DOI:
10.1039/D0QM00109K
(Research Article)
Mater. Chem. Front., 2020,
4, 1643-1647
Near-IR absorption and photocurrent generation using a first-of-its-kind boron difluoride formazanate non-fullerene acceptor†
Received
26th February 2020
, Accepted 6th April 2020
First published on 8th April 2020
Abstract
Herein, we report the synthesis and characterization of the first non-fullerene acceptor (NFA) containing a boron difluoride formazanate (BF2fz) core end-capped with N-annulated perylene diimides (PDIs). Electronic coupling between the BF2fz core and the PDI endcaps enabled tuning of the lowest unoccupied molecular orbital, leading to near-panchromatic optical absorption. Post-deposition solvent vapor annealing of the new NFA resulted in a significant red-shift in the optical spectra, which stretched into the near-IR. Proof-of-concept organic photovoltaic (OPV) devices were constructed to demonstrate the potential of this new material as an NFA. SVA treatment of the active layer resulted in a 2-fold increase in power conversion efficiency (PCE), due mainly to increases in the BF2PDI2 generated photocurrent that extended into the near-IR.
Introduction
For applications in organic photovoltaics (OPVs), π-conjugated materials are perfectly suited for use as non-fullerene acceptors (NFAs) because both physical and optoelectronic properties may be optimized to match the electron-donor material with which they are paired in the active layer.1,2 One class of non-fullerene acceptors (NFAs) recognized for high redox and thermal stability, as well as high molar absorptivity, are perylene diimides (PDIs).3,4 Despite such favorable qualities, the efficiency of charge-extraction for PDI-based NFA materials is often hindered by the formation of large π–π stacking domains throughout the BHJ.1 To promote more favorable phase separation in the BHJ, PDI self-assembly may be disrupted through either direct dimerization of PDIs5–8 or insertion of a core between the PDI-moieties.9–20
The Welch research group has previously explored the optoelectronic influence of thienoisoindigo, thienyl- and pyridyl-diketopyrrolopyrrole cores that were acetylene-linked to N-annulated PDI (Fig. 1).21 Strong electronic communication between the cores and PDI endcaps adjusted the highest occupied molecular orbital (HOMO) energy level of these molecules, affording near-panchromatic absorptions ideally suited for OPV. In search of a core that could achieve similar absorptivity, while instead influencing the lowest unoccupied molecular (LUMO) energy level, we targeted an acetylene-terminated boron difluoride formazanate (BF2fz), a chromophore popularized by the Gilroy research group.22–24 The properties of BF2fz materials are highly sensitive to structural variation at the N-aryl substituents,25–27 meaning the optoelectronic properties can be tuned by the choice of endcap material. In this contribution, we report the synthesis, characterization, and NFA capability of a new π-conjugated material comprised of a BF2fz core acetylene-linked to N-annulated PDI endcaps (BF2PDI2).
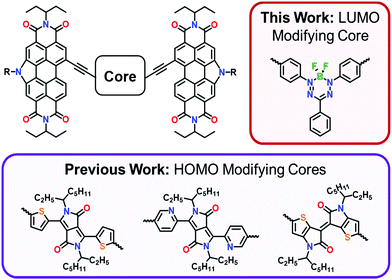 |
| Fig. 1 Depicting previously investigated HOMO-modifying and newly synthesized LUMO-modifying N-annulated PDI-core-PDI materials. R = hexyl (HOMO modifying cores) or 2-ethylhexyl (LUMO modifying core). | |
Characterization
The BF2fz core22 and N-annulated PDI (Br-PDIN-EH)8 end caps were connected via a Sonogashira cross-coupling reaction to generate BF2PDI2 (see ESI,† for full experimental details). The identity of BF2PDI2 was confirmed by 1H, 11B, 13C, and 19F NMR spectroscopy, as well as MALDI TOF mass spectrometry and CHN elemental analysis (Fig. S1–S7, ESI†). The 11B and 19F NMR spectra exhibited diagnostic 1
:
2
:
1 triplet and 1
:
1
:
1
:
1 quartet coupling patterns, respectively.25 Moreover, the 1H NMR spectrum showed no evidence of a formazan related N-H peak (at ∼15 ppm),22 strongly suggesting the BF2fz core remained intact throughout synthesis and purification.
The thermal properties of BF2PDI2 were measured using a combination of differential scanning calorimetry and thermal gravimetric analysis (Fig. S8 and S9, ESI†). No obvious glass transitions or melting points were observed between 100–300 °C, while the onset of molecular decomposition was not observed until >300 °C. The high thermal stability observed in BF2PDI2 may be attributed to significant π-conjugation between the BF2fz core and the N-annulated PDI endcaps. This notion was supported by the density functional theory (DFT) optimized structure of BF2PDI2 at the B3LYP-6-31G(d,p) level of theory (Fig. S14, ESI†). The PDI units were nearly co-planar with each other, with only a small degree of bending caused by the BF2fz core. This DFT optimized ‘dragonfly’ structure corresponded well with previously determined X-ray crystallography structures for compounds using the same BF2fz core.23,24
Next, the electronic properties of BF2PDI2 were probed using cyclic voltammetry (CV), with additional information provided by DFT calculations. The CV of BF2PDI2 was comprised of three irreversible oxidation events, as well as four fully reversible reduction events (Fig. 2). While the number oxidation events could be determined by differential pulse voltammetry (Fig. S13, ESI†), the precise electrochemical origin may not be readily assigned. On the other hand, the first and fourth reductions (E1/2 = −0.85 and −1.96 V vs. Fc+/0) may be attributed to BF2fz core, while the second and third reduction events (E1/2 = −1.32 and −1.60 V vs. Fc+/0) may be attributed to N-annulated PDI as the current passed during these redox processes was 2× larger. By DFT calculations, the first oxidation event appeared to be delocalized across the entire molecule, while the first reduction event was largely centered on the BF2fz core (Fig. S15, ESI†). The predicted HOMO–LUMO energy levels correlate well with the observed CV data. Together, these data strongly suggest that the BF2fz core has a higher electron affinity than the N-annulated PDI endcaps, which served to lower the LUMO energy level of BF2PDI2, when compared to related compounds with different cores.21
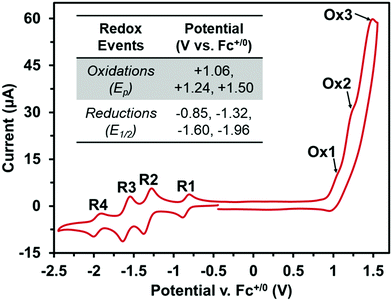 |
| Fig. 2 Cyclic voltammogram of BF2PDI2, measured at 100 mV s−1 in CH2Cl2 under argon with 0.1 M TBAPF6 supporting electrolyte (WE = glassy carbon, CE = Pt-wire, pseudo-RE = Ag/AgCl). Inset are all measured oxidation (Ox) and reduction (R) events, referenced to Fc+/0. | |
The optical properties of BF2PDI2 were measured by UV-visible-nearIR absorption spectroscopy (UV-vis-nIR). The UV-vis-nIR profile of BF2PDI2 in solution exhibited a broad absorbance between 450–750 nm, with an absorption maximum (λmax) at 543 nm attributable to the PDI endcaps and a low energy shoulder just beyond 600 nm attributed to the BF2fz core (Fig. 3). By overlaying the optical spectra of BF2PDI2 with its constituent fragments (BF2Ace2 and PDIN-EH, Fig. S11, ESI†), it was revealed that the low energy shoulder attributed to BF2fz core was red-shifted in BF2PDI2, emphasizing the extension of π-conjugation induced by the PDI endcaps. When spin-cast into a thin-film, the optical profile of BF2PDI2 underwent several notable changes. Beyond the slightly red-shifted onset of absorption (λonset), the broadening of λmax was also accompanied by an appreciable increase in the intensity of the low-energy shoulder, consistent with enhanced electron delocalization along the π-conjugated backbone.21
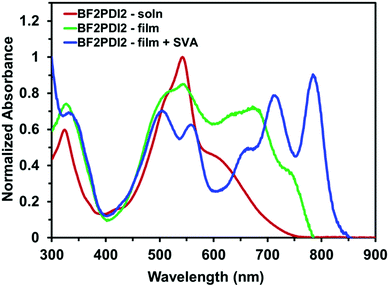 |
| Fig. 3 UV-vis-nIR absorption profile of BF2PDI2 in CHCl3 solution (red), thin-film spin-cast from o-dichlorobenzene (green), and the same thin-film solvent vapor annealed from CHCl3 (blue). | |
In the past, we have shown that post-deposition solvent vapor annealing (SVA) can induce structural order within the solid-state morphology of similar PDI–core–PDI materials.11,28–31 Exposure of the BF2PDI2 thin-film to CHCl3 solvent vapors caused the once broad solid-state optical profile to resolve into two distinct absorption regions. The PDI-based λmax region was split into two peaks (λ = 508 and 560 nm), while the low-energy shoulder developed fine structure (λmax = 790 nm) that extended well into the near-IR (λonset = 850 nm). This behaviour is strongly indicative of molecular reorganization and/or aggregation of BF2PDI2 into ordered domains with strong intermolecular electronic-coupling in the solid-state.32–34
OPV devices
The NFA capabilities of BF2PDI2 were assessed by constructing some proof-of-concept OPV devices with the following inverted architecture: ITO/ZnO/ternary active layer/MoOx/Ag (full experimental details in ESI†). A ternary active layer comprised of electron-donor polymer PPDT2FBT (FBT), PC61BM, and BF2PDI2 was employed for OPV devices. FBT was selected because of its complementary absorption with the solvent vapor annealed film of BF2PDI2 (Fig. S16, ESI†). PC61BM was added to this ternary blend to assist with charge mobility.35 Using a FBT
:
BF2PDI2
:
PC61BM blend ratio of (1
:
1
:
0.5) at 10 mg mL−1 total concentration, the best OPV devices achieved an open circuit voltage (Voc) of 0.69 V, a short circuit current (Jsc) of 1.1 mA cm−2, and a fill factor (FF) of 41%, leading to a power conversion efficiency (PCE) of 0.3% (Table S1, ESI†).
Post-deposition treatment of these films with CHCl3 SVA resulted in the desired optical profile shift of BF2PDI2, giving the OPV devices a near-panchromatic absorbance (Fig. 4A). Device performance was maximized after 5 min of CHCl3 SVA, where both the Jsc and FF increased to 1.9 mA cm−2 and 49%, respectively, leading to a 2-fold increase in PCE to 0.6% (Fig. 4B). Longer SVA treatments led to OPV performances similar to as-cast devices (Fig. S17 and Table S2, ESI†). This phenomena has previously been observed with our N-annulated PDI–core–PDI materials and was attributed to over-annealing of the active layer.11,28
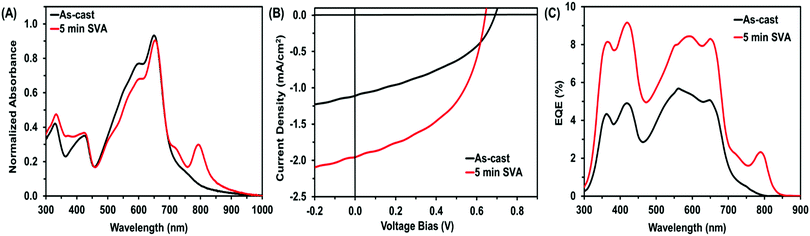 |
| Fig. 4 Optical absorption profile (A), J–V curves (B), an external quantum efficiency plots (C) comparing the effects of solvent vapor annealing with CHCl3 on ternary blend OPV devices with a FBT : BF2PDI2 : PC61BM (1 : 1 : 0.5) active layer at 10 mg mL−1 total concentration. | |
To better understand the observed enhancement in OPV performance for FBT
:
BF2PDI2
:
PC61BM (1
:
1
:
0.5) ternary blends that were SVA using CHCl3 for 5 min, photoluminescence (PL) and external quantum efficiency (EQE) experiments were performed. PL measurements of the ternary blend showed efficient quenching of the FBT polymer before and after SVA treatment (Fig. S18, ESI†). Analysis of the EQE spectra, the measured photocurrent clearly displays contributions from all components of the ternary blend (Fig. 4C). Following SVA treatment, photocurrent generation was extended well into the near-IR (λmax = 790 nm), clearly emphasizing the increased contribution from the new NFA material, BF2PDI2.
Surface morphology differences between the as-cast and SVA treated devices were also analyzed by atomic force microscopy (AFM). AFM height images showed significant differences between the as-cast and SVA treated devices (Fig. 5), as the root-mean square surface roughness of the films increased from 1.9 nm to 4.8 nm. X-ray diffraction (XRD) measurements of ternary blend films before and after CHCl3 SVA failed to show any distinguishing features that could be attributed to BF2PDI2 (Fig. S19, ESI†).36 Together, these data suggest that SVA enhanced OPV performance by inducing BF2PDI2 aggregation into phase separated domains, rather than crystallization.
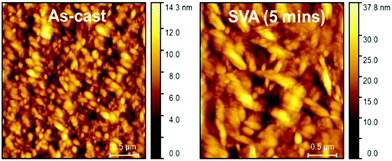 |
| Fig. 5 Atomic force microscopy height images of ternary blend OPV devices with an FBT : BF2PDI2 : PC61BM (1 : 1 : 0.5) active layer at 10 mg mL−1 total concentration. | |
Conclusions
In conclusion, we have synthesized and fully characterized a first-of-its-kind NFA containing a BF2fz core. The use of acetylene-linkers enabled through-conjugation between the BF2fz core and the PDI endcaps, leading to an altered LUMO energy level that resulted in near-panchromatic absorption for BF2PDI2. The new compound is a rare example of an acceptor–acceptor–acceptor type π-conjugated architecture. Treatment of solid-state BF2PDI2 with CHCl3 SVA resulted in a significant bathochromic shift of the optical spectra, leading to near-IR absorption. The overall performance in a series of proof-of-concept OPV devices, where BF2PDI2 was used as an NFA in a ternary blend system, was improved 2-fold by SVA treatment of the active layer. The increase in PCE could be mainly attributed to favorable aggregation of BF2PDI2 in the active layer which increased the generated photocurrent. This notion was supported by EQE and AFM measurements. This work demonstrates the utility of the BF2fz organic dye to construct narrow bandgap π-conjugated materials for use in organic electronics and provides the groundwork for further development.
Conflicts of interest
There are no conflicts to declare.
Acknowledgements
GCW acknowledges the Canada Research Chairs Program, CFI JELF (34102), NSERC DG (2019-04392), and the University of Calgary. JBG acknowledges CFI JELF (33977), NSERC DG (2018-04240), and the University of Western Ontario. JSD acknowledges NSERC CGS-D Scholarship. JK acknowledges Alberta Graduate Excellence Scholarship (AGES) program. Compute Canada and Westgrid are acknowledged for computational resources. This research was undertaken thanks in part to funding from the Canada First Research Excellence Fund (CFREF).
References
- S. Rajaram, R. Shivanna, S. K. Kandappa and K. S. Narayan, Nonplanar Perylene Diimides as Potential Alternatives to Fullerenes in Organic Solar Cells, J. Phys. Chem. Lett., 2012, 3, 2405–2408 CrossRef CAS PubMed
.
- C. B. Nielsen, S. Holliday, H.-Y. Chen, S. J. Cryer and I. McCulloch, Non-Fullerene Electron Acceptors for Use in Organic Solar Cells, Acc. Chem. Res., 2015, 48, 2803–2812 CrossRef CAS PubMed
.
- Z. Liu, Y. Wu, Q. Zhang and X. Gao, Non-Fullerene Small Molecule Acceptors Based on Perylene Diimides, J. Mater. Chem. A, 2016, 4, 17604–17622 RSC
.
- W. Chen and Q. Zhang, Recent Progress in Non-Fullerene Small Molecule Acceptors in Organic Solar Cells (OSCs), J. Mater. Chem. C, 2017, 5, 1275–1302 RSC
.
- D. Sun, D. Meng, Y. Cai, B. Fan, Y. Li, W. Jiang, L. Huo, Y. Sun and Z. Wang, Non-Fullerene-Acceptor-Based Bulk-Heterojunction Organic Solar Cells with Efficiency over 7%, J. Am. Chem. Soc., 2015, 137, 11156–11162 CrossRef CAS PubMed
.
- D. Meng, D. Sun, C. Zhong, T. Liu, B. Fan, L. Huo, Y. Li, W. Jiang, H. Choi, T. Kim, J. Y. Kim, Y. Sun, Z. Wang and A. J. Heeger, High-Performance Solution-Processed Non-Fullerene Organic Solar Cells Based on Selenophene-Containing Perylene Bisimide Acceptor, J. Am. Chem. Soc., 2016, 138, 375–380 CrossRef CAS PubMed
.
- A. D. Hendsbee, J.-P. Sun, W. K. Law, H. Yan, I. G. Hill, D. M. Spasyuk and G. C. Welch, Synthesis, Self-Assembly, and Solar Cell Performance of N-Annulated Perylene Diimide Non-Fullerene Acceptors, Chem. Mater., 2016, 28, 7098–7109 CrossRef CAS
.
- S. V. Dayneko, A. D. Hendsbee and G. C. Welch, Combining Facile Synthetic Methods with Greener Processing for Efficient Polymer-Perylene Diimide Based Organic Solar CellsSmall, Methods, 2018, 2, 1800081 Search PubMed
.
- Y. Lin, Y. Wang, J. Wang, J. Hou, Y. Li, D. Zhu and X. Zhan, A Star-Shaped Perylene Diimide Electron Acceptor for High-Performance Organic Solar Cells, Adv. Mater., 2014, 26, 5137–5142 CrossRef CAS PubMed
.
- Y. Duan, X. Xu, H. Yan, W. Wu, Z. Li and Q. Peng, Pronounced Effects of a Triazine Core on Photovoltaic Performance–Efficient Organic Solar Cells Enabled by a PDI Trimer-Based Small Molecular Acceptor, Adv. Mater., 2017, 29, 1605115 CrossRef PubMed
.
- S. M. McAfee, S. V. Dayneko, P. Josse, P. Blanchard, C. Cabanetos and G. C. Welch, Simply Complex: The Efficient Synthesis of an Intricate Molecular Acceptor for High-Performance Air-Processed and Air-Tested Fullerene-Free Organic Solar Cells, Chem. Mater., 2017, 29, 1309–1314 CrossRef CAS
.
- J. Liu, S. Xie, S. Feng, M. Li, L. Wu, X. Xu, X. Chen, C. Li and Z. Bo, A Propeller-Shaped Perylene Diimide Hexamer as a Nonfullerene Acceptor for Organic Solar Cells, J. Mater. Chem. C, 2018, 6, 9336–9340 RSC
.
- H. Lin, S. Chen, H. Hu, L. Zhang, T. Ma, J. Y. L. Lai, Z. Li, A. Qin, X. Huang, B. Tang and H. Yan, Reduced Intramolecular Twisting Improves the Performance of 3D Molecular Acceptors in Non-Fullerene Organic Solar Cells, Adv. Mater., 2016, 28, 8546–8551 CrossRef CAS PubMed
.
- Q. Wu, D. Zhao, A. M. Schneider, W. Chen and L. Yu, Covalently Bound Clusters of Alpha-Substituted PDI—Rival Electron Acceptors to Fullerene for Organic Solar Cells, J. Am. Chem. Soc., 2016, 138, 7248–7251 CrossRef CAS PubMed
.
- J. Zhang, Y. Li, J. Huang, H. Hu, G. Zhang, T. Ma, P. C. Y. Chow, H. Ade, D. Pan and H. Yan, Ring-Fusion of Perylene Diimide Acceptor Enabling Efficient Nonfullerene Organic Solar Cells with a Small Voltage Loss, J. Am. Chem. Soc., 2017, 139, 16092–16095 CrossRef CAS PubMed
.
- Q. Zhang, X. Xu, S. Chen, G. B. Bodedla, M. Sun, Q. Hu, Q. Peng, B. Huang, H. Ke, F. Liu, T. P. Russell and X. Zhu, Phenylene-Bridged Perylenediimide-Porphyrin Acceptors for Non-Fullerene Organic Solar Cells, Sustain, Energy Fuels, 2018, 2, 2616–2624 CAS
.
- T. A. Welsh, A. Laventure, T. Baumgartner and G. C. Welch, Dithienophosphole-Based Molecular Electron Acceptors Constructed Using Direct (Hetero)Arylation Cross-Coupling Methods, J. Mater. Chem. C, 2018, 6, 2148–2154 RSC
.
- T. A. Welsh, A. Laventure, A. F. Alahmadi, G. Zhang, T. Baumgartner, Y. Zou, F. Jäkle and G. C. Welch, Borane Incorporation in a Non-Fullerene Acceptor To Tune Steric and Electronic Properties and Improve Organic Solar Cell Performance, ACS Appl. Energy Mater., 2019, 2, 1229–1240 CrossRef CAS
.
- Z. Luo, T. Liu, Z. Chen, Y. Xiao, G. Zhang, L. Huo, C. Zhong, X. Lu, H. Yan, Y. Sun and C. Yang, Isomerization of Perylene Diimide Based Acceptors Enabling High-Performance Nonfullerene Organic Solar Cells with Excellent Fill Factor, Adv. Sci., 2019, 6, 1802065 CrossRef PubMed
.
- J. D. B. Koenig, A. Laventure and G. C. Welch, Harnessing Direct (Hetero)Arylation in Pursuit of a Saddle-Shaped Perylene Diimide Tetramer, ACS Appl. Energy Mater., 2019, 2, 8939–8945 CrossRef CAS
.
- J. R. Cann, C. Cabanetos and G. C. Welch, Spectroscopic Engineering toward Near-Infrared Absorption of Materials Containing Perylene Diimide, ChemPlusChem, 2017, 82, 1359–1364 CrossRef CAS PubMed
.
- S. M. Barbon and J. B. Gilroy, Boron Difluoride Formazanate Copolymers with 9,9-Di-n-Hexylfluorene Prepared by Copper-Catalyzed Alkyne–Azide Cycloaddition Chemistry, Polym. Chem., 2016, 7, 3589–3598 RSC
.
- J. S. Dhindsa, R. R. Maar, S. M. Barbon, M. O. Avilés, Z. K. Powell, F. Lagugné-Labarthet and J. B. Gilroy, A π-Conjugated Inorganic Polymer Constructed from Boron Difluoride Formazanates and Platinum(II) Diynes, Chem. Commun., 2018, 54, 6899–6902 RSC
.
- J. S. Dhindsa, A. Melenbacher, S. M. Barbon, M. J. Stillman and J. B. Gilroy, Altering the Optoelectronic Properties of Boron Difluoride Formazanate Dyes via Conjugation with Platinum(II)-Acetylides, Dalton Trans., 2020 10.1039/C9DT03417J
.
- R. R. Maar, S. M. Barbon, N. Sharma, H. Groom, L. G. Luyt and J. B. Gilroy, Evaluation of Anisole-Substituted Boron Difluoride Formazanate Complexes for Fluorescence Cell Imaging, Chem. – Eur. J., 2015, 21, 15589–15599 CrossRef CAS PubMed
.
- R. R. Maar, R. Zhang, D. G. Stephens, Z. Ding and J. B. Gilroy, Near-Infrared Photoluminescence and Electrochemiluminescence from a Remarkably Simple Boron Difluoride Formazanate Dye, Angew. Chem., Int. Ed., 2019, 58, 1052–1056 CrossRef CAS PubMed
.
- J. B. Gilroy and E. Otten, Formazanate Coordination Compounds: Synthesis, Reactivity, and Applications, Chem. Soc. Rev., 2020, 49, 85–113 RSC
.
- S. M. McAfee, A.-J. Payne, S. V. Dayneko, G. P. Kini, C. E. Song, J.-C. Lee and G. C. Welch, A Non-Fullerene Acceptor with a Diagnostic Morphological Handle for Streamlined Screening of Donor Materials in Organic Solar Cells, J. Mater. Chem. A, 2017, 5, 16907–16913 RSC
.
- S. M. McAfee, A.-J. Payne, A. D. Hendsbee, S. Xu, Y. Zou and G. C. Welch, Toward a Universally Compatible Non-Fullerene Acceptor: Multi-Gram Synthesis, Solvent Vapor Annealing Optimization, and BDT-Based Polymer
Screening, Sol. RRL, 2018, 2, 1800143 CrossRef
.
- D. Zomerman, J. Kong, S. M. McAfee, G. C. Welch and T. L. Kelly, Control and Characterization of Organic Solar Cell Morphology Through Variable-Pressure Solvent Vapor Annealing, ACS Appl. Energy Mater., 2018, 1, 5663–5674 CAS
.
- A.-J. Payne, N. A. Rice, S. M. McAfee, S. Li, P. Josse, C. Cabanetos, C. Risko, B. H. Lessard and G. C. Welch, Donor or Acceptor? How Selection of the Rylene Imide End Cap Impacts the Polarity of π-Conjugated Molecules for Organic Electronics, ACS Appl. Energy Mater., 2018, 1, 4906–4916 CrossRef CAS
.
- A.-J. Payne, S. Li, S. V. Dayneko, C. Risko and G. C. Welch, An Unsymmetrical Non-Fullerene Acceptor: Synthesis via Direct Heteroarylation, Self-Assembly, and Utility as a Low Energy Absorber in Organic Photovoltaic Cells, Chem. Commun., 2017, 53, 10168–10171 RSC
.
- M. E. Farahat, C.-S. Tsao, Y.-C. Huang, S. Hsiung Chang, W. Budiawan, C.-G. Wu and C.-W. Chu, Toward Environmentally Compatible Molecular Solar Cells Processed from Halogen-Free Solvents, J. Mater. Chem. A, 2016, 4, 7341–7351 RSC
.
- G. L. Schulz and S. Ludwigs, Controlled Crystallization of Conjugated Polymer Films from Solution and Solvent Vapor for Polymer Electronics, Adv. Funct. Mater., 2017, 27, 1603083 CrossRef
.
- S. B. Srivastava, S. K. Srivastava and S. P. Singh, Molecular-Shape-Induced Efficiency Enhancement in PC61BM and PC71BM Based Ternary Blend Organic Solar Cells, J. Phys. Chem. C, 2017, 121, 17104–17111 CrossRef CAS
.
- F. Tintori, A. Laventure and G. C. Welch, Additive Induced Crystallization of a Twisted Perylene Diimide Dimer within a Polymer Matrix, Soft Matter, 2019, 15, 5138–5146 RSC
.
Footnote |
† Electronic supplementary information (ESI) available. See DOI: 10.1039/d0qm00109k |
|
This journal is © the Partner Organisations 2020 |
Click here to see how this site uses Cookies. View our privacy policy here.