated HAP:Ln3+ (Ln = Eu or Tb) NPs promising for cell imaging. Additionally, different ion co-doped CaPs-based biomaterials have unexpected fluorescence properties. Ma and co-workers reported spindle-shaped Eu/Tb co-doped fluorinated HAP NPs that could be excited by a 488 nm laser and which exhibited both green and red light emissions.47 The emission in the green region (500–580 nm) decreased to the benefit of the emission in the red region (580–720 nm) by changing the amount of Eu and Tb, resulting in the balance of dual color emission. Therefore, Eu/Tb co-doped fluorinated HAP NPs are very useful for dual-color cell imaging. Recently, Xie and colleagues designed a luminescence enhanced (elevated up to about 120%) imaging agent based on Eu/Gd co-doped HAP nanocrystals through the energy transfer from Gd3+ to Eu3+.48 These biocompatible, biodegradable, and luminescence enhanced Eu/Gd co-doped HAP nanocrystals had the potential to act as FLI agents both in vitro and in vivo.
By encapsulation of fluorophores, CaPs-based biomaterials are promising for early diagnosis of solid tumors. Muddana and colleagues determined the principle photophysical properties of Cy3 loaded CaP NPs using steady-state and time-resolved fluorescence spectroscopy.19 The fluorescence intensity, quantum efficiency and fluorescence lifetimes of Cy3 had been significantly enhanced after encapsulating in CaP NPs. The enhanced photophysical properties and excellent biocompatibility made them ideal for bioimaging. As shown in Fig. 4, the Adair group used bioresorbable CaP to encapsulate the near-infrared (NIR) fluorophore ICG for bioimaging.21,22 The ICG-loaded CaP NPs (ICG-CPNPs, Fig. 4A–C) had better optical properties including higher emission intensity (Fig. 4D), 4.7-fold longer fluorescence half-life (Fig. 4E) and a 2-fold increase in quantum efficiency relative to the free fluorophore, which provided increased brightness and prolonged signal intensity (Fig. 4F–I). By conjugation with various targeting ligands such as human holotransferrin, anti-CD71 antibody, and short gastrin peptides, the ICG-loaded and silicate-doped CaPs NPs could actively target to breast and pancreatic cancer lesions for cancer diagnosis.22
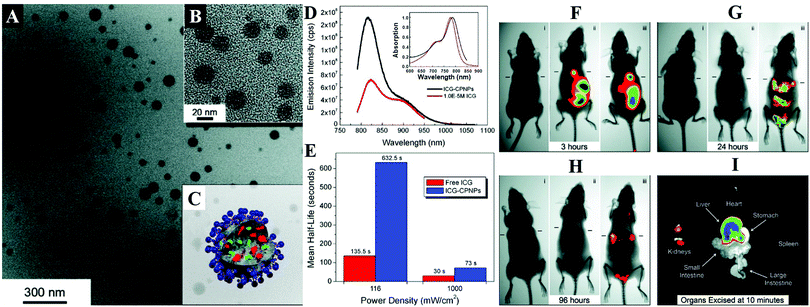 |
| Fig. 4 (A) TEM micrograph and (B) magnified image of ICG-loaded CaP NPs (ICG-CPNPs). (C) Schematic representation of the CaP NP architecture showing the encapsulated imaging agent (green) and any alternate payload (red) with full surface functionalization (blue). (D) Absorption (inset) and fluorescence spectra of free ICG (red) and ICG-CPNPs (black) in aqueous solution. (E) Fluorescence half-life for a free ICG fluorophore (red) and ICG-CPNPs (blue) suspension of matching absorption (10−6 M) in PBS under 785 nm excitation at two power densities. (F–H) NIR transillumination images (Ex. 755 nm, Em. 830 nm) taken at various times track fluorescence signals and pharmacokinetic distributions for the ICG-CPNPs and controls delivered systemically via tail vein injections in nude mice implanted with subcutaneous human breast adenocarcinoma tumors. Hash marks next to each mouse indicate the position of the 5 mm tumors. Two control samples, (i) carboxylate-terminated CaP NPs without the ICG encapsulant and (ii) free ICG, match the particle concentration and fluorophore content (1013 particles per mL and 10−5 M, respectively) of a (iii) PEGylated ICG-CPNPs sample. The excised organs in panel (I) illustrate the biliary clearance route at 10 min postinjection of PEG-ICG-CPNPs. Reprinted with permission from ref. 21. Copyright 2008, American Chemical Society. | |
Additionally, QD-conjugated CaPs were developed for cell imaging.33 For instance, Zhao et al. reported a bio-nanoplatform based on carbon dots conjugated with F-substituted nano-HAP for bioimaging, which exhibited bright blue fluorescence under UV illumination, excellent photostability and colloidal stability, low cytotoxicity and good biocompatibility.62
4.1.2 CaF2-Based FLI.
CaF2 is a unique host material for generating upconversion luminescence. In recent years, lanthanide-doped CaF2 UCNPs have evoked considerable interest due to their superior upconversion fluorescence efficiencies, for instance, monodispersed CaF2:Yb3+/Er3+ nanocrystals with bright green upconversion luminescence.386 Dong and colleagues reported a remarkable two-photon excited fluorescence efficiency in the “biological window” of CaF2:Tm3+,Yb3+ NPs with an outstanding tissue penetration depth as large as 2 mm, which were ideal candidates for in vivo deep FLI.387 Moreover, Zheng and co-workers fabricated monodisperse and highly emissive sub-10 nm CaF2:Ln3+ (Ln = Ce, Tb; Yb, Er; Yb, Tm) core-only and core/shell nanoprobes (Fig. 5A–J) through a high temperature coprecipitation route and sodium co-doping.390 The ultrasmall CaF2:Ce,Tb nanoprobes with intense long-lived photoluminescence (PL) were employed for the detection of avidin and tumor marker urokinase-type plasminogen activator receptor (uPAR) in homogeneous time-resolved (TR) fluorescence resonance energy transfer (TR-FRET, Fig. 5N–R) and heterogeneous TRPL bioassays (Fig. 5K–M). The long-lived luminescence of Ln3+ and the small size of NPs led to record-low LOD (limit of detection) of 164 pm and 48 pm for the detection of avidin in homogeneous TR-FRET and heterogeneous TRPL bioassays, respectively.
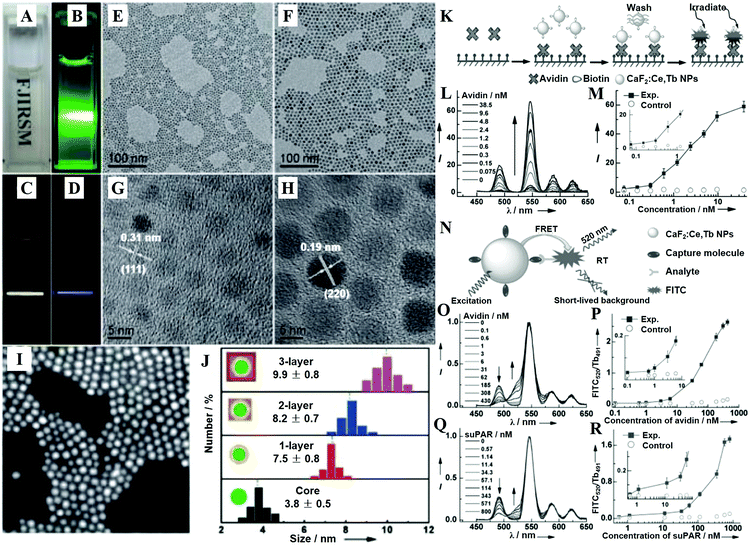 |
| Fig. 5 (A) Photograph showing the transparency of the CaF2:Ln3+ (Ln = Ce, Tb; Yb, Er; Yb, Tm) NPs dispersed in cyclohexane. (B) DS-luminescence photograph of CaF2:Ce,Tb NPs dispersed in cyclohexane under laser irradiation at 280 nm. Upconversion luminescence (UCL) photographs of the 1 ML CaF2:Yb,Er@CaF2 (C) and CaF2:Yb,Tm@CaF2 core/shell NPs (D) dispersed in cyclohexane under NIR laser irradiation at 980 nm. TEM (E and F) and HRTEM (G and H) images of CaF2:Yb,Er core-only (E and G) and 1 ML (F and H) core/shell NPs. (I) HAADF-STEM image of 1 ML CaF2:Yb,Er@CaF2 core/shell NPs. (J) Size distribution of CaF2:Yb,Er core-only and core/shell NPs as obtained from 200 particles in the TEM images. (K) Schematic representation of the heterogeneous TRPL detection of avidin. (L) TRPL spectra of the bioassays with biotinylated CaF2:Ce,Tb NPs as probes as a function of avidin concentration. (M) Calibration curve for TRPL detection: integrated PL intensity versus the concentration of avidin. The control experiment was conducted with nonbiotinylated NPs as bioprobes. (N) Schematic illustration showing the principle behind TRFRET detection. (O) TR-FRET spectra of the bioassay with biotinylated CaF2:Ce,Tb NPs as bioprobes as a function of avidin concentration. (P) Calibration curve for TR-FRET detection: FITC520/Tb491 ratio of the integrated PL intensities versus the concentration of avidin. The control experiments were conducted with nonbiotinylated NPs as bioprobes. (Q) TR-FRET spectra of the bioassay with ATF-coupled CaF2:Ce,Tb NPs as bioprobes as a function of suPAR concentration. (R) Calibration curve for TR-FRET detection: FITC520/Tb491 versus the concentration of suPAR. The control experiments were conducted with non-ATF-coupled NPs as bioprobes. All spectra in (B) and (D) were normalized at the maximum emission peak at 546 nm. Reprinted with permission from ref. 390. Copyright 2013, Wiley-VCH. | |
CaF2 is often chosen as an epitaxial shell material for UCNPs to enhance their upconversion emissions and biocompatibility, which is more effective in the resistance of quenching in aqueous medium than the typical β-NaYF4 shell.398 The CaF2 shells that are grown epitaxially over the NaxYF3+x:Yb,Er UCNPs can promote the red emission by 450 times, leading to promising in vitro and in vivo bioimaging applications.396 The ultrasmall NaYbF4:Tb@CaF2 NPs also exhibit a significant enhancement (690-fold) in cooperative sensitization upconversion emission intensity compared with the parent of NaYbF4:Tb by introducing the CaF2 inert shell to shield the sensitizer from quenchers.400 In addition, the Han group reported novel and biocompatible core/shell (α-NaYbF4:Tm3+)/CaF2 NPs (Fig. 6B–D) that exhibit highly efficient NIRin (∼980 nm)–NIRout (∼800 nm) upconversion (Fig. 6A and E) for high contrast and deep tissue bioimaging.393,394 The biocompatible CaF2 shell resulted in a 35-fold increase in the intensity of upconversion PL from the core by suppressing surface quenching effects. After covalently conjugated with polyethylenimine (PEI), the obtained (α-NaYbF4:Tm3+)/CaF2 UCNPs showed reasonable cytocompatibility at a concentration and exposure time adequate for efficient labeling of rat mesenchymal stem cells, and thus they were promising for cell labeling and in vivo tracking.
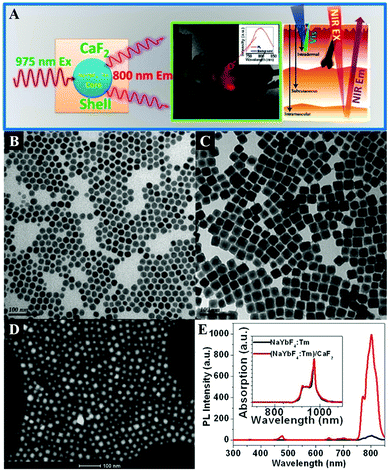 |
| Fig. 6 (A) Illustration of the core/shell (α-NaYbF4:Tm3+)/CaF2 NPs with highly efficient NIRin–NIRout upconversion for high contrast and deep bioimaging. TEM images of (B) NaYbF4:0.5% Tm3+ core and (C) (NaYbF4:0.5% Tm3+)/CaF2 core/shell NPs. (D) High-angle annular dark field scanning transmission electron microscopy image of (NaYbF4:0.5% Tm3+)/CaF2 NPs with resolved core/shell structures; both the core (bright) and the shell (dark) are clearly visible. (E) Upconversion photoluminescence spectrum of (NaYbF4:0.5% Tm3+)/CaF2 core/shell nanoparticles under laser excitation at 975 nm. Reprinted with permission from ref. 393. Copyright 2012, American Chemical Society. | |
4.2 Magnetic resonance imaging (MRI)
MRI can provide highly quantitative and qualitative anatomical details of soft tissues, resulting in the precise diagnosis of malignant tumors. By combination of CaXs with paramagnetic ions such as Gd3+ and Mn2+ or Fe3O4 NPs, CaXs-based MRI can be achieved. For example, Mi and colleagues developed organic–inorganic hybrid NPs composed of a CaP core, a PEGylated shell and gadolinium(III)-diethylenetriaminepentaacetic acid (DTPA) (Gd-DTPA/CaP NPs) for non-invasive diagnosis of solid tumors, which showed a significant enhanced retention ratio in blood circulation and high accumulation in tumor positions via the enhanced permeability and retention (EPR) effect.43 As a result, Gd-DTPA revealed a greater than 6 times increase of relaxivity in the NP system compared to the free form, and eventually, selective and elevated contrast enhancements in the tumor positions. Similarly, they reported another MRI CA that rapidly amplifies MRI signals in response to pH by confining Mn2+ within pH-sensitive CaP NPs with a PEG shell (PEGMnCaP, Fig. 7A–C).42 At a low pH, such as in solid tumours, the CaP disintegrated and released Mn2+ ions (Fig. 7D–G) that bound to proteins to increase the relaxivity, thus enhancing the contrast (Fig. 7H). As a result, these NPs could rapidly and selectively brighten solid tumours, identify hypoxic regions within the tumour mass and detect invisible millimetre-sized metastatic tumours in the liver (Fig. 7I–L). The amplification of the relaxivity from the pH-triggered release and complexation of Mn2+ ions with biomolecules provided a comprehensive and effective tool for enhancing the contrast of the tumour microenvironment or metastatic niches, which could potentially be used to monitor the biological processes of tumours and improve cancer diagnosis.
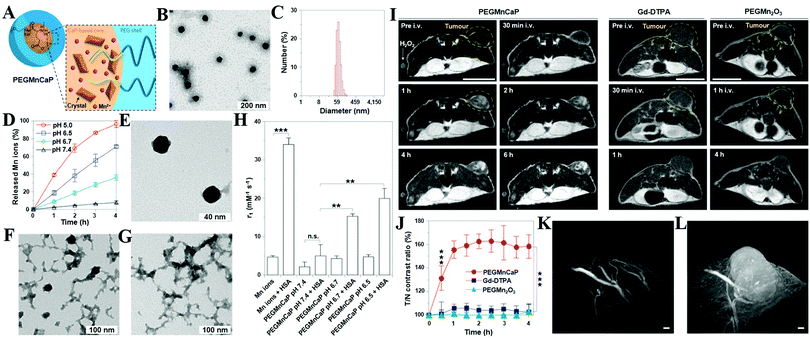 |
| Fig. 7 (A) Schematic illustration of the hybrid structure of PEGMnCaP. (B) TEM image of PEGMnCaP stained with uranyl acetate. (C) Number-average diameter of PEGMnCaP characterized by DLS. (D) Release profiles of PEGMnCaP under physiological conditions at different pH. (E–G) TEM images of PEGMnCaP after 4 h incubation at pH 7.4 (E), 6.7 (F) and 5.5 (G) in phosphate-buffered saline. (H) The r1 relaxivity of PEGMnCaP in physiological environments at different pH and with/without proteins (for example, HSA). (I) In vivo MR images of subcutaneous C26 tumour-bearing mice pre- and post-intravenous injection (i.v.) of PEGMnCaP (left), Gd-DTPA (centre) and PEGMn2O3 (right) measured with 1T MRI. Scale bar, 1 cm. (J) A comparison of the T/N contrast ratio after the administration of PEGMnCaP, Gd-DTPA and PEGMn2O3. (K and L) 3D MRI of C26 tumours before (K) and 1 h after (L) the intravenous injection of PEGMnCaP measured with 7T MRI. Scale bars, 50 μm. Reprinted with permission from ref. 42. Copyright 2016, Nature Publishing Group. | |
4.3 Ultrasound imaging (USI)
USI is based on the backscattering or reflection of high-frequency sound waves from the tissue with different ultrasonic properties, and thus it can show the structure of tissues inside the body. It is a common non-invasive diagnostic tool which is easy to operate, and it is more safe and comfortable for patients than other diagnostic methods. However, it has a low imaging resolution because tumor tissues have similar ultrasonic properties to normal tissues, which increases the difficulty of distinguishing tumor tissues from normal ones. Fortunately, CaCs-based ultrasound enhanced contrast agents (UECAs) have been developed to improve the resolution of USI. CaCs biomaterials are able to generate CO2 gas at tumoral acidic pH, which can significantly enhance the USI signal and contribute to the persistence of strong echogenic USI because the continuously generated CO2 nanobubbles coalesce into microbubbles. As an example, Kim et al. encapsulated CaC NPs into a flexible and biocompatible pluronic-based nanocarrier to construct a UECA, which showed high colloidal stability in serum-containing media and significant USI contrast enhancement at the tumor site in 1 h, in contrast to no change in the liver, followed by a rapid clearance from the body within 24 h.307
4.4 Multimodal imaging
Multimodal imaging is promising to furnish complementary information (such as anatomic, physiologic, molecular, and genomic information) for accurate diagnosis, monitoring therapy response, guiding drug discovery/development, and so on. In order to improve the imaging accuracy and overcome the defects of different imaging strategies, the development of multifunctional probes for multimodal bioimaging is highly desirable. Liu et al. prepared Gd-doped HAP NRs and then effectively post-labeled samarium-153 (153Sm) with a labeling rate of ∼100% and a radio-labeling stability in vitro of ∼100% over 48 h, which could serve as a dual-modal probe for in vivo SPECT and MRI.44 Similarly, Ashokan and co-workers reported 99m-technetium-methylene diphosphonate (99mTc-MDP) labeled ICG/Gd3+ co-doped CaP NPs for combined NIR (Fig. 8A and B), magnetic (Fig. 8C and D) and nuclear (Fig. 8E and F) trimodal imaging in vivo (Fig. 8G–L).23,24 Besides, Li and colleagues used a lanthanide-based core–shell–shell nanocomposite NaYbF4:Tm@CaF2@NaDyF4 as a multifunctional CA for trimodal MRI/CT/FLI lymphatic bioimaging.401 The introduction of a middle CaF2 layer was necessary as a barrier to reduce cross-relaxation and the surface quenching effect, which enhanced the upconversion emission of Tm3+.
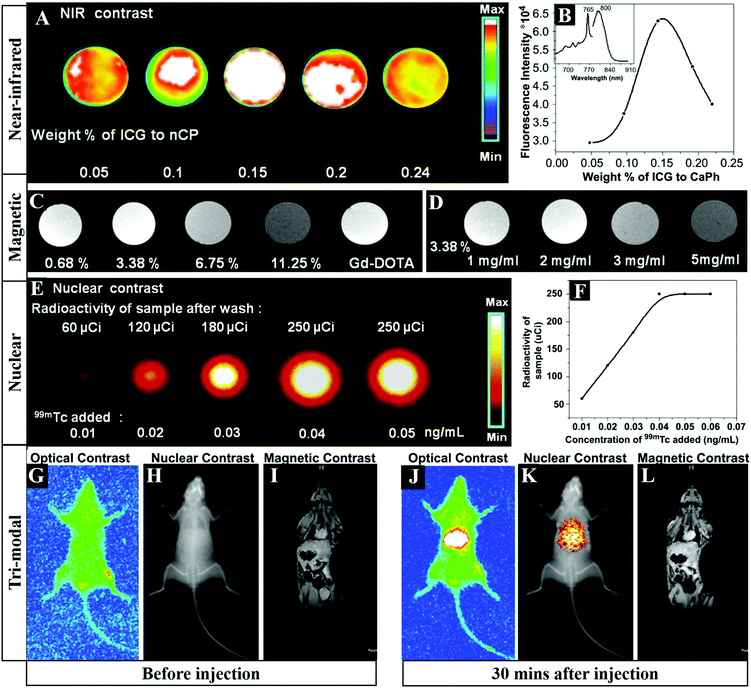 |
| Fig. 8 FLI (A) and integrated emission intensity (B) of CaP samples doped with increasing concentrations of ICG. The inset in (B) represents the fluorescence spectra of ICG doped CaP. (C) T1 weighted magnetic contrast images of 1 mg mL−1 ICG-CaP samples doped with different atomic percentages of Gd3+. (D) T1 weighted magnetic contrast image of ICG-CaP doped with different concentrations of 3.38% Gd3+. (E) Nuclear contrast image of ICG-Gd-CaP (20 mg mL−1) conjugated with varying concentrations of 99mTc-MDP. (F) Graphical representation of the amount of radioactivity from the labeled samples as a function of 99mTc added during synthesis. (G–L) Tri-modal imaging including NIR FLI, nuclear emission overlaid over the X-ray image and T1 based MRI in Swiss albino mice before (G–I) and 30 min after (J–L) tail vein injection of ICG-Gd–CaP–99mTc. Reprinted with permission from ref. 24. Copyright 2013, Elsevier. | |
5. CaXs biomaterials for treatment
CaXs biomaterials are widely investigated as nanocarriers for therapeutic applications including chemotherapy, gene therapy, HTT, PDT, RT, calcification therapy and combination therapy because of the low cost, easy synthesis and surface functionalization, outstanding biocompatibility and high resorbability, as well as pH-dependent solubility, along with the positively charged Ca2+ ions that are exposed on the surface and have a high affinity for negatively charged acidic groups such as carboxylic and phosphoric groups.154,156,525 Moreover, HAP NPs possess cancer-specific cytotoxicity due to their enhanced cellular uptake and mitochondrial targeting together with the prolonged elevation of intracellular Ca2+ ion concentration, which could significantly delay the tumor growth.526,527
5.1 Chemotherapy and gene therapy
5.1.1 CaPs-Based drug/gene delivery systems.
Pure CaPs nanocarriers.
CaPs-Based biomaterials are widely used as nanocarriers for drug,70–75 protein174–178 and gene193–196 delivery. The adsorption and desorption kinetics of bioactive molecules are strongly dependent on the interaction between drugs and CaPs NPs. Palazzo et al. investigated the adsorption and desorption of the anticancer drugs cisplatin, alendronate and di(ethylenediamineplatinum)medronate (DPM) towards two kinds of HAP materials with different morphologies and physicochemical properties.76 The negatively charged alendronate was strongly adsorbed on the negatively charged surface of HAP by ligand exchange in which the two phosphonate groups of drug molecules replaced two surface phosphate groups of HAP. Since the adsorption of the two platinum complexes was driven by electrostatic attraction, the positively charged cisplatin was strongly adsorbed, while the neutral DPM complex showed lower affinity towards the negatively charged surface of HAP. Significantly, the HAP with a phosphate-rich surface was favored to adsorb positively charged hydrolysis species of cisplatin, while the HAP with a Ca-rich surface was favored to adsorb negatively charged alendronate. Moreover, Iafisco et al. reported that the Ca/P ratio of CaP plays an important role in the adsorption and release of anticancer platinum bisphosphonates, in which the CaP with a higher Ca/P ratio showed greater affinity for platinum complexes.77 Therefore, the properties of CaPs biomaterials can be modulated to produce CaPs/drug conjugates for specific therapeutic applications.
Protein drugs such as peptide antigens,161 vaccines179 and antibacterial immunoglobulin Y (IgY)181,182 can be adsorbed on the CaPs nanomaterials via electrostatic attraction, especially the carboxyl groups of proteins with high affinity for Ca2+ ions.156–158 The protein adsorption capacity of CaPs can be controlled by surface functionalization187–189 and by changing the surface structure including the preferential growth direction and porous morphology.166 The protein release rate can also be controlled by changing their particle size, surface area and phase composition.155
CaPs-Based nanocarriers are frequently used to transfer genes into living cells,197–199 which could overcome the host innate immune responses compared with the viral vector, and have minimal cytotoxicity compared with other inorganic nanocarriers. The key parameters for effective CaPs-based transfection are an optimal concentration of Ca2+ and Cl− ions and a nanosized non-agglomerated precipitate, and thus the mechanical removal of microscale agglomerates by filtering and the addition of Ca2+ and Cl− ions can enhance the binding of genes to NPs and final expression.246 CaPs NPs are commonly covered and stabilized by DNA molecules as soon as they are synthesized by a precipitation method in aqueous solution, which can form a stable colloid and did not lose their ability for cell transfection even after several weeks of storage.210,212 Neumann and co-workers investigated Ca uptake in the human bladder carcinoma cell line T24 after transfection with the standard CaP transfection method and with DNA-covered CaP NPs by isotope labelling with 45Ca and by time lapse imaging microscopy using Fura-2.214 It was found that the intracellular Ca homeostasis and the cell viability of T24 cells were not affected after transfection using DNA-covered CaP, which was in remarkable contrast to cells transfected by the standard CaP method.
To further improve the transfection efficiency, one more layer of CaPs was covered on the surface of DNA-coated CaPs, and a subsequent outermost layer of DNA or polyelectrolyte on the NPs led to colloidal stabilization, and thus such a multishell structure can avoid the degradation of genes within the cells by lysosomes.247,248 Moreover, the surface-functionalization of CaPs NPs with a layer of PEI endows a positively charged surface, which exhibits high adsorption capacity and transfection rates for anionic nucleic acids with almost complete cell viability.229
Ion-doped CaPs nanocarriers.
For CaPs nanocarriers, the ion-doping strategy not only causes the change of their physicochemical properties including composition, crystallinity, morphology and solubility,149 but also modulates their cellular internalization425 and payload delivery performance.162 For example, Fe-doped HAP exhibits a decreased crystallite size and crystallinity along with a morphological change from spherulites to rods, and leads to highly prolonged drug release compared to the undoped HAP.115 Silicate ions on the silicate-substituted HAP(100) surface cause a shield effect on the adsorption of proteins, which is due to charge repulsion and steric hindrance of silicates.154 Besides, Zn-doped HAP nanocarriers exhibit a highly selective protein adsorption property.185 Thus, ion-doping is a powerful strategy to modulate the physicochemical properties of CaPs-based nanocarriers for specific applications.145,173
Hierarchical or hollow CaPs nanocarriers.
To improve the drug/gene loading capacity, hierarchical or hollow CaPs nanostructures are prepared. Ye et al. reported that HAP hollow nanospheres and nanotubes had much higher drug payload than traditional HAP NPs by using vancomycin as the model drug.111 After coupling with a layer of citrate molecules on their surfaces, the HAP nanotubes could further improve the drug load efficiency and form an excellent pH-controlled open/closed gate for drug release with the addition of cationic polyelectrolytes. Yang et al. reported HAP NPs with a hollow core and mesoporous shell structure for pH-responsive intracellular drug delivery.103 Compared with solid NPs, the hollow and mesoporous structures could significantly enhance the drug-loading capacity. Meanwhile, the thin shell structure reduced the burst release of drug and provided the pH-responsive property. Moreover, the Zhu group prepared yolk–shell porous CaPs microspheres (Fig. 9A),152 hollow porous CaPs microspheres (Fig. 9B–E)130,136,153 and CaPs nanoflowers (Fig. 9F)186 for drug/protein delivery by using phosphorus-containing biomolecules as organic phosphorus sources. Besides, the Cao group reported the hybrid HAP microparticles with a hollow HAP core and a polymer multilayer shell for thermal/pH dual-responsive drug delivery.140–142
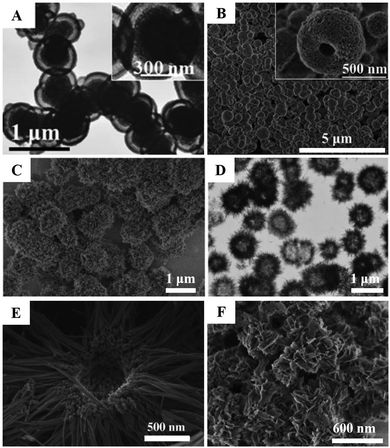 |
| Fig. 9 Typical hierarchical and/or hollow CaPs nanocarriers that were synthesized by using biocompatible phosphorus-containing biomolecules as an organic phosphorus source. (A) CaP yolk–shell porous microspheres. Reprinted with permission from ref. 152. Copyright 2015, Wiley-VCH. (B) ACP hollow porous microspheres. Reprinted with permission from ref. 136. Copyright 2015, Royal Society of Chemistry. (C and D) HAP hollow porous microspheres. Reprinted with permission from ref. 153. Copyright 2013, Wiley-VCH. (E) HAP hollow porous microspheres. Reprinted with permission from ref. 130. Copyright 2013, Wiley-VCH. (F) HAP nanoflowers. Reprinted with permission from ref. 186. Copyright 2016, Royal Society of Chemistry. | |
Organic–inorganic hybrid CaPs nanocarriers.
The uncontrolled growth and poor colloidal stability of CaPs nanocarriers are the main obstacles for their application in cancer treatment. To overcome these problems, biocompatible organic molecules such as chitosan,122,133,203,237 collagen,123 alginate,102,143 cyclodextrin,164 hyaluronic acid (HA),97,112 polyelectrolytes,129,171 block copolymers117,249–252 and lipids119,219,245 are introduced in the synthesis of CaPs NPs to form organic–inorganic hybrid biomaterials. The organic phase such as a block copolymer is usually used as a template to control the synthesis of CaPs nanocarriers, which can significantly enhance the colloidal stability and form uniform organic–inorganic hybrid NPs. These hybrid NPs have an adjustable diameter, excellent colloidal stability and serum tolerability, as well as fairly high drug/gene loading capacity.107,110,200,201 The drug/gene loaded hybrid NPs can be taken up by cells through endocytosis, followed by dissociation in the intracellular environment along with the release of payloads in a controlled manner.201 More interestingly, organic–inorganic hybrid CaPs NPs show higher gene binding capacity and transfection efficiency compared to CaP precipitates prepared according to the standard CaP transfection procedure.217
For instance, Giger et al. proposed a versatile method to stabilize CaP–DNA NPs by using poly(ethylene glycol)-functionalized bisphosphonate and PEGylated chelating agents.226,236 The prepared NPs exhibited good physical stability over several days, sustained ability of cell transfection as well as low toxicity. Xie and colleagues reported anionic-charged hybrid NPs composed of PEG grafted carboxymethyl chitosan and CaP for siRNA delivery through a single-step self-assembly method in aqueous solution (Fig. 10A).203 The hybrid NPs exhibited efficient siRNA encapsulation and enhanced colloidal and serum stability, which could enter into HepG2 cells by endocytosis, dissociate in the endosome, and then achieve endosomal escape of siRNA effectively (Fig. 10B), resulting in the apoptosis of HepG2 cells. The siRNA-loaded hybrid NPs passively accumulated in the tumor regions by the EPR effect, resulting in efficient gene silencing and tumor growth inhibition. In addition, Pittella et al. developed smart polymer/CaP/siRNA hybrid NPs with biocompatibility, reversible stability and endosomal escape functionality, by using a pH sensitive block copolymer of PEG and charge-conversional polymer (PEG–CCP) as building blocks.204,205 In this case, anionic functional groups of PEG–CCP could convert into cationic groups in the endosomal acidic condition, which facilitated the endosomal escape of siRNA. The hybrid NPs possessed excellent siRNA-loading efficiency (∼80% of dose) and favored efficient gene silencing in pancreatic cancer cells without obvious cytotoxicity. Systemic administration of the vascular endothelium growth factor (VEGF) siRNA loaded hybrid NPs could significantly inhibit the pancreatic tumor growth, and the VEGF gene silencing efficiency of tumor could reach ∼68%, which was due to the enhanced tumor accumulation of siRNA.
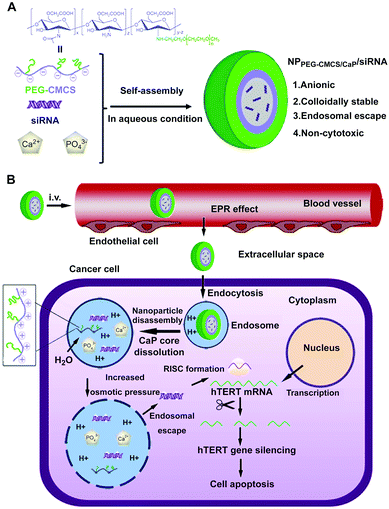 |
| Fig. 10 Schematic diagram of (A) formation of siRNA loaded PEG-CMCS/CaP hybrid anionic NPs (NPPEG-CMCS/CaP/siRNA). (B) NPPEG-CMCS/CaP/siRNA for systemic delivery of hTERT siRNA in anticancer therapy. Reprinted with permission from ref. 203. Copyright 2014, Elsevier. | |
For drug delivery, Min and co-workers fabricated CaP-reinforced core–shell–corona micelles as a nanocarrier to deliver doxorubicin (DOX) for cancer therapy.98 As shown in Fig. 11, the polymer micelles of poly(ethylene glycol)-b-poly(L-aspartic acid)-b-poly(L-phenylalanine) (PEG–PAsp–PPhe) provided three distinct functional domains in the aqueous phase, including the hydrated PEG outer corona for prolonged circulation, the anionic PAsp middle shell for CaP mineralization, and the hydrophobic PPhe inner core for DOX loading (Fig. 11A). The DOX release of DOX-loaded mineralized micelles (DOX-CaP-PM) was efficiently inhibited at physiological pH, whereas at an endosomal pH (pH 4.5), the DOX release was facile due to the rapid dissolution of CaP mineral layers in the middle shell domains (Fig. 11B and C). Due to the prolonged blood circulation and EPR effect, the CaP-mineralized micelles exhibited high tumor accumulation and excellent therapeutic efficacy compared with the DOX-loaded nonmineralized polymer micelles (DOX-NPM) (Fig. 11D and E). Similarly, Han et al. developed biostable CaPs-based NPs with high tumor targetability through the PEG-conjugated HA NP-involved mineralization, for pH-dependent drug release and effective antitumor efficacy.97,112
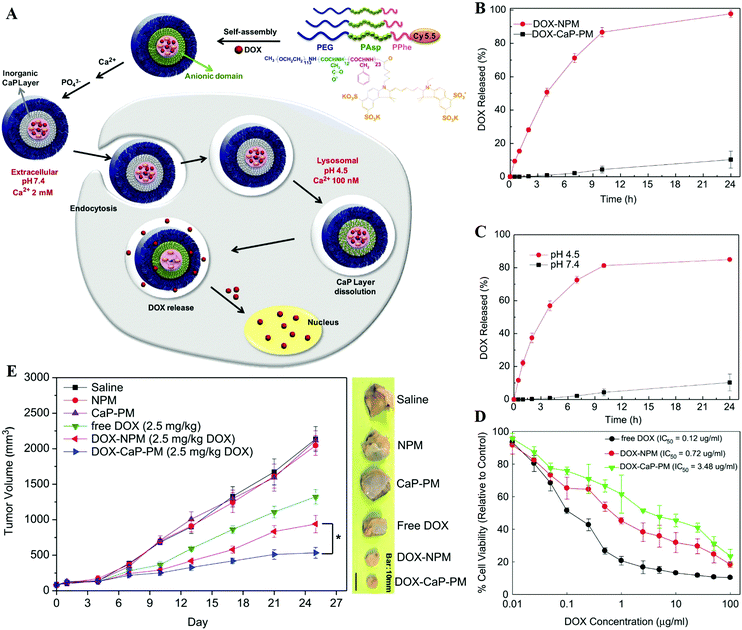 |
| Fig. 11 (A) Illustration of shell-specific CaP mineralization of DOX-loaded polymer micelles and triggered release of DOX by acidic intracellular compartments. (B) DOX release profiles from DOX-NPM and DOX-CaP-PM at pH 7.4 and (C) pH-controlled DOX release from DOX-CaP-PM. (D) In vitro cytotoxicity of DOX, DOX-NPM, and DOX-CaP-PM for MDA-MB231 cells after 24 h. (E) Changes in tumor volumes after the injection of saline, NPM, CaP-PM, free DOX, DOX-NPM (7.8 wt% DOX), and DOX-CaP-PM (7.8 wt% DOX), respectively. Reprinted with permission from ref. 98. Copyright 2014, Elsevier. | |
On the other hand, surface modification is an effective strategy to prepare the colloid stabilized CaPs nanocarriers. For example, chitosan-modified HAP NPs exhibited narrow size distribution, good hemocompatibility, high celecoxib encapsulation efficiency and a sustained release profile.99 The celecoxib-loaded NPs showed significant antiproliferation, apoptosis and time-dependent cytoplasmic uptake in colon cancer cells, and showed great inhibition of tumor growth in vivo. Luo and colleagues developed a water-dispersible DDS based on oleic acid-coated HAP NPs, using docetaxel (DTX) as a model drug.109 The drug encapsulation efficiency was 83.6% and the drug release could be sustained over 30 days. These nanocarriers exhibited obvious cytotoxicity, and the DTX-induced apoptosis was increased through activation of caspase-2 in androgen independent prostate cancer cells. In another case, Liang and co-workers prepared a core–shell nanostructure composed of HAP NPs as the core and alginate as the shell by a pre-gel method.102 This core–shell nanostructure exhibited excellent biocompatibility, superior drug loading capacity, and enhanced drug release efficacy. Moreover, the guest molecules such as organic dyes and therapeutic anticancer drugs could be loaded spatially into the core or shell layer, and the drug release kinetics could be controlled by acid-assisted dissolution.
For gene delivery, Mostaghaci et al. reported a reproducible, one-step procedure for the preparation of amino-modified CaP NPs by using N-(2-aminoethyl)-3-aminopropyltrimethoxysilane as the modifying and dispersing agent, and then loaded with DNA.238,244 The amino-modified CaP NPs with a narrow size distribution and positive charge at physiological pH showed low toxicity and reproducible transfection in A549 and HEK293 cells. Besides, Tan and colleagues utilized poly(L-arginine) and dextran as the polyelectrolytes to modify CaP NPs through a layer-by-layer (LbL) method. The obtained CaP NPs could act as siRNA delivery vehicles for SPARC (secreted protein, acidic, and rich in cysteine) silencing.242 Moreover, Frede and co-workers developed multi-shell NPs composed of a CaP core coated with siRNA, encapsulated into poly(D,L-lactide-co-glycolide acid) (PLGA), and modified with PEI as the final outer layer, for the local treatment of colonic inflammation. The multi-shell NPs could effectively deliver siRNA into the cytoplasm of epithelial cells and immune cells in vivo, thus inducing active gene silencing.202 More recently, inspired by the unique endocytosis pathway where Ras activation in cancer cells drives protein internalization via macropinocytosis, Huang and colleagues developed an efficient DDS to target the Ras-activated cancer cells via the macropinocytosis-mediated mechanism.253 They used apolipoprotein E3-reconstituted high-density lipoprotein to encapsulate the siRNA-loaded CaP core and facilitate it to penetrate the blood–brain barrier (BBB), which could induce cancer cells to ‘drink drugs’ for targeting activating transcription factor-5 (ATF5), an overexpressed anti-apoptotic transcription factor in glioblastoma, resulting in remarkable RNA-interfering efficiency, increased glioblastoma cell apoptosis and tumour cell growth inhibition both in vitro and in xenograft tumour models.
Furthermore, the Huang group developed lipid coated CaPs (LCP) nanocarriers for efficient drug/gene delivery owing to their versatility in encapsulating many types of therapeutic compounds.124–126,167–169,216,231,528 The LCP NPs could disassemble at low pH in the endosome, and cause endosome swelling and bursting to release the entrapped drug/gene.216 With the help of targeting ligands, such as anisamide231 and cRGD,241 the LCP NPs could effectively deliver drugs/genes to solid tumors with a good therapeutic effect. As shown in Fig. 12, the encapsulation of acyclovir monophosphate (ACVP) into LCP NPs (A-LCP, entrapment efficiency of ∼70%) (Fig. 12A and B) could turn the antiviral into an anticancer drug (Fig. 12C and D) by facilitating the targeted delivery of ACVP to the tumor, which resulted in excellent antitumor efficacy in vivo (Fig. 12E and F) due to their ability to induce dramatic apoptosis of tumor cells, as well as significantly inhibit tumor cell proliferation and cell cycle progression (Fig. 12G–I).124 Moreover, quercetin phosphate loaded LCP NPs significantly improved the bioavailability and metabolic stability of the parent quercetin, which could remodel the tumor microenvironment and increase the penetration of second-wave NPs into the tumor nests.127 After releasing from the LCP NPs, quercetin phosphate converted back to the parent quercetin and down-regulated Wnt16 expression, resulting in a synergistic antitumor effect with cisplatin NPs in a stroma-rich bladder carcinoma model. In addition, the encapsulation of a biologically active nucleotide analog (i.e. gemcitabine triphosphate) into LCP NPs could induce cell death and arrest the cell cycle in the S phase, as well as trigger the apoptosis of tumor cells and significantly reduce the tumor cell proliferation and the progress of cell cycle.125
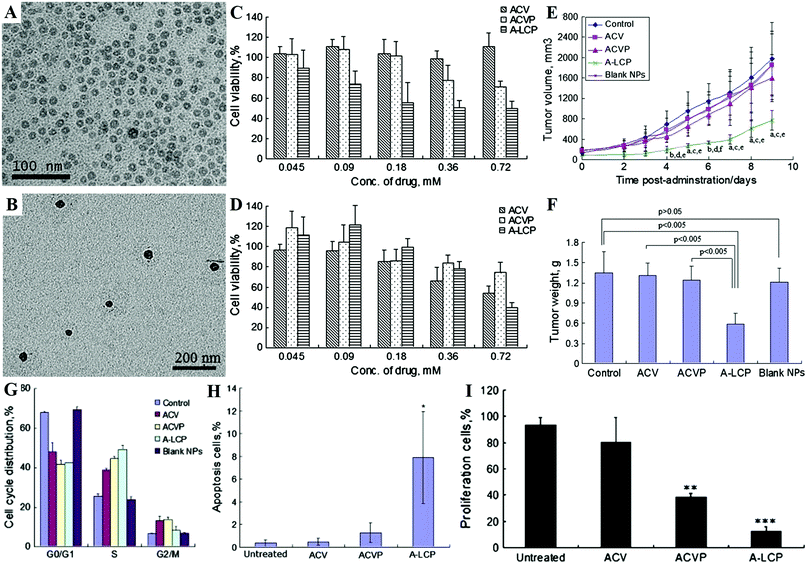 |
| Fig. 12 TEM images of the A-LCP core (A) and NPs (B). Cytotoxicity of ACV, ACVP and A-LCP NPs against HSV-TK− H460 cells (C) and HSV-TK+ H460 cells (D) in vitro assessed by MTT assay. (E) Tumor growth kinetics with dosing every other day for 5 times. aP < 0.05 and bP < 0.01 vs. the control, cP < 0.05 and dP < 0.01 vs. free ACV, and eP < 0.05 and fP < 0.005 vs. free ACVP. (F) Tumor weights of tumor-bearing mice after the treatment. (G) Cell cycle distribution of H460 cells treated with ACV, ACVP, A-LCP NPs and blank LCP NPs for 48 h. Quantification of in vivo tumor cell apoptosis (H) and tumor cell proliferation (I) induced by A-LCP NPs from H460 tumor-bearing nude mice after a schedule of 3 doses. Reprinted with permission from ref. 124. Copyright 2013, Elsevier. | |
The co-delivery of a chemotherapeutic agent (such as gemcitabine monophosphate) and an oncogene-modulating siRNA by LCP NPs could combine chemotherapy and gene therapy to induce cell apoptosis and reduce their proliferation, leading to dramatic tumor growth inhibition with negligible in vivo toxicity.126 In addition, the Trp2 peptide and CpG adjuvant co-loaded and mannose-modified LCP NPs can induce potent cytotoxic T-lymphocyte response, and follow by superior inhibition of tumor growth in both melanoma and its lung metastasis.167 However, due to the immunosuppressive microenvironment of tumors, the LCP-based Trp2 vaccine is less effective against later stage B16F10 melanoma in a subcutaneous syngeneic model. To improve the LCP-based Trp2 vaccine activity and modulate the tumor microenvironment, the transforming growth factor-β siRNA168 and CDDO-Me (a broad spectrum inhibitor)169 are loaded by liposome–protamine–HA NPs and poly-lacticglycolic-acid NPs, respectively, which exhibit an increase of antitumor efficacy and apoptotic tumor tissue than LCP-based Trp2 vaccine alone in B16F10 melanoma.
Importantly, LCP NPs with different surface modifications such as the density of PEG and lipids show different in vivo behaviors after intravenous injection.528 The location of LCP NPs in hepatocytes or Kupffer cells is dependent on the density of PEG and the surface lipids. LCP NPs can be transferred from hepatocytes to Kupffer cells by the decrease of surface PEG density per NP. In another case, 1,2-dioleoyl-3-trimethylammonium-propane (DOTAP) modified LCP NPs exhibited higher accumulation in the hepatocytes compared to LCP NPs with dioleoylphosphatidylcholine (DOPC) modification. Moreover, the apolipoprotein E (apoE) may serve as an endogenous targeting ligand for LCP-DOTAP NPs, but not for LCP-DOPC NPs. Therefore, the surface modifications of NPs directly decide their location and fate in vivo.
CaPs as the coating layer or gate-keeper.
CaPs coatings on the surface of NPs could dramatically enhance their stability, biocompatibility and drug/gene loading capacity, and endow them with a facility for surface modification and pH-dependent controllable drug release properties.91–95 The CaPs coated polymer micelles have the following advantages: (i) good stability against dilution and micelle-disrupting chemicals, and (ii) controllable drug release to avoid the premature leakage of drugs.108,120 For example, Choi et al. reported a versatile RNAi nanoplatform that was engineered by combination of an artificial RNA receptor (Zn(II)–DPA) with tumor-targetable and drug-loadable HA NPs, and then modified it with a CaP coating by in situ mineralization (Fig. 13A and B).239 This nanoplatform could effectively encapsulate small-molecule drugs within its hydrophobic inner core and strongly secure various RNA molecules (e.g. siRNAs, miRNAs, and oligonucleotides) by utilizing Zn(II)–DPA and a robust CaP coating (Fig. 13C–F). Their results suggested that this gene/drug delivery system promises to be a highly effective nonviral way to deliver chemo- and RNAi therapeutics into host cells (Fig. 13G–K). Moreover, Murphy and colleagues reported that CaP mineral-coated poly(lactide-co-glycolide) (PLG) microspheres could serve as effective carriers for protein binding and sustained release.190 More importantly, proteins bound within the nanostructured CaP coatings could enhance their stability against extreme external stressors and maintain their bioactivity. The protein stabilization effect could be attributed to the maintenance of protein conformational structure, which was related to the nanosized features of CaP coatings.191
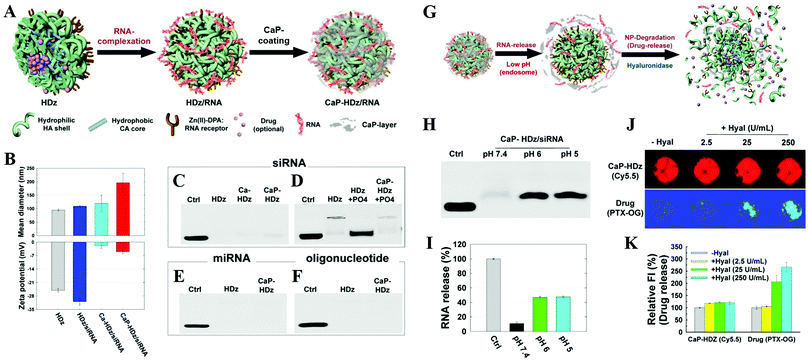 |
| Fig. 13 Preparation of the versatile RNAi nanoplatform. (A) The nanoplatform can strongly secure RNA molecules (and small-molecule lipophilic drugs if needed) using artificial RNA receptors (Zn/DPA) as well as a CaP-layer. (B) Mean diameter and zeta-potential results of HDz-NP, HDz/siRNA-NF, Ca-HDz/siRNA-NF, and CaP-HDz/siRNA NF. Electrophoretic retardation analysis of siRNA (C and D), miRNA (E), and oligonucleotide (F) (all at 10 pmol) binding with HDz and CaP-HDz-NPs (all at 2 μg of HDz-NP). (G) RNA release and degradation mechanism of CaP-HDz/siRNA-NF. (H and I) siRNAs complexed with CaP-HDz-NFs can be firmly secured at neutral pH (pH 7.4) but are readily released from CaP-HDz-NPs in endosomal/lysosomal pH conditions (pH 6 and 5). (J and K) Monitoring of enzyme-triggered NF degradation and drug release from NFs incorporated with both lipophilic drug, paclitaxel (PTX), and siRNA. Reprinted with permission from ref. 239. Copyright 2014, American Chemical Society. | |
Additionally, the CaPs coating layer can be used as the drug/gene depot, and different drugs can be spatially loaded in different regions. For instance, Li et al. synthesized amphiphilic gelatin@CaPs NPs for the co-delivery of two drug molecules (hydrophilic drug (DOX) and hydrophobic drug (camptothecin, CPT)), which could avoid the interaction within different drugs.138 More importantly, the degradable amphiphilic gelatin core and pH-sensitive CaP shell promised the release of CPT and DOX progressively. Due to the synergistic cell cycle arrest/apoptosis-inducing effect between CPT and DOX, the co-delivery system exhibited better antitumor efficacy. In another case, Li and colleagues developed a multifunctional nanoplatform by the combination of herceptin–gelatin conjugates, iron oxide, and CaP NPs, which could encapsulate the hydrophobic curcumin (CUR) and hydrophilic DOX in the gelatin core and the CaP shell, respectively.139 This multifunctional nanoplatform not only provided a dual-targeting functionality with a bioligand and magnetic targeting resulted in significantly elevated cellular uptake, but also co-loaded CUR and DOX as a dual-DDS for the combination therapy.
Besides NPs, viruses can be coated by the CaPs layer to overcome their inherent limitations and open their potential therapeutic applications. For example, Wang and colleagues modified viruses with a CaP shell by in situ biomineralization to fabricate the artificial viral core–shell hybrid NPs for virus reprocessing and protection.422 The CaP-coated viruses could stealthily bypass the receptor barriers with enhanced infectivity and expanded tropism, which could circumvent neutralizing antibodies and ensure systemic administration of viruses under the preimmunized conditions at relatively low dosage. In another case, as shown in Fig. 14A and B, Chen and co-workers explored a PEG-LCP-oncolytic adenovirus (PLC-OncoAd) delivery system based on PEG-modified LCP NP coated oncolytic adenovirus (ZD55-IL-24), which could shield OncoAd from sequestration in the liver, prolong its circulation time, reduce its systemic toxicity, and protect it from neutralization by pre-existing neutralizing antibodies and evade the innate immune response (Fig. 14C and D).423 After systemic administration, the PLC-OncoAd delivery system induced negligible hepatic toxicity and innate immune response, and exhibited an efficient tumor targeting ability and antitumor effect (Fig. 14E). By increasing the dose of PLC-OncoAd, the therapeutic efficacy and prognosis were improved. Hence, the PLC-OncoAd delivery system represented a promising improvement for oncolytic adenovirus-based cancer gene therapy in vivo.
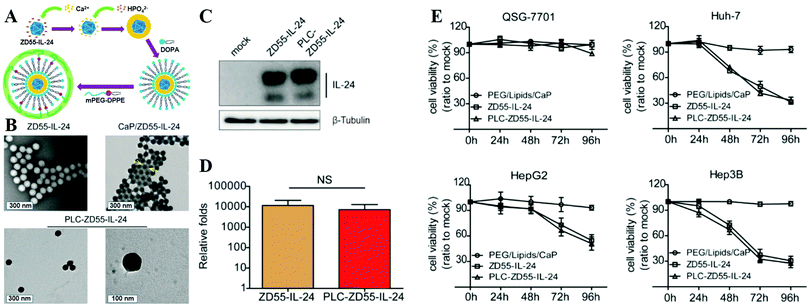 |
| Fig. 14 (A) Synthetic route used to prepare PLC-ZD55-IL-24. (B) TEM image of naked ZD55-IL-24, CaP/ZD55-IL-24 coprecipitation, and PLC-ZD55-IL-24. The arrows indicate the CaP shell. (C) Western blot analysis of IL-24 expression in Huh-7 cells. (D) Viral proliferation assessment in Huh-7 cells. (E) Cell viability was determined using the MTT assay. HCC cell lines (Huh-7, Hep3B, and HepG2) and the normal human liver cell line (QSG-7701) were treated with PEG/lipids/CaP, ZD55-IL-24 and PLC-ZD55-IL-24 at 160 VPs per cell. Reprinted with permission from ref. 423. Copyright 2016, American Chemical Society. | |
It is well known that CaPs are featured with pH sensitive dissolution behavior, so they are widely employed as a pH-responsive gate-keeper, which not only protects payloads from nonspecific triggers but also induces on-demand release of them. The “zero premature release” of the CaPs-based gate-keeper possesses great clinical significance in delivering cytotoxic drugs, which can significantly reduce the systemic toxicity and bring about effective anticancer treatment. For example, Banerjee et al. reported CaP nanocapsule crowned multi-walled carbon nanotubes (MWCNTs) for intracellular DOX delivery, which could efficiently prevent untimely drug release at physiological pH, but promote drug release at acidic milieu as observed in subcellular compartments such as lysosomes (∼5.0) upon pH triggered CaP dissolution.134
Besides MWCNTs, the pores of mesoporous silica NPs can also be blocked by surface mineralization of CaPs for controlled drug release.128,144 For instance, Rim et al. developed mesoporous silica nanocontainers covered with pH-controlled and absorbable CaP nanocoatings (Fig. 15A–C) that allow the controlled release of entrapped drugs within acidifying intracellular compartments such as endosomes and lysosomes.113 The CaP coatings could hold the encapsulated anticancer drugs under extracellular conditions, whereas they could be dissolved into nontoxic ions within intracellular endosomes to initiate drug release within tumor cells (Fig. 15D–G). Similarly, Chen and co-workers constructed CaP/HA hybrid capped mesoporous silica nanocontainers, which could control the release of loaded anticancer drugs in acidic subcellular environments after receptor mediated endocytosis.96 The presence of another layer of HA on the CaP surfaces could improve the stabilization of the nanocomposites and confer the target ability toward CD44 overexpressed cancer cells, which significantly enhanced the cellular uptake. Recently, Choi et al. reported a light-responsive gate-keeper system that was composed of a light-activated pH-jump reagent as an intermediary and a CaP coating as a shielding layer for spatiotemporal-controlled nitric oxide (NO) delivery (Fig. 16A–C).424 The CaP coating not only acted as a shielding barrier for diazeniumdiolate but also imparted pH responsiveness to the NO donor. When exposing the NO donor to physiological conditions, the acids generated from the light-activated pH-jump agent could accelerate the degradation of the CaP coating layers, resulting in NO release from diazeniumdiolate (Fig. 16D and E).
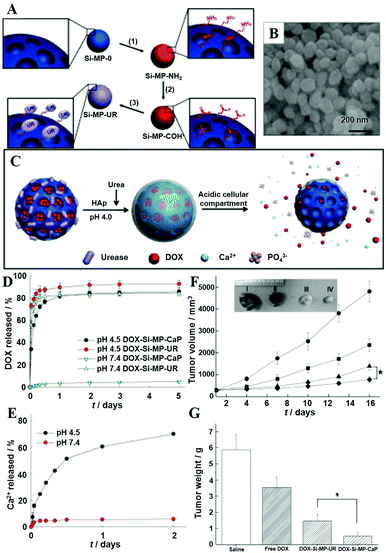 |
| Fig. 15 (A) Synthetic route to mesoporous silica NPs-urease (Si-MP-UR): (1) 3-aminopropyltriethoxysilane (APTES); (2) removal of cetyltrimethylammonium bromide, glutaraldehyde; (3) Jack bean urease (UR). (B) Field-emission scanning electron microscopy image of Si-MP-UR. (C) Schematic of surface CaP mineralization of DOX-loaded Si-MP-UR and triggered drug release under intracellular endo/lysosomal conditions. (D) DOX release profiles from DOX-Si-MP-UR and DOX-Si-MP-CaP under pH control. (E) Kinetics of calcium dissolution from DOX-Si-MP-CaP under pH control. (F) In vivo therapeutic efficacy after a single intratumoral injection of saline (●), free DOX (■), DOX-Si-MP-UR (▲), and DOX-SiMP-CaP (◆) at a DOX-equivalent dose of 10 mg kg−1. Inset: Images of excised tumors after 16 days post-treatment. I: saline, II: free DOX, III: DOX-Si-MP-UR, IV: DOX-Si-MP-CaP. (G) Tumor weights after 16 days post-treatment. The results represent the means ± SDs (n = 4); *P < 0.05. Reprinted with permission from ref. 113. Copyright 2011, Wiley-VCH. | |
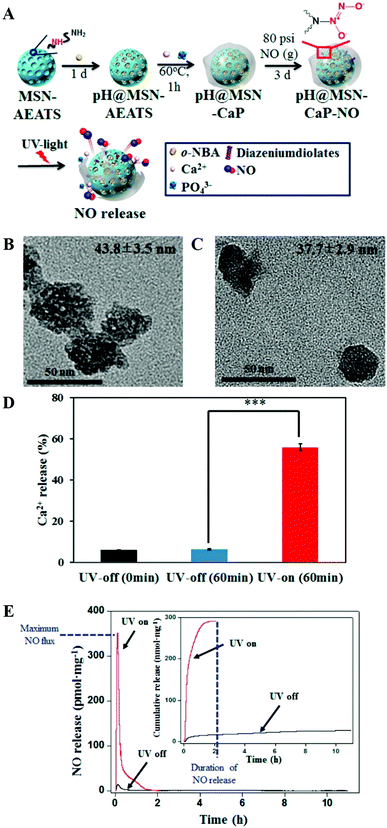 |
| Fig. 16 (A) Scheme for the preparation of the pH@MSN-CaP-NO gate-keeper. (B) TEM image of pH@MSN-CaP-NO without light irradiation. (C) TEM image of pH@MSN-CaP-NO after 1 h of light irradiation. (D) Quantification of calcium dissolution from pH@MSN-CaP-NO before and after UV irradiation. (E) Real-time NO release profile of pH@MSN-CaPNO after light irradiation (red) and in the dark (black). The inset represents the cumulative NO release. Reprinted with permission from ref. 424. Copyright 2016, American Chemical Society. | |
In situ drug/gene loading of CaPs.
Thanks to the mild preparation conditions of CaPs, various guest molecules can be in situ loaded into CaPs nanocarriers during their synthesis process, which not only increases the loading capacity but also cuts down on the loading process.80,132,220–225 Moreover, the in situ drug loading strategy can incorporate drug molecules into the bulk of nanocarriers, which could avoid the quick release of drugs and protect drug molecules (especially proteins and genes) from the destruction of enzymes in the organisms. Meanwhile, the guest molecules such as proteins and genes can act as stabilizers.106,211 For example, Kim and colleagues used CaPs microspheres to load alendronate in situ by an emulsification (water-in-oil) method.79 In this case, the nucleation/growth of CaPs occurred within the water droplets, which was initiated by a urea-mediated solution precipitation technique. Besides, Tang et al. reported that the in situ loading of drugs in the formation of CaPs nanocarriers could achieve ultrahigh drug-loading capacity and prolonged drug release.114 Similarly, Olton and co-workers reported the synthesis of mono-dispersed CaP–pDNA NPs in situ by mixing the Ca and phosphate precursors in a controlled and regulated manner.213 The mono-dispersed CaP–pDNA NPs had higher transfection efficiencies because these NPs not only effectively condensed (70% efficient) but also efficiently bound (90% efficient) the pDNA. Interestingly, the cellular uptake of CaPs–pDNA NPs in HeLa and COS-7 cells was mediated by both clathrin- and caveolae-dependent endocytosis, and the caveolae mechanism was the major contributor.227
Cao et al. reported a non-viral vector based on CaP NPs for the generation of virus-free induced pluripotent stem cells (iPSCs) from human umbilical cord mesenchymal stem cells (HUMSCs) via co-delivery of four plasmids (Oct4, Sox2, Klf4, and c-Myc).233 The co-delivery of four Yamanaka factors via in situ plasmid-encapsulated CaP NPs provided a simple, safe, and efficient method for the generation of virus-free iPSCs, which showed great potential in the field of regenerative medicine. As shown in Fig. 17, Zhang and colleagues conjugated PEG with siRNA via a disulfide linkage to form PEG-SS-siRNA, which was subsequently utilized as the template for the mineralization of CaP to obtain nanosized hybrid micelles.215 In this system, a larger amount of siRNA was complexed with CaP as the hybrid inner core, and surrounded by a dense PEG shell. The as-prepared micelles exhibited enhanced siRNA incorporation efficiency and gene-silencing efficacy, prompt responsivity toward the intracellular reducing environment, and prolonged stability in the serum-containing medium.
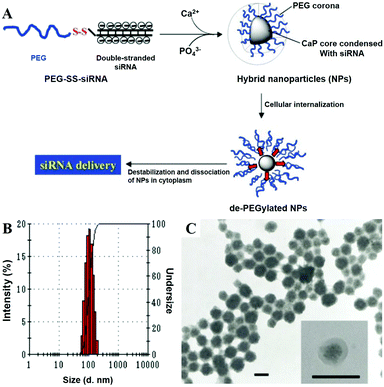 |
| Fig. 17 (A) Schematic demonstration of the PEG-SS-siRNA/CaP nanoparticle and its morphological investigations by (B) DLS and by (C) TEM (scale bar: 100 nm). Reprinted with permission from ref. 215. Copyright 2009, Wiley-VCH. | |
Besides drugs and genes, proteins can also be loaded in situ by CaPs. Liu et al. comparatively studied the bovine serum albumin (BSA)-loaded CaP nanocarriers for controlled protein delivery via both in situ and ex situ processes.160 The amount of loaded BSA decreased with increasing pH but a larger amount was observed in the ex situ compared to the in situ process above pH = 8.0. More importantly, bioactive polypeptide drugs can solidify to form uniform NPs via biomimetic mineralization. As an example, Chen and co-workers reported a potent platform for exendin-4 delivery through an in situ biomimetic mineralization strategy.192 The exendin-4 was mineralized to form nanosized minerals by the reaction between its acidic amino acid residues and Ca2+ ions in a supersaturated solution with a negligible influence on peptide bioactivity. Mineralized exendin-4 particles were spontaneously absorbed by a living body under physiologically supersaturated conditions, resulting in gradual dissociation and sustained drug release. In this case, the glucose level of type 2 diabetic mice was effectively controlled for a long period of time by mineralized exendin-4 without obvious side effects. Therefore, in situ loading provides a simpler preparation process for CaPs-based drug/gene/protein delivery systems.
CaPs-Based targeted drug/gene delivery.
NPs functionalized with tumor specific targeting moieties are promising strategies for active drug/gene delivery in cancer targeting therapy. By combination with various targeting ligands such as folic acid (FA), HA and antibodies, nanostructured CaPs are widely used for efficient targeted drug/gene delivery of tumours.165,207 For instance, Iafisco et al. functionalized CaP NPs with a DO-24 monoclonal antibody (mAb) against the Met/Hepatocyte Growth Factor receptor (Met/HGFR) which was over-expressed on different types of carcinomas.100 These CaP–mAb NPs could specifically bind to the cells that expressed the receptor of GTL-16. Additionally, Hu et al. reported membrane-core NPs that were composed of CaP cores, arginine-rich peptides, cationic and PEGylated lipid membranes, and galactose targeting ligands for efficient nuclear delivery of plasmid DNA (Fig. 18A–C).209 These membrane-core NPs exhibited rapid and extensive hepatic accumulation and were predominantly internalized by hepatocytes, while the inclusion of such peptides in NPs was sufficient to elicit high degrees of nuclear translocation of plasmid DNA (Fig. 18D–F).
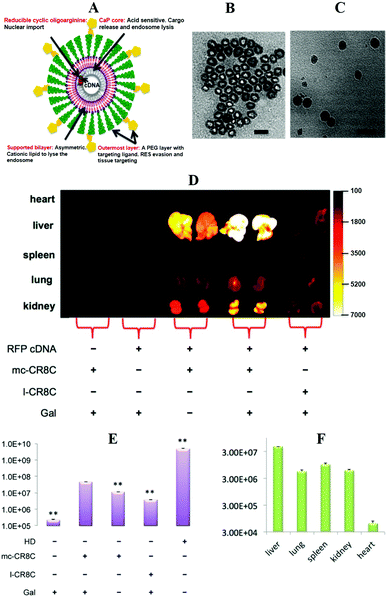 |
| Fig. 18 (A) Representative multifunctional features of membrane-core LCPs consisting of CaP cores encapsulating DNA and octaarginine peptides, and an asymmetric lipid membrane functionalized with galactose ligands on a stealthy PEG layer. (B) TEM image of LCP cores loaded with DNA and octaarginine peptides (scale bar: 50 nm). (C) TEM image of final LCPs, stained with uranyl acetate (scale bar: 100 nm). (D–F) Transgene expression in the major organs of C57BL/6 mice 24 h after intravenous injection of plasmids formulated in different groups of LCP (plasmid dose: 0.3 mg kg−1). (D) Comparison of expressed RFP fluorescence, as determined with a Kodak IVIS system (ex/em: 550/600 nm). Control DNA was a luciferase plasmid. (E) Comparison of expressed luciferase in the liver. All are compared with the group of Gal-targeted LCP containing mc-CR8C. (F) Comparison of expressed luciferase in various major organs after the administration of plasmids formulated in the Gal-targeted LCP containing mc-CR8C. Reprinted with permission from ref. 209. Copyright 2013, American Chemical Society. | |
HA, as an active tumor-targeting ligand that binds CD44 receptors, could confer CaPs nanocarriers with targeting ability. For example, Xiong and colleagues reported the HA modified HAP NPs (Fig. 19A) for efficient DOX delivery into both mitochondria and nuclei (Fig. 19B).101 The DOX-loaded HAP-HA NPs (DOX/HAP-HA) exhibited high drug loading efficiency (214.55 ± 51.05 μg mg−1) and pH-responsive drug release (Fig. 19C). The mitochondrial and nuclei dual-targeted system could markedly enhance mitochondrial cytochrome C leakage and activate the intracellular apoptotic cascade, thereby potentiating the antineoplastic effect (Fig. 19D and E). Excitingly, the in vivo anti-tumor effect on mice bearing Heps xenografts confirmed the tumor-targeting capacity and enhanced antitumor efficacy (Fig. 19F–H) of DOX/HAP-HA. In another case, Lee and colleagues prepared 3,4-dihydroxy-L-phenylalanine (DOPA) conjugated HA stabilized CaP NPs (CaP/siRNA/DOPA-HA) for targeted siRNA delivery of tumors.206 The superior ability to maintain the integrity of encapsulated siRNA and the solution stability of NPs allowed this formulation to achieve improved tumor accumulation of siRNA and a high level of target gene silencing in solid tumors. Considering its biocompatibility, transfection efficacy, and tumor targeting capability, this CaP/siRNA/DOPA-HA-based gene delivery platform shows great potential for systemic siRNA delivery and targeted cancer therapy. Recently, Qiu and co-workers developed HA-functionalized CaP NPs with a uniform spherical core–shell morphology for an injectable and targetable delivery of siRNA.208 The coating of hydrophilic HA improved the physical stability of NPs over one month, and effectively delivered EGFR-targeted siRNA into A549 cells through CD44-mediated endocytosis and significantly down-regulated the level of EGFR expression, which resulted in significant tumor growth inhibition.
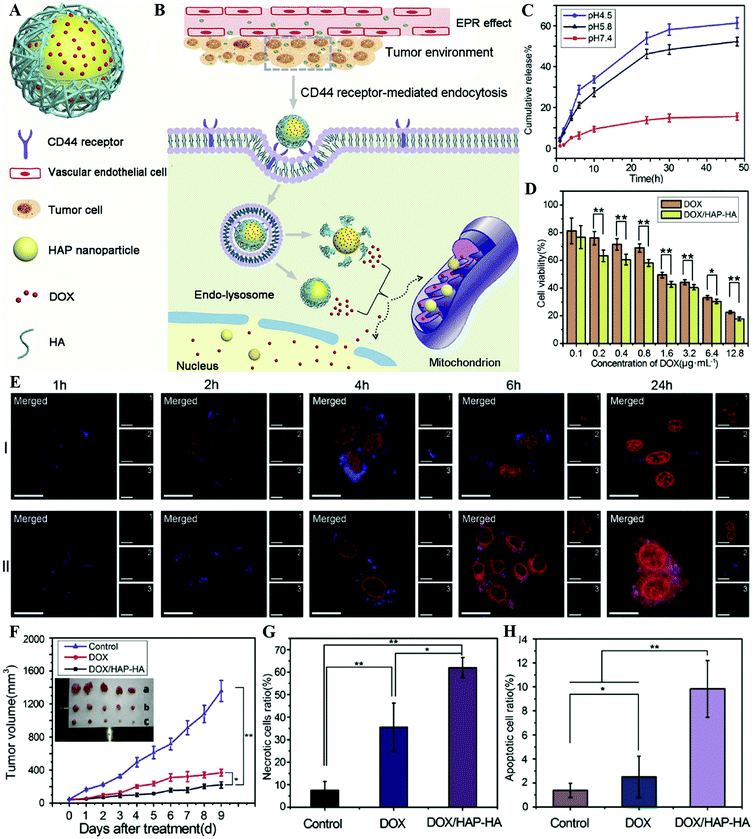 |
| Fig. 19 (A) The main components of DOX/HAP-HA NPs: DOX, HAP NPs and HA shell. (B) The antitumor mechanism of DOX/HAP-HA NPs, i.e. drug delivery into the cell nuclei and mitochondria. (C) In vitro release of DOX/HAP-HA NPs at different pH. (D) In vitro cytotoxicity of DOX/HAP-HA NPs in HepG2 cells for 48 h. (E) Intracellular distribution of DOX/HAP-HA in HepG2 cells at different times observed by CLSM. Cells were incubated with (I) free DOX and (II) DOX/HAP-HA NPs. For each panel, 1: DOX (red); 2: mitochondria stained by James Green B (blue); 3: cells under light field; merged: overlay of 1, 2 and 3. The scale bar is 20 mm. (F) The Heps tumor growth curves after intravenous injection of different formulations of DOX at a dose of 5 mg kg−1. The percentage of necrotic cells (G) and apoptotic cells (H) after treatment. Reprinted with permission from ref. 101. Copyright 2016, Elsevier. | |
Besides targeting moieties, magnetic CaPs nanocarriers have the function of magnetic targeting drug delivery and enhanced gene transfection.95 For example, Wu et al. prepared magnetic CaP NPs by treating CaP with Fe ions through a wet-chemical process, in which the hetero-epitaxial growth of magnetite was found.218 The magnetic CaP NPs displayed a high binding affinity of plasmid DNA and enhanced the transfection efficiency of rat marrow-derived mesenchymal stem cells with the gene for glial cell line-derived neurotrophic factor. By using an external magnet, the magnetic CaP NPs could target the selected site through magnetic targeting and the in vivo transfection could be realized.
5.1.2 CaCs-Based drug/gene delivery system.
Pure CaCs nanocarriers.
Nanostructured CaCs are useful nanocarriers for drug/protein/gene delivery owing to their pH-sensitivity, outstanding biocompatibility and biodegradability, low cost, and easiness of large-scale production.529 CaCs nanocarriers could incorporate guest molecules into the solid NPs with chemical stability. For example, granulocyte colony-stimulating factor or betamethasone phosphate incorporated CaCs are chemically stable and release slowly, which exhibit a smaller initial increase in plasma concentration and a subsequent sustained release compared with free drug solution.345 Interestingly, Zhang and colleagues reported vaterite CaC nanoplate assemblies with an exposed high-energy {001} facet, which showed a maximum DOX-loading capacity up to 65% and a high encapsulation efficiency of around 80%.333 Along with its sustainable release performance and the inhibition of initial burst release, this DDS had a long-term chemotherapeutic efficacy. This work highlighted the crystal surface structure (such as high-energy facets) and chemistry at the atomic level for the rational design of a DDS with high drug-loading capacity and sustainable-release property.
Hollow or hierarchical CaCs nanocarriers.
The hollow or hierarchical structure of CaCs, due to high specific surface area and high drug loading capacity, makes them more suitable for drug delivery. As an example, Wei et al. proposed a facile method to prepare self-organized hollow spheres of CaCs nanocrystallites (Fig. 20A–C), and used them as an anticancer drug delivery vehicle for DOX (Fig. 20D–H).344 The DOX-loaded CaCs hollow spheres exhibited the following advantages: localized drug release by the pH-sensitive structure, enhanced cytotoxicity by increasing cellular uptake, perinuclear accumulation, and nuclear entry (Fig. 20I–K). In another case, Ma and co-workers reported a facile approach to synthesize hierarchical porous CaC multi-shelled hollow nanospheres with controllable shell numbers using LbL thermal decomposition of organic acid salts and templates, which possessed high drug loading capacity and a sustained drug release profile.339
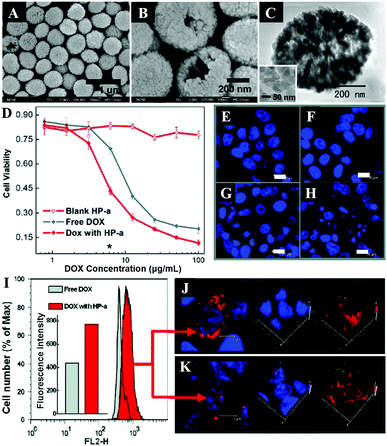 |
| Fig. 20 SEM images showing (A) the uniform particle size of the spheres and (B) some defects on the surface indicating the hollow structure of the CaCs spheres (HP-a). (C) TEM image of a single sphere with low contrast at the center with the inset showing the high-resolution TEM image of the primary building units. (D) Cytotoxicity of HepG2 cells exposed to free DOX, blank HP-a, and DOX loaded HP-a for 48 h. The right panel shows the nuclei of original HepG2 cells (E) and the HepG2 cells incubated with blank HP-a (F), free DOX (G), and DOX loaded HP-a (H) after 48 h. The concentrations of additives corresponded to the star-marked point in (D) (scale bar: 10 μm). (I) HepG2 cell uptake of free DOX and DOX loaded HP-a. Perinuclear accumulation (J) and nuclear location (K) of DOX loaded HP-a (red dot). The experiments were performed at 6 h after incubating HepG2 cells with DOX or DOX loaded HP-a, at the concentration corresponding to the star-marked point in (D) (scale bar: 5 μm). Reprinted with permission from ref. 344. Copyright 2008, American Chemical Society. | |
Compared to the crystallized CaCs, nanoscaled ACC NPs can be readily obtained. However, the biomedical applications of ACC NPs are partly limited because of their high aqueous instability. To overcome this challenge, Zhao et al. developed a novel nanoreactor based on hierarchical ACC–DOX@silica NPs.342 As shown in Fig. 21A–D, the nanoreactor was made up of a preloaded ACC–DOX core and a silica shell. In this nanoreactor, the acidic stimulus, controlled by the silica shell, could trigger the inner reactions in the ACC–DOX core. After dissociating from the core, the protonated DOX molecule would adhere to the negatively charged silica shell through a pH-dependent electrostatic attraction instead of being released directly. Thus, this DDS maintained a low drug leakage in physiological and lysosomal/endosomal environments, and responded specifically to the weakly acidic (pH 6.5) microenvironment of cancer cells, resulting in efficient cell death (Fig. 21E–H).
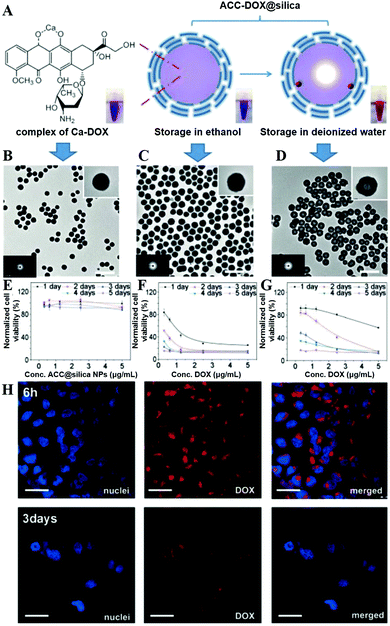 |
| Fig. 21 (A) Schematic representation of the ACC–DOX@silica nanoreactor and its stability in ethanol and water. Inset images (from left to right) are the suspensions of ACC–DOX in ethanol, ACC–DOX@silica in ethanol, and ACC–DOX@silica in water. Representative TEM images of (B) ACC–DOX nanoparticles in ethanol, (C) ACC–DOX@silica in ethanol, and (D) ACC–DOX@silica in water. The scale bars are 500 nm. The inset images in (B–D) are the enlarged TEM images and electron diffraction images. (E–G) Cell viability of HeLa cells cocultured with (E) ACC@silica nanoparticles, (F) DOX, and (G) ACC–DOX@silica nanoparticles after various periods of 1–5 days. The samples were all at pH 7.4. (H) Confocal laser scanning microscopy images of HeLa cells cocultured with ACC–DOX@silica nanoparticles after 6 h and 3 days. The images in the left column are the nuclei stained with Hoechst 33258 (blue), the images in the middle column are with DOX (red), and the images in the right column are merged images of the left and middle columns. The scale bars are 50 mm. Reprinted with permission from ref. 342. Copyright 2015, Wiley-VCH. | |
Organic–inorganic hybrid CaCs nanocarriers.
Another strategy to improve the stability of ACC in physiological environments is by adding biocompatible organic molecules as the stabilizer. The CaCs-based organic–inorganic hybrid NPs usually have a small and uniform size, a unique structure and high biocompatibility, which make them very suitable for drug/protein delivery. In our previous study, the highly stable ACC/ATP composite nanospheres were obtained by using ATP as stabilizer, in which ATP played a unique role in the stabilization of ACC nanospheres in aqueous solution.363 The obtained ACC/ATP nanospheres were highly stable in phosphate buffered saline for a relatively long period of time (12 days), and exhibited a high hemoglobin (Hb) adsorption capacity that reached 858 mg g−1 at a Hb concentration of 3.0 mg mL−1. In another case, Tewes et al. reported inhalable composite microparticles that were made up of ACC and hyaluronan for peptide and protein pulmonary aerosol delivery, which not only preserved peptide and protein activities but also improved their bioavailability.367
In order to increase the drug loading capacity and realize controlled drug release behavior, the surface of CaCs-based DDSs is often modified by polyelectrolytes to form organic–inorganic hybrid nanocarriers via the LbL self-assembly approach.316 After surface modification, the cellular internalization of CaCs-based DDSs obviously improved.340 With the help of targeting ligands, CaCs-based targeted DDSs are also explored. For instance, various targeting ligands including FA, aptamer, anisamide, chondroitin sulfate, and tumor necrosis factor-related apoptosis inducing ligand (TRAIL) have been conjugated with CaCs-based nanomaterials to construct targeted DDSs.320–323,343
In situ payload loading of CaCs.
CaCs nanomaterials can be easily obtained by mineralizing inorganic Ca salt with carbonate under environmentally benign conditions. During the mineralization process, guest molecules such as drugs, proteins, enzymes or genes could be encapsulated by an in situ way.348,359 Compared with traditional encapsulation strategies, this in situ payload loading can avoid the post-treatments and multistep processes. For example, Magnabosco et al. demonstrated that calcite single crystals were able, during their growth in the presence of DOX, to entrap drug molecules inside their lattice along specific crystallographic directions rather than simply adsorbing on their surface.330
CaCs nanocarriers for gene delivery.
CaCs-Based gene therapy is a promising strategy in cancer therapy. For instance, Sun et al. examined gene transfer in nine cell types by using surface-induced mineralization and DNA-doped CaCs nanocomposites.348 These nanocomposites possessed great potential for customizing gene transfer in realizing gene- and cell-based therapy and probing functions of genes. In addition, Wei et al. functionalized ACC hybrid nanospheres with the Ca(II)-IP6 compound (CaIP6) for efficient delivery of siRNA targeting AIB1 or AKT1 of human breast cancers, which resulted in cell proliferation inhibition, apoptosis induction and cell cycle arrest as well as tumor growth attenuation.349,357
5.1.3 CaSi-Based drug/gene delivery systems.
Nanostructured CaSi biomaterials have high biocompatibility and biodegradability, high specific surface area, mesoporous/hollow structure, high drug-loading capacity and pH-responsive release, which make them appealing as drug delivery carriers.9,10 As shown in Fig. 22A–D, Wu and colleagues reported a low-cost, surfactant-free sonochemical synthesis of hierarchically nanostructured CaSi mesoporous microspheres (HNMS-CSH) with a large specific surface area (290 m2 g−1) and pore volume (1.20 cm3 g−1).379 The prepared HNMS-CSH has an ultra-high drug-loading capacity (2.29 g of ibuprofen (IBU) per gram carrier) (Fig. 22E). In this system, the cumulative amount of released IBU showed a linear relationship with the natural logarithm of release time (Fig. 22F and G). Afterwards, they prepared CaSi ultrathin nanosheets with a large specific surface area (505 m2 g−1) and demonstrated their high protein (Hb, 878 mg g−1) and drug (IBU, 2.2 g g−1) adsorption capacities via a reaction-rate controlled precipitation process.380 By using the block copolymer monomethoxy(polyethyleneglycol)-block-poly(lactide-co-glycolide) as the template, they prepared amorphous CaSi/block copolymer hybrid NPs with a high drug loading capacity (IBU, 1.9 g g−1; DTX, 82 mg g−1; Hb, 995 mg g−1) and pH-responsive drug release performance.381
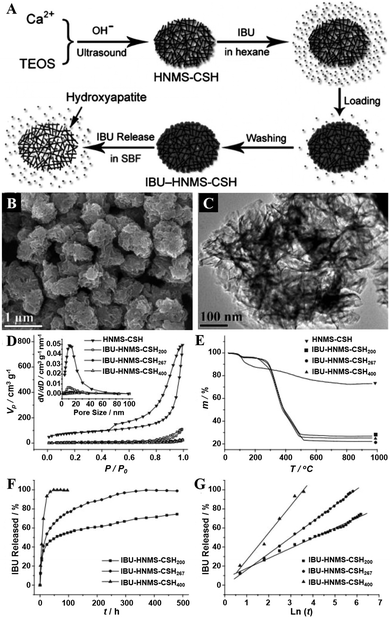 |
| Fig. 22 Illustration of the strategy (A) for the preparation and drug-delivery study of HNMS-CSH. SEM (B) and TEM (C) images of HNMS-CSH. (D) N2-Adsorption–desorption isotherm and BJH-desorption pore-size-distribution curves of HNMS-CSH and IBU–HNMS-CSHn. (E) TG curves of HNMS-CSH and IBU–HNMS-CSHn. (F) IBU-release profiles of the IBU–HNMS-CSHn drug-delivery systems in SBF. (G) The cumulative IBU-released-percentage versus natural-logarithm-of-time plots for IBU–HNMS-CSHn systems (n = 200, 267, and 400). Reprinted with permission from ref. 379. Copyright 2010, Wiley-VCH. | |
To investigate the interaction of CaSi nanocarriers and loaded drugs, Guo et al. used X-ray absorption near edge structure spectroscopy to investigate the interaction between drug molecules and CaSi nanocarriers.530,531 Their results clearly indicated that acidic functional groups of drug molecules linked to active sites (Ca–OH and Si–OH groups) on the surface of CaSi nanocarriers by electrostatic interactions. By using scanning transmission X-ray microscopy, they mapped an individual mesoporous CaSi microsphere before and after the loading of IBU, which could determine the absolute thickness of IBU and reveal drug distribution in the CaSi microsphere.532
Additionally, CaSi can be coated on the surface of other inorganic materials to form core–shell nanostructures. For instance, Kang et al. reported a single-step procedure to simultaneously load and protect high concentrations of siRNA in porous silicon NPs (pSiNPs) by precipitating a CaSi shell simultaneously with drug loading (Fig. 23A).385 The CaSi shell was readily modified with cell targeting (RVG peptide from rabies virus glycoprotein) and cell-penetrating (myristoylated transportan) peptides. This pSiNP-based siRNA delivery system exhibited excellent cellular targeting and in vitro gene knockdown, as well as efficient delivery of siRNA in the in vivo brain injury model (Fig. 23B and C). In another case, Lu et al. synthesized a core–shell structured mesoporous nanocomposite consisting of magnetic iron oxide NPs as the core and CaSi as the shell by using a two liquid phase system via ultrasound irradiation.382 The nanocomposite displayed superparamagnetic behavior, high specific surface area (474 m2 g−1) and pore volume (2.75 cm3 g−1), as well as high protein/drug loading capacities for Hb, DTX, and IBU.
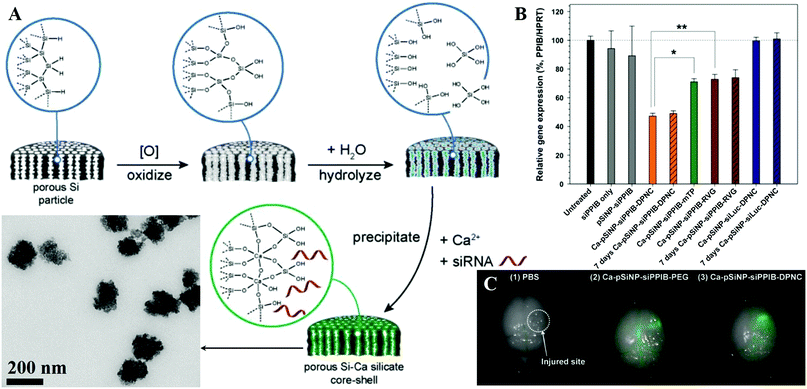 |
| Fig. 23 (A) Schematic illustration of the preparation of siRNA-loaded, CaSi-coated porous silicon nanoparticles (Ca-pSiNP-siRNA). (B) The silencing of relative PPIB gene expression in Neuro-2a cells after treatment with siRNA against the PPIB gene (siPPIB), aminated porous silicon nanoparticle (pSiNP) loaded with siPPIB (pSiNPsiPPIB), pSiNP-siPPIB construct prepared with a CaSi shell and containing both cell-targeting and cell-penetrating peptides on the outer shell in a dual peptide nanocomplex (Ca-pSiNP-siPPIB-DPNC), the pSiNP-siPPIB-CaSi shell construct containing only a cell-penetrating peptide on the outer shell (Ca-pSiNP-siPPIB-mTP), the pSiNP-siPPIB-CaSi shell construct containing only the cell-targeting peptide on the outer shell (Ca-pSiNP-siPPIB-RVG), and the pSi nanoparticle-CaSi shell construct containing a negative control siRNA sequence against luciferase, and containing both the cell-targeting and the cell-penetrating peptides on the outer shell (Ca-pSiNP-siLuc-DPNC). The “7 days” designations indicate that the nanoparticle construct was stored in ethanol at 4 °C for 7 d prior to the experiment. The cell penetrating peptide is a myristoylated transportan, and the cell targeting peptide is a domain derived from the rabies virus glycopeptide (RVG). Statistical analyses were performed with Student's t test (*P < 0.01, **P < 0.03). (C) Ex vivo fluorescence images of harvested organs after intravenous injection of (1) saline as a control, (2) Ca-pSiNP-siRNA-PEG, and (3) Ca-pSiNP-siRNA-DPNC. All siRNA constructs contained covalently attached dy677 fluorophore (Em: 700 nm). Reprinted with permission from ref. 385. Copyright 2016, Wiley-VCH. | |
5.2 Hyperthermia therapy (HTT)
Hyperthermia therapy (HTT) is based on the fact that magnetic NPs on inductive heating with a high frequency and alternating magnetic field produce hyperthermia and kill oncocytes (namely magnetic HTT), or is based on the photothermal conversion materials that can absorb light energy and transform it into localized heat to produce controlled local necrosis of oncocytes (namely photothermal therapy, PTT). In recent years, HTT has drawn increasing attention since it is a promising therapeutic modality for treating oncological diseases. As shown in Fig. 24, Xu and co-workers developed an injectable and biodegradable material using CaP cement containing Fe3O4 NPs for minimally invasive and efficient magnetic hyperthermia ablation of tumors (Fig. 24A).259 After directly injected into the center of tumors under the guidance of USI, the magnetic CaP cement was well-restricted within the tumor tissues without leakage (Fig. 24B), and tumors were completely ablated after 180 s of induction heating (Fig. 24C–F). In another case, Hou and colleagues prepared magnetic CaPs NPs by a co-precipitation method with the addition of Fe2+ ions.254,255 Upon exposure to the magnetic field, the mice injected subcutaneously with magnetic CaP NPs showed dramatic reduction of tumor volume without recurrence.
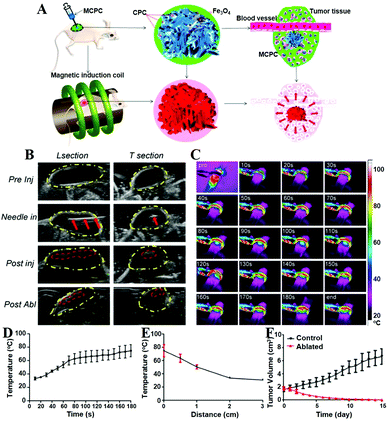 |
| Fig. 24 (A) Schematic illustration of magnetic CaP cement (MCPC) for minimally invasive and efficient magnetic hyperthermia ablation of tumors. (B) Ultrasound-guided injection and the USI of the injected 0.36 g of 10% MCPC in tumor pre- and post-180 s of magnetic induction heating. (L section: longitudinal section; T section: transverse section; Pre Inj: pre-injection; Post inj: post-injection; Post abl: post-ablation; yellow dotted line: tumor; red dotted line: MCPC; red arrows: needle). (C) The thermal images of tumor containing 0.36 g of 10% MCPC that was exposed to an alternating magnetic field for 180 s. (D) The corresponding time–temperature curve. (E) The distance–temperature curve for 0.36 g of 10% MCPC embedded in the tumor after 180 s of magnetic induction heating. (F) The time–tumor volume curve. Reprinted with permission from ref. 259. Copyright 2015, American Chemical Society. | |
Additionally, nanostructured CaPs and CaCs integrated with photothermal conversion materials such as gold and polyaniline NPs to form hybrid biomaterials represent a useful alternative in PTT. For example, gold-layered CaP NPs can efficiently ablate human epithelial cancer through PTT,26 and polyaniline loaded CaC microparticles display high efficiency for PTT.373
5.3 Photodynamic therapy (PDT)
PDT has become an emerging strategy for cancer therapy due to its specific spatiotemporal selectivity and minimal invasiveness.533–535 PDT is based on the cytotoxicity of reactive oxygen species (ROS) that are generated by PSs when energy from their excited state is transferred to the surrounding oxygen, thus inducing the death of cancerous cells. The delivery of PSs is a key step in PDT. Nanostructured CaPs-based263,266,267 and CaCs-based370,371,536–538 biomaterials are ideal carriers for PS delivery. For example, Lee et al. employed Chlorin e6 (Ce6)-loaded PEG-PAsp-PPhe polymer micelles as templates for the mineralization of CaP.263 The CaP layer effectively inhibited Ce6 release at the physiological pH value, and enhanced the phototoxicity upon irradiation compared to free Ce6. Similarly, Nomoto and co-workers prepared Ce6-loaded CaP/PEG-PAsp hybrid NPs, which reduced the photochemical damage in the bloodstream while inducing effective PDT efficacy inside tumor cells in response to the acidic conditions.266 In another case, Schwiertz et al. used polymer-functionalized CaP NPs as efficient PS carriers for PDT on cells and bacteria.261 The efficiency of PDT strongly depended on a number of parameters including particle charge, the kind of PS and polymer, cell culture medium and incubation time. Besides, Barth and colleagues reported the nontoxic and nonaggregating calcium phosphosilicate NPs (CPSNPs) to load ICG for targeted PDT of leukemia.262 The antibody conjugated CPSNPs specifically targeted CD117 or CD96, which dramatically enhanced both in vitro and in vivo efficacy of PDT. These leukemia-targeted CPSNPs offered the promise to effectively treat relapsing and multidrug-resistant leukemia and to improve the life of leukemia patients. Interestingly, CaF2:Tm NPs had intense afterglow emission with a long lifetime.411 Since the wavelength of the afterglow emission of the NPs fairly matched with the absorption band of the PpIX sensitizer, the PpIX conjugated CaF2:Tm NPs were recommended as a source of energy to improve PDT.
5.4 PhotoImmunoNanoTherapy
The therapeutic application of ICG-CPSNPs as PSs for PDT was shown to inhibit tumor growth in murine models of breast cancer, pancreatic cancer, and metastatic osteosarcoma by decreasing inflammation-expanded immature myeloid cells, and this therapeutic modality was termed as PhotoImmunoNanoTherapy (Fig. 25A).268 PhotoImmunoNanoTherapy utilizing ICG-CPSNPs exerted an antitumor effect by inducing a sphingosine kinase 2-dependent increase in sphingosine-1-phosphate (S1P) and dihydrosphingosine-1-phosphate (dhS1P), and the generated dhS1P could ablate immature myeloid cells that are often expanded as a function of tumor burden. This allowed the expansion of immune effector cells from hematopoietic progenitor cells, and this subsequently led to an antitumor immune response. Thus, PhotoImmunoNanoTherapy could decrease tumor-associated inflammation (Fig. 25B) and immature myeloid cells (Fig. 25C) in a sphingosine kinase 2-dependent manner, with the potential to be a revolutionary treatment for cancers with inflammatory and immunosuppressive phenotypes (Fig. 25D–I). This mechanism was in addition to, and independent from, traditional oxidative-mediated tumor cell death that is a hallmark of PDT.
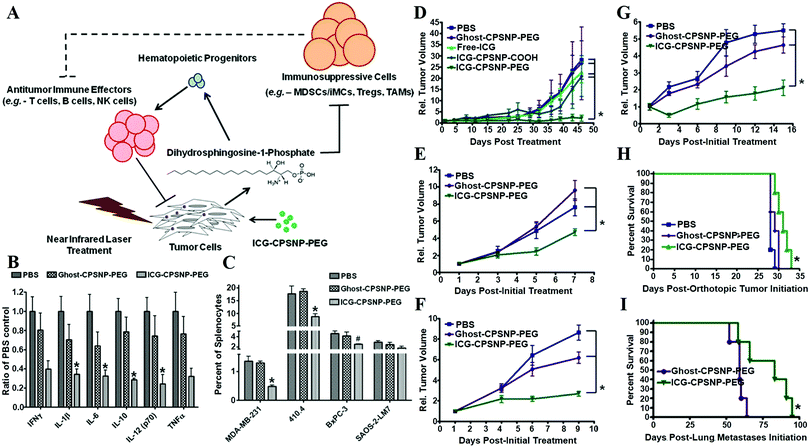 |
| Fig. 25 (A) Schematic illustration demonstrating that PhotoImmunoNanoTherapy utilizing ICG-CPSNP-PEG exerts an antitumor effect by generating dihydrosphingosine-1-phosphate (dhS1P) to ablate immature myeloid cells. PhotoImmunoNanoTherapy diminishes the systemic inflammatory milieu (B) and the immature myeloid immune milieu (C). (D–I) PhotoImmunoNanoTherapy decreases tumor burden and improves survival (ICG, indocyanine green; ghost, empty; CPSNP, calcium phosphosilicate nanoparticles; COOH, citrate-functionalized; PEG, PEGylated). Tumor growth following PhotoImmunoNanoTherapy was monitored in athymic nude mice engrafted with human MDA-MB-231 breast cancer cells (D), BALB/cJ mice engrafted with murine 410.4 breast cancer cells (E), NOD.CB17-Prkdcscid/J mice engrafted with murine 410.4 breast cancer cells (F) and C57BL/6J mice engrafted with murine Panc-02 pancreatic cancer cells (G). (H) The survival of athymic nude mice orthotopically implanted with human BxPC-3-GFP pancreatic cancer cells was monitored following PhotoImmunoNanoTherapy. (I) The survival of athymic nude mice with experimental lung metastases of human SAOS-2-LM7 osteosarcoma cells was monitored following PhotoImmunoNanoTherapy. Reprinted with permission from ref. 268. Copyright 2013, American Chemical Society. | |
5.5 Calcification therapy
Calcification and mineral accumulation in organisms are ubiquitous biological processes. However, abnormal Ca deposition in soft tissues may cause pathological diseases such as vascular calcification, skin necrosis and kidney stones. Inspired by biomineralization, the Tang group reported an artificial CaP mineral shell on yeast cells to protect and modify the living cells.269 The thin mineral shell on the surface of cells altered their behavior and inhibited their proliferation, even caused their necrosis when the mineral layer remained for a long time. Based on these findings, they further developed a drug-free tumor therapy strategy by using cancer cell targeting calcification (CCTC, Fig. 26A and B).270 Because the folate receptors (FR) which are overexpressed in many human carcinomas but expressed at low levels in normal cells (Fig. 26C and D) could selectively adsorb FA molecules, and the FA molecules could specifically bind Ca2+ from biological fluids to facilitate Ca mineral nucleation (Fig. 26E and F) owing to their carboxylate residues, the abundant FRs on cancer cells could be used to develop CCTC. As shown in Fig. 26G–M, the resultant Ca mineral layer observably induced cancer cell death (Fig. 26G and H), and in vivo assessments further confirmed that the CCTC treatment could efficiently inhibit tumor growth, successfully control tumor metastasis, and markedly improve survival rates without damaging normal cells compared with conventional chemotherapy (Fig. 26I–M). Notably, both FA and Ca2+ ions are essential ingredients in human metabolism, and thus CCTC was a successful drug-free method for tumor therapy, which provided an alternative cancer therapy characterized by selective calcification-based substitution of sclerosis for tumor disease.
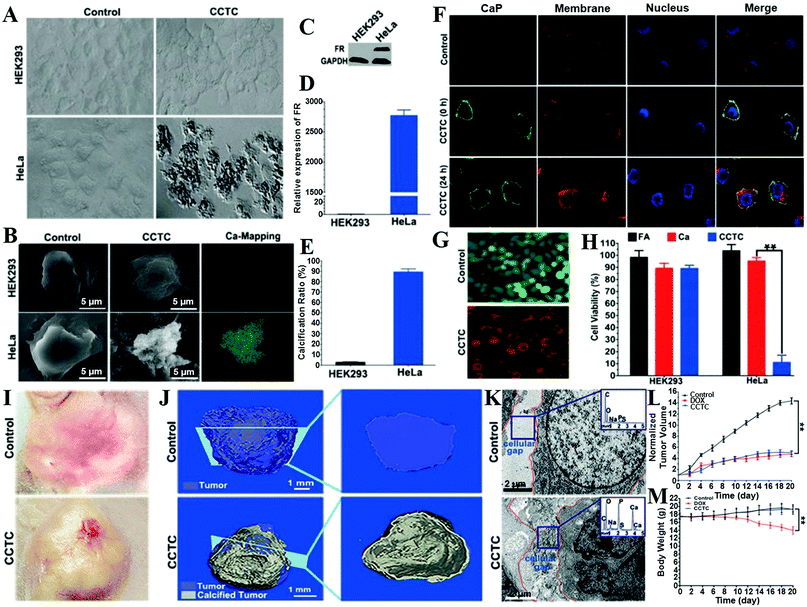 |
| Fig. 26 Optical (A) and SEM (B) observations of HEK293 and HeLa cells. Relative expression of FR in HEK293 and HeLa cells by western blot (C) and qPCR (D). (E) Calcification ratio of HEK293 and HeLa cells. (F) Fluorescent detection of calcified HeLa cells by confocal laser scanning microscopy (CLSM). CaP was stained by calcein (green), the cell membrane was stained by PKH26 (red), and the nucleus was stained by Hoechst33342 (blue). (G) Live/dead staining suggested that the CCTC-treated HeLa cells were dead at 24 h. (H) Quantitative analysis of cell viability with different treatments (**P < 0.01). (I) Optical images of calcified tumors; the tumor tissue became disrupted after CCTC treatment. (J) μCT detection of the tumors. The left column shows a three-dimensional reconstruction, and the right is a typical tomography section marked on the left. (K) TEM observation and EDX (inset) of calcified matter in tumor cellular gaps. (L) Normalized tumor growth curves in the control, DOX, and CCTC treatment groups (volume vs. time). (M) Body weights of mice after the different treatments. Reprinted with permission from ref. 270. Copyright 2016, Wiley-VCH. | |
5.6 Combination therapy
Combination therapy with different types of therapeutics can significantly reduce multidrug resistance and minimize dose, and as a result, improve the efficiency of cancer therapies. For instance, drug-loaded magnetite CaPs are used as combination cancer treatment of hyperthermia and chemotherapy.257,260 The therapeutic antibody linked CaP/Au nanocomposites are promising for bimodal cancer treatment by combination of a biocompatible mineral plasmon resonant for a localized PTT and a therapeutic antibody for a systemic treatment.26 In addition, Fe3O4@CaP capped Au nanocages can integrate magnetic targeting, PTT and chemotherapy for synergistically killing cancer cells.27 Furthermore, the Hsu group used anisamide-targeted LCP NPs to efficiently deliver HIF1α siRNA to the cytoplasm of sigma receptor expressing SCC4 and SAS cells, which effectively reduced HIF1α expression, increased cell death, and significantly inhibited cell growth following photosan-mediated PDT in cultured cells.264 When combined with PDT, HIF1α siRNA loaded NPs enhanced the regression in tumor size resulting in a ∼40% decrease in volume after 10 days; thus combination therapy was substantially more effective than either HIF1α siRNA or PDT alone. Meanwhile, they also confirmed that PDT combined with targeted VEGF-A gene therapy by using LCP NPs was a potential therapeutic modality to achieve enhanced therapeutic outcome for human head and neck squamous cell carcinoma.265
CaCs-Based biomaterials are often used for the co-delivery of genes and drugs,346,347 which combines gene therapy and chemotherapy for tumor treatments to achieve maximum therapeutic efficiency. By integration with Au NPs, CaCs nanocarriers can combine PTT, chemotherapy and gene therapy into one nanotheranostic. For example, Kong et al. reported biodegradable Au NRs@CaC NPs (Fig. 27A) coated with pH-responsive acetalated dextran and phospholipid as an advanced platform for the combination of photothermal effects with versatile molecular (including hydrophilic and hydrophobic drugs, as well as the model enzyme, green fluorescent protein or antibody) targeted therapeutics, which showed good biocompatibility, photothermal stability, and pH responsiveness.372 The photothermal effects of the therapeutics co-loaded CaC-based hybrid particles could promote ultrafast therapeutics release, which efficiently induced cancer cell death and reduced multidrug resistance and HER2 expression with synergism (Fig. 27D and C).
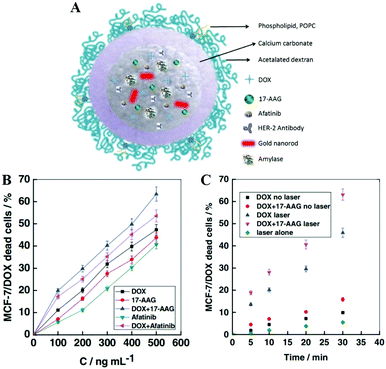 |
| Fig. 27 (A) Schematic illustration of the biocompatible CaC-based hybrid platform with photothermal and pH responsiveness for advancing biomedical applications by co-delivery of versatile therapeutics, enzyme, and antibody. (B) Cell viability of Au NRs@CaC@POPC hybrid particles, loaded with either single drugs (DOX, 17-AAG, and afatinib) or multiple drugs (DOX + 17-AAG and DOX + afatinib), in DOX-resistant MCF-7/DOX cells after 24 h incubation determined by the live/dead assay (CaC@POPC + 10 × 10−9 M Au NRs set as negative control). (C) Cell viability of DOX and DOX + 17-AAG loaded AuNRs@CaC@POPC particles under laser irradiation at 650 nm at different time intervals or without laser irradiation in MCF-7/DOX cells after 2 h incubation using live/dead assay. Laser alone (no particles, no drugs) is set as control. Reprinted with permission from ref. 372. Copyright 2016, Wiley-VCH. | |
6. CaXs biomaterials for theranostics
The rational design and synthesis of novel theranostic agents with both imaging and therapeutic functions have profound impact on molecular diagnostics, imaging, and therapeutics, which can significantly enhance the accuracy and efficiency of cancer therapy. Nanostructured CaXs biomaterials show potential for both bioimaging and cancer therapy, and thus they can be designed as novel nanotheranostics for imaging-guided therapy.
6.1 CaPs for theranostics
6.1.1 FLI-guided therapy.
Self-activated luminescent CaPs are promising for FLI-guided therapy.271–275 As an example, Singh et al. reported fluorescent HAP NR-based biomaterials for drug delivery, which expressed a strong blue emission at 427 nm, high drug loading efficiency (10%), long releasing time (over 3 days), and in situ FLI within the cells.275 In addition, Yang and colleagues utilized self-activated luminescent HAP to coat mesoporous silica NPs for controlled drug delivery and monitoring drug release.271 These NPs loaded with captopril drug still showed strong blue emission, and the luminescence intensity kept increasing when the drug was released, which showed great potential for controlled drug delivery, in vivo monitoring, and disease diagnosis.
Besides, CaPs biomaterials are flexibly designed as a FLI-guided therapy platform by integrating with FLI CAs and therapeutic agents.276–280,284–291 For instance, Yang and co-workers reported that IBU-loaded and Eu-doped HAP NPs still showed red luminescence of Eu3+ (5D0–7F1,2) under ultraviolet (UV) irradiation, and the emission intensities of Eu3+ varied with the release amount of IBU, and thus this DDS could easily track and monitor the drug release by the change of luminescence intensity.276 The Adair group designed a methodology to encapsulate both organic fluorophores and therapeutic drugs within a pH-responsive and nontoxic CaPs NP matrix.20,287 The CaPs-based encapsulation system with the assistance of FLI could assess the efficiency of drug delivery and possible mechanisms of NP bioabsorption. Similarly, Bastakoti and colleagues developed CaP-based core–shell–corona NPs with the capability of accommodating hydrophobic fluorescent dyes (Nile red) into the core and hydrophilic drugs (cisplatin or DOX) into the CaP shell, respectively.288,289 The fluorescent dyes were used for FLI to determine the location of the NPs and to give an observable indication of drug delivery, while the CaP shell could enhance the fluorescence of the encapsulated dyes and realize pH-triggered drug-release. In another case, Haedicke et al. used CaP NPs to conjugate with (i) Temoporfin as a PS, (ii) RGDfK peptide for favored tumor targeting and (iii) the fluorescent dye molecule DY682-NHS for enabling NIR FLI, which can realize FLI-guided PDT of tumors.291
6.1.2 MRI-guided therapy.
CaPs combined with MRI CAs are multifunctional DDSs for MRI-guided therapy. For example, the multifunctional carbon/CaP/Fe3O4 composite NPs that integrate high drug loading, pH/NIR-sensitive PTT and MRI into one nanoplatform can be employed as MRI-guided pH/NIR dual-responsive drug delivery vehicles for simultaneous PTT and chemotherapy.301 The CaPs-based core–shell NPs of DOX–CaP shell on amphiphilic gelatin–iron oxide core can act as a multifunctional DDS with improved cytocompatibility, pH-responsive drug release and MRI, which is a promising multifunctional nanodevice for nanotherapeutic approaches.302 In another case, Liu and co-workers developed a novel ultrasonically triggered drug delivery vehicle with MRI properties by encapsulating iron oxide NPs in HAP-coated liposomes, which could be used not only as a MRI-guided drug delivery vehicle capable of ultrasonically triggered release but also as a MRI reporter to probe ultrasonic triggering.30,31 Interestingly, Mi and colleagues developed PEGylated Gd-DTPA/CaP NPs for MRI-guided Gd neutron capture therapy (GdNCT) of tumors (Fig. 28A–C).298,299 The high r1 relaxivity of Gd-DTPA/CaP NPs sensitively enhanced the contrast of neoplastic tissues for MRI-guided tumor positioning (Fig. 28D–F), and the high accumulation of the NP-delivered Gd-DTPA in tumor tissues effectively damaged cancer cells by γ-rays or electron emission from the Gd nuclides, resulting in high therapeutic efficacy for GdNCT against solid tumors (Fig. 28G–I). Thus, Gd-DTPA/CaP NPs significantly improved the pharmacological properties of Gd-DTPA, such as plasma clearance, tumor accumulation, contrast specificity, and antitumor effects in GdNCT, demonstrating potential for noninvasive MRI and NCT.
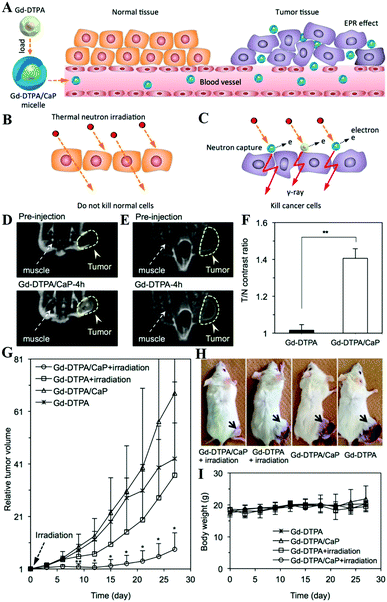 |
| Fig. 28 (A) The accumulation of Gd-DTPA delivered by Gd-DTPA/CaP in tumors through the EPR effect. (B) Low energy thermal neutron irradiation does not kill normal cells without neutron capture therapy agents. (C) Thermal neutron irradiation could kill or cause hazardous damage to cancer cells by the γ-rays emitted from the Gd nuclides after nuclear reaction with captured thermal neutrons. (D and E) In vivo images of mice bearing subcutaneous C26 tumors after intravenous injection of Gd-DTPA/CaP (D) and Gd-DTPA (E). (F) A comparison of the tumor-to-normal tissue (T/N) contrast intensity calculated from (D) and (E) by comparing the signal intensity of the regions of interest (ROI) between tumors and normal tissues. (G) The growth curve of the subcutaneous C26 tumors after administration of Gd-DTPA/CaP and treatment with thermal neutron irradiation for 1 h. The tumors were irradiated 24 h after the intravenous injection of Gd-DTPA/CaP (day 0). (H) Images of mice bearing subcutaneous C26 tumors 27 days after GdNCT. (I) Relative changes in the body weight of mice after thermal neutron irradiation. Reprinted with permission from ref. 298. Copyright 2015, American Chemical Society. | |
6.1.3 Photoacoustic imaging (PAI)-guided therapy.
Photoacoustic imaging (PAI) is based on the phenomenon that an object produces a sound signal under light irradiation, which can create multiscale/multicontrast images of living biological structures ranging from organelles to organs.539 Many biomolecules such as Hb and melanin are ideal endogenous PAI CAs owing to their strong NIR absorption and high photothermal conversion efficiency. By combination of CaPs with such biomolecules, biocompatible nanotheranostics could be prepared for PAI-guided therapy. As shown in Fig. 29, Zhang and colleagues fabricated spherical polydopamine (PDA)/mesoporous CaP hollow Janus NPs (PDA/mCaP H-JNPs) by using poly(acrylic acid) (PAA) NPs as the template, and then selectively functionalized with ICG and methoxy-poly(ethylene glycol)thiol (PEG-SH) on PDA domains to achieve superior PAI capability and stability. The mesoporous CaP with a hollow cavity served as a storage space and passage for the anti-cancer drug DOX. The resultant PEG-ICG-PDA/mCaP H-JNPs exhibited excellent biocompatibility, competent drug loading capability, high photothermal conversion efficiency, strong NIR absorbance, and pH/NIR dual-responsive properties, for PAI-guided synergistic cancer chemo–phototherapy.304
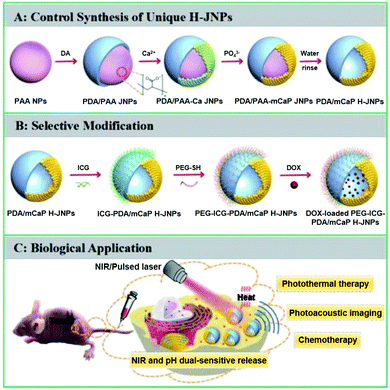 |
| Fig. 29 (A) Schematic showing the controlled synthesis of PEG-ICG-PDA/mCaP H-JNPs, (B) biofunctionalization of H-JNPs, (C) PAI-guided chemo–photothermal synergistic cancer therapy. Reprinted with permission from ref. 304. Copyright 2017, Royal Society of Chemistry. | |
6.1.4 Nuclear imaging-guided therapy.
Nuclear imagings such as SPECT, PET and CT have been widely used for preclinical and clinical imaging of diseases owing to their high sensitivity and noninvasiveness.540,541 By integrating with radionuclides, CaPs-based biomaterials can be used for nuclear imaging-guided therapy.300 For example, the Huang group reported that 111In labeled LCP NPs preferentially accumulated in the lymph nodes via lymphatic drainage, which were used for SPECT/CT imaging-guided lymphatic gene delivery.67 In addition, they also developed radioisotope 177Lu loaded LCP NPs as cancer theranostics with high specific drug loading, which allowed in vivo anticancer therapy in addition to radiographic imaging via the dual decay modes of 177Lu.295 The radionuclide loading could be increased by several orders of magnitude without affecting the encapsulation efficiency or the morphology of 177Lu-LCP. As a result, treatment with just one dose of 177Lu-LCP showed significant in vivo tumor inhibition by causing apoptotic cell death via double-stranded DNA breaks while causing a remodeling of the tumor microenvironment to a more disordered and less malignant phenotype. Besides radionuclides, Wang and colleagues reported a novel, facile and reproducible method to construct uniform FA-Au@PAA/mCaP JNPs.305 The exposed Au domains with FA-PEG-SH could achieve cancer cell-specific targeting and high contrast for CT imaging, while the PAA/mCaP domains with a mesoporous structure served as a storage space and passage for the anti-cancer drug DOX. Together with their excellent stability and good biocompatibility, the obtained FA-Au@PAA/mCaP JNPs could act as an efficient nanoplatform for CT imaging and active-targeted chemotherapy in vitro.
6.1.5 Multimodal imaging-guided therapy.
CaPs combined with Au NPs can integrate CT imaging and FLI into one nanoplatform for dual-modality imaging-guided therapy. As shown in Fig. 30A, Li et al. developed a simple, scalable, and reproducible route to synthesize novel multifunctional spherical Au nanocluster assemblies encapsulated by a PAA/CaP shell with aggregation enhanced fluorescence property (designated as AuNCs-A@PAA/CaP) (Fig. 30B and C).25 These AuNCs-A@PAA/CaP NPs had a high payload of DOX as synergetic pH-sensitive (Fig. 30H) drug delivery vehicles which could be employed for CT (Fig. 30D–F) and FLI (Fig. 30G) dual-modality imaging-guided liver cancer chemotherapy (Fig. 30I–L) in vivo, and thus they had great promise for guiding and monitoring the chemotherapeutic process using simultaneous dual-modality imaging.
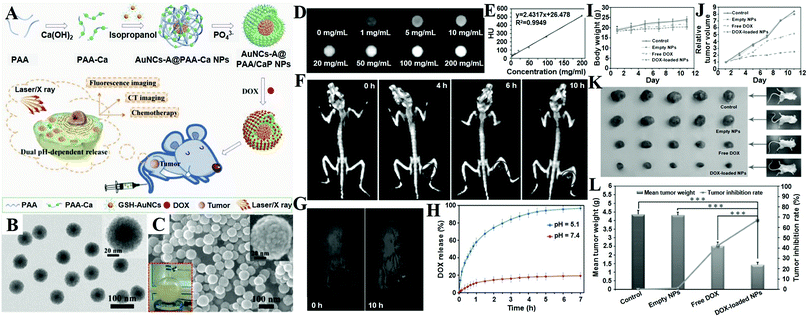 |
| Fig. 30 (A) Schematic illustration of the synthetic strategy for AuNCs-A@PAA/CaP NPs as pH-responsive drug delivery vehicles for dual-modality CT and FLI-guided liver cancer therapy in vitro and in vivo. TEM (B) and SEM (C) images of AuNCs-A@PAA/CaP NPs; inset: the corresponding high magnification images of a single NP. (D) CT phantom images of AuNCs-A@PAA/CaP NPs at various concentrations. (E) X-ray attenuation intensity (HU) as a function of the concentration of AuNCs-A@PAA/CaP NPs. (F) In vivo 3D X-ray CT imaging of H-22 tumor-bearing mice before and after intravenous injection of AuNCs-A@PAA/CaP NPs at different time points. (G) In vivo FLI of H-22-tumor-bearing mice before and 10 h after intravenous injection of AuNCs-A@PAA/CaP NPs. (H) DOX-release profiles for DOX-loaded AuNCs-A@PAA/CaP NPs measured at pH 5.1 and 7.4 in PBS buffer at 37 °C, respectively. Changes in the (I) body weight and (J) relative tumor volume of mice after the treatment with saline solution as control, empty NPs, free DOX, and DOX-loaded AuNCs-A@PAA/CaP NPs. (K) Representative photographs of excised tumors from euthanized mice on the 11th day posttreatment. (L) Comparative therapeutic efficacy study of an in vivo animal model. Reprinted with permission from ref. 25. Copyright 2015, Wiley-VCH. | |
In addition, both Gd-doped self-activated luminescent CaPs and Gd/lanthanide ion co-doped CaPs have exciting luminescent and MRI performance, which can be used for FLI and MRI dual-modality imaging-guided drug delivery.45,281–283 For example, Li and co-workers constructed aptamer-capped Gd-doped luminescent and mesoporous strontium HAP NRs with strong blue autofluorescence and paramagnetism, which served as a good CA for cancer-cell-responsive drug delivery and targeting FLI and MRI.45 Chen and colleagues reported multifunctional Eu3+/Gd3+ dual-doped CaPs with high drug loading capacity and sustained drug release behavior.281,282In vitro and in vivo imaging tests indicated that Eu3+/Gd3+ dual-doped CaPs had potential for applications such as a multiple-model imaging agent for FLI, MRI and CT, and thus they were promising for applications in the biomedical fields such as multifunctional DDSs with imaging guidance. Besides, CaPs integrated with lanthanide-doped Gd-based UCNPs are also used for FLI/MRI dual-modality imaging-guided drug delivery.293,294 For instance, the CaP covered NaGdF4:Ce/Tb hybrid system is capable of releasing guest drugs from the CaP-blocked pore under pH control.293 After introducing a layer of aptamer molecules on CaP, the afforded multifunctional theranostic platform is readily taken up by cells without premature leakage via receptor-mediated endocytosis and visualized through dual-mode imaging (FLI and MRI), resulting in enhanced therapeutic efficiency with minimized side effects.
6.2 CaCs for theranostics
6.2.1 FLI-guided therapy.
CaCs-Based nanomaterials can be designed as FLI-guided therapy platforms by the combination of drugs and FLI CAs. As an example, Begum et al. demonstrated a bioinspired method involving the macromolecular assembly of an anionic polypeptide with a cationic peptide-oligomer that allowed for in situ encapsulation of antibiotics like tetracycline in the CaC microstructure.368 This tetracycline-loaded sample exhibited a pH dependent drug-release profile and excellent antibacterial activity. The encapsulated drug or dye-conjugated peptide emitted fluorescence suitable for FLI and detection, hence making tetracycline-loaded CaC a multifunctional material.
6.2.2 MRI-guided therapy.
CaCs loaded with MRI CAs could realize MRI-guided cancer therapy. For example, the PEG modified CaC NPs were found to efficiently load both Mn2+-chelated Ce6 (Ce6(Mn)) and DOX, which showed high sensitivity to pH and rapidly degraded under a slightly acidic environment, resulting in the effective release of loaded therapeutic agents (Fig. 31A).371 The multifunctional nanocarrier showed an interesting pH-dependent T1 signal enhancement under MRI due to the released Ce6(Mn) (Fig. 31B–D), which could be utilized for real-time monitoring of drug release (Fig. 31E–G). Together with the synergistic anti-tumor effect of PDT and chemotherapy, the tumor-pH-activated nanocarrier based on biodegradable CaC nanoparticles could realize MRI-monitored cancer therapy.
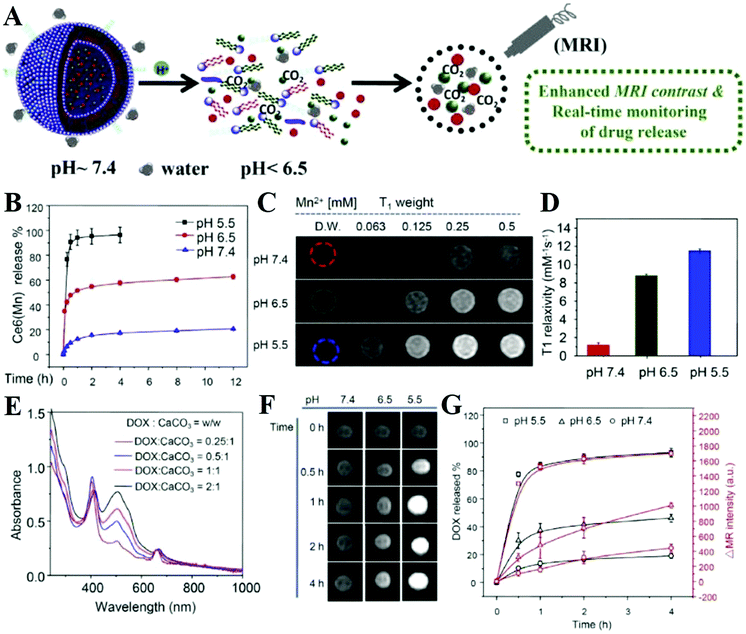 |
| Fig. 31 (A) Schematic illustration showing the pH-responsive decomposition of Ce6(Mn)@CaCO3-PEG NPs. (B) Time-dependent Ce6(Mn) release from Ce6(Mn)@CaCO3-PEG in PBS with different pH values. (C) T1-Weighted MRI of Ce6(Mn)@CaCO3-PEG with various concentrations taken 4 h after incubation in PBS at three different pH values. (D) T1 relaxivities of Ce6(Mn)@CaCO3-PEG at different pH values corresponding to figure (C). (E) UV-Vise-NIR spectra of DOX loaded Ce6(Mn)@CaCO3-PEG with different feeding concentrations of DOX. (F) Time-dependent T1-MRI of Ce6(Mn)@CaCO3-PEG(DOX) after incubation in PBS at different pH values. (G) Correlation between the percentage of released DOX and enhanced T1-MR signals over time at three pH values. Reprinted with permission from ref. 371. Copyright 2016, Elsevier. | |
6.2.3 USI-guided therapy.
CaCs can generate CO2 gas in acidic environments such as tumors and fat-rich plaques, which can enhance the USI signal and dissolve locally accumulated fat to prevent the blockage of blood vessels. Keum et al. reported a CaC-coated polycaprolactone (PCL) stent with the elution of CO2 microbubbles in a pH-responsive manner, which could successfully remove a large amount of fat without causing any severe damage or toxicity to tissues, and enabled real-time USI of the stent as a harmless CA, and thus it was effectively applied for noninvasive USI and prevention of restenosis after stent implantation.308 In another case, Lee and co-workers encapsulated CaCs using poly(D,L-lactide-co-glycolide) and then modified them using rabies virus glycoprotein (RVG) peptide (a targeting moiety to neuroblastoma) to construct cancer-targeting and gas-generating NPs (RVG-GNPs) (Fig. 32A).306 The obtained RVG-GNPs could effectively accumulate at tumor tissues and substantially release CO2 bubbles under tumor acidic conditions to enhance USI signals (Fig. 32B–G). Moreover, intravenous administration of RVG-GNPs could inhibit tumor growth without the use of other therapeutic agents (Fig. 32H and I), and thus they were useful as theranostics for the detection and treatment of cancers.
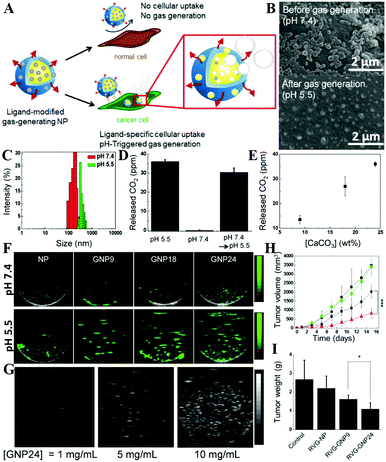 |
| Fig. 32 Schematic illustration (A) of ligand-modified gas-generating NPs for cancer-specific cellular uptake and pH-triggered gas generation. (B) SEM images of GNPs before and after gas generation. (C) Changes in the size and size distribution of GNPs after gas generation. (D) Amount of CO2 gas released from GNP24 and (E) effect of GNP CaC content on gas generation at pH 5.5. (F) In vitro ultrasound signals of non-gas-generating nanoparticles (NP) and gas-generating nanoparticles (GNP9, GNP18, and GNP24) under neutral and acidic conditions (10 mg mL−1). (G) Dose-dependent ultrasound signals generated from GNP24 at pH 5.5. (H) Changes in the tumor volume and (I) the final tumor weight of mice treated with saline (diamond), RVG-NP (square), RVG-GNP9 (circle), and RVG-GNP24 (triangle) (10 mg kg−1 polymer/mouse and 20 mg kg−1 docetaxel/mouse; five daily intravenous injections; *P < 0.05, ***P < 0.001). Reprinted with permission from ref. 306. Copyright 2016, Elsevier. | |
In fact, CaCs-based DDSs are intrinsic theranostic platforms for USI-guided therapy. As shown in Fig. 33, the Lee group developed DOX-loaded CaC hybrid NPs (DOX-CaC-MNPs) through a block copolymer templated in situ mineralization approach (Fig. 33A).369 The prepared DOX-CaC-MNPs displayed strong echogenic signals (Fig. 33D and E) and effective antitumor therapeutic activity (Fig. 33F–H) by triggering the DOX release simultaneously with CO2 bubble generation in the tumoral weak acid environment (Fig. 33B and C), and thus these theranostic NPs could express USI and simultaneous therapeutic functions for cancer treatment. Besides, they also constructed Ce6-loaded CaC-mineralized NPs that were considered as promising theranostics for USI-guided PDT in the field of tumor therapy.370
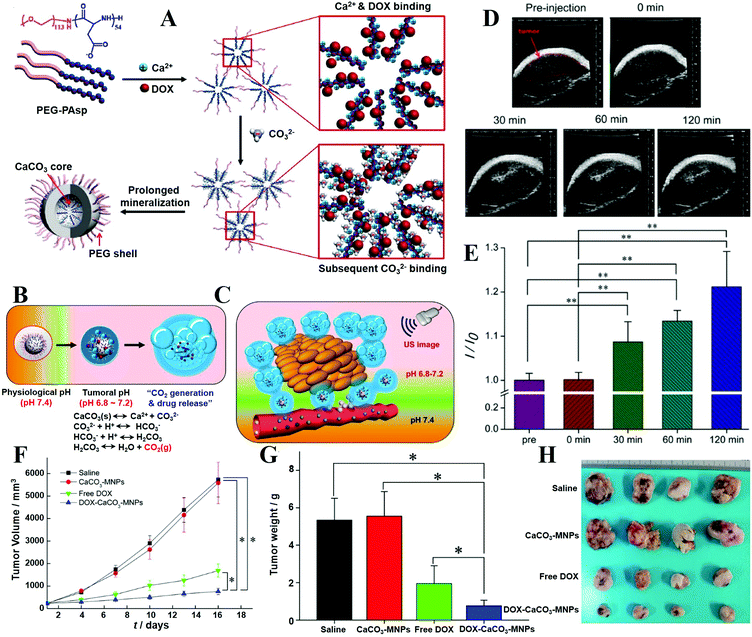 |
| Fig. 33 Schematic illustration of (A) the fabrication process of DOX-CaC-MNPs, (B) the mechanism of CO2 generation and drug release, and (C) bubble generation and drug release after accumulation of DOX-CaC-MNPs at tumor tissues. (D) In vivo US imaging of the SCC-7 tumor by intratumoral injection of DOX-CaC-MNPs, (E) histogram of a US intensity profile of the tumor as a function of time (I0 is the ultrasound intensity of preinjection). (F) Changes in tumor volumes after injection of saline, CaC-MNPs, free DOX, and DOX-CaC-MNPs; (G) tumor weights after 16 days post-treatment; and (H) images of excised tumors of each group with controls (saline and CaC-MNPs), free DOX, and DOX-CaC-MNPs after 16 days post-treatment. Reprinted with permission from ref. 369. Copyright 2015, American Chemical Society. | |
6.3 CaF2 for theranostics
6.3.1 FLI-guided therapy.
CaF2-Based DDSs have exciting luminescent performance, which can be used for FLI-guided therapy.409 For example, the Lin group prepared CaF2:Ce3+/Tb3+ hollow microspheres and then filled the pH-responsive PAA inside the cavity to obtain CaF2:Ce3+/Tb3+–PAA hybrid microspheres with strong green fluorescence and pH-dependent drug release behavior. These hybrid microspheres could easily track and monitor the drug release by means of the change in luminescence intensity.405,406
6.3.2 Multimodal imaging-guided therapy.
CaF2-Based NPs can also be designed as a multifunctional theranostic platform for multimodal imaging-guided therapy. For instance, alkali ion doped CaF2-based UCNPs exhibit enhanced upconversion luminescence and high contrast CT imaging signals, and thus they are promising for FLI/CT dual-modal imaging and chemotherapy.408 In addition, Qiao and co-workers used NaGdF4:Yb,Er@CaF2 UCNPs as the core and PS-grafted mesoporous silica as the shell to construct core–shell structured NPs, which integrated FLI, MRI and PDT into one nanoplatform for FLI/MRI dual-modality imaging-guided PDT.410 This nanoplatform exhibited excellent PDT efficiency through an energy transfer process between the UCNP core and PS molecules. In another case, Deng and colleagues constructed hollow structured CaF2:Yb3+/Er3+/Mn2+–poly(2-aminoethyl methacrylate) (UCNP–PAMA) hybrid microspheres for Pt(IV) pro-drug delivery (Fig. 34A and B).407 The UCNP–PAMA hybrid microspheres showed orange fluorescence for FLI (Fig. 34C), and the presence of functional Mn2+ and Yb3+ offered enhanced T1-weighted MRI (Fig. 34D) and CT (Fig. 34E) imaging, respectively. Due to the introduction of PAMA into the hollow nanospheres the Pt(IV) pro-drug could be bonded on the polymer network to construct an anti-cancer drug carrier. Thus, the multifunctional UCNP–PAMA–Pt(IV) hybrid microspheres had potential for FL/MRI/CT trimodal imaging-guided chemotherapy with low toxic side effects and high tumor inhibition rates in vivo (Fig. 34F and G).
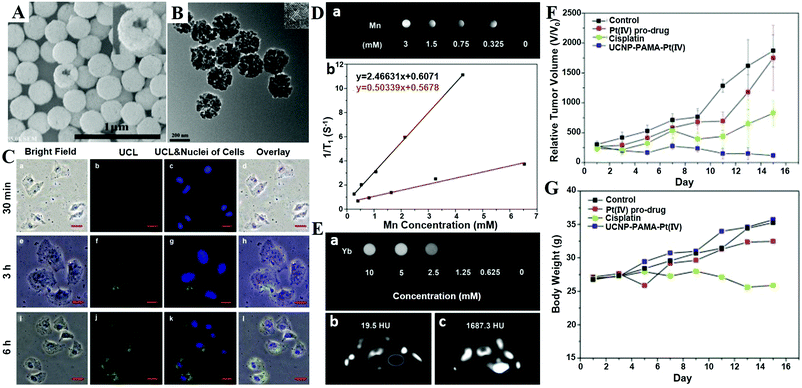 |
| Fig. 34 SEM (A) and TEM (B) images of UCNP–PAMA hybrid microspheres. The insets in graphs (A) and (B) represent the broken microsphere after ultrasound treatment and the HRTEM image of hybrid microspheres, respectively. (C) Inverted fluorescence microscope images of HeLa cells incubated with UCNP–PAMA for 30 min (a–d), 3 h (e–h) and 6 h (i–l) at 37 °C. Each series can be divided into the bright-field image, upconversion luminescence image (UCL), UCL and nuclei of cells (stained blue by Hoechst 33342 for visualization) and the overlay of the above three, respectively. All scale bars are 50 mm. (D) In vitro T1-weighted MRI of UCNP–PAMA hybrid microspheres at different Mn concentrations (a); relaxation rate R1versus different molar concentrations of Mn in UCNP–PAMA (b). (E) In vitro CT images of UCNP–PAMA at different concentrations of Yb ions (a); CT images of a tumor-bearing Balb/c mouse: pre-injection (b) and after injection (c) of hybrid microspheres in situ. In vivo anti-tumor efficacy of UNNP–PAMA–Pt(IV) on tumor-bearing Kunming mice. Changes in relative tumor volume (F) and body weight (G) achieved in mice after the treatment with saline solution as control, Pt(IV) pro-drug, cisplatin and UCNP–PAMA–Pt(IV), respectively. Reprinted with permission from ref. 407. Copyright 2015, Elsevier. | |
7. Conclusions and future outlooks
CaXs biomaterials that are derived from organisms have excellent biocompatibility, bioactivity and biodegradability, and thus they are widely investigated for a variety of biomedical applications. Recent advances in nanotechnology and nanomedicine have promoted the rapid development of nanostructured CaXs biomaterials including CaPs, CaCs, CaSi and CaF2 for diagnosis, treatment and theranostics owing to their easy preparation and functionalization, high drug loading capability and gene transfection efficiency, pH-responsive drug release, as well as good biosafety and bioabsorbability. Among these CaXs biomaterials, most of the studies are focused on CaPs, since the Ca2+ and PO43− ions are abundant in the human body and the body could quickly restore the balance of these ions by metabolism. Moreover, nanostructured CaPs have good stability in solution and are easy to prepare at room temperature. Although CaCs also have similar biosafety and bioabsorbability as CaPs, their instability has limited their biomedical applications. Additionally, since Si and F are trace elements in the human body, both CaSi and CaF2 cannot be used at a high dose. Besides, CaF2 NPs with high crystallinity and outstanding luminescence property are usually prepared at high temperature. To sum up, all of the CaPs, CaCs and CaSi are ideal nanocarriers and gate-keepers for pH-responsive and targeted drug/gene/protein delivery. Especially, CaCs are ideal USI CAs with gas-generation properties in response to tumour environments, and nanostructured CaF2 is a unique phosphor host to generate upconversion luminescence for PLI.
As summarized in this review, various CaXs biomaterials with different size, shape, structure, composition and surface modification have been exploited for biomedical applications. According to the “Physical approaches to biomaterial design”,542 some universal guidelines for the bioapplications of CaXs are summarized as follows. (1) Nanosized CaXs with a small size of less than 200 nm are suitable for intravenous injection, while CaXs biomaterials with a large size are more suitable for local injection or oral administration. (2) In order to enhance their hemocompatibility and targeting ability, the surface modification of CaXs NPs with various ligands is highly desirable. (3) In contrast with spherical CaXs, nonspherical CaXs are more favorable for cellular internalization and vascular dynamics. (4) The surface microstructure, texture and porosity of CaXs NPs affect the interaction between NPs and cells, and a rough surface is more conducive over a flat surface.
Although CaXs biomaterials have been widely investigated, there are still many challenges that need to be tackled before their clinical translation. In order to optimize and accelerate the clinical translation of CaXs biomaterials, the following concerns should be considered in the future.
(1) It is important to further study the physicochemical properties and formation mechanism of natural CaXs biominerals, which can guide the design and synthesis of CaXs biomaterials. By understanding the biomineralization process, we can integrate various therapeutic agents and imaging CAs into the CaXs platform through a biomimetic mineralization strategy, which can guarantee the biocompatibility of the obtained CaXs biomaterials. Moreover, the biomineralization can also help us to understand the metabolism processes of CaXs minerals, which contributes to evaluate the biosafety of CaXs biomaterials. Interestingly, inspired by biomineralization, the accumulation of CaXs mineral on the surface of tumour cells can be developed into a novel therapeutic strategy namely calcification therapy. When the mineral layer remains for a long time, the calcification therapy could alter the behavior of cells, inhibit their proliferation, and finally cause their necrosis.
(2) To further improve the performance of CaXs biomaterials, it is essential to precisely control their sizes, components, and surface coatings so that batch to batch variation is minimized. Meanwhile, the precision preparation methods of CaXs are highly desirable to integrate different functions into one platform. Among all reported preparation methods, the biomineralization method is suitable for the preparation of CaXs DDSs, since it is simple, convenient and environment-friendly. Moreover, CaXs biomaterials that are obtained by biomineralization exhibit improved stability, biosafety and absorbability. In addition, multifunctional CaXs biomaterials can be constructed by adding different functional biomolecules (such as PDA, melanin, and so on) during the biomimetic mineralization process. To obtain the highly crystalline CaXs biomaterials, the hydrothermal, solvothermal and microwave-assisted synthesis methods are good choices.
(3) For biomedical applications, CaXs biomaterials need to be designed according to their specific applications, because the functions of CaXs are strongly dependent on their physicochemical properties. For example, small sized CaXs NPs with pH-responsive solubility can serve as intelligent DDSs for drug delivery. The nanostructured CaCs are more suitable for USI than other CaXs, while CaF2 is more suitable for FLI than other CaXs. Moreover, specific surface modification of CaXs biomaterials is also helpful to target specific cells/tissues, and step over various biological barriers, such as the blood–brain barrier, cellular membranes, extracellular matrix, mucus, and so on.
(4) Although CaXs are usually regarded as biocompatible and biodegradable materials, there is still a need for systematic investigation of their long-term effects such as toxicity, biocompatibility, immunogenicity, and pharmacokinetics (absorption, distribution, metabolism, and excretion, ADME) from small animal models (e.g. mice, rats) to large animal models (e.g. monkeys) before clinical translation. Recently, some published articles reported the cytotoxicity of CaPs NPs based on the observation that the suddenly released ions disturb the osmotic pressure balance and lead to cell necrosis.543–546 The cytotoxicity of HAP NPs is shape and cell dependent, and the needle/plate-shaped HA induce the most significant cell-specific cytotoxicity and IL-6 (inflammatory cytokines) expression but show the least particle–cell association.544 Moreover, HAP NPs have a time-dependent toxicological effect on a natural pulmonary surfactant because of protein adsorption onto the HAP NPs.546 The depletion of surfactant proteins from phospholipid vesicles caused conversion of original large vesicles into much smaller vesicles with poor surface activity. These small vesicles, in turn, inhibited the biophysical function of surfactant films after adsorption at the air–water interface. Although there is no report on the cytotoxicity of CaSi and CaF2 NPs, the effect of excess SiO32− and F− ions produced by their degradation should be taken into consideration, which may cause some adverse biophysical effects. In a word, CaXs biomaterials will be developed as safe and versatile clinical solutions for diagnosis, treatment, and theranostics of diseases.
Abbreviations
ACC | Amorphous calcium carbonate |
ACP | Amorphous calcium phosphate |
ACVP | Acyclovir monophosphate |
ATP | Adenosine triphosphate |
CAs | Contrast agents |
CaXs | Calcium-based |
CaPs | Calcium phosphates |
CaCs | Calcium carbonates |
CaSi | Calcium silicate |
CaF2 | Calcium fluoride |
CCP | Charge-conversional polymer |
CDHA | Calcium-deficient hydroxyapatite |
Ce6 | Chlorin e6 |
CPT | Camptothecin |
CT | X-ray computed tomography |
CUR | Curcumin |
DCPD | Dicalcium phosphate dihydrate |
DDS | Drug delivery system |
DOPA | 3,4-Dihydroxy-L-phenylalanine |
DOPC | Dioleoylphosphatidylcholine |
DOTAP | 1,2-Dioleoyl-3-trimethylammonium-propane |
DOX | Doxorubicin |
DOX-CaP-PM | DOX-loaded mineralized micelles |
DOX-NPM | DOX-loaded nonmineralized micelles |
DPM | Di(ethylenediamineplatinum)medronate |
DTPA | Diethylenetriaminepentaacetic acid |
DTX | Docetaxel |
EPR | Enhanced permeability and retention |
FA | Folic acid |
FAP | Fluorapatite |
FLI | Fluorescence imaging |
FR | Folate receptors |
FRET | Fluorescence resonance energy transfer |
GdNCT | Gd neutron capture therapy |
HA | Hyaluronic acid |
HAP | Hydroxyapatite |
Hb | Hemoglobin |
HTT | Hyperthermia therapy |
HUMSCs | Human umbilical cord mesenchymal stem cells |
IBU | Ibuprofen |
ICG | Indocyanine green |
iPSCs | Induced pluripotent stem cells |
JNPs | Janus nanoparticles |
K
sp
| Thermodynamic solubility product |
LbL | Layer-by-layer |
LCP | Lipid coated CaPs |
LOD | Limit of detection |
MCPM | Monocalcium phosphate monohydrate |
MRI | Magnetic resonance imaging |
NCT | Neutron capture therapy |
NIR | Near-infrared |
NPs | Nanoparticles |
NRs | Nanorods |
OCP | Octacalcium phosphate |
PAA | Poly(acrylic acid) |
PAI | Photoacoustic imaging |
PDA | Polydopamine |
PDT | Photodynamic therapy |
PEG | Poly(ethylene glycol) |
PEG–PAsp–PPhe | Poly(ethylene glycol)-b-poly(L-aspartic acid)-b-poly(L-phenylalanine) |
PEI | Polyethylenimine |
PET | Positron emission tomography |
PL | Photoluminescence |
PLG | Poly(lactide-co-glycolide) |
PLGA | Poly(D,L-lactide-co-glycolide acid) |
PS | Photosensitizer |
PTT | Photothermal therapy |
QDs | Quantum dots |
RE | Rare earth |
ROS | Reactive oxygen species |
RT | Radiation therapy |
RVG | Rabies virus glycoprotein |
SPECT | Single photon emission computer tomography |
TR | Time-resolved |
UCL | Upconversion luminescence |
UCNPs | Upconversion nanoparticles |
UECAs | Ultrasound enhanced contrast agents |
uPAR | Urokinase-type plasminogen activator receptor |
USI | Ultrasound imaging |
UV | Ultraviolet |
Conflicts of interest
There are no conflicts to declare.
Acknowledgements
This work is financially supported by the startup fund from Shenzhen University, the National Science Foundation of China (51573096, 81401465, 51703132, 31771036), the Basic Research Program of Shenzhen (JCYJ20170412111100742, JCYJ20160422091238319), the Postdoctoral Science Foundation of China (2017M612727), and the National Postdoctoral Program for Innovative Talents (BX201600111).
Notes and references
- G. W. Zamponi, Nat. Rev. Drug Discovery, 2016, 15, 19–34 CrossRef CAS PubMed
.
- D. J. Posson, R. Rusinova, O. S. Andersen and C. M. Nimigean, Nat. Commun., 2015, 6, 8342 CrossRef CAS PubMed
.
- S. Chumnarnsilpa, R. C. Robinson, J. M. Grimes and C. Leyrat, Nat. Commun., 2015, 6, 8254 CrossRef CAS PubMed
.
- H. Guo, P. Feng, W. Chi, X. Sun, X. Xu, Y. Li, D. Ren, C. Lu, J. David Rochaix, D. Leister and L. Zhang, Nat. Commun., 2016, 7, 12173 CrossRef PubMed
.
- W. Habraken, P. Habibovic, M. Epple and M. Bohner, Mater. Today, 2016, 19, 69–87 CrossRef CAS
.
- M. Zhang and K. Kataoka, Nano Today, 2009, 4, 508–517 CrossRef CAS
.
- M. Epple, K. Ganesan, R. Heumann, J. Klesing, A. Kovtun, S. Neumann and V. Sokolova, J. Mater. Chem., 2010, 20, 18–23 RSC
.
- S. Sharma, A. Verma, B. V. Teja, G. Pandey, N. Mittapelly, R. Trivedi and P. R. Mishra, Colloids Surf., B, 2015, 133, 120–139 CrossRef CAS PubMed
.
- Y.-J. Zhu and T.-K. Sham, Expert Opin. Drug Delivery, 2014, 11, 1337–1342 CrossRef CAS PubMed
.
- Y.-J. Zhu, X.-X. Guo and T.-K. Sham, Expert Opin. Drug Delivery, 2017, 14, 215–228 CrossRef CAS PubMed
.
- A. M. Pietak, J. W. Reid, M. J. Stott and M. Sayer, Biomaterials, 2007, 28, 4023–4032 CrossRef CAS PubMed
.
- S. V. Dorozhkin, Biomaterials, 2010, 31, 1465–1485 CrossRef CAS PubMed
.
- K. Eldesoqi, C. Seebach, C. Nguyen Ngoc, S. Meier, C. Nau, A. Schaible, I. Marzi and D. Henrich, PLoS One, 2013, 8, e79058 Search PubMed
.
- M. P. Ginebra, C. Canal, M. Espanol, D. Pastorino and E. B. Montufar, Adv. Drug Delivery Rev., 2012, 64, 1090–1110 CrossRef CAS PubMed
.
- J. Zhang, W. Liu, V. Schnitzler, F. Tancret and J. M. Bouler, Acta Biomater., 2014, 10, 1035–1049 CrossRef CAS PubMed
.
- C. M. Curtin, G. M. Cunniffe, F. G. Lyons, K. Bessho, G. R. Dickson, G. P. Duffy and F. J. O'Brien, Adv. Mater., 2012, 24, 749–754 CrossRef CAS PubMed
.
- J. A. Inzana, D. Olvera, S. M. Fuller, J. P. Kelly, O. A. Graeve, E. M. Schwarz, S. L. Kates and H. A. Awad, Biomaterials, 2014, 35, 4026–4034 CrossRef CAS PubMed
.
- T. T. Morgan, T. M. Goff and J. H. Adair, Nanoscale, 2011, 3, 2044–2053 RSC
.
- H. S. Muddana, T. T. Morgan, J. H. Adair and P. J. Butler, Nano Lett., 2009, 9, 1559–1566 CrossRef CAS PubMed
.
- T. T. Morgan, H. S. Muddana, E. İ. Altınoğlu, S. M. Rouse, A. Tabaković, T. Tabouillot, T. J. Russin, S. S. Shanmugavelandy, P. J. Butler, P. C. Eklund, J. K. Yun, M. Kester and J. H. Adair, Nano Lett., 2008, 8, 4108–4115 CrossRef CAS PubMed
.
- E. İ. Altınoğlu, T. J. Russin, J. M. Kaiser, B. M. Barth, P. C. Eklund, M. Kester and J. H. Adair, ACS Nano, 2008, 2, 2075–2084 CrossRef PubMed
.
- B. M. Barth, R. Sharma, E. İ. Altınoğlu, T. T. Morgan, S. S. Shanmugavelandy, J. M. Kaiser, C. McGovern, G. L. Matters, J. P. Smith, M. Kester and J. H. Adair, ACS Nano, 2010, 4, 1279–1287 CrossRef CAS PubMed
.
- A. Ashokan, P. Chandran, A. R. Sadanandan, C. K. Koduri, A. P. Retnakumari, D. Menon, S. Nair and M. Koyakutty, Nanotoxicology, 2012, 6, 652–666 CrossRef CAS PubMed
.
- A. Ashokan, G. S. Gowd, V. H. Somasundaram, A. Bhupathi, R. Peethambaran, A. K. Unni, S. Palaniswamy, S. V. Nair and M. Koyakutty, Biomaterials, 2013, 34, 7143–7157 CrossRef CAS PubMed
.
- L. Li, L. Zhang, T. Wang, X. Wu, H. Ren, C. Wang and Z. Su, Small, 2015, 11, 3162–3173 CrossRef CAS PubMed
.
- J. Kang, J. Yang, J. Lee, S. J. Oh, S. Moon, H. J. Lee, S. C. Lee, J.-H. Son, D. Kim, K. Lee, J.-S. Suh, Y.-M. Huh and S. Haam, J. Mater. Chem., 2009, 19, 2902–2905 RSC
.
- P. Shi, K. Qu, J. Wang, M. Li, J. Ren and X. Qu, Chem. Commun., 2012, 48, 7640–7642 RSC
.
- C. Huang, Y. Zhou, Z. Tang, X. Guo, Z. Qian and S. Zhou, Dalton Trans., 2011, 40, 5026–5031 RSC
.
- H. Liu, J. Wu, J. H. Min, P. Hou, A. Y. Song and Y. K. Kim, Nanotechnology, 2011, 22, 055701 CrossRef PubMed
.
- T. Y. Liu, H. H. Huang, Y. J. Chen and Y. J. Chen, Acta Biomater., 2011, 7, 578–584 CrossRef CAS PubMed
.
- T. Y. Liu and T. C. Huang, Acta Biomater., 2011, 7, 3927–3934 CrossRef CAS PubMed
.
- K. Roy, R. K. Kanwar and J. R. Kanwar, Biomaterials, 2015, 71, 84–99 CrossRef CAS PubMed
.
- R. Zhou, M. Li, S. Wang, P. Wu, L. Wu and X. Hou, Nanoscale, 2014, 6, 14319–14325 RSC
.
- W.-Y. Cai, L.-D. Feng, S.-H. Liu and J.-J. Zhu, Adv. Funct. Mater., 2008, 18, 3127–3136 CrossRef CAS
.
- V. Lauth, B. Loretz, C.-M. Lehr, M. Maas and K. Rezwan, Chem. Mater., 2016, 28, 3796–3803 CrossRef CAS
.
- S. Guo, M. Yang, M. Chen, J. Zhang, K. Liu, L. Ye and W. Gu, Dalton Trans., 2015, 44, 8232–8237 RSC
.
- A. Lebugle, F. Pellé, C. Charvillat, I. Rousselot and J. Y. Chane-Ching, Chem. Commun., 2006, 606–608 RSC
.
- S. P. Mondéjar, A. Kovtun and M. Epple, J. Mater. Chem., 2007, 17, 4153–4159 RSC
.
- J. Hui, X. Zhang, Z. Zhang, S. Wang, L. Tao, Y. Wei and X. Wang, Nanoscale, 2012, 4, 6967–6970 RSC
.
- X. Zhang, J. Hui, B. Yang, Y. Yang, D. Fan, M. Liu, L. Tao and Y. Wei, Polym. Chem., 2013, 4, 4120–4125 RSC
.
- H. Zeng, X. Li, F. Xie, L. Teng and H. Chen, J. Mater. Chem. B, 2014, 2, 3609–3617 RSC
.
- P. Mi, D. Kokuryo, H. Cabral, H. Wu, Y. Terada, T. Saga, I. Aoki, N. Nishiyama and K. Kataoka, Nat. Nanotechnol., 2016, 11, 724–730 CrossRef CAS PubMed
.
- P. Mi, D. Kokuryo, H. Cabral, M. Kumagai, T. Nomoto, I. Aoki, Y. Terada, A. Kishimura, N. Nishiyama and K. Kataoka, J. Controlled Release, 2014, 174, 63–71 CrossRef CAS PubMed
.
- Y. Liu, Y. Sun, C. Cao, Y. Yang, Y. Wu, D. Ju and F. Li, Biomaterials, 2014, 35, 3348–3355 CrossRef CAS PubMed
.
- Z. Li, Z. Liu, M. Yin, X. Yang, Q. Yuan, J. Ren and X. Qu, Biomacromolecules, 2012, 13, 4257–4263 CrossRef CAS PubMed
.
- X. Zheng, M. Liu, J. Hui, D. Fan, H. Ma, X. Zhang, Y. Wang and Y. Wei, Phys. Chem. Chem. Phys., 2015, 17, 20301–20307 RSC
.
- B. Ma, S. Zhang, J. Qiu, J. Li, Y. Sang, H. Xia, H. Jiang, J. Claverie and H. Liu, Nanoscale, 2016, 8, 11580–11587 RSC
.
- Y. Xie, W. He, F. Li, T. S. Perera, L. Gan, Y. Han, X. Wang, S. Li and H. Dai, ACS Appl. Mater. Interfaces, 2016, 8, 10212–10219 CAS
.
- A. Doat, M. Fanjul, F. Pellé, E. Hollande and A. Lebugle, Biomaterials, 2003, 24, 3365–3371 CrossRef CAS PubMed
.
- A. Escudero, M. E. Calvo, S. Rivera-Fernandez, J. M. de la Fuente and M. Ocana, Langmuir, 2013, 29, 1985–1994 CrossRef CAS PubMed
.
- J. Pan, D. Wan, Y. Bian, H. Sun, C. Zhang, F. Jin, Z. Huang and J. Gong, AIChE J., 2013, 59, 4494–4501 CrossRef CAS
.
- R. Sun, K. Chen, X. Wu, D. Zhao and Z. Sun, CrystEngComm, 2013, 15, 3442–3447 RSC
.
- D. E. Wagner, K. M. Eisenmann, A. L. Nestor-Kalinoski and S. B. Bhaduri, Acta Biomater., 2013, 9, 8422–8432 CrossRef CAS PubMed
.
- M. H. Chen, T. Yoshioka, T. Ikoma, N. Hanagata, F. H. Lin and J. Tanaka, Sci. Technol. Adv. Mater., 2014, 15, 055005 CrossRef PubMed
.
- Y. Xie, T. S. Perera, F. Li, Y. Han and M. Yin, ACS Appl. Mater. Interfaces, 2015, 7, 23819–23823 CAS
.
- D. Wan, W. Liu, L. Wang, H. Wang and J. Pan, Nanotechnology, 2016, 27, 105703 CrossRef PubMed
.
- H. Liu, F. Chen, P. Xi, B. Chen, L. Huang, J. Cheng, C. Shao, J. Wang, D. Bai and Z. Zeng, J. Phys. Chem. C, 2011, 115, 18538–18544 CAS
.
- I. Roy, A. Bhardwaj, J. C. Joshi, A. Ray and K. Gulati, RSC Adv., 2014, 4, 40449–40455 RSC
.
- F. Oltolina, L. Gregoletto, D. Colangelo, J. Gomez-Morales, J. M. Delgado-Lopez and M. Prat, Langmuir, 2015, 31, 1766–1775 CrossRef CAS PubMed
.
- Z. Wang, Y. Liu, J. Jia, S. Chen, W. Qin, Q. Hu and B. Z. Tang, J. Mater. Chem. B, 2016, 4, 5265–5271 RSC
.
- Y. Guo, D. Shi, J. Lian, Z. Dong, W. Wang, H. Cho, G. Liu, L. Wang and R. C. Ewing, Nanotechnology, 2008, 19, 175102 CrossRef PubMed
.
- Y. Zhao, L. Shi, J. Fang and X. Feng, Nanoscale, 2015, 7, 20033–20041 RSC
.
- S. Guo, S. Lu, P. Xu, Y. Ma, L. Zhao, Y. Zhao, W. Gu and M. Xue, Dalton Trans., 2016, 45, 7665–7671 RSC
.
- K. Deshmukh, M. M. Shaik, S. R. Ramanan and M. Kowshik, ACS Biomater. Sci. Eng., 2016, 2, 1257–1264 CrossRef CAS
.
- S. Yao, Y. Zhang, J. Zhang, X. Zhang, B. Li and Y. Zhao, J. Mater. Chem. B, 2014, 2, 7988–7995 RSC
.
- S. S. Syamchand, S. Priya and G. Sony, Microchim. Acta, 2014, 182, 1213–1221 CrossRef
.
- Y. C. Tseng, Z. Xu, K. Guley, H. Yuan and L. Huang, Biomaterials, 2014, 35, 4688–4698 CrossRef CAS PubMed
.
- A. K. Sanchez Lafarga, F. P. Pacheco Moises, A. Gurinov, G. G. Ortiz and G. G. Carbajal Arizaga, Mater. Sci. Eng., C, 2015, 48, 541–547 CrossRef CAS PubMed
.
- H. Zhang, X. Qu, H. Chen, H. Kong, R. Ding, D. Chen, X. Zhang, H. Pei, H. A. Santos, M. Hai and D. A. Weitz, Adv. Healthcare Mater., 2017, 6, 1700664 CrossRef PubMed
.
- M. Lelli, N. Roveri, C. Marzano, J. D. Hoeschele, A. Curci, N. Margiotta, V. Gandin and G. Natile, Dalton Trans., 2016, 45, 13187–13195 RSC
.
- M. Iafisco, B. Palazzo, M. Marchetti, N. Margiotta, R. Ostuni, G. Natile, M. Morpurgo, V. Gandin, C. Marzano and N. Roveri, J. Mater. Chem., 2009, 19, 8385–8392 RSC
.
- Q. He, L. Pan, Y. Wang and F. C. Meldrum, Cryst. Growth Des., 2015, 15, 723–731 CAS
.
- Y. J. Shyong, M. H. Wang, H. C. Tseng, C. Cheng, K. C. Chang and F. H. Lin, J. Med. Chem., 2015, 58, 8463–8474 CrossRef CAS PubMed
.
- I. Rodriguez-Ruiz, J. M. Delgado-Lopez, M. A. Duran-Olivencia, M. Iafisco, A. Tampieri, D. Colangelo, M. Prat and J. Gomez-Morales, Langmuir, 2013, 29, 8213–8221 CrossRef CAS PubMed
.
- D. Li, J. He, W. Cheng, Y. Wu, Z. Hu, H. Tian and Y. Huang, J. Mater. Chem. B, 2014, 2, 6089–6096 RSC
.
- B. Palazzo, M. Iafisco, M. Laforgia, N. Margiotta, G. Natile, C. L. Bianchi, D. Walsh, S. Mann and N. Roveri, Adv. Funct. Mater., 2007, 17, 2180–2188 CrossRef CAS
.
- M. Iafisco, B. Palazzo, G. Martra, N. Margiotta, S. Piccinonna, G. Natile, V. Gandin, C. Marzano and N. Roveri, Nanoscale, 2012, 4, 206–217 RSC
.
- X. Cheng and L. Kuhn, Int. J. Nanomed., 2007, 2, 667–674 CAS
.
- C. W. Kim, Y. P. Yun, H. J. Lee, Y. S. Hwang, I. K. Kwon and S. C. Lee, J. Controlled Release, 2010, 147, 45–53 CrossRef CAS PubMed
.
- S. Koocheki, S. S. Madaeni and P. Niroomandi, Int. J. Nanomed., 2011, 6, 825–833 CAS
.
- G. Li, S. Dong, J. Qu, Z. Sun, Z. Huang, L. Ye, H. Liang, X. Ai, W. Zhang and X. Chen, Liver Int., 2010, 30, 585–592 CrossRef CAS PubMed
.
- C. Qi, Y.-J. Zhu, X.-Y. Zhao, J. Zhao, F. Chen, G.-F. Cheng and Y.-J. Ruan, Mater. Res. Bull., 2013, 48, 1536–1540 CrossRef CAS
.
- C. Qi, Y.-J. Zhu, Y.-G. Zhang, Y.-Y. Jiang, J. Wu and F. Chen, J. Mater. Chem. B, 2015, 3, 7347–7354 RSC
.
- Y. Cai, H. Pan, X. Xu, Q. Hu, L. Li and R. Tang, Chem. Mater., 2007, 19, 3081–3083 CrossRef CAS
.
- G. J. Ding, Y. J. Zhu, C. Qi, B. Q. Lu, J. Wu and F. Chen, J. Colloid Interface Sci., 2015, 443, 72–79 CrossRef CAS PubMed
.
- C. Qi, Y. J. Zhu, X. Y. Zhao, B. Q. Lu, Q. L. Tang, J. Zhao and F. Chen, Chem. – Eur. J., 2013, 19, 981–987 CrossRef CAS PubMed
.
- C. Qi, Y. J. Zhu and F. Chen, Chem. – Asian J., 2013, 8, 88–94 CrossRef CAS PubMed
.
- R. Vani, S. B. Raja, T. S. Sridevi, K. Savithri, S. N. Devaraj, E. K. Girija, A. Thamizhavel and S. N. Kalkura, Nanotechnology, 2011, 22, 285701 CrossRef CAS PubMed
.
- K. W. Wang, Y. J. Zhu, X. Y. Chen, W. Y. Zhai, Q. Wang, F. Chen, J. Chang and Y. R. Duan, Chem. – Asian J., 2010, 5, 2477–2482 CrossRef CAS PubMed
.
- M. Filippousi, P. I. Siafaka, E. P. Amanatiadou, S. G. Nanaki, M. Nerantzaki, D. N. Bikiaris, I. S. Vizirianakis and G. Van Tendeloo, J. Mater. Chem. B, 2015, 3, 5991–6000 RSC
.
- N. Abbasi Aval, J. Pirayesh Islamian, M. Hatamian, M. Arabfirouzjaei, J. Javadpour and M. R. Rashidi, Int. J. Pharm., 2016, 509, 159–167 CrossRef CAS PubMed
.
- M. Akrami, M. Khoobi, M. Khalilvand-Sedagheh, I. Haririan, A. Bahador, M. A. Faramarzi, S. Rezaei, H. A. Javar, F. Salehi, S. K. Ardestani and A. Shafiee, RSC Adv., 2015, 5, 88096–88107 RSC
.
- S. R. Rout, B. Behera, T. K. Maiti and S. Mohapatra, Dalton Trans., 2012, 41, 10777–10783 RSC
.
- B. Q. Lu, Y. J. Zhu, F. Chen, C. Qi, X. Y. Zhao and J. Zhao, Chem. – Asian J., 2014, 9, 2908–2914 CrossRef CAS PubMed
.
- Z. Tang, Y. Zhou, H. Sun, D. Li and S. Zhou, Eur. J. Pharm. Sci., 2014, 87, 90–100 CAS
.
- Z. Chen, Z. Li, Y. Lin, M. Yin, J. Ren and X. Qu, Biomaterials, 2013, 34, 1364–1371 CrossRef CAS PubMed
.
- H. S. Han, J. Lee, H. R. Kim, S. Y. Chae, M. Kim, G. Saravanakumar, H. Y. Yoon, D. G. You, H. Ko, K. Kim, I. C. Kwon, J. C. Park and J. H. Park, J. Controlled Release, 2013, 168, 105–114 CrossRef CAS PubMed
.
- K. H. Min, H. J. Lee, K. Kim, I. C. Kwon, S. Y. Jeong and S. C. Lee, Biomaterials, 2012, 33, 5788–5797 CrossRef CAS PubMed
.
- P. Venkatesan, N. Puvvada, R. Dash, B. N. Prashanth Kumar, D. Sarkar, B. Azab, A. Pathak, S. C. Kundu, P. B. Fisher and M. Mandal, Biomaterials, 2011, 32, 3794–3806 CrossRef CAS PubMed
.
- M. Iafisco, J. M. Delgado-Lopez, E. M. Varoni, A. Tampieri, L. Rimondini, J. Gomez-Morales and M. Prat, Small, 2013, 9, 3834–3844 CrossRef CAS PubMed
.
- H. Xiong, S. Du, J. Ni, J. Zhou and J. Yao, Biomaterials, 2016, 94, 70–83 CrossRef CAS PubMed
.
- Y. H. Liang, C. H. Liu, S. H. Liao, Y. Y. Lin, H. W. Tang, S. Y. Liu, I. R. Lai and K. C.-W. Wu, ACS Appl. Mater. Interfaces, 2012, 4, 6720–6727 CAS
.
- Y.-H. Yang, C.-H. Liu, Y.-H. Liang, F.-H. Lin and K. C. W. Wu, J. Mater. Chem. B, 2013, 1, 2447–2450 RSC
.
- Y. Lv, H. Huang, B. Yang, H. Liu, Y. Li and J. Wang, Carbohydr. Polym., 2014, 111, 101–107 CrossRef CAS PubMed
.
- H. Shi, Q. Cheng, S. Yuan, X. Ding and Y. Liu, Chem. – Eur. J., 2015, 21, 16547–16554 CrossRef CAS PubMed
.
- U. Mukesh, V. Kulkarni, R. Tushar and R. S. R. Murthy, J. Biomed. Nanotechnol., 2009, 5, 99–105 CrossRef CAS PubMed
.
- S. W. Cao, Y. J. Zhu, J. Wu, K. W. Wang and Q. L. Tang, Nanoscale Res. Lett., 2010, 5, 781–785 CrossRef CAS PubMed
.
- H. J. Lee, S. E. Kim, I. K. Kwon, C. Park, C. Kim, J. Yang and S. C. Lee, Chem. Commun., 2010, 46, 377–379 RSC
.
- Y. Luo, Y. Ling, W. Guo, J. Pang, W. Liu, Y. Fang, X. Wen, K. Wei and X. Gao, J. Controlled Release, 2010, 147, 278–288 CrossRef CAS PubMed
.
- Q. L. Tang, Y. J. Zhu, Y. R. Duan, Q. Wang, K. W. Wang, S. W. Cao, F. Chen and J. Wu, Dalton Trans., 2010, 39, 4435–4439 RSC
.
- F. Ye, H. Guo, H. Zhang and X. He, Acta Biomater., 2010, 6, 2212–2218 CrossRef CAS PubMed
.
- S.-Y. Han, H. S. Han, S. C. Lee, Y. M. Kang, I.-S. Kim and J. H. Park, J. Mater. Chem., 2011, 21, 7996–8001 RSC
.
- H. P. Rim, K. H. Min, H. J. Lee, S. Y. Jeong and S. C. Lee, Angew. Chem., Int. Ed., 2011, 50, 8853–8857 CrossRef CAS PubMed
.
- Q. L. Tang, Y. J. Zhu, J. Wu, F. Chen and S. W. Cao, Nanomedicine, 2011, 7, 428–434 CrossRef CAS PubMed
.
- V. S. Chandra, G. Baskar, R. V. Suganthi, K. Elayaraja, M. I. Joshy, W. S. Beaula, R. Mythili, G. Venkatraman and S. N. Kalkura, ACS Appl. Mater. Interfaces, 2012, 4, 1200–1210 Search PubMed
.
- J. R. Kanwar, G. Mahidhara and R. K. Kanwar, Nanomedicine, 2012, 7, 1521–1550 CrossRef CAS PubMed
.
- Y. Sun, X.-Y. Chen, Y.-J. Zhu, P.-F. Liu, M.-J. Zhu and Y.-R. Duan, J. Mater. Chem., 2012, 22, 5128–5136 RSC
.
- X. Y. Zhao, Y. J. Zhu, F. Chen and J. Wu, Chem. – Asian J., 2012, 7, 1610–1615 CrossRef CAS PubMed
.
- L. Chen, Y. Ding, Y. Wang, X. Liu, R. Babu, W. Ravis and W. Yan, Int. J. Nanomed., 2013, 8, 137–145 Search PubMed
.
- J. J. Khandare, A. Jalota-Badhwar, N. Taneja, R. R. Mascarenhas, K. Vadodaria, K. R. Zope and S. S. Banerjee, Part. Part. Syst. Charact., 2013, 30, 494–500 CrossRef CAS
.
- B. Kundu, D. Ghosh, M. K. Sinha, P. S. Sen, V. K. Balla, N. Das and D. Basu, Ceram. Int., 2013, 39, 9557–9566 CrossRef CAS
.
- J. Wang, B. Chen, D. Zhao, Y. Peng, R. X. Zhuo and S. X. Cheng, Int. J. Pharm., 2013, 446, 205–210 CrossRef CAS PubMed
.
- K. Watanabe, Y. Nishio, R. Makiura, A. Nakahira and C. Kojima, Int. J. Pharm., 2013, 446, 81–86 CrossRef CAS PubMed
.
- J. Yao, Y. Zhang, S. Ramishetti, Y. Wang and L. Huang, J. Controlled Release, 2013, 170, 414–420 CrossRef CAS PubMed
.
- Y. Zhang, W. Y. Kim and L. Huang, Biomaterials, 2013, 34, 3447–3458 CrossRef CAS PubMed
.
- Y. Zhang, L. Peng, R. J. Mumper and L. Huang, Biomaterials, 2013, 34, 8459–8468 CrossRef CAS PubMed
.
- K. Hu, L. Miao, T. J. Goodwin, J. Li, Q. Liu and L. Huang, ACS Nano, 2017, 11, 4916–4925 CrossRef CAS PubMed
.
- C.-X. Zhao, L. Yu and A. P. J. Middelberg, J. Mater. Chem. B, 2013, 1, 4828–4833 RSC
.
- X. Y. Zhao, Y. J. Zhu, F. Chen, B. Q. Lu, C. Qi, J. Zhao and J. Wu, Chem. – Asian J., 2013, 8, 1306–1312 CrossRef CAS PubMed
.
- X. Y. Zhao, Y. J. Zhu, C. Qi, F. Chen, B. Q. Lu, J. Zhao and J. Wu, Chem. – Asian J., 2013, 8, 1313–1320 CrossRef CAS PubMed
.
- F. Zheng, S. Wang, M. Shen, M. Zhu and X. Shi, Polym. Chem., 2013, 4, 933–941 RSC
.
- G. S. Kumar, R. Govindan and E. K. Girija, J. Mater. Chem. B, 2014, 2, 5052 RSC
.
- R. M. Samarasinghe, R. K. Kanwar, K. Kumar and J. R. Kanwar, Nanomedicine, 2014, 9, 819–837 CrossRef CAS PubMed
.
- S. S. Banerjee, K. J. Todkar, G. V. Khutale, G. P. Chate, A. V. Biradar, M. B. Gawande, R. Zboril and J. J. Khandare, J. Mater. Chem. B, 2015, 3, 3931–3939 RSC
.
- T. S. P. Cellet, G. M. Pereira, E. C. Muniz, R. Silva and A. F. Rubira, J. Mater. Chem. B, 2015, 3, 6837–6846 RSC
.
- G.-J. Ding, Y.-J. Zhu, C. Qi, B.-Q. Lu, F. Chen and J. Wu, J. Mater. Chem. B, 2015, 3, 1823–1830 RSC
.
- X. Hao, X. Hu, C. Zhang, S. Chen, Z. Li, X. Yang, H. Liu, G. Jia, D. Liu, K. Ge, X.-J. Liang and J. Zhang, ACS Nano, 2015, 9, 9614–9625 CrossRef CAS PubMed
.
- W. M. Li, C. W. Su, Y. W. Chen and S. Y. Chen, Acta Biomater., 2015, 15, 191–199 CrossRef CAS PubMed
.
- W. M. Li, C. S. Chiang, W. C. Huang, C. W. Su, M. Y. Chiang, J. Y. Chen and S. Y. Chen, J. Controlled Release, 2015, 220, 107–118 CrossRef CAS PubMed
.
- S. Xu, J. Shi, D. Feng, L. Yang and S. Cao, J. Mater. Chem. B, 2014, 2, 6500–6507 RSC
.
- J. Wei, J. Shi, Q. Wu, L. Yang and S. Cao, J. Mater. Chem. B, 2015, 3, 8162–8169 RSC
.
- Q. Wu, J. Shi, J. Wei, L. Yang and S. Cao, RSC Adv., 2015, 5, 70101–70108 RSC
.
- E. Campodoni, A. Adamiano, S. M. Dozio, S. Panseri, M. Montesi, S. Sprio, A. Tampieri and M. Sandri, Nanomedicine, 2016, 11, 2119–2130 CrossRef CAS PubMed
.
- Y. Guo, Q. Fang, H. Li, W. Shi, J. Zhang, J. Feng, W. Jia and L. Yang, Chem. Commun., 2016, 52, 10652–10655 RSC
.
- M. Iafisco, C. Drouet, A. Adamiano, P. Pascaud, M. Montesi, S. Panseri, S. Sarda and A. Tampieri, J. Mater. Chem. B, 2016, 4, 57–70 RSC
.
- Y. P. Guo, L. H. Guo, Y. B. Yao, C. Q. Ning and Y. J. Guo, Chem. Commun., 2011, 47, 12215–12217 RSC
.
- Y.-P. Guo, Y.-B. Yao, Y.-J. Guo and C.-Q. Ning, Microporous Mesoporous Mater., 2012, 155, 245–251 CrossRef CAS
.
- Y.-P. Guo, T. Long, S. Tang, Y.-J. Guo and Z.-A. Zhu, J. Mater. Chem. B, 2014, 2, 2899–2909 RSC
.
- C. Qi, Y.-J. Zhu, F. Chen and J. Wu, J. Mater. Chem. B, 2015, 3, 7775–7786 RSC
.
- C. Qi, Y. J. Zhu, B. Q. Lu, X. Y. Zhao, J. Zhao and F. Chen, J. Mater. Chem., 2012, 22, 22642–22650 RSC
.
- G. Zhu, Y. Hu, Y. Yang, R. Zhao and R. Tang, RSC Adv., 2015, 5, 23958–23964 RSC
.
- G. J. Ding, Y. J. Zhu, C. Qi, T. W. Sun, J. Wu and F. Chen, Chem. – Eur. J., 2015, 21, 9868–9876 CrossRef CAS PubMed
.
- C. Qi, Y. J. Zhu, B. Q. Lu, X. Y. Zhao, J. Zhao, F. Chen and J. Wu, Chem. – Eur. J., 2013, 19, 5332–5341 CrossRef CAS PubMed
.
- X. Chen, T. Wu, Q. Wang and J. W. Shen, Biomaterials, 2008, 29, 2423–2432 CrossRef CAS PubMed
.
- S. Dasgupta, A. Bandyopadhyay and S. Bose, Acta Biomater., 2009, 5, 3112–3121 CrossRef CAS PubMed
.
- X. Chen, Q. Wang, J. Shen, H. Pan and T. Wu, J. Phys. Chem. C, 2007, 111, 1284–1290 CAS
.
- K. Kandori, T. Kuroda, S. Togashi and E. Katayama, J. Phys. Chem. B, 2011, 115, 653–659 CrossRef CAS PubMed
.
- M. Iafisco, M. Di Foggia, S. Bonora, M. Prat and N. Roveri, Dalton Trans., 2011, 40, 820–827 RSC
.
- A. J. Khopade, S. Khopade and N. K. Jain, Int. J. Pharm., 2002, 241, 145–154 CrossRef CAS PubMed
.
- T. Y. Liu, S. Y. Chen, D. M. Liu and S. C. Liou, J. Controlled Release, 2005, 107, 112–121 CrossRef CAS PubMed
.
- I. Y. Saleem, M. Vordermeier, J. E. Barralet and A. G. Coombes, J. Controlled Release, 2005, 102, 551–561 CrossRef CAS PubMed
.
- S. Dasgupta, S. S. Banerjee, A. Bandyopadhyay and S. Bose, Langmuir, 2010, 26, 4958–4964 CrossRef CAS PubMed
.
- S. Ng, J. Guo, J. Ma and S. C. J. Loo, Acta Biomater., 2010, 6, 3772–3781 CrossRef CAS PubMed
.
- S. P. Victor and C. P. Sharma, Colloids Surf., B, 2011, 85, 221–228 CrossRef CAS PubMed
.
- M. Iafisco, E. Varoni, M. Di Foggia, S. Pietronave, M. Fini, N. Roveri, L. Rimondini and M. Prat, Colloids Surf., B, 2012, 90, 1–7 CrossRef CAS PubMed
.
- P. J. Reardon, J. Huang and J. Tang, Adv. Healthcare Mater., 2013, 2, 682–686 CrossRef CAS PubMed
.
- Z. Xu, S. Ramishetti, Y. C. Tseng, S. Guo, Y. Wang and L. Huang, J. Controlled Release, 2013, 172, 259–265 CrossRef CAS PubMed
.
- Z. Xu, Y. Wang, L. Zhang and L. Huang, ACS Nano, 2014, 8, 3636–3645 CrossRef CAS PubMed
.
- Y. Zhao, M. Huo, Z. Xu, Y. Wang and L. Huang, Biomaterials, 2015, 68, 54–66 CrossRef CAS PubMed
.
- C. Qi, Y. J. Zhu and F. Chen, ACS Appl. Mater. Interfaces, 2014, 6, 4310–4320 CAS
.
- A. Salama and M. El-Sakhawy, Carbohydr. Polym., 2014, 113, 500–506 CrossRef CAS PubMed
.
- T. Lan, Z.-q. Shao, J.-q. Wang and M.-j. Gu, Chem. Eng. J., 2015, 260, 818–825 CrossRef CAS
.
- Y. Wang, H. Hao and S. Zhang, Mater. Sci. Eng., C, 2016, 61, 545–552 CrossRef CAS PubMed
.
- N. Nocerino, A. Fulgione, M. Iannaccone, L. Tomasetta, F. Ianniello, F. Martora, M. Lelli, N. Roveri, F. Capuano and R. Capparelli, Int. J. Nanomed., 2014, 9, 1175–1184 Search PubMed
.
- P. Das and N. R. Jana, ACS Appl. Mater. Interfaces, 2016, 8, 8710–8720 CAS
.
- M. Iafisco, P. Sabatino, I. G. Lesci, M. Prat, L. Rimondini and N. Roveri, Colloids Surf., B, 2010, 81, 274–284 CrossRef CAS PubMed
.
- M. Tagaya, T. Ikoma, N. Hanagata, D. Chakarov, B. Kasemo and J. Tanaka, Sci. Technol. Adv. Mater., 2010, 11, 045002 CrossRef PubMed
.
- W. Zhang, Y. Chai, X. Xu, Y. Wang and N. Cao, Appl. Surf. Sci., 2014, 322, 71–77 CrossRef CAS
.
- D. Chiu, W. Zhou, S. Kitayaporn, D. T. Schwartz, K. Murali-Krishna, T. J. Kavanagh and F. Baneyx, Bioconjugate Chem., 2012, 23, 610–617 CrossRef CAS PubMed
.
- C. C. Lau, P. J. T. Reardon, J. C. Knowles and J. Tang, ACS Biomater. Sci. Eng., 2015, 1, 947–954 CrossRef
.
- X. Chen, B. Yang, C. Qi, T. W. Sun, F. Chen, J. Wu, X. P. Feng and Y. J. Zhu, Dalton Trans., 2016, 45, 1648–1656 RSC
.
- F. Chen, B. Yang, C. Qi, T.-W. Sun, Y.-Y. Jiang, J. Wu, X. Chen and Y.-J. Zhu, RSC Adv., 2015, 5, 100682–100688 RSC
.
- X.-Y. Zhao, Y.-J. Zhu, F. Chen, B.-Q. Lu and J. Wu, CrystEngComm, 2013, 15, 206–212 RSC
.
- X.-Y. Zhao, Y.-J. Zhu, F. Chen, B.-Q. Lu, C. Qi, J. Zhao and J. Wu, CrystEngComm, 2013, 15, 7926–7935 RSC
.
- E. Fujii, M. Ohkubo, K. Tsuru, S. Hayakawa, A. Osaka, K. Kawabata, C. Bonhomme and F. Babonneau, Acta Biomater., 2006, 2, 69–74 CrossRef PubMed
.
- C. Qi, Y.-J. Zhu, C.-T. Wu, T.-W. Sun, Y.-Y. Jiang, Y.-G. Zhang, J. Wu and F. Chen, RSC Adv., 2016, 6, 9686–9692 RSC
.
- M. Iafisco, B. Palazzo, G. Falini, M. D. Foggia, S. Bonora, S. Nicolis, L. Casella and N. Roveri, Langmuir, 2008, 24, 4924–4930 CrossRef CAS PubMed
.
- K. Kandori, S. Oda, M. Fukusumi and Y. Morisada, Colloids Surf., B, 2009, 73, 140–145 CrossRef CAS PubMed
.
- V. O. Kollath, F. V. d. Broeck, K. Fehér, J. C. Martins, J. Luyten, K. Traina, S. Mullens and R. Cloots, Chem. – Eur. J., 2015, 21, 10497–10505 CrossRef PubMed
.
- L. Jongpaiboonkit, T. Franklin-Ford and W. L. Murphy, Adv. Mater., 2009, 21, 1960–1963 CrossRef CAS
.
- X. Yu, A. H. Biedrzycki, A. S. Khalil, D. Hess, J. M. Umhoefer, M. D. Markel and W. L. Murphy, Adv. Mater., 2017, 29, 1701255 CrossRef PubMed
.
- W. Chen, G. Wang, B. C. Yung, G. Liu, Z. Qian and X. Chen, ACS Nano, 2017, 11, 5062–5069 CrossRef CAS PubMed
.
- Y. Liu, T. Wang, F. He, Q. Liu, D. Zhang, S. Xiang, S. Su and J. Zhang, Int. J. Nanomed., 2011, 6, 721–727 CrossRef CAS PubMed
.
- V. D. Mauro, M. Iafisco, N. Salvarani, M. Vacchiano, P. Carullo, G. B. Ramírez-Rodríguez, T. Patrício, A. Tampieri, M. Miragoli and D. Catalucci, Nanomedicine, 2016, 11, 891–906 CrossRef PubMed
.
- G. J. Wu, L. Z. Zhou, K. W. Wang, F. Chen, Y. Sun, Y. R. Duan, Y. J. Zhu and H. C. Gu, J. Colloid Interface Sci., 2010, 345, 427–432 CrossRef CAS PubMed
.
- A. Nouri, R. Castro, J. L. Santos, C. Fernandes, J. Rodrigues and H. Tomas, Int. J. Pharm., 2012, 434, 199–208 CrossRef CAS PubMed
.
- G. Zhang, T. Liu, Y. H. Chen, Y. Chen, M. Xu, J. Peng, S. Yu, J. Yuan and X. Zhang, Clin. Cancer Res., 2009, 15, 201–207 CrossRef CAS PubMed
.
- J. Klesing, S. Chernousova, A. Kovtun, S. Neumann, L. Ruiz, J. M. Gonzalez-Calbet, M. Vallet-Regi, R. Heumann and M. Epple, J. Mater. Chem., 2010, 20, 6144–6148 RSC
.
- P. N. Kumta, C. Sfeir, D. H. Lee, D. Olton and D. Choi, Acta Biomater., 2005, 1, 65–83 CrossRef PubMed
.
- Y. Kakizawa, S. Furukawa, A. Ishii and K. Kataoka, J. Controlled Release, 2006, 111, 368–370 CrossRef CAS PubMed
.
- Y. Kakizawa, S. Furukawa and K. Kataoka, J. Controlled Release, 2004, 97, 345–356 CrossRef CAS PubMed
.
- A. Frede, B. Neuhaus, R. Klopfleisch, C. Walker, J. Buer, W. Muller, M. Epple and A. M. Westendorf, J. Controlled Release, 2016, 222, 86–96 CrossRef CAS PubMed
.
- Y. Xie, H. Qiao, Z. Su, M. Chen, Q. Ping and M. Sun, Biomaterials, 2014, 35, 7978–7991 CrossRef CAS PubMed
.
- F. Pittella, K. Miyata, Y. Maeda, T. Suma, S. Watanabe, Q. Chen, R. J. Christie, K. Osada, N. Nishiyama and K. Kataoka, J. Controlled Release, 2012, 161, 868–874 CrossRef CAS PubMed
.
- F. Pittella, M. Zhang, Y. Lee, H. J. Kim, T. Tockary, K. Osada, T. Ishii, K. Miyata, N. Nishiyama and K. Kataoka, Biomaterials, 2011, 32, 3106–3114 CrossRef CAS PubMed
.
- M. S. Lee, J. E. Lee, E. Byun, N. W. Kim, K. Lee, H. Lee, S. J. Sim, D. S. Lee and J. H. Jeong, J. Controlled Release, 2014, 192, 122–130 CrossRef CAS PubMed
.
- D. Kozlova, S. Chernousova, T. Knuschke, J. Buer, A. M. Westendorf and M. Epple, J. Mater. Chem., 2012, 22, 396–404 RSC
.
- C. Qiu, W. Wei, J. Sun, H. T. Zhang, J. S. Ding, J. C. Wang and Q. Zhang, Nanoscale, 2016, 8, 13033–13044 RSC
.
- Y. Hu, M. T. Haynes, Y. Wang, F. Liu and L. Huang, ACS Nano, 2013, 7, 5376–5384 CrossRef CAS PubMed
.
- T. Welzel, I. Radtke, W. Meyer-Zaika, R. Heumann and M. Epple, J. Mater. Chem., 2004, 14, 2213–2217 RSC
.
- S. Bisht, G. Bhakta, S. Mitra and A. Maitra, Int. J. Pharm., 2005, 288, 157–168 CrossRef CAS PubMed
.
- V. Sokolova, A. Kovtun, O. Prymak, W. Meyer-Zaika, E. A. Kubareva, E. A. Romanova, T. S. Oretskaya, R. Heumann and M. Epple, J. Mater. Chem., 2007, 17, 721–727 RSC
.
- D. Olton, J. Li, M. E. Wilson, T. Rogers, J. Close, L. Huang, P. N. Kumta and C. Sfeir, Biomaterials, 2007, 28, 1267–1279 CrossRef CAS PubMed
.
- S. Neumann, A. Kovtun, I. D. Dietzel, M. Epple and R. Heumann, Biomaterials, 2009, 30, 6794–6802 CrossRef CAS PubMed
.
- M. Zhang, A. Ishii, N. Nishiyama, S. Matsumoto, T. Ishii, Y. Yamasaki and K. Kataoka, Adv. Mater., 2009, 21, 3520–3525 CrossRef CAS
.
- J. Li, Y. C. Chen, Y. C. Tseng, S. Mozumdar and L. Huang, J. Controlled Release, 2010, 142, 416–421 CrossRef CAS PubMed
.
- K.-W. Wang, L.-Z. Zhou, Y. Sun, G.-J. Wu, H.-C. Gu, Y.-R. Duan, F. Chen and Y.-J. Zhu, J. Mater. Chem., 2010, 20, 1161–1166 RSC
.
- H.-C. Wu, T.-W. Wang, M. C. Bohn, F.-H. Lin and M. Spector, Adv. Funct. Mater., 2010, 20, 67–77 CrossRef CAS
.
- C. Zhou, B. Yu, X. Yang, T. Huo, L. J. Lee, R. F. Barth and R. J. Lee, Int. J. Pharm., 2010, 392, 201–208 CrossRef CAS PubMed
.
- I. Roy, S. Mitra, A. Maitra and S. Mozumdar, Int. J. Pharm., 2003, 250, 25–33 CrossRef CAS PubMed
.
- X. Cao, W. Deng, Y. Wei, W. Su, Y. Yang, Y. Wei, J. Yu and X. Xu, Int. J. Nanomed., 2011, 6, 3335–3349 CAS
.
- A. Oyane, H. Araki, Y. Sogo, A. Ito and H. Tsurushima, CrystEngComm, 2013, 15, 4994–4997 RSC
.
- M. A. Hassan, I. S. Ahmed, P. Campbell and T. Kondo, Eur. J. Pharm. Sci., 2012, 47, 768–773 CrossRef CAS PubMed
.
- S. Naqvi, A. N. Maitra, M. Z. Abdin, M. Akmal, I. Arora and M. Samim, J. Mater. Chem., 2012, 22, 3500–3507 RSC
.
- S. Hou, H. Ma, Y. Ji, W. Hou and N. Jia, ACS Appl. Mater. Interfaces, 2013, 5, 1131–1136 CAS
.
- E. V. Giger, J. Puigmarti-Luis, R. Schlatter, B. Castagner, P. S. Dittrich and J. C. Leroux, J. Controlled Release, 2011, 150, 87–93 CrossRef CAS PubMed
.
- D. Y. Olton, J. M. Close, C. S. Sfeir and P. N. Kumta, Biomaterials, 2011, 32, 7662–7670 CrossRef CAS PubMed
.
- Q. Cai, Y. Zhu, J. Q. He, Z. H. Wang, F. Su, F. J. Xu, X. P. Yang and W. T. Yang, J. Mater. Chem., 2012, 22, 9358–9367 RSC
.
- J. Klesing, S. Chernousova and M. Epple, J. Mater. Chem., 2012, 22, 199–204 RSC
.
- G. Li, L. Ye, J. Pan, M. Long, Z. Zhao, H. Yang, J. Tian, Y. Wen, S. Dong, J. Guan and B. Luo, Liver Int., 2012, 32, 998–1007 CrossRef CAS PubMed
.
- J. Li, Y. Yang and L. Huang, J. Controlled Release, 2012, 158, 108–114 CrossRef CAS PubMed
.
- J. Tang, J. Y. Chen, J. Liu, M. Luo, Y. J. Wang, X. W. Wei, X. Gao, B. L. Wang, Y. B. Liu, T. Yi, A. P. Tong, X. R. Song, Y. M. Xie, Y. Zhao, M. Xiang, Y. Huang and Y. Zheng, Int. J. Pharm., 2012, 431, 210–221 CrossRef CAS PubMed
.
- X. Cao, W. Deng, R. Qu, Q. Yu, J. Li, Y. Yang, Y. Cao, X. Gao, X. Xu and J. Yu, Adv. Funct. Mater., 2013, 23, 5403–5411 CrossRef CAS
.
- Y. Chen, L. Yang, S. Huang, Z. Li, L. Zhang, J. He, Z. Xu, L. Liu, Y. Cao and L. Sun, Int. J. Nanomed., 2013, 8, 3107–3118 Search PubMed
.
- T. Devarasu, R. Saad, A. Ouadi, B. Frisch, E. Robinet, P. Laquerrière, J.-C. Voegel, T. Baumert, J. Ogier and F. Meyer, J. Mater. Chem. B, 2013, 1, 4692–4700 RSC
.
- E. V. Giger, B. Castagner, J. Raikkonen, J. Monkkonen and J. C. Leroux, Adv. Healthcare Mater., 2013, 2, 134–144 CrossRef CAS PubMed
.
- K. Lee, M. H. Oh, M. S. Lee, Y. S. Nam, T. G. Park and J. H. Jeong, Int. J. Pharm., 2013, 445, 196–202 CrossRef CAS PubMed
.
- B. Mostaghaci, B. Loretz, R. Haberkorn, G. Kickelbick and C.-M. Lehr, Chem. Mater., 2013, 25, 3667–3674 CrossRef CAS
.
- K. Y. Choi, O. F. Silvestre, X. Huang, K. H. Min, G. P. Howard, N. Hida, A. J. Jin, N. Carvajal, S. W. Lee, J.-I. Hong and X. Chen, ACS Nano, 2014, 8, 4559–4570 CrossRef CAS PubMed
.
- L. J. del Valle, O. Bertran, G. Chaves, G. Revilla-López, M. Rivas, M. T. Casas, J. Casanovas, P. Turon, J. Puiggalí and C. Alemán, J. Mater. Chem. B, 2014, 2, 6953–6966 RSC
.
- N. Khatri, D. Baradia, I. Vhora, M. Rathi and A. Misra, J. Controlled Release, 2014, 182, 45–57 CrossRef CAS PubMed
.
- Y. F. Tan, R. C. Mundargi, M. H. Chen, J. Lessig, B. Neu, S. S. Venkatraman and T. T. Wong, Small, 2014, 10, 1790–1798 CrossRef CAS PubMed
.
- B. Choi, Z. K. Cui, S. Kim, J. Fan, B. M. Wu and M. Lee, J. Mater. Chem. B, 2015, 3, 6448–6455 RSC
.
- B. Mostaghaci, J. Susewind, G. Kickelbick, C. M. Lehr and B. Loretz, ACS Appl. Mater. Interfaces, 2015, 7, 5124–5133 CAS
.
- J. Tang, L. Li, C. B. Howard, S. M. Mahler, L. Huang and Z. P. Xu, J. Mater. Chem. B, 2015, 3, 6805–6812 RSC
.
- C. E. Pedraza, D. C. Bassett, M. D. McKee, V. Nelea, U. Gbureck and J. E. Barralet, Biomaterials, 2008, 29, 3384–3392 CrossRef CAS PubMed
.
- V. V. Sokolova, I. Radtke, R. Heumann and M. Epple, Biomaterials, 2006, 27, 3147–3153 CrossRef CAS PubMed
.
- X. Zhang, A. Kovtun, C. Mendoza-Palomares, M. Oulad-Abdelghani, F. Fioretti, S. Rinckenbach, D. Mainard, M. Epple and N. Benkirane-Jessel, Biomaterials, 2010, 31, 6013–6018 CrossRef CAS PubMed
.
- G. Dördelmann, D. Kozlova, S. Karczewski, R. Lizio, S. Knauer and M. Epple, J. Mater. Chem. B, 2014, 2, 7250–7259 RSC
.
- Y. Kakizawa and K. Kataoka, Langmuir, 2002, 18, 4539–4543 CrossRef CAS
.
- P. Gao, X. Zhang, H. Wang, Q. Zhang, H. Li, Y. Li and Y. Duan, Oncotarget, 2015, 7, 2855–2866 Search PubMed
.
- Y. Maeda, F. Pittella, T. Nomoto, H. Takemoto, N. Nishiyama, K. Miyata and K. Kataoka, Macromol. Rapid Commun., 2014, 35, 1211–1215 CrossRef CAS PubMed
.
- J. L. Huang, G. Jiang, Q. X. Song, X. Gu, M. Hu, X. L. Wang, H. H. Song, L. P. Chen, Y. Y. Lin, D. Jiang, J. Chen, J. F. Feng, Y. M. Qiu, J. Y. Jiang, X. G. Jiang, H. Z. Chen and X. L. Gao, Nat. Commun., 2017, 8, 15144 CrossRef PubMed
.
- C. H. Hou, S. M. Hou, Y. S. Hsueh, J. Lin, H. C. Wu and F. H. Lin, Biomaterials, 2009, 30, 3956–3960 CrossRef CAS PubMed
.
- C. H. Hou, C. W. Chen, S. M. Hou, Y. T. Li and F. H. Lin, Biomaterials, 2009, 30, 4700–4707 CrossRef CAS PubMed
.
- A. Tampieri, T. D'Alessandro, M. Sandri, S. Sprio, E. Landi, L. Bertinetti, S. Panseri, G. Pepponi, J. Goettlicher, M. Banobre-Lopez and J. Rivas, Acta Biomater., 2012, 8, 843–851 CrossRef CAS PubMed
.
- C.-L. Tseng, K.-C. Chang, M.-C. Yeh, K.-C. Yang, T.-P. Tang and F.-H. Lin, Ceram. Int., 2014, 40, 5117–5127 CrossRef CAS
.
- S. Panseri, M. Montesi, M. Sandri, M. Iafisco, A. Adamiano, M. Ghetti, G. Cenacchi and A. Tampieri, J. Biomed. Nanotechnol., 2016, 12, 909–921 CrossRef CAS PubMed
.
- C. Xu, Y. Zheng, W. Gao, J. Xu, G. Zuo, Y. Chen, M. Zhao, J. Li, J. Song, N. Zhang, Z. Wang, H. Zhao and Z. Mei, ACS Appl. Mater. Interfaces, 2015, 7, 13866–13875 CAS
.
- J.-Y. Huang, M.-H. Chen, W.-T. Kuo, Y.-J. Sun and F.-H. Lin, Ceram. Int., 2015, 41, 2399–2410 CrossRef CAS
.
- J. Schwiertz, A. Wiehe, S. Grafe, B. Gitter and M. Epple, Biomaterials, 2009, 30, 3324–3331 CrossRef CAS PubMed
.
- B. M. Barth, E. İ. Altınoğlu, S. S. Shanmugavelandy, J. M. Kaiser, D. Crespo-Gonzalez, N. A. DiVittore, C. McGovern, T. M. Goff, N. R. Keasey, J. H. Adair, J. Loughran, P. Thomas, D. F. Claxton and M. Kester, ACS Nano, 2011, 5, 5325–5337 CrossRef CAS PubMed
.
- S. U. Lee, K. H. Min, S. Y. Jeong, H. Bae and S. C. Lee, Chem. – Asian J., 2013, 8, 3222–3229 CrossRef CAS PubMed
.
- W. H. Chen, R. L. Lecaros, Y. C. Tseng, L. Huang and Y. C. Hsu, Cancer Lett., 2015, 359, 65–74 CrossRef CAS PubMed
.
- R. L. Lecaros, L. Huang, T. C. Lee and Y. C. Hsu, Mol. Ther., 2016, 24, 106–116 CrossRef CAS PubMed
.
- T. Nomoto, S. Fukushima, M. Kumagai, K. Miyazaki, A. Inoue, P. Mi, Y. Maeda, K. Toh, Y. Matsumoto, Y. Morimoto, A. Kishimura, N. Nishiyama and K. Kataoka, Biomater. Sci., 2016, 4, 826–838 RSC
.
- D. Y. Seong and Y. J. Kim, J. Photochem. Photobiol., B, 2015, 146, 34–43 CrossRef CAS PubMed
.
- B. M. Barth, S. S. Shanmugavelandy, J. M. Kaiser, C. McGovern, E. İ. Altınoğlu, J. K. Haakenson, J. A. Hengst, E. L. Gilius, S. A. Knupp, T. E. Fox, J. P. Smith, T. M. Ritty, J. H. Adair and M. Kester, ACS Nano, 2013, 7, 2132–2144 CrossRef CAS PubMed
.
- B. Wang, P. Liu, W. Jiang, H. Pan, X. Xu and R. Tang, Angew. Chem., Int. Ed., 2008, 120, 3616–3620 CrossRef
.
- R. Zhao, B. Wang, X. Yang, Y. Xiao, X. Wang, C. Shao and R. Tang, Angew. Chem., Int. Ed., 2016, 55, 5225–5229 CrossRef CAS PubMed
.
- P. Yang, P. Yang, X. Teng, J. Lin and L. Huang, J. Mater. Chem., 2011, 21, 5505–5510 RSC
.
- G. S. Kumar and E. K. Girija, Ceram. Int., 2013, 39, 8293–8299 CrossRef CAS
.
- F. Jiang, D. P. Wang, S. Ye and X. Zhao, J. Mater. Sci.: Mater. Med., 2014, 25, 391–400 CrossRef CAS PubMed
.
- R. K. Singh, T.-H. Kim, K. D. Patel, C. Mahapatra, K. Dashnyam, M. S. Kang, H.-W. Kim and S. Bose, J. Am. Ceram. Soc., 2014, 97, 3071–3076 CrossRef CAS
.
- R. K. Singh, T.-H. Kim, K. D. Patel, J.-J. Kim and H.-W. Kim, J. Mater. Chem. B, 2014, 2, 2039–2050 RSC
.
- P. Yang, Z. Quan, C. Li, X. Kang, H. Lian and J. Lin, Biomaterials, 2008, 29, 4341–4347 CrossRef CAS PubMed
.
- N. Niu, D. Wang, S. Huang, C. Li, F. He, S. Gai, X. Li and P. Yang, CrystEngComm, 2012, 14, 1744–1752 RSC
.
- Z. Hou, P. Yang, H. Lian, L. Wang, C. Zhang, C. Li, R. Chai, Z. Cheng and J. Lin, Chem. – Eur. J., 2009, 15, 6973–6982 CrossRef CAS PubMed
.
- A. Al-Kattan, S. Girod-Fullana, C. Charvillat, H. Ternet-Fontebasso, P. Dufour, J. Dexpert-Ghys, V. Santran, J. Bordere, B. Pipy, J. Bernad and C. Drouet, Int. J. Pharm., 2012, 423, 26–36 CrossRef CAS PubMed
.
- F. Chen, P. Huang, C. Qi, B. Q. Lu, X. Y. Zhao, C. Li, J. Wu, D. X. Cui and Y. J. Zhu, J. Mater. Chem. B, 2014, 2, 7132–7140 RSC
.
- F. Chen, P. Huang, Y. J. Zhu, J. Wu and D. X. Cui, Biomaterials, 2012, 33, 6447–6455 CrossRef CAS PubMed
.
- F. Chen, P. Huang, Y. J. Zhu, J. Wu, C. L. Zhang and D. X. Cui, Biomaterials, 2011, 32, 9031–9039 CrossRef CAS PubMed
.
- G. Zhu, R. Zhao, Y. Li and R. Tang, J. Mater. Chem. B, 2016, 4, 3903–3910 RSC
.
- H.-B. Shang, F. Chen, J. Wu, C. Qi, B.-Q. Lu, X. Chen and Y.-J. Zhu, RSC Adv., 2014, 4, 53122–53129 RSC
.
- S. P. Victor, W. Paul, M. Jayabalan and C. P. Sharma, CrystEngComm, 2014, 16, 9033–9042 RSC
.
- S. P. Victor, W. Paul, M. Jayabalan and C. P. Sharma, CrystEngComm, 2014, 16, 6929–6936 RSC
.
- M. Kester, Y. Heakal, T. Fox, A. Sharma, G. P. Robertson, T. T. Morgan, E. İ. Altınoğlu, A. Tabaković, M. R. Parette, S. M. Rouse, V. Ruiz-Velasco and J. H. Adair, Nano Lett., 2008, 8, 4116–4121 CrossRef CAS PubMed
.
- B. P. Bastakoti, Y. C. Hsu, S. H. Liao, K. C. Wu, M. Inoue, S. Yusa, K. Nakashima and Y. Yamauchi, Chem. – Asian J., 2013, 8, 1301–1305 CrossRef CAS PubMed
.
- B. P. Bastakoti, K. C. Wu, M. Inoue, S. Yusa, K. Nakashima and Y. Yamauchi, Chem. – Eur. J., 2013, 19, 4812–4817 CrossRef CAS PubMed
.
- D. Li, Z. Liang, J. Chen, J. Yu and R. Xu, Dalton Trans., 2013, 42, 9877–9883 RSC
.
- K. Haedicke, D. Kozlova, S. Grafe, U. Teichgraber, M. Epple and I. Hilger, Acta Biomater., 2015, 14, 197–207 CrossRef CAS PubMed
.
- C. Heng, X. Zheng, M. Liu, D. Xu, H. Huang, F. Deng, J. Hui, X. Zhang and Y. Wei, Appl. Surf. Sci., 2016, 386, 269–275 CrossRef CAS
.
- L. Zhou, Z. Chen, K. Dong, M. Yin, J. Ren and X. Qu, Biomaterials, 2014, 35, 8694–8702 CrossRef CAS PubMed
.
- M. Liu, H. Liu, S. Sun, X. Li, Y. Zhou, Z. Hou and J. Lin, Langmuir, 2014, 30, 1176–1182 CrossRef CAS PubMed
.
- A. B. Satterlee, H. Yuan and L. Huang, J. Controlled Release, 2015, 217, 170–182 CrossRef CAS PubMed
.
- H. T. Ong, J. S. C. Loo, F. Y. C. Boey, S. J. Russell, J. Ma and K.-W. Peng, J. Nanopart. Res., 2007, 10, 141–150 CrossRef
.
- S. Albernaz Mde, C. A. Ospina, A. M. Rossi and R. Santos-Oliveira, Artif. Cells, Nanomed., Biotechnol., 2014, 42, 88–91 CrossRef PubMed
.
- P. Mi, N. Dewi, H. Yanagie, D. Kokuryo, M. Suzuki, Y. Sakurai, Y. Li, I. Aoki, K. Ono, H. Takahashi, H. Cabral, N. Nishiyama and K. Kataoka, ACS Nano, 2015, 9, 5913–5921 CrossRef CAS PubMed
.
- N. Dewi, P. Mi, H. Yanagie, Y. Sakurai, Y. Morishita, M. Yanagawa, T. Nakagawa, A. Shinohara, T. Matsukawa, K. Yokoyama, H. Cabral, M. Suzuki, Y. Sakurai, H. Tanaka, K. Ono, N. Nishiyama, K. Kataoka and H. Takahashi, J. Cancer Res. Clin. Oncol., 2016, 142, 767–775 CrossRef CAS PubMed
.
- M. F. Cipreste, A. M. Peres, A. A. C. Cotta, F. H. Aragón, A. d. M. Antunes, A. S. Leal, W. A. A. Macedo and E. M. B. de Sousa, Mater. Chem. Phys., 2016, 181, 301–311 CrossRef CAS
.
- M. Gou, S. Li, L. Zhang, L. Li, C. Wang and Z. Su, Chem. Commun., 2016, 52, 11068–11071 RSC
.
- W. M. Li, S. Y. Chen and D. M. Liu, Acta Biomater., 2013, 9, 5360–5368 CrossRef CAS PubMed
.
- S. P. Victor, W. Paul, V. M. Vineeth, R. Komeri, M. Jayabalan and C. P. Sharma, Colloids Surf., B, 2016, 145, 539–547 CrossRef CAS PubMed
.
- M. Zhang, L. Zhang, Y. Chen, L. Li, Z.-M. Su and C. Wang, Chem. Sci., 2017, 8, 8067–8077 RSC
.
- H. Wang, S. Li, L. Zhang, X. Chen, T. Wang, M. Zhang, L. Li and C. Wang, Nanoscale, 2017, 9, 14322–14326 RSC
.
- J. Lee, H. S. Min, D. G. You, K. Kim, I. C. Kwon, T. Rhim and K. Y. Lee, J. Controlled Release, 2016, 223, 197–206 CrossRef CAS PubMed
.
- M. Kim, J. H. Lee, S. E. Kim, S. S. Kang and G. Tae, ACS Appl. Mater. Interfaces, 2016, 8, 8409–8418 CAS
.
- D. H. Keum, J. H. Mun, B. W. Hwang, J. Kim, H. Kim, W. Jo, D. H. Ha, D. W. Cho, C. Kim and S. K. Hahn, Small, 2017, 13, 1602925 CrossRef PubMed
.
- A. F. Moreira, V. M. Gaspar, E. C. Costa, D. de Melo-Diogo, P. Machado, C. M. Paquete and I. J. Correia, Eur. J. Pharm. Biopharm., 2014, 88, 1012–1025 CrossRef CAS PubMed
.
- J. Wang, J.-S. Chen, J.-Y. Zong, D. Zhao, F. Li, R.-X. Zhuo and S.-X. Cheng, J. Phys. Chem. C, 2010, 114, 18940–18945 CAS
.
- P. Liang, D. Zhao, C. Q. Wang, J. Y. Zong, R. X. Zhuo and S. X. Cheng, Colloids Surf., B, 2013, 102, 783–788 CrossRef CAS PubMed
.
- X. Ma, S. Yuan, L. Yang, L. Li, X. Zhang, C. Su and K. Wang, CrystEngComm, 2013, 15, 8288–8299 RSC
.
- V. E. Bosio, M. L. Cacicedo, B. Calvignac, I. Leon, T. Beuvier, F. Boury and G. R. Castro, Colloids Surf., B, 2014, 123, 158–169 CrossRef CAS PubMed
.
- J. L. Wu, C. Q. Wang, R. X. Zhuo and S. X. Cheng, Colloids Surf., B, 2014, 123, 498–505 CrossRef CAS PubMed
.
- M. Q. Gong, J. L. Wu, B. Chen, R. X. Zhuo and S. X. Cheng, Langmuir, 2015, 31, 5115–5122 CrossRef CAS PubMed
.
- C. Wang, C. He, Z. Tong, X. Liu, B. Ren and F. Zeng, Int. J. Pharm., 2006, 308, 160–167 CrossRef CAS PubMed
.
- C. Peng, Q. Zhao and C. Gao, Colloids Surf., A, 2010, 353, 132–139 CrossRef CAS
.
- X. Ma, L. Li, L. Yang, C. Su, Y. Guo and K. Jiang, Mater. Lett., 2011, 65, 3176–3179 CrossRef CAS
.
- N. Qiu, H. Yin, B. Ji, N. Klauke, A. Glidle, Y. Zhang, H. Song, L. Cai, L. Ma, G. Wang, L. Chen and W. Wang, Mater. Sci. Eng., C, 2012, 32, 2634–2640 CrossRef CAS
.
- W. Cui, Y. Cui, J. Zhao and J. Li, J. Mater. Chem. B, 2013, 1, 1326–1332 RSC
.
- Y. Guo, W. Jia, H. Li, W. Shi, J. Zhang, J. Feng and L. Yang, J. Mater. Chem. B, 2016, 4, 5650–5653 RSC
.
- C. Zhou, T. Chen, C. Wu, G. Zhu, L. Qiu, C. Cui, W. Hou and W. Tan, Chem. – Asian J., 2015, 10, 166–171 CrossRef CAS PubMed
.
- S. K. Kim, M. B. Foote and L. Huang, Cancer Lett., 2013, 334, 311–318 CrossRef CAS PubMed
.
- B. V. Parakhonskiy, C. Foss, E. Carletti, M. Fedel, A. Haase, A. Motta, C. Migliaresi and R. Antolini, Biomater. Sci., 2013, 1, 1273–1281 RSC
.
- D. Preisig, D. Haid, F. J. Varum, R. Bravo, R. Alles, J. Huwyler and M. Puchkov, Eur. J. Pharm. Sci., 2014, 87, 548–558 CAS
.
- X. Ying, C. Shan, K. Jiang, Z. Chen and Y. Du, RSC Adv., 2014, 4, 10841–10844 RSC
.
- G. A. Islan, M. L. Cacicedo, V. E. Bosio and G. R. Castro, J. Colloid Interface Sci., 2015, 439, 76–87 CrossRef CAS PubMed
.
- L. Liu, X. Zhang, X. Liu, J. Liu, G. Lu, D. L. Kaplan, H. Zhu and Q. Lu, ACS Appl. Mater. Interfaces, 2015, 7, 1735–1745 CAS
.
- S. S. Liu, L. J. Liu, L. Y. Xiao, Q. Lu, H. S. Zhu and D. L. Kaplan, J. Mater. Chem. B, 2015, 3, 8314–8320 RSC
.
- G. Magnabosco, M. Di Giosia, I. Polishchuk, E. Weber, S. Fermani, A. Bottoni, F. Zerbetto, P. G. Pelicci, B. Pokroy, S. Rapino, G. Falini and M. Calvaresi, Adv. Healthcare Mater., 2015, 4, 1510–1516 CrossRef CAS PubMed
.
- H. Shi, L. Li, L. Zhang, T. Wang, C. Wang, D. Zhu and Z. Su, CrystEngComm, 2015, 17, 4768–4773 RSC
.
- V. Vergaro, P. Papadia, S. Leporatti, S. A. De Pascali, F. P. Fanizzi and G. Ciccarella, J. Inorg. Biochem., 2015, 153, 284–292 CrossRef CAS PubMed
.
- J. Zhang, Y. Li, H. Xie, B. L. Su, B. Yao, Y. Yin, S. Li, F. Chen and Z. Fu, ACS Appl. Mater. Interfaces, 2015, 7, 15686–15691 CAS
.
- L. Zhang, W. Zhu, Q. Lin, J. Han, L. Jiang and Y. Zhang, Int. J. Nanomed., 2015, 10, 3291–3302 CrossRef CAS PubMed
.
- C. F. Dai, W. Y. Wang, L. Wang, L. Zhou, S. P. Li and X. D. Li, Mater. Sci. Eng., C, 2016, 69, 577–583 CrossRef CAS PubMed
.
- C.-F. Dai, W.-Y. Wang, L. Wang, L. Zhou, S.-P. Li and X.-D. Li, RSC Adv., 2016, 6, 68335–68340 RSC
.
- T. Isa, Z. A. Zakaria, Y. Rukayadi, M. N. Mohd Hezmee, A. Z. Jaji, M. U. Imam, N. I. Hammadi and S. K. Mahmood, Int. J. Mol. Sci., 2016, 17, 713 CrossRef PubMed
.
- T. Yang, Z. Wan, Z. Liu, H. Li, H. Wang, N. Lu, Z. Chen, X. Mei and X. Ren, Mater. Sci. Eng., C, 2016, 63, 384–392 CrossRef CAS PubMed
.
- X. Ma, X. Zhang, L. Yang, G. Wang, K. Jiang, G. Wu, W. Cui and Z. Wei, Nanoscale, 2016, 8, 8687–8695 RSC
.
- R. Poojari, S. Kini, R. Srivastava and D. Panda, Colloids Surf., B, 2016, 143, 131–138 CrossRef CAS PubMed
.
- L. Saidykhan, M. Z. Abu Bakar, Y. Rukayadi, A. U. Kura and S. Y. Latifah, Int. J. Nanomed., 2016, 11, 661–673 CrossRef CAS PubMed
.
- Y. Zhao, Z. Luo, M. Li, Q. Qu, X. Ma, S. H. Yu and Y. Zhao, Angew. Chem., Int. Ed., 2015, 54, 919–922 CrossRef CAS PubMed
.
- Y. Guo, J. Zhang, L. Jiang, X. Shi, L. Yang, Q. Fang, H. Fang, K. Wang and K. Jiang, Chem. Commun., 2012, 48, 10636–10638 RSC
.
- W. Wei, G.-H. Ma, G. Hu, D. Yu, T. Mcleish, Z.-G. Su and Z.-Y. Shen, J. Am. Chem. Soc., 2008, 130, 15808–15810 CrossRef CAS PubMed
.
- Y. Ueno, H. Futagawa, Y. Takagi, A. Ueno and Y. Mizushima, J. Controlled Release, 2005, 103, 93–98 CrossRef CAS PubMed
.
- S. Chen, D. Zhao, F. Li, R.-X. Zhuo and S.-X. Cheng, RSC Adv., 2012, 2, 1820–1826 RSC
.
- D. Zhao, C. J. Liu, R. X. Zhuo and S. X. Cheng, Mol. Pharmaceutics, 2012, 9, 2887–2893 CrossRef CAS PubMed
.
- B. Sun, K. K. Tran and H. Shen, Biomaterials, 2009, 30, 6386–6393 CrossRef CAS PubMed
.
- J. Wei, T. Cheang, B. Tang, H. Xia, Z. Xing, Z. Chen, Y. Fang, W. Chen, A. Xu, S. Wang and J. Luo, Biomaterials, 2013, 34, 1246–1254 CrossRef CAS PubMed
.
- X. W. He, T. Liu, Y. X. Chen, D. J. Cheng, X. R. Li, Y. Xiao and Y. L. Feng, Cancer Gene Ther., 2008, 15, 193–202 CrossRef CAS PubMed
.
- T.-y. Cheang, S.-m. Wang, Z.-j. Hu, Z.-H. Xing, G.-q. Chang, C. Yao, Y. Liu, H. Zhang and A.-W. Xu, J. Mater. Chem., 2010, 20, 8050–8055 RSC
.
- U. Reibetanz, M. H. A. Chen, S. Mutukumaraswamy, Z. Y. Liaw, B. H. L. Oh, S. Venkatraman, E. Donath and B. Neu, Biomacromolecules, 2010, 11, 1779–1784 CrossRef CAS PubMed
.
- S. Chen, F. Li, R. X. Zhuo and S. X. Cheng, Mol. BioSyst., 2011, 7, 2841–2847 RSC
.
- X. Kong, S. Xu, X. Wang, F. Cui and J. Yao, J. Biomed. Mater. Res., Part A, 2012, 100, 2312–2318 Search PubMed
.
- C.-Q. Wang, M.-Q. Gong, J.-L. Wu, R.-X. Zhuo and S.-X. Cheng, RSC Adv., 2014, 4, 38623–38629 RSC
.
- D. Zhao, C. Q. Wang, R. X. Zhuo and S. X. Cheng, Colloids Surf., B, 2014, 118, 111–116 CrossRef CAS PubMed
.
- H. Zhou, J. Wei, Q. Dai, L. Wang, J. Luo, T. Cheang and S. Wang, Int. J. Nanomed., 2015, 10, 4255–4266 CAS
.
- J. H. Byeon, Sci. Rep., 2016, 6, 28782 CrossRef CAS PubMed
.
- M. Fujiwara, K. Shiokawa, K. Morigaki, Y. Zhu and Y. Nakahara, Chem. Eng. J., 2008, 137, 14–22 CrossRef CAS
.
- Z. Lu, J. Zhang, Y. Ma, S. Song and W. Gu, Mater. Sci. Eng., C, 2012, 32, 1982–1987 CrossRef CAS
.
- L. N. Hassani, F. Hindré, T. Beuvier, B. Calvignac, N. Lautram, A. Gibaud and F. Boury, J. Mater. Chem. B, 2013, 1, 4011–4019 RSC
.
- V. Lauth, M. Maas and K. Rezwan, J. Mater. Chem. B, 2014, 2, 7725–7731 RSC
.
- C. Qi, Y. J. Zhu, B. Q. Lu, X. Y. Zhao, J. Zhao, F. Chen and J. Wu, Small, 2014, 10, 2047–2056 CrossRef CAS PubMed
.
- A. N. Koo, K. H. Min, H. J. Lee, J. H. Jegal, J. W. Lee and S. C. Lee, Chem. – Asian J., 2015, 10, 2380–2387 CrossRef CAS PubMed
.
- E. S. Vasquez, J. L. Cunningham, J. B. McMahan, C. L. Simpson and K. B. Walters, J. Mater. Chem. B, 2015, 3, 6411–6419 RSC
.
- S. Donatan, A. Yashchenok, N. Khan, B. Parakhonskiy, M. Cocquyt, B. E. Pinchasik, D. Khalenkow, H. Mohwald, M. Konrad and A. Skirtach, ACS Appl. Mater. Interfaces, 2016, 8, 14284–14292 CAS
.
- F. Tewes, O. L. Gobbo, C. Ehrhardt and A. M. Healy, ACS Appl. Mater. Interfaces, 2016, 8, 1164–1175 CAS
.
- G. Begum, T. N. Reddy, K. P. Kumar, K. Dhevendar, S. Singh, M. Amarnath, S. Misra, V. K. Rangari and R. K. Rana, ACS Appl. Mater. Interfaces, 2016, 8, 22056–22063 CAS
.
- K. H. Min, H. S. Min, H. J. Lee, D. J. Park, J. Y. Yhee, K. Kim, I. C. Kwon, S. Y. Jeong, O. F. Silvestre, X. Chen, Y.-S. Hwang, E.-C. Kim and S. C. Lee, ACS Nano, 2015, 9, 134–145 CrossRef CAS PubMed
.
- D. J. Park, K. H. Min, H. J. Lee, K. Kim, I. C. Kwon, S. Y. Jeong and S. C. Lee, J. Mater. Chem. B, 2016, 4, 1219–1227 RSC
.
- Z. Dong, L. Feng, W. Zhu, X. Sun, M. Gao, H. Zhao, Y. Chao and Z. Liu, Biomaterials, 2016, 110, 60–70 CrossRef CAS PubMed
.
- F. Kong, H. Zhang, X. Zhang, D. Liu, D. Chen, W. Zhang, L. Zhang, H. A. Santos and M. Hai, Adv. Funct. Mater., 2016, 26, 6158–6169 CrossRef CAS
.
- A. Neira-Carrillo, E. Yslas, Y. A. Marini, P. Vasquez-Quitral, M. Sanchez, A. Riveros, D. Yanez, P. Cavallo, M. J. Kogan and D. Acevedo, Colloids Surf., B, 2016, 145, 634–642 CrossRef CAS PubMed
.
- M. Zhang and J. Chang, Ultrason. Sonochem., 2010, 17, 789–792 CrossRef CAS PubMed
.
- J. Zhang and Y. Zhu, Microporous Mesoporous Mater., 2014, 197, 244–251 CrossRef CAS
.
- M. S. Islam, H. N. Choi, W. S. Choi and H.-J. Lee, J. Mater. Chem. B, 2015, 3, 1001–1009 RSC
.
- W. Fan, Y. Li, Q. Sun, T. Ma and B. Fan, J. Nanobiotechnol., 2016, 14, 72 CrossRef PubMed
.
- Y.-F. Shen, C.-C. Ho, M.-Y. Shie, K. Wang and H.-Y. Fang, Materials, 2016, 9, 306 CrossRef PubMed
.
- J. Wu, Y. J. Zhu, S. W. Cao and F. Chen, Adv. Mater., 2010, 22, 749–753 CrossRef CAS PubMed
.
- J. Wu, Y. J. Zhu and F. Chen, Small, 2013, 9, 2911–2925 CrossRef CAS PubMed
.
- J. Wu, Y. J. Zhu, F. Chen, X. Y. Zhao, J. Zhao and C. Qi, Dalton Trans., 2013, 42, 7032–7040 RSC
.
- B. Q. Lu, Y. J. Zhu, H. Y. Ao, C. Qi and F. Chen, ACS Appl. Mater. Interfaces, 2012, 4, 6969–6974 CAS
.
- W. Xue, A. Bandyopadhyay and S. Bose, Acta Biomater., 2009, 5, 1686–1696 CrossRef CAS PubMed
.
- M. Fujiwara, K. Shiokawa and T. Kubota, Mater. Sci. Eng., C, 2012, 32, 2484–2490 CrossRef CAS
.
- J. Kang, J. Joo, E. J. Kwon, M. Skalak, S. Hussain, Z. G. She, E. Ruoslahti, S. N. Bhatia and M. J. Sailor, Adv. Mater., 2016, 28, 7962–7969 CrossRef CAS PubMed
.
- G. Wang, Q. Peng and Y. Li, J. Am. Chem. Soc., 2009, 131, 14200–14201 CrossRef CAS PubMed
.
- N.-N. Dong, M. Pedroni, F. Piccinelli, G. Conti, A. Sbarbati, J. E. Ramírez-Hernández, L. M. Maestro, M. C. I.-d. l. Cruz, F. Sanz-Rodriguez, A. Juarranz, F. Chen, F. Vetrone, J. A. Capobianco, J. G. Solé, M. Bettinelli, D. Jaque and A. Speghini, ACS Nano, 2011, 5, 8665–8671 CrossRef CAS PubMed
.
- S. Sasidharan, A. Jayasree, S. Fazal, M. Koyakutty, S. V. Nair and D. Menon, Biomater. Sci., 2013, 1, 294–305 RSC
.
- J. Zhao, Y. J. Zhu, J. Wu and F. Chen, J. Colloid Interface Sci., 2015, 440, 39–45 CrossRef CAS PubMed
.
- W. Zheng, S. Zhou, Z. Chen, P. Hu, Y. Liu, D. Tu, H. Zhu, R. Li, M. Huang and X. Chen, Angew. Chem., Int. Ed., 2013, 52, 6671–6676 CrossRef CAS PubMed
.
- I. X. Cantarelli, M. Pedroni, F. Piccinelli, P. Marzola, F. Boschi, G. Conti, A. Sbarbati, P. Bernardi, E. Mosconi, L. Perbellini, L. Marongiu, M. Donini, S. Dusi, L. Sorace, C. Innocenti, E. Fantechi, C. Sangregorio and A. Speghini, Biomater. Sci., 2014, 2, 1158–1171 RSC
.
- A. H. Li, M. Lu, J. Yang, L. Chen, X. Cui and Z. Sun, Dalton Trans., 2016, 45, 5800–5807 RSC
.
- G. Chen, J. Shen, T. Y. Ohulchanskyy, N. J. Patel, A. Kutikov, Z. Li, J. Song, R. K. Pandey, H. Ågren, P. N. Prasad and G. Han, ACS Nano, 2012, 6, 8280–8287 CrossRef CAS PubMed
.
- L. Zhao, A. Kutikov, J. Shen, C. Duan, J. Song and G. Han, Theranostics, 2013, 3, 249–257 CrossRef CAS PubMed
.
- B.-B. Ding, K. Liu, F. Zhang, Y. Wang, S. Cheng, Y. Lu and H.-S. Qian, CrystEngComm, 2015, 17, 5900–5905 RSC
.
- H. Dong, L. D. Sun, Y. F. Wang, J. Ke, R. Si, J. W. Xiao, G. M. Lyu, S. Shi and C. H. Yan, J. Am. Chem. Soc., 2015, 137, 6569–6576 CrossRef CAS PubMed
.
- K. Prorok, A. Bednarkiewicz, B. Cichy, A. Gnach, M. Misiak, M. Sobczyk and W. Strek, Nanoscale, 2014, 6, 1855–1864 RSC
.
- J. Shen, G. Chen, T. Y. Ohulchanskyy, S. J. Kesseli, S. Buchholz, Z. Li, P. N. Prasad and G. Han, Small, 2013, 9, 3213–3217 CrossRef CAS PubMed
.
- Y. Su, X. Liu, P. Lei, X. Xu, L. Dong, X. Guo, X. Yan, P. Wang, S. Song, J. Feng and H. Zhang, Dalton Trans., 2016, 45, 11129–11136 RSC
.
- M. Xue, X. Zhu, X. Qiu, Y. Gu, W. Feng and F. Li, ACS Appl. Mater. Interfaces, 2016, 8, 17894–17901 CAS
.
- Y. Li, Y. Gu, W. Yuan, T. Cao, K. Li, S. Yang, Z. Zhou and F. Li, ACS Appl. Mater. Interfaces, 2016, 8, 19208–19216 CAS
.
- S. Hao, L. Yang, H. Qiu, R. Fan, C. Yang and G. Chen, Nanoscale, 2015, 7, 10775–10780 RSC
.
- A. Astegno, E. Maresi, V. Marino, P. Dominici, M. Pedroni, F. Piccinelli and D. Dell'Orco, Nanoscale, 2014, 6, 15037–15047 RSC
.
- V. Marino, A. Astegno, M. Pedroni, F. Piccinelli and D. Dell'Orco, Nanoscale, 2014, 6, 412–423 RSC
.
- C. Zhang, C. Li, C. Peng, R. Chai, S. Huang, D. Yang, Z. Cheng and J. Lin, Chem. – Eur. J., 2010, 16, 5672–5680 CrossRef CAS PubMed
.
- Y. Dai, C. Zhang, Z. Cheng, P. Ma, C. Li, X. Kang, D. Yang and J. Lin, Biomaterials, 2012, 33, 2583–2592 CrossRef CAS PubMed
.
- X. Deng, Y. Dai, J. Liu, Y. Zhou, P. Ma, Z. Cheng, Y. Chen, K. Deng, X. Li, Z. Hou, C. Li and J. Lin, Biomaterials, 2015, 50, 154–163 CrossRef CAS PubMed
.
- W. Yin, G. Tian, W. Ren, L. Yan, S. Jin, Z. Gu, L. Zhou, J. Li and Y. Zhao, Dalton Trans., 2014, 43, 3861–3870 RSC
.
- Y. Li, Y. Zhou, X. Li, J. Sun, Z. Ren, W. Wen, X. Yang and G. Han, RSC Adv., 2016, 6, 38365–38370 RSC
.
- X. F. Qiao, J. C. Zhou, J. W. Xiao, Y. F. Wang, L. D. Sun and C. H. Yan, Nanoscale, 2012, 4, 4611–4623 RSC
.
- M. Zahedifar, E. Sadeghi, M. M. Shanei, A. Sazgarnia and M. Mehrabi, J. Lumin., 2016, 171, 254–258 CrossRef CAS
.
- L. P. Singh, S. K. Srivastava, R. Mishra and R. S. Ningthoujam, J. Phys. Chem. C, 2014, 118, 18087–18096 CAS
.
- S. V. Dorozhkin, Materials, 2009, 2, 399–498 CrossRef CAS
.
- L. C. Palmer, C. J. Newcomb, S. R. Kaltz, E. D. Spoerke and S. I. Stupp, Chem. Rev., 2008, 108, 4754–4783 CrossRef CAS PubMed
.
- S. V. Dorozhkin, Materials, 2009, 2, 1975–2045 CrossRef CAS
.
- M. F. Cipreste, I. Gonzalez, T. Maria da Mata Martins, A. M. Goes, W. Augusto de Almeida Macedo and E. M. Barros de Sousa, RSC Adv., 2016, 6, 76390–76400 RSC
.
- L. J. Wang and G. H. Nancollas, Chem. Rev., 2008, 108, 4628–4669 CrossRef CAS PubMed
.
- O. Bertran, G. Revilla-Lopez, J. Casanovas, L. J. Del Valle, P. Turon, J. Puiggali and C. Aleman, Chem. – Eur. J., 2016, 22, 6631–6636 CrossRef CAS PubMed
.
- T.-J. Lin and H. Heinz, J. Phys. Chem. C, 2016, 120, 4975–4992 CAS
.
- Y. Lu, A. A. Aimetti, R. Langer and Z. Gu, Nat. Rev. Mater., 2016, 2, 16075 CrossRef
.
- A. Ereath Beeran, F. B. Fernandez, A. John and H. Varma Pr, RSC Adv., 2015, 5, 36742–36752 RSC
.
- X. Wang, Y. Deng, S. Li, G. Wang, E. Qin, X. Xu, R. Tang and C. Qin, Adv. Healthcare Mater., 2012, 1, 443–449 CrossRef CAS PubMed
.
- J. Chen, P. Gao, S. Yuan, R. Li, A. Ni, L. Chu, L. Ding, Y. Sun, X. Y. Liu and Y. Duan, ACS Nano, 2016, 10, 11548–11560 CrossRef CAS PubMed
.
- H. W. Choi, J. Kim, J. Kim, Y. Kim, H. B. Song, J. H. Kim, K. Kim and W. J. Kim, ACS Nano, 2016, 10, 4199–4208 CrossRef CAS PubMed
.
- Z. Zhao, M. Espanol, J. Guillem-Marti, D. Kempf, A. Diez-Escudero and M. P. Ginebra, Nanoscale, 2016, 8, 1595–1607 RSC
.
- J. L. Arias and M. S. Fernández, Chem. Rev., 2008, 108, 4475–4482 CrossRef CAS PubMed
.
- M. Cusack and A. Freer, Chem. Rev., 2008, 108, 4433–4454 CrossRef CAS PubMed
.
- L. B. Gower, Chem. Rev., 2008, 108, 4551–4627 CrossRef CAS PubMed
.
- J. H. E. Cartwright, A. G. Checa, J. D. Gale, D. Gebauer and C. I. Sainz-Díaz, Angew. Chem., Int. Ed., 2012, 51, 11960–11970 CrossRef CAS PubMed
.
- Y. Liu, Y. J. Cui and R. Guo, Langmuir, 2012, 28, 6097–6105 CrossRef CAS PubMed
.
- M. Saharay, A. O. Yazaydin and R. J. Kirkpatrick, J. Phys. Chem. B, 2013, 117, 3328–3336 CrossRef CAS PubMed
.
- D. Gebauer, P. N. Gunawidjaja, J. Y. Ko, Z. Bacsik, B. Aziz, L. Liu, Y. Hu, L. Bergstrom, C. W. Tai, T. K. Sham, M. Eden and N. Hedin, Angew. Chem., Int. Ed., 2010, 49, 8889–8891 CrossRef CAS PubMed
.
- S. E. Wolf, J. Leiterer, M. Kappl, F. Emmerling and W. Tremel, J. Am. Chem. Soc., 2008, 130, 12342–12347 CrossRef CAS PubMed
.
- B. Parakhonskiy, M. V. Zyuzin, A. Yashchenok, S. Carregal-Romero, J. Rejman, H. Mohwald, W. J. Parak and A. G. Skirtach, J. Nanobiotechnol., 2015, 13, 53 CrossRef PubMed
.
- A. Som, R. Raliya, L. Tian, W. Akers, J. E. Ippolito, S. Singamaneni, P. Biswas and S. Achilefu, Nanoscale, 2016, 8, 12639–12647 RSC
.
- X. Wang, R. Kong, X. Pan, H. Xu, D. Xia, H. Shan and J. R. Lu, J. Phys. Chem. B, 2009, 113, 8975–8982 CrossRef CAS PubMed
.
- A. W. Xu, Q. Yu, W. F. Dong, M. Antonietti and H. Cölfen, Adv. Mater., 2005, 17, 2217–2221 CrossRef CAS
.
- C. Qi, J.-J. Huang, F. Chen, J. Wu, C.-N. Hao, Y.-Q. Shi, J.-L. Duan and Y.-J. Zhu, J. Mater. Chem. B, 2014, 2, 8378–8389 RSC
.
- A. T. Nagaraja, S. Pradhan and M. J. McShane, J. Colloid Interface Sci., 2014, 418, 366–372 CrossRef CAS PubMed
.
- B. Guillemet, M. Faatz, F. Gröhn, G. Wegner and Y. Gnanou, Langmuir, 2006, 22, 1875–1879 CrossRef CAS PubMed
.
- J.-F. Chen, H.-M. Ding, J.-X. Wang and L. Shao, Biomaterials, 2004, 25, 723–727 CrossRef CAS PubMed
.
- Y. J. Yang, X. Tao, Q. Hou and J. F. Chen, Acta Biomater., 2009, 5, 3488–3496 CrossRef CAS PubMed
.
- M.-Y. Ma, Y.-J. Zhu, L. Li and S.-W. Cao, J. Mater. Chem., 2008, 18, 2722–2727 RSC
.
- A. Wang, Y. Yang, Y. Qi, W. Qi, J. Fei, H. Ma, J. Zhao, W. Cui and J. Li, ACS Appl. Mater. Interfaces, 2016, 8, 8900–8907 CAS
.
- Y. Zhao, L. N. Lin, Y. Lu, S. F. Chen, L. Dong and S. H. Yu, Adv. Mater., 2010, 22, 5255–5259 CrossRef CAS PubMed
.
- I. Marchenko, A. Yashchenok, T. Borodina, T. Bukreeva, M. Konrad, H. Mohwald and A. Skirtach, J. Controlled Release, 2012, 162, 599–605 CrossRef CAS PubMed
.
- R. Luo, S. S. Venkatraman and B. Neu, Biomacromolecules, 2013, 14, 2262–2271 CrossRef CAS PubMed
.
- A. Yashchenok, B. Parakhonskiy, S. Donatan, D. Kohler, A. Skirtach and H. Möhwald, J. Mater. Chem. B, 2013, 1, 1223–1228 RSC
.
- J. J. Richardson, J. W. Maina, H. Ejima, M. Hu, J. Guo, M. Y. Choy, S. T. Gunawan, L. Lybaert, C. E. Hagemeyer, B. G. De Geest and F. Caruso, Adv. Sci., 2015, 2, 1400007 CrossRef PubMed
.
- Y. Ju, J. Cui, H. Sun, M. Mullner, Y. Dai, J. Guo, N. Bertleff-Zieschang, T. Suma, J. J. Richardson and F. Caruso, Biomacromolecules, 2016, 17, 2268–2276 CrossRef CAS PubMed
.
- C. E. Hansen, D. R. Myers, W. H. Baldwin, Y. Sakurai, S. L. Meeks, L. A. Lyon and W. A. Lam, ACS Nano, 2017, 11, 5579–5589 CrossRef CAS PubMed
.
- B. V. Parakhonskiy, Y. I. Svenskaya, C. Yashchenok Acapital Em, H. A. Fattah, O. A. Inozemtseva, F. Tessarolo, R. Antolini and D. A. Gorin, Colloids Surf., B, 2014, 118, 243–248 CrossRef CAS PubMed
.
- H. Chen and S. Leng, Ceram. Int., 2015, 41, 2209–2213 CrossRef CAS
.
- W. Lai, C. Chen, X. Ren, I. S. Lee, G. Jiang and X. Kong, Mater. Sci. Eng., C, 2016, 62, 166–172 CrossRef CAS PubMed
.
- Y. Guo, Y. Zhou, D. Jia and H. Tang, Microporous Mesoporous Mater., 2009, 118, 480–488 CrossRef CAS
.
- J. Forsgren, M. Andersson, P. Nilsson and A. Mihranyan, Adv. Healthcare Mater., 2013, 2, 1469–1476 CrossRef CAS PubMed
.
- S. Xu, K. Lin, Z. Wang, J. Chang, L. Wang, J. Lu and C. Ning, Biomaterials, 2008, 29, 2588–2596 CrossRef CAS PubMed
.
- H. Li and J. Chang, Acta Biomater., 2013, 9, 5379–5389 CrossRef CAS PubMed
.
- K. Lin, L. Xia, H. Li, X. Jiang, H. Pan, Y. Xu, W. W. Lu, Z. Zhang and J. Chang, Biomaterials, 2013, 34, 10028–10042 CrossRef CAS PubMed
.
- M. Y. Shie and S. J. Ding, Biomaterials, 2013, 34, 6589–6606 CrossRef CAS PubMed
.
- X. Guo, Z. Wang, J. Wu, Y. Hu, J. Wang, Y.-J. Zhu and T.-K. Sham, CrystEngComm, 2015, 17, 4117–4124 RSC
.
- B.-Q. Lu, Y.-J. Zhu, G.-F. Cheng and Y.-J. Ruan, Mater. Lett., 2013, 104, 53–56 CrossRef CAS
.
- H. H. Xu, J. L. Moreau, L. Sun and L. C. Chow, Biomaterials, 2008, 29, 4261–4267 CrossRef CAS PubMed
.
- M. Sadat-Shojai, M. T. Khorasani, E. Dinpanah-Khoshdargi and A. Jamshidi, Acta Biomater., 2013, 9, 7591–7621 CrossRef CAS PubMed
.
- A. C. Tas, Acta Biomater., 2014, 10, 1771–1792 CrossRef CAS PubMed
.
- K. L. Lin, C. T. Wu and J. Chang, Acta Biomater., 2014, 10, 4071–4102 CrossRef CAS PubMed
.
- B. V. Parakhonskiy, A. Haase and R. Antolini, Angew. Chem., Int. Ed., 2012, 51, 1195–1197 CrossRef CAS PubMed
.
- D. B. Trushina, T. V. Bukreeva and M. N. Antipina, Cryst. Growth Des., 2016, 16, 1311–1319 CAS
.
- N. Puvvada, P. K. Panigrahi and A. Pathak, Nanoscale, 2010, 2, 2631–2638 RSC
.
- B. V. Parakhonskiy, A. M. Yashchenok, S. Donatan, D. V. Volodkin, F. Tessarolo, R. Antolini, H. Mohwald and A. G. Skirtach, ChemPhysChem, 2014, 15, 2817–2822 CrossRef CAS PubMed
.
- M. E. Zilm, M. Staruch, M. Jain and M. Wei, J. Mater. Chem. B, 2014, 2, 7176–7185 RSC
.
- H.-C. Wu, T.-W. Wang, J.-S. Sun, W.-H. Wang and F.-H. Lin, Nanotechnology, 2007, 18, 165601 CrossRef
.
- H. Zhao, Y. D. Zhu, J. Sun, D. Wei, K. F. Wang, M. Liu, H. S. Fan and X. D. Zhang, Chem. Commun., 2014, 50, 12519–12522 RSC
.
- Y.-P. Guo, T.-S. Lin, Y. Zhou, D.-C. Jia and Y.-J. Guo, Microporous Mesoporous Mater., 2010, 127, 245–249 CrossRef CAS
.
- B. P. Bastakoti, M. Inuoe, S. Yusa, S. H. Liao, K. C. Wu, K. Nakashima and Y. Yamauchi, Chem. Commun., 2012, 48, 6532–6534 RSC
.
- R. V. Bell, L. A. Rochford, R. T. M. de Rosales, M. Stevens, J. V. M. Weaver and S. A. F. Bon, J. Mater. Chem. B, 2015, 3, 5544–5552 RSC
.
- K. K. Perkin, J. L. Turner, K. L. Wooley and S. Mann, Nano Lett., 2005, 5, 1457–1461 CrossRef CAS PubMed
.
- W. Tjandra, P. Ravi, J. Yao and K. C. Tam, Nanotechnology, 2006, 17, 5988–5994 CrossRef CAS
.
- H. T. Schmidt, B. L. Gray, P. A. Wingert and A. E. Ostafin, Chem. Mater., 2004, 16, 4942–4947 CrossRef CAS
.
- H. T. Schmidt and A. E. Ostafin, Adv. Mater., 2002, 14, 532–535 CrossRef CAS
.
- E. Y. Zeynep, D. Antoine, C. Brice, B. Frank and J. Christine, J. Mater. Chem. B, 2015, 3, 7227–7236 RSC
.
- A. Sugawara, S. Yamane and K. Akiyoshi, Macromol. Rapid Commun., 2006, 27, 441–446 CrossRef CAS
.
- Q. Wang, P. Liu, Y. Sun, T. Gong, M. Zhu, X. Sun, Z. Zhang and Y. Duan, Nanotoxicology, 2015, 9, 190–200 CrossRef CAS PubMed
.
- B. Palazzo, D. Walsh, M. Iafisco, E. Foresti, L. Bertinetti, G. Martra, C. L. Bianchi, G. Cappelletti and N. Roveri, Acta Biomater., 2009, 5, 1241–1252 CrossRef CAS PubMed
.
- A. Bouladjine, A. Al-Kattan, P. Dufour and C. Drouet, Langmuir, 2009, 25, 12256–12265 CrossRef CAS PubMed
.
- K. Ganesan, A. Kovtun, S. Neumann, R. Heumann and M. Epple, J. Mater. Chem., 2008, 18, 3655–3661 RSC
.
- E. T. Hwang, R. Tatavarty, J. Chung and M. B. Gu, ACS Appl. Mater. Interfaces, 2013, 5, 532–537 CAS
.
- X. Cheng, Z. Huang, J. Li, Y. Liu, C. Chen, R.-A. Chi and Y. Hu, Cryst. Growth Des., 2010, 10, 1180–1188 CAS
.
- D. Jiang, D. Li, J. Xie, J. Zhu, M. Chen, X. Lu and S. Dang, J. Colloid Interface Sci., 2010, 350, 30–38 CrossRef CAS PubMed
.
- M. G. Ma, Int. J. Nanomed., 2012, 7, 1781–1791 CrossRef CAS PubMed
.
- H. Zhang, M. Liu, H. Fan and X. Zhang, Cryst. Growth Des., 2012, 12, 2204–2212 CAS
.
- E. Lester, S. V. Y. Tang, A. Khlobystov, V. L. Rose, L. Buttery and C. J. Roberts, CrystEngComm, 2013, 15, 3256–3260 RSC
.
- F. Ren, Y. Leng, Y. Ding and K. Wang, CrystEngComm, 2013, 15, 2137–2146 RSC
.
- Y. Yang, Q. Wu, M. Wang, J. Long, Z. Mao and X. Chen, Cryst. Growth Des., 2014, 14, 4864–4871 CAS
.
- X. Jin, X. Chen, Y. Cheng, L. Wang, B. Hu and J. Tan, J. Colloid Interface Sci., 2015, 450, 151–158 CrossRef CAS PubMed
.
- X. Jin, J. Zhuang, Z. Zhang, H. Guo and J. Tan, J. Colloid Interface Sci., 2015, 443, 125–130 CrossRef CAS PubMed
.
- Y. Wang, X. Ren, X. Ma, W. Su, Y. Zhang, X. Sun and X. Li, Cryst. Growth Des., 2015, 15, 1949–1956 CAS
.
- K. Lin, P. Liu, L. Wei, Z. Zou, W. Zhang, Y. Qian, Y. Shen and J. Chang, Chem. Eng. J., 2013, 222, 49–59 CrossRef CAS
.
- S. Y. Park, K.-I. Kim, S. P. Park, J. H. Lee and H. S. Jung, Cryst. Growth Des., 2016, 16, 4318–4326 CAS
.
- B. Q. Lu, Y. J. Zhu, F. Chen, C. Qi, X. Y. Zhao and J. Zhao, Chem. – Eur. J., 2014, 20, 7116–7121 CrossRef CAS PubMed
.
- H. Cao, L. Zhang, H. Zheng and Z. Wang, J. Phys. Chem. C, 2010, 114, 18352–18357 CAS
.
- F. Chen, Y. J. Zhu, X. Y. Zhao, B. Q. Lu and J. Wu, CrystEngComm, 2013, 15, 4527–4531 RSC
.
- C. Qi, Y. J. Zhu, G. J. Ding, J. Wu and F. Chen, RSC Adv., 2015, 5, 3792–3798 RSC
.
- X.-Y. Zhao, Y.-J. Zhu, B.-Q. Lu, F. Chen, C. Qi, J. Zhao and J. Wu, Mater. Res. Bull., 2014, 55, 67–70 CrossRef CAS
.
- Y. J. Zhu and F. Chen, Chem. Rev., 2014, 114, 6462–6555 CrossRef CAS PubMed
.
- W. Amer, K. Abdelouahdi, H. R. Ramananarivo, M. Zahouily, A. Fihri, K. Djessas, K. Zahouily, R. S. Varma and A. Solhy, CrystEngComm, 2014, 16, 543–549 RSC
.
- K.-W. Wang, Y.-J. Zhu, F. Chen, G.-F. Cheng and Y.-H. Huang, Mater. Lett., 2011, 65, 2361–2363 CrossRef CAS
.
- P. J. Reardon, J. Huang and J. Tang, J. Biomed. Mater. Res., Part A, 2015, 103, 3781–3789 CrossRef CAS PubMed
.
- C. Qi, Q. L. Tang, Y. J. Zhu, X. Y. Zhao and F. Chen, Mater. Lett., 2012, 85, 71–73 CrossRef CAS
.
- K. S. Suslick, Science, 1990, 247, 1439–1445 CAS
.
- K. S. Suslick, S. B. Choe, A. A. Cichowlas and M. W. Grinstaff, Nature, 1991, 353, 414–416 CrossRef CAS
.
- G. E. Poinern, R. K. Brundavanam, N. Mondinos and Z. T. Jiang, Ultrason. Sonochem., 2009, 16, 469–474 CrossRef CAS PubMed
.
- M. Jevtić, M. Mitrić, S. Škapin, B. Jančar, N. Ignjatović and D. Uskoković, Cryst. Growth Des., 2008, 8, 2217–2222 Search PubMed
.
- G. Zhou, W. Song, Y. Hou, Q. Li, X. Deng and Y. Fan, J. Biomed. Mater. Res., Part A, 2014, 102, 3704–3712 CrossRef PubMed
.
- A. López-Marzo, J. Pons and A. Merkoçi, J. Mater. Chem., 2012, 22, 15326–15335 RSC
.
- M. Mehrali, S. F. Seyed Shirazi, S. Baradaran, M. Mehrali, H. S. Metselaar, N. A. Kadri and N. A. Osman, Ultrason. Sonochem., 2014, 21, 735–742 CrossRef CAS PubMed
.
- J. Chen, Y. Wang, X. Chen, L. Ren, C. Lai, W. He and Q. Zhang, Mater. Lett., 2011, 65, 1923–1926 CrossRef CAS
.
- G. H. An, H. J. Wang, B. H. Kim, Y. G. Jeong and Y. H. Choa, Mater. Sci. Eng., A, 2007, 449-451, 821–824 CrossRef
.
- J. S. Cho and Y. C. Kang, J. Alloys Compd., 2008, 464, 282–287 CrossRef CAS
.
- S. K. Ghosh, S. K. Roy, B. Kundu, S. Datta and D. Basu, Mater. Sci. Eng., B, 2011, 176, 14–21 CrossRef CAS
.
- A. Fahami, B. Nasiri-Tabrizi and R. Ebrahimi-Kahrizsangi, Ceram. Int., 2012, 38, 6729–6738 CrossRef CAS
.
- K. Lin, X. Liu, J. Chang and Y. Zhu, Nanoscale, 2011, 3, 3052–3055 RSC
.
- A. J. Nathanael, S. I. Hong, D. Mangalaraj and P. C. Chen, Chem. Eng. J., 2011, 173, 846–854 CrossRef CAS
.
- C. Zhang and J. Lin, Chem.
Soc. Rev., 2012, 41, 7938–7961 RSC
.
- S. Moudgil and J. Y. Ying, Adv. Mater., 2007, 19, 3130–3135 CrossRef CAS
.
- Y. Han, S. Li, X. Cao, L. Yuan, Y. Wang, Y. Yin, T. Qiu, H. Dai and X. Wang, Sci. Rep., 2014, 4, 7134 CrossRef CAS PubMed
.
- Y. Sun, Y. Chen, X. Ma, Y. Yuan, C. Liu, J. Kohn and J. Qian, ACS Appl. Mater. Interfaces, 2016, 8, 25680–25690 CAS
.
- Y. Liu, Y. Hu and L. Huang, Biomaterials, 2014, 35, 3027–3034 CrossRef CAS PubMed
.
- S. M. Dizaj, M. Barzegar-Jalali, M. H. Zarrintan, K. Adibkia and F. Lotfipour, Expert Opin. Drug Delivery, 2015, 12, 1649–1660 CrossRef PubMed
.
- X. Guo, J. Wu, Y. M. Yiu, Y. Hu, Y. J. Zhu and T. K. Sham, Phys. Chem. Chem. Phys., 2013, 15, 15033–15040 RSC
.
- X. Guo, Z. Wang, J. Wu, Y. M. Yiu, Y. Hu, Y. J. Zhu and T. K. Sham, J. Phys. Chem. B, 2015, 119, 10052–10059 CrossRef CAS PubMed
.
- X. Guo, Z. Wang, J. Wu, J. Wang, Y. J. Zhu and T. K. Sham, Nanoscale, 2015, 7, 6767–6773 RSC
.
- L. Cheng, C. Wang, L. Feng, K. Yang and Z. Liu, Chem. Rev., 2014, 114, 10869–10939 CrossRef CAS PubMed
.
- W. Fan, P. Huang and X. Chen, Chem. Soc. Rev., 2016, 45, 6488–6519 RSC
.
- K. K. Ng and G. Zheng, Chem. Rev., 2015, 115, 11012–11042 CrossRef CAS PubMed
.
- S. L. Yefimova, I. I. Bespalova, G. V. Grygorova, A. V. Sorokin, P. V. Mateychenko, X. Q. Cui and Y. V. Malyukin, Microporous Mesoporous Mater., 2016, 236, 120–128 CrossRef CAS
.
- Y. I. Svenskaya, A. M. Pavlov, D. A. Gorin, D. J. Gould, B. V. Parakhonskiy and G. B. Sukhorukov, Colloids Surf., B, 2016, 146, 171–179 CrossRef CAS PubMed
.
- Y. Svenskaya, B. Parakhonskiy, A. Haase, V. Atkin, E. Lukyanets, D. Gorin and R. Antolini, Biophys. Chem., 2013, 182, 11–15 CrossRef CAS PubMed
.
- L. V. Wang and S. Hu, Science, 2012, 335, 1458–1462 CrossRef CAS PubMed
.
- X. Sun, W. Cai and X. Chen, Acc. Chem. Res., 2015, 48, 286–294 CrossRef CAS PubMed
.
- S. Goel, C. G. England, F. Chen and W. Cai, Adv. Drug Delivery Rev., 2017, 113, 157–176 CrossRef CAS PubMed
.
- S. Mitragotri and J. Lahann, Nat. Mater., 2009, 8, 15–23 CrossRef CAS PubMed
.
- M. Motskin, D. M. Wright, K. Muller, N. Kyle, T. G. Gard, A. E. Porter and J. N. Skepper, Biomaterials, 2009, 30, 3307–3317 CrossRef CAS PubMed
.
- X. Zhao, S. Ng, B. C. Heng, J. Guo, L. Ma, T. T. Tan, K. W. Ng and S. C. Loo, Arch. Toxicol., 2013, 87, 1037–1052 CrossRef CAS PubMed
.
- Z. Liu, Y. Xiao, W. Chen, Y. Wang, B. Wang, G. Wang, X. Xu and R. Tang, J. Mater. Chem. B, 2014, 2, 3480–3489 RSC
.
- Q. Fan, Y. E. Wang, X. Zhao, J. S. C. Loo and Y. Y. Zuo, ACS Nano, 2011, 5, 6410–6416 CrossRef CAS PubMed
.
|
This journal is © The Royal Society of Chemistry 2018 |
Click here to see how this site uses Cookies. View our privacy policy here.