DOI:
10.1039/D1RA06239E
(Paper)
RSC Adv., 2021,
11, 30041-30045
Efficient artificial light-harvesting system constructed from supramolecular polymers with AIE property†
Received
17th August 2021
, Accepted 26th August 2021
First published on 8th September 2021
Abstract
Supramolecular luminescent materials in water have attracted much interest due to their excellent tunability, multi-color emission, and environment-friendly behavior. However, hydrophobic chromophores are often affected by poor solubility and aggregation-caused quenching effects in aqueous media. Herein, we report a water-phase artificial light-harvesting system based on an AIE-type supramolecular polymer. Specifically, dispersed nanoparticles in water were prepared from an AIE chromophore-bridged ditopic ureidopyrimidinone (M) based supramolecular polymer with the assistance of surfactants. By co-assembling the hydrophobic chromophores NDI as energy acceptor into the nanocarriers, artificial light-harvesting systems (M–NDI) could be successfully constructed, exhibiting efficient energy transfer and high antenna effects. Furthermore, the spectral emission of the system could be continuously tuned with a relatively small number of acceptors. This work develops an efficient supramolecular light-harvesting system in water, which has potential applications in dynamic luminescent materials.
Introduction
Solar energy harvesting and transfer are key points during the photosynthesis process.1 The natural light-harvesting systems (LHSs) are complex supramolecular assemblies, in which large numbers of chromophores need to be densely stacked for high photosynthetic efficiency.2 In artificial systems, scientists usually designed special scaffolds to accommodate chromophores to avoid aggregation-caused quenching (ACQ) and enhance Förster resonance energy transfer (FRET) between chromophores.3 Such scaffolds are ranging from macrocycles,4 DNA,5 and cyclic peptides.6 The introduction of aggregation-induced emission fluorogens (AIEgens) into this research field greatly simplifies the construction process of special scaffolds.7 Specifically, the strategy of combining AIEgens and self-assembly, referred as supramolecular assembly-induced emission (SAIE), provides new motivations to develop new AIE materials.8 Inspired by the above concept, a lot of AIE-type light-harvesting materials by mimicking photosynthesis have been fabricated for different applications, such as fluorescent probes,9 luminescent materials,10 bio-imaging,11 and photocatalysis.12
Supramolecular polymers, driven by non-covalent interactions between monomeric building blocks, show outstanding reversibility, degradability, and stimuli-responsiveness, exhibiting broad potential applications in environmental and materials science.13 Supramolecular polymerization is an excellent strategy for constructing LHSs, which not only can assemble chromophores together to pack tightly but also can enhance SAIE of the chromophores.14 Among different types of non-covalent interactions, ureidopyrimidinone (UPy)-based quadruple hydrogen bonding (QHB) displays great potential on account of its strong binding constant and self-complementary.15 In our previous studies, QHB has been used by us to construct various supramolecular polymers16 and other supramolecular architectures.17 In this work, we present the construction of a light-harvesting system by employing QHB supramolecular polymers with the AIE property.
The building block of the supramolecular polymer is a fixed tetraphenylethylene (FTPE)18 group bridged ditopic UPy monomer (M), which also served as the energy donor (Fig. 1). Herein, the FTPE group endows M with SAIE behavior, while the UPy motif provides M with supramolecular polymerization capability via QHB. As a result, M can form AIE-type supramolecular polymers in organic solvents, such as chloroform or dichloromethane (DCM). Furthermore, the supramolecular polymer can be further self-assembled into water-dispersed NPs by using the mini-emulsion method based on the surfactant cetyltrimethyl ammonium bromide (CTAB). Moreover, by loading the synthesized dye NDI as energy acceptor to the NPs, an artificial LHS in aqueous media could be successfully fabricated. Due to the FRET process between M and NDI, the aqueous NPs were endowed with efficient light-harvesting capability and tunable emission. In our system, each component plays multiple roles to minimize the complexity of the system. For example, M is not only a supramolecular polymerization unit, but also a light harvesting antenna and energy donor. CTAB not only acts as a surfactant, but also mimics membrane lipids in natural photosynthesis. NDI has the dual-role of collecting energy from M and achieving long-wavelength emission simultaneously. Therefore, the M–NDI system appeared to be an interesting light-harvesting platform with great potentials in luminescent materials and bioimaging agents.
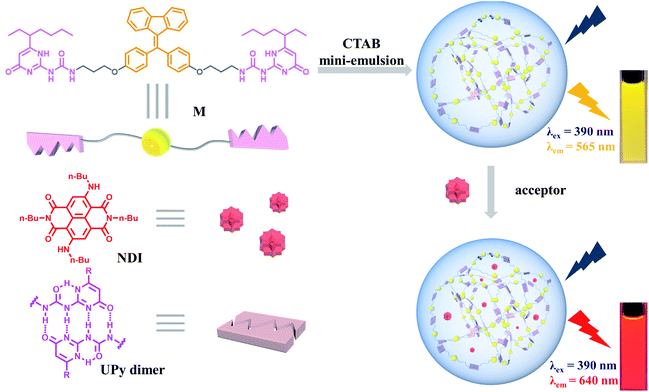 |
| Fig. 1 Schematic illustration of the construction of light-harvesting NPs based on M and NDI. | |
Results and discussion
Monomer M can be obtained by condensation of A and B in dry CHCl3 at room temperature (Scheme 1 and Fig. S7–S9, ESI†). Precursor A was synthesized according to our previous report,19 while intermediate B was prepared according to literature report.20 The association of M monomers through QHB was confirmed by 1H NMR. The characteristic N–H signals occurred in the down field region (between 10.0 and 14.0 ppm) indicates that the UPy units are dimerized by QHB (Fig. S7, ESI†). Through the logarithmic curve of specific viscosity versus monomer concentration, it can be found that the slope (2.02) has not changed (Fig. S1, ESI†), indicating that M can form a supramolecular polymer when the concentration is very low (<2 mM).
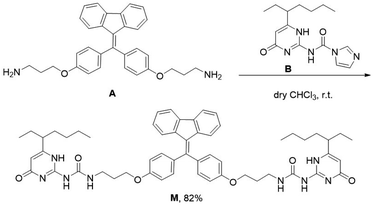 |
| Scheme 1 Synthesis of M. | |
In order to study the AIE property of M, the fluorescence spectra of M in mixed hexane/DCM solutions were studied. It showed no emission when M was dissolved in the pure good solvent DCM (Fig. 2a). When increasing the poor solvent hexane to 92%, a moderate emission was shown. As hexane increased to 98%, the intensity of the spectrum shows an enhanced increase. By utilizing a mini-emulsion approach, we fabricated water-dispersed NPs from supramolecular polymer of M. 50 μL solution of M ([M] = 10 mM) in DCM was added to an aqueous solution of CTAB (10 mL, [CTAB] = 1 mM), followed by ultrasonication for 30 min to produce the NPs. The obtained NPs solution of M ([M] = 5 × 10−5 M) showed an obvious Tyndall effect, suggesting the existence of large number of nano-assemblies (Fig. 2b, inset (2)). By contrast, in the solution of M in DCM no Tyndall effect was observed, indicating that M was fully dissolved in the solvent (Fig. 2b, inset (1)). Moreover, the solution of M NPs displayed a bright yellow fluorescence under a UV lamp, suggesting that the SAIE behavior was occurring (Fig. 2b, inset (4)). The absolute fluorescence quantum yield of the sample was measured to be 2.91% (Fig. S3a, ESI†). On the contrary, the solution of M in DCM has no fluorescence under a UV irradiation (Fig. 2b, inset (3)). Meantime, the fluorescence measurements of M in different forms showed that there is no emission spectrum in DCM, but there is a strong emission spectrum in aqueous media (Fig. 2b). The strong emission of M NPs in water is owing to the restrict motion of FTPE group inside the NPs. These above results indicate that supramolecular polymeric NPs of M have been successfully constructed with the help of CTAB in water.
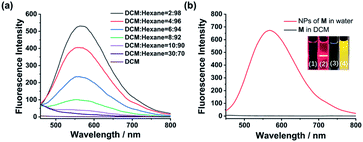 |
| Fig. 2 (a) Fluorescence spectra of M versus hexane fraction in DCM/hexane mixtures. (b) Fluorescence spectra of molecule M in DCM and NPs of M in water upon excitation at 390 nm. Insets: the Tyndall effect of (1) M in DCM and (2) NPs of M in water; photographs of (3) M in DCM and (4) NPs of M in water under UV lamp irradiation. [M] = 5 × 10−5 M. | |
The size and morphology of the NPs were further characterized by dynamic light scattering (DLS) and transmission electron microscopy (TEM). DLS of the M NPs showed that they are well-defined nanoaggregates with the Z-average hydrodynamic diameter of 180–200 nm (Fig. 3a). The TEM image of the M NPs displayed a regular spherical morphology with diameters about 150 nm, good agreement with the DLS data (Fig. 3b). The hydrophobic micro-environment provided by CTAB and the entangled supramolecular polymers resulted in the efficient encapsulation of the acceptor molecules, which could be loaded into the NPs by mini-emulsifying them simultaneously with M. The morphology of the obtained M–NDI NPs was then also characterized by DLS and TEM (Fig. 3c and d). Compared with the M NPs, the NDI-loaded one also exhibit well-defined spherical shape, but are larger in size, which might be due to the hydrophobicity of the acceptor fluorophore.
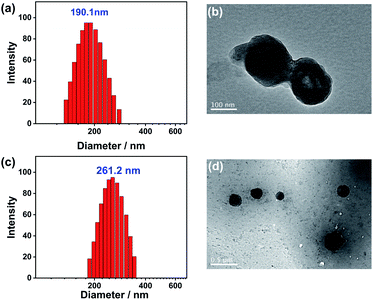 |
| Fig. 3 DLS data of (a) NPs of M and (c) NPs of M–NDI in water at 25 °C. TEM images of (b) NPs of M and (d) NPs of M–NDI ([M] = 5 × 10−5 M, [NDI] = 5 × 10−7 M). | |
The light-harvesting properties of the NDI-loaded NPs were further investigated. As shown in Fig. 4a, the absorption wavelength of NDI overlaps well with the emission peak of the M NPs, making the possibility of efficient FRET between the M (donor, D) and NDI (acceptor, A). Upon the addition of NDI to the NPs, when excited at 390 nm the fluorescence intensity of M at 565 nm dramatically decreased. Meantime, the emission peak of NDI at 640 nm continuously increased (Fig. 4b). Moreover, a remarkable change in fluorescence color from yellow to red was observed (Fig. 3c, inset). Furthermore, the absolute fluorescence quantum yield of M–NDI (A/D = 1%) exhibited a remarkable increase (13.35%, Fig. S3b, Table S2, ESI†), which might be because in this ratio NDI can harvest most of the excitation energy from M and subsequently emit as much as possible. To further confirm the occurrence of the energy-transfer process, time-resolved fluorescence spectroscopy was investigated. The fluorescence lifetime of the M NPs (Fig. 3c) was fitted as a double exponential decay, which showed τ1 = 1.61 ns and τ2 = 6.61 ns when monitored at 565 nm (Fig. S2, Table S1, ESI†). On the contrary, in the M–NDI system (A/D = 1%), the fluorescence lifetimes decreased to τ1 = 0.60 ns and τ2 = 4.87 ns respectively when monitored at 565 nm, indicating that the excitation energy was indeed transferred from the donor M to the acceptor NDI and that the M–NDI LHS had been successfully fabricated. In order to evaluate the capability of M–NDI LHS, both the energy-transfer efficiency (ΦET) and the antenna effect (AE) were further investigated. Notably, ΦET represents the absorbed donor energy by the acceptor compared to the overall excitation energy of the donor; while AE is the amplification factor of the acceptor fluorescence when exciting the donor instead of directly exciting the acceptor. The ΦET of such system was 58.2% when co-assembling 1% NDI with M (Fig. S4 and Table S3, ESI†). Notably, the ΦET values gradually decreased with the decrease of NDI ratio (Fig. 4). Moreover, an AE value of 16.0-fold emission enhancement was observed when M/NDI = 500/1 (Fig. 4, Fig. S5, and Table S4, ESI†), indicating that the obtained M–NDI NPs can serve as an efficient LHS in aqueous media. We also carried out a control experiment. By employing the precursor A which bears FTPE core but without UPy group as antenna and energy donor (Fig. S6, ESI†), very poor energy transfer efficiencies and antenna effects for the system were observed, indicating that the quadruple hydrogen bonded supramolecular polymer are crucial for fabricating the LHSs.
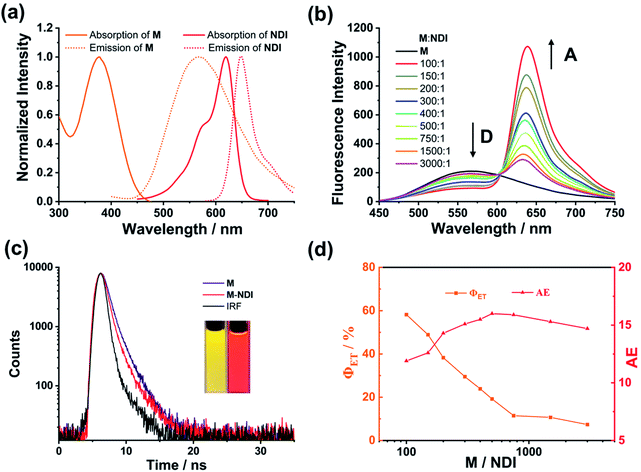 |
| Fig. 4 (a) Normalized absorption spectra (solid line) and emission spectra (dashed line) of M and NDI, respectively. (b) Fluorescence spectra of NPs of M in water with different concentrations of NDI, [M] = 5 × 10−5 M. (c) Fluorescence decay profiles of NPs of M and M–NDI, [M] = 5 × 10−5 M, [NDI] = 5 × 10−7 M. (d) Energy transfer efficiency (ΦET) and antenna effect (AE) at different M/NDI ratios. | |
The efficient M–NDI LHS creates a promising method to tune the fluorescence of the NPs in aqueous media. The system shows a ratio-dependent emission color change. As shown from the CIE 1931 chromaticity diagram (Fig. 5a), the NPs of M locates in the yellow area without the energy acceptor NDI. However, with the ratio of NDI increased from 3000
:
1 to 100
:
1, the fluorescence color of the LHS in water changed from yellow through orange to bright red gradually (Fig. 5b and c), indicating that an efficient energy transfer process had happened from the energy donor M to the energy acceptor NDI. It should be noting that this energy-transfer materials can be stored in water for a long time (1–2 weeks) without photobleaching. The reason for this is not only due to the efficient AIE property induced by FTPE group in M, but also because of the high fidelity of the QHB supramolecular polymers which was wrapped and stabilized by CTAB micelles.
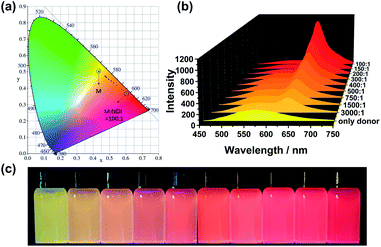 |
| Fig. 5 (a) The CIE chromaticity diagram of photoluminescence color changes by varying the ratios of chromophores. (b) The fluorescence spectra of M in water with different concentrations of NDI. (c) Photographs of NPs in water with different M–NDI ratios. [M] = 5 × 10−5 M. | |
Conclusions
In summary, we have fabricated an efficient artificial energy-transfer light-harvesting system in aqueous media based on supramolecular polymer. The supramolecular polymer was constructed by an FTPE group bridged ditopic UPy molecule M through quadruple hydrogen bonding. With the assistance of CTAB, the supramolecular polymers can further form stable nanoparticles in water by mini-emulsion method. By co-assembling hydrophobic fluorescent dye NDI simultaneously, an efficient artificial light-harvesting system M–NDI could be successfully constructed based on FRET process. By taking the advantages of SAIE, the ratio of donor and acceptor in nanoparticles could be easily controlled and the emission of the materials could be easily tuned with efficient energy-transfer and antenna effect. The composition of the system is relatively simple, and each module shows multi-functions, which provides the possibility for practical application. Therefore, the aqueous light-harvesting system developed here may have potential applications in dynamic luminescent materials and bio-imaging area.
Conflicts of interest
There are no conflicts to declare.
Acknowledgements
We gratefully thank the financial support from the National Natural Science Foundation of China (21702020).
Notes and references
-
(a) D. I. Arnon, Nature, 1959, 184, 10–21 CrossRef CAS PubMed;
(b) D. Gust and T. A. Moore, Science, 1989, 244, 35–41 CrossRef CAS PubMed;
(c) N. S. Wigginton, Science, 2016, 352, 1185–1186 Search PubMed.
-
(a) S. Guo, Y. Song, Y. He, X. Y. Hu and L. Wang, Angew. Chem., Int. Ed., 2018, 57, 3163–3167 CrossRef CAS PubMed;
(b) J. J. Li, Y. Chen, J. Yu, N. Cheng and Y. Liu, Adv. Mater., 2017, 29, 1701905 CrossRef PubMed;
(c) Z. Xu, S. Peng, Y. Y. Wang, J. K. Zhang, I. Lazar Alexandra and D. S. Guo, Adv. Mater., 2016, 28, 7666–7671 CrossRef CAS PubMed.
-
(a) Y.-X. Hu, W.-J. Li, P.-P. Jia, X.-Q. Wang, L. Xu and H.-B. Yang, Adv. Opt. Mater.,, 2020, 8, 2000265 CrossRef CAS;
(b) T. Xiao, W. Zhong, L. Zhou, L. Xu, X.-Q. Sun, R. B. P. Elmes, X.-Y. Hu and L. Wang, Chin. Chem. Lett., 2019, 30, 31–36 CrossRef CAS.
-
(a) K. Acharyya, S. Bhattacharyya, H. Sepehrpour, S. Chakraborty, S. Lu, B. Shi, X. Li, P. S. Mukherjee and P. J. Stang, J. Am. Chem. Soc., 2019, 141, 14565–14569 CrossRef CAS PubMed;
(b) A. Sautter, B. K. Kaletaş, D. G. Schmid, R. Dobrawa, M. Zimine, G. Jung, I. H. M. van Stokkum, L. De Cola, R. M. Williams and F. Würthner, J. Am. Chem. Soc., 2005, 127, 6719–6729 CrossRef CAS PubMed;
(c) B. Valeur, E. Bardez, J.-M. Lehn, L. Jullien and J. Canceill, Angew. Chem., Int. Ed., 1995, 33, 2438–2439 CrossRef;
(d) J. Pruchyathamkorn, W. J. Kendrick, A. T. Frawley, A. Mattioni, F. Caycedo-Soler, S. F. Huelga, M. B. Plenio and H. L. Anderson, Angew. Chem., Int. Ed., 2020, 59, 16455–16458 CrossRef CAS PubMed.
-
(a) P. Ensslen and H.-A. Wagenknecht, Acc. Chem. Res., 2015, 48, 2724–2733 CrossRef CAS PubMed;
(b) P. K. Dutta, R. Varghese, J. Nangreave, S. Lin, H. Yan and Y. Liu, J. Am. Chem. Soc., 2011, 133, 11985–11993 CrossRef CAS PubMed.
-
(a) Q. Song, S. Goia, J. Yang, S. C. L. Hall, M. Staniforth, V. G. Stavros and S. Perrier, J. Am. Chem. Soc., 2021, 143, 382–389 CrossRef CAS PubMed;
(b) T. Wang, X. Fan, J. Xu, R. Li, X. Yan, S. Liu, X. Jiang, F. Li and J. Liu, ACS Macro Lett., 2019, 8, 1128–1132 CrossRef CAS.
-
(a) Y. Hong, J. W. Y. Lam and B. Z. Tang, Chem. Soc. Rev., 2011, 40, 5361–5388 RSC;
(b) M. Cheng, C. Qian, Y. Ding, Y. Chen, T. Xiao, X. Lu, J. Jiang and L. Wang, ACS Macro Lett., 2020, 2, 425–429 CrossRef CAS;
(c) F. Würthner, Angew. Chem., Int. Ed., 2020, 59, 14192–14196 CrossRef PubMed.
-
(a) N. Song, D. X. Chen, M. C. Xia, X. L. Qiu, K. Ma, B. Xu, W. Tian and Y. W. Yang, Chem. Commun., 2015, 51, 5526–5529 RSC;
(b) H. Wu and T. Xiao, Front. Chem., 2020, 8, 610093 CrossRef CAS PubMed;
(c) T. Xiao, L. Zhang, H. Wu, H. Qian, D. Ren, Z.-Y. Li and X.-Q. Sun, Chem. Commun., 2021, 57, 5782–5785 RSC;
(d) X. Z. Yan, T. R. Cook, P. Wang, F. H. Huang and P. J. Stang, Nat. Chem., 2015, 7, 342–348 CrossRef CAS PubMed.
-
(a) Z. Liu, X. Sun, X. Dai, J. Li, P. Li and Y. Liu, J. Mater. Chem. C, 2021, 9, 1958–1965 RSC;
(b) M. Su, Y.-N. Jing, H. Bao and W.-M. Wan, Mater. Chem. Front., 2020, 4, 2435–2442 RSC;
(c) L. Ji, Y. Sang, G. Ouyang, D. Yang, P. Duan, Y. Jiang and M. Liu, Angew. Chem., Int. Ed., 2019, 58, 844–848 CrossRef CAS PubMed.
-
(a) D. Li, J. Wang and X. Ma, Adv. Opt. Mater., 2018, 6, 1800273 CrossRef;
(b) Y. Li, Y. Dong, L. Cheng, C. Qin, H. Nian, H. Zhang, Y. Yu and L. Cao, J. Am. Chem. Soc., 2019, 141, 8412–8415 CrossRef CAS PubMed;
(c) H.-Q. Peng, C.-L. Sun, L.-Y. Niu, Y.-Z. Chen, L.-Z. Wu, C.-H. Tung and Q.-Z. Yang, Adv. Funct. Mater., 2016, 26, 5483–5489 CrossRef CAS;
(d) Q. Wang, Q. Zhang, Q.-W. Zhang, X. Li, C.-X. Zhao, T.-Y. Xu, D.-H. Qu and H. Tian, Nat. Commun., 2020, 11, 158 CrossRef PubMed;
(e) X.-H. Wang, N. Song, W. Hou, C.-Y. Wang, Y. Wang, J. Tang and Y.-W. Yang, Adv. Mater., 2019, 31, 1903962 CrossRef PubMed;
(f) T. Xiao, X. Wei, H. Wu, K. Diao, Z.-Y. Li and X.-Q. Sun, Dyes Pigm., 2021, 188, 109161 CrossRef CAS.
- X. M. Chen, Q. Cao, H. K. Bisoyi, M. Wang, H. Yang and Q. Li, Angew. Chem., Int. Ed., 2020, 59, 10493–10497 CrossRef CAS PubMed.
-
(a) D. Zhang, W. Yu, S. Li, Y. Xia, X. Li, Y. Li and T. Yi, J. Am. Chem. Soc., 2021, 143, 1313–1317 CrossRef CAS PubMed;
(b) X. Li, Y. Wang, A. Song, M. Zhang, M. Chen, M. Jiang, S. Yu, R. Wang and L. Xing, Chin. J. Chem., 2021, 39 DOI:10.1002/cjoc.202100293;
(c) W. J. Li, X. Q. Wang, D. Y. Zhang, Y. X. Hu, W. T. Xu, L. Xu, W. Wang and H. B. Yang, Angew. Chem., Int. Ed., 2021, 60, 18761–18768 CrossRef CAS PubMed;
(d) M. Hao, G. Sun, M. Zuo, Z. Xu, Y. Chen, X. Y. Hu and L. Wang, Angew. Chem., Int. Ed., 2020, 59, 10095–10100 CrossRef CAS PubMed;
(e) Z. Zhang, Z. Zhao, Y. Hou, H. Wang, X. Li, G. He and M. Zhang, Angew. Chem., Int. Ed., 2019, 58, 8862–8866 CrossRef CAS PubMed.
-
(a) T. Aida, E. W. Meijer and S. I. Stupp, Science, 2012, 335, 813–817 CrossRef CAS PubMed;
(b) L. Yang, X. Tan, Z. Wang and X. Zhang, Chem. Rev., 2015, 115, 7196–7239 CrossRef CAS PubMed;
(c) E. Krieg, M. M. C. Bastings, P. Besenius and B. Rybtchinski, Chem. Rev., 2016, 116, 2414–2477 CrossRef CAS PubMed;
(d) T. Xiao, L. Zhou, X.-Q. Sun, F. Huang, C. Lin and L. Wang, Chin. Chem. Lett., 2020, 31, 1–9 CrossRef CAS.
-
(a) D. Dai, Z. Li, J. Yang, C. Wang, J. R. Wu, Y. Wang, D. Zhang and Y. W. Yang, J. Am. Chem. Soc., 2019, 141, 4756–4763 CrossRef CAS PubMed;
(b) P. Wang, X. Miao, Y. Meng, Q. Wang, J. Wang, H. Duan, Y. Li, C. Li, J. Liu and L. Cao, ACS Appl. Mater. Interfaces, 2020, 12, 22630–22639 CrossRef CAS PubMed;
(c) T. Xiao, H. Wu, G. Sun, K. Diao, X. Wei, Z.-Y. Li, X.-Q. Sun and L. Wang, Chem. Commun., 2020, 56, 12021–12024 RSC.
- R. P. Sijbesma, F. H. Beijer, L. Brunsveld, B. J. B. Folmer, J. H. K. K. Hirschberg, R. F. M. Lange, J. K. L. Lowe and E. W. Meijer, Science, 1997, 278, 1601–1604 CrossRef CAS PubMed.
-
(a) L. Qi, Y. Ding, T. Xiao, H. Wu, K. Diao, C. Bao, Y. Shen, Z. Li, X. Sun and L. Wang, Chin. J. Org. Chem., 2020, 40, 3847 CrossRef;
(b) T. Xiao, W. Zhong, L. Qi, J. Gu, X. Feng, Y. Yin, Z.-Y. Li, X.-Q. Sun, M. Cheng and L. Wang, Polym. Chem., 2019, 10, 3342–3350 RSC;
(c) T. Xiao, L. Xu, J. Götz, M. Cheng, F. Würthner, J. Gu, X. Feng, Z.-Y. Li, X.-Q. Sun and L. Wang, Mater. Chem. Front., 2019, 3, 2738–2745 RSC.
-
(a) T. Xiao, W. Zhong, W. Yang, L. Qi, Y. Gao, A. C. H. Sue, Z.-Y. Li, X.-Q. Sun, C. Lin and L. Wang, Chem. Commun., 2020, 56, 14385–14388 RSC;
(b) T. Xiao, L. Xu, J. Wang, Z.-Y. Li, X.-Q. Sun and L. Wang, Org. Chem. Front., 2019, 6, 936–941 RSC.
-
(a) X. Gu, J. Yao, G. Zhang, Y. Yan, C. Zhang, Q. Peng, Q. Liao, Y. Wu, Z. Xu, Y. Zhao, H. Fu and D. Zhang, Adv. Funct. Mater., 2012, 22, 4862–4872 CrossRef CAS;
(b) J.-H. Wang, H.-T. Feng, J. Luo and Y.-S. Zheng, J. Org. Chem., 2014, 79, 5746–5751 CrossRef CAS PubMed;
(c) T. Xiao, L. Zhou, X. Wei, Z. Li and X. Sun, Chin. J. Org. Chem., 2020, 40, 944–949 CrossRef.
- T. Xiao, J. Wang, Y. Shen, C. Bao, Z.-Y. Li, X.-Q. Sun and L. Wang, Chin. Chem. Lett., 2021, 32, 1377–1380 CrossRef CAS.
- H. M. Keizer, R. P. Sijbesma and E. W. Meijer, Eur. J. Org. Chem., 2004, 2004, 2553–2555 CrossRef.
Footnote |
† Electronic supplementary information (ESI) available: Experimental details, additional NMR spectra, and HR-ESI-MS spectra of individual compound. See DOI: 10.1039/d1ra06239e |
|
This journal is © The Royal Society of Chemistry 2021 |
Click here to see how this site uses Cookies. View our privacy policy here.