DOI:
10.1039/D2NR03770J
(Communication)
Nanoscale, 2022,
14, 16427-16435
One-dimensional metal thiophosphate nanowires by cluster assembly†
Received
10th July 2022
, Accepted 11th October 2022
First published on 15th October 2022
Abstract
One-dimensional (1D) atomic wires with precise structures are not only excellent platforms for exploring novel 1D physics, but also promising building blocks to assemble functional materials and devices. However, stable atomic wires remain limited and are hard to search using global optimization algorithms. Inspired by the emerging layered ternary chalcogenides, here we offer a design strategy for rational assembly of metal thiophosphate (MPS4) nanowires based on the concept of a superatom. ortho-Thiophosphate [PS4] clusters are linked by proper main-group and transition metal atoms to form closed electronic shells, endowing the assembled nanowires with high dynamic and thermal stabilities. Diverse and exotic electronic band structures are hosted by these ternary MPS4 nanowires, such as the coexistence of a spin–orbit Dirac point protected by nonsymmorphic symmetry and a flat band near the Fermi level, with nanowires being bipolar magnetic semiconductors for electrical control of spin orientation. These 1D Lego blocks can be further built into higher-order architectures via vdW interaction or covalent bonding. This assembly approach generally produces stable atomic wires with designated compositions and structure symmetries to induce peculiar quantum states for future applications.
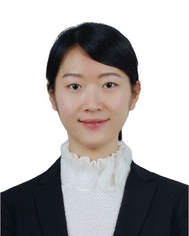 Si Zhou | Dr Si Zhou is a professor at the School of Physics at Dalian University of Technology. She has focused on cluster science and low-dimensional materials using theoretical methods such as first-principles calculations and genetic algorithms. Her achievements include the prediction of many innovative low-dimensional structures for electronics, spintronics, optics, and energy conversion, some of which have been realized in experiments. She has published over 90 peer-reviewed articles as the first- or corresponding-author in Chem. Rev., J. Am. Chem. Soc., Angew. Chem., Int. Ed., Nano Lett., ACS Nano, etc., with citations numbering over 6000 and an H-index of 39. |
Introduction
With the boom of two-dimensional (2D) layered materials, searching for atomic-scale Lego blocks has aroused tremendous interest. Artificial nanostructures with peculiar physical properties and integrated devices with multiple functions can be conveniently constructed by assembly of these van der Waals (vdW) Lego units.1 Stimulated by 2D transition metal dichalcogenides (TMDs) and trihalides, 1D atomic wires of binary transition metal compounds with various stoichiometries have been synthesized in experiments, such as the isolated chains of M6X6 (M = Mo, W; X = S, Se, Te)2–5 and Mo5S4,6 and the single-chain or the few-chain MTe3 (M = Ti, Hf, Nb, V)7,8 and NiY2 (Y = Cl, Br)9 stabilized in carbon nanotubes. The geometrical structures and fundamental properties of binary nanowires with different stoichiometries have been theoretically explored and compared with their 2D counterparts.10–13 Intriguingly, most 1D transition metal chalcogenide nanowires exhibit metallic behavior, while a large number of TMD monolayers are semiconductors.14–16 Transition metal halide nanowires present diverse electronic and magnetic properties, somewhat similar to reported 2D halides (e.g. CrI3, FeCl2, and VI3) that comprise magnetic semiconductors and half metals.17–20
Recently, ternary metal phosphorus trichalcogenides in the form of MPX3 (M = Mn, Fe, Co, Ni, Cu, Zn, Cd, etc.; X = S, Se) emerged as a new family of 2D materials.21 They have a common layered structure with (P2X6)4− bipyramids arranged in a triangular lattice that sandwich the metal layer. The MPX3 monolayers have moderate band gaps of 1.5–3.5 eV falling in the range of visible light wavelengths, and are thereby attractive for optoelectronics, photodetectors, and photocatalysts.22 Intrinsic long-range magnetic order exists in some 2D ternary compounds. For instance, antiferromagnetism is observed in MnPS3, FePS3, and NiPS3 monolayers.23 MnPS3 exhibits the anisotropic behavior of magnetic moments, capable of coupling the valley degree of freedom to the antiferromagnetic order.24 The CuInP2S6 crystal and thin films have been demonstrated to be ferroelectric with an order to disorder transition temperature of 315 K. An out-of-plane electric polarization has been predicted for the CuInP2S6 monolayer.25
Apparently, greater chemical diversity and structural complexity would induce richer physics in the 2D ternary compounds with regard to 2D binary chalcogenides and halides. It brings intriguing questions as to whether the atomic wires of ternary transition metal compounds can stably exist. How do their electronic structures depend on the elemental composition? How do their fundamental properties compare with those of their 2D counterparts? Computational screening the existing bulk materials with layered or chain-like structures has led to the discovery of many 2D and 1D materials.26–28 The theoretical exfoliation energies can be used to evaluate the possibility for cleaving low-dimensional building blocks from their bulk crystals, that is, growth by the “top-down” approach. Then, the elemental substitution is useful to further extend the chemical compositions of monolayers or chains. However, such conventions only predict 2D and 1D materials with very limited structures, and omit a large number of possible structures that may be fabricated by the “bottom-up” approach on proper substrates or templates.
Here we develop a strategy for the rational design of 1D metal thiophosphate nanowires in the form of MPS4 by cluster assembly. Based on the concept of a superatom, ortho-thiophosphate [PS4] units are linked by selective main-group metal atoms and transition metal atoms, such that the tetrahedral [PS4] cluster has a magic number of electrons to satisfy the electronic-shell closure. Following this assembly rule, a total of 26 MPS4 nanowires are constructed. Their energetic, dynamic, and thermal stabilities are comprehensively examined by density functional theory (DFT) calculations to validate our assembly rule. The electronic and magnetic structures of these MPS4 nanowires are investigated to illuminate their potential for device applications. The stable 1D MPS4 building blocks are further exploited to assemble higher-order architectures.
Computational methods
DFT calculations were carried out by the Vienna ab initio simulation package (VASP),29,30 using the planewave basis set with an energy cutoff of 500 eV, the projector augmented wave (PAW) potentials,31,32 and the generalized gradient approximation (GGA)33 parametrized by Perdew, Burke and Ernzerhof (PBE) for the exchange–correlation functional.34 A vacuum space of 15 Å was applied to the directions perpendicular to the axial direction of MPS4 nanowires. Uniform k-point meshes, with density 0.05 Å−1 and 0.0002 Å−1, were used to sample the Brillouin zone of the nanowires for geometry optimization and band structure calculation, respectively.35 The cell parameters of MPS4 nanowires along the axial direction were fully optimized and the ionic positions were relaxed with the convergence criteria for total energy and force of 10−5 eV and 0.01 eV Å−1, respectively. The Heyd–Scuseria–Ernzerhof (HSE06) hybrid functional36 was used to calculate the electronic band structures of simple metal thiophosphate nanowires assembled from group-IIIA atoms (B, Al, Ga, In) and [PS4] units, which predicts band gaps of about 1.2 eV larger than the values given by the PBE functional. For transition metal thiophosphate nanowires and monolayers, the DFT+U method37 was adopted to compute their band structures to account for the strong correlation effect. For consistency, we used the Hubbard on-site Coulomb parameter U = 3 eV for all the transition metal MPS4 nanowires. The obtained electronic band structures have features similar to those calculated by the PBE functional. Other U values were also tested, which generally affect the band gap, but do not influence the metallic systems. The detailed results obtained via different methods are compared in Table S1 and Fig. S1 of the ESI.† The phonon dispersion was calculated by the Phonopy code38 interfaced with the density functional perturbation theory (DFPT) implemented in VASP. The thermal stability of the assembled MPS4 nanowires was characterized by ab initio molecular dynamics (AIMD) simulations implemented in VASP. The molecular orbitals of a [PS4]3− cluster and natural population of the on-site charge were calculated by the Gaussian16 package,39 using the PBE functional accompanied with LANL2DZ basis sets.
Results and discussion
To design metal thiophosphate nanowires, we consider the ortho-thiophosphate [PS4] units in a tetrahedral geometry linked by metal atoms to form a chain, as illustrated in Fig. 1a. The rule for assembling MPS4 nanowires is to reach stable electronic configurations for both [PS4] clusters and M atoms after their bonding. For highly symmetric clusters, the jellium model suggests that the molecular orbitals generated by valence electrons resemble the shapes of atomic orbitals.40 A cluster will have enhanced stability when its number of valence electrons coincides with a closed-shell structure (so-called “magic number”). For a tetrahedral cluster with Td symmetry, the molecular orbitals split in energy and the magic numbers can be determined as 2, 8, 10, 16, 20, 26, 32, etc.41 As a free [PS4] unit carries 29 valence electrons, it has to gain three electrons from the M linkers to reach a magic number and electronic-shell closure. Intuitively, group-IIIA atoms (B, Al, Ga, and In) with three valence electrons, as well as transition metal elements with open d shells and exhibiting variable oxidation states, may satisfy such criteria and form stable 1D nanowires with the [PS4] unit.
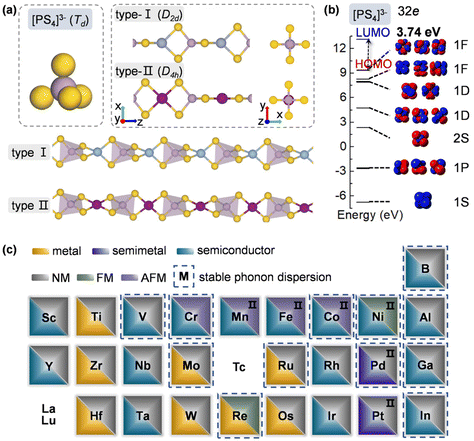 |
| Fig. 1 (a) Geometrical structure of a [PS4]3− cluster (top left panel) and the assembled 1D MPS4 nanowire (top right panel and bottom two panels) from different views. The P, S, and M atoms are shown in purple, yellow, and blue (magenta) colors, respectively. (b) Energy diagrams and molecular orbitals of [PS4]3−. The energy is relative to the vacuum level. The HOMO–LUMO gap is given. (c) Electronic and magnetic properties of 1D MPS4 nanowires, where M is either a transition metal element or a group-IIIA element. The dashed boxes indicate nanowires with a stable phonon dispersion. The systems with type-II structures are indicated by the symbol “II” in the upper right corner. | |
Next, we constructed MPS4 nanowires by linking [PS4] clusters with group-IIIA and transition metal atoms. To determine the ground-state geometry and magnetic order, we adopted different supercells (1 × 1 × 2 and 1 × 1 × 4 unit cells) to arrange the adjacent [PS4] clusters in different orientations and tested different spin configurations for the magnetic atoms. As displayed in Fig. 1a, the two most stable structure phases are found for the assembled MPS4 nanowires. In the type-I configuration, the adjacent [PS4] clusters are in the same orientation, such that each M atom stays in the center of S4 tetrahedra as that of a P atom. The nanowire has D2d symmetry and an M–S–P angle of about 90°. In the type-II configuration, the two adjacent [PS4] units rotate by 90° along the axial direction, such that each M atom stays in the center of the S4 rectangle, resulting in D4h symmetry for the nanowire.
Upon geometry optimization, all the considered 1D chains are stable. For both phases, the [PS4] unit in the nanowire well maintains its tetrahedral structure. We examined the molecular orbitals of a free [PS4]3− ion, which mimics the [PS4] building block in the MPS4 nanowires. It exhibits highly symmetric superatomic orbitals, as displayed in Fig. 1b. The 32 valence electrons fill the electronic shell of 1S21P62S21D101F61F6||1F0 under the symmetry-adapted orbital model,41,42 where the 1F orbital splits and the two lower energy levels are fully occupied, with a large gap of 3.74 eV between the highest occupied molecular orbital (HOMO) and lowest unoccupied molecular orbital (LUMO). These results manifest the superatomic nature of the tetrahedral [PS4] cluster and indicate its high stability by gaining a proper number of electrons when assembled into MPS4 nanowires.
The ground-state structure phases, electronic and magnetic properties, and dynamical stabilities of the assembled MPS4 nanowires are summarized in Fig. 1c. Most of these 1D systems prefer the type-I structure with D2d symmetry, while six MPS4 nanowires (M = Mn, Fe, Co, Ni, Pd, and Pt) have the type-II structure with D4h symmetry. In particular, there are 12 MPS4 nanowires that have stable phonon dispersions (Fig. 2a and Fig. S2†), comprising either the group-IIIA elements (M = B, Al, Ga, and In) or selective transition metal elements (M = V, Cr, Co, Ni, Mo, Re, Ru, and Pd). We have also tested some other main group elements, such as those elements from group-IA (Na), group-IIA (Mg), group-IVA (Sn, Pb), and group-VA (Bi). Their assembled 1D MPS4 structures are either broken down during optimization or have unstable phonon dispersions, which in turn corroborate our proposed assembly criteria. In the following content, we will focus on the 12 MPS4 nanowires that are dynamically stable.
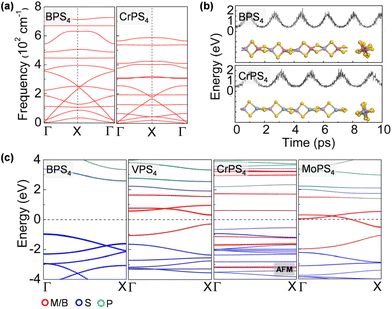 |
| Fig. 2 (a) Phonon dispersions of BPS4 and CrPS4 nanowires. (b) Energy profile during AIMD simulation for BPS4 and CrPS4 nanowires at 300 K. The energy is referred to that of the equilibrium structure at 0 K. The insets are snapshot structures at 10 ps (left: side-view; right: top-view). The M, P, and S atoms are shown in blue (pink), purple, and yellow colors, respectively. (c) Electronic band structures of selective MPS4 nanowires. The contributions from different atoms are shown by color. The Fermi level is shifted to zero (dashed lines). | |
The thermal stability of MPS4 nanowires was assessed by an AIMD simulation. As depicted in Fig. 2b and Fig. S3,† single chains of MPS4 have outstanding thermal stability at 300 K. The structure does not show any noticeable distortion during a simulation time of 10 ps. Taking the BPS4 and CrPS4 nanowires as representatives, the variations of M–S and P–S bond lengths are within 0.14 Å and 0.19 Å for BPS4 and 0.16 Å and 0.12 Å for CrPS4, respectively, signifying the structural robustness of these 1D ternary compound materials. We further characterized the energetic stability of 1D MPS4 nanowires by their formation energy defined as,
| Eform = E(MPS4) − [E(H3PS4) − 3/2E(H2)] − E(M) | (1) |
where
E(MPS
4),
E(H
3PS
4), and
E(H
2) are the total energies of MPS
4 nanowire per formula, a free H
3PS
4 and H
2 molecule, respectively;
E(M) is the energy of the M atom in its most stable bulk form. As presented in
Tables 1 and
2, group-IIIA MPS
4 (M = B, Al, Ga, In) and 3d transition metal MPS
4 (M = V, Cr, Co Ni) nanowires have negative values of
Eform = −1.44 to −0.08 eV, while MoPS
4, RuPS
4, PdPS
4, and RePS
4 nanowires have positive
Eform values. A negative value of
Eform indicates that the formation of an MPS
4 nanowire by metal ion substitution of H
3PS
4 acid is exothermic. These proposed 1D MPS
4 nanowires may be synthesized by the assembly of [PS
4]
3− ions, and group-IIIA metal atoms or transition metal atoms in 1D templates with a hollow cavity. A variety of transition metal chalcogenide and halide chains have been grown by vapor transport in open-ended carbon nanotubes and boron nitride nanotubes in the experiment.
3,43,44
Table 1 Formation energy (Eform) and band gap (Eg) of 1D group-IIIA MPS4 nanowires; inter-chain distance (d) and band gap (Eg) of the assembled 3D MPS4
|
1D |
Bulk |
|
E
form (eV) |
E
g (eV) |
d (Å) |
E
g (eV) |
BPS4 |
−0.93 |
3.57 |
3.72 |
3.21 |
AlPS4 |
−2.42 |
4.14 |
3.71 |
3.55 |
GaPS4 |
−1.34 |
4.01 |
3.74 |
3.17 |
InPS4 |
−0.80 |
3.73 |
3.82 |
3.12 |
Table 2 Magnetic state, band gap (Eg), magnetic moment (M), and exchange energy (Em), of 1D transition metal MPS4 nanowires and the assembled MPS4 monolayers. Formation energy (Eform) is also given for 1D MPS4. For ferromagnets, band gaps for spin-up and spin-down channels are provided
1D |
State |
E
g (eV) |
M (μB) |
E
m (meV) |
E
form (eV) |
VPS4 |
NM |
0.60 |
— |
— |
−1.21 |
CrPS4 |
AFM |
1.15 |
2.78 |
−99 |
−1.44 |
CoPS4 |
AFM |
0.81 |
1.53 |
−8 |
−0.16 |
NiPS4 |
FM |
1.73/0.22 |
0.51 |
26 |
−0.08 |
MoPS4 |
NM |
Metal |
— |
— |
0.13 |
RuPS4 |
NM |
Metal |
— |
— |
0.69 |
PdPS4 |
NM |
0 |
— |
— |
0.06 |
RePS4 |
FM |
1.41/metal |
0.92 |
44 |
1.06 |
2D |
State |
E
g (eV) |
M (μB) |
E
m (meV) |
VPS4 |
AFM |
0.03 |
1.80 |
−15 |
CrPS4 |
FM |
0.66/1.69 |
2.91 |
6 |
CoPS4 |
NM |
0.71 |
— |
— |
NiPS4 |
AFM |
Metal |
0.41 |
−27 |
MoPS4 |
NM |
Metal |
— |
— |
RuPS4 |
NM |
0.37 |
— |
— |
PdPS4 |
NM |
Metal |
— |
— |
RePS4 |
NM |
Metal |
— |
— |
The electronic band structures of the assembled MPS4 nanowires are given in Fig. 2c, 3, 4 and Fig. S4.† The group-IIIA MPS4 (M = B, Al, Ga, and In) nanowires are semiconductors with large band gaps of 3.57–4.14 eV predicted by the HSE06 hybrid functional. Encouragingly, these 1D ternary compounds exhibit diverse electronic and magnetic properties, including those of semiconductors, metals, semimetals, and ferromagnetic and antiferromagnetic semiconductors, and thereby serve as a rich family of 1D candidates for electronics, spintronics, and optoelectronics. We calculated the magnetic anisotropy energy (MAE) given by the energy difference of the magnetization direction parallel and perpendicular to the axial direction. Most of the magnetic MPS4 nanowires have the easy-axis perpendicular to the chain with MAE values of 0.02–0.75 meV per magnetic atom. Only the CoPS4 nanowire has the spin orientation along the chain direction in an antiferromagnetic order. As shown by the electronic band structures in Fig. 2c and Fig. S4,† CrPS4 and CoPS4 nanowires are both antiferromagnetic semiconductors with band gaps of 1.15 and 0.81 eV and magnetic moments of 2.78 and 1.53μB per metal atom, respectively. The VPS4 nanowire is a non-magnetic semiconductor with band gap of 0.60 eV, while the single chains of MoPS4 and RuPS4 exhibit metallic behavior. It is worth mentioning that the CoPS4 nanowire can have a non-collinear magnetic order, with the preference of spin orientation alternately parallel and perpendicular to the wire, as shown in Fig. S5.† However, the energy of the non-collinear magnetic order is 1.6–4.8 meV per Co atom higher than that of the collinear antiferromagnetic ground state.
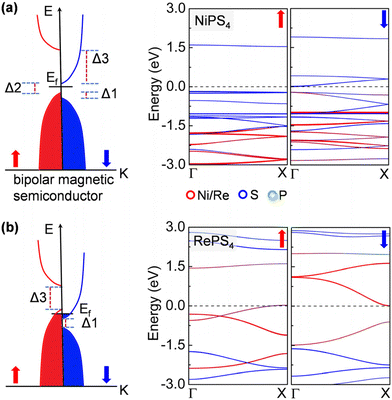 |
| Fig. 3 Electronic band structures of (a) NiPS4 and (b) RePS4 nanowires. The contributions from different atoms or orbitals are shown by the colors. The Fermi level is shifted to zero (red dashed lines). The left panels are schematic plots of their band structures. | |
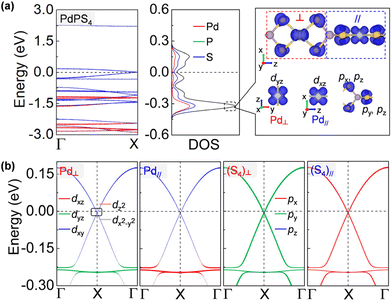 |
| Fig. 4 (a) Electronic band structures and density of states (DOS) of a PdPS4 nanowire calculated without SOC. The right panel displays the partial charge density of the flat band at about −0.3 eV. (b) Band structure of a PdPS4 nanowire calculated with SOC. The contributions from different atoms or orbitals are shown by the colors. The Fermi level is shifted to zero (dashed lines). | |
Remarkably, the NiPS4 nanowire is a bipolar magnetic semiconductor, as is featured by its different conduction band minimum (CBM) and valence band maximum (VBM) in terms of electronic spins.45 As shown in Fig. 3a, the VBM and CBM are contributed to by the majority and minority spin carriers, respectively, both from S atoms. There is a small gap of 0.20 eV, termed the spin–flip gap (Δ2), between VBM and CBM. By applying a small positive (negative) gate voltage, the minority (majority) spin carriers are created, allowing for the electrical control of these carriers’ spin orientations. The spin splitting near the Fermi level is mainly contributed to by S atoms. The RePS4 chain exhibits similar features to that of NiPS4, but has no gap at the Fermi level. As displayed in Fig. 3b, the spin-up and spin-down channels are slightly doped. The electronic states near the Fermi level are dominated by Re atoms. When applying a small gate voltage, minority spin carriers are generated with a large spin-conserved gap (Δ3) of 1.46 eV, while a negative gate voltage is required to generate majority spin carriers with a large spin-conserved gap (Δ1) of 0.62 eV. Therefore, these nanowires can carry completely spin-polarized currents under a proper electric field, allowing for exciting applications, such as a bipolar field effect spin filter and field effect spin valve, as well as detection and separation of entangled electrons from superconductors for quantum information processing.46
More impressively, the PdPS4 nanowire in the type-II configuration is a semimetal that is robust against spin–orbit coupling (SOC) protected by its nonsymmorphic symmetry.47 As shown in Fig. 4a, the CBM and VBM touch each other nearly at the Fermi level. A gap is not opened by adopting denser k-points with 0.0001 Å−1, larger SOC strength, or other U values (see Fig. S6†).48 This type of Dirac point that is robust against SOC is termed a spin–orbit Dirac point (SDP) and is distinct in nature from the SOC-vulnerable Dirac point. It has been reported in some 2D materials, such as RhB4,49 MXenes50 and HfGe0.92Te.51 Even more excitingly, the PdPS4 chain simultaneously harbors SDP and a nearly flat band near the Fermi level (at about −0.2 eV). A flat momentum-energy dispersion is the singularity in density of states, and the charge carriers in it have a zero group velocity and an infinite effective mass. It has been realized in Kagome lattice systems, twisted bilayer graphene and transition metal diachalcogenides,52–55 and may lead to high-temperature superconductivity, fractional Chern insulators, Wigner crystal formations, and so on.56 According to the density of states in Fig. 4a and b, the flat band of a PdPS4 nanowire is localized on the Pd–S4 planar unit, contributed to by the S p orbital and Pd d orbital perpendicular to the Pd–S4 plane. As the neighboring Pd–S4 units are perpendicular to each other, their orbital hybridization is very weak, which may be the origin of the flat dispersion. Therefore, the PdPS4 nanowire with its unique structure is a great 1D platform for exploring the coexistence of multiple exotic quantum states.
Next, we explore the possibility of assembling these 1D MPS4 building blocks into higher-order architectures. The interaction between two MPS4 nanowires is examined. Our results show that the group-IIIA MPS4 (M = B, Al, Ga, In) nanowires favor vdW interactions between neighboring chains, while the transition metal MPS4 nanowires are covalently bonded when approaching each other. As a result, group-IIIA MPS4 (M = B, Al, Ga, In) nanowires form quasi-1D bulk structures as illustrated in Fig. 5a. The supercell consists of parallel MPS4 chains arranged in a rectangular lattice. The chains in the center and at the corner of the supercell are shifted along the axial direction. The vertical distance between the center atoms of two neighboring nanowires is about 6.0 Å, and the separation between the outermost S atoms of two neighboring nanowires is more than 3.7 Å (see Table 1), manifesting the vdW interactions between them. Accordingly, needle-shaped crystallites of the above quasi-1D BPS4 and AlPS4 have been synthesized experimentally.57,58Fig. 5c and Fig. S7† display the electronic band structures of three-dimensional (3D) MPS4 by HSE06 calculations. The band gaps of bulk MPS4 (M = B, Al, Ga, In) are reduced to 3.12–3.55 eV with regard to the single chains. The band along the inter-chain direction is dispersive owing to the moderate interaction between neighboring chains. The band dispersions along the inter-chain direction and axial direction are noticeably different, implying the anisotropic electronic transport behavior of these unique quasi-1D structures, which are desirable for certain device applications, such as anisotropic field effect transistors and photodetectors, thermoelectronics, and piezoelectric and ferroelectric devices.59,60
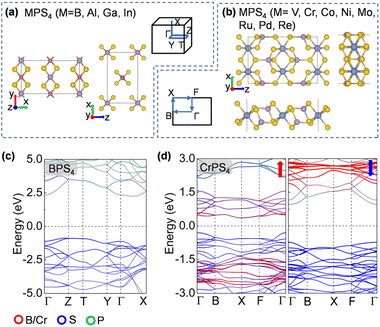 |
| Fig. 5 (a) and (b) Atomic structures of assembled bulk MPS4 (M = B, Al, Ga, In) and an MPS4 (M = V, Cr, Co, Ni, Mo, Ru, Pd, and Re) monolayer, respectively, displayed from different perspectives. The M, P, and S atoms are shown in blue (pink), purple, and yellow colors, respectively. The corresponding Brillouin zones are illustrated. (c) and (d) Electronic band structures of assembled bulk BPS4 and monolayer CrPS4, respectively. The contributions from different atoms or orbitals are shown by color. The Fermi level is shifted to zero (dashed lines). | |
The transition-metal-containing MPS4 (M = V, Cr, Co, Ni, Mo, Ru, Pd, and Re) nanowires favor covalent bonding between adjacent chains, leading to the formation of the monolayer structure shown in Fig. 5b. The tetrahedral [PS4] unit is slightly deformed, while each Cr atom is six-fold coordinated by S atoms and surrounded by four [PS4] units, resulting in an anisotropic rectangular lattice structure. Actually, such a layered structure of CrPS4 has been synthesized in the experiment,61,62 which again corroborates our proposed assembly rule for building stable nanostructures via [PS4] clusters. As shown in Fig. 5d, the CrPS4 monolayer is a ferromagnetic semiconductor with a magnetic moment of 2.91μB per Cr atom, and band gap of 0.66 eV and 1.69 eV for the spin-up and spin-down channels, respectively, in good agreement with previous theoretical reports.63 The VPS4 and NiPS4 monolayers are antiferromagnets, while the others are non-magnetic (see Table 2 and Fig. S8† for details). The magnetic moment prefers to align perpendicular to the layer for all these 2D magnets.
The robustness of the magnetism in cluster-assembled 1D and 2D MPS4 structures is evaluated by the exchange energy Em, defined as the energy difference between the lowest-lying antiferromagnetic state (EAFM) and ferromagnetic state (EFM) per MPS4 formula via:
Spin-polarized charge density distributions for FM and low-energy AFM configurations of 1D and 2D MPS4 structures are presented in Fig. 6a and b. The 1D nanowires of CrPS4, CoPS4, NiPS4 and RePS4 have Em = −99 meV, −8 meV, 26 meV, and 44 meV per formula unit (f.u.), respectively (Table 2). Their magnetic orders can be understood by the super-superexchange interaction along the M–S–P–S–M long superexchange interaction through the Cr–S–Cr bonds with a bond angle of about 90°, as shown in Fig. 6c.64–67 VPS4 and NiPS4 monolayers are antiferromagnetic with Em = −15 meV f.u.−1 and −27 meV f.u.−1, respectively. The former shows competition between FM and AFM coupling along the two in-plane directions, while the latter favors AFM order along both in-plane directions. As a reference, an existing 2D magnetic material like the CrI3 monolayer has Em = 28 meV f.u.−1 and a measured Curie temperature of about 45 K;19 the MnPS3 monolayer has Em = −13 meV f.u.−1 and a measured Néel temperature of about 103 K.68 The present 1D MPS4 nanowires have exchange energies competitive with the values of synthetic 2D magnetic materials, offering stable building blocks for integration of heterostructures and devices with desirable functions.
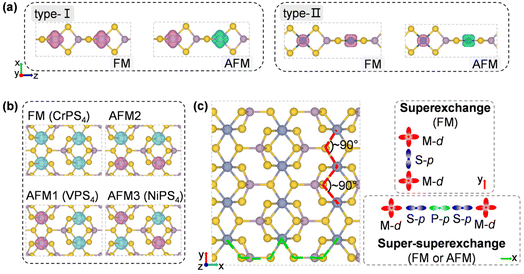 |
| Fig. 6 (a) and (b) Spin density distributions of an MPS4 nanowire and MPS4 monolayer in FM and low-energy AFM states. The ground-state magnetic configuration is indicated for VPS4, CrPS4, and NiPS4 monolayers, respectively. (c) Schematic illustration of superexchange and super-superexchange interactions for the MPS4 monolayer. | |
Conclusions
In summary, based on the superatom concept, we exploited the tetrahedral [PS4] cluster as a building block to construct assemblies of different dimensions by DFT calculations. Ternary MPS4 nanowires with outstanding dynamic and thermal stabilities are obtained by linking [PS4] units with group-IIIA (B, Al, Ga, and In) atoms or transition metal (V, Cr, Co, Ni, Mo, Ru, Pd, and Re) atoms. The assembled nanowires with unique structure symmetries exhibit diverse and peculiar electronic band structures, such as the coexistence of a SOC Dirac point and a nearly flat band, and being bipolar magnetic semiconductors. Moreover, these MPS4 nanowires can be further assembled into quasi-1D bulk structures via vdW interactions as well as layered 2D structures by covalent bonding that are feasible in the experiment.
Conflicts of interest
There are no conflicts to declare.
Acknowledgements
This work was financially supported by the National Natural Science Foundation of China (91961204, 11974068, 12222403) and XinLiaoYingCai Project of Liaoning province, China (XLYC1905014). The authors acknowledge the computer resources provided by the Shanghai Supercomputer Center and the Supercomputing Center of Dalian University of Technology.
References
- A. K. Geim and I. V. Grigorieva, Nature, 2013, 499, 419–425 CrossRef CAS.
- C. A. Slade, A. M. Sanchez and J. Sloan, Nano Lett., 2019, 19, 2979–2984 CrossRef CAS.
- M. Nagata, S. Shukla, Y. Nakanishi, Z. Liu, Y. C. Lin, T. Shiga, Y. Nakamura, T. Koyama, H. Kishida, T. Inoue, N. Kanda, S. Ohno, Y. Sakagawa, K. Suenaga and H. Shinohara, Nano Lett., 2019, 19, 4845–4851 CrossRef CAS.
- J. Lin, O. Cretu, W. Zhou, K. Suenaga, D. Prasai, K. I. Bolotin, N. T. Cuong, M. Otani, S. Okada, A. R. Lupini, J. C. Idrobo, D. Caudel, A. Burger, N. J. Ghimire, J. Yan, D. G. Mandrus, S. J. Pennycook and S. T. Pantelides, Nat. Nanotechnol., 2014, 9, 436–442 CrossRef CAS PubMed.
- N. Kanda, Y. Nakanishi, D. Liu, Z. Liu, T. Inoue, Y. Miyata, D. Tomanek and H. Shinohara, Nanoscale, 2020, 12, 17185–17190 RSC.
- X. Liu, T. Xu, X. Wu, Z. Zhang, J. Yu, H. Qiu, J. H. Hong, C. H. Jin, J. X. Li, X. R. Wang, L. T. Sun and W. Guo, Nat. Commun., 2013, 4, 1776 CrossRef PubMed.
- S. Meyer, T. Pham, S. Oh, P. Ercius, C. Kisielowski, M. L. Cohen and A. Zettl, Phys. Rev. B, 2019, 100, 041403(R) CrossRef.
- S. Stonemeyer, J. D. Cain, S. Oh, A. Azizi, M. Elasha, M. Thiel, C. Song, P. Ercius, M. L. Cohen and A. Zettl, J. Am. Chem. Soc., 2021, 143, 4563–4568 CrossRef CAS.
- M. V. Kharlamova, L. V. Yashina, A. A. Eliseev, A. A. Volykhov, V. S. Neudachina, M. M. Brzhezinskaya, T. S. Zyubina, A. V. Lukashin and Y. D. Tretyakov, Phys. Status Solidi B, 2012, 249, 2328–2332 CrossRef CAS.
- S. Oh, S. Chae, B. J. Kim, K. H. Choi, W.-S. Jang, J. Jang, Y. Hussain, D. K. Lee, Y.-M. Kim, H. K. Yu and J.-Y. Choi, RSC Adv., 2018, 8, 33980–33984 RSC.
- Y. Zhou, L. Wang, S. Chen, S. Qin, X. Liu, J. Chen, D.-J. Xue, M. Luo, Y. Cao, Y. Cheng, E. H. Sargent and J. Tang, Nat. Photonics, 2015, 9, 409–415 CrossRef CAS.
- J. Lee, B. J. Kim, Y. K. Chung, W. G. Lee, I. J. Choi, S. Chae, S. Oh, J. M. Kim, J. Y. Choi and J. Huh, J. Raman Spectrosc., 2020, 51, 1100–1107 CrossRef CAS.
- M. N. Kozlova, Y. V. Mironov, E. D. Grayfer, A. I. Smolentsev, V. I. Zaikovskii, N. A. Nebogatikova, T. Y. Podlipskaya and V. E. Fedorov, Chemistry, 2015, 21, 4639–4645 CrossRef CAS.
- C. Shang, L. Fu, S. Zhou and J. Zhao, JACS Au, 2021, 1, 147–155 CrossRef CAS PubMed.
- M.-S. Qiu, H.-H. Guo, Y. Zhang, B.-J. Dong, S. Ali and T. Yang, Chin. Phys. B, 2019, 28, 106103 CrossRef CAS.
- G. H. Han, D. L. Duong, D. H. Keum, S. J. Yun and Y. H. Lee, Chem. Rev., 2018, 118, 6297–6336 CrossRef CAS PubMed.
- Y.-P. Wang and M.-Q. Long, Phys. Rev. B, 2020, 101, 024411 CrossRef CAS.
- G.-D. Zhao, X. Liu, T. Hu, F. Jia, Y. Cui, W. Wu, M.-H. Whangbo and W. Ren, Phys. Rev. B, 2021, 103, 014438 CrossRef CAS.
- B. Huang, G. Clark, E. Navarro-Moratalla, D. R. Klein, R. Cheng, K. L. Seyler, D. Zhong, E. Schmidgall, M. A. McGuire, D. H. Cobden, W. Yao, D. Xiao, P. Jarillo-Herrero and X. Xu, Nature, 2017, 546, 270–273 CrossRef CAS PubMed.
- Y. Zhu, H. Li, T. Chen, D. Liu and Q. Zhou, Vacuum, 2020, 182, 109694 CrossRef CAS.
- M. Zhu, H. Kou, K. Wang, H. Wu, D. Ding, G. Zhou and S. Ding, Mater. Horiz., 2020, 7, 3131–3160 RSC.
- K. Z. Du, X. Z. Wang, Y. Liu, P. Hu, M. I. Utama, C. K. Gan, Q. Xiong and C. Kloc, ACS Nano, 2016, 10, 1738–1743 CrossRef CAS.
- R. Gusmão, Z. Sofer, D. Sedmidubský, Š. Huber and M. Pumera, ACS Catal., 2017, 7, 8159–8170 CrossRef.
- T. Olsen, J. Phys. D: Appl. Phys., 2021, 54, 314001 CrossRef CAS.
- S. Huang, Z. Shuai and D. Wang, J. Mater. Chem. A, 2021, 9, 2734–2741 RSC.
- F. Caruso, M. R. Filip and F. Giustino, Phys. Rev. B: Condens. Matter Mater. Phys., 2015, 92, 125134 CrossRef.
- W.-G. Lee, S. Chae, Y. K. Chung, S. Oh, J.-Y. Choi and J. Huh, Phys. Status Solidi RRL, 2019, 13, 18500517 Search PubMed.
- M. S. Stark, K. L. Kuntz, S. J. Martens and S. C. Warren, Adv. Mater., 2019, 31, 1808213 CrossRef.
- G. Kresse and J. Furthmuller, Phys. Rev. B: Condens. Matter Mater. Phys., 1996, 54, 11169–11186 CrossRef CAS.
- G. Kresse and J. Hafner, Phys. Rev. B: Condens. Matter Mater. Phys., 1993, 47, 558–561 CrossRef CAS.
- G. Kresse and D. Joubert, Phys. Rev. B: Condens. Matter Mater. Phys., 1999, 59, 1758–1775 CrossRef CAS.
- P. E. Blochl, Phys. Rev. B: Condens. Matter Mater. Phys., 1994, 50, 17953–17979 CrossRef.
- J. P. Perdew, K. Burke and M. Ernzerhof, Phys. Rev. Lett., 1996, 77, 3865–3868 CrossRef CAS PubMed.
- J. P. Perdew, M. Ernzerhof and K. Burke, J. Chem. Phys., 1996, 105, 9982–9985 CrossRef CAS.
- H. J. Monkhorst and J. D. Pack, Phys. Rev. B: Condens. Matter Mater. Phys., 1976, 13, 5188–5192 CrossRef.
- A. V. Krukau, O. A. Vydrov, A. F. Izmaylov and G. E. Scuseria, J. Chem. Phys., 2006, 125, 224106 CrossRef PubMed.
- V. I. Anisimov, J. Zaanen and O. K. Andersen, Phys. Rev. B: Condens. Matter Mater. Phys., 1991, 44, 943–954 CrossRef CAS.
- K. Parlinski, Z. Q. Li and Y. Kawazoe, Phys. Rev. Lett., 1997, 78, 4063–4066 CrossRef CAS.
-
M. J. Frisch, G. W. Trucks, H. B. Schlegel, G. E. Scuseria, M. A. Robb, J. R. Cheeseman, G. Scalmani, V. Barone, G. A. Petersson, H. Nakatsuji, X. Li, M. Caricato, A. V. Marenich, J. Bloino, B. G. Janesko, R. Gomperts, B. Mennucci, H. P. Hratchian, J. V. Ortiz, A. F. Izmaylov, J. L. Sonnenberg, D. Williams-Young, F. Ding, F. Lipparini, F. Egidi, J. Goings, B. Peng, A. Petrone, T. Henderson, D. Ranasinghe, V. G. Zakrzewski, J. Gao, N. Rega, G. Zheng, W. Liang, M. Hada, M. Ehara, K. Toyota, R. Fukuda, J. Hasegawa, M. Ishida, T. Nakajima, Y. Honda, O. Kitao, H. Nakai, T. Vreven, K. Throssell, J. A. Montgomery, Jr., J. E. Peralta, F. Ogliaro, M. J. Bearpark, J. J. Heyd, E. N. Brothers, K. N. Kudin, V. N. Staroverov, T. A. Keith, R. Kobayashi, J. Normand, K. Raghavachari, A. P. Rendell, J. C. Burant, S. S. Iyengar, J. Tomasi, M. Cossi, J. M. Millam, M. Klene, C. Adamo, R. Cammi, J. W. Ochterski, R. L. Martin, K. Morokuma, O. Farkas, J. B. Foresman and D. J. Fox, Wallingford CT, 2016.
- W. A. de Heer, Rev. Mod. Phys., 1993, 65, 611–676 CrossRef CAS.
- T. Tsukamoto, N. Haruta, T. Kambe, A. Kuzume and K. Yamamoto, Nat. Commun., 2019, 10, 3727 CrossRef.
-
S. Xing, L. Wu, Z. Wang, X. Chen, H. Liu, S. Han, L. Lei, L. Zhou, Q. Zheng, L. Huang, X. Lin, S. Chen, L. Xie, X. Chen, H.-J. Gao, Z. Cheng, J. Guo, S. Wang and W. Ji, 2022, DOI:10.48550/arXiv.2110.09058.
- J. Sloan, S. J. Grosvenor, S. Friedrichs, A. I. Kirkland, J. L. Hutchison and M. L. H. Green, Angew. Chem., Int. Ed., 2002, 41, 1156–1159 CrossRef CAS.
- T. Pham, S. Oh, P. Stetz, S. Onishi, C. Kisielowski, M. L. Cohen and A. Zettl, Science, 2018, 361, 263–266 CrossRef CAS PubMed.
- X. Li and J. Yang, Phys. Chem. Chem. Phys., 2013, 15, 15793–15801 RSC.
- X. Li and J. Yang, Natl. Sci. Rev., 2016, 3, 365–381 CrossRef CAS.
- S. M. Young and C. L. Kane, Phys. Rev. Lett., 2015, 115, 126803 CrossRef PubMed.
- S. Borisenko, Q. Gibson, D. Evtushinsky, V. Zabolotnyy, B. Büchner and R. J. Cava, Phys. Rev. Lett., 2014, 113, 027603 CrossRef CAS PubMed.
- Z. Gao, Q. Wang, W. Wu, Z. Tian, Y. Liu, F. Ma, Y. Jiao and S. A. Yang, Phys. Rev. B, 2021, 104, 245423 CrossRef CAS.
- H. Fashandi, V. Ivády, P. Eklund, A. L. Spetz, M. I. Katsnelson and I. A. Abrikosov, Phys. Rev. B: Condens. Matter Mater. Phys., 2015, 92, 155142 CrossRef.
-
L. Chen, L. Q. Zhou, Y. Zhou, C. Liu, Z. N. Guo, S. Y. Gao, W. H. Fan, J. F. Xu, Y. X. Guo, K. Liao, J. O. Wang, H. M. Weng and G. Wang, 2022, DOI:10.48550/arXiv.2201.05833.
- M. Kang, L. Ye, S. Fang, J.-S. You, A. Levitan, M. Han, J. I. Facio, C. Jozwiak, A. Bostwick, E. Rotenberg, M. K. Chan, R. D. McDonald, D. Graf, K. Kaznatcheev, E. Vescovo, D. C. Bell, E. Kaxiras, J. van den Brink, M. Richter, M. P. Ghimire, J. G. Checkelsky and R. Comin, Nat. Mater., 2020, 19, 163–169 CrossRef CAS.
- L. Ye, M. Kang, J. Liu, F. von Cube, C. R. Wicker, T. Suzuki, C. Jozwiak, A. Bostwick, E. Rotenberg, D. C. Bell, L. Fu, R. Comin and J. G. Checkelsky, Nature, 2018, 555, 638–642 CrossRef CAS.
- D. Marchenko, D. V. Evtushinsky, E. Golias, A. Varykhalov, T. Seyller and O. Rader, Sci. Adv., 2018, 4, eaau0059 CrossRef CAS.
- M. I. B. Utama, R. J. Koch, K. Lee, N. Leconte, H. Li, S. Zhao, L. Jiang, J. Zhu, K. Watanabe, T. Taniguchi, P. D. Ashby, A. Weber-Bargioni, A. Zettl, C. Jozwiak, J. Jung, E. Rotenberg, A. Bostwick and F. Wang, Nat. Phys., 2021, 17, 184–188 Search PubMed.
- J.-W. Rhim and B.-J. Yang, Adv. Phys.: X, 2021, 6, 1901606 Search PubMed.
- A. Kuhn, R. Eger, P. Ganter, V. Duppel, J. Nuss and B. V. Lotsch, Z. Anorg. Allg. Chem., 2014, 640, 2663–2668 CrossRef CAS.
- F. Pielnhofer, L. M. Schoop, A. Kuhn, R. Eger, J. Nuss, H. Nuss and B. V. Lotsch, Z. Anorg. Allg. Chem., 2019, 645, 267–271 CrossRef CAS.
- B.-Z. Sun, Z. Ma, C. He and K. Wu, Phys. Chem. Chem. Phys., 2015, 17, 29844–29853 RSC.
- L. Li, W. Han, L. Pi, P. Niu, J. Han, C. Wang, B. Su, H. Li, J. Xiong, Y. Bando and T. Zhai, InfoMat, 2019, 1, 54–73 CrossRef CAS.
- J. Son, S. Son, P. Park, M. Kim, Z. Tao, J. Oh, T. Lee, S. Lee, J. Kim, K. Zhang, K. Cho, T. Kamiyama, J. H. Lee, K. F. Mak, J. Shan, M. Kim, J. G. Park and J. Lee, ACS Nano, 2021, 15, 16904–16912 CrossRef CAS.
- A. K. Budniak, N. A. Killilea, S. J. Zelewski, M. Sytnyk, Y. Kauffmann, Y. Amouyal, R. Kudrawiec, W. Heiss and E. Lifshitz, Small, 2020, 16, 1905924 CrossRef CAS PubMed.
- Q. Chen, Q. Ding, Y. Wang, Y. Xu and J. Wang, J. Phys. Chem. C, 2020, 124, 12075–12080 CrossRef CAS.
- J. Xu, C. Xu, J.-B. Liu, L. Bellaiche, H. Xiang, B.-X. Liu and B. Huang, npj Comput. Mater., 2019, 5, 114 CrossRef CAS.
- P. W. Anderson, Phys. Rev., 1959, 115, 2–13 CrossRef CAS.
- J. B. Goodenough, Phys. Rev., 1955, 100, 564–573 CrossRef CAS.
- J. Kanamori, J. Phys. Chem. Solids, 1959, 10, 87–98 CrossRef CAS.
- J. Yang, Y. Zhou, Q. Guo, Y. Dedkov and E. Voloshina, RSC Adv., 2020, 10, 851–864 RSC.
Footnote |
† Electronic supplementary information (ESI) available: Detailed structural parameters, stability, and electronic properties results of 1D, 2D and bulk MPS4. See DOI: https://doi.org/10.1039/d2nr03770j |
|
This journal is © The Royal Society of Chemistry 2022 |
Click here to see how this site uses Cookies. View our privacy policy here.