Addressing main challenges in the tertiary treatment of urban wastewater: are homogeneous photodriven AOPs the answer?
Received
24th February 2022
, Accepted 13th August 2022
First published on 17th August 2022
Abstract
Homogeneous photodriven advanced oxidation processes (HP-AOPs) have been increasingly investigated in past years as a possible alternative solution to conventional tertiary treatment methods of urban wastewater. Despite the encouraging results in successfully addressing some of the main challenges, such as pathogen inactivation, removal of contaminants of emerging concerns (CECs), and antibiotic resistance (AR) control, their full-scale application is still poor. In this review, the main challenges in the tertiary treatment of urban wastewater are identified and the advances of HP-AOPs in addressing such challenges are critically discussed, emphasizing the respective advantages and drawbacks, even compared to consolidated tertiary treatment methods. Differences between solar- and UV-driven HP-AOPs, acidic vs. neutral pH photo-Fenton, as well as between homogeneous and heterogeneous photodriven AOPs are also analyzed and critically discussed. An approach for selecting model pollutants (e.g., CECs, pathogens, and AR indicators) as well as to validate under realistic conditions the results achieved under controlled laboratory conditions is also recommended. The most relevant issues and gaps in the knowledge are identified and discussed. A large number of investigations available in the scientific literature strongly support the capacity of HP-AOPs to effectively address the challenges in the tertiary treatment of urban wastewater, and the time for their full-scale application is almost mature. While the UV/H2O2 process is already applied as a final step in potable water reuse treatment trains, solar photo-Fenton has been quite exhaustively and successfully investigated in the tertiary treatment of urban wastewater so far and it is expected to be implemented at full scale in raceway pond reactors. In conclusion, 10 recommendations to take into account when designing the experimental plan are provided. Hopefully, this manuscript is also useful to water professionals and managers to learn possible effective and sustainable alternatives to conventional processes.
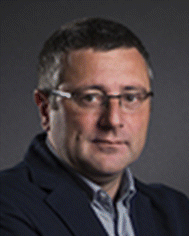 Luigi Rizzo | Luigi Rizzo is Associate Professor in Sanitary and Environmental Engineering at University of Salerno (Italy). He is Coordinator of the “International PhD School on Advanced Oxidation processes”, external expert of European Commission Committees, editor of “Water Science and Technology” journal, and associate editor of the “Journal of Environmental Chemical Engineering”. His main research interest is water/wastewater treatment by advanced oxidation processes (AOPs). He has published 150 papers (107 in peer-reviewed and indexed journals) (8100 citations and 45 h-index in SCOPUS). He was ranked 82th (out of 77 304) in the category Environmental Science according to Stanford's “Top 100 000 Scientists (2020)” list. |
Water impact
Urban wastewater treatment plants (WTPs) are the main hotspots for the release of contaminants of emerging concern into the environment and determinants of antibiotic resistance. Homogenous photodriven advanced oxidation processes are among the most investigated and potentially successful solutions to address such challenges. Their investigation as tertiary treatment processes in WTPs is critically reviewed and recommendations to improve the experimental design and accelerate their full-scale application are provided.
|
1. Introduction
Synthetic or natural chemicals such as active ingredients of pharmaceuticals and personal care products, pesticides, and flame retardants, also grouped as contaminants of emerging concern (CECs), are being continuously released in the environment.1 Though some of them occur at low concentrations in freshwater and wastewater (in the range of ng L−1–μg L−1), they still result in toxic effects.1,2 Moreover, among pharmaceuticals, the occurrence of antibiotics in the environment is a reason of further concern due to their contribution to the spread of antibiotic resistance (AR).3,4 In addition to such pollutants of concern, microplastics also result in a serious threat to the environment.5 Urban wastewater treatment plants (WTPs) are among the main hotspots for the release of all these pollutants into the environment because, due to the lack of specific regulations in most countries, they are not designed to specifically remove them.6 To improve the removal of such pollutants, most existing WTPs should be upgraded and new WTPs should be designed with proper (tertiary) treatment methods. While microplastics can be effectively removed through (advanced) separation methods,7 for CECs and AR determinants, such as antibiotic-resistant bacteria (ARB) and genes (ARGs), separation and biological processes are poorly effective or completely ineffective;6,8 thus, other solutions should be investigated and implemented. Advanced oxidation processes (AOPs) have been widely and successfully investigated at the lab and pilot scale, in the removal of most CECs6 as well as in the inactivation of ARB and ARGs,9 in particular, the so-called homogenous photodriven AOPs (HP-AOPs). HP-AOPs have attracted increasing interest from the scientific community because they are more effective than other AOPs in the removal of the abovementioned pollutants and potentially easy to upscale.6 However, these processes are not yet applied at the full scale because of the lack of specific regulations on CECs (except Switzerland), as well as ARB and ARGs that do not make HP-AOPs economically competitive with conventional tertiary treatment methods. However, the increasing interest and awareness about the risk related to their release into the environment has brought the problem to the attention of the competent authorities, which in some cases have taken the action of monitoring specific contaminants (such as in Europe with the EU watch list (WL)10), suggesting a forthcoming regulation. A positive step in this direction at the European level comes from the recent approval of the first EU regulation on wastewater reuse (Regulation 2020/741).11 It will come in force in the EU countries on June 2023, and the relevant stakeholders are expected to finalize a risk assessment plan where the CECs, AR determinants, and microplastics, among other pollutants, should also be taken into account. If one or more of the corresponding indicators should be higher than the respective risk threshold value, the WTP should be upgraded or designed accordingly.
In this review paper, HP-AOPs and challenges in the tertiary treatment of urban wastewater are first introduced (in chapters 2 and 3, respectively). Subsequently, the advances in the investigation of HP-AOPs application to urban wastewater treatment in addressing such challenges are critically discussed (chapter 4), emphasizing the respective advantages and drawbacks, even compared to consolidated tertiary treatment methods (chapter 7). A focus on specific issues including differences between solar and UV driven HP-AOPs (chapter 5), acidic vs. neutral pH photo-Fenton process (paragraph 4.1.1), as well as between homogeneous and heterogeneous photodriven AOPs (chapter 6) are also analyzed and critically discussed. An approach for selecting the model pollutants (e.g., CECs, pathogens, and AR indicators) as well as to validate under realistic conditions results achieved under controlled laboratory conditions is recommended (paragraph 3.7). A comparison among tertiary treatment methods under realistic conditions and different end-points as well as respective advantages and drawbacks are discussed and summarized (chapter 8). Finally, 10 recommendations to take into account when designing the experimental plan are provided (chapter 9).
2. Homogeneous photodriven advanced oxidation processes (HP-AOPs)
AOPs produce highly oxidizing species, including reactive oxygen species (ROS) and radicals, which are among the most powerful substances that can degrade organic pollutants, oxidize inorganic species, and inactivate several microorganisms. AOPs include homogeneous (e.g., UV/H2O2, Fenton, photo Fenton, ozonation, and UV/Cl2) and heterogeneous (solid semiconductors + light source, such as UV/TiO2 and heterogeneous photo-Fenton) processes. Among homogeneous processes, HP-AOPs have attracted increasing interest from the scientific community due to their efficiency in the removal of several contaminants and inactivation of microorganisms as well as their potential for short-term application at full scale as tertiary treatment methods of urban wastewater.6 In the following paragraphs, the most investigated HP-AOPs are briefly introduced.
2.1 Photo-Fenton and photo-Fenton-like processes
Fe(III) hydroxy complexes such as Fe(OH)2+ and Fe2(OH)24+, which occur in aqueous solutions under acidic pH conditions, absorb light in the UV and the visible regions. In particular, Fe(OH)2+, the most important species, results in the formation of Fe2+ and hydroxyl radical (HO˙), according to the following reaction.12 | Fe(OH)2+ + hν → Fe2+ + HO˙ | (1) |
The reduced iron can react with H2O2 to produce HO˙ and Fe3+(2), which in turn results in the formation of Fe2+ and HO˙ under UV radiation (3). | Fe2+ + H2O2 → Fe3+ + OH− + HO˙ | (2) |
| Fe3+ + H2O + hν → Fe2+ + H+ + HO˙ | (3) |
The efficiency of the photo-Fenton process in the removal of the target pollutants in aqueous matrices depends on the emission spectrum of the light source, the concentration and absorbance of the photoactive species, the characteristics of the aqueous matrix in terms of oxidant demand, as well as the occurrence and concentration of other light-absorbing species, which finally result in the so-called inner filter effect.12–14 It is noteworthy that Fe(III) complexes are characterized by higher absorbance and quantum yield than dissolved Fe(III), and therefore may be more important in photo-Fenton systems.12
As the photo-Fenton process is typically effective under acidic pH (<3) conditions, it is not an attractive option for water and urban wastewater treatment because they are characterized by neutral pH. Therefore, the target aqueous matrix should be first acidified and subsequently neutralized before effluent (re)use or disposal, leading to sludge formation and increased treatment costs. Different approaches have been investigated to overcome these limitations, including (i) process operation at mild conditions (low reagent concentrations, particularly of the metal to minimize its precipitation at pH >3);15 (ii) use of chelating agents (e.g., ethylenediamine-N,N′-disuccinic acid (EDDS) and nitrilotriacetic acid (NTA)) to form complexes with the metal and avoid its precipitation at neutral pH;16,17 (iii) heterogeneous photo-Fenton process;18,19 and (iv) non-iron Fenton catalysts.20,21 Fe(III) forms complexes with some organic compounds, typically characterized by higher molar absorption coefficients in the near-UV and visible regions than aqueous complexes. Their excitation leads to the production of Fe2+ and a ligand radical (4) with wavelength-dependent quantum yields.12
|  | (4) |
2.2 UV/H2O2
Hydrogen peroxide photolysis through UV light produces the HO˙ radical, according to the following reaction.Although the photolysis of H2O2 is characterized by a relatively high quantum yield (Φ ∼1 for HO˙ production, and ∼0.5 for H2O2 loss), its contribution to the degradation of the target pollutants in real water matrices may be reduced due to the absorption of light by dissolved organic matter.12,22 Moreover, H2O2 overdosing results in HO˙ scavenging with the formation of HO2˙ (6).23 | H2O2 + HO˙ → HO2˙ + H2O | (6) |
Despite these limitations, it is possibly the only HP-AOP applied at full scale so far in potable water reuse,24,25 and is used as a benchmark in different research works for comparison with other AOPs or treatment methods.
2.3 UV/free chlorine
UV/free chlorine process (UV/FC) has been recently investigated in the degradation of a variety of pollutants in aqueous matrices.26,27 The formation of radicals is strongly affected by the pH of the aqueous solution because when chlorine (e.g., gas or hypochlorite) is added to water, hypochlorous acid (HOCl) is almost instantaneously formed and, being a weak acid, it dissociates to form hydrogen and hypochlorite ion (OCl−). When FC (HOCl + OCl−) aqueous solution is irradiated by UV light, HO˙ and chlorine radicals are produced according to the following reactions.28–30 | HOCl + HO˙ → + ClO˙ + H2O | (10) |
| OCl− + HO˙ → + ClO˙ + OH− | (11) |
| HOCl + Cl˙ → ClO˙ + H+ + Cl− | (12) |
| OCl− + Cl˙ → ClO˙ + Cl− | (13) |
This process is arousing growing interest as a possible alternative to the UV/H2O2 process25 or as a solution to limit the formation of chlorination byproducts.31
2.4 UV/peracetic acid
The formation of chlorination byproducts and chlorine toxicity have increased the interest toward the use of less toxic chemicals in wastewater disinfection. Peracetic acid (PAA) has been increasingly used in WTPs as an alternative to the chlorination process.32–34 PAA (CH3CO3H) aqueous solutions include a mixture of acetic acid (CH3COOH), H2O2, PAA, and water, according to the following reaction. | CH3COOH + H2O2 ↔ CH3CO3H + H2O | (14) |
Coupling PAA with a light source results in the formation of hydroxyl and organic radicals, according to the following reaction.35 | CH3CO3H + hν → CH3COO˙ + HO˙ | (15) |
UV/PAA has been recently investigated in the removal of CECs as well as in the inactivation of bacteria in wastewater.36,37
2.5 Sulfate radicals
Sulfate radical (SO4˙−)-based AOPs rely on the formation of highly reactive and short-lived SO4˙− by cleaving the peroxide bond in the persulfate (PS) molecule via energy and electron transfer reactions.38–40 These processes have been attracting a lot of interest in the scientific community due to several potential advantages compared to HO˙-based AOPs including (i) higher redox potential, (ii) higher radical formation yield, (iii) a wider variety of methods for PS activation (e.g., UV radiation, heat, reduced metals, metal oxides, and some composites), (iv) less dependence on the operational parameters (in particular on pH), and (v) lower costs of storage and transportation of PS salts.40 Similar to ˙OH-based AOPs, SO4˙−-based AOPs efficiency is negatively affected by the occurrence and concentration of natural organic matter (NOM), but to a lower extent.41 However, unlike ˙OH-based AOPs, the efficiency of SO4˙− AOPs is substrate specific, making these processes effective in the removal of selected target pollutants rather than in the removal of a wide variety of pollutants from complex aqueous matrices.40
3. Challenges in the tertiary treatment of urban wastewater
3.1 Contaminants of emerging concern
CECs are synthetic or natural chemicals commonly detected in the environment that are suspected to have adverse ecological and/or human health effects.8 In particular, they include pharmaceuticals, personal care products, pesticides, and flame retardants, and are typically detected in freshwater and wastewater at concentrations in the range of ng L−1–μg L−1.42 Conventional urban WTPs are among the main point sources of their release into the environment because they have not been designed for their removal.8 Due to their poor biodegradability, CECs accumulate into the environment with possible chronic toxic and human health (through food chain) effects.2 Moreover, WTPs effluents reuse is also a cause for concern because CECs can accumulate in plants irrigated with treated wastewater with possible adverse effects on human health43,44 and ecosystems as well as on crop performance and quality.45 The results from the scientific literature have increased the awareness among stakeholders and policy makers about the risks related to the release of CECs into the environment;46 despite the determination of target pollutants and their concentrations being difficult to regulate, some steps forward have been taken. For example, Switzerland implemented a new Water Protection Act in 2016, which regulates the removal of a group of CECs from urban wastewater.47 In 2015, the European Commission established the first surface water WL under the Water Framework Directive, a mechanism for obtaining Union-wide monitoring data on CECs and substances that may pose a significant risk to or via the aquatic environment.10 The list was updated in 2018 when the insecticide metaflumizone and the antibiotics amoxicillin and ciprofloxacin were added,48 and recently, candidate substances for the 3rd WL have been proposed.49 Moreover, the European Union recently introduced a risk assessment-based approach to identify additional water quality requirements in wastewater reuse regulation (EU 2020/741), including “(d) pharmaceuticals; (e) other substances of emerging concern; and (f) anti-microbial resistance”, which are necessary to ensure the sufficient protection of the environment and of human and animal health in wastewater reuse practices.11
Ozonation and activated carbon adsorption (AC) are considered among the best available technologies (BATs) to remove CECs from secondary-treated urban wastewater, and they have been implemented at full scale in different countries, including the USA,50 Italy,51 Switzerland, and Germany.52 Unfortunately, ozonation results in the formation of toxic oxidation/disinfection byproducts, and AC treatment is not effective in the inactivation of bacteria. Therefore, alternative options, such as HP-AOPs, have been successfully investigated as tertiary treatment methods for urban wastewater.6
3.2 Antibiotic resistance
The overuse and misuse of antibiotics promote the spread of AR in clinical settings and the environment through mobile genetic elements, including ARB and ARGs.53,54 WTPs are among the main hotspots for the dissemination of AR into the environment.4 Biological secondary treatment promotes bacterial growth under selective pressures of antibiotics, heavy metals, and other pollutants that may act as co-selectors.55,56 Besides vertical gene transfer (VGT) from parent to offspring, AR spread can take place through different horizontal gene transfer (HGT) mechanisms, including conjugation, transformation, and transduction (Fig. 1).57
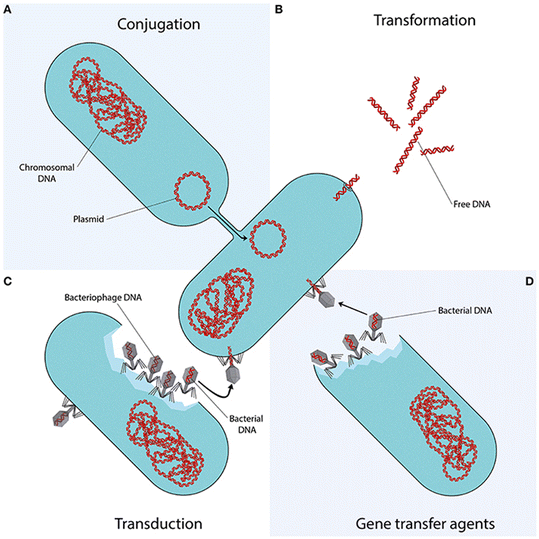 |
| Fig. 1 Mechanisms of horizontal gene transfer. Reprinted.57 | |
Recently, another mechanism named vesiduction, mediated by membrane vesicles, has been proposed as an additional pathway for AR as it plays an essential role in the HGT of ARGs, especially at the bacterial biofilm of water environments.58 WTPs are designed to meet the limits set by national regulations for effluent disposal or reuse; therefore, no treatment unit in the WTP is designed to minimize the release of AR determinants (antibiotics, ARB, and ARGs), with the result that they are continuously released into the environment.4 Even the currently applied disinfection methods may not be effective in controlling AR spread,59,60 and the presently used methods for monitoring the process efficiency do not allow to characterize such a risk.61 In particular conditions, the disinfection process may even increase AR transfer. Chlorination was found to promote the exchange of ARGs across bacterial genera by natural transformation.62 In particular, the authors observed that chlorine-tolerant injured bacteria resulted in higher plasmid transformation frequency than untreated bacteria.
3.3 Toxicity
Chemicals typically used in wastewater disinfection can result in possible adverse effects on the receiving ecosystem. Chlorination, the most widely used disinfectant in wastewater treatment, resulted in cytotoxicity in Chinese hamster ovary cells in partially treated agricultural wastewater effluents collected from two vegetable farms.63 In particular, a higher applied cumulative disinfectant concentration over time (Ct) (6.8 times higher) increased the toxicity by 1.27-fold as compared to the lower Ct value (P < 0.05). When chlorination was compared to the UV/H2O2 process in surface water disinfection in terms of pathogen inactivation and cytotoxicity potential (by means of 3-(4,5-dimethylthiazol-2-yl)-2,5-diphenylte-trazolium colorimetric test on a human-derived cell line), 120 min treatment was necessary to reduce the cytotoxicity of UV/H2O2 at the control level, while cytotoxicity was always higher than the control test for the chlorination process even after an unrealistic treatment time (420 min).64 Although ozonation was found to be less cytotoxic than chlorination in agricultural wastewater,63 phyto- and eco-toxicity were observed to increase in ozonated urban wastewater.65 In Germany, the chemical disinfection of wastewater was stopped in the 1990s because of the toxic impact of chemicals on the aquatic flora.66 Due to the toxic effects of chlorine and ozone on disinfected wastewater, in the recent years, alternative disinfection processes have been implemented in full-scale WTPs, including UV-C radiation and PAA. In particular, in Italy, PAA has been implemented in several WTPs to replace the chlorination process34,67 because WTPs effluents disinfected with PAA result in limited toxic, mutagenic, and genotoxic effects on different aquatic organisms compared to the chlorination process.68 Although they do not result in the formation of known/regulated disinfection byproducts (DBPs), AOPs may promote the formation of oxidation intermediates more toxic than the parent compounds.69,70
3.4 Disinfection and disinfection byproducts
Typically, the last step in the urban wastewater treatment train is disinfection, which aims to minimize the release of pathogens into the environment. Urban wastewater disinfection is performed through chemical (e.g., chlorine gas or hypochlorites, ozone, and PAA) or physical (UV-C radiation) agents. Chemical disinfectants, such as chlorine, inactivate bacteria primarily by damaging the outer cell membrane and subsequently the cytoplasmic components, but they are not effective against all bacteria which, if only damaged, can regrow.71 Moreover, most common chemical disinfectants result in the formation of toxic DBPs, such as trihalomethanes (THMs), haloacetic acids (for chlorination), bromates, and nitrosodymethylamine (for ozonation).72,73 Resistant pathogens and/or stringent limits (such as those for wastewater reuse) ask for increased chemical disinfectant doses, consequently resulting in higher concentrations of DBPs. UV-C disinfection directly targets intracellular DNA which, under favorable post-treatment conditions, can be repaired, thus enhancing cell viability and leading to bacterial regrowth.74 AOPs do not result in the formation of known/regulated DBPs, and the produced radicals can cause oxidative damage to bacterial cells, including lipid peroxidation and protein oxidation.9
3.5 Wastewater reuse (and bacterial regrowth)
Wastewater reuse, in particular for crops irrigation, is an increasingly applied practice in several countries because of the water scarcity problem (even worsened by climate changes) and increasing water demand.75,76 One of the main challenges in wastewater reuse is to meet the stringent limits of specific bacterial indicators. According to the recently established EU Regulation on wastewater reuse (2020/741), the limit for E. coli to meet for “class A” effluents is <10 CFU/100 mL.11 Moreover, a preliminary validation monitoring is requested to assess whether the performance targets (log
10 reduction) are complied with (Table 1). The indicator microorganisms selected are E. coli for pathogenic bacteria, F-specific coliphages, somatic coliphages, or coliphages for pathogenic viruses, and Clostridium perfringens spores or spore-forming sulfate-reducing bacteria for protozoa.
Table 1 Validation monitoring of “class A”a reclaimed water for agricultural irrigation (Table 4, EU regulation 2020/741)11
Indicator microorganismsb |
Performance targets for the treatment chain (log 10 reduction) |
All food crops consumed raw, where the edible part is in direct contact with reclaimed water and root crops consumed raw. All irrigation methods.
The reference pathogens Campylobacter, rotavirus, and Cryptosporidium may also be used for validation monitoring purposes instead of the proposed indicator microorganisms. The following log 10 reduction performance targets shall then apply: Campylobacter (≥5.0), rotavirus (≥6.0), and Cryptosporidium (≥5.0).
Total coliphages is selected as the most appropriate viral indicator. However, if the analysis of total coliphages is not feasible, at least one of them (F-specific or somatic coliphages) shall be analyzed.
Clostridium perfringens spores is selected as the most appropriate protozoa indicator. However, spore-forming sulfate-reducing bacteria are an alternative if the concentration of Clostridium perfringens spores does not make it possible to validate the requested log 10 removal.
|
E. coli
|
≥5.0 |
Total coliphages/F-specific coliphages/somatic coliphages/coliphagesc |
≥6.0 |
Clostridium perfringens spores/spore-forming sulfate-reducing bacteriad |
≥4.0 (in case of Clostridium perfringens spores) |
≥5.0 (in case of spore-forming sulfate-reducing bacteria) |
Bacterial regrowth after disinfection is a serious problem in wastewater treatment and reuse for crop irrigation due to the risk to public health. Although the mechanisms of light and photodriven disinfection processes (i.e., during storage before reuse) remain poorly understood, bacterial regrowth can occur through (i) reactivation from a viable but non-culturable state as environmental conditions are no longer stressful for the bacterial community, (ii) repair of photo-induced DNA damage, and (iii) reproduction of disinfection-resistant bacteria.74 Apparently, contrasting results are available in the scientific literature on the effect of wastewater disinfection processes, but such discrepancies depend on the different operating conditions (e.g., mild vs. intensive disinfectant/UV light doses, reactor design, and wastewater characteristics) and analytical methods. A poor experimental design and using just cultivation methods do not allow a reliable characterization of the potential for bacterial regrowth under realistic conditions. Flow cytometry and qPCR applied on 24 h/48 h-incubated disinfected samples have been recommended as complementary methods to plate counting to learn if the changes in bacterial density take place after a sufficient post-disinfection storage time.61
3.6 Microplastics
Plastic debris and particles with characteristic size between 1 and 5 mm are commonly defined as microplastics (MPs),77 while those smaller than 1 mm are categorized as nanoplastics (NPs).78 Major concerns are related to their release into the environment because they can be accidentally ingested, with adverse effect on different organisms even through the food chain.5 When they reach the WTPs through sewage, and although WTPs are not designed to remove them, they are quite effectively removed in different treatment steps.79 Nevertheless, the amount of MPs and NPs in the effluents of WTPs is still high, being estimated in the range of 24–209% due to the possible fragmentation of MPs in NPs during the treatment steps.79 Although disinfection methods, including ozonation,80 UV radiation, and chlorination,81 have been found to reduce the MPs to some extent in wastewater as well as to impact their integrity,79 the most effective methods include separation processes, particularly membrane-based technologies.7 Therefore, although MPs and NPs have been introduced as challenging pollutants in wastewater treatment, their removal by HP-AOPs has not received particular attention by the scientific community so far and will not be reviewed and discussed in this paper.
3.7 CECs removal, pathogens inactivation, and AR control: selection of model pollutants and validation tests under realistic conditions
3.7.1 CECs removal.
HP-AOPs have been widely investigated for the removal of CECs and the inactivation of pathogens. The effect of radicals on CECs and pathogens also depends on the target pollutant/microorganism. For example, carbamazepine (CBZ) has been widely investigated as the model CEC and it is quite refractory to the oxidation/degradation by HP-AOPs compared to other model CECs,82–84 which make it a good indicator to compare different processes. On the other hand, diclofenac is easily degraded by HP-AOPs and although it has also been widely used as a model CEC, particularly because of its possible toxic effects, it is not a good indicator to compare different HP-AOPs because it is quite photosensitive.82,83 However, these examples show that the two mechanisms should be also taken into account when selecting the target CECs, namely, direct photolysis and indirect photolysis. Although the reactivity of CBZ toward hydroxyl and sulfate radicals is high and similar to that of other CECs, its degradation in urban wastewater drastically decreases.85 This compound is less degraded by direct/indirect photolysis. Direct photolysis mechanism depends on the type and intensity of radiation, compound concentration, and characteristics of the wastewater, and indirect photolysis depends on the generation of ROS or excited triplet states from the photolysis of NOM, some inorganics, or even other pollutants occurring in the secondary effluent.86–88 Wastewater contains bicarbonate (HCO3−) and carbonate (CO32−) ions, which can be transformed to carbonate radical anion (CO3˙−) when secondary urban wastewater is treated by AOPs to remove CECs, and play a role in their degradation.89
CECs can also be sensitive to water temperature, which may cause the overestimation of HP-AOPs efficiency, particularly in solar-driven applications. This is the case of antibiotic chloramphenicol, which has been investigated as a model CEC because of its occurrence in surface water and wastewater and its carcinogenic effects, and it was found to be degraded (25%) already at 40 °C after 180 min exposure.90 Accordingly, the correct approach for selecting the most suitable model CEC (or mixture of CECs) for evaluating the effect of the investigated process as well as to compare different processes should take into account not only its (i) toxicity, (ii) frequency, and concentration at which it is detected in water and wastewater, (iii) its relevance for the specific case study, and (iv) final use (e.g., disposal or reuse) of the treated effluent, but also its possible refractoriness to the investigated process or its sensitivity to the corresponding control tests. Moreover, the tests should not be finalized only in deionized water solutions or simulated wastewater using non-realistic concentrations (mg L−1) of the target CEC, but also in real (secondary treated) wastewater (eventually spiked with 100–200 μg L−1 initial concentrations, if the analytical instrument (e.g., UPLC) and method do not allow to detect the real concentration (ng L−1) at which the target CEC occurs in the real wastewater sample). It is well known that the wastewater matrix can drastically affect AOPs efficiency due to the occurrence of organic matter and radical scavengers;91 therefore, operating the process only in deionized aqueous solutions or simulated water/wastewater may significantly overestimate the process efficiency.15,82
3.7.2 Pathogen inactivation.
If the scope of the investigation is to evaluate the disinfection process efficiency to make the effluent in compliance with specific regulations, the model pathogens should be selected according to the relevant regulation, taking into account the possible fate of the treated wastewater, namely, disposal into the environment or reuse. The limit for wastewater reuse is typically really stringent compared to the standard for effluent disposal into a receiving water body. For example, in Italy, 10 E. coli/100 mL (set by Ministry Decree 93/2006) and 5000 E. coli/100 mL (set by Legislative Decree 152/2006) are the limit and the guide value for the reuse and effluent disposal in surface water, respectively. However, when the scope of the study is to evaluate the effect on particular microorganisms, which are suspected to be resistant to disinfection processes and/or are not yet regulated, other microorganisms can be selected accordingly. In order to achieve reliable results, disinfection tests on indigenous microorganisms in real wastewater matrix should also be performed to validate the results observed under laboratory-controlled conditions using lab growth microorganisms in saline solutions. This approach is strongly recommended as the optimum conditions have been identified because indigenous pathogens can be more resistant to disinfection process than their respective lab-grown counterparts.92
3.7.3 AR control.
Unlike CECs and pathogens, inconsistent and incomplete information/data are available on the possible control of AR spread by AOPs. Such inconsistency in the results available in the scientific literature and, above all, in the conclusions withdrawn by the respective research groups, also depends on the fact that no accepted/shared indicators are available, and when reference is made to some indicators (for example, the abundance and type of ARB and ARGs), the available data are not yet sufficient to identify unique and shared threshold values, and it is difficult to predict if it will ever be possible to do it.61 However, some recommendations on possible indicators/model pollutants can be provided according to the mechanisms of AR transfer explained in paragraph 3.2. The conjugation mechanism suggests that the abundance of bacterial cells can be an indicator of the potential for AR transfer; therefore, if the disinfection process drastically decreases the bacterial abundance, we can reasonably assume that the risk of AR transfer decreases accordingly. However, withdrawing the conclusion that the risk of AR has been significantly reduced because antibiotic resistant strain cells of a specific bacterial family were effectively inactivated is a conclusion not supported by sufficient evidence for different reasons: (i) the target AR bacterial family is only a small fraction of the whole bacterial population typically occurring in wastewater; (ii) the disinfection process possibly selected for the bacterial population by increasing the relative abundance of other bacterial families more resistant than the target one and eventually with higher potential to transfer AR; (iii) the disinfection process will result in a release of ARGs and other mobile genetic elements from damaged/death bacterial cells, which may still contribute to AR transfer through transduction and natural transformation mechanisms, respectively. Therefore, the cultivation methods for the enumeration of the general bacterial population (such as total coliforms, faecal coliforms, and heterotrophic plate count), eventually coupled to flow cytometry and the quantification of relevant ARGs through quantitative real time polymerase reaction (qPCR), should be used to evaluate the relative efficiency of the investigated disinfection process compared to a consolidated one in terms of AR control.93,94
4. Tertiary treatment of urban wastewater by HP-AOPs
In this paragraph, the investigation of HP-AOPs as tertiary treatment methods of urban wastewater and particularly their effect on CECs and AR is discussed. Noteworthily, while the removal of CECs by different HP-AOIPs has been widely investigated, AR has attracted the interest of the scientific community working on AOPs only recently; the need for molecular methods to analyze ARGs and better characterize the effect of treatment processes on AR make such an investigation difficult, thus explaining the lower number of manuscripts available in the scientific literature on this topic. Moreover, the higher complexity of biological/molecular characterization compared to chemical analysis has resulted in some inconsistency among the different results published so far. Although AOPs can successfully inactivate ARB in urban wastewater,71,95,96 this may be not sufficient to effectively reduce the risk of AR spread through HGT mechanisms due to the release of mobile genetic elements (including ARGs) from damaged/death bacterial cells and the results achieved at the lab scale under controlled conditions, far from the realistic ones, may overestimate HP-AOPs efficiency in controlling AR spread.
4.1 Photo-Fenton process
The (photo-)Fenton process is typically effective under acidic pH conditions (optimum <3); therefore, to make the process more attractive as a tertiary treatment method of urban wastewater, the use of chelating agents has been investigated to make the process effective even under neutral pH conditions.
4.1.1 Acidic vs. neutral pH conditions: are chelating agents an effective/sustainable solution for the tertiary treatment of urban wastewater?.
The (photo-)Fenton reaction is effective at pH <3 because as the pH increases, iron starts to precipitate drastically, thus reducing the process efficiency. Therefore, different strategies have been investigated so far, including heterogeneous photo-Fenton process,97 mild conditions,15 and addition of chelating agents to form complexes with iron and minimize metal precipitation at neutral pH.16 The use of chelating agents in the photo-Fenton process has been widely investigated in the tertiary treatment of urban wastewater for disinfection,98 CECs removal,99 and AR control100 because it allows to avoid the typical drawbacks of the acidic pH process, including acidification, subsequent pH neutralization, and sludge formation, with an expectedly increased process cost. Among these, aminopolycarboxylic compounds such as ethylene diaminetetraacetic acid (EDTA), ethylenediamine-N,N′-disuccinic acid (EDDS), and nitrilotriacetic acid (NTA), can form stable complexes with Fe3+ ions in a wide pH range, with optimal ratio (Fe
:
L) in the range of 1
:
1.5–1
:
2.0.16 Two organic acids, namely, oxalic (OA) and citric (CA) acids, have also been investigated in the removal of CECs.101,102 The main advantages and drawbacks of some chelating agents applied in photo-Fenton treatment are summarized in Table 2.
Table 2 Advantages and drawbacks of chelating agents typically used in photo-Fenton treatment
Chelating agent |
Advantages |
Drawbacks |
Ref. |
EDDS |
High biodegradability |
Lower bacterial inactivation efficiency (E. coli and total coliform) compared to other chelating agents, sunlight/H2O2, and ozonation |
14, 16, 17, 103–106 |
Wide pH range (3–9) applicability |
Stability drastically affected by temperature |
No toxicity |
Expensive compared to other chelating agents |
Good efficiency in CECs removal (>60%) from secondary-treated wastewater |
EDTA |
High stability |
Poor biodegradability |
14, 17, 107, 101 |
Good efficiency in CECs removal (77% of sulfamethoxazole) from secondary-treated wastewater |
It can contribute to aquatic toxicity |
Lower E. coli inactivation efficiency compared to EDDS |
NTA |
High stability during SPF |
Potentially carcinogenic to humans |
101–112
|
High biodegradability |
Good efficiency in CECs removal (comparable to EDDS) from secondary-treated wastewater |
High inactivation of (lab) E. coli (6 log unit) |
Cheaper than EDDS |
OA |
High biodegradability |
Lower CECs removal efficiency compared to EDTA and NTA |
16, 101 |
Complex preparation easier than other complexing agents |
Large amounts required for complexation, resulting in high TOC and increased process cost |
CA |
High biodegradability |
Higher CA dose (Fe : CA 1 : 4) compared to EDDS (Fe : EDDS = 1 : 2) is requested to achieve comparable efficiencies |
16, 102 |
High removal rate of CECs (90%), comparable to EDDS, can be achieved but in low carbonates (5 mg L−1) wastewater |
Low reactivity with the metal compared to other chelating agents |
However, a comparison between the solar photo-Fenton (SPF) process operated under acidic and neutral pH conditions for the removal of CECs from urban wastewater led to some troubles regarding the supposedly higher operating cost of the acidic SPF.103 Acidic pH option was found to be cheaper than the neutral pH one, mainly due to the impact of the chelating agent EDDS on the operating costs. The 15 cm depth SPF raceway pond reactor (RPR) was operated in continuous mode at 30 min HRT, 0.1 mM of iron (5.58 mg L−1), 0.88 mM of H2O2, and treatment capacity of 2250 L m−2 d−1 for 7.5 h operation per day, with the aim to achieve 80% CEC removal, and the total unitary costs were estimated at 0.25 € per m3 and 0.56 € per m3 at acidic and neutral pH, respectively. However, before making these results conclusive, it should be taken into account that the operation under acidic pH conditions increases the conductivity of the effluent due to the acidification/neutralization processes, making this option poorly attractive in case of wastewater reuse for crop irrigation. Moreover, the higher operating costs of the neutral pH condition is mainly due to the use of EDDS as a chelating agent, which account for 68% of such cost. Actually, the choice of the most appropriate chelating agent for the SPF process is dictated by the boundary conditions. The choice should fall on the chelating agent, which results in a fair compromise among the process efficiency, treatment scope, investment, and management costs. For example, EDDS has proven to be more effective than NTA in the removal of sulfamethoxazole and imidacloprid from real secondary-treated urban wastewater104 but less effective than other chelating agents in the inactivation of E. coli and total coliforms.106 Therefore, depending on the scope of the treatment (e.g., CECs removal, bacteria inactivation, or both of them), the kinetics can be faster or slower, consequently affecting the reactor volume and investment costs. Moreover, EDDS is more expensive than other chelating agents, which this will affect the management costs. The investigation of new approaches as well as alternative and cheaper chelating agents is desirable and recommended to make the neutral pH process competitive in terms of operating costs. This investigation should not only evaluate the process efficiency in terms of CECs removal, but it should take into account even (i) other end points (namely, disinfection efficiency and final toxicity), (ii) chelating agent stability and biodegradability, (iii) eventually implementing LCA tools to make a final decision on the most suitable approach for the tertiary treatment of urban wastewater by SPF. Accordingly, some recent studies have already moved in such directions. For example, López-Vinent and co-authors investigated an alternative approach by mixing different chelating agents (namely, EDDS, EDTA, and diethylenetriamine pentaacetic acid (DTPA)) to overcome the respective limitations.14 The best performance in micropollutant degradation (propranolol, acetamiprid, and sulfamethoxazole) and E.coli inactivation was observed for the mixing between EDDS and EDTA.
4.1.2 Effect of the photo-Fenton process on antibiotic resistance.
When the efficiency of the SPF process in controlling AR spread was investigated in RPR at neutral pH (20 mg Fe2+ L−1, 50 mg H2O2 L−1) using real urban wastewater, although E. coli and Enterococcus sp. cefotaxime resistant bacteria were effectively inactivated (detection limit (1 CFU mL−1) achieved after 30–40 min, 3.2–4.7 kJ L−1), no ARGs removal was observed.113 Similar results on ARGs were also observed when SPF (5 mg Fe2+ L−1, 50 mg H2O2 L−1, pH 3) was operated at the pilot scale through a compound parabolic collector (CPC)-based reactor.100 The aqueous matrix drastically affects the process efficiency. ARB inactivation and ARGs removal rates by photo-Fenton with EDDS as the chelating agent (0.1
:
0.2
:
0.3 mM of Fe(III)
:
EDDS
:
H2O2) decreased significantly as the complexity of the aqueous matrix increased, with removal rates in the order deionized water > synthetic wastewater > real wastewater.114 When the experimental apparatus/reactor and operating conditions were moved away from the real ones, different results were observed. Giannakis et al. observed a good efficiency of the SPF process on AR, but the operating conditions (10 mg L−1 H2O2 and 1 mg L−1 Fe2+), experimental apparatus (lab scale Suntest apparatus with a 150 W xenon lamp and an irradiation level of 750 W m−2), and particularly ARGs characterization (they were not quantified through qPCR but monitored only through PCR) did not allow to quantify the actual effect of the process on the target ARGs.96 In another work, Vilela et al. evaluated the effect of the SPF process (50 mg L−1 of H2O2 and 30 mg L−1 of Fe2+, 60 min treatment, neutral pH, 400 mL glass reactor placed inside a solar simulator, 268 W m−2) on the inactivation of resistance-conferring plasmids (RCPs) in synthetic secondary wastewater (SWW) using lab-grown bacteria (E. coli BL21 transformed with plasmids by heat-shock).115 The authors observed the total inactivation of RCPs by SPF within 30 min treatment in SWW. When the same research group investigated the effect of SPF (using the same experimental apparatus and H2O2 and Fe2+ doses) on the removal of several ARGs in real wastewater, the observed total removal for β-lactams and fluoroquinolones ARGs after 240 min treatment was in the range of 55–61%.116
4.2 UV/hydrogen peroxide
4.2.1 CECs removal.
UV/H2O2 is a well-established process, commonly used for organic contaminant control in drinking water treatment plants and as a disinfection/refining step in wastewater-derived reverse osmosis permeate for groundwater recharge.25 When the process was operated under sunlight, the effect in terms of CECs degradation (CBZ, flumequine (FLU), and thiabendazole (TBZ), 100 μg L−1 each) in secondary-treated urban wastewater was found to be pollutant-specific and followed the order FLU > TBZ > CBZ (94%, 50%, and 12% after 90 min treatment, cumulative energy (QUV) = 4.6 kJ L−1).117 Taking into account that a small amount of iron naturally occurs in water and wastewater, the addition of H2O2 in the presence of sunlight can also promote a photo-Fenton reaction, improving the radical formation and the subsequent CECs removal. When the UV-C/H2O2 process was investigated, the six target CECs were removed >96% from three different secondary-treated municipal wastewaters (activated sludge (AS) (99%) > moving bed biofilm reactor (MBBR) (97%) > coagulation/flocculation (96%)) after 5 min treatment, and 99–100% removal was observed after 10 min treatment.118
4.2.2 Microorganisms' inactivation.
H2O2 photolysis is particularly effective in water and wastewater disinfection. Sunlight/H2O2 can result in the inactivation rates of some log units for different microorganisms in real wastewater.119,120 Agulló-Barceló et al. evaluated the effect of different solar-driven AOPs on naturally occurring E. coli, spores of sulphite-reducing clostridia (SRC), somatic coliphages (SPH), and F-specific RNA bacteriophages (FBPH) and sunlight/H2O2 (20 mg L−1) process, resulting in 5.3, 3.0, and 2.3 log inactivation for E. coli, FBPH, and SPH, respectively.119 Microorganisms inactivation by the UV-C/H2O2 process in the range of 4 (2.5 min)–6 (10 min) log units in real treated municipal wastewater was observed to depend on the type of the treatment (namely, primary, AS, MBBR, and CF).121 As the inactivation mechanisms of microorganisms by sunlight/or UV-C/H2O2 processes are of concern, an intracellular photo-Fenton reaction can also take place because H2O2 can diffuse through the cell wall and subsequently react with naturally-occurring iron inside the cell.122–124
4.2.3 Effect on AR.
When the effect of the UV-C/H2O2 process (2.0 mM of H2O2, 500 mL reactor volume, 0.45 W m−2, 80 min treatment) was investigated in the inactivation of the AR E. coli J53 strain (3.45 × 106 CFU mL−1) and its kanamycin (aphA) and tetracycline (tetA) resistant genes inoculated in sterilized real tertiary treated urban wastewater, the complete removal of both genomic and plasmid DNA was observed unlike that of the control test with the UV-C process.125 However, in a previous work, Yoon et al. investigated the inactivation efficiency of plasmid-encoded ARGs (ampR and kanR), both in the extracellular form (e-ARG) and present within E. coli (intracellular form, i-ARG) by the UV-C/H2O2 process (∼0.3 mW cm−2, 120 mL reactor volume, 10 mg L−1 of H2O2), and observed almost the same rates of e-ARG removal between UV-C control and UV-C/H2O2 in real wastewater, unlike the phosphate-buffered solutions, where e-ARG removal was faster for UV-C/H2O2 compared to UV-C treatment.126 Zhang et al. also observed the significant removal of ARGs (2.8–3.5 log of sul1, tetX, and tetG) by UV-C/H2O2 (30 min treatment, UV-C fluence not provided) in secondary-treated urban wastewater, but only under conditions not feasible at full-scale (pH 3.5 and 340 mg L−1 of H2O2).127 Moreover, when the process was investigated using a UV lamp emitting in the range of 320–450 nm under realistic conditions for wastewater treatment (pH 7.6 and 20 mg L−1 of H2O2), it was not effective in the removal of the target ARGs even after 240 min treatment.128
4.3 UV/free chlorine
UV/free chlorine (FC) process has been increasingly investigated in the past years and it seems to be an attractive alternative option to other AOPs in the tertiary treatment of urban wastewater, such as UV/H2O2, typically applied as a final step in potable water reuse treatment train.25
4.3.1 Bacterial inactivation, CECs removal, and effect on chlorination byproducts.
UV-C/FC, while effectively inactivating bacteria129 and reducing CECs, did not result in the significant formation of THMs (in particular, chloroform (CLF)) compared to the chlorination process, even after 48 h storage of treated wastewater (Fig. 2).31 Unlike the chlorination process, where FC reacts with organic matter to form THMs, in the UV-C/FC process, part of FC reacts with UV-C radiation to form radicals and it is no longer available to form THMs.31 These results are consistent with a subsequently published work where the UV-C/FC process was found to be more effective than the corresponding control tests with UV-C radiation and chlorination in the control of THMs and haloacetic acids.130
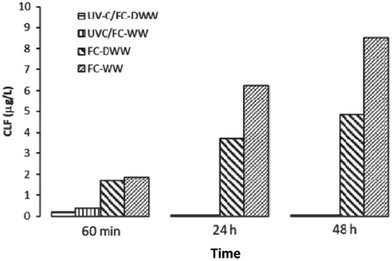 |
| Fig. 2 Chloroform (CLF) formation after FC and UV-C/FC treatment, respectively: comparison between the end of the treatment (60 min), and 24 h and 48 h post-treatment incubation (Reprinted,31 Fig. 6, copyright 2020, with permission from Elsevier). | |
When compared to other HP-AOPs (namely, SPF and sunlight/H2O2) and conventional disinfection methods (chlorination and UV-C irradiation), the sunlight/FC process was found to be the more effective one in the inactivation of E. coli and Enterococcus as well as in controlling post-treatment bacterial regrowth in inoculated roof-harvested rainwater.131
4.3.2 Effect on AR.
The experimental apparatus and operating conditions strongly affect the process efficiency in terms of ARGs inactivation, and completely different results have been observed in the scientific literature, from high removal to enrichment of the target ARGs after disinfection treatment. The UV-C/FC process was more effective than UV-C and chlorination as a standalone process in the removal of tetM and blaTem genes from aqueous solutions.132 More specifically, the UV-C/FC process (9.03 mW cm−2) reduced the tetM and blaTem genes by 0.98–3.20
log and 1.28–3.36
log, respectively, as the chlorine dose was increased from 0.5 to 20.0 mg L−1. However, when more realistic conditions were investigated (real secondary-treated wastewater and 0.91 mW cm−2 UV-C dose), the target ARGs (belonging to phenicol, tetracycline, macrolide, fluoroquinolone, oxazolidinone, and penam classes of antibiotics) were found to be enriched by all the three disinfection treatments (UV-C, chlorination, and UV-C/FC), the most prominent increase being observed for UV-C/FC.130 These results are consistent with those observed by Ping et al.133 that compared the effect of HP-AOPs (including UV-C/FC process) with conventional disinfection processes (namely, UV-C, chlorination, and PAA) in the simultaneous removal of antibiotics and ARGs in real secondary-treated wastewater. Low (18 mJ cm−2) and high (108 mJ cm−2) UV doses (1 min and 6 min irradiation times) and low NaClO concentration (3.92 mg L−1) were investigated, and β-lactam (−35.9%) and macrolides (−12.0%) ARGs remarkably increased after UV-C/FC treatment.
4.4 UV/PAA
The UV/PAA process has been poorly investigated as a tertiary treatment of urban wastewater compared to other HP-AOPs. Previous works have been mainly focused on bacterial inactivation34,36,134,135 and, only recently, its effect on CECs and AR has been addressed.37,82,133,136
4.4.1 Effect on CECs.
Rizzo et al. investigated the effect of the light source (sunlight Vs UV-C), water matrix (groundwater Vs wastewater), and PAA initial concentration on CECs.82 All the three variables investigated significantly affected process efficiency. While the results in terms of CECs degradation by sunlight/PAA are consistent with other solar-driven AOPs, CBZ removal by UV-C/PAA (22% within 180 min, 1 mg L−1 of PAA, CBZ 100 μg L−1) was not in agreement with the results observed by Cai et al. (90% within 30 min, 1 mg L−1 of PAA, CBZ initial concentration 1 μM);37 however, even these differences can be explained by the different experimental set-up, reactor design, and operating conditions. In order to evaluate the applicability of the UV/PAA process as a tertiary treatment step of urban wastewater, a comparison with other processes is necessary. According to previous works available in scientific literature, the UV/PAA process is less effective than the UV/H2O2 process in the removal of CECs from both deionized aqueous solutions136 and real wastewater.90 Moreover, it is worthy to note that the presence of H2O2 in the commercially-available PAA solutions can significantly affect the removal efficiency of the target pollutants by the UV/PAA process. When H2O2 was quenched, the half-life time of chloramphenicol in real wastewater increased from 20 min of the UV/PAA process including H2O2 in the PAA solution to 99 min of UV/PAA without H2O2 in the PAA solution.90 Finally, when considering the possible application of the UV/PAA process, the scavenging effect of PAA on hydroxyl radicals at high PAA concentration37,136 should also be taken into account.
4.4.2 Effect on AR.
Rizzo et al. also investigated the effect of photodriven-PAA advanced oxidation process on ARB.82 However, the results in terms of ARB inactivation were not so consistent with the previous work, possibly due to the differences in the terms of water matrix, E. coli population (total vs. AR E. coli), and initial bacterial density.34 The effect on the ARGs of the UV-C/PAA process (4 mg L−1 of PAA, 18 mJ cm−2 (low) or 108 mJ cm−2 (high), 1 and 6 min irradiation times) in real secondary-treated urban wastewater has also been recently investigated.133 The results showed that the UV-C/PAA treatment with a high UV dosage was effective to some extant in the removal of almost all the target ARGs (from 3.2% to 38.9%), while it stimulated a slight increase in sul2 and tetC ARGs.
4.5 Sulphate radicals
Sulphate radicals-based HP-AOPs have only been recently investigated as the tertiary treatment of urban wastewater, and less scientific literature is available.
4.5.1 Effect on CECs.
UV/PS (S2O82−) was compared to UV/H2O2 and UV/FC for the degradation of six representative trace organic contaminants under conditions relevant for potable water reuse, and UV/S2O82− was found to be the most efficient treatment.137 Among the three HP-AOPs, the treatment efficiency of UV/S2O82− was found to be more sensitive to pH, chloride, and inorganic carbon. The UV-vis/PS/Fe(II) process using solar irradiation was found to be more effective than PS/Fe(II) and UV-vis/PS processes in the removal of CBZ from both deionized water and secondary-treated urban wastewater.138 UV-C/peroxymonosulfate (PMS) and UV-C/PMS/Fe(II) were also investigated at full scale at low dosages (0.05–0.5 mM) and short UV-C contact time (4–18 s) for the removal of antibiotics and ARGs from wastewater effluents at the Estiviel WTP (Toledo, Spain).139 In particular, PMS (0.5 mM) combined with UV-C (7 s contact time) was the most efficient process in terms of antibiotics removal compared to other processes (including UV-C/H2O2).
4.5.2 Effect on bacterial inactivation and AR.
Unlike that observed in the removal of antibiotics, UV-C alone was the most effective process, even better than UV-C/PMS and UV-C/PMS/Fe(II) in the removal of ARGs from real wastewater at full scale.133 These results find confirmation in the previously mentioned work.125 When the effect of UV-C- and UV-C-driven HP-AOPs (using hydrogen peroxide, PS, and PMS as oxidant, respectively) were investigated in terms of inactivation of AR E. coli J53 strain, only a slight difference was observed compared to UV-C disinfection. However, when the effect on the genetic materials was investigated, any quantifiable genomic DNA in terms of aphA and tetA was observed, unlike the UV-C treatment alone, where genomic and plasmid DNA still persisted after treatment.
5. Sunlight vs. artificial light HP-AOPs
Photodriven AOPs can also be implemented using solar radiation as a light source, thus saving energy costs. This option may be feasible for small WTPs. For medium-large WTPs, sunlight-driven photo-Fenton and AOPs are not a feasible solution due to the high surface area necessary for the reactors. Solar-driven AOPs have been widely investigated in CPC-based reactors (Fig. 3a), but this technology is quite expensive (the unit cost being estimated as high as 400 € per m2); therefore, cheaper solutions, such as the RPR system (estimated cost 10 € per m2),140 have been recently investigated for the tertiary treatment of urban wastewater by the SPF process.103–105 Though RPR systems can absorb sunlight less efficiently than CPC reactors, they are characterized by a larger treated volume/surface ratio, and the liquid depth can easily be varied to eventually increase the treatment capacity (Fig. 3b). However, although the RPR solution decreases the investment cost compared to CPC, surface footprint is still a limitation. Therefore, it is of interest to learn if and to which extent UV-C-driven AOPs perform better (reduced contact time and, consequently, reduced reactor surface area) than solar-driven ones.
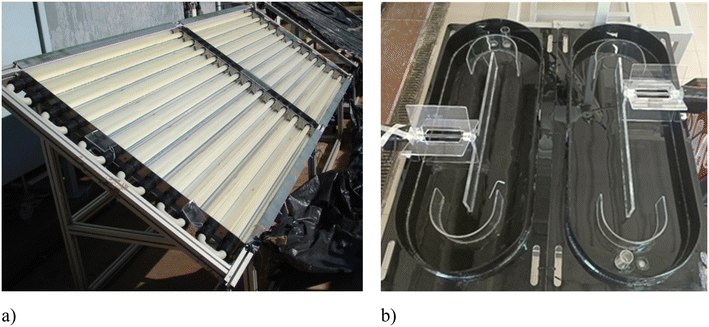 |
| Fig. 3 CPC-based reactor (a) and RPR plant (b). | |
Recently, iminodisuccinic acid (IDS) has been investigated in water and wastewater treatment by the photo-Fenton-like process as the complexing agent of Cu2+ (ref. 21) and Fe3+.141 Interestingly, UV-C/H2O2/Cu-IDS was more effective than the other investigated processes (UV-C/H2O2/Cu, UV-C/H2O2/Fe, and UV-C) in the inactivation of E. coli (complete inactivation (3.5
log units) in 10 min) at natural pH (7.8 ± 0.5) in real wastewater,21 while the sunlight/Fe-IDS/H2O2 process was found to be more effective than the UV-C-driven and pH 3 photo-Fenton processes in the degradation of phenol from aqueous solutions (Table 3).141
Table 3 Kinetic constant (k), R-squared (R2), and the half-life time (t1/2) for phenol (50 mg L−1, 0.53 mM) removal (as COD) by photo-Fenton and UV/H2O2: sunlight vs. UV-C, neutral (chelating agent) vs. acidic pH (data taken from,141Table 1, Copyright 2021, with permission from Elsevier)
Process |
Reagent s−1 (mM) |
R
2
|
k (1/min) |
t
1/2 (min) |
UV-C/Fe-IDS/H2O2 |
0.021/5.53 |
0.9745 |
−0.0073 |
68 |
Sunlight/Fe-IDS/H2O2 |
0.021/5.53 |
0.9891 |
−0.0114 |
44 |
UV-C/Fe2+/H2O2 (pH 3) |
0.021/5.53 |
0.9318 |
−0.0025 |
1000 |
Sunlight/Fe2+/H2O2 (pH 3) |
0.021/5.53 |
0.8726 |
−0.0029 |
500 |
UV-C/H2O2 |
5.53 |
0.9498 |
−0.0010 |
172 |
Sunlight/H2O2 |
5.53 |
0.9731 |
−0.0005 |
200 |
The higher efficiency of the photo-Fenton process under sunlight can be explained by the improved catalytic effect of the complex under longer wavelengths as they are able to overcome the inner filter effect.142 However, while for the other complexing agents, different works are available in the scientific literature, including the effect on CECs removal and comparisons among different chelating agents, such results are not yet available for IDS.
The sunlight/H2O2 process is less effective than the UV-C/H2O2 process because the photolytic cleavage of H2O2 into ˙OH is mainly due to the molar absorption coefficient of H2O2 (ε = 18.6 1/(M cm)) at λ = 254 nm, and UV-C radiation is only a small fraction of the solar spectrum. Such differences can be better appreciated by comparing the absorbance spectrum of an H2O2 solution (200 mg L−1) in deionized water, and the irradiance spectra of sunlight and a UV-C lamp, respectively (Fig. 4).
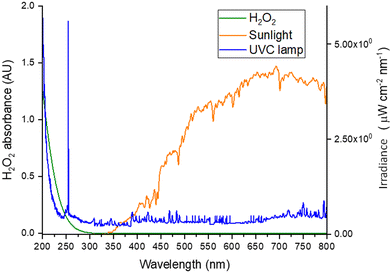 |
| Fig. 4 The absorbance spectrum of an H2O2 deionized water solution (200 mg L−1) and the irradiance spectra of sunlight (detected on the morning of a sunny day, July 7th 2022, at the Fisciano campus of University of Salerno (Italy) (40°76′N and 14°79′W)) and an UV-C lamp (16 W, with an emission peak at 254 nm, Sankyo Denky GT10T5L) were measured by an Ocean Optics (USA) spectrometer (model HR2000, equipped with a CC-3-UV-S cosine corrector with Spectralon as the diffusing material). | |
The higher performance of UV-C/H2O2 compared to the sunlight/H2O2 process was confirmed from the data in Table 2: 50 mg L−1 (0.53 mM) of phenol (measured as COD) was oxidized faster by UV-C/H2O2 (k = −0.0010 1/min; t1/2 = 172 min) rather than sunlight/H2O2 (k = −0.0005 1/min; t1/2 = 200 min).141 Despite such a limitation, the sunlight/H2O2 process has been successfully investigated as a disinfection process (internal photo Fenton mechanism)124 and, to some extent, for the removal of CECs from water and wastewater.118,120,121
The effect of the light source was also investigated in the photolysis of PAA as a tertiary treatment method of urban wastewater by evaluating its efficiency in terms of the inactivation of AR E. coli and degradation of CECs. In particular, the UV-C/PAA process was found to be effective in the degradation of chloramphenicol in deionized water (25 mg L−1 initial concentration, t1/2 = 44 min, 20 mg L−1 of PAA) but not effective with the sunlight/PAA process (t1/2 = 410 min, same chloramphenicol and PAA concentrations).90 The UV-C/PAA process was faster (QUV = 0.3 kJ L−1 and 0.2 mg PAA L−1 were sufficient to get the LOD) than the solar driven one (QUV = 4.4 kJ L−1 and 0.2 mg PAA L−1) in the inactivation of AR E. coli.82 However, longer exposure time and higher PAA initial dose were necessary to effectively remove the target CECs and, even in this case, the solar-driven process efficiency was lower than the UV-C-driven process.
6. Homogeneous vs. heterogeneous photodriven AOPs
Heterogeneous photodriven AOPs (HtP-AOPs) have been also investigated as the tertiary treatment of urban wastewater at the lab and pilot scale.83,143 However, their possible application to water and wastewater treatment at full scale is expected to require a longer time compared to HP-AOPs due to (i) the higher interference of real aqueous matrices, (ii) process limitations (e.g., low photoconversion efficiency), and (iii) technological issues (i.e., removal of the photocatalyst after treatment or its immobilization on a support, catalyst preparation method, reactor design and upscale, and energy consumption).6,97 Although progresses in materials science have contributed to improved photocatalytic activity, HtP-AOPs are still less effective than HP-AOPs.143–145 In particular, solar HtP-AOPs, namely those implementing semiconductors (such as TiO2) as the catalyst, have found to be less effective than homogenous solar-driven AOPs because, in addition to the abovementioned limitations, TiO2 poorly absorbs radiation in the solar spectrum. Despite the attempts to improve the process performance under solar light by expanding the absorption spectrum of the photocatalyst in the visible region through TiO2 doping with nitrogen (N-TiO2), the N-TiO2/sunlight process was less effective than the other investigated processes (sunlight/H2O2, SPF, and SPF with EDDS) in the removal of CECs from real secondary-treated urban wastewater.143 Even when TiO2 was used in the suspended form for the inactivation of a multidrug resistant E. coli strain at the pilot-scale in a compound parabolic collector reactor, sunlight/TiO2 (50 mg L−1) was found to be less effective (longer irradiation time requested for 5
log units inactivation) than SPF (Fe2+ = 0.090 mM (5.02 mg L−1), H2O2 = 0.294 mM, pH 4) and sunlight/H2O2 (0.588 mM) processes.120 However, SPF was found to be less effective than solar TiO2-based HtP-AOPs in the degradation of three CECs, but in this case, the experimental conditions were not optimized and SPF was operated at pH 5.5.83 Under these operating conditions, SPF also resulted in a higher environmental impact, evaluated through life cycle assessment (LCA) tool, when compared to other solar-driven AOPs, including heterogeneous ones, for the removal of three CECs (CBZ, diclofenac, and sulfamethoxazole) from a secondary-treated wastewater.146 Accordingly, although SPF, either with EDDS or at acidic pH, can be more effective than HtP-AOPs, it may result in a higher environmental impact; therefore, a comparison with the support of LCA is desirable to identify the most appropriate and sustainable solution for full-scale application.
7. HP-AOPs vs. conventional tertiary treatment methods
7.1 HP-AOPs vs. ozonation
When evaluating the possible implementation of HP-AOPs at full scale as a tertiary treatment method of urban wastewater, a comparison with the best available technologies, such as ozonation, is necessary.52 Though ozonation results in some concerns due to the formation of toxic byproducts (such as bromate, nitrosamines, formaldehyde, and acetaldehyde) and mutagenic activity,147 it is applied in central Europe countries (particularly in Switzerland and Germany) to remove CECs from urban wastewater.6 Therefore, to reduce toxic and mutagenic effects, a post-treatment with biological filtration or activated carbon (BAC) adsorption is applied,148 thus increasing the capital and management costs. The combination of ozonation with BAC allowed to achieve more than 90% removal for a wide range of CECs as well as a reduction of 70% of non-specific toxicity and more than 95% estrogenicity in full-scale wastewater reclamation plants located in Australia.148 The effect of BAC on AR control and pathogens as ozonation post-treatment was also investigated but did not significantly improve the ARGs (intl1 and sul1) and pathogens (but for C. perfringens and somatic coliphages) compared to the ozonation process alone.149
Solar-driven homogenous AOPs seem to be an attractive option alternative to ozonation for the tertiary treatment of urban wastewater in small WTPs. SPF with EDDS has been compared to the ozonation process in the inactivation of pathogens,104 CECs removal, and effect on the final toxicity.105 Sunlight/H2O2 and SPF with EDDS were operated at near neutral pH in RPRs and compared to the ozonation process at the pilot scale in the inactivation of three pathogens (namely, E. coli, Salmonella spp., and Enterococcus spp.) naturally occurring in secondary-treated urban wastewater.104 The inactivation rates were found to be in the following order of ozonation (83 mg O3 L−1 h−1) > sunlight/H2O2 (50 mg L−1) > SPF (1
:
1 molar ratio, 0.1 mM of Fe (5.58 mg L−1) and 50 mg L−1 of H2O2) for all the target pathogens. SPF with EDDS was also compared to ozonation (i) in the degradation of selected CECs (namely, caffeine, CBZ, diclofenac, sulfamethoxazole, and trimethoprim) at the initial concentration of 100 μg L−1 each and (ii) in terms of the effluent toxicity.105 Ozonation was faster than SPF to achieve 80% degradation of each CEC within the early 15 min (18.0 mg O3 L−1 consumed), but while the acute toxicity evaluated by AS did not show any toxic effect, highest acute toxicity to D. magna was observed in ozonated wastewater samples. Moreover, significant chronic toxicity was observed for almost all the analyzed samples though the processes successfully removed (80%) the target CECs; this result can be explained by the formation of oxidation intermediates more toxic than the parent compounds.69 SPF and sunlight/H2O2 process efficiency are drastically affected by radical scavengers such as carbonates; therefore, wastewater characterized by high carbonate concentrations (>70 mg L−1) would need pretreatment to decrease their concentration, thus increasing the treatment cost.
Ozonation was also compared to different HP-AOPs (namely, UV/H2O2, UV/Cl2, O3/UV, H2O2/O3/UV, and Cl2/O3/UV) operated at the pilot-scale as a tertiary treatment method of municipal wastewater in terms of energy efficiency, CECs (namely, CBZ, fluoxetine, gemfibrozil, primidone, sulfamethoxazole, and trimethoprim) removal, DBPs formation, and pathogens inactivation.150 Different ozone doses (1.5–9 mg L−1) and UV fluences (191–981 mJ cm−2) were investigated. Ozonation was the most energy-efficient process in terms of electrical energy per order (EEO) parameter for CECs oxidation (with UV/H2O2 and UV/Cl2 being the highest energy demanding processes). Among the ozone-based AOPs, only H2O2/O3/UV did not form the ozonation byproduct bromate. Ozonation (at 1.5–6 mg L−1 doses) was the least effective process to inactivate somatic coliphages, total coliform, E. coli, and enterococci, while HP-AOPs were the most effective ones, resulting in the complete inactivation of selected microorganisms (1.5 mg L−1 ozone dose and 191–465 mJ cm−2 UV fluence).
The abovementioned results show that ozonation is an effective option for the tertiary treatment of urban wastewater, but possible additional costs for a post-treatment unit to reduce effluent toxicity and ozonation byproducts as well as to improve pathogen inactivation should be evaluated before making the final decision.
7.2 HP-AOPs vs. chlorination
Chlorination is among the most-used wastewater disinfection processes at full scale. However, the concern for the formation of toxic byproducts has promoted the use of alternative disinfection processes, such PAA and UV radiation. HP-AOPs have also been investigated as possible alternatives to chlorination, but the higher cost compared to chlorination has not yet allowed their implementation at full scale. However, prospectively arguing, more stringent regulatory restrictions on WTPs effluents (namely, toxicity, DBPs, CECs removal, and AR control) may speed-up the implementation of HP-AOPs. In addition to the fact that they do not form regulated DBPs, other advantages have been observed. Although chlorination has resulted in faster inactivation kinetics of an indigenous multidrug resistant (MDR) E. coli strain in secondary-treated real urban wastewater compared to the sunlight/H2O2 process, it did not effectively inactivate bacterial cells that could regrow faster in post-treatment 48 h regrowth tests (Fig. 5).71
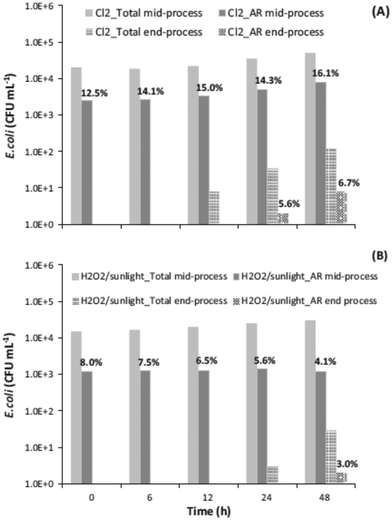 |
| Fig. 5 Regrowth of total and MDR E. coli after (A) chlorination (1 mg L−1) (initial total E. coli concentration 4.0 × 104 CFU mL−1 and initial MDR concentration 3.0 × 103 CFU mL−1) and (B) sunlight/H2O2 (50 mg L−1) (initial total E. coli concentration 2.0 × 104 CFU mL−1 and initial MDR concentration 1.0 × 103 CFU mL−1) (reprinted from ref. 71, Fig. 6, Copyright 2015, with permission from Elsevier). | |
The differences observed in the two investigated processes with regard to post-treatment regrowth can be ascribed to the different inactivation mechanisms of the disinfection processes. Xu et al. investigated the action of the chlorination process on E. coli and observed that membrane permeability damage, even at low doses of chlorine (<5 mg L−1), lead to the leakage of cellular adenosine triphosphate (ATP),151 while the effect on DNA (slight lesion) was observed only at high chlorine doses (>5 mg L−1). Moreover, 5 mg L−1 chlorine with 30 min contact time inhibited DNA damage repair function.
Unlike chlorination mechanisms, in light-assisted H2O2 disinfection, different works available in the scientific literature agree in identifying the light-enhanced internal photo-Fenton reaction as the inactivation mechanism.124 The prevailing inactivation mechanisms involve the following processes: i) light damages the DNA and enzymes responsible for its repair (direct action); ii) light disrupts ROS-scavenging enzymes in the cells (indirect action); (iii) H2O2 penetrates the cell; iv) iron into the cytoplasm reacts with H2O2 to start a (photo)Fenton reaction with light-reducing ferric to ferrous iron; (v) and the added H2O2 damages the cell membrane.
8. Comparison among the processes under realistic conditions and different end-points
In order to speed up the full-scale application of HP-AOPs, it is not sufficient to compare them with other processes and consolidated technologies, but it is more important to investigate the realistic conditions (namely, real wastewater, indigenous bacteria, and environmentally-relevant CECs concentrations) using different end-points (namely, CECs removal, pathogens inactivation, effluent toxicity, and DBPs formation (when relevant)) simultaneously.52 Ahmed et al. investigated the simultaneous removal of CECs, ARB, and ARGs by photo-Fenton at neutral pH (using EDDS as the chelating agent, 0.1
:
0.2
:
0.3 mM of Fe(III)
:
EDDS
:
H2O2) and demonstrated that the respective concentrations can be decreased to the limit of detection within 10, 30, and 15 min, respectively, in ultrapure water.114 But when the process was investigated in real wastewater, the detection limit was not achieved for ARB inactivation, even after 60 min treatment. In another work, SPF resulted in poor CECs removal when compared to other solar-driven AOPs in the simultaneous removal of CECs and biological contaminants (also including ARB and ARGs) from secondary-treated urban wastewater.83 This only apparent contrasting results compared to other evidences about SPF efficiency available in scientific literature can be explained by the experimental conditions, the process being operated without the addition of a chelating agent nor acidifying the solution to a pH value lower than 3, but adjusting the pH to a circumneutral value (5.5), which possibly resulted in iron precipitation, making the process less effective.
Sgroi et al. compared ozone-based and photodriven AOPs at the pilot scale as tertiary treatment methods of urban wastewater, using different end points (namely, CECs removal, energy efficiency, disinfection byproducts formation, and pathogen inactivation).150 While ozonation resulted in the most energy-efficient technology for the removal of the target CECs, the high ozone doses needed lead to the high formation of DBPs (namely, bromate and NDMA). In particular, the significant concentration of bromate (roughly 10 μg L−1, the limit set for drinking water in several countries) was detected for an ozone dose as high as 9 mg L−1, while a concentration lower than 10 ng L−1 NDMA (the notification value set by the California Department of Public Health (CDPH) in drinking water and in wastewater treated for potable reuse) was detected for all the ozone-based investigated processes. On the contrary, when ozone was combined with UV technology, the energy costs were significantly reduced compared to the UV/H2O2 and UV/Cl2 processes. The authors concluded that, considering all the end points, the UV/H2O2/O3 technology may represent a very promising option for the tertiary treatment of urban wastewater.
The limiting factor in the tertiary treatment method and consequently the operating conditions (namely, reagent doses and treatment time) depend on the secondary treatment (e.g., AS, MBR, and MBBR), the end-points, the scenario (e.g., effluent disposal vs. reuse), and regulation. Giannakis et al. investigated the effect of 5 photodriven (3 AOPs) processes on 3 different secondary treatment systems (namely, AS, MBBR, and CF) for the simultaneous inactivation of microorganisms, regrowth inhibition, and CECs removal.121 Using 3-log inactivation for microorganisms (analyzed through a non-selective agar, allowing the enumeration of different bacteria) and 80% removal of six CECs (according to Swiss legislation limits for micropollutants) as minimum thresholds, the best strategies in terms of the limiting factor (bacteria inactivation (and regrowth inhibition) vs. CECs removal) and treatment time could be defined for UV/H2O2 and photo-Fenton processes, respectively.
Table 4 summarizes the possible advantages, drawbacks, and recommendations for the application of HP-AOPs in the tertiary treatment of urban wastewater, according to the scientific literature reviewed in this manuscript.
Table 4 Possible advantages, drawbacks, and recommendations for the application of HP-AOPs in the tertiary treatment of urban wastewater
Advanced treatment |
Advantages |
Drawbacks |
Recommendations |
UV/H2O2 |
• Moderate-good CECs removal at lab/pilot scale |
• Limited efficiency and large surface area required when solar radiation is used as the light source |
• Toxicity should be monitored |
• Full scale evidence of high antibiotics removal |
• Possible formation of toxic oxidation intermediates |
• Effective as a disinfection process |
• Higher energy demand compared to ozonation |
• Sunlight/H2O2 can be more effective than SPF in the inactivation of microorganisms |
Photo-Fenton |
• SPF highly effective in CECs removal using chelating agents |
• Possible formation of toxic oxidation intermediates |
• Toxicity should be monitored |
• Quite effective as a disinfection process as well |
• To operate the process at neutral pH, the addition of chelating agents is necessary (increased operating costs) |
• SPF highly effective in ARGs removal at the lab scale under controlled conditions |
• Large surface area required for the solar-driven process |
UV/FC |
• High CECs removal |
• Formation of chlorination byproducts |
• Chlorination byproducts and toxicity should be monitored |
• Reduced formation of chlorination byproducts compared to chlorination process |
• Compliance of residual chlorine with limit for effluent disposal or reuse |
• Lower cost of sodium hypochlorite compared to hydrogen peroxide |
• Higher energy demand compared to ozonation |
• Sunlight/chlorine more effective than other solar-driven AOPs and UV-C in the inactivation of E. coli and Entero |
UV/PAA |
• Moderate-good CEC removal at lab/pilot scale under UV-C radiation |
• Less effective than UV/H2O2 in the removal of CECs |
• Toxicity should be monitored |
• Effective as a disinfection process |
• High TOC and scavenging effect, if high PAA dose is necessary |
• Not recommended when high PAA doses are necessary |
• Possible formation of toxic oxidation intermediates |
UV/PS and UV/PMS |
• Full scale evidence of high antibiotics removal efficiency |
• More sensitive to water matrix (pH, chloride, and inorganic carbon) than other HP-AOPs |
|
• No improvement/less effective than UV-C in the removal of ARGs in real secondary-treated wastewater |
UV/H2O2/O3 |
• High CECs removal |
• Possible formation of toxic oxidation intermediates and ozonation byproducts |
• Ozonation byproducts toxicity should be monitored |
• Reduced formation of ozonation byproducts compared to ozonation process |
• Higher energy demand compared to ozonation |
• Effective as disinfection process |
• Evidence at pilot scale of lower energy consumption compared to UV/H2O2 and UV/Cl2 |
Although the mineralization of the CECs is desirable because it would also mean that potentially toxic oxidation intermediates have been removed, it is in fact not feasible at all because it would entail high investment (higher volumes) and operating (higher dosages of reagents for longer reaction times) costs. Therefore, it is advisable to remove the CECs and, if after their removal, the toxicity is higher than the pre-treatment wastewater, the treatment time is extended until an effluent with a lower toxicity than the influent is achieved. According to the above discussion, process selection and design as well as the operating conditions should be a compromise among different end-points.
9. Conclusions
The actual and most relevant challenge in the tertiary treatment of urban wastewater is not the identification of the optimal and sustainable conditions to obtain the removal of a particularly refractory contaminant or a resistant microorganism, but rather obtaining the simultaneous removal or minimization of multiple contaminants under conditions that are relatively (compared to other treatments) more sustainable for the environment and safer for human health. Scientists have shown, also through pilot- and full-scale tests under realistic conditions, that HP-AOPs can effectively address the challenges in the tertiary treatment of urban wastewater and that the time for their full-scale application has almost come. Although it is hard to say to which extent they can minimize the risk of toxicity related to the release of residual concentrations of CECs or the spread of AR, we can say that they are more effective than conventional disinfection processes (such as chlorination, PAA, and UV-C radiation) and competitive with ozonation. On the other hand, the lack of a specific regulation or law in most countries on the removal of CECs and on the control of AR hinders the full-scale implementation of these processes because they are more expensive than conventional disinfection methods. It is unlikely that WTPs managers will implement more expensive processes than traditional ones even though, as highlighted in the article, they are not effective in removing CECs and controlling AR. Among the HP-AOPs reviewed in this manuscript, the UV/H2O2 process is already applied as a final step in potable water reuse treatment trains, but it may have higher energy demand compared to other possible solutions (such as ozonation); however, other end points should be evaluated when making the final decision, and LCA would be a useful tool to compare different solutions. SPF has been quite exhaustively investigated and can be successfully implemented at full scale in small WTPs in RPR, particularly when wastewater is characterized by a relatively low carbonate concentration (<70 mg L−1). That said, these HP-AOPs may be implemented in countries, such as Switzerland and Germany, where specific regulation is in force or concern for CECs and AR pushes WTPs managers in such direction, respectively.
Regarding the other HP-AOPs reviewed in this manuscript, a few steps are left to obtain exhaustive information/data about their possible implementation at full-scale as a tertiary treatment method of urban wastewater. To fill this gap, some advices for further investigation may be useful. In particular, the following 10 recommendations should be considered when designing the experimental plan to investigate an HP-AOP as a possible solution for the tertiary treatment of urban wastewater.
1. Scale-up of the investigated system (e.g., from lab to pilot);
2. Include tests on real wastewater;
3. Investigate the effect of the process on relevant CECs at real (or at least realistic for secondary-treated urban wastewater) concentrations. Accordingly, the occurrence, toxicity (eventually, availability of risk threshold values for humans), photosensitivity (direct photolysis), contribution of indirect photolysis to the degradation of the target CEC in real wastewater, possible refractoriness to conventional, as well as to new tertiary treatment methods should be considered when selecting the target CECs;
4. Investigate the effect on indigenous bacteria (and microorganisms in general);
5. Investigate the effect on antibiotic resistant determinants taking the possible AR transfer mechanisms into account;
6. Investigate the process efficiency using different end-points simultaneously;
7. Evaluate the impact of the investigated process using proper indicators (namely, ecological, microbiological, chemical, and toxicological ones) according to the final destination of the effluent (namely, reuse or disposal into different aquatic environments or into the soil);
8. Evaluate the investigated process taking international standards for effluent disposal or reuse into account;
9. Compare the investigated process with consolidated ones;
10. Use Life Cycle Assessment tools and multi-criteria analysis to compare the investigated processes.
Acronyms
AC | Activated carbon |
AR | Antibiotic resistance |
ARB | Antibiotic resistant bacteria |
ARGs | Antibiotic resistant genes |
AS | Activated sludge |
BAC | Biological activated carbon |
BATs | Best available technologies |
CA | Citric acid |
CBZ | Carbamazepine |
CECs | Contaminants of emerging concern |
CLF | Chloroform |
CPC | Compound parabolic collector |
DBPs | Disinfection byproducts |
EDDS | Ethylenediamine-N,N′-disuccinic acid |
FC | Free chlorine |
HP-AOPs | Homogeneous photodriven AOPs |
MBBR | Moving bed biofilm reactor |
MBR | Membrane biological reactor |
MPs | Microplastics |
NOM | Natural organic matter |
NPs | Nanoplastics |
NTA | Nitrilotriacetic acid |
OA | Oxalic acid |
PAA | Peracetic acid |
PMS | Peroxymonosulfate |
PS | Persulphate |
Q
UV
| Cumulative energy |
ROS | Reactive oxygen species |
RPR | Raceway pond reactor |
SPF | Solar photo-Fenton |
THMs | Trihalomethanes |
WL | Watch list |
WTP | Wastewater treatment plant |
Disclaimer
This manuscript reflects only the author's view and the European Commission/PRIMA are not responsible for any use that may be made of the information it contains.
Conflicts of interest
There are no conflicts to declare.
Acknowledgements
This paper is supported by the PRIMA programme under grant agreement No 1822, project “Decision support-based approach for Sustainable Water reuse application in Agricultural Production – DSWAP”. The PRIMA programme is supported by the European Union. The support from the University of Salerno through FARB-Rizzo 2021 project (ORSA219878) is also acknowledged. Finally, I'd like to thank Antonio Faggiano and Marco De Carluccio for the relevant measurements as well as for preparing Fig. 4.
References
- V. K. Parida, D. Saidulu, A. Majumder, A. Srivastava, B. Gupta and A. K. Gupta, Emerging contaminants in wastewater: A critical review on occurrence, existing legislations, risk assessment, and sustainable treatment alternatives, J. Environ. Chem. Eng., 2021, 9, 105966, DOI:10.1016/j.jece.2021.105966.
- S. Fan, B. Wang, H. Liu, S. Gao, T. Li, S. Wang, Y. Liu, X. Liu and Y. Wan, Trophodynamics of Organic Pollutants in Pelagic and Benthic Food Webs of Lake Dianchi: Importance of Ingested Sediment As Uptake Route, Environ. Sci. Technol., 2017, 51, 14135–14143, DOI:10.1021/acs.est.7b03681.
- I. Michael, L. Rizzo, C. S. McArdell, C. M. Manaia, C. Merlin, T. Schwartz, C. Dagot and D. Fatta-Kassinos, Urban wastewater treatment plants as hotspots for the release of antibiotics in the environment: A review, Water Res., 2013, 47, 957–995, DOI:10.1016/j.watres.2012.11.027.
- L. Rizzo, C. Manaia, C. Merlin, T. Schwartz, C. Dagot, M. C. Ploy, I. Michael and D. Fatta-Kassinos, Urban wastewater treatment plants as hotspots for antibiotic resistant bacteria and genes spread into the environment: a review, Sci. Total Environ., 2013, 447, 345–360, DOI:10.1016/j.scitotenv.2013.01.032.
- S. Franzellitti, L. Canesi, M. Auguste, R. Wathsala and E. Fabbri, Microplastic exposure and effects in aquatic organisms: A physiological perspective, Environ. Toxicol. Pharmacol., 2019, 68, 37–51, DOI:10.1016/j.etap.2019.03.009.
- L. Rizzo, S. Malato, D. Antakyali, V. G. Beretsou, M. B. Đolić, W. Gernjak, E. Heath, I. Ivancev-Tumbas, P. Karaolia, A. R. Lado Ribeiro, G. Mascolo, C. S. McArdell, H. Schaar, A. M. T. Silva and D. Fatta-Kassinos, Consolidated vs new advanced treatment methods for the removal of contaminants of emerging concern from urban wastewater, Sci. Total Environ., 2019, 655, 986–1008, DOI:10.1016/j.scitotenv.2018.11.265.
- J. Sun, X. Dai, Q. Wang, M. C. M. van Loosdrecht and B. J. Ni, Microplastics in wastewater treatment plants: detection, occurrence and removal, Water Res., 2019, 152, 21–37, DOI:10.1016/j.watres.2018.12.050.
- P. Krzeminski, M. C. Tomei, P. Karaolia, A. Langenhoff, C. M. R. Almeida, E. Felis, F. Gritten, H. R. Andersen, T. Fernandes, C. M. Manaia, L. Rizzo and D. Fatta-Kassinos, Performance of secondary wastewater treatment methods for the removal of contaminants of emerging concern implicated in crop uptake and antibiotic resistance spread: A review, Sci. Total Environ., 2019, 648, 1052–1081, DOI:10.1016/j.scitotenv.2018.08.130.
- I. Michael-Kordatou, P. Karaolia and D. Fatta-Kassinos, The role of operating parameters and oxidative damage mechanisms of advanced chemical oxidation processes in the combat against antibiotic-resistant bacteria and resistance genes present in urban wastewater, Water Res., 2018, 129, 208–230, DOI:10.1016/j.watres.2017.10.007.
- Commission Imlementing Decision (EU) 2015/495 of 20 March 2015 Establishing a Watch List of Substances for Union-wide Monitoring in the Field of Water Policy Pursuant to Directive 2008/105/EC of the European Parliament and of the Council, pp. 40–42.
- Regulation (EU) 2020/741 of the European parliament and of the council of 25 May 2020 on minimum requirements for water reuse, Official Journal of the European Union L 177/32, 5.6.2020.
- J. Pignatello, E. Oliveros and A. MacKay, Advanced Oxidation Processes for Organic Contaminant Destruction Based on the Fenton Reaction and Related Chemistry, Crit. Rev. Environ. Sci. Technol., 2006, 36(1), 1–84, DOI:10.1080/10643380500326564.
- I. Sciscenko, S. Garcia-Ballesteros, C. Sabater, M. A. Castillo, C. Escudero-Oñate, I. Oller and A. Arques, Monitoring photolysis and (solar photo)-Fenton of enrofloxacin by a methodology involving EEM-PARAFAC and bioassays: Role of pH and water matrix, Sci. Total Environ., 2020, 719, 137331, DOI:10.1016/j.scitotenv.2020.137331.
- N. López-Vinent, A. Cruz-Alcalde, J. Giménez and S. Esplugas, Mixtures of chelating agents to enhance photo-Fenton process at natural pH: Influence of wastewater matrix on micropollutant removal and bacterial inactivation, Sci. Total Environ., 2021, 786, 147416, DOI:10.1016/j.scitotenv.2021.147416.
- N. Klamerth, L. Rizzo, S. Malato, M. I. Maldonado, A. Agüera and A. R. Fernández-Alba, Degradation of fifteen emerging contaminants at μg L−1 initial concentrations by mild solar photo-Fenton in MWTP effluents, Water Res., 2010, 44, 545–554, DOI:10.1016/j.watres.2009.09.059.
- U. J. Ahile, R. A. Wuana, A. U. Itodo, R. Sha'Ato and R. F. Dantas, A review on the use of chelating agents as an alternative to promote photo-Fenton at neutral pH: Current trends, knowledge gap and future studies, Sci. Total Environ., 2020, 710, 134872, DOI:10.1016/j.scitotenv.2019.134872.
- P. Prete, A. Fiorentino, L. Rizzo, A. Proto and R. Cucciniello, Review of aminopolycarboxylic acids-based metal complexes application to water and wastewater treatment by (photo-)Fenton process at neutral pH, Curr. Opin. Green Sustainable Chem., 2021, 28, 100451, DOI:10.1016/j.cogsc.2021.100451.
- G. Iervolino, V. Vaiano, D. Sannino, L. Rizzo, A. Galluzzi, M. Polichetti, G. Pepe and P. Campiglia, Hydrogen production from glucose degradation in water and wastewater treated by Ru-LaFeO3/Fe2O3 magnetic particles photocatalysis and heterogeneous photo-Fenton, Int. J. Hydrogen Energy, 2018, 43, 2184–2196, DOI:10.1016/j.ijhydene.2017.12.071.
- S. Ben Ayed, M. Azam, S. I. Al Resayes, F. Ayari and L. Rizzo, Cationic dye degradation and real textile wastewater treatment by heterogeneous photo Fenton using a novel natural catalyst, Catalysts, 2021, 11, 1358, DOI:10.3390/catal11111358.
- A. D. Bokare and W. Choi, Review of iron-free Fenton-like systems for activating H2O2 in advanced oxidation processes, J. Hazard. Mater., 2014, 275, 121–135, DOI:10.1016/j.jhazmat.2014.04.054.
- A. Fiorentino, R. Cucciniello, A. Di Cesare, D. Fontaneto, P. Prete, L. Rizzo, G. Corno and A. Proto, Disinfection of urban wastewater by a new photo-Fenton like process using Cu-iminodisuccinic acid complex as catalyst at neutral pH, Water Res., 2018, 146, 206–215, DOI:10.1016/j.watres.2018.08.024.
- S. Vilhunen, M. Vilve, M. Vepsäläinen and M. Sillanpää, Removal of organic matter from a variety of water matrices by UV photolysis and UV/H2O2 method, J. Hazard. Mater., 2010, 179, 776–782, DOI:10.1016/j.jhazmat.2010.03.070.
- A. S. Stasinakis, Use of selected advanced oxidation processes ({AOPs}) for wastewater treatment - a mini review, Global NEST J., 2008, 10, 376–385, DOI:10.30955/gnj.000598.
-
S. Khan and A. Branch, Potable Water Reuse - What can Australia learn from global experience? WaterRA Project #3039 Final Report, © Water Research Australia Limited 2019, ISBN 978-1-921732-50-8.
- M. Kwon, A. Royce, Y. Gong, K. P. Ishida and M. I. Stefan, UV/chlorine vs. UV/H2O2 for water reuse at Orange County Water District, CA: a pilot study, Environ. Sci.: Water Res. Technol., 2020, 6, 2416, 10.1039/d0ew00316f.
- J. Fang, Y. Fu and C. Shang, The roles of reactive species in micropollutant degradation in the UV/free chlorine system, Environ. Sci. Technol., 2014, 48, 1859–1868, DOI:10.1021/es4036094.
- G. Cerreta, M. A. Roccamante, I. Oller, S. Malato and L. Rizzo, Contaminants of emerging concern removal from real wastewater by UV/free chlorine process: A comparison with solar/free chlorine and UV/H2O2 at pilot scale, Chemosphere, 2019, 236, 124354, DOI:10.1016/j.chemosphere.2019.124354.
- D. Wang, J. R. Bolton and R. Hofmann, Medium pressure UV combined with chlorine advanced oxidation for trichloroethylene destruction in a model water, Water Res., 2012, 46, 4677–4686, DOI:10.1016/j.watres.2012.06.007.
- K. Guo, Z. Wu, S. Yan, B. Yao, W. Song, Z. Hua, X. Zhang, X. Kong, X. Li and J. Fang, Comparison of the UV/chlorine and UV/H2O2 processes in the degradation of PPCPs in simulated drinking water and wastewater: kinetics, radical mechanism and energy requirements, Water Res., 2018, 147, 184–194, DOI:10.1016/j.watres.2018.08.048.
- Z. Hua, K. Guo, X. Kong, S. Lin, Z. Wu, L. Wang, H. Huang and J. Fang, PPCP degradation and DBP formation in the solar/free chlorine system: effects of pH and dissolved oxygen, Water Res., 2019, 150, 77–85, DOI:10.1016/j.watres.2018.11.041.
- G. Cerreta, M. A. Roccamante, P. Plaza-Bolaños, I. Oller, A. Aguera, S. Malato and L. Rizzo, Advanced treatment of urban wastewater by UV-C/free chlorine process: Micro-pollutants removal and effect of UV-C radiation on trihalomethanes formation, Water Res., 2020, 169, 115220, DOI:10.1016/j.watres.2019.115220.
- M. Antonelli, A. Turolla, V. Mezzanotte and C. Nurizzo, Peracetic acid for secondary effluent disinfection: a comprehensive performance assessment, Water Sci. Technol., 2013, 68(12), 2638–2644, DOI:10.2166/wst.2013.542.
- A. Di Cesare, E. M. Eckert, S. D'Urso, R. Bertoni, D. C. Gillan, R. Wattiez and G. Corno, Co-occurrence of integrase 1, antibiotic and heavy metal resistance genes in municipal wastewater treatment plants, Water Res., 2016, 94, 208–214, DOI:10.1016/j.watres.2016.02.049.
- F. Formisano, A. Fiorentino, L. Rizzo, M. Carotenuto, L. Pucci, M. Giugni and G. Lofrano, Inactivation of Escherichia coli and Enterococci in urban wastewater by sunlight/PAA and sunlight/H2O2 processes, Process Saf. Environ. Prot., 2016, 104, 178–184, DOI:10.1016/j.psep.2016.09.003.
- C. Caretti and C. Lubello, Wastewater disinfection with PAA and UV combined treatment: a pilot plant study, Water Res., 2003, 37, 2365–2371, DOI:10.1016/S0043-1354(03)00025-3.
- J. Koivunen and H. Heinonen-Tanski, Inactivation of enteric microorganisms with chemical disinfectants, UV irradiation and combined chemical/UV treatments, Water Res., 2005, 39, 1519–1526, DOI:10.1016/j.watres.2005.01.021.
- M. Cai, P. Sun, L. Zhang and C.-H. Huang, UV/Peracetic acid for degradation of pharmaceuticals and reactive species evaluation, Environ. Sci. Technol., 2017, 51, 14217–14224, DOI:10.1021/acs.est.7b04694.
- S. Waclawek, H. V. Lutze, K. Grubel, V. V. T. Padil, M. Cernik and D. D. Dionysiou, Chemistry of persulfates in water and wastewater treatment: A review, Chem. Eng. J., 2017, 330, 44–62, DOI:10.1016/j.cej.2017.07.132.
- I. A. Ike, K. G. Linden, J. D. Orbell and M. Duke, Critical review of the science and sustainability of persulphate advanced oxidation processes, Chem. Eng. J., 2018, 338, 651–669, DOI:10.1016/j.cej.2018.01.034.
- J. Lee, U. von Gunten and J.-H. Kim, Persulfate-Based Advanced Oxidation: Critical Assessment of Opportunities and Roadblocks, Environ. Sci. Technol., 2020, 54, 3064–3081, DOI:10.1021/acs.est.9b07082.
- Y. Q. Zhang, J. F. Zhang, Y. J. Xiao, V. W. C. Chang and T. T. Lim, Kinetic and mechanistic investigation of azathioprine degradation in water by UV, UV/H2O2 and UV/persulfate, Chem. Eng. J., 2016, 302, 526–534, DOI:10.1016/j.cej.2016.05.085.
- Y. Luo, W. Guo, H. H. Ngo, L. D. Nghiem, F. I. Hai, J. Zhang, S. Liang and X. C. Wang, A review on the occurrence of micropollutants in the aquatic environment and their fate and removal during wastewater treatment, Sci. Total Environ., 2014, 473–474, 619–641, DOI:10.1016/j.scitotenv.2013.12.06.
- T. Malchi, Y. Maor, G. Tadmor, M. Shenker and B. Chefetz, Irrigation of Root Vegetables with Treated Wastewater: Evaluating Uptake of Pharmaceuticals and the Associated Human Health Risks, Environ. Sci. Technol., 2014, 48, 9325–9333, DOI:10.1021/es5017894.
- R. Delli Compagni, M. Gabrielli, F. Polesel, A. Turolla, S. Trapp, L. Vezzaro and M. Antonelli, Risk assessment of contaminants of emerging concern in the context of wastewater reuse for irrigation: An integrated modelling approach, Chemosphere, 2020, 242, 125185, DOI:10.1016/j.chemosphere.2019.125185.
- S. Mansilla, J. Portugal, J. M. Bayona, V. Matamoros, A. M. Leiva, G. Vidal and B. Piña, Compounds of emerging concern as new plant stressors linked to water reuse and biosolid application in agriculture, J. Environ. Chem. Eng., 2021, 9, 105198, DOI:10.1016/j.jece.2021.105198.
- L. Rizzo, R. Krätke, J. Linders, M. Scott, M. Vighi and P. de Voogt, Proposed EU minimum quality requirements for water reuse in agricultural irrigation and aquifer recharge: SCHEER scientific advice, Curr. Opin. Environ. Sci. Health, 2018, 2, 7–11, DOI:10.1016/j.coesh.2017.12.004.
-
FOEN, Water quality: revision of the water protection act, 2015, https://www.bafu.admin.ch/bafu/fr/home/themes/formation/communiques.msg-id-59323.html Search PubMed.
- Commission Implementing Decision (EU) 2018/840 of 5 June 2018 establishing a watch list of substances for Union-wide monitoring in the field of water policy pursuant to Directive 2008/105/EC of the European Parliament and of the Council and repealing Commission Implementing Decision (EU) 2015/495 (notified under document C(2018) 3362).
-
L. Gomez Cortes, D. Marinov, I. Sanseverino, A. Navarro Cuenca, M. Niegowska, E. Porcel Rodriguez and T. Lettieri, Selection of substances for the 3rd Watch List under the Water Framework Directive, JRC Technical Report 2020, DOI:10.2760/194067.
- L. F. Angeles, R. A. Mullen, I. J. Huang, C. Wilson, W. Khunjar, H. I. Sirotkin, A. E. McElroy and D. S. Aga, Assessing pharmaceutical removal and reduction in toxicity provided by advanced wastewater treatment systems, Environ. Sci.: Water Res. Technol., 2020, 6, 62–77, 10.1039/c9ew00559e.
- S. Galafassi, R. Sabatino, M. Belen Sathicq, E. M. Eckert, D. Fontaneto, G. Dalla Fontana, R. Mossotti, G. Corno, P. Volta and A. Di Cesare, Contribution of microplastic particles to the spread of resistances and pathogenic bacteria in treated wastewaters, Water Res., 2021, 201, 117368, DOI:10.1016/j.watres.2021.117368.
- L. Rizzo, W. Gernjak, P. Krzeminski, S. Malato, C. S. McArdell, J. A. Sanchez Perez, H. Schaar and D. Fatta-Kassinos, Best available technologies and treatment trains to address current challenges in urban wastewater reuse for irrigation of crops in EU countries, Sci. Total Environ., 2020, 710, 136312, DOI:10.1016/j.scitotenv.2019.136312.
- J. Davies and D. Davies, Origins and Evolution of Antibiotic Resistance, Microbiol. Mol. Biol. Rev., 2010, 74, 417–433, DOI:10.1128/MMBR.00016-10.
- D. Cacace, D. Fatta-Kassinos, C. M. Manaia, E. Cytryn, N. Kreuzinger, L. Rizzo, P. Karaolia, T. Schwartz, J. Alexander, C. Merlin, H. Garelick, H. Schmitt, D. de Vries, C. U. Schwermer, S. Meric, C. B. Ozkal, M.-N. Pons, D. Kneis and T. U. Berendonk, Antibiotic resistance genes in treated wastewater and in the receiving water bodies: A pan-European survey of urban settings, Water Res., 2019, 162, 320–330, DOI:10.1016/j.watres.2019.06.039.
- C. Seiler and T. Berendonk, Heavy metal driven co-selection of antibiotic resistance in soil and water bodies impacted by agriculture and aquaculture, Front. Microbiol., 2012, 3, 399, DOI:10.3389/fmicb.2012.00399.
- X. Liu, H. Wang, L. L. Li, C. Deng, Y. Chen, H. Ding and Z. Yu, Do microplastic biofilms promote the evolution and co-selection of antibiotic and metal resistance genes and their associations with bacterial communities under antibiotic and metal pressures?, J. Hazard. Mater., 2022, 424(Part A), 127285, DOI:10.1016/j.jhazmat.2021.127285.
- C. J. H. von Wintersdorff, J. Penders, J. M. van Niekerk, N. D. Mills, S. Majumder, L. B. van Alphen, P. H. M. Savelkoul and P. F. G. Wolffs, Dissemination of Antimicrobial Resistance in Microbial Ecosystems through Horizontal Gene Transfer, Front. Microbiol., 2016, 7, 173, DOI:10.3389/fmicb.2016.00173.
- Q. Jiang, M. Feng, C. Ye and X. Yu, Effects and relevant mechanisms of non-antibiotic factors on the horizontal transfer of antibiotic resistance genes in water environments: A review, Sci. Total Environ., 2022, 806, 150568, DOI:10.1016/j.scitotenv.2021.150568.
- M. Munir, K. Wong and I. Xagoraraki, Release of antibiotic resistant bacteria and genes in the effluent and biosolids of five wastewater utilities in Michigan, Water Res., 2011, 45, 681–693, DOI:10.1016/j.watres.2010.08.033.
- C. W. McKinney and A. Pruden, Ultraviolet disinfection of antibiotic resistant bacteria and their antibiotic resistance genes in Water and wastewater, Environ. Sci. Technol., 2012, 46, 13393–13400, DOI:10.1021/es303652q.
- A. Di Cesare, G. Corno, C. M. Manaia and L. Rizzo, Impact of disinfection processes on bacterial community in urban wastewater: Should we rethink microbial assessment methods?, J. Environ. Chem. Eng., 2020, 8, 104393, DOI:10.1016/j.jece.2020.104393.
- M. Jin, L. Liu, D. Wang, D. Yang, W. Liu, J. Yin, Z. Yang, H. Wang, Z. Qiu, Z. Shen, D. Shi, H. Li, J. Guo and J. Li, Chlorine disinfection promotes the exchange of antibiotic resistance genes across bacterial genera by natural transformation, ISME J., 2020, 14(7), 1847–1856, DOI:10.1038/s41396-020-0656-9.
- S. K. Dong, N. Massalha, M. J. Plew and T. H. Nguyen, The impact of disinfection Ct values on cytotoxicity of agricultural wastewaters: Ozonation vs. chlorination, Water Res., 2018, 144, 482–490, DOI:10.1016/j.watres.2018.07.065.
- A. Costa Miranda, M. Lepretti, L. Rizzo, I. Caputo, V. Vaiano, O. Sacco, W. Silva Lopes and D. Sannino, Surface water disinfection by chlorination and advanced oxidation processes: Inactivation of an antibiotic resistant E. coli strain and cytotoxicity evaluation, Sci. Total Environ., 2016, 554–555, 1–6, DOI:10.1016/j.scitotenv.2016.02.189.
- I. C. Iakovides, K. Manoli, P. Karaolia, I. Michael-Kordatou, C. M. Manaia and D. Fatta-Kassinos, Reduction of antibiotic resistance determinants in urban wastewater by ozone: Emphasis on the impact of wastewater matrix towards the inactivation kinetics, toxicity and bacterial regrowth, J. Hazard. Mater., 2021, 420, 126527, DOI:10.1016/j.jhazmat.2021.126527.
- T. Kistemann, E. Rind, A. Rechenburg, C. Koch, T. Claßen, S. Herbst, I. Wienand and M. Exner, A comparison of efficiencies of microbiological pollution removal in six sewage treatment plants with different treatment systems, Int. J. Hyg. Environ. Health, 2008, 211, 534–545, DOI:10.1016/j.ijheh.2008.04.003.
- A. Di Cesare, D. Fontaneto, J. Doppelbauer and G. Corno, Fitness and recovery of bacterial communities and antibiotic resistance genes in urban wastewaters exposed to classical disinfection treatments, Environ. Sci. Technol., 2016, 50, 10153–10161, DOI:10.1021/acs.est.6b02268.
- L. Domínguez Henao, A. Turolla and M. Antonelli, Disinfection by-products formation and ecotoxicological effects of effluents treated with peracetic acid: A review, Chemosphere, 2018, 213, 25–40, DOI:10.1016/j.chemosphere.2018.09.005.
- L. Rizzo, Bioassays as a tool for evaluating advanced oxidation processes in water and wastewater treatment, Water Res., 2011, 45, 4311–4340, DOI:10.1016/j.watres.2011.05.035.
- M. C. V. M. Starling, P. P. Souza, A. Le Person, C. C. Amorim and J. Criquet, Intensification of UV-C treatment to remove emerging contaminants by UV-C/H2O2 and UV-C/S2O82?: Susceptibility to photolysis and investigation of acute toxicity, Chem. Eng. J., 2019, 376, 120856, DOI:10.1016/j.cej.2019.01.135.
- A. Fiorentino, G. Ferro, M. C. Alferez, M. I. Polo-López, P. Fernández-Ibañez and L. Rizzo, Inactivation and regrowth of multidrug resistant bacteria in urban wastewater after disinfection by solar-driven and chlorination processes, J. Photochem. Photobiol., B, 2015, 148, 43–50, DOI:10.1016/j.jphotobiol.2015.03.029.
- H. Selcuk, L. Rizzo, N. Nikolaou, S. Meric, M. Bekbolet and V. Belgiorno, DBPs formation and toxicity monitoring in different origin water treated by ozone-alum/PACl coagulation, Desalination, 2007, 210, 31–43, DOI:10.1016/j.desal.2006.05.030.
- U. von Gunten, E. Salhi, C. K. Schmidt and W. A. Arnold, Kinetics and mechanisms of N nitrosodimethylamine formation upon ozonation of N,N dimethylsulfamide-containing waters: bromide catalysis, Environ. Sci. Technol., 2010, 44, 5762–5768, DOI:10.1021/es1011862.
- M. Wang, M. Ateia, D. Dion Awfa and C. Yoshimura, Regrowth of bacteria after light-based disinfection - What we know and where we go from here, Chemosphere, 2021, 268, 128850, DOI:10.1016/j.chemosphere.2020.128850.
-
P. Drechsel, D. D. Mara, C. Bartone and S. M. Scheierling, Improving wastewater use in agriculture: an emerging priority, World Bank Policy Research Working Paper, No. 5412, 2010, p. 111, DOI:10.1596/1813-9450-5412.
- N. V. Paranychianakis, M. Salgot, S. A. Snyder and A. N. Angelakis, Water reuse in EU states: necessity for uniform criteria to mitigate human and environmental risks, Crit. Rev. Environ. Sci. Technol., 2015, 45, 1409–1468, DOI:10.1080/10643389.2014.955629.
- A. L. Andrady, The plastic in microplastics: a review, Mar. Pollut. Bull., 2017, 119(1), 12–22, DOI:10.1016/j.marpolbul.2017.01.082.
- J. P. Da Costa, P. S. M. Santos, A. C. Duarte and T. Rocha-Santos, (Nano)plastics in the environment e sources, fates and effects, Sci. Total Environ., 2016, 566–567, 15–26, DOI:10.1016/j.scitotenv.2016.05.041.
- M. Enfrin, L. F. Dumée and J. Lee, Nano/microplastics in water and wastewater treatment processes – Origin, impact and potential solutions, Water Res., 2019, 161, 621–638, DOI:10.1016/j.watres.2019.06.049.
- H. Hidayaturrahman and T. G. Lee, A study on characteristics of microplastic in wastewater of South Korea: identification, quantification, and fate of microplastics during treatment process, Mar. Pollut. Bull., 2019, 146, 696–702, DOI:10.1016/j.marpolbul.2019.06.071.
- S. Galafassi, A. Di Cesare, L. Di Nardo, R. Sabatino, A. Valsesia, F. Sirio Fumagalli, G. Corno and P. Volta, Microplastic retention in small and medium municipal wastewater treatment plants and the role of the disinfection, Environ. Sci. Pollut. Res., 2022, 29(7), 10535–10546, DOI:10.1007/s11356-021-16453-2.
- L. Rizzo, T. Agovino, S. Nahim-Granados, M. Castro-Alférez, P. Fernández-Ibáñez and M. I. Polo-López, Tertiary treatment of urban wastewater by solar and UV-C driven advanced oxidation with peracetic acid: effect on contaminants of emerging concern and antibiotic resistance, Water Res., 2019, 149, 272–281, DOI:10.1016/j.watres.2018.11.031.
- N. F. F. Moreira, C. Narciso-da-Rocha, M. Inmaculada Polo-Lopez, L. M. Pastrana-Martínez, J. L. Faria, C. M. Manaia, P. Fernandez-Ibanez, O. C. Nunes and A. M. T. Silva, Solar treatment (H2O2, TiO2-P25 and GO-TiO2 photocatalysis, photo-Fenton) of organic micropollutants, human pathogen indicators, antibiotic resistant bacteria and related genes in urban wastewater, Water Res., 2018, 135, 195–206, DOI:10.1016/j.watres.2018.01.064.
- K. Kowalska, G. Maniakova, M. Carotenuto, O. Sacco, V. Vaiano, G. Lofrano and L. Rizzo, Removal of carbamazepine, diclofenac and trimethoprim by solar driven advanced oxidation processes in a compound triangular collector based reactor: A comparison between homogeneous and heterogeneous processes, Chemosphere, 2020, 238, 124665, DOI:10.1016/j.chemosphere.2019.124665.
- R. Matta, S. Tlili, S. Chiron and S. Barbati, Removal of carbamazepine from urban wastewater by sulfate radical oxidation, Environ.
Chem. Lett., 2011, 9, 347–353, DOI:10.1007/s10311-010-0285-z.
- E. Lee, C. M. Glover and F. L. Rosario-Ortiz, Photochemical Formation of Hydroxyl Radical from Effluent Organic Matter: Role of Composition, Environ. Sci. Technol., 2013, 47, 12073–12080, DOI:10.1021/es402491t.
- S. Yan, B. Yao, L. Lian, X. Lu, S. A. Snyder, R. Li and W. Song, Development of Fluorescence Surrogates to Predict the Photochemical Transformation of Pharmaceuticals in Wastewater Effluents, Environ. Sci. Technol., 2017, 51, 2738–2747, DOI:10.1021/acs.est.6b05251.
- M. Figueredo, E. M. Rodríguez, E. M. Cordero and F. J. Beltrán, UVA LEDs and solar light photocatalytic oxidation/ozonation as a tertiary treatment using supported TiO2: With an eye on the photochemical properties of the secondary effluent, J. Environ. Chem. Eng., 2022, 10, 107371, DOI:10.1016/j.jece.2022.107371.
- L. Wojnárovits, T. Tóth and E. Takács, Rate constants of carbonate radical anion reactions with molecules of environmental interest in aqueous solution: A review, Sci. Total Environ., 2020, 717, 137219, DOI:10.1016/j.scitotenv.2020.137219.
- L. Rizzo, G. Lofrano, C. Gago, T. Bredneva, P. Iannece, M. Pazos, N. Krasnogorskaya and M. Carotenuto, Antibiotic contaminated water treated by photo driven advanced oxidation processes: ultraviolet/H2O2 Vs ultraviolet/peracetic acid, J. Cleaner Prod., 2018, 205, 67–75, DOI:10.1016/j.jclepro.2018.09.101.
- A. R. Lado Ribeiro, N. F. F. Moreira, G. Li Puma and A. M. T. Silva, Impact of water matrix on the removal of micropollutants by advanced oxidation technologies, Chem. Eng. J., 2019, 363, 155–173, DOI:10.1016/j.cej.2019.01.080.
- Z. Palková, Multicellular microorganisms: laboratory versus nature, EMBO Rep., 2004, 5(5), 470–476, DOI:10.1038/sj.embor.7400145.
- I. Zammit, R. B. M. Marano, V. Vaiano, E. Cytryn and L. Rizzo, Changes in antibiotic resistance gene levels in soil after irrigation with treated wastewater: a comparison between heterogeneous photocatalysis and chlorination, Environ. Sci. Technol., 2020, 54, 7677–7686, DOI:10.1021/acs.est.0c01565.
- A. Di Cesare, M. De Carluccio, E. M. Eckert, D. Fontaneto, A. Fiorentino, G. Corno, P. Prete, R. Cucciniello, A. Proto and L. Rizzo, Combination of flow cytometry and molecular analysis to monitor the effect of UVC/H2O2 vs UVC/H2O2/Cu-IDS processes on pathogens and antibiotic resistant genes in secondary wastewater effluents, Water Res., 2020, 184, 116194, DOI:10.1016/j.watres.2020.116194.
- P. Karaolia, I. Michael, I. García-Fernández, A. Agüera, S. Malato, P. Fernández-Ibáñez and D. Fatta-Kassinos, Reduction of clarithromycin and sulfamethoxazole resistant Enterococcus by pilot-scale solar-driven Fenton oxidation, Sci. Total Environ., 2014, 468–469, 19–27, DOI:10.1016/j.scitotenv.2013.08.027.
- S. Giannakis, T. M. Le, J. M. Entenza and C. Pulgarin, Solar photo-Fenton disinfection of 11 antibiotic-resistant bacteria (ARB) and elimination of representative AR genes. Evidence that antibiotic resistance does not imply resistance to oxidative treatment, Water Res., 2018, 143, 334–345, DOI:10.1016/j.watres.2018.06.062.
- G. Iervolino, I. Zammit, V. Vaiano and L. Rizzo, Limitations and Prospects for Wastewater Treatment by UV and Visible-Light-Active Heterogeneous Photocatalysis: A Critical Review, Top. Curr. Chem., 2020, 378, 7, DOI:10.1007/s41061-019-0272-1.
- P. Villegas-Guzman, S. Giannakis, R. A. Torres-Palma and C. Pulgarin, Remarkable enhancement of bacterial inactivation in wastewater through promotion of solar photo-Fenton at near-neutral pH by natural organic acids, Appl. Catal., B, 2017, 205, 219–227, DOI:10.1016/j.apcatb.2016.12.021.
- G. D. Silva, E. O. Marson, L. L. Batista, C. Ueira-Vieira, M. C. V. M. Starling and A. G. Trovó, Contrasting the performance of photo-Fenton at neutral pH in the presence of different organic iron-complexes using hydrogen peroxide or persulfate as oxidants for naproxen degradation and removal of antimicrobial activity, Process Saf. Environ. Prot., 2021, 147, 798–807, DOI:10.1016/j.psep.2021.01.005.
- P. Karaolia, I. Michael-Kordatou, E. Hapeshi, J. Alexander, T. Schwartz and D. Fatta-Kassinos, Investigation of the potential of a Membrane BioReactor followed by solar Fenton oxidation to remove antibiotic-related microcontaminants, Chem. Eng. J., 2017, 310, 491–502, DOI:10.1016/j.cej.2016.04.113.
- A. De Luca, R. F. Dantas and S. Esplugas, Assessment of iron chelates efficiency for photo-Fenton at neutral pH, Water Res., 2014, 61, 232–242, DOI:10.1016/j.watres.2014.05.033.
- S. Miralles-Cuevas, I. Oller, J. A. Sánchez Pérez and S. Malato, Removal of pharmaceuticals from MWTP effluent by nanofiltration and solar photo-Fenton using two different iron complexes at neutral pH, Water Res., 2014, 64, 23–31, DOI:10.1016/j.watres.2014.06.032.
- J. A. Sánchez Pérez, S. Arzate, P. Soriano-Molina, J. L. García Sánchez, J. L. Casas López and P. Plaza-Bolaños, Neutral or acidic pH for the removal of contaminants of emerging concern in wastewater by solar photo-Fenton? A techno-economic assessment of continuous raceway pond reactors, Sci. Total Environ., 2020, 736, 139681, DOI:10.1016/j.scitotenv.2020.139681.
- G. Maniakova, I. Salmerón, S. Nahim-Granados, S. Malato, I. Oller, L. Rizzo and M. I. Polo-López, Sunlight advanced oxidation processes Vs ozonation for wastewater disinfection and safe reclamation, Sci. Total Environ., 2021, 787, 147531, DOI:10.1016/j.scitotenv.2021.147531.
- G. Maniakova, I. Salmerón, M. Aliste, M. I. Polo-López, I. Oller, S. Malato and L. Rizzo, Solar photo-Fenton at circumneutral pH using Fe(III)-EDDS compared to ozonation for tertiary treatment of urban wastewater: contaminants of emerging concern removal and toxicity assessment, Chem. Eng. J., 2022, 431, 133474, DOI:10.1016/j.cej.2021.133474.
- U. J. Ahile, R. A. Wuana, A. U. Itodo, R. Sha'Ato, J. A. Malvestiti and R. F. Dantas, Are iron chelates suitable to perform photo-Fenton at neutral pH for secondary effluent treatment?, J. Environ. Manage., 2021, 278, 111566, DOI:10.1016/j.jenvman.2020.111566.
- M. Sillanpää, Environmental fate of EDTA and DTPA, Rev. Environ. Contam. Toxicol., 1997, 152, 85–111, DOI:10.1007/978-1-4612-1964-4_3.
-
IARC Monographs on the Evaluation of Carcinogenic Risks to Humans, ed. E. Heseltine, Some Chemicals that Cause Tumours of the Kidney or Urinary Bladder in Rodents and Some Other Substances, Lyon, 1990, vol. 73, pp. 385–399 Search PubMed.
-
EU, Risk assessment, Trisodium Nitrilotriacetate, CAS-No.: 5064-31-3. EINECS-No 225-768-6, Draft of 20.08.2008 Search PubMed.
- EU 2010, Opinion on Trisodium nitrilotriacetate (NTA), Scientific Committee on Consumer Safety, (1391, 201), https://ec.europa.eu/health/sites/health/files/scientific_committees/consumer_safety/docs/sccs_o_046.pdf.
- A. Mejri, P. Soriano-Molina, S. Miralles-Cuevas and J. A. Sánchez Pérez, Fe3+-NTA as iron source for solar photo-Fenton at neutral pH in raceway pond reactors, Sci. Total Environ., 2020, 736, 139617, DOI:10.1016/j.scitotenv.2020.139617.
- S. Miralles-Cuevas, P. Soriano-Molina, I. de la Obra, E. Gualda-Alonso and J. A. Sánchez Pérez, Simultaneous bacterial inactivation and microcontaminant removal by solar photo-Fenton mediated by Fe3+-NTA in WWTP secondary effluents, Water Res., 2021, 205, 117686, DOI:10.1016/j.watres.2021.117686.
- A. Fiorentino, B. Esteban, J. A. Garrido-Cardenas, K. Kowalska, L. Rizzo, A. Aguera and J. A. S. Pérez, Effect of solar photo-Fenton process in raceway pond reactors at neutral pH on antibiotic resistance determinants in secondary treated urban wastewater, J. Hazard. Mater., 2019, 378, 120737, DOI:10.1016/j.jhazmat.2019.06.014.
- Y. Ahmed, J. Zhong, Z. Yuan and J. Guo, Simultaneous removal of antibiotic resistant bacteria, antibiotic resistance genes, and micropollutants by a modified photo-Fenton process, Water Res., 2021, 197, 117075, DOI:10.1016/j.watres.2021.117075.
- P. B. Vilela, A. S. Martins, M. C. V. M. Starling, F. A. R. de Souza, G. F. F. Pires, A. P. Aguilar, M. E. A. Pinto, T. A. O. Mendes and C. C. de Amorim, Solar photon-Fenton process eliminates free plasmid DNA harbouring antimicrobial resistance genes from wastewater, J. Environ. Manage., 2021, 285, 112204, DOI:10.1016/j.jenvman.2021.112204.
- P. B. Vilela, R. P. Mendonça Neto, M. C. V. M. Starling, A. S. da Martins, G. F. F. Pires, F. A. R. Souza and C. C. Amorim, Metagenomic analysis of MWWTP effluent treated via solar photo-Fenton at neutral pH: Effects upon microbial community, priority pathogens, and antibiotic resistance genes, Sci. Total Environ., 2021, 801, 149599, DOI:10.1016/j.scitotenv.2021.149599.
- G. Ferro, M. I. Polo-López, A. Martínez-Piernas, P. Fernández-Ibáñez, A. Agüera and L. Rizzo, Cross-contamination of residual emerging contaminants and antibiotic resistant bacteria in lettuce crops and soil irrigated with wastewater treated by sunlight/H2O2, Environ. Sci. Technol., 2015, 49, 11096–11104, DOI:10.1021/acs.est.5b02613.
- S. Giannakis, F. A. Gamarra Vives, D. Grandjean, A. Magnet, L. F. De Alencastro and C. Pulgarin, Effect of advanced oxidation processes on the micropollutants and the effluent organic matter contained in municipal wastewater previously treated by three different secondary methods, Water Res., 2015, 84, 295–306, DOI:10.1016/j.watres.2015.07.030.
- M. Agulló-Barceló, M. I. Polo-López, F. Lucena, J. Jofre and P. Fernández-Ibánez, Urban wastewater disinfection for agricultural reuse: effect of solar driven AOPs in the inactivation of a multidrug resistant E. coli strain, Appl. Catal., B, 2013, 136–137, 341–350, DOI:10.1016/j.apcatb.2014.10.043.
- G. Ferro, A. Fiorentino, M. Castro Alferez, M. I. Polo-López, L. Rizzo and P. Fernández-Ibáñez, Urban wastewater disinfection for agricultural reuse: effect of solar driven AOPs in the inactivation of a multidrug resistant E. coli strain, Appl. Catal., B, 2015, 178, 65–73, DOI:10.1016/j.apcatb.2014.10.043.
- S. Giannakis, M. Voumard, D. Grandjean, A. Magnet, L. F. De Alencastro and C. Pulgarin, Micropollutant degradation, bacterial inactivation and regrowth risk in wastewater effluents: Influence of the secondary (pre)treatment on the efficiency of Advanced Oxidation Processes, Water Res., 2016, 102, 505–515, DOI:10.1016/j.watres.2016.06.066.
- F. Sciacca, J. A. Rengifo-Herrera, J. Wéthé and C. Pulgarin, Dramatic enhancement of solar disinfection (SODIS) of wild Salmonella sp. In PET bottles by H2O2 addition on natural water of Burkina Faso containing dissolved iron, Chemosphere, 2010, 78, 1186–1191, DOI:10.1016/j.chemosphere.2009.12.001.
- I. García-Fernández, M. I. Polo-López, I. Oller and P. Fernández-Ibánez, Bacteria and fungi inactivation using Fe3+/sunlight, H2O2/sunlight and nearneutral photo-Fenton: A comparative study, Appl. Catal., B, 2012, 121–122, 20–29, DOI:10.1016/j.apcatb.2012.03.012.
- S. Giannakis, M. I. Polo López, D. Spuhler, J. A. Sánchez Pérez, P. Fernández Ibáñez and C. Pulgarin, Solar disinfection is an augmentable, in situ-generated photo-Fenton reaction-Part 1: A review of the mechanisms and the fundamental aspects of the process, Appl. Catal., B, 2016, 199, 199–223, DOI:10.1016/j.apcatb.2016.06.009.
- I. Arslan-Alaton, A. Karatas, Ö. Pehlivan, O. Koba Ucun and T. Ölmez-Hancı, Effect of UV-A-assisted iron-based and UV-C-driven oxidation processes on organic matter and antibiotic resistance removal in tertiary treated urban wastewater, Catal. Today, 2021, 361, 152–158, DOI:10.1016/j.cattod.2020.02.037.
- Y. Yoon, H. J. Chung, D. Yoong, W. Di, M. C. Dodd, H. G. Hur and Y. Lee, Inactivation efficiency of plasmid-encoded antibiotic resistance genes during water treatment with chlorine, UV, and UV/H2O2, Water Res., 2017, 123, 783–793, DOI:10.1016/j.watres.2017.06.056.
- Y. Zhang, Y. Zhuang, J. Geng, H. Ren, K. Xu and L. Ding, Reduction of antibiotic resistance genes in municipal wastewater effluent by advanced oxidation processes, Sci. Total Environ., 2016, 550, 184–191, DOI:10.1016/j.scitotenv.2016.01.078.
- G. Ferro, F. Guarino, S. Castiglione and L. Rizzo, Antibiotic resistance spread potential in urban wastewater effluents disinfected by UV/H2O2 process, Sci. Total Environ., 2016, 560–561, 29–35, DOI:10.1016/j.scitotenv.2016.04.047.
- J. Wu, S. Cheng, Y. Duan, S. Shen, B. Jiang, Y. Li, P. Shi, W. Li, Q. Zhang and A. Li, Kinetics and efficacy of membrane/DNA damage to Bacillus subtilis and autochthonous bacteria during UV/chlorine treatment under different pH and irradiation wavelengths, Chem. Eng. J., 2021, 422, 129885, DOI:10.1016/j.cej.2021.129885.
- S. S. Shekhawat, N. M. Kulshreshtha, V. Vivekanand and A. B. Gupta, Impact of combined chlorine and UV technology on the bacterial diversity, antibiotic resistance genes and disinfection by-products in treated sewage, Bioresour. Technol., 2021, 339, 125615, DOI:10.1016/j.biortech.2021.125615.
- A. Fiorentino, G. Lofrano, R. Cucciniello, M. Carotenuto, O. Motta, A. Proto and L. Rizzo, Disinfection of roof harvested rainwater inoculated with E. coli and Enterococcus and post-treatment bacterial regrowth: Conventional vs solar driven advanced oxidation processes, Sci. Total Environ., 2021, 801, 149763, DOI:10.1016/j.scitotenv.2021.149763.
- S. Phattarapattamawong, N. Chareewan and C. Polprasert, Comparative removal of two antibiotic resistant bacteria and genes by the simultaneous use of chlorine and UV irradiation (UV/chlorine): Influence of free radicals on gene degradation, Sci. Total Environ., 2021, 755, 142696, DOI:10.1016/j.scitotenv.2020.142696.
- Q. Ping, T. Yan, L. Wang, Y. Li and Y. Lin, Insight into using a novel ultraviolet/peracetic acid combination disinfection process to simultaneously remove antibiotics and antibiotic resistance genes in wastewater: Mechanism and comparison with conventional processes, Water Res., 2022, 110, 118019, DOI:10.1016/j.watres.2021.118019.
- J. B. De Souza, F. Queiroz Valdez, R. F. Jeranoski, C. M. de Sousa Vidal and G. Soares Cavallini, Water and wastewater disinfection with peracetic acid and UV radiation and using advanced oxidative process PAA/UV, Int. J. Photoenergy, 2015, 1–7, 860845, DOI:10.1155/2015/860845.
- A. H. Hassaballah, T. Bhatt, J. Nyitrai, N. Dai and L. Sassoubre, Inactivation of E. coli, Enterococcus spp., somatic coliphage, and Cryptosporidium parvum in wastewater by peracetic acid (PAA), sodium hypochlorite, and combined PAA-ultraviolet disinfection, Environ. Sci.: Water Res. Technol., 2020, 6, 197, 10.1039/C9EW00837C.
- J. Hollman, J. Albino Dominic and G. Achari, Degradation of pharmaceutical mixtures in aqueous solutions using UV/peracetic acid process: Kinetics, degradation pathways and comparison with UV/H2O2, Chemosphere, 2020, 248, 125911, DOI:10.1016/j.chemosphere.2020.125911.
- W. Li, T. Jain, K. Ishida and H. Liu, A mechanistic understanding of the degradation of trace organic contaminants by UV/hydrogen peroxide, UV/persulfate and UV/free chlorine for water reuse, Environ. Sci.: Water Res. Technol., 2017, 3, 128, 10.1039/c6ew00242k.
- M. M. Ahmed and S. Chiron, Solar photo-Fenton like using persulphate for carbamazepine removal from domestic wastewater, Water Res., 2014, 48, 229–236, DOI:10.1016/j.watres.2013.09.033.
- J. Rodríguez-Chueca, S. V. della Giustina, J. Rocha, T. Fernandes, C. Pablos, A. Encinas, D. Barceló, S. Rodríguez-Mozaz, C. M. Manaia and J. Marugán, Assessment of full-scale tertiary wastewater treatment by UV-C based-AOPs: Removal or persistence of antibiotics and antibiotic resistance genes?, Sci. Total Environ., 2019, 652, 1051–1061, DOI:10.1016/j.scitotenv.2018.10.223.
- I. Carra, L. Santos-Juanes, F. Gabriel, A. Fernández, S. Malato and J. A. Sánchez Pérez, New approach to solar photo-Fenton operation. Raceway ponds as tertiary treatment technology, J. Hazard. Mater., 2014, 279, 322–329, DOI:10.1016/j.jhazmat.2014.07.010.
- A. Fiorentino, P. Prete, L. Rizzo, R. Cucciniello and A. Proto, Fe3+−iminodisuccinate as a new green catalyst for water treatment by photo-Fenton process at neutral pH, J. Environ. Chem. Eng., 2021, 9, 106802, DOI:10.1016/j.jece.2021.106802.
- M. N. Chong, B. Jin, C. W. K. Chow and C. Saint, Recent developments in photocatalytic water treatment technology: a review, Water Res., 2010, 44, 2997–3027, DOI:10.1016/j.watres.2010.02.039.
- G. Maniakova, K. Kowalska, S. Murgolo, G. Mascolo, G. Libralato, G. Lofrano, O. Sacco, M. Guida and L. Rizzo, Comparison between heterogeneous and homogeneous solar driven advanced oxidation processes for urban wastewater treatment: Pharmaceuticals removal and toxicity, Sep. Purif. Technol., 2020, 236, 116249, DOI:10.1016/j.seppur.2019.116249.
- W. Gernjak, M. I. Maldonado, S. Malato, J. Cáceres, T. Krutzler, A. Glaser and R. Bauer, Pilot-plant treatment of olive mill wastewater (OMW) by solar TiO2 photocatalysis and solar photo-Fenton, Sol. Energy, 2004, 77, 567–572, DOI:10.1016/j.solener.2004.03.030.
- M. G. Alalm, A. Tawfik and S. Ookawara, Comparison of solar TiO2 photocatalysis and solar photo-Fenton for treatment of pesticides industry wastewater: Operational conditions, kinetics, and costs, J. Water Process Eng., 2015, 8, 55–63, DOI:10.1016/j.jwpe.2015.09.007.
- J. F. J. R. Pesqueira, M. F. R. Pereira and A. M. T. Silva, A life cycle assessment of solar-based treatments (H2O2, TiO2 photocatalysis, circumneutral photo-Fenton) for the removal of organic micropollutants, Sci. Total Environ., 2021, 761, 143258, DOI:10.1016/j.scitotenv.2020.143258.
- M. Mišík, F. Ferk, H. Schaar, M. Yamada, W. Jaeger, S. Knasmueller and N. Kreuzinger, Genotoxic activities of wastewater after ozonation and activated carbon filtration: Different effects in liver-derived cells and bacterial indicators, Water Res., 2020, 186, 116328, DOI:10.1016/j.watres.2020.116328.
- J. Reungoat, B. I. Escher, M. Macov, F. X. Argaud, W. Gernjak and J. Keller, Ozonation and biological activated carbon filtration of wastewater treatment plant effluents, Water Res., 2012, 46, 863–872, DOI:10.1016/j.watres.2011.11.064.
- D. Sauter, C. Stange, V. Schumacher, A. Tiehm, R. Gnirssa and T. Wintgens, Impact of ozonation and biological post treatment of municipal wastewater on microbiological quality parameters, Environ. Sci.: Water Res. Technol., 2021, 7, 1643–1656, 10.1039/d1ew00312g.
- M. Sgroi, S. A. Snyder and P. Roccaro, Comparison of AOPs at pilot scale: Energy costs for micro-pollutants oxidation, disinfection by-products formation and pathogens inactivation, Chemosphere, 2021, 273, 128527, DOI:10.1016/j.chemosphere.2020.128527.
- L. Xu, C. Zhang, P. Xu and X. C. Wang, Mechanisms of ultraviolet disinfection and chlorination of Escherichia coli: Culturability, membrane permeability, metabolism, and genetic damage, J. Environ. Sci., 2018, 65, 356–366, DOI:10.1016/j.jes.2017.07.006.
|
This journal is © The Royal Society of Chemistry 2022 |
Click here to see how this site uses Cookies. View our privacy policy here.