DOI:
10.1039/D1OB01558C
(Review Article)
Org. Biomol. Chem., 2022,
20, 73-97
Covalent nanosynthesis of fluorene-based macrocycles and organic nanogrids
Received
8th August 2021
, Accepted 19th October 2021
First published on 3rd December 2021
Abstract
Gridization is an alternative way to create macromolecules of various sizes in addition to linear and dendritic polymerization as well as cyclization. Organic nanogrids are an expanding family of macrocycle-like closed structures at the nanoscale, but with a series of well-defined extension edges and vertices. Cyclic nanogrids can be used as nanoscale building blocks for the fabrication of not only rotaxanes, catenanes, knots, 3D cages, but also nanopolymers, covalent organic frameworks (COFs), metal–organic frameworks (MOFs), and complex molecular cross-scale architectures. In this review, the history of fluorene-based macrocycles has first been explored, followed by the development of the synthetic methodologies; in particular, fluorene-based nanogrids are highlighted owing to their features and applications. Typically, fluorenes are fused arenes with a hybrid entity between tetrahedral Csp3 and Csp2. Four ingenious connection modes of fluorene-based macrocycles, including 2,7-, 3,6-, 9,9-, and 2,9-linkages, fully demonstrate the geometric possibilities of the macrocycles and nanogrids. Such fluorene-based nanogrids will give birth to organic intelligence.
1. Introduction
In addition to linear and dendritic polymerization as well as cyclization, gridization is an alternative way to create macromolecules at various sizes. Macrocycles have been extensively studied owing to their key roles in molecular recognition,1 supramolecular host–guest systems,2 catalysis,3 and materials chemistry.4 However, the topological design of completely cyclic structures presents clear difficulties in terms of their interconnection. The edges and vertices of nanogrids that are an alternative to macrocycles are severely ignored. More recently, we gained insight into the powerful gridization of molecular building blocks, especially gridochemistry initiated by reorganizing the π-systems of organic semiconductors.5 In this review, we systemically describe the development evolution from fluorene-based macrocycles to organic nanogrids, and clarify their current knowledge.
Fluorene was discovered accidentally in 1867 by the French scientist Berthelot during the distillation of crude anthracene oil. Several methods have recently been reported for the synthesis of fluorenes from low-cost stuffs.6 Fluorene segment has a unique structure, in which a five-membered ring is fused with two benzene rings,7 and can usually be found in bowl-shaped arenes,8 drugs,9 molecular motors,10 and organic semiconductors.11 Moreover, fluorenes offer a paradigm for fluorene-like building blocks, such as heterofluorenes,12 diazafluorenes,13 cyclopentadithiophenes (CPDT),14 indenofluorenes,15 and truxenes.16
Fluorenes suffer from many reactions, including electropolymerization reactions,17 Friedel–Crafts (F–C) reactions,18 Suzuki coupling reactions, and Yamamoto coupling reactions.19 Many types of fluorene derivatives have been reported; examples include small molecules, such as sterically hindered diarylfluorenes20 and spirobifluorenes;21 linear polyfluorenes,22 including soluble poly-dialkylfluorenes23 and dendritic oligofluorene;24 and closed-loop structures, including covalent organic frameworks (COFs),25 metal-organic frameworks (MOFs),26 macrocycles,27 and gridarenes.28 Fluorene and its derivatives have extensive practical applications owing to their high-efficiency blue emission29 and excellent charge-transport properties,30 including perovskite solar cells,31 fluorescent organic light-emitting diodes (OLEDs),32 phosphorescent OLEDs,33 thermally activated delayed fluorescence (TADF) OLEDs,34 photocatalysts,35 nonvolatile memory devices,36 organic lasers,37 and organic field-effect transistors.38
Fluorene-based closed-loop structures have received extensive attention in recent years. Numerous methodologies have emerged over the past decades, realizing a diverse and sophisticated range of fluorene-based macrocycles. Fundamentally, the connection patterns of fluorenes are crucial in fluorene-based macrocycles. Nine kinds of substitution sites, numbered sequentially in turn from 1 (the next position of Csp3 atom) to 9 (Csp3), are available in the fluorene. Unsurprisingly, multiple active sites of fluorenes allowed four connection patterns: 2,7-, 3,6-, 9,9, and 2,9-linkages (Fig. 1). The 2,7-linkage and 3,6-linkage are commonly used to install fully conjugated cyclic polyfluorenes.39 The 2,9- and 9,9-positions mainly facilitate the installation of nonconjugated structures connected by Csp2–Csp3 bonds.5a,40 These nonconjugated structures offer two main advantages: (I) good expandability and solubility and (II) 2,9- and 9,9-linked macrocycles have strong steric hindrance that may inhibit intermolecular stacking.
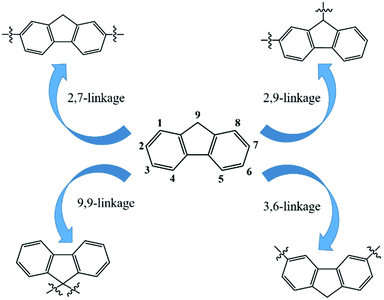 |
| Fig. 1 Four connection modes of a fluorene. | |
In 2014, our group discovered the key paradigm of the 2,9- linkage for the installation of the a new fluorene-based gridarene having a shape like the 日 character.18 Gridarenes become a new class of fluorene-based nanostructures with well-defined edges and vertices; these compounds have extendability, installability, programmability, and scalability5 properties that differ significantly from those of other covalent organic macrocycles, such as calix[n]arenes,41 pillar[n]arenes,42 three-dimensional (3D) cages,43 catenanes,44 and rotaxanes.45 In the background, this review presents an overview of synthetic approaches for fluorene-based cyclic compounds based on four different connection models of fluorenes, involving 2,7-, 3,6-, 9,9-, and 2,9-linked patterns. In particular, the features and applications of fluorene-based nanogrids are highlighted. Finally, there is a perspective on fluorene-based organic intelligence.
2. 2,7-Linked fluorene-based macrocycles
2,7-Linkages of fluorenes generally result in linear polymers;46 only under specific reaction conditions and with specific substrates can multidimensional expansion of 2,7-linkages occur to form π-conjugated macrocycles. Among them, fluorene-based cycloparaphenylene (CPP)-like compounds, as a representative π-conjugated macrocycle, are quite important in organic electronics owing to their unique electronic structures and remarkable strain-dependent fluorescence.47 Fluorene-based macrocycles based on 2,7-linkages were synthesized as early as 1985,48 and numerous synthetic methodologies have been reported over the last decade. Commonly used synthetic methods fall into one of four categories: template methods, that include bridging templates49 and Yamago Pt-macrocyclization;50 coupling reaction, including McMurry, Glaser, and Stille coupling reactions and so on;51 condensation reactions;1 and click reactions.52
2.1. Porphyrin template method
The first example of macrocyclizaion via a porphyrin template was reported by Mullen in 2006.49 In this study, substrates 1 and 2 underwent a two-step synthesis to form product 3 at a total yield of 68%; then, 3 was subjected to a Ni-catalyzed Yamamoto coupling reaction and the removal of the template led to the formation of the 12-membered macrocycle 4 at a total yield of approximately 1.6% (Scheme 1).49 Subsequently, in 2009, by replacing carbazole with fluorene, the synthesis of 7, containing twelve fluorenes, at 1.5% yield was performed under the same conditions from intermediate 6. Only traces of the 12-membered macrocycles were produced, clearly demonstrating the superior efficacy of the porphyrin template method, and the utilization of a template can effectively prevent the formation of linear oligomers (Scheme 2).27
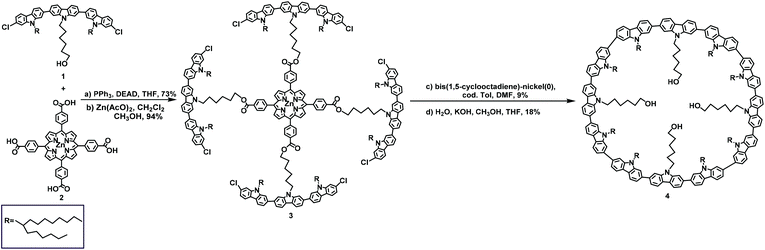 |
| Scheme 1 Bridging template method for the synthesis of a carbazole-based macrocycle. | |
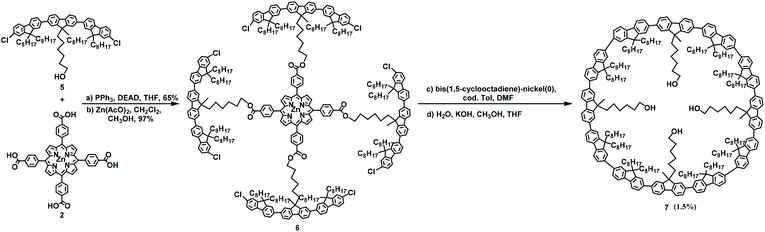 |
| Scheme 2 Bridging template method for the synthesis of a fluorene-based macrocycle. | |
2.2. Yamago Pt-macrocyclization
In 2010, Yamago and co-workers utilized 4,4′-bis(trimethylstannyl)biphenyl to interact with PtCl2(cod) and synthesize square platinum complexes. The subsequent ligand exchange with the 1,1′-bis(diphenylphosphino)ferrocene (Dppf) ligand, through a bromine-induced reduction elimination process, led to successful synthesis of the smallest CPP compound.53 Such a strategy is referred to as Yamago Pt-macrocyclization, which has been widely applied to the corresponding construction of structural units comprising carbon nanotube (CNT) and single-wall carbon nanotube (SWNT) molecules owing to its mild and neutral reaction conditions.50,54
The first example of cyclofluorenes was reported by Yamago et al. in 2015 (Scheme 3).50 In this work, substrate 8 was reacted with the ligand PtCl2(cod) and Dppf, respectively, and then intermediate 9 was synthesized with a total yield of about 27%. The triangle-shaped macrocycle 10 was then obtained from 9 with a yield of 22%. However, the cyclic tetramer 12 was accidently obtained with a yield of 27% when replacing PtCl2(cod) with PtCl2(nbd). However, in this reaction process, highly volatile and toxic trimethyltin chloride by-products were produced. Hence, our group included the nontoxic organoboron reagents 13 in the reaction to fabricate the square platinum complex 14b. Triphenylphosphine was subsequently added for the reduction/elimination process. The construction of the corresponding structural units comprising the cyclic tetramer 15b with a total yield of 14% (Scheme 3) was successfully realized through this procedure.39a In 2018, Poriel's group used the same reaction to construct 14a, by using ethyl rather than propyl at the 9-position of the fluorene, and succeeded in synthesizing the tetrameric nanoring 15a with a yield of 12% (Scheme 3).47a The effect of the alkyl chain length on the size of polyfluorene was inventively proposed via comparison with previous studies reported by Yamago and Huang. In 2020, they used the same methods to synthesize a pentamer for the first time by changing the 9-position alkyl chain length of the fluorene.55 The results revealed that when this 9-position contained a butyl group, the cyclic tetramer 15c with a yield of 17% and pentamer 15d with a yield of 6% were obtained. However, substituting a hexyl for a butyl generates product 15e with only 7% of the pentamer (Scheme 3). Analysis of the crude products using 1H nuclear magnetic resonance (1H NMR) in this work further confirmed that the alkyl chain length at the 9-position of the fluorene plays a key role in the synthesis of macrocycles with different sizes.
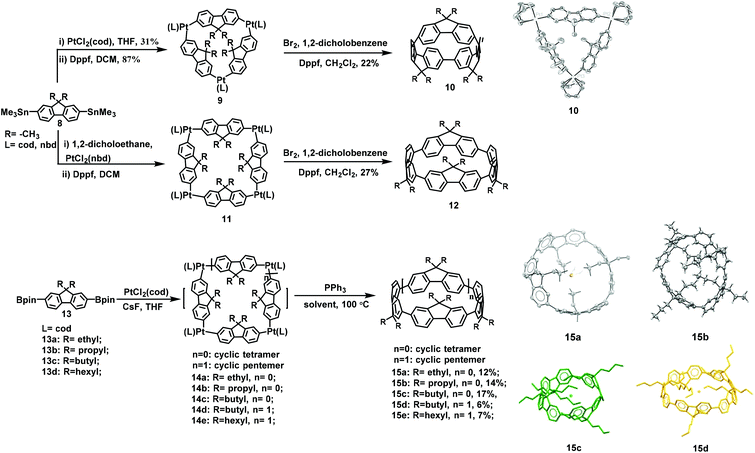 |
| Scheme 3 Platinum-mediated synthesis of macrocycles and single crystal structures of macrocycles 10 and 15a–d. | |
As observed from the results discussed, macrocycles of various sizes were obtained at different concentrations with the same utilization of ligand PtCl2(cod) in this reaction. For example, Yamago reported that only the trimer was obtained in dilute concentrations,50 whereas both Huang and Poriel observed macrocyclic and linear tetramers in a concentrated solution, and no trimer was formed.39a,47a The effect of concentration on the shape of the macrocycles has been previously investigated.56 Hence, it is doubtful whether the concentration of precursors will affect the shape of cyclic oligomers. These findings will greatly promote the synthesis of novel fluorene-based polymers and their applications in organic electronics.
2.3. Friedel–Crafts (F–C) reaction
Recently, Chen's group reported a new class of symmetric pentagon macrocycles synthesized in good yields by simple F–C reaction. In this reaction, when substrates 16 and paraformaldehyde were reacted in the presence of a catalytic amount of BF3·Et2O, only pentagon macrocycles 17 were isolated in 71–73% yields, while no other cyclic analogues were obtained (Scheme 4).57 Moreover, it has been proved that all resulting macrocycles could form 1
:
1 complexes with fullerenes C60 and C70 with high association constants of more than 105 M−1.
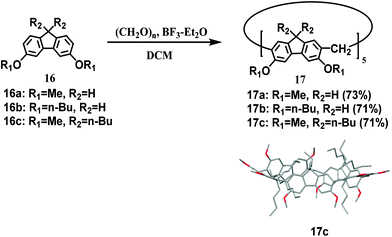 |
| Scheme 4 Synthesis of pentagon macrocycles 17 and the single crystal structure of 17c. | |
2.4. Coupling reactions
To overcome the low yield and complex steps in the synthesis of macrocyclic compounds, in 2008, Pei et al. designed and synthesized the U-shaped building blocks 18 and 19. McMurry and Glaser coupling reactions (Scheme 5) were then used to synthesize two shape-persistent elliptic macrocycles MC1 and MC2 in 29% and 52% isolated yields, respectively.51a
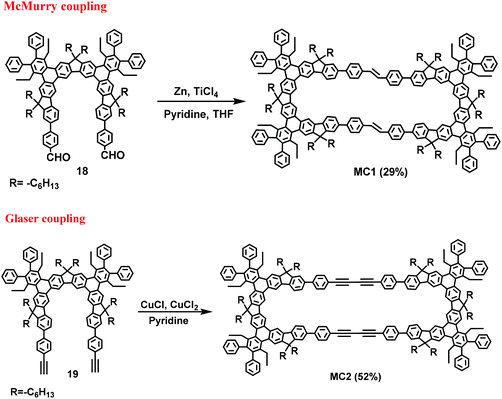 |
| Scheme 5 McMurry and Glaser coupling reaction for the synthesis of MC1 and MC2. | |
In 2015, Jäkle et al. fabricated a conjugated bipolar B/N macrocycle MC3 by introducing a boron atom and carbazole. In this paper, the synthesis of MC3 in 24% yield was achieved when compounds 20 and 3,6-bis(trimethyl-stannyl)carbazole (21) were employed as the starting materials (Scheme 6) via a Pd-catalyzed Stille coupling reaction.51b
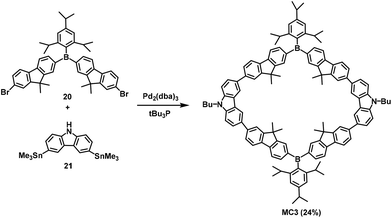 |
| Scheme 6 Stille coupling reaction for the synthesis of MC3. | |
2.5. Condensation reaction
Jäkle's research group has conducted in-depth research on conjugated organoboron macrocycles other than the abovementioned macrocycle MC3 (Scheme 6). In 2011, they used organometallic condensation to synthesize new highly luminescent macrocycles MC4 including six Lewis acid boron centers.58 In this reaction, the resulting BBr2-terminated species 22 was first treated with 2,7-bis(trimethylstannyl)-9,9-dimethylfluorene (FlSn2) under high-dilution conditions, and then reacted with TipCu (Tip = 2,4,6-triisopropylphenyl) to prepare the macrocyclic hexamer MC4 in 31% yield (Scheme 7). However, it is difficult to synthesize macrocyclic tetramers owing to the high strain energy. Hence, as reported in 2015, they replaced 2,7-substituted fluorene with 3,6-substituted carbazole and synthesized the conjugated bipolar B/N macrocyclic tetramers MC5 by the same method (Scheme 8).51b The target macrocycle MC5 was afforded with a yield of 61% from substrates 23 and FlSn2.
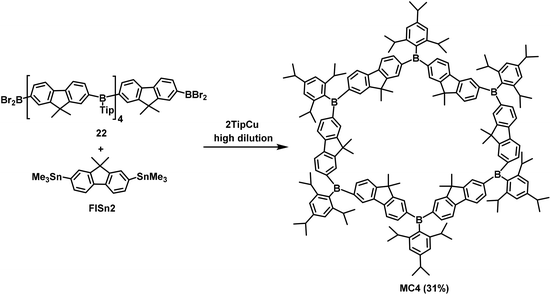 |
| Scheme 7 Organometallic condensation reaction for the synthesis of MC4. | |
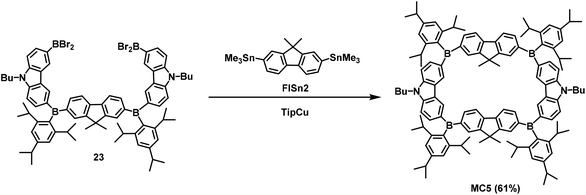 |
| Scheme 8 Organometallic condensation reaction for the synthesis of MC5. | |
To seek new macrocycles with potential applications in ion channels and the assembly of molecular machines, Wang's group synthesized a series of corona[n](het)arenes connected through heteroatoms, and controlled the macrocyclic conformation and recognition properties of such molecules by changing the oxidation state of the bridging heteroatoms. In 2016, they performed a one-pot macrocyclic condensation method of compounds 24 and 25 to prepare the target macrocycle 26 in 63% yield. Subsequently, the Diels–Alder reaction provided macrocyle 30 in 98% yield when the cyclopentanone-derived enamine 28 was treated with a mixture of p-toluenesulfonic acid and acetic acid. Similarly, norbornadiene 27 was employed instead of enamine 28 to react with 26 to prepare macrocycle 29 with a yield of 95%. Finally, the bridged sulfur atoms in macrocycle 30 were oxidized under catalysis with m-chloroperbenzoic acid (m-CPBA) to produce product 31 with a 97% yield (Scheme 9).1
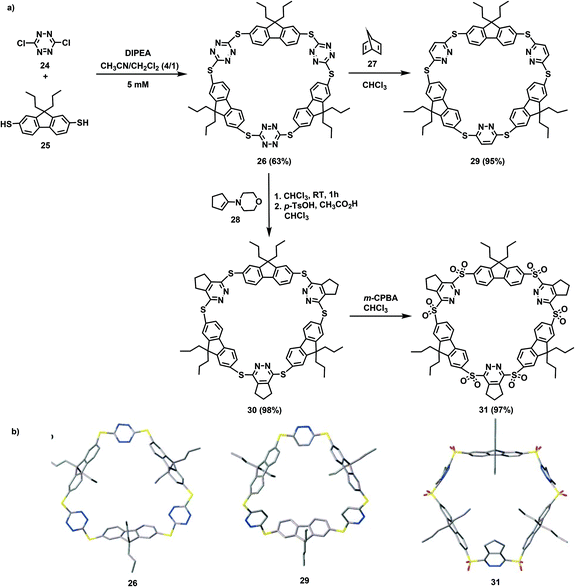 |
| Scheme 9 (a) The route for the synthesis of fluorene-based macrocycles. (b) X-ray crystal structures of 26, 29, and 31. | |
2.6. Click reaction
Click reactions have been widely used in organic synthesis owing to its high yield and mild reaction conditions.59 In 2017, Levine's group performed the click reaction on substrates 32 and 33 to prepare macrocycle 34 with a high isolated yield of 71% (Scheme 10).52 The results from Levine's group revealed that macrocycle 34 has high sensing capabilities for polycyclic aromatic hydrocarbons (PAHs) and fluoride anions.
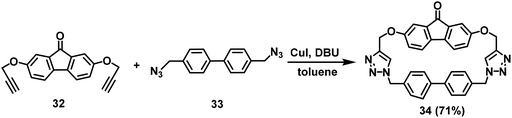 |
| Scheme 10 Synthesis of macrocycle 34via click reaction. | |
In addition to the compounds mentioned above, the synthesis of fluorene-based COFs and MOFs has also been well studied. As early as 2009, Zhang's group synthesized and characterized seven novel MOFs based on 9,9-dipropylfluorene-2,7-dicarboxylic acid.26c In 2016, Huang and Zhou's group prepared a series of fluorene-based MOFs to detect heavy metal ions in aqueous solutions.60 In 2017, Poriel's group successfully fabricated four chiral fluorene-based MOFs.26a Subsequently, in 2020, a series of novel Zn-based coordination MOFs synthesized from three spirodifluorene tetracarboxylate ligands were reported by Poriel's group.26b In addition, in 2017, Xu's group synthesized a fluorene-based COF,25 with improved electrical conductivity. The material offers considerable promise for the future applications of 2D COFs in the field of thermoelectricity.
3. 3,6-Linked fluorene-based macrocycles
The 3,6-linkage in fluorene-based π-conjugated compounds is another popular method for the construction of new compounds and polymers. The 3,6-substituents of fluorene synthons are expected to change the conformation of polyfluorenes, resulting in a greater nonlinear conformation compared to poly(9,9-dialkylfluorene-2,7-diyl).33c,61 Single crystalline structures of the cyclic tetrafluorenes connected at the 3,6-positions61 indicated that the 2,7-position39a,47a had a more curved configuration, resulting in higher strain energy than that of the 3,6-position linkages.
In 2008, the first 3,6-linked macrocycle 36 was developed by Hogen-Esch and coworkers via Yamamoto coupling using 3,6-dibromo-9,9-dihexyl-9H-fluorene 35 as the starting material (Scheme 11).61a In this reaction, a series of fluorene-based macrocyclic four- to thirteen-membered polymers was obtained, and the corresponding cyclic tetramer 36 was formed with up to 37% yield, as calculated from 1H NMR, under the optimal conditions.
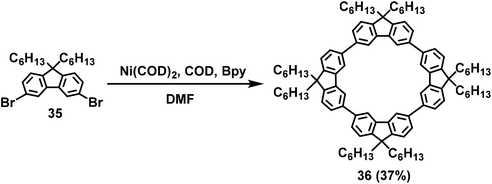 |
| Scheme 11 Polymerization of monomer 36via Yamamoto coupling reaction. | |
In 2010, Zhu and co-workers employed 9,9-dibutyl-9H-fluorene-3,6-dicarbaldehyde 37 as a precursor in the synthesis of the ethylene-bridged 3,6-substituted fluorene dimer 38 with a yield of 8.9% via the McMurry coupling reaction (Scheme 12).61b
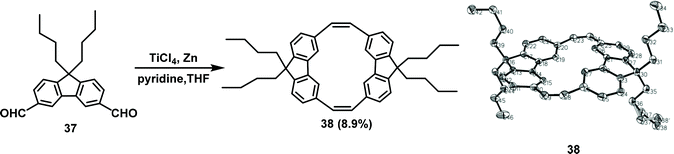 |
| Scheme 12 McMurry coupling reaction for synthesizing dimer 38 and the single crystal structure of 38. | |
Likewise, in 2017, via a Pd-catalyzed Stille coupling reaction, Wu et al. synthesized the acetylene-bridged fluorene-based macrocyclic trimer 41a, tetramer 41b, and pentamer 41c in 6%, 8%, and 4% yields, respectively, starting from 3,6-dibromofluorene 39 and 1,2-bis(tributylstannyl)ethyne 40 (Scheme 13).39b The resulting macrocyclic yield was less than 10%, but was comparable with that reported for ethylene-bridged 3,6-fluorene dimers obtained via the McMurry coupling reaction.
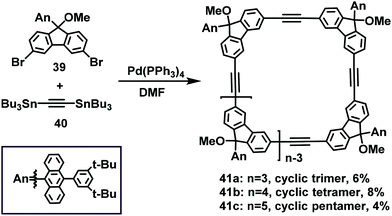 |
| Scheme 13 Stille coupling reaction for synthesizing fluorenyl macrocycles. | |
Similarly, Wu's group employed 3,6-dibromofluorene 42 and 3,6-diboronic acid ester 43 as substrates in the synthesis of uniform fluorene-based macrocycles. The cyclic tetramer 44a with the largest content in the Suzuki coupling reaction and the Yamamoto coupling reaction was formed with up to 20% and 32% yields, respectively (Scheme 14).39b Combined with previous work reported by Fomina et al.,61a their conclusions were that 3,6-linked fluorene synthons were more suitable for generating cyclic tetramers via the Yamamoto coupling reaction compared to 2,7-linked fluorene synthons, which are typically used to prepare linear polyfluorenes.62
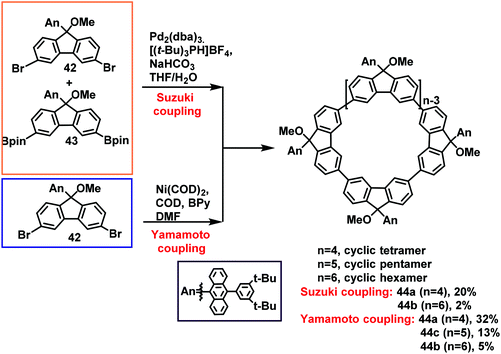 |
| Scheme 14 Two strategies for the synthesis of multiple macrocycles. | |
Although some progress has been made in the synthesis of 3,6-position fluorene-based macrocycles, the application of such compound types remains limited in organic electronics.
4. 9,9-Linked fluorene-based macrocycles
The 9,9-linkage of fluorenyl macrocycles represent a typical class of nonconjugated structures. This type of fluorene-based macrocycle attached at the 9,9-position belongs intrinsically to calix[n]arenes that are fabricated via two main methods, i.e., F–C reactions63 and self-assembly,64 which are described in the following sections.
4.1. Friedel–Crafts (F–C) reaction
In 2004, Lee et al. used the F–C reaction to synthesize a fluorene-based calixarene-like compound.65 In this paper, compound 46 was first obtained from fluorenone 45 and pyrrole with a yield of 45% under catalysis with trifluoromethanesulfonic acid (TFA). Then, compound 46 and acetone were employed as raw materials to synthesize macrocycle 47 with a yield of 31% via a BF3-mediated F–C reaction (Scheme 15).
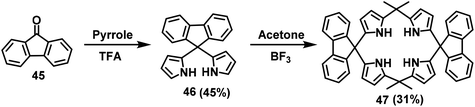 |
| Scheme 15 Synthesis of the fluorene-based macrocycle 47. | |
In 2007, Lu and coworkers took precursor 48 containing triphenylamine and oligofluorenes as raw materials to synthesize multi-H shaped macrocycles (TPAF3)3 and (TPAF5)3 in yields of up to 71% and 68%, respectively. The synthesis was performed in mesitylene solution via an F–C reaction catalyzed by methanesulfonic acid (Scheme 16).63 These two novel macrocycles were used as the hole-transporting emission layer for a pure blue light-emitting device. In 2014, Huang's group reported the first triphenylamine-fluorene host for exciplex-based highly efficient white phosphorescent OLEDs. A cyclic oligomer 50a with alternating fluorenyl and triphenylamine derivatives was fabricated with a yield of 80% by performing a F–C reaction with trifluoromethanesulfonic acid intervention on the 49a substrate (Scheme 17).66 In 2015, Ye's group synthesized substrate 49b by introducing diphenylphosphine oxygen, and prepared the macrocyclic compound 50b in a yield of up to 41.8% (Scheme 17).40 The resulting compounds exhibited excellent thermal and electrochemical properties and were efficient host materials for blue phosphorescent organic light-emitting diodes (OLEDs).
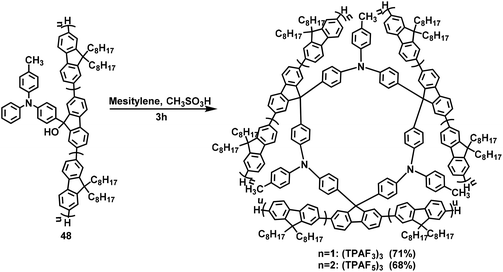 |
| Scheme 16 Synthetic route for the preparation of (TPAF3)3 and (TPAF5)3. | |
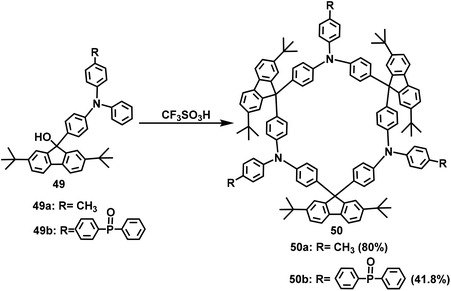 |
| Scheme 17 Synthesis of the fluorene-based oligomers 50a and 50b. | |
In addition to triphenylamine, carbazole and its derivatives were also used to prepare efficient materials suitable for OLEDs. In 2011, Huang's group used carbazole rather than triphenylamine to synthesize two macrocycles. (ECAF3)4 and (TCAF3)4 were prepared in yields of 38% and 42%, respectively, through a simple p-toluenesulfonic acid-mediated F–C reaction (Scheme 18).67 Both compounds exhibited good thermal stability and high luminous efficiency. The results also revealed that the introduction of carbazole improved the hole-injection capability without affecting the electron-injection ability. In 2017, a similar (Cz-F)4 macrocycle was synthesized with a yield of 50.1% via the F–C reaction starting from building block 52 (Scheme 18)68 and successfully applied to blue phosphorescent OLEDs.
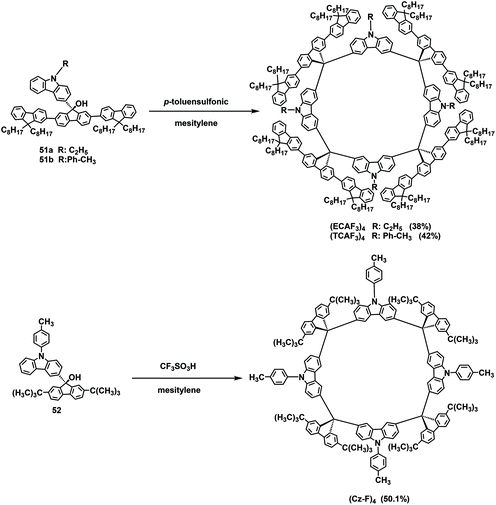 |
| Scheme 18 Synthesis of (ECAF3)4, (TCAF3)4 and (Cz-F)4. | |
4.2. Self-assembly
The current methods for the synthesis of fluorene-based macrocycles use templates or reactions in dilute solutions, or involve complex steps. However, these factors can be overcome when a simple self-assembly method is employed. As early as 1969, a tetrameric compound obtained via self-assembly and was accidentally discovered by Wittig and coworkers.69 In 1998, Ipaktschi provided the first confirmation of the existence of tetramers through their single-crystal structures. During the synthesis process, dibrominated compounds 53 were used as the starting materials for the synthesis of cyclic tetramers 54 with different alkyl chains at yields of approximately 97% (Scheme 19).70
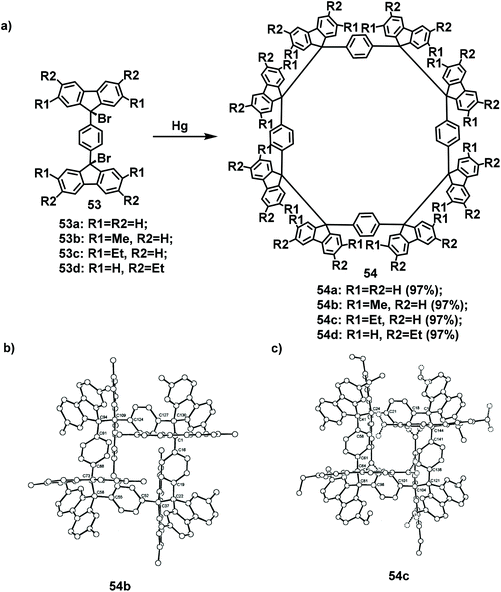 |
| Scheme 19 (a) Synthetic route to cyclic tetramers 54. (b) X-ray crystal structure of 54b. (c) X-ray crystal structure of 54c. | |
In 1999, Ipaktschi used this method to construct highly branched systems and multifunctional metallocenes (Scheme 20).64 In this study, the diol compound 55a was reduced with a SnCl2/HCl solution in the solvent of THF resulting in tetramer 56a with a yield of 82%. Macrocycle 56b was fabricated in benzene solution with the dichlorinated compound 55b in the presence of Sncl2 with a yield of 63%. These results indicated that the substituents with large steric hindrance had no effect on the desired tetramerization process. Furthermore, the spontaneous tetramerization process was mainly driven by the four weak Csp3–Csp3 bonds of the fluorenes and the gauche conformation of the difluorenyl units.
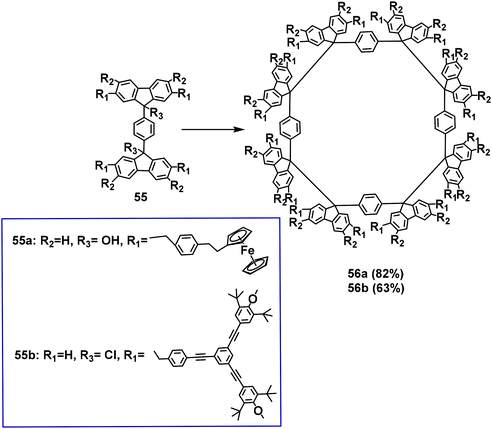 |
| Scheme 20 Synthesis schemes of cyclic tetramers 56a and 56b. | |
In 2000, Ipaktschi investigated the influence of substituents and conjugation effects on the size of macrocycles (Scheme 21).71 Subsequently, a series of tetramers 58 with different substituents was synthesized with high yields of 96%–98% when compounds 57 were used as the substrates under treatment with mercury. The hex-1-ynyl group attached to the 3- and 6-positions of the fluorene was evaluated to explore the conjugative influence of the substituent on the tetramerization. The tetramer 60 was obtained with a yield of 25%. In summary, the results confirmed that neither the steric hindrance of the substituents nor the conjugate length influenced the tetramerization process.
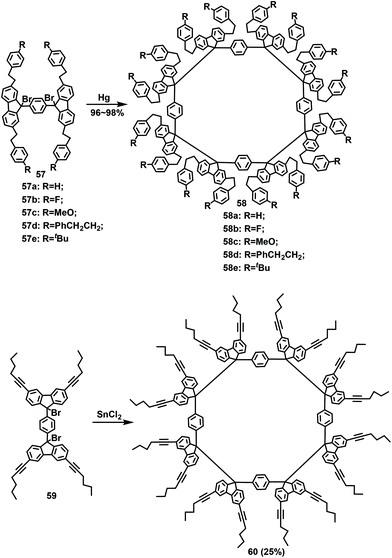 |
| Scheme 21 Two synthetic methods of cyclic tetramers. | |
In 2016, Wuest and coworkers used spirodifluorene rather than benzene to prepare macrocycles (Scheme 22).72 In the initial stage of the process, the dibrominated precursor 61a was used as the raw material to fabricate the then-largest XRD-verifiable macrocycle 63 with a yield of 35%. Subsequently, to increase the solubility of the final products, tert-butyl groups were added and an unexpected macrocycle 64 was prepared with up to 57% yield. This suggests that with no substituents on the fluorene, the special arrangement of intramolecular C–H⋯π interactions favors the synthesis of hexamers, whereas the presence of the tert-butyl group hinders the intermolecular interactions, resulting in tetramers.
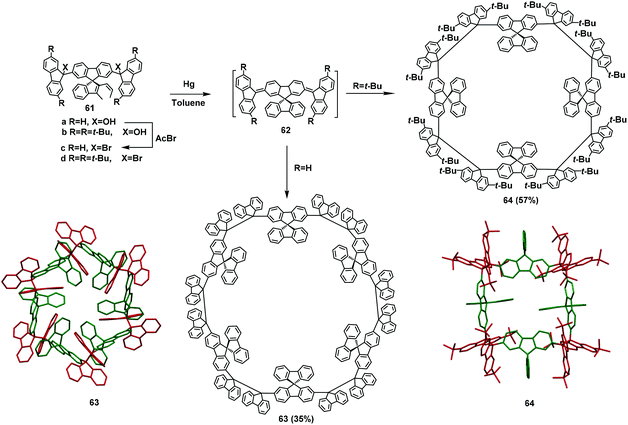 |
| Scheme 22 Synthesis of macrocyclic oligomers 63 and 64 and their single crystal structures. | |
In summary, self-assembly is a simple method that can help synthesize 9,9-linked fluorene-based macrocycles in high yields. The factors affecting the size of the macrocycles have been investigated, but a large gap in the synthesis principle of this method persists.
5. 2,9-Linked fluorene-based nanogrids
The 2,9-linkage of fluorenes is poorly studied compared with other linkages owing to the failure observation of their unique nature of nanogrids, besides the limited synthetic methodologies available. However, this linkage mode exhibits potential advantages, such as fused fixation for more rigidity and site-selectivity for extension owing to the guiding role of the 7-position. As a result, the preparation of gridarenes was achieved. Gridarenes are a new class of non-conjugated cycle-like structures with well-defined edges and vertices serving as the inter-connectable sites. Organic nanogrids connected at the 2,9-position of fluorene exhibit low reorganization energy73 and their polygrids exhibit adjustable persistence length of the chain in polygrids.5a Their extendability, installability, programmability, and scalability,5a allow the construction of nanoarchitectures with the potential applications in solar cells, memory devices,36 and OLEDs.
Inspired by the grid complex developed by Lehn and colleagues in 1994,74 our group proposed the gridization of organic semiconductors and focused on the design and synthesis of nanogridarenes linked by covalent bonds. In the beginning of the process, a series of rigid H-shaped nanoscale molecules was designed and synthesized. Further study of such structures revealed that rigid molecules with fixed shapes have significant potential for use as active components in storage devices and photodetectors.75 Hence, to enhance the rigidity of such compounds, diverse types of nanogridarenes were designed and syntheized with the 2,9-linkage modes. Until now, six types of nanogrids have been created according to various Chinese symbols and geometric shapes,5b including ladder-type, windmill-type, angle-lost-type, tic-tac-toe-type, strain-type and three dimension-type gridarenes (Scheme 23).
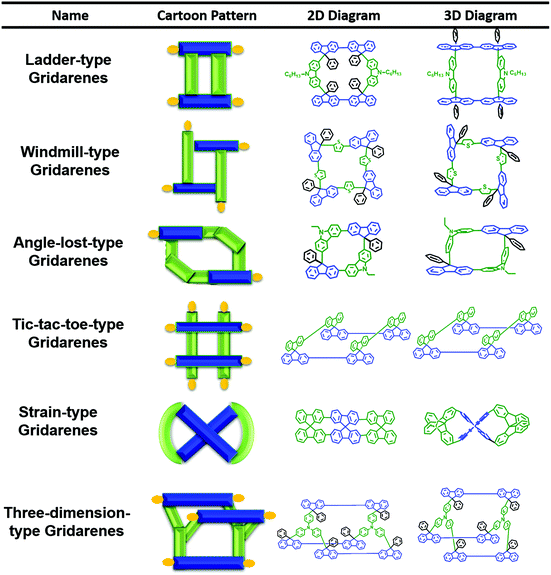 |
| Scheme 23 Six types of gridarenes. From left to right, cartoon patterns, two-dimensional (2D), and three-dimensional (3D) diagrams. | |
Currently, some methods have been reported for the preparation of fluorene-based nanogrids, including coupling reactions51a and template methods.27 Compared with these reactions and methods, the commonly used BF3·Et2O-mediated F–C reaction presented in our work has many benefits, such as simple steps, mild conditions, high-efficiency, and metal-free features.28,76 The synthesis of different types of gridarenes via F–C gridization is described in the following sections.
5.1. Ladder-type gridarenes
Ladder-type gridarenes have four reaction sites extending in two opposite directions toward the same plane, leading to a one-dimensional expansion for the installation of ladder-type nanopolymers. In 2017, our research group performed a retrosynthetic analysis on ladder-type gridarenes, and designed three synthesis routes involving I-type, L-type (A1B1-type building blocks), and U-type synthons. The three routes were studied and compared, and the results indicated that the L-type building block 65 could directly generate the ladder-type gridarene 66 in 58% yield using F–C reaction (Scheme 24).77 However, ladder-type gridarenes contain a fluorenyl group with a chiral carbon at the 9-position, based on density functional theory calculations, resulting in more than twelve stereoisomers. The low yield of 66 complicated the separation of the corresponding isomers.
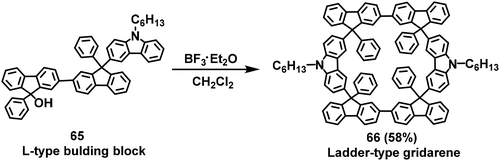 |
| Scheme 24 Synthesis of a ladder-type gridarene via F–C reaction. | |
After that, a stepwise synthesis route was designed for the synthesis of ladder-type gridarenes with higher yields than previously reported.78 The synthesis process involved the reaction of the synthesized substrate 67 with substrate 68, leading to the formation of the building block 69 with a yield of 50% via a Suzuki coupling reaction. Subsequently, the ladder-type gridarene DFCz was synthesized at 95% yield via F–C reaction (Scheme 25). In this paper, five relatively stable stereoisomers of ladder-type gridarenes are listed (Scheme 25), and some of the gridarenes were separated and characterized by 1H NMR spectroscopy. The results revealed that a highly symmetrical configuration a could be obtained with a yield of 10% and the configuration c with 20% yield, and a mixture of the remaining three conformations was obtained with a yield of 65%. This work provided a solid foundation for the subsequent separation of isomers.
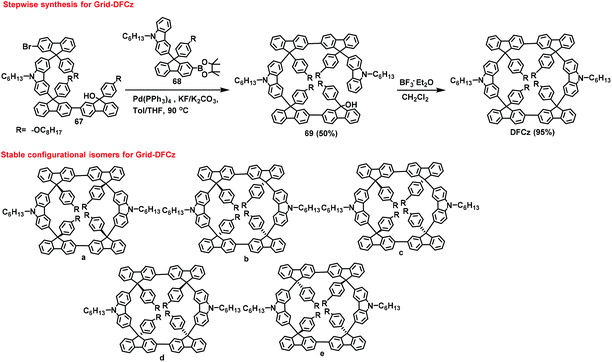 |
| Scheme 25 Stepwise synthesis route and stable configurations of DFCz. | |
In 2019, our group succeeded in combining fluorene and thiophene to create a new series of donor–acceptor gridarenes for use in memristors (Scheme 26).36 Similarly, the fluorene-based L-type synthon 70 containing benzothiadiazole (BT) as the crossbeam and thiophene as the π-bridge was initially acquired, with F–C self-condensation subsequently used to synthesize the D–A type gridarene Grid-BT. Three D–A gridarenes were prepared with yields of 15%, 26%, and 33% for G-Th, G-DTh, and G-TTh, respectively, by introducing different π-bridge motifs (thiophene, dithiophene, and trithiophene, respectively). The gridarene G-DTh doped with tetrabutylammonium hexafluorophosphate (TBAPF6) two-terminal memristors exhibited excellent characteristics, such as mimic learning processes, synaptic potentiation, and depression plasticity. This research expanded the applications of gridarenes, and provided a route for the synthesis of D–A type gridarenes with tailored electrical and optical properties.
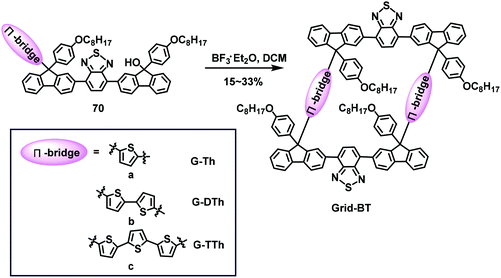 |
| Scheme 26 Synthesis of D–A-type gridarenes via F–C reaction. | |
In 2014, our group used bithiophene 72 as a holder for reaction with the H-type precursors 71 with the aim of reducing the molecular structure rotation. A novel stereoscopic macrocyclic “日” shaped nanostructure, GF, which is equivalent to the assembly of two ladder-type gridarenes, was successfully constructed (Scheme 27).18 In this work, the impact of the alkyl chain length on the yield of the final products was investigated, and 4-(octyloxy)phenyl-containing groups contributed to 26% of the yield. This suggested that the distance between tertiary alcohols will reduce the probability of intermolecular reactions as the length of the imported alkyl chain increases, thereby increasing the yield. In short, a new range of soluble nanomonomers was successfully prepared. The monomer reaction sites at the apex are favorable for establishing complex three-dimensional structures, which can provide new ideas for the design and synthesis of novel nanostructures.
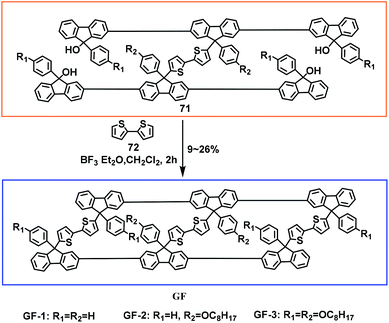 |
| Scheme 27 Synthetic routes to fluorene-based gridarene GF. | |
In 2017, to demonstrate the extendibility of organic nanogrids, an interrupted conjugated one-dimensional nanopolygrid was designed starting from the brominated L-type synthon 73 was designed, and F–C self-condensation was used to prepare the ladder-type nanogridarene 74 (Scheme 28).19a The nanogrid monomers 74 were further transformed into one-dimensional nonconjugated nanopolymers (polygrids) through the general Yamamoto polymerization. The excellent optical properties of the nanopolymers suggest their potential for applications for optoelectronic devices. These results have enabled the design of grid-based nanopolymers.
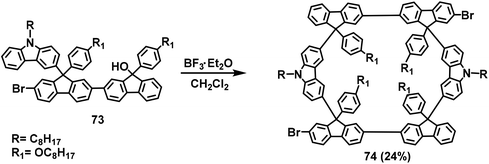 |
| Scheme 28 Synthesis of gridarene nanomonomer 74. | |
In 2019, based on previous work, a new fully conjugated nanopolymer was prepared with the aim of improving the properties and stability of these compounds. Conjugated ladder-type polymers were constructed by coupling reactions to two reaction sites on the same side. In this contribution, the Suzuki coupling reaction was used to prepare precursors 78 and 79 with yields of 57% and 40%, respectively. The brominated gridarene G-C8 was then obtained via the F–C reaction with a yield of 45% (Scheme 29);19b then, conjugated nanopolymers were prepared via the Yamamoto coupling reaction. Compared with traditional linear polyfluorenone (PFO) and polyfluorene (PH), which have large steric hindrance, the nanopolymers had greater thermal, spectral, and morphological stability. This indicates that gridarene-based nanopolymers may become the starting point for next-generation polymeric materials.
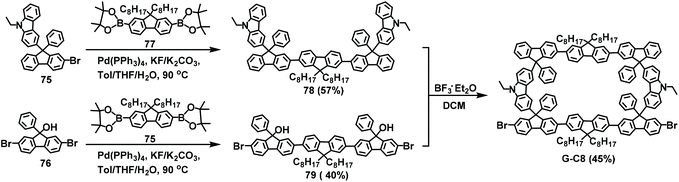 |
| Scheme 29 Synthetic route to gridarene nanomonomer G-C8. | |
5.2. Windmill-type gridarenes
In 2018, inspired by ancient Chinese windows, breakthroughs were made in the preparation of windmill-type gridarenes with the help of multiple controllable reaction sites on the fluorene and thiophene.28 As potential candidates for constructing complex nanostructures, windmill-type gridarenes differ significantly from ladder-type nanogrids in both their geometry and expandable reactive sites. These gridarenes consist of four reaction sites extending in four directions in the same plane, which can then be used to construct complex one-dimensional or two-dimensional polygrids. In this work, the synthesis of windmill-type gridarenes using I-type synthon 80 or L-type synthon 81 as substrates was investigated (Scheme 30). The experimental results revealed that when the I-type building block was used as a raw material under catalysis with BF3·Et2O, triangular gridarene (WG3), square windmill-like gridarene (WG4), and pentagonal gridarene (WG5) were obtained at 36%, 18%, and 10% yields, respectively. However, when the L-type building block was used as a starting compound, the desired WG4 was isolated at a yield of 53%. This resulted from the fact that the L-type synthon was more selective than the I-type synthon and tended to synthesize windmill-type gridarenes with four vertical angles at a higher yield.
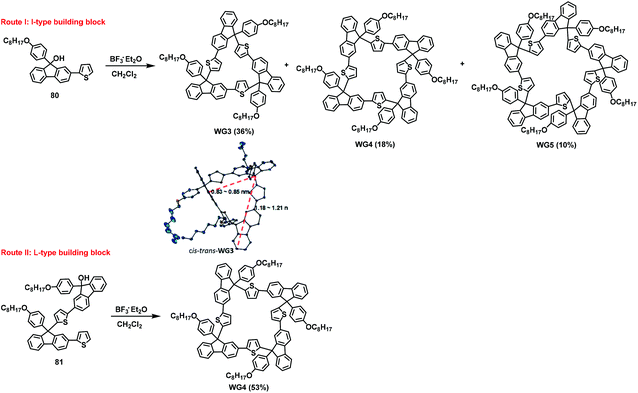 |
| Scheme 30 Different sizes of gridarenes were synthesized using I-type and L-type synthons and X-ray crystal structure of cis–trans-WG3. | |
Different from the edge-on-type nanogrids, planar windmill-type gridarenes are also key nanounits. The main differences between edge-on windmill-type and planar windmill-type gridarenes mainly depend on the connection modes of the 9-positions of the fluorene. It is obvious that the 9-positions of the fluorene of windmill-type gridarenes have Csp3 connection, while those of planar windmill-type gridarenes have Csp2 connection. In general, the 2,9-position linkage makes it difficult to obtain such planar gridarenes. However, by changing Csp3 into Csp2 at the 9-position of fluorenes, a type of planar windmill-type gridarene was designed. In 2018, through a base-catalyzed self-condensation reaction, Amaya and colleagues prepared macrocycles using substrate 82 and investigated the photochemical behavior of the macrocycle isomers (Scheme 31).79 In this reaction, isomers EZEZ and ZZZZ with a total yield of 11% were obtained under light conditions, whereas only EZEZ, with a yield of 10%, was acquired under dark conditions. Density functional theory (DFT) calculations revealed that the strain energy was the lowest for EZEZ and ZZZZ; hence, these isomers were easily obtained. This research group further investigated the transformation between the two isomers, and determined that the conversion rate from EZEZ to ZZZZ reached up to 90%, as indicated by 1H NMR. Nevertheless, ZZZZ was only 20% isomerized to EZEZ under a high-pressure Hg lamp, mainly because the strain energy of the EZEZ isomer was greater than that of the ZZZZ isomer. Similarly, the results revealed that the transition from the ZZZZ isomer to the EZEZ isomer would generate the intermediate EZZZ, which would be selectively transformed into the more stable EZEZ and ZZZZ isomers under light conditions. However, the ZZZZ isomer could only be obtained under the action of heat, confirming that the EZEZ isomer was a dynamic product. A light-induced reversible transformation between isomers results in a considerable change in the molecular configuration, although the determination of the properties requires further study.
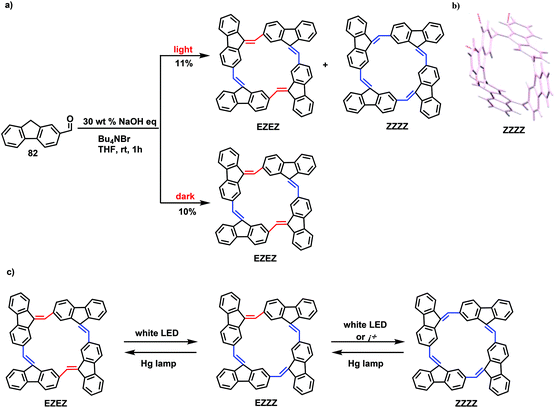 |
| Scheme 31 (a) Synthetic route to EZEZ and ZZZZ. (b) X-ray crystal structure of ZZZZ. (c) Scheme for the isomerization of EZEZ and ZZZZ. | |
5.3. Angle-lost-type gridarenes
Angle-lost-type gridarenes, also referred to as drawing hands gridarenes (DHGs), resemble a square compound lacking two angles and have potential covalent multibonds for nanolinkages. Many studies have focused on the synthesis of gridarenes, but it is quite difficult to control the stereoisomerism of these structures. Hence, DHGs were synthesized starting from mono-crescent-shaped synthons 83, and the effects of supramolecular attraction–repulsion interactions on the stereoselectivity of nanogridarenes (Scheme 32) were verified.5a Based on the prototype reaction, a methoxy-containing crescent-shaped synthon was used as the substrate, and catalytic CF3SO3H and additive CF3COOH were used to drive the reaction. A 1 min reaction at room temperature realized DHG yields of 77%, and first-ever transient control over the kinetic product meso-DHGs with a diastereomeric excess (de) of up to 75.6% was achieved. Therefore, the author investigated the time dependence of the reaction pattern, and found that the yield and de value decreased gradually as the reaction time increased, dropping to 36.5% and 19.0% at 54 h. To clarify the meso-selectivity of substituents in different locations, the methoxy group was replaced with a methyl group, thereby reducing the de value, which confirmed that the oxygen in the methoxy group was important for the reaction. Changing the R2 groups revealed that the substituents could affect the DHG yield, to a certain extent, without destroying the meso-selectivity. To verify the applicability of meso-selectivity, new dicrescent-shaped monomers DCs were successfully designed and then subjected to polygridization. The results revealed that rapid termination and quenching could effectively inhibit the formation of insoluble crosslinked polymers. The polygridization of PDHGs from the monomer DC-F provided 60% yield and 79.5% de of meso-selectivity for the reaction times of 6–25 s, and DC-F monomer concentrations of 15–60 mM. This was achieved without the formation of insoluble crosslinked polymers. However, inevitably, replacing DC-F with DC-SFX generated insoluble crosslinked polymers with a yield of only 30%.
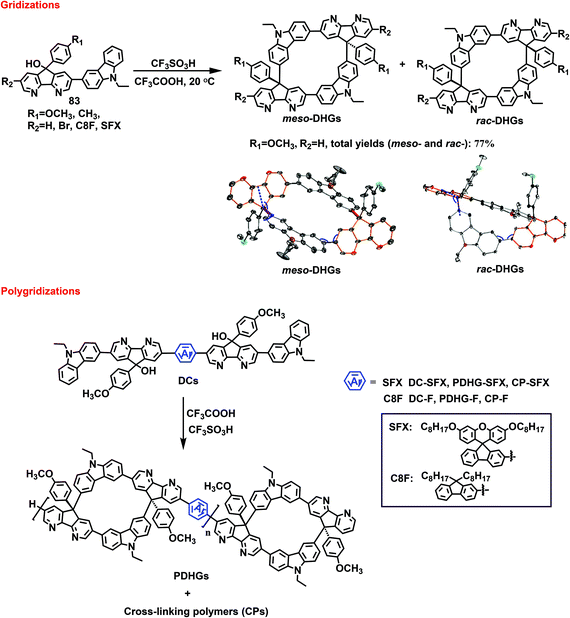 |
| Scheme 32 Synthetic routes to DHGs and PDHGs, and single crystal structures of meso-DHGs and rac-DHGs. | |
5.4. Tic-tac-toe-type gridarenes
The tic-tac-toe–type gridarenes were synthesized from spirobifluorene with eight active substitution sites, and can be extended in four directions to achieve three-dimensional expansion. In 2017, Alonso-Gomez et al. presented that the introduction of axially chiral spiranic building blocks into molecules can increase their chiroptical responses, thereby improving their chiroptical application of them.80 In this study, after enantiomeric separation, spirodifluorene (P)-3 was employed as starting materials to render diethynylspirane (P)-4 in 73% yield via Sonogashira coupling reaction. Subsequent treatment of (P)-4 with NaOH in toluene afforded monodeprotected (P)-5 in 80%. (P)-5 was subjected to Glaser coupling reaction to give dimer (P2)-6 in 74% yield, which then reacted with NaOH in toluene affording the deprotected dimer (P2)-7 with a yield of 82%. Finally, the desired tetrameric macrocycle (P4)-2 in 25% yield and the dimeric macrocycle (P2)-1 in 5% yield was obtained via Glaser coupling reaction (Scheme 33). Analysis of the Hausdorff chirality measure (HCM) indicated that macrocycles (P2)-1 and (P4)-2 possessing high indexes of chirality, which laid a strong foundation for chiroptical application. Afterwards, in 2020, they successfully synthesized trimeric macrocycles other than dimers and tetramers by changing the reaction conditions.81 In this paper, Sonogashira coupling reaction was carried out on rac-2 to obtain rac-3-TMS in 83% yield, and subsequent trimethylsilyl deprotection afforded rac-3 in 75% yield. Finally, the desired trimeric macrocycle was prepared in 37% yield as a stereomeric mixture in the presence of catalyst Pd(PPh3)2Cl2 and CuI (Scheme 34). And the structures of compounds (P,P,P)/(M,M,M)-1 were confirmed via single crystal structures. Similarly, the developed macrocycles showed remarkable photophysical and chiroptical responses.
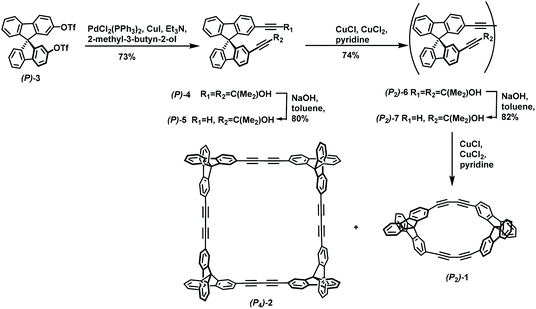 |
| Scheme 33 The reaction routes for the synthesis of (P4)-2 and (P2)-1. | |
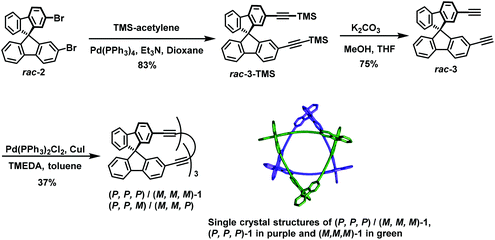 |
| Scheme 34 The reaction routes for the synthesis of a macrocycle and the single crystal structure of (P,P,P)/(M,M,M)-1. | |
In 2019, our group synthesized a series of tic-tac-toe–type gridarenes with different sizes through Yamamoto coupling reaction, which only contained spirodifluorene monomer. The experimental results revealed that trimer [3]GSBF, tetramer [4]GSBF, and pentamer [5]GSBF were synthesized at 30%, 25%, and 4% yield, respectively (Scheme 35).82
 |
| Scheme 35 Synthetic routes to GSBFs. | |
Subsequently, in 2020, Amaya and co-workers performed a Yamamoto coupling reaction on chiral spirodifluorene building blocks to prepare chiral cyclic spirodifluorenylenes 2-[n]. The results revealed that the total yieds of 2-[3], 2-[4], and 2-[5] for the R-isomers were 40%, at the ratio of 1.0, 0.91, and 0.38, and the S-isomers of 2-[n] were obtained at a total yield of 34%, at the ratio of 1.0, 0.80, and 0.35 (Scheme 36).83 In this paper, the S-isomers of 2-[n] were demonstrated by single crystal diffraction. Combined with our previous work on the synthesis of tic-tac-toe–type gridarenes.79 it is clear that the chirality of trimer to pentamer products is inseparable from the chirality of the spirodifluorene substrates.
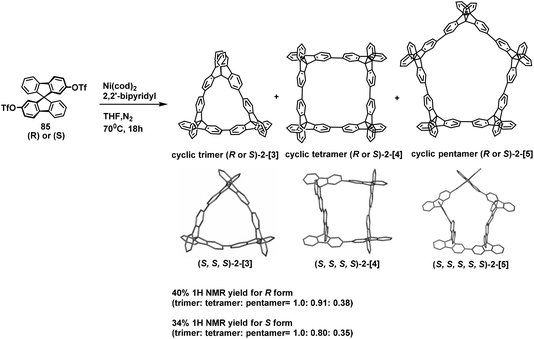 |
| Scheme 36 Synthesis of chiral cyclic [n]spirodifluorenylenes 2-[n] and single crystal structures of 2-[n]. | |
In 2019, Ohe's group carried out Sonogashira coupling reaction twice in succession and successfully synthesized the conjugated double helicates macrocycle (s,s)-1-SBF.84 During this reaction, substrates (s)-3 and A were subjected to Sonogashira coupling reaction to prepare (s,s)-4 in 67% yield, and subsequent deprotection to give (s,s)-5 completely. (s,s)-2 was afforded in 17% yield by implementing Sonogashira coupling reaction on (s,s)-5 and A. The final product (s,s)-1-SBF was rendered with a yield of 62% via reductive aromatization (Scheme 37).
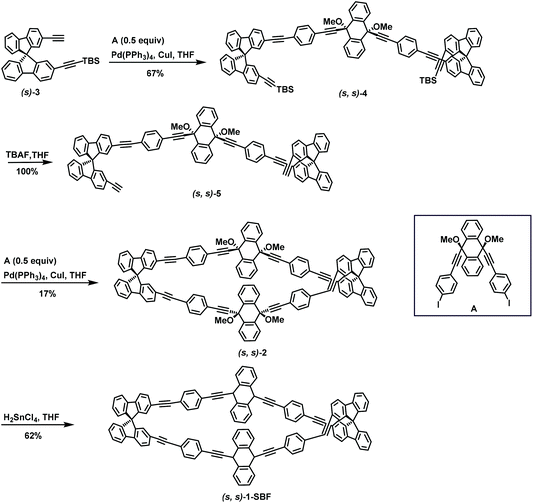 |
| Scheme 37 Sonogashira coupling reaction for the synthesis of (s,s)-1-SBF. | |
5.5. Strain-type gridarenes
Strained gridarenes, as a hybrid paradigm for strained macrocycles and grids, afford state-of-the-art models and versatile nanobricks for advanced materials. In 2019, Amaya's group designed and synthesized novel strained gridarenes containing three spirodifluorenes (Scheme 38). Route I revealed that the desired macrocycle (R,P,R/S,M,S)-1 was afforded in 5% yield when substrates (R/S)-2 and 86 were utilized via SPhos-Pd-mediated Suzuki–Miyaura cross-coupling. In Route II, when (R)-5 or (S)-5 was used to react with 2,2′,7,7′-tetrabromo-9,9′-spirodifluorene 87, the yield of macrocycle (R,P,R/S,M,S)-1 was increased to 15% under the same reaction conditions.85 In the same year, Cong's group reported a strained gridarene with a figure-of-eight geometry containing cyclic paraphenylene and pentiptycene via using photochemical synthesis.86 Subsequently, Stępień et al. used 9,9′-bicarbazole as a scaffold for the synthesis of a novel strained gridarene.87 Similarly, in 2020, Jasti's group fabricated a series of spirodifluorene-based strained gridarenes of different sizes (Scheme 39).88 They first used substrate 88a to react with 2,2′,7,7′-tetrabromo-9,9′-spirodifluorene 89 to obtain product 90a at a remarkable 55% yield. Then, strained macrocycle 91a was fabricated with a yield of 45% by implementing the deprotection and reductive aromatization of compound 90a. Larger macrocycles 90b and 90c were obtained at yields of 30% and 35%, respectively, when the same method was used for the 8-ring 88b and 10-ring 88c substrates, with strained macrocycles 91b and 91c obtained at yields of 39% and 37%, respectively. They also prepared the strained macrocycle 91d containing bipyridyls with a yield of 32% to explore the effect of subtle adjustments in raw materials.
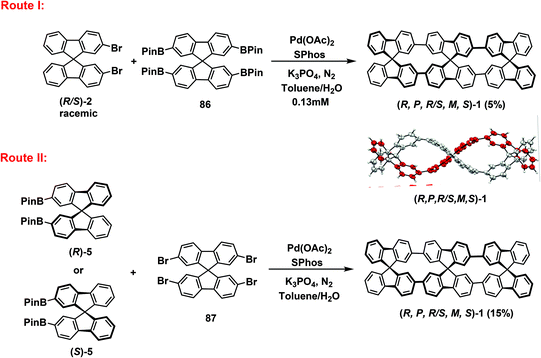 |
| Scheme 38 Synthetic route to (R,P,R/S,M,S)-1 and the single crystal structure of (R,P,R/S,M,S)-1. | |
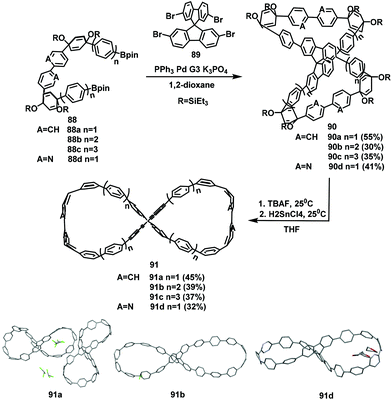 |
| Scheme 39 Synthetic route to strained gridarenes 91 and single crystal structures of 91a, 91b, 91d. | |
5.6. Three dimension (3D)-type gridarenes
Up to now, although the study of fluorene-based macrocycles and nanogrids has been fairly constant, there are only a few examples of 3D cages based on fluorenes. In 2008, Pei's group designed and synthesized a series of novel fluorene-based 3D nanocages. First, the preparation of nanocages 94 from aldehydes 92 and amines 93 was carried out in a mixture of 1,1,2,2-tetrachloroethane (TCE) and dichloromethane (DCM). Subsequently, a reduction reaction was applied to substrates 93 to produce the final products 94 at yields of 67%–72% (Scheme 40).89
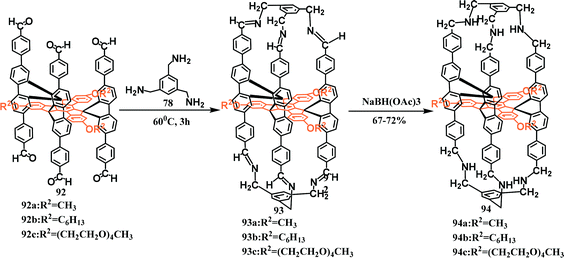 |
| Scheme 40 Synthesis of fluorene-based 3D-cages. | |
However, the architecture of the 3D cage is such that connections are difficult. The 2,9-linkage of fluorene can be designed into a 3D grid for cages by the A2 + B3 type reaction. In 2019, triphenylamine (TPA) was deemed to be a B3-type unit and was used to replace the commonly used electron-rich B2-type building blocks. In this work, the TPA was reacted with difluorene ditertiary alcohols to construct a class of novel gridarenes with a three-dimensional structure (Scheme 41).90 The obtained products have many configurations owing to the chirality of the ditertiary alcohol. Hence, an understanding of the influence of the substrate configuration (mix-DFOH, meso-DFOH, and rac-DFOH) is necessary. The results indicated that, compared with the other two configurations, rac-DFOH was more conducive to cyclization processes that generate 3D-grid-FTPA with a high yield of 55.1%. meso-DFOH is quite suitable for the generation of oligomers, as 31.4% of the target product can be prepared, and mix-DFOH generated a 40.8% product yield. These findings provide a solid foundation for further research.
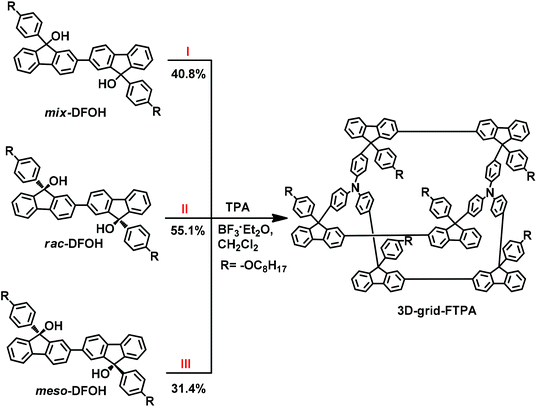 |
| Scheme 41 Three synthetic routes for synthesizing 3D-grid-FTPA. | |
Some progresses have been made in the preparation and characterization of 3D nanogridarenes. Future work will continuously include the ongoing study of the development and application of 3D gridarenes.
6. Conclusion and outlook
In a word, notable developments in the synthesis of fluorene-based macrocyclic structures have been summarized. Fluorene-2,7-diyl cyclic structures have been studied extensively owing to their full conjugation features for the design of organic semiconductors and strained features as the substructures of carbon nanotube. Such 2,7-linked macrocycles have great potential in the application of chiroptical responses and ionic recognition. The 3,6-linked fluorene-based macrocycles become another usefully optoelectronic materials with face-on features that exhibit the potential design space with other linkage modes. The 9,9-linkage of fluorenyl macrocycles presents a type of nonconjugated calixarene-type structure. The macrocycles obtained by incorporating the 9-positions of fluorene with triphenylamine or carbazole have practical applications in OLEDs. The macrocycle-like nanogrids, also referred to as gridarenes, are an evolving class of organic nanostructures with well-defined edges and vertices by means of the unique 2,9-linkage modes, and exhibit potential extendability, installability, programmability, and scalability. Inspired by Chinese symbols and window lattices, the synthesis of ladder-type, windmill-type, angle-lost-type, tic-tac-toe-type, strain-type and three dimension-type gridarenes based on F–C reaction has been achieved. Owing to the presence of multiple reaction sites, these compounds can be further constructed into polygridarenes, COFs, and MOFs. Moreover, owing to their excellent optoelectronic properties, these nanogrids exhibit significant promise for the application of optoelectronic materials. In conclusion, fluorenes serve as a paradigm for providing diverse nanosynthons that have great potential to revolutionize molecular synthesis and help society to develop molecular and organic intelligence.
Conflicts of interest
There are no conflicts to declare.
Acknowledgements
This work was supported by the National Natural Science Foundation of China (21774061 and 2207112), Natural Science Research Project of Universities in Jiangsu Province (20KJB150038), National Key Laboratory (2009DS690095), Natural Science Foundation major research program integration project (91833306) and Postgraduate Research & Practice Innovation Program of Jiangsu Province (KYCX21_0701), National Natural Science Foundatio n major res earch program integratio n project (Grant Num ber 91833306), The Open Project from State Key Laboratory of Supramolecular Structure and Materials at Jilin University (No. sklssm202108).
References
- Q. H. Guo, L. Zhao and M. X. Wang, Chem. – Eur. J., 2016, 22, 6947 CrossRef CAS PubMed
.
- F. Huang and L. Isaacs, Acc. Chem. Res., 2014, 47, 1923 CrossRef CAS PubMed
.
- J. Shang, Y. Liu and T. Z. Pan, Front. Chem., 2021, 9, 635315 CrossRef CAS
.
- S. P. Wang, Y. F. Shen, B. Y. Zhu, J. Wu and S. J. Li, Chem. Commun., 2016, 52, 10205 RSC
.
-
(a) D. Q. Lin, Y. Wei, A. Z. Peng, H. Zhang, C. X. Zhong, D. Lu, H. Zhang, X. P. Zheng, L. Yang, Q. Y. Feng, L. H. Xie and W. Huang, Nat. Commun., 2020, 11, 1756 CrossRef CAS
;
(b) X. M. Xie, Y. Wei, D. Q. Lin, C. X. Zhong, L. H. Xie and W. Huang, Chin. J. Chem., 2020, 38, 103 CrossRef CAS
.
-
(a) J. Song, Y. L. Li, W. Sun, C. L. Yi, H. Wu, H. T. Wang, K. R. Ding, K. Xiao and C. Liu, New J. Chem., 2016, 40, 9030 RSC
;
(b) J. Song, W. Sun, Y. L. Li, F. L. Wei, C. Liu, Y. Qian and S. F. Chen, Chem. – Asian J., 2016, 11, 211 CrossRef CAS
.
- R. P. Kaiser, I. Caivano and M. Kotora, Tetrahedron, 2019, 75, 2981 CrossRef CAS
.
- K. Kawasumi, Q. Y. Zhang, Y. Segawa, L. T. Scott and K. Itami, Nat. Chem., 2013, 5, 739 CrossRef CAS PubMed
.
- D. Dunn, G. Hostetler, M. Iqbal, V. R. Marcy, Y. G. Lin, B. Jones, L. D. Aimone, J. Gruner, M. A. Ator, E. R. Bacon and S. Chatterjee, Bioorg. Med. Chem. Lett., 2012, 22, 3751 CrossRef CAS PubMed
.
- J. C. M. Kistemaker, P. Stacko, J. Visser and B. L. Feringa, Nat. Chem., 2015, 7, 890 CrossRef CAS PubMed
.
- L. H. Xie, C. R. Yin, W. Y. Lai and Q. L. Fan, Prog. Polym. Sci., 2012, 37, 1192 CrossRef CAS
.
-
(a) D. W. H. MacDowell and A. T. Jeffries, J. Org. Chem., 1970, 35, 871 CrossRef CAS
;
(b) T. M. Kadayat, C. Park, K. Y. Jun, T. B. T. Magar, G. Bist, H. Y. Yoo, Y. Kwon and E. S. Lee, Bioorg. Med. Chem., 2015, 23, 160 CrossRef PubMed
.
- J. Druey and P. Schmidt, Helv. Chim. Acta, 1950, 33, 1080 CrossRef CAS
.
- A. Kraak, A. K. Wiersema, P. Jordens and H. Wynberg, Tetrahedron, 1968, 24, 3381 CrossRef CAS
.
- D. Thirion, C. Poriel, J. Rault-Berthelot, F. Barriere and O. Jeannin, Chem. – Eur. J., 2010, 16, 13646 CrossRef CAS
.
- H. Zhang, X. Liu, T. T. Lu, P. Lv and W. Y. Lai, Chem. – Eur. J., 2019, 25, 3909 CrossRef CAS PubMed
.
-
(a) J. Raultberthelot and J. Simonet, J. Electroanal. Chem., 1985, 182, 187 CrossRef CAS
;
(b) C. Poriel, Y. Ferrand, P. Le Maux, C. Paul-Roth, G. Simonneaux and J. Rault-Berthelot, J. Electroanal. Chem., 2005, 583, 92 CrossRef CAS
;
(c) C. Poriel, Y. Ferrand, P. Le Maux, J. Rault-Berthelot and G. Simonneaux, Inorg. Chem., 2004, 43, 5086 CrossRef CAS
.
- L. Wang, G. W. Zhang, C. J. Ou, L. H. Xie, J. Y. Lin, Y. Y. Liu and W. Huang, Org. Lett., 2014, 16, 1748 CrossRef CAS PubMed
.
-
(a) Q. Y. Feng, Y. L. Han, M. N. Yu, B. Li, Y. Wei, L. H. Xie and W. Huang, Chin. J. Poly. Sci., 2017, 35, 87 CrossRef CAS
;
(b) Q. Y. Feng, S. L. Xie, K. S. Tan, X. J. Zheng, Z. T. Yu, L. J. Li, B. Liu, B. Li, M. N. Yu, Y. Yu, X. W. Zhang, L. H. Xie and W. Huang, ACS Appl. Polym. Mater., 2019, 1, 2441 CrossRef CAS
.
- K. T. Wong, R. T. Chen, F. C. Fang, C. C. Wu and Y. T. Lin, Org. Lett., 2005, 7, 1979 CrossRef CAS PubMed
.
- L. H. Xie, J. Liang, J. Song, C. R. Yin and W. Huang, Curr. Org. Chem., 2010, 14, 2169 CrossRef CAS
.
-
(a) L. J. Feng, M. J. Zhong, S. Z. Zhang, M. Wang, Z. Y. Sun and Q. Chen, Des. Monomers Polym., 2019, 22, 150 CrossRef CAS PubMed
;
(b) L. H. Xie, S. H. Yang, J. Y. Lin, M. D. Yi and W. Huang, Philos. Trans. R. Soc., A, 2013, 371, 20120337 CrossRef
;
(c) R. Abbel, A. P. H. J. Schenning and E. W. Meijer, J. Polym. Sci., Part A: Polym. Chem., 2009, 47, 4215 CrossRef CAS
.
- M. Fukuda, K. Sawada and K. Yoshino, J. Polym. Sci., Part A: Polym. Chem., 1993, 31, 2465 CrossRef CAS
.
- S. Wang, J. W. Hong and G. C. Bazan, Org. Lett., 2005, 7, 1907 CrossRef CAS
.
- L. Y. Wang, B. Dong, R. Ge, F. X. Jiang and J. K. Xu, ACS Appl. Mater. Interfaces, 2017, 9, 7108 CrossRef CAS
.
-
(a) J. Robin, N. Audebrand, C. Poriel, J. Canivet, G. Calvez, T. Roisnel, V. Dorcet and P. Roussel, CrystEngComm, 2017, 19, 2042 RSC
;
(b) F. Moreau, N. Audebrand and C. Poriel, CrystEngComm, 2020, 22, 293 RSC
;
(c) H. D. Guo, X. M. Guo, S. R. Batten, J. F. Song, S. Y. Song, S. Dang, G. L. Zheng, J. K. Tang and H. J. Zhang, Cryst. Growth Des., 2009, 9, 1394 CrossRef CAS
.
- S. C. Simon, B. Schmaltz, A. Rouhanipour, H. J. Raeder and K. Muellen, Adv. Mater., 2009, 21, 83 CrossRef CAS
.
- Y. Wei, Q. Y. Feng, H. Liu, X. L. Wang, D. Q. Lin, S. L. Xie, M. D. Yi, L. H. Xie and W. Huang, Eur. J. Org. Chem., 2018, 7009 CrossRef CAS
.
- C. Poriel, L. Sicard and J. Rault-Berthelot, Chem. Commun., 2019, 55, 14238 RSC
.
- M. Pasini, U. Giovanella, P. Betti, A. Bolognesi, C. Botta, S. Destri, W. Porzio, B. Vercelli and G. Zotti, ChemPhysChem, 2010, 10, 2143 CrossRef
.
-
(a) Y. K. Wang, Z. C. Yuan, G. Z. Shi, Y. X. Li, Q. Li, F. Hui, B. Q. Sun, Z. Q. Jiang and L. S. Liao, Adv. Funct. Mater., 2016, 26, 1375–1381 CrossRef CAS
;
(b) N. J. Jeon, H. Na, E. H. Jung, T. Y. Yang, Y. G. Lee, G. Kim, H. W. Shin, S. I. Seok, J. Lee and J. Seo, Nat. Energy, 2018, 3, 682–689 CrossRef CAS
.
- C. Poriel and J. Rault-Berthelot, Adv. Funct. Mater., 2020, 30, 1910040 CrossRef CAS
.
-
(a) F. Lucas, O. A. Ibraikulov, C. Quinton, L. Sicard, T. Heiser, D. Tondelier, B. Geffroy, N. Leclerc, J. Rault-Berthelot and C. Poriel, Adv. Opt. Mater., 2020, 8, 1901225 CrossRef CAS
;
(b) F. Lucas, C. Quinton, S. Fall, T. Heiser, D. Tondelier, B. Geffroy, N. Leclerc, J. Rault-Berthelot and C. Poriel, J. Mater. Chem. C, 2020, 8, 16354 RSC
;
(c) Q. Wang, F. Lucas, C. Quinton, Y. K. Qu, J. Rault-Berthelot, O. Jeannin, S. Y. Yang, F. C. Kong, S. Kumar, L. S. Liao, C. Poriel and Z. Q. Jiang, Chem. Sci., 2020, 11, 4887 RSC
.
-
(a) J. J. Liang, Y. Li, Y. Yuan, S. H. Li, X. D. Zhu, S. Barlow, M. K. Fung, Z. Q. Jiang, S. R. Marder and L. S. Liao, Mater. Chem. Front., 2018, 2, 917 RSC
;
(b) X. Q. Wang, S. Y. Yang, Q. S. Tian, C. Zhong, Y. K. Qu, Y. J. Yu, Z. Q. Jiang and L. S. Liao, Angew. Chem., Int. Ed., 2021, 60, 5213 CrossRef CAS
.
- W. W. Yong, H. Lu, H. Li, S. Wang and M. T. Zhang, ACS Appl. Mater. Interfaces, 2018, 10, 10828 CrossRef CAS
.
- Y. Yu, L. Y. Bian, Y. Zhang, Z. Liu, Y. T. Li, R. Zhang, R. L. Ju, C. Z. Yin, L. Yang, M. D. Yi, L. H. Xie and W. Huang, ACS Omega, 2019, 4, 5863 CrossRef CAS
.
- T. Virgili, M. Anni, M. L. De Giorgi, R. Borrego Varillas, B. M. Squeo and M. Pasini, Molecules, 2019, 25, 79 CrossRef
.
-
(a) S. Bebiche, P. A. Cisneros-Perez, T. Mohammed-Brahim, M. Harnois, J. Rault-Berthelot, C. Poriel and E. Jacques, Mater. Chem. Front., 2018, 2, 1631 RSC
;
(b) Z. P. Fan, X. Y. Li, X. E. Luo, X. Fei, B. Sun, L. C. Chen, Z. F. Shi, C. L. Sun, X. F. Shao and H. L. Zhang, Adv. Funct. Mater., 2017, 27, 1702318 CrossRef
.
-
(a) Y. Y. Liu, J. Y. Lin, Y. F. Bo, L. H. Xie, M. D. Yi, X. W. Zhang, H. M. Zhang, T. P. Loh and W. Huang, Org. Lett., 2016, 18, 172 CrossRef CAS PubMed
;
(b) X. F. Lu, S. Lee, Y. Hong, P. Hoa, T. Y. Gopalakrishna, T. S. Herng, T. Tanaka, M. E. Sandoval-Salinas, W. D. Zeng, J. Ding, D. Casanova, A. Osuka, D. Kim and J. S. Wu, J. Am. Chem. Soc., 2017, 139, 13173 CrossRef CAS PubMed
.
- K. Chen, H. R. Zhao, Z. K. Fan, G. Yin, Q. M. Chen, Y. W. Quan, S. H. Li and S. H. Ye, Org. Lett., 2015, 17, 1413 CrossRef
.
- E. Roka, M. Vecsernyes, I. Bacskay, C. Felix, M. Rhimi, A. W. Coleman and F. Perret, Chem. Commun., 2015, 51, 9374 RSC
.
- T. Ogoshi, S. Kanai, S. Fujinami, T.-A. Yamagishi and Y. Nakamoto, J. Am. Chem. Soc., 2008, 130, 5022 CrossRef CAS
.
- T. Tozawa, J. T. A. Jones, S. I. Swamy, S. Jiang, D. J. Adams, S. Shakespeare, R. Clowes, D. Bradshaw, T. Hasell, S. Y. Chong, C. Tang, S. Thompson, J. Parker, A. Trewin, J. Bacsa, A. M. Z. Slawin, A. Steiner and A. I. Cooper, Nat. Mater., 2009, 8, 973 CrossRef CAS
.
- Q. Wu, P. M. Rauscher, X. L. Lang, R. J. Wojtecki, J. J. de Pablo, M. J. A. Hore and S. J. Rowan, Science, 2017, 358, 1434 CrossRef CAS
.
- T. Kumpulainen, M. R. Panman, B. H. Bakker, M. F. Hilbers, S. Woutersen and A. M. Brouwer, J. Am. Chem. Soc., 2019, 141, 19118 CrossRef CAS
.
- M. Knaapila, R. Stepanyan, B. P. Lyons, M. Torkkeli and A. P. Monkman, Adv. Funct. Mater., 2006, 16, 599 CrossRef CAS
.
-
(a) L. Sicard, O. Jeannin, J. Rault-Berthelot, C. Quinton and C. Poriel, ChemPluschem, 2018, 83, 874 CrossRef CAS
;
(b) X. L. Yang, X. B. Xu and G. J. Zhou, J. Mater. Chem. C, 2015, 3, 913 RSC
;
(c) Y. Li, C. Barlose, J. Jorgensen, B. D. Carlsen and K. A. Jorgensen, Chem. – Eur. J., 2017, 23, 38 CrossRef CAS
;
(d) C. Poriel and J. Rault-Berthelot, J. Mater. Chem. C, 2017, 5, 3869 RSC
.
- M. W. Haenel, H. Irngartinger and C. Krieger, Chem. Ber., 1985, 118, 144 CrossRef CAS
.
- S. H. Jung, W. Pisula, A. Rouhanipour, H. J. Raeder, J. Jacoba and K. Muellen, Angew. Chem., Int. Ed., 2006, 45, 4685 CrossRef CAS
.
- E. Kayahara, R. Qu, M. Kojima, T. Iwamoto, T. Suzuki and S. Yamago, Chem. – Eur. J., 2015, 21, 18939 CrossRef CAS PubMed
.
-
(a) W. J. Liu, Y. Zhou, Q. F. Zhou, Y. G. Ma and J. Pei, Org. Lett., 2008, 10, 2123 CrossRef CAS PubMed
;
(b) P. K. Chen, X. D. Yin, N. Baser-Kirazli and F. Jäkle, Angew. Chem., Int. Ed., 2015, 54, 10768 CrossRef CAS
.
- I. S. Tamgho, S. Chaudhuri, M. Verderame, D. J. DiScenza and M. Levine, RSC Adv., 2017, 7, 28489 RSC
.
- S. Yamago, Y. Watanabe and T. Iwamoto, Angew. Chem., Int. Ed., 2010, 49, 757 CrossRef CAS
.
-
(a) H. Bai, Q. Chen, Y. F. Zhao, Y. B. Wu, H. G. Lu, J. Li and S. D. Li, J. Mol. Model., 2013, 19, 1195 CrossRef CAS
;
(b) S. Hitosugi, W. Nakanishi, T. Yamasaki and H. Isobe, Nat. Commun., 2011, 2, 492 CrossRef
;
(c) E. Kayahara, T. Iwamoto, H. Takaya, T. Suzuki, M. Fujitsuka, T. Majima, N. Yasuda, N. Matsuyama, S. Seki and S. Yamago, Nat. Commun., 2013, 4, 2694 CrossRef PubMed
.
- L. Sicard, F. Lucas, O. Jeannin, P. A. Bouit, J. Rault-Berthelot, C. Quinton and C. Poriel, Angew. Chem., Int. Ed., 2020, 59, 11066 CrossRef CAS
.
- M. Fujita, O. Sasaki, T. Mitsuhashi, T. Fujita, J. Yazaki, K. Yamaguchi and K. Ogura, Chem. Commun., 1996, 13, 1535 RSC
.
- J. Q. Wang, Y. Han and C. F. Chen, Chem. Commun., 2021, 57, 3987 RSC
.
- P. K. Chen and F. Jaekle, J. Am. Chem. Soc., 2011, 133, 20142 CrossRef CAS PubMed
.
- W. Wu, J. W. Wang, Y. K. Wang, Y. J. Huang, Y. F. Tan and Z. Q. Weng, Angew. Chem., Int. Ed., 2017, 56, 10476 CrossRef CAS PubMed
.
- L. Li, Q. Chen, Z. G. Niu, X. H. Zhou, T. Yang and W. Huang, J. Mater. Chem. C, 2016, 4, 1900 RSC
.
-
(a) N. Fomina and T. E. Hogen-Esch, Macromolecules, 2008, 41, 3765 CrossRef CAS
;
(b) Y. B. Song, W. Xu and D. B. Zhu, Tetrahedron Lett., 2010, 51, 4894 CrossRef CAS
.
- I. A. Adams and P. A. Rupar, Macromol. Rapid Commun., 2015, 36, 1336 CrossRef CAS
.
- Q. G. Kong, D. Zhu, Y. W. Quan, Q. M. Chen, J. F. Ding, J. P. Lu and Y. Tao, Chem. Mater., 2007, 19, 3309 CrossRef CAS
.
- J. Ipaktschi, R. Hosseinzadeh and P. Schlaf, Angew. Chem., Int. Ed., 1999, 38, 1658 CrossRef CAS
.
- M. Y. Song, H. K. Na, E. Y. Kim, S. J. Lee, K. I. Kim, E. M. Baek, H. S. Kim, D. K. An and C. H. Lee, Tetrahedron Lett., 2004, 45, 299 CrossRef CAS
.
- Z. K. Fan, N. Q. Li, Y. W. Quan, Q. M. Chen, S. H. Ye, Q. L. Fan, W. Huang and H. Xu, J. Mater. Chem. C, 2014, 2, 9754 RSC
.
- Y. Lu, Y. Zhou, Y. W. Quan, Q. M. Chen, R. F. Chen, Z. Y. Zhang, Q. L. Fan, W. Huang and J. F. Ding, Org. Lett., 2011, 13, 200 CrossRef CAS PubMed
.
- N. Q. Li, Z. K. Fan, Y. Y. Fang, L. Li, Y. W. Quan, Q. M. Chen, S. H. Ye, Q. L. Fan and W. Huang, J. Phys. Chem. C, 2017, 121, 8692 CrossRef CAS
.
- G. Wittig, E. Dreher, W. Reuther, H. Weidinger and R. Steinmetz, Justus Liebigs Ann. Chem., 1969, 726, 188 CrossRef
.
- J. Ipaktschi, R. Hosseinzadeh, P. Schlaf, E. Dreiseidler and R. Goddard, Helv. Chim. Acta, 1998, 81, 1821 CrossRef CAS
.
- J. Ipaktschi, R. Hosseinzadeh, P. Schlaf and T. Eckert, Helv. Chim. Acta, 2000, 83, 1224 CrossRef CAS
.
- D. Beaudoin, O. Levasseur-Grenon, T. Maris and J. D. Wuest, Angew. Chem., Int. Ed., 2016, 55, 894 CrossRef CAS
.
- L. Yang, J. Mao, C. Z. Yin, M. A. Ali, X. P. Wu, C. Y. Dong, Y. Y. Liu, Y. Wei, L. H. Xie, X. Q. Ran and W. Huang, New J. Chem., 2019, 43, 7790 RSC
.
- P. N. W. Baxter, J. M. Lehn, J. Fischer and M. T. Youinou, Angew. Chem., Int. Ed. Engl., 1994, 33, 2284 CrossRef
.
-
(a) L. H. Xie, X. Y. Hou, C. Tang, Y. R. Hua, R. J. Wang, R. F. Chen, Q. L. Fan, L. H. Wang, W. Wei, B. Peng and W. Huang, Org. Lett., 2006, 8, 1363 CrossRef CAS
;
(b) Y. Z. Chang, Q. Shao, L. Y. Bai, C. J. Ou, J. Y. Lin, L. H. Xie, Z. D. Liu, X. D. Chen, G. W. Zhang and W. Huang, Small, 2013, 9, 3218 CAS
;
(c) Y. Qian, Q. Wei, G. Del Pozo, M. M. Mroz, L. Lueer, S. Casado, J. Cabanillas-Gonzalez, Q. Zhang, L. Xie, R. Xia and W. Huang, Adv. Mater., 2014, 26, 2937 CrossRef CAS
.
- L. H. Xie, X. Y. Hou, Y. R. Hua, C. Tang, F. Liu, Q. L. Fan and W. Huang, Org. Lett., 2006, 8, 3701 CrossRef CAS PubMed
.
- G. W. Zhang, Y. Wei, J. S. Wang, Y. Y. Liu, L. H. Xie, L. Wang, B. Y. Ren and W. Huang, Mater. Chem. Front., 2017, 1, 455 RSC
.
- S. X. Sun, C. J. Ou, D. Q. Lin, Z. Y. Zuo, Y. Yan, Y. Wei, Y. Y. Liu, L. H. Xie and W. Huang, Tetrahedron, 2018, 74, 5833 CrossRef CAS
.
- T. Amaya, H. Fujimoto, T. Tanaka and T. Moriuchi, Org. Lett., 2018, 20, 2055 CrossRef CAS PubMed
.
- S. Castro-Fernandez, R. Yang, A. P. Garcia, I. L. Garzon, H. Xu, A. G. Petrovic and J. L. Alonso-Gomez, Chem. – Eur. J., 2017, 23, 11747 CrossRef CAS PubMed
.
- A. Ozcelik, D. Aranda, S. Gil-Guerrero, X. A. Pola-Otero, M. Talavera, L. X. Wang, S. K. Behera, J. Gierschner, A. Pena-Gallego, F. Santoro, R. Pereira-Cameselle and J. L. Alonso-Gomez, Chem. – Eur. J., 2020, 26, 17342 CrossRef CAS PubMed
.
-
X. L. Wang, Molecular engineering of spirodifluorene-based tic-tac-toe-type nanogridarenes D, Nanjing University of Posts and Telecommunications, Nanjing, 2019 Search PubMed
.
- K. Zhu, K. Kamochi, T. Kodama, M. Tobisu and T. Amaya, Chem. Sci., 2020, 11, 9604 RSC
.
- K. Miki, T. Noda, M. Gon, K. Tanaka, Y. Chujo, Y. Mizuhata, N. Tokitoh and K. Ohe, Chem. – Eur. J., 2019, 25, 9211 CrossRef CAS
.
- J. Oniki, T. Moriuchi, K. Kamochi, M. Tobisu and T. Amaya, J. Am. Chem. Soc., 2019, 141, 18238 CrossRef CAS PubMed
.
- W. Xu, X. D. Yang, X. B. Fan, X. Wang, C. H. Tung, L. Z. Wu and H. Cong, Angew. Chem., Int. Ed., 2019, 58, 3943 CrossRef CAS
.
- K. Senthilkumar, M. Kondratowicz, T. Lis, P. J. Chmielewski, J. Cybinska, J. L. Zafra, J. Casado, T. Vives, J. Crassous, L. Favereau and M. Stępień, J. Am. Chem. Soc., 2019, 141, 7421 CrossRef CAS
.
- T. A. Schaub, E. A. Prantl, J. Kohn, M. Bursch, C. R. Marshall, E. J. Leonhardt, T. C. Lovell, L. N. Zakharov, C. K. Brozek, S. R. Waldvogel, S. Grimme and R. Jasti, J. Am. Chem. Soc., 2020, 142, 8763 CrossRef
.
- J. Luo, T. Lei, X. G. Xu, F. M. Li, Y. G. Ma, K. Wu and J. Pei, Chem. Eur. J., 2008, 14, 3860 CrossRef CAS
.
- Y. Wei, M. C. Luo, G. W. Zhang, J. Q. Lei, L. H. Xie and W. Huang, Org. Biomol. Chem., 2019, 17, 6574 RSC
.
|
This journal is © The Royal Society of Chemistry 2022 |
Click here to see how this site uses Cookies. View our privacy policy here.