DOI:
10.1039/D0CB00047G
(Review Article)
RSC Chem. Biol., 2020,
1, 86-97
Labelling of DNA and RNA in the cellular environment by means of bioorthogonal cycloaddition chemistry
Received
16th April 2020
, Accepted 27th May 2020
First published on 2nd June 2020
Abstract
Labelling of nucleic acids as biologically important cellular components is a crucial prerequisite for the visualization and understanding of biological processes. Efficient bioorthogonal chemistry and in particular cycloadditions fullfill the requirements for cellular applications. The broadly applied Cu(I)-catalyzed azide–alkyne cycloaddition (CuAAC), however, is limited to labellings in vitro and in fixed cells due to the cytotoxicity of copper salts. Currently, there are three types of copper-free cycloadditions used for nucleic acid labelling in the cellular environment: (i) the ring-strain promoted azide–alkyne cycloaddition (SPAAC), (ii) the “photoclick” 1,3-dipolar cycloadditions, and (iii) the Diels–Alder reactions with inverse electron demand (iEDDA). We review only those building blocks for chemical synthesis on solid phase of DNA and RNA and for enzymatic DNA and RNA preparation, which were applied for labelling of DNA and RNA in situ or in vivo, i.e. in the cellular environment, in fixed or in living cells, by the use of bioorthogonal cycloaddition chemistry. Additionally, we review the current status of orthogonal dual and triple labelling of DNA and RNA in vitro to demonstrate their potential for future applications in situ or in vivo.
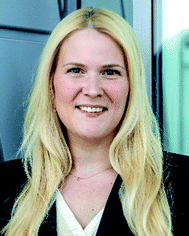
Dorothée Ganz
| Dorothée Ganz was trained as a medical-technical laboratory assistant, she studied Chemical Biology at the Karlsruhe Institute of Technology (KIT, Germany) and obtained the MSc degree in the research area of organ-on-a-chip models. Through her years of work in the microbiology department of the Municipal Clinic Karlsruhe, she also gained experience in the field of medical microbiology. For her doctoral research she joined the Institute of Organic Chemistry at KIT in 2019 and works under the supervision of Hans-Achim Wagenknecht. She follows her interests in chemical biology and works on metabolic labelling of nucleic acids by bioorthogonally reactive building blocks in order to investigate intracellular metabolic processes. |
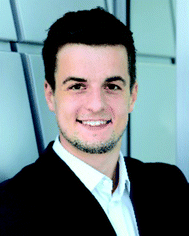
Dennis Harijan
| Dennis Harijan received his BSc degree in Chemical Biology at the Karlsruhe Institute of Technology (KIT, Germany) under the supervision of Anne S. Ulrich in 2016. Then he moved into the field of nucleic acid chemistry and investigated new building blocks for the bioorthogonal labelling of DNA and RNA. He obtained the MSc degree in 2018 and is currently working as a doctoral researcher under the supervision of Hans-Achim Wagenknecht and develops new cyclopropane- and cyclopropenone-modified nucleosides for the metabolic labelling of nucleic acids. |
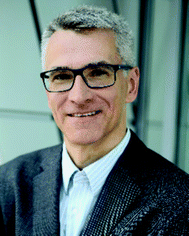
Hans-Achim Wagenknecht
| Hans-Achim Wagenknecht studied chemistry in Freiburg (Germany), obtained his diploma in 1995 (glycosidase inhibitors, Jochen Lehmann), got his doctoral degree in 1998 in bioorganic chemistry (porphyrine enzyme models, Wolf-D. Woggon) in Basel (Switzerland) and worked as postdoc with Jacqueline K. Barton at Caltech (USA). His independent career started in 2000 in Munich (Germany). In 2003, he obtained the habilitation (charge transfer in synthetically modified DNA) and in 2005 he got a professorship at Regensburg (Germany). Since 2010 he holds the chair for organic chemistry at the Karlsruhe Institute of Technology (KIT, Germany). His research is focused on bioorganic chemistry with nucleic acids and peptides, fluorescent imaging, DNA architectonics, photochemistry and chemical photocatalysis. |
Introduction
Labelling of cellular components is a crucial prerequisite for the visualization and understanding of biological processes.1,2 Among the different cellular components, proteins represent the main carrier of biological functions. Hence, it is not surprising that the chemical toolbox for protein labelling is well developed.3–7 Among the non-proteinaceous biomolecules, nucleic acids play a central role for cellular functions and regulations. DNA is the carrier of genetic information and is regulated by epigenetic modifications.8 RNA is central for transcription and translation of the genetic information into the protein function. Moreover, the function of non-coding RNAs, such as miRNA,9 lncRNA,10 and eRNA,11,12 is currently an increasingly important topic of research, and their imaging and detection inside cells are challenges for future research. It is of utmost importance to label and image those biomolecules “beyond the proteome”,13 inside their natural cellular environment.5,14–16 This requires the use of bioorthogonal chemistry17 which was developed mainly for proteins and carbohydrates,3–7,18 and has been very recently applied for nucleic acids, too. However, DNA and RNA chemistries are different from protein and carbohydrate chemistry, and bioorthogonal labelling chemistry cannot be simply transferred from proteins over carbohydrates to DNA and RNA.19,20
“Click” chemistry21 is considered to be biocompatible, if the reactions do not require toxic catalysts, organic solvents, high temperatures or high pressure.22 Cycloadditions in general, and azide–alkyne cycloadditions in particular, are intensively used for nucleic acid labelling.23–25 One major reason is the advantage that both functional groups, azides and alkynes, are bioorthogonal and react chemoselectively with each other.26 Since the early discovery by Huisgen,27 the azide–alkyne cycloaddition, however, can only be used for biolabelling in the presence of a Cu(I) catalyst that accelerates this reaction such that it efficiently works at biological temperature.28,29 Chelating Cu(I) ligands, like tris(benzyltriazolylmethyl)amine and water-soluble derivatives, suppress Cu(I)-induced oxidative DNA and RNA damages.30,31 The cytotoxicity of Cu(I) salts is, however, a principal problem for labellings in the cellular environment. This limits the applicability of this reaction to fixed cells (vide infra). There are rare examples of protein labellings in living cells;32–34 to the best of our knowledge, there is no published example of a Cu(I)-catalyzed azide–alkyne cycloaddition (CuAAC) for nucleic acid labelling in living cells. Among the copper-free click-type reactions, there are currently three main reactions used for nucleic acid labelling: (i) the ring-strain promoted azide–alkyne cycloadditions (SPAAC),35 (ii) the “photoclick” 1,3-dipolar cycloadditions (PC)36 and (iii) the Diels–Alder reactions with inverse electron demand (iEDDA).5–7 According to the literature, these three reaction types are considered to be bioorthogonal.37 The available building blocks for these reactions were summarized recently by us19,20 and others.38,39 Herein, we review the labelling of DNA and RNA in the cellular environment of fixed (in situ) or living cells (in vivo) by the use of bioorthogonal cycloaddition chemistry. Additionally, we review the current status of orthogonal dual and triple labelling of DNA and RNA in the test tube (in vitro) to demonstrate their potential for future applications in situ or in vivo.
Discussion
Labelling of presynthesized reactive oligonucleotides inside cells
Postsynthetic labellings of reactive oligonucleotides18,40 are performed inside cells to check if the applied bioconjugation chemistry is indeed bioorthogonal. Such reactive nucleic acids were prepared by two major strategies (Fig. 1). (i) DNA and RNA was synthesized on solid phase (SPS) using a synthesizer and phosphoramidites with reactive groups (RG) as building blocks.41,42 Using this method, the sequence and the number of incorporated building blocks with reactive groups was controlled by the sequential synthesis. The reactive groups must be stable during DNA or RNA synthesis which includes strong acids for detritylation, strong oxidizers to convert the P(III) building blocks into P(V) and strong bases during workup. Unfortunately, not all bioorthogonally reactive groups withstand these conditions. For instance, tetrazines are highly reactive groups for iEDDA reactions, but are not stable enough for DNA and RNA solid-phase chemistry.43 (ii) The enzymatic approach is a milder strategy to prepare DNA and RNA because it is performed in aqueous solution at neutral pH and without harsh chemicals.44 2′-Deoxyribonucleotide (dNTPs) and ribonucleoside triphosphates (NTPs) are the building blocks and have to be prepared as substrates for DNA and RNA polymerases.45 Primer extension (PEX) or other enzymatic oligonucleotide extensions (EX) yield shorter oligonucleotides,46 and PCR amplifies longer pieces of DNA. If a modified dN*TP (e.g. dA*TP) with a reactive group is mixed with the other three unmodified dNTPs (e.g. dGTP, dCTP and TTP) the reactive group is incorporated at every site where the corresponding dNTP is inserted by the enzyme. This multilabelling with reactive groups may be a disadvantage of this way of DNA and RNA preparation due to its inhibitory effect on polymerases and the lack of control on the locations.
 |
| Fig. 1 Incorporation of 2′-deoxyribonucleotides and ribonucleotides with reactive groups (RG) into DNA and RNA (marked in red) for intracellular transport and subsequent postsynthetic labelling with reactive counterparts (marked in blue) inside cells. | |
The SPAAC is an important alternative for the copper(I)-catalyzed reaction (k2 = 10–200 M−1 s−1),47 although the second rate order constants for conventional cyclooctynes are significantly lower (k2 = 0.0012 M−1 s−1).16,48 The reaction kinetics were improved by increased ring strain. One of the most reactive cyclooctynes is biarylazacyclooctyne (BARAC, k2 = 0.96 M−1 s−1),49 but it is also quite lipophilic.50 The carboxymethylmonobenzocyclooctyne (COMBO) is an alternative and very promising bioorthogonally reactive cyclooctyne that was invented by Kele et al. It is much smaller than the dibenzoannulated cyclooctynes, like BARAC, and thus less lipophilic, but shows similar reactivity (k2 = 0.8 M−1 s−1).51 We synthesized 2′-deoxyuridines as new DNA building blocks with the COMBO moiety attached to the 5-position using a rigid ethynyl linker52 or the flexible propyl linker.53 The DNA building block 1 (Fig. 2) was chemically incorporated into oligonucleotides using SPS. HeLa cells were transfected with the synthetic DNA modified with 1 and were treated by azide-modified dyes.53 The azide group quenches the fluorescence of the coumarin chromophore. The azide moiety gets converted into the triazoyl moiety by the SPAAC with the modified DNA inside the living HeLa cells, and thus the fluorescence of the dyes lights up. Confocal microscopy revealed specific DNA staining in the nuclei. In a different approach, Rentmeister et al. modified the 5′-cap of the eGFP mRNA in vitro with the azide–RNA building block 2 using the corresponding nucleoside triphosphate and the methyltransferase Ecm1.54 Mammalian cells were transfected with these azide-modified RNA and then reacted with BARAC-modified labels. After the in-cell SPAAC, the cells were fixed for better imaging. Alternatively, the poly(A) tail of mRNA could be labelled in a chemoenzymatic extension approach using the triphosphate of RNA building block 3 and yeast poly(A) polymerase.55 In contrast to the previous approach with the 5′-cap, the enzyme installs multiple building blocks 3 to the eGFP mRNA. Confocal imaging of HeLa cells revealed that such poly(A) tail-modified mRNA is active for translation. Taken together these tools for intracellular labelling of mRNA are extremely important for studying subcellular localization and dynamic processes of target mRNAs in the future.
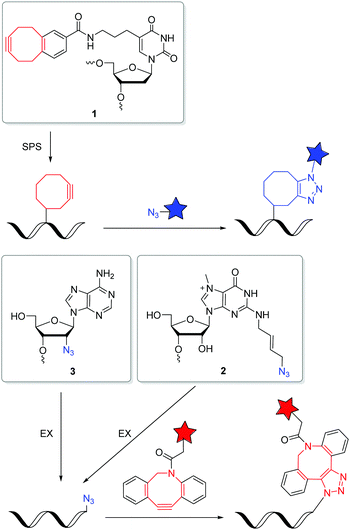 |
| Fig. 2 DNA and RNA building blocks 1–3 for labelling by SPAAC in the cellular environment. | |
The iEDDA reactions between 1,2,4,5-tetrazines and strained alkenes or alkynes are currently the fastest copper-free alternatives for bioorthogonal labelling.56–58 Another advantage of this type of reactions is that the first cycloaddition is followed by an irreversible nitrogen release in a retro-Diels–Alder reaction of the bicyclic intermediates to dihydropyridazines (with alkenes) or pyridazines (with alkynes). The iEDDA reactivity strongly depends on the substituents at the 1,2,4,5-tetrazines.59–62 The major problem for nucleic acid labelling by using 1,2,4,5-tetrazines as reactive groups, however, is the general instability towards the harsh and, in particular, basic conditions of the SPS,43 but also towards the enzymatic DNA preparation by PEX.20 There are only very few examples of 1,2,4,5-tetrazine-modified DNA and RNA in literature.20,43,63 To avoid this general problem, the alternative strategy is to incorporate the strained alkenes and alkynes into nucleic acids. In particular norbornenes,64 bicyclo[6.1.0]nonynes (BCN),65trans-cyclooctenes (TCO)66 and 1-methylcyclopropenes (MCp)67 were established as functional groups for the iEDDA labelling of biomolecules, at first in proteins and later also in nucleic acids. However, there are only a few examples in literature for this type of labelling with presynthesized DNA or RNA in the cellular environment. Kath-Schorr et al. prepared norbornene-modified RNA.68 The RNA building block 4 (Fig. 3) was incorporated at defined positions in predefined sequences by SPS. Mammalian cells (NCI-H460) were transfected with this presynthesized siRNA and treated with various 1,2,4,5-tetrazine-fluorophore conjugates. Confocal microscopy revealed that the ATTO647-labelled antisense strand of the siRNA duplex colocalizes with the iEDDA reaction product of the sense strand. We applied the DNA building block 5 with the small cyclopropene group attached to the 7-position of the 7-deaza-2′-deoxyadenosine core for primer extension experiments with standard DNA polymerases.69 This enzymatically prepared DNA strands were transfected into HeLa cells and reacted with a 1,2,4,5-tetrazine-TAMRA dye. Similar to the previous approach, the successful iEDDA reaction inside living cells was demonstrated by the colocalization of the TAMRA dye with the fluorescein label that was attached to the primer. These results demonstrate that strained alkenes represent an important chemical tool for the detection and investigation of RNA and DNA functions in cells, because they minimize pertubations of RNA functions by a large fluorophore and postpone the labelling to the final imaging of the living cells. Devaraj et al. used a template-dependent chemical ligation of fluorogenic probes for the detection of nucleic acids. Two different DNA pieces were presynthesized; the first piece of DNA was labelled by a quenched 1,2,4,5-tetrazine-fluorophore at the 5′-end and the second one by the MCp-modified building block 6 at the 3′-end. The ligation of the two modified oligonucleotides and the subsequent fluorogenic turn-on took place exclusively in the presence of the complementary template, compared to just minimal background reactions in the absence of the template. The enforced proximity of both reaction partners for the iEDDA reaction increases the effective molarity to a 50–120 mM range, depending on the linkers. This system was used for the detection of DNA in mammalian cells.70 In another approach, the dienophile was replaced by the 7-azabenzonorbornadiene building block 7 and the tetrazine by a fluorogenic tetrazine–BODIPY, which led to an increase in turnover amplification after template-dependent ligation. The modified probes were used for the detection of the miRNA template mir-21 inside human cancer cell lines.63
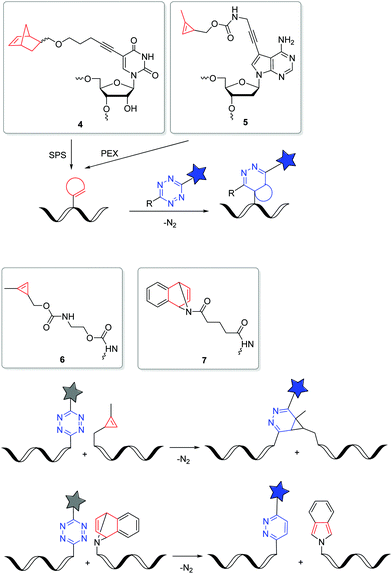 |
| Fig. 3 DNA and RNA building blocks 4–7 for labelling by iEDDA reactions in the cellular environment. | |
Metabolic labelling with reactive nucleosides and nucleotides
Metabolic labelling (ML) of nucleic acids focuses on the uptake of reactive nucleosides71,72 as well as nucleoside mono-,73 or triphosphates74 by living cells. After endogenous, enzymatic conversion into active nucleotides, they can be incorporated into nascent nucleic acids by DNA or RNA polymerases through successive rounds of replication or transcription, respectively. The provided label can postsynthetically be detected via a bioorthogonal reaction with a fluorescent reporter molecule (Fig. 4).
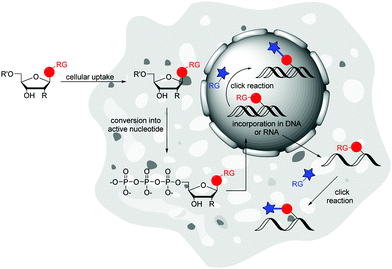 |
| Fig. 4 Principle of metabolic labelling (ML) of nucleic acids: cellular uptake of reactive nucleosides or nucleotides, which are endogenous converted into active nucleotides and thus can be incorporated into DNA or RNA. Detection is followed by “click” reaction with fluorescent reporter molecule. | |
The incorporation of modified nucleosides into DNA and RNA is restricted by the acceptance of the nucleoside kinases. These enzymes are highly selective for the natural substrates due to the specific structures of active centres and the binding contacts. This initial phosphorylation step to the nucleoside monophosphates yield the building blocks for DNA and RNA polymerases. There are some approaches to overcome this limitation. The crystal structure of the uridine–cytidine kinase revealed an open structure at the C5 position.72 Likewise, the adenosine kinase showed cavernous open spaces at the C2, N6 and N7 positions.72 A modification at one of these sites can increase the acceptance of the nucleoside.72,75 Luedtke et al. worked with genetically modified cells that expressed a kinase of the herpes simplex virus that has reduced selectivity.76 Another and less intrusive method is the use of so-called pronucleotides. These are nucleoside monophosphates in which the phosphate group is masked by two organic ester or amide groups to increase hydrophobicity and allows cell penetration. Once inside the cell, the chemical masks are cleaved by endogenous enzymes and the active nucleoside monophosphate is released.73,77 Common are the phosphoresters of 5-bispivaloyloxymethyl (POM) and 5-bis(4-acetoxybenzyl) (AB),73 the phosphoramidites that are used for the “ProTide” protection groups77 and cyclosal triesters.78 To the best of our knowledge, there have been no publications on the introduction of nucleoside diphosphates into living cells, probably due to the inherent instability of the protected phosphate groups.79 Also for nucleoside triphosphates there is a single publication by Zawada and Kraus, where a synthetic transporter complex is used for translocate nucleotides across the plasma membrane and release the active nucleotide into the cytoplasma.74
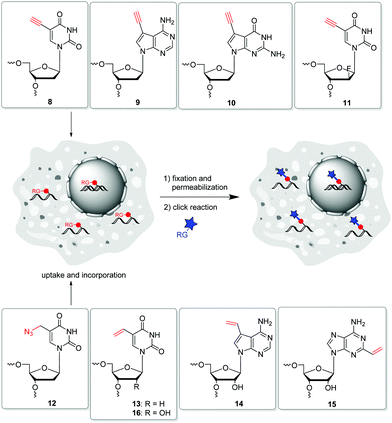 |
| Fig. 5 Metabolic labelling of DNA with the modified building blocks 8–13 and of RNA with 14–16. | |
It may be mentioned at this point that DNA and RNA polymerases also exhibit a certain substrate selectivity. This limitation is mainly explored for in vitro experiments to guide the incorporation of new nucleoside building blocks into DNA and RNA.80–82 Polymerase engineering yields advanced polymerases with improved functions by design, screening and targeted evolution. The intention is to improve their ability to incorporate, extend and replicate a wide variety of modified nucleotide analogues.83 Recently, Spitale's group published the metabolic incorporation of exogenous uracil and uracil analogues into cellular RNA after specifically identifying that the uridine mono-phosphate synthase (UMPS) pathway and uracil phosphoribosyltransferase (UPRT) pathway yields the respective nucleotides. This provided a more complex understanding of the metabolic pathways responsible for the incorporation of bioorthogonal reporter molecules.84
Subsequent to introducing the nucleoside into the cell and converting it to the active nucleotide, several strategies are now available to postsynthetically detect the labelled nucleic acids in the cellular environment. Due to the toxicity of some reactive nucleosides,76,85 the cytotoxic reaction conditions86 and the cell preparations for labelling,71 as well as the limited membrane permeability of fluorescent reporter molecules,87 postsynthetic labelling of nucleic acids was performed predominantly in fixed cells.
In 2008, Salic and Mitchison firstly published an in vivo strategy for the metabolic labelling of DNA using copper(I)-catalyzed azide–alkyne cycloaddition (CuAA). The incorporation of 5-ethynyl-2′-deoxyuridine 8 (Fig. 5) and its postsynthetic detection via reaction with fluorescent azides was a major breakthrough in the field of nascent DNA imaging, which was previously been done only by use of antibody staining.88,89 Despite the frequent use, 8 has been shown to be a highly toxic antimetabolite that can cause DNA strand damage leading to cell death.90 Hence, further terminal alkyne-modified nucleosides were introduced, which had less toxic properties.91 In particular, the purine derivatives 7-deaza-7-ethynyl-2′-deoxyadenosine 9 and 7-deaza-7-ethynyl-2′-deoxyguanosine 10
92 as well as the arabinosyl derivative 11 of 5-ethynyl-2′-deoxuridine published by Luedtke et al. showed lower toxicity in cell experiments.93 Thus, these analogues were better adapted for long-term in vivo incubation. As an azide modified nucleoside, 5-(azidomethyl)-2′-deoxyuridine 12 was shown to be a viable alternative for postsynthetic labelling by CuAAC.76
A copper-free alternative was recently published by Luedtke et al., which was also the first time ever that a SPAAC was applied for metabolic labelling of nucleic acids and as an in vivo experiment.94 Before this approach, SPAACs were restricted to in vitro experiments due to the limited water solubility and poor uptake of the reactants.48,95 Luedtke's three-component “double click” strategy used a cationic Sondheimer diyne derivative for noncovalent intercalation into double-stranded DNA.96 This creates a spatial proximity to the previously metabolically incorporated azide-modified nucleosides and yields efficient SPAACs. The resulting highly strained alkyne products then react in a second SPAAC with an azide, tagged to a fluorescent dye.
Another copper-free method introduced by Luedtke's group is based on iEDDA chemistry of 1,2,4,5-tetrazines and electron-rich dienophils.58 The reaction offers some major advantages: (i) it does not require a catalyst, (ii) it is irreversible due to the release of nitrogen, and (iii) it is compatible with cell media and therefore qualified for biological applications. 5-Vinyl-2′-deoxyuridine 13 was applied for metabolic labelling because it could successfully be incorporated into nascent DNA and detected by the reaction with a tetrazine-modified TAMRA dye. Based on this strategy, the incorporation of other vinyl-modified nucleosides, including 7-deaza-7-vinyl-adenosine 14 and 2-vinyl-adenosine 15 was also demonstrated for the metabolic labelling of RNA.72,97
However, only a few of the above described methods for metabolic labelling of nucleic acids have the potential for labelling and detection in living cells. Imaging in fixed cells only provides information by a snapshot of living cells and is additionally affected by artefacts often caused by the fixation process.98 In contrast, live cell imaging allows the monitoring of many dynamic intracellular processes in real time, which results in advanced kinetic and spatial information.99,100
The CuAAC is highly restricted for the use in living systems by the intrinsic cytotoxicity of copper, causing oxidative DNA damage resulting in cell death in a short time.86,101 Where copper is no longer a problem in the SPAAC reaction, the restriction here is the limited cell permeability of the reaction partners. Due to the very bulky modifications, the acceptance of the kinases is an additional impediment for this reaction type.102,103 These limitations do not apply for metabolic labelling by iEDDA reaction. The vinyl-modified nucleosides are small enough to be taken up by the cell. They are accepted by the kinases, incorporated into nascent nucleic acids and do not show any significant cytotoxic properties. The postsynthetic labelling reaction takes place under physiological conditions. The major limiting factor is the essential denaturation of the DNA with 2 M HCl to allow reaction with the fluorescent tetrazine–dye. This prevents the detection of native DNA in the well preserved cellular environment, as well as the application in living systems.71 With regard to these issues, the following approach is promising; not an iEDDA reaction, but a hetero-Diels–Alder reaction was used by Tang et al. for labelling DNA. They demonstrated the metabolic incorporation of vinyl thioether thymidine as dienophile to enable hetero-Diels–Alder cycloaddition with o-quinolinone quinone methide (oQQF) under physiological conditions. The major advantage of this concept is that there is no need to denature the cellular DNA by HCl. However, labelling with the fluorescent oQQF was only performed in fixed cells.104
Due to these non-biocompatible reaction conditions and preparations, the detection of labelled nucleic acids is still lacking usability in living systems. Another factor is the fluorescent reporter molecule which is used. While there is no problem with the availability of cell permeable “clickable” fluorescent dyes, which are most organic fluorophores that can be synthesised or purchased, it is more a problem to remove unbound dye from the inside of cells.105–107 While in fixed cells unbound dye can simply be washed out, this is a major challenge in living cells where it accumulates and causing fluorescent background signal. This problem may be solved by fluorogenic probes.108,109 In particular azides110 and tetrazines7,111 functions quench the fluorescence of dyes.
To the best of our knowledge, there are so far two publications reporting the detection of nucleic acids in living cells and even in living mice. (i) Last year, Xu et al. described the metabolic labelling of RNA with 5-vinyluridine 16 and the subsequent iEDDA reaction with a functionalized tetrazine linked to a biotin moiety. Detection is followed by fluorescent streptavidin-Alexa 488 reporter. Due to the high affinity of biotin and streptavidin, 16 can be linked to other molecules carrying streptavidin. This method may be paving the way to experiments that explore the dynamic interactions of RNA with other molecules.112 In a further step, 16 was used to visualize tumors in living mice. For this approach, a light-up strategy of the fluorescence reporter was used to eliminate the fluorescent background, since in living systems the unbound dye cannot be removed. The BODIPY-tetrazine derivative developed for this purpose is the profluorophore, which only develops a strong fluorescence signal through the iEDDA reaction with the vinyl function of the nucleoside.106 The injected modified nucleoside was able to accumulate in tumour tissue due to the increased metabolic activity of the tumour cells. The detection was subsequently performed with a light-up strategy using the fluorogenic BODIPY–tetrazine dye, which could be specifically detected in living mice. (ii) The second example applies the PC reaction which is the 1,3-dipolar cycloaddition between nitrile imines, that are generated by photolysis of tetrazoles, and olefines. This type of photoreaction was discovered by Huisgen et al.113 However, high temperatures or alternatively the use of light in the UV-B range do not meet the criteria of bioorthogonal labelling. The group of Lin et al. synthesized modified diaryltetrazoles for protein labelling which can be photolysed by light in the UV-A range.114 In principle, PC-type modifications of nucleic acids should now be applicable even to living cells. To the best of our knowledge, however, there is only one example published by Zhang et al. The group applied 5-vinyl-2′-deoxyuridine as building block 13 for metabolic labelling of DNA. Live cell labelling of DNA was reported to be efficient and fluorogenic with a water-soluble tetrazole and upon irradiation by 350 nm light.115
Orthogonal dual and triple labelling in vitro
The field of dual or even triple bioorthogonality is particularly challenging, but highly desired for investigations of structure changes and dynamics, in particular with FRET-based imaging probes. To achieve selective simultaneous labelling, a mutual orthogonality of the reactions must be given. Hildebrand et al. demonstrated for protein chemistry that TCO reacts with a 1,2,4,5-tetrazine in an iEDDA cycloaddition and an azide reacts with a dibenzocyclooctyne in a SPAAC and cross reactivity was excluded.116 iEDDA reactions117 with strained alkenes, in particular TCO and MCp, are orthogonal to SPAAC, but BCNs show cross reactivity.37,118 While some dual labelling schemes have been investigated and established for proteins119–122 and carbohydrates,123 also a few can be found for nucleic acids (Fig. 6 and 7).71,124–126 Luedtke et al. synthesized the 5-vinyl-2′-deoxyuridine building block 13 for metabolic labelling by iEDDA cycloaddition and the 5-ethynyl-2′-deoxyuridine building block 8 by CuAAC labelling and evidenced their chemical orthogonality on fixed HeLa cells using two different fluorescent dyes.71 Jäschke et al. introduced the more reactive dienophile modifications into DNA. The TCO-bearing phosphoramidites 17–19 were successfully attached to the 5′-end of DNA by SPS, and react with dansyl-modified 1,2,4,5-tetrazines.124 Together with the alkyne-bearing building block 20 and an azide as reactive partner for the CuAAC dual labelling of DNA was realized in a one-pot reaction. This reaction combination was extended in another approach for efficient triple labelling of DNA and RNA by using the SPAAC for an additional level of orthogonality.125 For the iEDDA cycloaddition the norbornene-modified building block 4 was attached at the 5′-end of the oligonucleotides. Applications with comparatively much more reactive TCO- and BCN-modifications showed high yields of by-product, which were probably caused by the usually disfavored reactions between azides and these dienophiles. For the CuAAC reaction, building blocks 20 and 21 were incorporated by SPS into DNA or RNA, respectively, while the 2′-azide-modified guanosine building block 22 was used for SPAAC. This approach could allow to study individual molecules by single molecule FRET (smFRET) and offer valuable insights into folding dynamics and presence of multiple folding states.125
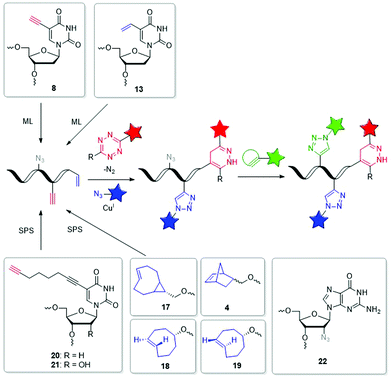 |
| Fig. 6 Building blocks 17–22 such as 4, 8 and 13 for orthogonal dual and triple labelling of DNA and RNA by CuAAC, iEDDA and SPAAC. | |
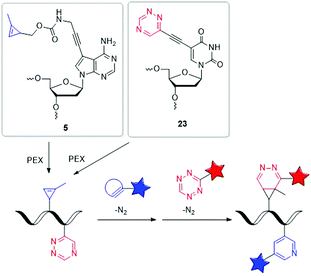 |
| Fig. 7 Building blocks 5 and 23 for orthogonal dual labelling of DNA by two different iEDDA reactions. | |
Our group managed to incorporate the MCp-bearing 7-deaza-2′-deoxyadenosine building block 5 and the 1,2,4-triazine-modified 2′-deoxyuridine building block 23 simultaneously into DNA strands by PEX.127 For the dual labelling, two different iEDDA reactions were used, which reacted in an orthogonal fashion with each other. As reactive partner for the 1,2,4-triazine group of 23 a BCN-modified dye and for the MCp group of 5 a 1,2,4,5-tetrazine-modified dye was chosen. Careful gel electrophoretic analysis revealed a dual and orthogonal labelling of the PEX DNA products. In the meantime, this mutual orthogonality of iEDDA reactions with 1,2,4-triazines and 1,2,4,5-tetrazines was also confirmed by Prescher et al.128 Although so far only performed in the test tube, such multiple position-selective postsynthetic modifications of nucleic acids offer a useful tool for bioorthogonal labelling and enables multi-component nucleic acid imaging in cells in the future.
Conclusions
Bioorthogonal cycloaddition chemistry requires reactive groups incorporated into DNA or RNA. This can be achieved either as presynthesized oligonucleotides with reactive groups by solid-phase chemistry, by polymerase-assisted preparation methods, such as primer extension (and subsequent intracellular transport), or by metabolic labelling of nucleosides with reactive groups. Recently, three main “click”-type reactions were explored in the cellular environment. The Cu(I)-catalyzed azide–alkyne cycloaddition (CuAAC) is limited to its use in fixed cells and has been extensively used in the context of metabolic labelling. The ring-strain promoted azide–alkyne cycloaddition (SPAAC) and the Diels–Alder reactions with inverse electron demand (iEDDA) are considered to be bioorthogonal and there are many available building blocks. Both reactions were explored as postsynthetic labelling methods in the cellular environment by presynthesized oligonucleotides or by metabolic incorporation into nascent DNA or RNA. The PC reaction is currently quite undeveloped with respect to potential for in vivo labelling. In particular, the spatiotemporal resolution that is associated in general with the use of light, could be a significant advantage for cellular applications in comparison to the more broadly applied SPAAC and iEDDA reactions. However, to ensure further promising and advanced approaches in particular for in vivo metabolic labelling of nucleic acids, the current limitations for bioorthogonal ligation have to be overcome, as they are not entirely suitable for living systems. Therefore, there is still a need to develop more flexible and biocompatible strategies to continuously expand the bioorthogonal toolbox for DNA and RNA labelling. Although multiple position-selective postsynthetic modification of nucleic acids can be performed in the test tube, it must be developed as a tool for multibioorthogonal labelling for nucleic acid imaging in cells in the future. Due to continuous research and the diversity of potential nucleoside analogues, the metabolic labelling of nucleic acids will offer important biological applications for imaging that have not yet been fully exploited.
Conflicts of interest
There are no conflicts to declare.
Acknowledgements
Financial support by the Deutsche Forschungsgemeinschaft (GRK 2039/2 and Wa 1386/15-2) and KIT is gratefully acknowledged.
Notes and references
- V. Ntziachristos, Fluorescence Molecular Imaging, Annu. Rev. Biomed. Eng., 2006, 8, 1–33 CrossRef CAS PubMed
.
- R. Weissleder and V. Ntziachristos, Shedding light onto live molecular targets, Nat. Med., 2003, 9, 123–128 CrossRef CAS PubMed
.
- Y. Tian and Q. Lin, Fitness Factors for Biooorthogonal Chemical Probes, ACS Chem. Biol., 2019, 14, 2489–2496 CrossRef CAS PubMed
.
- S. Mayer and K. Lang, Tetrazines in Inverse-Electron-Demand Diels-Alder Cycloadditions and Their Use in Biology, Synthesis, 2017, 830–848 CAS
.
- R. D. Row and J. A. Prescher, Constructing New Bioorthogonal Reagents and Reactions, Acc. Chem. Res., 2018, 51, 1073–1081 CrossRef CAS PubMed
.
- M. R. Karver, R. Weissleder and S. A. Hilderbrand, Bioorthogonal Reaction Pairs Enable Simultaneous, Selective, Multi-Target Imaging, Angew. Chem., Int. Ed., 2012, 51, 920–922 CrossRef CAS PubMed
.
- H. Wu and N. K. Devaraj, Advances in Tetrazine Bioorthogonal Chemistry Driven by the Synthesis of Novel Tetrazines and Dienophiles, Acc. Chem. Res., 2018, 51, 1249–1259 CrossRef CAS PubMed
.
- A. Hofer, Z. J. Liu and S. Balasubramanian, Detection, Structure and Function of Modified DNA Bases, J. Am. Chem. Soc., 2019, 141, 6420–6429 CrossRef CAS PubMed
.
- T. Tian, J. Wang and X. Zhou, A review: microRNA detection methods, Org. Biomol. Chem., 2015, 13, 2226–2238 RSC
.
- J. J. Quinn and H. Y. Chang, Unique features of long non-coding RNA biogenesis and function, Nat. Rev. Genet., 2016, 17, 47–62 CrossRef CAS PubMed
.
- Y. Murakawa, M. Yoshihara, H. Kawaji, M. Nishikawa, H. Zayed, H. Suzuki, F. Consortium and Y. Hayashizaki, Enhanced Identification of Transcriptional Enhances Provides Mechanistic Insights into Diseases, Trends Genet., 2016, 32, 76–88 CrossRef CAS PubMed
.
- M. T. Y. Lam, W. Li, M. G. Rosenfeld and C. K. Glass, Enhancer RNAs and regulated transcriptional programs, Trends Biochem. Sci., 2014, 39, 170–182 CrossRef CAS PubMed
.
- P. V. Chang and C. R. Bertozzi, Imaging beyond the proteome, Chem. Commun., 2012, 48, 8864–8879 RSC
.
- P. Shieh and C. R. Bertozzi, Design strategies for bioorthogonal smart probes, Org. Biomol. Chem., 2014, 12, 9307–9320 RSC
.
- K. Lang and J. W. Chin, Cellular Incorporation of Unnatural Amino Acids and Bioorthogonal Labeling of Proteins, Chem. Rev., 2014, 114, 4764–4806 CrossRef CAS PubMed
.
- C. P. Ramil and Q. Lin, Bioorthogonal chemistry: strategies and recent developments, Chem. Commun., 2013, 49, 11007–11022 RSC
.
- P. Shieh and C. R. Bertozzi, Design strategies for bioorthogonal smart probes, Org. Biomol. Chem., 2014, 12, 9307–9320 RSC
.
- I. Ivancová, D. Leone and M. Hocek, Reactive modifications of DNA nucleobases for labelling, bioconjugations and cross-linking, Curr. Opin. Chem. Biol., 2019, 52, 136–144 CrossRef PubMed
.
- K. Krell, D. Harijan, D. Ganz, L. Doll and H.-A. Wagenknecht, Postsynthetic Modifications of DNA and RNA by Means of Copper-Free Cycloadditions as Bioorthogonal Reactions, Bioconjugate Chem., 2020,(31), 990–1011 CrossRef CAS PubMed
.
- M. Merkel, S. Arndt, D. Ploschik, G. B. Cserép, U. Wenge, P. Kele and H.-A. Wagenknecht, Scope and Limitations of Typical Copper-Free Bioorthogonal Reactions with DNA: Reactive 2′-Deoxyuridine Triphosphates for Postsynthetic Labeling, J. Org. Chem., 2016, 81, 7527–7538 CrossRef CAS PubMed
.
- H. C. Kolb, M. G. Finn and K. B. Sharpless, Click Chemistry: Diverse Chemical Function From a Few Good Reactions, Angew. Chem., Int. Ed., 2001, 40, 2004–2021 CrossRef CAS PubMed
.
- C. J. Zeman, S. Kim, F. Zhang and K. S. Schanze, Direct Observation of the Reduction of Aryl Halides by a Photoexcited Perylene Diimide Radical Anion, J. Am. Chem. Soc., 2020, 142, 2204–2207 CrossRef CAS PubMed
.
- P. M. Gramlich, C. T. Wirges, A. Manetto and T. Carell, Postsynthetic DNA Modification through the Copper-Catalyzed Azide-Alkyne Cycloaddition Reaction, Angew. Chem., Int. Ed., 2008, 47, 8350–8358 CrossRef CAS PubMed
.
- A. H. El-Sagheer and T. Brown, Click chemistry with DNA, Chem. Soc. Rev., 2010, 39, 1388–1405 RSC
.
- E. Paredes and S. R. Das, Click Chemistry for Rapid Labeling and Ligation of RNA, ChemBioChem, 2011, 12, 125–131 CrossRef CAS PubMed
.
- M. F. Debets, C. W. J. V. D. Doelen, F. P. J. T. Rutjes and F. L. V. Delft, Azide: A Unique Dipole for Metal-Free Bioorthogonal Ligations, ChemBioChem, 2010, 11, 1168–1184 CrossRef CAS PubMed
.
- R. Huisgen, 1,3-Dipolar Cycloaddition. Past and Future, Angew. Chem., Int. Ed. Engl., 1962, 2, 565–598 CrossRef
.
- V. V. Rostovstev, L. G. Green, V. V. Fokin and K. B. Sharpless, A Stepwise Huisgen Cycloaddition Process: Copper(i)-Catalyzed Regioselective “Ligation” of Azides and Terminal Alkynes, Angew. Chem., Int. Ed., 2002, 41, 2596–2599 CrossRef
.
- C. W. Tornoe, C. Christensen and M. Meldal, Peptidetriazoles on Solid Phase: [1,2,3]-Triazoles by Regiospecific Copper(I)-Catalyzed 1,3-Dipolar Cycloadditions of Terminal Alkynes to Azides, J. Org. Chem., 2002, 67, 3057–3064 CrossRef CAS PubMed
.
- T. R. Chan, R. Hilgraf, K. B. Sharpless and V. V. Fokin, Polytriazoles as Copper(I)-Stabilizing Ligands in Catalysis, Org. Lett., 2004, 6, 2853–2855 CrossRef CAS PubMed
.
- C. Besanceney-Webler, H. Jiang, T. Zheng, L. Feng, D. S. D. Amo, W. Wang, L. M. Klivansky, F. L. Marlow, Y. Liu and P. Wu, Increasing the Efficacy of Bioorthogonal Click Reactions for Bioconjugation: A Comparative Study, Angew. Chem., Int. Ed., 2011, 50, 8051–8056 CrossRef CAS PubMed
.
- D. C. Kennedy, C. S. McKay, M. C. B. Legault, D. C. Danielson, J. A. Blake, A. F. Pegoraro, A. Stolow, Z. Mester and J. P. Pezacki, Cellular Consequences of Copper Complexes Used to Catalyze Bioorthogonal Click Reactions, J. Am. Chem. Soc., 2011, 133, 17993–18001 CrossRef CAS PubMed
.
- V. Hong, N. F. Steinmetz, M. Manchester and M. G. Finn, Labeling Live Cells by Copper-Catalyzed Alkyne-Azide Click Chemistry, Bioconjugate Chem., 2010, 21, 1912–1916 CrossRef CAS PubMed
.
- M. Yang, J. Li and P. R. Chen, Transition metal-mediated bioorthogonal protein chemistry in living cells, Chem. Soc. Rev., 2014, 43, 6511–6526 RSC
.
- J. J. Jewett and C. R. Bertozzi, Cu-free click cycloaddition reactions in chemical biology, Chem. Soc. Rev., 2010, 39, 1272–1279 RSC
.
- C. P. Ramil and Q. Lin, Photoclick chemistrry: a fluorogenic light-triggered in vivo ligation reaction, Curr. Opin. Chem. Biol., 2014, 21, 89–95 CrossRef CAS PubMed
.
- D. M. Patterson and J. A. Prescher, Orthogonal bioorthogonal chemistries, Curr. Opin. Chem. Biol., 2015, 28, 141–149 CrossRef CAS PubMed
.
- M. Hocek, Enzymatic Synthesis of Base-Functionalized Nucleic Acids for Sensing, Cross-linking, and Modulation of Protein-DNA Binding and Transcription, Acc. Chem. Res., 2019, 52, 1730–1737 CrossRef CAS PubMed
.
- S. Kath-Schorr, Cycloadditions for Studying Nucleic Acids, Top. Curr. Chem., 2016, 374, 4 CrossRef PubMed
.
- W. Schmucker and H.-A. Wagenknecht, Organic Chemistry of DNA Functionalization; Chromophores as DNA Base Substitutes versus DNA Base/2′-Modifications, Synlett, 2012, 2435–2448 CAS
.
- C. B. Reese, Oligo- and poly-nucleotides: 50 years of chemical synthesis, Org. Biomol. Chem., 2005, 3, 3851–3868 RSC
.
- S. L. Beaucage and M. H. Caruthers, Deoxynucleoside phosphoramidites—A new class of key intermediates for deoxypolynucleotide synthesis, Tetrahedron Lett., 1981, 22, 1859–1862 CrossRef CAS
.
- G. B. Cserép, O. Demeter, E. Bätzner, M. Kállay, H.-A. Wagenknecht and P. Kele, Sythesis and Evaluation of Nicotinic Acid Derived Tetrazines for Bioorthogonal Labeling, Synthesis, 2015, 2738–2744 Search PubMed
.
- M. Hocek, Enzymatic Synthesis of Base-Functionalized Nucleic Acids for Sensing, Cross-linking, and Modulation of Protein-DNA Binding and Transcription, Acc. Chem. Res., 2019, 52, 1730–1737 CrossRef CAS PubMed
.
- J. Singh, A. Ripp, T. M. Haas, D. Qiu, M. Keller, P. A. Wender, J. S. Siegel, K. K. Baldrigde and H. J. Jessen, Synthesis of Modified Nucleoside Oligophosphates Simplified: Fast, Pure, and Protecting Group Free, J. Am. Chem. Soc., 2019, 141, 15013–15017 CrossRef CAS PubMed
.
- A. Hottin, K. Betz, K. Diederichs and A. Marx, Structural Basis for the KlenTaq DNA Polymerase Catalysed Incorporation of Alkene- versus Alkyne-Modified Nucleotides, Chem. – Eur. J., 2017, 23, 2109–2118 CrossRef CAS PubMed
.
- K. Wang, A. Sachdeva, D. J. Cox, N. W. Wilf, K. Lang, S. Wallace, R. A. Mehl and J. W. Chin, Optimized orthogonal translation of unnatural amino acids enables spontaneous protein double-labelling and FRET, Nat. Chem., 2014, 6, 393–403 CrossRef CAS PubMed
.
- K. Lang and J. W. Chin, Bioorthogonal Reactions for Labeling Proteins, ACS Chem. Biol., 2014, 9, 16–20 CrossRef CAS PubMed
.
- J. C. Jewett, E. M. Sletten and C. R. Bertozzi, Rapid Cu-Free Click Chemistry with Readily Synthesized Biarylazacyclooctynes, J. Am. Chem. Soc., 2010, 132, 36878–36890 CrossRef PubMed
.
- M. F. Debets, J. C. M. v. Hest and F. P. J. T. Rutjes, Bioorthogonal labelling of biomolecules: new functional handles and ligation methods, Org. Biomol. Chem., 2013, 11, 6439–6455 RSC
.
- B. R. Varga, M. Kallay, K. Hegyi, S. Beni and P. Kele, A Non-Fluorinated Monobenzocyclooctyne for Rapid Copper-Free Click Reactions, Chem. – Eur. J., 2012, 18, 822–828 CrossRef CAS PubMed
.
- C. Stubinitzky, G. B. Cserép, E. Bätzner, P. Kele and H.-A. Wagenknecht, 2′-Deoxyuridine Conjugated with a Reactive Monobenzocyclooctyne as a DNA Building Block for Copper-Free Click-type Postsynthetic Modification of DNA, Chem. Commun., 2014, 50, 11218–11221 RSC
.
- Á. Eördögh, J. Steinmeyer, K. Peewasan, U. Schepers, H.-A. Wagenknecht and P. Kéle, Polarity Sensitive Bioorthogonally Applicable Far-Red Emitting Labels for Postsynthetic Nucleic Acid Labeling by Copper-Catalzed and Copper-Free Cycloaddition, Bioconjugate Chem., 2016, 27, 457–464 CrossRef PubMed
.
- J. M. Holstein, L. Anhäuser and A. Rentmeister, Modifying the 5′-Cap for Click Reactions of Eurkaryotic mRNA and To Tune Translation Efficiency in Living Cells, Angew. Chem., Int. Ed., 2016, 55, 10899–10903 CrossRef CAS PubMed
.
- L. Anthäuser, S. Hüwel, T. Zobel and A. Rentmeister, Multiple covalent fluorescence labeling of eukaryotic mRNA at the poly(A) tail enhances translation and can be performed in living cells, Nucleic Acids Res., 2019, 47, e42 CrossRef PubMed
.
- R. A. Carboni and R. V. Lindsey, Reactions of Tetrazines with Unsaturated Compounds. A New Synthesis of Pyridazines, J. Am. Chem. Soc., 1959, 81(16), 4342–4346 CrossRef CAS
.
- D. L. Boger and S. M. Sakya, Inverse electron demand Diels-Alder reactions of 3,6-bis(methylthio)-1,2,4,5-tetrazine. 1,2-Diazine introduction and direct implementation of a divergent 1,2,4,5-tetrazine.fwdarw. 1,2-Diazine.fwdarw. benzene (indoline/indole) Diels-Alder strategy, J. Org. Chem., 1988, 53(7), 1415–1423 CrossRef CAS
.
- D. R. Soenen, J. M. Zimpleman and D. L. Boger, Synthesis and Inverse Electron Demand Diels−Alder Reactions of 3,6-Bis(3,4-dimethoxybenzoyl)-1,2,4,5-tetrazine, J. Org. Chem., 2003, 68(9), 3593–3598 CrossRef CAS PubMed
.
- J. Balcar, G. Chrisam, F. X. Huber and J. Sauer, Reaktivität von stickstoff-heterocyclen genenüber cyclooctin als dienophil, Tetrahedron Lett., 1983, 24(14), 1481–1484 CrossRef CAS
.
- M. R. Karver, R. Weissleder and S. A. Hilderbrand, Synthesis and Evaluation of a Series of 1,2,4,5-Tetrazines for Bioorthogonal Conjugation, Bioconjugate Chem., 2011, 22(11), 2263–2270 CrossRef CAS PubMed
.
- D. L. Boger, R. P. Schaum and R. M. Garbaccio, Regioselective Inverse Electron Demand Diels−Alder Reactions of N-Acyl 6-Amino-3-(methylthio)-1,2,4,5-tetrazines, J. Org. Chem., 1998, 63(18), 6329–6337 CrossRef CAS PubMed
.
- A. Hamasaki, R. Ducray and D. L. Boger, Two Novel 1,2,4,5-Tetrazines that Participate in Inverse Electron Demand Diels−Alder Reactions with an Unexpected Regioselectivity, J. Org. Chem., 2006, 71(1), 185–193 CrossRef CAS PubMed
.
- H. Wu, B. T. Cisneros, C. M. Cole and N. K. Devaraj, Bioorthogonal Tetrazine-Modified Transfer Reactions Facilitate Reaction Turnover in Nucleic-Acid Templated Detection of MicroRNA, J. Am. Chem. Soc., 2014, 136, 17942–17945 CrossRef CAS PubMed
.
- A.-C. Knall, M. Hollauf and C. Slugovc, Kinetic studies of inverse electron demand Diels–Alder reactions (iEDDA) of norbornenes and 3,6-dipyridin-2-yl-1,2,4,5-tetrazine, Tetrahedron Lett., 2014, 55(34), 4763–4766 CrossRef CAS PubMed
.
- W. Chen, D. Wang, C. Dai, D. Hamelberg and B. Wang, Clicking 1,2,4,5-tetrazine and cyclooctynes with tunable reaction rates, Chem. Commun., 2012, 48(12), 1736–1738 RSC
.
- R. Rossin, P. Renart Verkerk, S. M. van den Bosch, R. C. M. Vulders, I. Verel, J. Lub and M. S. Robillard,
In Vivo Chemistry for Pretargeted Tumor Imaging in Live Mice, Angew. Chem., Int. Ed., 2010, 49(19), 3375–3378 CrossRef CAS PubMed
.
- D. N. Kamber, L. A. Nazarova, Y. Liang, S. A. Lopez, D. M. Patterson, H.-W. Shih, K. N. Houk and J. A. Prescher, Isomeric Cyclopropenes Exhibit Unique Bioorthogonal Reactivities, J. Am. Chem. Soc., 2013, 135(37), 13680–13683 CrossRef CAS PubMed
.
- A. M. Pyka, C. Domnick, F. Braun and S. Kath-Schorr, Diels-Alder Cycloadditions on Synthetic RNA in Mammalian Cells, Bioconjugate Chem., 2014, 25, 1438–1443 CrossRef CAS PubMed
.
- D. Ploschik, F. Rönicke, H. Beike, R. Strasser and H.-A. Wagenknecht, DNA Primer Extension with Cyclopropenylated 7-Deaza-2′-deoxyadenosine and Efficient Bioorthogonal Labeling in Vitro and in Living Cells, ChemBioChem, 2018, 19, 1949–1953 CrossRef CAS PubMed
.
- J. Šečkutė, J. Yang and N. K. Devaraj, Rapid oligonucleotide-templated fluorogenic tetrazine ligations, Nucleic Acids Res., 2013, 41(15), e148 CrossRef PubMed
.
- U. Rieder and N. W. Luedtke, Alkene–Tetrazine Ligation for Imaging Cellular DNA, Angew. Chem., Int. Ed., 2014, 53(35), 9168–9172 CrossRef CAS PubMed
.
- M. Kubota, S. Nainar, S. M. Parker, W. England, F. Furche and R. C. Spitale, Expanding the Scope of RNA Metabolic Labeling with Vinyl Nucleosides and Inverse Electron-Demand Diels-Alder Chemistry, ACS Chem. Biol., 2019, 14, 1698–1707 CrossRef CAS PubMed
.
- M. Tera, S. M. K. Glasauer and N. W. Luedtke, In Vivo Incorporation of Azide Groups into DNA by Using Membrane-Permeable Nucleotide Triesters, ChemBioChem, 2018, 19, 1939–1943 CrossRef CAS PubMed
.
- Z. Zawada, A. Tatar, P. Mocilac, M. Budesinsky and T. Kraus, Transport of Nucleoside Triphosphates into Cells by Artificial Molecular Transporters, Angew. Chem., Int. Ed., 2018, 57(31), 9891–9895 CrossRef CAS PubMed
.
- A. R. Van Rompay, A. Norda, K. Linden, M. Johansson and A. Karlsson, Phosphorylation of uridine and cytidine nucleoside analogs by two human uridine-cytidine kinases, Mol. Pharmacol., 2001, 59(5), 1181–1186 CrossRef CAS PubMed
.
- A. B. Neef and N. W. Luedtke, An Azide-Modified Nucleoside for Metabolic Labeling of DNA, ChemBioChem, 2014, 15, 789–793 CrossRef CAS PubMed
.
- C. McGuigan, R. N. Pathirana, N. Mahmood, K. G. Devine and A. J. Hay, Aryl phosphate derivatives of AZT retain activity against HIV1 in cell lines which are resistant to the action of AZT, Antiviral Res., 1992, 17(4), 311–321 CrossRef CAS PubMed
.
- N. Huynh, C. Dickson, D. Zencak, D. H. Hilko, A. Mackay-Sim and S. A. Poulsen, Labeling of Cellular DNA with a Cyclosal Phosphotriester Pronucleotide Analog of 5-ethynyl-2'-deoxyuridine, Chem. Biol. Drug Des., 2015, 86(4), 400–409 CrossRef CAS PubMed
.
- H. J. Jessen, T. Schulz, J. Balzarini and C. Meier, Bioreversible protection of nucleoside diphosphates, Angew. Chem., Int. Ed., 2008, 47(45), 8719–8722 CrossRef CAS PubMed
.
- K. Betz, F. Streckenbach, A. Schnur, T. Exner, W. Welte, K. Diederichs and A. Marx, Structures of DNA polymerases caught processing size-augmented nucleotide probes, Angew. Chem., Int. Ed., 2010, 49(30), 5181–5184 CrossRef CAS PubMed
.
- A. Hottin, K. Betz, K. Diederichs and A. Marx, Structural Basis for the KlenTaq DNA Polymerase Catalysed Incorporation of Alkene- versus Alkyne-Modified Nucleotides, Chem. – Eur. J., 2017, 23(9), 2109–2118 CrossRef CAS PubMed
.
- V. Raindlova, M. Janouskova, M. Slavickova, P. Perlikova, S. Bohacova, N. Milisavljevic, H. Sanderova, M. Benda, I. Barvik, L. Krasny and M. Hocek, Influence of major-groove chemical modifications of DNA on transcription by bacterial RNA polymerases, Nucleic Acids Res., 2016, 44(7), 3000–3012 CrossRef CAS PubMed
.
- D. Loakes and P. Holliger, Polymerase engineering: towards the encoded synthesis of unnatural biopolymers, Chem. Commun., 2009,(31), 4619–4631 RSC
.
- S. Nainar, B. J. Cuthbert, N. M. Lim, W. E. England, K. Ke, K. Sophal, R. Quechol, D. L. Mobley, C. W. Goulding and R. C. Spitale, An optimized chemical-genetic method for cell-specific metabolic labeling of RNA, Nat. Methods, 2020, 17(3), 311–318 CrossRef CAS PubMed
.
- F. Kohlmeier, A. Maya-Mendoza and D. A. Jackson, EdU induces DNA damage response and cell death in mESC in culture, Chromosome Res., 2013, 21(1), 87–100 CrossRef CAS PubMed
.
- D. C. Kennedy, C. S. McKay, M. C. B. Legault, D. C. Danielson, J. A. Blake, A. F. Pegoraro, A. Stolow, Z. Mester and J. P. Pezacki, Cellular Consequences of Copper Complexes Used to Catalyze Bioorthogonal Click Reactions, J. Am. Chem. Soc., 2011, 133, 17993–18001 CrossRef CAS PubMed
.
- C. W. Cunningham, A. Mukhopadhyay, G. H. Lushington, B. S. J. Blagg, T. E. Prisinzano and J. P. Krise, Uptake, distribution and diffusivity of reactive fluorophores in cells: implications toward target identification, Mol. Pharming, 2010, 7(4), 1301–1310 CrossRef CAS PubMed
.
- A. Salic and T. J. Mitchison, A chemical method for fast and sensitive detection of DNA synthesis in vivo, Proc. Natl. Acad. Sci. U. S. A., 2008, 105(7), 2415–2420 CrossRef CAS PubMed
.
- R. M. Hamelik and A. Krishan, Click-iT assay with improved DNA distribution histograms, Cytometry, Part A, 2009, 75(10), 862–865 CrossRef PubMed
.
- H. Zhao, H. D. Halicka, J. Li, E. Biela, K. Berniak, J. Dobrucki and Z. Darzynkiewicz, DNA damage signaling, impairment of cell cycle progression, and apoptosis triggered by 5-ethynyl-2'-deoxyuridine incorporated into DNA, Cytometry, Part A, 2013, 83(11), 979–988 CrossRef PubMed
.
- L. Guan, G. W. van der Heijden, A. Bortvin and M. M. Greenberg, Intracellular detection of cytosine incorporation in genomic DNA by using 5-ethynyl-2′-deoxycytidine, ChemBioChem, 2011, 12(14), 2184–2190 CrossRef CAS PubMed
.
- A. B. Neef, F. Samain and N. W. Luedtke, Metabolic labeling of DNA by purine analogues in vivo, ChemBioChem, 2012, 13(12), 1750–1753 CrossRef CAS PubMed
.
- A. B. Neef and N. W. Luedtke, Dynamic metabolic labeling of DNA in vivo with arabinosyl nucleosides, Proc. Natl. Acad. Sci. U. S. A., 2011, 108(51), 20404–20409 CrossRef CAS PubMed
.
- M. Tera and N. W. Luedtke, Three-Component Bioorthogonal Reactions on Cellular DNA and RNA, Bioconjugate Chem., 2019, 30, 2991–2997 CrossRef CAS PubMed
.
- R. Chadwick, S. Gyzen, S. Liogier and A. Adronov, Scalable Synthesis of Strained Cyclooctyne Derivatives, Synthesis, 2014, 669–677 Search PubMed
.
- M. Tera, Z. Harati Taji and N. W. Luedtke, Intercalation-enhanced “Click” Crosslinking of DNA, Angew. Chem., Int. Ed., 2018, 57(47), 15405–15409 CrossRef CAS PubMed
.
- J. T. George and S. G. Srivatsan, Vinyluridine as a Versatile Chemoselective Handle for the Post-transcriptional Chemical Functionalization of RNA, Bioconjugate Chem., 2017, 28(5), 1529–1536 CrossRef CAS PubMed
.
- J. W. Lichtman and J.-A. Conchello, Fluorescence microscopy, Nat. Methods, 2005, 2(12), 910–919 CrossRef CAS PubMed
.
- D. J. Stephens and V. J. Allan, Light microscopy techniques for live cell imaging, Science, 2003, 300(5616), 82–86 CrossRef CAS PubMed
.
- L. Parhamifar, L. Wu, H. Andersen and S. M. Moghimi, Live-cell fluorescent microscopy platforms for real-time monitoring of polyplex–cell interaction: Basic guidelines, Methods, 2014, 68(2), 300–307 CrossRef CAS PubMed
.
- G. R. Abel, Jr., Z. A. Calabrese, J. Ayco, J. E. Hein and T. Ye, Measuring and Suppressing the Oxidative Damage to DNA During Cu(I)-Catalyzed Azide-Alkyne Cycloaddition, Bioconjugate Chem., 2016, 27(3), 698–704 CrossRef PubMed
.
- N. J. Agard, J. A. Prescher and C. R. Bertozzi, A Strain-Promoted [3+2] Azide-Alkyne Cycloaddition for Covalent Modification of Biomolecules in Living Systems, J. Am. Chem. Soc., 2004, 126, 15046–15047 CrossRef CAS PubMed
.
- M. F. Debets, S. S. van Berkel, S. Schoffelen, F. P. Rutjes, J. C. van Hest and F. L. van Delft, Aza-dibenzocyclooctynes for fast and efficient enzyme PEGylation via copper-free (3+2) cycloaddition, Chem. Commun., 2010, 46(1), 97–99 RSC
.
- A. Gubu, L. Li, Y. Ning, X. Zhang, S. Lee, M. Feng, Q. Li, X. Lei, K. Jo and X. Tang, Bioorthogonal Metabolic DNA Labelling using Vinyl Thioether-Modified Thymidine and o-Quinolinone Quinone Methide, Chem. – Eur. J., 2018, 24(22), 5895–5900 CrossRef CAS PubMed
.
- F. Doll, A. Buntz, A.-K. Späte, V. D. Schart, A. Timper, W. Schrimpf, C. R. Hauck, A. Zumbusch and V. Wittmann, Visualization of Protein-Specific Glycosylation Inside Living Cells, Angew. Chem., Int. Ed., 2016, 55, 2262–2266 CrossRef CAS PubMed
.
- J. C. T. Carlson, L. G. Meimetis, S. A. Hilderbrand and R. Weissleder, BODIPY–Tetrazine Derivatives as Superbright Bioorthogonal Turn-on Probes, Angew. Chem., Int. Ed., 2013, 52(27), 6917–6920 CrossRef CAS PubMed
.
- K. E. Beatty, J. D. Fisk, B. P. Smart, Y. Y. Lu, J. Szychowski, M. J. Hangauer, J. M. Baskin, C. R. Bertozzi and D. A. Tirrell, Live-cell imaging of cellular proteins by a strain-promoted azide-alkyne cycloaddition, ChemBioChem, 2010, 11(15), 2092–2095 CrossRef CAS PubMed
.
- A. Nadler and C. Schultz, The Power of Fluorogenic Probes, Angew. Chem., Int. Ed., 2013, 52, 2408–2410 CrossRef CAS PubMed
.
- E. Kozma and P. Kele, Fluorogenic probes for super-resolution microscopy, Org. Biomol. Chem., 2019, 17, 215–233 RSC
.
- A. Herner, I. Nikic, M. Kallay, E. A. Lemke and P. Kele, A new family of bioorthogonally applicable fluorogenic labels, Org. Biomol. Chem., 2013, 11, 3297–3306 RSC
.
- A. Wieczorek, P. Werther, J. Euchner and R. Wombacher, Green- to far-red-emitting fluorogenic tetrazine probes – synthetic
access and no-wash protein imaging inside living cells, Chem. Sci., 2017, 8, 1506–1510 RSC
.
- H. S. Liu, T. Ishizuka, M. Kawaguchi, R. Nishii, H. Kataoka and Y. Xu, A Nucleoside Derivative 5-Vinyluridine (VrU) for Imaging RNA in Cells and Animals, Bioconjugate Chem., 2019, 30(11), 2958–2966 CrossRef CAS PubMed
.
- J. S. Clovis, A. Eckell, R. Huisgen and R. Sustmann, 1.3-Dipolare Cycloadditonen, XXV. Der Nachweis des freien Diphenylnitrilimins als Zwischenstufe bei Cycloadditionen, Chem. Ber., 1967, 100, 60–70 CrossRef CAS
.
- Y. Wang, W. J. Hu, W. Song, R. K. V. Lim and Q. Lin, Discovery of Long-Wavelength Photoactivatable Diaryltetrazoles for Bioorthogonal 1,3-Dipolar Cycloadditions, Org. Lett., 2008, 10, 3725–3728 CrossRef CAS PubMed
.
- Y. Wu, G. Guo, J. Zheng, D. Xing and T. Zhang, Fluorogenic “Photoclick” Labeling and Imaging of DNA with Coumarin-Fused Tetrazole in Vivo, ACS Sens., 2019, 4, 44–51 CrossRef CAS PubMed
.
- M. R. Karver, R. Weissleder and S. A. Hilderbrand, Bioorthogonal Reaction Pairs Enable Simultaneous, Selective, Multi-Target Imaging, Angew. Chem., Int. Ed., 2012, 51(4), 920–922 CrossRef CAS PubMed
.
- B. L. Oliveira, Z. Guo and G. J. L. Bernardes, Inverse electron demand Diels–Alder reactions in chemical biology, Chem. Soc. Rev., 2017, 46, 4895–4950 RSC
.
- A.-C. Knall and C. Slugovc, Inverse electron demand Diels-Alder (iEDDA)-initiated conjugation: a (high) potential click chemistry scheme, Chem. Soc. Rev., 2013, 42, 5131–5142 RSC
.
- T. Plass, S. Milles, C. Koehler, J. Szymański, R. Mueller, M. Wießler, C. Schultz and E. A. Lemke, Amino Acids for Diels–Alder Reactions in Living Cells, Angew. Chem., Int. Ed., 2012, 51(17), 4166–4170 CrossRef CAS PubMed
.
- D. M. Patterson, L. A. Nazarova, B. Xie, D. N. Kamber and J. A. Prescher, Functionalized Cyclopropenes As Bioorthogonal Chemical Reporters, J. Am. Chem. Soc., 2012, 134(45), 18638–18643 CrossRef CAS PubMed
.
- I. Nikić, T. Plass, O. Schraidt, J. Szymański, J. A. G. Briggs, C. Schultz and E. A. Lemke, Minimal Tags for Rapid Dual-Color Live-Cell Labeling and Super-Resolution Microscopy, Angew. Chem., Int. Ed., 2014, 53(8), 2245–2249 CrossRef PubMed
.
- A. Sachdeva, K. Wang, T. Elliott and J. W. Chin, Concerted, Rapid, Quantitative, and Site-Specific Dual Labeling of Proteins, J. Am. Chem. Soc., 2014, 136(22), 7785–7788 CrossRef CAS PubMed
.
- A. Niederwieser, A.-K. Späte, L. D. Nguyen, C. Jüngst, W. Reutter and V. Wittmann, Two-Color Glycan Labeling of Live Cells by a Combination of Diels–Alder and Click Chemistry, Angew. Chem., Int. Ed., 2013, 52(15), 4265–4268 CrossRef CAS PubMed
.
- J. Schoch, M. Staudt, A. Samanta, M. Wiessler and A. Jäschke, Site-Specific One-Pot Dual Labeling of DNA by Orthogonal Cycloaddition Chemistry, Bioconjugate Chem., 2012, 23(7), 1382–1386 CrossRef CAS PubMed
.
- M.-L. Winz, E. C. Linder, J. Becker and A. Jäschke, Site-specific one-pot triple click labeling for DNA and RNA, Chem. Commun., 2018, 54(83), 11781–11784 RSC
.
- P. V. Chang and C. R. Bertozzi, Imaging beyond the proteome, Chem. Commun., 2012, 48, 8864–8879 RSC
.
- U. Reisacher, D. Ploschik, F. Rönicke, G. B. Cserép, P. Kele and H.-A. Wagenknecht, Copper-free dual labeling of DNA by triazines and cyclopropenes as minimal orthogonal and bioorthogonal functions, Chem. Sci., 2019, 10(14), 4032–4037 RSC
.
- D. N. Kamber, Y. Liang, R. J. Blizzard, F. Liu, R. A. Mehl, K. N. Houk and J. A. Prescher, 1,2,4-Triazines Are Versatile Bioorthogonal Reagents, J. Am. Chem. Soc., 2015, 137(26), 8388–8391 CrossRef CAS PubMed
.
|
This journal is © The Royal Society of Chemistry 2020 |
Click here to see how this site uses Cookies. View our privacy policy here.