DOI:
10.1039/C9NR05671H
(Paper)
Nanoscale, 2019,
11, 21759-21766
One-pot synthesis of hollow PDA@DOX nanoparticles for ultrasound imaging and chemo-thermal therapy in breast cancer†
Received
5th July 2019
, Accepted 22nd August 2019
First published on 22nd August 2019
Abstract
Constructing nanocarriers with high drug loading capacity is a challenge, which limits the effective delivery of drugs to solid tumors. Here, we reported a one-pot synthesis of hollow nanoparticles (NPs) encapsulated by doxorubicin (DOX) and modified with polydopamine (PDA) to form PDA@DOX NPs for breast cancer treatment. PDA@DOX NPs demonstrated exceptionally high capacity (53.16%) for loading DOX. In addition, when PDA@DOX NPs were administered systemically, they exhibited responsive aggregation in the tumor sites and demonstrated a good controlled release effect for DOX due to the weak acidic environment of the tumor sites and targeting near-infrared (NIR) light irradiation. The PDA outer layer absorbed the near-infrared (NIR) light and facilitated simultaneous generation of heat energy for destroying the tumor cells to release the drug upon NIR irradiation. Moreover, this NIR-activated combined/synergistic therapy exhibited remarkably complete tumor growth suppression in a breast cancer mouse model. Importantly, NPs exhibited a good ultrasound performance both in vitro and in vivo, which could monitor the treatment process. In conclusion, this NIR-activated PDA@DOX NP system is demonstrated as a good US-guided combination (chemotherapy + PTT) therapy platform with high loading capacity and controlled drug release characteristics, which is promising for the treatment of breast cancer.
Introduction
As per the American Cancer Society (2016 statistics), in women, the most prevalent cancer is breast cancer and it is the second highest cause of cancer-associated death. The main clinical treatment for treating tumors is chemotherapy by systemic injections.1 The risk of recurrence and death due to breast cancer in a 10-year period can be moderately reduced by enhancing the dose intensity of adjuvant chemotherapy.2 However, traditional chemotherapeutic drugs are non-targeting and have short circulation in blood, which can cause insufficient therapeutic dosage at the tumor site through intravenous administration, leading to low chemo-efficacy, drug resistance and failure of chemotherapy.3,4 The current deficits in treatments can be avoided through advancements in chemotherapeutic methodologies, especially in drug delivery systems at the nanoscale. Nanoscale drug carriers have the advantages of small sizes, long blood circulation times, large loading ratios and unique therapeutic properties of high accumulation in the tumor sites, controlled release, and synergistic photothermal therapy (PTT) and/or photodynamic therapy (PDT).5–8 Therefore, it is required to produce nanomaterial-based drug carriers to enhance the efficiency of chemotherapy with a concurrent reduction in systemic toxicity.
Nowadays, several strategies for improving the therapeutic efficacy of nanocarriers are being addressed through different approaches. For instance, the accumulation of the number of nanocarriers in tumors can be enhanced by decreasing the size of the nanoparticles (NPs) in addition to surface modification, which can extend their circulation in blood and provide better penetration through biological barriers.9,10 An additional approach is to construct NPs with multiple roles working synergistically in the treatment process and thus reducing the doses, resulting in proper efficiency.11–13 PTT is one such modality in breast cancer treatment that has garnered much attention. Through this treatment, a near-infrared (NIR) light is converted to thermal energy, which ultimately kills cancer cells photothermally14 and also improves the efficiency of chemotherapy via localized heating produced by the photothermal effect.15,16 In this context, the functional theranostic nanosystem development is receiving increasing attention.17,18 So far, the market has more than 11 types of nanomedicines, and more than 45 types of nanomedicines are under advanced phases of clinical trials.19 These nanomedicines provide numerous options for drug nanocarrier development; these include HNTs (halloysite nanotubes) loaded with GNRs (gold nanorods) and DOX, which are found to provide effective combination (chemotherapy + PTT) therapy synergistically. The DOX-related toxicity to normal tissues can be reduced based on the fact that the same curative effect at a low dosage will be ensured.20 Accordingly, a nanocarrier with exceptionally high pH-/NIR-responsive drug release and drug loading capacity for the combination (chemotherapy + PTT) therapy has been reported by Mu et al.21 Thus, chemotherapy combined with PTT can be potentially promising in breast cancer treatment.
In this study, a PDA-based hollow drug delivery system by combining chemotherapy (DOX) and PTT with the capacity of ultrasound imaging was developed. Especially, mesoporous ZIF-90@DOX was used as a hard template to prepare hollow PDA@DOX, attributing to a high drug payload. The developed PDA@DOX NPs have several benefits: (1) the hollow structure endowed NPs with a high drug (DOX) loading rate for chemotherapy; (2) the tumors were directly destroyed by the PDA layer through the deposition of thermal energy and also by thermal-responsive DOX delivery to further stimulate and raise the therapeutic effect; (3) the gas within the hollow structure cavities could enhance ultrasound (US) imaging for specific guidance of therapy. Thus, PDA@DOX NPs have a great clinical potential in the combination therapy against tumors.
Results and discussion
Synthesis and characterization of PDA@DOX
The hollow-structured PDA@DOX was successfully synthesized in two steps in an aqueous solution (Fig. 1a). First, DOX-encapsulated nano ZIF-90 was synthesized through the one-step self-assembling method. The size and morphology of ZIF-90@DOX (Fig. 1b), as characterized by transmission electron microscopy (TEM), showed that it possessed spherical morphology and smooth surface. Then, the PDA layer was coated on the ZIF-90@DOX core through the hard-templating method, followed by the removal of the templates to leave a hollow interior. For this, ZIF-90@DOX NPs were mixed with dopamine after being disseminated in a Tris-HCl buffer (pH 8.5). The dopamine molecules self-polymerized in aerobic and slightly alkaline conditions to form lean, adherent PDA layers on the surface of the ZIF-90@DOX nanoparticles. PDA@DOX NPs were also examined by TEM after coating. As seen in Fig. 1c, a hollow structure with a black outer layer is clearly observed, and no evident surface feature due to the coating of PDA is noticed. This may be because the smooth, uniform coating of PDA did not significantly affect the spherical shape of ZIF-90@DOX NPs. Hollow PDA NPs were synthesized by the same process without using DOX. Then, dynamic light scattering (DLS) and zeta potential experiments were performed to characterize both PDA and PDA@DOX NPs. The mean diameters of PDA and PDA@DOX were 152.83 ± 4.71 nm (PDI < 0.19) and 159.87 ± 1.62 nm (PDI < 0.16), with negative zeta potentials of −14.57 ± 0.35 mV and −3.46 ± 0.02 mV in PBS, respectively (Fig. 1d and e). The stability during long-term storage in physiological conditions was also tested (Fig. S1, ESI†). The UV-vis absorption spectrum of PDA@DOX (Fig. 1c) shows sharp absorption peaks of DOX and PDA@DOX at 235 and 288 nm, respectively, indicating that DOX was successfully loaded inside hollow PDA. Then, the loading amount of DOX was calculated to be 53.16% (5.32 mg DOX/4.69 mg PDA), indicating that hollow PDA@DOX could be used as a drug carrier with an excellent loading rate.
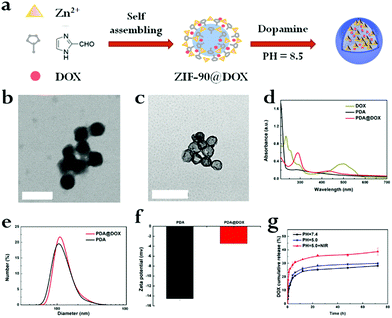 |
| Fig. 1 (a) The process of synthesis of PDA@DOX NPs. (b) Images of ZIF-90@DOX as visualized through TEM. (c) TEM images of PDA@DOX. (d) The UV-vis absorbance spectrum of DOX, PDA and PDA@DOX. DLS curves (e) and zeta potential (f) of PDA and PDA@DOX. (g) In vitro cumulative release of DOX from PDA@DOX (pH = 7.4, 7.4 + NIR, 5.0 or 5.0 + NIR) in PBS for 3 days at 37 °C. Inset scale bar is 200 nm for all images. | |
In vitro drug release and US imaging
Next, the drug release attribute of PDA@DOX NPs in different conditions (pH = 7.4, 7.4 + NIR, 5.0, 5.0 + NIR) was determined, followed by UV-vis spectroscopy for quantification. The DOX standard curve was first obtained (Fig. S2, ESI†). As per Fig. 1f, it can be inferred that after 120 h, 28.19% of DOX is released from NPs at pH 7.4, while 29.94% of DOX is released at pH 5.0. This result indicated that the acidic environments led to increased DOX release, which was faster at pH 5.0 than at pH 7.4. This may be due to alterations in the charge interactions of DOX with PDA along with increased solubility of DOX in water at lower pH.21 Moreover, the amine groups on DOX may be fully protonated at acidic pH to significantly enhance the solubility and hydrophilicity of DOX in aqueous media.21 This drug release action in response to the changes in pH is crucial for applications in vivo because the microenvironments of the tumors, endosomes, and intracellular lysosomes are all acidic and promote the release of the drug from PDA@DOX NPs in tumor cells. Besides the pH-responsive drug release, another use of NIR irradiation is to initiate the release of the drug from PDA@DOX. An 808 nm NIR (1.5 W cm−2) light was used to determine the kinetics of the drug release from PDA@DOX NPs in response to NIR irradiation. The result indicated that the DOX release from PDA@DOX under NIR irradiation at pH 5.0 (38.72%) was higher than that at pH 5.0 without NIR irradiation; this indicated that the drug-releasing system's temperature may increase due to the NIR irradiation of the tumor site and DOX release could be enhanced at a high temperature. This result might be attributed to the fact that the PDA outer layer degraded at a higher temperature. Hence, PDA@DOX NPs showed NIR- and pH-sensitivity and were beneficial in alleviating the side effects, which acted systemically, augmenting the antitumor efficiency.
To investigate the suitability of PDA@DOX NPs for US imaging, assays using varying concentrations of PDA@DOX NP solutions were carried out in vitro. US images were tested with the Resona 7S clinical ultrasonic equipment (Mindray, China) in two modes: the B mode and the contrast enhanced ultrasound (CEUS) mode. The CEUS images were quantitatively analysed via time-intensity curves (TICs). The US images in both the modes were observed (Fig. 2a), and the CEUS mode was observed to present more obvious and stronger signals than the B-mode, thus indicating greater sensitivity of CEUS to the PDA@DOX NP signal. The contrast peak intensity was quantitatively accumulated (Fig. 2b) and showed the same trend as the concentration-dependent CEUS images. The PBS control group gave no obvious US signal. Thus, PDA@DOX NPs can be used as ideal US contrast agents and are more appropriate for the imaging conditions of CEUS in the existing US instruments.
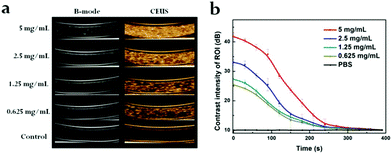 |
| Fig. 2 (a) In vitro US imaging on various concentrations of PDA@DOX. (b) Time-dependent contrast intensity of ROI. | |
In vitro photothermal performance and cell damage
We investigated the potential of PDA@DOX NPs to act as a photothermal agent in an aqueous solution. At 808 nm NIR irradiation continuously (1.5 W cm−2), both photothermal performance and photothermal stability studies were noted and saved by an infrared thermal camera. PDA is considered as a distinguished photosensitizer approved by the FDA, which can absorb light to generate heat, and it is a very promising agent in clinical use.22 The near-infrared light is one of the most frequently used wavelengths in PTT, and 808 nm has been widely used in breast cancer therapy.23,24Fig. 3a shows temperature increase of the PDA@DOX aqueous solution dependent on concentration after NIR exposure (1.5 W cm−2, 10 min). The aqueous solution with 400 μg mL−1 PDA@DOX NPs showed dramatic temperature increase of 19.1 °C in 10 min, while other groups did not reach 40 °C. Contrarily, the temperature change of pure water barely showed a response to NIR. Therefore, the concentration of 400 μg mL−1 PDA@DOX was considered optimum for further experiments. We then studied the effect of NIR intensity on the temperature change. When the density of NIR was enhanced from 0.3 W cm−2 to 2.0 W cm−2, there was stable increase in the temperature of the PDA@DOX aqueous solution from 25.0 to 45.5 °C. The photothermal performance of NIR at 1.5 W cm−2 (44.1 °C) was quite close to that at 2.0 W cm−2. In contrast, the temperature of the PDA@DOX aqueous solution only reached up to 41.1 °C at 1.0 W cm−2 and 36.1 °C at 0.5 W cm−2 after 10 min NIR irradiation (Fig. 3b). Therefore, 1.5 W cm−2 was considered as optimum at 400 μg mL−1 PDA@DOX concentration in the following investigations. Furthermore, upon NIR irradiation, the photothermal stability of PDA@DOX NPs is important because of their role as PTT agents. As per the differences in the temperatures of the PDA@DOX aqueous solutions over 10 ON/OFF cycles for 808 nm NIR irradiation at 400 μg mL−1, 1.5 W cm−2, there was no remarkable decrease at the end of the cycle (Fig. 3c).
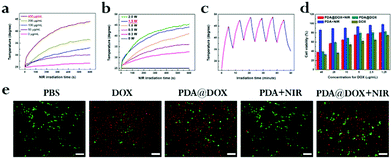 |
| Fig. 3 The elevation in temperature of PDA@DOX aqueous solution at (a) concentration of 400 μg mL−1 for 10 min and (b) power density of 1.5 W cm−2 for 10 min exposed to 808 nm NIR irradiation. (c) The variation in temperature of PDA@DOX aqueous solution for 10 ON/OFF cycles after 808 nm NIR irradiation at 400 μg mL−1, 1.5 W cm−2, 10 min. (d) The viability of 4T1 cells after treatment with PDA@DOX, PDA and DOX in the presence or absence of NIR irradiation (1.5 W cm−2, 10 min). (e) Fluorescence images of PANC-1 cells incubated with PDA@DOX, PDA and DOX in the presence or absence of NIR irradiation at 1.5 W cm−2, 10 min. The cells were stained by Calcein-AM/PI; green for alive and red for dead cells. For all images, inset scale bar is 50 μm. | |
Cellular uptake
The excellence of PDA@DOX NPs in photothermal conversion in vitro guarantees their PTT performance at the cell level. The uptake of DOX and PDA@DOX by cells was first analysed by laser scanning confocal microscopy (LSCM) and flow cytometry. The cell membrane was stained green with FITC phalloidin (Ex = 488 nm, Em = 540–580 nm), the nuclei were stained blue with Hoechst (Ex = 405 nm, Em = 420–480 nm), and autofluorescence imaging of DOX was red (Ex = 488 nm, Em = 600–660 nm). As shown in Fig. S3,† both DOX and PDA@DOX (red fluorescent substances) were detected in the cytoplasm, indicating an efficient uptake of NPs by the cells. Then, the cellular uptake of DOX and PDA@DOX NPs (Fig. S4a and S4b, ESI†) was quantified by flow cytometry. After being co-incubated with 4T1 cells, the mean fluorescence intensity (MFI) values were much higher for the DOX and PDA@DOX groups (1580.33 and 1100.67, respectively) compared to that for the PBS group (202.33). These results suggested that DOX and PDA@DOX can be ingested by the 4T1 cells after 8 h incubation. Next, we conducted a CCK-8 assay to assess the potential toxicity of PDA to the 4T1 cells in vitro and observed no appreciable negative effect to the viability of the cells after incubation even at 1 mg mL−1, suggesting that PDA is non-toxic and safe to cells (Fig. S5, ESI†). Then, the inhibition effect of free DOX, PDA and PDA@DOX NPs in the presence or absence of NIR irradiation on the growth of the 4T1 cells was evaluated by the CCK-8 assay (Fig. 3d). The cell viability results showed a decreasing trend with the increase in the concentration of NPs. Many more cells were killed by the “chemotherapy + PTT” treatment compared to the observations for the PDA + NIR and PDA@DOX groups; this confirmed that PDA@DOX was a potentially efficient PTT agent and drug carrier because the PTT-treated cancer cells had enhanced sensitivity to chemotherapeutic drugs.20 The calcein-AM/PI double staining kit was also used to evaluate cell activity (green for living cells and red for dead cells) under different treatments (Fig. 3e). The results also confirmed that the combination therapy of PTT and a chemotherapeutic drug is highly efficient in destroying tumor cells and is more advantageous than the single-mode treatment.
In vivo US imaging
Furthermore, in vivo US-imaging experiments were performed to evaluate NP localization during the cancer treatment in 4T1 tumor-bearing nude mice (Fig. 4a). The variations in the US signals were concurrently monitored using the B-mode and CEUS dual imaging system, which is commonly used for clinical US examinations. In comparison to the observations prior to injection, the US signal of the PDA@DOX group was amplified 2 min after a tail-vein injection in the tumor region. CEUS exhibited a better effect of tumor enhancement than the B-mode, which was consistent with the conclusion of in vitro US imaging. We selected a circular region of the tumor to determine the enhanced CEUS signal in the tumor area; the peak contrast intensity value was measured and plotted every 30 seconds (Fig. 4b). The signal intensity of the tumor region was observed to increase gradually in the time periods from 2 minutes to 5 minutes and reached a peak value. This may be due to the small size of the targeted NPs, which facilitated their accumulation in the tumor tissue because of the EPR effect. These results thus indicate that the use of PDA@DOX NPs can be established for the real-time monitoring of tumors by US imaging.
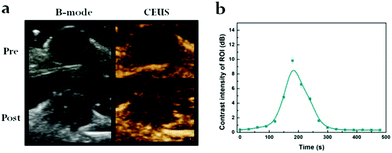 |
| Fig. 4 (a) In vivo US imaging (B-mode, CEUS) of tumor in 4T1-bearing mouse. (b) Time-dependent contrast intensity of ROI. | |
In vivo distribution, tumor inhibition, biocompatibility and pharmacology
As PDA@DOX NPs have an exceptionally good in vitro photothermal performance, the images (photothermal) and changes in the temperature of mice tumor were captured at predetermined time (1, 2, 4, 8, 12 and 14 h) intervals using an infrared thermal imaging camera. In contrast, the tumor temperature of saline-treated mice had no notable fluctuation after 10 min irradiation. The tumor temperature of mice after treatment with PDA@DOX rapidly increased from 48.9 °C to 55.0 °C and aggregated most in the tumor sites at 8 h after intravenous injection (Fig. 5a and S6, ESI†). Moreover, the temperature change curves were obtained (Fig. S7, ESI†). The cancer cells can be inactivated at 41 °C due to their poor blood supply.25 Therefore, the results demonstrated that PDA@DOX has an outstanding photothermal performance and is a promising photosensitizer for antitumor therapy.
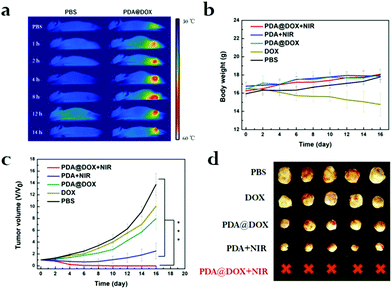 |
| Fig. 5 (a) In vivo photothermal imaging of PDA@DOX at predetermined time. Body weight (b) and tumor volume (c) of mice after different treatments (5 mice per group). (d) The corresponding tumor photographs resected from mice on the 16th day (***p < 0.05 in inset (c)). | |
According to in vivo photothermal imaging, PDA@DOX is speculated to have a prominent antitumor effect in tumor-bearing mice. As presented in Fig. 5b, during these periods, there is no obvious loss of the body weight of the mice in all the treatment groups except in the DOX group. The decrease in body weight was due to DOX toxicity as it exhibited certain toxicity in mice. However, no toxicity to mice was observed due to PDA@DOX NPs. Thus, PDA@DOX NPs may be a potentially superior nanosystem for cancer therapy. Therefore, there was reduction in the toxicity to normal tissues while simultaneously maintaining a good therapeutic effect. Moreover, the tumor volume calculated every 2 days is shown in Fig. 5c (p < 0.05). The relative tumor volume of the tested groups showed a notable therapeutic effect compared to that of the PBS group. By comparing the efficacies of free DOX and the PDA@DOX group, PDA@DOX was proven to perform better, which illustrated that the use of a drug carrier can improve the therapeutic effect through better biocompatibility, longer duration of blood circulation and pH-response in the tumor sites. The tumor was first suppressed after the PTT treatment and then began to recur on the sixth to the eleventh day in the PTT only group. In a marked contrast, significant tumor suppression was observed in the PDA@DOX + NIR group. These outcomes confirmed the NIR light's limitation of penetrating depth; thus, it is often difficult for PTT to kill tumors completely, which might result in recurrence of the tumor growth. Therefore, the PTT combined chemotherapy can play a synergistic role and improve the therapeutic effect in breast cancer. Furthermore, when the tumor tissues were assessed by H&E staining after 24 h of treatment (Fig. S8, ESI†), significant cell necrosis and apoptosis could be observed on the graph (black arrow), further indicating that PDA@DOX + NIR possessed effective tumor-suppressing capacity. On day 16, resection of each tumor tissue was done and the image was captured (Fig. 5d). Furthermore, the biocompatibility of PDA analysed by the H&E staining (Fig. S9, ESI†) of the main organs (lungs, heart, kidneys, spleen, and liver) and blood routine examination (Fig. S10, ESI†) on day 21 after the injection of saline and PDA showed no obvious damage.
The pharmacological effects of PDA@DOX were assessed in vivo, and the pharmacokinetics of DOX were further studied (Fig. S11, ESI†). As expected, the half-life (t1/2) of PDA@DOX (3.13 hours) in the blood circulation was significantly prolonged compared to that of free DOX, which was 0.64 hours. The maximum concentration of PDA@DOX in the plasma 3 min after injection was 5.36 times (64.70 mg mL−1) the level of free DOX. In addition, the area under the curve (AUC 0–24 h) of PDA@DOX (102.70 mg mL−1 h−1) was about 13.46 times that of free DOX (7.63 mg mL−1 h−1). The above experimental data showed that PDA@DOX significantly prolongs the half-life of blood circulation and improves the pharmacokinetic parameters of DOX in vivo. Therefore, it is probably enriched at the tumor sites by delaying the rate of clearance of the drug in the blood.
Materials and methods
Materials
Zn(CH3COOH)2·2H2O and imidazolate-2-carboxyaldehyde (ICA) were purchased from Infinity Scientific Co. Ltd (Beijing, China). DOX hydrochloride, N,N-dimethylformamide (DMF) and FITC phalloidin were procured from Sigma-Aldrich (China). Dopamine hydrochloride and Hoechst were procured from Aladdin (China). The live-dead cell staining kit Alcein-AM/PI was purchased from Yeasen Biological Technology (China). Deionized water from Millipore Milli-Q grade (18.2 MΩ resistivity) was used in all the experiments. The chemicals were used with no further purification.
Animals and cell lines
4T1 mouse breast cancer cells were procured from the Shanghai Gefan Biotechnology CO (Shanghai, China). Cells were cultured in an RPMI 1640 medium (Hyclone, USA) containing fetal bovine serum (10%; Hyclone, USA) at 37 °C in an incubator with 5% CO2. Nude mice (5–6 weeks old) were procured from BiKai Biological products sales center in Nanjing, China. All experimental protocols were reviewed and consented by Ningbo University's Regional Ethics Committee for Animal Experiments (Permit No. SYXK Zhe 2013-0191).
Synthesis of PDA@DOX
PDA@DOX NPs were synthesized by a previously reported method26,27 (Fig. 1a). Briefly, 2 mL each of Zn(CH3COOH)2·2H2O (0.1 M) and ICA (imidazolate-2-carboxyaldehyde; 0.2 M) was mixed with 5 mg mL−1 DOX vigorously. After stirring for 5 min, DMF (10 mL) was added into the reaction mixture and stirred for additional 20 min. The products were washed with DMF and methanol and purified by centrifugation. ZIF-90@DOX NPs were then stored at 4 °C in the absence of light after vacuum drying for 12 h at room temperature. Then, ZIF-90@DOX NPs were coated with PDA by mixing with dopamine hydrochloride (mass ratio of 3
:
1) in Tris-HCl buffer (10 mmol L−1, pH 8.5) for 24 h at room temperature with no rotation. PDA@DOX NPs were finally acquired by washing thrice with deionized water and drying for 12 h in vacuum at room temperature. A similar process was used to synthesize hollow PDA nanoparticles without using DOX.
Photothermal performance and photothermal stability studies
Experiments were carried out using a digital photothermal imaging system to evaluate the PDA@DOX (1.0 mL, dispersed in a cuvette) photothermal performance at 808 nm irradiation. (1) We changed the concentration of PDA@DOX and the power density was fixed at 1.5 W cm−2 for 10 min irradiation. (2) We changed the power density and the concentration of PDA@DOX was fixed at 400 μg mL−1 for 10 min irradiation. Photothermal stability of the exposure of PDA@DOX to 808 nm NIR was measured by controlling its exposure at 5 min intervals for 10 ON/OFF cycles.
pH-Dependent drug release and biocompatibility
The measurement of the in vitro cumulative release of DOX from PDA@DOX was done through a dynamic dialysis method.28 Briefly, 1 mL of the PDA@DOX solution (1 mg mL−1) was placed in a dialysis bag (MWCO: 2.0 kDa), dialyzing in an oscillation incubator at 100 rpm. The dialysis bags contained 20 mL PBS under different conditions (pH = 7.4, 5.0, 5.0 + NIR). After NIR irradiation at 808 nm (1.5 W cm−2, 10 min), each time, 1 mL sample was removed and replaced with the same volume of mixed solution. The drug release was calculated by determining the amount of DOX using a UV-Vis spectrophotometer. The biocompatibility of PDA was calculated by standard MTT assays using 4T1 cell lines as the model. Cells were seeded in a 96-well plate at 5 × 103 per well and incubated overnight in 5% CO2 at 37 °C. PDA (100 μl) was added to the media at different concentrations (80, 60, 40, 20, 10, 5, 2.5, 1.25 and 0.625 μg mL−1), and the cells were further incubated for 24 hours. At the end of incubation, a CCK-8 solution (10 μL) was added per well. The optical density was examined using a microplate reader at the wavelength of 450 nm.
Cellular uptake
The investigation of the cellular uptake of DOX and PDA@DOX was done by CLSM. 4T1 cells (2.0 mL) were seeded in confocal culture dishes (NETS Co., USA) at a density of 1 × 105 and incubated for 24 hours. DOX and PDA@DOX (100 μg mL−1) were added and incubated for 8 hours. After washing for three times with PBS, fixation of cells was done with formaldehyde (4%) for 30 min; they were treated with Triton (0.1%) for the next 5 min, and BSA (1.0%) was added. Then, FITC phalloidin (5 μg mL−1) and Hoechst 33258 Stain (10 μg ml−1) solutions were added in dishes for 30 min at room temperature. Finally, the samples were measured by CLSM. The cellular uptake was also analyzed by flow cytometry, and the 4T1 cells were seeded into six-well plates coated with 0.01% poly(Lys) at a density of 1 × 105 cells per well. Cells (2 mL) were incubated in a complete 1640 medium overnight and allowed to adhere prior to their removal and treatment with a fresh medium containing DOX and PDA@DOX (100 μg mL−1) for another 8 hours. Then, the cells were washed thrice with PBS to remove free NPs and detached with trypsin-EDTA. The result was analyzed by flow cytometry (FACSCalibur, BD, USA).
In vitro therapy
4T1 cells were seeded in 96-well plates (100 μL, 5 × 104 cells per mL) and permitted to adhere for 24 h. Then, the culture medium was replaced by a 100 μL 1640 medium containing free DOX, PDA, PDA@DOX (40, 20, 10, 5, 2.5 and 1.25 μg mL−1 for DOX) and incubated for 8 h. After washing with PBS for three times, 100 μL of 1640 was added into cells. Then, the cells were irradiated by 808 nm NIR for 10 min (1.5 W cm−2) and incubated for 16 h under the following different conditions: (1) cells + DOX, (2) cells + PDA@DOX, (3) cells + PDA + NIR, (4) cells + PDA@DOX + NIR. A microplate reader was used to examine the cell viability according to the absorbance at 450 nm.
Staining of live/dead cells
Live/dead cell staining is measured by a Calcein-AM/PI double stain kit. 4T1 cells (100 μL, 5 × 104 cells per mL) were seeded in 96-well plates for 24 h. The culture medium was then replaced by 100 μL 1640 medium containing free DOX, PDA, PDA@DOX (10 μg mL−1 for DOX) and incubated for another 8 h. After washing with PBS for three times, 100 μL of DMEM was added to the cells. Then, the cells were irradiated by 808 nm NIR for 10 min (1.5 W cm−2) and incubated for 16 h under the following different conditions: (1) DOX, (2) PDA@DOX, (3) PDA + NIR, (4) PDA@DOX + NIR. Finally, the cells were stained by Calcein-AM/PI solution for 30 min at 37 °C. The imaging was evaluated by a fluorescence microscope.
In vitro and in vivo ultrasound imaging (USI)
US imaging was carried out with Resona 7S clinical ultrasonic equipment (China). First, 2 mL PDA@DOX in a series of concentrations (5, 2.5, 1.25 and 0.625 mg ml−1) was placed into a self-made gelatine phantom and B-mode imaging and CEUS imaging were acquired through a linear array transducer (L14-5WU) at a central frequency of 7.5 MHz (AP 5.50%, MI 0.079). The relative average intensities of the interest area were calculated within a circular region inside the tubes with a diameter of 5 mm. For in vivo USI, two 4T1 tumor-bearing nude mice were anaesthetized with 6% chloral hydrate through intraperitoneal injections. After injecting 100 μL PBS and PDA@DOX (2.0 mg mL−1), the image was observed and acquired by a linear array transducer (L11-3U) at a central frequency of 7.5 MHz (AP 1.82%, MI 0.085).
In vivo biocompatibility
For toxicity evaluation, mice treated with PDA were used to evaluate the in vivo biocompatibility. Healthy nude mice were injected with 100 μL PDA (5.0 mg mL−1), while 100 μL of PBS was injected as control and observed over 21 days for behavioural changes. Following this, blood and the main organs were extracted from all mice after sacrificing them. The blood was used for routine examination. The organs (i.e., lung, heart, kidney, liver, and spleen) were preserved in 4% paraformaldehyde solution and stained with H&E staining to assess the toxicity of PDA.
In vivo photothermal imaging
When the tumor grew to 50–100 mm3 size, mice were injected with PDA@DOX NPs (100 μL, 5.0 mg mL−1) and normal saline (100 μL) through tail vein. Then, the mice were irradiated by an 808 nm laser (1.5 W cm−2, 10 min) after injection at predetermined time (1, 2, 4, 8, 12 and 14 h) intervals. Then, the photothermal imaging was done using a thermal-infrared camera and the temperature changes in the tumor tissues of mice were recorded at the same time.
In vivo anticancer effect
In order to further evaluate the combined efficacy of animal experiments, the mice with tumors were injected with 2 × 105 4T1 cells in the flank of nude mice. After the tumor growth to 50–100 mm3, the nude mice were arbitrarily divided into six groups, five members per group (i.e., free DOX, PDA, PDA@DOX, PDA + NIR, PDA@DOX + NIR and PBS), and we injected 100 μL free DOX, PDA, PDA@DOX (5 mg kg−1 for DOX) intravenously through the tail vein on day 0. After 8 hours, mice were anaesthetized using intraperitoneal injections with 6% chloral hydrate. The tumor was given 808 nm NIR irradiation at 1.5 W cm−2 for 10 min. PBS was injected as control. Then, 24 h after treatment, tumors and major organs from one mouse of each group were selected for hematoxylin–eosin staining (H&E). The tumor sizes were calculated with a calliper every two days as follows: tumor volume (mm3) = long diameter × short diameter2/2. The body weights of all the groups were also monitored every two days. After 14 days, all nude mice were sacrificed to select tumors for taking pictures.
Pharmacokinetics study
Female Balb/C mice (7–8 weeks) were injected with DOX and PDA@DOX (5 mg kg−1 for DOX) through the tail vein (n = 3). Blood samples were collected from the retro-orbital venous plexus at timed intervals (3 min, 10 min, 20 min, 1 h, 2 h, 4 h, 8 h, 12 h, and 24 h) and put in blood routine anticoagulant tubes (BDEDTA, US). After 10 min centrifugation at 4500 rpm, the supernatant (150–200 μL) was mixed with the same volume of acetonitrile solution to precipitate the plasma proteins and centrifuged. The supernatant was taken for UV-vis absorbance spectrum analysis.
Conclusions
To summarize, we synthesized a novel nanoplatform for effective combined chemo-thermal therapy toward breast cancer. The hollow PDA@DOX structure provided high DOX loading capacity (53.16%) and more gas inside to greatly enhance the US images. Moreover, PDA@DOX NPs exhibited acid–base-dependent drug release under the tumor microenvironment that was acidic in nature and a targeted drug release function under NIR illumination, which led to efficient tumor site enrichment for the complete suppression of the tumor growth. Thus, they can simultaneously serve as nanocarriers with the ability of dual stimuli-responsive drug delivery, as photosensitizers for combination (chemotherapy + PTT) therapy, and as contrast agents for US imaging to monitor the PTT process. Therefore, the novel combination treatment nanoplatform of PDA@DOX is a safe, effective, and potentially promising choice for breast cancer therapy.
Conflicts of interest
There are no conflicts to declare.
Acknowledgements
This work was supported by the National Natural Science Foundation of China (Grant No. 81527803, 81420108018, U1432114, 81550110258, 8161101589, 81650410654), National Key R&D Program of China (No. 2018YFC0115900, 2018YFC0910600), Key Breakthrough Program of Chinese Academy of Sciences (KGZD-EW-T06), Zhejiang Science and Technology Project (No. 2019C03077), the Hundred Talents Program of Chinese Academy of Sciences (2010-735), Youth Natural Science Fund Project of Zhejiang Province (LQ19H180004) and Natural Science Fund Project of Ningbo (2018A610380). We acknowledge Ruifen Zou for help in animal experiment, and Libin Chen for help of Ultrasound imaging performance.
Notes and references
- K. D. Miller,
et al., Cancer treatment and survivorship statistics, 2016, CA-Cancer J. Clin., 2016, 66, 271–289 CrossRef PubMed.
- R. Gray,
et al., Increasing the dose intensity of chemotherapy by more frequent administration or sequential scheduling: a patient-level meta-analysis of 37
298 women with early breast cancer in 26 randomised trials, Lancet, 2019, 393, 1440–1452 CrossRef.
- A. Coates,
et al., On the receiving end–patient perception of the side-effects of cancer chemotherapy, Eur. J. Cancer Clin. Oncol., 1983, 19, 203–208 CrossRef CAS PubMed.
- H. Lage, An overview of cancer multidrug resistance: a still unsolved problem, Cell. Mol. Life Sci., 2008, 65, 3145–3167 CrossRef CAS PubMed.
- M. Gautam,
et al., Aerosol technique-based carbon-encapsulated hollow mesoporous silica nanoparticles for synergistic chemo-photothermal therapy, Acta Biomater., 2019, 88, 448–461 CrossRef CAS PubMed.
- W. Hou,
et al., pH-Sensitive self-assembling nanoparticles for tumor near-infrared fluorescence imaging and chemo-photodynamic combination therapy, Nanoscale, 2016, 8, 104–116 RSC.
- T. Li,
et al., Surface-engineered nanobubbles with pH-/light-responsive drug release and charge-switchable behaviors for active NIR/MR/US imaging-guided tumor therapy, NPG Asia Mater., 2018, 10, 1046–1060 CrossRef CAS.
- A. Zhang,
et al., Carbon-gold hybrid nanoprobes for real-time imaging, photothermal/photodynamic and nanozyme oxidative therapy, Theranostics, 2019, 9, 3443–3458 CrossRef PubMed.
- C. Saraiva,
et al., Nanoparticle-mediated brain drug delivery: Overcoming blood-brain barrier to treat neurodegenerative diseases, J. Controlled Release, 2016, 235, 34–47 CrossRef CAS PubMed.
- B. Xu,
et al., A Single-Atom Nanozyme for Wound Disinfection Applications, Angew. Chem., Int. Ed., 2019, 58, 4911–4916 CrossRef CAS PubMed.
- J. Y. Yhee,
et al., Nanoparticle-Based Combination Therapy for Cancer Treatment, Curr. Pharm. Des., 2015, 21, 3158–3166 CrossRef CAS PubMed.
- F. Zhang,
et al., Interventional Photothermal Therapy Enhanced Brachytherapy: A New Strategy to Fight Deep Pancreatic Cancer, Adv. Sci., 2019, 6, 1801507 CrossRef PubMed.
- X. Pan,
et al., Metal-Organic-Framework-Derived Carbon Nanostructure Augmented Sonodynamic Cancer Therapy, Adv. Mater., 2018, 30, 1800180 CrossRef PubMed.
- Q. Yang,
et al., Polypyrrole-coated phase-change liquid perfluorocarbon nanoparticles for the visualized photothermal-chemotherapy of breast cancer, Acta Biomater., 2019, 90, 337–349 CrossRef CAS.
- W. Gao,
et al., Targeting and destroying tumor vasculature with a near-infrared laser-activated “nanobomb” for efficient tumor ablation, Biomaterials, 2017, 139, 1–11 CrossRef CAS PubMed.
- W. Li,
et al., Overcoming photodynamic resistance and tumor targeting dual-therapy mediated by indocyanine green conjugated gold nanospheres, J. Controlled Release, 2017, 258, 171–181 CrossRef CAS PubMed.
- H. Lee,
et al., Combination of chemotherapy and photodynamic therapy for cancer treatment with sonoporation effects, J. Controlled Release, 2018, 283, 190–199 CrossRef CAS PubMed.
- Y. Zhao,
et al., Drug Delivery System Based on Near-Infrared Light-Responsive Molybdenum Disulfide Nanosheets Controls the High-Efficiency Release of Dexamethasone To Inhibit Inflammation and Treat Osteoarthritis, ACS Appl. Mater. Interfaces, 2019, 11, 11587–11601 CrossRef CAS PubMed.
- T. Sun,
et al., Engineered nanoparticles for drug delivery in cancer therapy, Angew. Chem., Int. Ed., 2014, 53, 12320–12364 CAS.
- J. Zhang,
et al., Rod in Tube: A Novel Nanoplatform for Highly Effective Chemo-Photothermal Combination Therapy toward Breast Cancer, ACS Appl. Mater. Interfaces, 2019, 11, 3690–3703 CrossRef CAS PubMed.
- Q. Mu,
et al., Biconcave Carbon Nanodisks for Enhanced Drug Accumulation and Chemo-Photothermal Tumor Therapy, Adv. Healthcare Mater., 2019, 8, e1801505 CrossRef PubMed.
- Z. Zhu and M. Su, Polydopamine Nanoparticles for Combined Chemo- and Photothermal Cancer Therapy, Nanomaterials, 2017, 7, 160 CrossRef PubMed.
- Y. Tang,
et al., Transformable nanotherapeutics enabled by ICG: towards enhanced tumor penetration under NIR light irradiation, Nanoscale, 2019, 11, 6217–6227 RSC.
- L. Zeng,
et al., Doxorubicin-loaded NaYF4:Yb/Tm-TiO2 inorganic photosensitizers for NIR-triggered photodynamic therapy and enhanced chemotherapy in drug-resistant breast cancers, Biomaterials, 2015, 57, 93–106 CrossRef CAS PubMed.
- W. J. Lokerse,
et al., In depth study on thermosensitive liposomes: Optimizing formulations for tumor specific therapy and in vitro to in vivo relations, Biomaterials, 2016, 82, 138–150 CrossRef CAS PubMed.
- Z. Jiang,
et al., Dual ATP and pH responsive ZIF-90 nanosystem with favorable biocompatibility and facile post-modification improves therapeutic outcomes of triple negative breast cancer
in vivo, Biomaterials, 2019, 197, 41–50 CrossRef CAS PubMed.
- J. Xi,
et al., Mn(2+)-coordinated PDA@DOX/PLGA nanoparticles as a smart theranostic agent for synergistic chemo-photothermal tumor therapy, Int. J. Nanomed., 2017, 12, 3331–3345 CrossRef CAS PubMed.
- E. L. Jin,
et al., Acid-Active Cell-Penetrating Peptides for in Vivo Tumor-Targeted Drug Delivery, J. Am. Chem. Soc., 2013, 135, 933–940 CrossRef CAS PubMed.
Footnotes |
† Electronic supplementary information (ESI) available: Standard curves of DOX, uptake of DOX and PDA@DOX, biocompatibility of PDA, photothermal temperature changes in tumor, the real photographs and H&E images, hematological analysis, pharmacokinetic of PDA@DOX and DOX. See DOI: 10.1039/c9nr05671h |
‡ These authors contributed equally to this work. |
|
This journal is © The Royal Society of Chemistry 2019 |
Click here to see how this site uses Cookies. View our privacy policy here.