DOI:
10.1039/D2NH00006G
(Review Article)
Nanoscale Horiz., 2022,
7, 463-479
Electrodes and electrocatalysts for electrochemical hydrogen peroxide sensors: a review of design strategies
Received
4th January 2022
, Accepted 1st March 2022
First published on 1st March 2022
Abstract
H2O2 sensing is required in various biological and industrial applications, for which electrochemical sensing is a promising choice among various sensing technologies. Electrodes and electrocatalysts strongly influence the performance of electrochemical H2O2 sensors. Significant efforts have been devoted to electrode nanostructural designs and nanomaterial-based electrocatalysts. Here, we review the design strategies for electrodes and electrocatalysts used in electrochemical H2O2 sensors. We first summarize electrodes in different structures, including rotation disc electrodes, freestanding electrodes, all-in-one electrodes, and representative commercial H2O2 probes. Next, we discuss the design strategies used in recent studies to increase the number of active sites and intrinsic activities of electrocatalysts for H2O2 redox reactions, including nanoscale pore structuring, conductive supports, reducing the catalyst size, alloying, doping, and tuning the crystal facets. Finally, we provide our perspectives on the future research directions in creating nanoscale structures and nanomaterials to enable advanced electrochemical H2O2 sensors in practical applications.
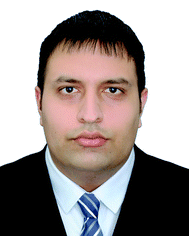
Muhammad Adil Riaz
| Dr Muhammad Adil Riaz completed his MPhil in Chemical & Biomolecular Engineering from the Hong Kong University of Science and Technology in 2016. His research project in MPhil focused on designing novel graphene-based nanoporous sorbent materials for environmental remediation. He then pursued his doctoral study in Chemical Engineering at the University of Sydney, Australia, from 2017 to 2021. He worked with Professor Yuan Chen on designing novel metal–carbon nanocomposites for hydrogen peroxide electrochemical detection. He has 12 publications in high-impact factor scientific journals such as Sensors & Actuators B, Journal of Hazardous Materials and Carbon, etc. |
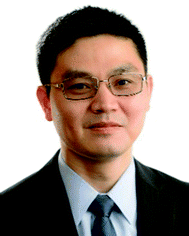
Yuan Chen
| Yuan Chen received a bachelor's degree from Tsinghua University and a PhD from Yale University. He is a professor at The University of Sydney. His research focuses on carbon materials and their sustainable energy and environmental applications, including batteries, supercapacitors, electrocatalysts, membranes, and antibacterial coatings. He is a fellow of the Royal Society of Chemistry and the Institution of Chemical Engineers. He is currently serving as an editor for Carbon and Journal of Alloys and Compounds. |
1. Introduction
Hydrogen peroxide (H2O2) can be generated in cells by the oxygen metabolism of organic molecules. It plays several essential functions in biological systems. Cells use H2O2 as a reactant in oxidative biosynthesis against bacterial pathogens or as a signaling molecule to regulate various physiological processes.1 As an antioxidant defense mechanism, cells secrete enzymes to decompose H2O2 into H2O and O2 to maintain an average intracellular concentration of 1–100 nM or an intercellular concentration of 10–1000 nM. A high H2O2 concentration induces oxidative stress to cells, damages cell membranes and DNA, and causes errors in cell signaling, leading to various diseases (Fig. 1a and b).2 The fluctuation of H2O2 concentration in the cellular microenvironment of specific organs/tissues has been used as an indicator for cardiovascular, neurological (Alzheimer and Parkinson) diseases, and diabetes. The average H2O2 concentration (1–5 μM) in the blood can increase to 30–50 μM in the case of inflammation. The urinary H2O2 concentration can also indicate oxidative stress.3,4 Cancer cells have a high H2O2 concentration, which initiates tumor formation and helps in the progression and metastasis of tumors.5 H2O2 is often overproduced in the microenvironment of tumor cells due to aberrant metabolism, which can be used as a cancer diagnostic tool.6 H2O2 can also provide an indirect measurement of glucose because H2O2 is produced as a by-product during the enzymatic electrochemical sensing of glucose.7 H2O2 is a biomarker for lung diseases, such as chronic obstructive pulmonary disease, asthma, and lung cancers. It can be detected in exhaled breath vapors from patients.8 Furthermore, increased H2O2 concentration in the epidermis can also be used to monitor various skin disorders.9
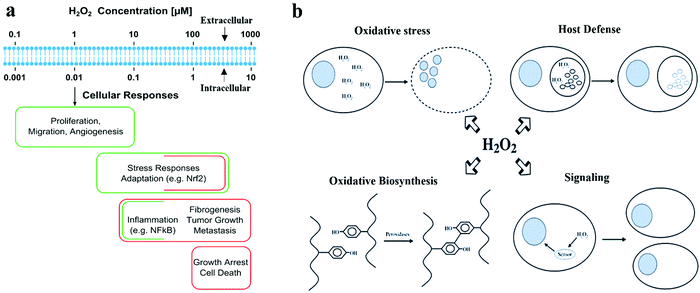 |
| Fig. 1 Biological roles of H2O2. (a) Different H2O2 concentrations generate varied responses at the cellular level. Reproduced with permission from ref. 2. Copyright 2017, Elsevier. (b) Four main biological functions of H2O2. Reproduced with permission from ref. 1. Copyright 2006, Mary Ann Liebert Inc. | |
Apart from the biological significance, H2O2 is widely used as an oxidizer, bleaching agent, and disinfectant in many fields. Vaporized H2O2 is a common sterilant used in industry and healthcare facilities. Thus, monitoring H2O2 is critical to avoid unwanted exposure to workers as higher concentrations of H2O2 produce various undesirable effects,10 such as H2O2 induced skin blanching11 and portal venous gas embolism from accidental concentrated H2O2 ingestions.12 Various sensors are available to monitor H2O2 vapors at the ppm level to prevent such accidents. The H2O2 workplace airborne exposure limit by OSHA is 1 ppm over an 8 hour shift. H2O2 is also used in hospitals for instrument disinfection,16–18 orthopedic surgery,19 and treatment of brain ischemia as an antiseptic.13 Furthermore, it is used in industry to sanitize fruits/vegetables after harvest, disinfect food packaging, and as a preservative in milk to increase its shelf life.14 As a bleaching agent, H2O2 is used in teeth whitening products,15 cosmetics,16 and the paper/pulp industry.17 As an oxidizing agent, it is used to degrade environmental pollutants and as odor control in wastewater.18 Therefore, there is a clear need to measure H2O2 in stationary and flowing aqueous systems to avoid potentially harmful exposures.
Various methods have been utilized for H2O2 sensing based on different sensing principles. Colorimetric sensors use chemicals to react with H2O2 in solution and detect its presence qualitatively by observing color fading of analyte solution or quantitatively by measuring changes in the absorbance of visible light.19 Fluorescence sensors utilize luminescent materials that can absorb UV light and re-emit it as visible light where H2O2 induced quenching of signals provides quantitative sensing.20,21 Fluorescence microscopic imaging has been used to visualize intracellular H2O2 near cellular organelles, such as mitochondria.22 Some optical sensors based on electrochemiluminescence utilize electron stimuli to generate ROS, which reacts with H2O2 to generate luminescent species in their excited state. The emitted light intensity from these luminescent species is proportional to the H2O2 concentration.23–25 Sensors based on field-effect transistors detect H2O2 by measuring the current intensity changes between the source and drain electrodes induced by surface potential changes of the gate electrode upon H2O2 binding.26 Surface-enhanced Raman spectroscopy sensors measure the change in Raman scattering of active plasmonic surfaces, such as Au or Ag, upon exposure to H2O2.27 Surface plasmon resonance sensors measure variations in the angle of reflection of incident light of active plasmonic surfaces induced by H2O2 adsorption.28 There are also some less common H2O2 sensing methods, including calorimetric,29 magnetic,30 and coulometric approaches,31 as well as gas/liquid chromatography.32,33
Electrochemical sensors utilize electron-driven chemical reactions of H2O2 for sensing, such as the oxidation/reduction of H2O2 by electrocatalysts on the surface of electrodes, which generate electrical currents based on H2O2 concentration. The oxidation of H2O2 (H2O2 → O2 + 2H+ + 2e−; E0 = 0.68 V vs. normal hydrogen electrode (NHE)) releases electrons at anodes. Reducing H2O2 (H2O2 + 2H+ + 2e− → 2H2O; E0 = 1.77 V vs. NHE) requires receiving electrons from cathodes. They are further categorized into amperometric, potentiometric, or impedance sensors based on their measured concentration-dependent variables, i.e., current, voltage, or resistance, respectively. Electrochemical sensors are most widely used for H2O2 sensing due to their high sensitivity, low cost, and simple operation. They can also be combined with other methods to form multiple sensing platforms, for example, photoelectrochemical sensors,34–37 which utilize visible light to drive the electrochemical reaction, plasmon-assisted electrochemical sensors,38,39 organic electrochemical transistors,40 and spectroelectrochemical sensors.41
Although significant efforts have been devoted to developing electrochemical H2O2 sensors, to the best of our knowledge, no comprehensive review has described both recent state-of-the-art studies of electrocatalyst materials used in electrochemical H2O2 sensors and the design of electrodes to achieve ultrasensitive H2O2 detection. Here, we first describe several widely used electrode structures, including rotation disc electrodes, freestanding electrodes, all-in-one electrodes, and representative commercial H2O2 probes. Next, we discuss the latest electrocatalyst design strategies in two areas: increasing the number of active sites and improving their intrinsic catalytic activities for H2O2 redox reactions. Finally, we propose several research directions that we expect to play critical roles in enabling practical applications.
2. Electrode structures of electrochemical H2O2 sensors
A key component of H2O2 electrochemical sensors is the electrodes, and electrochemical reactions occur on the electrode surfaces. Several types of electrodes have been used in research labs or in field applications, including glassy carbon electrodes (GCEs) and freestanding electrodes used in 3-electrode cells and integrated all-in-one electrodes, such as screen-printed electrodes (SPEs) (see Fig. 2) or integrated probes in commercial products (Table 1).
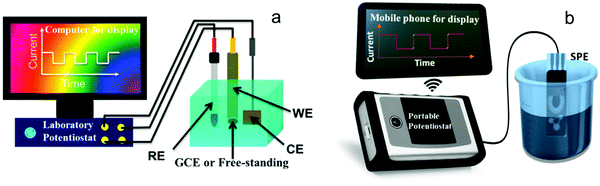 |
| Fig. 2 Common electrochemical H2O2 sensing platforms used in research labs. (a) A 3-electrode cell controlled by a potentiostat. Adapted with permission from ref. 42. Copyright 2015, American Chemical Society. (b) A portable sensing platform using an all-in-one screen-printed electrode (SPE) and a hand-held commercial potentiostat (PalmSens). | |
Table 1 Comparison of different types of electrodes used in H2O2 electrochemical sensors
Electrodes |
Advantages |
Drawbacks |
Glassy carbon electrodes (in 3-electrode cells) |
High sensitivity and reproducibility |
No intrinsic catalytic activity (requires the deposition of catalysts with binders) |
|
Low noise in the signal |
|
|
Fast detection |
High cost |
|
High conductivity |
Limited to research labs |
|
Freestanding electrodes (in 3 electrode cells) |
Wide detection ranges |
High noise in the signal |
|
High intrinsic activity |
Low reproducibility |
|
No binders required |
Long response time |
|
Integrated all-in-one electrodes (e.g., screen-printed electrodes or all-in-one probes) |
Suitable for portable detections |
Low sensitivity |
|
Low cost |
Low conductivity substrates (mostly made of plastics or ceramics) |
|
Low sample volume |
|
|
Can be flexible/wearable |
Low or no catalytic activity |
|
Many commercial products |
Additional surface modifications may be required |
Table 2 Comparison of freestanding macroscopic 3D working electrodes
Freestanding electrodes |
Advantages |
Drawbacks |
Carbon-based |
High conductivity |
Low intrinsic catalytic activity (require surface modifications and the deposition of active catalysts) |
|
Low cost |
|
|
Biocompatibility |
|
|
Metal-based |
High intrinsic catalytic activity |
Low surface area |
|
High conductivity |
High cost |
|
Unconventional materials |
Low cost |
Low intrinsic catalytic activity (require surface modifications and the deposition of active catalysts) |
Glass, Si, ITO, FTO, PET, and ceramics |
Robust in many practical applications |
|
|
Electrode arrays (micro and nano) |
Multiplexing |
High fabrication cost |
|
Minimized mass transfer resistance |
Low utilization of surfaces |
A typical configuration of electrochemical H2O2 sensors involves a 3-electrode cell, including a working electrode, a reference, and a counter electrode. The electrodes are immersed in H2O2 containing analytes and controlled by a potentiostat. A bulky potentiostat would limit sensors’ portability. The reference and counter electrodes are typically silver/silver chloride (Ag/AgCl) and platinum (Pt) wire/mesh, respectively. Several types of working electrodes have been used with significantly different sensing performances, including rotation disc electrodes (RDEs), freestanding electrodes in compact 2D films, 3D foams, or 1D wires, and microelectrode arrays. Another electrode configuration combines all three electrodes on a single substrate suitable for portable on-site detection. We describe these working electrodes and their characteristics below.
2.1. Rotation disc electrodes
RDEs rotate themselves during operation, effectively preventing mass transfer limits in solutions. They offer high sensitivity, reproducibility, and signal/noise ratios. GCEs are the most commonly used in H2O2 sensing among various available carbon or metal-based RDEs. Glassy carbon has high electrical conductivity, is resistant to chemical corrosion, and is inert to H2O2 reduction or oxidation. Electrocatalysts are deposited on GCEs to catalyze reactions involving H2O2. However, the cost of GCEs is relatively high. Their small surface area also constrains the mass loading of electrocatalysts, which restricts the lower detection limits of H2O2 sensors. Furthermore, it is also tedious to prepare electrocatalyst ink and deposit the ink on GCEs. Thus, the usage of GCEs is usually restricted to research labs.
2.2. Freestanding electrodes
Freestanding electrodes use mechanically stable and electrically conductive substrates to host electrocatalysts. They have much larger surface areas than GCEs to accommodate a high mass loading of electrocatalysts. Sometimes, electrocatalysts are directly synthesized on substrates of freestanding electrodes, which avoids electrocatalyst ink preparation and deposition and the use of nonconductive polymer binders in ink, improving the electrical conductivity of electrodes. Freestanding electrodes have different structures, as illustrated in Fig. 3a, including compact 2D films, 3D foams, and 1D wires.
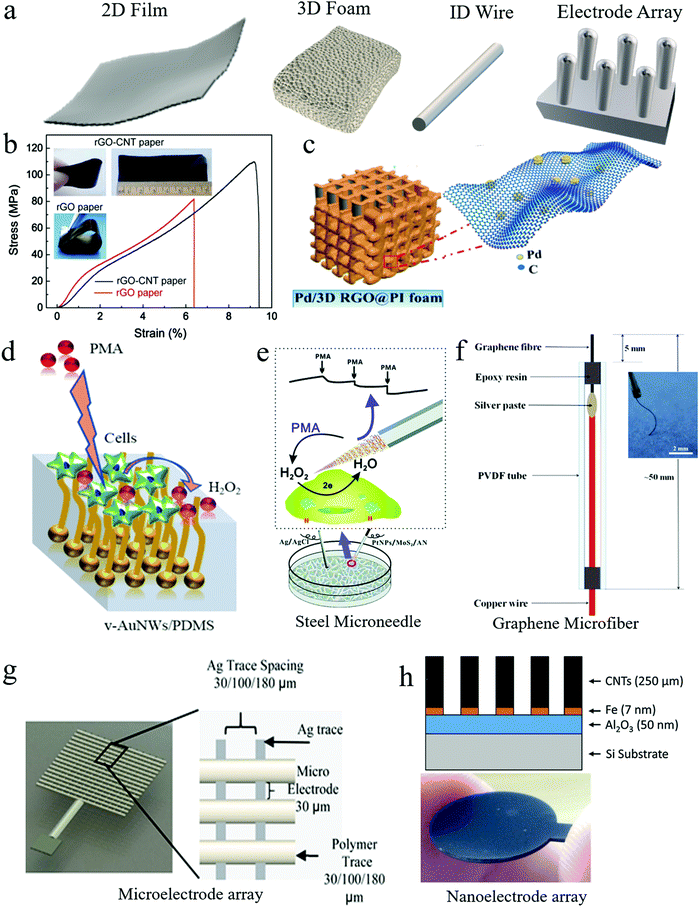 |
| Fig. 3 Freestanding working electrodes used for H2O2 sensing. (a) Freestanding electrodes in different shapes: compact 2D film, porous 3D foam, and 1D wire. (b) An rGO–CNT paper electrode demonstrated good mechanical strength and flexibility. Reproduced with permission from ref. 52. Copyright 2015, Elsevier. (c) Bendable 3D polyimide foam electrode coated with rGO nanosheets and Pd nanoparticles. Reproduced with permission from ref. 59. Copyright 2019, American Chemical Society. (d) Vertical Au nanowire-based stretchable electrodes on a polydimethylsiloxane (PDMS) substrate. Reproduced with permission from ref. 66. Copyright 2018, American Chemical Society. (e) A steel microneedle electrode for H2O2 sensing in living cells. Reproduced with permission from ref. 67. Copyright 2017, Royal Society of Chemistry. (f) A graphene microfiber-based flexible electrode. Reproduced with permission from ref. 68. Copyright 2018, Elsevier. (g) A 3D printed Ag microelectrode array. Reproduced with permission from ref. 69. Copyright 2016, Elsevier. (h) A CNT nanoelectrode array supported on a Si substrate. Reproduced with permission from ref. 72. Copyright 2017, Elsevier. | |
2.2.1 2D film electrodes.
Compact 2D film-shaped freestanding electrodes use various 2D compact films as substrates, including paper and films made of different carbon materials.43–45 For example, photo paper coated with Ag nanoparticles was used as freestanding electrodes for H2O2 sensing.43 Carbon materials offer high electrical conductivity. For example, carbon fiber films44 and electrospun carbon nanofiber films45 contain highly conductive 1D electron transport channels. Carbon cloth made up of micrometer-sized carbon fiber bundles has also been used.46 Furthermore, graphene films offer high electrical conductivity and large surface areas, obtained by either assembling reduced graphene oxide (rGO) nanosheets47–49 or direct synthesis by chemical vapor deposition (CVD).50,51 Carbon nanotubes (CNTs) have been incorporated in rGO films to prevent the stacking of graphene nanosheets, resulting in freestanding rGO–CNT paper electrodes with larger surface areas and good mechanical strength and flexibility (Fig. 3b).52 On the contrary, although graphite paper also has high electrical conductivity, it is seldom used to fabricate freestanding electrodes for H2O2 sensing because of its low surface area.
2.2.2 3D foam electrodes.
3D metal foams provide a large surface area to host electrocatalysts, and they also have interconnected channels to enable efficient mass transfer. They are often used as substrates in freestanding electrodes. For example, Cu foam-based freestanding electrodes have delivered ultra-high sensitivity (up to 6349 μA mM−1 cm−2) in H2O2 sensing.53,54 Similarly, Ni foams loaded with electrocatalysts have also acted as freestanding electrodes.55 Alternatively, 3D graphene foams with hierarchically porous structures and stacked graphene nanosheets can also serve as freestanding electrodes. For example, Cao et al. affixed a CVD-grown 3D graphene foam on a glass wafer, coating one side of the graphene foam with Ag paste as an electrode for amperometric sensing of H2O2.56 Loading catalytically active metallic nanoparticles (e.g., Pt, Cu2O and Prussian blue) can further boost the H2O2 sensing performances of graphene foam electrodes.49,57,58 Furthermore, Yang et al. coated rGO nanosheets on a 3D polyimide foam, followed by electrodeposition of Pd nanoparticles (Fig. 3c). The resulting Pd/3D rGO@PI foam showed high catalytic activity for H2O2 reduction.59
2.2.3 1D wire electrodes.
1D wire-shaped freestanding electrodes are attractive for H2O2 sensing, and in particular micrometer size fiber electrodes can be used to monitor neurotransmitters. For example, microelectrodes made of commercial carbon fibers (7 μm in diameter) were used in an in vitro system for H2O2 sensing in 2010, demonstrating a sensing limit of 2 μM in rat brain tissues.60 Carbon fiber-based microelectrodes also enabled highly selective amperometric sensing of dynamic H2O2 concentration changes in a rat brain induced by microinfusion of artificial cerebrospinal fluid.61 The surface of carbon fiber electrodes was often further functionalized to introduce electroactive species, which boosted their sensing performances.62,63 Selective H2O2 sensing in the brain is often challenging because other molecules, such as adenosine and histamine, can also be oxidized under a similar voltage as H2O2. A size-exclusion membrane (1,3-phenylenediamine) was electrodeposited on carbon fiber-based microelectrodes, blocking other neurochemicals and enabling selective H2O2 sensing.64 Furthermore, the volume of analytes in biochemical applications is sometimes tiny. Zhang et al. combined micropipette tips with carbon microfiber electrodes to achieve efficient H2O2 sensing in 20 μL samples where H2O2 was generated as an intermediate reaction product of glucose electro-oxidation.65 Metal wires and nanowires have also been used as 1D wire electrodes. Zhai et al. used vertical Au nanowires (v-AuNWs) with nanoparticle heads on one side and nanowire tails on the opposite side to seamlessly integrate with soft and curvilinear biological tissues (Fig. 3d).66 The resulting nanowire electrodes achieved an H2O2 detection limit of 80 μM with a linear range of 0.2–10.4 mM at 20% strain. Furthermore, a stainless-steel microneedle with its tip coated with electroactive nanomaterials was used as a 1D wire electrode for H2O2 sensing in living cells (Fig. 3e).67 Carbon nanomaterials, such as CNTs and graphene, can also be integrated into fibers as 1D wire freestanding electrodes, offering a high surface area and excellent electrical conductivity. For example, Peng et al. synthesized graphene fibers of 50 μm using a hydrothermal method. A graphene fiber was sealed in a tube with epoxy resin with an exposed section of 5 mm in length (Fig. 3f). The graphene fiber electrode demonstrated good H2O2 sensitivity and biocompatibility with living cells.68
2.2.4 Microelectrode/nanoelectrode arrays.
Besides relying on a single electrode, microelectrode arrays (MEAs) or nanoelectrode arrays (NEAs) have been fast-developing directions for electrochemical H2O2 sensors. In general, electrode arrays can increase the sensing sensitivity and provide the opportunity to obtain multiplexed data. Yang et al. 3D printed an MEA (6 × 2 mm) made of Ag microelectrodes (30 × 15 μm), in which individual microelectrodes were spaced 30, 100, or 180 μm apart from each other.69 The authors studied the effects of microelectrode density in MEAs on the overall H2O2 sensing performance of MEAs. It was revealed that a high microelectrode density (30 μm apart) had the highest sensitivity. However, the overlap of the diffusion layer from neighboring microelectrodes increased the noise level (12 nA) and sensing limit (2.7 μM). In contrast, the MEA with a low electrode density (180 μm apart) showed a reduced noise level (1.2 nA) and higher sensing limit (1.7 μM) but suffered from low sensitivity. Hence, the authors concluded that an intermediate microelectrode density (100 μm apart) would be optimum for H2O2 sensing. NEAs are expected to further improve the sensing performance with a higher nanoelectrode density and minimized diffusion layer overlapping. When NEAs are supported on a macroscopic conductive substrate, they can also be used as a freestanding electrode. Several studies have explored NEAs for H2O2 sensing, such as arrays of Au nanospheres supported on a Si substrate70 and Fe oxide nanopatterns supported on an indium tin oxide substrate.71Fig. 3h shows vertically aligned CNT nanoarrays grown on a Si wafer as an NEA. The authors studied the effect of flow patterns on its electrochemical performance and concluded that through-flow sensing produced much higher signals than stirred sensing.72
2.3. All-in-one electrodes
All-in-one electrodes have working, counter, and reference electrodes fabricated together on one substrate as a single chip or packaged into one container as a single probe. Their integrated structure and compact design are most suitable for portable sensing applications. We describe both these types of all-in-one electrodes and some representative commercial products in the subsections below.
2.3.1. Single chips with multiple electrodes.
Ink containing various active materials can be printed on inert substrates, such as ceramics, plastics, or paper, to fabricate multiple electrodes as sensing chips. These chips are often designed for low-cost disposable one-time use. However, because of their less conductive substrates, their electrochemical performance is usually inferior to that obtained using GCEs. Different printing or patterning methods have been used to fabricate electrodes. Screen printing is one of the most widely used methods because of its excellent versatility. Commercial companies, such as Pine Research, Dropsens, and Lanprintech, provide various SPEs. Many research studies have utilized these commercial SPEs for H2O2 sensing with working electrodes further modified by additional electrocatalysts to enhance the H2O2 sensing performance. For example, N-doped graphene nanoribbons,73 chitosan-decorated pristine graphene,74 3D graphene/Co oxides,75 and Au nanoparticles supported on carbon black76 have been used to modify SPEs. These electrocatalysts were deposited on SPEs by drop-casting,73,76 or electrodeposition.74 Commercial companies also provide SPEs modified with electrocatalysts specifically designed for H2O2 sensing. For example, Dropsens and Rusens sell SPEs modified by ferrocyanide and Prussian blue, respectively. Zimmer & Peacock sell SPEs modified by some enzymes.
Furthermore, sensing chips on flexible substrates can be used as wearable sensors in contact with human skin for monitoring various health and environmental conditions.77–80 However, few studies have explored such chips for H2O2 sensing, which may be because continuous H2O2 monitoring in human body fluids is not in high demand, unlike glucose monitoring which is required in diabetes patients. Also, H2O2 is usually not present in interstitial fluids (sweat or tears) from the skin and body, unlike some other analytes (e.g., lactate, glucose, Ca). Nonetheless, Santhiago et al. fabricated an H2O2 sensing chip by printing electrode patterns on cellulosic paper using ink containing carbon black and redox-active Prussian blue nanoparticles.81 Apart from its H2O2 sensing capability, the chip showed excellent mechanical stability with excellent folding stability (>20
000 cycles), and its electronic circuit can be crumpled without significant sensing performance loss. Maier et al. also fabricated H2O2 sensing chips by printing carbon materials and Prussian blue on a paper substrate.8 The chips were used to monitor the concentration of H2O2 in artificial breaths. They were integrated into commercial masks for H2O2 sensing of the exhaled breath of patients with respiratory diseases, reducing the high cost typically required for breath collection and analysis.
2.3.2. Single probes with packaged electrodes.
Using separated reference electrodes and working electrodes often brings various inconveniences in practical applications. Thus, various commercial products have integrated a reference electrode with an H2O2 sensing working electrode into a single probe (see the commercial products illustrated in Fig. 4a and b). There are also many research studies on developing single probes for challenging measurement conditions. For example, Fig. 4c shows a probe with microelectrodes sealed in an insulated container, used to monitor H2O2 related ROS generated in cold atmospheric plasma under ultrahigh voltages.82 It is desirable to evaluate the defense potential of biofilms against electrochemically produced H2O2 in bioelectrochemical systems. However, it is impossible to measure H2O2 concentrations under the interference of a strong electric field using common electrodes.83Fig. 4d shows an all-in-one probe used in a bioelectrochemical system to monitor H2O2 concentration. The probe was also used to measure H2O2 decomposition in filamentous fungus to understand the microorganism's antioxidant defense mechanism. The fungus was alive after the electrochemical test, indicating the benefit of using an integrated probe.84
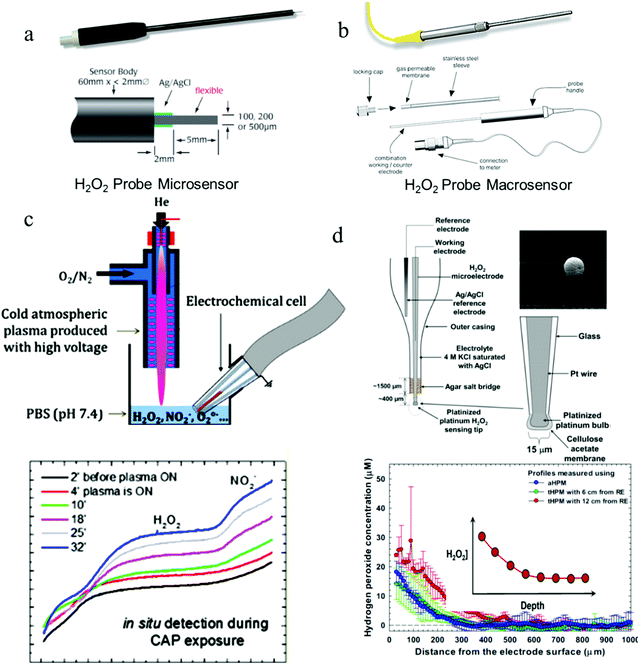 |
| Fig. 4 All-in-one probe sensors for H2O2 sensing. (a and b) Structures of commercial H2O2 probes for biological applications (WPI HPO sensors). (c and d) H2O2 probes for H2O2 measurement under the interference of high voltages and currents, respectively. Reproduced with permission from ref. 82 and 83. Copyright 2019, American Chemical Society & Copyright 2015 Elsevier respectively. | |
2.3.3. Representative commercial H2O2 probes.
Commercial electrochemical H2O2 sensors usually utilize designs of a single probe or a single chamber, which encapsulates all electrodes. They are convenient to be used in practical scenarios. For example, an HPO macro-sensor from World Precision Instruments (WPI) encapsulates an H2O2 sensing electrode and a reference electrode in a single 2 mm diameter stainless steel probe. The probe also contains internally refillable electrolytes and a gas permeable membrane to prevent unwanted species from entering the probe tip. An H2O2 microsensor from WPI uses a 100 μm diameter carbon fiber working electrode tip, enclosed in a hypodermic needle to insert into tissues beneath the skin. An L-shape working electrode design allows tissue bath studies. A list of representative commercial all-in-one H2O2 sensors and their key features are provided in Table 3.
Table 3 Representative commercial H2O2 electrochemical sensors and their key features
Probe name |
Electrode materials |
Housing materials |
Key features |
World Precision Instruments (WPI) ISO-HPO-X sensors |
Platinum wire sensing electrode (HPO-2) |
Stainless-steel |
HPO-2 macrosensor: 100 nM–100 μM range, 8 pA μM−1 sensitivity, for uses in cell cultures and related applications. |
|
|
Activated carbon fiber sensing electrode (HPO-100) |
|
HPO-100 microsensor: 1 nM–1 mM range, sensitivity ≥ 1 pA nM−1, for use in tissues and microvessels. |
|
Seko Kontrol 500 Probe |
Platinum and copper electrodes |
ABS plastic material IP65 |
0–500 ppm, for uses in swimming pools and wastewater with a flow rate up to 30 L h−1 and pressure up to 5 bar. |
|
ChemDAQ's Steri-Trac H2O2 area monitor |
A precious metal electrode on a porous PTFE membrane |
Plastic material |
0–10 ppm, 0.1 ppm resolution, for uses to monitor workplace exposure of sterilant H2O2 vapors, and wireless sensing with audible and visual alarms. |
|
Gazdetect B12 H2O2 detector |
Sensor material: Noryl |
NEMA 4X polystyrene |
0–10 ppm with 4–20 mA output display powered with 24 V for uses in vapor sensing, high corrosion resistance, and humidity tolerance (up to 100%). |
|
Edaphic Scientific (ES) H2O2 micro-sensor |
Silicone (membrane), glass (sensor), epoxy resin |
Titanium |
0.02–10%, for uses to in situ measuring and monitoring aqueous H2O2 concentrations in wastewater (colored and turbid as well). It can be used in water depths of up to 100 m, 100 bar. |
|
ProMinent DULCOTEST H2O2 sensors (PER1 and PEROX) |
Diaphragm-covered electrodes |
Shaft/diaphragm material: PVC/silicone or stainless-steel/PVDF |
0.5–2000 mg L−1, PER1 for uses to measure H2O2 concentration in chemically contaminated and polluted flowing water. PEROX for uses in measuring H2O2 concentration without cross-sensitivity to chlorine. |
|
Analytical Technologies Inc. (ATI) H2O2 Q46 |
Platinum electrode covered with a membrane |
NEMA 4X (IP-66) and polycarbonate enclosure |
0–2000 ppm, 0.05 ppm sensitivity, for uses to continuously measure the concentration of H2O2 in cooling water, aquarium, and wastewater plants. |
|
Dräger XS EC H2O2 Sensor |
Porous polymer membrane coated electrodes |
Plastic |
0–20 ppm, 0.1 ppm resolution, for uses to measure vaporized H2O2 in ambient air |
3. Electrocatalysts for H2O2 sensing
Electrochemical H2O2 sensing usually depends on 2-electron oxidation or reduction reactions of H2O2 on the surface of electrocatalysts. Thus, the performance of H2O2 sensors is strongly affected by the catalytic activity of electrocatalysts. In general, the activity of electrocatalysts is determined by the intrinsic activity of their catalytic sites and the total number of accessible catalytic sites. In recent years, various approaches have been explored to improve the activity of electrocatalysts used in electrochemical H2O2 sensors. We review these advances in the following two subsections, and the performance of representative electrocatalysts used for ultrasensitive H2O2 sensing is compared in Table 4.
Table 4 Design strategies and performance comparison of representative electrocatalysts used for ultrasensitive H2O2 sensing with a nanomolar level detection limit
Electrocatalysts |
Design strategy |
Potential (V) |
Sensitivity μA mM−1 cm−2 |
Detection limit |
Ref. |
Electrocatalysts deposited on glassy carbon electrodes |
1% Cu–N/C |
N-doping, pore structuring |
−0.7 |
2992 |
40 |
135
|
Pt-skin Pt3Co z-NWs/C |
More active facets, alloying, conductive support |
−0.05 |
3030 |
0.4 |
166
|
MoS2 NPs |
Ultra-small nanoparticles |
−0.25 |
2580 |
2.5 |
129
|
Au67Cu33 NWs |
Ultrathin nanowires, alloying |
+0.48 |
2710 |
2.0 |
131
|
PtFe Clusters/rGO |
Alloying, pore structuring, conductive support |
+0.1 |
4450 |
2.0 |
173
|
|
Electrocatalysts used in freestanding electrodes |
ZnCo2O4/Co3O4/Cu foam |
Alloying, conductive support, metal oxides |
−0.1 |
3260 |
1.0 |
148
|
Fe3N–Co2N nanowire arrays on carbon cloth |
Metal nitrides, alloying, conductive support |
−0.2 |
2273.8 |
59 |
174
|
Ni(OH)2/rGO/Cu2O on Cu foil |
Metal oxides, conductive support (nano and macro) |
+0.6 |
1706.3 |
200 |
175
|
CuO nanowire array on Cu foam |
Metal oxide, conductive support |
−0.4 |
5750 |
560 |
53
|
3.1. Increasing the number of active sites
3.1.1. Pore structuring.
A porous structure is often desirable for electrocatalysts, which facilitates the mass transfer of electrolytes/analytes to reach catalytically active sites and provides a large surface area to host more catalytically active sites. Porous structures can be created by either top-down and bottom-up methods. The calcination of precursors with well-defined porous structures, such as metal–organic frameworks, is one of the most widely used top-down methods. Furthermore, polymers and various organic compounds are often used as sacrificial materials to create pores. They would degrade and release gases during the calcination. Another common top-down method is to use chemical or physical etching to create pores.85 Alternatively, using various templates is one the most common bottom-up methods, where nanostructures are formed on templates mimicking their shape. Hard templates, such as silica or other metal oxide nanoparticles, need to be removed using concentrated acids or base solutions. Soft templates, such as block polymers or surfactants, can be removed more easily by calcination in air or dissolution in mild solvents. Hard templates are more often used to synthesize crystalline nanostructures because the required high-temperature annealing conditions may destroy the porous structures formed by soft templates.86 2D materials, such as graphene and inorganic nanosheets, can self-assemble into 3D materials with hierarchal porous structures. However, less control of the pore size and shape is currently achieved using 2D materials.
The methods described above have been explored to create various porous electrocatalysts for H2O2 sensing. For example, Yao et al. used a soft micelle template to prepare Pd nanostructures with 2 nm lamellar pores (Fig. 5a), which demonstrated a high sensitivity of 201 μA mM−1 cm2 in H2O2 sensing.87 3D hierarchal porous N-doped carbon electrocatalysts were synthesized for H2O2 sensing. They were obtained by carbonizing a mixture of glucose (acting as the carbon source) and melamine (acting as an N dopant and pore-forming agent) in two steps at 600 and 900 °C, respectively. The resulting electrocatalyst has a high surface area of 431.4 m2 g−1 with an average pore size of 10–23 nm.88 Das et al. inkjet-printed graphene ink into 3D graphene electrocatalysts and then irradiated them with a UV light laser.89 When the laser power increased from 40 to 85 mJ cm−2, the catalyst surface morphology changed dramatically (Fig. 5b), and the sensitivity of H2O2 sensing increased from 0.54 to 3.32 μA mM−1. The authors proposed that the light irradiation led to the formation of a unique 3D petal-like porous structure and a reduction in graphene sheet electrical resistance.89 Several other studies also synthesized porous graphene foam electrocatalysts for H2O2 sensing by bottom-up assembling graphene oxide nanosheets.49,57,58
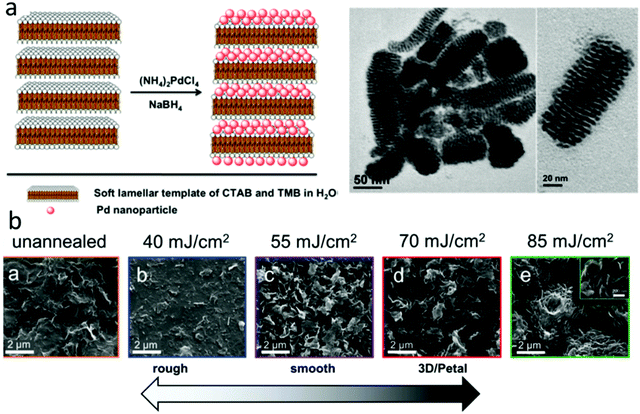 |
| Fig. 5 Electrocatalysts with porous structures for H2O2 sensing. (a) A bottom-up method to synthesize Pd nanostructures with lamellar pores. Reproduced with permission from ref. 87. Copyright 2017, American Chemical Society. (b) Top-down laser etching approach for H2O2 sensing. Reproduced with permission from ref. 89. Copyright 2016, Royal Society of Chemistry. | |
3.1.2. Conductive supports.
Electrocatalysts containing metallic nanoparticles often suffer from poor stability due to the fast agglomeration of nanoparticles at high temperatures or under working conditions, which can be alleviated by anchoring metallic nanoparticles on conductive supports. Different methods have been used, including co-precipitation, chemical reduction, hydrothermal reduction, electrodeposition, dip coating, and sputtering. Conductive supports made of carbon materials are the most popular choice because of their low cost, high surface area, and good biocompatibility. They can be categorized based on their graphitic degrees. Partially graphitic carbon materials, such as carbon black, are widely used in commercial Pt/C electrocatalysts because of their low cost and high electrical conductivity.90 Carbon nanofibers prepared by carbonization of polymer precursors are also widely used as conductive supports for metal nanoparticles in the electrocatalysts used for H2O2 sensing.45,91–95 Fully graphitic carbons, such as CNTs63,96–101 and graphene,36,47,52,102–112 are frequently used as conductive supports due to their large surface area and high electrical conductivity. Furthermore, graphene quantum dots have also been used as conductive supports to anchor metallic nanoparticles for H2O2 sensing.113,114 One key advantage of nanocarbon supports such as CNTs is their excellent biocompatibility, due to which natural enzyme-based redox catalysts can be immobilized on them for biosensing. Zhang et al.115 loaded horseradish peroxidase enzymes on poly-L-lysine functionalized single-walled CNTs for amplified amperometric H2O2 sensing with a detection range of 1 μM to 10 mM. In another study,116 glucose oxidase was immobilized on CNT via a bridge of ionic liquid-like units for electrochemical biosensing. Beyond carbon supports, some atomically thin metal or non-metal-based layered materials have also been explored as conductive supports in electrocatalysts used for H2O2 sensing, including layered double hydroxides,117 metal dichalcogenides,118,119 MXene,120 or phosphorene.121
Macroscopic porous foams or aerogels are also popular as conductive supports, especially in fabricating freestanding electrodes. Porous carbon foams with hierarchal pore structures can effectively facilitate the mass transfer of electrolytes/analytes.122 Some metal foams have high electrical conductivity and show intrinsic catalytic activity for H2O2 sensing. Nanostructured electrocatalysts directly synthesized on metal foams have demonstrated some of the best performances in H2O2 sensing reported so far. For example, Huang et al. fabricated copper oxide (CuO) nanowire arrays on Cu foam using a facile two-step method involving alkaline oxidation of the Cu foam and subsequent low-temperature Cu(OH)2 thermolysis in the air at 190 °C, as illustrated in Fig. 6a.53 This electrocatalyst showed a high H2O2 sensitivity of 5750 μA mM−1 cm−2 when used as a freestanding electrode. The electrochemical impedance spectroscopy results revealed that its unique porous nanowire array structure enabled faster electron and mass transfers than planar CuO grown on the Cu foam. In another work, Cu(OH)2 nanowires were first grown by electrochemically anodizing Cu foam and then phosphidizing in an inert atmosphere at 300 °C to form pipet-like Cu3P nanowires with root and tip diameters of 300 and 100 nm, respectively (Fig. 6b).54 These Cu3P nanowires were intimately connected with the Cu foam support, achieving a sensing limit of 2 nM H2O2.
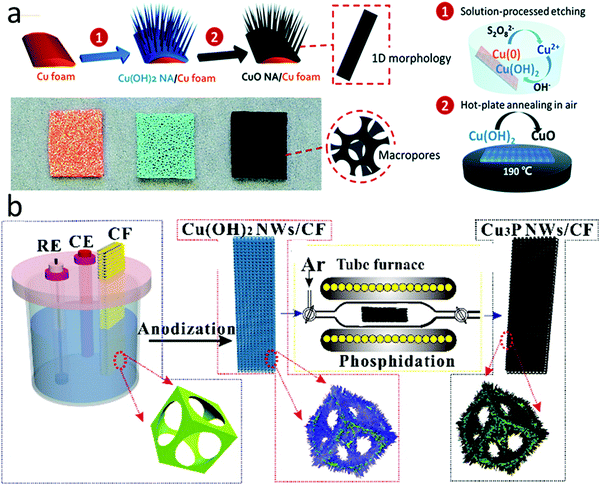 |
| Fig. 6 Nanostructured electrocatalysts synthesized on metal foams. (a) CuO nanowires grown on Cu foam. Reproduced with permission from ref. 53. Copyright 2015, Royal Society of Chemistry. (b) Cu3P nanowires grown on Cu foam. Reproduced with permission from ref. 54. Copyright 2016, American Chemical Society. | |
3.1.3. Reducing catalyst size.
Reducing the size of metal or metal composite nanoparticles/nanosheets/nanowires may expose more catalytically active catalyst sites, resulting in improved H2O2 sensing activity.123 Various methods, such as impregnation, co-precipitation, atomic layer deposition, or space confinement, have been utilized for this purpose.124,125 Depositing a low mass loading of metal nanoparticles on a large surface area support can alleviate the aggregation of single atoms or metal clusters into large particles. For example, Riaz et al. loaded 4.8 wt% Pt on a high surface area nanocarbon support, which achieved an H2O2 sensitivity higher than that of 20 wt% Pt/C.126 Li et al. anchored Co nanoparticles (10–50 nm) and atomic Co–Nx moieties on N doped CNT arrays by the pyrolysis of a sandwich-like urea@ZIF-67 complex.127 The electrocatalysts were deposited on SPEs and demonstrated high sensitivity and a low detection limit of 32.4 nM. Gao et al. synthesized electrocatalysts containing thiol-stabilized nanoclusters with sub-nanometer cores of 6 Cu atoms (0.37 nm in length and 0.27 nm in width). Due to their small size, the electrocatalyst showed a lower detection limit and a broader linear H2O2 sensing range than Cu2O nanoparticle-based electrocatalysts.128 Wang et al. obtained ultra-small MoS2 nanoparticles with a narrow size distribution (1–2 nm) by sonication of bulk MoS2 and density gradient centrifugation at 12000 rpm. Due to their large surface area and abundant edge sites, these nanoparticles showed a high H2O2 sensitivity of 2580 μA mM−1 cm−2 with a low detection limit of 2.5 nM.129 Shu et al. expanded the interlayer distance of MoS2 from 6.15 to 9.40 Å using excessive thiourea and a shorter hydrothermal reaction time, resulting in interlayer expanded MoS2 with exposed monolayer nanosheets. The interlayer expanded MoS2 showed much higher H2O2 sensing sensitivity (1706.0 μA mM−1 cm−2) and a low detection limit (0.2 μM) compared to non-expanded MoS2.130 Wang et al. synthesized ultrathin Au–Cu nanowires (∼4 nm diameter) (Fig. 7a), which achieved a low detection limit of 2.0 nM.131 Sun et al. synthesized atomic thick PtNi nanowires with an average diameter of 0.9 nm and an average length of 120 nm, and then assembled them on rGO nanosheets (Fig. 7b).132 The electrocatalyst showed high sensitivity in H2O2 sensing with a detection limit of 0.3 nM. Similarly, ultrathin Ag nanosheets (<10 nm) were also utilized for H2O2 sensing.133 A fast-growing strategy is to reduce the size of catalytic sites to single atoms, forming single atom catalysts. Metal–nitrogen–carbon (M–N–C) active sites are active for H2O2 oxidation and reduction. Some studies have synthesized M–N–C electrocatalysts by carbonizing zeolitic imidazolate frameworks for H2O2 sensing.134,135 They demonstrated excellent performances with low metal mass loadings.
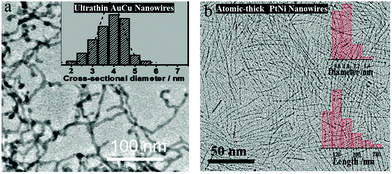 |
| Fig. 7 Ultra-small metal nanowires for H2O2 sensing. (a) Au–Cu nanowires and (b) Pt–Ni nanowires. Reproduced with permission from ref. 131 and 132. Copyright 2014 & 2017, American Chemical Society. | |
3.2. Increasing intrinsic activity
3.2.1. Alloying.
Alloys of multiple metals often show higher intrinsic activity than single metals due to the synergistic effect and optimum electronic structures in alloys. The synergistic effect means combining two metals resulting in an overall higher activity than the sum of individual metals used. In many cases, there is an excellent enhancement in their specific physical and chemical properties than in individual metals. For example, Guler et al.136 synthesized Pd@Ag alloy nanoparticles on rGO supports which showed a higher sensitivity 1307 μA mM−1 cm−2 than sensors using individual metal nanoparticles (966 μA mM−1 cm−2 for Pd/rGO/GC and 150 μA mM−1 cm−2 for Ag/rGO/GC).
Various binary136–139 and ternary140 alloys of noble metals and transition metal alloys110,141–143 have been explored as electrocatalysts for H2O2 sensing. Some alloy particles have unique structures, such as core–shell structures144,145 and yolk–shell structures,146 which may further boost the catalytic activity. Besides alloy nanoparticles, alloy nanowires132 and nanosheets147 of binary metals have also been explored for H2O2 sensing.
Nagaiah et al. alloyed Pd with a second noble metal (i.e., Au or Pt) by electrodeposition on graphite electrodes. The alloy electrocatalysts showed improved performances in H2O2 sensing. Visualization of local catalytic activity by scanning electrochemical microscopy revealed that the catalytic activity for H2O2 oxidation followed the trend: Pd50Pt50 > Pd70Pt30 > Pd > Pd90Pt10. A similar trend was also observed for Pd/Au alloys.147 In another study, bimetallic transition metal oxide (ZnCo2O4) nanoflowers of 5 μm in diameters were grown on Co3O4 nanowires of 100 nm in diameter.148 The unique morphology and synergic effects between two metal species enabled high sensitivity with a low detection limit of 1 nM.
3.2.2. Doping.
Doping elements, such as O, N, and S, into metals results in metal oxides,75,103,149,150 metal nitrides,151,152 and metal sulfides,153,154 respectively. Some of them demonstrated higher catalytic activities in H2O2 sensing than pure metal electrocatalysts. More studies have also studied doping these elements into carbon materials, resulting in metal-free carbon electrocatalysts. Extensive efforts have been devoted to understanding the catalytic activity of N-doped carbon electrocatalysts. It is generally believed that doped N atoms induce higher positive charges at surrounding C atoms, making these C sites more favorable for H2O2 oxidation/reduction.155,156 For example, DFT calculations showed that N atoms in graphene cause charge enhancement on adjacent C and H atoms. H2O2 physisorption on doped graphene requires less adsorption energy (−0.15 to −0.47 eV) than undoped graphene (−0.10 eV). N atoms may also provide better stabilization for H2O2 oxidation/reduction intermediates.155 N doped CNTs also showed higher activity in H2O2 sensing.156 For example, N doped CNTs displayed an anodic sensitivity of 830 mA M−1 cm−2 at 0.05 V, and a cathodic sensitivity of 270 mA M−1 cm−2 at −0.25 V, lowering the overpotential required for H2O2 oxidation and reduction.157 Some studies claimed that co-doping N and S into carbon materials by pyrolyzing molecules containing N and S atoms, such as thiadiazole and thiourea, can further improve the activity of carbon electrocatalysts in H2O2 sensing due to the synergistic effects between N and S.158,159
Another approach to obtain N-doped carbon electrocatalysts is to directly carbonize N-containing polymers, such as polyacrylonitrile (PAN) and polypyrrole (PPy). For example, pollack et al. synthesized an N-doped electrocatalyst by pyrolyzing electrospun PAN nanofibers at 1050 °C.160 X-ray photoelectron spectroscopy results suggested that pyridinic and graphitic N have higher activity for H2O2 reduction than other N species. In another study, Zhang et al. synthesized N-doped carbon nanoparticles embedded in CNFs by pyrolyzing electrospun PPy/PAN nanofibers, yielding a high sensitivity of 383.9 μA mM−1 cm−2 for H2O2 sensing.161
Furthermore, graphitic carbon nitride (g-C3N4) also shows high activity for H2O2 reduction. Gomez et al. synthesized g-C3N4 nanosheets for H2O2 sensing.162 Melamine powder was first thermally annealed at 550 °C in an inert atmosphere to yield yellow color crystals and then further sonicated in 0.5 M H2SO4 solution for 30 min to obtain exfoliated g-C3N4 nanosheets as electrocatalysts. Liu et al. studied the effect of protonation of g-C3N4 nanosheets on H2O2 sensing performance.163 g-C3N4 nanosheets were treated with concentrated HCl for 3 h at room temperature before they were exfoliated by ultrasonication in HNO3. DFT calculation results revealed that the protonation changed the band structure of g-C3N4 from semiconducting to conducting (no bandgap), leading to better electrical conductivity. Protonated g-C3N4 nanosheets showed a better H2O2 sensing performance.
3.2.3. Tuning crystal facets.
Different surface facets of nanocrystals may have different catalytic activities.164 Nanocrystals in different shapes have different proportions of surface facets, which can be controlled by nucleation via adjusting their experimental synthesis parameters, such as the concentration of precursors and temperature. Nuclei growth can occur either in thermodynamic or kinetic regimes, and the interplay of these two regimes strongly influence the final structure of nanocrystals. High index surface facets usually have higher catalytic activities than low-index facets because they have more kinks, atomic steps, edges, and low coordinated atoms as active sites. However, high index facets are often unstable due to their high surface energy. High index facets can be formed by fast reduction of precursors in stabilizing agents to direct nuclei growth in the kinetic regime. They can also be formed by facet replication of seeds or selective etching.165
Electrocatalysts with tuned crystal facets have been used for H2O2 sensing. For example, Luo et al. synthesized a Pt skin of high index facets on carbon-supported Pt3Co nanowires by annealing them in an inert atmosphere at 400 °C (Fig. 8a).166 The presence of the Pt skin with nearly two atomic layers of 0.5 nm led to a low sensing limit of 0.4 nM and a current density of −1.47 mA cm−2pt, which was 3 and 8 times higher than Pt3Co nanowires without high index facets and commercial Pt/C electrocatalysts, respectively. In another work, Pt–Cu octahedra were synthesized by introducing Cl ions into Co and Pt salt precursors. Pt–Cu octahedra with (111) facets showed a better H2O2 sensing performance than Pt–Cu spherical nanoparticles.167 Dai et al. synthesized Cu7S4 nanocrystals with high index facets using a solvothermal method, demonstrating a better H2O2 sensing performance than CuS spheres or flowers.168 Similarly, the Ni7S6 phase with a 3D flower-like structure showed a better H2O2 sensing performance than other phases, such as NiS, Ni3S4, and Ni9S8.169 Fe3O4 nanostructures with a stick shape and α-crystal phase showed better H2O2 sensing performances than other crystal phases and morphologies (Fig. 8b).170 Au nanoparticles decorated on pyramidal Si with (111) facets showed enhanced H2O2 sensor performances compared to that on planar Si with (100) facets.171 Zhang et al. synthesized CuO–CoO core–shell nanostructures on Cu foam using a chemical bath deposition method with sequential Cu and Co hydroxide formation, followed by calcination in an inert atmosphere.172 The CoO shell changed from nanosheets to nanoneedles when the calcination time increased from 2 to 3.5 h. CuO–CoO-2.5 h achieved a sensitivity of 6349 μA mM−1 cm−2 due to its unique leaf-like morphology. DFT calculations showed that the CoO(200) surface had better electron transport properties than the CoO(111) surface, irrespective of the core surfaces (CuO(111) or CuO(200)). A longer calcination time of 3 or 3.5 h resulted in less CoO(200) surfaces.
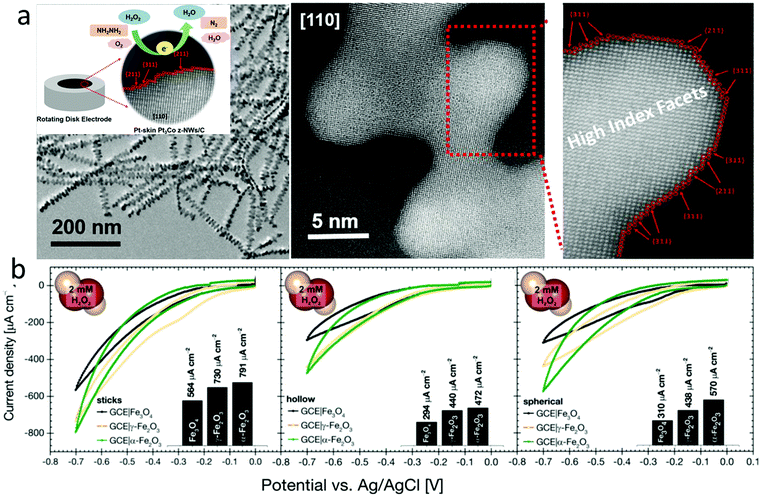 |
| Fig. 8 (a) Pt skin with high index facets enabled ultrasensitive H2O2 sensing. Reproduced with permission from ref. 166. Copyright 2018, American Chemical Society. (b) Comparison of Fe3O4 in different morphologies and crystal structures for H2O2 sensing. Reproduced with permission from ref. 170. Copyright 2018, Wiley-VCH Verlag GmbH & Co. | |
4. Summary and perspectives
We summarize the design strategies of electrodes and electrocatalysts for ultrasensitive electrochemical H2O2 sensors with detection limits down to the nanomole and sub-nanomole ranges, which is often encountered in cellular detection of H2O2. Both electrode structures and electrocatalysts are critical to the performance of electrochemical H2O2 sensors. Their designs are often intertwined together with electrocatalysts seamlessly integrated into electrode structures at the nanometer scale. Novel freestanding electrodes and SPEs are opening up more applications in portable on-site monitoring of H2O2. MEAs and NEAs have attracted significant interest in increasing sensitivity and providing multiplexed data. The fast development of electrocatalyst design strategies involving increasing the number of active sites and intrinsic activity at the nanoscale has improved the performance, as shown in Table 2. Based on the current research progress, we propose that the following areas should be priorities in upcoming research:
(1) Multiple electrocatalyst design strategies involving increasing the number of active sites and intrinsic activity should be seamlessly integrated to improve the electrocatalyst performance. Recent studies have demonstrated some success; for example, 3D nanoporous alloyed Pt–Fe clusters were anchored on an rGO support, which combined the strategies of nanoscale pore structuring, conducive support, reducing the catalyst size, and alloying.173 Appropriate combinations of the design strategies discussed in this review will likely lead to novel electrocatalysts with improved performance.
(2) Single atoms anchored in high surface area porous and conductive supports can provide well-defined and high density catalytic active sites. Recent studies have reported transition metal (e.g., Fe176 and Co177) SACs for H2O2 sensing. Pt is one the most active metals as electrocatalysts for H2O2 oxidation/reduction. Although Pt SACs have been used in other electrocatalytic applications,124,125 they have not been explored for H2O2 sensing. Pt SACs with a high Pt mass loading and good stability have excellent potential to replace currently used commercial electrocatalysts in electrochemical H2O2 sensors.
(3) Achieving durable sensing performances over a long-time operation requires stable electrocatalysts without fast degradation. The degradation of electrocatalysts has been studied for energy conversion processes and energy storage devices,126 but it is rarely discussed for electrocatalysts used in electrochemical H2O2 sensors. There is a clear gap in current research in this respect.
(4) Most H2O2 sensing studies reported in the literature have tested the performance in pure solutions or simple mixtures, which are very different from complex practical samples. For example, blood, food (e.g., milk and juice), and turbid water may contain various interferents, fats, proteins, and suspended particles. Testing the H2O2 sensor performance in practical samples would be the first step in understanding how the sensor performance changes in complex solution environments. Next, electrode fouling is expected to be a critical issue for sensing applications in practical samples. Designing electrocatalysts and electrodes with good antifouling properties will be required for many practical sensing applications.
(5) Due to the existence of electroactive interferents at a similar potential of H2O2 sensing, it is challenging to develop H2O2 sensors with high selectivity in practical samples. The current method is to avoid the potential window of potential interferents. This approach may not work in many practical samples. Some natural enzymes have high selectivity due to their unique 3D structures and catalytic binding sites. However, the stability of natural enzymes is often poor, and their active sites are often buried in complex protein structures with limited access.178 Developing artificial nano-enzymes that mimic selective catalytic active sites of natural enzymes with 3D porous nanoscale structures to improve their mass and electron transfer would be a promising research direction.179,180
(6) Continuous H2O2 concentration monitoring is required in some applications, such as tracking disinfectants or environmental pollutants in wastewater. Due to different contact modes between fluids and electrodes, the fluid flow patterns would strongly influence the H2O2 sensor performance. Thus, designing electrodes suitable for different fluid movement conditions is required. For example, flow-injection cells are often used to assess the practical utility of sensors,72 which can assist the development of suitable electrodes for electrochemical H2O2 sensors.
(7) H2O2 sensing in vapors is rarely reported in the literature. Many medical and industrial settings require detecting aerosolized H2O2 in the vapor phase (at ppm level) after the disinfection operation of buildings or rooms. We speculate that designing suitable electrodes to combine gas adsorption and sensing may help in the development of efficient electrochemical H2O2 sensors used in the vapor phase.
(8) Current commercial sensors for on-site H2O2 monitoring in air or water would cost hundreds of dollars (e.g., Steri-Trac area monitor: $1250, Gazdetect B12 detector: $1080, and Dräger XS sensor: $810). Simplifying sensor designs and lowering their manufacturing costs through nanoscale innovations in electrodes, electrocatalysts, and other components of electrochemical H2O2 sensors have the potential to yield more affordable sensors.
Conflicts of interest
There are no conflicts to declare.
Acknowledgements
This work is supported by the Australian Research Council under the Future Fellowships scheme (FT160100107).
References
- J. R. Stone and S. Yang, Antioxid. Redox Signaling, 2006, 8, 243–270 CrossRef CAS PubMed
.
- H. Sies, Redox Biol., 2017, 11, 613–619 CrossRef CAS PubMed
.
- H. J. Forman, A. Bernardo and K. J. Davies, Arch. Biochem. Biophys., 2016, 603, 48–53 CrossRef CAS PubMed
.
- J. W. Yuen and I. F. Benzie, Free Radical Res., 2003, 37, 1209–1213 CrossRef CAS PubMed
.
- M. P. Lisanti, U. E. Martinez-Outschoorn, Z. Lin, S. Pavlides, D. Whitaker-Menezes, R. G. Pestell, A. Howell and F. Sotgia, Cell Cycle, 2011, 10, 2440–2449 CrossRef CAS PubMed
.
- T. F. Langford, B. K. Huang, J. B. Lim, S. J. Moon and H. D. Sikes, Nat. Commun., 2018, 9, 3145 CrossRef PubMed
.
- D. Bruen, C. Delaney, L. Florea and D. Diamond, Sensors, 2017, 17, 1866 CrossRef PubMed
.
- D. Maier, E. Laubender, A. Basavanna, S. Schumann, F. Guder, G. A. Urban and C. Dincer, ACS Sens., 2019, 4, 2945–2951 CrossRef CAS PubMed
.
- S. Jankovskaja, A. Labrousse, L. Prevaud, B. Holmqvist, A. Brinte, J. Engblom, M. Rezeli, G. Marko-Varga and T. Ruzgas, Mikrochim. Acta, 2020, 187, 656 CrossRef CAS PubMed
.
- B. E. Watt, A. T. Proudfoot and J. A. Vale, Toxicol. Rev., 2004, 23, 51–57 CrossRef CAS PubMed
.
- H. P. Chan and H. I. Maibach, Cutaneous Ocul. Toxicol., 2008, 27, 307–309 CrossRef CAS PubMed
.
- S. M. Hendriksen, N. L. Menth, B. C. Westgard, J. B. Cole, J. W. Walter, T. C. Masters and C. J. Logue, Am. J. Emerg. Med., 2017, 35, 809.e805–809.e808 CrossRef PubMed
.
- M. Armogida, R. Nistico and N. B. Mercuri, Br. J. Pharmacol., 2012, 166, 1211–1224 CrossRef CAS PubMed
.
- P. Singh and N. Gandhi, Food Rev. Int., 2015, 31, 236–261 CrossRef CAS
.
- M. Goldberg, M. Grootveld and E. Lynch, Clin. Oral. Invest., 2010, 14, 1–10 CrossRef PubMed
.
- H. J. Sung, M. F. Khan and Y. H. Kim, Int. J. Biol. Macromol., 2019, 136, 20–26 CrossRef CAS PubMed
.
- M. L. Bianchi, R. Crisol and U. Schuchardt, Bioresour. Technol., 1999, 68, 17–21 CrossRef CAS
.
- M. Ksibi, Chem. Eng. J., 2006, 119, 161–165 CrossRef CAS
.
- S. Chen, X. Hai, X. W. Chen and J. H. Wang, Anal. Chem., 2014, 86, 6689–6694 CrossRef CAS PubMed
.
- Y. Gao, G. Wang, H. Huang, J. Hu, S. M. Shah and X. Su, Talanta, 2011, 85, 1075–1080 CrossRef CAS PubMed
.
- Y. Cui, F. Chen and X. B. Yin, Biosens. Bioelectron., 2019, 135, 208–215 CrossRef CAS PubMed
.
- L. Yang, N. Li, W. Pan, Z. Yu and B. Tang, Anal. Chem., 2015, 87, 3678–3684 CrossRef CAS PubMed
.
- S. Sakura, Anal. Chim. Acta, 1992, 262, 49–57 CrossRef CAS
.
- S. A. Kitte, W. Gao, Y. T. Zholudov, L. Qi, A. Nsabimana, Z. Liu and G. Xu, Anal. Chem., 2017, 89, 9864–9869 CrossRef CAS PubMed
.
- Y. Zheng, Z. Ning, D. Pan, D. Han, Y. Xu, S. Liu, Y. Zhang and Y. Shen, Sens. Actuators, B, 2020, 321, 128557 CrossRef CAS
.
- J. W. Park, S. J. Park, O. S. Kwon, C. Lee and J. Jang, Anal. Chem., 2014, 86, 1822–1828 CrossRef CAS PubMed
.
- Z. Yu, Y. Park, L. Chen, B. Zhao, Y. M. Jung and Q. Cong, ACS Appl. Mater. Interfaces, 2015, 7, 23472–23480 CrossRef CAS PubMed
.
- J. Matsui, K. Akamatsu, N. Hara, D. Miyoshi, H. Nawafune, K. Tamaki and N. Sugimoto, Anal. Chem., 2005, 77, 4282–4285 CrossRef CAS PubMed
.
- Y. Zhang and S. Tadigadapa, Biosens. Bioelectron., 2004, 19, 1733–1743 CrossRef CAS PubMed
.
- M. Dong, W. Zheng, Y. Chen, Y. Xianyu, B. Ran, Z. Qian and X. Jiang, Anal. Chem., 2018, 90, 9148–9155 CrossRef CAS PubMed
.
- F. Vianello, L. Zennaro and A. Rigo, Biosens. Bioelectron., 2007, 22, 2694–2699 CrossRef CAS PubMed
.
- M. Song, J. Wang, B. Chen and L. Wang, Anal. Chem., 2017, 89, 11537–11544 CrossRef CAS PubMed
.
- O. Tantawi, A. Baalbaki, R. El Asmar and A. Ghauch, Sci. Total Environ., 2019, 654, 107–117 CrossRef CAS PubMed
.
- L. Li, Y. Zhang, L. Zhang, S. Ge, H. Liu, N. Ren, M. Yan and J. Yu, Anal. Chem., 2016, 88, 5369–5377 CrossRef CAS PubMed
.
- L. Wang, W. Zhu, W. Lu, L. Shi, R. Wang, R. Pang, Y. Cao, F. Wang and X. Xu, Biosens. Bioelectron., 2019, 142, 111577 CrossRef CAS PubMed
.
- M. Nallal, G. Anantha Iyengar and K. Pill-Lee, ACS Appl. Mater. Interfaces, 2017, 9, 37166–37183 CrossRef CAS PubMed
.
- F. X. Hu, J. Miao, C. Guo, H. B. Yang and B. Liu, Chem. Eng. J., 2021, 407, 127203 CrossRef CAS
.
- C. Del Real Mata, R. Siavash Moakhar, I. I. Hosseini, M. Jalali and S. Mahshid, Nanoscale, 2021, 13, 14316–14329 RSC
.
- S. Zhao, M. Riedel, J. Patarroyo, N. Bastus, V. Puntes, Z. Yue, F. Lisdat and W. J. Parak, Nanoscale, 2021, 13, 980–990 RSC
.
- X. Guo, Q. Cao, Y. Liu, T. He, J. Liu, S. Huang, H. Tang and M. Ma, Anal. Chem., 2020, 92, 908–915 CrossRef CAS PubMed
.
- J. Duay, J. Elliott, J. B. Shear and K. J. Stevenson, Anal. Chem., 2015, 87, 10109–10116 CrossRef CAS PubMed
.
- J. N. Tiwari, V. Vij, K. C. Kemp and K. S. Kim, ACS Nano, 2016, 10, 46–80 CrossRef CAS PubMed
.
- A. Ghosale, K. Shrivas, R. Shankar and V. Ganesan, Anal. Chem., 2017, 89, 776–782 CrossRef CAS PubMed
.
- S. Du, Z. Ren, J. Wu, W. Xi and H. Fu, Nano Res., 2016, 9, 2260–2269 CrossRef CAS
.
- M. A. Riaz, Z. Yuan, A. Mahmood, F. Liu, X. Sui, J. Chen, Q. Huang, X. Liao, L. Wei and Y. Chen, Sens. Actuators, B, 2020, 319, 128243 CrossRef CAS
.
- X. Gao, S. DelaCruz, C. Zhu, S. Cheng, D. Gardner, Y. Xie, C. Carraro and R. Maboudian, Carbon, 2019, 148, 64–71 CrossRef CAS
.
- Y. Shim, M. H. Nam, S. W. Hyuk, S. Y. Yoon and J. M. Song, Anal. Chim. Acta, 2015, 853, 501–507 CrossRef CAS PubMed
.
- M. Zhang, A. Halder, C. Hou, J. Ulstrup and Q. Chi, Bioelectrochemistry, 2016, 109, 87–94 CrossRef CAS PubMed
.
- C. Cheng, C. Zhang, X. Gao, Z. Zhuang, C. Du and W. Chen, Anal. Chem., 2018, 90, 1983–1991 CrossRef CAS PubMed
.
- Y. Yuan, F. Zhang, H. Wang, J. Liu, Y. Zheng and S. Hou, RSC Adv., 2017, 7, 30542–30547 RSC
.
- Y. Yuan, Y. Zheng, J. Liu, H. Wang and S. Hou, Microchim. Acta, 2017, 184, 4723–4729 CrossRef CAS
.
- Y. Sun, K. He, Z. Zhang, A. Zhou and H. Duan, Biosens. Bioelectron., 2015, 68, 358–364 CrossRef CAS PubMed
.
- J. Huang, H. Li, Y. Zhu, Q. Cheng, X. Yang and C. Li, J. Mater. Chem. A, 2015, 3, 8734–8741 RSC
.
- Z. Li, Y. Xin, W. Wu, B. Fu and Z. Zhang, Anal. Chem., 2016, 88, 7724–7729 CrossRef CAS PubMed
.
- X. Lu, X. Xiao, Z. Li, F. Xu, H. Tan, L. Sun and L. Wang, Anal. Methods, 2014, 6, 235–241 RSC
.
- X. Cao, Z. Zeng, W. Shi, P. Yep, Q. Yan and H. Zhang, Small, 2013, 9, 1703–1707 CrossRef CAS PubMed
.
- C. C. Kung, P. Y. Lin, F. J. Buse, Y. Xue, X. Yu, L. Dai and C. C. Liu, Biosens. Bioelectron., 2014, 52, 1–7 CrossRef CAS PubMed
.
- L. Chen, X. Wang, X. Zhang and H. Zhang, J. Mater. Chem., 2012, 22, 22090 RSC
.
- M. Yang, C. Zhang, Q. Lv, G. Sun, C. Bi, S. Guo, H. Dong and L. Liu, ACS Appl. Mater. Interfaces, 2020, 12, 934–944 CrossRef CAS PubMed
.
- A. L. Sanford, S. W. Morton, K. L. Whitehouse, H. M. Oara, L. Z. Lugo-Morales, J. G. Roberts and L. A. Sombers, Anal. Chem., 2010, 5205–5210 CrossRef CAS PubMed
.
- R. Li, X. Liu, W. Qiu and M. Zhang, Anal. Chem., 2016, 88, 7769–7776 CrossRef CAS PubMed
.
- H. Li, H. Zhao, H. He, L. Shi, X. Cai and M. Lan, Sens. Actuators, B, 2018, 260, 174–182 CrossRef CAS
.
- Y. Zhang, J. Xiao, Y. Sun, L. Wang, X. Dong, J. Ren, W. He and F. Xiao, Biosens. Bioelectron., 2018, 100, 453–461 CrossRef CAS PubMed
.
- L. R. Wilson, S. Panda, A. C. Schmidt and L. A. Sombers, Anal. Chem., 2018, 90, 888–895 CrossRef CAS PubMed
.
- D. W. Zhang, J. X. Liu, J. Nie, Y. L. Zhou and X. X. Zhang, Anal. Chem., 2013, 85, 2032–2036 CrossRef CAS PubMed
.
- Q. Zhai, Y. Wang, S. Gong, Y. Ling, L. W. Yap, Y. Liu, J. Wang, G. P. Simon and W. Cheng, Anal. Chem., 2018, 90, 13498–13505 CrossRef CAS PubMed
.
- J. X. Zhou, L. N. Tang, F. Yang, F. X. Liang, H. Wang, Y. T. Li and G. J. Zhang, Analyst, 2017, 142, 4322–4329 RSC
.
- Y. Peng, D. Lin, J. Justin Gooding, Y. Xue and L. Dai, Carbon, 2018, 136, 329–336 CrossRef CAS
.
- H. Yang, T. Rahman, D. Du, R. Panat and Y. Lin, Sens. Actuators, B, 2016, 230, 600–606 CrossRef CAS PubMed
.
- Y. Sun, L. Hang, D. Men, H. Li, D. Liu, X. Li, L. Wen and Y. Li, J. Mater. Chem. C, 2016, 4, 9864–9871 RSC
.
- S. Z. Bas, C. Cummins, D. Borah, M. Ozmen and M. A. Morris, Anal. Chem., 2018, 90, 1122–1128 CrossRef CAS PubMed
.
- B. J. Brownlee, K. M. Marr, J. C. Claussen and B. D. Iverson, Sens. Actuators, B, 2017, 246, 20–28 CrossRef CAS
.
- L. B. Shi, X. H. Niu, T. T. Liu, H. L. Zhao and M. B. Lan, Microchim. Acta, 2015, 182, 2485–2493 CrossRef CAS
.
- Q. Zhu, B. Liang, Y. Cai, Q. Cao, T. Tu, B. Huang, L. Fang and X. Ye, Talanta, 2018, 190, 70–77 CrossRef CAS PubMed
.
- S. Kogularasu, M. Govindasamy, S.-M. Chen, M. Akilarasan and V. Mani, Sens. Actuators, B, 2017, 253, 773–783 CrossRef CAS
.
- F. Arduini, C. Zanardi, S. Cinti, F. Terzi, D. Moscone, G. Palleschi and R. Seeber, Sens. Actuators, B, 2015, 212, 536–543 CrossRef CAS
.
- H. Y. Nyein, W. Gao, Z. Shahpar, S. Emaminejad, S. Challa, K. Chen, H. M. Fahad, L. C. Tai, H. Ota, R. W. Davis and A. Javey, ACS Nano, 2016, 10, 7216–7224 CrossRef CAS PubMed
.
- W. Gao, S. Emaminejad, H. Y. Y. Nyein, S. Challa, K. Chen, A. Peck, H. M. Fahad, H. Ota, H. Shiraki, D. Kiriya, D. H. Lien, G. A. Brooks, R. W. Davis and A. Javey, Nature, 2016, 529, 509–514 CrossRef CAS PubMed
.
- R. K. Mishra, L. J. Hubble, A. Martin, R. Kumar, A. Barfidokht, J. Kim, M. M. Musameh, I. L. Kyratzis and J. Wang, ACS
Sens., 2017, 2, 553–561 CrossRef CAS PubMed
.
- J. R. Sempionatto, R. K. Mishra, A. Martin, G. Tang, T. Nakagawa, X. Lu, A. S. Campbell, K. M. Lyu and J. Wang, ACS Sens., 2017, 2, 1531–1538 CrossRef CAS PubMed
.
- M. Santhiago, C. C. Correa, J. S. Bernardes, M. P. Pereira, L. J. M. Oliveira, M. Strauss and C. C. B. Bufon, ACS Appl. Mater. Interfaces, 2017, 9, 24365–24372 CrossRef CAS PubMed
.
- F. Girard-Sahun, V. Badets, P. Lefrancois, N. Sojic, F. Clement and S. Arbault, Anal. Chem., 2019, 91, 8002–8007 CrossRef CAS PubMed
.
- E. Atci, J. T. Babauta and H. Beyenal, Sens. Actuators, B, 2016, 226, 429–435 CrossRef CAS
.
- C. S. Santos, R. Bannitz-Fernandes, A. S. Lima, C. A. Tairum, I. Malavazi, L. E. S. Netto and M. Bertotti, Anal. Chem., 2018, 90, 2587–2593 CrossRef CAS PubMed
.
- J. Qi, W. Zhang and R. Cao, ChemCatChem, 2018, 10, 1206–1220 CrossRef CAS
.
- L. Zhang, L. Jin, B. Liu and J. He, Front. Chem., 2019, 7, 22 CrossRef CAS PubMed
.
- L. Yao, Y. Yan and J.-M. Lee, ACS Sustainable Chem. Eng., 2017, 5, 1248–1252 CrossRef CAS
.
- N. Lu, T. Zhang, X. Yan, Y. Gu, H. Liu, Z. Xu, H. Xu, X. Li, Z. Zhang and M. Yang, Nanoscale, 2018, 10, 14923–14930 RSC
.
- S. R. Das, Q. Nian, A. A. Cargill, J. A. Hondred, S. Ding, M. Saei, G. J. Cheng and J. C. Claussen, Nanoscale, 2016, 8, 15870–15879 RSC
.
- Y. Liu, H. Li, S. Gong, Y. Chen, R. Xie, Q. Wu, J. Tao, F. Meng and P. Zhao, Sens. Actuators, B, 2019, 290, 249–257 CrossRef CAS
.
- N. Isoaho, S. Sainio, N. Wester, L. Botello, L.-S. Johansson, E. Peltola, V. Climent, J. M. Feliu, J. Koskinen and T. Laurila, RSC Adv., 2018, 8, 12742–12751 RSC
.
- Y. Ni, Y. Liao, M. Zheng and S. Shao, Microchim. Acta, 2017, 184, 3689–3695 CrossRef CAS
.
- T. Laurila, S. Sainio, H. Jiang, N. Isoaho, J. E. Koehne, J. Etula, J. Koskinen and M. Meyyappan, ACS Omega, 2017, 2, 496–507 CrossRef CAS PubMed
.
- Y. Yang, R. Z. Fu, J. J. Yuan, S. Y. Wu, J. L. Zhang and H. Y. Wang, Microchim. Acta, 2015, 182, 2241–2249 CrossRef CAS
.
- H. Guan, Y. Zhao, J. Zhang, Y. Liu, S. Yuan and B. Zhang, Sens. Actuators, B, 2018, 261, 354–363 CrossRef CAS
.
- H. C. Kazici, F. Salman, A. Caglar, H. Kivrak and N. Aktas, Fullerenes, Nanotubes, Carbon Nanostruct., 2018, 26, 145–151 CrossRef CAS
.
- L. Venosta, M. V. Bracamonte, M. C. Rodríguez, S. E. Jacobo and P. G. Bercoff, Sens. Actuators, B, 2017, 248, 460–469 CrossRef CAS
.
- J.-X. Liu and S.-N. Ding, Sens. Actuators, B, 2017, 251, 200–207 CrossRef CAS
.
- V. S. Joshi, J. Kreth and D. Koley, Anal. Chem., 2017, 89, 7709–7718 CrossRef CAS PubMed
.
- B. Huang, Y. Wang, Z. Lu, H. Du and J. Ye, Sens. Actuators, B, 2017, 252, 1016–1025 CrossRef CAS
.
- J. Anojčić, V. Guzsvány, O. Vajdle, D. Madarász, A. Rónavári, Z. Kónya and K. Kalcher, Sens. Actuators, B, 2016, 233, 83–92 CrossRef
.
- W. Wang, H. Tang, Y. Wu, Y. Zhang and Z. Li, Biosens. Bioelectron., 2019, 132, 217–223 CrossRef CAS PubMed
.
- B. Li, H.-Y. Song, Z.-P. Deng, L.-H. Huo and S. Gao, Sens. Actuators, B, 2019, 288, 641–648 CrossRef CAS
.
- T. N. N. Dau, V. H. Vu, T. T. Cao, V. C. Nguyen, C. T. Ly, D. L. Tran, T. T. N. Pham, N. T. Loc, B. Piro and T. T. Vu, Sens. Actuators, B, 2019, 283, 52–60 CrossRef CAS
.
- X. Yang, Y. Ouyang, F. Wu, Y. Hu, Y. Ji and Z. Wu, Sens. Actuators, B, 2017, 238, 40–47 CrossRef CAS
.
- M. Sookhakian, E. Zalnezhad and Y. Alias, Sens. Actuators, B, 2017, 241, 1–7 CrossRef CAS
.
- Z. Li, W. Wang, H. Cao, Q. Zhang, X. Zhou, D. Wang, Y. Wang, S. Zhang, G. Zhang, C. Liu, Y. Zhang, R. Liu and J. Jiang, Adv. Mater. Interfaces, 2017, 2, 1700224 Search PubMed
.
- B. Amanulla, S. Palanisamy, S. M. Chen, V. Velusamy, T. W. Chiu, T. W. Chen and S. K. Ramaraj, J. Colloid Interface Sci., 2017, 487, 370–377 CrossRef CAS PubMed
.
- T. D. Thanh, J. Balamurugan, S. H. Lee, N. H. Kim and J. H. Lee, Biosens. Bioelectron., 2016, 85, 669–678 CrossRef CAS PubMed
.
- L. Wang, Y. Zhang, C. Cheng, X. Liu, H. Jiang and X. Wang, ACS Appl. Mater. Interfaces, 2015, 7, 18441–18449 CrossRef CAS PubMed
.
- H. Wang, Y. Bu, W. Dai, K. Li, H. Wang and X. Zuo, Sens. Actuators, B, 2015, 216, 298–306 CrossRef CAS
.
- V. Mani, R. Devasenathipathy, S.-M. Chen, S.-F. Wang, P. Devi and Y. Tai, Electrochim. Acta, 2015, 176, 804–810 CrossRef CAS
.
- J. Ju and W. Chen, Anal. Chem., 2015, 87, 1903–1910 CrossRef CAS PubMed
.
- J. Xi, C. Xie, Y. Zhang, L. Wang, J. Xiao, X. Duan, J. Ren, F. Xiao and S. Wang, ACS Appl. Mater. Interfaces, 2016, 8, 22563–22573 CrossRef CAS PubMed
.
- Y. Zhang, J. Li, Y. Shen, M. Wang and J. Li, J. Phys. Chem. B, 2004, 108, 15343–15346 CrossRef CAS
.
- Y. Zhang, Y. Shen, D. Han, Z. Wang, J. Song, F. Li and L. Niu, Biosens. Bioelectron., 2007, 23, 438–443 CrossRef PubMed
.
- H. Farhat, C. Taviot-Gueho, G. Monier, V. Briois, C. Forano and C. Mousty, J. Phys. Chem. C, 2020, 124, 15585–15599 CrossRef CAS
.
- B. Habibi, F. F. Azhar, J. Fakkar and Z. Rezvani, Anal. Methods, 2017, 9, 1956–1964 RSC
.
- A. Aziz, M. Asif, G. Ashraf, M. Azeem, I. Majeed, M. Ajmal, J. Wang and H. Liu, Microchim. Acta, 2019, 186, 671 CrossRef CAS PubMed
.
- L. Lorencova, T. Bertok, J. Filip, M. Jerigova, D. Velic, P. Kasak, K. A. Mahmoud and J. Tkac, Sens. Actuators, B, 2018, 263, 360–368 CrossRef CAS
.
- S. Yan, B. Wang, Z. Wang, D. Hu, X. Xu, J. Wang and Y. Shi, Biosens. Bioelectron., 2016, 80, 34–38 CrossRef CAS PubMed
.
- S. Dong, A. Q. Dao, B. Zheng, Z. Tan, C. Fu, H. Liu and F. Xiao, Electrochim. Acta, 2015, 152, 195–201 CrossRef CAS
.
- E. Mazzotta, T. Di Giulio, V. Mastronardi, P. P. Pompa, M. Moglianetti and C. Malitesta, ACS Appl. Nano Mater., 2021, 4, 7650–7662 CrossRef CAS
.
- X. F. Yang, A. Wang, B. Qiao, J. Li, J. Liu and T. Zhang, Acc. Chem. Res., 2013, 46, 1740–1748 CrossRef CAS PubMed
.
- L. Zhang, K. Doyle-Davis and X. Sun, Energy Environ. Sci., 2019, 12, 492–517 RSC
.
- M. A. Riaz, S. Zhai, L. Wei, Z. Zhou, Z. Yuan, Y. Wang, Q. Huang, X. Liao and Y. Chen, Sens. Actuators, B, 2019, 283, 304–311 CrossRef CAS
.
- Z. Li, R. Liu, C. Tang, Z. Wang, X. Chen, Y. Jiang, C. Wang, Y. Yuan, W. Wang, D. Wang, S. Chen, X. Zhang, Q. Zhang and J. Jiang, Small, 2019, 1902860, DOI:10.1002/smll.201902860,
.
- X. Gao, S. He, C. Zhang, C. Du, X. Chen, W. Xing, S. Chen, A. Clayborne and W. Chen, Adv. Sci., 2016, 3, 1600126 CrossRef PubMed
.
- T. Wang, H. Zhu, J. Zhuo, Z. Zhu, P. Papakonstantinou, G. Lubarsky, J. Lin and M. Li, Anal. Chem., 2013, 85, 10289–10295 CrossRef CAS PubMed
.
- Y. Shu, W. Zhang, H. Cai, Y. Yang, X. Yu and Q. Gao, Nanoscale, 2019, 11, 6644–6653 RSC
.
- N. Wang, Y. Han, Y. Xu, C. Gao and X. Cao, Anal. Chem., 2015, 87, 457–463 CrossRef CAS PubMed
.
- Y. Sun, M. Luo, Y. Qin, S. Zhu, Y. Li, N. Xu, X. Meng, Q. Ren, L. Wang and S. Guo, ACS Appl. Mater. Interfaces, 2017, 9, 34715–34721 CrossRef CAS PubMed
.
- B. Ma, C. Kong, X. Hu, K. Liu, Q. Huang, J. Lv, W. Lu, X. Zhang, Z. Yang and S. Yang, Biosens. Bioelectron., 2018, 106, 29–36 CrossRef CAS PubMed
.
- Z. Wu, L.-P. Sun, Z. Zhou, Q. Li, L.-H. Huo and H. Zhao, Sens. Actuators, B, 2018, 276, 142–149 CrossRef CAS
.
- Z. Li, Y. Jiang, C. Liu, Z. Wang, Z. Cao, Y. Yuan, M. Li, Y. Wang, D. Fang, Z. Guo, D. Wang, G. Zhang and J. Jiang, Environ. Sci.: Nano, 2018, 5, 1834–1843 RSC
.
- M. Guler, V. Turkoglu, A. Bulut and M. Zahmakiran, Electrochim. Acta, 2018, 263, 118–126 CrossRef CAS
.
- Z. Bai, W. Dong, Y. Ren, C. Zhang and Q. Chen, Langmuir, 2018, 34, 2235–2244 CrossRef CAS PubMed
.
- X. Cui, S. Wu, Y. Li and G. Wan, Microchim. Acta, 2014, 182, 265–272 CrossRef
.
- C. Zhang, Y. Zhang, X. Du, Y. Chen, W. Dong, B. Han and Q. Chen, Talanta, 2016, 159, 280–286 CrossRef CAS PubMed
.
- W. Dong, Y. Ren, Z. Bai, Y. Yang, Z. Wang, C. Zhang and Q. Chen, Talanta, 2018, 189, 79–85 CrossRef CAS PubMed
.
- Y. Sun, M. Luo, X. Meng, J. Xiang, L. Wang, Q. Ren and S. Guo, Anal. Chem., 2017, 89, 3761–3767 CrossRef CAS PubMed
.
- J. Li, J. Jiang, Z. Xu, M. Liu, S. Tang, C. Yang and D. Qian, Sens. Actuators, B, 2018, 260, 529–540 CrossRef CAS
.
- M. Hassan, Y. Jiang, X. Bo and M. Zhou, Talanta, 2018, 188, 339–348 CrossRef CAS PubMed
.
- M. Asif, H. Liu, A. Aziz, H. Wang, Z. Wang, M. Ajmal, F. Xiao and H. Liu, Biosens. Bioelectron., 2017, 97, 352–359 CrossRef CAS PubMed
.
- H. Mei, W. Wu, B. Yu, H. Wu, S. Wang and Q. Xia, Sens. Actuators, B, 2016, 223, 68–75 CrossRef CAS
.
- L. Zhou, L. Kuai, W. Li and B. Geng, ACS Appl. Mater. Interfaces, 2012, 4, 6463–6467 CrossRef CAS PubMed
.
- T. C. Nagaiah, D. Schafer, W. Schuhmann and N. Dimcheva, Anal. Chem., 2013, 85, 7897–7903 CrossRef CAS PubMed
.
- V. Mani, S. Selvaraj, T.-K. Peng, H.-Y. Lin, N. Jeromiyas, H. Ikeda, Y. Hayakawa, S. Ponnusamy, C. Muthamizhchelvan and S.-T. Huang, ACS Appl. Nano Mater., 2019, 2, 5049–5060 CrossRef CAS
.
- Z.-L. Wu, C.-K. Li, J.-G. Yu and X.-Q. Chen, Sens. Actuators, B, 2017, 239, 544–552 CrossRef CAS
.
- M. Yang, D. S. Kim, T. J. Lee, S. J. Lee, K. G. Lee and B. G. Choi, J. Colloid Interface Sci., 2016, 468, 51–56 CrossRef CAS PubMed
.
- D. Yin, X. Bo, J. Liu and L. Guo, Anal. Chim. Acta, 2018, 1038, 11–20 CrossRef CAS PubMed
.
- T. Deepalakshmi, D. T. Tran, N. H. Kim, K. T. Chong and J. H. Lee, ACS Appl. Mater. Interfaces, 2018, 10, 35847–35858 CrossRef CAS PubMed
.
- M. Wang, J. Ma, X. Guan, W. Peng, X. Fan, G. Zhang, F. Zhang and Y. Li, J. Alloys Compd., 2019, 784, 827–833 CrossRef CAS
.
- A. Ebrahimi, K. Zhang, C. Dong, S. Subramanian, D. Butler, A. Bolotsky, L. Goodnight, Y. Cheng and J. A. Robinson, Sens. Actuators, B, 2019, 285, 631–638 CrossRef CAS
.
- P. Wu, P. Du, H. Zhang and C. Cai, Phys. Chem. Chem. Phys., 2013, 15, 6920–6928 RSC
.
- X. Xu, S. Jiang, Z. Hu and S. Liu, ACS Nano, 2010, 4, 4292–4298 CrossRef CAS PubMed
.
- J. M. Goran, E. N. Phan, C. A. Favela and K. J. Stevenson, Anal. Chem., 2015, 87, 5989–5996 CrossRef CAS PubMed
.
- T. Zhang, C. Li, Y. Gu, X. Yan, B. Zheng, Y. Li, H. Liu, N. Lu, Z. Zhang and G. Feng, Talanta, 2017, 165, 143–151 CrossRef CAS PubMed
.
- T. Zhang, Y. Gu, C. Li, X. Yan, N. Lu, H. Liu, Z. Zhang and H. Zhang, ACS Appl. Mater. Interfaces, 2017, 9, 37991–37999 CrossRef CAS PubMed
.
- B. Pollack, S. Holmberg, D. George, I. Tran, M. Madou and M. Ghazinejad, Sensors, 2017, 17, 2407 CrossRef PubMed
.
- X. Zhang, D. Liu, B. Yu and T. You, Sens. Actuators, B, 2016, 224, 103–109 CrossRef CAS
.
- C. G. Gomez, A. M. Silva, M. C. Strumia, L. B. Avalle and M. I. Rojas, Nanoscale, 2017, 9, 11170–11179 RSC
.
- L. Liu, H. Lv, C. Wang, Z. Ao and G. Wang, Electrochim. Acta, 2016, 206, 259–269 CrossRef CAS
.
- F. A. C. Pastrián, A. G. M. da Silva, A. H. B. Dourado, A. P. de Lima Batista, A. G. S. de Oliveira-Filho, J. Quiroz, D. C. de Oliveira, P. H. C. Camargo and S. I. Córdoba de Torresi, ACS Catal., 2018, 8, 6265–6272 CrossRef
.
- A. R. Poerwoprajitno, L. Gloag, S. Cheong, J. J. Gooding and R. D. Tilley, Nanoscale, 2019, 11, 18995–19011 RSC
.
- M. Luo, Y. Sun, Y. Qin, S. Chen, Y. Li, C. Li, Y. Yang, D. Wu, N. Xu, Y. Xing, L. Wang, P. Gao and S. Guo, Chem. Mater., 2018, 30, 6660–6667 CrossRef CAS
.
- T. Bian, H. Liu, B. Sun, B. Xiao, Y. Jiang, C. Jin, A. Yuan, H. Zhang and D. Yang, J. Alloys Compd., 2019, 788, 1334–1340 CrossRef CAS
.
- Y. Dai, X. Zhu, H. Liu, Y. Lin, W. Sun, Y. Sun, C. Ding, C. Luo and Q. Wei, Biosens. Bioelectron., 2018, 112, 143–148 CrossRef CAS PubMed
.
- W. Wu, Y. Li, J. Jin, H. Wu, S. Wang and Q. Xia, Sens. Actuators, B, 2016, 232, 633–641 CrossRef CAS
.
- P. Jakubec, O. Malina, J. Tuček, I. Medřík, Z. Medříková, P. Slovák, J. Kašlík and R. Zbořil, Adv. Mater. Interfaces, 2018, 1801549, DOI:10.1002/admi.201801549,
.
- C.-W. Huang, J. A. A. Valinton, Y. Hung, Jr. and C.-H. Chen, Sens. Actuators, B, 2018, 266, 463–471 CrossRef CAS
.
- W. Zhang, G. Fan, H. Yi, G. Jia, Z. Li, C. Yuan, Y. Bai and D. Fu, Small, 2018, 14, 1703713 CrossRef PubMed
.
- F. Lin, Y. Sun, J. Lai, K. Wang, Y. Tang, Y. Chao, Y. Yang, J. Feng, F. Lv, P. Zhou, M. Huang and S. Guo, Small Methods, 2018, 2, 1800073 CrossRef
.
- D. Zhou, X. Cao, Z. Wang, S. Hao, X. Hou, F. Qu, G. Du, A. M. Asiri, C. Zheng and X. Sun, Chem. – Eur. J., 2017, 23, 5214–5218 CrossRef CAS PubMed
.
- X. Wu, F. Li, C. Zhao and X. Qian, Sens. Actuators, B, 2018, 274, 163–171 CrossRef CAS
.
- S. Ding, Z. Lyu, L. Fang, T. Li, W. Zhu, S. Li, X. Li, J. C. Li, D. Du and Y. Lin, Small, 2021, 17, 2100664 CrossRef CAS PubMed
.
- Y. Shu, Z. Li, Y. Yang, J. Tan, Z. Liu, Y. Shi, C. Ye and Q. Gao, ACS Appl. Nano Mater., 2021, 4, 7954–7962 CrossRef CAS
.
- J. Masa and W. Schuhmann, Nano Energy, 2016, 29, 466–475 CrossRef CAS
.
- T. M. Benedetti, C. Andronescu, S. Cheong, P. Wilde, J. Wordsworth, M. Kientz, R. D. Tilley, W. Schuhmann and J. J. Gooding, J. Am. Chem. Soc., 2018, 140, 13449–13455 CrossRef CAS PubMed
.
- P. B. O'Mara, P. Wilde, T. M. Benedetti, C. Andronescu, S. Cheong, J. J. Gooding, R. D. Tilley and W. Schuhmann, J. Am. Chem. Soc., 2019, 141, 14093–14097 CrossRef PubMed
.
|
This journal is © The Royal Society of Chemistry 2022 |
Click here to see how this site uses Cookies. View our privacy policy here.