DOI:
10.1039/C7NR06373C
(Paper)
Nanoscale, 2018,
10, 132-141
Bacterial species-identifiable magnetic nanosystems for early sepsis diagnosis and extracorporeal photodynamic blood disinfection†
Received
26th August 2017
, Accepted 25th October 2017
First published on 26th October 2017
Abstract
Despite the numerous bacteria detection and elimination techniques available nowadays, sensitive diagnosis and treatment of sepsis (caused by the presence of bacteria in the bloodstream), especially at the early stage, remain big challenges. Here we report a nanosystem for early sepsis diagnosis and complete extracorporeal blood disinfection, based on iron oxide magnetic nanoparticles functionalized with chlorin e6 molecules and bacterial species-identifiable aptamers (Fe3O4-Ce6-Apt). We demonstrate that the Fe3O4-Ce6-Apt nanosystem can achieve simultaneous blood bacterial species identification and enrichment in a single step, and the enriched bacteria can be easily detected with the assistance of fluorescence microscopic determination. Based on this Fe3O4-Ce6-Apt nanosystem, successful diagnosis of sepsis caused by a single (Staphylococcus aureus) or multiple species (Staphylococcus aureus and Escherichia coli) of bacteria in mice has been realized. Compared to the gold standard blood culture method, this Fe3O4-Ce6-Apt nanosystem-based strategy has a comparable detection sensitivity (around 10 colony-forming units) but a significantly shortened diagnosis turnaround time (within 1.5 h), revealing its great potential for early sepsis diagnosis in clinical settings. Moreover, benefitting from the strong photodynamic effect of the Fe3O4-Ce6-Apt nanosystem, complete extracorporeal blood disinfection has been achieved. Remarkably, we also demonstrate that the disinfected blood can be reused for mice transfusion application without inducing adverse reactions, indicating the fruitful potential of the Fe3O4-Ce6-Apt nanosystem for sepsis treatment. Apart from the sepsis-associated applications, we believe that the Fe3O4-Ce6-Apt nanosystem could find wide applications in the fields of health and environmental sciences that require bacteria monitoring and sterilization.
Introduction
Sepsis, caused by the presence of pathogenic bacteria in the bloodstream, is a serious infection syndrome that can cause tissue damage and multisystem organ failure, resulting in patient morbidity and high mortality in the clinic.1–3 Sepsis has been recognized to be a significant worldwide concern because more than 18 million people worldwide are suffering from sepsis annually with a mortality rate reaching 28–50%.4,5 In addition, septic shock studies have shown that patient mortality increases dramatically, as much as 7.6% per hour, before the antibiotic administration.6 This condition is becoming even more devastating because of the rapid emergence of drug-resistant bacteria in recent years.7 A consensus has been reached among worldwide researchers that patient survival rate can be greatly increased if sepsis is effectively diagnosed and treated at the early stage.8 This situation raises the urgent need for the development of high performance strategies of rapid detection of pathogenic bacteria in the bloodstream, as well as efficient bacteria elimination without using antibiotics.
For bloodstream bacterial detection, blood culture and the polymerase chain reaction (PCR)-based assay are two typical strategies.9–12 However, the blood culture method usually requires laborious and time-consuming (typically several days) biochemical processes (e.g., long incubation duration, morphological analysis of bacterial colonies, and metabolite production measurement), which is far from satisfying the aim of rapid detection,9 while for the PCR-based assay, the complicated procedures (i.e., cell lysis, nucleic-acid extraction, and signal amplification) and occurrence of false-positive results become a hindrance to its practical diagnosis applications.11 Although several elegant techniques have been developed for sensitive detection of bacteria in bloodstream infections including surface-enhanced Raman scattering (SERS),13 mass spectrometry,14 and nuclear magnetic resonance (NMR),15 unfortunately, the complicated and expensive instruments required by these techniques make them impractical for bedside diagnosis. Hence, a rapid bacterial detection technique (i.e. within 2 h) with high accuracy for early sepsis diagnosis is urgently needed. Because of the extremely low concentration of bacteria (about 10–100 cells per mL) in the bloodstream and the co-existence of massive blood cells and proteins, bacterial enrichment and species identification are two crucial tasks in sepsis diagnosis.16,17 However, these two tasks are typically conducted separately through complicated procedures in the clinic, resulting in significantly prolonged turnaround time and low accuracy. Hence, the combination of bacterial enrichment and species identification in one step could be an efficient approach to achieve rapid and sensitive diagnosis of sepsis at the early stage. Considering the unique capability of magnetic nanosystems to rapidly enrich bacteria, as well as aptamer's excellent merit of accurate identification of targeted microbes,18–21 it is highly anticipated that aptamer-modified magnetic nanosystems can act as a promising platform for simultaneous bacterial enrichment and species identification, which are the ultimate functions for early sepsis diagnosis.
Regarding sepsis therapy, extracorporeal blood disinfection or cleaning has aroused great interest based on the fact that blood bacteria loading is the main cause of the mortality of patients with sepsis.22 By sterilizing the blood flowing from the sepsis patient and then transfusing it back, extracorporeal blood disinfection provides an efficient way for bacterial sepsis treatment.23–25 Several methods for extracorporeal blood disinfection have been developed, such as antibiotics,26 heat treatments,27 ultraviolet light irradiation,28 and photodynamic therapy (PDT).29,30 In particular, PDT exhibits antimicrobial activity toward drug-resistant bacteria and presents no risk to plasma, making it a promising solution for extracorporeal blood disinfection.29 However, the clinical applications based on PDT are still hampered because of the non-specific accumulation of photosensitizers in erythrocytes and platelets, which cause unexpected side effects.31 Based on these, we hypothesize that, by introducing photosensitizers into the magnetic nanosystem modified with bacterial species-identifiable aptamers, more efficient and specific PDT for extracorporeal blood disinfection can be achieved.
Herein, we present bacterial species-identifiable magnetic nanosystems for early sepsis diagnosis and efficient extracorporeal blood disinfection, based on iron oxide magnetic nanoparticles functionalized with chlorin e6 molecules and bacterial species-identifiable aptamers (Fe3O4-Ce6-Apt) (Fig. 1). The aptamer modification is employed to confer bacteria capture and species identification capabilities on the magnetic nanoparticles. Ce6 is a widely used photosensitizer that shows high photosensitizing efficacy and low dark toxicity.32 Polyethylene glycol (PEG) molecules are introduced onto the magnetic nanoparticles to increase their stability and biocompatibility.33 We demonstrate that the Fe3O4-Ce6-Apt nanosystem can achieve simultaneous blood bacterial species identification and magnetic enrichment in a single step, and the enriched bacteria can be easily detected with the assistance of fluorescence microscopic determination. Based on this Fe3O4-Ce6-Apt nanosystem, successful diagnosis of sepsis caused by a single (Staphylococcus aureus (S. aureus)) or multiple species (S. aureus and Escherichia coli (E. coli)) of bacteria in mice has been realized. Compared to the gold standard blood culture method, this Fe3O4-Ce6-Apt nanosystem-based strategy exhibits a comparable detection sensitivity (around 10 colony-forming units (CFU)) but a significantly shortened diagnosis turnaround time (within 1.5 h), revealing its great potential for early sepsis diagnosis in clinical settings. Moreover, benefitting from the strong photodynamic effect of the Fe3O4-Ce6-Apt nanosystem, complete extracorporeal blood disinfection can be achieved. It is worth noting that the disinfected blood can be reused for mice transfusion application without inducing adverse reactions, indicating the fruitful potential of the Fe3O4-Ce6-Apt nanosystem for sepsis treatment.
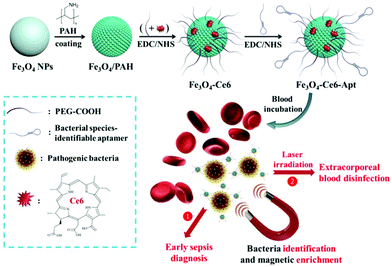 |
| Fig. 1 Preparation and conceptual illustration of the Fe3O4-Ce6-Apt nanosystem for early sepsis diagnosis and extracorporeal blood disinfection. | |
Results and discussion
Synthesis and characterization of the Fe3O4-Ce6-Apt nanosystem
The Fe3O4-Ce6-Apt nanosystem was prepared by first coating negatively charged iron oxide magnetic nanoparticles (Fe3O4 NPs) with the PAH polyelectrolyte, followed by subsequent conjugations of Ce6, PEG, and aptamer molecules through a chemical covalent coupling method (Fig. 1).34,35 PEG modification was employed to increase the biocompatibility and stability of the Fe3O4-Ce6-Apt nanosystem in blood.33Fig. 2a depicts a transmission electron microscopy (TEM) image of the Fe3O4-Ce6-Apt nanosystem, and its solution under external magnet imposition. It was found that the prepared Fe3O4-Ce6-Apt nanosystem exhibited strong magnetic responses, and had a homogeneous spherical structure with an average size of 17.2 ± 1.5 nm. Compared to micro-sized magnetic particles such as the commercial Dynabeads products, the nano-sized Fe3O4-Ce6-Apt has higher surface to volume ratio and thus exhibits a higher binding capacity to targets.36 The UV-vis absorption spectrum results showed that the Fe3O4-Ce6-Apt nanosystem possessed the characteristic absorption peaks (at 403 and 664 nm) of free Ce6,37 indicating the successful conjugation of Ce6 (Fig. 2b). From the agarose gel electrophoresis of the Fe3O4-Ce6-Apt nanosystem, it was found that the migration of aptamer in Fe3O4-Ce6-Apt was completely retarded, confirming the successful linkage of aptamer (Fig. S1†).38 In addition, the whole preparation process of the Fe3O4-Ce6-Apt nanosystem could be monitored and confirmed by the increased hydrodynamic size (Fig. S2†) and decreased zeta potential (Fig. 2c). The considerably small zeta potential (around −10 mV) of the Fe3O4-Ce6-Apt nanosystem made electrostatic interaction between Fe3O4-Ce6-Apt and blood components difficult, which is quite important to minimize the effect of the Fe3O4-Ce6-Apt nanosystem on blood components during sepsis diagnosis and blood disinfection.
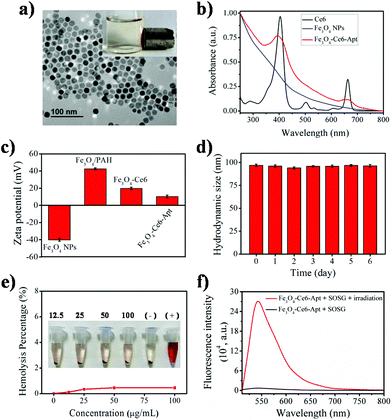 |
| Fig. 2 Characterization of the Fe3O4-Ce6-Apt nanosystem. (a) TEM image of Fe3O4-Ce6-Apt. The inset shows the Fe3O4-Ce6-Apt solution with an external magnet imposition. (b) UV-vis absorption spectra of Ce6, Fe3O4 NPs and Fe3O4-Ce6-Apt. (c) Zeta potentials of Fe3O4 NPs, Fe3O4/PAH, Fe3O4-Ce6 and Fe3O4-Ce6-Apt in DI water. (d) Hydrodynamic sizes of Fe3O4-Ce6-Apt in blood during 6 days of storage. (e) The haemolytic assay of RBCs after incubation with the Fe3O4-Ce6-Apt at various concentrations for 4 h. In this assay, the DI water and PBS buffer were used as the positive and negative controls, respectively. (f) Fluorescence spectra of SOSG (10 nmol) in the Fe3O4-Ce6-Apt solution before and after NIR laser (660 nm, 0.8 W cm−2) irradiation for 5 min. SOSG normally shows weak blue fluorescence, but emits strong green fluorescence in the presence of 1O2. In the experiments, the aptamer toward S. aureus was used to prepare the Fe3O4-Ce6-Apt nanosystem. | |
The stability of the Fe3O4-Ce6-Apt nanosystem was investigated by dynamic light scattering measurements. As shown in Fig. 2d, no significant increase of hydrodynamic size was found for the Fe3O4-Ce6-Apt nanosystem during 6 days of storage in blood, revealing its high structural stability and excellent solubility. In addition, the potential toxicity of the Fe3O4-Ce6-Apt nanosystem toward red blood cells (RBCs) was evaluated by a haemolytic assay. It was found that no obvious hemolysis of RBCs was observed even after incubation with the Fe3O4-Ce6-Apt nanosystem at high concentration (100 μg mL−1), pointing to good blood biocompatibility of the nanosystem (Fig. 2e). Moreover, the microbial proliferation in the presence of the Fe3O4-Ce6-Apt nanosystem was assessed to evaluate the potential toxicity of Fe3O4-Ce6-Apt toward bacteria. As shown in Fig. S3,† bacterial growth in the presence of Fe3O4-Ce6-Apt was similar to the control experiment, indicating that the Fe3O4-Ce6-Apt nanosystem did not exhibit an antimicrobial effect, which was a prerequisite for bacteria detection. Furthermore, the capability of the Fe3O4-Ce6-Apt nanosystem to generate singlet oxygen (1O2) under near-infrared (NIR) laser irradiation was investigated to evaluate its photodynamic performance. As depicted in Fig. 2f, strong green fluorescence of singlet oxygen sensor green (SOSG), a widely used indicator of 1O2,39 was detected in the Fe3O4-Ce6-Apt nanosystem upon irradiation, indicating the outstanding 1O2 generation capability of the Fe3O4-Ce6-Apt nanosystem for subsequent blood photodynamic disinfection. Compared to free Ce6 molecules, the Fe3O4-Ce6-Apt nanosystem was found to exhibit significantly enhanced photostability under laser irradiation, indicating the protective role of Fe3O4 NP carriers in preventing the photobleaching of Ce6 under irradiation (Fig. S4†).
Fe3O4-Ce6-Apt nanosystem-based bacterial identification and enrichment
To evaluate the bacteria identification and enrichment performance of the Fe3O4-Ce6-Apt nanosystem, separation of bacteria-spiked blood samples after incubation with the Fe3O4-Ce6-Apt nanosystem and magnetic enrichment was carried out. S. aureus, the most fatal pathogen in sepsis,40 was chosen as the model bacterium and the aptamer toward S. aureus was used to prepare the Fe3O4-Ce6-Apt nanosystem. As shown in Fig. 3a, the Fe3O4-Ce6-Apt nanosystem exhibited an incubation time-dependent bacterial separation, and nearly complete bacterial enrichment was achieved within short times (about 60 min). Consistently, a large number of Fe3O4-Ce6-Apt particles (as indicated by red arrows in Fig. 3b) were clearly observed on the surface of bacteria from the TEM image of enriched S. aureus, confirming the capability of the Fe3O4-Ce6-Apt nanosystem for robust bacterial identification and enrichment. In addition, the Fe3O4-Ce6-Apt nanosystem demonstrated higher capability to enrich bacteria, by up to ten times, than the Fe3O4-Ce6 (without aptamer modification), indicating the crucial role of aptamer in bacterial identification and capture (Fig. S5†). Moreover, the selectivity of the prepared Fe3O4-Ce6-Apt nanosystem for S. aureus identification and enrichment was studied. As shown in Fig. 3c, only the S. aureus (as indicated by red arrows) with a round shape was fluorescently labelled in the mixture of S. aureus and E. coli after incubation with the FITC dye-modified Fe3O4-Ce6-Apt nanosystem, confirming the identification selectivity of the Fe3O4-Ce6-Apt nanosystem. Consistently, based on the Fe3O4-Ce6-Apt nanosystem, a much higher enrichment efficiency (around 95%) of S. aureus was achieved than that of E. coli (below 13%), indicating robust enrichment selectivity of the Fe3O4-Ce6-Apt nanosystem (Fig. 3d).
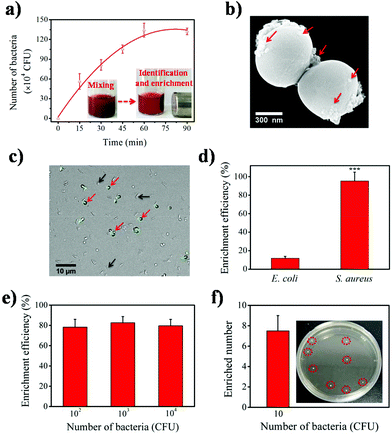 |
| Fig. 3 Fe3O4-Ce6-Apt nanosystem-based bacterial identification and enrichment. (a) Quantitative evaluation of the enriched S. aureus numbers at different incubation times. The insets depict the S. aureus (106 CFU)-spiked blood samples incubated with Fe3O4-Ce6-Apt before and after external magnet imposition. (b) Representative TEM image of the S. aureus cell enriched with the Fe3O4-Ce6-Apt. (c) Overlapping bright field and fluorescence microscopy images of the mixture of E. coli (106 CFU, indicated by a black arrow) and S. aureus (106 CFU, indicated by a red arrow) after incubation with the Fe3O4-Ce6-Apt for 60 min. (d) Quantitative evaluation of the enrichment efficiency of the Fe3O4-Ce6-Apt nanosystem toward E. coli (106 CFU) and S. aureus (106 CFU), respectively. The enrichment efficiency is defined as the proportion of bacteria enriched from the test blood samples. (e) S. aureus enrichment efficiencies of the Fe3O4-Ce6-Apt nanosystem at low bacterial concentrations ranging from 102 to 104 CFU in blood. (f) Assay of the Fe3O4-Ce6-Apt nanosystem for bacterial identification and enrichment under trace concentration (10 CFU). The inset shows one of the agar plates of the enriched bacteria. In (a), (d), (e) and (f), the values for enrichment efficiency and bacteria number represent the mean of three independent experiments and the error bars indicate the standard deviation (SD) from the mean. ***P < 0.001. | |
It is worth mentioning that the concentration of bacteria used in the aforementioned tests was relatively high (about 106 CFU). To evaluate the performance of the Fe3O4-Ce6-Apt nanosystem, bacteria enrichment at low bacterial concentrations was necessary and further examined. As shown in Fig. 3e, for low bacterial concentrations from 102 to 104 CFU, the Fe3O4-Ce6-Apt nanosystem still possessed considerably strong capability for bacteria enrichment with efficiency as high as 80%. Remarkably, the Fe3O4-Ce6-Apt nanosystem enabled efficient bacteria identification and enrichment even at trace concentration (10 CFU) at which around seven bacteria were captured. These results solidly revealed the extremely high sensitivity of the proposed nanosystem (Fig. 3f). More importantly, the performance of the Fe3O4-Ce6-Apt nanosystem was found to be not influenced by the bacterial medium (e.g., DI water, PBS buffer, DMEM cell medium and blood), which was very important for real applications that may involve different clinical samples (Fig. S6†).
Fe3O4-Ce6-Apt nanosystem-based early sepsis diagnosis
By exploiting the outstanding capability of the Fe3O4-Ce6-Apt nanosystem for bacterial identification and enrichment, we proposed a simple but efficient strategy for rapid sepsis diagnosis. As depicted in Fig. 4a, the sepsis blood sample was first incubated with the Fe3O4-Ce6-Apt nanosystem followed by magnetic separation to achieve simultaneous bacteria identification and enrichment. Subsequently, the enriched bacteria were stained using a commercial bacteria-targeted dye (SYTO 9 with green color) and imaged by fluorescence microscopy to quantitatively evaluate the number of enriched bacteria. This whole process can be completed in 1.5 h, and thus is very suitable for early sepsis diagnosis. To demonstrate the feasibility of this Fe3O4-Ce6-Apt nanosystem-based strategy for sepsis diagnosis, the blood-infected mice model was constructed by intravenously injecting 100 CFU of S. aureus bacteria into the bloodstream of mice through the tail vein, and sepsis blood samples were collected from the orbital venous plexus of infected mice 1 h later. As shown in Fig. 4b, for the sepsis blood sample with an extremely low concentration of S. aureus (8 CFU as shown in the agar plate after gold standard bacterial blood culture procedures), bacterial cells (3 bacteria were observed and determined from the fluorescence microscopy images) were successfully detected by the Fe3O4-Ce6-Apt nanosystem-based strategy. The reduced bacterial number was attributed to the loss of bacterium during the processes of magnetic enrichment and SYTO 9 dye staining. Moreover, parallel trials on sepsis blood samples were conducted to confirm the practicability of the Fe3O4-Ce6-Apt nanosystem-based strategy, and the results are summarized in Fig. 4c. It was found that, for all five sepsis blood samples, the Fe3O4-Ce6-Apt nanosystem-based strategy provided consistent results in 1.5 h, compared to the gold standard blood culture method that typically takes 20–24 h. It is worth noting that the bacterial concentrations used in our current studies (from 6 to 12 CFU) were at the lower limit of real early sepsis in the clinic (about 10–100 CFU mL−1).16 This highlights the high performance of the Fe3O4-Ce6-Apt nanosystem-based strategy for early sepsis diagnosis with a high accuracy but a significantly shortened turnaround time.
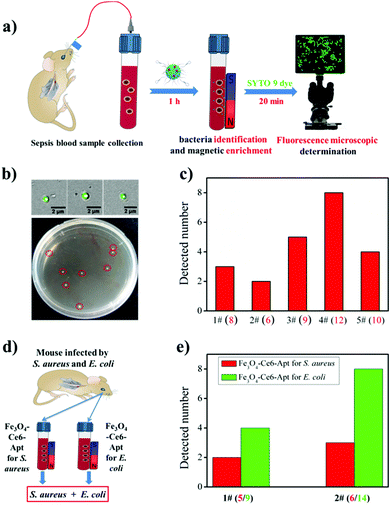 |
| Fig. 4 Fe3O4-Ce6-Apt nanosystem-based early sepsis diagnosis. (a) Illustration of the process of the Fe3O4-Ce6-Apt nanosystem-based strategy for sepsis diagnosis. (b) The fluorescence microscopic result of one S. aureus sepsis blood sample based on the Fe3O4-Ce6-Apt nanosystem-based strategy, and the corresponding agar plate result of the same S. aureus sepsis blood sample using the gold standard bacterial blood culture method. (c) The detected bacterial numbers of five parallel S. aureus sepsis blood samples obtained by the Fe3O4-Ce6-Apt nanosystem-based strategy. The red-color values in brackets represent the numbers of bacterial colonies grown on the agar plate of the five S. aureus sepsis blood samples using the gold standard blood culture method. (d) Illustration of the application of the Fe3O4-Ce6-Apt nanosystem array for the diagnosis of sepsis caused by a mixture of S. aureus and E. coli species. (e) The detected bacterial numbers of two blood samples of sepsis caused by a mixture of S. aureus and E. coli species obtained by the Fe3O4-Ce6-Apt array-based strategy. The red and green color values in brackets represent the corresponding numbers of bacterial colonies of S. aureus and E. coli of the two sepsis blood samples, respectively, grown on the agar plate using the gold standard bacterial blood culture method. | |
In addition to the diagnosis of sepsis caused by a single bacterial species, the performance of the Fe3O4-Ce6-Apt nanosystem-based strategy for the analysis of multiple bacterial species-caused sepsis was evaluated. Here, the sepsis blood was collected from the mice that were infected by the mixture of S. aureus and E. coli, two common species in clinical sepsis,40,41 as demonstrated in Fig. 4d. For the two parallel trials, the Fe3O4-Ce6-Apt nanosystem-based strategy provided consistent results like the gold standard blood culture method but in shorter time than we expected, indicating its great potential for complicated sepsis diagnosis (related results can be found in Fig. S7† and Fig. 4e). Inspired by these findings, the array of Fe3O4-Ce6-Apt nanosystems with specificity for the main pathogen of sepsis (e.g., S. aureus, E. coli, Streptococcus pyogenes, and Pseudomonas aeruginosa) would be constructed;42,43 thus rapid detection of unknown sepsis samples could be achieved without the need for blood culture. This would provide an accurate and quick technique for the clinical applications.
Fe3O4-Ce6-Apt nanosystem-based extracorporeal photodynamic blood disinfection
By transfusing the sterilized sepsis blood back to the patient, extracorporeal blood disinfection provides a promising approach for bacterial sepsis treatment.24 From the above studies, the Fe3O4-Ce6-Apt nanosystem was identified to exhibit high activity in 1O2 generation (Fig. 2f), as well as bacterial identification and enrichment (Fig. 3). Thus we hypothesized that the Fe3O4-Ce6-Apt nanosystem could also be used for extracorporeal blood disinfection based on bacteria-targeted photodynamic therapy. To assess this, a two-step extracorporeal blood disinfection assay (photo-killing plus magnetic removal) was designed based on the Fe3O4-Ce6-Apt nanosystem (Fig. 5a). The S. aureus-spiked blood sample was incubated with the Fe3O4-Ce6-Apt nanosystem for 1 h and then irradiated with a NIR laser. The performance of the Fe3O4-Ce6-Apt nanosystem for photodynamic eradication of blood bacteria was summarized in Fig. 5b. It was clear that no S. aureus colony was found in the agar plate of S. aureus-spiked blood after Fe3O4-Ce6-Apt incubation and laser irradiation, indicating the death of bacteria. Furthermore, the antimicrobial activity of the Fe3O4-Ce6-Apt nanosystem triggered by its photodynamic behaviour was validated based on scanning electron microscopy (SEM)-based bacterial morphology studies and the live/dead bacterial staining assay, and the results are summarized in Fig. 5c. In detail, normal S. aureus cells in blood, after incubation with the Fe3O4-Ce6-Apt nanosystem but prior to laser irradiation, emitted green color and had smooth bodies. In contrast, S. aureus cells after incubation with the Fe3O4-Ce6-Apt nanosystem and laser irradiation were stained yellow (the overlapping of green and red colors) and had a lysed morphology. These findings clearly confirmed the photodynamic antimicrobial activity of the Fe3O4-Ce6-Apt nanosystem through disrupting the bacterial cell wall and membrane.44 In addition, we found that the temperature increase of the blood sample incubated with the Fe3O4-Ce6-Apt nanosystem by laser irradiation was negligible, only 1.5 °C (Fig. S8†). Hence this Fe3O4-Ce6-Apt nanosystem-based photodynamic treatment would not cause direct heat damage to blood components. Remarkably, S. aureus-specific photodynamic elimination in sepsis blood samples containing both S. aureus and E. coli species was realized based on the Fe3O4-Ce6-Apt nanosystem toward S. aureus (for details please refer to Fig. S9 and Fig. S10†). This unique bacterial species-targeted photodynamic antimicrobial performance is believed to be very useful in clinical anti-infection fields because efficient pathogen elimination without affecting the probiotics in the human body is still a big challenge.45
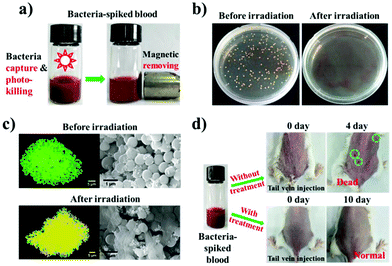 |
| Fig. 5 Fe3O4-Ce6-Apt nanosystem-based extracorporeal photodynamic blood disinfection. (a) Fe3O4-Ce6-Apt nanosystem-based two-step extracorporeal blood disinfection assay. (b) Agar plate photographs and (c) representative SEM and overlapping fluorescence images for the live/dead bacterial staining assay of the S. aureus (106 CFU)-spiked blood samples incubated with the Fe3O4-Ce6-Apt nanosystem (50 μg mL−1 of iron element) before and after NIR laser (660 nm, 0.8 W cm−2) irradiation for 5 min. Two fluorescent dyes were used in the live/dead bacterial staining assay in which SYTO 9 with green color labelled both live and dead bacteria, while propidium iodide with red color only stained dead bacteria. (d) Photographs of the mice transfused with S. aureus (106 CFU)-spiked blood samples with and without disinfection treatment at different time points post transfusion. | |
After photo-killing of bacteria, removal of the dead bacteria cells is a necessity because they would cause a fatal immune response.46 In addition, compared to the extracorporeal blood disinfection assay based on pure magnetic separation, our proposed two-step (photo-killing plus magnetic removing) strategy showed enhanced disinfection results (Fig. S11†). To evaluate the feasibility of Fe3O4-Ce6-Apt nanosystem-based extracorporeal blood disinfection for sepsis therapy, the S. aureus-spiked blood samples after disinfection treatment were intravenously transfused back to the mice from which the blood samples were collected, and the mice growth was monitored. As shown in Fig. 5d, for the control group in which the mice were transfused with the S. aureus-spiked blood samples (no disinfection treatment), mice death was found at 4 day post-transfusion with numerous red spots (indicated by green circles) being observed on the skin. After dissection and tissue bacterial culture with the gold standard bacterial culture method, a large number of bacterial colonies were found in the agar plates of blood, liver and lung of the transfused mice, indicating the sepsis-induced multisystem organ failure and death (Fig. S12†). In contrast, for the mice transfused with S. aureus-spiked blood samples with disinfection treatment, mice growth was not influenced and no bacterial colony was observed in the agar plates of tissues (Fig. 5d and Fig. S12†). These revealed the successful extracorporeal blood disinfection of the Fe3O4-Ce6-Apt nanosystem for sepsis therapy. Based on these results, we believe that the Fe3O4-Ce6-Apt nanosystem could be safely incorporated with microfluidics for the development of automated extracorporeal blood disinfection devices for clinical sepsis therapy.47
Experimental
Chemicals and reagents
Iron(III) chloride hexahydrate (FeCl3·6H2O), sodium oleate, 1-octadecene, poly(allylamine hydrochloride) (PAH; Mw = 15
000 Da), phosphate buffered saline (PBS), Tris-acetate-EDTA (TAE) buffer, N-(3-(dimethylamino)propyl-N′-ethylcarbodiimide) hydrochloride (EDC), N-hydroxysulfosuccinimide sodium salt (NHS), Luria–Bertani broth medium (LB) and tetramethylammonium hydroxide were obtained from Sigma-Aldrich. Carboxyl-terminated PEG (mPEG-COOH; Mw = 5000 Da) was purchased from Bomei Biotechnology Company. Chlorin e6 (Ce6) was obtained from J&K Chemical, and the bacterial species-identifiable aptamers were obtained from Sangon Biotech. The live/dead bacterial staining agent, gel red and singlet oxygen sensor green (SOSG) were purchased from Thermo Fisher. MS-6 agarose and 5× loading buffer for agarose gel electrophoresis were purchased from Takara Biotechnology. All other chemicals were obtained from Adamas-beta, and used without further purification. Deionized (DI) water (Millipore Milli-Q grade, 18.2 MΩ) was used in all the experiments.
Characterization
The morphology and size of the Fe3O4-Ce6-Apt nanosystem were observed using a transmission electron microscope (TEM) (Tecnai G20, FEI). The UV-vis absorption and fluorescence were monitored using a UV-vis spectrophotometer (UV-3600, Shimadzu) and a fluorescence spectrometer (FLS980, Edinburgh), respectively. The hydrodynamic size and zeta potential of the Fe3O4-Ce6-Apt nanosystem were recorded using a nanosizer (Zetasizer Nano ZS90, Malvern). Confocal fluorescence images were taken by utilizing a confocal laser scanning microscope (FV1200, Olympus). The concentration of iron element in the Fe3O4-Ce6-Apt nanosystem was determined by inductively coupled plasma mass spectrometry (ICP-MS) (ELEMENT 2, Thermo).
Preparation of magnetic Fe3O4 nanoparticles
Magnetic Fe3O4 nanoparticles were synthesized by thermal decomposition of the iron–oleate complex according to a previously reported method.48 First, 2.7 g of FeCl3·6H2O and 9.125 g of sodium oleate were added to a mixture containing 20 mL of ethanol, 15 mL of deionized (DI) water and 35 mL of hexane. The mixture was kept in a round bottle flask and refluxed at 70 °C for 4 h to generate the iron–oleate complex. Then the resulting brown solution was transferred to a separatory funnel, and washed three times with DI water. To remove hexane, the solution was then evaporated for another 2 h, yielding a dark brown iron–oleate complex. 4.5 g of the iron–oleate complex obtained was then dissolved in a mixture containing 12.5 g of 1-octadecene and 0.76 g of sodium oleate, and the reaction mixture was heated at 320 °C for 30 min in the presence of nitrogen. Finally, the generated black solution was naturally cooled to room temperature, and 25 mL of 2-propanol was added to precipitate the Fe3O4. The obtained magnetic nanoparticles were rinsed with hexane and ethanol and finally dispersed in 5 mL of hexane for subsequent experiments.
To make the prepared Fe3O4 nanoparticles water soluble, an equal volume of ethanol was added to a hexane dispersion of Fe3O4 nanoparticles, and the mixture was magnetically decanted. After adding 0.2 M of tetramethylammonium hydroxide to the precipitate, the solution was shaken for 5 min. 2-Propanol was then added and the mixture solution was magnetically decanted again. Finally, the Fe3O4 nanoparticles were washed with acetone, and dispersed in DI water for subsequent PAH coating.
Preparation of the Fe3O4-Ce6-Apt nanosystem
PAH coating of Fe3O4 nanoparticles was implemented following a method published previously.49 In brief, 0.1 g of PAH was dissolved in 10 mL of a 1 mM NaCl solution, followed by the addition of 1 mL of an as-prepared Fe3O4 nanoparticle solution. The mixture was stirred at a speed of 900 rpm for 3 h at room temperature. Lastly, the generated PAH-coated Fe3O4 nanoparticles (Fe3O4/PAH) were centrifuged at 12
000 rpm for 15 min and dispersed in 1 mL of DI water. In order to prepare Fe3O4-Ce6, mPEG-COOH and Ce6 were covalently conjugated to the amino group of PAH based on the EDC/NHS covalent coupling method.34,50 First, 12 mg of mPEG-COOH and 0.3 mg of Ce6 were dissolved in a mixture of dimethylformamide (DMF) and DI water (2 mL, VDMF
:
VDI water = 3
:
1), and then 4.1 mg of EDC and 4.5 mg of NHS were added to activate the carboxyl group. After 30 min, 500 μL of Fe3O4/PAH nanoparticles were added to the mixture and stirred for 24 h. The Fe3O4-Ce6 nanoparticles were finally obtained by centrifugation at 12
000 rpm for 15 min and re-dispersed in DI water for subsequent aptamer functionalization.
The Fe3O4-Ce6-Apt nanosystem was prepared by modifying the Fe3O4-Ce6 nanoparticles with bacterial species-identifiable aptamers (aptamers for S. aureus: 5′-HOOC-GCTAACCCCCCCAGTCCGTCCTCCCAGCCTCACACCGCCA-3′; aptamers for E. coli: 5′-HOOC-ACACCATAATATGCCGTAAGGAGAGGCCTGTTGGGAGCGCCGTAGAG-3′).51,52 First, 2.9 nmol of aptamers were dissolved in 100 μL of DI water, and then 0.0056 mg of EDC and 0.0063 mg of NHS were added to activate the carboxyl group of aptamers for 30 min. Afterwards, 1 mL of the prepared Fe3O4-Ce6 solution was added and stirred for 24 h. Finally, the Fe3O4-Ce6-Apt nanosystem was collected through centrifugation and suspended in DI water for further characterization and application.
Agarose gel electrophoresis
The aptamer modification of the Fe3O4-Ce6-Apt nanosystem was analyzed by agarose gel electrophoresis using the literature reported procedures.38 First, 20 μL of the Fe3O4-Ce6-Apt nanosystem (1 mg mL−1 of iron element) was mixed with 4 μL of 5× loading buffer, and 15 μL of the mixture solution was then loaded onto 2% agarose gel containing 2.5 μL of gel red. Then, we conducted the agarose gel electrophoresis in 1× TAE running buffer for 40 min at a voltage of 110 V. Finally, the experimental results were recorded under UV light using a Gel Image System (Gel Doc 2000, Bio-Rad).
Hematolysis experiment
To investigate the potential toxicity of the Fe3O4-Ce6-Apt nanosystem on red blood cells, a hematolysis experiment was performed. First, 1.2 mL of fresh blood extracted from the eyeball of healthy mice was stabilized in EDTA solution, and subsequently separated by centrifugation at a speed of 2000 rpm for 10 min. After centrifugation, the upper blood serum was removed and the precipitate was washed several times with PBS (pH 7.4) to obtain red blood cells. Then, the diluted red cell suspension (20% in PBS) was mixed with a series of different concentrations (12.5, 25, 50, and 100 μg mL−1 of iron element) of the Fe3O4-Ce6-Apt nanosystem. The red cell samples in DI water and PBS buffer were used as the positive and negative controls, respectively. All the samples were incubated at 37 °C for 2 h. After that, the samples were centrifuged at 2000 rpm for 10 min and the absorbance of supernatants at 540 nm was measured using a microplate reader.
Singlet oxygen generation from the Fe3O4-Ce6-Apt nanosystem
The SOSG reagent was used to investigate the singlet oxygen generation capability of the Fe3O4-Ce6-Apt nanosystem. In brief, we irradiated the Fe3O4-Ce6-Apt nanosystem (50 μg mL−1 of iron element) using a NIR laser (660 nm, 0.8 W cm−2) for 5 min, and then 20 μL of SOSG (500 μM) was added. The generation of singlet oxygen from the Fe3O4-Ce6-Apt nanosystem could be quantitatively assessed by the analysis of the fluorescence spectrum of SOSG.
Bacteria culture
Bacteria S. aureus (ATCC 6538) and E. coli (ATCC 8739) were used in the experiments. The bacteria were cultured in LB medium at 37 °C overnight, and harvested at the exponential growth phase through centrifugation. The concentration of bacteria could be monitored photometrically by measuring the optical density (OD) at a wavelength of 600 nm, in which an OD600 value of 0.1 corresponds to the concentrations of 2 × 108 and 4 × 108 CFU mL−1 for S. aureus and E. coli, respectively.
Effect of the Fe3O4-Ce6-Apt nanosystem on bacteria growth
By incubating the Fe3O4-Ce6-Apt nanosystem (50 μg mL−1 of iron element) with S. aureus LB solutions (105 CFU) on 96 well plates at 37 °C and measuring the OD600 values of the mixture at different time intervals, the effect of the Fe3O4-Ce6-Apt nanosystem on bacterial growth was systematically investigated. The S. aureus LB solution (105 CFU mL−1) without the Fe3O4-Ce6-Apt nanosystem incubation was used as the control.
Fe3O4-Ce6-Apt nanosystem-based bacterial identification and enrichment
Fresh whole blood obtained from the eyeball of healthy mice was spiked with different concentrations of bacteria (10, 102, 103, 104, 105, and 106 CFU mL−1) to produce bacteria-contaminated blood samples. For bacterial identification and enrichment experiments, the Fe3O4-Ce6-Apt nanosystem (50 μg mL−1 of iron element) was incubated with 1 mL of bacteria-contaminated blood samples for different times (15, 30, 45, 60, and 90 min) at 37 °C. After incubation, an external magnet was applied to achieve magnetic enrichment of the identified bacteria. The enrichment efficiency (C%) is defined as the proportion of bacteria separated from the test blood samples, and it is calculated based on the equation: C% = 1 − (Na/Nb). In this equation, Na and Nb represent the number of bacteria in the test blood samples after and before magnetic enrichment, respectively, and they were determined using the standard colony counting method in our experiments.
Early sepsis diagnosis in mice
For early sepsis diagnosis, a mice model of sepsis was created by injecting 50 μL of bacterial PBS suspension (100 CFU, single S. aureus or mixed S. aureus and E. coli) into the bloodstream of Balb/c mice through the tail vein. At 1 h post infection, 400 μL of sepsis blood samples were collected from the orbital venous plexus of infected mice. In the sepsis diagnosis experiments, the Fe3O4-Ce6-Apt nanosystem (50 μg mL−1 of iron element) was incubated with the test blood samples at 37 °C for 1 h followed by magnetic enrichment. Subsequently, the acquired precipitates after enrichment were mixed with 100 μL of SYTO 9 dye PBS solution (10 nM) for 20 min to label the enriched bacteria, and fluorescence microscopy was employed to determine the number of enriched bacteria. In the above experiments, the female Balb/c mice (6 weeks, 20 g) used were obtained from Nanjing Peng Sheng Biological Technology Co. Ltd, and were allowed to acclimatize for one week in the lab before experiment. All animal experiments were performed in compliance with the protocols approved by the Soochow University Laboratory Animal Center.
Extracorporeal photodynamic blood disinfection
1 mL of fresh blood extracted from the orbital venous plexus of one selected healthy mouse was spiked with 106 CFU of bacteria (single S. aureus or mixed S. aureus and E. coli) to produce bacteria-contaminated blood samples. For extracorporeal blood disinfection, the bacteria-contaminated blood sample was incubated with our Fe3O4-Ce6-Apt nanosystem (50 μg mL−1 of iron element) at 37 °C for 1 h, and the mixture was then treated with near-infrared (NIR) laser (660 nm, 0.8 W cm−2) irradiation for 5 min to inactivate the bacteria. The antimicrobial performance was assessed by SEM-based bacterial morphology studies and the live/dead bacterial staining assay. To investigate the potential of the Fe3O4-Ce6-Apt nanosystem-based extracorporeal blood disinfection method for sepsis therapy, 50 μL of the disinfected blood mentioned above was intravenously transfused back to the mice from which the blood samples were collected. The growth conditions of the transfused mice were monitored in real time, and mice dissection and tissue bacterial culture were carried out at specific time post transfusion. Healthy mice transfused with no treated sepsis blood samples were used as the control.
SEM-based morphology studies of bacteria
The morphology of bacteria in the bacteria-contaminated blood samples before and after NIR laser irradiation was examined by field-emission scanning electron microscopy (FESEM). In brief, bacteria-contaminated blood samples incubated with the Fe3O4-Ce6-Apt nanosystem before and after NIR laser irradiation were fixed with 2% glutaraldehyde for 2 h, respectively. Then, the samples were dehydrated with a series of ethanol solutions (50%, 70%, 90%, and 100%) for 10 min at each step. Lastly, the dehydrated samples were dropped on silicon wafers and imaged via FESEM after nitrogen drying and platinum coating.
Live/dead bacterial staining assay
The bacteria-contaminated blood samples incubated with Fe3O4-Ce6-Apt nanosystem before and after NIR laser irradiation were incubated with 100 μL of a dye solution containing 1 μM SYTO 9 and 1 μM propidium iodide. After 20 min incubation, the bacteria were imaged through a confocal laser scanning microscope. The SYTO 9 dye with green color can stain both intact (live) and membrane-damaged (dead) bacteria, while the propidium iodide dye with red color can only stain membrane-damaged (dead) bacteria.
Conclusions
In summary, we report a strategy, based on a bacterial species-identifiable magnetic nanosystem (Fe3O4-Ce6-Apt), for early sepsis diagnosis and extracorporeal photodynamic blood disinfection. Our results demonstrate that the Fe3O4-Ce6-Apt nanosystem can simultaneously achieve blood bacterial species identification and magnetic enrichment in a single step, and the enriched bacteria can be easily detected with the assistance of fluorescence microscopic determination. Based on this Fe3O4-Ce6-Apt nanosystem, successful diagnosis of sepsis caused by a single (S. aureus) or multiple species (S. aureus and E. coli) of bacteria in mice has been realized. Compared to the gold standard blood culture method, the Fe3O4-Ce6-Apt nanosystem-based strategy provides an equally high detection sensitivity (around 10 CFU) but a significantly shortened diagnosis turnaround time (within 1.5 h), revealing its great potential for effective early stage sepsis diagnosis in clinical settings. Moreover, benefitting from the strong photodynamic effect of the Fe3O4-Ce6-Apt nanosystem, complete extracorporeal blood disinfection has been achieved. Remarkably, the disinfected blood can be reused for mice transfusion application without inducing adverse reactions, indicating the potential applications of the Fe3O4-Ce6-Apt nanosystem for sepsis treatment. Apart from the sepsis-related applications, the Fe3O4-Ce6-Apt nanosystem could find wide applications in the fields of health and environmental sciences that require bacteria monitoring and sterilization.
Conflicts of interest
These are no conflicts of interest to declare.
Acknowledgements
This work was supported by the National Natural Science Foundation of China (31500802, 21628201, and 21405108), the Natural Science Foundation of Jiangsu Province (BK20150350 and BK20141170), and a project funded by the Priority Academic Program Development of Jiangsu Higher Education Institutions (PAPD). This work was also supported by the 333 Project of Jiangsu Province.
References
- J. Cohen, Nature, 2002, 420, 885–891 CrossRef CAS PubMed
.
- R. S. Hotchkiss and I. E. Karl, N. Engl. J. Med., 2003, 348, 138–150 CrossRef CAS PubMed
.
- R. C. Bone, Ann. Intern. Med., 1991, 115, 457–469 CrossRef CAS PubMed
.
- M. Goto and M. N. Al-Hasan, Clin. Microbiol. Infect., 2013, 19, 501–509 CrossRef CAS PubMed
.
- D. C. Angus, W. T. Linde-Zwirble, J. Lidicker, G. Clermont, J. Carcillo and M. R. Pinsky, Crit. Care Med., 2001, 29, 1303–1310 CrossRef CAS PubMed
.
- D. M. Yealy, J. A. Kellum, D. T. Huang, A. E. Barnato, L. A. Weissfeld, F. Pike, T. Terndrup, H. E. Wang, P. C. Hou, F. LoVecchio, M. R. Filbin, N. I. Shapiro and D. C. Angus, N. Engl. J. Med., 2014, 370, 1683–1693 CrossRef CAS PubMed
.
- B. M. Geilich, A. L. van de Ven, G. L. Singleton, L. J. Sepulveda, S. Sridhar and T. J. Webster, Nanoscale, 2015, 7, 3511–3519 RSC
.
- H. Shen, J. Wang, H. Liu, Z. Li, F. Jiang, F.-B. Wang and Q. Yuan, ACS Appl. Mater. Interfaces, 2016, 8, 19371–19378 CAS
.
- B. S. Reisner and G. L. Woods, J. Clin. Microbiol., 1999, 37, 2024–2026 CAS
.
- N. Mancini, S. Carletti, N. Ghidoli, P. Cichero, R. Burioni and M. Clementi, Clin. Microbiol. Rev., 2010, 23, 235–251 CrossRef CAS PubMed
.
- T. Meyer, G. Franke, S. K. A. Polywka, M. Lutgehetmann, J. Gbadamosi, T. Magnus and M. Aepfelbacher, J. Clin. Microbiol., 2014, 52, 1751–1753 CrossRef CAS PubMed
.
- K. Hsieh, B. S. Ferguson, M. Eisenstein, K. W. Plaxco and H. T. Soh, Acc. Chem. Res., 2015, 48, 911–920 CrossRef CAS PubMed
.
- M. R. Hoonejani, A. Pallaoro, G. B. Braun, M. Moskovits and C. D. Meinhart, Nanoscale, 2015, 7, 16834–16840 RSC
.
- R. A. Allardyce, V. S. Langford, A. L. Hill and D. R. Murdoch, J. Microbiol. Methods, 2006, 65, 361–365 CrossRef CAS PubMed
.
- H. J. Chung, C. M. Castro, H. Im, H. Lee and R. Weissleder, Nat. Nanotechnol., 2013, 8, 369–375 CrossRef CAS PubMed
.
- L. G. Reimer, M. L. Wilson and M. P. Weinstein, Clin. Microbiol. Rev., 1997, 10, 444–465 CAS
.
- P. C. Ray, S. A. Khan, A. K. Singh, D. Senapati and Z. Fan, Chem. Soc. Rev., 2012, 41, 3193–3209 RSC
.
- J. Chen, B. Duncan, Z. Wang, L.-S. Wang, V. M. Rotello and S. R. Nugen, Nanoscale, 2015, 7, 16230–16236 RSC
.
- H. Gu, P.-L. Ho, K. W. T. Tsang, L. Wang and B. Xu, J. Am. Chem. Soc., 2003, 125, 15702–15703 CrossRef CAS PubMed
.
- L. Zhang, W.-F. Dong and H.-B. Sun, Nanoscale, 2013, 5, 7664–7684 RSC
.
- M. Sunbul and A. Jaschke, Angew. Chem., Int. Ed., 2013, 52, 13401–13404 CrossRef CAS PubMed
.
- Y.-C. Chuang, S.-C. Chang and W.-K. Wang, PLoS One, 2010, 5, e14133 CAS
.
- J. H. Kang, M. Super, C. W. Yung, R. M. Cooper, K. Domansky, A. R. Graveline, T. Mammoto, J. B. Berthet, H. Tobin, M. J. Cartwright, A. L. Wstters, M. Rottman, A. Waterhouse, A. Mammoto, N. Gamini, M. J. Rodas, A. Kole, A. Jiang, T. M. Valentin, A. Diaz, K. Takahashi and D. E. Ingber, Nat. Med., 2014, 20, 1211–1216 CrossRef CAS PubMed
.
- R. Bellomo, P. Honore, J. Matson, C. Ronco and J. Winchester, Int. J. Artif. Organs, 2005, 28, 996130017414–458 Search PubMed
.
- J.-J. Lee, K. J. Jeong, M. Hashimoto, A. H. Kwon, A. Rwei, S. A. Shankarappa, J. H. Tsui and D. S. Kohane, Nano Lett., 2014, 14, 1–5 CrossRef CAS PubMed
.
- T. F. Didar, M. I. Cartwright, M. Rottman, A. R. Graveline, N. Gamini, A. L. Watters, D. C. Leslie, T. Mammoto, M. J. Rodas, J. H. Kang, A. Waterhouse, B. T. Seiler, P. Lombardo, E. I. Qendro, M. Super and D. E. Ingber, Biomaterials, 2015, 67, 382–392 CrossRef CAS PubMed
.
- T. Burnouf and M. Radosevich, Blood Rev., 2000, 14, 94–110 CrossRef CAS PubMed
.
- M. Wainwright, Curr. Med. Chem., 2002, 9, 127–143 CrossRef CAS PubMed
.
- L. Marciel, L. Teles, B. Moreira, M. Pacheco, L. M. O. Lourenco, M. G. P. M. S. Neves, J. P. C. Tome, M. A. F. Faustino and A. Almeida, Future Med. Chem., 2017, 9, 365–379 CrossRef CAS PubMed
.
- S. Li, S. Cui, D. Yin, Q. Zhu, Y. Ma, Z. Qian and Y. Gu, Nanoscale, 2017, 9, 3912–3924 RSC
.
- S. Verma, G. M. Watt, Z. Mal and T. Hasan, Photochem. Photobiol., 2007, 83, 996–1005 CrossRef CAS PubMed
.
- T. Liu, C. Wang, W. Cui, H. Gong, C. Liang, X. Shi, Z. Li, B. Sun and Z. Liu, Nanoscale, 2014, 6, 11219–11225 RSC
.
- Y.-Q. Li, B. K. Chandran, C. T. Lim and X. Chen, Adv. Sci., 2015, 2, 1500118 CrossRef PubMed
.
- S. Yin, Y.-L. Wu, B. Hu, Y. Wang, P. Cai, C. K. Tan, D. Qi, L. Zheng, W. R. Leow, N. S. Tan, S. Wang and X. Chen, Adv. Mater. Interfaces, 2014, 1, 1300043 CrossRef
.
- Y.-Q. Li, B. Zhu, Y. Li, W. R. Leow, R. Goh, B. Ma, E. Fong, M. Tang and X. Chen, Angew. Chem., Int. Ed., 2014, 53, 5837–5841 CrossRef CAS PubMed
.
- C.-Y. Wen, Y.-Z. Jiang, X.-Y. Li, M. Tang, L.-L. Wu, J. Hu, D.-W. Pang and J.-B. Zeng, ACS Appl. Mater. Interfaces, 2017, 9, 9416–9425 CAS
.
- Z. Zhao, R. Yan, J. Wang, H. Wu, Y. Wang, A. Chen, S. Shao and Y.-Q. Li, J. Mater. Chem. B, 2017, 5, 3572–3579 RSC
.
- S. Guo, Y. Huang, Q. Jiang, Y. Sun, L. Deng, Z. Liang, Q. Du, J. Xing, Y. Zhao, P. C. Wang, A. Dong and X.-J. Liang, ACS Nano, 2010, 4, 5505–5511 CrossRef CAS PubMed
.
- R. Franco, M. I. Panayiotidis and J. A. Cidlowski, J. Biol. Chem., 2007, 282, 30452–30465 CrossRef CAS PubMed
.
- A. Tarkowski and H. Wagner, Mol. Med. Today, 1998, 4, 15–18 CrossRef CAS PubMed
.
- Z. M. N. Quezado, C. Natanson, W. Karzai, R. L. Danner, C. A. Koev, Y. Fitz, D. P. Dolan, S. Richmond, S. M. Banks, L. Wilson and P. Q. Eichacker, J. Appl. Physiol., 1998, 84, 107–115 CrossRef CAS PubMed
.
- L. C. Wu, O. Zaborina, A. Zaborin, E. B. Chang, M. Musch, C. Holbrook, J. Shapiro, J. R. Turner, G. H. Wu, K. Y. C. Lee and J. C. Alverdy, Gastroenterology, 2004, 126, 488–498 CrossRef CAS
.
- N. L. Ben Zakour, C. Venturini, S. A. Beatson and M. J. Walker, J. Clin. Microbiol., 2012, 50, 2224–2228 CrossRef CAS PubMed
.
- I. Irwansyah, Y.-Q. Li, W. Shi, D. Qi, W. R. Leow, M. B. Y. Tang, S. Li and X. Chen, Adv. Mater., 2015, 27, 648–654 CrossRef CAS PubMed
.
- S. Mukherjee, H. Zheng, M. G. Derebe, K. M. Callenberg, C. L. Partch, D. Rollins, D. C. Propheter, J. Rizo, M. Grabe, Q.-X. Jiang and L. V. Hooper, Nature, 2014, 505, 103–107 CrossRef PubMed
.
- M. Gottar, V. Gobert, T. Michel, M. Belvin, G. Duyk, J. A. Hoffmann, D. Ferrandon and J. Royet, Nature, 2002, 416, 640–644 CrossRef CAS PubMed
.
- B. Kherzi and M. Pumera, Nanoscale, 2016, 8, 17415–17421 RSC
.
- J. Jiang, H. Gu, H. Shao, E. Devlin, G. C. Papaefthymiou and J. Y. Ying, Adv. Mater., 2008, 20, 4403–4407 CrossRef CAS
.
- Z. Zhao, R. Yan, X. Yi, J. Li, J. Rao, Z. Guo, Y. Yang, W. Li, Y.-Q. Li and C. Chen, ACS Nano, 2017, 11, 4428–4438 CrossRef CAS PubMed
.
- P. Cai, W. R. Leow, X. Wang, Y.-L. Wu and X. Chen, Adv. Mater., 2017, 29, 1605529 CrossRef PubMed
.
- Y.-C. Chang, C.-Y. Yang, R.-L. Sun, Y.-F. Cheng, W.-C. Kao and P.-C. Yang, Sci. Rep., 2013, 3, 1863 CrossRef PubMed
.
- J. G. Bruno, M. P. Carrillo, T. Phillips and C. J. Andrews, J. Fluoresc., 2010, 20, 1211–1223 CrossRef CAS PubMed
.
Footnote |
† Electronic supplementary information (ESI) available. See DOI: 10.1039/c7nr06373c |
|
This journal is © The Royal Society of Chemistry 2018 |
Click here to see how this site uses Cookies. View our privacy policy here.