DOI:
10.1039/C8RA01734D
(Paper)
RSC Adv., 2018,
8, 12562-12572
Wet-chemically prepared low-dimensional ZnO/Al2O3/Cr2O3 nanoparticles for xanthine sensor development using an electrochemical method†
Received
27th February 2018
, Accepted 19th March 2018
First published on 3rd April 2018
Abstract
A reliable xanthine (XNT) chemical sensor was fabricated using a facile wet-chemical method (by co-precipitation) to prepare ZnO/Al2O3/Cr2O3 nanoparticles (NPs) in an alkaline medium at low temperature. Powder X-ray diffraction (XRD), X-ray photoelectron spectroscopy (XPS), field emission scanning electron microscopy (FESEM), energy-dispersive X-ray spectroscopy (EDS), Fourier transform infrared spectroscopy (FTIR) and ultraviolet-visible spectroscopy (UV-vis) were implemented for detailed characterization of the NPs. To fabricate the working electrode as a XNT chemical sensor probe, a glassy carbon electrode (GCE) with a 0.0316 cm2 surface area was coated with an ethanolic slurry of the prepared ZnO/Al2O3/Cr2O3 NPs to make a thin layer and used to analyse XNT in a phosphate buffer system. To evaluate the analytical performances of the XNT chemical sensor, the calibration curve of XNT was plotted as the relationship of current versus the concentration of XNT. The plotted calibration curve was found to be linear over the LDR (linear dynamic range) of 0.05 nM to 5.0 μM. The assembled XNT electrochemical sensor exhibited the highest sensitivity (70.8861 μA μM−1 cm−2), the lowest detection limit (1.34 ± 0.07 pM), good reproducibility performance with high accuracy and long-term stability with standard results under ambient conditions. This is a simple route to selectively detect XNT with wet-chemically prepared co-doped ZnO/Al2O3/Cr2O3 nanomaterials using a reliable electrochemical method at a large scale for safety within healthcare fields.
Introduction
Chemically, XNT is known as 3,7-dihydropurine-2,6-dione, and is an intermediate metabolized product of purine in the human body. Therefore, it is found in the human body at concentrations ranging from 0.5–2.5 μM in blood serum and 40–160 μM in urine.1,2 In the metabolism pathway of purine, XNT is oxidized to uric acid by an enzymatic reaction in the presence of XNT oxides (XOs). For excess XNT levels above the normal range, a number of pathological indicators such as hyperuricemia, tumour hyperthermia, xanthinuria, perinatal asphyxia, pre-eclampsia, leukemia, cerebral ischemia, and gout are recognized in the human body.3–5 The derivatives of XNT (caffeine and theobromine) are found as mild stimulants in drinks such as coffee and tea. To produce high quality foods, the meat of fresh fish is used in food industries. Due to the degradation of ATP in dead fish, XNT is produced naturally.6,7 To determine XNT in human serum and food, a reliable and efficient method is needed for safety within the health and food sectors.8,9 HPLC (high-performance liquid chromatography),10,11 voltammetric and amperometric,12,13 and electrophoresis14 methods are extensively implemented to detect XNT quantitatively in an aqueous medium. In recent years, a number of research activities to detect XNT using electrochemical methods based on various metal oxides and hybrid materials have been reported.15–18 The aim of this research approach is to develop an electrochemical sensor using an electrochemical method based on ZnO/Al2O3/Cr2O3 NPs.
ZnO is a semi-conductive oxide with a 3.3 eV band gap energy, a 60 meV exciton binding energy, a high range of resistivity (10−3–105 Ω cm) and transparency in the visible wave region.19–23 Therefore, ZnO shows good optical, electrical and piezo-electrical properties24,25 and these outstanding properties make ZnO a strong candidate in the field of sensor applications. As a sensor material, ZnO has been applied to detect many toxic chemicals such as acetone,26 ethanol,27 4-aminophenol,28 and bisphenol A29 in an aqueous medium. With attractive photo-electronic characteristics, Al2O3 has also been applied as a chemical and biological sensor.30–32 Another active transition metal oxide Cr2O3 has also found use in catalysis, wear resistant materials, colorants, and optical and electronic devices.33–36 Besides this, the binary combination of ZnO–Al2O3 has found use as a sensor to detect catechol, hydroquinone37 and methyl violet dye.38 Moreover, ZnO–Cr2O3 has been reported as an efficient sensor material to detect ethanol39 and LPG.40
To execute this study, an electrochemical sensor was fabricated by deposition of ZnO/Al2O3/Cr2O3 NPs onto a GCE as a uniform thin layer with the help of a conducting Nafion binder. Thus, the resulting working electrode probe (ZnO/Al2O3/Cr2O3 NPs/binder/GCE) for XNT was implemented to detect XNT in an optimized phosphate buffer system using an electrochemical method under ambient conditions. This research approach might be a reliable and easy method to develop future sensitive chemical and biological sensors based on various doped or undoped ternary metal oxides.
Materials and methodology
Materials and methods
To prepare the ternary metal oxides, analytical grade chemicals such as Al2(SO4)3·18H2O, Zn(NO3)2·6H2O, and Cr(NO3)3·9H2O were purchased and used as received. As part of this investigation, various toxins namely 2,4-DNP (2,4-dinitrophenol), 2-APy (2-acetyl pyridine), BC (benzyl chloride), DEM (diethyl malonate), EG (ethylene glycol), M-THyd (M-tolyl hydrazine hydrochloride), M-Xy (M-xylol), Zdehy (zimtaldehyde), and XNT were obtained from Sigma-Aldrich (USA). A Thermo-Scientific K-α1 1066 X-ray photoelectron spectroscopy (XPS) instrument with an excitation radiation source (A1 Kα1, beam spot size = 300.0 μm, pass energy = 200.0 eV and pressure ∼10−8 torr) was used to study the binding energy and oxidation state of the elements existing in the synthesized ZnO/Al2O3/Cr2O3 NPs. To characterize their ability to absorb visible light, the prepared NPs were examined using a Thermo-Scientific 300 UV-vis spectrometer. A Thermo-Scientific NICOLET iS50 (Madison, WI, USA) FTIR spectrometer was used to identify the functional groups in the ZnO/Al2O3/Cr2O3 NPs. The elemental composition and structural morphology were inspected using FESEM analysis equipped with XEDS. The phase crystallinity and particle size of the prepared NPs were evaluated using powder X-ray diffraction (XRD) with an ARL™ X’TRA powder diffractometer. The electrochemical investigation was carried out using a Keithley electrometer (6517A, USA).
Synthesis of the ZnO/Al2O3/Cr2O3 NPs
To synthesise nanomaterials of transition metal oxides, the wet-chemical (co-precipitation process) method is an efficient, conventional method in an alkaline medium with pH 10.5. Al2(SO4)3·18H2O, Zn(NO3)2·6H2O, and Cr(NO3)3·9H2O are used to prepare a 0.1 M solution of each salt individually. 100.0 mL round bottom flasks with deionized water and 50.0 mL of each solution in a 250.0 mL conical flask were taken for the synthesis of the doped nanomaterials. Then, the conical flask was put on a hot plate at a temperature of 80 °C with continuous magnetic stirring. Consequently, a prepared 0.1 M NH4OH solution was added dropwise into the mixture in the conical flask to gradually increase the pH of the solution up to 10.5. Due to the addition of ammonium hydroxide, the pH of the mixture was enriched slowly and the metal ions started to co-precipitate in the form of metal hydroxides and initiated the formation of the nuclei for crystal formation. At pH 10.5, all the metal ions had co-precipitated out quantitatively in the form of a Zn(OH)2·Al(OH)3·Cr(OH)3·nH2O crystal and with the temperature conditions and magnetic stirring, the process took several hours. A similar process for the formation of nanocrystals in an aqueous medium has been reported earlier.41–43 The reactions in the conical flask are proposed below: |
NH4OH(l) ⇆ NH4(aq)+ + OH(aq)−
| (i) |
|
Zn(NO3)2(s) → Zn(aq)2+ + 2NO3(aq)−
| (ii) |
|
Al2(SO4)3(s) → 2Al(aq)3+ + 3SO4(aq)−
| (iii) |
|
Cr(NO3)3(s) → Cr(aq)3+ + 3NO3(aq)−
| (iv) |
|
Zn(aq)2+ + Al(aq)3+ + Cr(aq)3+ + 8OH(aq)− ⇆ Zn(OH)2·Al(OH)3·Cr(OH)3(s)↓
| (v) |
Then, the precipitate was separated from the aqueous medium and washed with acetone and deionized water properly. After that, the resultant crystals were kept to dry inside an oven at a temperature of 110 °C overnight. Finally, the dehydrated Zn(OH)2·Al(OH)3·Cr(OH)3(s) sample was subjected to calcination at a temperature of 500 °C for 6 hours in a high temperature muffle furnace in the presence of atmospheric oxygen. The metal hydroxides were converted to the metal oxides: ZnO·Al2O3·Cr2O3. The proposed reaction inside the muffle furnace is given as follows:
|
Zn(OH)2·2Al(OH)3·2Cr(OH)3(s) → ZnO·Al2O3·Cr2O3 + 7H2O(↑)
| (vi) |
Fabrication of the GCE
The working electrode of the desired sensor was fabricated using a GCE. To do this, the slurry of ZnO/Al2O3/Cr2O3 NPs was prepared in ethanol and deposited onto the glassy carbon electrode in a very uniform thin layer. Then, the modified GCE was kept under ambient conditions for an hour to dry. To improve the binding strength between the NPs and the GCE, a drop of Nafion (5% Nafion suspension in ethanol) was added onto the modified GCE, which was put inside an oven at a temperature of 35 °C for a time adequate to dry the working electrode ZnO/Al2O3/Cr2O3 NPs/binder/GCE entirely. The desired sensor was assembled using an electrometer (a Keithley electrometer), where ZnO/Al2O3/Cr2O3 NPs/binder/GCE acted as working electrode and Pt-wire as a counter electrode. Depending on the concentration, a number of XNT solutions ranging from 0.05 mM to 0.05 nM were prepared and used as target analytes to evaluate the analytical performance of the anticipated XNT sensor. A curve was plotted (known as a calibration curve) as the relationship of current vs. the concentration of XNT. From the maximum linearity (r2) of the calibration curve, the linear dynamic range (LDR) of the projected XNT sensor was estimated. Using the slope of the calibration curve, the sensitivity and detection limit (DL) of the XNT sensor were calculated. During the investigation, the buffer solution in the measuring beaker was kept at a constant 10.0 mL throughout the analysis. The Keithley electrometer was used as a simple two electrode system.
Results and discussion
Optical and structural properties of the ZnO/Al2O3/Cr2O3 NPs
Normally, metal oxides show a characteristic absorption peak in the region 400–800 cm−1 in FTIR investigations. To study the FTIR spectrum of the synthesized ZnO/Al2O3/Cr2O3 NPs, FTIR analysis was implemented in the region 400–4000 cm−1. As observed in Fig. 1(a), two major absorption peaks at 400 and 1100 cm−1 were exhibited, which are associated with the stretching vibrations of the Zn–O and C–O functional groups, respectively.44–46 The photocatalytic activity of the ZnO/Al2O3/Cr2O3 NPs was investigated by the implementation of UV-vis analysis in the range of 280–800 nm as shown in Fig. 1(b). As shown in Fig. 1(b), a characteristic absorption band was observed at 289 nm.47–49 The band gap energy of the ZnO/Al2O3/Cr2O3 NPs was estimated using the following equation and is found to be 4.29 eV:where Ebg is the band gap energy and λ is the maximum absorption wavelength.
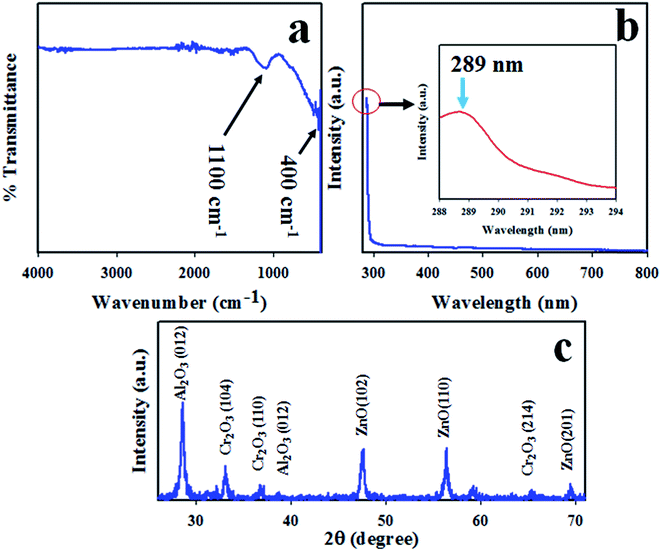 |
| Fig. 1 The optical properties and crystallinity of the synthesized ZnO/Al2O3/Cr2O3 NPs. (a) FTIR analysis, (b) UV-vis spectrum, and (c) phase crystallinity. | |
The crystallinity of ZnO/Al2O3/Cr2O3 NPs was studied by execution of XRD analysis with a radiation source of Cu Kα at 1.5406 ångströms. The resulting XRD pattern recorded over the 2θ range of 26–74° is shown in Fig. 1(c). As shown in Fig. 1(c), the observed peaks of ZnO are shown as (101), (102) and (201) planes, which are indicated by JCPDS no. 00-036-1451 and previous reports of ZnO.50–52 The diffracted peaks of Cr2O3 such as (104), (110), and (214) are also shown in Fig. 1(c) and have great similarity to JCPDS no. 01-089-6744 and those from previous authors.53,54 Besides this, the reflected XRD peaks (012) and (110) of Al2O3 match those of JCPDS no. 077-1716 and reports for Al2O3.55,56 Using the Scherrer equation, the crystal size of the NPs has been estimated from the Al2O3 peak (012) and is found to be 22.54 nm.
|
D = 0.9λ/(β cos θ)
| (viii) |
where
λ is the wavelength of the X-ray radiation (1.5418 Å) and
β is the full width at half maximum (FWHM) of the peak at diffracted angle
θ.
XPS analysis of the ZnO/Al2O3/Cr2O3 NPs
To measure the surface composition and oxidation state of the synthesized ZnO/Al2O3/Cr2O3 NPs, XPS analysis was performed as shown in Fig. 2. As demonstrated in Fig. 2, the XPS spectra show the peaks associated with Zn, O, Al and Cr only. The other peaks are due to the presence of the impurities. The high resolution XPS spectrum of Zn 2p presented in Fig. 2(a) shows two split spin orbitals centered at 1021.4 eV for Zn 2p3/2 and 1044.4 eV for Zn 2p1/2. The spin energy separation is 23.0 eV, which is the characteristic value for the Zn2+ oxidation state.57–59 The O 1s spectrum shows a highly intense peak at 532.0 eV as shown in Fig. 2(b), which can be ascribed to lattice oxygen with an oxidation state of O−2 in the ZnO/Al2O3/Cr2O3 NPs.60,61 As shown in Fig. 2(d), the core level Cr 2p XPS spectrum shows two split spin orbitals at 576.0 eV for Cr 2p3/2 and 586.4 eV for Cr 2p1/2 corresponding to the Cr3+ oxidation state.62,63 The XPS peak positioned at 74.5 eV is related to the Al3+ state as represented in Fig. 2(c).64,65
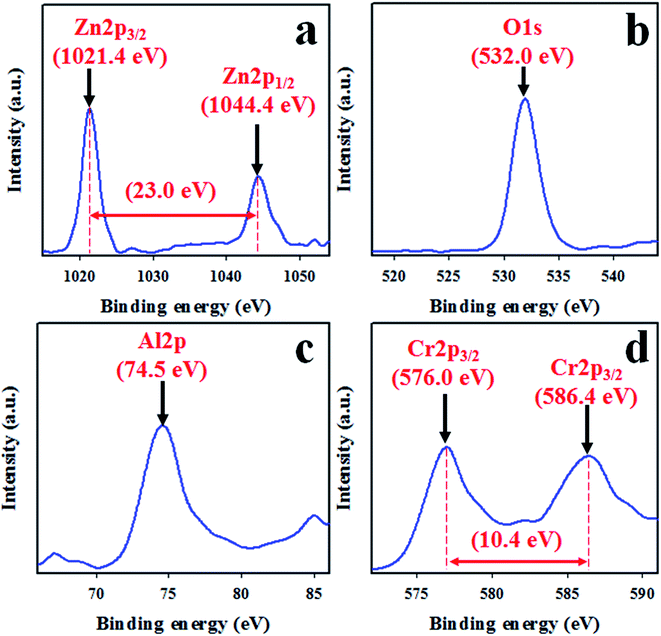 |
| Fig. 2 High resolution XPS spectra of the ZnO/Al2O3/Cr2O3 NPs. (a) The core level XPS spectrum of Zn 2p, (b) O 1s orbitals, (c) Al 2p orbitals, and (d) the 3/2 spin orbitals of Cr 2p. | |
Morphological and elemental analysis of the ZnO/Al2O3/Cr2O3 NPs
FESEM equipped with EDS was implemented to identify the structural morphology of the synthesized ZnO/Al2O3/Cr2O3 NPs. As demonstrated in Fig. 3(a and b), both the low and high magnification FESEM images confirmed that the prepared ZnO/Al2O3/Cr2O3 oxides are aggregated nanoparticles in shape. An identical result was also observed from EDS analysis as shown in Fig. 3(c and d). The elemental composition of the mixed oxides was found to be 60.13% O, 14.42% Al, 13.51% Cr, and 11.95% Zn and is presented in Fig. 3(d). Therefore, EDS analysis established that the synthesized NPs contained O, Al, Zn, and Cr only and peaks associated with the presence of impurities were not observed.
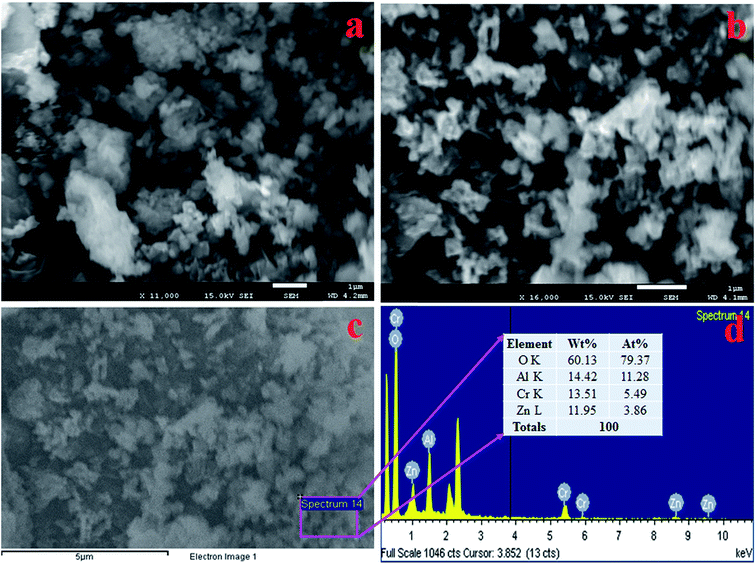 |
| Fig. 3 Structural and compositional analysis of the prepared ZnO/Al2O3/Cr2O3 NPs. (a and b) Low and high magnification FESEM images and (c and d) elemental analysis with EDS. | |
Analytical performance of the XNT sensor
The synthesized ZnO/Al2O3/Cr2O3 NPs were used to fabricate the desired electrochemical sensor. To assemble the working electrode of this electrochemical sensor, the ZnO/Al2O3/Cr2O3 NPs were deposited onto a GCE as a uniform layer of thin film with a Nafion conducting binder. At the very beginning of this study, the assembled electrochemical (applying the I–V method) sensor was tested to find out the most desirable buffer system. To execute this testing, a number of buffer systems with various pH values ranging from 5.7–8.0 were analyzed as shown in Fig. 4(a). As can be observed in Fig. 4(a), the anticipated electrochemical sensor shows the highest I–V response at pH 7.0. Therefore, it can be concluded that the proposed sensor based on ZnO/Al2O3/Cr2O3 NPs/binder/GCE has maximum electrochemical activity at pH 7.0. To examine the selectivity of the assembled electrochemical sensor, environmental toxins were analyzed with micro-level concentrations at pH 7.0. The corresponding I–V data for 2,4-DNP, 2-APy, BC, DEM, EG, M-THyd, M-Xy, Zdehy, and XNT are presented in Fig. 4(b), for which the applied potential range of 0 to +1.5 V was used at pH 7.0. Obviously, XNT shows the best I–V response among these toxins. To estimate the analytical performance of the XNT electrochemical sensor, it was diluted in deionized water to make up a number of XNT solutions based on concentrations ranging from 0.05 mM to 0.05 nM which were applied as analytes to measure I–V responses (Fig. 4(c)). As shown in Fig. 4(c), the I–V responses are distinctive from lower to higher concentration and this performance is observed at applied potentials higher than +1.0 V and at pH 7.0. To plot the calibration curve, the current data at the applied potential +1.5 V were collected from Fig. 4(c) and plotted as concentration of XNT versus current as presented in Fig. 4(d). The calibration curve was found to be linear over the concentration range of 0.05 nM to 5.0 μM which was identified as the linear dynamic range (LDR), and basically, this is a wide range of concentrations. The current data for the LDR has been fitted with the regression co-efficient value r2 = 0.9907 which provides evidence of the linearity of the calibration curve. As revealed in Fig. 4(d), the current data is consistently scattered lengthways in the plot which provides an indication of the reliability of the analytical method. The sensitivity of the anticipated XNT sensor based on ZnO/Al2O3/Cr2O3 NPs/binder/GCE was assessed from the slope of the calibration curve by considering the active surface area of the GCE (0.0316 cm2). The value was estimated to be 70.8861 μA μM−1 cm−2. Likewise, the detection limit (DL) was also calculated from the slope of the calibration curve by considering the signal to noise ratio at 3. This obtained the result of 1.34 ± 0.07 pM, which is a significantly lower value than the reported value.
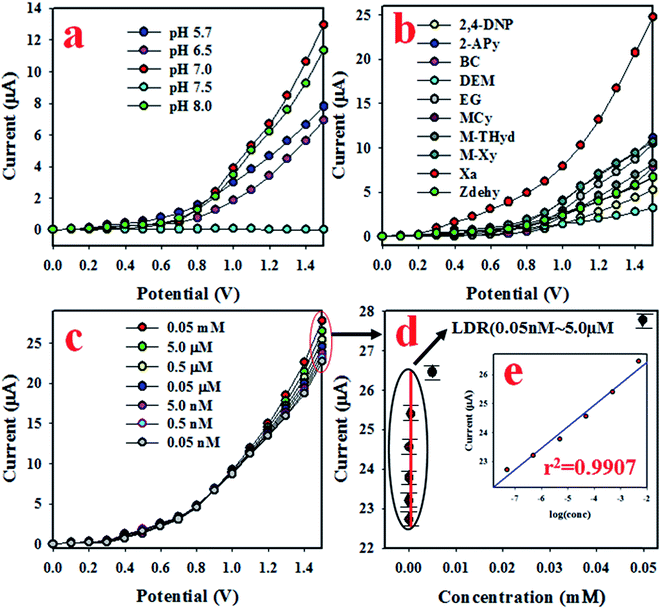 |
| Fig. 4 The estimation of sensor analytical behavior and performance. (a) pH optimization, (b) estimation of the selectivity, (c) concentration variation from lower to higher range, and (d) the calibration curve [inset log(conc.) vs. current]. | |
To quantify the capability to reproduce identical electrochemical responses in the optimized buffer system, the proposed XNT electrochemical sensor was used in a reproducibility test. This test was effected with a 5.0 nM concentration of XNT at applied potentials from 0 to +1.5 V. As shown in Fig. 5(a), the seven runs are virtually identical which provides information about the reliability of the XNT sensor. To assess the accuracy of the reproducibility study, the current data were analyzed to estimate the relative standard deviation (RSD) and an excellent RSD was obtained (1.08%). A similar XNT sensor reproducibility test was executed for an elongated period, as shown in Fig. 6. Fig. 6(a–d) shows the equivalent information to Fig. 5(a). Therefore, it can be summarized that the participating XNT sensor is able to significantly detect XNT in real environmental samples. The response time of the sensor is an important criterion to quantify the efficiency. Therefore, the response time of the XNT sensor based on ZnO/Al2O3/Cr2O3 NPs/binder/GCE was calculated using a 5.0 nM concentration of XNT. As revealed in Fig. 5(b), the resulting response time of the XNT sensor is around 22 s, a result which might be considered as having high potential. To confirm the selectivity and interference effect of the XNT sensor, the electrochemical responses were investigated as shown in Fig. 5(c and d). It can be summarized that the anticipated XNT sensor is highly selective toward XNT without any interference effects in the presence of other toxins.
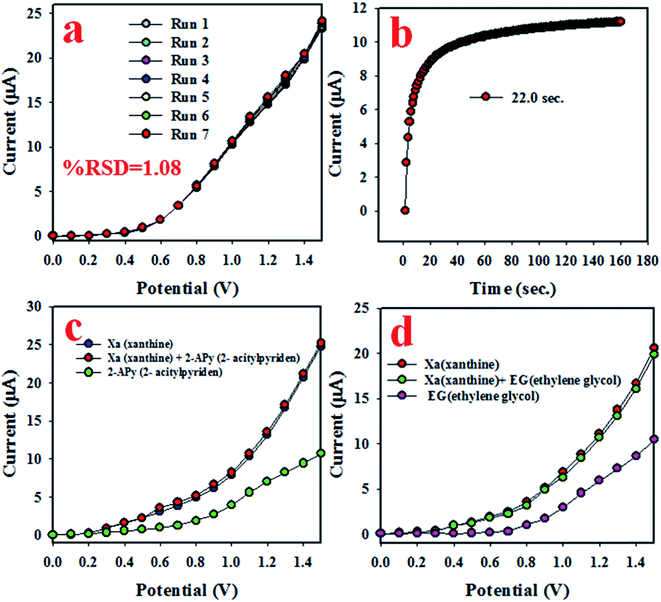 |
| Fig. 5 Reliability investigations of the XNT sensor based on ZnO/Al2O3/Cr2O3 NPs/binder/GCE: (a) testing reproducible performance, (b) response time, and (c and d) interference tests. | |
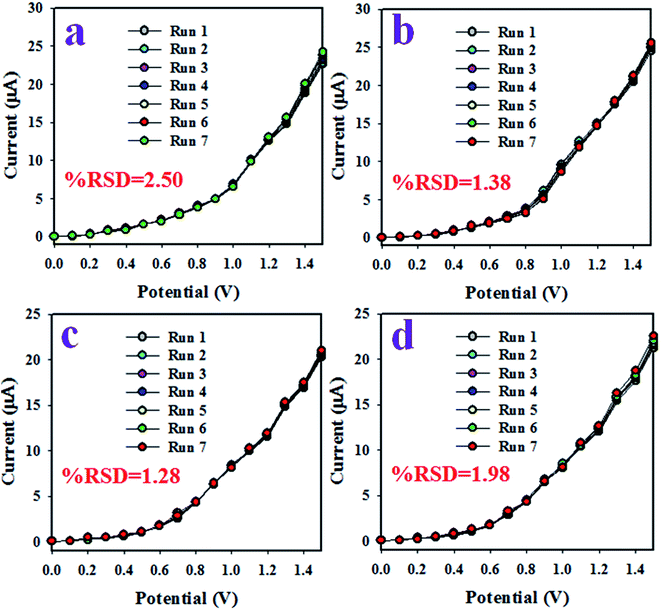 |
| Fig. 6 (a–d) The reproducibility performance for various elongated times (4 days). | |
As shown in Fig. 4(c), the I–V response varies with the corresponding concentration of XNT. The proposed XNT sensor with ZnO/Al2O3/Cr2O3 NPs/binder/GCE was implemented successfully to detect XNT in an optimized phosphate buffer system. A control experiment was performed (Fig. S1†) and is included in the ESI.† Here, a control experiment was performed based on different compositions of fabricated GCEs such as Al2O3, ZnO, Cr2O3, ZnO/Al2O3, Al2O3/Cr2O3, ZnO/Cr2O3, and ZnO/Al2O3/Cr2O3 nanoparticles. Based on these observations, the highest sensor response was exhibited with ZnO/Al2O3/Cr2O3 NPs/binder/GCE compared to those of other fabricated electrodes such as Al2O3, ZnO, Cr2O3, ZnO/Al2O3, Al2O3/Cr2O3, and ZnO/Cr2O3.
During the initial stages of the sensing study of the XNT sensor, the surface coverage on the anticipated working electrode was smaller due to absorption of very few XNT molecules and the corresponding XNT oxidation reaction proceeded. With the increase in XNT concentration in the detecting system, both the surface coverage and rate of reaction increased gradually and approached steady state equilibrium conditions. With further enrichment of the XNT concentration, equilibrium current density was observed in the detecting system. This equilibrium current density is presented in Fig. 4(d) and the current data points are consistently distributed along the linear plot. Therefore, this investigation provides evidence of the reliability of the method. As presented in Fig. 5(b), the response time of the XNT sensor is 22 s and this amount of time is necessary for the XNT sensor to produce a steady state equilibrium I–V response. Therefore, the XNT sensor based on ZnO/Al2O3/Cr2O3 NPs/binder/GCE exhibited appreciable analytical performance characteristics such as good sensitivity (70.8861 μA μM−1 cm−2), a broad linear dynamic range (0.05 nM to 5.0 μM), a very low detection limit (1.34 ± 0.07 pM), a short response time (22 s), reproducibility with a constant I–V response, and high selectivity without interference effects. Also studied was the sensor performance based on various time spans such as intra-day and inter-day validations, which are presented in Fig. 6(a–d). A comparison of the XNT sensor performances was carried out and is included in Table 1. As indicated in Table 1, the XNT sensor based on ZnO/Al2O3/Cr2O3 NPs/binder/GCE shows better performance when comparing the sensitivity, detection limit and linear dynamic range.66
Table 1 A comparison of the sensor performance with that of similar work based on different electrode fabrications using an electrochemical approacha
Modified GCE |
DL |
LDR |
Sensitivity |
Ref. |
DL (detection limit) and LDR (linear dynamic range). |
Co/ZnO NPs/GCE |
178.8 pM |
5.0 nM to 0.05 mM |
53.1 μA μM−1 cm−2 |
66 |
ZnO/Al2O3/Cr2O3 NPs/GCE |
1.34 pM |
0.05 nM to 5.0 μM |
70.9 μA μM−1 cm−2 |
This work |
Possible sensing mechanism of XNT
During the electrochemical analysis of XNT using the I–V method, the XNT molecules are adsorbed onto the surface of the working electrode based on ZnO/Al2O3/Cr2O3 NPs/binder/GCE, where the XNT molecules are oxidized to uric acid (Scheme 1). As illustrated in Scheme 1, due to oxidation of the XNT molecule using the I–V method, an electron is produced which is responsible for the higher conductivity of the sensing phosphate buffer medium. A similar pattern of electrochemical oxidation of XNT has been reported previously.15,16,67
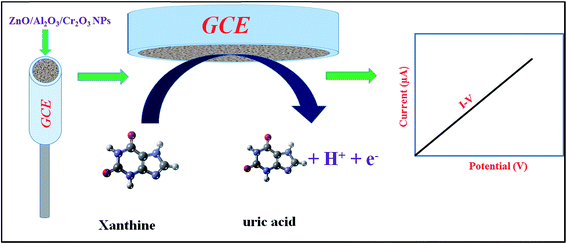 |
| Scheme 1 A prototype representation of the electrochemical oxidation of XNT in an aqueous medium using an electrochemical method. | |
Validity of the XNT sensor with real environmental samples
To test the validity of the proposed XNT electrochemical sensor based on ZnO/Al2O3/Cr2O3 NPs/binder/GCE, environmental samples such as extracts from a PC-water bottle, a PC-baby bottle, a PVC food packaging bag and industrial effluent have been analyzed using a recovery method. The results of the analyses are presented in Table 2. In Table 2, the obtained results are quite reasonable and acceptable.
Table 2 Analyses of real environmental samples with the ZnO/Al2O3/Cr2O3 NPs/binder/GCE sensor
Sample |
Added XNT concentration (nM) |
Measured melamine conc.a by ZnO/Al2O3/Cr2O3 NPs/GCE (nM) |
Average recoveryb (%) |
RSDc (%) (n = 3) |
R1 |
R2 |
R3 |
Mean of three repeated determinations (signal to noise ratio of 3) with ZnO/Al2O3/Cr2O3 NPs/GCE. The concentration of XNT determined/the concentration taken (unit: nM). Relative standard deviation value indicates precision among three repeated measurements (R1, R2, R3). |
Industrial effluent |
5.000 |
4.97128 |
4.96702 |
4.94034 |
99.18 |
0.34 |
PC-baby bottle |
5.000 |
4.88659 |
4.92789 |
4.87152 |
97.91 |
0.60 |
PVC-water bottle |
5.000 |
4.89598 |
4.93011 |
4.92118 |
98.32 |
0.36 |
PVC-food packaging bag |
5.000 |
4.95423 |
4.95025 |
4.94347 |
98.99 |
0.11 |
Conclusion
The electrochemical sensor was fabricated using wet-chemically prepared ZnO/Al2O3/Cr2O3 nanoparticles and implemented to selectively detect XNT in a phosphate buffer phase. The fabricated sensor shows good sensitivity, a wider linear dynamic range, a much lower detection limit, a quick response time, and excellent reproducibility performance. Besides this, it was found to be stable long-term in a phosphate buffer medium without any interference effects and able to successfully detect XNT in real environmental samples. This has introduced a new route for efficient detection of XNT using an electrochemical approach with ternary ZnO/Al2O3/Cr2O3 nanoparticles for safety within healthcare and biomedical fields.
Conflicts of interest
There are no conflicts to declare.
Acknowledgements
The Center of Excellence for Advanced Materials Research (CEAMR), Chemistry Department, King Abdulaziz University, Jeddah, Saudi Arabia is highly acknowledged for financial support and research facilities.
References
- R. Boulieu, C. Bory, P. Baltassat and C. Gonnet, Hypoxanthine and xanthine levels determined by high-performance liquid chromatography in plasma, erythrocyte, and urine samples from healthy subjects: the problem of hypoxanthine level evolution as a function of time, Anal. Biochem., 1983, 129, 398–404 CrossRef CAS PubMed
. - R. Kant, R. Tabassum and B. D. Gupta, Xanthine oxidase functionalized Ta2O5 nanostructures as a novel scaffold for highly sensitive SPR based fiber optic xanthine sensor, Biosens. Bioelectron., 2018, 99, 637–645 CrossRef CAS PubMed
. - P. Kalimuthu, S. Leimkuhler and P. V. Bernhardt, Low-potential amperometric enzyme biosensor for xanthine and hypoxanthine, Anal. Chem., 2012, 84, 10359–10365 CrossRef CAS PubMed
. - W. Pu, H. Zhao, L. Wu and X. Zhao, A colorimetric method for the determination of xanthine based on the aggregation of gold nanoparticles, Microchim. Acta, 2015, 182, 395–400 CrossRef CAS
. - S. Yun and L. Jinzhou, Electrochemical sensor using glassy carbon electrode modified with acylpyrazolone-multiwalled carbon nanotube composite film for determination of xanthine, J. Solid State Electrochem., 2012, 16, 689–695 CrossRef
. - J. H. T. Luong, K. B. Male and A. L. Nguyen, Application of polarography for monitoring the fish post mortem metabolite transformation, Enzyme Microb. Technol., 1989, 11, 277–282 CrossRef CAS
. - D. Shan, Y. Wang, H. Xue and S. Cosnier, Sensitive and selective xanthine amperometric sensors based on calcium carbonate nanoparticles, Sens. Actuators, B, 2009, 136, 510–515 CrossRef CAS
. - D. Shan, Y. Wang, M. Zhu, H. Xue, S. Cosnier and C. Wang, Development of a high analytical performance-xanthine biosensor based on layered double hydroxides modified-electrode and investigation of the inhibitory effect by allopurinol, Biosens. Bioelectron., 2009, 24, 1171–1176 CrossRef CAS PubMed
. - D. Shan, Y. Wang, H. Xue and S. Cosnier, Sensitive and selective xanthine amperometric sensors based on calcium carbonate nanoparticles, Sens. Actuators, B, 2009, 136, 510–515 CrossRef CAS
. - M. Czauderna and J. Kowalczyk, Quantification of allantoin, uric acid, xanthine and hypoxanthine in ovine urine by high-performance liquid chromatography and photodiode array detection, J. Chromatogr. B: Biomed. Sci. Appl., 2000, 744, 129–138 CrossRef CAS
. - N. Cooper, R. Khosravan, C. Erdmann, J. Fiene and J. W. Lee, Quantification of uric acid, xanthine and hypoxanthine in human serum by HPLC for pharmacodynamics studies, J. Chromatogr. B: Anal. Technol. Biomed. Life Sci., 2006, 837, 1–10 CrossRef CAS PubMed
. - U. A. Kirgoz, S. Timur, J. Wang and A. Telefoncu, Xanthine oxidase modified glassy carbon paste electrode, Electrochem. Commun., 2004, 6, 913–916 CrossRef
. - J. Pei and X. Y. Li, Xanthine and hypoxanthine sensors based on xanthine oxidase immobilized on a CuPtCl6 chemically modified electrode and liquid chromatography electrochemical detection, Anal. Chim. Acta, 2000, 414, 205–213 CrossRef CAS
. - T. Richter, L. L. Shultz-Lockyear, R. D. Oleschuk, U. Bilitewski and D. J. Harrison, Bi-enzymatic and capillary electrophoretic analysis of non-fluorescent compounds in microfluidic devices: determination of xanthine, Sens. Actuators, B, 2002, 81, 369–376 CrossRef CAS
. - R. Devi, S. Yadav and C. S. Pundir, Electrochemical detection of xanthine in fish meat by xanthine oxidase immobilized on carboxylated multiwalled carbon nanotubes/polyaniline composite film, Biochem. Eng. J., 2011, 58–59, 148–153 CrossRef CAS
. - S. Z. Baş, H. Gülce and S. Yıldız, Amperometric xanthine biosensors based on electrodeposition of platinum on polyvinylferrocenium coated Pt electrode, J. Mol. Catal. B: Enzym., 2011, 72, 282–288 CrossRef
. - R. Devi, S. Yadav and C. S. Pundir, Au-colloids–polypyrrole nanocomposite film based xanthine biosensor, Colloids Surf., A, 2012, 394, 38–45 CrossRef CAS
. - R. Ojani, A. Alinezhad and Z. Abedi, A highly sensitive electrochemical sensor for simultaneous detection of uric acid, xanthine and hypoxanthine based on poly(L-methionine) modified glassy carbon electrode, Sens. Actuators, B, 2013, 188, 621–630 CrossRef CAS
. - R. Ayouchi, D. Leinen, F. Martın, M. Gabas, E. Dalchiele and J. R. Ramos-Barradoa, Preparation and characterization of transparent ZnO thin films obtained by spray pyrolysis, Thin Solid Films, 2003, 426, 68–77 CrossRef CAS
. - R. Romero, M. C. López, D. Leinen, F. Martín and J. R. Ramos-Barrado, Electrical properties of the n-ZnO/c-Si heterojunction prepared by chemical spray pyrolysis, Mater. Sci. Eng., B, 2004, 110, 87–93 CrossRef
. - H. Zhang, D. Yang, S. Li, X. Ma, Y. Ji, J. Xu and D. Que, Controllable growth of ZnO nanostructures by citric acid assisted hydrothermal process, Mater. Lett., 2005, 59, 1696–1700 CrossRef CAS
. - G. Fang, D. Li and B. L. Yao, Fabrication and characterization of transparent conductive ZnO: Al thin films prepared by direct current magnetron sputtering with highly conductive ZnO(ZnAl2O4) ceramic target, J. Cryst. Growth, 2003, 247, 393–400 CrossRef CAS
. - H. K. Kim, K. S. Lee and H. A. Kang, Characteristics of indium zinc oxide top cathode layers grown by box cathode sputtering for top-emitting organic light-emitting diodes, J. Electrochem. Soc., 2006, 153, H29–H33 CrossRef CAS
. - A. Bougrine, A. El Hichou, M. Addou, J. Ebothé, A. Kachouane and M. Troyon, Structural, optical and cathodoluminescence characteristics of undoped and tin-doped ZnO thin films prepared by spray pyrolysis, Mater. Chem. Phys., 2003, 80, 438–445 CrossRef CAS
. - A. El Hichou, M. Addou, A. Bougrine, R. Dounia, J. Ebothé, M. Troyon and M. Amrani, Cathodoluminescence properties of undoped and Al-doped ZnO thin films deposited on glass substrate by spray pyrolysis, Mater. Chem. Phys., 2004, 83, 43–47 CrossRef CAS
. - M. M. Rahman, M. M. Alam, A. M. Asiri and M. A. Islam, Fabrication of selective chemical sensor with ternary ZnO/SnO2/Yb2O3 nanoparticles, Talanta, 2017, 170, 215–223 CrossRef CAS PubMed
. - M. M. Rahman, M. M. Alam, A. M. Asiri and M. A. Islam, Ethanol sensor development based on ternary-doped metal oxides (CdO/ZnO/Yb2O3) nanosheets for environmental safety, RSC Adv., 2017, 7, 22627–22639 RSC
. - M. M. Rahman, M. M. Alam, A. M. Asiri and M. R. Awual, Fabrication of 4-aminophenol sensor based on hydrothermally prepared ZnO/Yb2O3 nanosheets, New J. Chem., 2017, 41, 9159–9169 RSC
. - M. A. Subhan, P. C. Saha, M. M. Alam, A. M. Asiri, M. Al-Mamund and M. M. Rahman, Development of bis-phenol A sensor based on Fe2MoO4·Fe3O4·ZnO nanoparticles for sustainable environment, J. Environ. Chem. Eng., 2018, 6, 1396–1403 CrossRef CAS
. - Z. Jiang, J. Wang, L. Meng, Y. Huang and L. Liu, A highly efficient chemical sensor material for ethanol: Al2O3/Graphene nanocomposites fabricated from graphene oxide, Chem. Commun., 2011, 47, 6350–6352 RSC
. - T. H. Kwon, S. H. Park, J. Y. Ryu and H. H. Choi, Zinc oxide thin film doped with Al2O3, TiO2 and V2O5 as sensitive sensor for trimethylamine gas, Sens. Actuators, B, 1998, 46, 75–79 CrossRef CAS
. - L. M. Hagelsieb, P. E. Lobert, R. Pampin, D. Bourgeois, J. Remacle and D. Flandre, Sensitive DNA electrical detection based on interdigitated Al/Al2O3 microelectrodes, Sens. Actuators, B, 2004, 98, 269–274 CrossRef
. - R. Gupta, E. Mitchell, J. Candler, P. Kahol, K. Ghosh and L. Dong, Facile synthesis and characterization of nanostructured chromium oxide, Powder Technol., 2014, 254, 78–81 CrossRef CAS
. - H. Ma, Y. Xu, Z. Rong, X. Cheng, S. Gao, X. Zhang, H. Zhao and L. Huo, Highly toluenesensing performance
based on monodispersed Cr2O3 porous microspheres, Sens. Actuators, B, 2012, 174, 325–331 CrossRef CAS
. - S. Inturi, M. Suidan and P. Smirniotis, Influence of synthesis method on leaching of the Cr-TiO2 catalyst for visible light liquid phase photo-catalysis and their stability, Appl. Catal., B, 2015, 180, 351–361 CrossRef
. - P. Gibot, F. Schnell and D. Spitzer, Ca3(PO4)2 biomaterial: a nontoxic template to prepare highly porous Cr2O3, Mater. Lett., 2015, 161, 172–174 CrossRef CAS
. - M. Nazari, S. Kashanianb, P. Moradipour and N. Maleki, A novel fabrication of sensor using ZnO-Al2O3 ceramic nanofibers to simultaneously detect catechol and hydroquinone, J. Electroanal. Chem., 2018, 812, 122–131 CrossRef CAS
. - M. A. Subhan, P. C. Saha, M. M. Rahman, M. A. R. Akand, A. M. Asiri and M. Al-Mamun, Enhanced photocatalytic activity and chemical sensor development based on ternary B2O3-Zn6Al2O9-ZnO nanomaterials for environmental safety, New J. Chem., 2017, 41, 7220–7231 RSC
. - W. Wang, Z. Li, W. Zheng, H. Huang, C. Wang and J. Sun, Cr2O3-sensitized ZnO electrospun nanofibers based ethanol detectors, Sens. Actuators, B, 2010, 143, 754–758 CrossRef CAS
. - D. R. Patil and L. A. Patil, Cr2O3-modified ZnO thick film resistors as LPG sensors, Talanta, 2009, 77, 1409–1414 CrossRef CAS PubMed
. - M. M. Rahman, M. M. Alam and A. M. Asiri, 2-Nitrophenol sensor-based wet-chemically prepared binary doped Co3O4/Al2O3 nanosheets by an electrochemical approach, RSC Adv., 2018, 8, 960–970 RSC
. - M. M. Rahman, M. M. Alam and A. M. Asiri, Sensitive 1,2-dichlorobenzene chemi-sensor development based on solvothermally prepared FeO/CdO nanocubes for environmental safety, J. Ind. Eng. Chem., 2018 DOI:10.1016/j.jiec.2018.01.019
. - M. M. Rahman and J. Ahmed, Cd-doped Sb2O4 nanostructures modified glassy carbon electrode for efficient detection of melamine by electrochemical approach, Biosens. Bioelectron., 2018, 102, 631–636 CrossRef CAS PubMed
. - S. J. Salih and A. K. Smail, Synthesis, characterization and evaluation of antibacterial efficacy of zinc oxide nanoparticles, Pharmaceutical and Biological Evaluations, 2016, 3, 3 Search PubMed
. - V. Manoj, M. Karthika, V. S. R. P. Kumar, S. Boomadevi, K. Jeyadheepan, R. K. Karn, R. J. B. Balaguru and S. K. Pandiyan, Synthesis of ZnO nanoparticles using carboxymethyl cellulose hydrogel, Asian J. Appl. Sci., 2014, 7, 798–803 CrossRef CAS
. - M. M. AbdElhady, Preparation and Characterization of Chitosan/Zinc Oxide Nanoparticles for Imparting Antimicrobial and UV Protection to Cotton Fabric, Int. J. Carbohydr. Chem., 2012, 2012, 840591 Search PubMed
. - K. Fooladsaz, M. Negahdary, G. Rahimi, A. H. Tamijani, S. Parsania, H. Akbari-dastjerdi, A. Sayad, A. Jamaleddini, F. Salahi and A. Asadi, Dopamine Determination with a Biosensor Based on Catalase and Modified Carbon Paste Electrode with Zinc Oxide Nanoparticles, Int. J. Electrochem. Sci., 2012, 7, 9892–9908 CAS
. - R. Srivastava, Investigation on Temperature Sensing of Nanostructured Zinc Oxide Synthesized via Oxalate Route, J. Sens. Technol., 2012, 2, 8–12 CrossRef CAS
. - M. Noroozi, S. Radiman and A. Zakaria, Influence of Sonication on the Stability and Thermal Properties of Al2O3 Nanofluids, J. Nanomater., 2014, 10 Search PubMed
. - M. R. Arefi and S. R. Zarchi, Synthesis of Zinc Oxide Nanoparticles and Their Effect on the Compressive Strength and Setting Time of Self-Compacted Concrete Paste as Cementitious Composites, Int. J. Mol. Sci., 2012, 13, 4340–4350 CrossRef CAS PubMed
. - A. K. Zak, R. Razali, W. H. A. Majid and M. Darroudi, Synthesis and characterization of a narrow size distribution of zinc oxide nanoparticles, Int. J. Nanomed., 2011, 6, 1399–1403 Search PubMed
. - Y. Zhang, F. Zhu, J. Zhang and L. Xia, Converting Layered Zinc Acetate Nanobelts To One-Dimensional Structured ZnO Nanoparticle Aggregates and
Their Photocatalytic Activity, Nanoscale Res. Lett., 2008, 3, 201–204 CrossRef CAS
. - X. Li, Z. Zhang and L. Tao, A novel microarray chemiluminescence method based on chromium oxide nanoparticles catalysis for indirect determination of the explosive triacetone triperoxide at the scene, Analyst, 2013, 138, 1596–1600 RSC
. - L. Farrell, E. Norton, B. J. O’Dowd, D. Caffrey, I. V. Shvets and K. Fleischer, Spray pyrolysis growth of a high figure of merit, nano-crystalline, p-type transparent conducting material at low temperature, Appl. Phys. Lett., 2015, 107, 031901 CrossRef
. - S. V. P. Vattikuti and C. Byon, Synthesis and Structural Characterization of Al2O3-Coated MoS2 Spheres for Photocatalysis Applications, J. Nanomater., 2015, 978409 Search PubMed
. - M. H. Zadeh, M. Razavi, O. Mirzaee and R. Ghaderi, Characterization of properties of Al–Al2O3 nano-composite synthesized via milling and subsequent casting, J. King Saud Univ., Eng. Sci., 2013, 25, 75–80 Search PubMed
. - D. A. Zatsepin, A. F. Zatsepin, D. W. Boukhvalov, E. Z. Kurmaev, Z. V. Pchelkina and N. V. Gavrilov, Electronic structure and photoluminescence properties of Zn-ion implanted silica glass before and after thermal annealing, J. Non-Cryst. Solids, 2016, 432, 183–188 CrossRef CAS
. - Y. Cong, D. Han, J. Dong, S. Zhang, X. Zhang and Y. Wang, Fully transparent high performance thin film transistors with bilayer ITO/Al-Sn-Zn-O channel structures fabricated on glass substrate, Sci. Rep., 2017, 7, 1497 CrossRef PubMed
. - H. Tian, H. Fan, G. Dong, L. Ma and J. Ma, NiO/ZnO p–n heterostructures and their gas sensing properties for reduced operating temperature, RSC Adv., 2016, 6, 109091–109098 RSC
. - Y. Jung, W. Yang, C. Y. Koo, K. Song and J. Moon, High performance and high stability low temperature aqueous solution-derived Li–Zr co-doped ZnO thin film transistors, J. Mater. Chem., 2012, 22, 5390–5397 RSC
. - Z. Zulkifli, M. Subramanian, T. Tsuchiya, M. S. Rosmi, P. Ghosh, G. Kalita and M. Tanemur, Highly transparent and conducting C: ZnO thin film for field emission displays, RSC Adv., 2014, 4, 64763–64770 RSC
. - X. Li, C. He, Y. Bai, B. Ma, G. Wang and H. Tan, Stabilization/solidification on chromium(III) wastes by C3A and C3A hydrated matrix, J. Hazard. Mater., 2014, 268, 61–67 CrossRef CAS PubMed
. - C. Ruan, K. Xie, L. Yang, B. Ding and Y. Wu, Efficient carbon dioxide electrolysis in a symmetric solid oxide electrolyzer based on nanocatalyst loaded chromate electrodes, Int. J. Hydrogen Energy, 2014, 39, 10338–10348 CrossRef CAS
. - L. Nie, A. Meng, J. Yu and M. Jaroniec, Hierarchically Macro-Mesoporous Pt/α-Al2O3 Composite Microspheres for Efficient Formaldehyde Oxidation at Room Temperature, Sci. Rep., 2013, 3, 3215 CrossRef PubMed
. - H. Cui, J. Zhou, G. Z. Yang, Y. Sun and C. X. Wang, Growth, modulation and electronic properties of Al2O3-coatings SiC nanotubes via simple heating evaporation process, CrystEngComm, 2011, 13, 902–906 RSC
. - M. M. Rahman, H. B. Balkhoyor and A. M. Asiri, Ultra-sensitive xanthine sensor development based on wet-chemically prepared Co/ZnO nanoparticles, Mater. Express, 2017, 7, 2 Search PubMed
. - M. Dervisevic, E. Custiuc, E. Çevik, Z. Durmus, M. Şenel and A. Durmus, Electrochemical biosensor based on REGO/Fe3O4 bionanocomposite interface for xanthine detection in fish sample, Food Control, 2015, 57, 402–410 CrossRef CAS
.
Footnote |
† Electronic supplementary information (ESI) available. See DOI: 10.1039/c8ra01734d |
|
This journal is © The Royal Society of Chemistry 2018 |
Click here to see how this site uses Cookies. View our privacy policy here.