Photosynthetic response mechanisms in typical C3 and C4 plants upon La2O3 nanoparticle exposure†
Received
2nd September 2019
, Accepted 20th November 2019
First published on 21st November 2019
Abstract
Lanthanum oxide nanoparticles (La2O3 NPs) have raised enormous concerns due to the potential environmental risks they pose to agricultural production. Previous studies have demonstrated that the growth and photosynthesis of higher plants could be inhibited by La2O3 NPs. Due to the different photosynthetic pathways of C3 and C4 plants, their photosynthetic responses and defense mechanisms under NP exposure have not previously been investigated. To examine different regulatory strategies for photosynthesis in C3 and C4 plants, typical C3 (soybean) and C4 (maize) plants were selected in the present study, and their photosynthetic performance upon La2O3 NP exposure was compared. After 10 mg L−1 La2O3 NP exposure for 4 days, the net photosynthetic rate was reduced significantly by 8.77% and 55.52% in soybean and maize, respectively. Soybean's maximum quantum yield of PSII (Fv/Fm) was decreased by 10.53% and electron transport was blocked, resulting in low utilization efficiency of solar energy. Meanwhile for maize, the relative gene expressions for phosphoenolpyruvate carboxylase (PEPC) and ribulose-1,5-bisphosphate carboxylase/oxygenase (Rubisco) were downregulated, and the activity of PEPC and Rubisco were reduced by 19.08% and 7.16%, respectively. Thus, carbon fixation was restricted. Consequently, excess reactive oxygen species (ROS) were generated in the chloroplasts of maize. This is the first study focusing on differences in photosynthetic responses between C3 and C4 plants upon NP exposure, which provides useful knowledge for developing targeted regulation strategies to apply nano-agricultural technology in higher plants with different photosynthetic pathways.
Environmental significance
Lanthanum oxide nanoparticles (La2O3 NPs) have raised enormous concerns due to their potential environmental risks. Previous studies have shown that La2O3 NPs inhibit photosynthesis in higher plants. In order to develop targeted regulation strategies in applying nano-agricultural technology in higher plants, photosynthesis in typical C3 (soybean) and C4 (maize) plants upon La2O3 NP exposure was comprehensively compared in this study. The photosynthesis of maize seedlings was more vulnerable than soybean to La2O3 NP exposure, and the response mechanisms were species-dependent. Specifically, the inhibition of La2O3 NPs on soybean photosynthesis was mainly due to the low utilization efficiency of solar energy, while the reduction of photosynthesis on maize was mainly due to the restriction of carbon fixation.
|
1. Introduction
Rare earth oxide nanoparticles (REO NPs) have played an increasingly important role in high-technology industries on account of their magnetic, catalytic and optical properties.1 As a representative member of REO NPs, lanthanum oxide (La2O3) NPs have been widely used in various products and processes, such as automobile exhaust purification, agricultural films, optical fibers and electrode materials.2,3 Due to their extensive uses, La2O3 NPs are inevitably released into agricultural soils through vehicle exhausts, water irrigation, and land application of biosolids,4,5 which are anticipated to pose potential environmental risks. Until now, several studies regarding the inhibition of La2O3 NPs on plant growth have been carried out. Ma et al.6 reported that La2O3 NPs significantly reduced the root elongation of seven higher plants including radish, rape, tomato, lettuce, wheat, cabbage and cucumber. However, the negative impacts of La2O3 NPs differed in the different growth stages of the plants. The inhibition on wheat mainly occurred during the seed incubation stage, while it occurred during both seed soaking and incubation stages in lettuce and rape. The high dissolution of La2O3 NPs at the root surface induced by the root exudates and subsequent formation of LaPO4 in the intercellular spaces and middle lamellas of roots can reduce the phosphorus content in roots, which might be the reason for the phytotoxicity in cucumber.2,7 In addition, La2O3 NPs significantly decreased the biomass of both roots and shoots in lettuce and maize.3,8 In maize, La2O3 NPs downregulated the AQP genes and blocked water uptake.3 Moreover, in the research of Yue et al.,9 the growth inhibition of maize upon La2O3 NP exposure was not only found to be due to reduced water uptake but also the lower availability of CO2 for photosynthesis via stomatal closure.
Photosynthesis is a vital metabolic process that drives sugar production and ultimately crop yield. The elevation of photosynthetic efficiency is a promising approach for increasing crop yield.10 Therefore, it is necessary to study which processes limit photosynthesis in plants under NP exposure. Plants can be divided into C3 and C4 plants depending on their different photosynthetic pathways. Generally, C4 plants exhibit a 50% higher photosynthetic efficiency than most C3 plants.11 The maximum conversion efficiency of solar energy to plant biomass is 6% for C4 plants, while it is only 4.6% for C3 plants.12 The superior anatomical and biochemical properties of C4 plants result in a greater photosynthetic resilience as compared to C3 plants, especially under certain abiotic stresses, such as heat, drought and unusual CO2 concentration conditions.13–17 There are several studies investigating photosynthesis of C3 or C4 plants under NP exposure. For example, Ag NPs have been shown to inhibit ribulose-1,5-bisphosphate carboxylase/oxygenase (Rubisco) activity and photosynthesis in Spirodela polyrhiza, a C3 plant.18 TiO2 and CeO2 NPs have also been found to reduce the CO2 assimilation rate of Clarkia unguiculata (C3 plant) under high light and nutrient conditions.19 For sorghum, a C4 plant, CeO2 NPs have been shown to eliminate reactive oxygen species (ROS) and elevate photosynthetic efficiency under drought stress.20 However, until now, no research has compared photosynthetic differences between C3 and C4 plants under NP exposure. Photosynthesis occurs in both soybean and maize, but the photosynthetic pathways and initial carbon fixation enzymes are different, resulting in various degrees of carbon fixation. Hence, the differences between the possible photosynthetic mechanisms of C3 and C4 plants under NP exposure are proposed here.
In the present study, typical C3 (soybean) and C4 (maize) plants were chosen to explore discrepancies in photosynthesis between higher plants with different photosynthetic pathways in the presence of La2O3 NPs. The following three aspects were specifically examined: (1) the toxicity and internalization of La2O3 NPs in soybean and maize seedlings; (2) the discrepancies in photosynthetic efficiency of soybean and maize seedlings in response to La2O3 NPs; and (3) the comparative analysis of the toxicity mechanisms involved in the photosynthesis of soybean and maize seedlings induced by La2O3 NPs. This is the first instance of a direct comparison of photosynthetic changes in typical C3 and C4 plants upon exposure to La2O3 NPs. This study could help in the development of targeted regulation strategies for applying nanotechnology in sustainable agricultural practices.
2. Materials and methods
2.1. La2O3 NPs preparation and characterization
La2O3 NPs (99.99%, 10–100 nm) were purchased from US Research Nanomaterials (Houston, TX, USA). The NP suspensions were prepared by adding La2O3 NP powder into deionized water and subsequently sonicating the mixture for 30 min to increase particle dispersion. The morphology of the La2O3 NPs was observed by transmission electron microscopy (TEM, JEM-2100, Japan) operated at 200 kV. The TEM image indicates that the sizes of the rod-shaped La2O3 NPs range from 30 nm to 150 nm (Fig. S1†). The particle sizes (424.68 ± 64.47 nm) and ζ-potential (13.90 ± 2.16 mV) of La2O3 NP suspensions were measured with a Malvern Nano-ZS analyzer (Malvern Instrument Inc., UK).
2.2. Plant cultivation and La2O3 NP exposure
Soybean (Glycine max (L.) Merr.) and maize (Zea mays L.) seeds were purchased from Qingdao seed station (Qingdao, China). Seeds were sterilized with 10% H2O2 solution for 10 min and then rinsed with deionized water repeatedly.21 The sterile seeds were incubated in the dark for 3 days at 25 °C with an average humidity of 60%. After that, uniform seedlings were transplanted into ceramic pots. Each ceramic pot contained 1/4 strength of the nutrient solution.21 After the third leaf appeared in the maize plants and the first pair of euphylla expanded completely in soybean (the time when photosynthesis of both plants began), the plants were transplanted into deionized water containing La2O3 NPs. In our previous studies, 50 mg L−1 NPs inhibited plant growth,3,22 while other studies also indicated that NPs (>50 mg L−1) significantly inhibited the physiological and biochemical processes of plants.23–25 Thus, 0, 5, 10 and 50 mg L−1 were selected as the exposure concentrations. Deionized water with a lower ionic strength was used to minimize NP aggregation and highlight the effects of the NPs.21 In our previous study,3 0.50 mg L−1 La3+ was released from 50 mg L−1 La2O3 NPs in deionized water in the presence of plants after exposure for 48 h, and the ionic control indicated that less than 1 mg L−1 of La3+ had no significant impact on maize growth. Based on this, the NP suspension was changed every 48 hours in order to exclude the contribution of La3+ to the phytotoxicity. Plants were harvested as a function of the exposure time (0–6 days) and La2O3 NP concentration (0, 5, 10 and 50 mg L−1). After the harvest, the seedling roots were washed using 20 mM EDTA-Na2 solution (five times) followed by five rinses with deionized water to avoid possible attachment of La2O3 NPs.22 The morphological parameters of the roots were analyzed by the WinRHIZO Pro 2017 b (Regent Instruments Inc., Canada). The dry biomass was measured after oven-drying at 70 °C for 48 h. The La content in the shoots and roots of both plants was detected according to the methods outlined in previous research with some slight modifications.26 Specifically, the dry samples were cut into pieces, and were digested with a mixture of HNO3 and HCl on a heating plate (120 °C) for 20 min. After cooling, the samples were digested completely using a microwave digestion system (MARS 6, CEM, USA). The total La content in the digested solution was determined using triple quadrupole inductively coupled plasma mass spectrometry (ICP-MS) (iCAP-TQ, Thermo Fisher, Germany). The uptake efficiency was calculated using the equation, uptake efficiency = La content/root surface area, which represents NP uptake by the roots per unit area.
2.3. Determination of photosynthetic parameters
The relative chlorophyll content (SPAD) was measured using a Chlorophyll Meter (SPAD-502 plus, Konica Minolta Inc., Japan) in soybean and maize leaves exposed to 10 mg L−1 La2O3 NPs for 4 days. The second leaf from the top of each plant was chosen. Each chosen leaf was measured three times and the average of the three readouts was recorded. The gas exchange measurements, including net photosynthetic rate (Pn), stomatal conductance (Gs), transpiration rate (E) and intracellular CO2 concentrations (Ci), were obtained using the CIRAS-3 portable gas exchange system (CIRAS-3, PP-Systems, USA). At the time of measurement, the ambient CO2 concentration was kept constant at 400 μmol mol−1, while the relative humidity was 60%, maximum photosynthetic photon flux density (PPFD) was 500 μmol m−2 s−1 and leaf temperature was 25 °C.
Chlorophyll a fluorescence transient was simultaneously measured using a plant efficiency analyzer (Pocket PEA, Hansatech Instruments Ltd., UK). The parameters obtained from dark-adapted leaves at room temperature were analyzed using the JIP test. The JIP test reflects the changes in light absorption, energy transformation and PSII photoactivity.27 The fluorescence intensity rose from an initial minimum fluorescence intensity Fo (O step) to a maximal fluorescence intensity Fm (P step) during 1 s of illumination with saturating red light. The maximum quantum yield of PSII (Fv/Fm) was calculated using the equation: Fv/Fm = 1 − (Fo/Fm).27 Two intermediate steps (J step and I step) appeared at 2 and 30 ms between the above-mentioned two extrema fluorescence parameters, respectively. To obtain an OJIP curve, the original fluorescence transient was standardized between O and P steps. The Wk curve was normalized by chlorophyll a fluorescence transient from Fo to F300μs. The W step was then calculated using the equation: Wt = (Ft − Fo)/(F300μs − Fo).27 The derived fluorescence parameters were calculated using the OJIP curve, and these parameters are listed in Table S1.†28
2.4. Activity of the key enzymes
The activity of phosphoenolpyruvate carboxylase (PEPC) and Rubisco were spectrophotometrically determined according to methods used in previous studies with slight modifications.18,29 Briefly, the leaves were wiped and cut off, and then ground into fine powders in liquid nitrogen using a mortar and pestle. The powders were quickly homogenized in a pre-cooling buffer solution at pH 8.0 that contained 50 mM Tris, 0.1 mM EDTA, 15 mM MgCl2 and 10% glycerol. The extracts were centrifuged at 12
000g for 15 min. Afterwards, the supernatants were used to measure the activity of Rubisco and PEPC. A multifunctional microplate reader (Varioskan Lux, Thermo Scientific, Finland) was applied to monitor the absorbance change at 340 nm. The activity values of Rubisco and PEPC were normalized using protein content.
2.5. Quantitative real-time (qRT)-PCR analysis
After 10 mg L−1 La2O3 NP exposure for 4 days, the leaves of both soybean and maize were separately harvested. The excised leaves were immediately frozen in liquid nitrogen and stored at −80 °C until further analysis. The total RNA was extracted and reverse-transcribed to cDNA using a MiniBEST Plant RNA Extraction Kit (Takara, Japan) and PrimeScript™ RT Master Mix (Takara, Japan) following the manufacturer's instructions. For each qRT-PCR reaction, a final volume of 25 μL was used with 12.5 μL of 2× TB Green Premix Ex TaqII (Takara, Japan), 1 μL forward and 1 μL reverse primer, and 1 μL cDNA sample and 8.5 μL RNase free H2O. The specific primer sequences are listed in Table S2.†30 Analysis was then performed on the CFX 96 touch Real Time PCR System (BioRad, USA). The qRT-PCR protocol was 95 °C for 30 s, 40 cycles of 95 °C for 5 s and 60 °C for 30 s, and 95 °C for 10 s, and a melt curve was held in 0.5 °C increments from 60 °C to 95 °C. Sequentially, the relative gene expression was calculated using the 2−ΔΔCT method.31
2.6. ROS accumulation in chloroplasts
For ROS in vivo detection, the leaves were collected and wiped from each treatment. Leaf discs (∼5 mm diameter) were obtained from leaves by using a puncher. These were transferred into 25 μM 2′,7′-dichlorodihydrofluorescein diacetate (H2DCFDA, Sigma Aldrich) dye, and incubated for 30 min in the dark.32 The dye was dissolved in dimethylsulfoxide (DMSO). Leaf discs were placed between a glass slide and a coverslip, and the edges were sealed with nail polish to ensure that no air bubbles remained. The samples were then imaged using a confocal microscope (Nikon A1+ Confocal Superresolution Imaging System, Japan). Confocal imaging settings were as follows: 20× wet objective; 488 nm laser excitation; PMT: 500–600 nm. The fluorescence signals from the ROS dyes were collected and measured by NIS-Elements AR.
2.7. Statistical analysis
Statistical analysis of the growth curve data was performed using SPSS version 25.0 (IBM, Armonk, NY, USA) by a one-way ANOVA with Tukey HSD post hoc test after the verification of normality and homoscedasticity assumption. Statistical analysis of the other data was carried out using SPSS by an independent-sample t-test. All the experiments were carried out at least in triplicate, and a significant difference was considered at p < 0.05.
3. Results and discussion
3.1. La internalization in plant tissues
Previous studies suggested that NPs can pass through the root epidermis to accumulate in the roots, and then translocate to the shoots through the xylem.33 In the present study, La was detected in the roots and shoots of both soybean and maize seedlings after 10 mg L−1 La2O3 NP exposure for 4 days, respectively (Fig. 1A). The La content in the whole soybean seedlings was twice as much as it was in maize. A possible reason for this is that soybean has larger leaf surface areas which could result in a higher transpiration rate. The transpiration rate of soybean was 10.75 mmol H2O m−2 s−1, while it was 2.05 mmol H2O m−2 s−1 for maize (Fig. 2D). The driving force for long distance translocation of water and nutrients mainly depends on the transpiration rate. Thus, soybean may have a greater ability to take up NPs than maize. The La content in roots was remarkably higher than that in shoots of both plants (Fig. 1A). This showed that La2O3 NPs were probably transported from roots to shoots,21 and fewer La2O3 NPs were possibly translocated from roots to shoots. The low translocation might be due to the fact that positively charged La2O3 NPs are easily absorbed in large quantities by negatively charged xylem.34 In addition, the La content in soybean roots was significantly higher than that in maize roots; however, the La content in soybean shoots was significantly lower than that in maize shoots (Fig. 1A). Also, the uptake efficiency of soybean roots, which indicates the efficiency of the root system to take up NPs, was much higher than that of maize roots (Fig. 1B). The xylem wall in soybean normally has more negative charge (e.g. carboxyl groups) and could be able to adsorb more positively charged particles than that in maize.34 The positively charged La2O3 NPs used in this study were more readily adsorbed on the xylem wall and were more difficult to transport to the shoots in soybean compared to maize.35 After entering the plant tissues, La might further affect plant growth.7
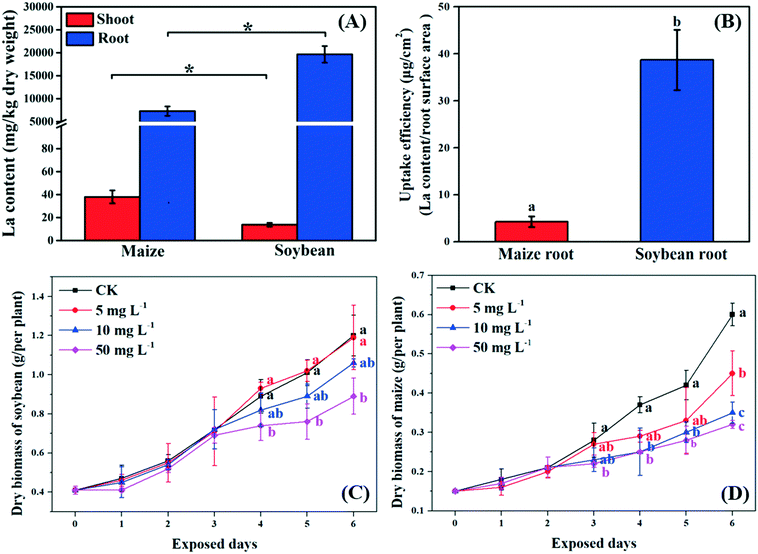 |
| Fig. 1 La content in maize and soybean tissues (A) and uptake efficiency of their roots (B). The growth curves of soybean (C) and maize (D) after exposure to 10 mg L−1 La2O3 NPs for 4 days. Values represent mean ± SD (n = 6). Different letters indicate significant differences between the control and the treatment (p < 0.05). The letters are only shown when differences among means are statistically significant. The significant differences between maize and soybean are marked with “*” (p < 0.05). | |
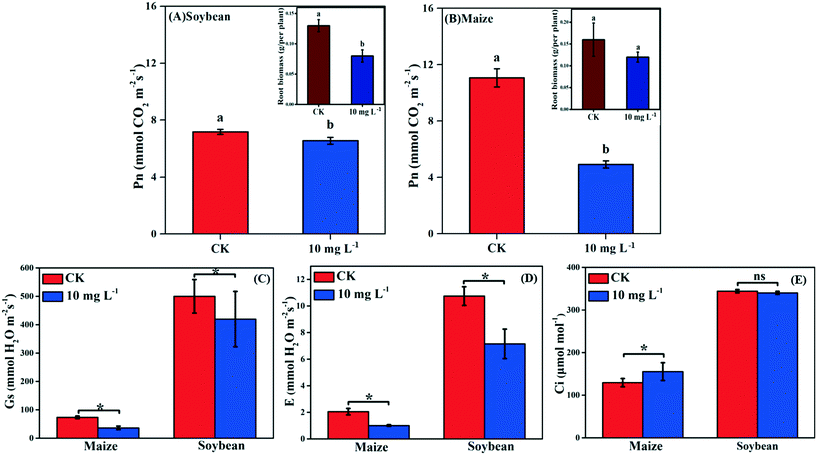 |
| Fig. 2 The gas exchange parameters: net photosynthetic rate (Pn) of soybean (A) and maize (B); stomatal conductance (Gs) (C); transpiration rate (E) (D); and intracellular CO2 concentrations (Ci) (E) of soybean and maize subjected to 10 mg L−1 La2O3 NPs for 4 days. Data marked with * (p < 0.05) exhibit significant differences with the control. Data with different letters are significantly different (p < 0.05). | |
3.2. Growth of C3 and C4 plants after exposure to La2O3 NPs
As shown in Fig. 1C and D, the biomass of soybean and maize seedlings exposed to La2O3 NPs was dose- and time-dependent and the impacts differed between the two types of plants. After exposure for 1 and 2 days, the biomass of both plants showed no significant change. After 3 days, only the biomass of maize exposed to 50 mg L−1 La2O3 NPs was decreased by 21.43%. After 4 days, the biomass of maize was reduced significantly by 32.43% and 32.43% at 10 mg L−1 and 50 mg L−1, respectively, while the biomass of soybean was reduced significantly at 50 mg L−1 by 16.85%. Although the difference was not significant in soybean with 10 mg L−1 La2O3 NP exposure for 4 days, the biomass started to exhibit a downward trend. This shows that maize seedlings are more sensitive than soybean seedlings to La2O3 NPs exposure. Additionally, the biomass of both soybean and maize seedlings started to decrease significantly after 10 mg L−1 La2O3 NP exposure for 4 days (Fig. 1A and B). Thus, 10 mg L−1 La2O3 NPs was the lowest observed concentration that exhibited inhibitory impacts on plant growth. 10 mg L−1 and 4 days were chosen as the exposure concentration and time to further investigate the physiological characteristics and photosynthetic mechanisms of plants upon La2O3 NP exposure.
As shown in Fig. S2,† roots were more sensitive to La2O3 NPs than shoots in both plants, which is similar to the findings of our previous study.21 The possible reason is that NPs come in direct contact with roots during exposure. The root biomass may also be reduced by the weakened photosynthesis, owing to the fact that root growth is supported by photosynthates, and more than 75% of photosynthates are translocated to the root system for optimal growth of roots.36 As shown in the small panels of Fig. 2A and B, the root biomass of both the soybean and maize seedings was significantly decreased, likely due to the inhibition of photosynthesis. Moreover, less photosynthates were translocated to the roots of plants with small and weak roots (NP-exposed), in comparison with those with well-developed roots (un-exposed control) (Fig. S3 and S4†).36 Except for the significant reduction of the root biomass, the root morphology was noticeably changed. As shown in Fig. S3 and S4,† all the root morphological parameters, including root length, surface area, volume and root tips, were reduced significantly as compared to the control.
3.3. Photosynthesis of C3 and C4 plants after La2O3 NP exposure
Photosynthesis is an essential metabolic process; it is the product of photosynthesis that provides necessary nutrients for plant growth and development. As shown in Fig. 2A and B, the Pn of soybean and maize seedlings was decreased by 8.77% and 55.52% in response to La2O3 NPs, respectively. The photosynthesis of maize (C4) was decreased to a greater extent than that of soybean (C3). However, under abiotic stresses such as climate variation, C4 plants have an absolute advantage over C3 plants in photosynthesis.13–16 This might be because the defense mechanisms of C3 plants and C4 plants against xenobiotics differ in the case of climate variation. Under harsh climate conditions, the photosynthesis in C3 plants is mainly reduced by stomatal limitation, which might interfere with CO2 diffusion pathways, while in C4 plants the “CO2 concentrating mechanism” has the capability to maintain photosynthesis even at relatively low CO2 concentrations.37 When Pn decreases with Ci reduction, the main limiting factor of photosynthesis is stomatal limitation. However, when Pn decreases with the increase of Ci, the limiting factor of photosynthesis is non-stomatal limitation.38 In the present study, after La2O3 NP exposure, although obvious decreases were observed for Gs and E in both plants (Fig. 2C and D), Ci did not change in soybean seedlings and even increased in maize seedlings (Fig. 2E). This indicates that CO2 diffusion pathways were not impeded in both plants, and that the non-stomatal limitation played a dominant role in the decreasing photosynthesis. To better understand the inhibition mechanism of photosynthesis between C3 and C4 plants in response to La2O3 NPs, light reaction and carbon fixation were investigated, as discussed below.
3.4. Effect of La2O3 NPs on light reaction of C3 and C4 plants
As the first stage of photosynthesis, the light reaction starts with the absorption of light energy by chlorophyll, and then goes through water-splitting and electron transport, finally providing ATP and NADPH for the dark reaction. Fig. 3A shows that there was no significant difference in the relative chlorophyll content between the control and treatment groups in both soybean and maize seedlings, indicating that the absorption efficiency of light energy was not affected by La2O3 NPs. Nevertheless, light energy absorbed by chlorophyll could not be fully utilized by the primary photochemical reactions and was partially consumed in the form of fluorescence and thermal energy dissipation.39,40 Herein, to better understand the role of La2O3 NPs in the light reaction of photosynthesis, chlorophyll a fluorescence transient was studied. Chlorophyll a fluorescence transient is regarded as a rapid and noninvasive technique for detecting alterations of photosynthetic capacity.39,40 The Fv/Fm ratio is the most common indicator, which reflects the maximum light energy conversion efficiency of PSII and the potential photosynthetic capacity of the plant.40 As shown in Fig. 3B, Fv/Fm in the soybean seedlings with La2O3 NP treatment was significantly decreased in comparison with the control, while no obvious change was observed in maize seedlings. Therefore, the PSII in soybean may be more vulnerable to La2O3 NPs than that in maize. Similarly, 10 mg L−1 Cr2O3 NPs decreased the Fv/Fm ratio in soybean, probably because the thylakoid membrane was broken and the ultrastructure of chloroplasts was destroyed.23
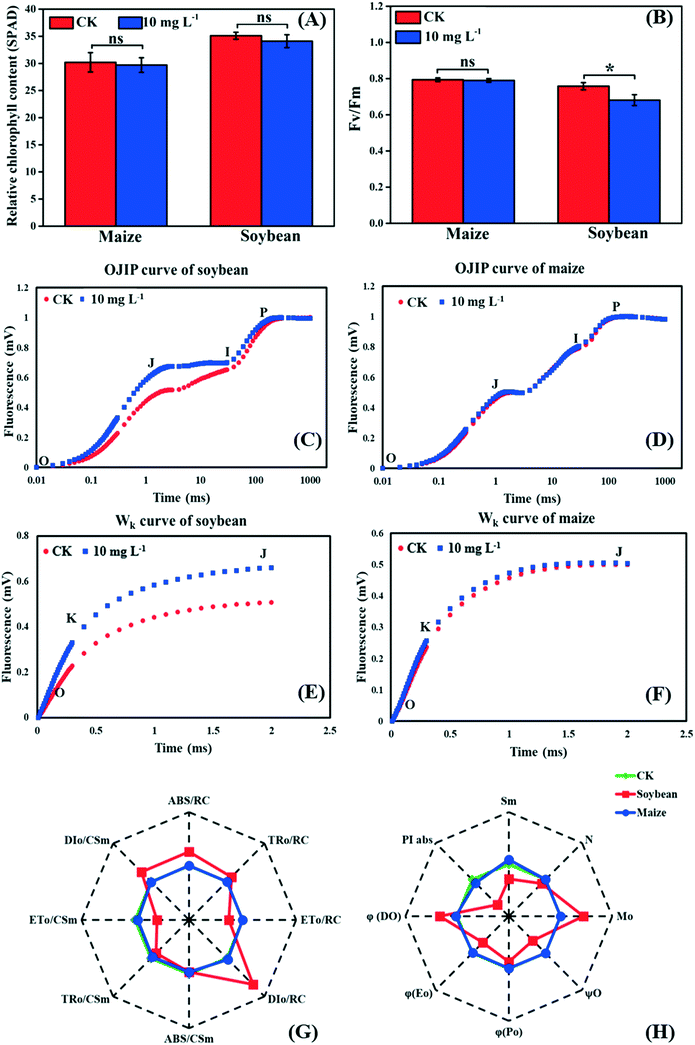 |
| Fig. 3 Effects of La2O3 NPs on light reaction of soybean and maize. (A) Relative chlorophyll content. (B) The maximum quantum yield of PSII (Fv/Fm). (C and D) The OJIP curve of soybean and maize leaves. (E and F) Wk curve of soybean and maize leaves (O step at about 20 μm; K step at about 300 μm; J step at about 2 ms; I step at about 30 ms; P step indicates the maximum fluorescence). (G and H) Spider-plot presentation of the chlorophyll fluorescence parameters in soybean and maize. The description of these parameters is listed in table S1.† Data marked with * (p < 0.05) exhibit significant differences with the control. | |
The JIP test also reflects the function of photosynthetic apparatus and the PSII primary photochemical reaction, and can be carried out by chlorophyll a fluorescence transient.41 To further investigate the inhibition sites of La2O3 NPs on PSII, the PSII electron donor side, the reaction centers (RCs), and the electron acceptor side were analyzed using the JIP test. According to the OJIP curves (Fig. 3D), we observed a distinct increase of the J step in soybean seedlings exposed to La2O3 NPs in comparison to the control, indicating that the electron transport from QA to QB was limited. The rise of the J step might be due to D1 protein degradation and/or inactivation because QB is bound to the D1 protein.42 At the same time, the J step did not change in maize seedlings (Fig. 3F). This result manifested in La2O3 NPs restricting electron transport from QA to QB in soybean, while this had no effect in maize. PSII electron acceptor side includes QA, QB and plastoquinone (PQ). The fluorescence parameters of Mo, Sm, N, φEo and ψO mainly reflect the change in the PSII electron acceptor side (Table S1†). As shown in the spider-plot (Fig. 3G and H), all presented parameters were affected to a different extent by La2O3 NP treatment in soybean but were barely changed in maize. This illustrates that La2O3 NPs blocked the electron transport in the PSII electron acceptor side of soybean, while the electron was smoothly transported through PSII electron acceptor side of maize. The increase of the I step indicates that electron transport from PQ to the PSI electron acceptor side is blocked.27 Meanwhile, the I step displayed no significant change in both plants, illustrating that the electron transport from PQ to the PSI electron acceptor side remained unchanged upon La2O3 NP exposure (Fig. 3C and D).
When the PS II electron donor side is damaged, there would be more inflection points in the OJIP curve.43 Herein, Wk curves could be obtained between the O and J steps. The increase of the K step fluorescence is widely considered to be a specific indicator of injury to the oxygen evolving complex (OEC) on the PSII electron donor side.27Fig. 3E and F show the Wk curves in soybean and maize seedlings upon La2O3 NP exposure. Similarly, La2O3 NPs significantly elevated the K step in soybean, indicating that the OEC was damaged and water splitting might be inhibited in soybean. On the contrary, the K step in maize after La2O3 NP exposure almost coincided with that of the unexposed control, which indicated that maize could maintain homeostasis in OEC in response to La2O3 NPs.
The fluorescence parameters shown in Fig. 3G represent energy capture and RCs activity of PSII.28 PSII in soybean was more sensitive to La2O3 NPs than maize and thermal energy dissipation was elevated by La2O3 NPs in soybean. The performance index (PIabs) precisely reflects the state of the photosynthetic apparatus, and is more sensitive than Fv/Fm.28 PIabs was reduced by 68.30% in soybean exposed to La2O3 NPs, while there was no obvious difference in maize (Fig. 3H). Hence, La2O3 NPs could reduce the energy conversion efficiency of PSII, block the electron transport from QA to QB and damage OEC in soybean. The light reaction of soybean was thus more susceptible to La2O3 NPs than maize.
3.5. The carbon fixation of C3 and C4 plants upon La2O3 NP exposure
As the non-stomatal limitation played a predominant role in this study, photosynthesis may be inhibited by the reduction of key enzymes in the dark reaction. For C3 plants, CO2 is fixed by the Calvin cycle in mesophyll cells, where the rate-limiting factor is Rubisco.44 Meanwhile, for C4 plants, CO2 is fixed not only by the Calvin cycle in bundle sheath cells but also by C4 photosynthesis in mesophyll cells. The initial carbon fixation of C4 photosynthesis is mainly catalyzed by PEPC.12,37,45 As shown in Fig. 4A, La2O3 NPs made no difference to Rubisco activity in soybean. However, Rubisco activity in maize exposed to La2O3 NPs was significantly decreased by 7.16% compared to the control (Fig. 4B and C). Meanwhile, PEPC activity in maize was significantly reduced by 19.08% in comparison with the control. This suggested that La2O3 NPs had no impact on the carbon fixation in soybean, whereas they significantly inhibited carbon fixation in maize. The inhibition of Rubisco and PEPC could slow down the CO2 assimilation in maize (expressed by Pn) (Fig. 2B). In addition, the expression levels of genes involved in Rubisco and PEPC were determined in maize. The relative expression of the PEPC gene (pepc), the Rubisco large subunit (rbcL) and the Rubisco small subunit (rbcS) were downregulated in maize exposed to La2O3 NPs as compared to the control (Fig. 4D–F). The low gene expression of some enzymes involved in carbon fixation processes (pepc, rbcL and rbcS) could decrease PEPC and Rubisco synthesis and further inhibit photosynthetic efficiency. As a matter of fact, xenobiotics have been shown to influence the above-mentioned gene expression. For example, exogenous alpha lipoic acid has been shown to mitigate drought stress of maize through inducing gene expression associated with carbon fixation (pepc, rbcL and rbcS), enhancing the photosynthetic performance.30 Moreover, as shown in Fig. 5, upon La2O3 NP exposure, the chloroplasts of maize produced excess ROS, which has the potential to disturb the whole energy transfer in photosynthesis. The generation of excess ROS might be due to additional electrons accumulating in chloroplast stroma from the inactivation of Rubisco.46
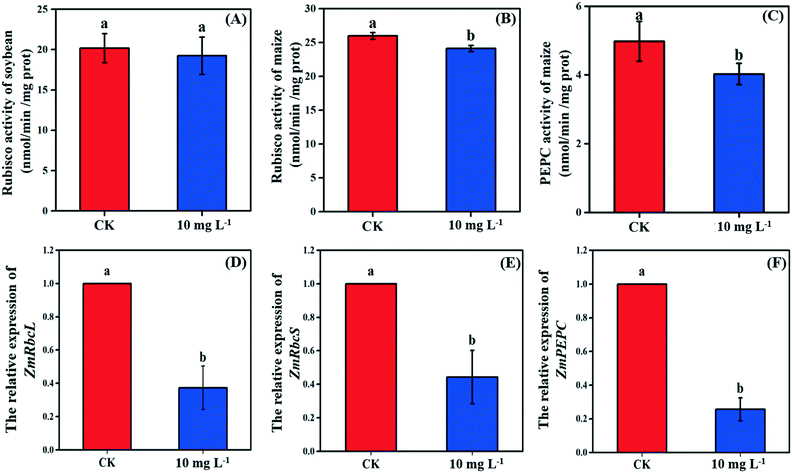 |
| Fig. 4 The activity of key enzymes (Rubisco and PEPC) involved in carbon fixation of soybean (A) and maize (B and C), and the relative gene expression of Rubisco (D and E) and PEPC (F) of maize subjected to 10 mg L−1 La2O3 NPs for 4 days. Data with different letters represent significant differences (p < 0.05). | |
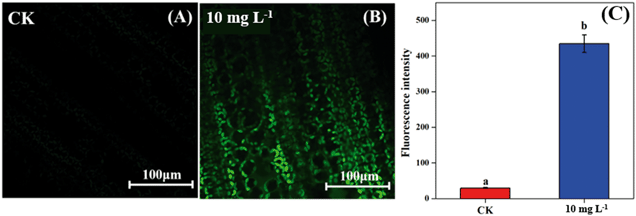 |
| Fig. 5 Confocal images with fluorescence of ROS in maize leaves (A: control, B: 10 mg L−1 La2O3 NP exposure) and mean fluorescence intensity of confocal images (C). Data with different letters represent significant differences (p < 0.05). | |
Previous studies have shown that NPs inhibited carbon fixation in C3 plants. For example, Ag NPs inhibited Rubisco activity and generated additional ROS within chloroplasts in the aquatic plant, Spirodela polyrhiza (C3 plant).18 However, in the present study, carbon fixation in C3 plants did not change in response to La2O3 NPs. These differences might be due to the fact that both roots and leaves of aquatic plants come in contact with NPs, while only roots of soybean come in contact with NPs in this work. In the terrestrial plant, Clarkia unguiculata (C3 plant), TiO2 and CeO2 NPs reduced the CO2 assimilation rate and induced superfluous ROS production under high light and nutrient conditions.19 The different results are likely because Clarkia unguiculata was exposed to complex combined stresses, while the plants were only exposed to NPs in this study. For C4 plants, Djanaguiraman et al.20 indicated that foliar-sprayed CeO2 NPs could alleviate drought-induced oxidative stress by ROS scavenging, and lead to higher sorghum photosynthetic efficiency and grain yield. This was because CeO2 NPs had excellent catalytic activity accompanied by oxygen vacancy generation or elimination, thereby cerium ions reversibly transit between the Ce4+ and Ce3+ oxidation states to scavenge ROS.1 However, a comparison of the response mechanisms during photosynthesis between C3 and C4 plants exposed to NPs has not been reported so far. In the present study, the damage mechanisms in C3 and C4 plants in response to La2O3 NPs were comparatively studied. Specifically, La2O3 NPs restricted the photosynthesis of the C3 plants (soybean), mainly by inhibiting the light utilization process rather than the carbon fixation process. On the contrary, La2O3 NPs inhibited the photosynthesis of the C4 plants (maize), mainly by inhibiting the carbon fixation process. There are three possible reasons to explain the different impacts of La2O3 NPs on the photosynthesis between C3 and C4 plants. Firstly, photorespiration is essential for photosynthetic apparatus against photoinactivation via the consumption of superfluous ATP and NADPH in the case of ROS accumulation and alleviating the overreduction of QA.46,47 As a bifunctional enzyme, Rubisco not only initiates the carbon fixation pathway but also catalyzes the oxidation reaction during photorespiration.44 The ratio of carboxylation to oxygenation depends on the CO2/O2 ratio.11 In C3 plants, only 220 μbar of CO2 could enter into chloroplasts involved in carboxylation when the ambient concentration is approximately 380 μbar.37 However, the whole C4 photosynthesis could finally increase the CO2 concentration by more than 5-fold via Rubisco in C4 plants as compared to C3 plants, which is called the “CO2 concentrating mechanism”.12,37,45 The “CO2 concentrating mechanism” might cause the elimination or minimization of photorespiration and then reduce the ability of C4 plants to scavenge excess ROS.12,37 C4 plants are more sensitive to the effects of NPs in comparison to C3 plants. Secondly, the structural differences in the leaves between C3 and C4 plants might result in different defense capabilities against xenobiotics. In terms of anatomy, compared with C3 plants, C4 plants have a unique structure called the Kranz anatomy, which consists of closely packed mesophyll and plump bundle sheath cells. For C4 plants, the first step of CO2-fixing occurs in mesophyll cells, and the Calvin cycle takes place in plump bundle sheath cells.17 However, all photosynthetic reactions of C3 plants occur in mesophyll cells owing to the fact that there are few chloroplasts in the small bundle sheath cells.17 NPs could be transferred from roots to leaves via long-distance transport along with water through the xylem of the vascular system.34 The vascular system is surrounded by bundle sheath cells and mesophyll cells from inside to outside. Accordingly, NPs could firstly affect the reactions in the bundle sheath cells, and then restrict the chain reactions in the mesophyll cells in C4 plants. However, compared to C4 plants, photosynthesis of C3 plants is only affected by the change of mesophyll cells. Thirdly, NPs could enter roots through the epidermis and cortex, become transported to the shoots,21,35,48 subsequently translocate into the leaves, and even transport from leaf extracellular air spaces to mesophyll chloroplasts.24,32 After La2O3 NP exposure, genes encoding for transporters and electron carriers, as well as the PSII protein located in chloroplast and thylakoid membrane, were downregulated in crop plants.49 In this study, La was detected in the shoots of both plants, and soybean leaves were found to have more La than maize leaves (Fig. 1A). It is possible that La2O3 NPs were transferred from roots to the leaves and then entered the chloroplasts. Also, the inter-thylakoid gaps range between 50 and 200 nm.50 Thus, they are larger than the size of La2O3 NPs used in the present study. More particles could insert within thylakoid stacks in soybean chloroplasts than in maize chloroplasts, and would compete for electrons and then impede electron transport.19 In summary, the mechanisms of the inhibited photosynthesis between C3 and C4 plants upon La2O3 NP exposure were different.
A previous study suggested that NPs exhibited a hormesis effect on plants, i.e., a beneficial effect at a low dose and a detrimental effect at high dose.25 At a low dose, NPs might activate the defense systems appropriately and have enormous potential to promote crop growth and resilience, thus increasing quality and yield. Also, a low dose has economic benefit for the application of nanotechnology in the agricultural industry. Recently, scientists have been trying to explore the opportunities and challenges of nanotechnology in agricultural production.51,52 Nanotechnology is widely thought to have the potential to play an important role in agroecological systems, through improving crop yield and resilience in order to meet the fast-growing demands for food. The processes and mechanisms of photosynthesis augmented by NPs to increase crop yields have been summarized in our previous review.33 Appropriate application methods, e.g., foliar spray, and NPs with negative charges or greater biocompatible coatings have also been proposed to improve the bioavailability of NPs in agricultural applications. However, Sinclair et al.53 suggested that a large change in the CO2-fixing enzyme could only trigger a minimal alteration in crop yield. On the contrary, Heyneke et al.11 summarized that even a slight increment of photosynthetic rate can raise crop yield dramatically. It is known that photosynthesis is a fundamental metabolic process for crop growth10 and photosynthates could be translocated from the source organs (the site of its synthesis) to sink organs via the phloem.54 Thus, future research should focus on the perspective of source and sink relationship in C3 and C4 plants in the mature stage upon NP exposure at a lower dosages. Corresponding strategies could then be proposed to increase the efficiency of nanotechnology application in sustainable agricultural systems.
4. Conclusion
In this present study, a comparison of the photosynthesis between seedlings of C3 and C4 plants upon La2O3 NP exposure was made for the first time. The results suggest that the photosynthesis of both soybean (typical C3 plant) and maize (typical C4 plant) could be inhibited in response to La2O3 NPs, and maize is more sensitive than soybean. However, the damage mechanisms are different between the two plants. Specifically, La2O3 NPs significantly inhibited the photosynthesis of soybean by hindering the light utilization and electron transport, which could further reduce the supply of ATP and NADPH. However, the inhibition of photosynthesis in maize was mainly due to restricted carbon fixation. The activity of the key enzymes, Rubisco and PEPC, were both reduced through the downregulation of their relative gene expression (pepc, rbcL and rbcS). Excess electrons were then accumulated, and superfluous ROS were generated. The results provide basic knowledge and new perspectives for developing targeted regulation strategies to apply nano-agricultural technology in higher plants with different photosynthetic pathways.
Conflicts of interest
The authors report no conflict of interest to this work.
Acknowledgements
This research was supported by the National Natural Science Foundation of China (41820104009, 41530642, 41807378) and USDA-NIFA Hatch program/UMass CAFE (MAS 00549).
References
- C. Xu and X. Qu, Cerium oxide nanoparticle: a remarkably versatile rare earth nanomaterial for biological applications, NPG Asia Mater., 2014, 6, e90 CrossRef CAS.
- Y. Ma, X. He, P. Zhang, Z. Zhang, Z. Guo, R. Tai, Z. Xu, L. Zhang, Y. Ding, Y. Zhao and Z. Chai, Phytotoxicity and biotransformation of La2O3 nanoparticles in a terrestrial plant cucumber (Cucumis sativus), Nanotoxicology, 2011, 5, 743–753 CrossRef CAS PubMed.
- L. Yue, C. Ma, X. Zhan, J. C. White and B. Xing, Molecular mechanisms of maize seedling response to La2O3 NP exposure: water uptake, aquaporin gene expression and signal transduction, Environ. Sci.: Nano, 2017, 4, 843–855 RSC.
- K. L. Garner, S. Suh and A. A. Keller, Assessing the risk of engineered nanomaterials in the environment: development and application of the nanoFate model, Environ. Sci. Technol., 2017, 51, 5541–5551 CrossRef CAS PubMed.
- L. Rossi, H. Sharifan, W. Zhang, A. P. Schwab and X. Ma, Mutual effects and in planta accumulation of co-existing cerium oxide nanoparticles and cadmium in hydroponically grown soybean (Glycine max (L.) Merr.), Environ. Sci.: Nano, 2018, 5, 150–157 RSC.
- Y. Ma, L. Kuang, X. He, W. Bai, Y. Ding, Z. Zhang, Y. Zhao and Z. Chai, Effects of rare earth oxide nanoparticles on root elongation of plants, Chemosphere, 2010, 78, 273–279 CrossRef CAS PubMed.
- Y. Ma, P. Zhang, Z. Zhang, X. He, Y. Li, J. Zhang, L. Zheng, S. Chu, K. Yang, Y. Zhao and Z. Chai, Origin of the different phytotoxicity and biotransformation of cerium and lanthanum oxide nanoparticles in cucumber, Nanotoxicology, 2015, 9, 262–270 CrossRef CAS PubMed.
- R. De la Torre Roche, A. Servin, J. Hawthorne, B. Xing, L. A. Newman, X. Ma, G. Chen and J. C. White, Terrestrial trophic transfer of bulk and nanoparticle La2O3 does not depend on particle size, Environ. Sci. Technol., 2015, 49, 11866–11874 CrossRef CAS PubMed.
- L. Yue, F. Chen, K. Yu, Z. Xiao, X. Yu, Z. Wang and B. Xing, Early development of apoplastic barriers and molecular mechanisms in juvenile maize roots in response to La2O3 nanoparticles, Sci. Total Environ., 2019, 653, 675–683 CrossRef CAS PubMed.
- E. Heyneke and A. R. Fernie, Metabolic regulation of photosynthesis, Biochem. Soc. Trans., 2018, 46, 321–328 CrossRef CAS PubMed.
- K. Kajala, S. Covshoff, S. Karki, H. Woodfield, B. J. Tolley, M. J. Dionora, R. T. Mogul, A. E. Mabilangan, F. R. Danila, J. M. Hibberd and W. P. Quick, Strategies for engineering a two-celled C4 photosynthetic pathway into rice, J. Exp. Bot., 2011, 62, 3001–3010 CrossRef CAS PubMed.
- X. G. Zhu, S. P. Long and D. R. Ort, What is the maximum efficiency with which photosynthesis can convert solar energy into biomass?, Curr. Opin. Biotechnol., 2008, 19, 153–159 CrossRef CAS PubMed.
- W. Yamori, K. Hikosaka and D. A. Way, Temperature response of photosynthesis in C3, C4, and CAM plants: temperature acclimation and temperature adaptation, Photosynth. Res., 2014, 119, 101–117 CrossRef CAS PubMed.
- A. Gomez-Garay, B. Pintos, J. A. Manzanera, C. Lobo, N. Villalobos and L. Martin, Uptake of CeO2 nanoparticles and its effect on growth of Medicago arborea in vitro plantlets, Biol. Trace Elem. Res., 2014, 161, 143–150 CrossRef CAS PubMed.
- D. Killi, F. Bussotti, A. Raschi and M. Haworth, Adaptation to high temperature mitigates the impact of water deficit during combined heat and drought stress in C3 sunflower and C4 maize varieties with contrasting drought tolerance, Physiol. Plant., 2017, 159, 130–147 CrossRef CAS PubMed.
- M. Wang, B. Xie, Y. Fu, C. Dong, L. Hui, L. Guanghui and H. Liu, Effects of different elevated CO2 concentrations on chlorophyll contents, gas exchange, water use efficiency, and PSII activity on C3 and C4 cereal crops in a closed artificial ecosystem, Photosynth. Res., 2015, 126, 351–362 CrossRef CAS PubMed.
- L. Wang, A. Czedik-Eysenberg, R. A. Mertz, Y. Si, T. Tohge, A. Nunes-Nesi, S. Arrivault, L. K. Dedow, D. W. Bryant, W. Zhou, J. Xu, S. Weissmann, A. Studer, P. Li, C. Zhang, T. LaRue, Y. Shao, Z. Ding, Q. Sun, R. V. Patel, R. Turgeon, X. Zhu, N. J. Provart, T. C. Mockler, A. R. Fernie, M. Stitt, P. Liu and T. P. Brutnell, Comparative analyses of C4 and C3 photosynthesis in developing leaves of maize and rice, Nat. Biotechnol., 2014, 32, 1158 CrossRef CAS PubMed.
- H. S. Jiang, L. Y. Yin, N. N. Ren, S. T. Zhao, Z. Li, Y. Zhi, H. Shao, W. Li and B. Gontero, Silver nanoparticles induced reactive oxygen species via photosynthetic energy transport imbalance in an aquatic plant, Nanotoxicology, 2017, 11, 157–167 CrossRef CAS PubMed.
- J. R. Conway, A. L. Beaulieu, Ni. L. Beaulieu, S. J. Mazer and A. A. Keller, Environmental stresses increase photosynthetic disruption by metal oxide nanomaterials in a soil-grown plant, ACS Nano, 2015, 9, 11737–11749 CrossRef CAS PubMed.
- M. Djanaguiraman, R. Nair, J. P. Giraldo and P. V. V. Prasad, Cerium oxide nanoparticles decrease drought-induced oxidative damage in sorghum leading to higher photosynthesis and grain yield, ACS Omega, 2018, 3, 14406–14416 CrossRef CAS PubMed.
- Z. Wang, X. Xie, J. Zhao, X. Liu, W. Feng, J. C. White and B. Xing, Xylem- and phloem-based transport of CuO nanoparticles in maize (Zea mays L.), Environ. Sci. Technol., 2012, 46, 4434–4441 CrossRef CAS PubMed.
- J. Zhao, W. Ren, Y. Dai, L. Liu, Z. Wang, X. Yu, J. Zhang, X. Wang and B. Xing, Uptake, distribution, and transformation of CuO NPs in a floating plant Eichhornia crassipes and related stomatal responses, Environ. Sci. Technol., 2017, 51, 7686–7695 CrossRef CAS PubMed.
- J. Li, Y. Song, K. Wu, Q. Tao, Y. Liang and T. Li, Effects of Cr2O3 nanoparticles on the chlorophyll fluorescence and chloroplast ultrastructure of soybean (Glycine max), Environ. Sci. Pollut. Res., 2018, 25, 19446–19457 CrossRef CAS PubMed.
- V. Nhan le, C. Ma, Y. Rui, S. Liu, X. Li, B. Xing and L. Liu, Phytotoxic mechanism of nanoparticles: destruction of chloroplasts and vascular bundles and alteration of nutrient absorption, Sci. Rep., 2015, 5, 11618 CrossRef PubMed.
- V. L. R. Pullagurala, I. O. Adisa, S. Rawat, B. Kim, A. C. Barrios, I. A. Medina-Velo, J. A. Hernandez-Viezcas, J. R. Peralta-Videa and J. L. Gardea-Torresdey, Finding the conditions for the beneficial use of ZnO nanoparticles towards plants-A review, Environ. Pollut., 2018, 241, 1175–1181 CrossRef PubMed.
- R. Gornati, A. Longo, F. Rossi, M. Maisano, G. Sabatino, A. Mauceri, G. Bernardini and S. Fasulo, Effects of titanium dioxide nanoparticle exposure in Mytilus galloprovincialis gills and digestive gland, Nanotoxicology, 2016, 10, 807–817 CrossRef CAS PubMed.
- Z. Zhang, G. Li, H. Gao, L. Zhang, C. Yang, P. Liu and Q. Meng, Characterization of photosynthetic performance during senescence in stay-green and quick-leaf-senescence Zea mays L. inbred lines, PLoS One, 2012, 7, e42936 CrossRef CAS PubMed.
- R. J. Strasser, M. Tsimilli-Michael, S. Qiang and V. Goltsev, Simultaneous in vivo recording of prompt and delayed fluorescence and 820-nm reflection changes during drying and after rehydration of the resurrection plant Haberlea rhodopensis, Biochim. Biophys. Acta, 2010, 1797, 1313–1326 CrossRef CAS PubMed.
- R. A. Boyd, A. Gandin and A. B. Cousins, Temperature responses of C4 photosynthesis: biochemical analysis of Rubisco, phosphoenolpyruvate carboxylase, and carbonic anhydrase in Setaria viridis, Plant Physiol., 2015, 169, 1850–1861 CAS.
- A. Sezgin, C. Altuntas, M. Demiralay, S. Cinemre and R. Terzi, Exogenous alpha lipoic acid can stimulate photosystem II activity and the gene expressions of carbon fixation and chlorophyll metabolism enzymes in maize seedlings under drought, J. Plant Physiol., 2019, 232, 65–73 CrossRef CAS PubMed.
- K. J. Livak and T. D. Schmittgen, Analysis of relative gene expression data using real-time quantitative PCR and the 2-△△CT method, Methods, 2001, 25, 402–408 CrossRef CAS PubMed.
- H. Wu, N. Tito and J. P. Giraldo, Anionic cerium oxide nanoparticles protect plant photosynthesis from abiotic stress by scavenging reactive oxygen species, ACS Nano, 2017, 11, 11283–11297 CrossRef CAS PubMed.
- Y. Liu, L. Yue, Z. Wang and B. Xing, Processes and mechanisms of photosynthesis augmented by engineered nanomaterials, Environ. Chem., 2019, 16, 430–445 CrossRef CAS.
-
P. J. White, in Marschner's Mineral Nutrition of Higher Plants, Elsevier, 3rd edn, 2012, pp. 49–70 Search PubMed.
- P. Zhang, Y. Ma, C. Xie, Z. Guo, X. He, E. Valsami-Jones, I. Lynch, W. Luo, L. Zheng and Z. Zhang, Plant species-dependent transformation and translocation of ceria nanoparticles, Environ. Sci.: Nano, 2019, 6, 60–67 RSC.
- T. Shiroya, G. R. Lister, G. Krotkov, C. D. Nelson and V. Slankis, Translocation of the products of photosynthesis to roots of pine seedlings, Can. J. Bot., 1962, 40, 1125–1135 CrossRef.
- O. Ghannoum, C4 photosynthesis and water stress, Ann. Bot., 2009, 103, 635–644 CrossRef CAS PubMed.
- G. D. Farquhar and T. D. Sharkey, Stomatal conductance and photosynthesis, Annu. Rev. Plant Physiol., 1982, 33, 317–345 CrossRef CAS.
- N. R. Baker, Chlorophyll fluorescence: a probe of photosynthesis in vivo, Annu. Rev. Plant Biol., 2008, 59, 89–113 CrossRef CAS PubMed.
- K. Maxwell and G. N. Johnson, Chlorophyll fluorescence—a practical guide, J. Exp. Bot., 2000, 51, 659–668 CrossRef CAS PubMed.
- Y. T. Li, Y. Liang, Y. N. Li, X. K. Che, S. J. Zhao, Z. S. Zhang and H. Y. Gao, Mechanisms by which Bisphenol A affect the photosynthetic apparatus in cucumber (Cucumis sativus L.) leaves, Sci. Rep., 2018, 8, 4253 CrossRef PubMed.
- L. Jin, X. Che, Z. Zhang, Y. Li, H. Gao and S. Zhao, The mechanisms by which phenanthrene affects the photosynthetic apparatus of cucumber leaves, Chemosphere, 2017, 168, 1498–1505 CrossRef CAS PubMed.
-
R. J. Strasser, M. Tsimilli-Michael and A. Srivastava, in Chlorophyll a fluorescence: a signature of photosynthesis, Springer, Dordrecht, 2004, pp. 321–362 Search PubMed.
- M. A. Parry, P. J. Andralojc, J. C. Scales, M. E. Salvucci, A. E. Carmo-Silva, H. Alonso and S. M. Whitney, Rubisco activity and regulation as targets for crop improvement, J. Exp. Bot., 2013, 64, 717–730 CrossRef CAS PubMed.
- R. T. Furbank and M. D. Hatch, Mechanism of C4 Photosynthesis: the size and composition of the inorganic carbon pool in bundle sheath cells, Plant Physiol., 1987, 85, 958 CrossRef CAS PubMed.
- K. Asada, Production and scavenging of reactive oxygen species in chloroplasts and their functions, Plant Physiol., 2006, 141, 391–396 CrossRef CAS PubMed.
- U. Heber, R. Bligny, P. Streb and R. Douce, Photorespiration is essential for the protection of the photosynthetic apparatus of C3 plants against photoinactivation under sunlight, Bot. Acta, 1996, 109, 307–315 CrossRef CAS.
- E. Spielman-Sun, E. Lombi, E. Donner, D. Howard, J. M. Unrine and G. V. Lowry, Impact of surface charge on cerium oxide nanoparticle uptake and translocation by wheat (Triticum aestivum), Environ. Sci. Technol., 2017, 51, 7361–7368 CrossRef CAS PubMed.
- L. Pagano, A. D. Servin, R. De La Torre-Roche, A. Mukherjee, S. Majumdar, J. Hawthorne, M. Marmiroli, E. Maestri, R. E. Marra, S. M. Isch, O. P. Dhankher, J. C. White and N. Marmiroli, Molecular response of crop plants to engineered nanomaterials, Environ. Sci. Technol., 2016, 50, 7198–7207 CrossRef CAS PubMed.
-
P. H. Raven, R. F. Evert and S. E. Eichhorn, in Biology of Plants, W. H. Freeman and Company, New York, NY, 7th edn, 2005 Search PubMed.
- G. V. Lowry, A. Avellan and L. M. Gilbertson, Opportunities and challenges for nanotechnology in the agri-tech revolution, Nat. Nanotechnol., 2019, 14, 517–522 CrossRef CAS PubMed.
- M. Kah, N. Tufenkji and J. C. White, Nano-enabled strategies to enhance crop nutrition and protection, Nat. Nanotechnol., 2019, 14, 532–540 CrossRef CAS PubMed.
- T. R. Sinclair, L. C. Purcell and C. H. Sneller, Crop transformation and the challenge to increase yield potential, Trends Plant Sci., 2004, 9, 70–75 CrossRef CAS PubMed.
- S. M. Yu, S. F. Lo and T. H. D. Ho, Source-sink communication: regulated by hormone, nutrient, and stress cross-signaling, Trends Plant Sci., 2015, 20, 844–857 CrossRef CAS PubMed.
Footnote |
† Electronic supplementary information (ESI) available. See DOI: 10.1039/c9en00992b |
|
This journal is © The Royal Society of Chemistry 2020 |
Click here to see how this site uses Cookies. View our privacy policy here.