DOI:
10.1039/D2NA00426G
(Review Article)
Nanoscale Adv., 2022,
4, 4972-4996
Research progress on near-infrared long persistent phosphor materials in biomedical applications
Received
30th June 2022
, Accepted 22nd October 2022
First published on 24th October 2022
Abstract
After excitation is stopped, long persistent phosphor materials (LPPs) can emit light for a long time. The most important feature is that it allows the separation of excitation and emission in time. Therefore, it plays a vital role in various fields such as data storage, information technology, and biomedicine. Owing to the unique mechanism of storage and luminescence, LPPs can avoid the interference of sample autofluorescence, as well as show strong tissue penetration ability, good afterglow performance, and rich spectral information in the near-infrared (NIR) region, which provides a broad prospect for the application of NIR LPPs in the field of biomedicine. In recent years, the development and applications in biomedical fields have been advanced significantly, such as biological imaging, sensing detection, and surgical guidance. In this review, we focus on the synthesis methods and luminescence mechanisms of different types of NIR LPPs, as well as their applications in bioimaging, biosensing detection, and cancer treatment in the field of biomedicine. Finally, future prospects and challenges of NIR LPPs in biomedical applications are also discussed.
1. Introduction
After the excitation using special light waves (UV, visible light, X-ray, etc., process ①), the excitation source energy stored in crystal defects is gradually released from LPPs in the form of photons (process ②), which can emit light for minutes, hours, or even longer (Fig. 1).1–4 In China, the phenomenon of long afterglow can be traced back to the Song Dynasty, which has a history of thousands of years.5,6 In Western countries, the first mention of such luminescent materials was in the early 17th century. When smelting gold from ore, an Italian shoemaker discovered a material that was glowing red at night. Following further investigations, the luminescence was found to be due to the presence of barium sulfide in the ore. After roasting, it was revealed that the ore could be largely transformed into barium sulfide, which is a long-lasting luminous substance. However, the afterglow performance of LPPs is currently weak, which limits the development of applications.5 At the end of the twentieth century, Matsuzawa reported SrAl2O4:Eu2+, Dy3+ phosphors capable of producing quite bright and long-term phosphorescence, which piqued the interest of researchers.7 Thereafter, researchers began to investigate LPPs in terms of luminescence mechanism and preparation methods, eventually applying such materials to the fields of safety instructions and luminous coatings.8 In 2007, Chermont et al.9 used the composite Ca0.2Zn0.9Mg0.9Si2O6:Eu2+, Dy3+, Mn2+ for NIR biomarker imaging and diagnosis in vivo, establishing the research precedent for LPPs in the field of biomedicine. NIR long-afterglow luminescence technology was quickly brought to the forefront of the attention of the scientific community through this pioneering work.
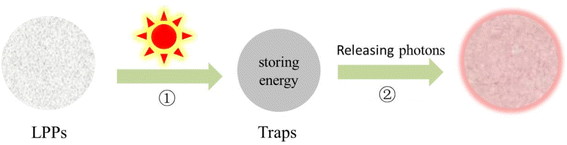 |
| Fig. 1 Schematic diagram of LPPs luminescence. | |
For the biomedical field, NIR LPPs are ideal replacements for traditional fluorescent materials. The reasons are as follows. Firstly, it allows the separation of excitation and emission in time, which completely avoids the background interference of tissue autofluorescence and scattered light. Secondly, it has high sensitivity and signal-to-noise ratio.10,11 Finally, the NIR light region is the emission range of LPPs that has less absorption and scattering, allowing strong penetration into biological tissues and opening up a new field for biomedicine.12 NIR light was discovered as the first invisible light. The American Society for Testing and Materials (ASTM) stipulates that its wavelength range is between 700 nm and 2500 nm. According to different wavelengths, the NIR light region is divided into region I (NIR-I, 700–1100 nm) and region II/III (NIR-II/III, 1100–2500 nm).9,10 The majority of the reported NIR LPPs are in the NIR-I region. For example, Cr3+ is a satisfactory wide emission center with an emission range of 650 nm–1000 nm. In addition, Mn4+, Mn2+, divalent rare earth ions Eu2+, Yb2+, tetravalent rare earth ions Pr4+, Ce4+, Tb4+, and other-doped LPPs are excellent emission centers for synthesizing NIR-I LPPs. However, only a few NIR-II/III LPPs with deeper tissue penetration and imaging contrast have been found. Some nanosized fluoride hosts (NaYF4/NaGdF4/NaLuF4) have been reported to have long afterglow emission in the NIR-II.13–15 For example, Zhuang et al.13 reported monodisperse nanoscale NaYF4:Ln3+ with narrow-band emission tunable from 480 nm to 1060 nm. The mechanism of persistent luminescence in NaYF4:Ln3+ has been proposed through thermoluminescence measurements and a host reference binding energy (HRBE) protocol. A new 3D optical information storage application is realized, proving its application potential in anti-counterfeiting, biomedicine, and other fields. There are also some bulk hosts such as lead sulfide (PbS) quantum dots (QDs),16 CsPbX3 (X = Cl, Br, I),17 Ba[Mg2Al2N4]:Eu2+, Tm3+,18 Y3Al2Ga3O12:Er3+, Cr3+,19 which also have NIR-II long afterglow luminescence properties. Nonetheless, NIR-II has fewer types and narrower application fields than NIR-I materials, requiring more attention. These NIR LPPs are available in data storage,20 information technology,21 biomedicine,22 and other fields. Although many excellent reviews have been reported to comprehensively summarize the synthesis, luminescence mechanism, and biomedical applications of LPPs, there are few related reviews of NIR LPPs.23–26 Therefore, this review provides a comprehensive summary of the luminescence mechanism and synthesis methods of different types of NIR LPPs, as well as their applications in the biomedical field (Fig. 2). In addition, this paper discusses future directions and challenges.
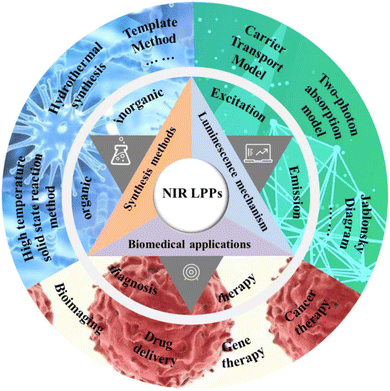 |
| Fig. 2 Summary of the biomedical applications of NIR LPPs. | |
2. Luminescence mechanism
LPPs are a class of thermoluminescent materials that emit light at room temperature, which belongs to the electron capture material.27 The luminescence phenomenon in the electron-trapping materials is formed by the trap-level structure in the materials, such as some inorganic metal oxides, organic composites, inorganic-organic hybrids, and other LPPs. However, owing to the limited availability of analytical techniques and the complexity of the energy-level structure, there is no clear unified mechanistic model for the luminescence mechanism of this material. The currently discovered mechanisms of luminescence include the carrier transport model,28–30 tunneling model,31 two-photon absorption model,32 Vk center model,33 and displacement coordinate model.34,35 At the same time, there is a principle called the Jablonsky diagram, which is used to explain the organic long afterglow luminescence.36,37 Most of these luminescence mechanisms use existing band theory and other theoretical knowledge to explain the phenomena found in experiments. The following discussion mainly introduces several typical luminescence mechanism models and the main NIR LPPs.
2.1 Carrier transport model
In 1971, Abbtuscato V. et al.38 proposed that the delayed luminescence of SrAl2O4:Eu2+ was caused by electron transport. It was not until 1996 that Matsuzawa et al.7 proposed the hole transfer model, which is the first model used to explain the luminescence of SrAl2O4:Eu2+, Dy3+. Based on this study, Kyota Uheda et al.39 proposed that the delayed luminescence mechanism of ZnGa2O4:Mn2+ is related to electron transfer and holes. The luminescence mechanism models proposed by these researchers were all about the energy transfer caused by electrons or holes. Since both electrons and holes belong to the charged particles that can move, it is recognized as the carrier transport model.29–31
Qu et al. investigated the red LPP of Y2O2S:Eu, Ti, Mg using the carrier transport model, and its luminescence mechanism model is shown in Fig. 3a. When the luminescence center of Eu2+ is excited at 382 nm, the spin-level transition occurs inside the ion, and the electron transitions to the conduction band to form an electron flow (process ①). A portion of the electron flow is transformed into the excited state of Eu2+ and then released back to the ground state in the form of photons, resulting in yellow fluorescence (process ②). Another part of the electron flow was captured by the Ti4+ electron trap. When enough energy is captured, a thermal escape phenomenon will occur. The captured electrons will combine with the luminescent center of Eu2+, resulting in the red afterglow phenomenon (process ③).28
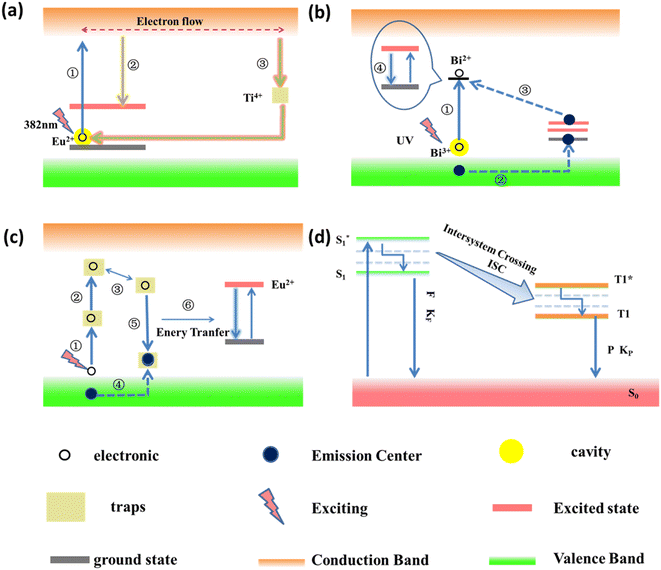 |
| Fig. 3 Diagram of the luminescence mechanism of various materials: (a) carrier model. Reproduced from ref. 28 with permission from the Royal Society of Chemistry, copyright 2019. (b) Tunneling model. Reproduced from ref. 40 with permission from the Royal Society of Chemistry, copyright 2017. (c) Two-photon absorption model. Reproduced from ref. 32 with permission from Elsevier, copyright 2001 (d) Jablonsky diagram. Reproduced from ref. 36 with the springer nature, copyright 2019, and from ref. 37 with the springer nature, copyright 2019 (F: fluorescence. P: phosphorescence). | |
2.2 Tunneling model
In 1958, Hougeustraten et al.41 first discovered the tunneling model when studying sulfides, which was first used to explain the luminescence mechanism of Eu2+, Dy2+ co-doped long afterglow materials. In 1981, Chang et al.42 observed that there was no photoconductivity in the afterglow phenomenon of Zn2SiO4:Mn3+ and BaFBr:Eu2+ at low temperatures, which further improved the light-emitting mechanism of the “tunneling” model. The tunneling model is separated into the full-tunneling model and the half-tunneling model. It has been discovered by researchers as a fundamental model of pyroelectric light emission. The luminescence mechanism of KGaGeO4:Bi3+ was investigated using a tunneling model by Sun et al.40 A schematic diagram of its tunneling mechanism model is shown in Fig. 3b. The electron in the emitting center of Bi3+ is excited by UV (process ①), and Bi2+ is formed. At the same time, holes are generated (process ②). The holes recombine with Bi2+ through a tunneling process (process ③). The released energy is obtained by the Bi3+ emitting centers, which leap from the ground state to the excited state through the thermal escape phenomenon. Due to the non-radiative leap and specific energy-level leap, the cyan-to-blue afterglow emission phenomenon of Bi3+ arises (process ④).
2.3 Two-photon absorption model
The two-photon absorption model is a relatively new mechanistic model, which was proposed by Aitasalo et al.32 when they were studying Eu2+ and Dy3+ or Nd3+ co-doped aluminate systems in 2001. Fig. 3c shows a schematic diagram of the luminescence mechanism model of CaAl2O4:Eu2+. Electrons in the valence band are excited to deep traps (process ①). When the energy is absorbed, they are excited to a shallow trap (process ②). Due to thermal disturbance, the electrons enter another trap (process ③). The holes in the valence band will enter the hole trap (process ④). The oxygen vacancies in the matrix are electron traps. Alkaline earth metal ion vacancies are hole traps. Electron traps capture electrons, and hole traps capture holes to form “electron–hole” pairs, which will undergo non-radiative recombination (process ⑤). Then, the energy is transferred to the Eu2+ luminescent center, resulting in a long afterglow phenomenon (process ⑥).43–45
2.4 Luminescence mechanism of organic LPPs
At present, the process of organic long afterglow luminescence is still unclear. The researchers turned to study the phenomena of luminescence in greater depth. A plausible mechanism for proposing organic-extended afterglow luminescence is based on experimental verification and related theoretical calculations. That is, the Jablonski energy-level diagram is used to explain the luminescence mechanism of organic LPPs, as shown in Fig. 3d. When the organic molecule is excited by the external field force, it transitions from the ground state S0 to the lowest excited state S1. Molecules in the S1 state are transformed into the triple excited state T1 through the channeling between the systems. Molecules in the T1 state are aggregated and captured by the H-aggregation, forming a stable triple-excited state.
produces long afterglow phenomena at very low radiative and nonradiative decay rates.36,37
2.5 Luminescence mechanism of inorganic-organic hybrid LPPs
In addition to inorganic and organic materials, researchers have hybridized the two to fully exploit the benefits of both, ushering in a new era in the field of long afterglow luminescence. As an inorganic-organic hybrid material, MOFs have the rigid porous structure of inorganic materials, and this structure can trap and stabilize the emitting molecules. Therefore, the coordination of organic fluorescent units and common metals is an effective way for MOF materials to achieve long afterglow luminescence.46 The porous structure of MOFs contains a large number of guest molecules in their pores. There are also abundant charge-transfer processes occurring inside, which provide the possibility for a variety of luminescence pathways. The luminescence mechanism of MOF materials is illustrated in Fig. 4a.47–50
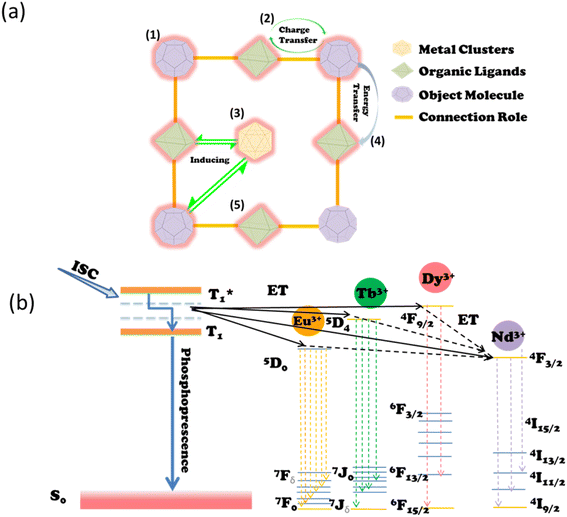 |
| Fig. 4 (a) Multiple luminescence pathways of MOFs, including (1) metal-centered luminescence (2) metal and organic ligand-based charge transfer luminescence (3) guest-induced luminescence (4) metal and organic ligand-based transfer luminescence and (5) organic ligand-based. Reproduced from ref. 58 with permission from China national knowledge internet, copyright 2021. (b) Schematic diagram of the energy conversion between metals in LnMOFs (ISC, Intersystem Crossing; ET, Energy Transfer). Reproduced from ref. 57 with permission from the Royal Society of Chemistry, copyright 2019. | |
Process ① is based on metal center luminescence. The luminescence mechanism mainly exists in two kinds of metal ions. One is relatively rare with d–d electron transfer of transition metal ions and s–p electron transfer of the main group of metal ions. The other is the most important lanthanide rare earth and actinide ions luminescence. It is recognized as the process of the luminescence “antenna effect”. This effect usually selects for the introduction of organic molecules with chromophoric groups and chelation of rare earth ions. The process of sensitizing rare earth ions is through the energy absorbed by organic molecules, thereby emitting light.51,52 Process ③ is guest-induced luminescence. It is mainly to endow MOFs with new luminescence ability by utilizing the permanent regular pores or pore properties of the material. We usually exchange or replace some chromophore molecules, rare earth ions, quantum dots, and other materials with strong luminescent properties into these pores. Such a luminescence mechanism conferred by the exchange of the guest is called guest-induced luminescence.53–55 Process ⑤ is the luminescence process of organic ligands, which is the same as the luminescence process of organic long afterglow luminescent materials. The difference is that the stability and rigidity of the organic ligands increase. Processes ② and ④ are based on metal-to-organic ligand luminescence and metal-to-metal energy transfer luminescence processes. The former is based on charge transfer luminescence. It has two typical luminescence modes: charge transfer luminescence from metal to organic ligand (MLCT) and charge transfer luminescence from organic ligand to metal (LMCT).56 The latter is based on energy transfer between metals to emit light. It is not only limited to single-metal MOF materials but has gradually expanded to the study of MOF materials containing multiple metals, especially heterometallic organic framework materials (HMOFs) and multi-metal hybrid MOF materials. The primary distinction between these two materials is that the different metal ions in HMOF have different coordination environments and coordination modes, and their crystal structures change. In contrast, mixed metal ions in multi-metal hybrid materials, typically, only, partially replace the original metal ions, while the coordination environment and coordination mode remain unchanged, resulting in crystal structures that are frequently identical to those of pure metal materials. Therefore, researchers agree that the multi-metal mixed MOF materials are more conducive to the study of the energy transfer luminescence mechanism between the metal and the metal. Zeng et al.57 designed novel bimetallic lanthanide metal–organic framework materials (Ln-MOFs) with luminescent colors systematically investigated by energy transfer from visible light-emitting M3+ to NIR emitting Nd3+-doped in M1−xNdxL2 for color tuning. Color tuning was investigated by doping M1−xNdxL2 with Nd3+ emitted in the NIR. The doping of Eu3+, Tb3+, or Dy3+ MOFs to form NIR and visible luminescence bimetallic Ln-MOFs opens up a new direction, the applied NIR spectral emission of bimetallic Ln-MOFs provides convenient applications in the field of future optical imaging. A schematic diagram of the metal-to-metal energy transfer luminescence of the Ln-MOFs is shown in Fig. 4b.
2.6 Main NIR LPPs
It is well known that the afterglow characteristics of LPPs mainly depend on the trap center and the emission center. The former is used to store energy from excitation light sources, the latter is used to emit energy after excitation has ceased.59 Obviously, it is pretty significant to consider suitable NIR luminescence centers and traps for designing and synthesizing suitable NIR LPPs. The main matrix of LPPs contains sulfide, aluminate, silicate, gallate, germanate, titanate, and so on. Doping ions are generally introduced as luminescent centers or co-activators to improve the afterglow properties of the host. These doping ions are either rare earth ions (Eu2+, Tb3+, Nd3+, Er3+, Pr3+, Ho3+, Sm3+, Tm3+, Yb2+, Yb3+, Dy3+, etc.) or transition metal ions (Cr3+, Mn4+, Mn2+, Ni2+, Co2+, etc). Only a few ions (Er3+, Yb3+, Nd3+, Pr3+, Cr3+, Mn4+, Mn2+, Ni2+, Co2+, etc.) have NIR emission properties. Cr3+, Mn4+, Mn2+, RE3+, Eu2+, Yb2+, Pr4+, Ce4+, Tb4+, etc. play an important role in the emission in the NIR region. Yb2+, Pr3+, Nd3+, Co2+, Ni2+, etc. have made great contributions to the design and synthesis of NIR-II/III LPPs.60–68 In addition, NIR emission in the second and third regions can also be achieved through a continuous energy transfer strategy between two or more different emission centers19,69,70 (as shown in Table 1).
Table 1 Comparison of the afterglow properties of common NIR PLNPs and their different applications in biomedical fields
NIR partition |
Different substrates |
Main material |
Dopant ions |
Excitation |
Emission |
color |
Afterglow time |
Biomedical applications |
References |
NIR-I |
Sulfide |
CaS |
Eu2+, Dy3+ |
254 nm |
550–800 nm |
Red |
18 min |
— |
63
|
ZnS |
Cu, Cl/Cu, Al |
Cathode ray |
420–620 nm |
Green |
— |
— |
71
|
Perovskite |
CsPbX3 (X = Cl, Br, I) |
— |
365 nm |
400–700 nm |
Green/red |
∼8 h |
— |
72
|
Aluminate |
Sr3Al2O6 |
Eu2+, Dy3+ |
473 nm |
550–700 nm |
Red |
>600 s |
— |
73
|
LaAlO3 |
Mn4+ |
254 nm |
680–750 nm |
Red |
∼10 min |
Imaging |
74
|
Cr3+ |
UV |
734 nm |
Red |
100 min |
Optical imaging |
76
|
SrAl2O4 |
Eu2+, Dy3+ |
— |
∼520 nm |
Green |
— |
Imaging, monitoring |
77
|
Gallate |
ZnGa2O4 |
— |
UV/LED |
700 nm |
Red |
∼2400 s |
In vivo optical bioimaging and drug-delivery |
78
|
Cr3+ |
250 nm |
695 nm |
Red |
∼60 min |
Imaging, background-free bioassay, detection of hemoglobin |
79–82
|
UV |
700 nm |
Red |
>80 min |
Optical imaging of vascularization, tumours and grafted cells |
83
|
X-ray |
600–750 nm |
Red |
>5 min |
Optical imaging |
84
|
Cr, Gd |
Red LED/UV |
600–900 nm |
Red |
∼1800 s |
Multimodal imaging |
85
|
Iron oxide |
254 nm |
650–800 nm |
Red |
∼1200 s |
Multimodal imaging |
86
|
Cr3+, Bi3+ |
254 nm |
600–700 nm |
Red |
∼40 min |
Bioenvironmental temperature sensing detection |
87
|
Y3Ga5O12 |
Cr3+, Si4+ |
655 nm |
650–950 nm |
Red |
10 min |
In vivo imaging |
88
|
Gd3Ga5O12 |
Cr3+, Nd3+ |
UV |
745 nm |
Red |
>3 h |
Multimodal imaging and cancer therapy |
89
|
Gd2.99Ce0.01Al1.995Cr0.005Ga3O12 |
Cr3+ |
800 nm |
650–900 nm |
Red |
10 min |
Multimodal imaging |
90
|
Germanate |
MgGeO3 |
Mn2+, Yb3+ |
254 nm |
300–750 nm |
Red |
∼1800 s |
— |
91
|
K4CaGe3O9 |
Mn2+, Yb3+ |
254 nm |
300–700 nm |
— |
5 h |
— |
92
|
Gallium germanate |
Zn1.1Ga1.8Ge0.1O4 |
Cr3+ |
UV |
690–750 nm |
Red |
10 min |
Bioenvironmental testing |
93
|
LED |
1 h |
Red LED |
695 nm |
Red |
2 h |
Drug delivery, chemotherapy |
95
|
Zn1.1Ga1.8Ge0.1O4 |
Cr3+, Eu3+ |
696 nm |
700–800 nm |
Red |
50 min |
Drug delivery and tumor targeted imaging |
96
|
254 nm |
675–750 nm |
Red |
3 h |
In vivo chemotherapy |
97
|
Zn1.25Ga1.5Ge0.25O4 |
Cr3+, Yb3+, Er3+ |
254 nm |
660–780 nm |
Red |
50 min |
Drug delivery |
98
|
Titanate |
CaZnTiO3 |
Pr3+ |
314 nm |
600–650 nm |
Red |
>2.5 h |
Photothermal therapy, biomonitoring |
99
|
Na0.5Gd0.5TiO3 |
Cr3+ |
650 nm |
760 nm |
Red |
∼60 min |
Low-irradiance imaging |
100
|
Nitride |
Ba[Mg2Al2N4] |
Eu2+, Tm3+ |
660 nm |
600–900 nm |
Red |
∼1000 s |
— |
18
|
Stannate |
SrSnO3 |
Bi2+ |
808 nm |
700–900 nm |
Red |
>30 min |
In vivo bioimaging |
101
|
Sr2SnO4 |
Tm3+ |
254 nm |
440–850 nm |
Red |
>30 min |
— |
64
|
Other |
F-L-Yb |
— |
980 nm |
900–1100 nm |
Red |
500 μs |
Time-resolved imaging |
102
|
Conjugated polymers MEH-PPV |
— |
465 nm |
780 nm |
Red |
∼1 h |
Tracking and molecular optics imaging |
103
|
Semiconductor polymers SPNs |
— |
Biothio-activation |
780 nm |
Red |
∼30 min |
Detection of drug-induced hepatotoxicity |
104
|
Luminous nanodots AGL AIE dots |
— |
465 nm |
600–650 nm |
Red |
250 s |
Surgical imaging navigation |
105
|
Bioluminescent probes FD-1029 |
— |
560 nm |
1029 nm |
Red |
300 min |
NIR-II imaging |
106
|
Nanocomposites LPLNP-PPT/TRAIL |
— |
254 nm |
694 nm |
Red |
∼2 h |
Mesenchymal stem cell therapy engineering (MSC) treatment and follow-up |
107
|
NIR-II/III |
Fluoride |
NaYF4/NaGdF4/NaLuF4 |
Ln3+/Tb2+ |
X-ray |
480–1060 nm |
Green/red |
>1800 s |
Bioimaging |
13–15
|
Titanate |
CaTiO3 |
Yb3+, Bi3+ |
365 nm |
900–110 0 nm |
Red |
>80 h |
— |
67
|
Gallium germanate |
Zn3Ga2Ge2O10 |
Ni2+ |
300 nm |
900–1700 nm |
Red |
>2 h |
— |
65
|
Stannate |
Zn2Ga2Sn0.5O6 |
Yb3+, Ni2+ |
254 nm |
1100–1600 nm |
Red |
>5 min |
— |
66
|
Gallate |
Y3Al2Ga3O12 |
Cr3+, Er3+ |
254 nm |
690 nm, 1532 nm |
Red |
∼1000 min |
— |
19
|
Nd3+, Ce3+, Cr3+ |
460 nm |
850–1200 nm |
Red |
>10 h |
— |
69
|
ZnGa2O4 |
Co2+, Ni2+ |
— |
900–1700 nm |
Red |
>30 min |
— |
68
|
Zn1+ySnyGa2−x−2yO4 |
Ni2+ |
UV |
1270–1430 nm |
Red |
∼300 s |
With potential for optical imaging |
108
|
Zn0.6Ca0.4Ga2O4 |
Cr3+, Yb3+ |
254 nm |
950–1100 nm |
Red |
>10 min |
With potential for optical imaging |
109
|
Aluminates |
LaAlO3 |
Cr3+/Er3+ |
UV |
734 nm, 1553 nm |
Red |
∼60 min |
With potential for optical imaging in vivo |
60
|
Phosphate |
Ba3(PO4)2 |
Mn5+ |
200–900 nm |
1100–1300 nm |
Red |
550 s |
In vivo NIR-II fluorescence imaging |
110
|
3. Synthesis methods
3.1 Synthesis methods of inorganic and inorganic-organic hybrid LPPs
Synthesis methods have a significant impact on the micromorphology, afterglow properties, luminous efficiency, and defects of long afterglow materials, which are all closely related to their applications.111 As researchers continue to explore and study their synthesis methods. Different types of synthesis methods have been discovered and reported. The high-temperature solid-state reaction method is the most ancient, mature, and widely used. The raw materials mixed by the ball mill were heated under certain temperatures and conditions.112,113 The use of co-solvents such as P2O5 and B2O3 was discovered to lower the sintering temperature and improve the material's afterglow qualities.114 Although the above synthesis method is simple and low-cost, it requires long-time high-temperature sintering and the prepared product is damaged by ball milling, which reduces the afterglow performance of the material. In 1990, Indian researchers used the combustion method to prepare LPPs to solve the drawbacks of the high-temperature solid phase reaction method, such as large particles and damaged crystal shape.115 The method utilizes the heat released by the combustion reaction of the mixed raw materials to promote the spontaneous and continuous chemical reaction without a complicated heating device. In short, it is a promising synthesis method. Both Zhang116 and Ni et al.117 have successfully manufactured various of LPPs using this technology in recent years.
During the synthesis of various LPPs, it was discovered that the afterglow properties and particle shape of the materials can be greatly improved by doping with different types and contents of rare earth or transition metals, or even by adding alcohol and other substances in the synthesis.118,119 However, the existing traditional synthesis methods have been unable to meet the requirements of high-doping content or controlled appearance of materials. Gradually, the sol-gel method, co-precipitation method, and template method were developed. These methods have proven to be effective in improving LPPs with desirable properties. Nevertheless, a drawback that should not be overlooked is that these methods do not provide direct access to LPPs and require a traditional calcination process. The advent of hydrothermal synthesis has made sudden progress in the field of preparation, which not only greatly reduces the temperature of the reaction, but also enables the synthesis of products in a single step. In recent years, Yang,120 Ankoji,121 Wang,122 and other teams have successfully prepared different LPPs with excellent afterglow properties using this method using high-temperature and high-pressure reactors. The synthesis conditions in this method are mild, and the particles are nanoscale and uniform. Of course, there are also disadvantages such as being limited to the oxidation system and reducing the brightness of the product. With the rapid development of modern science and technology, the synthesis methods of LPPs are also advancing, and many new methods are emerging, such as microwave method,123 flame spray pyrolysis,124,125 vapor deposition,126,127 electrostatic spinning,128,129 and ultrasonic synthesis.130,131Fig. 5 shows a brief flowchart of the different synthesis methods. In short, researchers are making progress toward better LPP synthesis. A comparison of different common synthesis methods of LPP in terms of preparation time, temperature, and morphological appearance is shown in Table 2.
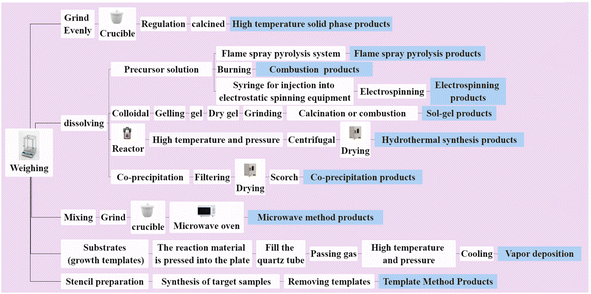 |
| Fig. 5 Several brief flow charts for the preparation of LPPs. | |
Table 2 Comparison of common preparation methods for LPPs in various aspects
Preparation method |
Preparation principle |
Preparation time |
Preparation temperature |
Advantages |
Disadvantages |
Grain size |
Ref. |
High temperature solid state reaction method |
Combustion between solid powders |
A few hours |
>1000 °C |
Simple process, convenient operation, low cost, high brightness, wide range of applications |
High temperature, long time, large particles |
Micron level |
74
|
Combustion method |
High temperature scorching |
A few minutes |
>1000 °C |
Shorter time and more energy efficient than high temperature solid phase reaction preparation |
A large amount of ammonia gas will be generated and pollute the environment. Lower brightness than the product synthesized by high temperature solid phase reaction method |
Nanoscale to micron scale |
133
|
Sol–gel method |
Sol-gel pathway and polymerizable complex pathway |
A few hours |
≤200 °C |
Good product uniformity, small particle size, strong luminosity |
An auxiliary method. High cost and long duration. More damaging to the organism |
Nanoscale to micron scale |
135–138
|
Hydrothermal synthesis method |
Closed environment high temperature and high pressure synthesis |
A few hours |
100–240 °C |
Mild synthesis conditions, stable system, uniform particle |
Low brightness, limited to oxide system |
Nanoscale to micron scale |
139–141
|
Co-precipitation method |
Sedimentation principle |
A few hours |
Room temperature |
Smaller particle size, higher purity, more uniform particles, and longer process compared to high-temperature solid phase reaction products |
An auxiliary method. Easy to generate other impurity mixture, temperature is not easy to control |
Nanoscale to micron scale |
142
|
Template method |
Use of extrinsic templates to control material appearance |
A few hours |
Room temperature |
Uniform particle size, easily controlled size and shape, mild conditions |
An auxiliary method. The stencil is more restrictive on the morphological pattern |
Nanoscale to micron scale |
109
|
Vapor deposition |
Chemical or physical changes occur between gases and coalesce after cooling |
A few minutes |
>1000 °C |
No polluting gas is released during the reaction, and the product is bright, which is a clean and energy-saving preparation method |
High cost |
Nanoscale to micron scale |
126
|
Microwave method |
Heating with microwave |
A few hours |
— |
Fast and efficient, energy-saving, simple operation, uniform particles, less pollution |
Not well suited for industrial production |
Nanoscale to micron scale |
123
|
Flame spray pyrolysis |
Using surface tension, electric field force, coulomb force, and other interactions |
Depending on the flow rate of oxygen in the generator, generally 5 L min−1 |
>1000 °C |
Fast and efficient, uniform particles, low strength, no pollution |
High cost and long time |
Micron level |
124
|
Electrostatic spinning |
Use of polymer electrostatic atomization form |
Depending on the speed of the electronic pump injection, generally 2 ml h−1 |
>1000 °C |
Uniform size, easy to control shape and appearance, high brightness, simple and easy to operate |
High cost, easy to cause environmental pollution |
Nanoscale to micron scale |
128
|
3.2 Design strategies for organic LPPs
Thus far, crystal induction,145 hydrogen bonding effect,146,147 host–guest doping,148 and other methods have been used to create a rigid environment, which effectively reduces the non-radiative leap rate. The high nuclear charge of halogen atoms with heavy atomic effect149,150 effectively enhances the inter-system scramble of molecules in the S1 state to form a stable triple excited state, resulting in the organic long afterglow phenomenon. The difficulty in obtaining pure organic LPPs can be attributed to two major factors. Firstly, the spin coupling between the lowest excited singlet state (S1) and the lowest excited triplet state (T1) is very weak in pure organic material molecules. The second reason is that the non-radiative leap rate is fast enough to allow the absorbed energy to be radiated as heat or other forms of energy.151 Therefore, researchers have focussed on developing organic LPPs with an excellent performance by increasing the probability of intersystem crossing, stabilizing triplet excited states, or reducing nonradiative transition rates. From both molecular and material design perspectives, organic ultra-long phosphorescence emission at room temperature has been achieved in a series of material systems, including single-component crystals, multi-component crystals, carbon dots, and polymers.
In terms of molecules, organic LPPs can be created by incorporating aromatic carbonyl groups, heavy atoms, heavy atoms, heteroatoms containing lone pairs of electrons and paramagnetic molecular groups,152–155 deuterium substitution,156 host–guest doping,157,158 aggregation structures,159,160etc. for materials; they can be designed by rigid structure induction,161–163 polymer formation,164 resonance structures,165 and thermal activation.166 Most reported materials have been carefully designed and synthesized. Organic LPPs urgently should start from the existing afterglow materials and design the construction strategy of organic LPPs without any chemical modification. Table 3 shows the design strategies for organic LPPs.
Table 3 Design strategies for organic LPPs
Type |
Design strategy |
Reason |
Applications |
Ref. |
Molecular design |
Introduction of aromatic carbonyl groups, heavy atoms, heteroatoms containing lone pairs of electrons, and molecular groups with paramagnetic properties |
Promote n–π* jumps, enhance inter-system scramble processes, and increase the stability of triple excited states |
Application to small molecule crystals, organic ionic crystals, carbon dots, and other single-component series |
152–155
|
Deuterated |
Weakening the intramolecular vibrations and reducing the rate constant of the non-radiative decay of the triple excited state |
Organic molecules have a large number of hydrogen atoms present, and under certain conditions, they can all be deuterated |
156
|
Material design |
The role between the subject and object molecules |
Restriction of guest molecular motion and inhibition of non-radiative leaps |
Application to multi-component eutectic, host–guest doping, amorphous films, and other types |
157
|
Construction of effective aggregation structures (e.g. H aggregation structures, strong π–π stacking, ionic bonding, etc.) |
Inhibition of nonradiative leaps, stabilization of trilinear excitons, and slow release |
Application to organic ionomers, materials containing π-bonds, etc. that are prone to forming aggregate structures |
159
|
Rigid solid-phase crystalline structure induced |
Introduction of amine groups, carboxyl groups, carbonyl groups, etc. groups, carbonyl groups, etc., to improve molecular interactions |
Application to single crystal and eutectic systems |
161–163
|
Formation of polymers |
Stabilization of the triplet state in organic molecules to reduce the non-radiative deactivation of the triplet state (T1) to the ground state (S0) |
For example, single component room temperature phosphorescent polymers in solid films or nanoparticles |
164
|
Resonant structures |
More inter-system crossing channels, significantly enhanced the self-selected orbital coupling effect, facilitating the inter-system crossing |
Afterglow molecules based on the existence of resonance structures such as N–P O/N–P S |
165
|
Thermal activation process |
A special type of three-mode emission that can be produced by the radiative decay of S1, T1, and at room temperature |
In distorted donor–acceptor structure afterglow molecules |
166
|
4. NIR LPPs in biomedical applications
In biological applications, NIR LPPs can avoid autofluorescence of biological tissues without situ excitation, while they have excellent afterglow properties in the NIR region. NIR LPPs exhibit superior responsiveness and biological tissue penetration compared to standard LPPs, boosting detection signal-to-noise ratio and sensitivity while minimizing background noise.11 The afterglow properties of NIR LPPs are changeable under different substrates or even the same substrate under varied doping conditions, allowing for a wide range of applications. As shown in Table 1, we compared the afterglow properties and biomedical applications of different NIR LPPs. NIR LPPs commonly used in the biomedical field are nanoscale, which are called NIR-persistent luminescence nanoparticles (PLNPs). Thanks to ongoing research and functionalized modifications by researchers, NIR PLNPs have been used in a variety of fields, including biosensing detection, bioimaging, drug delivery, and cancer therapy.
4.1 Biosensing detection
NIR PLNPs can be used as bioprobes for monitoring and identifying changes in the biological environment in the field of sensing and monitoring biomarkers. Currently, researchers have developed a number of biological probes based on NIR PLNPs for monitoring various active chemicals in living organisms. These probes have high sensitivity, signal-to-noise ratio, and excellent detection performance.
4.1.1 Detection of proteins.
In 2012, Maldiney et al. used biotin-coated Eu, Dy, and Mn-doped silicate nanoparticles to detect avidin for the first time.167 It is a pioneer in protein detection using materials. At present, it has been used to detect various proteins such as hemoglobin (Hb), alpha-fetoprotein (AFP), and others.82,168 Hb plays a crucial role in the transport of oxygen atoms in mammals. It is also a typical biomarker for certain diseases, such as leukemia, heart disease, and anemia.169,170 Although many detection techniques have been developed, the detection of Hb in red blood cells still suffers from low sensitivity and poor specificity. Liu et al.82 synthesized a ZnGa2O4:Cr (ZGC) probe with water-soluble and NIR luminescence properties. It was found that Hb could neither shield the probe's excitation light nor absorb its emission, but could rapidly quench it. There is a partial overlap between the absorption spectrum of Hb and the emission spectrum of the probe, which is different from the common energy transfer mechanism. Therefore, it is classified as a typical collisional quenching process. The dynamic quenching mechanism gradually and significantly suppressed the persistent luminescence phenomenon with the addition of hemoglobin. The probe has a specific response to hemoglobin, laying the groundwork for hemoglobin detection. Fig. 6a shows a diagram of this probe for the ultra-sensitive detection of hemoglobin. It also possesses great selectivity and ultra-sensitivity to Hb due to its easy operation, and this technology has been effectively used in the clinical detection of human Hb content.
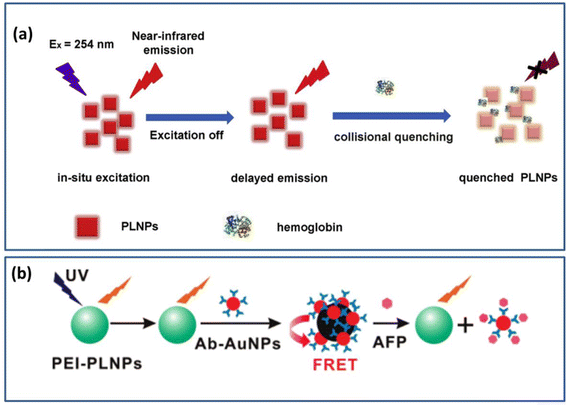 |
| Fig. 6 (a) Schematic diagram for ultra-sensitive detection of hemoglobin. Reproduced from ref. 82 with permission from Elsevier, copyright 2020. (b) Schematic diagram of these PLNPs for the determination of AFP content. Reproduced from ref. 168 with permission from the American Chemical Society, copyright 2011. | |
Serum levels of AFP can rise abnormally in conditions such as chronic hepatitis, cirrhosis, and liver cancer. The current clinical serum marker to determine primary liver cancer is AFP. Therefore, the measurement of serum AFP levels can be used as a diagnostic criterion for early liver disease.171,172 In 2011, an assay for the inhibition of AFP by fluorescence resonance energy transfer (FRET) was reported by Yan et al.168 They used polyethyleneimine (PEI) for the surface functionalization of Ca1.86Mg0.14ZnSi2O7:Eu2+, Dy3+ water-soluble PLNPs were first synthesized. At this time, the material will have a long afterglow in the NIR after being excited by the UV. After coating with gold nanomaterials, the long afterglow phenomenon will disappear. This work exploits the fact that AFP can disrupt the structure of PEI PLNPs and restore their afterglow properties to detect AFP in serum. A schematic diagram of the PLNPs for the determination of AFP content is shown in Fig. 6b. The detection method has also been successfully applied to the clinical detection of AFP content in human serum, which is of great significance for the early detection of liver cancer.
4.1.2 Detection of the biological environment.
NIR LPPs can be used not only for tracking and monitoring biological environments in vitro but in vivo. For example, tracking macrophages and visualizing inflammatory tissue. Significantly, NIR LPPs are functionally modified and typically coated with polyethylene glycols, folic acid, and other compounds prior to bioavailability to improve drug delivery and biosafety. It has been found that the NIR LPPs modified by surface functionalization can achieve low or even non-toxic effects, which is a very ideal material for biological probes.83,173 Sun et al.174 used a sol-gel method to create Zn1.1Ga1.8Ge 0.1O4:Cr3+ (ZGG) with low cytotoxicity and NIR-sustained luminescence properties, which they used to create a model for tracking the inflammatory tissue in mice in 2018. This study demonstrated a simple and novel method for visualizing inflammation in vivo with high sensitivity, paving the way for a new method for the early detection of 12 inflammation-related diseases. The following year, Chen et al.93 reported cell-penetrating peptide-functionalized LPPs (PLNP-TAT) as a luminescent marker for non-invasive tracking of J774A.1 macrophages in a mouse model of inflammation. Fig. 7 shows a schematic diagram of PLNP-TAT-labeled macrophages homing to inflamed tissues.
 |
| Fig. 7 Schematic representation of PLNP-TAT labeled macrophages homing to inflammatory tissues. Reproduced from ref. 93 with permission from the American Chemical Society, copyright 2019. | |
In addition, it is also useful in the construction of surgical guidance systems. Tissue autofluorescence can be efficiently prevented because ZGGO:Cr particles can sustain NIR luminescence after stimulation stops. Wang et al.94 demonstrated that the ZGGO:Cr particles created are suitable candidates for autofluorescence-free bioimaging. By building an aptamer-guided ZGGO:Cr bioprobe, it can be used for real-time monitoring of biological processes and the development of surgical guidance systems.
4.2 Bioimaging
The excellent chemical stability and photostability of NIR LPPs lay the foundation for their application in biomedicine. Bioimaging takes advantage of the continuous luminescence of the material after excitation with different wavelengths of light, such as X-rays, UV, and LED lights.15,84,93–95 Regarding biological imaging, various different techniques have been developed, such as optical imaging, up-conversion imaging, magnetic resonance imaging, time-resolved imaging, and other single-modality imaging techniques and multi-modality imaging techniques.
4.2.1 Optical imaging.
Optical imaging technology is a type of imaging that converts optical signals into electrical signals by exploiting the unique optical differences between the area to be imaged and the background (such as light scattering, absorption, luminescence, etc.).175 This technique not only allows for in vitro diagnosis, such as cellular or single-molecule diagnostics, but it also allows for image guidance during surgery, such as tumor excision and minimally invasive surgery in vivo. Utilizing BALB/carbon nude mouse molds, Lin et al.84 generated PLNPs [SiO2@ZnGa2O4:Cr3+@SiO2 (SZGOS)] using a simple silica template approach for in vivo NIR luminescence imaging. Model mice injected intravenously with a certain dose of SZGOS solution were found to have a high concentration of luminescent material in the liver area after a period of irradiation with soft X-rays. This phenomenon was used as a target area for the effect of SZGOS luminescence imaging in vivo. The main signal of persistent luminescence was detected in the liver region for more than 2 hours by observing the persistent luminescence images (Fig. 8a). Notably, upon re-irradiation with soft X-rays, the luminescence signal was fully activated again, indicating that the nanomaterial can be repeatedly activated in situ for long-term imaging in vivo (Fig. 8b). In the same year, Katayama et al.76 also developed LaAlO3:Cr3+, which can continuously emit light at 734 nm in the NIR-I after being activated by Cr3+. By observing the phenomenon of light transfer and the induced continuous emission, it is proved that this material can be used for repeated imaging in vivo.
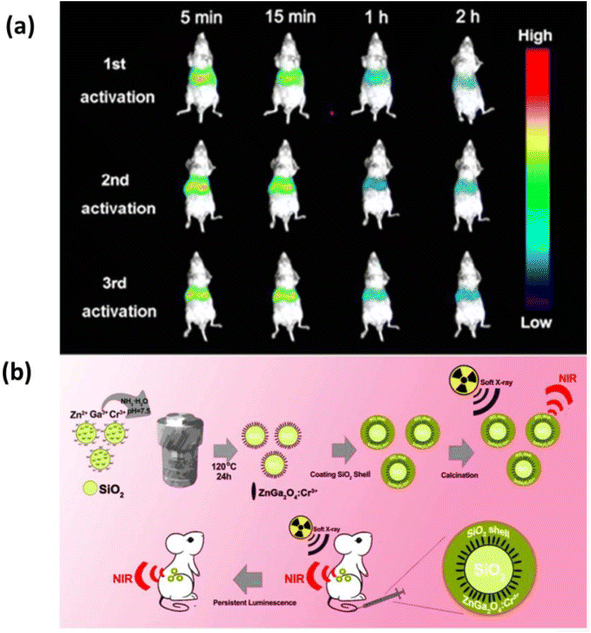 |
| Fig. 8 (a) Continuous luminescence imaging of soft X-ray activated BALB/carbon nude mice by intravenous injection of SZGOS solution. (b) Schematic diagram for the synthesis of SZGOS nanoparticles and their application in long-term in vivo bioimaging. Reproduced from ref. 84 with permission from the American Chemical Society, copyright 2017. | |
4.2.2 Fluorescence lifetime imaging.
Fluorescence lifetime imaging (FLIM) is the best in vivo time-resolved imaging technique available. Not only does it penetrate deep into the tissues in the NIR region of 650–1800 nm, but it also offers the unique advantage of quantitative visualization of molecular events in vivo. It is also independent of local luminescence intensity, fluorophore concentration, and biological tissue thickness. It is well known that the fluorescence lifetime and afterglow mechanisms are different. The fluorescence lifetime refers to the time for an electron to transition from the excited state back to the ground state. In contrast, afterglow refers to the process of capturing and releasing electrons. Due to the long lifetime of lanthanides, Ning et al.102 designed and synthesized a sensitive Yb3+ probe with deep permeability, good reversibility, and high spatial and temporal resolution, which is capable of in situ real-time dynamic monitoring pH changes, in vitro and in vivo (Fig. 9a shows the synthesis schematic for the design of a Yb3+ probe). The advantages of NIR emitting Ln for molecular imaging in vivo were also successfully demonstrated in a mouse model for monitoring pH in the gastrointestinal tract in vivo, as shown in Fig. 9b and c for the fluorescence imaging intensity and FLIM imaging.
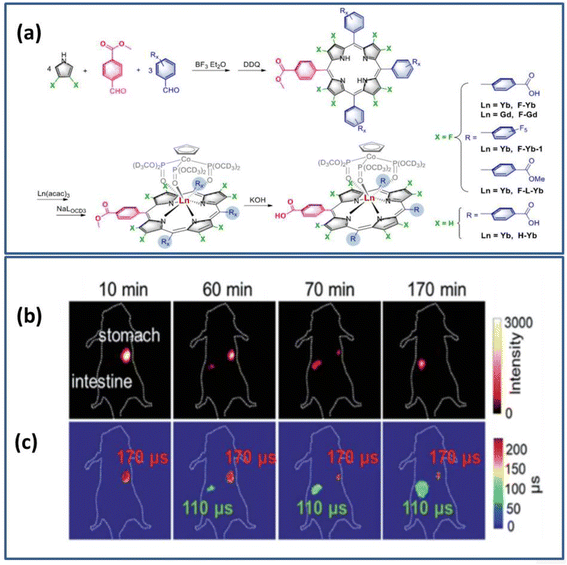 |
| Fig. 9 (a) Schematic diagram of the design and synthesis of the Yb3+ probe (b) NIR intensity fluorescence imaging (exposure time, 25 ms) (c) FLIM image (exposure time, 250 ms). λex = 532 nm; λem = 1000 nm long range. Reproduced from ref. 102 with permission from the Royal Society of Chemistry, copyright 2019. | |
4.2.3 Nuclear magnetic resonance imaging.
Based on different attenuation for the energy released in different structural environments within a substance, magnetic resonance imaging (MRI) is the imaging of electromagnetic waves emitted through the detection of an applied gradient magnetic field using the principle of nuclear magnetic resonance. This imaging technique is a promising technical imaging tool, which is multi-parametric and high-contrast imaging without damage.176,177 Wang et al.178 used hydrothermal synthesis to create NIR PLNPs (ZnGa2O4:Cr3+) and used these PLNPs to create a multimodal probe from hyaluronic acid functionalized Gd2O3 (HA-Gd2O3). As shown in Fig. 10a, the preparation of HA-Gd2O3-PLNPs probes is shown schematically. HA-Gd2O3 was used as an MRI contrast agent and PLNPs as a light source. In vivo imaging was successfully achieved in mice under the excitation of LED. The potential of multimodal probes for tumor-targeted NIR sustained luminescence and magnetic resonance imaging In vivo and in vitro was demonstrated.
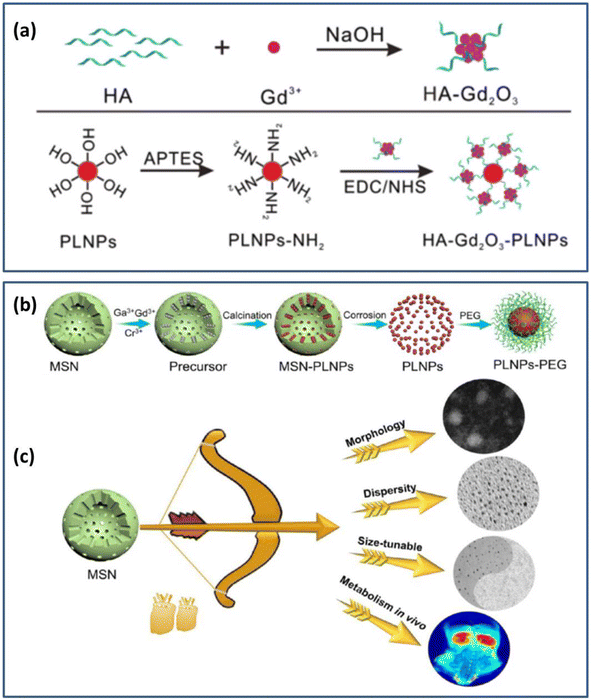 |
| Fig. 10 (a) Schematic diagram for the preparation of the HA-Gd2O3-PLNPs probe. Reproduced from ref. 178 with permission from the Royal Society of Chemistry, copyright 2017. (b) Synthesis and surface functionalization of MSNs. (c) Performance optimization of MSNs. Reproduced from ref. 179 with permission from the American Chemical Society, copyright 2021. | |
Wang and Li et al.179 synthesized PLNPs using mesoporous silica nanoparticles (MSNs) as a template. After excitation with 254 nm light for 15 min, it could exhibit long afterglow properties in the NIR range of 750–800 nm. Magnetic resonance dual-mode imaging was achieved by fine-tuning the morphology, size dispersion, and other properties of this material after coating it with polyethylene glycol. Fig. 10b and c are schematic diagrams of the synthesis and modification of the material, as well as the fine-tuning of the material properties. More notably, this work achieved renal clearance without toxicity to the organism, further promoting the application of the material in kidney-related diseases and cancer.
4.2.4 Multimodal imaging.
In addition to unimodal imaging applications, multimodal imaging techniques integrate two or more imaging modalities and can be used to diagnose diseases, mediate surgery, and so forth. Multimodal imaging can overcome the limitations of unimodal techniques and take advantage of each unimodal imaging technique to observe lesions with higher specificity, which provides a more powerful technical tool for treating diseases and curing the untreated. Abdukayum et al.180 achieved the first combination of NIR optical imaging and MRI by coupling gadolinium spray glucosamine (Gd-DTPA) with PLNPs. Sengar et al.90 firstly used a synthetic Gd2.99Ce0.01Al1.995Cr0.005Ga3O12 (GAGG:Ce, Cr) multimode probe for host cell imaging and cytotoxicity experiments. After the surface functional modification of folic acid, the probe has the potential of two-photon bioimaging in the NIR I region (650–900 nm) under the excitation of two-photon NIR (800 nm). In addition, the material has good paramagnetic properties, so it can be used for MRI. The researchers also performed toxicity tests on four different cell lines, demonstrating that the probe has not only good biocompatibility but also low toxicity, which achieved two-photon excitation imaging and NMR imaging. The following year, the group of Maldiney combined superparamagnetic iron oxide nanoparticles with chromium-doped zinc gallate, which was the first bimodal optical MRI nanoprobes (MPNHs) based on the development of magnetic persistent nanoparticles obtained from red light technology. The probe not only realizes real-time imaging with high spatial resolution in vivo but also laid the foundation for magnetic vectorization and cell therapy in vitro.86 Simultaneously, they attempted to use Gd ions instead of iron oxide nanoparticles to combine with chromium-doped zinc gallate. They also built a probe of the same type, which successfully realized real-time monitoring and imaging after the surface functional of polyethylene glycol. Under the surface functional modification of polyethylene glycol, the probe can significantly delay the uptake of the reticuloendothelial system in vivo when injected into healthy mice, providing novel nanotechnology for diagnosis in the biomedical field.85
In recent years, researchers have investigated and studied NIR LPPs after they have entered the human body through various routes. The goal is to improve imaging targeting and develop better administration routes, indicating their bright future for in vivo bioimaging. In 2016, Li et al.141 compared the targeted of synthetic monodisperse tridoped zinc gallate (ZGGO:Cr3+, Yb3+, Er3+) after surface functionalization with folic acid by using both gavage and caudal intravenous routes of administration. Fig. 11 shows the fabrication of the material, surface functionalization modification, and oral in vivo imaging, schematically. The targeting performance of orally folic acid functionalized ZGGO:Cr3+, Yb3+, Er3+ for tumor-targeted bioimaging is shown to be superior to that of traditional intravenous injection for the first time, indicating a good strategy for disease detection and drug delivery route selection.
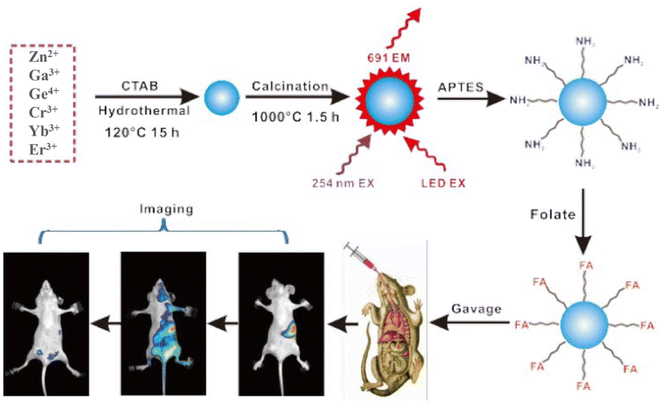 |
| Fig. 11 ZGGO:Cr3+, Yb3+, Er3+ synthesis and surface functionalization for oral in vivo bioimaging. Reproduced from ref. 141 with permission from the Royal Society of Chemistry, copyright 2016. | |
4.2.5 NIR-II/III bioimaging.
The longer the wavelength, the greater the penetration of biological tissue and the lesser the corresponding damage. The second and third regions of NIR light are gaining popularity. Shi et al.89 constructed a multifunctional nanoplatform from Cr3+, Nd3+ co-doped Gd3Ga5O12 synthesized using the template method, which exhibited excellent luminescence performance at 1067 nm under excitation at 808 nm. These particles also have a magnetic resonance effect due to the presence of Gd. Coupled with NIR-II luminescence properties, it exhibited multimodal imaging properties. Nie et al.108 successfully prepared Ni2+-doped Zn1+ySnyGa2−x−2yO4 nanomaterials. The emission peaks in the second emission window in the NIR range from 1270 to 1430 nm. During the tunability, high resolution and low light interference could be achieved, it provides greater application potential in the biomedical field. Er3+, Cr3+, and Sm3+-doped LaAlO3 nanomaterials reported by Xu et al.60 are promising biological probes with NIR-I (734 nm) and NIR-II (1553 nm) luminescence properties. In vivo optical imaging, especially in the NIR-III biological window, has great application potential.
4.3 Cancer treatment
There is currently no cure for cancer; it is one of the deadliest diseases in the world today. It is a new biomedical technology that organically combines diagnosis, monitoring, and treatment of the disease.181,182 LPPs have made significant contributions to the field of cancer diagnosis and treatment through their unique persistent luminescence properties. Researchers have combined drugs with LPPs to guide adjuvant-targeted cancer therapy and surgical guidance through persistent luminescence imaging.89,183 Up to now, there are different treatments such as drug delivery, chemotherapy, photothermal therapy, and photodynamic therapy.
4.3.1 Drug delivery.
Drug carrier refers to control of the distribution in the organism and the release rate of the drug by changing the route of administration, so as to deliver the drug to the target tissue or organ. It can deliver some defective drugs with high side effects or poor solubility into the body, thereby reducing damage to the human body and improving drug efficacy.184 For example, mesoporous silica and liposomes are commonly used drug-coating materials. In 2014, Maldiney et al.78 successfully constructed a core–shell structure optical imaging probe using mesoporous silica and chromium-doped zinc gallate, which was particularly used for the optical detection of living tissue and drug delivery. The probe successfully loaded the anticancer drug doxorubicin, which started the gradual release of the disease in a pH-sensing manner, showing the advantages of drug release localization and non-invasiveness.
In addition to conventional drugs as carriers in vitro, biofilm-camouflaged drug carriers usually have better biocompatibility. Liu et al.98 combined RBC membrane vesicles and PLNPs (ZGGO@mSiO2) into RBC bio-nanoprobes using bionanotechnology. Due to the long afterglow features of PLNPs in the NIR, adriamycin was employed on this probe, which can be used for long-term bioimaging and monitoring of mice in situ. This study demonstrates that medications are successfully transported to the tumor's growth site, resulting in tumor growth reduction. Various nanoplatforms for the application of bionanotechnology to the diagnosis and treatment of diseases have been proposed. Fig. 12 shows the preparation of erythrocyte membrane bio-inspired PLNP nanocarriers for imaging and drug delivery in vivo.
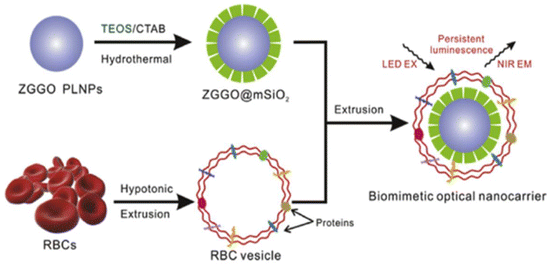 |
| Fig. 12 Schematic diagram of the preparation of erythrocyte membrane bioinspired PLNP nanocarriers for imaging and drug delivery in vivo. Reproduced from ref. 98 with permission from Elsevier, copyright 2018. | |
4.3.2 Chemotherapy.
Chemotherapy is currently the most commonly used cancer treatment in clinical practice. Although it has obvious therapeutic effects, it has disadvantages such as poor targeting, relatively large side effects, and large damage to normal cells of the human body.181 With good biocompatibility, biodegradability, and low toxicity, liposomes are a classical and widely used carrier, which can be well used for drug delivery and cancer therapy when intelligently combined with rare earth-doped NIR LPPs. In 2019, Zhang et al.97 used a hydrothermal synthesis method combined with further calcination to create a hydroxylated probe (Zn1.1Ga1.8Ge0.1O4:0.5%Cr3+, 0.5%Eu3+). The design synthesis strategy is depicted schematically in Fig. 13a. HepG2 tumor-bearing mice were subjected to in vivo chemotherapy after surface functional modification and drug-loading system (PHLIP-SS-GFLG-MSPLNPs). Fig. 13b is a schematic diagram of continuous tumor luminescence imaging and chemotherapy.
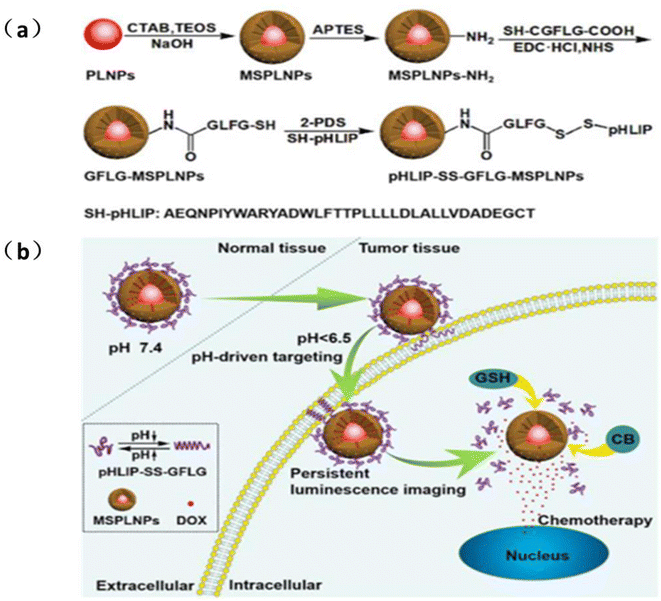 |
| Fig. 13 (a) Design strategy and synthetic route of pH-LIP-SS-GFLG-MSPLNPs (b) schematic diagram of pH-LIP-SS-GFLG-MSPLNPs@DOX as a pH-driven targeted nanoprobe for the sustained luminescence imaging of tumors and chemotherapy. Reproduced from ref. 97 with permission from the American Chemical Society, copyright 2020. | |
4.3.3 Photothermal therapy.
Photothermal therapy (PTT) is a treatment method for killing cancer cells by injecting materials with high photothermal conversion efficiency into the human body and then converting light energy into heat energy to gather around tumor tissues through targeted identification technology under the irradiation of external light sources. Despite growing interest, the method is still in its early stages and faces numerous challenges. Indocyanine green (ICG) for PTT guidance has great potential in cancer therapy. Zheng et al.99 first designed and prepared ICG and LPPs co-loaded mesoporous SiO2 nanocarriers [(ICG+PLPs)@mSiO2], which were successfully used for persistent luminescence imaging-guided PTT (Fig. 14). Chen et al.185 also developed a multi-chargeable mesoporous SiO2/ZnGa2O4:Cr3+(mZGC) NIR LPPs, which was successfully applied to photoluminescence imaging-guided PTT by loading ICG into ICG@mZGC.
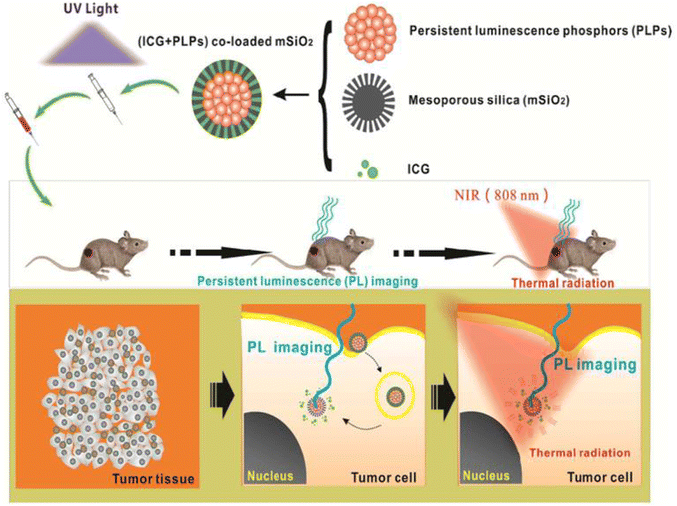 |
| Fig. 14 Continuous luminescence imaging-guided in vivo PTT application of (ICG+PLPs)@mSiO2 is depicted in this diagram. The schematic depicts the drug system's composition, which was injected into mice after UV excitation and guided in vivo PTT for cancer treatment using continuous luminescence imaging that tracked the drug's aggregation and diffusion in vivo. Reproduced from ref. 99 with permission from the American Chemical Society, copyright 2016. | |
4.3.4 Photodynamic therapy.
Photodynamic therapy (PDT), a newly emerged clinical cancer treatment method using photosensitizers, involves irradiating the tumor site with specific wavelengths to selectively activate the photosensitized drugs gathered in the tumor tissue to produce singlet oxygen (ROS) thereby killing cancer cells. This method requires long-time irradiation of visible light or ultraviolet light, which not only limits tissue penetration and penetration strength but also causes skin and tissue damage. However, the afterglow characteristics of NIR long-persistence luminescent materials can be used as the internal persistent light source of PDT, which can allow deep tissue treatment and avoid skin tissue damage.186,187 Fan et al.188 successfully constructed an injectable ZGC-persistent luminescence implant with stronger afterglow intensity and longer afterglow lifetime than ZnGa1.996O4:Cr0.004(ZGC) PLNPs, which can be repeatedly charged with LED for deep tissue afterglow imaging and PDT therapy greatly improving the therapeutic effect. Abdurahman et al.189 reported that the combination of a photosensitizer (Si-Pc) and an 808 nm NIR-persistent luminescent material can provide an internal light source to generate singlet oxygen for photodynamic therapy without continuous external light source irradiation. In 2018, Liu et al.190 composed ZnGa1.996O4:Cr0.004 (PLNPs) and photosensitizer IR780 into temperature-responsive “wax-seal” therapeutic nanoplatforms. When activated by thermal stimulation, PLNPs can not only serve as an internal light source to continuously excite photosensitizers and generate reactive oxygen species, but also provide high-resolution imaging of cancer (Fig. 15).
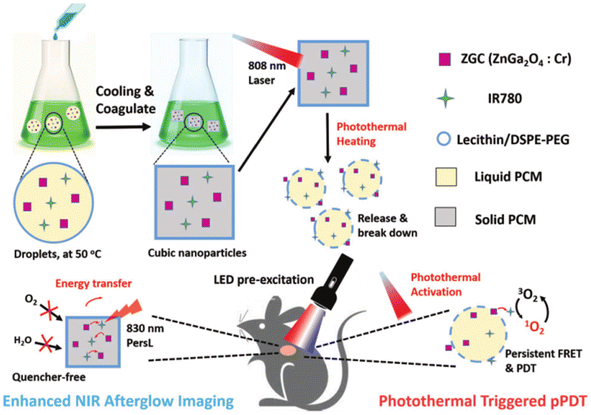 |
| Fig. 15 Design of the “wax-seal” design of the IR-ZGC nanoplatform. Reproduced from ref. 190 with permission from Wiley-VCH, copyright 2018. | |
4.3.5 Gene therapy.
Gene therapy is a new type of cancer treatment that introduces exogenous normal genes into the target cells to correct or compensate for diseases caused by defective and abnormal genes for therapeutic purposes. This method has high specificity and effectiveness and fewer side effects in post-surgical recovery.191–193 Wu et al.107 placed the therapeutic plasmid on polyetherimide (PEI) to modify PLNPs. A dual-functional persistent luminescent nanocomposite (LPLNP) was prepared, which was used for effective luminescence while tracking the homing and gene therapy of engineered mesenchymal stem cells (MSCs) glioblastoma (Fig. 16a and b). Qin et al.194 designed and synthesized a local therapeutic system consisting of gold nanorods (Au NRs) loaded with TERT siRNA on the surface of ZnGa2O4:Cr (ZGOC) nanofibers. It amplified gene phagocytosis, siRNA release in the cytoplasm of the host Au NRs, and silencing effects through a potential LED-induced mild photothermal effect.
 |
| Fig. 16 (a) Schematic diagram of a surface functional modification of LPLNP (b) Schematic diagram of cell tracking and gene therapy. Reproduced from ref. 107 with permission from Wiley-VCH, copyright 2017. | |
4.3.6 Combination therapy.
In recent years, a new type of treatment called combination therapy has been developed. Combination therapy is a treatment that combines two or more therapies to treat cancer, such as the combination of surgical treatment, chemotherapy, photothermal, and photodynamic therapy. A variety of different combination therapies have been reported. Zhao et al.195 reported acid-activated NIR PLNPs, which were endowed with tumor targeting and prolonged blood circulation under the surface functionalization of polyethylene glycol. Combined with the long-persistence properties of the material, the long-persistence imaging of tumors and the combined treatment of PTT/PDT are realized. Wu et al.183 designed a multifunctional persistent luminescent nanoplatform (PHF) with human serum albumin (HSA) combined with an IR780 probe and Fe3+ modified near-infrared emitting persistent luminescent nanoparticles (PLNP), which can be used for multimodal imaging of tumors and also enabling Fenton-like combined photodynamic and photothermal therapy that can effectively overcome harsh treatment conditions to kill tumor cells (Fig. 17), demonstrating that the multifunctional persistent luminescent nanoplatform has great potential for cancer therapy.
 |
| Fig. 17 Schematic of multimodality imaging and combination therapy. Reproduced from ref. 183 with permission from Wiley-VCH, copyright 2017. | |
5. Summary and outlook
In this review, we systematically summarize the luminescence mechanism, synthesis methods, and biomedical applications of NIR LPPs. As a promising alternative to traditional fluorescent materials, NIR LPPs have received widespread attention in the biomedical field because of their unique NIR afterglow characteristics. NIR LPPs can separate excitation and emission in time while avoiding background interference from fluorescence and scattered light. The most important one is that the emission range is in the NIR region. It has strong biological tissue penetration, high resolution and sensitivity, small absorption, and less scattering, which provides great convenience for its application in the biomedical field. Even though NIR LPPs have made significant growth in recent years, further work is required, including but not limited to:
(i) Synthesis method. At present, many synthesis methods have been reported, among which traditional synthesis methods include the high-temperature solid phase reaction method, combustion method, co-precipitation method, template method, and hydrothermal synthesis method. Based on the traditional synthesis method, electrostatic spinning, gas deposition, and electrospray pyrolysis are discovered, as well as a synthesis process that is helped by current technical methods such as microwave and ultrasound. Nevertheless, none of these synthesis methods is the best. It cannot simultaneously meet the requirements of morphological appearance, uniformity, and afterglow properties of NIR long afterglow materials, but only improves one or several of these properties. The most desirable synthesis method is that NIR LPPs with excellent morphology, homogeneity, afterglow properties, good stability, biocompatibility, recyclability, and low or even no toxicity can be synthesized by simple operations. At the same time, few or no pollutants are produced during the reaction. Therefore, the synthesis method needs further research.
(ii) Material Design. The design of materials includes two aspects. One is the design of the material itself, and the other is the design of the material after synthesis and before biological application. First of all, the design of the material itself refers to the design of the material synthesis. At present, the main thing is to choose some transition metal ions or rare earth ions as the luminescent center of the material when synthesizing the material, so as to achieve the purpose of improving the afterglow performance of the material. At the same time, the degradability of the material should also be considered, which greatly contributes to the biosafety of the material. In 2020, Lecuyer et al.196 demonstrated the degradability of ZGC in a lysosome-like medium following in vivo application in a simulated cellular environment, providing safety and credibility for the use of ZGC in the biomedical field. In addition, most of the currently reported NIR LPPs are focused on NIR-I. Fewer studies have been conducted in the NIR-II/III region, which has better tissue penetration and imaging capabilities, a strong investment in the research of NIR-II/III region luminescent materials is needed. The second is that after the material is synthesized, some materials with good biocompatibility are usually selected for functionalization and modification. This not only can improve the drug delivery and biocompatibility of the material but also can reduce the damage of the material itself to the organism. However, there are still a limited number of applications. Polyethylene glycol, folic acid, and other substances are available. Better, more functionalized modification materials are still in the works.
(iii) Excitation light. Excitation light is one of the most important factors for the afterglow properties of NIR LPPs, but most of the current applications involve UV excitation, and the afterglow time of the excited materials is not sufficient to support biological applications, requiring multiple repeated excitations. Therefore, future research on excitation light will focus on NIR light, radiation, and X-rays to better develop LPPs with NIR-II/III region luminescence.
(iv) Luminescence mechanism. Many different luminescence mechanisms have been reported, among which the most applied is the carrier model. No luminescence mechanism can be widely accepted by the general public yet. The luminescence properties of NIR LPPs are related to the type and depth of the traps present in the material and their ability to trap electrons. The study of the luminescence mechanism of the material also means the study of how the traps exist in the material and how the traps trap and release electrons during the luminescence process. Therefore, it is necessary to study how traps are involved in the luminescence process.
(v) Biological applications. There are three main applications of NIR LPPs in the biomedical field. They are biosensing detection, bioimaging, and cancer therapy. Among these, biosensing detection and cancer therapy are realized with the help of bioimaging technology. Breakthroughs in bioimaging technology have been achieved. With the guidance of bioimaging, the material and the drug combination system can now be applied to chemotherapy, PTT, PDT, gene therapy, and combination therapy. Despite the great progress in the research of NIR LPPs in the biomedical field, there is still a lack of experience in the research of these materials in clinical trials, mainly focusing on laboratory studies. Therefore, the biggest challenge of NIR LPPs in the biomedical field is the transition from laboratory research to clinical trials. Therefore, a bioapplication system for NIR LPPs should be constructed, so as to achieve efficient targeted drug transport, enable sufficient internalization of drugs in cells and tissues, promote the clinicalization of NIR LPPs, and make NIR LPPs better available for the biomedical field.
In conclusion, NIR LPPs are still in their initial stage of research. In the future development, NIR LPPs with better afterglow properties and application potential may emerge. We have to focus on the luminescence mechanism of this material and have a very in-depth luminescence mechanism in order to better build on the strengths and avoid the weaknesses, and design and synthesize NIR LPPs with better afterglow properties for better application potential in the biomedical field. We also believe that achieving this goal is very interesting and worthy of our expectations.
Conflicts of interest
There are no conflicts to declare.
Acknowledgements
We gratefully appreciate the financial support from the National Natural Science Foundation of China (No. 81903780), the Shandong Provincial Natural Science Foundation of China (No. ZR2020MB108), the Shandong University of Traditional Chinese Medicine Youth Science Fund (No. 2018zk24), the Health Commission of Shandong Province (No. 202004010938), Ji Nan Science & Technology Bureau (Grant No. 2020GXRC005), Jinan Education Bureau (JNSX2021041), the Funded by Shandong University of Traditional Chinese Medicine, Chinese Medicine Neuroimmunopharmacology Young Scientific Research Innovation Team (NO. 22202112). We would also like to thank the Experimental Center, Shandong University of Traditional Chinese Medicine, Jinan 250355, PR China.
References
- Y. Liu, J. Kuang, B. Lei and C. Shi, J. Mater. Chem. A, 2005, 15, 4025–4031 RSC.
- Z. Pan, Y. Y. Lu and F. Liu, Nat. Mater., 2011, 11, 58–63 CrossRef PubMed.
- Y. Wu, Y. Li, X. Qin and J. Qiu, Chem.–Asian J., 2016, 11, 2537–2541 CrossRef CAS PubMed.
- X. Chen, Y. Li, K. Huang, L. Huang, X. Tian, H. Dong, R. Kang, Y. Hu, J. Nie, J. Qiu and G. Han, Adv. Mater., 2021, 33, e2008722 CrossRef PubMed.
- H. Jorma, Electrochem. Soc. Interface, 2009, 18, 42–45 CrossRef.
- J. Xu, J. Ueda and S. Tanabe, J. Am. Ceram. Soc., 2017, 100, 4033–4044 CrossRef CAS.
- Y. A. Matsuzawa, N. Takeuchi and Y. Murayama, J. Electrochem. Soc., 1996, 143, 2670–2673 CrossRef.
- R. E. Rojas-Hernandez, F. Rubio-Marcos, M. A. Rodriguez and F. F. Jose, Renewable Sustainable Energy Rev., 2018, 81, 2759–2770 CrossRef CAS.
- C. Chanéac, Q. l. M. d. Chermont, J. Seguin, F. Pelle, S. Maîtrejean, J.-P. Jolivet, D. Gourier, M. Bessodes and D. Scherman, Proc. Natl. Acad. Sci., India, Sect. B, 2007, 104, 9266–9271 Search PubMed.
- N. Liu, J. Shi, Q. Wang, J. Guo, Z. Hou, X. Su, H. Zhang and X. Sun, Small, 2020, 16, e2001494 CrossRef PubMed.
- L. Liang, N. Chen, Y. Jia, Q. Ma, J. Wang, Q. Yuan and W. J. N. R. Tan, Nano Res., 2019, 12, 1279–1292 CrossRef CAS.
- Z. Xue, X. Li, Y. Li, M. Jiang, G. Ren, H. Liu, S. Zeng and J. Hao, Nanoscale, 2017, 9, 7276–7283 RSC.
- Y. Zhuang, D. Chen, W. Chen, W. Zhang, X. Su, R. Deng, Z. An, H. Chen and R. J. Xie, Light: Sci. Appl., 2021, 10, 132–142 CrossRef CAS PubMed.
- X. Ou, X. Qin, B. Huang, J. Zan, Q. Wu, Z. Hong, L. Xie, H. Bian, Z. Yi, X. Chen, Y. Wu, X. Song, J. Li, Q. Chen, H. Yang and X. Liu, Nature, 2021, 590, 410–415 CrossRef CAS PubMed.
- P. Pei, Y. Chen, C. Sun, Y. Fan, Y. Yang, X. Liu, L. Lu, M. Zhao, H. Zhang, D. Zhao, X. Liu and F. Zhang, Nat. Nanotechnol., 2021, 16, 1011–1018 CrossRef CAS PubMed.
- B. Hou, Y. Cho, B. S. Kim, D. Ahn, S. Lee, J. B. Park, Y. W. Lee, J. Hong, H. Im, S. M. Morris, J. I. Sohn, S. Cha and J. M. Kim, J. Mater. Chem. C, 2017, 5, 3692–3698 RSC.
- B. Paul, S. Maiti, T. Paul, B. Chatterjee, S. Maiti, S. Sarkar, N. Besra, B. Das, K. Panigrahi, S. Thakur, U. Ghorai and K. Chattopadhyay, J. Mater. Chem. C, 2018, 11374–11383 Search PubMed.
- J. Ueda, J. L. Leaño, C. Richard, K. Asami, S. Tanabe and R.-S. Liu, J. Mater. Chem. C, 2019, 7, 1705–1712 RSC.
- J. Xu, D. Murata, J. Ueda, B. Viana and S. Tanabe, Inorg. Chem., 2018, 57, 5194–5203 CrossRef CAS PubMed.
- J. Yu, M. Luo, Z. Lv, S. Huang, H. H. Hsu, C. C. Kuo, S. T. Han and Y. Zhou, Nanoscale, 2020, 12, 23391–23423 RSC.
- H. Tan, T. Wang, Y. Shao, C. Yu and L. Hu, Front. Chem., 2019, 7, 387 CrossRef CAS PubMed.
- J. Wang, Q. Ma, Y. Wang, H. Shen and Q. Yuan, Nanoscale, 2017, 9, 6204–6218 RSC.
- T. L. Jianhua Liu, J. Séguin, N. Mignet, D. Scherman, B. Viana and C. Richard, Adv. Drug Delivery Rev., 2019, 138, 193–210 CrossRef PubMed.
- B. Viana, S. Sharma, D. Gourier, T. Maldiney, E. Teston, D. Scherman and C. Richard, J. Lumin., 2016, 879–887 CrossRef CAS.
- L. Liang, N. Chen, Y. Jia, Q. Ma, J. Wang, Q. Yuan and W. Tan, Nano Res., 2019, 12, 1279–1292 CrossRef CAS.
- G. Su, R. Shen, J. Tan and Q. Yuan, Chem. J. Chin. Univ., 2020, 41, 2404–2414 CAS.
- J. M. Ortega and M. Roncel, Physiol. Plant., 2021, 171, 268–276 CrossRef CAS PubMed.
- B. Qu, J. Wang, K. Liu, R. Zhou and L. Wang, Phys. Chem. Chem. Phys., 2019, 21, 25118–25125 RSC.
- S. Jiang, M. J. F. Digonnet, M. Pellerin, C. Coelho-Diogo, C. Bonhomme, N. Touatib, L. Binet, D. Gourier, J. Ueda, S. Tanabe, B. Viana and C. Chanéac, Opt. Compon. Mater. XIV, 2017, 10100, 46–52 Search PubMed.
- N. Majewska, T. Lesniewski, S. Mahlik, M. Grinberg, A. Chruscinska, D. Michalik and M. Sopicka-Lizer, Phys. Chem. Chem. Phys., 2020, 22, 17152–17159 RSC.
- A. J. J. Bos, Materials, 2017, 10, 1357–1379 CrossRef PubMed.
- J. H. o. a. Tuomas Aitasalo, H. ogne Jungner, M. Lastusaari and J. Niittykoski, J. Lumin., 2001, 94, 59–63 CrossRef.
- A. Zhang, C. Huang, H. Shi, W. Guo, X. Zhang, H. Xiang, T. Jia, F. Miao and N. Jia, Sens. Actuators, B, 2017, 238, 24–31 CrossRef CAS.
- K. M. Jianrong Qiu a, H. Inouye, S. Fujiwara, T. Mitsuyu and K. Hirao, J. Non-Cryst. Solids, 1999, 244, 185–188 CrossRef.
- Q. S. Tianzhi Zhang and S. Wang, Chinese J. Lumin., 1999, 20, 170–175 Search PubMed.
- W. Zhao, T. S. Cheung, N. Jiang, W. Huang, J. W. Y. Lam, X. Zhang, Z. He and B. Z. Tang, Nat. Commun., 2019, 10, 1595 CrossRef PubMed.
- L. Gu, H. Shi, L. Bian, M. Gu, K. Ling, X. Wang, H. Ma, S. Cai, W. Ning, L. Fu, H. Wang, S. Wang, Y. Gao, W. Yao, F. Huo, Y. Tao, Z. An, X. Liu and W. Huang, Nat. Photonics, 2019, 13, 406–411 CrossRef CAS.
- V. Abbruscato, J. Electrochem. Soc., 1971, 118, 930–933 CrossRef CAS.
- T. M. Kyota Uheda, H. Takizawa and T. Endo, J. Alloys Compd., 1997, 262, 60–64 CrossRef.
- W. Sun, R. Pang, H. Li, D. Li, L. Jiang, S. Zhang, J. Fu and C. Li, J. Mater. Chem. C, 2017, 5, 1346–1355 RSC.
- W. Hoogenstraaten, Philips Res. Rep., 1958, 13, 515–693 CAS.
- I. F. Chang and P. Thioulouse, J. Appl. Phys., 1982, 53, 5873–5875 CrossRef CAS.
- W. Chen, S. Zhang, M. Zhou, T. Zhao, X. Qin, X. Liu, M. Liu and P. Duan, J. Phys. Chem. Lett., 2019, 10, 3290–3295 CrossRef CAS PubMed.
- Y. Dou, Q. Zhu and K. Du, Chembiochem, 2021, 22, 1871–1883 CrossRef CAS PubMed.
- X. Dai, X. Zhang, I. M. Kislyakov, L. Wang, J. Huang, S. Zhang, N. Dong and J. Wang, Opt. Express, 2019, 27, 13744–13753 CrossRef CAS PubMed.
- Y. Zhao, H. Zeng, X. W. Zhu, W. Lu and D. Li, Chem. Soc. Rev., 2021, 50, 4484–4513 RSC.
- Y. Cui, Y. Yue, G. Qian and B. Chen, Chem. Rev., 2012, 112, 1126–1162 CrossRef CAS PubMed.
- Y. Cui, J. Zhang, H. He and G. Qian, Chem. Soc. Rev., 2018, 47, 5740–5785 RSC.
- J. Heine and K. Muller-Buschbaum, Chem. Soc. Rev., 2013, 42, 9232–9242 RSC.
- Y. Ning, G. Q. Jin, M. X. Wang, S. Gao and J. L. Zhang, Curr. Opin. Chem. Biol., 2022, 66, 102097 CrossRef CAS PubMed.
- L. Zhao, X. Song, X. Ren, H. Wang, D. Fan, D. Wu and Q. Wei, Biosens. Bioelectron., 2021, 191, 113409 CrossRef CAS PubMed.
- H. Xu, W. Yu, K. Pan, G. Wang and P. Zhu, Nano Res., 2020, 14, 720–729 CrossRef.
- W. Shang, X. Zhu, T. Liang, C. Du, L. Hu, T. Li and M. Liu, Angew. Chem., Int. Ed. Engl., 2020, 59, 12811–12816 CrossRef CAS PubMed.
- A. Halder, B. Bhattacharya, F. Haque, S. Dinda and D. Ghoshal, Chem, 2019, 25, 12196–12205 CrossRef CAS PubMed.
- C. Y. Liu, X. R. Chen, H. X. Chen, Z. Niu, H. Hirao, P. Braunstein and J. P. Lang, J. Am. Chem. Soc., 2020, 142, 6690–6697 CrossRef CAS PubMed.
- D. J. Vogel, T. M. Nenoff and J. M. Rimsza, ACS Appl. Mater. Interfaces, 2020, 12, 4531–4539 CrossRef CAS.
- M. Zeng, C. Zhan and J. Yao, J. Mater. Chem. C, 2019, 7, 2751–2757 RSC.
-
X. Feng, PhD thesis, Tianjin Polytech. Univ., 2021.
- T. Lyu and P. Dorenbos, J. Mater. Chem. C, 2018, 6, 369–379 RSC.
- J. Xu, D. Murata, Y. Katayama, J. Ueda and S. Tanabe, J. Mater. Chem. B, 2017, 5, 6385–6393 RSC.
- S. Kamimura, C.-N. Xu, H. Yamada, G. Marriott, K. Hyodo and T. Ohno, J. Ceram. Soc. Jpn., 2017, 125, 591–595 CrossRef CAS.
- S. Lin, H. Lin, C. Ma, Y. Cheng, S. Ye, F. Lin, R. Li, J. Xu and Y. Wang, Light: Sci. Appl., 2020, 9, 22 CrossRef CAS PubMed.
- D. C. Rodríguez Burbano, S. K. Sharma, P. Dorenbos, B. Viana and J. A. Capobianco, Adv. Opt. Mater., 2015, 3, 551–557 CrossRef.
- P. Xiong and M. Peng, J. Mater. Chem. C, 2019, 7, 8303–8309 RSC.
- F. Liu, Y. Liang, Y. Chen and Z. Pan, Adv. Opt. Mater., 2016, 4, 562–566 CrossRef CAS.
- C. Ma, H. Liu, F. Ren, Z. Liu, Q. Sun, C. Zhao and Z. Li, Cryst. Growth Des., 2020, 20, 1859–1867 CrossRef CAS.
- Z. Zou, C. Wu, X. Li, J. Zhang, H. Li, D. Wang and Y. Wang, Opt. Lett., 2017, 42, 4510–4512 CrossRef CAS PubMed.
- M. Pellerin, V. Castaing, D. Gourier, C. Chanéac and B. Viana, Oxide-based Mater. Devices IX.SPIE, 2018, 10533, 196–203 Search PubMed.
- J. Xu, S. Tanabe, A. D. Sontakke and J. Ueda, Appl. Phys. Lett., 2015, 107, 081903 CrossRef.
- J. Xu, D. Murata, B. So, K. Asami, J. Ueda, J. Heo and S. Tanabe, J. Mater. Chem. C, 2018, 6, 11374–11383 RSC.
- Y. Chen, J. Duh, B. Chiou and C. Peng, Thin Solid Films, 2001, 392, 50–55 CrossRef CAS.
- Z. Gong, W. Zheng, Y. Gao, P. Huang, D. Tu, R. Li, J. Wei, W. Zhang, Y. Zhang and X. Chen, Angew. Chem., Int. Ed. Engl., 2019, 58, 6943–6947 CrossRef CAS PubMed.
- P. Zhang, M. Xu, Z. Zheng, B. Sun and Y. Zhang, Mater. Sci. Eng., B, 2007, 136, 159–164 CrossRef CAS.
- J. Du, O. Q. De Clercq, K. Korthout and D. Poelman, Materials, 2017, 10, 1422 CrossRef PubMed.
- S. Li, Q. Zhu, X. Li, X. Sun and J.-G. Li, J. Alloys Compd., 2020, 827, 154365 CrossRef CAS.
- Y. Katayama, J. Ceram. Soc. Jpn., 2017, 125, 793–798 CrossRef CAS.
- J. C. G. Bünzli, Eur. J. Inorg. Chem., 2017, 2017, 5058–5063 CrossRef.
- T. Maldiney, B. Ballet, M. Bessodes, D. Scherman and C. Richard, Nanoscale, 2014, 6, 13970–13976 RSC.
- T. Luan, J. Liu, X. Yuan and J. G. Li, Nanoscale Res. Lett., 2017, 12, 219 CrossRef PubMed.
- S. J. Aurélie Bessière, K. Priolkar, A. Lecointre, B. Viana and D. Gourier, Opt. Express, 2011, 19, 10131–10137 CrossRef PubMed.
- Z. Zhou, W. Zheng, J. Kong, Y. Liu, P. Huang, S. Zhou, Z. Chen, J. Shi and X. Chen, Nanoscale, 2017, 9, 6846–6853 RSC.
- Y. Liu, Y. Wang, K. Jiang, S. Sun, S. Qian, Q. Wu and H. Lin, Talanta, 2020, 206, 120206 CrossRef CAS PubMed.
- T. Maldiney, A. Bessiere, J. Seguin, E. Teston, S. K. Sharma, B. Viana, A. J. Bos, P. Dorenbos, M. Bessodes, D. Gourier, D. Scherman and C. Richard, Nat. Mater., 2014, 13, 418–426 CrossRef CAS PubMed.
- X. H. Lin, L. Song, S. Chen, X. F. Chen, J. J. Wei, J. Li, G. Huang and H. H. Yang, ACS Appl. Mater. Interfaces, 2017, 9, 41181–41187 CrossRef CAS PubMed.
- T. Maldiney, B.-T. Doan, D. Alloyeau, M. Bessodes, D. Scherman and C. Richard, Adv. Funct. Mater., 2015, 25, 331–338 CrossRef CAS.
- E. Teston, Y. Lalatonne, D. Elgrabli, G. Autret, L. Motte, F. Gazeau, D. Scherman, O. Clement, C. Richard and T. Maldiney, Small, 2015, 11, 2696–2704 CrossRef CAS PubMed.
- E. Glais, M. Pellerin, V. Castaing, D. Alloyeau, N. Touati, B. Viana and C. Chanéac, RSC Adv., 2018, 8, 41767–41774 RSC.
- H. Yang, W. Zhao, X. Lin, Z. Liao, Z. Nie, L. Luo, W. Zhang, Z. Hu and J. Zhong, J. Lumin., 2020, 219, 116871 CrossRef CAS.
- J. Shi, X. Sun, S. Zheng, J. Li, X. Fu and H. Zhang, Biomater, 2018, 152, 15–23 CrossRef CAS PubMed.
- P. Sengar, D.-L. Flores, K. Chauhan, B. Can-Uc, K. Juarez-Moreno, O. E. Contreras, M. A. Digman and G. A. Hirata, Cryst. Growth Des., 2020, 20, 5880–5889 CrossRef CAS.
- B. L. Yan Cong, S. Yue, L. Zhang, W. Li and X.-j. Wang, J. Electrochem. Soc., 2009, 154, H272–H275 CrossRef.
- X. Wang, J. Ding and Y. Wang, J. Mater. Chem. C, 2018, 6, 7353–7360 RSC.
- L. J. Chen, X. Zhao and X. P. Yan, ACS Appl. Mater. Interfaces, 2019, 11, 19894–19901 CrossRef CAS PubMed.
- J. Wang, Q. Ma, X. X. Hu, H. Liu, W. Zheng, X. Chen, Q. Yuan and W. Tan, ACS Nano, 2017, 11, 8010–8017 CrossRef CAS PubMed.
- L. Chen, C. Yang and X. Yan, Anal. Chem., 2017, 13, 6936–6939 CrossRef PubMed.
- J. Shi, X. Sun, J. Li, H. Man, J. Shen, Y. Yu and H. Zhang, Biomater, 2015, 37, 260–270 CrossRef CAS PubMed.
- H. J. Zhang, X. Zhao, L. J. Chen, C. X. Yang and X. P. Yan, Anal. Chem., 2020, 92, 1179–1188 CrossRef CAS PubMed.
- J. M. Liu, D. D. Zhang, G. Z. Fang and S. Wang, Biomater, 2018, 165, 39–47 CrossRef CAS PubMed.
- B. Zheng, H. B. Chen, P. Q. Zhao, H. Z. Pan, X. L. Wu, X. Q. Gong, H. J. Wang and J. Chang, ACS Appl. Mater. Interfaces, 2016, 8, 21603–21611 CrossRef CAS PubMed.
- L. Huang, L. Lin, W. Xie, Z. Qiu, H. Ni, H. Liang, Q. Tang, L. Cao, J. Meng and F. Li, Chem. Mater., 2020, 32, 5579–5588 CrossRef CAS.
- X. Qin, Y. Li, D. Wu, Y. Wu, R. Chen, Z. Ma, S. Liu and J. Qiu, RSC Adv., 2015, 5, 101347–101352 RSC.
- Y. Ning, S. Cheng, J. Wang, X. Liu, Y. Feng, W. Li and J. Zhang, R. Soc. Chem., 2019, 10, 4227–4235 CAS.
- M. Palner, K. Pu, S. Shao and J. Rao, Angew. Chem., Int. Ed. Engl., 2015, 54, 11477–11480 CrossRef CAS PubMed.
- Q. Miao, C. Xie, X. Zhen, Y. Lyu, H. Duan, X. Liu, J. V. Jokerst and K. Pu, Nat. Biotechnol., 2017, 35, 1102–1110 CrossRef CAS PubMed.
- X. Ni, X. Zhang, X. Duan, H. L. Zheng, X. S. Xue and D. Ding, Nano Lett., 2019, 19, 318–330 CrossRef CAS PubMed.
- L. Lu, B. Li, S. Ding, Y. Fan, S. Wang, C. Sun, M. Zhao, C. X. Zhao and F. Zhang, Nat. Commun., 2020, 11, 4192–4203 CrossRef PubMed.
- S. Wu, C. Yang and X. Yan, Adv. Funct. Mater., 2017, 27, 1604992 CrossRef.
- J. Nie, Y. Li, S. Liu, Q. Chen, Q. Xu and J. Qiu, Sci. Rep., 2017, 7, 12392 CrossRef PubMed.
- Y. Lin, J. Hu, L. Wu, Q. Zou, D. Chen, D. Huang, H. Lu, S. B. Wang and H. Zhu, J Mater Chem B, 2021, 9, 1131–1137 RSC.
- R. Cao, X. Yu, X. Sun, C. Cao and J. Qiu, Spectrochim. Acta. A. Mol. Biomol. Spectrosc., 2014, 128, 671–673 CrossRef CAS PubMed.
- K. Huang, N. Le, J. S. Wang, L. Huang, L. Zeng, W. C. Xu, Z. Li, Y. Li and G. Han, Adv. Mater., 2022, 34, 2107962 CrossRef CAS PubMed.
- O. Hai, M. Pei, E. Yang, Q. Ren, X. Wu, J. Zhu, Y. Zhao and L. Du, J. Alloys Compd., 2021, 866, 158752 CrossRef CAS.
- C. L. Wu, Mod. Chem. Ind., 2019, 39, 197–202 Search PubMed.
-
G. Qi, PhD thesis, North China Electr. Power Univ., 2018.
- L. Sun, Q. Wang, X. Lin and J. Zhou, J. Shandong Univ. Sci. Technol., 2012, 31, 74–80 Search PubMed.
- N. Zhang, C. G. Liu, M. Zhang, J. Yang, S. N. Li, H. C. Zhu, D. T. Yan, C. S. Xu and Y. X. Liu, Spectrosc. Spect. Anal., 2020, 40, 373–378 CAS.
- L. Ni, M. Wang, Z. X. Zhu, M. Li, C. A. J. Yuan and J. Wu, Chin. J. Anal. Chem., 2022, 50, 103–111 Search PubMed.
- X. Wei, X. Huang, Y. Zeng, L. Jing, W. Tang, X. Li, H. Ning, X. Sun, Y. Yi and M. Gao, ACS Nano, 2020, 14, 12113–12124 CrossRef CAS PubMed.
- Z. Zhou, Y. Li and M. Peng, Chem. Eng. J., 2020, 399, 125688 CrossRef CAS.
- Y. Yang, H. Pan, L. Guan, D. Wang, J. Zhao, J. Yang, Z. Yang and X. Li, J. Mater. Res. Technol., 2020, 9, 3847–3855 CrossRef CAS.
- P. Ankoji and B. H. Rudramadevi, J. Mater. Sci.: Mater. Electron., 2018, 30, 2750–2762 CrossRef.
- Y. Wang, S. Chen and L. Geng, J. Rare Earths, 2021, 39, 913–920 CrossRef CAS.
- L. Pan, S. Liu, X. Zhang, O. Oderinde, F. Yao and G. Fu, J. Alloys Compd., 2018, 737, 39–45 CrossRef CAS.
- J. H. Lee, C. K. Yun, B. P. Seung and P. Hee Dong, Jpn. Soc. Appl. Phys., 2001, 40, 4083–4086 CrossRef.
- Y. C. Kang, D. J. Seo, S. B. Park and H. D. Park, Mater. Res. Bull., 2002, 37, 263–269 CrossRef CAS.
- Z. Li, Y. Zhang, X. Wu, C. Qin and G. Shi, Phys. Lett. A, 2017, 381, 3519–3522 CrossRef CAS.
- Z. Marton, H. B. Bhandari, C. Brecher, S. R. Miller, B. Singh and V. V. Nagarkar, IEEE Trans. Nucl. Sci., 2013, 60, 983–987 CAS.
- G. Dong, X. Xiao, L. Zhang, Z. Ma, X. Bao, M. Peng, Q. Zhang and J. Qiu, J. Mater. Chem., 2011, 21, 2194–2203 RSC.
- F. Ye, S. Dong, Z. Tian, S. Yao, Z. Zhou and S. Wang, Opt. Mater., 2015, 45, 64–68 CrossRef CAS.
- A. A. Tehrani, V. Safarifard, A. Morsali, G. Bruno and H. A. Rudbari, Inorg. Chem. Commun., 2015, 59, 41–45 CrossRef CAS.
- M. Y. Masoomi, A. Morsali and P. C. Junk, RSC Adv., 2014, 4, 47894–47898 RSC.
- Y. Jin, Y. Fu, Y. Hu, L. Chen, G. Ju and Z. Mu, Opt. Mater. Express, 2015, 3, 9435–9443 CAS.
- B. Cheng, Z. Zhang, Z. Han, Y. Xiao and S. Lei, CrystEngComm, 2011, 13, 3545–3550 RSC.
- B. Zhang, C. Zhao and D. Chen, Lumin, 2010, 25, 25–29 CAS.
- S. Demirci, S. Gültekin, S. A. Akalin, Ö. Öter, K. Ertekin and E. Çelik, Mater. Sci. Semicond. Process., 2015, 31, 611–617 CrossRef CAS.
- E. Teston, S. Richard, T. Maldiney, N. Lièvre, G. Y. Wang, L. Motte, C. Richard and Y. J. C. A. E. J. Lalatonne, Chem, 2015, 21, 7350–7354 CrossRef CAS PubMed.
- C. Matias, E. Nassar, M. Verelst and L. Rocha, J. Braz. Chem. Soc., 2015, 26, 2558–2570 CAS.
- D. Meroni, L. Porati, F. Demartin and D. Poelman, ACS Omega, 2017, 2, 4972–4981 CrossRef CAS PubMed.
- J. Yang, Y. Liu, D. Yan, H. Zhu, C. Liu, C. Xu, L. Ma and X. Wang, Dalton Trans., 2016, 45, 1364–1372 RSC.
- M. Xia, G. Wang, Z. Gu, Z. Qiu, C. Rong, J. Zhang, W. Zhou, L. Yu and S. Lian, Luminescence, 2017, 32, 999–1008 CrossRef CAS PubMed.
- Y. J. Li and X. P. Yan, Nanoscale, 2016, 8, 14965–14970 RSC.
- M. Pellerin, E. Glais, T. Lecuyer, J. Xu, J. Seguin, S. Tanabe, C. Chanéac, B. Viana and C. Richard, J. Lumin., 2018, 202, 83–88 CrossRef CAS.
- V. Boiko, M. Markowska, L. Consentino, M. L. Saladino and D. Hreniak, Opt. Mater., 2020, 107, 109956 CrossRef CAS.
- Y. Liu, J. Goebl and Y. Yin, Chem. Soc. Rev., 2013, 42, 2610–2653 RSC.
- Y. Oaki, Supra-Materials Nanoarchitectonics, 2017, 10, 221–246 Search PubMed.
- Y. Zhang, Y. Su, H. Wu, Z. Wang, C. Wang, Y. Zheng, X. Zheng, L. Gao, Q. Zhou and Y. Yang, J. Am. Chem. Soc., 2021, 143, 13675–13685 CrossRef CAS PubMed.
- G. Nidhankar, D. Mohana Kumari, S. Kumar, R. Chaubey, R. Gonnade, G. Kumar, R. Krishnan and S. Babu, Angew. Chem., Int. Ed., 2020, 59, 13079–13085 CrossRef PubMed.
- H. Chen, X. Yao, X. Ma and H. Tian, Adv. Opt. Mater., 2016, 4, 1397–1401 CrossRef CAS.
- C. DeRosa, C. Kerr, Z. Fan, M. Kolpaczynska, A. Mathew, R. Evans, G. Zhang and C. Fraser, ACS Appl. Mater. Interfaces, 2015, 7, 23633–23643 CrossRef CAS PubMed.
- H. Shi, L. Song, H. Ma, C. Sun, K. Huang, A. Lv, W. Ye, H. Wang, S. Cai, W. Yao, Y. Zhang, R. Zheng, Z. An and W. Huang, J. Phys. Chem. Lett., 2019, 10, 595–600 CrossRef PubMed.
- Z. An, C. Zheng, Y. Tao, R. Chen, H. Shi, T. Chen, Z. Wang, H. Li, R. Deng, X. Liu and W. Huang, Nat. Mater., 2015, 14, 685–690 CrossRef CAS PubMed.
- Y. Gong, G. Chen, Q. Peng, W. Z. Yuan, Y. Xie, S. Li, Y. Zhang and B. Z. Tang, Adv. Mater., 2015, 27, 6195–6201 CrossRef CAS PubMed.
- M. Shimizu, R. Shigitani, M. Nakatani, K. Kuwabara, Y. Miyake, K. Tajima, H. Sakai and T. Hasobe, J. Phys. Chem. C, 2016, 120, 11631–11639 CrossRef CAS.
- J. Wang, X. Gu, H. Ma, Q. Peng, X. Huang, X. Zheng, S. H. P. Sung, G. Shan, J. W. Y. Lam, Z. Shuai and B. Z. Tang, Nat. Commun., 2018, 9, 2963 CrossRef PubMed.
- H. Shi, Z. An, P.-Z. Li, J. Yin, G. Xing, T. He, H. Chen, J. Wang, H. Sun, W. Huang and Y. Zhao, Cryst. Growth Des., 2016, 16, 808–813 CrossRef CAS.
- S. Hirata, K. Totani, T. Watanabe, H. Kaji and M. Vacha, Chem. Phys. Lett., 2014, 591, 119–125 CrossRef CAS.
- N. Notsuka, R. Kabe, K. Goushi and C. Adachi, Adv. Funct. Mater., 2017, 27, 1703902 CrossRef.
- S. Hirata, K. Totani, H. Kaji, M. Vacha, T. Watanabe and C. Adachi, Adv. Opt. Mater., 2013, 1, 438–442 CrossRef.
- S. Cai, H. Shi, Z. Zhang, X. Wang, H. Ma, N. Gan, Q. Wu, Z. Cheng, K. Ling, M. Gu, C. Ma, L. Gu, Z. An and W. Huang, Angew. Chem., Int. Ed. Engl., 2018, 57, 4005–4009 CrossRef CAS PubMed.
- Z. Cheng, H. Shi, H. Ma, L. Bian, Q. Wu, L. Gu, S. Cai, X. Wang, W. W. Xiong, Z. An and W. Huang, Angew. Chem., Int. Ed. Engl., 2018, 57, 678–682 CrossRef CAS PubMed.
- M. S. Kwon, Y. Yu, C. Coburn, A. W. Phillips, K. Chung, A. Shanker, J. Jung, G. Kim, K. Pipe, S. R. Forrest, J. H. Youk, J. Gierschner and J. Kim, Nat. Commun., 2015, 6, 8947 CrossRef PubMed.
- D. Lee, O. Bolton, B. C. Kim, J. H. Youk, S. Takayama and J. Kim, J. Am. Chem. Soc., 2013, 135, 6325–6329 CrossRef CAS PubMed.
- M. S. Kwon, D. Lee, S. Seo, J. Jung and J. Kim, Angew. Chem., Int. Ed. Engl., 2014, 53, 11177–11181 CrossRef CAS PubMed.
- J. Samonina-Kosicka, C. A. DeRosa, W. A. Morris, Z. Fan and C. L. Fraser, Macromol, 2014, 47, 3736–3746 CrossRef CAS PubMed.
- Y. Tao, R. Chen, H. Li, J. Yuan, Y. Wan, H. Jiang, C. Chen, Y. Si, C. Zheng, B. Yang, G. Xing and W. Huang, Adv. Mater., 2018, 30, e1803856 CrossRef PubMed.
- J. Jin, H. Jiang, Q. Yang, L. Tang, Y. Tao, Y. Li, R. Chen, C. Zheng, Q. Fan, K. Y. Zhang, Q. Zhao and W. Huang, Nat. Commun., 2020, 11, 842 CrossRef CAS PubMed.
- T. Maldiney, M. U. Kaikkonen, J. Seguin, Q. le Masne de Chermont, M. Bessodes, K. J. Airenne, S. Ylä-Herttuala, D. Scherman and C. Richard, Bioconjugate Chem., 2012, 23, 472–478 CrossRef CAS PubMed.
- B. Y. Wu, H. F. Wang, J. T. Chen and X. P. Yan, J. Am. Chem. Soc., 2011, 133, 686–688 CrossRef CAS PubMed.
- M. H. Ahmed, M. S. Ghatge and M. K. Safo, Subcell. Biochem., 2020, 94, 345–382 CAS.
- X. Zhao, J. Zhou, G. Du and J. Chen, Trends Biotechnol., 2021, 39, 286–297 CrossRef CAS PubMed.
- B. Lin, X. Dong, Q. Wang, W. Li, M. Zhu and M. Li, Front. Cell Dev. Biol., 2021, 9, 635476 CrossRef PubMed.
- F. Ozdemir and A. Baskiran, J. Gastrointest. Cancer, 2020, 51, 1127–1132 CrossRef CAS PubMed.
- Q. Wang, S. Zhang, Z. Li and Q. Zhu, Nanoscale Res. Lett., 2018, 13, 64 CrossRef PubMed.
- X. Sun, J. Shi, S. Zheng, J. Li, S. Wang and H. Zhang, J. Lumin., 2018, 204, 520–527 CrossRef CAS.
- K. Jeong, Y. Kim, C. S. Kang, H.-J. Cho, Y.-D. Lee, I. C. Kwon and S. Kim, Opt. Mater. Express, 2016, 6, 1262–1280 CrossRef CAS.
- I. B. Alaya and M. Mars, Curr. Med. Imaging, 2020, 16, 892–901 CrossRef PubMed.
- S. Mule, E. Reizine, P. Blanc-Durand, L. Baranes, P. Zerbib, R. Burns, R. Nouri, E. Itti and A. Luciani, Cancers, 2020, 12, 23391–23423 CrossRef PubMed.
- Y. Wang, C. X. Yang and X. P. Yan, Nanoscale, 2017, 9, 9049–9055 RSC.
- J. Wang, Q. Li, H. Zhao, W. Yue, K. Zhang, X. Jiang and K. Li, ACS Nano, 2021, 16, 462–472 CrossRef PubMed.
- A. Abdukayum, C. X. Yang, Q. Zhao, J. T. Chen, L. X. Dong and X. P. Yan, Anal. Chem., 2014, 86, 4096–4101 CrossRef CAS PubMed.
- Y. Zhang, H. F. Chan and K. W. Leong, Adv. Drug Delivery Rev., 2013, 65, 104–120 CrossRef CAS PubMed.
- F. Chen, E. B. Ehlerding and W. Cai, J. Nucl. Med., 2014, 55, 1919–1922 CrossRef CAS PubMed.
- S. Wu, Z. Qiao, Y. Li, S. Hu, Y. Ma, S. Wei and L. Zhang, ACS Appl. Mater. Interfaces, 2020, 12, 25572–25580 CrossRef CAS PubMed.
- S. Mura, J. Nicolas and P. Couvreur, Nat. Mater., 2013, 12, 991–1003 CrossRef CAS PubMed.
- H. Chen, B. Zheng, C. Liang, L. Zhao, Y. Zhang, H. Pan, W. Ji, X. Gong, H. Wang and J. Chang, Mater. Sci. Eng., C, 2017, 79, 372–381 CrossRef CAS PubMed.
- J. Wang, Y. Li, R. Mao, Y. Wang, X. Yan and J. Liu, J. Mater. Chem. B, 2017, 5, 5793–5805 RSC.
- T. Ozdemir, Y.-C. Lu, S. Kolemen, E. Tanriverdi-Ecik and E. U. Akkaya, ChemPhotoChem, 2017, 1, 183–187 CrossRef CAS.
- W. Fan, N. Lu, C. Xu, Y. Liu, J. Lin, S. Wang, Z. Shen, Z. Yang, J. Qu, T. Wang, S. Chen, P. Huang and X. Chen, ACS Nano, 2017, 11, 5864–5872 CrossRef CAS PubMed.
- R. Abdurahman, C. X. Yang and X. P. Yan, Chem. Commun., 2016, 52, 13303–13306 RSC.
- G. Liu, S. Zhang, Y. Shi, X. Huang, Y. Tang, P. Chen, W. Si, W. Huang and X. Dong, Adv. Funct. Mater., 2018, 28, 1804317 CrossRef.
- S. K. Das, M. E. Menezes, S. Bhatia, X. Y. Wang, L. Emdad, D. Sarkar and P. B. Fisher, J. Cell. Physiol., 2015, 230, 259–271 CrossRef CAS PubMed.
- Z. Mirza and S. Karim, Semin. Cancer Biol., 2021, 69, 226–237 CrossRef CAS PubMed.
- E. B. Yahya and A. M. Alqadhi, Life Sci., 2021, 269, 119087 CrossRef CAS PubMed.
- L. Qin, P. Yan, C. Xie, J. Huang, Z. Ren, X. Li, S. Best, X. Cai and G. Han, Nanoscale, 2018, 13423–13442 Search PubMed.
- X. Zhao, K. C. Zhao, L. J. Chen, Y. S. Liu, J. L. Liu and X. P. Yan, Chem. Sci., 2020, 12, 442–452 RSC.
- T. Lecuyer, M. A. Durand, J. Volatron, M. Desmau, R. Lai-Kuen, Y. Corvis, J. Seguin, G. Wang, D. Alloyeau, D. Scherman, N. Mignet, F. Gazeau and C. Richard, Nanoscale, 2020, 12, 1967–1974 RSC.
|
This journal is © The Royal Society of Chemistry 2022 |
Click here to see how this site uses Cookies. View our privacy policy here.