DOI:
10.1039/D1NA00741F
(Review Article)
Nanoscale Adv., 2022,
4, 334-352
Novel insights into nanomaterials for immunomodulatory bone regeneration
Received
12th October 2021
, Accepted 13th November 2021
First published on 29th November 2021
Abstract
Bone defect repair caused by trauma, congenital malformation, tumors, infection or systemic diseases remains the focus of attention in regeneration medicine. Recent advances in osteoimmunology indicate that immune cells and correlative cytokines modulate the delicate balance between osteoblasts and osteoclasts and induce a favorable microenvironment for bone regeneration. With superior attributes that imitate the three-dimensional architecture of natural bone, excellent fabricability, mechanical and biological properties, nanomaterials (NMs) are becoming attractive in the field of bone tissue engineering. Particularly, it could be an effective strategy for immunomodulatory bone regeneration by engineering NMs involved in composition nature, nanoarchitectural morphology, surface chemistry, topography and biological molecules, whose mechanisms potentially refer to regulating the phenotype of high-plastic immune cells and inducing cytokine secretion to accelerate osteogenesis. Despite these prominent achievements, the employment of NMs is poorly translated into clinical trials due to the lack of knowledge about the interaction between NMs and the immune system. For this reason, we sketch out the hierarchical structure of bone and its natural healing process, followed by discussion about the effects of immune cells on bone regeneration. Novel horizons focusing on recent progressions in the architectural and physicochemical performances of NMs and their impacts on the body defence mechanism are also emphasized, hoping to provide novel insights for the fabrication of bone graft materials in tissue engineering.
 Ya Cui | Ya Cui received her Bachelor of Dental Surgery from Sichuan University in 2020. Currently, she is a PhD candidate under the supervision of Professor Lixia Mao in the Department of Oral & Cranio-Maxillofacial Surgery at the Shanghai Jiao Tong University School of Medicine. Her research interests are mainly focused on the study of bioactive nanomaterials for bone defect repair. |
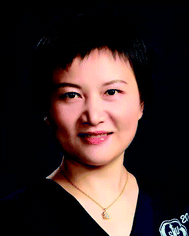 Lixia Mao | Lixia Mao received her Doctorate of Orthodontics from Shanghai Jiao Tong University in 2016. Currently, she is an associate chief physician in the Department of Oral & Cranio-Maxillofacial Surgery at the Shanghai Jiao Tong University School of Medicine. Her research interests are mainly focused on bioactive nanomaterials with a surface micro–nano-structure for bone defect repair and the related mechanism. |
1. Introduction
In recent years, bone damage caused by trauma, congenital malformation, tumors, infection or systemic diseases has brought enormous detriments to society. Although small bone trauma commonly heals by itself, critical bone defects need interventional therapies to achieve fracture healing.1 Conventionally, autologous bone grafts as the “gold standard” in clinics are limited by supply and commonly cause injury in the donor's extraction site. Furthermore, allografts require a complex implantation technique and are possibly rejected due to host-to-graft immune reactions.2 Currently, increasing studies are focused on novel advances in tissue engineering strategies applied in bone regeneration. Bone tissue engineering combines functional cells and growth factors with material scaffolds, playing crucial roles in restoring the injured areas of the skeletal system. Indeed, it is of great importance for the scientific community to design and develop updated materials to offer a favorable three-dimensional microenvironment for the adhesion and proliferation of various cells during the bone healing process.3 Despite much encouraging achievements, scaffold materials designed for bone regeneration are poorly translated into clinic trials potentially due to ambiguous interaction with the body defence system.
As an interdisciplinary research field, osteoimmunology mainly concentrates on the interplay between the skeletal and immune systems, providing a heuristic concept to explore effective therapies for bone defect repair and regeneration. Except most discussions relating to bone and innate immune response, the effects of adaptive immune reactions on the osteoimmunomodulation of homeostasis and diseases have also attracted the attention of researchers in recent times.4,5 Additionally, mesenchymal stem cells (MSCs) belong to multipotent stromal cells that possess the capability of tri-lineage differentiation into several different cell types, including adipocytes, osteoblasts or chondrocytes. Extensive studies have demonstrated that the therapeutic role of MSCs mainly depends on their immunomodulatory plasticity. MSCs can promote tissue repair via producing abundant cytokines and growth factors in response to local inflammatory microenvironment. Such plasticity in MSC immunomodulatory function is one of the intense subjects in regenerative medicine.6 Nevertheless, the interaction among immune cells, bone cells and stem cells in bone physiological microenvironment and their spatio-temporal coupling for successful bone regeneration are still not clear, which may impede clinical application of stem cell therapy in bone tissue engineering. Encouragingly, engineered materials with a specific nanoarchitecture hold great promise for the development of stem cell-based bone regeneration due to their positive effects on facilitating osteogenic differentiation and modulating immune response.7
It is inspiring that these restrictions could be addressed through the burgeoning field of nanomedicine as NMs themselves possess immune-activating or immunosuppressive effects and promote targeted co-delivery of biological molecules, and mimic the hierarchical structure of natural bone tissue.8 According to definition of the US Food and Drug Administration (FDA), nanotechnology is in the light of size as well as function, using the range of 1 to 100 nm, on the condition that the physical, chemical or biological effects of the material in question are attributed to its dimensions.9 Over the last few decades, research efforts on the engineering of nanoscale materials for emerging therapies of tumors and autoimmune diseases have made unprecedented progress. Recently, researchers have focused on collaboration between nanotechnology and osteoimmunology for the therapy of pathological bone loss caused by osteoporosis, periodontitis and rheumatoid arthritis, inspired by NMs applied in cancer immunotherapy.10
In this review we discuss how the biochemical and biophysical attributes of NMs affect their effects on immune reactions, inflammation, and bone remolding under physiological and pathological conditions, respectively. We also review the crucial role of advanced nanotechnology in relation to its applications in the research and development of immunomodulatory bone tissue engineering materials. In particular, we highlight how precise manipulation of cells or bioactive molecules at the nanoscale may be conducive to our increasing understanding and targeting of immune reactions for bone regeneration. The field of nanotechnology is vast; therefore we mainly concentrate on examples of chosen studies that emphasize the wide range of immunological applications of NMs in bone-related disease and regenerative therapy.
2. Bone structure and its natural healing process
Bone tissues as a type of widely existing load-bearing organ in vertebrates were endowed with a unique biological adaptive architecture, biomechanics and biology under the long-term evolution of nature, which exhibit high strength, stiffness and fracture toughness as well as play a crucial role in supporting the body and movement.11 In particular, the hierarchical architecture ranging from macro- (cortical and cancellous bone), microscopic (haversian system, osteon, and trabecular bone), submicroscopic (lamellar bone), nano- (collagen and mineral particles) to sub-nano levels (minerals and organic proteins) gives the specific heterogeneity and anisotropy of natural bone. Among them, collagen nanofibers and hydroxyapatite (HA) nanocrystals are the foundation of sophisticated bone structures.12,13 To some extent, bone can be regarded as a network skeleton connected by collagen microfibers deposited with flake or needle bone mineral nanocrystals. The multi-leveled structure of natural bone gives specific surficial/interfacial cues to direct bone regeneration through modulating the balance between bone growth and bone resorption in the participation of osteoblasts, osteoclasts and growth factors. Furthermore, three-dimensional modeling composed of the skeleton system in return can resist tough mechanical stress produced by motion and possess favorable flexibility.14
Fracture healing after injury is a spontaneous and complicated physiological process, which involves a series of osteoinductive and osteoconductive reactions, such as ossification of cartilage, induction of intracellular and extracellular molecular signal pathways, and time and space optimization. In particular, bone healing refers to a multi-cell coupled cascade response, during which inflammatory cells, vascular cells, bone progenitor cells, osteoblasts and osteoclasts play a leading role.15 After extravasated blood forms a hematoma, platelets, macrophages and inflammatory cells (e.g. granulocytes and lymphocytes) penetrate into the hematoma and secrete cytokines and growth factors to facilitate fibrin thrombosis and coagulation. Subsequently, capillaries extend into blood clots, macrophages degenerate and gradually form granulation tissue. Chondrocytes derived from MSCs proliferate, synthesize a cartilage matrix and gradually replace cellulose/granulation tissue. As the most active period of the osteogenic effect, it is characterized by the activity of osteoblasts and the formation of a bone mineralized matrix, forming woven bone with certain strength. Among them, osteogenic cells mainly come from periosteum, bone marrow, the circulatory system, blood vessels and surrounding tissues. In the final stage of fracture healing, the hard callus is reconstructed into the original cortical bone or trabecular bone.16–18 Taken together, this reconstruction is a coupling process of bone absorption and bone formation in the participation of osteoblasts and osteoclasts (Fig. 1).
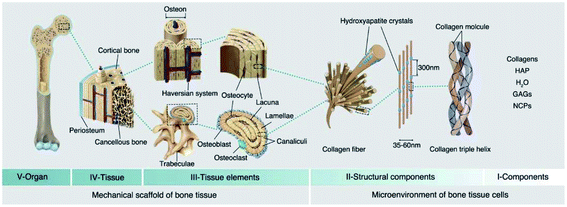 |
| Fig. 1 Hierarchical structure of natural bone. The V, IV, and III levels construct the mechanical support structure for bone tissues, and the II and I levels construct the microenvironment for bone tissue cells. HAP: hydroxyapatite; GAGs: glycosaminoglycans; NCPs: non-collagenous proteins. Reprinted with permission from ref. 12. Copyright (2021) Elsevier. | |
3. The role of the immune system in bone regeneration
3.1 The immune reaction, inflammation and bone regeneration
The immune system comprises a sophisticated network of cells and lymphoid organs that act in harmony to protect the host from a universe of pathogenic microorganisms.19 The innate immune system commonly involves every aspect of the host's immune defense mechanisms, which are encoded in their mature functional recognition molecules via the germline genes of the host. When the immune system recognizes microbial, toxic, or allergenic structures, the innate immunity acts as the first defensive system that immediately and non-specifically reacts against invading microbes. In contrast, the antigen-specific receptors in the adaptive immune system are generally expressed on the surfaces of T and B lymphocytes and exhibit complicated specificity for their target antigens. Unlike the innate mechanisms of host defense, adaptive immunity generally spends more time constituting an immune reaction that is systemic, continuous, and highly specific.20 In fact, although the innate and adaptive immunity are depicted as independent arms of the host defensive response, the cooperativity between them is crucial for an integrated, completely effective immune reaction.
Furthermore, the immune system also plays crucial roles in inflammatory reactions that occur when natural bone is damaged by microbes, toxins, heat, or any other substances. Broadly defined, inflammation is a normal physiological reaction that protects tissues from infection or injury, which can be divided into two categories including infectious pathogen-associated molecular patterns (PAMPs) and noninfectious damage-associated molecular patterns (DAMPs).21 These danger signals can induce acute inflammation that activate the immune system to remove damaging pathogens, promote bone repair, and restore its normal functions via up-regulation of anti-inflammatory mediators.22 Typically, an acute inflammatory phase consists of recruitment of monocytes from circulation and activation of inflammatory mediators to eliminate outside pathogenic agents, among which the release of anti-inflammatory factors, down-regulation of pro-inflammatory factors, and liquidation of apoptotic cells (through phagocytes) promote the recession of inflammation.23 However, in many cases, chronic inflammation will arise if the inflammation is non-resolving for some reasons. In general, chronic inflammation may induce the release of pro-inflammatory mediators, infiltration of monocytes and eventually issue in local tissue damage. The persistent inflammatory reaction that includes over-expressed inflammatory mediators, damaged tissues, and necrotic monocytes will become an inflammatory trigger and result in an adaptive immune reaction. Moreover, the host's own immune system will destruct host tissues and organs as infectious pathogens escape from host immunity surveillance and become tolerogenic, the consequences of which can lead to some bone-related inflammatory diseases, including osteoporosis, periodontitis and rheumatoid arthritis that eventually cause pathological bone loss.24
3.2 The main factors of immune system modulating bone regeneration
The immune system greatly affects osteogenesis and osteoclastogenesis during the bone remolding process in both positive and negative fashions. Therefore, it is an attractive approach to achieve successful bone regeneration via controlling the immune components of the fracture healing process. In this section, we highlight various immune cells that have an essential influence on bone tissue repair and regeneration. In many cases, these immune cells are based on cytokines or growth factors as media for osteoimmunomodulation (Fig. 2).
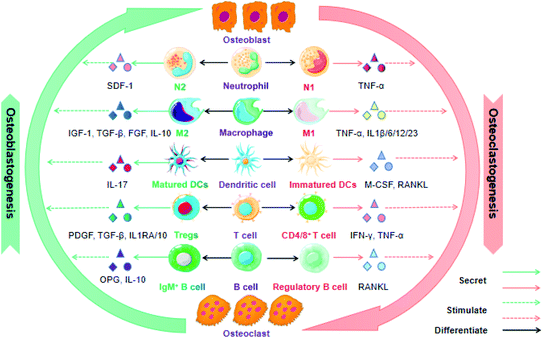 |
| Fig. 2 Crosstalk among immune cells and its role in bone regeneration. During the bone formation and healing process, various immune cells participate in the balance between osteoblastogenesis and osteoclastogenesis through the secretion of cytokines that can be utilized as bioactive molecules for osteoimmunomodulation. | |
3.2.1 Neutrophils.
Neutrophils are a thoroughly crucial part of the innate immune system and are the first cells recruited to inflammatory sites after damage and are answerable for wound detection and eliminating fomites.25 The prominent leukocyte population in human blood can release neutrophil extracellular traps (NETs) and induce phagocytosis, and subsequently control infection in the local area. It is worth noting that increasing evidence suggests that they are capable of phagocytosing debris whereas restricting NET production, which may play an essential role in damaged tissue repair and regeneration. Recent advances in tissue engineering have demonstrated that neutrophils can modulate macrophage recruitment and polarization, trigger the migration of macrophages to injury sites, and promote cardiac repair of acute myocardial infarction mice. Meanwhile, Hoemann et al. prepared chitosan-glycerol phosphate/blood implants in order to facilitate trabecular bone regeneration of drilled defects through the recruitment of arginase-1+ macrophages in a specific neutrophil-dependent mechanism. It was found that neutrophils could also polarize into the N2 phenotype under suitable interleukin (IL)-8 concentration, subsequently evoking bone marrow stromal cell (BMSC) recruitment and ectopic endochondral ossification through the SDF-1/CXCR4 axis and its downstream PI3K/AKT pathway and β-catenin-mediated migration.26 Despite these extraordinary advantages, neutrophils may release NETs to exert apparent paradoxical effects and aggravate tissue damage during inappropriate chronic inflammation, and therefore the function of neutrophils in bone repair and regeneration remains abstruse.
3.2.2 Monocytes and macrophages.
Similarly, macrophages are one of the major constituent parts of the innate immune system. In general, inflammatory macrophages are derived from monocyte precursors circulating in the bloodstream and established during foetal development.27 Highly plastic macrophages show the phenomenon of polarization to either the M1 or M2 type in terms of microenvironmental cues.28 In general, bone healing after injury includes the inflammatory phase, the repair phase, and the remodeling phase, and among them the initial inflammatory phase plays a significant role in eventual healing. M1 cells are considered to link with the initial stage of bone remolding because of the high expression of several surface markers (e.g. CD86, CD80, iNOS, and CCR7) in the acute inflammatory stage. In contrast, M2 cells act during the late phases of bone remolding, leading to the rapid progress of the active healing process. For instance, in the pathology of several chronic inflammatory diseases, macrophages will polarize into a pro-inflammatory M1 phenotype, resulting in an increasing of pro-inflammatory mediators and a reduction of anti-inflammatory and regulatory cytokines (e.g. IL-4, IL-10, IL-13, and TGF-β).29
To date, although macrophages promote bone repair in terms of their attractive potential in recruiting various cells (e.g. osteoprogenitors, vascular progenitor cells, MSCs, etc.) into the fracture area, the exact mechanism is still unclear. Most researchers agree that the ratio of M1/M2 modulates the outcomes of bone repair after scaffold implantation, during which the M1 macrophage is conducive to the premier acute inflammatory stage that sweeps away the debris of the bone defect area, while the M2 macrophage secretes growth factors and facilitates MSC-mediated bone regeneration in the later stages of bone remolding.30 Overall, the orientation of macrophage polarization and the quantity of macrophages may be effective predictors for successful bone remodeling and fracture healing.
3.2.3 Dendritic cells.
Indeed, antigen presenting cells (APCs) play a remarkable role in linking both innate and adaptive immune reactions, which make them particularly attractive targets for immunomodulation.8 For instance, Smith et al. concluded that nanotechnology interacting with the immune system mainly relied on APC-mediated immune response, and some pathways lead to reduced action or apoptosis of APCs in nanoscale immunosuppression.31,32 In particular, dendritic cells (DCs) are the major APCs that can identify various tissue injury by-products and release numerous inflammatory factors in acute inflammation to accelerate tissue healing.33 For instance, it has been shown that EP3 signaling in DCs promoted liver repair through eliciting IL-13-mediated remolding of macrophage phenotypes from the pro-inflammatory to pro-reparative. Furthermore, tolerogenic DCs are promising candidates for remolding and healing after myocardial infarction via modulating the regulatory T cell (Tregs) and macrophage polarization.34 The potential interaction between DCs with biomaterials is also critical for bone repair and regeneration. In terms of mechanisms, DCs most likely act as a bridge between innate and adaptive immunity during bone defect healing in order to activate naive T cells and control macrophage homeostasis.35 In addition, immature DCs have the potential of switching into osteoclasts after stimulation with RANKL and M-CSF and play an important role in resorbing bone because they are derived from the same myeloid precursor and the functions of both cell types are highly dependent on RANKL.36 Although their precise role during bone regeneration remains not fully understood, studies show that they have an essential influence on the fracture healing process.
3.2.4 T cells.
Increasing data indicates that T cells play a crucial role in bone repair and regeneration. Although potential mechanisms have been revealed, the precise effects of various T cell types and subsets as well as their level of accumulation at fracture sites are poorly understood. Generally noting, αβ T cells can be divided into both pro- and anti-regenerative sub-populations, while tissue resident γδ T cells have been recognized as being pro-regenerative.37
T cells contain a diverse range of subsets and produce multiple growth factors and cytokines, which play different roles in bone repair and regeneration. Both “helper” (CD4) and “cytotoxic” (CD8) T cells have been reported to inhibit bone regeneration and delay fracture healing. In contrast, Tregs have demonstrated the ability to promote fracture healing via modulating both the innate and adaptive immune response. They play a significant role in the maintenance of self-tolerance and controlling excessive inflammation after injury to some extent. For instance, Nagai and coworker fabricated a hydrogel-formulated prolyl hydroxylase inhibitor, which facilitated the regeneration of alveolar bone loss after being injected into the periodontal pockets of experimental mice. The potential mechanisms were associated with elevated CXCR4-dependent accumulation of Treg cells, decreased secretion of pro-inflammatory factors and increased expression of osteogenic genes.38 Concomitantly, Treg cells have the ability to switch an initial undesirable and anti-regenerative immune microenvironment caused by an increased CD8+ effector T cell level into a pro-regenerative microenvironment facilitating successful bone fracture healing.39
Additionally, γδ T cells also play an essential role in the modulation of bone physiology. For example, oral barrier homeostasis is vital for safeguarding tissue integrity and preventing periodontitis, and it is shown that γδ T cells promote the wound healing through the secretion of reparative cytokine amphiregulin.40 More recent evidence indicates that IL-17-producing γδ T cells promote bone regeneration and accelerate fracture healing, given that IL-17A elicits the proliferation and osteoblastic differentiation of injury-associated MSCs. Furthermore, Vγ6+ γδ T cells proliferate in the musculoskeletal tissue following a drill-hole bone defect and act as the key producer of IL-17A in fracture healing. Overall, it is IL17A−/− rather than IL17F−/− mice that exhibits impaired bone regeneration.41 Despite the encouraging headway in understanding the functions of T cells for bone repair and regeneration, precise mechanisms by which various T cell types and subsets regulate the immune reaction to fracture are poorly verified.
3.2.5 B cells.
There is little obtained evidence on the effects of B cells on fracture healing. To some extent, B cells are derived from the bone marrow and it would be expected that there would be several correlations between B cells and bone tissue. For example, IgM+ B cells possess the potential to promote bone formation and accelerate bone healing through the secretion of osteoprotegerin and IL-10. In addition, it is shown that the depletion of the adaptive immune response represents a promising strategy to facilitate bone regeneration, giving that mice deficient in both T and B cells display elevated fracture healing.42 Nevertheless, we would argue that there is still much to be explored with respect to the effects of B cells on the repair and regeneration of natural bone tissue (Fig. 3).
 |
| Fig. 3 Mechanisms of NM coupling immune signaling and osteogenesis in the bone homeostasis process. The physicochemical performances and bionic attributes of NMs play a crucial role in the crosstalk among immune cells, stem cells and bone cells during bone remolding. | |
4. Mechanisms of nanomaterials for osteoimmunomodulation
NMs possess a specific intrinsic effect on the host immune system after implanted into the body, which highly depend on their physicochemical properties. The surface chemistry, topography, grafted molecules that NMs take, the nanoarchitectural morphology, the hydrophobicity, the degradability, the biocompatibility, and the composition nature of NMs are critical parameters to be considered. The potential mechanisms by which engineered NMs effectively promote bone formation and bone healing through regulating immune cells, bone cells, cytokines and growth factors are discussed in detail below.
4.1 Chemical properties of nanomaterials
The surface chemistry of NMs has been reported to have a significant influence on the immune system, in particular phagocytes. It is of great importance for bone tissue repair to achieve surface modification of NMs through employing hydrophilic functional groups, surface charge, and biominerals.43 As one of the crucial chemical attributes required for NMs, hydrophilicity can be acquired by several methods such as hydrolysis or plasma modification. Simultaneously, surface charges are closely associated with the material hydrophilicity and absorption of biomolecules, which play an essential role in osteogenesis and osteoclastogenesis. Various NMs modified with either cations or anions can effectively induce bone formation and inhibit bone resorption.44 Recent advances in biomineralization on the surface of NMs have shown that this strategy significantly facilitates osteogenesis, which is achieved through sputter coating, thermal spraying, or utilization of a simulated body fluid.45 Although different surface modification strategies have been introduced to promote bone defect healing, the updated knowledge of bone regeneration via immune regulation through the surface attributes of NMs has been highlighted in recent time.
Previous studies have indicated that the hydrophilic surface of NMs facilitates less macrophage and foreign body giant cell formation and enhances the osseointegration of implants compared to the hydrophobic surface.46 For instance, recent studies have shown that surface-modified hydrophilic titanium (Ti) disks facilitate the production of osteogenic cytokines and influence the activity of macrophages. The hydrophilic surface was successfully fabricated through rinsed Ti disks under N2 and stored it in an isotonic saline solution at pH 4–6. The expression level of TGF-β/BMP signaling was elevated followed by the fostering of osteoblasts on these hydrophilic substrates. Furthermore, the hydrophilic Ti surface significantly promoted the polarization of macrophages toward the healing-associated M2 type via enhancing the secretion of an anti-inflammatory factor IL-10 as well as inhibiting the secretion of pro-inflammatory factors such as IL-1β, IL-6, and IL-8.47 Additionally, adsorbed proteins on the surface of gold nanoparticles were decreased and the material hydrophilicity was increased following the surface modification of polyethylene glycol, which weaken the excessive activation and phagocytosis role of macrophages, subsequently accelerating tissue repair.48 Therefore, surface-modified hydrophilic NMs can induce a favorable immune microenvironment to promote bone tissue repair and accelerate fracture healing.
The surface charge of materials has also an essential influence on the osteoimmune microenvironment. Recently, Brodbeck et al.49 prepared acrylamide materials with the anionic functional group of poly(acrylic acid), which effectively promoted the expression of an anti-inflammatory factor IL-10 and reduced the expression of a pro-inflammatory factor IL-8. Concurrently, the substrate with the cationic functional group of poly(dimethylaminopropylacrylamide) inhibited the secretion of IL-1RA and IL-10 that are crucial for mature osteoblasts. The above results demonstrated that the anionic substrate effectively increased osteogenesis compared to cationic substrates, given that anionic surfaces could inhibit the production of pro-inflammatory cytokines and induce a favorable bone repair microenvironment.
In the last decade, synthetic substitutes of bone grafts with biomineralization attributes have shown great application prospects in the regeneration of large bone defects. Meanwhile, novel fields about the osteoimmunology of minerals have also given fundamental knowledge to better understand bone tissue engineering.50 Increasing studies have demonstrated that the surface modification of materials with calcium phosphate nanocrystals can inhibit the secretion of pro-inflammatory factors (e.g. TNF-α, IL-1β) and enhance the adhesion of osteoblasts. In another case, Jin et al.51 prepared biomimicking hierarchical intrafibrillarly mineralized collagens (HIMCs) and proved that these biomineralized scaffolds not only mimicked the hierarchical structure and surface chemistry of bone tissue, also recruited host MSCs and accelerated endogenous bone regeneration by affecting macrophage polarization and intracellular communication through macrophage-derived extracellular vesicles. Taken together, the above studies reveal that the biomineralization attributes of nanoscale materials seem to directly promote bone regeneration via controlling the immune reaction.
4.2 Physical properties of nanomaterials
In addition to surface chemical properties, the physical properties of NMs such as topography, roughness, porosity, and pore size can also influence bone formation and related cellular function. The surface topography of NMs can modulate cellular reactions through transforming the cell shape and elasticity.52 It has been reported that the function, phenotype and polarization of macrophages are influenced via varying the material topography. For instance, Huang et al.53 successfully fabricated a Cu-containing nano-topographical bio-ceramic surface (Micro-Ti surface) in order to investigate the effect of Cu2+ release or surface topography on macrophage-mediated osteogenic and bactericidal activities. Although Cu2+ added directly into the culture medium or released from Cu-containing nano-substrates polarized macrophages to a pro-inflammatory M1 phenotype, the nano-topographical substrate played an anti-inflammatory role to some extent via up-regulating the integrin and TLR signaling. Furthermore, the Cu-Hier-Ti surface created a favorable immune microenvironment for the proliferation and differentiation of osteoblasts and intensify macrophage ability to engulf and kill bacteria compared to a Cu-free micro-topographical surface. Recently, nanoscaled roughness has also been shown to influence the adhesion, proliferation and differentiation of osteoblasts via modulating immune response. In a case, Ma et al.54 prepared UV-irradiation TiO2 surfaces with different roughness (6–12 nm) in order to investigate the effects of different nanoroughness groups on osteoimmunomodulation. The results showed that these materials with higher roughness implanted in rats induced a pro-inflammatory immune response in the implant. Although recent studies were focused on the influence of different topographical characteristics on osteogenesis, their effects on the behavior of immune cells are still poorly understood and need more investigation.
Indeed, the porosity and pore size of bone implants are of great importance for clinical applications due to the penetration of biomolecules such as oxygen or proteins, which can also influence osteogenesis and immunomodulation, acting as crucial topographical cues. In a case, Chen et al.55 fabricated anodic alumina with nanoporous architectures, in which surfaces with pores in diameters of 100 and 200 nm induced macrophages into an anti-inflammatory M2 phenotype. To some extent, it may be a promising strategy to modulate osteogenesis and immune response via controlling the pore size and porosity of NMs, nevertheless, the exact mechanism still needs further investigation (Fig. 4).
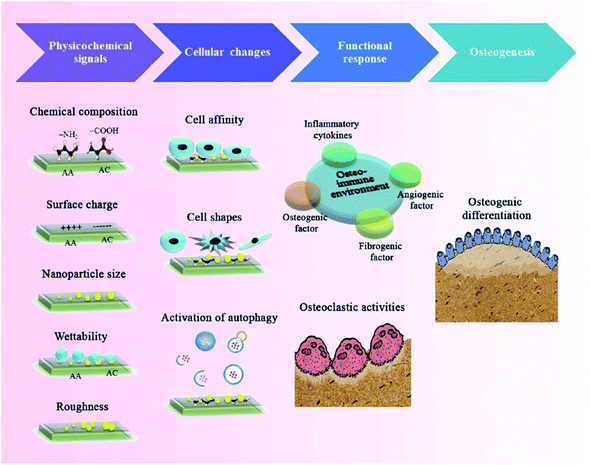 |
| Fig. 4 Schematic diagram of tuning physicochemical signals (e.g. chemistry and topography of nanoarchitectural surfaces) to modulate immune reactions during the bone healing process. Reprinted with permission from ref. 56. Copyright (2017) American Chemical Society Publications. | |
4.3 Structural effects of nanomaterials
It is well known that NMs can mimic the hierarchical architecture of natural bone, providing a favorable microenvironment for nutrient/waste interchange, intercellular communication, cell migration, proliferation and differentiation. Moreover, the nanostructure at different dimensional levels may offer specific environmental cues to modulate cell behaviors and evoke the corresponding immune reaction, subsequently facilitating the recruitment and osteogenic differentiation of stem cells in an early period of bone formation.57
Interestingly, nanoscale biomaterials for bone tissue engineering can be divided into one-dimensional (1D), two-dimensional (2D), and three-dimensional (3D) levels according to the dimensions of their microstructures. Among them, 1D nanostructures refer to materials with nanoscaled size in a spatial dimension, such as nanoparticles, nanospheres, nanowires, and nanorods. 2D nanosurfaces are nano-sized in two dimensions and generally include nanotubes, nanoflakes, and nanoribbons. Furthermore, the unique hierarchical structures of 3D NMs are fabricated based on 1D and 2D nanoarchitectures, which are more beneficial to bone regeneration. Increasing studies have indicated that 1D level NMs (e.g. nanoparticles, nanowires, nanorods, etc.) could enhance bone regeneration through regulating the immune response. For example, Bordoni et al.58 fabricated a specific type of bioactive material combining graphene oxide (GO) and calcium phosphate (CaP) with a nanoscale lateral dimension, which displayed intrinsic immunomodulatory attributes and excellent osteoinductive capacity. As a consequence, these 1D level nanoparticles could evoke the activation of monocytes through over-production of oncostatin M and increase the expression of osteogenic markers through up-regulation of BMP and Wnt signaling. Eventually, the pro-osteogenic role of maGO-CaP in vivo has been evidenced via injection into the tibia of mice, which obviously increased the local bone mass and bone formation rate. Overall, particular nanosystems with one dimensional structures may promote bone regeneration by a sophisticated process demanding a synergetic reaction between the immune and skeletal system. Besides 1D nanoeffects on bone repair, 2D nanoscale topographical surfaces also have an essential influence on bone regeneration. Recent studies have demonstrated that the nanoscale morphology and surface roughness of materials play a crucial role in the mutual interplay of bone cells and immune cells, suggesting that nanotopographical surfaces at the 2D level could modulate the osteoimmune microenvironment for better bone tissue repair. The details have been discussed above. It is worth noting that materials with 3D macro/micro/nano-scaled structures can mimic the hierarchical architecture of natural bone, which may provide multiple cues such as mechanical and immune signaling, in order to have a synergistic effect on the improvement of bone formation efficacy. As mentioned above, HIMC scaffolds successfully mimic the surface chemical attributes and hierarchical nanoarchitecture of natural bone. In particular, this unique bone-like staggered nanointerface could facilitate M2 macrophage polarization, IL-4 secretion and host MSC recruitment during endogenous bone regeneration. In vivo experiments showed that HIMC loaded with IL-4 implantation into critical-sized mandible defects significantly accelerated fracture healing via inducing the macrophage polarization into the M2 phenotype.51 In another case, Song et al.59 prepared a hierarchical zinc silicate/nanohydroxyapatite/collagen (ZS/HA/Col) material by the robocasting method that is an advanced 3D printing technique possessing the capability to fabricate geometrically sophisticated materials with specific topographic characteristics, highly interconnected porosity, and unique internal architecture. ZS/HA/Col scaffolds with 10% ZS promoted the differentiation of monocytes into tartrate-resistant acid phosphatase (TRAP)-positive cells, which subsequently recruited BMSCs and endothelial cells into bone defect areas. In particular, the p38 signaling pathway in monocytes was activated and the secretion of cytokines (e.g. BMP-2, Osterix, VEGF, CD31, SDF-1, TGF-β1, and PDGF) was increased. The above results indicated that hierarchical 10ZS/HA/Col scaffolds facilitated the recruitment and differentiation of BMSCs as well as neovascularization through the activation of p38 signaling via modulating monocytes and thereby creating a favorable osteoimmune microenvironment.
With the advancement of nanotechnology, NMs with complicated geometric architectures can be successfully fabricated and increasing studies have demonstrated that nanoscale structures may improve bone formation efficiency via influencing the mutual interplay between the immune and skeletal systems; nevertheless, the exact biological mechanisms are still unknown and require further investigation (Fig. 5).
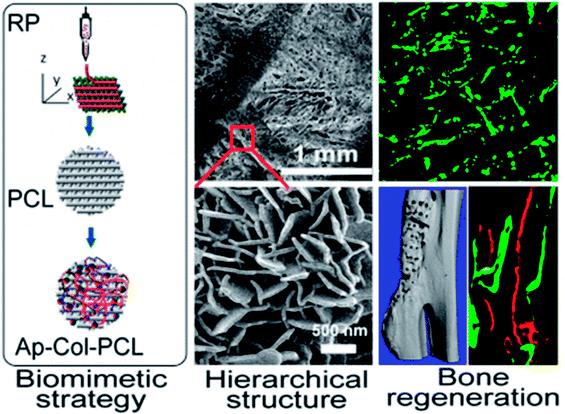 |
| Fig. 5 Schematic representation of biomimetically ornamented rapid prototyping fabrication of an apatite-collagen-polycaprolactone composite construct with a nano–micro–macro hierarchical structure for large bone defect treatment. Reprinted with permission from ref. 60. Copyright (2016) American Chemical Society Publications. | |
4.4 Nanomaterials as carriers for biomolecule delivery
Recent advances in nanomedicine have shown that engineered NMs can be utilized as targeting carried materials for the delivery of various drugs and bioactive molecules that regulate the cross effects among osteoblasts, osteoclasts and immunomodulatory cells.61 What needs to be emphasized is that the cross-talk between bone cells and immune cells is sophisticated, and care should be taken in the selection of immunomodulatory molecules, given that multiple associated signaling pathways are yet to be clarified. Nonetheless, studies on targeted delivery of biological molecules for the regulation of osteoimmunology have been extensively pursued. For instance, Li et al.62 prepared multi-functional TiO2 nanotubes achieving a controlled co-delivery of pro-osteogenic RGD peptide and anti-inflammatory cytokine IL-4 to create a favorable microenvironment for early bone formation. Initially, IL-4 wrapped in poly-dopamine (DOP) coating was implemented to achieve the functional modification of TiO2 nanotubes. Then, a carboxymethyl chitosan hydrogel layer was fabricated on the surface of DOP to incorporate RGD peptide. Experimental results showed that the multifunctional surface enhanced MSC recruitment and differentiation through affecting the BMP/SMAD/RUNX2 signaling pathway, and evoked macrophage switching into an anti-inflammatory M2 phenotype that promoted the secretion of reparative factors such as IL-10. Therefore, a Ti substrate tailored to delivery cell adhesive motifs and anti-inflammatory cytokines can synergistically create a favorable osteoimmune microenvironment to enhance early osteogenesis.
In addition, the delivery of proteins also displayed enhanced bone regeneration both for osteogenesis and immunomodulation. BMP-2 is a type of acknowledged growth factor with potentially immunomodulatory and osteoinductive effects and has been shown to induce macrophage polarization and promote cytokine secretion that are essential for osteogenesis and angiogenesis. In a case, Vantucci et al.63 prepared heparin methacrylamide microparticles (HMPs) that strongly bond BMP-2 in order to achieve spatiotemporal delivery profiles and reduce side effects due to excessive doses of BMP-2. Furthermore, HMP delivery vehicles with appropriate doses of BMP-2 stimulated immune effector cells (e.g. T cells) and increased the secretion of cytokines associated with bone regeneration. In conclusion, above results indicate that nanoscaled vehicle delivery of proteins could positively influence the systemic immune reaction to enhance bone formation. Nucleic acids are also a potential candidate for osteoimmunomodulation. In another case, a nanoplatform with multi-biologic delivery was successfully fabricated in order to release cytokines and miRNAs such as IL-2, TGF-β and miR-10a, which facilitated the local recruitment of T cells and evoked their differentiation into Tregs. In particular, recent studies have found that miR-10a is highly expressed in Tregs.64 Although few studies have reported the modulation of the osteoimmune microenvironment through nanocarrier-loaded genes in order to promote bone regeneration, it is possible to fabricate a polycation-plasmid nanoporous scaffold for osteoimmunomodulation in future.65
In conclusion, various strategies have been proposed to regulate inflammatory response and increase osteogenesis, such as delivery of cytokines, proteins, nucleic acids or immune modulators. Therefore, it is of great importance to research osteoimmunology and to reasonably select bioactive molecules with nanoscaled carriers to achieve successful treatment of bone related diseases (Fig. 6).
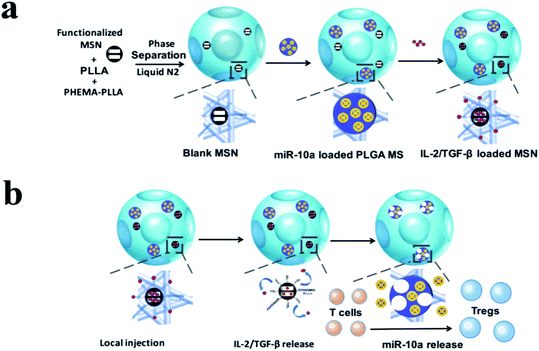 |
| Fig. 6 Schematic illustration of the fabrication of multi-functionalized poly(L-lactic acid) (PLLA) nanofibrous spongy microspheres (NF-SMS) and their intended release and function. (a) Illustrative flow chart of fabricating multi-functionalized PLLA NF-SMS with MSN to incorporate growth factors and PLGA MS to incorporate microRNA/HP polyplexes. (b) Schematic representation of the use of functionalized PLLA NF-SMS and their distinct releases of growth factors (IL-2/TGF-β) and miR-10a for T cell recruiting and transformation. The recruiting is achieved by the release of grow factors loaded in MSN. The Treg transformation is achieved by both extracellular IL-2/TGF-β release and efficient intracellular miR-10a delivery. Reprinted with permission from ref. 64. Copy right (2018) American Chemical Society Publications. | |
5. Engineering nanomaterials for immunomodulation and bone regeneration
Conventional bone graft materials such as bioactive ceramics, polymers or metals are incapable of accurately mimicking native bone compositions and structures for successful bone regeneration. To some extent, ceramics intrinsically easily cause local stress brittleness and produce poor flexibility but show a high elastic modulus. Polymeric scaffolds have been reported to maintain low mechanical stability and biocompatibility, and thus weaken the osteoinductive and osteoconductive capability. Furthermore, high stiffness as well as low biocompatibility restrict the application of metals (e.g. titanium alloy, cobalt chromium alloy and stainless steel) in bone tissue engineering.66 Concentrating on nanotechnology and nanomedicine, recent advances indicate that engineered NMs such as nanofibers, nanotubes, nanoparticles, nanospheres, nanocomposites, nanoporous scaffolds and nanostructured coatings are used for bone defect healing, which may be a promising strategy for bone regeneration. Importantly, NMs can mimic the hierarchical and nanoscaled characteristics of natural bone to provide unique “smart” graft materials that not only react to local mechanical stimuli but also conduct growth factors, promote cell communication and regulate immune response.67–69 For instance, Yin et al.70 prepared a biomimetic anti-inflammatory nanocapsule that can effectively facilitate M2 macrophage polarization, block cytokines, and play an active role in bone regeneration ex vivo and in vivo. Therefore, cytokine receptor enveloping nanosystems loaded with bioactive molecules may act as a macrophage polarization inducer and cytokine blocker.
Taken together, the nature of repairing materials evoking inflammatory response partly determined the success of bone regeneration; especially, intensive evidence has indicated that both the nanoscale design/size features and surface biochemistry of implanted materials could affect the inflammatory response in the bone microenvironment ranging from neutrophil infiltration to macrophage recruitment and eventually fibrous capsule formation. Therefore, the utilization of engineered NMs for osteoimmunomodulation to promote bone tissue repair and regeneration is an attractive strategy. We elaborate on various NMs promoting bone regeneration through immunomodulation in the following sections (Fig. 7).
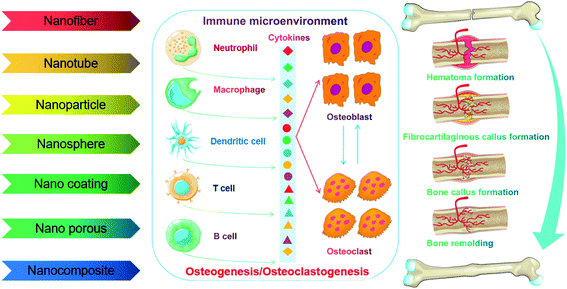 |
| Fig. 7 Illustration of NM engineering for the regulation of miscellaneous events during the bone remolding process associated with the immune reaction, inflammation, osteoblastogenesis, and osteoclastogenesis. | |
5.1 Nanofibers
Nanofibers have received extensive attention with the attributes of a high surface area to volume ratio, facile control of components, and the capacity to mimic matrix attributes including interconnected nanopores and surface nanotopography.71 With the use of a copper(I)-catalyzed azide–alkyne cycloaddition reaction, Sedghi et al.72 synthesized a novel type of composite nanofiber (diameter about 419–495 nm) with high bioactivity based on grafting polycaprolactone to chitosan for guided bone regeneration (GBR). As a result, the fabricated nanofibers showed higher mechanical properties, antibacterial activity and cell attachment of osteoblast-like MG63 cells in vitro, and enhanced in vivo bone mineralization ability due to the introduction of the triazole ring.
Additionally, these versatile materials can notably enhance osteogenesis and switch the macrophage phenotype towards the anti-inflammatory and pro-healing M2 extreme. For instance, electrospinning is the most common approach to fabricate nanofibers for tissue engineering, which generates fibers with diameters down to the nanoscale and are similar to the fibrous structures of the native extracellular matrix (ECM).73 Mathew et al.74 prepared a GBR membrane made of polycaprolactone (mPCL) electrospun fibers, which exhibited excellent antibacterial and immunomodulatory properties. Azithromycin loading on the mPCL membrane effectively inhibited the growth of Staphylococcus aureus and induced macrophage polarization toward the M2 phenotype in vivo. Self-assembly and phase separation have also been used to fabricate nanofiber scaffolds. The layer-by-layer (LbL) self-assembly method reserves multiple oppositely charged bio-recognition elements to produce a LbL film for the delivery of drugs or proteins. Han et al.75 prepared nanoparticle-assembling chitosan covered serum albumin and alginate on Ti scaffolds, which possessed hierarchical architectures and improved the biocompatibility and biofunctionality of the Ti surface. Some studies reported that scaffold fabrication by phase separation produces porous structures with a well-defined pore size and interconnected channels to allow cell communication and vascularization of the bone remolding phase. Therefore, nanofibers are potential materials that can be applied in bone regeneration. Specifically, aligned fibers with diameters of 600 nm or less have been used to fabricate bioactive surfaces or drug delivery systems, which may promote bone regeneration via regulating the immune response.76
5.2 Nanotubes
Carbon nanotubes (CNTs) are defined as column-form carbon tubes with nano-sized diameters, which is one of the most representative tubular nanoarchitectures. Increasing data have shown that CNTs are a class of promising engineered NMs for bone regeneration, exhibiting a unique 1D hollow structure and great electrical, thermal, mechanical and optical properties. They can promote the adhesion, spread and osteogenic differentiation of bone forming cells and inhibit osteoclastogenesis.77 Recent research has turned to their immunomodulatory effects on osteogenesis. For example, Dong et al.78 fabricated multi-walled carbon nanotubes (MWCNTs) that induced lessened viability of RAW 264.7 cells and moderately heightened the phagocytic activity of murine peritoneal macrophages. In addition, tailoring the surface of bio-polymer nanofibers with CNTs has also been reported to create a specific bimodal nanoscaled topography (500 nm nanofibers with 25 nm nanotubes) and hold advantages in modulating diverse in vivo and in vitro responses, including down-regulated macrophage gathering and expression of pro-inflammatory cytokines, enhanced angiogenic marker expression and new blood vessel formation, as well as up-regulated osteogenic signs (BMP2, OPN, and OCN) and accelerated bone regeneration.
In conclusion, CNTs can modulate the bone microenvironment and immune response for angiogenesis and osteogenesis, which ultimately orchestrate to promote fracture healing and bone regeneration processes. However, high-concentration MWCNTs could cause immunologic toxicity, which induces high levels of inflammation and immunosuppression and significant reduction of splenocytes.79 Although carbon nanotubes can switch the macrophage phenotype towards M2 for osteogenesis, mitigate the foreign body reaction and inhibit the expression of pro-inflammatory factors and the activation of related signaling, the high-concentration induced inflammatory reaction, cytotoxicity and non-biodegradability limit their application in bone tissue engineering.
5.3 Nanoparticles, nanospheres and nanocomposites
Nanoparticles and nanospheres intrinsically possess typical chemical properties, flexibility and porosity in design for the delivery of drugs, growth factors and genetic materials. It is worth noting that the utilization of nanoparticles as vehicles to deliver bioactive molecules has been reported to be an effective strategy for bone regeneration.80 For example, Nah et al.81 conjugated vitamin D to gold nanoparticles (VGNPs) through initially using thiol-PEG-vitamin D, in which this bio-inspired material (size of 60 nm) was well bound through the thiol groups between vitamin D and GNPs. In vitro, the fabricated VGNPs were found to significantly enhance the osteogenic differentiation of human adipose-derived stem cells (hADSCs). Based on these results, the incorporation of biomolecules and drugs into GNPs could be utilized as a new strategy to fabricate functional NMs for maintaining bone homeostasis.
Recently, the addition of nanoparticles to fabricate nanocomposites has been reported to enhance their mechanical stability, biocompatibility and immunoregulatory activity.82 In a case, Makvandi et al.83 synthesized injectable and thermosensitive hydrogels containing β-tricalcium phosphate, hyaluronic acid, and corn silk extract-nanosilver, exhibiting gelification temperature close to body temperature and negligible cytotoxicity. Furthermore, the nanocomposites facilitated osteogenic differentiation of MSCs and exhibited superior antibacterial activity toward Gram-positive and Gram-negative bacteria. Chitosan is a native biopolymer widely utilized in tissue engineering with excellent biocompatibility.84 Malathy et al.85 prepared a chitosan nanoparticle loading naringin with anti-inflammatory and antioxidant activities, which promoted osteoblastogenesis and inhibited osteoclastogenesis. In addition, the prefabricated magnetic nanoparticles (MNPs) enhanced bone remolding by up-regulating the expression of antiapoptotic factor B-cell lymphoma leukemia-2 (Bcl-2) in hADSCs.86 Zhu et al.87 also reported that MNPs could promote an acute inflammatory response while suppressing the chronic inflammatory response, leading to the recruitment of immune cells, remodeling of the ECM and eventually acceleration of bone healing. The above results suggest that these drug-loaded nanoparticles and nanospheres can control and targeted silence pro-inflammatory cytokines, holding great potential for osteoimmunomodulation. The following research should be oriented towards exploring the detailed contributions of the chemical composition and size characteristics of NMs to osteogenesis, angiogenesis and immune reaction during the bone remolding process, respectively.
5.4 Nanopore and nanoporous scaffolds
Nanopore and nanoporous scaffolds are defined as materials which possess pore sizes falling in the nano-dimension range, that is, <100 nm. Material porosity is a crucial design parameter of engineered scaffolds, which affects the infiltration of cells and blood vessels resulting in the promotion of integration with ambient tissue.88 In a case, Greiner et al.89 utilized SiO2 nanoparticles thermally cross-linked into a nanocomposite to fabricate biomimicking 31.93 ± 0.97 nm pores and induced osteogenic differentiation. This research revealed an endogenous mechanism of osteogenic differentiation of adult stem cells by nanoporous cues and provided a novel direction using materials with surface nanotopography bio-mimicking the native bone architecture.
Recently, some studies on topography-mediated bone regeneration indicated that the nanopore structure and pore size influence the cell shape and movement of macrophages, and subsequently regulate the expression and activation of autophagy and osteogenic signaling pathway and have essential effects on inflammatory reactions, osteoclastogenesis, and osteogenesis.90 Furthermore, nanopores of materials can evade the host's inflammatory system by resembling the native tissue/matrix architecture. For example, Velard et al.91 evaluated the inflammatory effects of several HA scaffolds with different nanopores, in which HA1 and HA6 were the most appropriate bone filling materials. HA1 powder evoked the most integrated osteoid generation and exhibited the largest matrix deposition. It also facilitated the most high-performance angiogenesis in the fracture site with little leukocytes infiltration or recruitment, although several CD68-positive cells surrounding the nanopore structure survived persistently. Similarly, HA6 evoked a slight inflammatory response with excellent angiogenesis of large blood vessels, minor resorption of the scaffold material and less infiltration of CD68-positive cells. Thus, HA scaffolds with nanopores could be a type of ideal material for immunomodulation in bone regeneration.
Based on above data, nanoporous structures may become an important direction for advanced immunotherapeutic strategy of bone graft materials. Nevertheless, reasonable design of these porous NMs in order to achieve accurate modulation of immune cells and bone cells during the bone repair process still faces many challenges.
5.5 Nanostructural coatings
As mentioned above, nanotopography is tunable and biomimetic to control the behaviour of immune cells in a predictable manner, which has been considered as a delicate strategy to develop a “smart” coating material for the modulation of the osteoimmune microenvironment.92 Several in vitro and in vivo data have supported the important role of surface topography in inflammatory reactions. Ti and titanium alloy implants are some of the most biocompatible and widely used metallic implant materials.93 Nanoscaled TiO2 coatings have been reported to attenuate the inflammatory activity of macrophages via inhibiting MAPK and NF-κB signaling pathways. Among them, 80 nm TiO2 coating surfaces may possess the weakest inflammatory reaction with lessened mRNA expression and protein secretion of MCP-1, MIP-1α, IL-1β, IL-6 and TNF-α.94,95 In addition, gold nanoparticle (AuNP) coatings with an appropriate scale of topography (20–30 nm) were nontoxic to mice and exhibited superior anti-inflammatory effects, in which the cell shapes of the macrophage changed from a more rounded shape into a more elongated spindle shape with apparent pseudopodia. AuNP coatings also precisely induced macrophage polarization from pro-inflammatory M1 toward the anti-inflammatory M2 extreme. The expression of inflammatory factors including IL-1β, IL-6, and TNF-α was lessened, but the expression of IL-10 produced by the bone-marrow-derived macrophage was up-regulated. It is well known that calcium ions are essential for physiological bone metabolism and have the capability to suppress the expression of inflammatory cytokine TNF-α via activating the CaSR signalling cascade and inhibiting NF-κB pathway.96 Silicon has also been reported to exhibit anti-oxidant and anti-inflammatory effects, which can inhibit the gene expression of TNF-α, COX-2, and iNOS and reduce the generation of NO and IL-6 in macrophages.97 Recently, Chen et al.98 fabricated barrier collagen membrane coating nanometer-sized Ca2ZnSi2O7, and in vitro and in vivo models indicated that this material strengthened the expression of pro-inflammatory cytokines including TNF-α, IL-1β, IL-6, and IL-18 and activated BMP, canonical Wnt/β-catenin and OSM signaling to induce osteogenic differentiation of BMSCs. Furthermore, it's worth noting that superparamagnetic coatings on magnetization drive macrophages towards M2-like polarization thus construct a favorable microenvironment for angiogenesis and osteogenesis.99
Taken together, tuning the chemistry and topography of NMs can change the cell morphology and motility, and evoke unique modifications in gene expression and phenotypic switches. Metal coating nanocomposites possess excellent prospects in osteogenesis and immunomodulation and may be a promising scaffold material for bone regeneration. Nevertheless, the immunoregulatory cues inherent to the nanostructure and nanotopography are not thoroughly clarified, which constitutes a vibrant field of future research (Table 1).
Table 1 Paradigms of engineering nanomaterials for immunomodulatory bone regeneration
Nanomaterial |
Type |
Size |
Immunomodulatory mechanism |
References |
Magnetic HA |
Nanoparticles |
13 ± 1 nm |
Magnetic HA suppresses the chronic inflammatory reaction but promotes an acute inflammatory reaction |
87
|
Gold-doped mesoporous silica (Au-MSNs) |
Nanoparticles |
15 nm |
Au-MSNs activate an anti-inflammatory reaction and promote the expression of osteogenic factors via macrophages |
100
|
Lithium-doped Ti scaffolds |
Nanoparticles |
52.5 ± 20.48 nm |
LiCl switches macrophages into the M2 phenotype and facilitates the production of anti-inflammatory and bone-related factors, and thus enhances osteogenic differentiation of rBMSCs |
101
|
Copper-doped mesoporous silica |
Nanospheres |
90–110 nm |
Copper-doped mesoporous silica initiates suitable inflammatory factors, induces osteogenic/angiogenic factors and inhibits osteoclastogenic factors via immune cells |
102
|
Europium-doped mesoporous silica |
Nanospheres |
230–300 nm |
Europium-doped mesoporous silica promotes osteogenesis and angiogenesis via inducing a favorable osteoimmune microenvironment |
103
|
Graphene oxide complexed with calcium phosphate (maGO-CaP) |
Nanocomposites |
18 ± 4 nm |
maGO-CaP stimulates monocytes to promote osteogenesis by the production of oncostatin M, up-regulation of Wnt and BMP signaling |
58
|
Biomimetic calcium deficient HA |
Nanopores |
5.2 nm |
Nanoporous HA induces a favourable osteoimmune microenvironment to facilitate bone regeneration |
104
|
Poly(L-lactic acid) nanofibrous spongy microspheres (PLLA-NF-SMS) |
Nanofibers |
15 nm |
PLLA-NF-SMS releases miRNA and growth factors, leading to Treg-mediated immune therapy against bone loss |
64
|
Medical polycaprolactone nanofibers (mPCL-NFs) |
Nanofibers |
500 nm |
mPCL-NFs are loaded with antibiotic azithromycin to induce macrophage polarization |
74
|
Carbon nanotubes (CNTs) |
Nanotubes |
25 nm |
The CNTs suppress the expression of pro-inflammatory factors and macrophage gathering, and promote angiogenesis and osteogenesis |
105
|
TiO2 nanotubes |
Nanotubes |
30–80 nm |
Nanostructured Ti surfaces influence osteogenesis by RANKL/OPG/M-CSF in response to a macrophage-mediated inflammatory reaction |
54
|
Silver-loaded TiO2 nanotubes (Ag@TiO2-NT) |
Nanotubes |
130–140 nm |
Ag@TiO2-NT scaffolds inhibit PI3K/Akt signaling and downstream effector GLUT1, activate autophagy, induce M2 polarization and finally promote bone repair |
106
|
Mg-doped nanoengineered Ti surface |
Nanostructured coatings |
100–200 nm |
Mg evokes macrophage polarization toward the M2 phenotype and exerts an advantageous effect on osteogenesis |
107
|
Hierarchical intrafibrillarly mineralized collagen (HIMC) |
Nanointerface |
154.2 ± 19.6 μm |
HIMC recruits host MSCs and promotes endogenous bone regeneration by the secretion of IL-4 and regulation of macrophage polarization |
51
|
6. Challenges and opportunities for clinical translation
The immune system is a strong and sophisticated defence system responsible for detecting and eliminating infectious organisms, cancer cells and particulate heterogeneous materials. As a crucial process of adapting immunity, immune regulation aims to acquire the optimal reaction both in terms of immunosuppression in autoimmunity, organ transplantation or inflammation disorders and immunostimulation in vaccination or cancer immunotherapy.19,32 Currently, nanotechnology has been extensively applied in medicine and the topic of NM-immune interaction has attracted extensive attention of researchers in recent decades.108 NMs can act as a modulator to control immune response directly through their components and structures or indirectly as integrated carriers of bioactive molecules. In particular, recent studies have indicated that engineered NMs possess great ability to control the polarization direction of macrophages and promote the osteogenic differentiation of MSCs and may be a promising candidate for immunomodulatory bone regeneration.109
Despite increasing studies focusing on the reciprocity of biomedical NMs with the immune system, such as NM-mediated immune reactions, tissue regeneration, drug delivery and tumor therapy, many knowledge gaps for the immunoreaction and immunotherapy concerning NMs still exist. The heterogeneity and complexity of the immune-NM interface make current studies hard due to the shortage of database systems concentrating on necessary immune-NM reciprocities.110 Indeed, the immune-NM interface exerts various driving forces (e.g. hydrogen bonding, metal coordination, stereoselective interaction, electrostatic interaction, etc.) that significantly influence the biological functions, surface properties, intracellular uptake and in vivo transformation pathways of NMs. Moreover, the expression of receptors on the surfaces of macrophages and secretion of related cytokines are modulated by entropic and nanothermodynamic potentials that occur at the immune-NM interfaces. NMs as a foreign agent may evoke body defensive reactions leading to the degradation and clearance of themselves and destroy the function of the immune system and result in pathological changes. Although NMs with good immunocompatibility possess high drug utilization efficiency, good targeting capability, long-term circulation in the body, low cytotoxicity and inflammatory response, the interaction between NMs and the immune system may result in some diseases like inflammatory arthritis, autoimmune diseases, and allergic inflammation, which still remains a huge challenge. In order to address these issues, biomimetic or modified coating of NMs with the ability of escaping clearance through the immune system may be an effective approach.111 Increasing researchers sustain the notion that bionic NM-immunotherapy can be applied throughout the biomedical field, such as cancer therapy, tumor imaging, tissue regeneration, stem autoimmunity, detecting atherosclerosis, tackling bacterial infections, broad-spectrum detoxicants, increasing nano-vaccine effectiveness, and nanocarriers with high specific targeting. Understanding how NMs regulate the immune system is important for the research and development of NMs for bone regeneration.112
However, the NM-induced immune reaction and its molecular mechanism are still ambiguous due to the lack of perfect databases to comprehensively recognize the biological properties of the NMs. Currently, no comprehensive databases characterize and organize the complex physical, chemical and biological properties of biomedical NMs. Therefore, it is of great difficulty to understand the corresponding connections regarding the types of complement activation pathways and the properties of NMs. Furthermore, unpredictable NM-immune response hampers the design and development of ideal NMs for immunotherapy. Intriguingly, recent advances in computer science have indicated that deep learning, a field in artificial intelligence (AI), can comprehensively analyse large data sets from previous samples to recognize and classify data and patterns, eventually finding an optimal solution to this conundrum.113 Considering the complex characteristics of immune response and NMs, deep machine learning and quantitative structure–activity relationship (QSAR) models will become intelligent approaches to explore and forecast the interactions between the immune system and NMs, improve nanomedicine formulations, lessen the workload spent on NM discovery, obtain desirable immunotherapy and eliminate poor immune response.114 For instance, Lin et al. developed a machine learning algorithm based on support vector machines, which could simultaneously screen thousands of articles with sensitive search strategies and evaluate the effects of different drugs on bone integration. The accuracy of this method reaches 95%, prominently reducing the workload by 93% compared with manual screening. Subsequently, this reliable machine learning algorithm may be used to evaluate the nanosafety and osteoimmunomodulatory effects of NMs. As a reminder, computational methods are not widespread and demands much solid data for training and improvement (Fig. 8).
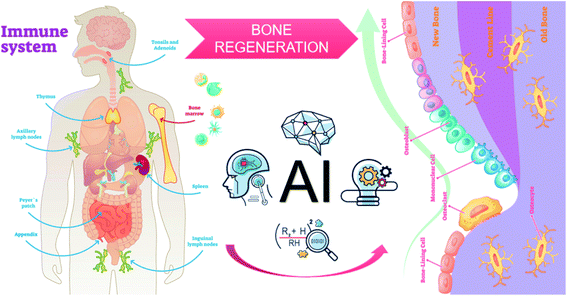 |
| Fig. 8 Combination of databases and AI for the design of immunomodulatory NMs in bone tissue engineering. | |
7. Conclusion and prospects
Emerging fields focusing on nanomedicine have appeared and have been greatly booming over the past few years. With increasing knowledge about the immunological variations induced via novel nanotechnology, it is promising to design intelligent biomedical NMs matching with targeted drug delivery, immune activation and immune therapy. Additionally, osteoimmunology is an attractive field concentrating on the molecular mechanisms of interaction between the skeletal and immune systems, and published investigation results provide a potential opportunity for the development of nano-osteoimmunology, and thus NMs can modulate immune response and inflammation for effective therapies of bone-related diseases. The surface chemistry, topography, particle size, porosity, released ions and structural effects of NMs can affect immune response, which accommodate the ratio of pro-inflammatory M1 and anti-inflammatory M2 macrophages in progressive bone remolding phases and facilitate the secretion of pro-regenerative factors, as well as possessing the capability of preventing “foreign body” reactions. Although current research concentrating on NM-based therapies has made rapid development, clinical translation is still relatively low. The promotion of clinical translation of nanomedicine in future will be the key challenge. Functionalization with tailor-made ligands with precise microenvironment responsiveness, cell-based nanocarrier delivery, and collaborative delivery of biomolecules with immunomodulatory roles are upcoming strategies for tackling these challenges.
Nanotechnology is also promising for immunomodulatory bone regeneration. With the development of osteoimmunology, materials in bone tissue engineering have recently advanced to modulating inflammatory response based on the increasing understanding of the interaction between the immune system and skeletal system. In order to develop ideal NMs with favorable immunomodulatory attributes, next studies need to investigate the underlying mechanism of how immune cells and cytokines regulate bone dynamics and create a beneficial microenvironment for successful bone regeneration. Furthermore, researchers will continuously improve and optimize the fabrication techniques and strategies of NMs to tune the physicochemical and mechanical surface properties for the precise modulation of bone homeostasis.
Finally, what needs to be emphasized is that safety is the primary concern of NMs in the human body. Although NMs have great potential for biomedical engineering, the exact mechanisms and related pathways about the immunological and toxicological effect of NMs are still not fully unraveled. Therefore, it is crucial to explore the impact of the size, shape and surface chemistry of NMs on a particular immune compartment or body organ as the nanostructure can potentially cause adverse reactions with the surrounding tissues. Indeed, much room remains to optimize the physicochemical properties of NMs, and improve the immune response and immunotherapy of NMs. In particular, computational methods, such as AI or deep machine learning, are upcoming in bionanotechnological research and could combine the sophisticated properties of NMs with the information of immunoreaction and immunotherapy and thereby forecast the immunoreaction or immunotherapy effects of new NMs, facilitating systematic optimization steps.
Funding
The National Natural Science Foundation of China, grant number: 81701020 and the Shanghai Municipal Commission of Health and Family Planning, grant number: 201740035.
Conflicts of interest
There are no conflicts to declare.
References
- D. Taylor, Fracture mechanics: How does bone break?, Nat. Mater., 2003, 2, 133–134 CrossRef CAS PubMed.
- S. Shahnaseri, M. Sheikhi and B. Hashemibeni,
et al., Comparison of autogenous bone graft and tissue-engineered bone graft in alveolar cleft defects in canine animal models using digital radiography, Indian J. Dent. Res., 2020, 31, 118–123 CrossRef PubMed.
- H. Hajiali, L. Ouyang and V. Llopis-Hernandez,
et al., Review of emerging nanotechnology in bone regeneration: progress, challenges, and perspectives, Nanoscale, 2021, 13, 10266–10280 RSC.
- M. Tsukasaki and H. Takayanagi, Osteoimmunology: evolving concepts in bone-immune interactions in health and disease, Nat. Rev. Immunol., 2019, 19, 626–642 CrossRef CAS PubMed.
- M. C. Walsh, N. Takegahara and H. Kim,
et al., Updating osteoimmunology: regulation of bone cells by innate and adaptive immunity, Nat. Rev. Rheumatol., 2018, 14, 146–156 CrossRef CAS PubMed.
- Y. Shi, Y. Wang and Q. Li,
et al., Immunoregulatory mechanisms of mesenchymal stem and stromal cells in inflammatory diseases, Nat. Rev. Nephrol., 2018, 14, 493–507 CrossRef CAS PubMed.
- S. J. Wang, D. Jiang and Z. Z. Zhang,
et al., Biomimetic Nanosilica-Collagen Scaffolds for In Situ Bone Regeneration: Toward a Cell-Free, One-Step Surgery, Adv. Mater., 2019, 31, e1904341 CrossRef PubMed.
- P. Zhang, Y. Zhai and Y. Cai,
et al., Nanomedicine-Based Immunotherapy for the Treatment of Cancer Metastasis, Adv. Mater., 2019, 31, e1904156 CrossRef PubMed.
- F. Albalawi, M. Z. Hussein and S. Fakurazi,
et al., Engineered Nanomaterials: The Challenges and Opportunities for Nanomedicines, Int. J. Nanomed., 2021, 16, 161–184 CrossRef PubMed.
- Y. Li and C. Liu, Nanomaterial-based bone regeneration, Nanoscale, 2017, 9, 4862–4874 RSC.
- R. Müller, Hierarchical microimaging of bone structure and function, Nat. Rev. Rheumatol., 2009, 5, 373–381 CrossRef PubMed.
- G. Zhu, T. Zhang and M. Chen,
et al., Bone physiological microenvironment and healing mechanism: Basis for future bone-tissue engineering scaffolds, Bioact. Mater., 2021, 6, 4110–4140 CrossRef CAS PubMed.
- U. G. Wegst, H. Bai and E. Saiz,
et al., Bioinspired structural materials, Nat. Mater., 2015, 14, 23–36 CrossRef CAS PubMed.
- R. M. Hoerth, M. Kerschnitzki and M. Aido,
et al., Correlations between nanostructure and micromechanical properties of healing bone, J. Mech. Behav. Biomed. Mater., 2018, 77, 258–266 CrossRef PubMed.
- T. A. Einhorn and L. C. Gerstenfeld, Fracture healing: mechanisms and interventions, Nat. Rev. Rheumatol., 2015, 11, 45–54 CrossRef PubMed.
- L. Claes, S. Recknagel and A. Ignatius, Fracture healing under healthy and inflammatory conditions, Nat. Rev. Rheumatol., 2012, 8, 133–143 CrossRef CAS PubMed.
- C. W. Schlickewei, H. Kleinertz and D. M. Thiesen,
et al., Current and Future Concepts for the Treatment of Impaired Fracture Healing, Int. J. Mol. Sci., 2019, 20, 5805–5831 CrossRef CAS PubMed.
- P. Zhou, D. Xia and Z. Ni,
et al., Calcium silicate bioactive ceramics induce osteogenesis through oncostatin M, Bioact. Mater., 2021, 6, 810–822 CrossRef CAS PubMed.
- D. D. Chaplin, Overview of the immune response, J. Allergy Clin. Immunol., 2010, 125, S3–S23 CrossRef PubMed.
- R. J. Vagnozzi, M. Maillet and M. A. Sargent,
et al., An acute immune response underlies the benefit of cardiac stem cell therapy, Nature, 2020, 577, 405–409 CrossRef CAS PubMed.
- H. Kumar, T. Kawai and S. Akira, Pathogen recognition by the innate immune system, Int. Rev. Immunol., 2011, 30, 16–34 CrossRef CAS PubMed.
- C. Yang, Inflammation in Tissue and Organ Injury, Repair and Regeneration, Curr. Protein Pept. Sci., 2019, 20, 768–769 CrossRef CAS PubMed.
- D. R. Germolec, K. A. Shipkowski and R. P. Frawley,
et al., Markers of Inflammation, Methods Mol. Biol., 2018, 1803, 57–79 CrossRef CAS PubMed.
- Y. T. Yeung, F. Aziz and A. Guerrero-Castilla,
et al., Signaling Pathways in Inflammation and Anti-inflammatory Therapies, Curr. Pharm. Des., 2018, 24, 1449–1484 CrossRef CAS PubMed.
- F. V. S. Castanheira and P. Kubes, Neutrophils and NETs in modulating acute and chronic inflammation, Blood, 2019, 133, 2178–2185 CrossRef CAS PubMed.
- B. Cai, D. Lin and Y. Li,
et al., N2-Polarized Neutrophils Guide Bone Mesenchymal Stem Cell Recruitment and Initiate Bone Regeneration: A Missing Piece of the Bone Regeneration Puzzle, Adv. Sci., 2021, e2100584 CrossRef PubMed.
- K. Hamidzadeh, S. M. Christensen and E. Dalby,
et al., Macrophages and the Recovery from Acute and Chronic Inflammation, Annu. Rev. Physiol., 2017, 79, 567–592 CrossRef CAS PubMed.
- J. Li, Y. J. Zhang and Z. Y. Lv,
et al., The observed difference of macrophage phenotype on different surface roughness of mineralized collagen, Regener. Biomater., 2020, 7, 203–211 CrossRef CAS PubMed.
- M. N. Michalski and L. K. McCauley, Macrophages and skeletal health, Pharmacol. Ther., 2017, 174, 43–54 CrossRef CAS PubMed.
- J. Pajarinen, T. Lin and E. Gibon,
et al., Mesenchymal stem cell-macrophage crosstalk and bone healing, Biomaterials, 2019, 196, 80–89 CrossRef CAS PubMed.
- V. Lenders, X. Koutsoumpou and A. Sargsian,
et al., Biomedical nanomaterials for immunological applications: ongoing research and clinical trials, Nanoscale Adv., 2020, 2, 5046–5089 RSC.
- J. Kubackova, J. Zbytovska and O. Holas, Nanomaterials for direct and indirect immunomodulation: A review of applications, Eur. J. Pharm. Sci., 2020, 142, 105139 CrossRef CAS PubMed.
- C. Qian and X. Cao, Dendritic cells in the regulation of immunity and inflammation, Semin. Immunol., 2018, 35, 3–11 CrossRef CAS PubMed.
- E. H. Choo, J. H. Lee and E. H. Park,
et al., Infarcted Myocardium-Primed Dendritic Cells Improve Remodeling and Cardiac Function After Myocardial Infarction by Modulating the Regulatory T Cell and Macrophage Polarization, Circulation, 2017, 135, 1444–1457 CrossRef CAS PubMed.
- F. J. Zhu, Y. L. Tong and Z. Y. Sheng,
et al., Role of dendritic cells in the host response to biomaterials and their signaling pathways, Acta Biomater., 2019, 94, 132–144 CrossRef CAS PubMed.
- D. Guérit, P. Marie and A. Morel,
et al., Primary myeloid cell proteomics and transcriptomics: importance of β-tubulin isotypes for osteoclast function, J. Cell Sci., 2020, 133, 120–135 Search PubMed.
- J. C. Ribot, N. Lopes and B. Silva-Santos, γδ T cells in tissue physiology and surveillance, Nat. Rev. Immunol., 2021, 21, 221–232 CrossRef CAS PubMed.
- K. Nagai, H. Ideguchi and T. Kajikawa,
et al., An injectable hydrogel-formulated inhibitor of prolyl-4-hydroxylase promotes T regulatory cell recruitment and enhances alveolar bone regeneration during resolution of experimental periodontitis, FASEB J., 2020, 34, 13726–13740 CrossRef CAS PubMed.
- C. Schlundt, S. Reinke and S. Geissler,
et al., Individual Effector/Regulator T Cell Ratios Impact Bone Regeneration, Front. Immunol., 2019, 10, 1954 CrossRef CAS PubMed.
- S. Krishnan, I. E. Prise and K. Wemyss,
et al., Amphiregulin-producing γδ T cells are vital for safeguarding oral barrier immune homeostasis, Proc. Natl. Acad. Sci. U. S. A., 2018, 115, 10738–10743 CrossRef CAS PubMed.
- T. Ono, K. Okamoto and T. Nakashima,
et al., IL-17-producing γδ T cells enhance bone regeneration, Nat. Commun., 2016, 7, 10928 CrossRef CAS PubMed.
- I. Könnecke, A. Serra and T. El Khassawna,
et al., T and B cells participate in bone repair by infiltrating the fracture callus in a two-wave fashion, Bone, 2014, 64, 155–165 CrossRef PubMed.
- M. Liu, N. A. Amro and G. Y. Liu, Nanografting for surface physical chemistry, Annu. Rev. Phys. Chem., 2008, 59, 367–386 CrossRef CAS PubMed.
- M. Bartkowski and S. Giordani, Supramolecular chemistry of carbon nano-onions, Nanoscale, 2020, 12, 9352–9358 RSC.
- D. de Melo Pereira and P. Habibovic, Biomineralization-Inspired Material Design for Bone Regeneration, Adv. Healthcare Mater., 2018, 7, e1800700 CrossRef PubMed.
- R. Siqueira, J. A. Ferreira and F. A. P. Rizzante,
et al., Hydrophilic titanium surface modulates early stages of osseointegration in osteoporosis, J. Periodontal Res., 2021, 56, 351–362 CrossRef CAS PubMed.
- Q. L. Ma, L. Z. Zhao and R. R. Liu,
et al., Improved implant osseointegration of a nanostructured titanium surface via mediation of macrophage polarization, Biomaterials, 2014, 35, 9853–9867 CrossRef CAS PubMed.
- I. Venditti, L. Fontana and I. Fratoddi,
et al., Direct interaction of hydrophilic gold nanoparticles with dexamethasone drug: loading and release study, J. Colloid Interface Sci., 2014, 418, 52–60 CrossRef CAS PubMed.
- W. G. Brodbeck, Y. Nakayama and T. Matsuda,
et al., Biomaterial surface chemistry dictates adherent monocyte/macrophage cytokine expression in vitro, Cytokine, 2002, 18, 311–319 CrossRef CAS PubMed.
- L. Cheng, Z. Chen and Z. Cai,
et al., Bioinspired Functional Black Phosphorus Electrospun Fibers Achieving Recruitment and Biomineralization for Staged Bone Regeneration, Small, 2020, 16, e2005433 CrossRef PubMed.
- S. S. Jin, D. Q. He and D. Luo,
et al., A Biomimetic Hierarchical Nanointerface Orchestrates Macrophage Polarization and Mesenchymal Stem Cell Recruitment To Promote Endogenous Bone Regeneration, ACS Nano, 2019, 13, 6581–6595 CrossRef CAS PubMed.
- X. Zheng, L. Xin and Y. Luo,
et al., Near-Infrared-Triggered Dynamic Surface Topography for Sequential Modulation of Macrophage Phenotypes, ACS Appl. Mater. Interfaces, 2019, 11, 43689–43697 CrossRef CAS PubMed.
- Q. Huang, Z. Ouyang and Y. Tan,
et al., Activating macrophages for enhanced osteogenic and bactericidal performance by Cu ion release from micro/nano-topographical coating on a titanium substrate, Acta Biomater., 2019, 100, 415–426 CrossRef CAS PubMed.
- Q. L. Ma, L. Fang and N. Jiang,
et al., Bone mesenchymal stem cell secretion of sRANKL/OPG/M-CSF in response to macrophage-mediated inflammatory response influences osteogenesis on nanostructured Ti surfaces, Biomaterials, 2018, 154, 234–247 CrossRef CAS PubMed.
- Z. Chen, S. Ni and S. Han,
et al., Nanoporous microstructures mediate osteogenesis by modulating the osteo-immune response of macrophages, Nanoscale, 2017, 9, 706–718 RSC.
- Z. Chen, A. Bachhuka and S. Han,
et al., Tuning Chemistry and Topography of Nanoengineered Surfaces to Manipulate Immune Response for Bone Regeneration Applications, ACS Nano, 2017, 11, 4494–4506 CrossRef CAS PubMed.
- C. Ma, Y. Jing and H. Sun,
et al., Hierarchical Nanofibrous Microspheres with Controlled Growth Factor Delivery for Bone Regeneration, Adv. Healthcare Mater., 2015, 4, 2699–2708 CrossRef CAS PubMed.
- V. Bordoni, G. Reina and M. Orecchioni,
et al., Stimulation of bone formation by monocyte-activator functionalized graphene oxide in vivo, Nanoscale, 2019, 11, 19408–19421 RSC.
- Y. Song, H. Wu and Y. Gao,
et al., Zinc Silicate/Nano-Hydroxyapatite/Collagen Scaffolds Promote Angiogenesis and Bone Regeneration via the p38 MAPK Pathway in Activated Monocytes, ACS Appl. Mater. Interfaces, 2020, 12, 16058–16075 CrossRef CAS PubMed.
- J. Wang, D. Wu and Z. Zhang,
et al., Biomimetically Ornamented Rapid Prototyping Fabrication of an Apatite-Collagen-Polycaprolactone Composite Construct with Nano-Micro–Macro Hierarchical Structure for Large Bone Defect Treatment, ACS Appl. Mater. Interfaces, 2015, 7, 26244–26256 CrossRef CAS PubMed.
- M. L. P. Vidallon and B. M. Teo, Recent developments in biomolecule-based nanoencapsulation systems for antimicrobial delivery and biofilm disruption, Chem. Commun., 2020, 56, 13907–13917 RSC.
- M. Li, F. Wei and X. Yin,
et al., Synergistic regulation of osteoimmune microenvironment by IL-4 and RGD to accelerate osteogenesis, Mater. Sci. Eng., C, 2020, 109, 110508 CrossRef CAS PubMed.
- C. E. Vantucci, L. Krishan and A. Cheng,
et al., BMP-2 delivery strategy modulates local bone regeneration and systemic immune responses to complex extremity trauma, Biomater. Sci., 2021, 9, 1668–1682 RSC.
- Z. Liu, X. Chen and Z. Zhang,
et al., Nanofibrous Spongy Microspheres To Distinctly Release miRNA and Growth Factors To Enrich Regulatory T Cells and Rescue Periodontal Bone Loss, ACS Nano, 2018, 12, 9785–9799 CrossRef CAS PubMed.
- B. Khorsand, N. Nicholson and A. V. Do,
et al., Regeneration of bone using nanoplex delivery of FGF-2 and BMP-2 genes in diaphyseal long bone radial defects in a diabetic rabbit model, J. Controlled Release, 2017, 248, 53–59 CrossRef CAS PubMed.
- A. Wubneh, E. K. Tsekoura and C. Ayranci,
et al., Current state of fabrication technologies and materials for bone tissue engineering, Acta Biomater., 2018, 80, 1–30 CrossRef CAS PubMed.
- Z. Peng, T. Zhao and Y. Zhou,
et al., Bone Tissue Engineering via Carbon-Based Nanomaterials, Adv. Healthcare Mater., 2020, 9, e1901495 CrossRef PubMed.
- R. Singla, S. M. S. Abidi and A. I. Dar,
et al., Nanomaterials as potential and versatile platform for next generation tissue engineering applications, J. Biomed. Mater. Res., Part B, 2019, 107, 2433–2449 CrossRef CAS PubMed.
- M. Qasim, D. S. Chae and N. Y. Lee, Advancements and frontiers in nano-based 3D and 4D scaffolds for bone and cartilage tissue engineering, Int. J. Nanomed., 2019, 14, 4333–4351 CrossRef CAS PubMed.
- C. Yin, Q. Zhao and W. Li,
et al., Biomimetic anti-inflammatory nano-capsule serves as a cytokine blocker and M2 polarization inducer for bone tissue repair, Acta Biomater., 2020, 102, 416–426 CrossRef CAS PubMed.
- Z. Du, Z. Zhao and H. Liu,
et al., Macroporous scaffolds developed from CaSiO(3) nanofibers regulating bone regeneration via controlled calcination, Mater. Sci. Eng., C, 2020, 113, 111005 CrossRef CAS PubMed.
- R. Sedghi, A. Shaabani and N. Sayyari, Electrospun triazole-based chitosan nanofibers as a novel scaffolds for bone tissue repair and regeneration, Carbohydr. Polym., 2020, 230, 115707 CrossRef CAS PubMed.
- Y. Wang, W. Cui and X. Zhao,
et al., Bone remodeling-inspired dual delivery electrospun nanofibers for promoting bone regeneration, Nanoscale, 2018, 11, 60–71 RSC.
- A. Mathew, C. Vaquette and S. Hashimi,
et al., Antimicrobial and Immunomodulatory Surface-Functionalized Electrospun Membranes for Bone Regeneration, Adv. Healthcare Mater., 2017, 6, 120–132 Search PubMed.
- L. Han, M. Wang and H. Sun,
et al., Porous titanium scaffolds with self-assembled micro/nano-hierarchical structure for dual functions of bone regeneration and anti-infection, J. Biomed. Mater. Res., 2017, 105, 3482–3492 CrossRef CAS PubMed.
- Y. Qian, X. Zhou and H. Sun,
et al., Biomimetic Domain-Active Electrospun Scaffolds Facilitating Bone Regeneration Synergistically with Antibacterial Efficacy for Bone Defects, ACS Appl. Mater. Interfaces, 2018, 10, 3248–3259 CrossRef CAS PubMed.
- M. Tanaka, Y. Sato and M. Zhang,
et al., In Vitro and In Vivo Evaluation of a Three-Dimensional Porous Multi-Walled Carbon Nanotube Scaffold for Bone Regeneration, Nanomaterials, 2017, 7, 46–63 CrossRef PubMed.
- X. Dong, L. Liu and D. Zhu,
et al., Effects of Carboxylated Multiwalled Carbon Nanotubes on the Function of Macrophages, J. Nanomater., 2015, 2015, 4 Search PubMed.
- T. Zhang, M. Tang and S. Zhang,
et al., Systemic and immunotoxicity of pristine and PEGylated multi-walled carbon nanotubes in an intravenous 28 days repeated dose toxicity study, Int. J. Nanomed., 2017, 12, 1539–1554 CrossRef CAS PubMed.
- T. J. Levingstone, S. Herbaj and J. Redmond,
et al., Calcium Phosphate Nanoparticles-Based Systems for RNAi Delivery: Applications in Bone Tissue Regeneration, Nanomaterials, 2020, 10, 146–174 CrossRef CAS PubMed.
- H. Nah, D. Lee and M. Heo,
et al., Vitamin D-conjugated gold nanoparticles as functional carriers to enhancing osteogenic differentiation, Sci. Technol. Adv. Mater., 2019, 20, 826–836 CrossRef CAS PubMed.
- H. J. Kim, S. Lee and J. M. Park,
et al., Development of a three-layer consecutive gene delivery system for enhanced bone regeneration, Biomaterials, 2021, 277, 121104 CrossRef CAS PubMed.
- P. Makvandi, G. W. Ali and F. Della Sala,
et al., Biosynthesis and characterization of antibacterial thermosensitive hydrogels based on corn silk extract, hyaluronic acid and nanosilver for potential wound healing, Carbohydr. Polym., 2019, 223, 115023 CrossRef CAS PubMed.
- Z. K. Cui, S. Kim and J. J. Baljon,
et al., Microporous methacrylated glycol chitosan-montmorillonite nanocomposite hydrogel for bone
tissue engineering, Nat. Commun., 2019, 10, 3523 CrossRef PubMed.
- S. Malathy and P. R. Iyer, Naringin Loaded Chitosan Nanoparticle for Bone Regeneration: A Preliminary in vitro Study, J. Nanomed. Nanotechnol., 2018, 9, 1024 Search PubMed.
- E. Brett, E. R. Zielins and A. Luan,
et al., Magnetic Nanoparticle-Based Upregulation of B-Cell Lymphoma 2 Enhances Bone Regeneration, Stem Cells Transl. Med., 2017, 6, 151–160 CrossRef CAS PubMed.
- Y. Zhu, P. Jiang and B. Luo,
et al., Dynamic protein corona influences immune-modulating osteogenesis in magnetic nanoparticle (MNP)-infiltrated bone regeneration scaffolds in vivo, Nanoscale, 2019, 11, 6817–6827 RSC.
- K. Hayashi and K. Ishikawa, Effects of nanopores on the mechanical strength, osteoclastogenesis, and osteogenesis in honeycomb scaffolds, J. Mater. Chem. B, 2020, 8, 8536–8545 RSC.
- J. F. Greiner, M. Gottschalk and N. Fokin,
et al., Natural and synthetic nanopores directing osteogenic differentiation of human stem cells, Nanomedicine, 2019, 17, 319–328 CrossRef CAS PubMed.
- X. Li, M. Wang and W. Zhang,
et al., A Magnesium-Incorporated Nanoporous Titanium Coating for Rapid Osseointegration, Int. J. Nanomed., 2020, 15, 6593–6603 CrossRef CAS PubMed.
- F. Velard, S. Schlaubitz and J. C. Fricain,
et al., In vitro and in vivo evaluation of the inflammatory potential of various nanoporous hydroxyapatite biomaterials, Nanomedicine, 2015, 10, 785–802 CrossRef CAS PubMed.
- S. Ni, D. Zhai and Z. Huan,
et al., Nanosized concave pit/convex dot microarray for immunomodulatory osteogenesis and angiogenesis, Nanoscale, 2020, 12, 16474–16488 RSC.
- Z. Ma, J. Li and F. Cao,
et al., Porous silicon carbide coated with tantalum as potential material for bone implants, Regener. Biomater., 2020, 7, 453–459 CrossRef CAS PubMed.
- Y. He, Z. Li and X. Ding,
et al., Nanoporous titanium implant surface promotes osteogenesis by suppressing osteoclastogenesis via integrin β1/FAKpY397/MAPK pathway, Bioact. Mater., 2022, 8, 109–123 CrossRef CAS PubMed.
- P. M. Yang, H. Z. Mu and Y. S. Zhang,
et al., Sequential release of immunomodulatory cytokines binding on nano-hydroxyapatite coated titanium surface for regulating macrophage polarization and bone regeneration, Med. Hypotheses, 2020, 144, 110241 CrossRef CAS PubMed.
- F. Romero-Gavilán, A. Cerqueira and E. Anitua,
et al., Protein adsorption/desorption dynamics on Ca-enriched titanium surfaces: biological implications, J. Biol. Inorg Chem., 2021, 26, 715–726 CrossRef PubMed.
- Y. Huang, C. Wu and X. Zhang,
et al., Regulation of immune response by bioactive ions released from silicate bioceramics for bone regeneration, Acta Biomater., 2018, 66, 81–92 CrossRef CAS PubMed.
- Z. Chen, L. Chen and R. Liu,
et al., The osteoimmunomodulatory property of a barrier collagen membrane and its manipulation via coating nanometer-sized bioactive glass to improve guided bone regeneration, Biomater. Sci., 2018, 6, 1007–1019 RSC.
- H. M. Yun, E. S. Lee and M. J. Kim,
et al., Magnetic Nanocomposite Scaffold-Induced Stimulation of Migration and Odontogenesis of Human Dental Pulp Cells through Integrin Signaling Pathways, PLoS One, 2015, 10, e0138614 CrossRef PubMed.
- H. Liang, C. Jin and L. Ma,
et al., Accelerated Bone Regeneration by Gold-Nanoparticle-Loaded Mesoporous Silica through Stimulating Immunomodulation, ACS Appl. Mater. Interfaces, 2019, 11, 41758–41769 CrossRef CAS PubMed.
- C. Yang, W. Wang and K. Zhu,
et al., Lithium chloride with immunomodulatory function for regulating titanium nanoparticle-stimulated inflammatory response and accelerating osteogenesis through suppression of MAPK signaling pathway, Int. J. Nanomed., 2019, 14, 7475–7488 CrossRef CAS PubMed.
- M. Shi, Z. Chen and S. Farnaghi,
et al., Copper-doped mesoporous silica nanospheres, a promising immunomodulatory agent for inducing osteogenesis, Acta Biomater., 2016, 30, 334–344 CrossRef CAS PubMed.
- M. Shi, L. Xia and Z. Chen,
et al., Europium-doped mesoporous silica nanosphere as an immune-modulating osteogenesis/angiogenesis agent, Biomaterials, 2017, 144, 176–187 CrossRef CAS PubMed.
- J. M. Sadowska, F. Wei and J. Guo,
et al., Effect of nano-structural properties
of biomimetic hydroxyapatite on osteoimmunomodulation, Biomaterials, 2018, 181, 318–332 CrossRef CAS PubMed.
- K. D. Patel, T. H. Kim and N. Mandakhbayar,
et al., Coating biopolymer nanofibers with carbon nanotubes accelerates tissue healing and bone regeneration through orchestrated cell- and tissue-regulatory responses, Acta Biomater., 2020, 108, 97–110 CrossRef CAS PubMed.
- Y. Chen, M. Guan and R. Ren,
et al., Improved Immunoregulation of Ultra-Low-Dose Silver Nanoparticle-Loaded TiO(2) Nanotubes via M2 Macrophage Polarization by Regulating GLUT1 and Autophagy, Int. J. Nanomed., 2020, 15, 2011–2026 CrossRef CAS PubMed.
- X. Qiao, J. Yang and Y. Shang,
et al., Magnesium-doped Nanostructured Titanium Surface Modulates Macrophage-mediated Inflammatory Response for Ameliorative Osseointegration, Int. J. Nanomed., 2020, 15, 7185–7198 CrossRef CAS PubMed.
- Q. Chen, J. Chen and Z. Yang,
et al., Nanoparticle-Enhanced Radiotherapy to Trigger Robust Cancer Immunotherapy, Adv. Mater., 2019, 31, e1802228 CrossRef PubMed.
- M. Zou, J. Sun and Z. Xiang, Induction of M2-Type Macrophage Differentiation for Bone Defect Repair via an Interpenetration Network Hydrogel with a GO-Based Controlled Release System, Adv. Healthcare Mater., 2021, 10, e2001502 CrossRef PubMed.
- R. Feng, F. Yu and J. Xu,
et al., Knowledge gaps in immune response and immunotherapy involving nanomaterials: Databases and artificial intelligence for material design, Biomaterials, 2021, 266, 120469 CrossRef CAS PubMed.
- J. Bourquin, A. Milosevic and D. Hauser,
et al., Biodistribution, Clearance, and Long-Term Fate of Clinically Relevant Nanomaterials, Adv. Mater., 2018, 30, e1704307 CrossRef PubMed.
- Y. Shi and T. Lammers, Combining Nanomedicine and Immunotherapy, Acc. Chem. Res., 2019, 52, 1543–1554 CrossRef CAS PubMed.
- O. Adir, M. Poley and G. Chen,
et al., Integrating Artificial Intelligence and Nanotechnology for Precision Cancer Medicine, Adv. Mater., 2020, 32, e1901989 CrossRef PubMed.
- A. V. Singh, M. H. D. Ansari and D. Rosenkranz,
et al., Artificial Intelligence and Machine Learning in Computational Nanotoxicology: Unlocking and Empowering Nanomedicine, Adv. Healthcare Mater., 2020, 9, e1901862 CrossRef PubMed.
|
This journal is © The Royal Society of Chemistry 2022 |
Click here to see how this site uses Cookies. View our privacy policy here.