DOI:
10.1039/D2NR02537J
(Review Article)
Nanoscale, 2022,
14, 15964-16002
Nanoarchitectonics beyond perfect order – not quite perfect but quite useful
Received
9th May 2022
, Accepted 25th September 2022
First published on 26th September 2022
Abstract
Nanoarchitectonics, like architectonics, allows the design and building of structures, but at the nanoscale. Unlike those in architectonics, and even macro-, micro-, and atomic-scale architectonics, the assembled structures at the nanoscale do not always follow the projected design. In fact, they do follow the projected design but only for self-assembly processes producing structures with perfect order. Here, we look at nanoarchitectonics allowing the building of nanostructures without a perfect arrangement of building blocks. Here, fabrication of structures from molecules, polymers, nanoparticles, and nanosheets to polymer brushes, layer-by-layer assembly structures, and hydrogels through self-assembly processes is discussed, where perfect order is not necessarily the aim to be achieved. Both planar substrate and spherical template-based assemblies are discussed, showing the challenging nature of research in this field and the usefulness of such structures for numerous applications, which are also discussed here.
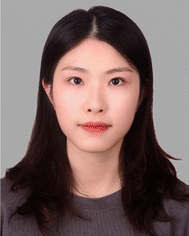 Lin Cao | Lin Cao received her M.S. degree in Food Science and Engineering from the National Engineering Research Center of Seafood, Dalian Polytechnic University, China. She is currently pursuing a Ph.D. degree in the Biotechnology faculty at Ghent University, Belgium. Her research interests include the synthesis of nanomaterials and organic-inorganic hybrid hydrogels with applications in biology and food science including functional compound target delivery, drug delivery, and tissue engineering. |
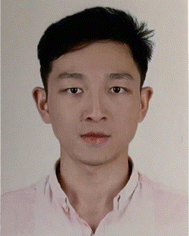 Yanqi Huang | Yanqi Huang received his M.S. degree in Powder Metallurgy Research from Central South University, China. He is currently pursuing his Ph.D. degree in the Biotechnology faculty at Ghent University, Belgium. His research interests include the synthesis of nano-inorganic materials and hydrogels with applications in biology including drug delivery systems and tissue engineering. |
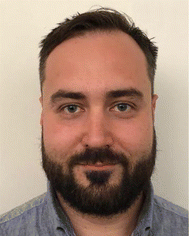 Bogdan Parakhonskiy | Bogdan Parakhonskiy received his Ph.D. from Lomonosov Moscow State University, Russia in 2009. He held a Marie Curie Postdoc fellowship at the University of Trento, Italy. After this, he worked as a group leader in the theranostic laboratory at Saratov State University, Russia. Since 2015 he has been the holder of a prestigious FWO (Flanders Research Foundation) fellowship at Ghent University, Belgium. His research interests focus on the design and modification of composite scaffolds and colloidal particles, biomineralization, and drug delivery. He is a pioneer in the development of carriers such as calcium carbonate particles, hydrogel matrices, and capsules. |
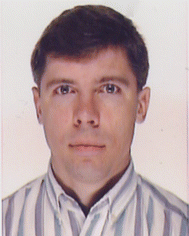 Andre G. Skirtach | Andre Skirtach was born in Ukraine and received an MSc degree in Physics from Lomonosov Moscow State University, Russia, and a Ph.D. degree in Chemistry from McGill University, Canada. Subsequently, he joined the National Research Council of Canada (NRC) in Ottawa. In 2000, Dr Skirtach and colleagues were involved in establishing a prominent startup—Trillium Phot., Inc. He then moved to the Max Planck Institute of Colloids and Interfaces, Golm, Germany, first working as a researcher and then as a Group Leader. In 2011, Dr Skirtach joined the Faculty of Bioscience Engineering, Ghent University, Belgium. His scientific research interests include nanotechnology and nanobiotechnology, cell biology, cell death and mechanobiology, development of microscopy and analytical methods, nanoparticles, polymeric capsules and planar interfaces, self-assembly and its applications. |
1. Introduction
While it exists at different size scales, such as macro-, micro-, and atomic or sub-nano, architectonics at the nanoscale, or nanoarchitectonics, is the focus of our analysis here. Historically, the early roots of architectonics can be linked to Aristotle, who put practical craftsmanship and the concept of technē in contrast to the more abstract thoughts of his teacher, Plato.1 Later on, architectonics can be traced to Kant who, however, developed its more abstract perception, in contrast to the directly related terms “man the knower”, “man the doer”, and “man the maker” of Aristotle. Even cellular sub-structures, composition, and anatomy have been linked to the term architectonics, albeit as cytoarchitectonics.2 In biomedicine, the term architectonics can have specificity, depending on the application area: for example, it was linked to microscopic and larger-scale organs in the neurosciences.3 Furthermore, architectonics can be linked to the construction and design of buildings. The sheer scale of building blocks in architecture enables the construction of buildings exactly according to projected design, and the same holds true for micro-architectonics due to the sizes of building blocks and advances in the microstructural sciences, particularly in the last century when microstructural sciences were quickly developing. Curiously, and as was insightfully reported by Ariga (NIMS, Japan) and co-workers, similar correspondence exists both at the micro- and atomic scales, where in the former case the geometrical factors eliminate the influence of molecular forces, while at the atomic scale, the interaction between atoms leads to a specific lattice arrangement or molecular structure.4 This does not necessarily hold true at the nanoscale due to molecular forces acting on the building blocks, predominantly molecules and nanoparticles. The attractiveness of applications in energy,5,6 environmental,7,8 and biomedical issues9,10 propelling development in the area of nanoarchitectonics is discussed complementary to earlier description.11
Fabricated nanomaterials often exhibit unique physical and chemical properties due to their high surface area and nanoscale size (at least one dimension measuring 100 nm or less).12 Top-down nanomaterial synthesis approaches have been extensively studied, incorporating metal nanoparticles,13,14 inorganic nanoparticles,15 nano aerogels,16 nanofibers,17 and so on, which have been applied in the corresponding fields. However, functional nanomaterials with a rational organization cannot be directly created only by nanotechnology-related top-down approaches,18 because the regulation of nanoscale components and structures is becoming crucial to the future of nanotechnology. In this context, bottom-up strategies based on self-assembly create well-considered materials by controlling the arrangement of atoms,19 molecules,20 macromolecules,21 or supramolecules,22 and have lately received great attention. These bottom-up self-assembly processes are similar to an ideal biological system for fabricating high-performance materials with incredibly high efficiency, selectivity, and multifunctionality.18 Overall, the types of self-assembly can be classified into static, dynamic, templated and biological self-assembly, and in self-assembly often the static type is used.23
The concept of nanoarchitectonics was originally proposed by Masakazu Aono in 2000.24,25 The term is supposed to be a novel technology system for the production of new functional materials from nanoscopic building blocks through harmonization of various processes, including atomic/molecular manipulation, chemical nanofabrication, self-assembly/organization, field-regulated organization, and bio-related treatments.26–28 Nanoarchitectonics represents a next-generation methodology that combines solid knowledge of nanotechnology with various other scientific disciplines such as supramolecular chemistry,29,30 organic/polymer synthesis,31,32 physical materials control,33 and biological materials conversion and organization.34,35 Driven by the extensive efforts of Ariga, nanoarchitectonics has been diversified and the range of applications extended.24,25,28,36 For example, functional building blocks such as conductive polymers, hydrogels, and ionic polymers have been prepared for application in smart energy supercapacitors due to their excellent electrochemical performance.37 The co-assembly of peptides and other bioactive components has become a facile strategy to form nano-structural units to achieve targeted release in tumor supramolecular nanotheranostics.38 It can be noted that the major difference between nanoscopic material synthesis and macroscopic manufacturing originated from various uncontrollable uncertainties such as thermal fluctuations, quantum effects, statistical distributions, and ingenious mutual interactions, which cannot be ignored in the nanoscale system.18 Therefore, actions and interactions in the nanoworld have to be coordinated rather than simply being summed up.4 It should be noted that analytical techniques, or more specifically nano-scale imaging, are of paramount importance for the development of nanoarchitectonics. That is why recent developments in high-resolution imaging employing, for example, scanning tunneling microscopy (STM), transmission and scattering electron microscopy (TEM and SEM), cryo-electron microscopy, and atomic force microscopy (AFM), which allow observation and manipulation of nanoscale materials, have deepened our understanding of nanoarchitectonics. In general, it can be also noted that assembled structures can be defined as those with perfect (high) order and those without perfect (low) order. The former (i.e. perfect, or what can be referred to as a high order) category can be further sub-divided into structures without defects and those with some (typically minor) defects; however, both types of such structures would have a distinct and repeatable pattern.39 The latter type of structure (with imperfect or low order) typically lacks a specific pattern.40,41
In this review, we analyze developments in nanoarchitectonics looking at structures assembled without perfect order, which can be empirically divided into polymer brushes, layer-by-layer (LbL) assembly, and hydrogels. Such assemblies contrast with those in which perfect order of constituent elements can be obtained, such as structures in self-assembly with perfect order, Fig. 1. In that regard, nanoarchitectonics is different from architectonics, micro-architectonics, and atomic scale, sub-nano architectonics, where design precisely follows the projected structure. We also analyze various applications in biomedical fields, where such assemblies are useful.
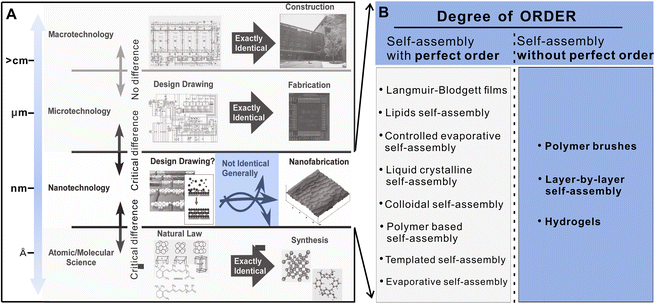 |
| Fig. 1 (A) Schematics of architectonics at different size scales.4 Grey areas are not covered by this article. (B) Degree of the order of different assembly modalities. Reproduced with permission from ref. 4. Copyright © 2015, Wiley. | |
2. Self-assembly structures with perfect order
Self-assembly refers to the organization of components into patterns or structures without external intervention;23 it is ubiquitous from macro- to micro-scale, and it is different from positional assembly where the permutation and combination of molecules are controlled artificially, but which largely depend on the characteristics of molecules and the non-covalent interactions.42 The above actions enable molecules to form specific organizations and be located at appropriate positions without external forces and to eventually spontaneously form ordered, rational, and many other useful structures. Notably, such non-covalent binding forces are not as strong as covalent bonds (400 kJ mol−1), making the system more flexible and allowing the reversibility and dynamics of self-assembled nanostructures to be maintained. Molecular units involved in self-assembly range from the nanoscale, and allow one to program materials at the nanoscale to achieve desired structures and properties. These superiorities render the technology important in nanotechnology, from chips to electric vehicles to performance optimization of biomaterials. Assembly at the nanoscale is a highly versatile methodology for establishing nanoarchitecture functional systems from a variety of small units. Self-assembly is mainly driven by various weak and reversible non-covalent interactions including hydrogen bonding, van der Waals interactions, electrostatic interactions, hydrophobic interactions, and π–π stacking. To go further, the construction of functional materials from nanoscale building blocks also calls for essential contributions from non-nanotechnology areas such as supramolecular chemistry with self-assembly/self-organization, materials fabrications, and biotechnology. Therefore, based on self-assembly, to further develop a way to control and modulate interactions within nanostructures, nanoarchitectonics, a progressive and novel concept, has emerged as a research paradigm alongside nanotechnology.
In general, one can distinguish self-assembly in: (a) nature,43 (b) colloids and nanoparticles,44 (c) mixed nanoparticle/polymer mixtures,45 and (d) molecular systems.46
(a) Self-assembly in nature. Over the past two decades, the principles of self-assembly in nature have inspired materials scientists to explore the fabrication of artificial materials with hierarchical structures and tailored properties, and to create functional devices. The self-assembling building blocks of peptides and nucleic acids bound to a variety of ligands perform intricate and diverse dynamic functions, and have been recognized as an outstanding family of structural units for the bottom-up fabrication of complex nano-biomaterials.43,47 The interactions relevant in nature's self-assembly are noncovalent forces including electrostatic interactions, hydrophobic effects, van der Waals interaction, hydrogen bonding, metal coordination and aromatic stacking, rather than covalent bonds. Incorporating self-assembly from nature into existing nanotechnology can offer new possibilities for the creation of reproducible hierarchical biomaterials with precise biomolecular and physical properties.48
(b) Colloidal self-assembly. An ensemble of colloidal nanocrystals can be led to self-assemble into an ordered superlattice driven by, for example, evaporation-based nanocrystal self-assembly,49 destabilization-based nanocrystal self-assembly,50 kinetics of nanocrystal self-assembly,51 and hydrocarbon-capped nanocrystal.52 The complex phase behavior and the flexibility of superlattice composition and structure suggest that controlled nanocrystal self-assembly could be an important enabler of next-generation materials design.44 Organic surfactants optimized for nanocrystal synthesis are also well suited for ordering nanocrystals into superlattices. Moreover, the effective nanocrystal size and shape can be achieved by modifying nanocrystal surfaces after synthesis. Self-assembly driven by chemical processes at the nanocrystal surfaces leads to a partial desorption of native hydrocarbon ligands,53 homopolymer surface ligands,54 block copolymer surface ligands,55 coarse-grained models for ligand-tethered nanocrystals,56 capping layers containing two or more ligand species as patchy particles,57 charged aliphatic surface ligands and charged nanocrystal cores,58 DNA-based surface ligands,59 toward biologically inspired self-assembly,60 and molecular switches as surface ligands.61 Self-assembly of nanocrystals can also make use of physical processes such as temperature,62 pressure regulation of interparticle coupling,63 template and structure,64 and external forces (electric/magnetic fields or fluid flows), but these processes do not extend to serial manipulation of building blocks (e.g., dragging individual particles into position).44
(c) Self-assembly in nanoparticle/polymer systems. For individual nanoparticles or polymers, the hydrophobic interactions of nanoparticles are capable of inducing nanoparticles into clusters with well-controlled sizes and interparticle distance;65 self-assembly of block copolymers is an unique approach for surface patterning with ordered structures.66 The synergistic interactions between self-organizing particles and a self-assembling matrix material can also lead to hierarchically ordered structures.45 One approach is to synthesize inorganic nanoparticles within the microdomain of well-ordered block-copolymer templates.67 Another approach is using the self-assembled micro-domain morphology of block copolymers to control the spatial arrangement of nanoscopic elements within the materials.68 These novel hybrid materials provide a means of controlling electrical, magnetic, or optical properties.69
(d) Molecular systems. In the field of molecular architectonics, different approaches are used. For example, biorecognition is often used as an effective method to construct novel supramolecules. Flexible control of the aspect ratio, higher-order layering, and ordering of self-assembled systems can be achieved by design and engineering development, combination and stepwise advancement of desired single molecules or modules at different scales and dimensions. In addition, the inherent multivalency of the assembled structure generally allows for more efficient biological effects and higher local concentrations of given chemical signals. At present, a large number of self-assembly systems based on inorganic nanoparticles, and organic substances including polysaccharides, peptides, proteins, and mixtures have been applied to drug delivery, biological scaffolds, and biosensing systems for regenerative tissue engineering, due to their multifunctional potential, biocompatibility, scalability, and tunability. Numerous self-assembly systems with perfect structures such as Langmuir–Blodgett (LB), lipid assembly, evaporative self-assembly, and templated self-assembly have been summarized, and the most typical structures are LB and lipid assembly.
In the past few decades, low-dimensional nanomaterials with low-level ordered structures have been prepared by means of film coating, solvent evaporation, and capillary forces, but their regularity, ordering, and controllability still require greater improvement. Driven by self-assembly technology, researchers discovered that LB technology can precisely control the molecular arrangement and assembly at the nanoscale. LB film is achieved by dispersing both hydrophilic and hydrophobic molecules at the gas–liquid interface, gradually compressing the volume occupied by the water surface, and finally transferring the film from the gas–liquid interface to the immersed solid matrix to obtain a Langmuir membrane with a monolayer arrangement70 (Fig. 2A and B). It is an ordered interfacial self-assembly technique on the substrate, and the film can be composed of single or multilayers of organic materials, and each dipping or reproduction step can uniformly adsorb the monolayer. In this way the thickness of the film can be precisely controlled, providing possibilities for the eventual realization of highly ordered, well-defined, controllable single/multilayer structures.71 Structures used in the process include liposomes, nanoparticles, polymers, proteins, and biomolecules, and this perfect structure has potential applications in semiconductor fabrication,72 supramolecular chemical media,70 and biotechnology.73 Besides, a perfect phospholipid bilayer structure was shown to be formed by a spontaneous orderly arrangement of the hydrophilic heads and hydrophobic tails of phospholipid molecules through the investigation of the structure of biological membranes. This kind of bilayer is the most important structure ubiquitous in living organisms and the best representative type of self-assembly technology in nature. Liposomes are spherical vesicles which possess a closed structure formed by the self-assembly of phospholipids in aqueous solutions (Fig. 2C). The driving force for this process is derived from the hydrophobic effect, which in turn organizes the amphiphilic phospholipid molecules to reduce unfavorable interactions between the hydrophobic acyl chains and the surrounding aqueous medium.74 This effect is triggered by various intermolecular forces including electrostatic interactions, hydrogen bonding, van der Waals, and dispersion forces.75 Importantly, similarity of the composition of liposomes and biological membranes endows the former good biocompatibility, while their amphiphilic structure enables them to load various hydrophilic and hydrophobic therapeutic drug molecules with favorable loading efficiency.76 Liposomes also allow modification of the surface by a variety of different chemical groups to obtain stimuli-responsive and smart properties, which ultimately guarantee targeted and controlled drug release.76 The encapsulation performance of liposomes can be enhanced by the increment of drug solubility and stability, which play a positive role in achieving sustained drug release and improving drug efficacy. More attractively, their subcellular size allows relatively high intracellular uptake and enhances bioavailability of drugs in vivo.77 However, liposomes are susceptible to rapid clearance during the transportation process in blood because of the adsorption of second-generation plasma proteins, although polymer coatings can effectively overcome this issue and maintain good pharmacokinetic properties of liposomes.78,79 In addition, the circulatory half-life of liposomes can be modulated by changing the composition of the lipid bilayer.80 In brief, the membrane-like composition and high-level orderly structure of liposomes promote their extensive application in medical fields, such as drug delivery vehicles, vaccination adjuvants, signal enhancers, or carriers with a focus on medical diagnostics and analytical biochemistry, and support matrices for various components.81
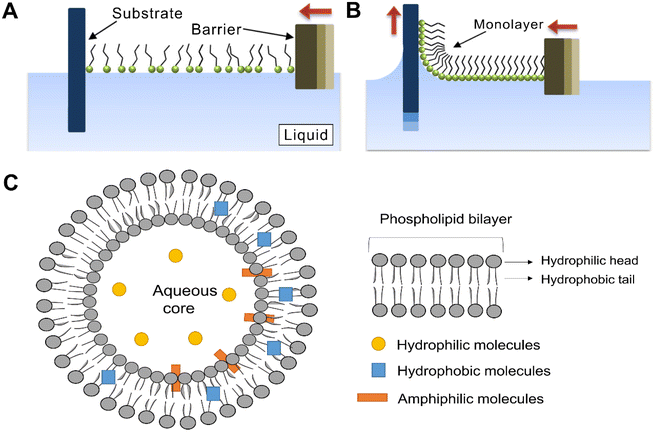 |
| Fig. 2 An illustration depicting the compression of a Langmuir–Blodgett film (A) by moving a barrier across the surface of a liquid covered by loosely packed amphiphilic molecules.81 (B) When fully compressed, the monolayer can be transferred to a substrate by a number of techniques including dip coating.82 (C) Schematic of the general structure of liposomes.83 Reproduced with permission from ref. 81 and 82. Copyright © 2014, Tsinghua University Press and Springer; and Copyright © 2021, Elsevier. | |
3. Nanoarchitectonics beyond perfect order: polymer brushes, LbL assembly, hydrogels, and other structures
Opposite to perfect (high order) structures84,85 which are without defects (Fig. 3A and B) or with minor defects (Fig. 3C and D), self-assembled nanostructures with imperfect (low or no) order and alignment are still often overlooked and even neglected, but this type of structure includes a broad range of nanomaterials, for example, those with an irregular crosslinking86,87 (Fig. 3E and F), those that are porous88 (Fig. 3G) and of uneven thickness89 (Fig. 3H). Nevertheless, non-perfect self-assembly of nanostructures is sufficient for control and thus to achieve their function.90,91 A summary of some of these structures, materials and interaction forces is presented in Table 1.
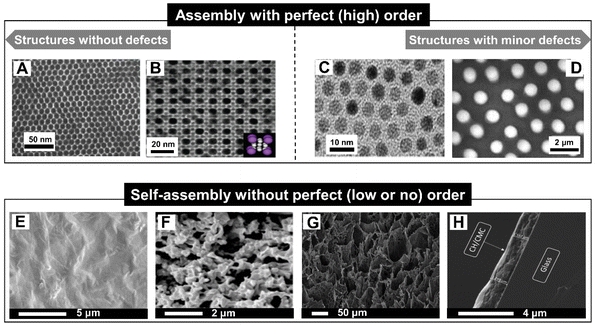 |
| Fig. 3 (A) TEM image of films with long-range ordered quantum dot superlattices.85 (B) (100) plane of cubAB13 type binary nanoparticle superlattices formed by 8.1 nm CdTe and 4.4 nm CdSe nanocrystals.92 (C) TEM image of an array of Au colloidal nanocrystals (Au NCs) capped with dodecanethiol.84 (D) SEM patterns of mesostructures of 5.7 nm cobalt nanocrystals having 13% size distributions subjected to a magnetic field perpendicular to the substrate during the evaporation time.39 (E) Surface SEM images and (F) cross-sectional SEM images of graphene oxide-polydimethyl diallyl ammonium chlor-hydrolyzed PVDF (GO-PDDA-h-PVDF) membrane.86 (G) SEM micrograph of hierarchical porous chitosan cryogels.88 (H) SEM image of glass coated with 20 bilayers of chitosan/carboxymethylcel complex.89 Perfectly self-assembled order (top) and imperfect self-assembled structures (bottom). Reproduced with permission from ref. 39, 84–86, 88, 89 and 92. Copyright © 2016, American Association for the Advancement of Science; Copyright © 2010, American Chemical Society; Copyright © 2009, American Association for the Advancement of Science; Copyright © 2007, American Chemical Society; Copyright © 2020, Elsevier; Copyright © 2018 Taylor & Francis; and Copyright © 2018, Elsevier. | |
Table 1 Summary of basic properties of polymer brushes, LbL assembly, hydrogels and other structures
|
Materials |
Forces |
Morphology |
Properties |
Ref. |
Polymer brushes |
Polymer single-crystal sheets + poly(ε-caprolactone) + glass substrate |
Chemical coupling |
Irregular chain-folding |
Super-density |
108
|
|
Hydrogen bond |
|
|
|
Diblock copolymers (liquid-crystalline polymer + polystyrene) |
Electrostatic interaction |
— |
High-density |
277
|
Block copolymers (polystyrene + polylactide) |
Electrostatic interaction |
Gyroid-like |
Potential responsive feature, capture and release properties |
112
|
LbL assembly |
Quantum dots (CdSe, CdZnS, CdTe, AuNPs, CdS, ZnS, MoS2, and carbon quantum dots) |
Electrostatic interaction |
Spherical/nanoflake-shaped/undulations and rough surface |
Transparency151 |
151, 152, 278 and 279 |
|
|
|
Ammonia-sensing152 |
|
|
|
|
Photoelectrochemical and photocatalytic278 |
|
|
|
|
Long-term stability and anti-fouling279 |
|
Polyelectrolyte polymers (poly(3-aminobenzylamine), poly(sodium 4-styrenesulfonate), poly(acrylic acid), poly(ethylene oxide)-block-poly(ε-caprolactone), polydopamine, poly-L-lysine, polysaccharides, proteins, poly-amino acids, and tannic acid) |
Electrostatic interaction |
Spherical/undulations and rough surface/porous |
Dopamine-sensing154 |
154–156, 158, 167, 280 and 281 |
|
Hydrogen bond |
|
Stimulated-release156 |
|
|
|
|
pH-Responsive158 and antimicrobial effect156,167 |
|
|
|
|
Enhanced biological properties158 |
|
Inorganic nanoparticles (SiO2, titanium, alumina, CaCO3, carbon, and magnetic iron oxide) + polyelectrolyte layers (poly(allylamine hydrochloride), poly (diallyldimethylammonium chloride), polysaccharides, proteins, poly(ε-caprolactone), and poly-amino acids) |
Electrostatic interaction |
Porous/spherical/irregular-shape/undulations and rough surface |
pH-Sensitive172,174 |
172–174, 176, 178, 190, 272 and 282–284 |
|
|
|
Improve cells growing173,272 |
|
|
|
|
Sensitive to bacteria composition282 |
|
|
|
|
Antimicrobial activity176 |
|
|
|
|
Drug loading190 |
|
|
|
|
Cancer cell killing283 |
|
Hydrogels |
Natural polymer (polysaccharides, and poly peptide) + substrate (silicon wafers, glass substrates, and titanium) |
Electrostatic interaction |
Porous/undulations and rough surface |
pH-Triggered programmable deformations and sequential double folding behavior222 |
222, 226 and 227 |
|
Hydrogen bond |
|
Resisting bacterials227,228 |
|
|
|
|
Attracting cells228 |
|
Natural polymer (polysaccharides, poly peptide, proteins, and DNA) |
Electrostatic interaction |
Porous/spherical/ellipsoid/nanofiber/undulations and rough surface |
Stimulated-release285 |
236, 245, 249, 285 and 286 |
|
Hydrogen bond |
|
Controlled stiffness and shearing-respondent245 |
|
|
|
|
Enhanced cell proliferation286 |
|
Other structures |
Semiconductor, metal, magnetic particles, and nanocrystals |
Hydrophobic |
Mostly flat substrates |
Responsiveness to different stimuli (light, magnetic, temperature, etc.) |
275, 276 and 287–289 |
|
Magnetic |
|
|
|
|
Steric |
|
|
|
|
Acoustic levitation |
|
|
|
Although the molecular arrangement or the morphology is imperfect in these materials, they are often used to address important issues and challenges, and it is worthwhile devoting effort to further explore their potential for nanoarchitectonics. In this regard, construction of functional self-assembled materials into thin films and coatings is essential for further expansion of the range of their applications, as they often control and even dictate interactions with the surrounding environment. More specifically, polymer brushes, hydrogels, LbL assembly, and other structures offer effective means for coating substrates with polymers, colloids, and biomolecules. Notably, thin film nanoarchitectonics can be an outstanding archetype for total three-dimensional (3D) nanoarchitectonics. Preparation of raw materials based on nanoscale self-assembly is an anticipated key concept for acquiring new functional materials, while constructing whole 3D structures at the nanoscale remains a difficult task even with currently existing technologies.93 The design of 3D structures is based on an extension of two-dimensional (2D) architectures, where a particular flexibility of the LbL self-assembly has been extensively used. Furthermore, hydrogels have been emerging in 3D nanoarchitectonics due to their controllability, for example by particles, mechanical performance, and versatility of design capabilities. Further controllable construction of 3D nanoarchitectonics similar to internal units of biological systems will greatly promote the progress of biomedical applications.
Here, we present an overview of recent developments in self-assembly research with special attention paid to examples of the construction of non-perfect hierarchical functional structures involving inorganic nanoparticles, organic molecules or hybrid structures.94 In particular, 2D nanoarchitectonics for surface adsorption including polymer brushes, LbL planar coatings and hydrogel films as well as 3D nanoarchitectonics including LbL particles and hydrogels are summarized. Some other structures with imperfect assembly are also highlighted and major strategies for preparing all these nanomaterials are discussed. Furthermore, we describe several application areas of 2D and 3D nanoarchitectonics assemblies using polymer brushes, LbL, hydrogels, and other structures in biomedicine, tissue engineering, drug delivery, and therapeutics. Finally, we provide an outlook describing potential applications and development steps of nanoarchitectonics for materials based on imperfectly assembled structures.
3.1 Polymer brushes
Over the past few decades, self-assemblies of polymer brushes, including homopolymer, block-copolymer, and mixed-polymer brushes, have invoked great interest in the fields of chemistry and materials science. Polymer brushes are special macromolecular structures wherein one end of the polymer chain is densely tethered to the surface of a planar matrix and the other end is stretched owing to the excluded volumetric repulsion.95–97 The introduction of polymer chains onto solid surfaces not only significantly changes the surface-related properties but also endows the obtained polymer brushes with exquisite properties such as adhesive behavior, colloidal stability, corrosion protection, stimuli-responsiveness, lubrication, friction properties, and so forth.98 Generally, polymer brushes can be synthesized using different strategies: physical adsorption,99 “grafting to”,100 and “grafting from”101 or “grafting through”102 strategies (Fig. 4). Physical adsorption is a reversible process involving the self-assembly of macromolecules with appropriate blocks of functional groups interacting with the groups on the surface.102 The “grafting to” approach is achieved by directly grafting pre-synthesized polymer chains to a substrate under efficient coupling reactions or specific interactions. In the “grafting from” method, polymer chains grow from the surface-immobilized initiators or RAFT agents to produce polymer brushes.103 Given the effects of the aggregation state of polymer chains and intermolecular steric hindrance on the properties of polymer brushes, “grafting from” displays less steric hindrance than “grafting to” to obtain polymer brushes with a relatively high grafting density and longer polymer chains. The “grafting through” strategy is a combination of “grafting from” and “grafting to” to create chains of macromolecules simultaneously in a solution and on a surface during the synthetic procedure. The lateral structures in polymer brushes depend on the relative lengths of building blocks and the energies of their interactions with each other and the solvent. The densely grafted macromolecules can self-assemble into hexagonally arranged micelles, inverse micelles, and stripes consisting of outer blocks;104 nevertheless, not densely grafted macromolecules would form “onion-like” and “garlic-like” micelle structures with the cores of less soluble blocks surrounded by more soluble blocks,105 or a “flower-like” micelles with dense cores and loose “petals”.106 With the great development of polymer materials, organic and inorganic chemistry, supramolecular chemistry, and various living/controlled polymerization methods have been employed in the fabrication of polymer brushes with control or optimization of composition, architecture, and the length of polymer chains.107 However, polymer brushes without perfect constructions still demonstrate functionality. In the following part, polymer brushes with random structures prepared by the self-assembly of macromolecules and self-assemblies of polymer brushes are the focus of discussion.
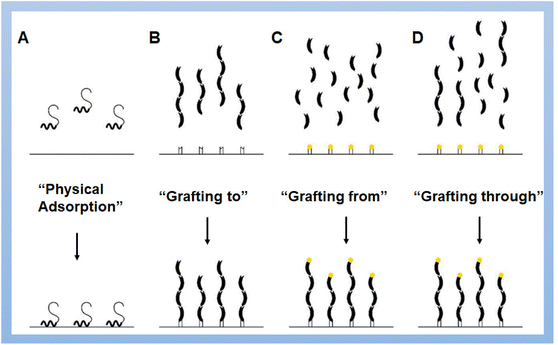 |
| Fig. 4 Major strategies of fabrication of polymer brushes: (A) physical adsorption of macromolecules, (B) “grafting to”, (C) “grafting from” and (D) “grafting through” methods.102 Reproduced with permission from ref. 102. Copyright © 2021, The authors, published by Elsevier. | |
Physical adsorption is a facile and straightforward method employing hydrogen bonds, van der Waals, or electrostatic interactions.102 Brandani and co-workers investigated the adsorption and desorption behavior of triblock copolymers on a self-assembled hydrophobic surface.99 The presence of polymer brushes was revealed by AFM. Nevertheless, this self-assembly-based approach cannot lead to desired dense and thick brushes, and a combination of self-assembly and other methods would be required. Zhou and co-workers reported a self-assembly-assisted grafting-to approach to synthesize polymer brushes on flat substrates.108 In this study, polymer chain ends were functionalized by an alkoxysilane group and pre-assembled into 2D polymer single crystals (PSCs). Chemically coupling the PSCs onto solid substrates led to the formation of polymer brushes with a grafting density as high as 2.12 chains per nm2. Moreover, polymer loop brushes can be obtained using oddly folded PSCs with bi-functionalized chain end (Fig. 5A). They provided a new pathway for synthesizing super-dense polymer brushes which are promising for various fields.
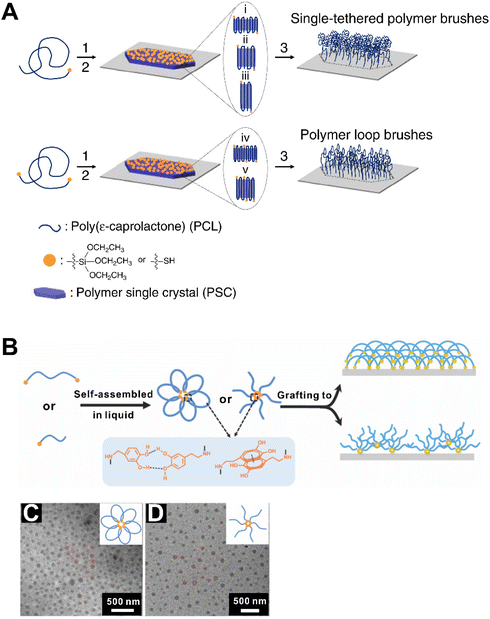 |
| Fig. 5 (A) Synthetic route for polymer brushes via the self-assemble assisted-grafting-to method.108 (B) Schematic illustration of polymer grafting to the substrate.109 TEM images of the triblock copolymer (C) and diblock copolymer (D) micelles and their corresponding illustrations.109 Reproduced with permission from ref. 108 and 109. Copyright © 2016, The authors; and Copyright © 2020, The Royal Society of Chemistry. | |
Self-assemblies of polymer brushes play an important role in fully realizing their functionalities. Grafting polymer brushes on inorganic plates is an example inspired by the adhesive nature of mussels and the antifouling properties of zwitterions. For example, Zhai and co-workers designed amphiphilic ABA triblock and AB diblock copolymers on silicon wafers, where A was a catechol-anchoring and B was a hydrophilic zwitterionic block.109 Amphiphilic block copolymers consisting of a hydrophilic poly[(sulfobetaine methacrylate)-co-(2-(dimethylamino) ethyl methacrylate)] (P(SBMA-co-DMAEMA)) block and a hydrophobic poly(dopamine methacrylamide) (PDMA) block are capable of self-assembling into star-like micelles and flower-like micelles due to the hydrophobic interactions in aqueous diblock and triblock copolymer solutions, respectively (Fig. 5B). TEM images showed that the micelles of self-assemblies of triblock copolymer and diblock copolymer were not uniform in size (Fig. 5C and D). The triblock polymer-coated surfaces showed excellent protein-reduction performance over diblock copolymer-coated surfaces at the same polymer concentration, which exhibited better antifouling properties. The force-versus-separation curves revealed that the loop conformation of triblock copolymers was attributed to an enhanced steric stabilization of the surface, and the loop conformation is probably the reason for improved protein-resistance capability. Thermo-responsiveness is another important property of polymer brushes.110 What's more, brushes can be made not only on flat surfaces but also on elongated structures, like microtubules, which provides new possibilities for the design of functional materials.111
Although the use of self-assembly methods for the synthesis of polymer brushes is not very common, the self-assembly behavior of polymer brushes is the key to a reliable design of functional 3D polymer materials. For example, block-type diblock molecular polymer brushes (MPBs) with polystyrene (PS) and polylactide (PLA) compartments were incorporated into surfactant-stabilized oil-in-water emulsions, and the MPBs formed solid nano/microparticles through self-assembly due to the increasing confinement in the nano-emulsion droplets during solvent evaporation.112 The self-assembly properties of MPBs have been used in a variety of different applications such as nanofabrication, cellular imaging and even mimicking complex biological functions such as size- and charge-selective molecular transport.113
3.2 LbL self-assembly of nanostructures
LbL assembly built by deposition of alternately charged polyelectrolyte multilayers provides significant benefits to the generation of functional materials.114–117 LbL assembly is a powerful technique for preparing multilayer films and coatings,118,119 allowing the application of various stimuli to control the nanoscale thickness of overall films and the composition of hierarchical materials.120–122 The LbL assembly process is often driven by electrostatic interactions, i.e., charge neutralization and re-saturation of the counterionic component materials upon adsorption onto the charged surface resulting in a charge reversal.123–125 This permits alternate adsorption of cationic and anionic polyelectrolytes at a surface.126 Besides, hydrogen-bonding interactions,127–129 bio-specific interactions,130 charge transfer interactions,131 electrochemical reactions,132 metal coordination,133 stereo-complex formation,134 sol/gel reactions,135 molecular interactions,136 or covalent bonding formation137 can also act as driving forces of LbL assembly. Another property of LbL assembly is pH-,138–141 salt-,142,143 redox,144 ionic strength145 responsiveness, and crosslinking capability.146 LbL assembly exhibits the unique characteristics of facile and inexpensive procedures, which can be divided into three mature operational strategies. Conventionally, multilayer nanoarchitectures would be obtained on a planar substrate by simply immersing the substrate into solutions for depositing layered materials114 (Fig. 6A). Further, spray- and spin-coating-assisted LbL assemblies, which benefit from industrial spin-coating equipment, have been developed for LbL processing in recent years36,147,148 (Fig. 6B and C). These innovative methods have been proved to form LbL films in sub-second to second time intervals per layer instead of traditionally used immersion adsorption steps which take several minutes. Different methods can result in differences in the stratification versus interpenetration of the layers that should be considered when designing films for different applications. Compared with the LB technique, which produces tightly arranged and well-defined ordered monolayers, the LbL approach generates quite flexible and non-crystalline multi-component assemblies. LbL-assembled materials often contain structural and morphological variations or even defects that hinder the formation of grain boundaries, which in turn are favorable for the facile diffusion of small molecules and open possibilities for dynamic functions. These imperfect features are highly advantageous for sequential nanoreactors, protective coatings, and drug delivery systems.149
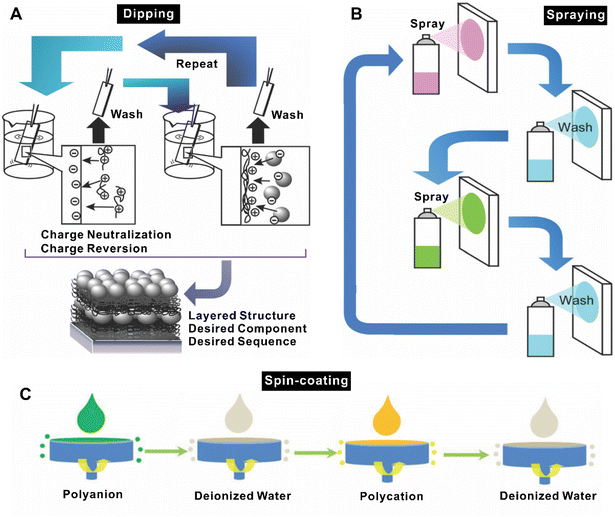 |
| Fig. 6 Schematic illustration of the LbL assembly process with (A) dipping method, (B) spray deposition method,36 and (C) consecutive spin-coating method.147 Reproduced with permission from ref. 36 and 147. Copyright © 2022, The Owner Societies; and Copyright © 2016, The Royal Society of Chemistry. | |
3.2.1 LbL self-assembly of 2D nanostructures on substrates.
Noble metal and semiconductor nanoparticles exhibit remarkable electronic and optical properties that are strikingly different from those of their bulk materials.150 These inorganic nanoparticles were deposited onto substrates by LbL self-assembly techniques to produce functional films, which have a wide range of applications in sensing. Lu and co-workers created nanoarchitectonics structures consisting of hydrophobic CdSe/CdZnS quantum dots (QDs) and hydrophilic CdTe and Au nanoparticles (AuNPs), linked by a functional silica layer on a slide glass.151 SEM images of AuNPs films and CdSe/CdZnS core/shell QDs films demonstrated that AuNPs or CdSe/CdZnS cannot be dispersed well in the slide glass at high concentration, and uneven aggregation occurred. TEM imaging of CdTe QDs films revealed similar results to those obtained for AuNPs films and CdSe/CdZnS core/shell QDs films. But these glass films are also promising for applications in biomedicine and clinical diagnostics due to their excellent stability and transparency. Zhang and co-workers first fabricated a molybdenum disulfide (MoS2)/tricobalt tetraoxide (Co3O4) nanocomposite film sensor on a substrate with interdigital electrodes via the LbL self-assembly route152 (Fig. 7A). The surface morphology of as-prepared Co3O4, MoS2, and MoS2/Co3O4 film was examined by SEM. Fig. 7B shows that the shapes of hydrothermally synthesized Co3O4 nanorods are not identical, and the size distribution is between 65 and 135 nm. On the other hand, MoS2 has a nanoflake-shaped structure, and its size distribution extends from 110 to 290 nm. In the MoS2/Co3O4 nanocomposite, Co3O4 nanorods and MoS2 nanosheets were in contact with each other without strict arrangement. The sensing performances of the MoS2/Co3O4 nanocomposite film sensor were evaluated by exposure to an ultra-low concentration of ammonia gas in the range of 0.1–5 ppm at room temperature. The experimental results revealed that imperfectly structured films possessed a high sensitivity, good repeatability, stability, selectivity, and fast response/recovery characteristics for NH3. Rodrigues and co-workers assembled LbL films of oppositely charged polymer-stabilized graphene layers as (GPDDA/GPSS)10, also including gold nanoparticles (AuNPs) in another architecture ((GPDDA/GPSS)1(AuNP/GPSS)10) for methyl parathion (MP) sensing153 (Fig. 7C). GPPDA was synthesized using graphene nanoplatelets stabilized in poly(diallyldimethylammonium chloride) (PDDA), and GPSS was synthesized using graphene nanoplatelets stabilized in poly(styrene sulfonate) sodium salt (PSS). SEM images showed that both (GPDDA/GPSS)10 and (GPDDA/GPSS)1(AuNP/GPSS)10 films were wrinkled and undefined arrangement structures. Moreover, the distribution of AuNPs on the graphene sheet surfaces was random (Fig. 7D and E). On the basis of structural characterization, (GPDDA/GPSS)10 and (GPDDA/GPSS)1(AuNP/GPSS)10 films interacted with MP through different adsorbing properties which were accounted for by the fact that the MP molecules can be distributed homogeneously on the film with AuNPs. Indium tin oxide (ITO) electrodes modified with the LbL films were applied by differential pulse voltammetry, achieving the detection of MP in real samples.
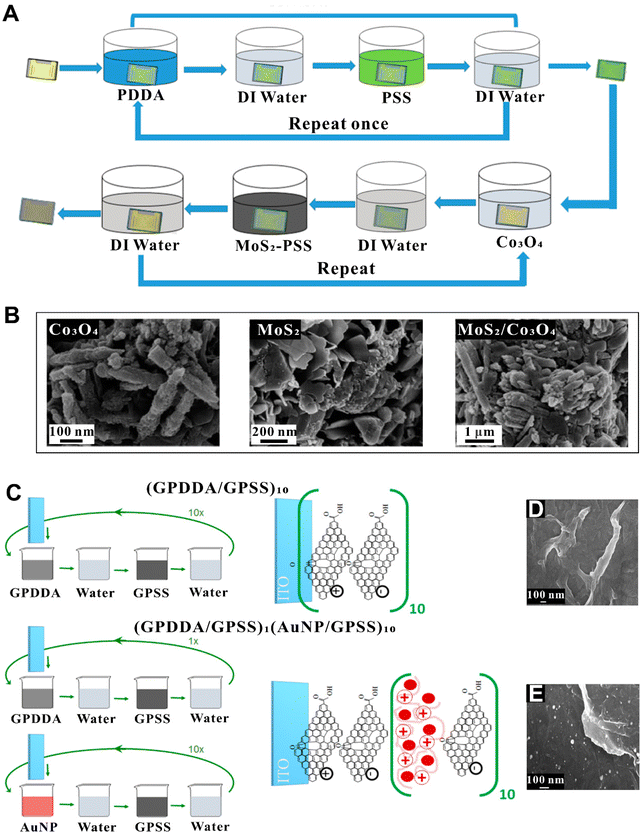 |
| Fig. 7 (A) LbL fabrication process of MoS2/Co3O4 nanocomposite film.152 (B) SEM images of Co3O4 (left), MoS2 (middle), and MoS2/Co3O4 (right).152 (C) Procedures to modify ITO electrodes with (GPPDA/GPSS)10 and (GPDDA/GPSS)1(AuNP/GPSS)10 LbL films.153 SEM images of LbL (GPDDA/GPSS)10 (D) and (GPDDA/GPSS)1(AuNP/GPSS)10 (E).153 Reproduced with permission from ref. 152 and 153. Copyright © 2017 and 2019 American Chemical Society. | |
For organic LbL systems, polyelectrolyte polymers are often deposited on flat substrates, where various materials can be also incorporated. A poly(3-aminobenzylamine)/PSS (PABA/PSS) multilayer thin film was developed, which was used as a electrochemical dopamine (DA) biosensor exhibiting a good sensitivity, linear range, low detection limit, long-term stability, and good reproducibility154 (Fig. 8A). The PABA was firstly synthesized by chemical oxidation of 3-aminobenzylamine (ABA) monomer using ammonium persulfate (APS) as an oxidant. The morphologies of PABA powder and PABA/PSS thin films observed by SEM, as shown in Fig. 8B. PABA powder was not uniform in size and did not line up neatly on PSS, even displaying irregular stacking in multilayer PABA/PSS thin films. However, the PABA/PSS thin film deposited onto fluorine-doped tin oxide (FTO)-coated glass substrate enabled detection of DA. The construction of biodegradable LbL self-assembled drug delivery surfaces was investigated by Kim and co-workers.155 The film was constructed based on the hydrogen bonding between poly(acrylic acid) (PAA) as a H-bond donor and biodegradable poly(ethylene oxide)-block-poly(ε-caprolactone) (PEO-b-PCL) micelles as a H-bond acceptor; this structure was assembled under acidic conditions (Fig. 8C). Due to the weak interactions of the hydrogen-bonded film on hydrophobic surfaces, flexible free-standing LbL thin film without an ordered structure can be generated (Fig. 8D) and can be allowed to disintegrate to release micelles upon exposure to physiological conditions. It was also demonstrated that such micelle LbL film loaded with antibacterial triclosan was fully functional in inhibiting the growth of Staphylococcus aureus (S. aureus). The micellar encapsulation of hydrophobic therapeutics does not require specific chemical interactions and takes up random positions, which can be used to control release properties.
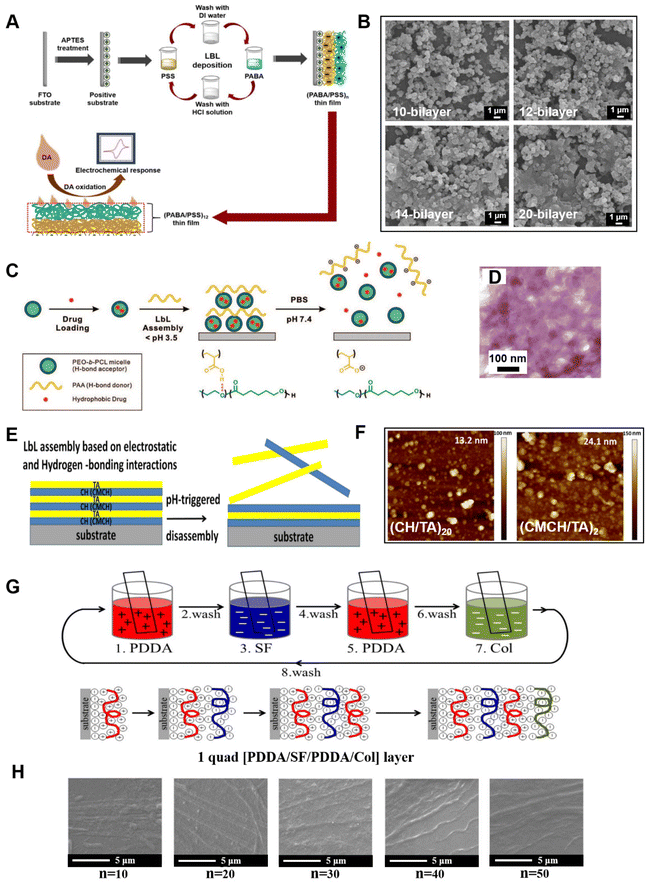 |
| Fig. 8 (A) Schematic diagram representing PABA/PSS LbL films assembled for the electrochemical detection of DA based on a biosensor on FTO substrate.154 (B) SEM images of different number bilayer PABA/PSS thin films.154 (C) Schematic representation of a hydrogen-bonding LbL assembly of block copolymer micelles for hydrophobic drug delivery carriers from surfaces.155 (D) TEM micrograph of a cross-section of free-standing (PEO-bPCL/PAA)60 film.155 (E) Schematic of the formation and pH-triggered disassembly of polyelectrolyte LbL films composed of the polycations CH or CMCH and the polyanion TA.159 (F) AFM images of (CH/TA)20 and (CMCH/TA)20 films.159 (G) Schematics of 1 quad PDDA-SF-PDDA-Col LbL self-assembly formation on a glass slide surface.161 (H) SEM images of the surface of [PDDA-SF-PDDA-Col]n film with different layers after LbL deposition.161 Reproduced with permission from ref. 154, 155, 159 and 161. Copyright © 2021, The authors; Copyright © 2008, American Chemical Society; Copyright © 2019 and 2019, Elsevier. | |
With increasing demand for being environmentally friendly, and for biodegradability, biocompatibility, and non-toxicity for nanomaterials, natural polymers (such as polysaccharides,156,157 proteins, and poly-amino acids158) are of great interest in LbL assembly research. Chitosan can react with other materials through interactions including electrostatic forces and hydrogen bonding, which allows fabrication of chitosan-based nanocomposites by LbL. For example, chitosan or N-(2-hydroxypropyl)-3-trimethylammonium chitosan chloride (CMCH) and tannic acid (TA) were employed to form LbL assembly multilayer films with pH-responsive and antibacterial properties159 (Fig. 8E). Both CH/TA and CMCH/TA LbL films with an irregular inner structure (Fig. 8F) exhibited a similar disassembly at pH 7.4 and showed antiadhesive activity against Escherichia coli (E. coli) and S. aureus. On the basis of N-grafted copolymers of chitosan with ether polyethylene glycol (PEG) or dextran (DEX) alternately deposited with dextran sulfate, LbL films with enhanced resistance to protein adsorption were obtained.160 Natural proteins also can be used in LbL film to provide improved biological compatibility. An osseointegration surface for dental implant materials was manufactured with LbL self-assembly161 (Fig. 8G). The films were composed of silk fibroin (SF), collagen (Col), and PDDA, and films of PDDA-SF-PDDA-Col were constructed with a different number of layers: n = 0, 10, 20, 30, 40, and 50 through an immersion method. These coatings displayed molecular mobility on their surfaces, which enhanced biological properties, osteoblast cell proliferation, alkaline phosphate (ALP) activity, and total protein absorption. SEM images revealed that the surfaces of LbL films were not flat and had partial stripes (Fig. 8H). The examples mentioned above are based on organic materials assembled on flat surfaces without specific alignment sequences, but these films still have their functionalities in certain fields. It should be noted that structure and order in polymeric systems have been investigated,162–164 but such order depends on self-assembly methods.
The aim of organic LbL films doped with inorganic components is typically to improve properties or to obtain additional functionalities in the resultant hybrid materials. Deng and co-workers designed a dual therapy implant coating on the 3D micro-/nanoporous sulfonated polyetheretherketone (SPEEK) via LbL self-assembly of Ag ions and Zn ions165 (Fig. 9A). Three types of nutrient elements incorporating SPEEK coatings (Ag-SPEEK, Zn-SPEEK, and Ag/Zn-SPEEK) were prepared and characterized. SEM images indicated that SPEEK formed a 3D network structure with interconnected micro- and nanopores, and the size of the non-uniform micropores varied between 0.5 and 1.0 μm (Fig. 9B). The antibacterial assays demonstrated that the Ag-decorated and Ag/ZnO-co-incorporated SPEEK coatings effectively inhibit the reproduction of E. coli and S. aureus with a bactericide rate up to 99%. In addition, osteoblasts exhibited a higher level of ALP activity and osteogenesis-related genes on the Ag/ZnO dual-co-modified SPEEK coatings. Ag/Zn-SPEEK implant coatings have better antibacterial activity, biocompatibility, and the capability to hasten osteoblastic differentiation of the implant, which might be related to the ion-induced synergistic effect and the 3D porous topological structure. Chen and co-workers reported fabrication of Ag nanoparticles (AgNPs)/cellulose nanofibril (CNF) comprehensive thin films via LbL spray-coating166 (Fig. 9C). Compared with AgNPs sprayed on SiO2 substrates, the incorporation of CNF substrates resulted in a more uniform distribution of AgNP agglomerates and individual AgNPs through their network structure and absorption of partially dissolved AgNP clusters. However, AFM images have shown that the deposition of AgNPs onto CNF thin film was still not well proportioned (Fig. 9D). Nevertheless, the increase of hydrophilicity of the AgNP/CNF composite films and the localized surface plasmon resonance intensity of AgNP suggested their potential applications in antifouling coatings or label-free biosensors. Li and co-workers successfully prepared a hybrid coating consisting of hydroxyapatite (HA), AgNPs, and chitosan on a polydopamine (PDA)-modified titanium (Ti) substrate by the LbL assembly process167 (Fig. 9E). PDA accelerated the formation of HA crystals and bridged the binding strength between HA and Ti substrate. The surface of substrate modified by polydopamine, and the hydroxyapatite crystals on the modified substrate both were not in order (Fig. 9F and G). It has been noted that the surface-deposited HA particles react with AgNO3 to form silver phosphate nanoparticles (SP NPs) during the period of immersion in AgNO3 solution. SP NPs with sizes ranging from 50 to 100 nm were partially agglomerated on the surface of HA to form small clusters (Fig. 9H). In this nanoarchitectonics system, chitosan nanofilm played an important role in controlling the release of Ag+ from the hybrid coating and effectively reducing the cytotoxicity of hybrid coating. The PDA/HA/Ag/CS coating exhibited a higher antimicrobial effect than HA and can damage the shape of bacterial cells (especially E. coli), and presented long-term osteogenesis.
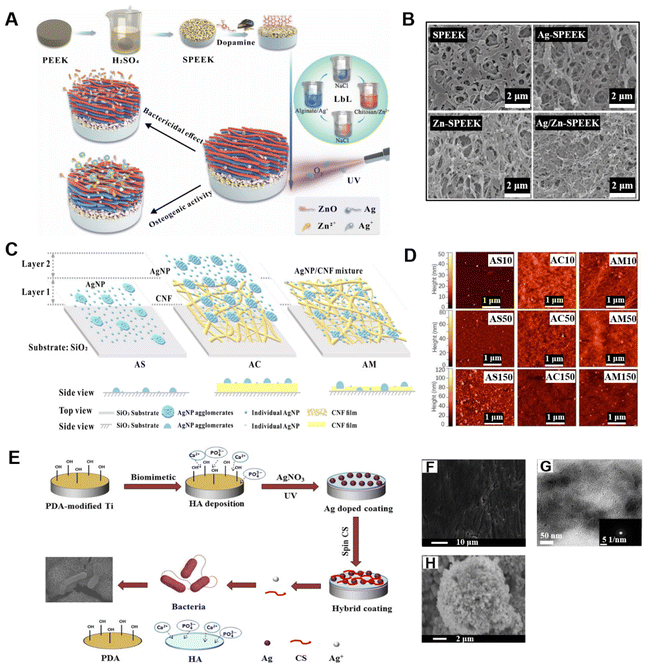 |
| Fig. 9 (A) Schematic drawing of the preparation of Ag/ZnO dual-decorated micro-/nanoporous SPEEK, and its bactericidal effect and osteogenic activity.165 (B) SEM images of SPEEK, Ag-SPEEK, Zn-SPEEK, and Ag/Zn-SPEEK coating.165 (C) Sketch of the layered structure of AgNPs on the SiO2 substrate, AgNPs spray-coated onto a cellulose CNF thin film, and a AgNP/CNF mixture on the SiO2 substrate, respectively.166 (D) AFM images of the AS, AC, and AM samples with 10, 50, and 150 spray cycles, respectively.166 (E) Schematic illustration of a hybrid coating on Ti implant via LbL method and its disruptive behavior on bacterial cell membrane.167 (F) Electron microscopy images of polydopamine modification of the Ti substrate.167 (G) TEM image of hydroxyapatite crystals on polydopamine-modified Ti.167 (H) SEM image of Ag doped on the surface of HA.167 Reproduced with permission from ref. 165, 166 and 167. Copyright © 2018, Wiley; Copyright © 2021, The authors, published by American Chemical Society; and Copyright © 2016, American Chemical Society. | |
3.2.2 LbL self-assembly on particles – 3D nanostructures for encapsulation.
LbL self-assembly can also provide an excellent shielding effect for the encapsulated substrates or molecules and achieve the versatility of the resulting hybrid system through the selection and reasonable matching of materials constituting hierarchical films. Such multilayers are mainly formed by alternate deposition of polyvalent polymers, colloids, and biomolecules on particles which serve as sacrificial templates for making polyelectrolyte multilayer capsules. Electrostatic interactions, and the matrix used are more likely to comprise micro/nano inorganic particles. Herein, the multilayer structures obtained by LbL self-assembly on particles used as substrates, including those possessing asymmetric shapes, non-ordered arrangements of molecules, and local defects, are also discussed.
Originally, purely polymeric capsules were developed.168,169 However, these hybrid systems with undesired structures still have an important value for research and application.170 Inorganic nanoparticles have attracted extensive attention in biomedicine due to their good physicochemical properties, stimuli-responsiveness, and signal-enhancing capabilities, etc. However, their low dispersion stability caused by high surface polarity, surface energy, and nano-effects will greatly limit their applications, while the introduction of polymer coatings provides a feasible solution to this problem. In addition, particles with perfect or highly symmetrical structures are not easily available under natural synthetic conditions due to the principle of minimum energy, which makes it difficult to obtain hybrid particles with perfect structures.
In general, hybrid particles are composed of heterogeneously distributed polymers and inorganic nanoparticles with different properties. Due to the irregular structures or morphologies, the agglomeration effect of nanoparticles, the uneven coating thickness and hole defects caused by the random cross-linking mechanism of polymer chains in the liquid phase, the morphology and surface structure of the so-called hybrid particles cannot be precisely controlled, resulting in a sequence-imperfect structure, which can be observed from the microstructure of hybrid particles. Even so, these poorly structured complexes formed by LbL self-assembly still exhibited a good performance.
Polymers or even inorganic nanoparticles forming the layers of such capsules have been deposited in an aqueous solution on such sacrificial templates (or cores) as melamine formaldehyde (MF),171 SiO2,172 titanium,173 alumina,174 CaCO3,175 carbon,176,177 magnetic iron oxide,178 and metal nanoparticles.179 These particles are homogeneously dispersed in the polymer solution, and single or multiple layers are deposited by inducing the cross-linking between polymer chains and the mutual adsorption caused by the charge effect between the polymer and the surface of the nanoparticles. LbL technology is the most typical method to obtain a multifunctional composite coating. The formed hybrid particles integrate the excellent properties of the two materials, which endows them with high encapsulation efficiency and stimuli-responsive ability, giving them great potential for applications in the encapsulation, delivery and targeted release of drugs as well as in biosensors.180,181 Furthermore, the resulting hybrid particles can be transformed into hollow capsules depending on the solubility of inorganic nanoparticles, such as CaCO3 particles, which is completely soluble in acidic conditions.182 The versatility of the capsules results from several aspects, including that release kinetics can be controlled as the number of layers affects release kinetics.183,184
Given that the spherical structure and porosity of vaterite endow it with relatively higher drug-loading efficiency, it can be defined as a good substrate for synthesizing LbL self-assembly system. Numerous combinations of polyelectrolyte layers with opposite surface charges have been applied to the surface of vaterite, such as dextran sulfate sodium salt/poly-L-arginine hydrochloride,185 chitosan/hyaluronic,186 sodium alginate/chitosan,187 poly(allylamine hydrochloride) (PAH)/PSS,188 poly-L-ornithine (PLO)/fucoidan,175 mucin/protamine,189 PDDA and PSS,190 cationic-polycyclodextrin/alginate,191 and DA-modified alginate/titanium(IV) bis(ammonium lactato) dihydroxid,192 and phenylboronic compounds for glucose responsiveness.193,194 Unlike the completely ordered molecular arrangement, although the LbL adsorption and non-covalent cross-linking of polymers lead to the formation of various imperfect surface structures, such as continuous and porous 3D network structures186,188,191 or the concave-convex inner surface because of the penetration of the polymer into the pores,182 the resulting surfaces are denser and smoother than the original matrix,175 and compared with porous CaCO3 particles without multilayers, the hybrid carriers show higher loading efficiency and a synergistic release mechanism. Moreover, some multilayer capsules can maintain structural stability in physiological conditions or a reductive environment186 (Fig. 10).
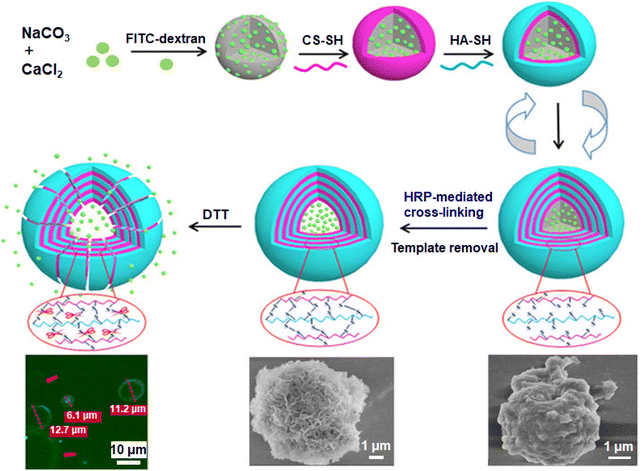 |
| Fig. 10 Schematic illustration of an enzymatical disulfide-crosslinked hollow CS/HA microcapsules by LbL on the CaCO3 sacrificial templates, the confocal laser scanning microscope (CLSM) images of FITC-dextran-loaded disulfide-crosslinked hollow chitosan/hyaluronic acid (CS/HA)4 microcapsules incubated in PBS (pH 7.4) containing 5 mmol L−1 dithiothreitol for 60 h at 37 °C (left bottom), and the SEM images of disulfide-crosslinked hollow (CS/HA)4 microcapsule (middle) and disulfide-crosslinked CaCO3@(CS/HA)4 microspheres (right bottom).186 Reproduced with permission from ref. 186. Copyright © 2018, American Chemical Society. | |
Mesoporous silica nanoparticle (MSN) is attractive as a stable carrier for biomolecules such as DNA, enzymes, proteins, etc., ultimately for drug delivery and biosensing due to facile preparation, controllable size, high surface area and biocompatibility. However, MSN usually requires surface modification to improve the comprehensive properties, and LbL self-assembly is one of the most favorable methods. Different polymer combinations can be adsorbed on the surface of MSN through the LbL technique. For example, HA/5,10,15,20-tetrakis(4-sulfonatophenyl)-porphyrin (TPPS4) can be alternately deposited on the particle surface by electrostatic adsorption to form a hybrid carrier, and the existence of the multilayer masks the mesoporous structure of SiO2, which reduces the specific surface area, pore size, and pore volume of the original matrix, effectively hindering the leakage of drug molecules from pores.195 Self-assembly structures are closely related to the properties of polymers, which in turn are affected by the charge distribution caused by such factors as the concentration of salt solution and pH.196 In addition, polyamidoamine (PAMAM) dendrimers/chondroitin sulfate, Lys/HA/polyglycerol methacrylate (PGMA) have been studied as well,197,198 and these polymer multilayers improve the stability of the hybrid system and the targeted release of drug molecules, despite the structural imperfections caused by the random cross-linking of the polymers.
Advanced sandwich-liked structures have been also developed. For example, Shi and co-workers first deposited oxidized alginate and protamine organic layers on the surface of CaCO3 particles via the LbL technique and then prepared a SiO2 template layer on the membrane by biomimetic silicidation, followed by biomimetic mineralization.199 An organic–inorganic hybrid outer membrane was formed, and with the removal of CaCO3 core and SiO2 template, mitochondria-like multi-chambered microcapsules with microcavity dimensions and nanomembrane spatial structure were obtained (Fig. 11A). The microstructures of the intima and adventitia showed high densification but exhibited different thicknesses (intima: 70–140 nm; adventitia: 200 nm), and the structures of the inner and outer surfaces of the adventitia were asymmetric (Fig. 11B–E). Although the nanoparticles were not arranged in an orderly manner, which was unavoidable during the deposition process, this double-sandwich structure had better activity, selectivity, and recovery stability of loaded enzymes compared with single-chamber microcapsules. Also, the highly interconnected slit-like pores inside the microcapsules provided channels for the free diffusion of molecules, and the double-layer structure can hinder the leakage of molecules as well. In addition, the presence of intermembrane space molecules built up osmotic pressure and improved particle stability.
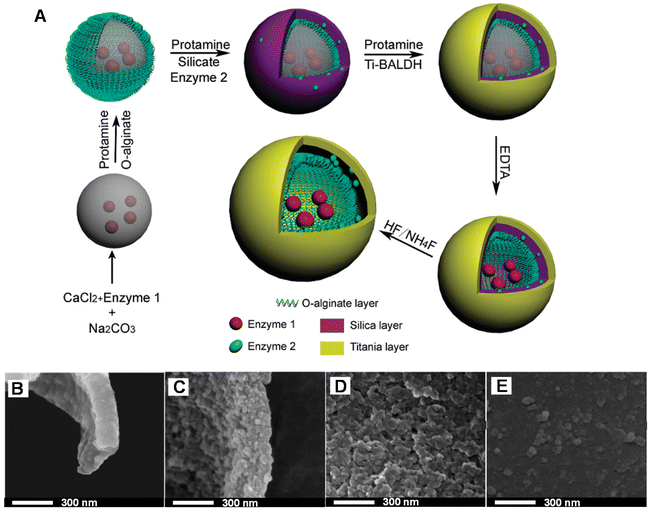 |
| Fig. 11 (A) Scheme of the construction of multienzyme system through synergy of LbL self-assembly and biomimetic mineralization. High-resolution SEM (HRSEM) image of the inner membrane (B) and inner surface (D) of hybrid double membrane microcapsules. HRSEM images of the outer membrane (C) and outer surface (E) of hybrid double membrane microcapsules.199 Reproduced with permission from ref. 199. Copyright © 2011, American Chemical Society. | |
Similar multilayers can be applied to mesoporous carbon particles with mono-dispersity to achieve surface modification and controlled drug release. For example, a hybrid carbon-based system was formed using polyethyleneimine (PEI) as the inner layer, followed by alternate deposition of PSS and PAH bilayers three times. In view of the surface asperity effect caused by the porous structure of carbon particles and the low charge in bare carbon, the first two PSS/PAH bilayers inevitably exhibited uneven thickness and local defects. Nonetheless, the presence of the polymer coating improved the biocompatibility and controllability of drug release of hybrid carriers, and provided room for the addition of specific receptors.200
Some metal salt particles are also considered as good candidates for matrix materials.201 For example, hedgehog-shaped particles (HPs) prepared from Zn(NO3)2 replicating the tip geometry of pollen grains were coated with polymers and exhibited remarkable stability.202 Indeed, in contrast to the easily agglomerated spherical or square particles with regular structure, the former particles with imperfect appearance showed high dispersion stability and were not affected by their hydrophobicity/hydrophilicity matches with the medium phase. To preserve this stability, the surface modification of the tip was designed with a high-density conformal coating consisting of polymers and AuNPs via the LbL technique. The combination of plasma activity, the dense buildup of NP, and the omnidispersible stability enabled HPs with multilayers to become more efficient surface-enhanced Raman scattering (SERS) probes for rapid multipath biosensing, since the sensitivity and reliability of SERS-based biosensing highly depend on the dispersion stability of particles.203 On the other hand, such HPs were shown to self-assemble into rod-like structures,204 which are scientifically interesting and practical from the application point of view, but imperfect structure-wise. Thus, in addition, those LbL-modified HPs offer unique capabilities for catalysts, aerosol drug carriers, and extraction processes, where dispersion stability in a wide range of polar media is critical, even though their structure is imperfect.
Notably, incorporating inorganic nanoparticles in the polymer film205 or spherical polyelectrolyte multilayer capsules brings additional functionalities. For example, the mechanical properties of capsules can be enhanced either by increasing the number of layers206 or incorporating inorganic nanoparticles207 as well as Raman signal peaks for easier detection and the antibacterial properties of the substrate material.208–210 Metal nanoparticle coatings prepared on the surface of polymer substrates through LbL technology can impart metallic properties to the surface of polymers, thus giving the possibility of further processing (e.g. electroplating), which opens up a new avenue for decorative coating and surface metallization of polymer materials.211 It should be noted that the application range of microcapsules, particularly in biomedicine, is steadily growing.170,212,213 Also, it can be added that polyelectrolyte multilayer capsules can be combined with polymeric films214,215 to further extend the range of applications.
3.3 Hydrogels
Hydrogels are materials with mechanical properties situated between those of elastic solids and viscous liquids.216 Hydrogels are hydrophilic gels with 3D network structures, which can be synthesized through different crosslinking methods using such polymers as natural polysaccharides, polypeptides, and derivatives. According to the nature of the cross-linking, hydrogels can be divided into chemical and physical types.42 The former is determined by covalent bonds, which have longer degradation times and stronger mechanical properties but may involve addition of cross-linking agents, which will affect the biological properties of the hydrogel.217 The formation of physical hydrogels is driven by electrostatic interaction, hydrogen bonding, and chain entanglement, and is affected by physical factors such as temperature, pressure, pH, ionic strength, and the type of added phase. These effects can be interfered with or interrupted by environmental changes, resulting in imperfect sequence distribution and length of polymers. However, the formation process does not involve the adoption of potentially toxic cross-linking agents while keeping excellent performance, which increases the appeal of physical hydrogels. According to the formation principle, physical hydrogels can also be called self-assembled hydrogels, and represent a new class of architecture for biomedical applications.
3.3.1 2D nanostructures of hydrogels on substrates.
In terms of the fabrication of hydrogel films, natural polymer-based hydrogel films are highly regarded due to their superior biocompatibility. Numerous accounts in the literature have reported that self-assembled natural hydrogel films were multifunctional for a various range of biomedical applications over the decades.218–221 For instance, Cui and co-workers designed smart hydrogel actuators constructed from chitosan and sodium alginate for assessing biomedical applications.222 The gradient chitosan/sodium alginate complex hydrogel film, which is nano-porous, ultra-strong, and has adjustable thickness, provides practical guidance for application in drug delivery, tissue engineering, soft robotics, and active implants. Acacia gum polysaccharide-based porous hydrogel films has been made by Singh and co-workers to explore antioxidant activity and improve its wound-healing potential.223
It is worth noting that an emerging approach to improve the physical–biochemical properties and the multifunctionality of biomaterials is the incorporation of functional hydrogels onto 2D surfaces. Surface-attached hydrogels are another tailored architecture comparable with polymer brushes and LbL assemblies as stable and durable polymer coatings which combine the advantages of both hydrogels and films.224,225 A large range of inorganic substrates such as silicon wafers, glass substrates, and titanium surfaces can be chosen to introduce hydrogel films.226–228 Lian and co-workers used a self-assembled peptide nanofibrous hydrogel composed of N-fluorenylmethoxycarbonyl diphenylalanine (Fmoc-FF) to construct a smart biointerface on the glassy carbon electrode.229 The interlaced nanofibers of Fmoc-FF hydrogel with some non-uniform pores acted as a matrix to embed an enzyme model for enzyme-based electrochemical biosensing, and was a robust substrate for cell adhesion to monitor H2O2 released from cells in situ. The polyimide-based thin film electrodes coated by a laminin-derived peptide can support neurons and reduce the risk of fibroblast-mediated fibrotic encapsulation.230 Notwithstanding the great potential of surface-attached hydrogel films, they are still rarely investigated except for very specific uses. This is probably due to the lack of a general strategy for the synthesis of reliable and reproducible self-assembled hydrogel films.226
3.3.2 3D nanostructures of hydrogels on particles.
Polysaccharide-based self-assembled polyelectrolytes including cellulose,231 HA,232 CS,217,233,234 alginic acid,235 pectin,236 gellan gum,237 alginate,238etc. (so-called physical hydrogels), can be prepared via electrostatic attraction of anions and cations without any chemical cross-linkers, and their final structures and properties are not only controlled by thermodynamics, but also related to the spatiotemporal sequence of cues that trigger self-assembly.235 Ultimately these hydrogels exhibit excellent biosafety, degradability, reversibility, and favorable drug-loading and release properties.239 These honeycomb or network-like frameworks composed of polymers usually possess a high porosity and interconnected pores which provide channels for drug diffusion, as well as cell adhesion and proliferation.217,233,240 The distribution characteristics of these pores of hydrogel films are non-uniform and are accompanied by various pore sizes and a high local density packing. Local fractures also appear in the 3D framework, caused by the strength of the driving force.217,233 Therefore, one can clearly find messy skeleton structures of the self-assembled hydrogels, rather than the regular structure similar to the football olefin or the Rubik's cube, although only changes in the morphology of hydrogels can be noticed from the macroscopically (Fig. 12).
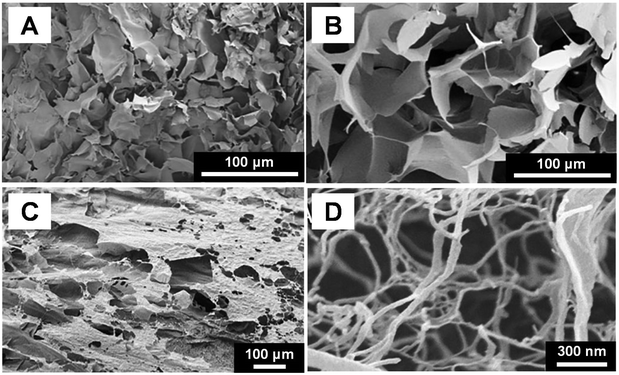 |
| Fig. 12 SEM images of the cross-sectional morphology of freeze-dried polysaccharides salecan hydrogel (A) and N,N,N-trimethyl chitosan hydrogel (B).217 (C) and (D) SEM images of hydrogel formed by cellulose nanofibrils.240 Reproduced with permission from ref. 217 and 240. Copyright © 2019, Elsevier; and Copyright © 2018, Acta Materialia Inc., published by Elsevier. | |
Polypeptides have received extensive attention since they not only self-assemble into supramolecular structures, but they can also influence cell adhesion and differentiation by regulating their own biochemical and physicochemical properties. Notably, hydrogels formed by covalent cross-linking of polypeptides triggered by UV radiation, extreme pH, high temperature, or chemical agents such as glutaraldehyde often lack biocompatibility, which limits their applications in the fields of drug delivery vectors and tissue engineering. While such strong non-covalent interactions as hydrogen bonding, hydrophobic or electrostatic interactions provide feasible solutions for the preparation of transient, reversible polypeptide-based hydrogels, this may be at the expense of self-assembly, healing, stability, and flexibility, which can be compensated for by a reasonable combination of peptides.
In addition, anisotropic particles with complex, non-spherical, and asymmetric structures are favored because of their unique advantages, such as anisotropic responses to external forces, anisotropic building blocks for complex structures, which makes them attractive in applications for drug delivery systems, cell culture, and biosensing.241 For example, Marquis and co-workers adapted dimethyl carbonate as continuous phase, or deforming pre-gelled droplets off-chip at a fluid–fluid interface to synthesize pectin hydrogel microparticles with ellipsoid, torus or mushroom-type morphologies. The connection between microfluidic diffusion-induced self-assembly and surface porosity was revealed by the microstructure of the surface of pectin hydrogel microparticles. In the case of internal gelation, the surface of the hydrogel was irregular and the pore size distribution of the microparticles surface ranged from 100 to 600 nm. Mushroom-shaped microparticles self-assembled to the undulating surface through a type of cap-to-tail binding in a topology-like manner and displayed rather loosely interconnected anisotropic “domains” (with a star-like structure) and local voids on the tail surface, which indicated that the gel crosslinking was not uniform, leading to the imperfect self-assembly structure236 (Fig. 13).
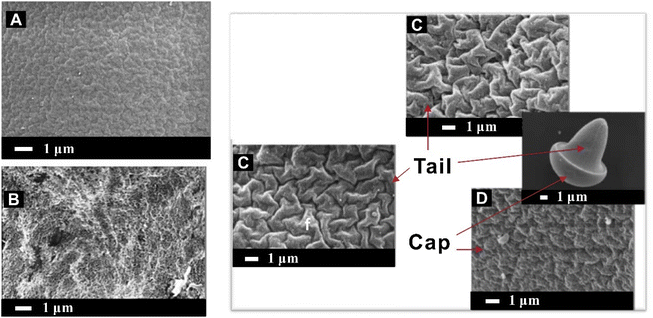 |
| Fig. 13 SEM micrographs of the surface of pectin hydrogel microparticles.236 (A) Surface of pectin hydrogel microparticles when solvent diffusion applied; (B) surface of pectin hydrogel microparticles without solvent diffusion; (C) tail surface of the mushroom-like morphology microparticles; (D) cap surface of the mushroom-like morphology microparticles. Reproduced with permission from ref. 236. Copyright © 2014, Elsevier. | |
For example, combining positively charged poly-L-lysine with negatively charged Fmoc-FF enhanced the coordination and self-healing properties of the resulting hydrogels, which exhibited a dense network-like structure composed of long nanofibers.242 Porous hydrogels with independently tunable biochemical, mechanical, and physicochemical performance parameters can be synthesized from self-assembled peptide amphiphile and PEG without any chemical modification or involvement of additional polymers. This hydrogel presented an opportunity for tissue engineering and regenerative medicine applications.243 Through the elimination of electrostatic repulsion of PBS, the polypeptide fibers and liposomes completed the gradual self-assembly, realizing the elongation, winding and gelation of the fibers, and the spherical liposomes were wrapped on the surface of the membrane structure by the fibers. Although not structurally appealing, this hybrid gel system combines the responsiveness of peptides to biological signals with the flexibility of loading and controlled release of liposomes.244
In addition, the microstructure and mechanical properties of self-assembled peptide hydrogels are closely related to cell proliferation, differentiation, and migration, which in turn can be determined by controlling the gelation speed through pH, ionic strength, and temperature. In general, higher ion concentrations can accelerate the hardening process of the gel, resulting in a more robust structure with smaller non-uniform mesh sizes, and extreme pH can cause this result as well.245,246 Relatively milder conditions will result in the formation of more homogeneous, relatively soft hydrogels, although the microstructures are not completely ordered.245
Proteins are formed from one or more peptide chains, and the highly cooperative coordination of their subunits gives them enormous advantages for self-assembly.247 The amino acid residues are essentially weak electrolytes, which are distributed on the surface of the protein and endow the protein with different charge properties under different conditions, which provides the basis for the protein to complete self-assembly through electrostatic interactions.248 But these amino acids are usually unevenly distributed and form localized charge centers, which are related to the heterogeneity of the protein surface and ultimately cause imperfections in the protein structure. Although strong electrostatic attraction results in periodically ordered protein-based gels, weak interactions are generally not sufficient to induce self-assembly.247 However, a number of protein-based hydrogels have been synthesized from self-assembled protein fibrils and have been employed in numerous applications. Fibrinogen contains important binding sites to facilitate cell interaction and is able to form 3D hydrogels and nanofibrous fibrinogen scaffold.249–253 For instance, Brüggemann's group introduced a simple and well-controllable bio-fabrication method to prepare nanofibrous fibrinogen hydrogel scaffolds by salt-induced self-assembly.249 Fibrinogen molecules change their conformation and are oriented during drying in the presence of salt ions. As the concentration of protein gradually increases upon drying, the oriented molecules aggregate into fibrils and induce fibrillogenesis220 (Fig. 14A). The structure of fibrinogen hydrogels induced by phosphate ions revealed a highly fascinating but not perfect porous fibrous network251 (Fig. 14B). Fibrinogen hydrogels have great potential for wound-healing applications since they support fibroblast adhesion, prevent E. coli infiltration,250 and their multiscale structures can be well-regulated without inducing any pathogenic amyloid transitions.252 Besides, amyloid fibrils also were investigated to form hydrogels which were triggered by various stimuli, such as heating/cooling,254,255 changes in pH,256 or chemical additives.257 Das and co-workers developed a series of amyloid hydrogels based on the self-recognition motif of α-synuclein for promoting stem cell differentiation to neurons.254 Hu and co-workers found that amyloid fibrils can be driven into reversible hydrogels with antibacterial activity by adding natural polyphenols to amyloid fibrils present in the nematic phase257 (Fig. 14C). They also reported that flavonoid–amyloid fibril hybrid hydrogels inhibited the core molecular links between gut microbes and host intestinal lipid absorption for obesity control.258 Although the morphology of amyloid fibril hybrid hydrogels was homogeneously distributed in the AFM image (Fig. 14D), the arrangement of fibers was not orderly. Nonetheless, protein-based hydrogels still have great potential in the field of biomedicine.
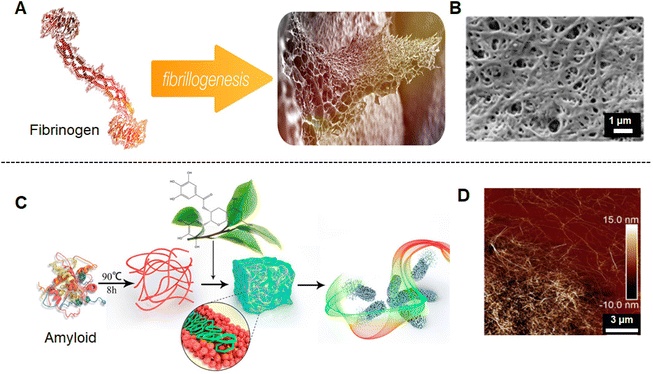 |
| Fig. 14 (A) Schematic illustration of fibrinogen assembles into fibrinogen hydrogel.251 (B) SEM image of fibrinogen hydrogel treated with phosphate ions after 5 h.251 (C) Schematic illustration of polyphenol-binding amyloid fibrils self-assembly into reversible hydrogels with antibacterial activity.257 (D) The AFM image of the morphology of the epigallocatechin gallate (EGCG)-induced amyloid fibril hydrogel.257 Reproduced with permission from ref. 251 and 257. Copyright © 2021, The authors, published by American Chemical Society; and Copyright © 2018, American Chemical Society. | |
DNA-based materials exhibit outstanding properties attributed to the supramolecular interactions among complementary base pairings of nucleic acids.259 Additionally DNA, as a strongly hydrophilic polyelectrolyte, is capable of absorbing large amounts of water, which sparks interest in DNA for hydrogel design.260 Among the most common chemical and physical techniques (including self-assembly, enzyme ligation, rolling circle amplification, and hybridization chain reaction) of synthesizing DNA-based hydrogels, self-assembly of Y-type DNA (three-way junction) by extended sticky ends was used to make the simplest form of DNA hydrogels.259 Xing and co-workers proposed a Y-shaped DNA and a double-stranded DNA as two kinds of building blocks to create pure DNA hydrogels with designable thermal and enzymatic responsive properties (Fig. 15A). The sticky ends of the Y-shaped DNA and double-stranded DNA as linkers are complementary to each other, and this hybridization may result in DNA-based hydrogel formation.261 Ma and co-workers designed floxuridine-containing DNA and RNA as building units and further assembled into a nucleic acid-based spherical nanogel that exhibited excellent inhibitory activity against cancer cells.262 As for single-stranded DNA (ssDNA), Hu and co-workers reported on the construction of pH-responsive DNA hydrogel based on the self-assembly of one short ssDNA (Fig. 15B). It was revealed that the self-assembly between the short ssDNA strands resulted in the formation of long linear DNA structures and looped structures, while the entanglement between these DNA assemblies at high concentrations led to the formation of 3D hydrogel networks.263 The microstructure of the freeze-dried DNA hydrogel was full of dense but irregular pores (Fig. 15C), confirming the existence of a cross-linked network structure of the system. DNA-based hydrogel as a novel kind of smart hydrogel with programmable functions, stimuli-responsiveness, excellent biocompatibility, and inherent biodegradability has high potential in biomedical applications.263,264
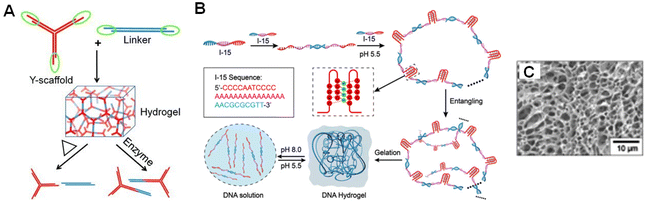 |
| Fig. 15 (A) Schematic illustration of DNA hydrogel formed from Y-shaped DNA. The DNA hydrogels exhibit thermal and enzymatic responsive properties.261 (B) Schematic representation of the self-assembly of ssDNA (I-15) into a pH-responsive DNA hydrogel.263 (C) SEM image of the freeze-dried I-15 DNA hydrogel.263 Reproduced with permission from ref. 261 and 263. Copyright © 2011, Wiley; and Copyright © 2022, American Chemical Society. | |
Hybrid hydrogels with the incorporation of inorganic ions have attracted great attention in recent years for their excellent mechanical properties. Inorganic components including SiO2,265 CaCO3,266 Fe3O4 particles,267 graphene oxide,268 nanoclay,269 and carbon nanotubes270 have been introduced into organic hydrogels to expand their applications. For instance, Abalymov and co-workers designed a hybrid thermally annealed hydrogel with sodium alginate and CaCO3, which has a porous and irregular particle arrangement morphology.271 These hybrid hydrogels have tunable mechanical properties and were employed to control cell adhesion and proliferation.271,272 Zhuang and co-workers demonstrated that an alginate/reduced graphene oxide double-network hybrid hydrogel exhibited a higher Young's modulus and a lower swelling ratio than the single-network hydrogel, which led to improved gel stability in highly concentrated alkali/salt solutions.273 Inorganic-doped hybrid hydrogels would be suitable representatives of toughened hydrogels because of the versatile functionalization of inorganic nanoparticles and their excellent mechanical strength and large specific surface area.265,274
3.4 Other structures
A large variety of other structures exist in which perfect and imperfect self-assembly was used. These include assemblies composed using hydrophobic, magnetic and forces upon evaporation including such materials as quantum dots, nanocrystals, magnetic nanoparticles or magnetic nanoparticle-assisted assembly, and acoustic levitation. These structures are different from the assemblies described above, but they constitute an additional class of materials where perfect (high) and imperfect (order) is either used or is relevant for their functions. For instance, Co nanocrystals with low size distribution can self-assemble either in fcc colloidal crystals to form supracrystals or in films with voids.275 Au nanocubes, used as model building blocks, were used in acoustic levitation self-assembly.276
4. Applications
In this section, we mainly discuss different biomedical applications of polymer brushes, LbL self-assembly nanostructures, and hydrogels, which have been summarized in Table 2.
Table 2 Selected applications of polymer brushes, LbL assembly, hydrogels and other structures
|
Materials |
Type of structure |
Functions |
Applications |
Ref. |
Polymer brushes |
Semiconducting polymer |
3D |
Light-responsive |
Cancer therapy |
351
|
|
|
Specific release |
|
|
|
Inhibits tumor growth |
|
|
|
Deliver CRISPR/Cas9 cassettes |
Gene therapy |
349
|
|
|
Control the endolysosomal escape |
|
|
|
Photothermal conversion |
|
LbL assembly |
Heparin + chitosan + polyurethane-coated decellularized scaffold |
2D |
Decreased hemolysis rate |
Vascular tissue engineering |
156
|
|
|
Prolonged in vitro coagulation time |
|
|
|
Enhanced resistance to platelet adhesion |
|
β-Cyclodextrin-modified silk fibroin + adamantane-modified hyaluronic acid |
2D |
Self-healing |
Nerve tissue engineering |
375
|
|
|
Antibacterial activity |
|
|
|
Enhanced cells proliferation |
|
Carbon nanotube + cellulose acetate nanofibers + chitosan |
3D |
Promote fibroblast attachment, spreading, and proliferation |
Tissue engineering |
301
|
Chitosan + caseine + corona pretreated polylactic acid substrates |
2D |
Drug loading |
Drug delivery |
319
|
|
|
Prolonged release |
|
Chitosan + dextran sulfate |
3D |
Drug loading |
Anti-cancer drug delivery |
376
|
|
|
Controlled release |
|
|
|
Inhibition of HepG2 cells |
|
Silica nanoparticles + poly-L-ornithine + carboxymethyl lentinan |
3D |
High drug loading |
Anti-cancer drug delivery |
377
|
|
|
Sustained release |
|
|
|
Anti-proliferative |
|
Indium tin oxide electrodes + polyallylamine hydrochloride + folic acid |
2D |
Detect cervical cancer cells |
Cancer diagnosis |
378
|
Poly(lactide-coglycolide) + (poly L-ornithine/fucoidan)4 |
3D |
Drug loading |
Therapeutic |
379
|
|
|
Controlled release |
|
|
|
Anti-tumor activity |
|
Silica nanoparticle + per-O-methyl-β-cyclo-dextrin-grafted-hyaluronic acid + TPPS4 |
3D |
Drug loading |
Therapeutic and diagnosis |
195
|
|
|
Preferential accumulation in tumor site |
|
|
|
Inhibited the tumor progression |
|
|
|
NIR fluorescence/MR imaging |
|
Hydrogels |
Polyethylene glycol-based Fmoc-FF peptide hybrid polyurethane + curcumin |
3D |
Self-healing |
Tissue engineering |
380
|
|
|
|
Suppressing the inflammation response |
|
|
|
|
Improve the cutaneous wound healing |
|
|
Helical N-acetyl-β3-peptides |
3D |
Compatibility with neural cells |
Neural tissue engineering |
381
|
|
|
|
Cells adhesion and proliferation |
|
|
Graphene oxide + nano-hydroxyapatite |
3D |
Enhance the proliferation, ALP activity and osteogenic gene expression |
Bone tissue engineering |
382
|
|
|
|
Healed calvarial defects |
|
|
Coumarin-tris derivatives |
3D |
Stimuli responsive |
Drug delivery |
383
|
|
Fmoc-FF peptide + alginate |
3D |
Sustained and controlled release |
Drug delivery |
341
|
|
Alginate + carboxymethyl cellulose |
3D |
pH-Sensitive |
Drug delivery |
384
|
|
|
|
Sustained release |
|
|
Alginate + silver nanoparticles |
3D |
Laser-induced |
Drug delivery |
363
|
|
|
|
Enhance the Raman signal |
Detector |
|
Alginate + silver nanoparticles + calcium carbonate |
3D |
Enhance the Raman signal |
Drug delivery |
362
|
|
|
|
|
Detector |
|
|
|
Ultrasound-induced |
Drug delivery |
209
|
|
|
|
Enhance the Raman signal |
Detector |
|
Rhein |
3D |
Sustained release |
Neuroinflammatory therapy |
385
|
|
|
|
Reversible stimuli-responses |
|
|
|
|
Bind to toll-like receptor 4 |
|
|
DNA (unmethylated cytosine-phosphate-guanine oligonucleotides) |
3D |
Drug loading |
Antitumor therapy |
386
|
|
|
|
pH-Responsive |
|
|
|
|
Controlled release |
|
|
|
|
Induced apoptosis of tumor cells |
|
|
Gold nanobipyramids conjugated silica nanoparticles + azobenzene and α-cyclodextrin-functionalized hyaluronic acid |
3D |
Sustained and selective drug delivery |
Chemotherapy and photothermal theranostic platform |
364
|
|
|
|
Long-term drug release |
|
|
|
|
NIR and enzyme dual responsive |
|
|
|
|
Attach to tumor cells |
|
Other structures |
Semiconductor, metal and magnetic particles |
2D or 3D |
Sensors and biosensors |
SERS |
367 and 387 |
|
|
Light responsive |
Detectors |
4.1 Tissue engineering
Tissue engineering is an interdisciplinary subject evolving from biomaterials and engineering development, and aims at the formation and regeneration of tissues or organs by assembling scaffolds, cells, and functional molecules into tissues.290–292 To mimic the extracellular matrix of native tissues, the ideal scaffolds must meet some specific requirements, involving structural, physical, chemical, and biological properties and functions.293,294 Taking into account the requirements, various multilayer nanofilms, nano-coatings, and 3D hierarchical structures have been designed for tissue engineering.
4.1.1 LbL self-assembly nanostructures.
Multilayer nanofilms constructed by the principle of nanoarchitectonics can direct cellular phenotypes like cell adhesion, cell growth, and cell differentiation. For instance, Col and HA were deposited on the plasma membrane of live mesenchymal stem cells (MSCs) using the LbL assembly technique295 (Fig. 16A). Although Col/HA multilayer films did not entirely block the cell plasma membrane surface, instead of leaving a vast extent of the plasma membrane in a naked state, this structure displayed openness and flexibility. The multilayer Col/HA film significantly supported cell survival in the attachment-deprived culture condition even in its mesh-like form. Moreover, films induced cellular metabolic activity and upregulated osteogenic differentiation with no deteriorating effect or inhibition of cellular attachment. Nano-coatings acted as protective shells for cells and as a way to functionalize the cell surface by modulating the physiochemical properties of the coating such as thickness, components, and charge of multilayers. For example, a LbL multilayer assembly method was employed to coat the MSCs’ surface with hydroxyapatite (HAp) and Col for bone tissue engineering applications.296 The cellular reactions of MSCs including adhesion, proliferation, and osteogenic differentiation phenotype were examined by altering the surface contents of HAp and Col on the micro-structured scaffold. Encapsulation of cells has been demonstrated to effectively control cell functions through modulating the communication to the cellular environment, providing protection for cells against physical damage, or attenuating deleterious host responses. Shin and co-workers successfully created multilayered dense and tightly connected cardiac tissue by alternately depositing cardiomyocytes and PLL-coated graphene oxide (PLL-GO)297 (Fig. 16B). The architecture of the multilayer constructs improved cardiac cell organization, maturation, and cell–cell electrical coupling, and enhanced biological activity, mechanical integrity, ease of handling, and retrievability of engineered tissue. Single cells were also encapsulated into LbL-assembled fibronectin-gelatin (FN-G) nanofilms by Nishiguchi and co-workers.298 This cell accumulation technique by FN-G encapsulation of single-cell surface easily provided approximately 8L of 3D tissues after only one day of incubation, and the layer number, cell type, and location were all successfully controlled by changing the number and order of seeded cell.
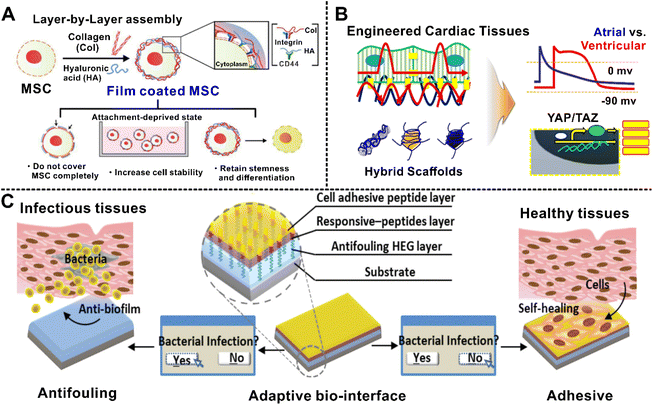 |
| Fig. 16 (A) Schematic illustration of the Col/HA LbL film on MSCs. Col and HA can bind with integrin and CD44, respectively, forming a stable multilayer on the MSC. The multilayer Col/HA films increased cell survival in the attachment-deprived culture condition.295 (B) Schematics displaying electric signal propagation through hybrid hydrogels in cardiac tissue engineering.297 (C) Illustration of the adaptive biointerface with dual responsiveness for tissue engineering. A cell-adhesive peptide layer, an infectious-environment-responsive peptide, and antifouling HEG layers were included in the self-assembled peptide surface. The adaptive capability of this biointerface was realized with a smart responsiveness toward healthy and infectious tissues.310 Reproduced with permission from ref. 295, 297 and 310. Copyright © 2017 and 2019, American Chemical Society; and Copyright © 2015, Wiley. | |
Compared with single inorganic or organic particles, hybrid systems based on LbL self-assembled polyelectrolytes, especially the multilayer polymer coating of inorganic or organic particles, have a relatively suitable structure with rough surfaces and reasonably tunable mechanical properties determined by the cross-linking mechanism of the polymers and the characteristics of the LbL self-assembly technique, which will facilitate cell adhesion, migration, proliferation, and allow filling of tissue defects. For example, the biomedical applications of polyester materials are restricted due to their hydrophobicity and weak bioactivity. The lack of appropriate cell-binding signals leaves them undesirable for cell adhesion and proliferation.299 It has been documented that modification of the scaffold surfaces with natural macromolecules by LbL self-assembly is a promising approach to mimic the organic component of nature and improve the biological properties of the substrates. He and co-workers constructed laminin/chitosan multilayers on poly-L-lactic acid (PLLA) nanofibers to provide a better environment for neuron growth and neurite outgrowth. Higher amounts of laminin embedded in the architecture and maintained a secondary structure close to their native form that better assisted in promoting cell proliferation than PLLA nanofibers.300 However, most research has focused on multilayer coating on nanofibers301,302 or metal alloys,303,304 and the functionalities of LbL coating on particles for tissue engineering need to be further conducted.
4.1.2 Hydrogels.
Self-assembled hydrogels with interconnected porous structures can provide channels for cell growth and migration, and the rough surface supports cell adhesion, growth, and differentiation as well.305–307 Such hydrogels have important applications as 3D scaffolds for tissue engineering. For example, unmodified chitosan hydrogel-encapsulated mesenchymal stem cells and chondrocytes can effectively support the proliferation and deposition of extra chondral matrix, and the addition of native type II collagen and chondroitin sulfate increases cell cohesion and cartilage-like cluster formation, and further promotes the regeneration of cartilage.308 The chitosan/polyglutamic acid hydrogel formed without the participation of any toxic cross-linking agent shows good shape-retaining ability, and the interconnected porous and rough surface structure are ideal for cell adhesion. It creates a favorable biological environment for tissue growth and proliferation, and also provides efficient channels for tissue growth and nutrient exchange, ultimately effectively inducing the growth of osteoblasts. The percentage of new bone formation after six weeks of hybrid system containing calcium sulfate dihydrate was as high as 70%.305 Self-assembled peptide-based hydrogels with 3D network structures which are similar to polysaccharide-based hydrogels have been explored for use as 3D tissue engineering scaffolds as well, due to their good biocompatibility, immunogenicity, degradability, and non-toxic byproducts, and have been extensively reviewed.221,309–311 The self-assembled peptide can be assembled as a dual-responsive bio-interface for infection prevention and tissue self-healing310 (Fig. 16C). These peptides can be induced to self-assemble to form hydrogels through changes in pH and ionic concentration, and hydrogels with a reasonable strength and enhanced specific tissue priorities (e.g., osteogenic differentiation, drug delivery, or tunable gelation) can be obtained through modulation of peptide sequence and peptide concentration.312–314 They can be easily injected and can be used as bone-filling materials, and their inherent network structure with relatively rough surface is conducive to cell adhesion, proliferation, migration, and differentiation.315 For example, the hydrogels comprising β-sheet-forming peptides rich in aspartic amino acids and of tricalcium phosphate (β-TCP)-loaded hydrogels can significantly induce osteoblasts in vitro, and in vivo studies on bone defect healing in rat distal femurs analyzed by micro-computerized tomography showed that the peptide hydrogel itself induced better bone regeneration in comparison with non-treated defects.316
4.2 Drug delivery
The development of smart drug-delivery systems for controlled drug-loading and manner of release has been an active area of research.317,318 Many aspects are essential when considering the design of novel drug-delivery platforms, including drug dosage, loading efficacy, controllable release, side effects, and toxicity. Hierarchical self-assembled systems offer impressive advantages of tailorability and versatility, which are crucial for controlled and targeted drug release.
4.2.1 LbL self-assembly of nanostructures.
Multilayer polysaccharide-based films are capable of high drug loadings due to their stable and porous structures. For example, the LbL deposition technique was applied for the build of multilayers which were performed by alternate dip-coating of corona pretreated polylactic acid substrates into chitosan and casein solutions.319 The cross-linking process of chitosan and casein resulted in stable and porous film structures, leading to the highest benzydamine loading of 217.4 μg cm−2. Drug-loading efficacy can also be readily tuned by changing the number of layers. Albright and co-workers have demonstrated that the antibacterial efficacy of antimicrobial-hosting coatings was enhanced by a simple increase of the number of assembled polymer layers.320 Multilayer films have abilities to protect drug molecules from harsh environmental factors which can cause the loss of therapeutic effects, and control the drug release rate by the rate of diffusion or by the rate of their dissolution or degradation. For instance, Redolfi Riva and co-workers reported a free-standing polymeric ultrathin film, composed of poly(methylmethacrylate) (PMMA), chitosan, and sodium alginate multilayers, which was loaded with anti-inflammatory drugs.321 The structural layer of PMMA augmented the mechanical performance of the films and hindered the dispersion of drugs by acting as a barrier for the directional release. Drug-release modeling with the Korsemeyer–Peppas model illustrated that drug release was characterized by a diffusion-controlled mechanism. Mohanta and co-workers fabricated LbL-assembled multilayer thin films and hollow microcapsules employing nano-crystalline cellulose (NCC) and chitosan as a carrier for drug delivery322 (Fig. 17A). The films have porous nanofibrous morphology and can be loaded with a substantial amount of the hydrophilic drug doxorubicin (Dox). The films also showed favorable interaction of NCC with the water-insoluble drug curcumin via hydrogen bonding and van der Waals interactions, in molecular docking studies. Both Dox and curcumin were released continuously in physiological conditions simulated by PBS buffer of pH 7.4. The sustained release manner of this film drug-delivery system prolongs the availability of drugs at the site of action. Hybrid inorganic–organic films fulfill great potential for antimicrobial drug delivery due to the synergetic effects of polymer with inorganic materials. Türk and co-workers developed an innovative LbL coated titanium hydroxide-(gentamicin-polydopamine) hybrid drug delivery platform323 (Fig. 17B). The multilayer coatings containing gentamicin demonstrated an improved drug-release time and in vitro inhibitory effect on the growth of S. aureus.
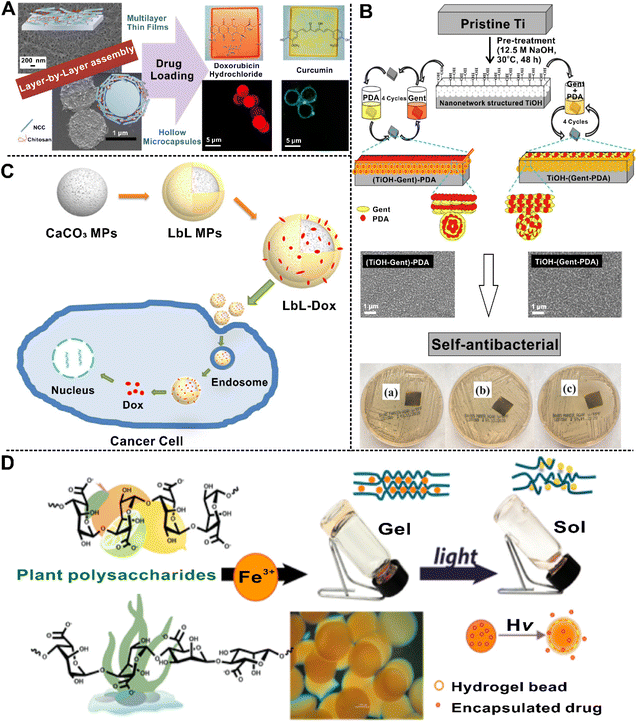 |
| Fig. 17 (A) Schematic representation of multilayer thin films and hollow microcapsules as a carrier for Dox and curcumin delivery.322 (B) Schematic illustration of fabrication processes of non-simultaneous titanium hydroxide-gentamicin-polydopamine ((TiOH-Gent)-PDA) and simultaneous TiOH-(Gent-PDA) LbL hybrid coatings. The multilayer hybrid drug delivery platforms demonstrated an inhibitory effect on the growth of the S. aureus.323 (C) Schematic representation showing the outline of the LbL MPs design and the effect of the MPs in the tumor environment.175 (D) Schematic illustration of light-responsive Fe3+-polysaccharide coordination hydrogels for controlled drug delivery.339 Reproduced with permission from ref. 322, 323, 175, and 339. Copyright © 2014, American Chemical Society; Copyright © 2021, Elsevier; Copyright © 2018, Springer; Copyright © 2015, American Chemical Society. | |
The LbL self-assembly systems based on particles have a high loading efficiency which is provided by the porosity of carriers. The trapping effect of the network structure will hinder the short-term and rapid release of drugs, and finally form a good drug sustained-release system. For example, the hybrid drug-delivery system formed by CaCO3 particles combined with various polyelectrolyte coatings has higher stability and longer-lasting drug-release efficiency than single nanocarriers without coating175 (Fig. 17C). Because the polyelectrolyte multilayer can stably exist in an acidic environment and undergo network shrinkage, the drug-burst effect caused by the easy degradability of CaCO3 in an acidic environment is alleviated.324,325 In addition, the multifunctional groups on the surface of the polyelectrolyte multilayer make surface modification easier, which is more efficient for cellular uptake and the targeted induction of hybrid carriers.158,326 Importantly, the preferential ionization of the outer layer will change the permeability of the multilayer in the medium with different environments and time, and finally shows different responsive release behaviors. For example, Elbaz et al. adopted poly-L-arginine/alginate to preferentially prepare two bilayers, followed by poly-L-arginine/Eudragit L100 and/chitosan/Eudragit L100 as the fifth and sixth layers, respectively. The former has no pH responsiveness, showing a delayed release effect in an acidic environment, but a rapid release in the intestinal environment; the latter shows pH responsiveness, which can effectively protect the drug in the gastric acid environment, and also plays an important role in limiting drug release in the intestinal environment, which is of great value for colon-targeted drug delivery.327 Also, the composite multilayer can provide a diversity of encapsulated drugs and provide the combination therapy of segmented release.328 Moreover, the addition of multifunctional nanoparticles before the self-assembly of polyelectrolyte multilayers will also confer stimuli-responsiveness to the hybrid carrier.329,330 Some polyelectrolyte multilayers have pH-dependent ionization degree, near-infrared light, and thermal sensitivity, and the resulting polymers show good pH, light, and thermal responsiveness, which provides a basis for targeted drug release.331–333
4.2.2 Hydrogels.
As mentioned above, self-assembled hydrogels have reliable biocompatibility compared with the hydrogels formed by chemical cross-linking and possess the general properties inherent in hydrogels, including high water content and network-like structure. It should be noted that this type of hydrogel has good self-healing properties and strength, which gives them great potential in drug delivery systems.334 For example, a 3D network-like self-assembled hydrogel system composed of anionic TEMPO-oxidized cellulose nanofibers and cationic guar gum through electrostatic/hydrogen bonding has good self-healing and strength, showing a good sustained-release effect in gastrointestinal conditions, since the nano-locking effect produced by the network structure delays the release of the drug.335 This is also reflected in the double-membrane hydrogel drug delivery system formed by cationic cellulose and sodium alginate.336 Injectable hydrogels prepared from ethylene glycol and chitosan have good stability and coordinated heat sensitivity, and are considered to be a good alternative material for the treatment of intervertebral disc herniation.337 Polysaccharide hydrogels formed with HA and CS effectively achieved the side effects of MMP inhibitors.338 The self-assembled hydrogels formed from alginic acid and pectin have good light responsiveness, which provides effective guidance for the controlled release of drugs339 (Fig. 17D). Ding and co-workers constructed a degradable, reversible hydrogel using dual physical dynamic bonding of host–guest chemistry and electrostatic interactions. This delivery vehicle exhibits a good biocompatibility and controlled release of growth factors in applications such as wound healing and ischemic tissue repair.340 A polysaccharide–peptide hybrid hydrogel formed by calcium ion-triggered co-assembly of Fmoc-FF peptide and alginate has better performance than alginate hydrogel or Fmoc-FF hydrogel. The high stability may be due to the synergistic effect of non-covalent and ionic interactions, and the hybrid hydrogel microspheres have a dense surface structure and a porous nanofiber network inside, which exhibit a well-controlled release effect on docetaxel. The release index n indicates that docetaxel is released from the hybrid beads mainly in a Fickian diffusion-mediated manner.341 The controlled release of drugs from self-assembled peptide hydrogels can be achieved by adjusting the mechanical strength and porosity determined by the self-assembly conditions, combined with pH value and properties of drug molecules.342 In addition, the mechanical responsiveness, self-healing, and thixotropy of peptide hydrogels are crucial for injectable drug loading systems, which enable smooth drug delivery without destroying the carrier and blocking pinholes.343 Also, the self-assembled peptide sequence can be effectively combined with some growth factors, hormones, and protein drugs to form a non-toxic and biodegradable hybrid drug-loading system, so as to successfully deliver drugs to myocardial tissue.344,345
4.3 Theranostics
Nano-carriers can be exploited alone for therapeutic purposes or for a combination of therapeutic and diagnostic objectives. In such a combined application, the nanoplatforms can deliver both imaging and therapeutic agents for precision medicine.346,347 Nanoarchitectonics research has focused on the fabrication of various nanostructures that have the capability to be used for cancer diagnosis and treatment.348 These delivery carriers are useful for theranostics, particularly as multicompartment carriers, which can be envisioned to encapsulate sensor molecules in some compartments and medically relevant molecules in other compartments. Owing to their unique physical and optical properties, the functionalities of therapeutics and imaging diagnosis can be achieved.
4.3.1 Polymer brushes.
Semiconducting polymer brushes (SPBs) are employed as advanced photonic theranostic nanostructures with good biocompatibility, stable photophysical properties, and sensitive and tunable optical responses from ultraviolet to near-infrared (NIR) II window.349 SPB with a typical amphiphilic structure is convenient for forming semiconducting polymer particles (SPNs) in solvent via self-assembly, which can improve the optical-electronic and nano-structural properties.295,350 For example, Cui and co-workers reported that the SPBs structure grafted with chemo-drug side chains through hypoxia-cleavable linkers can be directly self-assembled for cancer photoactivated chemotherapy treatment.351 After systemic administration of semi-conducting polymer nanoprodrug (SPNpd) into the tumor-bearing living mice through tail vein, the NIR fluorescence images were longitudinally acquired to observe the biodistribution and accumulation of SPNpd at the tumor site. The mouse tumor growth was significantly inhibited because of the release of bromoisophosphoramide mustard intermediate and killing of cancer cells by inducing their DNA crosslinking. Li and co-workers designed an amphiphilic SPB that can self-assemble in water to encapsulate dexamethasone (Dex) for the remote control of clustered regularly interspaced short palindromic repeat (CRISPR)/CRISPR-associated protein 9 (Cas9) genome-editing349 (Fig. 18A). A tumor model was used to demonstrate that SPNs can accumulate in the tumor and showed accelerated Dex release and gene endolysosomal escape after laser irradiation. Subsequently, the payload release of CRISPR/Cas9 cassettes allowed highly efficient genome editing. The unique feature of SPBs-based NIR-II emission was useful for tracking and guiding genome editing in vivo, which increased the accuracy of the genome editing manipulation.
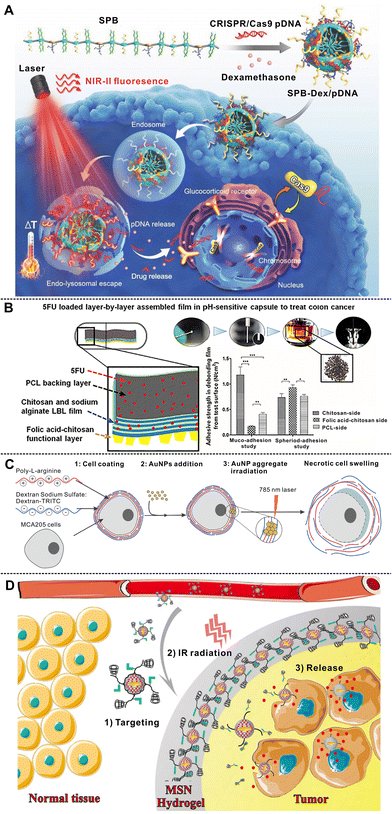 |
| Fig. 18 (A) Design of SPB-Dex nanoparticles for CRISPR/Cas9 delivery, and illustration of the intracellular genome editing process upon 808 nm laser irradiation.349 (B) Schematic representation of the construction of functionalized LbL self-assembled polymeric film, and the study of spheroid adhesion.352 (C) Schematic diagram of hybrid LbL-AuNP coatings around cells. By exposing LbL-coated cells with gold nanoparticle aggregates to a 785 nm laser, a strong localized heating can be induced resulting in necrotic cell swelling.353 (D) Schematic representation of the targeted drug delivery to tumor tissue based on Dox-loaded, polymer (azobenzene and α-cyclodextrin functionalized hyaluronic acid), and gold nanobipyramids conjugated mesoporous silica nanoparticles/hydrogel switchable system.364 Reproduced with permission from ref. 349, 352, 353 and 364. Copyright © 2019, Wiley; Copyright © 2020, Elsevier; Copyright © 2021, The authors, published by Elsevier; and Copyright © 2016, American Chemical Society. | |
4.3.2 LbL self-assembly nanostructures.
LbL self-assembled multilayer architectures are also highlighted in theranostics research because of their ease of design and the potential to achieve a variety of stimulus responses, such as pH, temperature, and light responsiveness. Janardhanam and co-workers prepared pH-sensitive functionalized LbL self-assembled polymeric film composed of chitosan and sodium alginate loaded with 5-Fluorouracil (5FU) as the model chemotherapeutic agent against colorectal cancer352 (Fig. 18B). Studies in a non-everted rat colon-sac model and open colon membrane model showed great permeation of 5FU across the colon wall when adhered to the chitosan side of LbL film. Cell monolayer and 3D-spheroid model studies indicated significant growth inhibition of cancer cells by 5FU-loaded film compared with free 5FU solution. Furthermore, a versatile theranostics nanoplatform can be archived by LbL self-assembly of metal particles and organic polymers. The unique photothermal conversion properties of metal nanoparticles suggested that they can perform as a phototherapeutic for cancer therapy. Our group proposed a new strategy of cancer fibrosarcoma MCA205 cells encapsulation in a hybrid coating containing LbL assembly polyelectrolytes functionalized with AuNPs aggregates353 (Fig. 18C). Upon exposure to laser illumination operating in a biologically “friendly” near-infrared spectral window, AuNPs aggregates can absorb the laser beam and convert it into heat, resulting in localized heat that caused immediate necrosis of the encapsulated cancer cells. Besides, functional inorganic nanoparticles such as silica nanoparticles can also be coated with polymers using the LbL technique, endowing them with additional characteristics, such as magnetic functions, electrochemical properties, and radioactivity, for various therapy and diagnostic applications. Mesoporous silica nanoparticles loading tirapazamine with LbL-assembled multilayer can be further constructed as an excellent photosensitive theranostic nanoplatform via host–guest interaction and the chelation with paramagnetic Gd3+. The nanoarchitectonics can be specifically taken up by CD44 receptor overexpressed tumor cells and respond to hyaluronidase to trigger the release of therapeutics, which exhibited a preference for accumulation at the tumor site and significantly inhibited the tumor progression. The in vivo tumor-targeting ability the nanoarchitectonics was investigated using NIR fluorescence and MR imaging.195 LbL organic nanoparticles for effective cancer photoimmunotherapy were realized through the combination of NIR therapy and regulatory T-cell modulation.354 LbL self-assembled poly(D,L-lactide-co-glycolide) (PLGA) nanoparticles coated with poly-L-histidine (PLH) and poly(ethylene glycol)-block-poly(L-glutamic acid) (PEG-b-PLG) with stimulus-responsive behavior, longer blood circulation, and better biocompatibility, can be successfully targeted to the Treg cells within the tumor microenvironment to abrogate their immunosuppressive function, which was expected to improve tumor ablation efficacy.354 Diagnostic functionality of LbL self-assembly nanostructures can include pH sensing,355 photothermal sensing,356 other ion-sensing capabilities357 or even mechanical sensing,203,358 which can also initiate the release.359
4.3.3 Hydrogels.
Self-assembled hydrogels have been employed to provide well-controlled drug release and targeted therapy in theranostics, affording greater patient comfort and efficient therapy. Injectable hydrogels have the potential for non-invasive administration, and can be delivered together with the aqueous pre-gel materials via syringe injection, and trapped at the target sites.360 Polymer-based hydrogels contain both natural and synthetic polymers with biocompatibility and robust structures, as well as a range of physiochemical and mechanical properties that can meet various requirements in vivo. Some inorganic materials such as metallic nanoparticles,209,361–363 silica,364,365 and carbon materials366 can be incorporated into hydrogel networks to render them multifunctional. Various applications have been developed using this type of structure, in which either electrostatic, hydrogen bonding or hydrophobic, steric, or magnetic forces have been used. A notable application is SERS, which enhances a weak Raman scattering signal.367 For example, in situ functionalization of the hydrogel capsules with silver nanoparticles can provide multiple functionalities: make the capsule's shell a sensor platform to enhance the Raman signal and as result detect the molecule; be an important structural element of the shell and enhance the mechanical property; and provide a sensitivity to the laser363 and ultrasound irradiation,209 which enable a remote release of the payload after administration.363 Chen and co-workers reported an injectable drug delivery system for sustained cancer treatment using polymer (azobenzene and α-cyclodextrin-functionalized hyaluronic acid) and gold nanobipyramids-conjugated mesoporous silica nanoparticles364 (Fig. 18D). The light-induced platform has the specific affinity to the CD44 antigen overexpressed on tumor cells, and in situ self-assembled into a hydrogel under NIR radiation. Due to the upregulation of hyaluronidase (HAase) around the tumor tissue, further degradation of the HA in the hydrogel will result in the release of drug from the hydrogel, which can then be taken up by tumor cells and delivered to the cell nuclei. The intrinsic characteristics of polysaccharides, especially their enzymatic degradation and non-immunogenicity, make them the preferred choice for hydrogel preparation for theranostics. Chitosan-based hydrogel can facilitate lysosomal degradation and does not induce immune responses, and has been employed as drug delivery carrier for cancer treatment.368 The initial burst release effect of the chitosan-based hydrogel can be inhibited by an alginate surface layer, which improved the sustained-release behavior and radiolabeling stability of the formulation.369 Notably, most cancers are associated with the abnormal activities of phosphatases and/or kinases. Peptide-based hydrogel can be used as a biocompatible molecular probe for detecting the activity of phosphatases and kinases, which is a simple and easy method for quick measurement. For example, Yang and co-workers designed a self-assembly/disassembly system of supramolecular hydrogel based on a phosphatase/kinase switch to examine the activities of phosphatase and kinases.370 The hydrogel turned into a gel-sol phase transition in the presence of adenosine triphosphates and kinase, then phosphatase was added to the resulting solution that transformed it back to the hydrogel.
Successful in vivo transformation indicated that this biomimetic approach for regulating the state of supramolecular hydrogel could be a promising way to detect the activities of phosphatases and kinases. Peptide-based hydrogel also acts as a carrier to deliver drugs to tumor sites, enhances drug stability, improves the optical properties of photosensitizers, and increases the immune response.371–373 A novel peptide-based self-assembled hybrid hydrogel with a high antitumor efficacy enabled photoacoustic and fluorescence imaging as demonstrated by Jin and co-workers, which served as a multifunctional vehicle for the delivery of drugs to tumor sites for cancer photothermal therapy.374
5. Conclusion and perspective
In this review, we describe recent research activity focusing on nanoarchitectonics. It is emphasized that architectonics at the nano-level, nanoarchitectonics, is different from that at macro- and micro-architectonics as well as atomic arrangements, particularly in crystals. Within the area of nanoarchitectonics, two sub-areas are described: self-assembly where order can be achieved and assembly with non-perfect order. Having provided a brief overview of the former, the focus of this review is on the latter area, where polymer brushes, LbL, and hydrogels are described. LB technology and lipids self-assembly are precise for constructing well-structured nano-units and have been summarized in many previous works. Conversely, nanostructures without perfect order and alignment have been classified as those based on 2D nanostructures (for example, for surface modification) and 3D nanostructures (for example, for encapsulation and drug delivery). According to different types of self-assembly, the principles of preparation are discussed, and the imperfect cross-linking structures are mentioned, and their biomedical applications are discussed as well. Polymer brushes, LbL nanostructure, hydrogels, and other structures are listed, revealing that each nanoarchitectonics assembly has its own characteristics and functionalities. (1) Polymer brush is one of the simplest strategies for functionalizing material surfaces, exhibiting fascinating physicochemical properties and versatile architectures. As a unique class of functional materials, polymer brushes combine the functionalities of matrix and the properties of tethered polymers, the self-assembly of polymers under solution can improve the nano-structural properties, including stimulus-responsiveness, superhydrophobicity, antifouling, and so forth. Therefore, they show great potential in theranostics by taking advantage of their structure and synergetic properties. (2) LbL assembly is an effective way for preparation of controlled nanoarchitectonics structures; both LbL assembly on organic/inorganic surfaces and particles are versatile and useful for a wide range of biomedical applications. Multilayer films constructed on flat surfaces using LbL assembly are advantageous for device structures and the preparation of biocompatible layered nanoarchitectures. The design of inorganic, organic, and hybrid LbL films is useful for development of ideas, protocols, and developments between these different research communities. LbL self-assembly structure on particles makes the physiochemical properties of particles complementary to that of polymers. High surface area may facilitate the increase of drug-loading concentration or even the spatial control of drug presentation. (3) Hydrogels are solid-like materials with large amounts of water, and are similar to the native extracellular matrix and possess the great ability to capture bioactive molecules. Natural supramolecular self-assembled hydrogels can improve cellular permeability and regulate the biological action of other pharmaceutical agents. The incorporation of particles in the matrix of hydrogel is a feasible approach to improve the strength of the hydrogel and reinforce the weak hydrogel network. Hybrid hydrogel provides multiple functions including sensitivity to several stimuli and programmable response. Even in nature not everything is perfectly ordered, like crystalline liquid, and tree skeleton made of cellulose; however, these structures still play quite a unique role there.
As mentioned above, although significant advances have been achieved with self-assembled hierarchical nanostructures, several challenges remain for their future developments. We believe that nanoarchitectonics is not a simple extension of what is observed in nanometer regions, and it is an amalgamation of many disciplines (such as supramolecular chemistry, materials fabrications, and biotechnology) and technologies (including microtechnology and nanotechnology). Nanoarchitectonics has two core missions for true development in nanoscience and related engineering outputs: (1) materials are architected through controlled and coordinated interactions to create unexpected functions, and (2) controlling nano-functions by simple macroscopic mechanical actions to utilize cutting-edge frontier science in daily life.388 However, the production of nano-sized objects has many uncertainty factors including thermal fluctuations, quantum effects, and statistical distributions, which may result in nano-specific phenomena that are unexpected or not easily controllable. Besides, most nano-sized objects have simple structures, which offer a limited level of nano-sized control. Other challenges are the selection of molecules and ‘decision-making’ based on the harmonization of multiple and sometimes ambiguous responses. Nanoarchitectonics based on self-assembly relies on both exploration of chemical designs389 and self-assembly processes.390 Self-assembly can be found in biomolecular evolutionary processes, which can be mimicked in artificial systems. In-depth studies of self-assembly processes of biological systems will help in establishing highly sophisticated functional nanoarchitectonics that have not been fully realized in artificial self-assembly. Moreover, by mixing two simple amphiphilic liquids,391 the self-assembly processes can bring a dramatic variation of materials even without creating entirely new molecules, and chemical modification can open up molecular engineering strategies to biology,29,392,393 and these procedures will provide improved building blocks for nanoarchitectonics for developing yet more applications, including nanomedicine.394
As discussed here, situated between micro- and atomic levels or sub-nano architectonics where fabricated objects typically follow the conceptual design, nanoarchitectonics produces structures which do not often follow the projected design due to the influence of molecular interactions. And in this area, the imperfect self-assembly methods discussed in this review provide a wealth of knowledge and enable a myriad of applications at this important nanoscale size range. All these factors make this area both interesting and challenging, underlining Richard Feynman's famous saying: “there is plenty of room at the bottom”, even if the bottom here is not at the lowest (atomic) size-scale level and even if it does not have a perfect (high) order assembly.
Abbreviations
STM | Scanning tunneling microscopy |
TEM | Transmission electron microscopy |
SEM | Scattering electron microscopy |
AFM | Atomic force microscopy |
CLSM | Confocal laser scanning microscope |
LbL | Layer-by-layer |
LB | Langmuir–Blodgett |
3D | Three-dimensional |
2D | Two-dimensional |
PSCs | Polymer single crystals |
P(SBMA-co-DMAEMA) | Poly[(sulfobetaine methacrylate)-co-(2-(dimethylamino) ethyl methacrylate)] |
PDMA | Poly(dopamine methacrylamide) |
MPBs | Molecular polymer brushes |
PS | Polystyrene |
PLA | Polylactide |
QDs | Quantum dots |
AuNPs | Au nanoparticles |
MoS2 | Molybdenum disulfide |
Co3O4 | Tricobalt tetraoxide |
MP | Methyl parathion |
PDDA | Poly(diallyldimethylammonium chloride) |
PSS | Poly(styrene sulfonate) sodium salt |
PABA | Poly(3-aminobenzylamine) |
DA | Dopamine |
ABA | 3-Aminobenzylamine |
APS | Ammonium persulfate |
PAA | Poly(acrylic acid) |
PEO-b-PCL | Poly(ethylene oxide)-block-poly(ε-caprolactone) |
S. aureus
|
Staphylococcus aureus
|
CMCH |
N-(2-Hydroxypropyl)-3-trimethylammonium chitosan chloride |
TA | Tannic acid |
E. coli
|
Escherichia coli
|
PEG | Polyethylene glycol |
DEX | Dextran |
SF | Silk fibroin |
Col | Collagen |
ALP | Alkaline phosphate |
SPEEK | Sulfonated polyetheretherketone |
AgNPs | Ag nanoparticles |
CNF | Cellulose nanofibril |
PDA | Polydopamine |
SP NPs | Silver phosphate nanoparticles |
MF | Melamine formaldehyde |
PAH | Poly(allylamine hydrochloride) |
PLO | Poly-L-ornithine |
HA | Hyaluronic acid |
MSN | Mesoporous silica nanoparticle |
TPPS4 | 5,10,15,20-Tetrakis(4-sulfonatophenyl)-porphyrin |
PAMAM | Polyamidoamine |
PGMA | Polyglycerol methacrylate |
PEI | Polyethyleneimine |
HPs | Hedgehog-shaped particles |
SERS | Surface-enhanced Raman scattering |
Fmoc-FF |
N-Fluorenylmethoxycarbonyl diphenylalanine |
EGCG | Epigallocatechin gallate |
ssDNA | Single-stranded DNA |
HAp | Hydroxyapatite |
PLLA | Poly-L-lactic acid |
PMMA | Poly(methylmethacrylate) |
Dox | Doxorubicin |
SPBs | Semiconducting polymer brushes |
Dex | Dexamethasone |
5FU | 5-Fluorouracil |
PLGA | Poly(D,L-lactide-co-glycolide) |
PLH | Poly-L-histidine |
PEG-b-PLG | Poly(ethylene glycol)-block-poly(L-glutamic acid) |
Author contributions
Lin Cao collected literature, wrote the first draft, and made subsequent modifications. Yanqi Huang provided support for literature collection and the first draft on the content of nanostructures for encapsulation and related applications. Bogdan V. Parakhonskiy and Andre G. Skirtach coordinated and organized this work, and provided valuable suggestions for improving the framework of the review and the final draft. Finally, all authors have contributed materially and intellectually through several joint papers on the development of self-assembly to nanoarchitectonics, which helped to shape its intellectual basis.
Conflicts of interest
The authors declare no conflict of interest.
Acknowledgements
This work is supported by the China Scholarship Council (CSC No. 202106910013 and 202006370040). This work is supported by FWO (Research Foundation Flanders): G043219N, G043322N, I002620N, and BOF-UGent: 01IO3618. This project has also received funding from FWO-F.N.R.S. under the Excellence of Science (EOS) program, grant number: 40007488. We would like to thank the Faculty of Bioscience Engineering, Department of Biotechnology of Ghent University for providing a good research platform.
References
-
M. J. Adler, in Aristotle for Everybody, Simon and Schuster, 1997 Search PubMed
.
- G. E. Smith, J. Anat., 1927, 61, 264–266 Search PubMed
.
- C. C. Hilgetag, S. F. Beul, S. J. van Albada and A. Goulas, Netw. Neurosci., 2019, 3, 905–923 CrossRef
.
- M. Aono and K. Ariga, Adv. Mater., 2016, 28, 989–992 CrossRef CAS PubMed
.
- D. Lin, Y. Liu, A. Pei and Y. Cui, Nano Res., 2017, 10, 4003–4026 CrossRef CAS
.
- J. Wan, S. D. Lacey, J. Dai, W. Bao, M. S. Fuhrer and L. Hu, Chem. Soc. Rev., 2016, 45, 6742–6765 RSC
.
- Y. Wu, H. Pang, Y. Liu, X. Wang, S. Yu, D. Fu, J. Chen and X. Wang, Environ. Pollut., 2019, 246, 608–620 CrossRef CAS PubMed
.
- F. Lu and D. Astruc, Coord. Chem. Rev., 2020, 408, 213180 CrossRef CAS
.
- A. Beck, L. Goetsch, C. Dumontet and N. Corvaïa, Nat. Rev. Drug Discovery, 2017, 16, 315–337 CrossRef CAS
.
- J. Shi, P. W. Kantoff, R. Wooster and O. C. Farokhzad, Nat. Rev. Cancer, 2017, 17, 20–37 CrossRef CAS PubMed
.
- K. Ariga, X. Jia, J. Song, J. P. Hill, D. T. Leong, Y. Jia and J. Li, Angew. Chem., Int. Ed., 2020, 59, 15424–15446 CrossRef CAS PubMed
.
-
C. Chircov and A. M. Grumezescu, Nanoarchitectonics in Biomedicine, Elsevier, 2019, pp. 1–21 Search PubMed
.
- H. Noh, C.-W. Kung, T. Islamoglu, A. W. Peters, Y. Liao, P. Li, S. J. Garibay, X. Zhang, M. R. DeStefano, J. T. Hupp and O. K. Farha, Chem. Mater., 2018, 30, 2193–2197 CrossRef CAS
.
- J. Fichtner, S. Watzele, B. Garlyyev, R. M. Kluge, F. Haimerl, H. A. El-Sayed, W.-J. Li, F. M. Maillard, L. Dubau, R. Chattot, J. Michalička, J. M. Macak, W. Wang, D. Wang, T. Gigl, C. Hugenschmidt and A. S. Bandarenka, ACS Catal., 2020, 10, 3131–3142 CrossRef CAS
.
- I. Ijaz, E. Gilani, A. Nazir and A. Bukhari, Green Chem. Lett. Rev., 2020, 13, 223–245 CrossRef CAS
.
- E. S. Madivoli, P. G. Kareru, A. N. Gachanja, D. S. Makhanu and S. M. Mugo, J. Inorg. Organomet. Polym., 2022, 32, 854–863 CrossRef CAS
.
- C. Jia, C. Chen, Y. Kuang, K. Fu, Y. Wang, Y. Yao, S. Kronthal, E. Hitz, J. Song, F. Xu, B. Liu and L. Hu, Adv. Mater., 2018, 30, 1801347 CrossRef
.
- K. Ariga and Y. Yamauchi, Chem. – Asian J., 2020, 15, 718–728 CrossRef
.
- T. Hasegawa, K. Terabe, T. Tsuruoka and M. Aono, Adv. Mater., 2012, 24, 252–267 CrossRef CAS
.
- Y. Dong, S. Zhang, Z. Wu, X. Li, W. L. Wang, Y. Zhu, S. Stoilova-McPhie, Y. Lu, D. Finley and Y. Mao, Nature, 2019, 565, 49–55 CrossRef CAS PubMed
.
- W. Cui, A. Wang, J. Zhao and J. Li, Adv. Colloid Interface Sci., 2016, 237, 43–51 CrossRef CAS
.
- F. Würthner, C. R. Saha-Möller, B. Fimmel, S. Ogi, P. Leowanawat and D. Schmidt, Chem. Rev., 2016, 116, 962–1052 CrossRef
.
- G. M. Whitesides and B. Grzybowski, Science, 2002, 295, 2418–2421 CrossRef CAS
.
- K. Ariga and J. Li, Adv. Mater., 2016, 28, 987–988 CrossRef CAS PubMed
.
- K. Ariga, Q. Ji, W. Nakanishi, J. P. Hill and M. Aono, Mater. Horiz., 2015, 2, 406–413 RSC
.
- K. Ariga, M. Li, G. J. Richards and J. P. Hill, J. Nanosci. Nanotechnol., 2011, 11, 1–13 CrossRef CAS
.
- K. Ariga, J. Li, J. Fei, Q. Ji and J. P. Hill, Adv. Mater., 2016, 28, 1251–1286 CrossRef CAS PubMed
.
- K. Ariga, Mater. Chem. Front., 2017, 1, 208–211 RSC
.
- K. Ariga, M. Nishikawa, T. Mori, J. Takeya, L. K. Shrestha and J. P. Hill, Sci. Technol. Adv. Mater., 2019, 20, 51–95 CrossRef CAS
.
- K. Ariga, T. Mori, T. Kitao and T. Uemura, Adv. Mater., 2020, 32, 1905657 CrossRef CAS PubMed
.
- Z. Sun, K. Ikemoto, T. M. Fukunaga, T. Koretsune, R. Arita, S. Sato and H. Isobe, Science, 2019, 363, 151–155 CrossRef CAS PubMed
.
- T. Mori, H. Tanaka, A. Dalui, N. Mitoma, K. Suzuki, M. Matsumoto, N. Aggarwal, A. Patnaik, S. Acharya, L. K. Shrestha, H. Sakamoto, K. Itami and K. Ariga, Angew. Chem., 2018, 130, 9827–9831 CrossRef
.
- K. Ariga, M. Ito, T. Mori, S. Watanabe and J. Takeya, Nano Today, 2019, 28, 100762 CrossRef
.
- B. L. Li, M. I. Setyawati, L. Chen, J. Xie, K. Ariga, C.-T. Lim, S. Garaj and D. T. Leong, ACS Appl. Mater. Interfaces, 2017, 9, 15286–15296 CrossRef CAS
.
- M. Gosak, R. Markovič, J. Dolenšek, M. Slak Rupnik, M. Marhl, A. Stožer and M. Perc, Phys. Life Rev., 2018, 24, 118–135 CrossRef
.
- K. Ariga, Y. Lvov and G. Decher, Phys. Chem. Chem. Phys., 2022, 24, 4097–4115 RSC
.
- J. Na, D. Zheng, J. Kim, M. Gao, A. Azhar, J. Lin and Y. Yamauchi, Small, 2022, 18, 2102397 CrossRef CAS PubMed
.
- S. Li, Q. Zou, R. Xing, T. Govindaraju, R. Fakhrullin and X. Yan, Theranostics, 2019, 9, 3249–3261 CrossRef CAS PubMed
.
- M.-P. Pileni, Acc. Chem. Res., 2007, 40, 685–693 CrossRef CAS PubMed
.
- A. G. Skirtach, C. Déjugnat, D. Braun, A. S. Susha, A. L. Rogach and G. B. Sukhorukov, J. Phys. Chem. C, 2007, 111, 555–564 CrossRef CAS
.
- B. V. Parakhonskiy, M. F. Bedard, T. V. Bukreeva, G. B. Sukhorukov, H. Möhwald and A. G. Skirtach, J. Phys. Chem. C, 2010, 114, 1996–2002 CrossRef CAS
.
- J. Kopeček and J. Yang, Angew. Chem., Int. Ed., 2012, 51, 7396–7417 CrossRef PubMed
.
- A. C. Mendes, E. T. Baran, R. L. Reis and H. S. Azevedo, Wiley Interdiscip. Rev.: Nanomed. Nanobiotechnol., 2013, 5, 582–612 CAS
.
- M. A. Boles, M. Engel and D. V. Talapin, Chem. Rev., 2016, 116, 11220–11289 CrossRef CAS
.
- Y. Lin, A. Böker, J. He, K. Sill, H. Xiang, C. Abetz, X. Li, J. Wang, T. Emrick, S. Long, Q. Wang, A. Balazs and T. P. Russell, Nature, 2005, 434, 55–59 CrossRef CAS
.
- M. P. Stoykovich, H. Kang, K. C. Daoulas, G. Liu, C.-C. Liu, J. J. de Pablo, M. Müller and P. F. Nealey, ACS Nano, 2007, 1, 168–175 CrossRef CAS
.
- P. Yin, H. M. T. Choi, C. R. Calvert and N. A. Pierce, Nature, 2008, 451, 318–322 CrossRef CAS PubMed
.
- K. H. Smith, E. Tejeda-Montes, M. Poch and A. Mata, Chem. Soc. Rev., 2011, 40, 4563 RSC
.
- T. P. Bigioni, X.-M. Lin, T. T. Nguyen, E. I. Corwin, T. A. Witten and H. M. Jaeger, Nat. Mater., 2006, 5, 265–270 CrossRef CAS
.
- J. Zhuang, H. Wu, Y. Yang and Y. C. Cao, J. Am. Chem. Soc., 2007, 129, 14166–14167 CrossRef CAS
.
- E. Rabani, D. R. Reichman, P. L. Geissler and L. E. Brus, Nature, 2003, 426, 271–274 CrossRef CAS PubMed
.
- D. V. Talapin, E. V. Shevchenko, C. B. Murray, A. V. Titov and P. Král, Nano Lett., 2007, 7, 1213–1219 CrossRef CAS
.
- D. Zherebetskyy, M. Scheele, Y. Zhang, N. Bronstein, C. Thompson, D. Britt, M. Salmeron, P. Alivisatos and L.-W. Wang, Science, 2014, 344, 1380–1384 CrossRef CAS
.
- S. Fischer, A. Salcher, A. Kornowski, H. Weller and S. Förster, Angew. Chem., 2011, 123, 7957–7960 CrossRef
.
- Y. Liu, Y. Li, J. He, K. J. Duelge, Z. Lu and Z. Nie, J. Am. Chem. Soc., 2014, 136, 2602–2610 CrossRef CAS PubMed
.
- R. L. Marson, T. D. Nguyen and S. C. Glotzer, MRS Commun., 2015, 5, 397–406 CrossRef CAS
.
- B. Cabane, J. Li, F. Artzner, R. Botet, C. Labbez, G. Bareigts, M. Sztucki and L. Goehring, Phys. Rev. Lett., 2016, 116, 208001 CrossRef
.
- Z. Tang, N. A. Kotov and M. Giersig, Science, 2002, 297, 237–240 CrossRef CAS
.
- R. Jin, G. Wu, Z. Li, C. A. Mirkin and G. C. Schatz, J. Am. Chem. Soc., 2003, 125, 1643–1654 CrossRef CAS PubMed
.
- T. I. N. G. Li, R. Sknepnek and M. Olvera de
la Cruz, J. Am. Chem. Soc., 2013, 135, 8535–8541 CrossRef CAS
.
- R. Klajn, J. F. Stoddart and B. A. Grzybowski, Chem. Soc. Rev., 2010, 39, 2203 RSC
.
- M. I. Bodnarchuk, M. V. Kovalenko, W. Heiss and D. V. Talapin, J. Am. Chem. Soc., 2010, 132, 11967–11977 CrossRef CAS
.
- J.-C. Cheng, L.-Y. Pan, X.-L. Huang, Y.-P. Huang, Y.-H. Wang, S.-P. Xu, F.-F. Li, Z.-W. Men and T. Cui, Nanomaterials, 2021, 11, 325 CrossRef CAS
.
- S.-Y. Zhang, M. D. Regulacio and M.-Y. Han, Chem. Soc. Rev., 2014, 43, 2301 RSC
.
- A. Sánchez-Iglesias, M. Grzelczak, T. Altantzis, B. Goris, J. Pérez-Juste, S. Bals, G. Van Tendeloo, S. H. Donaldson, B. F. Chmelka, J. N. Israelachvili and L. M. Liz-Marzán, ACS Nano, 2012, 6, 11059–11065 CrossRef
.
- Z. Nie and E. Kumacheva, Nat. Mater., 2008, 7, 277–290 CrossRef CAS
.
- J. Spatz, S. Mößmer, M. Möller, M. Kocher, D. Neher and G. Wegner, Adv. Mater., 1998, 10, 473–475 CrossRef CAS
.
- S. Mann and G. Ozin, Nature, 1996, 382, 313–318 CrossRef CAS
.
- J. He, R. Tangirala, T. Emrick, T. P. Russell, A. Böker, X. Li and J. Wang, Adv. Mater., 2007, 19, 381–385 CrossRef CAS
.
- K. Ariga, Y. Yamauchi, T. Mori and J. P. Hill, Adv. Mater., 2013, 25, 6477–6512 CrossRef CAS PubMed
.
- S. A. Hussain, B. Dey, D. Bhattacharjee and N. Mehta, Heliyon, 2018, 4, e01038 CrossRef PubMed
.
- M. Ito, Y. Yamashita, Y. Tsuneda, T. Mori, J. Takeya, S. Watanabe and K. Ariga, ACS Appl. Mater. Interfaces, 2020, 12, 56522–56529 CrossRef
.
- F. Gür, E. D. Kaya, B. Gür, A. Türkhan and Y. Onganer, Colloids Surf., A, 2019, 583, 124005 CrossRef
.
- D. D. Lasic and D. Papahadjopoulos, Science, 1995, 267, 1275–1276 CrossRef CAS
.
- J. N. Israelachvili, S. Marčelja and R. G. Horn, Q. Rev. Biophys., 1980, 13, 121–200 CrossRef CAS
.
- P. S. Zangabad, S. Mirkiani, S. Shahsavari, B. Masoudi, M. Masroor, H. Hamed, Z. Jafari, Y. D. Taghipour, H. Hashemi, M. Karimi and M. R. Hamblin, Nanotechnol. Rev., 2018, 7, 95–122 CrossRef CAS PubMed
.
- A. Laouini, C. Jaafar-Maalej, I. Limayem-Blouza, S. Sfar, C. Charcosset and H. Fessi, J. Colloid Sci. Biotechnol., 2012, 1, 147–168 CrossRef CAS
.
- W. Zhou, W. Liu, L. Zou, W. Liu, C. Liu, R. Liang and J. Chen, Colloids Surf., B, 2014, 117, 330–337 CrossRef CAS
.
- K. Son, M. Ueda, K. Taguchi, T. Maruyama, S. Takeoka and Y. Ito, J. Controlled Release, 2020, 322, 209–216 CrossRef CAS
.
- A. D. Bangham, Chem.
Phys. Lipids, 1993, 64, 275–285 CrossRef CAS PubMed
.
- H. Daraee, A. Etemadi, M. Kouhi, S. Alimirzalu and A. Akbarzadeh, Artif. Cells, Nanomed., Biotechnol., 2016, 44, 381–391 CrossRef CAS
.
- M. Celestin, S. Krishnan, S. Bhansali, E. Stefanakos and D. Y. Goswami, Nano Res., 2014, 7, 589–625 CrossRef CAS
.
- D. Guimarães, A. Cavaco-Paulo and E. Nogueira, Int. J. Pharm., 2021, 601, 120571 CrossRef
.
- M. V. Kovalenko, M. Scheele and D. V. Talapin, Science, 2009, 324, 1417–1420 CrossRef CAS
.
- C. R. Kagan, E. Lifshitz, E. H. Sargent and D. V. Talapin, Science, 2016, 353, aac5523 CrossRef
.
- Y. Shi, D. Wan, J. Huang, Y. Liu and J. Li, Chemosphere, 2020, 252, 126581 CrossRef CAS PubMed
.
- K. Manabe, Prog. Org. Coat., 2020, 142, 105599 CrossRef CAS
.
- T. Sen, B. Ozcelik and M. M. Ozmen, Int. J. Polym. Mater. Polym. Biomater., 2019, 68, 411–416 CrossRef CAS
.
- R. K. Kramer, F. E. G. Guimarães and A. J. F. Carvalho, J. Mol. Liq., 2019, 273, 368–373 CrossRef CAS
.
- T. A. Kolesnikova, D. Kohler, A. G. Skirtach and H. Möhwald, ACS Nano, 2012, 6, 9585–9595 CrossRef CAS
.
- M. F. Bédard, B. G. De Geest, H. Möhwald, G. B. Sukhorukov and A. G. Skirtach, Soft Matter, 2009, 5, 3297–3931 Search PubMed
.
- D. V. Talapin, J.-S. Lee, M. V. Kovalenko and E. V. Shevchenko, Chem. Rev., 2010, 110, 389–458 CrossRef CAS PubMed
.
- K. Ariga, Y. Yamauchi, G. Rydzek, Q. Ji, Y. Yonamine, K. C.-W. Wu and J. P. Hill, Chem. Lett., 2014, 43, 36–68 CrossRef CAS
.
- M. S. Saveleva, K. Eftekhari, A. Abalymov, T. E. L. Douglas, D. Volodkin, B. V. Parakhonskiy and A. G. Skirtach, Front. Chem., 2019, 7, 179 CrossRef CAS
.
- M. A. C. Stuart, W. T. S. Huck, J. Genzer, M. Müller, C. Ober, M. Stamm, G. B. Sukhorukov, I. Szleifer, V. V. Tsukruk, M. Urban, F. Winnik, S. Zauscher, I. Luzinov and S. Minko, Nat. Mater., 2010, 9, 101–113 CrossRef
.
- S. T. Milner, Science, 1991, 251, 905–914 CrossRef CAS
.
- O. Azzaroni, J. Polym. Sci., Part A: Polym. Chem., 2012, 50, 3225–3258 CrossRef CAS
.
- C. Feng and X. Huang, Acc. Chem. Res., 2018, 51, 2314–2323 CrossRef CAS
.
- P. Brandani and P. Stroeve, Macromolecules, 2003, 36, 9492–9501 CrossRef CAS
.
- M. Divandari, G. Morgese, S. N. Ramakrishna and E. M. Benetti, Eur. Polym. J., 2019, 110, 301–306 CrossRef CAS
.
-
S. Zapotoczny, Surface-Grafted Polymer Brushes, in Biomaterials Surface Science, ed. A. Taubert, J. F Mano and C. Rodriguez-Cabello, Wiley-VCH, Verlag GmbH & Co. KGaA, 2013, pp. 27–43 Search PubMed
.
- A. J. Wójcik, K. Wolski and S. Zapotoczny, Eur. Polym. J., 2021, 155, 110577 CrossRef
.
- B. Zhao and W. J. Brittain, Prog. Polym. Sci., 2000, 25, 677–710 CrossRef CAS
.
- B. M. D. O'Driscoll, G. H. Griffiths, M. W. Matsen, S. Perrier, V. Ladmiral and I. W. Hamley, Macromolecules, 2010, 43, 8177–8184 CrossRef
.
- E. B. Zhulina, C. Singh and A. C. Balazs, Macromolecules, 1996, 29, 6338–6348 CrossRef CAS
.
- Y. Yin, P. Sun, B. Li, T. Chen, Q. Jin, D. Ding and A.-C. Shi, Macromolecules, 2007, 40, 5161–5170 CrossRef CAS
.
- C. Feng, Y. Li, D. Yang, J. Hu, X. Zhang and X. Huang, Chem. Soc. Rev., 2011, 40, 1282–1295 RSC
.
- T. Zhou, H. Qi, L. Han, D. Barbash and C. Y. Li, Nat. Commun., 2016, 7, 11119 CrossRef CAS
.
- Y. Zhai, X. Chen, Z. Yuan, X. Han and H. Liu, Polym. Chem., 2020, 11, 4622–4629 RSC
.
- A. M. Jonas, K. Glinel, R. Oren, B. Nysten and W. T. S. Huck, Macromolecules, 2007, 40, 4403–4405 CrossRef CAS
.
- L. Ionov, V. Bocharova and S. Diez, Soft Matter, 2009, 5, 67–71 RSC
.
- A. Steinhaus, T. Pelras, R. Chakroun, A. H. Gröschel and M. Müllner, Macromol. Rapid Commun., 2018, 39, 1800177 CrossRef
.
- T. Pelras, C. S. Mahon and M. Müllner, Angew. Chem., Int. Ed., 2018, 57, 6982–6994 CrossRef CAS PubMed
.
- G. Decher, Science, 1997, 277, 1232–1237 CrossRef CAS
.
- W. Xu, A. A. Steinschulte, F. A. Plamper, V. F. Korolovych and V. V. Tsukruk, Chem. Mater., 2016, 28, 975–985 CrossRef CAS
.
- W. Xu, P. A. Ledin, Z. Iatridi, C. Tsitsilianis and V. V. Tsukruk, Angew. Chem., Int. Ed., 2016, 55, 4908–4913 CrossRef CAS
.
- J. J. Richardson, M. Björnmalm and F. Caruso, Science, 2015, 348, aaa2491 CrossRef
.
- O. Löhmann, M. Zerball and R. von Klitzing, Langmuir, 2018, 34, 11518–11525 CrossRef
.
- A. Baba, P. Taranekar, R. R. Ponnapati, W. Knoll and R. C. Advincula, ACS Appl. Mater. Interfaces, 2010, 2, 2347–2354 CrossRef CAS
.
- K. Ariga, M. Nishikawa, T. Mori, J. Takeya, L. K. Shrestha and J. P. Hill, Sci. Technol. Adv. Mater., 2019, 20, 51–95 CrossRef CAS PubMed
.
- M. Delcea, H. Möhwald and A. G. Skirtach, Adv. Drug Delivery Rev., 2011, 63, 730–747 CrossRef CAS PubMed
.
- C. Picart, J. Mutterer, L. Richert, Y. Luo, G. D. Prestwich, P. Schaaf, J.-C. Voegel and P. Lavalle, Proc. Natl. Acad. Sci. U. S. A., 2002, 99, 12531–12535 CrossRef CAS
.
- S. A. Sukhishvili, E. Kharlampieva and V. Izumrudov, Macromolecules, 2006, 39, 8873–8881 CrossRef CAS
.
- J. J. Richardson, J. Cui, M. Björnmalm, J. A. Braunger, H. Ejima and F. Caruso, Chem. Rev., 2016, 116, 14828–14867 CrossRef CAS
.
- E. V. Musin, A. L. Kim, A. V. Dubrovskii and S. A. Tikhonenko, Sci. Rep., 2021, 11, 14040 CrossRef CAS PubMed
.
- K. Ariga, E. Ahn, M. Park and B. Kim, Chem. – Asian J., 2019, 14, 2553–2566 CrossRef CAS
.
- W. B. Stockton and M. F. Rubner, Macromolecules, 1997, 30, 2717–2725 CrossRef CAS
.
- E. Kharlampieva, V. Kozlovskaya and S. A. Sukhishvili, Adv. Mater., 2009, 21, 3053–3065 CrossRef CAS
.
- J. F. Quinn and F. Caruso, Adv. Funct. Mater., 2006, 16, 1179–1186 CrossRef CAS
.
- Y. Lvov, K. Ariga, I. Ichinose and T. Kunitake, J. Chem. Soc., Chem. Commun., 1995, 2313 RSC
.
- Y. Shimazaki, M. Mitsuishi, S. Ito and M. Yamamoto, Langmuir, 1997, 13, 1385–1387 CrossRef CAS
.
- M. Li, S. Ishihara, M. Akada, M. Liao, L. Sang, J. P. Hill, V. Krishnan, Y. Ma and K. Ariga, J. Am. Chem. Soc., 2011, 133, 7348–7351 CrossRef CAS
.
- H. Xiong, M. Cheng, Z. Zhou, X. Zhang and J. Shen, Adv. Mater., 1998, 10, 529–532 CrossRef CAS
.
- T. Serizawa, K. Hamada and M. Akashi, Nature, 2004, 429, 52–55 CrossRef CAS PubMed
.
- I. Ichinose, H. Senzu and T. Kunitake, Chem. Mater., 1997, 9, 1296–1298 CrossRef CAS
.
- J. Borges and J. F. Mano, Chem. Rev., 2014, 114, 8883–8942 CrossRef CAS
.
- J. Chen, L. Huang, L. Ying, G. Luo, X. Zhao and W. Cao, Langmuir, 1999, 15, 7208–7212 CrossRef CAS
.
- A. A. Antipov, G. B. Sukhorukov, E. Donath and H. Möhwald, J. Phys. Chem. B, 2001, 105, 2281–2284 CrossRef CAS
.
- S. E. Burke and C. J. Barrett, Biomacromolecules, 2003, 4, 1773–1783 CrossRef CAS
.
- K. Yoshida, K. Suwa and J. Anzai, Materials, 2016, 9, 425 CrossRef
.
- J. M. Silva, S. G. Caridade, R. R. Costa, N. M. Alves, T. Groth, C. Picart, R. L. Reis and J. F. Mano, Langmuir, 2015, 31, 11318–11328 CrossRef CAS
.
- S. T. Dubas and J. B. Schlenoff, Langmuir, 2001, 17, 7725–7727 CrossRef CAS
.
- S. T. Dubas and J. B. Schlenoff, Macromolecules, 1999, 32, 8153–8160 CrossRef CAS
.
- D. Laurent and J. B. Schlenoff, Langmuir, 1997, 13, 1552–1557 CrossRef CAS
.
- R. Steitz, W. Jaeger and R. v. Klitzing, Langmuir, 2001, 17, 4471–4474 CrossRef CAS
.
- C.-A. Ghiorghita, F. Bucatariu and E. S. Dragan, Mater. Sci. Eng., C, 2019, 105, 110050 CrossRef CAS
.
- F.-X. Xiao, M. Pagliaro, Y.-J. Xu and B. Liu, Chem. Soc. Rev., 2016, 45, 3088–3121 RSC
.
- P. Schaaf, J.-C. Voegel, L. Jierry and F. Boulmedais, Adv. Mater., 2012, 24, 1001–1016 CrossRef CAS
.
- M. V. Zyuzin, A. S. Timin and G. B. Sukhorukov, Langmuir, 2019, 35, 4747–4762 CrossRef CAS PubMed
.
-
U. Kreibig and M. Vollmer, in Optical Properties of Metal Clusters, Springer Science & Business Media, 2013 Search PubMed
.
- S. Lu, M. Li, D. Liu, Y. Yang and P. Yang, J. Nanosci. Nanotechnol., 2018, 18, 288–295 CrossRef CAS PubMed
.
- D. Zhang, C. Jiang, P. Li and Y. Sun, ACS Appl. Mater. Interfaces, 2017, 9, 6462–6471 CrossRef
.
- G. H. S. Rodrigues, C. M. Miyazaki, R. J. G. Rubira, C. J. L. Constantino and M. Ferreira, ACS Appl. Nano Mater., 2019, 2, 1082–1091 CrossRef CAS
.
- T. Panapimonlawat, S. Phanichphant and S. Sriwichai, Polymer, 2021, 13, 1488 CAS
.
- B.-S. Kim, S. W. Park and P. T. Hammond, ACS Nano, 2008, 2, 386–392 CrossRef CAS PubMed
.
- J. Zhang, D. Wang, X. Jiang, L. He, L. Fu, Y. Zhao, Y. Wang, H. Mo and J. Shen, Chem. Eng. J., 2019, 370, 1057–1067 CrossRef CAS
.
- T. Mohan, R. Kargl, K. E. Tradt, M. R. Kulterer, M. Braćić, S. Hribernik, K. Stana-Kleinschek and V. Ribitsch, Carbohydr. Polym., 2015, 116, 149–158 CrossRef CAS
.
- P. F. Ferrari, E. Zattera, L. Pastorino, P. Perego and D. Palombo, Int. J. Biol. Macromol., 2021, 177, 548–558 CrossRef CAS
.
- M. Kumorek, I. M. Minisy, T. Krunclová, M. Voršiláková, K. Venclíková, E. M. Chánová, O. Janoušková and D. Kubies, Mater. Sci. Eng., C, 2020, 109, 110493 CrossRef CAS
.
- T. G. Shutava, K. S. Livanovich and A. A. Sharamet, Colloids Surf., B, 2019, 173, 412–420 CrossRef CAS
.
- S. Nawae, J. Meesane, N. Muensit and C. Daengngam, Mater. Des., 2018, 160, 1158–1167 CrossRef CAS
.
- X. Arys, A. Laschewsky and A. M. Jonas, Macromolecules, 2001, 34, 3318–3330 CrossRef CAS
.
- K. Glinel, A. Laschewsky and A. M. Jonas, Macromolecules, 2001, 34, 5267–5274 CrossRef CAS
.
- M. Szuwarzyński, K. Wolski, T. Kruk and S. Zapotoczny, Prog. Polym. Sci., 2021, 121, 101433 CrossRef
.
- Y. Deng, L. Yang, X. Huang, J. Chen, X. Shi, W. Yang, M. Hong, Y. Wang, M. S. Dargusch and Z. Chen, Macromol. Biosci., 2018, 18, 1800028 CrossRef
.
- Q. Chen, C. J. Brett, A. Chumakov, M. Gensch, M. Schwartzkopf, V. Körstgens, L. D. Söderberg, A. Plech, P. Zhang, P. Müller-Buschbaum and S. V. Roth, ACS Appl. Nano Mater., 2021, 4, 503–513 CrossRef CAS
.
- M. Li, X. Liu, Z. Xu, K. W. K. Yeung and S. Wu, ACS Appl. Mater. Interfaces, 2016, 8, 33972–33981 CrossRef CAS PubMed
.
- E. Donath, G. B. Sukhorukov, F. Caruso, S. A. Davis and H. Möhwald, Angew. Chem., Int. Ed., 1998, 37, 2201–2205 CrossRef
.
- F. Caruso, R. A. Caruso and H. Möhwald, Science, 1998, 282, 1111–1114 CrossRef CAS PubMed
.
- W. Tong, X. Song and C. Gao, Chem. Soc. Rev., 2012, 41, 6103 RSC
.
- A. J. Khopade and F. Caruso, Chem. Mater., 2004, 16, 2107–2112 CrossRef CAS
.
- Q.-L. Li, Y. Sun, Y.-L. Sun, J. Wen, Y. Zhou, Q.-M. Bing, L. D. Isaacs, Y. Jin, H. Gao and Y.-W. Yang, Chem. Mater., 2014, 26, 6418–6431 CrossRef CAS
.
- Z. Wang, X. Zhang, J. Gu, H. Yang, J. Nie and G. Ma, Carbohydr. Polym., 2014, 103, 38–45 CrossRef CAS PubMed
.
- M. Porta-i-Batalla, C. Eckstein, E. Xifré-Pérez, P. Formentín, J. Ferré-Borrull and L. F. Marsal, Nanoscale Res. Lett., 2016, 11, 372 CrossRef
.
- P. Wang, R. K. Kankala, J. Fan, R. Long, Y. Liu and S. Wang, J. Mater. Sci. Mater. Med., 2018, 29, 68 CrossRef
.
- S. Aslan, M. Deneufchatel, S. Hashmi, N. Li, L. D. Pfefferle, M. Elimelech, E. Pauthe and P. R. Van Tassel, J. Colloid Interface Sci., 2012, 388, 268–273 CrossRef CAS PubMed
.
- A. M. Yashchenok, D. N. Bratashov, D. A. Gorin, M. V. Lomova, A. M. Pavlov, A. V. Sapelkin, B. S. Shim, G. B. Khomutov, N. A. Kotov, G. B. Sukhorukov, H. Möhwald and A. G. Skirtach, Adv. Funct. Mater., 2010, 20, 3136–3142 CrossRef CAS
.
- L. Li, K. K. Sun, L. Fan, W. Hong, Z. S. Xu and L. Liu, Mater. Lett., 2014, 126, 197–201 CrossRef CAS
.
- Y. Chen, Z. Xiong, L. Peng, Y. Gan, Y. Zhao, J. Shen, J. Qian, L. Zhang and W. Zhang, ACS Appl. Mater. Interfaces, 2015, 7, 16338–16347 CrossRef CAS
.
- R. Ladj, A. Bitar, M. M. Eissa, H. Fessi, Y. Mugnier, R. Le Dantec and A. Elaissari, Int. J. Pharm., 2013, 458, 230–241 CrossRef CAS
.
- K. Radhakrishnan, J. Tripathy, D. P. Gnanadhas, D. Chakravortty and A. M. Raichur, RSC Adv., 2014, 4, 45961–45968 RSC
.
- J. Campbell, J. Abnett, G. Kastania, D. Volodkin and A. S. Vikulina, ACS Appl. Mater. Interfaces, 2021, 13, 3259–3269 CrossRef CAS
.
- I. Marchenko, A. Yashchenok, T. Borodina, T. Bukreeva, M. Konrad, H. Möhwald and A. Skirtach, J. Controlled Release, 2012, 162, 599–605 CrossRef CAS PubMed
.
- F. Cuomo, F. Lopez, M. Piludu, M. G. Miguel, B. Lindman and A. Ceglie, J. Colloid Interface Sci., 2015, 447, 211–216 CrossRef CAS
.
- Y. Tarakanchikova, A. Muslimov, I. Sergeev, K. Lepik, N. Yolshin, A. Goncharenko, K. Vasilyev, I. Eliseev, A. Bukatin, V. Sergeev, S. Pavlov, A. Popov, I. Meglinski, B. Afanasiev, B. Parakhonskiy, G. Sukhorukov and D. Gorin, J. Mater. Chem. B, 2020, 8, 9576–9588 RSC
.
- Y. Yang, H. Zhu, J. Wang, Q. Fang and Z. Peng, ACS Appl. Mater. Interfaces, 2018, 10, 33493–33506 CrossRef CAS
.
- J. Zhou, G. Romero, E. Rojas, L. Ma, S. Moya and C. Gao, J. Colloid Interface Sci., 2010, 345, 241–247 CrossRef CAS PubMed
.
- A. Yashchenok, B. Parakhonskiy, S. Donatan, D. Kohler, A. Skirtach and H. Möhwald, J. Mater. Chem. B, 2013, 1, 1223 RSC
.
- N. Balabushevich, E. Sholina, E. Mikhalchik, L. Filatova, A. Vikulina and D. Volodkin, Micromachines, 2018, 9, 307 CrossRef
.
- T. Paulraj, N. Feoktistova, N. Velk, K. Uhlig, C. Duschl and D. Volodkin, Macromol. Rapid Commun., 2014, 35, 1408–1413 CrossRef CAS PubMed
.
- S. Belbekhouche, N. Bousserrhine, V. Alphonse and B. Carbonnier, Food Hydrocolloids, 2019, 95, 219–227 CrossRef CAS
.
- X. Wang, Z. Jiang, J. Shi, Y. Liang, C. Zhang and H. Wu, ACS Appl. Mater. Interfaces, 2012, 4, 3476–3483 CrossRef CAS
.
- B. G. De Geest, A. M. Jonas, J. Demeester and S. C. De Smedt, Langmuir, 2006, 22, 5070–5074 CrossRef CAS
.
- D. Shi, M. Ran, H. Huang, L. Zhang, X. Li, M. Chen and M. Akashi, Polym. Chem., 2016, 7, 6779–6788 RSC
.
- W.-H. Chen, G.-F. Luo, W.-X. Qiu, Q. Lei, L.-H. Liu, S.-B. Wang and X.-Z. Zhang, Biomaterials, 2017, 117, 54–65 CrossRef CAS
.
- J. Gauczinski, Z. Liu, X. Zhang and M. Schönhoff, Langmuir, 2012, 28, 4267–4273 CrossRef CAS PubMed
.
- X. Xu, S. Lü, C. Gao, X. Bai, C. Feng, N. Gao and M. Liu, Mater. Des., 2015, 88, 1127–1133 CrossRef CAS
.
- Y. Wu, Y. Long, Q.-L. Li, S. Han, J. Ma, Y.-W. Yang and H. Gao, ACS Appl. Mater. Interfaces, 2015, 7, 17255–17263 CrossRef CAS
.
- J. Shi, L. Zhang and Z. Jiang, ACS Appl. Mater. Interfaces, 2011, 3, 881–889 CrossRef CAS PubMed
.
- B. Amritha Rammohan, L. Tayal, A. Kumar, S. Sivakumar and A. Sharma, RSC Adv., 2013, 3, 2008–2016 RSC
.
- Y. Zhu, W. Tong, C. Gao and H. Möhwald, Langmuir, 2008, 24, 7810–7816 CrossRef CAS PubMed
.
- D. G. Montjoy, J. H. Bahng, A. Eskafi, H. Hou and N. A. Kotov, J. Am. Chem. Soc., 2018, 140, 7835–7845 CrossRef CAS
.
- S. T. Sivapalan, B. M. DeVetter, T. K. Yang, M. V. Schulmerich, R. Bhargava and C. J. Murphy, J. Phys. Chem. C, 2013, 117, 10677–10682 CrossRef CAS
.
- L. Tang, T. Vo, X. Fan, D. Vecchio, T. Ma, J. Lu, H. Hou, S. C. Glotzer and N. A. Kotov, J. Am. Chem. Soc., 2021, 143, 19655–19667 CrossRef CAS PubMed
.
- E. V. Lengert, S. I. Koltsov, J. Li, A. V. Ermakov, B. V. Parakhonskiy, E. V. Skorb and A. G. Skirtach, Coatings, 2020, 10, 1131 CrossRef CAS
.
- M. Delcea, S. Schmidt, R. Palankar, P. A. L. Fernandes, A. Fery, H. Möhwald and A. G. Skirtach, Small, 2010, 6, 2858–2862 CrossRef CAS
.
- M. F. Bédard, A. Munoz-Javier, R. Mueller, P. del Pino, A. Fery, W. J. Parak, A. G. Skirtach and G. B. Sukhorukov, Soft Matter, 2009, 5, 148–155 RSC
.
- W. J. Anderson, K. Nowinska, T. Hutter, S. Mahajan and M. Fischlechner, Nanoscale, 2018, 10, 7138–7146 RSC
.
- E. Lengert, M. Saveleva, A. Abalymov, V. Atkin, P. C. Wuytens, R. Kamyshinsky, A. L. Vasiliev, D. A. Gorin, G. B. Sukhorukov, A. G. Skirtach and B. Parakhonskiy, ACS Appl. Mater. Interfaces, 2017, 9, 21949–21958 CrossRef CAS
.
- I. Y. Stetciura, A. V. Markin, A. N. Ponomarev, A. V. Yakimansky, T. S. Demina, C. Grandfils, D. V. Volodkin and D. A. Gorin, Langmuir, 2013, 29, 4140–4147 CrossRef CAS PubMed
.
- D. Chen, Y. Zhang, T. Bessho, J. Sang, H. Hirahara, K. Mori and Z. Kang, Chem. Eng. J., 2016, 303, 100–108 CrossRef CAS
.
- Y. Ju, C. Cortez-Jugo, J. Chen, T. Wang, A. J. Mitchell, E. Tsantikos, N. Bertleff-Zieschang, Y. Lin, J. Song, Y. Cheng, S. Mettu, M. A. Rahim, S. Pan, G. Yun, M. L. Hibbs, L. Y. Yeo, C. E. Hagemeyer and F. Caruso, Adv. Sci., 2020, 7, 1902650 CrossRef CAS
.
- A. Mateos-Maroto, L. Fernández-Peña, I. Abelenda-Núñez, F. Ortega, R. G. Rubio and E. Guzmán, Polymer, 2022, 14, 479 CAS
.
- D. Volodkin, A. Skirtach and H. Möhwald, Polym. Int., 2012, 61, 673–679 CrossRef CAS
.
-
D. Volodkin, A. Skirtach and H. Möhwald, Bioactive Surfaces, Springer Berlin Heidelberg, 2010, vol. 240, pp. 135–161 Search PubMed
.
- P. K. Vemula, N. Wiradharma, J. A. Ankrum, O. R. Miranda, G. John and J. M. Karp, Curr. Opin. Biotechnol., 2013, 24, 1174–1182 CrossRef CAS
.
- X. Hu, Y. Wang, L. Zhang and M. Xu, Food Chem., 2020, 306, 125632 CrossRef CAS PubMed
.
-
Z. Shariatinia and A. Barzegari, Polysaccharide Carriers for Drug Delivery, Elsevier, 2019, pp. 639–684 Search PubMed
.
- E. J. Bealer, S. Onissema-Karimu, A. Rivera-Galletti, M. Francis, J. Wilkowski, D. Salas-de la Cruz and X. Hu, Polymer, 2020, 12, 464 CAS
.
- G. Archana, K. Sabina, S. Babuskin, K. Radhakrishnan, M. A. Fayidh, P. A. S. Babu, M. Sivarajan and M. Sukumar, Carbohydr. Polym., 2013, 98, 89–94 CrossRef CAS PubMed
.
- J. J. Panda and V. S. Chauhan, Polym. Chem., 2014, 5, 4431–4449 RSC
.
- H. Cui, N. Pan, W. Fan, C. Liu, Y. Li, Y. Xia and K. Sui, Adv. Funct. Mater., 2019, 29, 1807692 CrossRef
.
- B. Singh, S. Sharma and A. Dhiman, Carbohydr. Polym., 2017, 165, 294–303 CrossRef CAS
.
- I. Tokarev and S. Minko, Soft Matter, 2009, 5, 511–524 RSC
.
- D. Kuckling, Colloid Polym. Sci., 2009, 287, 881–891 CrossRef CAS
.
- B. Chollet, M. Li, E. Martwong, B. Bresson, C. Fretigny, P. Tabeling and Y. Tran, ACS Appl. Mater. Interfaces, 2016, 8, 11729–11738 CrossRef CAS
.
- G. P. Sakala and M. Reches, Adv. Mater. Interfaces, 2018, 5, 1800073 CrossRef
.
- S. Yuran, A. Dolid and M. Reches, ACS Biomater. Sci. Eng., 2018, 4, 4051–4061 CrossRef CAS PubMed
.
- M. Lian, X. Chen, Y. Lu and W. Yang, ACS Appl. Mater. Interfaces, 2016, 8, 25036–25042 CrossRef CAS
.
- M. Righi, G. L. Puleo, I. Tonazzini, G. Giudetti, M. Cecchini and S. Micera, Sci. Rep., 2018, 8, 502 CrossRef
.
- P. Heidarian, A. Z. Kouzani, A. Kaynak, M. Paulino, B. Nasri-Nasrabadi, A. Zolfagharian and R. Varley, Carbohydr. Polym., 2020, 231, 115743 CrossRef CAS
.
- S. Trombino, C. Servidio, F. Curcio and R. Cassano, Pharmaceutics, 2019, 11, 407 CrossRef CAS
.
- X. Hu, L. Yan, Y. Wang and M. Xu, Chem. Eng. J., 2020, 388, 124189 CrossRef CAS
.
- X. Hu, Y. Wang, L. Zhang and M. Xu, Carbohydr. Polym., 2020, 234, 115920 CrossRef CAS
.
- Y. Xiong, K. Yan, W. E. Bentley, H. Deng, Y. Du, G. F. Payne and X.-W. Shi, ACS Appl. Mater. Interfaces, 2014, 6, 2948–2957 CrossRef CAS
.
- M. Marquis, J. Davy, B. Cathala, A. Fang and D. Renard, Carbohydr. Polym., 2015, 116, 189–199 CrossRef CAS
.
- A. Abalymov, E. Lengert, L. Van der Meeren, M. Saveleva, A. Ivanova, T. E. L. Douglas, A. G. Skirtach, D. Volodkin and B. Parakhonskiy, Biomater. Adv., 2022, 133, 112632 CrossRef PubMed
.
- A. Abalymov, L. Van Poelvoorde, V. Atkin, A. G. Skirtach, M. Konrad and B. Parakhonskiy, ACS Appl. Bio Mater., 2020, 3, 2986–2996 CrossRef CAS
.
- T. Wu, J. Huang, Y. Jiang, Y. Hu, X. Ye, D. Liu and J. Chen, Food Chem., 2018, 240, 361–369 CrossRef CAS PubMed
.
- S. D. Hujaya, G. S. Lorite, S. J. Vainio and H. Liimatainen, Acta Biomater., 2018, 75, 346–357 CrossRef CAS
.
- V. Kozlovskaya and E. Kharlampieva, Macromol. Biosci., 2022, 22, 2100328 CrossRef CAS
.
- R. Xing, S. Li, N. Zhang, G. Shen, H. Möhwald and X. Yan, Biomacromolecules, 2017, 18, 3514–3523 CrossRef CAS PubMed
.
- M. Goktas, G. Cinar, I. Orujalipoor, S. Ide, A. B. Tekinay and M. O. Guler, Biomacromolecules, 2015, 16, 1247–1258 CrossRef CAS PubMed
.
- N. C. Wickremasinghe, V. A. Kumar and J. D. Hartgerink, Biomacromolecules, 2014, 15, 3587–3595 CrossRef CAS
.
- H. Huang, A. I. Herrera, Z. Luo, O. Prakash and X. S. Sun, Biophys. J., 2012, 103, 979–988 CrossRef CAS
.
- D. J. Adams, M. F. Butler, W. J. Frith, M. Kirkland, L. Mullen and P. Sanderson, Soft Matter, 2009, 5, 1856 RSC
.
- Y. Bai, Q. Luo and J. Liu, Chem. Soc. Rev., 2016, 45, 2756–2767 RSC
.
- H. Nakamura, Q. Rev. Biophys., 1996, 29, 1–90 CrossRef CAS PubMed
.
- K. Stapelfeldt, S. Stamboroski, P. Mednikova and D. Brüggemann, Biofabrication, 2019, 11, 025010 CrossRef CAS PubMed
.
- N. Suter, A. Joshi, T. Wunsch, N. Graupner, K. Stapelfeldt, M. Radmacher, J. Müssig and D. Brüggemann, Mater. Sci. Eng., C, 2021, 126, 112156 CrossRef CAS PubMed
.
- D. Hense, A. Büngeler, F. Kollmann, M. Hanke, A. Orive, A. Keller, G. Grundmeier, K. Huber and O. I. Strube, Biomacromolecules, 2021, 22, 4084–4094 CrossRef CAS
.
- K. Stapelfeldt, S. Stamboroski, I. Walter, N. Suter, T. Kowalik, M. Michaelis and D. Brüggemann, Nano Lett., 2019, 19, 6554–6563 CrossRef CAS PubMed
.
- M. Kenny, S. Stamboroski, R. Taher, D. Brüggemann and I. Schoen, Adv. Healthcare Mater., 2022, 11, 2200249 CrossRef CAS PubMed
.
- S. Das, K. Zhou, D. Ghosh, N. N. Jha, P. K. Singh, R. S. Jacob, C. C. Bernard, D. I. Finkelstein, J. S. Forsythe and S. K. Maji, NPG Asia Mater., 2016, 8, e304–e304 CrossRef CAS
.
- R. S. Jacob, D. Ghosh, P. K. Singh, S. K. Basu, N. N. Jha, S. Das, P. K. Sukul, S. Patil, S. Sathaye, A. Kumar, A. Chowdhury, S. Malik, S. Sen and S. K. Maji, Biomaterials, 2015, 54, 97–105 CrossRef CAS PubMed
.
- S.-C. How, T.-H. Lin, C.-C. Chang and S. S.-S. Wang, Int. J. Biol. Macromol., 2021, 184, 79–91 CrossRef CAS PubMed
.
- B. Hu, Y. Shen, J. Adamcik, P. Fischer, M. Schneider, M. J. Loessner and R. Mezzenga, ACS Nano, 2018, 12, 3385–3396 CrossRef CAS PubMed
.
- B. Hu, M. Li, X. He, H. Wang, J. Huang, Z. Liu and R. Mezzenga, Biomater. Sci., 2022, 10, 3597–3611 RSC
.
- V. Morya, S. Walia, B. B. Mandal, C. Ghoroi and D. Bhatia, ACS Biomater. Sci. Eng., 2020, 6, 6021–6035 CrossRef CAS
.
- M. D. Frank-Kamenetskii, V. V. Anshelevich and A. V. Lukashin, Sov. Phys. Usp., 1987, 30, 317 CrossRef
.
- Y. Xing, E. Cheng, Y. Yang, P. Chen, T. Zhang, Y. Sun, Z. Yang and D. Liu, Adv. Mater., 2011, 23, 1117–1121 CrossRef CAS PubMed
.
- Y. Ma, H. Liu, Q. Mou, D. Yan, X. Zhu and C. Zhang, Nanoscale, 2018, 10, 8367–8371 RSC
.
- S. Hu, X. Du, Y. Bi, P.-P. He, Y. Mu, C. Liu, Q. Gao, M. Yin and W. Guo, ACS Appl. Polym. Mater., 2022, 4, 5199–5208 CrossRef CAS
.
- J. Gačanin, C. V. Synatschke and T. Weil, Adv. Funct. Mater., 2020, 30, 1906253 CrossRef
.
- S. Xia, S. Song, X. Ren and G. Gao, Soft Matter, 2017, 13, 6059–6067 RSC
.
- Y. Huang, L. Cao, B. V. Parakhonskiy and A. G. Skirtach, Pharmaceutics, 2022, 14, 909 CrossRef CAS PubMed
.
- X. Hu, G. Nian, X. Liang, L. Wu, T. Yin, H. Lu, S. Qu and W. Yang, ACS Appl. Mater. Interfaces, 2019, 11, 10292–10300 CrossRef CAS
.
- C. Pan, L. Liu, Q. Chen, Q. Zhang and G. Guo, ACS Appl. Mater. Interfaces, 2017, 9, 38052–38061 CrossRef CAS
.
- N. Sarkar, G. Sahoo and S. K. Swain, Nano-Struct. Nano-Objects, 2020, 23, 100507 CrossRef CAS
.
- I. M. Garnica-Palafox, H. O. Estrella-Monroy, N. A. Vázquez-Torres, M. Álvarez-Camacho, A. E. Castell-Rodríguez and F. M. Sánchez-Arévalo, Carbohydr. Polym., 2020, 236, 115971 CrossRef CAS PubMed
.
- A. Abalymov, L. Van der Meeren, M. Saveleva, E. Prikhozhdenko, K. Dewettinck, B. Parakhonskiy and A. G. Skirtach, ACS Biomater. Sci. Eng., 2020, 6, 3933–3944 CrossRef CAS PubMed
.
- A. Abalymov, L. Van der Meeren, D. Volodkin, B. Parakhonskiy and A. G. Skirtach, C, 2021, 7, 18 CAS
.
- Y. Zhuang, F. Yu, H. Chen, J. Zheng, J. Ma and J. Chen, J. Mater. Chem. A, 2016, 4, 10885–10892 RSC
.
- J. Zheng, P. Xiao, W. Liu, J. Zhang, Y. Huang and T. Chen, Macromol. Rapid Commun., 2016, 37, 265–270 CrossRef CAS PubMed
.
- I. Lisiecki and M.-P. Pileni, J. Phys. Chem. C, 2012, 116, 3–14 CrossRef CAS
.
- Q. Shi, W. Di, D. Dong, L. W. Yap, L. Li, D. Zang and W. Cheng, ACS Nano, 2019, 13, 5243–5250 CrossRef CAS PubMed
.
- K. Mukai, M. Hara, S. Nagano and T. Seki, Angew. Chem., Int. Ed., 2016, 55, 14028–14032 CrossRef CAS PubMed
.
- F.-X. Xiao, J. Miao and B. Liu, J. Am. Chem. Soc., 2014, 136, 1559–1569 CrossRef CAS PubMed
.
- Y. H. Deng, J. H. Chen, Q. Yang and Y. Z. Zhuo, Chem. Eng. Res. Des., 2021, 170, 423–433 CrossRef CAS
.
- A. G. Skirtach, D. V. Volodkin and H. Möhwald, ChemPhysChem, 2010, 11, 822–829 CrossRef CAS PubMed
.
- E. Kilic, M. V. Novoselova, S. H. Lim, N. A. Pyataev, S. I. Pinyaev, O. A. Kulikov, O. A. Sindeeva, O. A. Mayorova, R. Murney, M. N. Antipina, B. Haigh, G. B. Sukhorukov and M. V. Kiryukhin, Sci. Rep., 2017, 7, 44159 CrossRef
.
- J. Li, D. Khalenkow, D. Volodkin, A. Lapanje, A. G. Skirtach and B. V. Parakhonskiy, Colloids Surf., A, 2022, 650, 129547 CrossRef CAS
.
- J. Li, L. Van der Meeren, J. Verduijn, B. V. Parakhonskiy and A. G. Skirtach, Mater. Today Commun., 2022, 33, 104287 CrossRef CAS
.
- M. S. Savelyeva, A. A. Abalymov, G. P. Lyubun, I. V. Vidyasheva, A. M. Yashchenok, T. E. L. Douglas, D. A. Gorin and B. V. Parakhonskiy, J. Biomed. Mater. Res., Part A, 2017, 105, 94–103 CrossRef CAS PubMed
.
- H. Jiang and T. Kobayashi, Mater. Sci. Eng., C, 2017, 75, 478–486 CrossRef CAS PubMed
.
- Y. Shao, H. Jia, T. Cao and D. Liu, Acc. Chem. Res., 2017, 50, 659–668 CrossRef CAS PubMed
.
- M. P. Pileni, J. Phys. Chem. B, 2001, 105, 3358–3371 CrossRef CAS
.
- C.-W. Wang, A. Oskooei, D. Sinton and M. G. Moffitt, Langmuir, 2010, 26, 716–723 CrossRef CAS PubMed
.
- A. Saini, K. Theis-Bröhl, A. Koutsioubas, K. L. Krycka, J. A. Borchers and M. Wolff, Langmuir, 2021, 37, 4064–4071 CrossRef CAS PubMed
.
- A. Khademhosseini and R. Langer, Nat. Protoc., 2016, 11, 1775–1781 CrossRef CAS PubMed
.
- M. M. Stevens and J. H. George, Science, 2005, 310, 1135–1138 CrossRef CAS PubMed
.
- N. W. Choi, M. Cabodi, B. Held, J. P. Gleghorn, L. J. Bonassar and A. D. Stroock, Nat. Mater., 2007, 6, 908–915 CrossRef CAS PubMed
.
- J. J. Green and J. H. Elisseeff, Nature, 2016, 540, 386–394 CrossRef CAS PubMed
.
- T. Dvir, B. P. Timko, D. S. Kohane and R. Langer, Nat. Nanotechnol., 2011, 6, 13–22 CrossRef CAS PubMed
.
- D. Choi, J. Park, J. Heo, T. I. Oh, E. Lee and J. Hong, ACS Appl. Mater. Interfaces, 2017, 9, 12264–12271 CrossRef CAS PubMed
.
- T. G. Kim, S.-H. Park, H. J. Chung, D.-Y. Yang and T. G. Park, J. Mater. Chem., 2010, 20, 8927 RSC
.
- J. Lee, V. Manoharan, L. Cheung, S. Lee, B.-H. Cha, P. Newman, R. Farzad, S. Mehrotra, K. Zhang, F. Khan, M. Ghaderi, Y.-D. Lin, S. Aftab, P. Mostafalu, M. Miscuglio, J. Li, B. B. Mandal, M. A. Hussain, K. Wan, X. S. Tang, A. Khademhosseini and S. R. Shin, ACS Nano, 2019, 13, 12525–12539 CrossRef CAS PubMed
.
- A. Nishiguchi, H. Yoshida, M. Matsusaki and M. Akashi, Adv. Mater., 2011, 23, 3506–3510 CrossRef CAS PubMed
.
- R. Ravichandran, J. R. Venugopal, S. Sundarrajan, S. Mukherjee and S. Ramakrishna, Biomaterials, 2012, 33, 846–855 CrossRef CAS PubMed
.
- L. He, S. Tang, M. P. Prabhakaran, S. Liao, L. Tian, Y. Zhang, W. Xue and S. Ramakrishna, Macromol. Biosci., 2013, 13, 1601–1609 CrossRef CAS PubMed
.
- Y. Luo, S. Wang, M. Shen, R. Qi, Y. Fang, R. Guo, H. Cai, X. Cao, H. Tomás, M. Zhu and X. Shi, Carbohydr. Polym., 2013, 91, 419–427 CrossRef CAS PubMed
.
- K. Zhang, W. H. Chooi, S. Liu, J. S. Chin, A. Murray, D. Nizetic, D. Cheng and S. Y. Chew, Biomaterials, 2020, 256, 120225 CrossRef CAS PubMed
.
- X. Zhang, Z. Li, X. Yuan, Z. Cui and X. Yang, Appl. Surf. Sci., 2013, 284, 732–737 CrossRef CAS
.
- L.-Y. Cui, S.-C. Cheng, L.-X. Liang, J.-C. Zhang, S.-Q. Li, Z.-L. Wang and R.-C. Zeng, Bioact. Mater., 2020, 5, 153–163 CrossRef PubMed
.
- H.-D. Wu, J.-C. Yang, T. Tsai, D.-Y. Ji, W.-J. Chang, C.-C. Chen and S.-Y. Lee, Carbohydr. Polym., 2011, 85, 318–324 CrossRef CAS
.
- B. Choi, S. Kim, B. Lin, B. M. Wu and M. Lee, ACS Appl. Mater. Interfaces, 2014, 6, 20110–20121 CrossRef CAS PubMed
.
- X. Qi, T. Su, M. Zhang, X. Tong, W. Pan, Q. Zeng, Z. Zhou, L. Shen, X. He and J. Shen, ACS Appl. Mater. Interfaces, 2020, 12, 13256–13264 CrossRef CAS PubMed
.
- S. Afewerki, A. Sheikhi, S. Kannan, S. Ahadian and A. Khademhosseini, Bioeng. Transl. Med., 2019, 4, 96–115 CrossRef CAS PubMed
.
- D. M. Ryan and B. L. Nilsson, Polym. Chem., 2012, 3, 18–33 RSC
.
- L.-L. Li, G.-B. Qi, F. Yu, S.-J. Liu and H. Wang, Adv. Mater., 2015, 27, 3181–3188 CrossRef CAS PubMed
.
- F. Yergoz, N. Hastar, C. E. Cimenci, A. D. Ozkan, T. Tekinay, M. O. Guler and A. B. Tekinay, Biomaterials, 2017, 134, 117–127 CrossRef CAS PubMed
.
- A. Andukuri, W. P. Minor, M. Kushwaha, J. M. Anderson and H.-W. Jun, Nanomedicine, 2010, 6, 289–297 CrossRef CAS PubMed
.
- J.-K. Kim, J. Anderson, H.-W. Jun, M. A. Repka and S. Jo, Mol. Pharm., 2009, 6, 978–985 CrossRef CAS PubMed
.
- J. D. Hartgerink, E. Beniash and S. I. Stupp, Proc. Natl. Acad. Sci. U. S. A., 2002, 99, 5133–5138 CrossRef CAS PubMed
.
- M. Rivas, L. del Valle, C. Alemán and J. Puiggalí, Gels, 2019, 5, 14 CrossRef CAS PubMed
.
- N. Amosi, S. Zarzhitsky, E. Gilsohn, O. Salnikov, E. Monsonego-Ornan, R. Shahar and H. Rapaport, Acta Biomater., 2012, 8, 2466–2475 CrossRef CAS PubMed
.
- A. M. Diez-Pascual and A. Rahdar, ChemMedChem, 2022, 17, e202200142 CrossRef CAS PubMed
.
- K. Ariga, Y. M. Lvov, K. Kawakami, Q. Ji and J. P. Hill, Adv. Drug Delivery Rev., 2011, 63, 762–771 CrossRef CAS PubMed
.
- B. Pilicheva, Y. Uzunova, I. Bodurov, A. Viraneva, G. Exner, S. Sotirov, T. Yovcheva and M. Marudova, J. Drug Delivery Sci. Technol., 2020, 59, 101897 CrossRef CAS
.
- V. Albright, I. Zhuk, Y. Wang, V. Selin, B. van de Belt-Gritter, H. J. Busscher, H. C. van der Mei and S. A. Sukhishvili, Acta Biomater., 2017, 61, 66–74 CrossRef CAS PubMed
.
- E. Redolfi Riva, A. Desii, S. Sartini, C. La Motta, B. Mazzolai and V. Mattoli, Langmuir, 2013, 29, 13190–13197 CrossRef CAS PubMed
.
- V. Mohanta, G. Madras and S. Patil, ACS Appl. Mater. Interfaces, 2014, 6, 20093–20101 CrossRef CAS PubMed
, https://pubs.acs.org/doi/10.1021/am505681e.
- S. Türk and E. Yılmaz, J. Drug Delivery Sci. Technol., 2022, 67, 102943 CrossRef
.
- C. Du, J. Shi, J. Shi, L. Zhang and S. Cao, Mater. Sci. Eng., C, 2013, 33, 3745–3752 CrossRef CAS PubMed
.
- J. Wang, H. Hao and J. H. Cai, J. Macromol. Sci., Part B: Phys., 2019, 58, 535–550 CrossRef CAS
.
- J. Xing, Y. Cai, Y. Wang, H. Zheng and Y. Liu, Adv. Polym. Technol., 2020, 2020, 1–8 CrossRef
.
- N. M. Elbaz, A. Owen, S. Rannard and T. O. McDonald, Int. J. Pharm., 2020, 574, 118866 CrossRef CAS PubMed
.
- S. Correa, N. Boehnke, E. Deiss-Yehiely and P. T. Hammond, ACS Nano, 2019, 13, 5623–5634 CrossRef CAS PubMed
.
- Z. Li, D. Yuan, G. Jin, B. H. Tan and C. He, ACS Appl. Mater. Interfaces, 2016, 8, 1842–1853 CrossRef CAS PubMed
.
- Y. Wang, V. Bansal, A. N. Zelikin and F. Caruso, Nano Lett., 2008, 8, 1741–1745 CrossRef CAS PubMed
.
- N. Theodorakis, S.-F. Saravanou, N.-P. Kouli, Z. Iatridi and C. Tsitsilianis, Polymer, 2021, 13, 1228 CAS
.
- L. J. De Cock, S. De Koker, B. G. De Geest, J. Grooten, C. Vervaet, J. P. Remon, G. B. Sukhorukov and M. N. Antipina, Angew. Chem., Int. Ed., 2010, 49, 6954–6973 CrossRef CAS PubMed
.
- J. Shao, M. Xuan, T. Si, L. Dai and Q. He, Nanoscale, 2015, 7, 19092–19098 RSC
.
- T. A. Debele, S. L. Mekuria and H.-C. Tsai, Mater. Sci. Eng., C, 2016, 68, 964–981 CrossRef CAS PubMed
.
- L. Dai, T. Cheng, Y. Wang, H. Lu, S. Nie, H. He, C. Duan and Y. Ni, Cellulose, 2019, 26, 6891–6901 CrossRef CAS
.
- N. Lin, A. Gèze, D. Wouessidjewe, J. Huang and A. Dufresne, ACS Appl. Mater. Interfaces, 2016, 8, 6880–6889 CrossRef CAS PubMed
.
- Z. Li, H. Shim, M. O. Cho, I. S. Cho, J. H. Lee, S.-W. Kang, B. Kwon and K. M. Huh, Carbohydr. Polym., 2018, 184, 342–353 CrossRef CAS PubMed
.
- B. P. Purcell, D. Lobb, M. B. Charati, S. M. Dorsey, R. J. Wade, K. N. Zellars, H. Doviak, S. Pettaway, C. B. Logdon, J. A. Shuman, P. D. Freels, J. H. Gorman III, R. C. Gorman, F. G. Spinale and J. A. Burdick, Nat. Mater., 2014, 13, 653–661 CrossRef CAS PubMed
.
- G. E. Giammanco, C. T. Sosnofsky and A. D. Ostrowski, ACS Appl. Mater. Interfaces, 2015, 7, 3068–3076 CrossRef CAS PubMed
.
- X. Ding, J. Gao, H. Awada and Y. Wang, J. Mater. Chem. B, 2016, 4, 1175–1185 RSC
.
- Y. Xie, J. Zhao, R. Huang, W. Qi, Y. Wang, R. Su and Z. He, Nanoscale Res. Lett., 2016, 11, 184 CrossRef PubMed
.
- S. Sutton, N. L. Campbell, A. I. Cooper, M. Kirkland, W. J. Frith and D. J. Adams, Langmuir, 2009, 25, 10285–10291 CrossRef CAS PubMed
.
- A. Baral, S. Roy, A. Dehsorkhi, I. W. Hamley, S. Mohapatra, S. Ghosh and A. Banerjee, Langmuir, 2014, 30, 929–936 CrossRef CAS PubMed
.
- H. Guo, G. Cui, J. Yang, C. Wang, J. Zhu, L. Zhang, J. Jiang and S. Shao, Biochem. Biophys. Res. Commun., 2012, 424, 105–111 CrossRef CAS PubMed
.
- V. A. C. Puig-Sanvicens and C. E. Semino, Drug Delivery Transl. Res., 2013, 3, 330–335 CrossRef CAS PubMed
.
- D. Ni, C. A. Ferreira, T. E. Barnhart, V. Quach, B. Yu, D. Jiang, W. Wei, H. Liu, J. W. Engle, P. Hu and W. Cai, J. Am. Chem. Soc., 2018, 140, 14971–14979 CrossRef PubMed
.
- R. Xiong, S. J. Soenen, K. Braeckmans and A. G. Skirtach, Theranostics, 2013, 3, 141–151 CrossRef CAS PubMed
.
- E. Catanzaro, O. Feron, A. G. Skirtach and D. V. Krysko, Front. Immunol., 2022, 13, 925290 CrossRef CAS PubMed
.
- L. Li, Z. Yang, S. Zhu, L. He, W. Fan, W. Tang, J. Zou, Z. Shen, M. Zhang, L. Tang, Y. Dai, G. Niu, S. Hu and X. Chen, Adv. Mater., 2019, 31, 1901187 CrossRef PubMed
.
- C. Xie, X. Zhen, Q. Lei, R. Ni and K. Pu, Adv. Funct. Mater., 2017, 27, 1605397 CrossRef
.
- D. Cui, J. Huang, X. Zhen, J. Li, Y. Jiang and K. Pu, Angew. Chem., 2019, 131, 5981–5985 CrossRef
.
- L. S. L. Janardhanam, V. V. Indukuri, P. Verma, A. C. Dusane and V. V. K. Venuganti, Mater. Sci. Eng., C, 2020, 115, 111118 CrossRef CAS PubMed
.
- L. Van der Meeren, J. Verduijn, J. Li, E. Verwee, D. V. Krysko, B. V. Parakhonskiy and A. G. Skirtach, Appl. Surf. Sci. Adv., 2021, 5, 100111 CrossRef
.
- W. Ou, L. Jiang, R. K. Thapa, Z. C. Soe, K. Poudel, J.-H. Chang, S. K. Ku, H.-G. Choi, C. S. Yong and J. O. Kim, Theranostics, 2018, 8, 4574–4590 CrossRef CAS PubMed
.
- O. Kreft, A. M. Javier, G. B. Sukhorukov and W. J. Parak, J. Mater. Chem., 2007, 17, 4471 RSC
.
- D. Zhu, L. Feng, N. Feliu, A. H. Guse and W. J. Parak, Adv. Mater., 2021, 33, 2008261 CrossRef CAS PubMed
.
- L. I. Kazakova, L. I. Shabarchina, S. Anastasova, A. M. Pavlov, P. Vadgama, A. G. Skirtach and G. B. Sukhorukov, Anal. Bioanal. Chem., 2013, 405, 1559–1568 CrossRef CAS PubMed
.
- L. Van der Meeren, J. Li, B. V. Parakhonskiy, D. V. Krysko and A. G. Skirtach, Anal. Bioanal. Chem., 2020, 412, 5015–5029 CrossRef CAS PubMed
.
- S. Schmidt, P. A. L. Fernandes, B. G. De Geest, M. Delcea, A. G. Skirtach, H. Möhwald and A. Fery, Adv. Funct. Mater., 2011, 21, 1411–1418 CrossRef CAS
.
- M. H. Bakker, C. C. S. Tseng, H. M. Keizer, P. R. Seevinck, H. M. Janssen, F. J. Van Slochteren, S. A. J. Chamuleau and P. Y. W. Dankers, Adv. Healthcare Mater., 2018, 7, 1701139 CrossRef PubMed
.
- J. I. Kim, B. S. Lee, C. Chun, J.-K. Cho, S.-Y. Kim and S.-C. Song, Biomaterials, 2012, 33, 2251–2259 CrossRef CAS PubMed
.
- E. Lengert, A. M. Yashchenok, V. Atkin, A. Lapanje, D. A. Gorin, G. B. Sukhorukov and B. V. Parakhonskiy, RSC Adv., 2016, 6, 20447–20452 RSC
.
- E. Lengert, B. Parakhonskiy, D. Khalenkow, A. Zečić, M. Vangheel, J. M. Monje Moreno, B. P. Braeckman and A. G. Skirtach, Nanoscale, 2018, 10, 17249–17256 RSC
.
- X. Chen, Z. Liu, S. G. Parker, X. Zhang, J. J. Gooding, Y. Ru, Y. Liu and Y. Zhou, ACS Appl. Mater. Interfaces, 2016, 8, 15857–15863 CrossRef CAS PubMed
.
- C. Wang, N. Zhao, Y. Huang, R. He, S. Xu and W. Yuan, Chem. Eng. J., 2020, 401, 126100 CrossRef CAS
.
- K. N. Alagarsamy, S. Mathan, W. Yan, A. Rafieerad, S. Sekaran, H. Manego and S. Dhingra, Bioact. Mater., 2021, 6, 2261–2280 CrossRef CAS PubMed
.
- A. La Porta, A. Sánchez-Iglesias, T. Altantzis, S. Bals, M. Grzelczak and L. M. Liz-Marzán, Nanoscale, 2015, 7, 10377–10381 RSC
.
- N. Bhattarai, J. Gunn and M. Zhang, Adv. Drug Delivery Rev., 2010, 62, 83–99 CrossRef CAS PubMed
.
- K. Lv, Q. Li, L. Zhang, Y. Wang, Z. Zhong, J. Zhao, X. Lin, J. Wang, K. Zhu, C. Xiao, C. Ke, S. Zhong, X. Wu, J. Chen, H. Yu, W. Zhu, X. Li, B. Wang, R. Tang, J. Wang, J. Huang and X. Hu, Theranostics, 2019, 9, 7403–7416 CrossRef CAS PubMed
.
- Z. Yang, G. Liang, L. Wang and B. Xu, J. Am. Chem. Soc., 2006, 128, 3038–3043 CrossRef CAS PubMed
.
- K. Basu, A. Baral, S. Basak, A. Dehsorkhi, J. Nanda, D. Bhunia, S. Ghosh, V. Castelletto, I. W. Hamley and A. Banerjee, Chem. Commun., 2016, 52, 5045–5048 RSC
.
- W. Liu, H. Tang, L. Li, X. Wang, Z. Yu and J. Li, Cell Proliferation, 2021, 54, e13025 CAS
.
- R. Chang, Q. Zou, R. Xing and X. Yan, Adv. Ther., 2019, 2, 1900048 CrossRef
.
- H. Jin, G. Zhao, J. Hu, Q. Ren, K. Yang, C. Wan, A. Huang, P. Li, J.-P. Feng, J. Chen and Z. Zou, ACS Appl. Mater. Interfaces, 2017, 9, 25755–25766 CrossRef CAS PubMed
.
- H. Xuan, X. Tang, Y. Zhu, J. Ling and Y. Yang, ACS Appl. Bio Mater., 2020, 3, 1628–1635 CrossRef CAS PubMed
.
- F. Wang, J. Li, X. Tang, K. Huang and L. Chen, Colloids Surf., B, 2020, 190, 110925 CrossRef CAS PubMed
.
- D. Cai, L. Shi, R. Long, G. Ren, S. Wang and Y. Liu, Carbohydr. Polym., 2021, 261, 117847 CrossRef CAS PubMed
.
- A. R. Correia, I. Sampaio, E. J. Comparetti, N. C. S. Vieira and V. Zucolotto, Talanta, 2021, 233, 122506 CrossRef CAS PubMed
.
- J. Fan, Y. Liu, S. Wang, Y. Liu, S. Li, R. Long, R. Zhang and R. K. Kankala, RSC Adv., 2017, 7, 32786–32794 RSC
.
- F. Zhang, C. Hu, Q. Kong, R. Luo and Y. Wang, ACS Appl. Mater. Interfaces, 2019, 11, 37147–37155 CrossRef CAS PubMed
.
- S. Motamed, M. P. Del Borgo, K. Kulkarni, N. Habila, K. Zhou, P. Perlmutter, J. S. Forsythe and M. I. Aguilar, Soft Matter, 2016, 12, 2243–2246 RSC
.
- W. Nie, C. Peng, X. Zhou, L. Chen, W. Wang, Y. Zhang, P. X. Ma and C. He, Carbon, 2017, 116, 325–337 CrossRef CAS
.
- K. Lalitha, Y. S. Prasad, C. U. Maheswari, V. Sridharan, G. John and S. Nagarajan, J. Mater. Chem. B, 2015, 3, 5560–5568 RSC
.
- Y. Hu, S. Hu, S. Zhang, S. Dong, J. Hu, L. Kang and X. Yang, Sci. Rep., 2021, 11, 9142 CrossRef CAS PubMed
.
- J. Zheng, R. Fan, H. Wu, H. Yao, Y. Yan, J. Liu, L. Ran, Z. Sun, L. Yi, L. Dang, P. Gan, P. Zheng, T. Yang, Y. Zhang, T. Tang and Y. Wang, Nat. Commun., 2019, 10, 1604 CrossRef CAS PubMed
.
- H. Wei, Z. Zhao, Y. Wang, J. Zou, Q. Lin and Y. Duan, ACS Appl. Mater. Interfaces, 2019, 11, 46479–46489 CrossRef CAS PubMed
.
- M. Chang, M. Wang, Y. Chen, M. Shu, Y. Zhao, B. Ding, Z. Hou and J. Lin, Nanoscale, 2019, 11, 10129–10136 RSC
.
- K. Ariga, K. Minami, M. Ebara and J. Nakanishi, Polym. J., 2016, 48, 371–389 CrossRef CAS
.
- J. Yamaguchi and K. Itami, Bull. Chem. Soc. Jpn., 2017, 90, 367–383 CrossRef CAS
.
- L. Zhao, Q. Zou and X. Yan, Bull. Chem. Soc. Jpn., 2019, 92, 70–79 CrossRef CAS
.
- P. Calandra, D. Caschera, V. Turco Liveri and D. Lombardo, Colloids Surf., A, 2015, 484, 164–183 CrossRef CAS
.
- J. K. Scott and G. P. Smith, Science, 1990, 249, 386–390 CrossRef CAS PubMed
.
- G. P. Smith, Science, 1985, 228, 1315–1317 CrossRef CAS PubMed
.
- B. Pelaz, C. Alexiou, R. A. Alvarez-Puebla, F. Alves, A. M. Andrews, S. Ashraf, L. P. Balogh, L. Ballerini, A. Bestetti, C. Brendel, S. Bosi, M. Carril, W. C. W. Chan, C. Chen, X. Chen, X. Chen, Z. Cheng, D. Cui, J. Du, C. Dullin, A. Escudero, N. Feliu, M. Gao, M. George, Y. Gogotsi, A. Grünweller, Z. Gu, N. J. Halas, N. Hampp, R. K. Hartmann, M. C. Hersam, P. Hunziker, J. Jian, X. Jiang, P. Jungebluth, P. Kadhiresan, K. Kataoka, A. Khademhosseini, J. Kopeček, N. A. Kotov, H. F. Krug, D. S. Lee, C.-M. Lehr, K. W. Leong, X.-J. Liang, M. Ling Lim, L. M. Liz-Marzán, X. Ma, P. Macchiarini, H. Meng, H. Möhwald, P. Mulvaney, A. E. Nel, S. Nie, P. Nordlander, T. Okano, J. Oliveira, T. H. Park, R. M. Penner, M. Prato, V. Puntes, V. M. Rotello, A. Samarakoon, R. E. Schaak, Y. Shen, S. Sjöqvist, A. G. Skirtach, M. G. Soliman, M. M. Stevens, H.-W. Sung, B. Z. Tang, R. Tietze, B. N. Udugama, J. S. VanEpps, T. Weil, P. S. Weiss, I. Willner, Y. Wu, L. Yang, Z. Yue, Q. Zhang, Q. Zhang, X.-E. Zhang, Y. Zhao, X. Zhou and W. J. Parak, ACS Nano, 2017, 11, 2313–2381 CrossRef CAS PubMed
.
|
This journal is © The Royal Society of Chemistry 2022 |
Click here to see how this site uses Cookies. View our privacy policy here.