Ice nucleation imaged with X-ray spectro-microscopy
Received
27th September 2021
, Accepted 7th February 2022
First published on 7th February 2022
Abstract
Ice nucleation is one of the most uncertain microphysical processes, as it occurs in various ways and on many types of particles. To overcome this challenge, we present a heterogeneous ice nucleation study on deposition ice nucleation and immersion freezing in a novel cryogenic X-ray experiment with the capability to spectroscopically probe individual ice nucleating and non-ice nucleating particles. Mineral dust type particles composed of either ferrihydrite or feldspar were used and mixed with organic matter of either citric acid or xanthan gum. We observed in situ ice nucleation using scanning transmission X-ray microscopy (STXM) and identified unique organic carbon functionalities and iron oxidation state using near-edge X-ray absorption fine structure (NEXAFS) spectroscopy in the new in situ environmental ice cell, termed the ice nucleation X-ray cell (INXCell). Deposition ice nucleation of ferrihydrite occurred at a relative humidity with respect to ice, RHi, between ∼120–138% and temperatures, T ∼ 232 K. However, we also observed water uptake on ferrihydrite at the same T when deposition ice nucleation did not occur. Although, immersion freezing of ferrihydrite both in pure water droplets and in aqueous citric acid occurred at or slightly below conditions for homogeneous freezing, i.e. the effect of ferrihydrite particles acting as a heterogeneous ice nucleus for immersion freezing was small. Microcline K-rich feldspar mixed with xanthan gum was also used in INXCell experiments. Deposition ice nucleation occurred at conditions when xanthan gum was expected to be highly viscous (glassy). At less viscous conditions, immersion freezing was observed. We extended a model for heterogeneous and homogeneous ice nucleation, named the stochastic freezing model (SFM). It was used to quantify heterogeneous ice nucleation rate coefficients, mimic the competition between homogeneous ice nucleation; water uptake; deposition ice nucleation and immersion freezing, and predict the T and RHi at which ice was observed. The importance of ferrihydrite to act as a heterogeneous ice nucleating particle in the atmosphere using the SFM is discussed.
Environmental significance
Snow, hail, and about two-thirds of rain begins as ice crystals. Therefore, the initial formation of ice crystals, or ice nucleation, in the upper atmosphere is a critical step in the Earth's water cycle. Particles present in the air or within cloud droplets can catalyze ice nucleation in what is called heterogeneous ice nucleation. We studied this with a range of particle types in a newly developed cryogenic environmental cell that allows nano-focused X-ray experiments to be performed while ice nucleation is occurring. We have found that particles of an important mineral in airborne dust, ferrihydrite, aids ice nucleation and so is important for cloud formation. When the concentrations of such particle types are known, our work can be used to accurately predict the amount of ice formed for better representation of clouds in weather and climate models.
|
1 Introduction
Ice formation in the atmosphere is important for accurate representation of the hydrological cycle,1–3 of stratospheric water vapor,4,5 of multi-phase chemical reactions on ice,6–9 and thus for climate projection overall.10–12 Furthermore, ice plays a large role in precipitation, not only for snow, sleet and hail, but also for rain – about two-thirds of rain begins as ice crystals.13 In mixed-phase clouds where ice particles and liquid droplets co-exist, ice crystals grow and remove water vapor by sedimentation, which can affect cloud lifetime and reflectivity of solar radiation.5 Cirrus clouds are composed entirely of ice crystals and on average, they have a warming effect (positive radiative forcing) due to them being fairly transparent to solar radiation and strongly absorbing terrestrial radiation.14,15 Aerosol particles are playing a key role in the formation of ice. They have varied composition and properties, including organic and inorganic matter, as well as water soluble and insoluble components.16–18 This has led to a wide range of thermodynamic conditions over which they can nucleate ice. Aerosol-cloud interactions can influence Earth's radiation balance, atmospheric temperature, and cloud evolution.5,11,19–21 However, accurate representation of aerosol–ice cloud interactions and any resulting climatic effects requires a significant reduction in the uncertainties of quantifying how aerosol particles influence ice cloud abundance, albedo, height, and especially overall ice particle numbers in clouds.5,21
Predicting atmospheric ice cloud formation from aerosol particles is difficult, partly because ice can form in different ways depending on the aerosol composition and phase.20,21 Homogeneous ice nucleation can occur from micrometer sized liquid cloud droplets and aqueous aerosol particles below about 235 K.22–25 Heterogeneous ice nucleation in the atmosphere can occur from the liquid or the vapor phase of water on aerosol particles, termed ice nucleating particles (INPs). Furthermore, the ability to form ice can be highly variable between different substrates including insoluble particles, crystalline particles, biogenic particles or macromolecules, compressed surfactants on droplets and highly viscous (or glass-like) solid aerosol particles.20,23,26–28 Atmospheric particles experience continuously changing relative humidity with respect to water, RHw, and temperature, T, for a wide range of water vapor pressures. Therefore, knowing the precise RHw and T that define which phase transitions to ice are dominant is of great value. Immersion freezing (liquid-to-ice) is a heterogeneous ice nucleation mode that describes liquid water in an aqueous aerosol or cloud droplet initiating freezing on a particle surface immersed within the liquid. Another heterogeneous ice nucleation mode is deposition ice nucleation (vapor-to-ice), whereby water molecules from the supersaturated vapor phase nucleate ice and subsequently form an ice crystal on a particle surface without the involvement of a bulk liquid aqueous phase. Other freezing modes include contact freezing, condensation freezing, pore-condensation freezing or inside-out freezing,29–32 however these are not considered here. Surfactant substrates can nucleate ice heterogeneously,33–37 although, the impact of ambient organic surfactants on ice formation in atmospheric clouds is fairly understudied compared with laboratory based investigations.38 In particular, the kinetics of ice nucleation for immersion freezing have been quantified using a universal approach based on aqueous solution water activity in laboratory and field studies applicable for a variety of substrates previously mentioned including mineral, organic, biological, surfactant, and soot.39–42 Use of water activity in this study to predict freezing is detailed in a later section. It is important to note that an ice nucleating substrate can be solid-like (glassy or highly viscous), but not necessarily water insoluble.20,43–45 In this study, we have aimed to investigate whether deposition ice nucleation, immersion freezing or homogeneous ice nucleation is favored over the other depending on the particle types present.
Iron containing aerosol particles are a subset of mineral dust particles that are abundant46 and found in the residuals of ice crystals.47–50 Laboratory and model studies have revealed that common minerals and clay such as feldspar, a highly important atmospheric ice nucleating particle, kaolinite and other iron containing minerals are ice forming particles.40,51–59 Iron is frequently incorporated into airborne clay mineral particles and can be at about 5 weight percent as inclusions of iron oxy-hydroxides.60 Additionally, iron mass abundance is typically equal to that of calcium and aluminum in fine dust.46 Anthropogenic iron from urban and combustion sources can be present in about 1 in 20 particles61 and make up a high fraction of soluble iron globally, suspected to be due to an abundance of ferrihydrite.62 Ferrihydrite, or colloidal iron oxy-hydroxides, is an amorphous mineral phase responsible for the transition between soluble iron (i.e. iron ions) and hematite or goethite.63 Iron minerals such as hematite and goethite are heterogeneous ice nucleators and their freezing efficiency increases (i.e. freezing can occur at warmer temperatures) when milled or when particle surface area is increased by other chemical or physical treatments.64,65 The authors of these studies explain their results with milled and unmilled particles by correlating the freezing temperatures with differences in OH group surface concentration and arrangement, in addition to the degree of ice crystal lattice-match.64,65
Ferrihydrite is an amorphous iron oxy-hydroxide. In general, iron oxy-hydroxides amount to more than half of all iron in Asian and African dust aerosol particles,66,67 and to a quarter of iron containing particles from oil combustion.66 Although it is thermodynamically unstable,68,69 ferrihydrite is ubiquitous in nature70 and can remain untransformed for a few hundred days in the atmosphere.71 This long lifetime has been reportedly due to silica, clay minerals, and a range of organic substances (all present in Saharan soils and dust) that contribute to its stabilization.72 Importantly, ferrihydrite is also considered to be a product from the weathering process of iron containing clays such as illite or smectite69 and indeed present at the surface of such clay minerals.69,73 Unlike goethite and hematite, ferrihydrite has no clear structure and previously observed to be in the form of nanoaggregates.63,74,75 It exists in two types, exhibits 2 or 6 lines in X-ray diffractograms and has no fixed composition.63 Due to its porous nanostructure, ferrihydrite has a high specific surface area and offers a high number of accessible sites for adsorption and reaction. It is also considered to be the main source of bio-available iron, i.e. leached from the mineral and absorbed by organisms and thus is critically important to marine life.63,74,75 Thus, ferrihydrite can significantly impact atmospheric chemistry, iron fertilization in oceans, and in particular, ice cloud formation. Although, its capability to nucleate ice has not previously been identified, likely due to the difficulty in isolating it from ambient air and from other minerals, as well as being difficult to synthesize in the laboratory. Being quite unstable, ferrihydrite is also difficult to accurately identify and characterize in laboratory samples.
Here, we have used a unique pairing between scanning transmission X-ray microscopy coupled to near-edge X-ray absorption fine structure spectroscopy (STXM/NEXAFS) and a new ice nucleation experiment, termed the INXCell, to simultaneously observe the formation of ice in the vicinity of specific particles under tightly controlled temperature and humidity conditions. The composition and chemical state of particles (those that did and did not nucleate ice) were imaged in situ with stability and control of RHw and RHi, which was not previously achievable in a STXM experiment. Environmental scanning electron microscopy (ESEM) has previously been used for imaging ice nucleation in situ.76–78 Although spatial resolution of SEM is superior to STXM, energy-dispersive X-ray spectroscopy used with SEM has inferior chemical selectivity compared with STXM/NEXAFS.79,80 Our method also does not require sample transport or vacuum exposure in between ice nucleation observations and chemical investigation as in offline studies,48,50 eliminating sudden exposure of particles to vast swings of T and RHw. STXM/NEXAFS requires thin (∼100 nm) and delicate silicon nitride windows for an X-ray transparent support for the particles, however this results in very poor survivability of samples for offline studies due to transport, handling and preparation. Our INXCell also facilitates easier identification of single ice nucleating particles. This avoids the difficulty for offline studies due to the differences in orientation, contrast generation and spatial resolution between various microscopes, e.g., optical and X-ray, that can hinder re-identification of particles. These difficulties are evident due the fact that only one particle observed to nucleate ice was ever observed using STXM/NEXAFS offline,50 and ice was only tentatively observed in another X-ray environmental cell without knowledge of the nucleating T or RHi conditions.81 When considering ice nucleation capability, spatial resolution and chemical selectivity, the INXCell is superior to other techniques, e.g. Raman spectro-microscopy, typically having a 1 × 1 μm2 pixel size.82 This is important when investigating submicrometer sized particles or ambient particles that can be highly diverse.
We quantify the ice nucleation ability of bare ferrihydrite, ferrihydrite mixed with citric acid, and K-rich microcline feldspar mixed with xanthan gum. Citric acid was used as a proxy for oxygenated organic material common in atmospheric aerosol particles and has a similar viscosity behavior.83,84 Xanthan gum is a proxy for polymeric or oligomeric compounds common in atmospheric organic particles85,86 and primary marine organic matter.87,88 The glass transition of xanthan gum was previously documented,89 and beneficial to explain the impact on ice nucleation mode when particles are more solid or liquid-like. The INXCell encloses a volume between two X-ray transparent membranes where the local gaseous environment is provided from a controlled external flow and the temperature is controlled by blowing cold nitrogen gas against the membrane supporting the test particles. A platinum wire sensor printed onto the membrane was used to measure the temperature with high precision and accuracy. The pair of membranes provide a window through which the sample volume of INXCell can be observed in situ with STXM/NEXAFS spectroscopy.79,80,90 This allows the composition and chemical state of particles to be mapped in situ, as well as having the stability and control to image particles inside ice crystals and water droplets at saturation with respect to liquid or ice (RHw = 100% or RHi = 100%, respectively). Additionally, we have advanced a previous model for homogeneous ice nucleation and heterogeneous ice nucleation from the vapor phase to complement our experimental results that builds on our previous work.40 This model is designed to derive heterogeneous ice nucleation rates with carefully estimated uncertainties. Together, our new method and model makes it possible to investigate single ice nucleating particles in situ as a function of T, RHw, and RHi.
2 Experimental
The INXCell is based on STXM/NEXAFS,79,80,90 traditional cryogenic cold-stage optical microscopy ice nucleation techniques,91,92 and in general, water uptake and ice formation are imaged and spectroscopically probed.81,93–100 Controlling the environmental conditions with enough precision to navigate the phase changes while also keeping the sample area thin enough for transparency of the soft X-ray beam is very challenging, as illustrated by the fact that only one study has ever tentatively detected ice under controlled conditions,81 and that pure water droplets formed from the vapor phase have never before been observed using STXM/NEXAFS. A key feature in the design of the INXCell was the use of lithographic patterning to fabricate high precision temperature sensors directly onto sample substrates, placing the sensor directly in the measurement region and allowing precise determination of the thermodynamic conditions when ice nucleates on particles within the INXCell. Previous in situ ice nucleation studies with higher spatial resolution than optical microscopy have utilized environmental-scanning electron microscopy (E-SEM), in which a sample is imaged while resting on a thick, cold, and thermally conductive block in a humidified atmosphere.51,76–78,101 These studies have advanced our understanding of how morphological features of individual particles or surfaces can impact freezing and crystallization. However, E-SEM does not have chemical sensitivity, compared to STXM/NEXAFS, which can identify organic coatings and provide information on chemical bonding and metal speciation within individual particles. This is important due to the great chemical complexity that ambient INPs can have.50,78 Both INPs50 and the residuals of ice crystals48 have been probed using X-ray spectro-microscopy in offline analysis. In terms of in situ analysis, previous studies have successfully produced X-ray transparent, layered structures for the purpose of investigating time resolved changes in magnetic domains,102–105 electrochemical investigation106 and calorimetric studies.107,108In situ microreactors have been previously developed and used in the field of atmospheric chemistry and physics for investigating water uptake and phase transformations,81,93–97 and chemical98,99 and photochemical reaction cycling.100,109
2.1 INXCell design and description
Fig. 1 shows a schematic of the INXCell that highlights the two most important design features. The first is a patterned temperature sensor on the surface of the silicon nitride membrane. An image of the sensor is outlined in pink in Fig. 1 and is at the exact position of the particles on which ice nucleates. The sensor is a 40 nm thick and 10 μm wide platinum wire fabricated using standard electron beam lithography (VISTEC EBPG 5000Plus)102,105 and inspired by a previous study.108 Briefly, a 40 nm thick film of platinum was deposited with a serpentine pattern in a PMMA/MMA (poly methyl methacrylate/methyl methacrylate) bilayer resist prepared by electron beam lithography with a direct current magnetron sputter coater (AJA) at a base pressure <2 × 10−8 torr and deposition pressure of 3 mTorr. The platinum and resist were on a 1 mm square silicon nitride window having a 100 nm membrane thickness supported by a 0.2 mm thick silicon frame that was 5 mm square. The exposed resist was developed by immersion in a 1
:
3 by volume mixture of methyl isobutyl ketone and isopropyl alcohol and rinsed in pure isopropyl alcohol. A lift-off step was performed by immersing the patterned membrane in pure acetone to remove the resist and extraneous platinum over layer, but retaining the platinum wire pattern. Contacts extended from the membrane to the supporting silicon frame and were wire bonded (TPT HB05) to a custom printed circuit board102,105 connected to a high precision electrometer (Keithley Series 4200). The sensor resistance was around 600 Ω at room temperature and a sample specific temperature calibration, described in a later section, was performed.
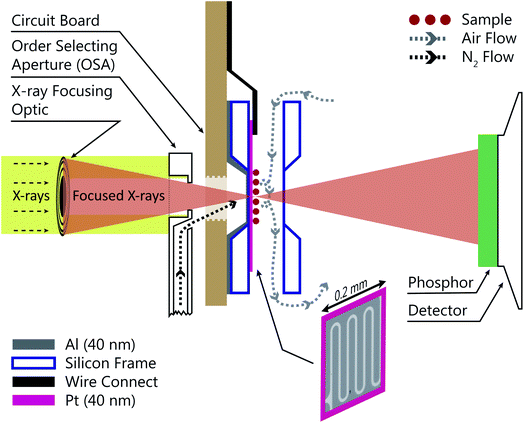 |
| Fig. 1 Sketch of the INXCell configuration. X-rays were focused by a Fresnel zone plate through a temperature controlled order selecting aperture (OSA) with an integrated gas jet. Particles inside the INXCell were exposed to humidified air and cooled by the impinging gas jet from the OSA. The INXCell was mounted on a circuit board (light brown) that was connected to a lithographically fabricated temperature sensor on the sample substrate with a thickness of 40 nm. An optical microscope image of the sensor is outlined in pink. Transmitted X-rays were detected using a phosphor screen coupled with a photomultiplier tube (PMT). Further details are given in the text. When desired, a 40 nm aluminum layer was condensed onto the reverse side (upstream facing) of the membrane to increase the lateral thermal conductivity. The flows of humidified sample air and dry nitrogen is depicted. The sketch is not drawn to scale. | |
The second important design consideration was to ensure that the sample of particles on the silicon nitride membrane was the coldest surface to prevent icing in undesirable locations of the INXCell construction. The requirement for X-ray transparency limits the thickness of the membranes enclosing the cell and supporting the sample particles to about 0.1 μm. This means that the membrane transports heat easily through the thickness of the membrane, but very poorly across its much larger width,108 and so the sample particles near the center of the membrane cannot be efficiently cooled by the cell body. Therefore, an additional form of cooling must be applied from a direction out of the plane of the membrane. This is further hindered by the space restriction of the STXM optics; the Fresnel zone plate (X-ray diffraction focusing optic) requires an order selecting aperture (OSA) positioned within a few hundred micrometers of the focus in order to block the unfocused, zero-order light, while allowing the first-order focus to pass through, as illustrated in Fig. 1. Therefore, we developed a temperature controlled OSA with a gas outlet that delivers a jet of cooled N2 to the front of the cell, removing heat from the center of the membrane and then being pumped from the experiment chamber by a turbomolecular pump. In some instances, a 40 nm layer of Al was deposited on the reverse side of the sample membrane to marginally increase thermal conductivity across its surface. The total pressure inside the cell was maintained at 150 mbar and the vacuum chamber pressure was ∼10−3 mbar. Finally, the body of the INXCell was also cooled and temperature controlled at about 1–2 K warmer than the OSA. It is this two-step cooling design, i.e. cooling of the INXCell body and further cooling of the sample surface utilizing the OSA, resulted in the sample position having the lowest temperature in the gas flow system and being the only area for ice nucleation to occur.
The INXCell chamber dimensions and airflow are identical to previous designs,93,98,99 with minor improvements such as thermally insulating the mounting plate attached from below. Dry helium was used as the carrier gas inside the cell at 20 cm3 min−1 (at equivalent standard temperature and pressure). First, all residual water vapor was removed from the He flow using a liquid nitrogen trap. The flow was split and directed to either a humidifier or a bypass. The temperature controlled humidifier was half-filled with ice held at −16 °C and separated from the air above using a layer of Nafion film. The humidified flow was mixed with the dry bypass flow to achieve a controlled frost point temperature down to about 215 K. Pressure was measured just before the inlet gas flow line of the INXCell and controlled at 150 mbar using a vacuum pump and a proportional-integral-derivative (PID) controlled solenoid valve. Outside of the INXCell in the STXM/NEXAFS vacuum chamber, a 100 cm3 min−1 flow of N2 was directed through the cooled OSA onto the reverse side of the sample substrate. This resulted in a vacuum chamber pressure of ∼10−3 mbar, sufficiently low to minimize the X-ray absorption outside of the gas cell. The N2 flow was pre-dried in the same water vapor trap as for the He flow with an additional pressure regulator.
2.2 Ice nucleation and humidity calibration procedure
The temperature of the INXCell, Tcell, and of the OSA supporting arm, TOSA, was measured with calibrated Pt-100 sensors and controlled using PID modules. Prior to starting any ice nucleation experiments, a temperature calibration of each lithographically patterned Pt sample sensor was performed. To do this, the sample resistance was measured while dry gas was flowing through the INXCell and OSA. The position of the INXCell and OSA was the same as when obtaining a focused STXM image. Then, the OSA and INXCell were cooled to the same T, i.e. TOSA = Tcell and the resistance of the Pt sample sensor, Rp, was measured. Therefore, the temperature of the particles on the membrane, Tp, was equal to TOSA and Tcell, and corresponded to Rp. This was repeated every 10 K over the range 210–290 K to derive a temperature-resistance calibration curve.
To conduct an ice nucleation experiment, TOSA and Tcell were initially set to the same temperature and RHi was set to just under <90%. When a cooling ramp began, Tcell was fixed and TOSA was cooled at a rate of 0.3 K min−1, which led to a change in RHi of about 2.8% min−1 and similar to other methods.92,110 During cooling, we continuously imaged the sample area at a single X-ray energy until ice formed. Ice crystals grew to hundreds of micrometers in size over a few minutes and were clearly identifiable in the STXM images as areas of dark contrast. As soon as ice was detected, TOSA was immediately increased to ∼ 1–3 K warmer than the temperature at which ice nucleated in attempt to stop crystal growth. Water uptake was identified when imaged particles instantaneously and clearly increased in size and X-ray absorption while being cooled. It is notable that these effects were much less pronounced than ice crystal appearance and growth92,110 (as seen in Fig. 4(a) and discussed in a later section).
Calibration of RHi was performed after each observation of ice nucleation following previous optical microscopy studies.91,92 Briefly, STXM images of ice crystals increasing or decreasing in size indicated when RHi was supersaturated (>100%) or subsaturated (<100%), respectively. We determined Tp at which an ice crystal did not change size over multiple images, i.e. for a time of ∼10 min. The measured temperature at which ice crystals did not change size is the so-called frost point temperature, Tfst. Then, we calculated
where
is the saturation vapor pressure over ice as a function of temperature. In some instances, ice nucleation did not occur and instead, only water droplets formed. Then,
where Tdew, is the so-called dew point temperature. The same calibration procedure was implemented except determining the temperature at which water droplets did not grow or shrink, equivalent to Tdew. After calibration, ice crystals were sublimated while continuously being imaged. The residual particles remaining beneath the sublimated crystals were then investigated using the highest spatial and X-ray energy resolution possible. The error on resistance was <±0.3 Ω, which translated to <±0.2 K in T. The uncertainty in both Tdew and Tfst was then chosen as ±0.2 K. This was propagated to
or
and then to the error in RHi found in Table 1. Note, the time between each X-ray image acquisition was about 40 s, corresponding to a systematic uncertainty range in RHi of about 2%, which is far less than the error given in Table 1. Cooling cycles were repeated N times given in Table 1. Before each cycle, particle temperature was increased to 275 K to prevent any effects from preactivation.92,111
Table 1 The relative humidity with respect to ice, RHi, and its uncertainty, σRHi, temperature, T and the water activity criterion, Δaw, at which ice nucleated on average for N freezing cycles having a frozen fraction of particles, f, surface area, A, heterogeneous ice nucleation rate coefficient, Jhet, and the ice crystal count normalized to A, ns
Name |
T/K |
RH
i
|
σ
RHi
|
Δaw |
N
|
f
|
A
|
J
het/cm−2 s−1 |
n
s/cm−2 |
Deposition ice nucleation on ferrihydrite.
Immersion freezing after water uptake on ferrihydrite.
Water uptake on ferrihydrite.
Immersion freezing after water uptake on ferrihydrite/citric acid particles.
Deposition ice nucleation on feldspar/xanthan gum particles.
Immersion freezing after water uptake on feldspar/xanthan gum particles.
Water uptake on feldspar/xanthan gum particles.
|
FHDepa |
231.7 |
131.3 |
±6.6 |
0.210 |
7 |
2 × 10−2 |
2.0 × 10−6 |
4.4 × 102 |
2.6 × 104 |
FHImb |
231.4 |
145.0 |
±6.7 |
0.301 |
1 |
2 × 10−2 |
2.0 × 10−6 |
2.7 × 102 |
1.6 × 104 |
FHIm |
234.9 |
138.5 |
±6.2 |
0.266 |
3 |
2 × 10−2 |
2.0 × 10−6 |
8.2 × 101 |
4.9 × 103 |
FHWUc |
231.6 |
141.5 |
±6.6 |
0.278 |
1 |
— |
2.0 × 10−6 |
— |
— |
FHWU |
235.7 |
127.4 |
±5.7 |
0.191 |
3 |
— |
2.0 × 10−6 |
— |
— |
FHWU |
240.0 |
138.2 |
±6.0 |
0.277 |
1 |
— |
2.0 × 10−6 |
— |
— |
FH + CAImd |
230.1 |
150.3 |
±7.0 |
0.332 |
1 |
1 × 10−5 |
1 × 10−4 |
8.0 × 102 |
4.8 × 104 |
FH + CAIm |
238.1 |
140.7 |
±6.2 |
0.289 |
2 |
1 × 10−5 |
1 × 10−4 |
1.8 × 102 |
1.1 × 104 |
FsXGDepe |
243.9 |
119.0 |
±6.4 |
0.143 |
4 |
6 × 10−3 |
9.6 × 10−6 |
4.6 × 102 |
2.8 × 104 |
FsXGImf |
255.3 |
113.9 |
±6.2 |
0.117 |
2 |
6 × 10−3 |
9.6 × 10−6 |
2.5 × 101 |
1.5 × 103 |
FsXGWUg |
255.8 |
108.3 |
±4.1 |
0.070 |
2 |
— |
9.6 × 10−6 |
— |
— |
2.3 Particle preparation
Ferrihydrite particles were dry deposited on the silicon nitride membrane. Briefly, ferrihydrite synthesis followed an adapted version of Schwertmann's method,112 where 100 ml of Fe(NO3)3 at 0.1 M was prepared in a beaker and titrated by 0.1 M of NaOH under strong stirring until the pH of the solution reached 7.5–8. The product in the form of precipitate was then washed six times with twice its volume of distilled water through a centrifugation procedure. Finally, the product was dried in an oven for 48 h at 40 °C.112 When desired, aqueous citric acid droplets were deposited on top first by nebulizing a 3 weight percent solution, and impacting them on top of the ferrihydrite particles. The solution droplets were generated using an ultrasonic nebulizer.98,99 K-rich feldspar particles mixed with xanthan gum were also dry deposited from a powder. Feldspar was purchased from the Bureau of Analysed Sample Ltd (BCS-CRM no. 376/1 SGT FELDSPAR 1) and sieved through a 250 μm mesh. This was milled again with a ball-milling machine for 5 min and sieved through a 64 μm mesh. A bulk sample was prepared by mixing a small volume of xanthan gum, feldspar and water in equal weight ratio. After drying for about two weeks, the mixture turned to a glass state, which was then crushed into a powder using a mortar and pestle. The nebulizer was cleaned before each use, first by scrubbing using laboratory detergent, then rinsing in distilled and deionized water (resistivity of 18.2 MΩ) and third, soaking in 10% weight percent HCl solution for one hour followed by another rinse prior to use. Tubing and pumps that supplied liquid to the nebulizer were cleaned by circulating the HCl solution for one hour, followed by a rinsing with distilled and deionized water. The mortar and pestle were cleaned following the same procedure. This ensured that undesired compounds were below detection, which was confirmed by generating sodium chloride particles after a cleaning procedure without detecting iron or carbon using STXM/NEXAFS. Confirmation of coatings on mineral particles was performed using STXM/NEXAFS where particles were imaged at the carbon pre-edge at 280.0 eV and either the peak absorption for citric acid at 288.5 eV or xanthan gum at 289.4 eV. X-ray absorption increased uniformly over the particles and appeared slightly enlarged indicating a complete coating. However, STXM images are 2-D and thus we cannot exclude the possibility that some bare feldspar was exposed at the top of the particles. However, we find this unlikely and maintain the presumption that feldspar was completely coated. Future studies should take care to quantify coating amount and heterogeneity. Multiple images of particles were used to derive 2-D projected surface area, which were then translated to 3-D particle surface area estimates assuming spherical geometry. The particle surface area per field of view from multiple images was scaled up to the total sample area estimated to be about 0.16 mm2. Due to time constraints using the synchrotron X-ray beamline, our experiments were limited to the investigation of ferrihydrite particles, ferrihydrite particles coated with citric acid and feldspar coated with xanthan gum. We suggest further experiments on ferrihydrite particles coated with xanthan gum and feldspar particles without any coatings as an addition to the current dataset.
2.4 STXM/NEXAFS procedure
The INXCell was developed and operated at the PolLux beamline of the Swiss Light Source using STXM/NEXAFS.79 X-rays were first focused using a Fresnel zone plate to a minimum pixel size of either 50 or 35 nm at the position of the sample, depending on the specific zone plate used. The custom designed OSA was positioned between the zone plate and sample to block unfocused and unwanted light. The transmission of X-ray photons through the particle was measured and converted to optical density, OD = −
ln(I/I0), where I0 and I are the incident and transmitted photon count, respectively, and with an uncertainty of
and
The sample was scanned over a desired field of view (FOV), while the focused X-rays remained at a fixed position. An image was acquired from hundreds to thousands of individual pixels, where OD was calculated for each pixel. Multiple OD images over the same FOV taken over a range of X-ray energies, referred to here as a “stack”, were aligned and processed using publicly available software.113 We primarily investigated the X-ray energy ranges between 278–320 eV, 525–550 eV and 700–735 eV, which correspond to the carbon K-edge, oxygen K-edge and iron L2,3-edges, respectively.
3 Results and discussion
3.1 Observation of ice nucleation
Ferrihydrite particles are effective heterogeneous INPs. Ice nucleation was observed as a function of T and RHi and shown in Fig. 2. Deposition ice nucleation was observed (when liquid water was not detected) at about 232 K and RHi = 132% seen as the filled blue diamond, which is the average of multiple freezing cycles. Individual RHi and T values for each cycle are shown as small blue open diamonds. Water uptake (open circles) for ferrihydrite particles was observed for only one cycle at T = 231 K, while it was always observed when T = 236 K. Note that water condensation was observed at subsaturated conditions for these temperatures, i.e. RHw < 100%. After water uptake, ice nucleation occurred (filled circles) at or slightly below conditions expected for homogeneous ice nucleation. This result implies that there was a competition between deposition ice nucleation and water uptake. At T = 240 K, ice nucleation was not observed, and water uptake occurred close to RHw = 100%. When ferrihydrite was present with citric acid, ice nucleation was observed at or slightly below conditions for homogeneous freezing when T = 230 K. Water uptake due to citric acid already occurred when RHi < 90% and was not quantified in this study. Additional information about freezing results and particle sample properties are found in Table 1. We compare our results with those of hematite64 having a similar sample surface area shown as small filled diamonds. Note, that these data points are onset conditions, i.e. the RHi and T at which ice and water uptake was first observed. Ice nucleation on hematite occurred at considerably less RHi with roughly the same scatter in the data. Clearly, ice nucleation for ferrihydrite is not so efficient compared to hematite, although its importance to atmospheric ice formation will be evaluated in a later section. Highly concentrated aqueous solutions of citric acid can be highly viscous and kinetically limit water uptake.83,114 The glass transition temperature at RHi = 100% has previously been estimated at 211.8 K (ref. 115) and likely, glassy citric acid is not important in our investigated T and RHi range.
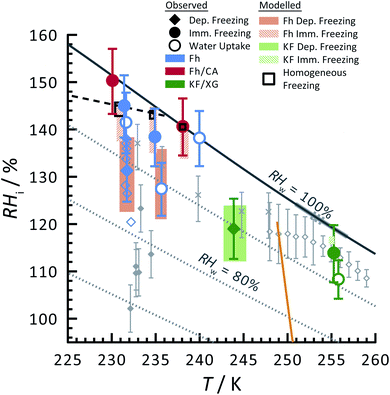 |
| Fig. 2 Ice nucleation observations from ferrihydrite, mixed ferrihydrite and citric acid, and mixed K-feldspar and xanthan gum particles as a function of relative humidity with respect to ice, RHi, and temperature, T. Deposition ice nucleation, immersion freezing and water uptake are indicated by different symbols given in the legend, where each are averages of repeat experiments. An example of individual data points are shown as small open blue diamonds for ferrihydrite deposition ice nucleation. Solid and hashed orange boxes indicate the modelled range for deposition and immersion freezing, respectively, from ferrihydrite. Solid and hashed light green boxes indicate deposition ice nucleation and immersion freezing, respectively, from feldspar. Black open boxes are the modelled range of homogeneous freezing. Grey filled diamonds are freezing due to hematite,64 crosses are freezing of K-feldspar particles with an electrical mobility diameter of 300 nm,58 and gray open diamonds are deposition ice nucleation of K-feldspar particles with diameters between 1–100 μm,51 respectively. The gray solid57 and dashed55 lines are a range of immersion freezing of K-feldspar in water droplets. The orange line is the expected glass transition temperature for aqueous xanthan gum solutions.89 The solid black line indicates water saturation, i.e. when the relative humidity with respect to water, RHw = 100%. Dotted gray lines indicate decreasing RHw by 10%. The black dashed line indicates homogeneous ice nucleation from a single water or aqueous solution droplet ∼ 10 μm in diameter. | |
Feldspar particles coated with xanthan gum nucleated ice via deposition ice nucleation at 244 K. Water uptake was not detected at this temperature. At T = 256 K, however, water uptake occurred at RHw = 91% followed by immersion freezing. A previous study showed that water uptake by xanthan gum at room temperature and RHw = 91% led to a growth in particle diameter by a factor of 1.2 compared to its dry diameter when RHw = 0%. These are concentrated xanthan gum solutions that are highly viscous and exhibit glass transition temperatures of about 256.8 K and 249.9 K when RHw = 11 and 84%, respectively,89 seen as the orange line in Fig. 2 extrapolated to RHw = 95%. It can be expected that the ability for xanthan gum to take up water is kinetically limited to the left of this glass transition line in Fig. 2. We compare our deposition ice nucleation results with those of onset deposition ice nucleation of feldspar particles without any organic present shown as gray crosses58 for particles with a 300 nm electrical mobility diameter and a frozen fraction of about 1 in 104 particles. We also compare with supermicron sized particles, shown as open diamonds.51 There is good agreement between the RHi where deposition ice nucleation was observed for feldspar particles with and without a xanthan gum coating. This implies that either the ice nucleation efficiency of glassy xanthan gum is similar to feldspar, or the xanthan gum did not affect ice nucleation properties of feldspar if water mobility through the xanthan gum was not limited and could access the feldspar particle surface. Ice nucleation studies using xanthan gum particles alone are certainly necessary to verify the former explanation, however, they were not possible due to time constraints. Diffusion coefficients of water through xanthan gum should also be determined as a function of RHw and T to elucidate if deposition ice nucleation was due to the glassy xanthan gum surface or the feldspar beneath. In contrast to our deposition ice nucleation results, immersion freezing was observed after water uptake due to the xanthan gum at RHw = 96%. Therefore, feldspar nucleated ice in aqueous xanthan gum at water subsaturated conditions. Feldspar immersion freezing has been previously shown to commonly follow a freezing point depression,40 meaning that ice nucleation from feldspar in aqueous solution is expected to occur at lower temperature than for particles in pure water. Previous studies on feldspar shown as the solid and dashed gray lines along RHw = 100% in Fig. 2 observed ice nucleation in pure water in agreement with our immersion freezing data considering the experimental error in RHi. The xanthan gum aqueous solution that formed may have been highly dilute. As described later, the immersion freezing data presented here for aqueous solution can be predicted similarly as immersion freezing in pure water using the aqueous solution water activity, aw, which is equivalent to RHw when the solution is in equilibrium with water vapor.22
3.2 X-ray imaging and NEXAFS spectroscopy of water droplets and ice particles
Fig. 3 shows X-ray images acquired at 700.0 and 709.6 eV of a water droplet with a ferrihydrite particle immersed inside at T = 236 K and RHw = 100%. The droplet is visible at both X-ray energies, however, the ferrihydrite particle is only visible in Fig. 3(b) at 709.6 eV, corresponding to the resonant absorption energy for iron(III). Ice formation did not occur at this low temperature while the liquid-vapor equilibrium was maintained for hours, demonstrating our ability to well-control thermodynamic conditions in the INXCell. If ice formed heterogeneously elsewhere on the sample, e.g. on the silicon nitride substrate or the Pt temperature sensor, the vapor pressure decrease would result in rapid evaporation of the droplet. This did not occur and implies that the substrate and Pt wire did not nucleate ice heterogeneously. In the presence of citric acid, droplets always formed followed by ice nucleation.
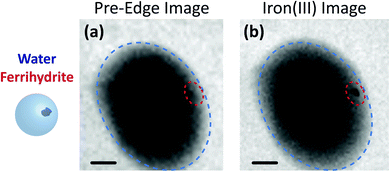 |
| Fig. 3 A water droplet imaged with a ferrihydrite particle immersed inside at 236 K. The image in (a) was taken at the iron pre-edge (700.0 eV) and the image in (b) was taken at the resonant energy (709.6 eV) corresponding to iron(III). The blue and red outlines indicate where the water and ferrihydrite particle are, respectively. The scale bar in both images is 2 μm. | |
Our ability to detect droplet formation, ice nucleation and identify residual particles from sublimating ice crystals is shown in Fig. 4 and 5. During cooling, X-ray optical density images at a coarse resolution were acquired. Our Pt temperature sensor is seen in Fig. 4(a) along with droplets that formed at 231.7 K. It is important to note that the droplets appeared simultaneously across the surface indicating a uniform water vapor distribution and very low temperature gradient within the dashed circled area in Fig. 4(a). In this particular cycle, ice formed 0.2 K lower in temperature seen in Fig. 4(b). The ice crystal grew significantly after cooling by another 0.2 K seen in Fig. 4(c). After a calibration procedure described above, the ice crystal was subjected to slow sublimation. Fig. 5 shows example images during sublimation for an ice crystal that formed on citric acid coated ferrihydrite. The shrinking ice crystals were imaged with a coarse resolution in Fig. 5(a) and (b). Finally, the ice completely sublimated, as seen in Fig. 5(c), and a high spatial resolution image was acquired, as seen in Fig. 5(d). We obtained NEXAFS spectra at the carbon K-edge and iron L2,3-edges shown in Fig. 5(e) and (f), respectively, of the residual particle indicated in the image and a non-residual particle elsewhere on the sample. Both have nearly identical spectral features indicating the presence of ferrihydrite and citric acid. Considering our nanoscale spatial resolution (∼35 nm) and our chemical sensitivity from NEXAFS spectroscopy, we have found no significant difference between these particles. Therefore, it is evident that ice nucleation randomly nucleated on these particles, where larger particles have a greater change to be INPs.
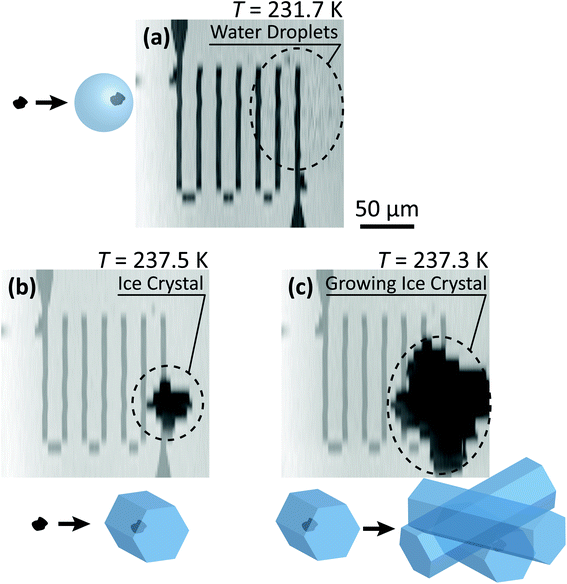 |
| Fig. 4 Example of an INXCell experiment in which (a) water droplets formed, following by (b) ice formation (c) and crystal growth on dry deposited ferrihydrite particles. The temperature, T, of the sample is indicated above the STXM images. The coarse spatial resolution was necessary to quickly image the nucleated and growing ice crystal. | |
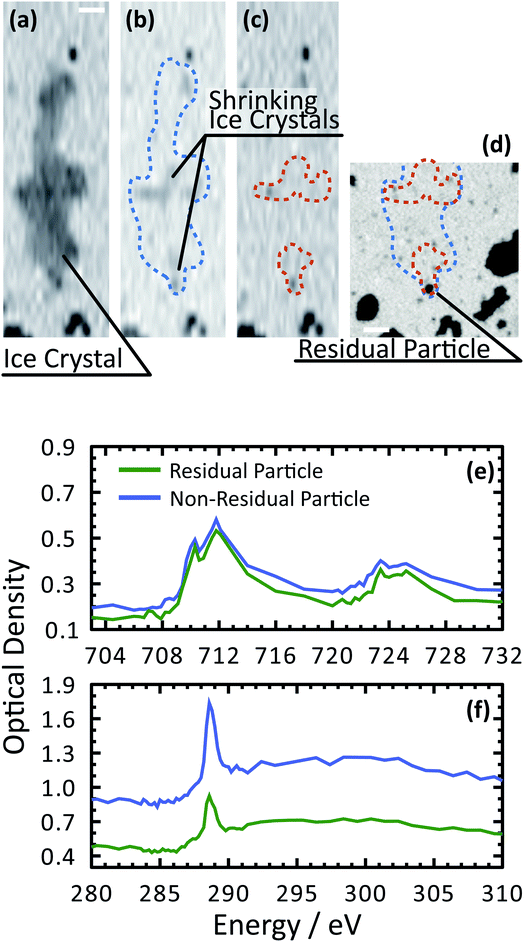 |
| Fig. 5 Demonstration of ice nucleation and spectroscopic identification of ferrihydrite particles coated with citric acid. (a)–(c) A sequence of X-ray images at 280 eV showing the last instances of a sublimating ice crystal. The coarse resolution is necessary to quickly image the shrinking crystal. The blue and orange outline indicates the crystal boundaries. (d) An X-ray image at 288.6 eV showing the crystal boundaries, residual particles after sublimation and organic rich particles across the sample. The scale bar is 2 μm for all images. NEXAFS spectra were acquired first (f) at the carbon K-edge and then (e) the iron L2,3-edges of a residual particle and a non-ice nucleating particle. | |
3.3 Modelling homogeneous and heterogeneous ice nucleation
Ice formation is well-established in nucleation theory, which is used to derive a freezing rate that is crucial to the nucleation process. Water clusters form with increasing numbers of molecules coinciding with increasing free energy until a potential energy barrier is overcome triggering energy release and thus nucleation followed by bulk crystallization.116 The homogeneous ice nucleation rate coefficient, Jhom, with units of cm−3 s−1 predicts water and aqueous solution droplet freezing in the atmosphere.22,24,116,117 The homogeneous ice nucleation rate is ωhom = JhomVd, where Vd is the volume of a droplet. A heterogeneous ice nucleating substrate reduces the energy barrier for ice nucleation, and the heterogeneous ice nucleation rate coefficient is Jhet, with units of cm−2 s−1. From this, the heterogeneous ice nucleation rate, ωhet, scales with the particle surface area, Ap, where ωhet = JhetAp. Immersion freezing from laboratory generated particles, from ambient particles and in atmospheric cloud models has been successfully described using the water activity, aw, of the bulk solution around the immersed particle,39–41,118,119 being either pure water or aqueous solution. The aw-based immersion freezing model (ABIFM) quantifies Jhet over a range of atmospherically relevant T and RHw using aw and shown to be valid thus far for every investigated organic, biogenic and mineral type of particles and for every organic or inorganic solute.39,40,119 Due to its vast applicability for heterogeneous freezing both at and below water saturation, we use the ABIFM to derive Jhet for immersion freezing due to ferrihydrite with and without coatings of citric acid, as well as immersion freezing due to feldspar coated with xanthan gum. Jhet for immersion freezing of feldspar in aqueous solution was previously derived,40 although this was not tested with feldspar in xanthan gum solutions. A aw-based deposition ice nucleation model (ABDINM) was not previously considered, because aqueous solution is thought not to be involved in deposition ice nucleation. Despite this, we use the ABIFM and ABDINM as convenient and reliable functional forms of Jhet for model implementation described below.
For immersion freezing, Jhet,im, as a function of the water activity criterion, Δaw,39,40 is parameterized as
| Jhet,im = mimΔaw(aw,T) + cim, | (1) |
where
mim and
cim are parameters specific to either ferrihydrite or feldspar. The term, Δ
aw, is the difference between solution water activity and the water activity along the ice melting point line at constant
T, or
| Δaw(aw,T) = aw − aiw(T), | (2) |
where
aw is assumed equal to
RHw the particle is exposed to in the INXCell, and
aiw(
T) is the ice–liquid equilibrium curve.
22,120 As previously mentioned, a major advantage of
eqn (1) is that it is independent of the nature of the solute and so is applicable to aqueous solutions of both xanthan gum and citric acid. In other words, a single value of Δ
aw defines a unique point in the
T versus aw phase diagram where
Jhet,im is constant and independent of any solute.
Eqn (1) therefore relates heterogeneous ice nucleation kinetics directly to thermodynamics.
39,40
To represent deposition ice nucleation, we have used the same functional form as eqn (1) where
| Jhet,dep = mdepΔaw(aw,T) + cdep, | (3) |
and
mdep and
cdep are parameters different from those for immersion freezing. We have expanded the stochastic freezing model (SFM) presented in Alpert and Knopf
40 to predict the freezing probability,
Pfrz, of a particle due to immersion freezing, deposition ice nucleation and homogeneous freezing as
| 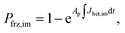 | (4) |
| 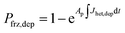 | (5) |
and
| 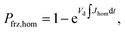 | (6) |
respectively.
Eqn (1)–(3) are used in
eqn (4) and
(5), while
Jhom is taken from literature
22,120 and used in
eqn (6). The integral with respect to time,
t, is included in
eqn (4)–(6) to account for a variable cooling rate,
i.e. T(
t), of the sample material. The updated SFM presented here simulates freezing by single particles,
40 each having a unique
Ap. The uncertainty in quantifying
Ap was previously shown to significantly impact freezing results.
40 Therefore, we derived
Ap and the distribution of particles sizes and total surface area on a sample from measurements of particle sizes on the sample estimated from STXM images. These were used to produce a surface area probability distribution from which the SFM randomly sampled
Ap for one simulation. The temperature in the SFM decreases continuously and after a small temperature step, Δ
T = 0.2 K, freezing of a particle is sampled from a binomial distribution as a Bernoulli trial using a probability parameter from
eqn (4)–(6). A success is considered an ice nucleation event and a single simulation is finished as soon as a freezing event is sampled. We then use a Monte Carlo method by repeating the simulation 10
5 times to determine the average conditions of
T,
RHw and
RHi at which ice occurs and their uncertainty, which is derived from the multiple simulations and from Poisson statistics at 99.9% confidence following Alpert and Knopf.
40 It is important to note that each simulation run will sample a new particle population from the measured size distribution to account for the uncertainty in surface area. Finally, parameters
mim,
mdep,
cim and
cdep were varied to best fit the average thermodynamic conditions at which ice was observed to form, while also matching the standard deviation from multiple cooling cycles.
One of the main goals of modelling ice nucleation using the SFM was to reproduce our results and uncertainties in RHi and T at which freezing was observed. In addition, we evaluated the variability in RHi and T that could be attributed to random freezing. As previously stated, only the first ice nucleation event on a sample with many other particles was observed and due to time constraints, we were limited on the number of repeated cooling cycles (see Table 1) that could be performed. Therefore, the SFM was employed to assess the statistical probability of a single ice nucleation event and compare it to the RHi and T variability.
The SFM was able to reproduce our measured conditions as seen in Fig. 2. Also, the standard deviation of modeled RHi derived entirely from stochastic variability was comparable to the RHi measurement uncertainty. Fitted values were mdep = 12.3525, cdep = 0.0516, mim = 15.0469 and cim = −2.0906 for ferrihydrite particles, and mdep = 13.2251 and cdep = 0.7716 for feldspar particles. Parameters for feldspar particle immersion freezing are mim,feld = 122.83 and cim,feld = −12.98 from Alpert and Knopf.40 New values of Jhet,dep and Jhet,im for both ferrihydrite and feldspar and their error are shown in Fig. 6(a). The SFM was run for deposition, immersion and homogeneous freezing simultaneously, where the latter two were allowed only after water uptake, which was observed for ferrihydrite at about RHw = 94.5%+4.7−4.5. Particles having citric acid or particles with xanthan gum above the glass transition (orange line in Fig. 2) were modelled to always have water and nucleate ice via immersion freezing, while deposition ice nucleation was not allowed. We reiterate that deposition ice nucleation on ferrihydrite without citric acid was observed in 7 out of 8 experiments around T = 232 K (see Table 1), while immersion freezing occurred once. Our model similarly predicted that deposition ice nucleation would occur about 85% of the time in competition with immersion freezing and homogeneous ice nucleation. At around T = 232 K, the SFM predicted that deposition, immersion and homogeneous freezing would occur on ferrihydrite at 75%, 5% and 20%, respectively. In addition, citric acid/ferrihydrite particles formed ice around conditions expected for homogeneous freezing. Although we did observe residual particles after freezing for both ferrihydrite particles and citric acid coated ferrihydrite particles, there remains a possibility that homogeneous ice nucleation may have occurred instead when considering the uncertainty in our observations, i.e. the error bars for immersion freezing overlap with expected homogeneous freezing temperatures. We find that immersion freezing may not be a competing way of nucleating ice for ferrihydrite particles. Instead, we claim that deposition ice nucleation can be important for heterogeneous ice nucleation if water uptake does not occur.
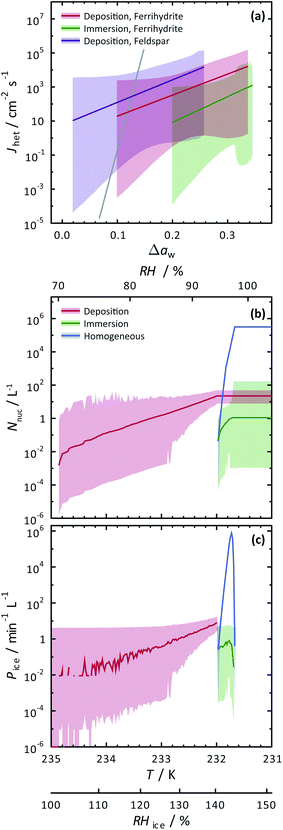 |
| Fig. 6 Calculated heterogeneous ice nucleation rate coefficients, Jhet, ice nucleation events, Nnuc, and ice particle production rates, Pice, are shown. (a) Jhet parameterizations and their certainty at 0.999 confidence are shown as the solid colored lines and shadings. The solid gray line is for immersion freezing due to feldspar from Alpert and Knopf.40 Modelled ice nucleation accounting for deposition ice nucleation, immersion freezing and homogeneous ice nucleation is shown in (b) and (c) from an aerosol population having ferrihydrite and non-ferrihydrite particles. Lognormal distributions with two modes were used with parameters N1 = 285 cm−3, μ1 = 0.05 μm, σ1 = 1.2, N2 = 15 cm−3, μ2 = 0.9 μm and σ2 = 0.5 for non-ferrihydrite particles and N3 = 14 cm−3, μ3 = 0.05 μm, σ3 = 1.2, N4 = 0.8 cm−3, μ4 = 0.9 μm and σ4 = 0.5 for ferrihydrite particles. | |
The SFM prediction of deposition ice nucleation around T = 244 K on mixed feldspar/xanthan gum particles is in good agreement with observations. We find that at subsaturated conditions, deposition ice nucleation may be important for atmospheric ice nucleation from feldspar particles. Deposition ice nucleation was predicted to occur 100% of the time, meaning that RHw = 100% was not reached to allow for droplet formation followed by immersion freezing. This is in agreement with our ice nucleation and water uptake observations. Around T = 255 K, we observed water uptake and immersion freezing below water saturation. Note that Table 1 details the number of repeat cooling cycles for each experiment. The SFM predicted immersion freezing of feldspar is in agreement with our observations, and verified the parameterization from Alpert and Knopf.40 It is important to note that our error bar derived from temperature and humidity uncertainty is much larger than the error from our model derived from stochastic freezing. This is not a disagreement since the SFM does not consider any temperature error, and only implies that stochastic error may not contribute very significantly to the total error in this case.
3.4 Atmospheric importance of ferrihydrite
To evaluate the importance of deposition ice nucleation and immersion freezing due to ferrihydrite particles, or homogeneous freezing, we applied the SFM to a hypothetical aerosol size distribution representative of the concentration of fine particles and coarse mode airborne dust particles.42,121,122 In this exercise, dust particles were arbitrarily set to 5% of the total particles. Using Jhet,dep and Jhet,im for ferrihydrite from Fig. 6(a), and allowing for multiple freezing events we modelled freezing and tracked the ice particle production rates and concentration of ice particles due to all three ways of ice nucleation. Note, that using the SFM in this way does not accurately represent atmospheric ice nucleation as it lacks air mass trajectories, crystal growth, water vapor depletion, cloud structure and other thermodynamic and meteorological processes that impact cloud microphysics. However, it does establish the potential importance of ferrihydrite to atmospheric ice nucleation. Importantly, it also evaluates the competition between immersion, deposition and homogeneous freezing in determining the range of conditions that one may be more important than the other. Further parcel or cloud resolving models would be necessary to give estimates that are more accurate.
Fig. 6(b) and (c) show the results of the SFM employing our representative size distribution with a cooling rate of 0.1 K min−1. The number of ice nucleation events, Nnuc, due to deposition ice nucleation (red lines) reach 10−2 L−1 of air before immersion or homogeneous freezing occurred at a rate, Pi < 10−2 min−1 L−1 near RHi = 100%. These initial freezing events are due to the largest coarse mode particles that have more surface area and thus a higher probability to freeze. At a maximum, Nnuc = 21 L−1 and Pi = 8 min−1 L−1 from deposition ice nucleation alone. Water uptake was modelled at RHw = 94.5%, which was similar to observations for ferrihydrite particles. After water uptake, deposition ice nucleation was not possible and immersion and homogeneous freezing was allowed. Homogeneous freezing contributed far more to the total Nnuc toward the end of the model run than immersion freezing shown in Fig. 6(b). In order to assign an uncertainty to these model predictions, we consider a hypothetical instrument counting ice particles similar to a continuous flow diffusion chamber (CFDC) that samples 1 L min−1 of air with a 10 s resident time and using a 10 min averaging interval. These CFDC instruments are routinely deployed in aircraft studies for measuring INP concentrations.42,123,124 Again, using Poisson statistics at 99.9% confidence, we derive upper and lower fiducial limits125 of Nnuc and Pi seen as the shaded areas. Ambient INPs and ice crystal concentrations are observed from 10−1 to 103 L−1 in atmospheric clouds, however these numbers are highly uncertain in general and not well-known for cirrus clouds.28 We find that low numbers of ice crystals in clouds could be due to deposition ice nucleation from ferrihydrite in dust, but also we claim that these low numbers, and thus low sampling statistics, are the leading cause of the great uncertainty associated with predictions. Reducing the uncertainties would require high volume sampling and long particle residence times in ice nucleation instrumentation to detect high numbers of INPs, which poses a significant experimental challenge. However, despite low numbers of ice nucleation events, careful evaluation of uncertainties, as done here, can still yield increased understanding of ice nucleating particle chemistry and physics. We have demonstrated that ferrihydrite particles have the ability to nucleate ice and should be considered in future deposition ice nucleation studies and derived quantitative values of Jhet and corresponding uncertainties following water activity to predict ice particle production.
Our INXCell and SFM are also well-suited for measuring and modelling the ice nucleation ability of field collected particles in combination with their chemical morphology. Due to the wide variability of particle types, in situ identification and composition mapping of organic coatings and metal oxidation states of atmospheric INPs will give valuable insights on the nature of ice nucleation. In doing so, future studies will be able to discriminate in situ INP types and their composition with discrimination of immersion freezing and deposition ice nucleation. We recommend further investigation on ambient particles using our new INXCell and newly developed SFM. Furthermore, experiments on complex laboratory generated particles using the INXCell would greatly benefit from spectroscopic and composition mapping data. This could be applied in future ice nucleation studies, e.g. on both fully or partially coated particles.
4 Conclusions
Ice nucleation due to ferrihydrite particles with and without citric acid, as well as feldspar with xanthan gum, was observed in a new instrument, referred to as the INXCell, that combines X-ray spectro-microscopy, a humidified environmental chamber and a cryogenic cold stage. This work showed that ice nucleation on single particles could be paired with in situ environmental spectroscopy. In this way, the detection of specific chemical components on and inside single particles could be distinguished such as inorganic material, organic material, iron, and corresponding carbon bonding functionalities and oxidation state. In particular, single ice nucleating ferrihydrite particles were identified through X-ray images and NEXAFS spectroscopy. Ferrihydrite nucleated ice at significantly lower values of RHi than expected for homogeneous ice nucleation. This occurred only when water uptake was completely avoided. When water uptake did occur, ice nucleation occurred at conditions close to homogeneous ice nucleation. One surprising finding was that even at the same humidity conditions, repeat cycles were performed that either did and did not result in observed water uptake. Therefore, heterogeneous ice nucleation via deposition ice nucleation from the water vapor phase was in competition with water uptake onto ferrihydrite particles. This was possibly due to the stochastic nature of heterogeneous ice nucleation, i.e. if ice formation did not occur by chance, then water uptake would eliminate any further chance of deposition ice nucleation. We also observed single ferrihydrite particles immersed inside of supercooled water droplets without any indication of ice nucleation and rapid crystallization. Feldspar deposition ice nucleation in the presence of xanthan gum was observed and was in agreement with previous ice nucleation results at water subsaturated conditions for feldspar ice nucleation studies without xanthan gum. Although xanthan gum is hygroscopic, no water uptake was observed on the mixed particles, likely due to the xanthan gum being a glass at the humidity and temperature at which ice nucleation was observed.
We quantified ice nucleation rate coefficients using observation of T and RHi at which ice formed using our newly developed model, the SFM, and carefully determined corresponding uncertainties. This was an extension of our previously developed immersion freezing model using a Monte Carlo technique.40 This model uses a water activity based description for quantifying freezing kinetics (Jhet) and thus, independent of any solute type present and applicable for both water saturated and water subsaturated conditions. The SFM could reproduce our observations of ice nucleation due to ferrihydrite and feldspar with and without citric acid or xanthan gum aqueous solution and within our experimental error. The range of RHi at which particle froze predicted by the SFM was almost identical to the scatter in our observations. Since the SFM variability is entirely based on a stochastic freezing process, we found that stochastic freezing was the major source of error for deposition ice nucleation and immersion freezing due to ferrihydrite particles and deposition ice nucleation due to feldspar particles. On the other hand, the variability of RHi predicted by the SFM for immersion freezing caused by feldspar was much smaller and thus, experimental temperature error was likely the major source of variability. The fact that stochastic freezing has the ability to explain our data scatter and error gives evidence that heterogeneous ice nucleation due to ferrihydrite and feldspar is dominated by stochastic freezing and validated the use of a nucleation rate coefficient following nucleation theory.
We have also investigated the potential importance of ferrihydrite as an atmospheric ice nucleating particle using our SFM, considering the competition between immersion freezing, deposition ice nucleation and homogeneous ice nucleation. Concentrations of ice particles from deposition ice nucleation were predicted on the order of 101 L−1 when RHi increased up to 145%. These crystal numbers are typical for cirrus cloud formation, and thus, ferrihydrite could potentially be important for atmospheric ice production if water uptake is avoided. Immersion freezing insignificantly contributed to the total ice particle concentration compared to homogeneous freezing, and therefore, immersion freezing of ferrihydrite is concluded to not be important for atmospheric ice production.
This work could only be possible using our new INXCell, which features a platinum resistive temperature sensor that was lithographically patterned onto the sample surface and a novel cryogenic cooling solution. This ensured a high precision measurement of the sample temperature as low as 230 K, demonstrated in this study. The major challenge to overcome was to ensure that the coldest area of the gas flow path throughout the inside of the entire INXCell construction was located at the X-ray transparent silicon nitride membrane where particles sit, and co-located where the temperature of the particles was measured. This yielded unprecedented accuracy and control of temperature and thus humidity. Inside the cell, this means that maximum relative humidity conditions are central, localized, predictable and uniform across a defined sample area. To our knowledge, measurement and equilibration of pure water droplets, i.e. at 100% relative humidity, with and without particle immersed using STXM/NEXAFS has never been done until now. The INXCell is advantageous for future studies of, e.g. phase transitions, chemical reactions, or multiphase chemistry, but potentially in other disciplines such as electrochemistry106 for fuel cell performance,126,127 involving the aqueous phase of liquid water where water saturation is maintained for long time scales.
Data availability
All data are publicly available online at https://doi.org/10.5281/zenodo.6034243. This includes schematic drawings of the order selecting aperture.
Code availability
All codes are publicly available online at https://doi.org/10.5281/zenodo.6034243.
Author contributions
P. A. A. wrote the manuscript. P. A. A., B. W., C. P. and M. A. conceptualized and planned the study. P. A. A. planned and conducted STXM/NEXAFS experiments supervised by B. W., C. P. and M. A., A. B., S. Y., H. Y. and K. K. also conducted STXM/NEXAFS experiments. P. A. A. and S. F. designed the INXCell supervised by B. W., S. F. performed lithography. Z. L. performed sputter coating and characterized the temperature sensors. P. A. A. conducted STXM/NEXAFS data analysis and interpretation of results. P. A. A. wrote and developed the SFM. All co-authors discussed the results and commented on the manuscript.
Conflicts of interest
We declare no conflict of interest.
Acknowledgements
This work was funded by the Swiss National Science Foundation (Grant 163074 and 188662) and by the European Union's Horizon 2020 research and innovation program under the Marie Skłodowska-Curie grant agreement (No 701647). The PolLux end station was financed by the German Ministerium für Bildung und Forschung (BMBF) through contracts 05K16WED and 05K19WE2. We acknowledge Jörg Raabe, Andres Laso and Blagoj Sarafimov for constructing the INXCell and support at the PolLux end station.
References
- U. Lohmann and J. Feichter, Atmos. Chem. Phys., 2005, 5, 715–737 CrossRef CAS.
- U. Lohmann and K. Diehl, J. Atmos. Sci., 2006, 63, 968–982 CrossRef.
- J. Mülmenstädt, O. Sourdeval, J. Delanoë and J. Quaas, Geophys. Res. Lett., 2015, 42, 6502–6509 CrossRef.
- A. W. Brewer, Q. J. R. Meteorol. Soc., 1949, 75, 351–363 CrossRef.
- T. Storelvmo, Annu. Rev. Earth Planet. Sci., 2017, 45, 199–222 CrossRef CAS.
- M. Kräemer, C. Schiller, C. Voigt, H. Schlager and P. J. Popp, Q. J. R. Meteorol. Soc., 2008, 134, 905–912 CrossRef.
- J. N. Crowley, M. Ammann, R. A. Cox, R. G. Hynes, M. E. Jenkin, A. Mellouki, M. J. Rossi, J. Troe and T. J. Wallington, Atmos. Chem. Phys., 2010, 10, 9059–9223 CrossRef CAS.
- T. Bartels-Rausch, H.-W. Jacobi, T. F. Kahan, J. L. Thomas, E. S. Thomson, J. P. D. Abbatt, M. Ammann, J. R. Blackford, H. Bluhm, C. Boxe, F. Domine, M. M. Frey, I. Gladich, M. I. Guzmán, D. Heger, T. Huthwelker, P. Klán, W. F. Kuhs, M. H. Kuo, S. Maus, S. G. Moussa, V. F. McNeill, J. T. Newberg, J. B. C. Pettersson, M. Roeselová and J. R. Sodeau, Atmos. Chem. Phys., 2014, 14, 1587–1633 CrossRef.
- C. D. Holmes, T. H. Bertram, K. L. Confer, K. A. Graham, A. C. Ronan, C. K. Wirks and V. Shah, Geophys. Res. Lett., 2019, 46, 4980–4990 CrossRef CAS.
- M. B. Baker and T. Peter, Nature, 2008, 451, 299–300 CrossRef CAS PubMed.
-
O. Boucher, D. Randall, P. Artaxo, C. Bretherton, G. Feingold, P. Forster, V.-M. Kerminen, Y. Kondo, H. Liao, U. Lohmann, P. Rasch, S. K. Satheesh, S. Sherwood, B. Stevens and X.-Y. Zhang, Clouds and Aerosols, in Climate Change 2013: The Physical Science Basis. Contribution of Working Group I to the Fifth Assessment Report of the Intergovernmental Panel on Climate Change, ed. S. Fuzzi, J. Penner, V. Ramaswamy and C. Stubenrauch, Cambridge University Press, Cambridge, United Kingdom and New York, NY, USA, 2013, ch. 7, pp. 571–657 Search PubMed.
- D. Rosenfeld, M. O. Andreae, A. Asmi, M. Chin, G. de Leeuw, D. P. Donovan, R. Kahn, S. Kinne, N. Kivekäs, M. Kulmala, W. Lau, K. S. Schmidt, T. Suni, T. Wagner, M. Wild and J. Quaas, Rev. Geophys., 2014, 52, 750–808 CrossRef.
- K. M. Lau and H. T. Wu, Geophys. Res. Lett., 2003, 30(24), 2290 CrossRef.
- G. M. McFarquhar, A. J. Heymsfield, J. Spinhirne and B. Hart, J. Atmos. Sci., 2000, 57, 1841–1853 CrossRef.
- B. Kärcher, Curr. Clim. Change Rep., 2017, 3, 45–57 CrossRef.
- C. D. O'Dowd, M. C. Facchini, F. Cavalli, D. Ceburnis, M. Mircea, S. Decesari, S. Fuzzi, Y. J. Yoon and J. P. Putaud, Nature, 2004, 431, 676–680 CrossRef PubMed.
- Q. Zhang, J. L. Jimenez, M. R. Canagaratna, J. D. Allan, H. Coe, I. Ulbrich, M. R. Alfarra, A. Takami, A. M. Middlebrook, Y. L. Sun, K. Dzepina, E. Dunlea, K. Docherty, P. F. DeCarlo, D. Salcedo, T. Onasch, J. T. Jayne, T. Miyoshi, A. Shimono, S. Hatakeyama, N. Takegawa, Y. Kondo, J. Schneider, F. Drewnick, S. Borrmann, S. Weimer, K. Demerjian, P. Williams, K. Bower, R. Bahreini, L. Cottrell, R. J. Griffin, J. Rautiainen, J. Y. Sun, Y. M. Zhang and D. R. Worsnop, Geophys. Res. Lett., 2007, 34, L13801 Search PubMed.
- A. Laskin, M. K. Gilles, D. A. Knopf, B. Wang and S. China, Annu. Rev. Anal. Chem., 2016, 9, 117–143 CrossRef PubMed.
- J. Haywood and O. Boucher, Rev. Geophys., 2000, 38, 513–543 CrossRef CAS.
- D. A. Knopf, P. A. Alpert and B. Wang, ACS Earth Space Chem., 2018, 2, 168–202 CrossRef CAS.
- N. Bellouin, J. Quaas, E. Gryspeerdt, S. Kinne, P. Stier, D. Watson-Parris, O. Boucher, K. S. Carslaw, M. Christensen, A.-L. Daniau, J.-L. Dufresne, G. Feingold, S. Fiedler, P. Forster, A. Gettelman, J. M. Haywood, U. Lohmann, F. Malavelle, T. Mauritsen, D. T. McCoy, G. Myhre, J. Mülmenstädt, D. Neubauer, A. Possner, M. Rugenstein, Y. Sato, M. Schulz, S. E. Schwartz, O. Sourdeval, T. Storelvmo, V. Toll, D. Winker and B. Stevens, Rev. Geophys., 2020, 58, e2019RG000660 CrossRef CAS PubMed.
- T. Koop, B. P. Luo, A. Tsias and T. Peter, Nature, 2000, 406, 611–614 CrossRef CAS PubMed.
- D. A. Hegg and M. B. Baker, Rep. Prog. Phys., 2009, 72, 056801 CrossRef.
- B. J. Murray, S. L. Broadley, T. W. Wilson, S. J. Bull, R. H. Wills, H. K. Christenson and E. J. Murray, Phys. Chem. Chem. Phys., 2010, 12, 10380–10387 RSC.
- B. Kärcher and A. Seifert, Q. J. R. Metereol. Soc., 2016, 142, 1320–1334 CrossRef.
- C. Hoose and O. Möhler, Atmos. Chem. Phys., 2012, 12, 9817–9854 CrossRef CAS.
- B. J. Murray, D. O'Sullivan, J. D. Atkinson and M. E. Webb, Chem. Soc. Rev., 2012, 41, 6519–6554 RSC.
- Z. A. Kanji, L. A. Ladino, H. Wex, Y. Boose, M. Burkert-Kohn, D. J. Cziczo and M. Krämer, Meteor. Mon., 2017, 58, 1–33 Search PubMed.
- N. Hoffmann, D. Duft, A. Kiselev and T. Leisner, Faraday Discuss., 2013, 165, 383–390 RSC.
- N. Fukuta and R. C. Schaller, J. Atmos. Sci., 1982, 39, 648–655 CrossRef.
- R. O. David, C. Marcolli, J. Fahrni, Y. Qiu, Y. A. Perez Sirkin, V. Molinero, F. Mahrt, D. Brühwiler, U. Lohmann and Z. A. Kanji, Proc. Natl. Acad. Sci. U. S. A., 2019, 116, 8184–8189 CrossRef CAS PubMed.
- A. J. Durant and R. A. Shaw, Geophys. Res. Lett., 2005, 32, L20814 CrossRef.
- M. Gavish, R. Popovitz-Biro, M. Lahav and L. Leiserowitz, Science, 1990, 250, 973–975 CrossRef CAS PubMed.
- L. H. Seeley and G. T. Seidler, J. Chem. Phys., 2001, 114, 10464–10470 CrossRef CAS.
- W. Cantrell and C. Robinson, Geophys. Res. Lett., 2006, 33, L07802 CrossRef.
- B. Zobrist, T. Koop, B. P. Luo, C. Marcolli and T. Peter, J. Phys. Chem. A, 2007, 111, 2149–2155 CAS.
- D. A. Knopf and S. Forrester, J. Phys. Chem. A, 2011, 115, 5579–5591 CrossRef CAS PubMed.
-
V. F. McNeill, N. Sareen and A. N. Schwier, in Atmospheric and Aerosol Chemistry, ed. V. McNeill and P. Ariya, Springer, Berlin, Heidelberg, 2014, vol. 339 of Topics in Current Chemistry-Series, pp. 201–259 Search PubMed.
- D. A. Knopf and P. A. Alpert, Faraday Discuss., 2013, 165, 513–534 RSC.
- P. A. Alpert and D. A. Knopf, Atmos. Chem. Phys., 2016, 16, 2083–2107 CrossRef CAS.
- S. China, P. A. Alpert, B. Zhang, S. Schum, K. Dzepina, K. Wright, R. C. Owen, P. Fialho, L. R. Mazzoleni, C. Mazzoleni and D. A. Knopf, J. Geophys. Res.: Atmos., 2017, 122, 3065–3079 CrossRef CAS.
- D. A. Knopf, C. Williamson, A. Kupc, K. D. Froyd, F. Erdesz, N. Wagner, M. Richardson, J. P. Schwarz, R.-S. Gao, J. M. Katich, P. Campuzano-Jost, B. A. Nault, J. C. Schroder, J. L. Jimenez, B. Weinzierl, M. Dollner, T. Bui and D. M. Murphy, Bull. Am. Meteorol. Soc., 2021, 102(10), E1952–E1971 Search PubMed.
- B. J. Murray, T. W. Wilson, S. Dobbie, Z. Q. Cui, S. Al-Jumur, O. Mohler, M. Schnaiter, R. Wagner, S. Benz, M. Niemand, H. Saathoff, V. Ebert, S. Wagner and B. Kärcher, Nat. Geosci., 2010, 3, 233–237 CrossRef CAS.
- R. Wagner, O. Möhler, H. Saathoff, M. Schnaiter, J. Skrotzki, T. Leisner, T. W. Wilson, T. L. Malkin and B. J. Murray, Atmos. Chem. Phys., 2012, 12, 8589–8610 CrossRef CAS.
- B. Wang, A. T. Lambe, P. Massoli, T. B. Onasch, P. Davidovits, D. R. Worsnop and D. A. Knopf, J. Geophys. Res.: Atmos., 2012, 117, D16209 Search PubMed.
- J. L. Hand, T. E. Gill and B. A. Schichtel, J. Geophys. Res.: Atmos., 2017, 122, 3080–3097 CrossRef.
- J. Cozic, S. Mertes, B. Verheggen, D. J. Cziczo, S. J. Gallavardin, S. Walter, U. Baltensperger and E. Weingartner, J. Geophys. Res.: Atmos., 2008, 113, D15209 CrossRef.
- N. Hiranuma, S. D. Brooks, R. C. Moffet, A. Glen, A. Laskin, M. K. Gilles, P. Liu, A. M. Macdonald, J. W. Strapp and G. M. McFarquhar, J. Geophys. Res.: Atmos., 2013, 118, 6564–6579 CrossRef CAS.
- D. J. Cziczo, K. D. Froyd, C. Hoose, E. J. Jensen, M. Diao, M. A. Zondlo, J. B. Smith, C. H. Twohy and D. M. Murphy, Science, 2013, 340, 1320–1324 CrossRef CAS PubMed.
- D. A. Knopf, P. A. Alpert, B. Wang, R. E. O'Brien, S. T. Kelly, A. Laskin, M. K. Gilles and R. C. Moffet, J. Geophys. Res.: Atmos., 2014, 119, 10365–10381 CrossRef.
- F. Zimmermann, S. Weinbruch, L. Schutz, H. Hofmann, M. Ebert, K. Kandler and A. Worringen, J. Geophys. Res.: Atmos., 2008, 113, D23204 CrossRef.
- F. Lüönd, O. Stetzer, A. Welti and U. Lohmann, J. Geophys. Res.: Atmos., 2010, 115, D14201 CrossRef.
- S. L. Broadley, B. J. Murray, R. J. Herbert, J. D. Atkinson, S. Dobbie, T. L. Malkin, E. Condliffe and L. Neve, Atmos. Chem. Phys., 2012, 12, 287–307 CrossRef CAS.
- V. Pinti, C. Marcolli, B. Zobrist, C. R. Hoyle and T. Peter, Atmos. Chem. Phys., 2012, 12, 5859–5878 CrossRef CAS.
- J. D. Atkinson, B. J. Murray, M. T. Woodhouse, T. F. Whale, K. J. Baustian, K. S. Carslaw, S. Dobbie, D. O'Sullivan and T. L. Malkin, Nature, 2013, 498, 355–358 CrossRef CAS PubMed.
- S. Hartmann, H. Wex, T. Clauss, S. Augustin-Bauditz, D. Niedermeier, M. Rösch and F. Stratmann, J. Atmos. Sci., 2016, 73, 263–278 CrossRef.
- A. Peckhaus, A. Kiselev, T. Hiron, M. Ebert and T. Leisner, Atmos. Chem. Phys., 2016, 16, 11477–11496 CrossRef CAS.
- M. Burkert-Kohn, H. Wex, A. Welti, S. Hartmann, S. Grawe, L. Hellner, P. Herenz, J. D. Atkinson, F. Stratmann and Z. A. Kanji, Atmos. Chem. Phys., 2017, 17, 11683–11705 CrossRef CAS.
- A. Kumar, C. Marcolli, B. Luo and T. Peter, Atmos. Chem. Phys., 2018, 18, 7057–7079 CrossRef CAS.
- G. Y. Jeong, M. Y. Park, K. Kandler, T. Nousiainen and O. Kemppinen, Atmos. Chem. Phys., 2016, 16, 12397–12410 CrossRef CAS.
- R. C. Moffet, H. Furutani, T. C. Rödel, T. R. Henn, P. O. Sprau, A. Laskin, M. Uematsu and M. K. Gilles, J. Geophys. Res.: Atmos., 2012, 117, D07204 CrossRef.
- A. Ito, S. Myriokefalitakis, M. Kanakidou, N. M. Mahowald, R. A. Scanza, D. S. Hamilton, A. R. Baker, T. Jickells, M. Sarin, S. Bikkina, Y. Gao, R. U. Shelley, C. S. Buck, W. M. Landing, A. R. Bowie, M. M. G. Perron, C. Guieu, N. Meskhidze, M. S. Johnson, Y. Feng, J. F. Kok, A. Nenes and R. A. Duce, Sci. Adv., 2019, 5, eaau7671 CrossRef CAS PubMed.
- U. Schwertmann, J. Friedl and H. Stanjek, J. Colloid Interface Sci., 1999, 209, 215–223 CrossRef CAS PubMed.
- N. Hiranuma, M. Paukert, I. Steinke, K. Zhang, G. Kulkarni, C. Hoose, M. Schnaiter, H. Saathoff and O. Möhler, Atmos. Chem. Phys., 2014, 14, 13145–13158 CrossRef CAS.
- E. Chong, K. E. Marak, Y. Li and M. A. Freedman, Phys. Chem. Chem. Phys., 2021, 23, 3565–3573 RSC.
- A. W. Schroth, J. Crusius, E. R. Sholkovitz and B. C. Bostick, Nat. Geosci., 2009, 2, 337–340 CrossRef CAS.
- Y. Takahashi, M. Higashi, T. Furukawa and S. Mitsunobu, Atmos. Chem. Phys., 2011, 11, 11237–11252 CrossRef CAS.
- Z. Shi, J. M. Zachara, L. Shi, Z. Wang, D. A. Moore, D. W. Kennedy and J. K. Fredrickson, Environ. Sci. Technol., 2012, 46, 11644–11652 CrossRef CAS PubMed.
- Z. Shi, M. D. Krom, T. D. Jickells, S. Bonneville, K. S. Carslaw, N. Mihalopoulos, A. R. Baker and L. G. Benning, Aeolian Res., 2012, 5, 21–42 CrossRef.
- Z. Shi, S. Bonneville, M. D. Krom, K. S. Carslaw, T. D. Jickells, A. R. Baker and L. G. Benning, Atmos. Chem. Phys., 2011, 11, 995–1007 CrossRef CAS.
- R. Raiswell and D. E. Canfield, Geochem Perspect Lett., 2012, 1, 1–220 CrossRef.
- J. L. Jambor and J. E. Dutrizac, Chem. Rev., 1998, 98, 2549–2586 CrossRef CAS PubMed.
- R. A. Scanza, D. S. Hamilton, C. Perez Garcia-Pando, C. Buck, A. Baker and N. M. Mahowald, Atmos. Chem. Phys., 2018, 18, 14175–14196 CrossRef CAS.
- T. Hiemstra, Geochim. Cosmochim. Acta, 2013, 105, 316–325 CrossRef CAS.
- C. Rodriguez-Navarro, F. di Lorenzo and K. Elert, Atmos. Chem. Phys., 2018, 18, 10089–10122 CrossRef CAS.
- F. Zimmermann, M. Ebert, A. Worringen, L. Schütz and S. Weinbruch, Atmos. Environ., 2007, 41, 8219–8227 CrossRef CAS.
- A. Kiselev, F. Bachmann, P. Pedevilla, S. J. Cox, A. Michaelides, D. Gerthsen and T. Leisner, Science, 2017, 355, 367–371 CrossRef CAS PubMed.
- B. Wang, D. A. Knopf, S. China, B. W. Arey, T. H. Harder, M. K. Gilles and A. Laskin, Phys. Chem. Chem. Phys., 2016, 18, 29721–29731 RSC.
- J. Raabe, G. Tzvetkov, U. Flechsig, M. Böge, A. Jaggi, B. Sarafimov, M. G. C. Vernooij, T. Huthwelker, H. Ade, D. Kilcoyne, T. Tyliszczak, R. H. Fink and C. Quitmann, Rev. Sci. Instrum., 2008, 79, 113704 CrossRef CAS PubMed.
-
R. C. Moffet, A. V. Tivanski and M. K. Gilles, Scanning Transmission X-ray Microscopy: Applications in Atmospheric Aerosol Research, in Fundamentals and Applications in Aerosol Spectroscopy, ed. R. Signorell and J. P. Reid, Taylor and Francis Group, LLC, Boca Raton, FL, 2011, ch. 17, pp. 419–462 Search PubMed.
- J.-D. Förster, C. Gurk, M. Lamneck, H. Tong, F. Ditas, S. S. Steimer, P. A. Alpert, M. Ammann, J. Raabe, M. Weigand, B. Watts, U. Pöschl, M. O. Andreae and C. Pöhlker, Atmos. Meas. Tech., 2020, 13, 3717–3729 CrossRef.
- G. P. Schill and M. A. Tolbert, J. Phys. Chem. C, 2014, 118, 29234–29241 CrossRef CAS.
- F. H. Marshall, R. E. H. Miles, Y.-C. Song, P. B. Ohm, R. M. Power, J. P. Reid and C. S. Dutcher, Chem. Sci., 2016, 7, 1298–1308 RSC.
- J. P. Reid, A. K. Bertram, D. O. Topping, A. Laskin, S. T. Martin, M. D. Petters, F. D. Pope and G. Rovelli, Nat. Commun., 2018, 9, 15002 Search PubMed.
- M. Kalberer, D. Paulsen, M. Sax, M. Steinbacher, J. Dommen, A. S. H. Prevot, R. Fisseha, E. Weingartner, V. Frankevich, R. Zenobi and U. Baltensperger, Science, 2004, 303, 1659–1662 CrossRef CAS PubMed.
- S. Yang, F. Duan, Y. Ma, H. Li, J. Wang, Z. Du, Y. Xu, T. Zhang, L. Zhu, T. Huang, T. Kimoto, L. Zhang and K. He, Sci. Total Environ., 2020, 746, 141209 CrossRef CAS PubMed.
- K. W. Dawson, M. D. Petters, N. Meskhidze, S. S. Petters and S. M. Kreidenweis, J. Geophys. Res.: Atmos., 2016, 121, 11803–11818 CAS.
- J. Y. Aller, J. C. Radway, W. P. Kilthau, D. W. Bothe, T. W. Wilson, R. D. Vaillancourt, P. K. Quinn, D. J. Coffman, B. J. Murray and D. A. Knopf, Atmos. Environ., 2017, 154, 331–347 CrossRef CAS.
- S. Basu, U. S. Shivhare and A. S. Mujumdar, Drying Technol., 2007, 25, 1581–1586 CrossRef CAS.
- A. L. Kilcoyne, T. Tyliszczak, W. F. Steele, S. Fakra, P. Hitchcock, K. Franck, E. Anderson, B. Harteneck, E. G. Rightor, G. E. Mitchell, A. P. Hitchcock, L. Yang, T. Warwick and H. Ade, J. Synchrotron Radiat., 2003, 10, 125–136 CrossRef CAS PubMed.
- M. Dymarska, B. J. Murray, L. M. Sun, M. L. Eastwood, D. A. Knopf and A. K. Bertram, J. Geophys. Res.: Atmos., 2006, 111, D04204 CrossRef.
- B. Wang and D. A. Knopf, J. Geophys. Res.: Atmos., 2011, 116, D03205 Search PubMed.
- T. Huthwelker, V. Zelenay, M. Birrer, A. Krepelova, J. Raabe, G. Tzvetkov, M. G. C. Vernooij and M. Ammann, Rev. Sci. Instrum., 2010, 81, 113706 CrossRef CAS PubMed.
- V. Zelenay, T. Huthwelker, A. Křepelová, Y. Rudich and A. Markus, Environ. Chem., 2011, 8, 450–460 CrossRef CAS.
- V. Zelenay, M. Ammann, A. Křepelová, M. Birrer, G. Tzvetkov, M. G. Vernooij, J. Raabe and T. Huthwelker, J. Aerosol Sci., 2011, 42, 38–51 CrossRef CAS.
- S. T. Kelly, P. Nigge, S. Prakash, A. Laskin, B. Wang, T. Tyliszczak, S. R. Leone and M. K. Gilles, Rev. Sci. Instrum., 2013, 84, 073708 CrossRef PubMed.
- R. E. O'Brien, B. Wang, S. T. Kelly, N. Lundt, Y. You, A. K. Bertram, S. R. Leone, A. Laskin and M. K. Gilles, Environ. Sci. Technol., 2015, 49, 4995–5002 CrossRef PubMed.
- S. S. Steimer, M. Lampimäki, E. Coz, G. Grzinic and M. Ammann, Atmos. Chem. Phys., 2014, 14, 10761–10772 CrossRef.
- P. A. Alpert, P. Corral Arroyo, J. Dou, U. K. Krieger, S. S. Steimer, J.-D. Förster, F. Ditas, C. Pöhlker, S. Rossignol, M. Passananti, S. Perrier, C. George, M. Shiraiwa, T. Berkemeier, B. Watts and M. Ammann, Phys. Chem. Chem. Phys., 2019, 21, 20613–20627 RSC.
- P. A. Alpert, J. Dou, P. C. Arroyo, F. Schneider, J. Xto, B. Luo, T. Peter, T. Huthwelker, C. N. Borca, K. D. Henzler, T. Schaefer, H. Herrmann, J. Raabe, B. Watts, U. K. Krieger and M. Ammann, Nat. Commun., 2021, 12, 1769 CrossRef CAS PubMed.
- N. B. Magee, A. Miller, M. Amaral and A. Cumiskey, Atmos. Chem. Phys., 2014, 14, 12357–12371 CrossRef CAS.
- S. Finizio, S. Wintz, E. Kirk and J. Raabe, Rev. Sci. Instrum., 2016, 87, 123703 CrossRef CAS PubMed.
- K. Zeissler, M. Mruczkiewicz, S. Finizio, J. Raabe, P. M. Shepley, A. V. Sadovnikov, S. A. Nikitov, K. Fallon, S. McFadzean, S. McVitie, T. A. Moore, G. Burnell and C. H. Marrows, Sci. Rep., 2017, 7, 15125 CrossRef CAS PubMed.
- S. Finizio, K. Zeissler, S. Wintz, S. Mayr, T. Weßels, A. J. Huxtable, G. Burnell, C. H. Marrows and J. Raabe, Nano Lett., 2019, 19, 7246–7255 CrossRef CAS PubMed.
- S. Finizio, S. Wintz, S. Mayr, A. J. Huxtable, M. Langer, J. Bailey, G. Burnell, C. H. Marrows and J. Raabe, Appl. Phys. Lett., 2020, 116, 182404 CrossRef.
- V. Prabu, M. Obst, H. Hosseinkhannazer, M. Reynolds, S. Rosendahl, J. Wang and A. P. Hitchcock, Rev. Sci. Instrum., 2018, 89, 063702 CrossRef PubMed.
- E. de Smit, I. Swart, J. F. Creemer, G. H. Hoveling, M. K. Gilles, T. Tyliszczak, P. J. Kooyman, H. W. Zandbergen, C. Morin, B. M. Weckhuysen and F. M. F. de Groot, Nature, 2008, 456, 222–225 CrossRef CAS PubMed.
- C. Baldasseroni, D. R. Queen, D. W. Cooke, K. Maize, A. Shakouri and F. Hellman, Rev. Sci. Instrum., 2011, 82, 093904 CrossRef CAS PubMed.
- J. Dou, P. A. Alpert, P. Corral Arroyo, B. Luo, F. Schneider, J. Xto, T. Huthwelker, C. N. Borca, K. D. Henzler, J. Raabe, B. Watts, H. Herrmann, T. Peter, M. Ammann and U. K. Krieger, Atmos. Chem.
Phys., 2021, 21, 315–338 CrossRef CAS.
- P. A. Alpert, D. A. Knopf and J. Y. Aller, Phys. Chem. Chem. Phys., 2011, 13, 19882–19894 RSC.
- D. A. Knopf, J. Mak, S. Gross and A. K. Bertram, Geophys. Res. Lett., 2006, 33, 1376–1377 CrossRef.
-
U. Schwertmann and R. M. Cornell, Ferrihydrite, in Iron Oxides in the Laboratory: Preparation and Characterization, John Wiley & Sons, Weinheim, Germany, Ltd, 2000, ch. 8, pp. 103–112 Search PubMed.
- R. C. Moffet, T. Henn, A. Laskin and M. K. Gilles, Anal. Chem., 2010, 82, 7906–7914 CrossRef CAS PubMed.
- D. M. Lienhard, A. J. Huisman, D. L. Bones, Y.-F. Te, B. P. Luo, U. K. Krieger and J. P. Reid, Phys. Chem. Chem. Phys., 2014, 16, 16677–16683 RSC.
- T. W. Wilson, B. J. Murray, R. Wagner, O. Möhler, H. Saathoff, M. Schnaiter, J. Skrotzki, H. C. Price, T. L. Malkin, S. Dobbie and S. M. R. K. Al-Jumur, Atmos. Chem. Phys., 2012, 12, 8611–8632 CrossRef CAS.
-
H. R. Pruppacher, and J. D. Klett, Microphysics of Clouds and Precipitation, Kluwer Academic Publishers, Netherlands, 1997 Search PubMed.
- T. Koop and B. J. Murray, J. Chem. Phys., 2016, 145, 211915 CrossRef PubMed.
- S. Fan, D. A. Knopf, A. J. Heymsfield and L. J. Donner, J. Atmos. Sci., 2017, 74, 3799–3814 CrossRef.
- D. A. Knopf, P. A. Alpert, A. Zipori, N. Reicher and Y. Rudich, npj Clim. Atmos. Sci., 2020, 3, 2 CrossRef PubMed.
- T. Koop and B. Zobrist, Phys. Chem. Chem. Phys., 2009, 11, 10839–10850 RSC.
- M. O. Andreae, A. Afchine, R. Albrecht, B. A. Holanda, P. Artaxo, H. M. J. Barbosa, S. Borrmann, M. A. Cecchini, A. Costa, M. Dollner, D. Fütterer, E. Järvinen, T. Jurkat, T. Klimach, T. Konemann, C. Knote, M. Krämer, T. Krisna, L. A. T. Machado, S. Mertes, A. Minikin, C. Pöhlker, M. L. Pöhlker, U. Pöschl, D. Rosenfeld, D. Sauer, H. Schlager, M. Schnaiter, J. Schneider, C. Schulz, A. Spanu, V. B. Sperling, C. Voigt, A. Walser, J. Wang, B. Weinzierl, M. Wendisch and H. Ziereis, Atmos. Chem. Phys., 2018, 18, 921–961 CrossRef CAS.
- C. A. Brock, C. Williamson, A. Kupc, K. D. Froyd, F. Erdesz, N. Wagner, M. Richardson, J. P. Schwarz, R.-S. Gao, J. M. Katich, P. Campuzano-Jost, B. A. Nault, J. C. Schroder, J. L. Jimenez, B. Weinzierl, M. Dollner, T. Bui and D. M. Murphy, Atmos. Meas. Tech., 2019, 12, 3081–3099 CrossRef CAS.
- G. M. McFarquhar, S. Ghan, J. Verlinde, A. Korolev, J. W. Strapp, B. Schmid, J. M. Tomlinson, M. Wolde, S. D. Brooks, D. Cziczo, M. K. Dubey, J. Fan, C. Flynn, I. Gultepe, J. Hubbe, M. K. Gilles, A. Laskin, P. Lawson, W. R. Leaitch, P. Liu, X. Liu, D. Lubin, C. Mazzoleni, A. Macdonald, R. C. Moffet, H. Morrison, M. Ovchinnikov, M. D. Shupe, D. D. Turner, S. Xie, A. Zelenyuk, K. Bae, M. Freer and A. Glen, Bull. Am. Meteorol. Soc., 2011, 92, 183–201 CrossRef.
- P. J. DeMott, A. J. Prenni, G. R. McMeeking, R. C. Sullivan, M. D. Petters, Y. Tobo, M. Niemand, O. Möhler, J. R. Snider, Z. Wang and S. M. Kreidenweis, Atmos. Chem. Phys., 2015, 15, 393–409 CrossRef.
- T. Koop, B. P. Luo, U. M. Biermann, P. J. Crutzen and T. Peter, J. Phys. Chem. A, 1997, 101, 1117–1133 CrossRef CAS.
- J. Biesdorf, P. Oberholzer, F. Bernauer, A. Kaestner, P. Vontobel, E. H. Lehmann, T. J. Schmidt and P. Boillat, Phys. Rev. Lett., 2014, 112, 248301 CrossRef CAS PubMed.
- J. Wu, X. Zhu, M. M. West, T. Tyliszczak, H.-W. Shiu, D. Shapiro, V. Berejnov, D. Susac, J. Stumper and A. P. Hitchcock, J. Phys. Chem. C, 2018, 122, 11709–11719 CrossRef CAS.
|
This journal is © The Royal Society of Chemistry 2022 |
Click here to see how this site uses Cookies. View our privacy policy here.