DOI:
10.1039/D2BM01694J
(Review Article)
Biomater. Sci., 2023,
11, 1625-1647
Expanding opportunities to engineer mucosal vaccination with biomaterials
Received
17th October 2022
, Accepted 20th December 2022
First published on 30th January 2023
Abstract
Mucosal vaccines are receiving increasing interest both for protecting against infectious diseases and for inducing therapeutic immune responses to treat non-infectious diseases. However, the mucosal barriers of the lungs, gastrointestinal tract, genitourinary tract, nasal, and oral tissues each present unique challenges for constructing efficacious vaccines. Vaccination through each of these mucosae requires transport through the mucus and across specialized epithelia to reach tissue-specific immune cells and lymphoid structures, necessitating finely tuned and multifunctional strategies. Serving as inspiration for mucosal vaccine design, pathogens have evolved elaborate, diverse, and multipronged approaches to penetrate and infect mucosae. This review is focused on biomaterials-based strategies, many inspired by pathogens, for designing mucosal vaccine platforms. Passive and active technologies are discussed, along with the microbial processes that they seek to mimic.
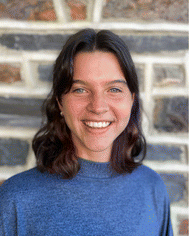 Helena Freire Haddad | Helena Freire Haddad obtained her BS in biomedical engineering from Northwestern University. She is currently a PhD student at Duke University. Her current work focuses on engineering mucus-penetrating materials for mucosal vaccine delivery and designing active immunotherapies to mitigate inflammatory diseases. |
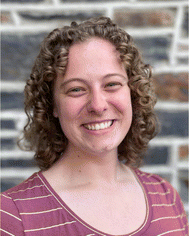 Emily F. Roe | Emily Roe obtained her BS in Materials Science and Engineering from North Carolina State University. She is currently a PhD candidate at Duke University. Her current work focuses on sublingual delivery of vaccines to prevent sexually transmitted infections and conjugation of proteins to self-assembling peptide nanofibers. |
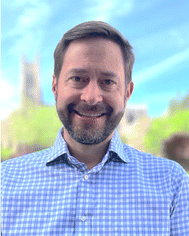 Joel H. Collier | Joel Collier is the Theodore Kennedy Professor of Biomedical Engineering at Duke University. He received his BS in Materials Science and Engineering from Rice University and his PhD in Biomedical Engineering from Northwestern University. His research group designs biomolecular materials for immunotherapies toward the treatment of infectious and inflammatory diseases. |
1. Introduction
Designing nanomaterials for mucosal vaccination requires thoughtful consideration of the challenges presented by the mucosal barrier. Size, charge, physicochemical properties, and receptor–ligand interactions all govern the ability of a material to penetrate the mucus gel and the underlying epithelium to deliver combinations of antigens, adjuvant molecules, and carriers to the submucosal space. The mucosa is a highly dynamic system where mucus is being continuously secreted and shed to regulate the transport of pathogens, small molecules, antigens, and endogenous substances into the epithelium. Mucin glycoproteins, the major structural component of mucus, form an enmeshment of fibers that trap foreign particulates. After overcoming the mucus layer, particulates must additionally transverse the epithelium. Whereas this system provides beneficial protection against pathogen invasion, it is a formidable barrier for vaccine delivery of nanomaterials.
A broad range of biomaterial strategies have been employed in the realm of mucosal delivery, leveraging tailored physicochemical properties to improve transport across the mucus layer. Polymeric modifications have been used to modulate charge, hydrophobicity, and mucoadhesion, improving retention and diffusion of antigen-containing carriers through the mucosa. Nanocarriers have been designed both for passive and active delivery of payloads across the mucosa, employing components such as mucolytic enzymes, ligand–receptor systems, and stimuli-responsive materials. Combinations of these strategies can mimic the natural mechanisms through which various pathogens penetrate and colonize host mucus. In this review, biomaterial platforms for mucosal vaccine delivery will be discussed, emphasizing mucus penetration strategies and the ability of these platforms to elicit robust and effective immune responses. Mucosal vaccination offers advantages for raising neutralizing immune responses both at mucosal sites of infection as well as systemically or in tissues remote from the vaccination site. Furthermore, in low-resource settings, mucosal vaccines may provide a route for higher compliance and convenience by affording needle-free administration. Previous reviews have discussed material design strategies for drug delivery through mucosal barriers,1 polymeric modifications to enhance mucus penetration,2 and materials for mucosal delivery of cancer vaccines.3 Herein, we will expand on these themes, focusing specifically on biomaterial and nanomaterial design for vaccines against infectious diseases, with an emphasis on bio-inspired strategies for mucosal penetration.
2. Mucosal delivery
2.1. Mucus physiology and physicochemical properties
Mucosae are the epithelial layers which line body cavities such as the gastrointestinal and respiratory tracts. Above the epithelial cells of mucous membranes lies mucus which is secreted by specialized cells or glands goblet cells in the respiratory, gastrointestinal, and genital tracts and parotid, submandibular, and sublingual glands making saliva for the oral cavity. Mucus can either be an adherent gel layer or suspended above the epithelial surface and is composed of glycoproteins, lipids, inorganic salts, and water, the last of which makes up to 95% of its weight.4 Mucins are the primary glycoproteins present in mucus, making up to 80% of the dry weight and responsible for the structure and gel forming nature of mucus.5 Approximately 20 mucin proteins have been identified in humans, 5 of which are gel forming (MUC 2, 5AC, 5B, 6, 19). Others are soluble but not gel forming (MUC 7, 8, 9, 20) or membrane bound (MUC 1, 3A, 3B, 4, 12, 13, 15, 16, 17, 21, 22). The major secreted airway mucins are MUC5AC and MUC5B. MUC2is primarily secreted in the gastrointestinal tract, and MUC7 is the major salivary protein.6,7 All mucins are heavily glycosylated proteins ranging from 10–40 MDa,8 with up to 30 carbohydrate chains per 100 amino acid residues.9 Due to this highly glycosylated nature, individual proteins have a bottle-brush appearance. Mucins additionally have a high sialic acid and sulfate content, leading to a highly negative charge which increases rigidity due to charge repulsion.8 The amino- and carboxy-termini of mucin proteins are rich with cysteines which leads to extensive disulfide crosslinking, creating a mesh-like network in which foreign materials are trapped. Designing materials for the controlled and predictable penetration of the mesh-like network formed by mucins to enable mucosal vaccination is the central focus of this review.
2.2. Principles of mucus penetration
Any mucosal vaccine, regardless of target tissue, must contend with the formidable barrier presented by mucus. Mucus adhesion and penetration is a complex process in which a mucous membrane adheres to another material. Ionic, covalent, hydrogen, and van der Waals bonds form between mucus and foreign materials, preventing these foreign material from accessing the epithelial layer, and allowing for clearance away from the mucous membrane.4 Mucus adhesion can be divided into two stages, the contact stage and the consolidation stage. In the contact, or wetting stage, the two surfaces come together either through direct physical contact by placing a material onto a mucous membrane, or by particle deposition based on fluid flow across the mucosae. At this stage, the forces between the two surfaces are generally low. The second consolidation stage forms stronger bonds between mucus and a foreign material for prolonged adhesion. The two theories which govern the increased bonds in the consolidation stage are interpenetration of the foreign material with mucus glycoproteins10 and dehydration of the mucus layer.11 In the interpenetration theory, mucoadhesive materials and mucins become physically tangled and interact via hydrogen bonds and van der Waals forces.12 The dehydration theory states that when materials capable of rapid gelation are placed in contact with mucus, water movement occurs out of mucus and into the foreign material until equilibrium is reached.11 While neither theory alone can explain the complexity of mucus adhesion, each contributes to the physical phenomena observed, and both are crucial in understanding how to exploit and control mucus penetration and adhesion to enable mucosal vaccination. Work by Ponchel et al. used the theory of interpenetration to examine the coefficient of diffusion and work of adhesion when tablets composed of poly(acrylic acid) (PAA) and hydroxypropyl carboxymethylcellulose were placed in contact with bovine mucin.13 The authors found that after reaching the swollen equilibrium state, the work of adhesion to detach the tablet from the mucus increased with increasing PAA content. Due to the non-crosslinked nature of PAA and the high capacity for swelling, the increased work of adhesion was attributed to increased interpenetration due to diffusion of PAA towards the mucus. Based on relationships between fracture energy and interpenetration depth, the authors demonstrated increased interpenetration with increasing PAA content.13 This work demonstrated how theories behind mucus adhesion and penetration can form a basis for biomaterials design in the context of mucosal vaccine delivery. For more context regarding the principles which govern mucus adhesion and penetration and how they can be applied to mucosal vaccine delivery, we direct the reader to several reviews which expand on the ideas discussed here.4,5
2.3. Mucosal tissues each present unique delivery challenges
Superficially, the mucosal tissues of the body share common features, yet each has highly specialized epithelia, mucus, lymphoid structures, resident immune cells, and commensal microbiota, presenting unique considerations for biomaterials-based vaccines appropriate for each tissue. Here, we will provide a brief synopsis of the mucosal physiology relevant for vaccination via the sublingual, buccal, gastrointestinal, nasal, and genital mucosae, noting features making each tissue unique (Fig. 1). The nasal epithelium is a ciliated pseudostratified columnar epithelium, with mucus secreted by goblet cells and cleared by the movement of cilia. Nasal associated lymphoid tissue, including adenoids, palatine tonsils, tubal tonsils, and lingual tonsils, contains the lymphocytes and leukocytes required to generate an adaptive immune response and plays an important role in generating Th1, Th2, and IgA-secreting B cells in the respiratory tract.14 In the respiratory and gastrointestinal tracts, specialized epithelial cells called M cells deliver antigens into organized lymphoid tissues.15 For sublingual or buccal vaccines delivered via the oral cavity, non-keratinized stratified squamous epithelium is covered with saliva secreted by parotid, submandibular, and sublingual glands in the oral cavity, which is cleared by regular swallowing. The oral cavity is immunologically privileged, without dedicated lymphoid tissues like other mucosae. Instead, antigen is delivered to Langerhans cells and myeloid dendritic cells, which sample antigens and transport them to lymph nodes to direct adaptive immune responses.16 Oral vaccines are usually designed for antigen delivery in the intestines, which are lined with simple columnar epithelia, with mucus produced by goblet cells, which increase in frequency through the gastrointestinal tract, with the lowest concentration of goblet cells located in the duodenum, and highest concentration in the rectum. Peyer's patches, groupings of lymphoid follicles in the small intestine, are the organized lymphoid tissue dedicated to generating immune responses in the gastrointestinal tract. They are responsible for generating a tolerogenic environment in response to commensal pathogens and function to bridge the innate and adaptive immune system within the gut.17 M cells surveil antigens and deliver them to Peyer's patches for further processing and generation of an immune response.15 The epithelium of the female genital tract is comprised of non-keratinized stratified squamous epithelium, with mucus secreted by cervical glands. Like in the oral cavity, there is no organized lymphoid tissue dedicated to the genital tract. Further, epithelial cells constitutively express MHCII molecules and can act as antigen presenting cells in the lower genital tract.18 The genital tract also has a broadly ranging commensal microbiome, with the upper genital tract being sterile and the vaginal tract and ectocervix supporting a diverse microbial community. Additionally, antibodies in the genital tract are dominated by IgG, and not IgA as with other mucosal surfaces.18,19 Although the various mucosae share common features such as an epithelium overlaid with mucus, the mechanisms of immune surveillance and generation of an immune response are unique to each tissue, and therefore each route of vaccination and must be considered in designing mucosal vaccines.
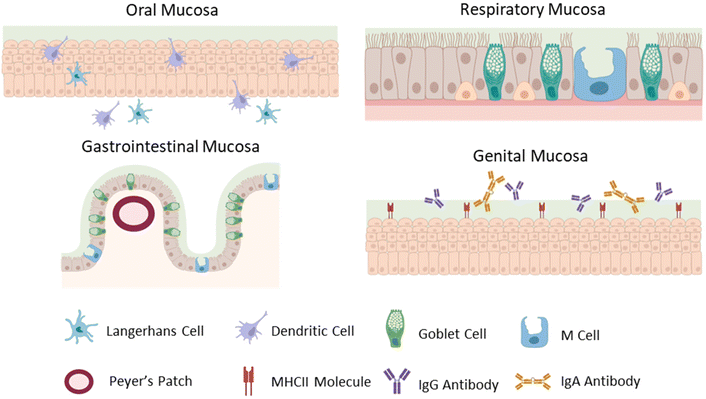 |
| Fig. 1 Mucosal epithelia of oral, respiratory, gastrointestinal, and genital tissues respectively (top to bottom). Major cells and structures at these sites include Langerhans cells, dendritic cells, goblet cells, M cells, Peyer's patches, MHCII, IgG and IgA. Created with BioRender.com. | |
3. Pathogen entry strategies: sources of inspiration
Through co-evolution with humans, pathogens have developed a bewildering array of finely tuned strategies for colonizing and infecting mucosal surfaces. Increasingly, these strategies are providing inspiration for the design of biomaterials for mucosal vaccination. In particular, microorganisms can disrupt and modulate the mucus layer, often through enzymatic activity and mechanical propulsion, allowing them to reach, penetrate, and infect the epithelium and beyond.
Cysteine proteases are utilized by protozoan parasites to invade tissues and have thus inspired drug development against diseases such as leishmaniasis, malaria, and Chagas disease.20–22 Many of these parasites infect through the bloodstream, however some gastrointestinal protozoans utilize cysteine proteases for entry through mucosal surfaces. The intestinal parasite Giardia duodenalis, which causes malabsorptive diarrhea, malnutrition, and chronic complications produces cysteine proteases capable of degrading mucus.23 These degrade human MUC2 mucin in a dose-dependent manner, and are sensitive to protease inhibitors. In a goblet cell line infected with G. duodenalis, mucin depletion occurred initially, but in later timepoints intracellular mucin was upregulated, which was attributed to an upregulation of mRNA encoding MUC2 production. This was confirmed in vivo, as infected mice exhibited higher levels of intracellular mucin, but had a thinner mucus layer covering the intestinal epithelium.23 Similarly, the protozoan Entamoeba histolytica utilizes cysteine proteases to colonize the colonic mucus, causing dysentery, bloody diarrhea, and associated complications.24 In the colon, MUC2 monomers are linked by disulfide bonds in the cysteine-rich N- and C-terminus, forming a thick polymeric mucus gel. Because both termini are less heavily O-glycosylated, the peptide chain is more exposed and makes them more vulnerable to protease activity. It was shown that E. histolytica protease targets this vulnerability and preferentially cleaves the C-terminus of MUC2, depolymerizing mucin chains and facilitating pathogen infection.25
Some pathogens have surface ligands that can interact with receptors on host cells. This is exemplified by yeasts’ ligand β-glucan that binds to dectin-1 on the surface of M cells,26 or the receptor binding proteins of viruses such as SARS-COV-2, which can be conjugated to virus-mimetic particles to create “synthetic pathogens”.27 Other pathogens employ the combined and concerted action of multiple polysaccharide-binding and polysaccharide-degrading enzymes to penetrate the mucus layer. For example, the influenza A virus displays two key antigenic proteins in its envelope, the sialic acid-binding hemagglutinin (HA) and the terminal sialic acid-cleaving neuraminidase (NA, also called sialidase). Cooperation between these two proteins enables motility within the sialylated mucus layer, both during viral entry into cells and during the shedding of virus particles. It is speculated that through NA-driven dynamic receptor interactions, spherical influenza viruses roll through the heavily O-glycosylated mucins until they reach the sialylated epithelium for infection.28 However, there is a growing appreciation that the spatial distribution of these two enzymes on the viral surface, along with the overall shape of the virus itself, can confer a highly specialized ability for the virus to move to and from the cells they infect. In a recent elegant demonstration of this principle, Vahey and Fletcher found that NA and HA are asymmetrically distributed on filamentous influenza virus particles, with a NA-rich pole and HA distributed on the rest of the surface.29 Use of a model sialylated surface facilitated the observation of virus particle motion, which followed a ratchet-like pattern. Interestingly, viral particles moved away from the NA-rich pole with directed motility. This polarization of mucus-binding and receptor-cleaving proteins and the resulting directional motility may inform biomaterial design for enhanced mucus penetration and mucosal vaccination.
pH-responsiveness is another broad feature utilized by mucosal pathogens that can inspire biomaterial strategies. For example, the flagellar motion of Vibrio Cholerae bacteria is significantly influenced by pH. Flagellar motion is likely essential for the bacteria to reach the epithelium and deliver cholera toxin, which leads to water and chloride influx to the intestine and the diarrhea that is a hallmark of the disease. This flagellar motility is dependent on the sodium transporter NADH:quinone oxidoreductase (NA1-NQR), a component of the respiratory chain that is more active at alkaline pH.30 Inhibiting NA1-NQR activity in vitro led to a reduced swimming speed of V. Cholerae, suggesting that this mechanism is important for increased motility, particularly in the intestinal tissues the bacteria infect. Such a principle can be incorporated into drug and vaccine delivery, by tailoring when materials are “active” for delivery in the gastrointestinal tract based on pH. In contrast, the bacterium Helicobacter Pylori does not rely on pH changes to locomote, but rather uses enzymatic activity to locally modulate the pH of the mucus layer. This gastric pathogen is then able to colonize the mucosa of the stomach and reach the underlying epithelial cells for infection. H. pylori produces urease, which hydrolyzes the urea in the stomach to produce ammonia and CO2, locally increasing pH. Both urease production and bacteria motility are crucial factors for the pathogen to survive, and these two factors have been shown to influence each other in the infection process.31 An increased pH changes the rheology of gastric mucus, liquifying the gel and therefore allowing for increased motility. Since the enmeshment of mucin fibers is a barrier for delivery, analogous local modulation of mucus’ viscoelasticity can be an attractive strategy for material design, discussed in the sections below.
Finally, microbes utilize biomechanical propulsion strategies such as flagellar motion to propel themselves actively through mucus and tissues. Through the whipping of these structures, they generate forces sufficient to propel themselves through biological fluids, including mucus, a strategy that has inspired propulsion-based delivery strategies discussed below.32
4. Passive material strategies for mucus penetration
4.1. Charge modulation
Manipulation of electrostatic interactions is a useful way to control mucus adhesion and mucus penetration. Increasing positive surface charge on particles generally increases mucus adhesion and retention due to interaction with negatively charged mucus.33 Negative charge, conversely, tends to increase mucus penetration due to repulsion from negatively charged mucins. To balance mucus penetration and mucus adhesion, one highly investigated strategy is the use of zwitterionic particles – which contain both positively and negatively charged regions. In an illustrative recent example, Hu et al. systematically investigated a variety of polymeric coatings on PLGA nanoparticles and showed that zwitterionic coatings in particular increased both mucus penetration and cellular uptake (Fig. 2a).34 The authors synthesized poly(lactic-co-glycolic acid) (PLGA) nanoparticles with a variety of coatings including polyethylene glycol (PEG), poly(vinyl alcohol) PVA, Pluronic F127 (F127) (a copolymer surfactant consisting of a central hydrophobic polypropylene glycol block and two hydrophilic polyethylene blocks), and polydopamine (PDA), a bioinspired polymer of dopamine commonly used as a hydrophilic coating. Multiple particle tracking was used to quantify mucus penetration (Fig. 2b), and TEM images were used to measure cellular uptake into mucus-producing HT29-MTX cells (Fig. 2c). Comparatively positively charged unmodified PLGA and PVA NPs resulted in limited mucus penetration and cellular uptake due to increased mucin interaction and adhesion. Highly negatively charged PLGA-PEG and PLGA-F127 demonstrated satisfactory mucus penetration but were not readily acquired by mucus-producing cells. Zwitterionic PLGA-PDA facilitated both rapid mucus penetration and increased cellular uptake. These NPs proved biocompatible and non-toxic when applied directly to the intestines in vivo – demonstrating the promise of this platform for effective delivery of antigens or drugs past the mucus barrier and to the epithelial cells of interest. Charge modulation, especially in combination with other modifications discussed here, is essential to enabling mucus penetration of biomaterials by balancing the forces of mucus adhesion and mucus penetration.
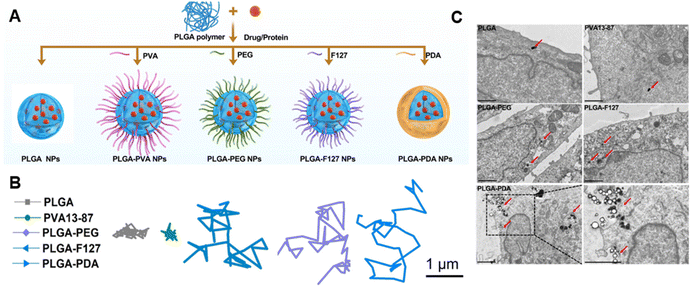 |
| Fig. 2 (A) Schematic illustration of nanoparticle synthesis and different coating techniques. PLGA NPs with different polymeric coronas: PLGA only (PLGA), poly(vinyl alcohol) MW 13 000–23 000, 80–97% hydrolyzed (PVA 13–87), polyethylene glycol (PLGA-PEG), Pluronic F127 (PLGA-F127), and polydopamine (PLGA-PDA). (B) Transport trajectory of FITC-labeled NPs in porcine mucus as measured by multiple particle tracking. PLGA and PVA 13–87 NPs are immobilized by mucin fibers while PGLA-PEG, PLGA-F127, and PLGA-PDA move freely through mucus. (C) Cellular uptake of different nanoparticles in HT29-MTX mucus-producing cells. Cells were incubated with NPs for 2 h, then collected, washed, and fixed with 2.5% glutaraldehyde and observed under TEM, scale bars: 2 μm. Adapted from Hu et al., Chem. Eng. J., 2022, 428, 132107 with permission from Elsevier. | |
4.2. Polymeric modification: natural polymers
A broad range of polymers have been investigated as surface modifiers for enabling mucosal vaccination, with polysaccharides receiving heightened interest. Chitosan and its derivatives in particular have been heavily investigated and have proven to be effective in achieving immune responses against antigens of interest. Many of these polymers have been found to provide increased mucus adhesion and residence time at mucosal sites after vaccination, leading to increased uptake of vaccine formulations.35
Chitosan is a biopolymer obtained by deacetylating chitin, which is found in the exoskeleton of crustaceans. Chitosan is abundant, biodegradable, and has been extensively used in a wide variety of applications, including water treatment, cosmetics, and biopharmaceutics, primarily as a metal ion chelation agent and coagulant.36 The degree of deacetylation is an important chemical characteristic of chitosan, as it controls the amount of free amines and thus charge present; the term chitosan generally refers to chitin that has been deacetylated more than 70%.36 Chitosan has several characteristics which can be exploited in mucosal vaccination strategies. Of particular interest in this review, chitosan is mucus-adhesive and can open tight junctions between epithelial cells,37 increasing time to clearance from mucosal sites and uptake of vaccine formulations respectively. Chitosan can also be used as an adjuvant – it activates dendritic cells via the STING (STimulator of INterferon Genes) pathway;38 however, does not directly induce cytokine production.39 Chitosan has also been shown to activate the NLRP3 inflammasome, leading to neutrophil recruitment, increased antigen presenting cell maturation, and a pro-inflammatory environment through the secretion of cytokines including IL-18 and IL-1β.40 Chitosan is often further chemically modified to enhance solubility or other desired characteristics. For example, N-trimethylation of chitosan41,42 and decreasing its molecular weight43 improves its solubility, and glycol chitosan44 and aminated chitosan45 further increase mucus adhesion. The ability to chemically modify and formulate chitosan using a variety of methods makes it a popular choice of biomaterial to increase the efficacy of mucosal vaccines with a variety of delivery routes including oral46,47 and intranasal.41,44,45 Sinani et al. modified chitosan to increase its mucoadhesive properties and adjuvanticity.45 The authors created aminated chitosan (aChi) and aminated plus thiolated chitosan (atChi). Using peak force detachment studies and work of adhesion measurements, they found that aChi and atChi had increased mucoadhesion compared with unmodified chitosan (Chi) due to increased interactions between amines on modified chitosan and sialic acid in mucus. The authors formed chitosan nanoparticles with encapsulated bovine serum albumin (BSA) as a control antigen via ionotropic gelation with aChi, atChi, and Chi. When these nanoparticles were delivered intranasally, mice vaccinated with aChi and atChi retained higher serum IgG and IgA 36 weeks after vaccination compared to free BSA + CpG adjuvant. This work demonstrates the versatility of chitosan in mucosal vaccine delivery, both as a tool to increase mucus adhesion and as an adjuvant. Although chitosan can significantly increase mucosal retention time in many cases, the balance between mucus adhesion and mucus penetration must still be considered. Additionally, owing to the natural sourcing of chitosan and other polysaccharides, they can be polydisperse and sacrifice some amount of control over their precise chemical composition. Where mucus penetration or precise control of material composition or molecular weight is of greater concern, other biomaterials strategies may be better suited.
Other polysaccharides are also commonly used to modulate mucus penetration of vaccine formulations for mucosal delivery. Alginate48 and cellulose derivatives are the most used polysaccharides outside of chitosan. As a recent example, Hanson et al. used a combination of carboxymethylcellulose (CMC) and alginate to develop a mucoadhesive film for sublingual vaccination.49 CMC provided increased mucus adhesion, while alginate provided mechanical stability as well as effective protein stabilization. They designed alginate/CMC films encapsulating an HIV antigen that raised immune responses when delivered as a sublingual vaccine. These data demonstrate the wide variety of polysaccharides which can be used to control biomaterial interaction with mucus.
4.3. Polymeric modifications: synthetic polymers
Synthetic polymers offer the opportunity to finely tailor interactions between nanomaterials and the mucosal surface. While naturally sourced polysaccharides mainly provide increased mucus adhesion, synthetic polymers can be tailored to provide increased mucus penetration. PEG is the most widely used and best understood polymer in this context, pioneered by the work of Justin Hanes’ group.50–53 More recently, alternatives to PEG have been investigated, including oxazolines, polypeptides, and zwitterionic polymers.
Polyethylene glycol.
PEG is a widely used biomedical polymer and is approved by the FDA for multiple applications.54,55 In the context of mucus penetration, it is understood to be mucus-inert-to penetrative and has been used to deliver vaccines orally,56 sublingually,57–59 and to the genital tract.60–62 Continued research into PEG has optimized its use as a modulator of mucus transport. Xu et al. examined the role of PEG density in mucus penetration (Fig. 3a).61 The authors prepared PLGA nanoparticles with varying densities of 5 kDa PEG by combining different ratios of PLGA and PLGA-PEG copolymer. 5% PEG was needed to achieve effective transport through mucus both in vitro and in vivo. Nanoparticles with at least 5% PEG had a high mean square displacement measured by multiple particle tracking (Fig. 3b) and high effective diffusivity, enough for effective diffusion through mucus. In vivo nanoparticles with higher percentages of PEG were more uniformly distributed with higher percent coverage in the vaginal canal after administration in mice (Fig. 3c) while lower percentages of PEG did not penetrate the mucus and were confined to the luminal facing tissue of vaginal rugae (Fig. 3d). This work highlights the level of control achievable with defined synthetic polymeric modifications. Precise control over the molecular weight, density, and nanomaterial synthesis allows PEG to be tuned to a variety of contexts and for a variety of antigens.
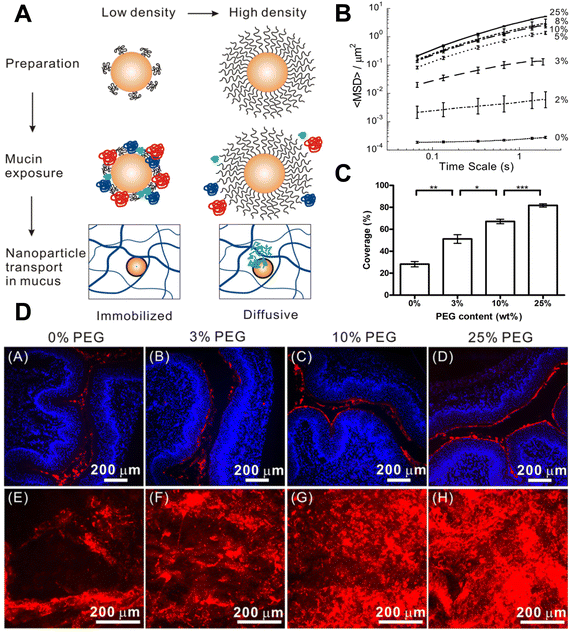 |
| Fig. 3 (A) Schematic illustrating the effect of nanoparticle PEG surface density on transport behavior in mucus (blue mesh). Varying molecular weight mucin particles (low: light blue, medium: dark blue, high: red) bind to the nanoparticle core where it is exposed. Low density PEG coatings adhere to mucus and mucin particles can access the nanoparticle core, while high density PEG coatings diffuse rapidly through mucus (trajectory shown in green). (B) Ensemble-averaged geometric mean square displacement as a function of time scale for PLGA NPs with varying PEG content in human cervicovaginal mucus. High density PEG coatings correspond to increased displacement. (C) Percent surface coverage by NPs on flattened vaginal tissue after administration in vivo of an estrus phase mouse. 10 minutes after administration, the entire vagina was removed and frozen for later imaging. (D) Fluorescence images of mouse vagina (blue: DAPI, red: NPs). A–D: 6 μm cryosections of natural conformation of vaginal tissue. E–H: 6 μm cryosections of vaginal tissue flattened between two glass slides to expose rugae. NPs with low density PEG coatings were confined to luminal facing surfaces while NPs with high density PEG coatings penetrated mucus and accessed vaginal rugae for more uniform coverage. Adapted with permission from Xu et al., ACS Nano, 2015, 9(9), 9217, Copyright © 2015 American Society of Chemistry. | |
Other synthetic polymers.
Polyethylene glycol has received particular interest, but other synthetic polymers have also been shown to enable mucus penetration for mucosal vaccine delivery. Further, recent concern over the immunogenicity of PEG has intensified the search for PEG alternatives.2,63 Oxazolines,64,65 polypeptides,58 and zwitterionic polymers have been investigated as alternatives to PEG for modulating nanoparticle penetration through and adhesion to mucus, and for increasing retention within mucosal tissues.66,67 For example, Mansfield et al. compared several oxazoline polymers to understand how side chain chemistry impacts mucus penetration.64 They functionalized silica nanoparticles with poly(2-methyl-2-oxazoline) (PMOZ), poly(2-ethyl-2-oxazoline) (PEOZ), or poly(2-n-propyl-2-oxazoline) (PNPOZ) (Fig. 4a) and found that the diffusion coefficient of PMOZ was higher than PEOZ, PNPOZ, or unmodified silica nanoparticles, with no significant difference between PNPOZ and unmodified nanoparticles (Fig. 4b). The increase in diffusion coefficient was attributed to the smaller, less hydrophobic side chain on PMOZ compared to PEOZ and PNPOZ. On fresh porcine mucosa sections, PMOZ penetrated further into the mucus at all time points compared to unmodified nanoparticles or other oxazoline modifications (Fig. 4c). PNPOZ exhibited the least ability to penetrate mucus, potentially due to its more hydrophobic side chains. This work demonstrates that specific control of the chemistry of synthetic polymer modifications is advantageous for adjusting mucus penetration.
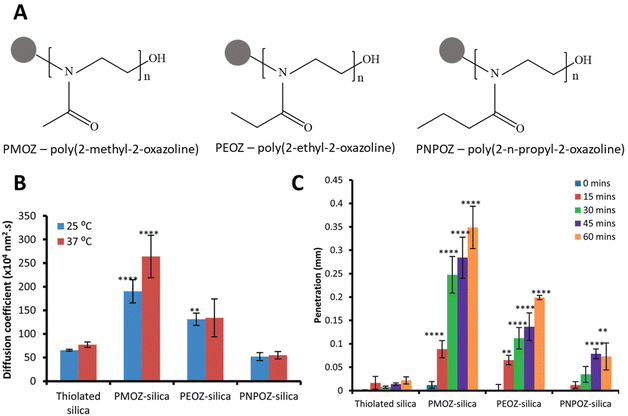 |
| Fig. 4 (A) Schematics of poly(2-alkyl-2-oxazoline) polymers attached to silica nanoparticles (B) diffusion coefficients of modified and unmodified silica nanoparticles through 1% w/v gastric mucin dispersion at 25 °C and 37 °C measured using nanoparticle tracking analysis. PMOZ demonstrated increased diffusion coefficients at both temperatures. (C) Mucus penetration of modified and unmodified silica nanoparticles over 1 hour on fresh porcine mucosa quantified by fluorescent microscopy of histology sections. Nanoparticles exhibited decreased penetration with increasing hydrophobicity of the side chain. (B) and (C) adapted from Mansfield et al., Biomater. Sci., 2016, 4(9), 1318 with permission from Royal Society of Chemistry. | |
4.4. Microneedle arrays
Microneedle arrays (MAs) are a tool used to effectively penetrate the epithelium and deliver drugs or vaccines in a pain-free manner. MAs typically contain needle-like structures less than 1 mm in length which can penetrate the epithelium. Microneedle delivery approaches have been most intensively investigated for transdermal delivery, although their application to mucosal routes (buccal, sublingual, and genital tract) have also received interest. Here we will provide a brief overview of how these materials can be used to deliver mucosal vaccines, which has been comprehensively reviewed elsewhere.68 MAs can be constructed from a wide variety of hard and soft materials, including but not limited to metals,69–71 synthetic polymers,72 hydrogels,73 and freeze-dried biomaterials.74,75 MAs have been used to penetrate both the mucus and epithelium in the oral cavity and genital tract.74 Antigen can be coated onto pre-existing needle structures or used to form the needle-like structures themselves.73–75 The use of MAs overcomes the challenges of mucus penetration by directly penetrating both the mucus and the epithelium to deliver antigen to APCs. Additionally, MAs control the dose of delivered antigen or drug, as none is lost to clearance by the mucus. Wang et al. demonstrated Mas delivering large (200 nm) and small (50 nm) liposomes for vaginal vaccine delivery.74 MAs were prepared by adding the liposomes into microneedle array inverse molds and subsequent lyophilization. Upon vaccination, the array was rehydrated, and liposomes are delivered directly through the mucosal epithelium. The authors found the large liposomes (mannosylated lipid A-liposomes, MLLs) were preferentially uptaken by mucosal resident dendritic cells. The small liposomes (PEGylated, or stealth, lipid A-liposomes, SLLs) however, were trafficked to draining lymph nodes to be picked up by macrophages. Upon vaginal vaccination with the engineered HSV2 antigen gD, mice were successfully protected from viral challenge when vaccinated with a MA compared to vaginal instillation without the MA, or intradermal microneedle delivery. The straightforward design of MAs provides a method for mucosal penetration which allows for the direct delivery of a variety of antigens to the mucosal epithelium with minimal re-design or functionalization of vaccine components, providing significant flexibility and applicability for a wide variety of antigens. This flexibility has also been exploited for applications in drug delivery, notably by the Prausnitz group; we direct the reader to their recent review on the use of MAs for drug delivery for a comprehensive description.76 MAs may be particularly useful in contexts where modification of antigen formulation is challenging, as the challenge of mucus penetration is overcome by the MA regardless of antigen formulation. However, MAs can only be placed in accessible areas, so they are challenging to apply to certain tissues such as the respiratory tract or gastrointestinal tract. For these tissues, other methods of mucus penetration may be better suited.
Each of the strategies discussed in this section represents an important tool to tune materials properties to facilitate material delivery through the mucus and uptake by immune cells to induce an immune response. Importantly, these factors do not exist in isolation, and many recent studies have sought to integrate multiple strategies to optimize material delivery. For example, positively charged cell penetrating peptides have been combined with alginate to enhance mucus penetration,77,78 and PEG and alginate have been combined to balance mucus penetration and adhesion.79 Additionally, materials of different classes may provide similar properties and benefits to achieve successful mucosal vaccination.80 Here we have discussed the ways in which incorporating a variety of biomaterials into a vaccine formulation can control the mucus penetration profile and enable mucosal vaccination.
5. Biomimetic and active mucus penetration strategies
As described above in section 3, pathogens use a variety of active strategies to locomote through mucus, concentrate at specific target cells, and achieve infection. In this section, biomaterial approaches for applying these strategies within active vaccine platforms will be discussed (Fig. 5), including technologies for self-propulsion, enzymatic disruption of mucus, ligand–receptor targeting, and various other pathogen-inspired approaches. In addition to these biomimetic active strategies, other stimuli-responsive materials such as photoactivatable adjuvants will also be addressed.81
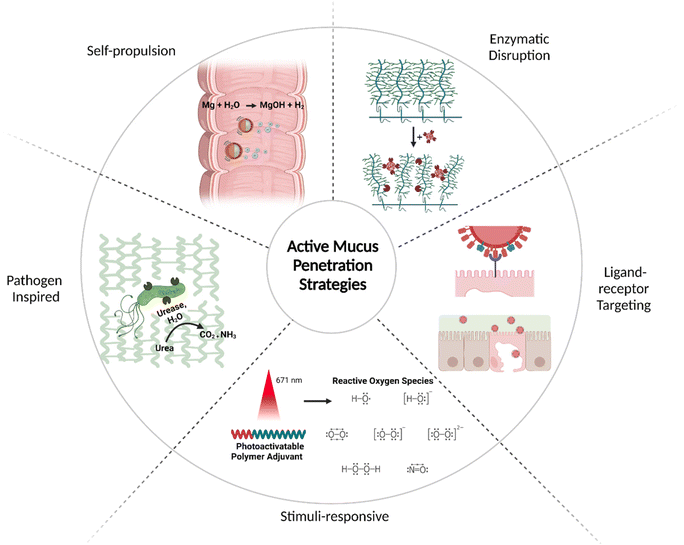 |
| Fig. 5 Active transport strategies for mucus penetration: self-propulsion,82 enzymatic disruption,83 ligand–receptor targeting,84 stimuli-responsive,81 and pathogen inspired (clockwise).85Created with BioRender.com. | |
5.1. Self-propulsion
Micro- and nanomotors have emerged as an active platform to improve delivery, target specific locations, and enhance tissue penetration. They utilize fuel sources from biological fluids, enzyme activity, or are remotely controlled to generate directional movement that propel cargos to a target tissue.86,87 In the context of mucosal delivery, micromotors have been recently explored as vehicles for the active delivery of cargos to the GI tract mucosa through propulsion and modulation of the mucus barrier.82,85,88–90
Zinc and magnesium are actively pursued as structural components of self-propelling micromotors, organized in an asymmetric fashion to achieve directional motility. Propulsion with zinc-based motors is achieved in acidic conditions, where zinc reacts with H+ ions to form Zn ions and release hydrogen gas. Since this system relies on an acidic environment, Zn-based motors have been explored particularly for gastric delivery. For example, a poly(3,4-ethylenedioxythiophene) (PEDOT)/Zn micromotor was developed as an acid-powered delivery system (Fig. 6a).88 PEDOT was deposited onto a porous cylindrical template, and subsequently Zn was deposited in the interior of the bilayer tube.88 In an acidic pH environment, the reaction with H+ ions propelled the motor at a speed of 60 μm s−1 for up to 10 minutes. In a mouse model, retention of the PEDOT/Zn motor in the stomach was enhanced when compared to a PEDOT/Pt tube that cannot self-propel, indicating the propulsion enhanced penetration of gastric mucus and improved tissue retention. While natural pathogens do not utilize Zn chemistry to propel themselves, this bioinspired micromotor propulsion mimics flagellar motility, as both use a source of “fuel” (albeit from different sources) to generate motion. To assess the ability of the micromotor to deliver gold nanoparticles as cargo were delivered via the PEDOT/Zn tubes. No toxicity or damage to the tissue was observed, and gold nanoparticles showed increased retention in mice's stomachs after 12 hours, indicating efficient mucus penetration. Similarly, a Mg-based PEDOT cylindrical motor has been employed for delivery in the intestines89 Unlike zinc, which generates hydrogen gas in acidic environments, magnesium reacts with water to produce MgOH and hydrogen gas, which allows for self-propulsion in neutral-to-basic environments such as the intestines. In the design of micromotors for intestinal delivery, enteric polymers and chitosan have been incorporated in the motor design.82,89 The commercial Eudragit L100-55 is an enteric polymer commonly used as a protective layer that prevents degradation by stomach acid, while chitosan promotes mucus adhesion as discussed in previous sections. By varying the thickness of the enteric coating, Mg micromotors can be activated at different portions of the intestine for specific targeting to the ileum, duodenum, or jejunum, by exposing the Mg core as the coating degrades.89
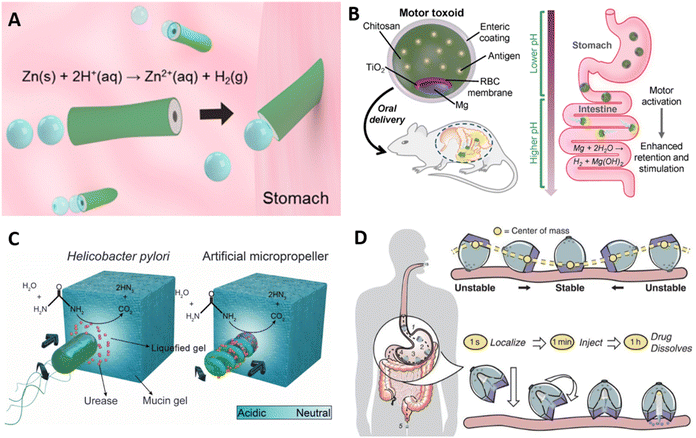 |
| Fig. 6 Self-propulsion material strategies for mucus penetration. (A) PEDOT/Zn micromotor evolves H2 gas in acidic environments, propelling the device through gastric tissue. Adapted with permission from Gao et al., ACS Nano, 2015, 9, 1, 117–123. Copyright © 2014 American Chemical Society (B) schematic of enteric Mg-based micromotor for oral vaccination, propelled by Mg hydrolysis. Adapted with permission from Wei et al., Nano Lett., 2019, 19, 1914–1921. Copyright © 2019 American Chemical Society (C) H. pylori mucus-penetrating mechanism and its artificial micropropeller counterpart. Hydrolysis of urease locally increases pH and liquifies mucin glycoproteins. Adapted with permission from Walker et al., Sci. Adv., 2015, 1, 11. Copyright © 2015, The Authors. (D) SOMA capsules self-orient and inject payload in a time-dependent manner in the stomach. Adapted with permission from Abramson et al., Science, 2019, 363(6427), 611–615. Copyright © 2019, The Authors. | |
Translating micromotors in vivo has been an important advancement in the field,88 however these systems have primarily employed model cargos such as gold nanoparticles or dyes to date, and specific disease-focused translation is still forthcoming. Wei et al. recently demonstrated the use of a biomimetic micromotor for oral vaccine delivery (Fig. 6b).82 Mg was asymmetrically coated with a thin TiO2 layer, the TiO2 layer was coated with a red blood cell membrane decorated with Staphylococcal α-toxin as the model antigen, then chitosan and enteric polymer. Importantly, the Mg core remained partially uncoated until encapsulation with the enteric polymer. As the enteric coating encounters a more neutral pH in the intestines, it dissolves exposing the asymmetric Mg core. Asymmetric generation of Mg(OH)2 and H2 gas then creates directional motion. Compared to a static version of the particle in which the Mg core was completely coated with TiO2 and could not propel, the self-propelled formulations increased antigen retention in the intestinal walls and increased fecal IgA levels. The approach also had the benefit of benign products and components: hydrogen is naturally produced as a product of fermentation in the intestines and is further metabolized or otherwise excreted through breath or flatus.91 Zinc is present in our diets, and MgOH is a commonly used antacid and laxative.92 While this approach was promising for generating mucosal immune responses, it remained unclear how the micromotor system compared to the efficacy and translatability of other simpler material platforms for oral vaccination.
Micromotor strategies have also been developed for the modulation of the mucus barrier. Walker et al. have designed a micromotor inspired by the Helicobacter pylori mechanism, discussed above in section 3, for gastric mucus penetration in vitro.85 Urease was immobilized on the surface of a helical magnetic motor, and propulsion and directional motility was assessed in a mucin gel model (Fig. 6c). Urea hydrolysis via urease releases ammonia, locally increasing the pH, and mucin glycoproteins that form viscoelastic gels in acidic environments are liquified in neutral or basic conditions. Without urease activity, the motors rotated under a rotating magnetic field but could not propel forward due to entanglement within the glycoprotein network. Efficient propulsion was observed in neutral pH mucin gels and in slightly acidic pH (4.5) under a magnetic field. Further studies will hopefully investigate motility under physiologically relevant conditions, as the physiological gastric pH falls between 1.5 and 3.5. Additionally, in vivo studies will clarify how this system may function in the stomach, the degree to which it may enhance antigen or drug uptake, and whether any of the components or byproducts exhibit unacceptable toxicity. However, the concept is intriguing and exemplifies an approach inspired by pathogen motility.
In addition to motors in the micro- and nanoscale, there are millimeter-scale devices that are able to penetrate the mucosa and deliver cargos. Recently, a self-orienting capsule (SOMA) that injects its drug payload into the gastric mucosa has been developed for the oral delivery of macromolecules.93,94 Unlike other micromotors that rely on chemical and enzymatic reaction for self-propulsion, this system is inspired by tortoises and their ability to self-orient based on their center of gravity (Fig. 6d). The millimeter-scale capsule comprises a heavy, stainless-steel bottom and a hollow low-density polycaprolactone shell containing a needle and the payload. In the stomach, it orients itself with gravity such as the bottom of the capsule is aligned with the stomach wall. A stainless steel spring providing 1.7 to 5 N of force is then triggered to insert a needle into the gastric mucosa. Gastric acid dissolves the coating of sucrose and isomalt (a sugar alcohol) that acts as a hydration-dependent actuator.93 The device was tested in a porcine model, both ex vivo and in vivo with fasted animals, and through endoscopy it was found that SOMA capsules self-oriented with a high degree of efficiency, while the control capsules, composed of only the hollow low-density polycaprolactone shell, had only 50% of success. This allows for the conclusion that contractility and movement of the stomach did not adversely affect capsule orientation. SOMA capsules have been used to deliver clinically relevant macro and small molecules, namely mRNA, Adalimumab, insulin, GLP and epinephrine.94,95 Therefore, this innovative platform provides a unique approach for the delivery of protein, nucleotide, and peptide vaccines, although the immunogenicity of vaccines delivered through this platform has yet to be investigated.
5.2. Mucosal disruption
Another active strategy to enhance mucosal delivery is the degradation of the mucus layer or the underlying epithelium to allow cargo transport. The first can be achieved via the incorporation of mucolytic enzymes into nanomaterials to locally disrupt the mucus matrix. Papain and bromelain, plant-derived cysteine proteases with mucolytic activity, have been investigated due to their ability to use cysteine residues to catalyze the cleavage of amino acids in mucoglycoprotein structures. This mechanism is analogous to the processes used by some protozoans to infect the gastrointestinal mucosa.25 Both enzymes locally destroy cross-linking density, increasing the mobility of the carriers and of the mucus itself.83 Müller et al. first functionalized papain into poly(acrylic acid) (PAA) nanoparticles through electrostatic interactions between the positively charged protein and negatively charged polymer.96 The resulting nanoparticles exhibited improved transport and diffusion in vitro, reduced mucus viscoelasticity, and the ability to be loaded with a model hydrophilic cargo. A rat model was subsequently used for assessing the oral delivery and mucus penetration of papain-PAA particles. A higher percentage of papain-functionalized particles were found to be retained in the proximal region of the intestines compared to plain PAA particles.97 While the incorporation of papain improved mucus penetration and particle absorption, for vaccine applications material parameters such as the thickness of the enteric polymer coating could be adjusted for improved delivery to key sites, such as the Peyer's patches in the ileum.
Papain-PAA nanoparticles were later compared with PAA functionalized with bromelain, a pineapple-derived enzyme better tolerated by humans and with fewer recorded cases of allergic reactions in the literature.83 Bromelain exhibited higher loading and enzymatic activity when functionalized to PAA, higher mucus permeation, and increased the diffusion coefficient of mucus, indicating a decrease in cross-linking density. In addition to mucolytic activity, bromelain also possess immunomodulatory activity.98,99 Inhibition of NFκ-B and COX-2 contributed to its anti-inflammatory effect, as well as the upregulation of PGE-1, an anti-inflammatory molecule. Additionally, the enzyme promoted activation of pro-inflammatory factors IL-1β, IL-6, TNF and IFN-γ in acute cellular stress, and it downregulated them in states of overt cytokine expression.100 Bromelain also has been shown to modulate T cell responses. While stimulating T cell-dependent, antigen-specific B-cell responses in vivo, the enzyme was shown to reduce IL-2 production and T cell activity without affecting CD4 proliferation by blocking Ras-1/Erk-2 signal transduction pathways.98 While multiple immunomodulatory responses to bromelain have been observed, the full mechanisms behind this ability to engage such responses remain to be fully understood. As these are unraveled, combining bromelain's mucolytic activity with immune functions may be explored in the development of vaccines and immunotherapies.
Other nanoparticle platforms have also explored proteases to enhance mucus penetration. Enzyme immobilization on PLGA nanoparticles provided increased stability of trypsin, papain and bromelain.101 The two latter exhibited increased mucus permeation in vitro in comparison to trypsin-PLGA and bare PLGA particles. Additionally, Pulsed-Field Gradient Spin-Echo NMR measurements showed an increased diffusion coefficient of mucus when incubated with enzyme-functionalized PLGA particles versus bare particles, indicating that enzymatic activity affected the physical properties of the mucus gel. Similar results were observed in a self-emulsifying drug delivery system composed of surfactants (Cremophor EL and Tween 80), and oily phases (Miglyol 840 and Labrafac TM Lipophile WL 1349) mixed at different ratios. Hydrophobic ion pairing of papain was performed to increase hydrophobicity and allow for enzyme loading.102 Formulations with ion pairing remained to a higher extent in the mucosa of pig intestines in an ex vivo assay, which correlates higher papain loading with increased mucus permeation.
On a different note, cell penetrating peptides (CPP) have been widely employed to disrupt the epithelium and deliver cargoes. Although the exact mechanism through which they work remains incompletely understood, suggested pathways include direct translocation by binding and forming pores in the cell membrane, endocytosis followed by transcytosis, or paracellular dislocation via interaction with tight junctions.103 Polyarginine in particular has been employed in mucosal vaccines to facilitate transport of macromolecules across the epithelium for oral delivery. In one example, the antigen OVA was coated with a 12-residue polyarginine (R12) before functionalization with PEG.78 The polymer's interaction with mucus was essential for the penetration of the mucus layer in vitro, while the incorporation of R12 enhanced transport through an epithelial cell monolayer. The complete material formulation decreased the transepithelial electrical resistance, a measure of integrity of tight junctions. While this facilitates penetration of the epithelium, the strategy might not be beneficial for the integrity of the tissue, although further examination is required for a complete understanding. In vivo, PEG-R12-OVA increased uptake in Peyer's patches, leading to heightened systemic (IgG) and mucosal (IgA) B cell responses in the GI and reproductive tracts, as well as IFNγ production.78 It also had a protective effect against an H. pylori challenge, reducing inflammation and the bacterial load in the GI tract. Although PEG-OVA alone was not tested, this study was a successful example of a multi-component material for penetration of both the mucus gel and the epithelium, as the latter is sometimes underprioritized in other mucus-penetration material designs.
Similarly, in our group, a 9-residue polyarginine (R9) has been used to enhance the adjuvanticity of epitope-bearing peptide nanofibers delivered sublingually.59 The self-assembled peptide-polymer platform PEG-Q11 was co-assembled into integrated nanofibers containing R9 and cyclic-dinucleotide (CDN) adjuvants. Polyarginine can be electrostatically assembled with CDNs, with which the guanidium side chain of arginine forms bidentate hydrogen bonds. Independently of adjuvant complexation, PEG-Q11/R9 enhanced DC uptake in vitro, as well as epitope presentation in MHCII to T cells. When PEG-Q11/R9 fibers were complexed with two CDN adjuvants, c-di-AMP and c-di-GMP, a synergistic effect was observed in vitro, with enhanced DC activation compared to PEG-Q11/R9 or CDNs alone, in a dose dependent manner when titrating R9 into the fibers. This result is specific to the R9/CDN interaction, as when polylysine (K9) was titrated into the fibers, theoretically with the same charge as R9, this synergistic effect was not observed, nor when the toll-like receptor 9 (TLR9) agonist CpG was co-administered, as this adjuvant is not expected to complex with R9. The platform was subsequently administered as a sublingual vaccine in mice, with a peptide epitope targeting M. tuberculosis. Vaccination raised T cell responses, serum antibody responses, and antibodies in the bronchoalveolar lavage fluid, but the improvements seen in vitro by adding R9 were lessened in vivo, and an intermediate concentration of R9 maximized immunization. Our hypothesis was that the positive charge of the fibers promoted mucoadhesion, a countervailing factor for improving vaccination. Optimal formulations possessed sufficient R9 for adjuvant complexation but without excess R9 to enhance mucoadhesion. This study illustrates that for complex multicomponent vaccines, a modular construction facilitates balancing of multiple factors and their interactions, which may be complexly dose dependent.
5.3. Receptor targeting
To facilitate transport through the mucosal epithelium, the targeting of key receptors has received active interest. For example, M cells are responsible for the rapid uptake and presentation of antigens from the lumen to the mucosa-associated lymphoid tissue (MALT), and therefore are promising targets in oral vaccine delivery.104 Different M cell ligands have been identified and employed to enhance transcytosis through M cell-specific binding.84,105–107 The Cho group identified the M cell peptide ligand CKS9 (CKSTHPLSC) through phage display and integrated it into a complex polymeric material construct for an oral vaccine against swine dysentery.84,105 The antigen, membrane protein B of Brachyspira hyodysenteriae (BmpB), was loaded into PLGA microparticles. The particles were then coated with water soluble chitosan (WSC) conjugated to CKS9 to create BmpB-CKS9-WSC-PLGA. As expected, the WSC component enhanced mucoadhesion and retention at the mucosa, therefore maximizing M cell interaction. In addition, the complete formulation elicited the highest sIgA titers in both the intestines and feces, as well as higher IgG titers over the course of 28 days in a murine model. The ligand was also employed in a thiolated enteric polymer by the same group.108 The synergistic effect between mucoadhesive polymer and the M-cell homing peptide enhanced cell uptake and led to a stronger B and T cell response in mice (Fig. 7a).
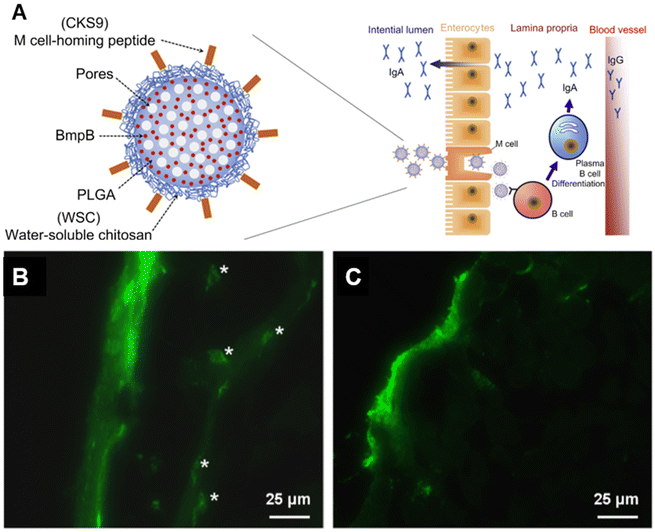 |
| Fig. 7 (A) Microparticles conjugated with M cell-homing peptide and antigen are uptaken by M cells in the intestinal lumen. Adapted from Liu et al., Biomaterials, 2014, 35(7), 2365–2373, with permission from Elsevier. All rights reserved. (B) C1q/CD91/CD40L targeting enhances VLP transport to the sublingual submucosal space and binding to DCs. (C) compared to CD40L/HIV VLPs without C1q ligand. (B) and (C) were adapted from Liu et al., Vaccines, 2021, 9(11), 1236. Copyright © 2021, The Authors. | |
Liu et al. have recently explored the ligand–receptor interaction to enhance mucosal penetration via the sublingual route.109 Epithelial cells in the human salivary glands highly express CD91, which is a receptor to the C1 complement subcomponent C1q. C1q binds to antigen–antibody complexes, activating C1 and initiating the classical complement pathway. The ligand was incorporated into chimeric C1q/CD40L/HIV virus-like particles (VLPs), which were used in sublingual vaccination to elicit mucosal immune responses against HIV in mice. Enhanced penetration of the sub-mucosal tissue was observed, as well as enhanced binding of the VLPs to DC-like cells in the submucosal space (Fig. 7b and c). VLPs containing the C1q component elicited higher titers of salivary IgA, enhanced IFN-γ T cell responses, lymphocyte homing to the gut, and DC activation. Interestingly, there were no significant changes in IgG levels compared to CD40L/HIV VLPs. Others have developed an exosome-based vaccine that share proteins and ligands with the target epithelial tissue, while mimicking the structure of SARS-COV-2.110 Along with other SARS-COV-2 mimetic structures, this will be discussed in the next section.
5.4. Other forms of pathogen-inspired mucus penetration
Another organism that has inspired vaccine delivery are bacteriophages, viruses that infect bacteria and are part of the human microbiome, colonizing the mucosal surfaces of the respiratory and GI tracts. Phages have been employed in vaccine delivery, as they can display proteins and peptides in the capsid and even encapsulate nucleic acids for DNA and RNA vaccines.111 They are stable in the GI tract and concentrated in mucus, making them a promising vehicle for oral vaccine delivery.112 Ig-like domains on phage capsids also bind to mucin glycoproteins, augmenting their mucoadhesive properties which are necessary for mucosal delivery.113,114 An oral lambda phage vaccine has been designed to display multiple peptide epitopes fused to the capsid head protein.115 Particle uptake in small intestines was shown to be dependent on MALT. In newborn calves, a dose-dependent antigen specific antibody response was observed in the Peyer's patches, with mostly IgA secreting cells and some IgG response. IgA responses were induced against three different peptide epitopes from a deer prion in the absence of adjuvant. This study highlights the promise of phages as a mucosal delivery vehicle that can adhere to mucus, trespass the epithelium via M cell activity, and induce mucosal immune responses.
Yeasts have also been explored for mucosal delivery. The unicellular organism can be used to encapsulate or display antigens, and its resistance to digestion in the stomach makes yeast an attractive oral carrier.26 β-Glucan is a primary component of the yeast cell wall, which is recognized by pattern recognition receptors such as dectin-1, that is present in APCs and M cells in the intestinal epithelium, thus enhancing acquisition and activation.26 Yeast is a simple, low cost, and non-pathogenic eukaryotic vehicle that can encapsulate or display antigens. Bal et al. combined the Dengue virus protein scEDIII along with the M cell targeting ligand Co1 in a recombinant S. cerevisiae strain expressing the protein on the cell surface.116 Oral immunization with the Co1-scEDII-AGA construct induced enhanced systemic and mucosal antibody responses compared to controls, demonstrating the potential in combining yeast wall carriers with targeting motifs to enhance mucosal uptake. Another study modified yeast capsules with the cationic polymer polyethyleneimine (PEI) to enhance the packaging and delivery of pseudovirus bearing the SARS-CoV-2 spike protein.117 Due to the negative charge of both the yeast and virus, antigen packaging was limited, so the charge modification of the wall allowed for more efficient loading and neutralized the particle, therefore enhancing mucus penetration.
Towards intranasal delivery, respiratory viruses provide inspiration for biomaterials-based vaccines. The SARS-CoV-2 spike protein targets the ACE2 receptor, present on alveolar epithelial cells, macrophages, and monocytes in the lung. In a strategy to exploit this pathway in vaccination, Lawanprasert and coworkers designed COVID-19 mimetic particles (CoMiP) to be rapidly phagocytosed by alveolar macrophages and deliver antigen-encoding plasmids to the mucosal surface of the respiratory tract.27 The nucleic acid cargo was electrostatically assembled with a poly-L-lysine peptide envelope to form a polyplex and was enveloped in a glycosaminoglycan coating of hyaluronic acid. The particles were able to successfully transfect macrophages with the four structural virus proteins (spike, envelope, membrane, and nucleocapsid), demonstrating the ability of the construct to transfect and induce production of several antigens. While CoMiP successfully induced antigen production in vitro, the in vivo humoral responses were weak, with an increase in total IgA production but no significance in antigen-specific IgG or IgA compared to saline. The authors hypothesized that the disconnect between in vitro and in vivo results may have been due to macrophage polarization effects. While hyaluronic acid is more likely to bind to cells with a higher CD44 expression (M1 phenotype), a lower expression of CD44, present in the M2 phenotype, favors intracellular particle uptake.118 Therefore, antigen production is lower in the cells that uptake CoMiP. The use of adjuvants or protein and peptide antigens may mitigate this effect.
Zheng et al. utilized a different approach to mimic the structure of SARS-CoV-2 for the design of an inhalable vaccine.119 In the lungs, in addition to mucus, alveolar epithelial cells also secrete surfactant that limits the entry of nanoparticles and hydrophilic molecules into the underlying epithelium. To surpass this additional barrier, a platform containing nucleic acid, capsid, and spike protein components of SARS-CoV-2 was designed. The first was made of poly(I:C), a synthetic double-stranded RNA adjuvant known to stimulate the TLR3 pathway. The capsid was made of biomimetic pulmonary surfactant liposomes (DPPC/DPPG/DPPE-COOH/Chol), with similar charge and composition to pulmonary surfactant, which encapsulated the poly(I:C) and displayed the receptor binding domains (RBDs) as the “spike” protein antigen. Surfactant proteins A and D were shown to be crucial for entry in macrophages by interacting with the material. In a mouse model, the intranasal administration of the complete construct led to increased immune activation, characterized by higher levels of IFN-β, IFN-γ and TNF production, increased antigen presentation, and increased anti-RBD IgG and IgA titers. Upon pseudovirus challenge, groups immunized with the negatively charged biomimetic vaccine showed enhanced immune activation and better protection compared to positively charged liposomes and particles not containing antigen.
Similarly, exosomes decorated with RBD were developed as another intranasal vaccine platform against COVID-19.110 Exosomes are extracellular vesicles that exhibit attractive targeting potential to the recipient cells through interactions between surface adhesion proteins. For example, exosomes derived from lung tissue share many protein ligands and receptors with the airway epithelium, leading to enhanced lung distribution and retention compared to liposomes, making the platform a promising vehicle for pulmonary delivery. Cheng and coworkers designed exosomes with RBD on the surface to produce RBD-Exo. Delivered intranasally, the particles elicited a Th1-biased response characterized by antibodies predominantly of the IgG2a subclass and higher frequency of CD4+ and CD8+ IFN-γ producing T cells, conversely to when RBD-Exo were delivered intravenously, which led to a Th2-biased responses. Vaccination with RBD-Exo elicited a strong systemic and mucosal humoral response, as well as a protective effect against SARS-CoV-2 and cross-protection against a more infectious variant. The platform was also shown to be stable at room temperature for over 3 months, a feature that can potentially address the supply chain issues associated with COVID-19 vaccines currently in the clinic.
To summarize, a variety of microorganisms colonize and penetrate the mucosal barrier, and by rationally designing materials to mimic their strategies, successful penetration of mucus and epithelium, as well as the induction of immune responses, can be achieved (Table 1). Both the direct use of microbes and microbial components as well as biomaterials inspired by these structures have seen advancement in recent years.
Table 1 Biomimetic material strategies inspired by pathogens with mucus penetrating mechanisms. Created with BioRender.com
Organism |
Mechanism |
Biomimetic strategy |
|
Inject RNA or DNA into cell. Protein capsid encapsulates RNA or DNA, which are injected into the host cell. Ig-like domain on capsid can bind to mucin glycoproteins |
SOMA capsules inject payload into epithelial cells via a hydration-dependent actuator93,94 |
Utilizing phage structure in an oral lambda display phage vaccine. Multiple peptide epitopes are fused to the capsid protein115 |
|
Spike, envelope, membrane and nucleocapsid proteins comprise the viral structure |
Biomimetic virus. Pulmonary surfactant liposome mimics the capsid, encapsulates the synthetic double-stranded RNA poly(I:C) and displays RBDs as the spike protein119 |
Spike protein targets the ACE2 receptor of alveolar epithelia, monocytes, and macrophages in the lung |
Covid mimetic particles (CoMiP). Poly-L-lysine encapsulates nucleic acid, subsequentially coated with hyaluronic acid27 |
|
β-glucan expressed on wall surface is recognized by dectin-1, a receptor on APCs and M cells in the intestinal epithelium, promoting uptake within Peyer's patches |
M cell peptide ligand CKS9 coating of polymeric materials84,108 and inclusion bodies120 |
Co1 M-cell ligand expressed with antigen on the surface of yeast116 |
Surface modification with the cationic polymer polyethyleneimine (PEI) to neutralize negative charge of yeast117 |
|
H. pylori synthesizes urease, which locally increases pH via the hydrolysis of urea to form ammonia. Mucin glycoproteins in the stomach are liquified in neutral or basic conditions. This facilitates propulsion of the bacteria by flagellar motion |
Self-propelling micromotors mimic force generated by flagella82,85,88–90 |
Helical magnetic micropropellers coated with urease. Propulsion observed under rotating magnetic field, only when urease activity occurred85 |
|
Secreted cysteine proteases cleave residues at the C-terminus of MUC2 monomers, depolymerizing mucin networks and facilitating pathogen entry |
PAA96 and PLGA101 nanoparticles functionalized with mucolytic proteases, bromelain and papain |
5.5. Stimuli-responsive
In combination with other mucus penetration strategies, stimuli-responsive materials can be employed to enhance delivery to the mucosa. External stimuli such as, pH, light and enzymatic activity can modify material properties in a temporal or spatial manner that facilitates mucosal vaccination.
Enteric polymer coatings have been extensively employed in materials for oral delivery to bypass the variable pH of the GI tract and reach the intestines.82,89,96,108 Many technologies employ commercially available trademarked enteric polymers, which are stable at an acidic pH but degrade and release cargo at neutral to basic pH. Varying the thickness of the coating can help target specific parts of the intestine such as Peyer's patches for vaccine delivery. For the design of nano and micromotors, an enteric coating has been applied to protect the Mg core until the material reaches the intestines, only then allowing the reaction with water to take place for self-propulsion.89 pH-sensitive polymers can also be combined with a mucoadhesive moiety for oral vaccine delivery, shielding the carrier from the harsh gastric environment while maximizing residence time in mucus. Lee et al. combined cellulose acetate phthalate (CAP), a pH responsive material soluble only above pH 6.2, with a thiolated polymer (T) that covalently binds to the cysteine-rich mucus glycoproteins.121 The platform was used in an oral vaccine against foot and mouth disease (FMD) by creating CAP-T microparticles loaded with antigen. Antigen release was significantly higher in simulated intestinal fluid compared to gastric fluid, and CAP-T particles exhibited increased mucoadhesive properties to porcine mucus. In vivo, higher titers of antigen-specific IgG were observed, as well as relative and fecal IgA, in comparison to the antigen alone or non-thiolated CAP carriers. This study demonstrated the synergistic effects of pH sensitive and mucoadhesive material strategies to enhance mucosal and systemic vaccine responses.
Jeong et al. designed a photo-responsive material platform, NanoVac, for intranasal delivery of an influenza vaccine.81 It consists of the influenza antigen HA electrostatically self-assembled with the photoactivatable polymeric adjuvant (PPA) (poly[(2-aminoethyl)aspartamide]-chlorin e6), that is stimulated with a light source applied to the nose after administration (Fig. 8a). The positive charge of the polymer increased mucoadhesion, and when stimulated with a 671 nm laser, the photosensitive Ce6 generated ROS that enhanced mucus penetration and modulated immune responses. When administered intranasally, fluorescence intensity in vivo and histological analysis showed a prolonged residence time in the nasal cavity for animals immunized with HA-NanoVac and exposed to laser irradiation, compared to free HA and no laser exposure. Additionally, irradiated HA-NanoVac enhanced immunogenicity. An increased count of mature DCs, CD4+ and CD8+ T cells, plasma and memory B cells was observed, as well as higher levels of IL-2. The ability of the NanoVac to induce antigen-specific responses was also investigated. IFN-γ production by splenocytes was higher in irradiated HA-NanoVac groups compared to free HA and to the non-irradiated counterpart. An increase in HA-specific CD8+ T cells, serum IgG and secreted IgA in the nasal mucosa was also observed with the complete formulation. Finally, when mice were exposed to a lethal influenza viral challenge, all animals immunized with irradiated HA-NanoVac survived, in contrast with all other groups that had at least 40% of animals reach the experimental endpoint before full recovery.
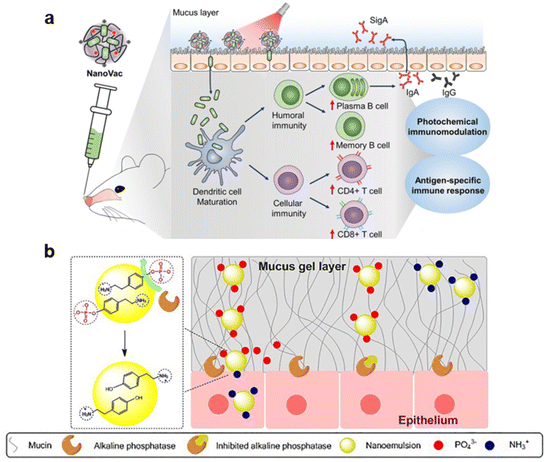 |
| Fig. 8 Stimuli-responsive materials to enhance mucosal vaccine delivery (A) electrostatically self-assembled HA and PAA polymeric adjuvant particles are stimulated by a light source, generating ROS that modulate immune responses. Adapted with permission from Jeong et al., Adv. Sci., 2021, 8, 2100118. Copyright © 2019, The Authors. (B) pTyr nano emulsions undergo charge reversal as the enzyme alkaline phosphatase cleaves phosphate groups and exposes amines. Adapted from Sharifi et al., J. Colloid Interface Sci., 2021, 585, 126–137, with permission from Elsevier. | |
Enzyme activity can also provide stimuli to alter material properties and enhance mucus penetration. For example, the insertion of an enzyme-cleavable moiety has been employed in polymeric nanoparticles for a ζ-potential-changing system.122–124 As discussed previously, charge has a significant effect in mucus interactions. Particles exhibiting a neutral to slightly negative charge penetrate mucus, however, positively charged particles are preferred for delivery and transport across the epithelial layer. Due to this paradoxical nature, particles that can transiently alter surface charge are attractive. Bonengel et al. linked 6-phosphogluconic acid to polyethylene imine and assembled the polymer with carboxymethyl cellulose in a poly-ionic complex.122 This approach relies on the brush border membrane bound alkaline phosphatase, an enzyme that dephosphorylates materials as they penetrate the mucus layer. The zeta potential changed from −6.44 mV to +2.8 mV after incubation with the isolated enzyme. By incubating particles with a Caco2 cell monolayer, fast phosphate release was observed up until 20 minutes, with a slow-release profile observed afterwards. While this study proved the principle of the zeta potential-changing system, mucus penetration remained to be investigated both in vitro and in vivo. Another reported approach consists of phosphorylated tyramine (pTyr) nanoemulsions for charge reversal (Fig. 8b).124 Successful formulations had a zeta potential that switched from −8.40 mV to 1.29 mV, with an abrupt increase in charge 1 hour after incubation with enzyme. In a transwell assay, pTyr particles permeated porcine mucus faster and to a greater extent than controls, and they were also acquired by Caco2 cells to a greater extent than when a phosphatase inhibitor was administered.
Although the application of these systems directly in vaccine delivery remains to be investigated, in vivo studies involving the oral delivery of insulin suggest translational potential for these materials.123 A biomimetic VLP with charge reversal abilities, P-R8-Pho, was designed by combining an 8-residue polyarginine (R8), with the anionic phosphoserine that is a substrate of alkaline phosphatase. The two components were conjugated to the surface of PLGA nanoparticles to obtain a densely charged but neutral surface. P-R8-Pho particles exhibited less aggregation and increased diffusion in porcine mucus, a switch from −1.87 mV to +7.37 mV, and increased cellular uptake in a Caco-2/E12 co-culture. In vivo, when administered orally, fluorescent P-R8-Pho particles exhibited the highest fluorescence intensity in histology sections of intestinal villi. Importantly, insulin-loaded P-R8-Pho nanoparticles successfully decreased blood glucose by 32%, and insulin was retained for longer than when freely administered.
6. Practical aspects for translation, remaining hurdles, and outlook
We have discussed strategies in biomaterial design to address the challenges of mucosal vaccine delivery, specifically the penetration of the mucus layer and epithelium to reach the target immune cells.
Physicochemical properties of materials play a large role in delivery, so it is crucial to understand aspects such as charge and hydrophobicity in material platform design. However, as noted in several examples above, they are difficult to independently isolate and experimentally control, multiple physicochemical features may exhibit unpredictable interactions, and these interactions may vary for different antigens and platforms. For example, charge modulation is usually addressed by employing different polymer chemistries and is a difficult phenomenon to study without introducing other factors that might affect mucosal delivery, such as altered hydrophobicity. A modular platform, in which charged polymers can be titrated, is advantageous for studying the influence of such factors. Kelly et al. were able to titrate different amounts of polyarginine into peptide nanofibers, increasing the amount of positive charge and finding that intermediate amounts of the polypeptide led to enhanced immune responses in vitro.59 This provides insight into the balance necessary to overcome the mucosa; while cell penetrating peptides enhance epithelial uptake, excess positive charge would prevent penetration of the negative charged mucus gel through excessive mucoadhesion. Similarly, to study mucoadhesion, the introduction of amine and thiol groups into chitosan is a straightforward approach for increasing mucoadhesion without completely changing the polymer platform.45 These modifications increase interaction with mucins, increasing adhesion, and lead to enhanced immune responses. It would be interesting to explore titrations of these functional groups to identify the tipping point where adhesion overpowers penetration, and whether such relationships hold true for different antigens.
It is also important to recognize some limitations of these technologies with respect to their immunogenicity and intended applications. As we have highlighted in this review, biomaterials are being more commonly used in applications where the generation of an immune response is the desired outcome, yet we have limited understanding of the inherent immunogenicity of many biomaterials. For example, while PEG has regulatory approval for a variety of applications and is widely used in pharmaceuticals, the generation of antibodies against PEG has recently gained increasing attention.63 Chitosan is also well understood to elicit an immune response, as it is used both to control mucus penetration and as a STING adjuvant.38,40 Other biomaterials may also be immunogenic; for example papain-functionalized particles can elicit allergic reactions, as cases of hypersensitivity to the enzyme have been reported when orally administered.125 Bromelain may be a better protease alternative, as fewer allergic responses have been documented to date.83 For yeast-mimicking materials, β-glucan ligands will induce PAMP-related inflammatory responses,26 triggering the release of inflammatory cytokines, which can be beneficial for raising protective immune responses against infectious agents. However, in the context of active immunotherapies against inflammatory diseases, enhanced inflammation would be detrimental. The same principle applies for the use of photoactivatable polymeric adjuvants, which induce the production of ROS.81 The immunological responses to many biomaterials discussed in this review are not fully understood, representing an important area for ongoing research. This is of particular importance for biomaterials used within vaccines and active immunotherapies, where it is crucial to balance inflammation with the immune response desired.
If isolating physicochemical material properties is already challenging, this becomes a larger issue when designing complex, biomimetic platforms. Often these may seem like a “Rube Goldberg machine”, overcomplicated materials with too many components to be practical. However, given the challenging nature of mucosal delivery, a multifactorial approach may be necessary to raise significant immune responses. Micromotors, for example, leverage self-propulsion via hydrolysis reactions in asymmetric constructs.82,88 Elegantly, by coating the reactive core, or switching it to an inert element, self-propulsion can be isolated as an influential factor that enhances immunogenicity. However, other components of these carriers may also have unpredictable influences on micromotor effectiveness, at it remains to be understood whether various polymer coatings influence properties such as antigenicity, trafficking, interaction with adjuvants, and so on. Walking the line between oversimplified and overcomplicated platforms is important, and the balance may be clarified through multifactorial investigation of the interplays between all factors. Then, the most influential components can be combined in efficacious platforms that utilize multiple strategies while minimizing complexity. For example, it may be found be that a mucoadhesive polymer coating is not necessary in micromotor-based vaccines, if the particles are able to propel deep enough into the tissue. Investigating these trade-offs will be an important part of translating such platforms.
Hurdles also remain for the translational aspects of such technologies. Many of the strategies hereby discussed have only been shown to be successful in vitro, penetrating a mucus gel or a mucus-secreting cell layer, and have yet to be explored in pre-clinical animal models. Therefore, assessing efficacy of such delivery systems in an in vivo setting is a crucial first step towards translatability, not only proving that they are able to deliver cargo to the mucosa but highlighting how they compare to current gold standards or individual material components in the case of complex delivery constructs. A clearer understanding of the clearance mechanisms, safety profile, dosing, pharmacokinetics, and toxicity of these materials will also need to be developed, especially in contexts where diverse material and biological components are present.
Although simplicity is a maxim of biomedical technology development, the world of pathogens suggests that some degree of complexity is important, as most pathogens use multiple overlapping strategies to penetrate the mucosa, reach the underlying tissue, and infect it. Flagellar activity, enzymatic disruption of mucins, local modulation of mucus properties, and ligand–receptor targeting are all strategies that are used in combination by pathogens and could be controlled using biomaterials. As the field of vaccinology is embracing the need for mucosal immune responses to successfully fight pathogens at the point of entry, overcoming the mucosal barrier will continue to receive increasing interest, and biomaterials platforms will continue to provide avenues for multifunctional approaches.
Conflicts of interest
JHC is an inventor on patents related to the topic of the review.
Acknowledgements
Related research in our group is supported by NIH 5R01AI167300.
References
- S. P. Bandi, S. Bhatnagar and V. V. K. Venuganti, Advanced materials for drug delivery across mucosal barriers, Acta Biomater., 2021, 119, 13–29 CrossRef CAS PubMed.
- V. V. Khutoryanskiy, Beyond PEGylation: Alternative surface-modification of nanoparticles with mucus-inert biomaterials, Adv. Drug Delivery Rev., 2018, 124, 140–149 CrossRef CAS PubMed.
- S. Ferber, R. J. Gonzalez, A. M. Cryer, U. H. von Andrian and N. Artzi, Immunology-Guided Biomaterial Design for Mucosal Cancer Vaccines, Adv. Mater., 2020, 32, 1903847 CrossRef CAS PubMed.
- J. D. Smart, The basics and underlying mechanisms of mucoadhesion, Adv. Drug Delivery Rev., 2005, 57, 1556–1568 CrossRef CAS PubMed.
- S. K. Lai, Y.-Y. Wang, D. Wirtz and J. Hanes, Micro- and macrorheology of mucus, Adv. Drug Delivery Rev., 2009, 61, 86–100 CrossRef CAS PubMed.
- P. R. Hoorens, M. Rinaldi, R. W. Li, B. Goddeeris, E. Claerebout, J. Vercruysse and P. Geldhof, Genome wide analysis of the bovine mucin genes and their gastrointestinal transcription profile, BMC Genomics, 2011, 12, 140 CrossRef CAS PubMed.
- N. Moniaux, F. Escande, N. Porchet, J. P. Aubert and S. K. Batra, Structural organization and classification of the human mucin genes, Front. Biosci. J. Virtual Libr., 2001, 6, D1192–D1206 CrossRef CAS PubMed.
- R. Shogren, T. A. Gerken and N. Jentoft, Role of glycosylation on the conformation and chain dimensions of O-linked glycoproteins: light-scattering studies of ovine submaxillary mucin, Biochemistry, 1989, 28, 5525–5536 CrossRef CAS PubMed.
- G. Lamblin, M. Lhermitte, A. Klein, N. Houdret, A. Scharfman, R. Ramphal and P. Roussel, The carbohydrate diversity of human respiratory mucins: a protection of the underlying mucosa?, Am. Rev. Respir. Dis., 1991, 144, S19–S24 CrossRef CAS PubMed.
- N. A. Peppas and J. J. Sahlin, Hydrogels as mucoadhesive and bioadhesive materials: a review, Biomaterials, 1996, 17, 1553–1561 CrossRef CAS PubMed.
- S. A. Mortazavi and J. D. Smart, An investigation into the role of water movement and mucus gel dehydration in mucoadhesion, J. Controlled Release, 1993, 25, 197–203 CrossRef CAS.
- E. Jabbari, N. Wisniewski and N. A. Peppas, Evidence of mucoadhesion by chain interpenetration at a poly (acrylic acid)/mucin interface using ATR-FTIR spectroscopy, J. Controlled Release, 1993, 26, 99–108 CrossRef CAS.
- G. Ponchel, F. Touchard and D. Duchqne, Bioadhesive analysis of controlled-release systems. Ill. Bioadhesive and release behavior of metronidazolecontaining poly(acrylic acid)-hydroxypropyl methyl cellulose systems, Int. J. Pharm., 1987, 65–70 CrossRef CAS.
- H. Kiyono and S. Fukuyama, NALT- versus PEYER'S-patch-mediated mucosal immunity, Nat. Rev. Immunol., 2004, 4, 699–710 CrossRef CAS PubMed.
- J.-P. Kraehenbuhl and M. R. Neutra, Epithelial M Cells: Differentiation and Function, Annu. Rev. Cell Dev. Biol., 2000, 16, 301–332 CrossRef CAS PubMed.
- V. Trincado, R. P. Gala and J. O. Morales, Buccal and Sublingual Vaccines: A Review on Oral Mucosal Immunization and Delivery Systems, Vaccines, 2021, 9, 1177 CrossRef CAS PubMed.
- C. Jung, J.-P. Hugot and F. Barreau, Peyer's Patches: The Immune Sensors of the Intestine, Int. J. Inflammation, 2010, 2010, 823710 Search PubMed.
- M. Johansson and N. Y. Lycke, Immunology of the human genital tract, Curr. Opin. Infect. Dis., 2003, 16, 43–49 CrossRef CAS PubMed.
- G. Lacroix, V. Gouyer, F. Gottrand and J.-L. Desseyn, The Cervicovaginal Mucus Barrier, Int. J. Mol. Sci., 2020, 21, 8266 CrossRef CAS PubMed.
- P. Das, M. N. Alam, D. Paik, K. Karmakar, T. De and T. Chakraborti, Protease inhibitors in potential drug development for Leishmaniasis, Indian J. Biochem. Biophys., 2013, 50, 363–376 CAS.
- M. Marco and J. M. Coteron, Falcipain Inhibition as a Promising Antimalarial
Target, Curr. Top. Med. Chem., 2012, 12(5), 408–444 CrossRef CAS PubMed.
- M. Ndao, C. Beaulieu, W. C. Black, E. Isabel, F. Vasquez-Camargo, M. Nath-Chowdhury, F. Massé, C. Mellon, N. Methot and D. A. Nicoll-Griffith, Reversible Cysteine Protease Inhibitors Show Promise for a Chagas Disease Cure, Antimicrob. Agents Chemother., 2014, 58, 1167–1178 CrossRef PubMed.
- C. B. Amat, J.-P. Motta, E. Fekete, F. Moreau, K. Chadee and A. G. Buret, Cysteine Protease–Dependent Mucous Disruptions and Differential Mucin Gene Expression in Giardia duodenalis Infection, Am. J. Pathol., 2017, 187, 2486–2498 CrossRef CAS PubMed.
- L. A. Baxt and U. Singh, New insights into Entamoeba histolytica pathogenesis, Curr. Opin. Infect. Dis., 2008, 21, 489–494 CrossRef PubMed.
- M. E. Lidell, D. M. Moncada, K. Chadee and G. C. Hansson, Entamoeba histolytica cysteine proteases cleave the MUC2 mucin in its C-terminal domain and dissolve the protective colonic mucus gel, Proc. Natl. Acad. Sci. U. S. A., 2006, 103, 9298–9303 CrossRef CAS PubMed.
- Y. Tan, L. Chen, K. Li, B. Lou, Y. Liu and Z. Liu, Yeast as carrier for drug delivery and vaccine construction, J. Controlled Release, 2022, 346, 358–379 CrossRef CAS PubMed.
- A. Lawanprasert, A. W. Simonson, S. E. Sumner, M. J. Nicol, S. Pimcharoen, G. S. Kirimanjeswara and S. H. Medina, Inhalable SARS-CoV-2 Mimetic Particles Induce Pleiotropic Antigen Presentation, Biomacromolecules, 2022, 23, 1158–1168 CrossRef CAS PubMed.
- E. de Vries, W. Du, H. Guo and C. A. M. de Haan, Influenza A Virus Hemagglutinin–Neuraminidase–Receptor Balance: Preserving Virus Motility, Trends Microbiol., 2020, 28, 57–67 CrossRef CAS PubMed.
- M. D. Vahey and D. A. Fletcher, Influenza A virus surface proteins are organized to help penetrate host mucus, eLife, 2019, 8, e43764 CrossRef PubMed.
- N. T. Q. Nhu, J. S. Lee, H. J. Wang and Y. S. Dufour, Alkaline pH Increases Swimming Speed and Facilitates Mucus Penetration for Vibrio cholerae, J. Bacteriol., 2021, 203(7), e00607–e00620 CrossRef CAS PubMed.
- J. P. Celli, B. S. Turner, N. H. Afdhal, S. Keates, I. Ghiran, C. P. Kelly, R. H. Ewoldt, G. H. McKinley, P. So, S. Erramilli and R. Bansil, Helicobacter pylori moves through mucus by reducing mucin viscoelasticity, Proc. Natl. Acad. Sci. U. S. A., 2009, 106, 14321–14326 CrossRef CAS PubMed.
- S. Kojima, K. Yamamoto, I. Kawagishi and M. Homma, The Polar Flagellar Motor of Vibrio cholerae Is Driven by an Na + Motive Force, J. Bacteriol., 1999, 181, 1927–1930 CrossRef CAS PubMed.
- C. Y. Dombu, M. Kroubi, R. Zibouche, R. Matran and D. Betbeder, Characterization of endocytosis and exocytosis of cationic nanoparticles in airway epithelium cells, Nanotechnology, 2010, 21, 355102 CrossRef PubMed.
- S. Hu, Z. Yang, S. Wang, L. Wang, Q. He, H. Tang, P. Ji and T. Chen, Zwitterionic polydopamine modified nanoparticles as an efficient nanoplatform to overcome both the mucus and epithelial barriers, Chem. Eng. J., 2022, 428, 132107 CrossRef CAS.
- C.-S. Cho, S.-K. Hwang, M.-J. Gu, C.-G. Kim, S.-K. Kim, D.-B. Ju, C.-H. Yun and H.-J. Kim, Mucosal Vaccine Delivery Using Mucoadhesive Polymer Particulate Systems, Tissue Eng. Regener. Med., 2021, 18, 693–712 CrossRef PubMed.
- M. Rinaudo, Chitin and chitosan: Properties and applications, Prog. Polym. Sci., 2006, 31, 603–632 CrossRef CAS.
- R. Popescu, M. V. Ghica, C.-E. Dinu-Pîrvu, V. Anuţa, D. Lupuliasa and L. Popa, New Opportunity to Formulate Intranasal Vaccines and Drug Delivery Systems Based on Chitosan, Int. J. Mol. Sci., 2020, 21, 5016 CrossRef CAS PubMed.
- E. C. Carroll, L. Jin, A. Mori, N. Muñoz-Wolf, E. Oleszycka, H. B. T. Moran, S. Mansouri, C. P. McEntee, E. Lambe, E. M. Agger, P. Andersen, C. Cunningham, P. Hertzog, K. A. Fitzgerald, A. G. Bowie and E. C. Lavelle, The Vaccine Adjuvant Chitosan Promotes Cellular Immunity via DNA Sensor cGAS-STING-Dependent Induction of Type I Interferons, Immunity, 2016, 44, 597–608 CrossRef CAS PubMed.
- C. Villiers, M. Chevallet, H. Diemer, R. Couderc, H. Freitas, A. Van Dorsselaer, P. N. Marche and T. Rabilloud, From secretome analysis to immunology: chitosan induces major alterations in the activation of dendritic cells via a TLR4-dependent mechanism, Mol. Cell. Proteomics, 2009, 8, 1252–1264 CrossRef CAS PubMed.
- H. Li, S. B. Willingham, J. P.-Y. Ting and F. Re, Cutting Edge: Inflammasome Activation by Alum and Alum's Adjuvant Effect Are Mediated by NLRP3, J. Immunol., 2008, 181, 17–21 CrossRef CAS PubMed.
- M. Dabaghian, A. M. Latifi, M. Tebianian, H. NajmiNejad and S. M. Ebrahimi, Nasal vaccination with r4M2e.HSP70c antigen encapsulated into N-trimethyl chitosan (TMC) nanoparticulate systems: Preparation and immunogenicity in a mouse model, Vaccine, 2018, 36, 2886–2895 CrossRef CAS PubMed.
- J. Mosafer, A.-H. Sabbaghi, A. Badiee, S. Dehghan and M. Tafaghodi, Preparation, characterization and in vivo evaluation of alginate-coated chitosan and trimethylchitosan nanoparticles loaded with PR8 influenza virus for nasal immunization, Asian J. Pharm. Sci., 2019, 14, 216–221 CrossRef PubMed.
- S. Biswas, M. Chattopadhyay, K. K. Sen and M. K. Saha, Development and characterization of alginate coated low molecular weight chitosan nanoparticles as new carriers for oral vaccine delivery in mice, Carbohydr. Polym., 2015, 121, 403–410 CrossRef CAS PubMed.
- D. Pawar and K. S. Jaganathan, Mucoadhesive glycol chitosan nanoparticles for intranasal delivery of hepatitis B vaccine: enhancement of mucosal and systemic immune response, Drug Delivery, 2016, 23, 185–194 CrossRef CAS PubMed.
- G. Sinani, M. Sessevmez, M. K. Gök, S. Özgümüş, H. O. Alpar and E. Cevher, Modified chitosan-based nanoadjuvants enhance immunogenicity of protein antigens after mucosal vaccination, Int. J. Pharm., 2019, 569, 118592 CrossRef CAS PubMed.
- K. Zhao, G. Chen, X. Shi, T. Gao, W. Li, Y. Zhao, F. Zhang, J. Wu, X. Cui and Y.-F. Wang, Preparation and Efficacy of a Live Newcastle Disease Virus Vaccine Encapsulated in Chitosan Nanoparticles, PLoS One, 2012, 7, e53314 CrossRef CAS PubMed.
- P. Cao, F. Y. Han, L. Grøndahl, Z. P. Xu and L. Li, Enhanced Oral Vaccine Efficacy of Polysaccharide-Coated Calcium Phosphate Nanoparticles, ACS Omega, 2020, 5, 18185–18197 CrossRef CAS PubMed.
- S. Dehghan, M. T. Kheiri, K. Abnous, M. Eskandari and M. Tafaghodi, Preparation, characterization and immunological evaluation of alginate nanoparticles loaded with whole inactivated influenza virus: Dry powder formulation for nasal immunization in rabbits, Microb. Pathog., 2018, 115, 74–85 CrossRef CAS PubMed.
- S. M. Hanson, S. Singh, A. Tabet, K. J. Sastry, M. Barry and C. Wang, Mucoadhesive wafers composed of binary polymer blends for sublingual delivery and preservation of protein vaccines, J. Controlled Release, 2021, 330, 427–437 CrossRef CAS PubMed.
- B. C. Tang, M. Dawson, S. K. Lai, Y.-Y. Wang, J. S. Suk, M. Yang, P. Zeitlin, M. P. Boyle, J. Fu and J. Hanes, Biodegradable polymer nanoparticles that rapidly penetrate the human mucus barrier, Proc. Natl. Acad. Sci. U. S. A., 2009, 106, 19268–19273 CrossRef CAS PubMed.
- S. K. Lai, D.
E. O'Hanlon, S. Harrold, S. T. Man, Y.-Y. Wang, R. Cone and J. Hanes, Rapid transport of large polymeric nanoparticles in fresh undiluted human mucus, Proc. Natl. Acad. Sci. U. S. A., 2007, 104, 1482–1487 CrossRef CAS PubMed.
- M. Yang, S. K. Lai, Y.-Y. Wang, W. Zhong, C. Happe, M. Zhang, J. Fu and J. Hanes, Biodegradable nanoparticles composed entirely of safe materials that rapidly penetrate human mucus, Angew. Chem., Int. Ed., 2011, 50, 2597–2600 CrossRef CAS PubMed.
- Y.-Y. Wang, S. K. Lai, J. S. Suk, A. Pace, R. Cone and J. Hanes, Addressing the PEG mucoadhesivity paradox to engineer nanoparticles that ‘slip’ through the human mucus barrier, Angew. Chem., Int. Ed., 2008, 47, 9726–9729 CrossRef CAS PubMed.
- A. A. D'souza and R. Shegokar, Polyethylene glycol (PEG): a versatile polymer for pharmaceutical applications, Expert Opin. Drug Delivery, 2016, 13, 1257–1275 CrossRef PubMed.
- Z. A. Parray, M. I. Hassan, F. Ahmad and A. Islam, Amphiphilic nature of polyethylene glycols and their role in medical research, Polym. Test., 2020, 82, 106316 CrossRef CAS.
- K. Maisel, L. Ensign, M. Reddy, R. Cone and J. Hanes, Effect of surface chemistry on nanoparticle interaction with gastrointestinal mucus and distribution in the gastrointestinal tract following oral and rectal administration in the mouse, J Control Release, 2015, 197, 48–57 CrossRef CAS PubMed.
- S. H. Kelly, E. E. Opolot, Y. Wu, B. Cossette, A. K. Varadhan and J. H. Collier, Tabletized Supramolecular Assemblies for Sublingual Peptide Immunization, Adv. Healthcare Mater., 2021, 10, 2001614 CrossRef CAS PubMed.
- S. H. Kelly, Y. Wu, A. K. Varadhan, E. J. Curvino, A. S. Chong and J. H. Collier, Enabling sublingual peptide immunization with molecular self-assemblies, Biomaterials, 2020, 241, 119903 CrossRef CAS PubMed.
- S. H. Kelly, B. J. Cossette, A. K. Varadhan, Y. Wu and J. H. Collier, Titrating Polyarginine into Nanofibers Enhances Cyclic-Dinucleotide Adjuvanticity in Vitro and after Sublingual Immunization, ACS Biomater. Sci. Eng., 2021, 7, 1876–1888 CrossRef CAS PubMed.
- K. Maisel, M. Reddy, Q. Xu, S. Chattopadhyay, R. Cone, L. M. Ensign and J. Hanes, Nanoparticles coated with high molecular weight PEG penetrate mucus and provide uniform vaginal and colorectal distribution in vivo, Nanomedicine, 2016, 11, 1337–1343 CrossRef CAS PubMed.
- Q. Xu, L. M. Ensign, N. J. Boylan, A. Schön, X. Gong, J.-C. Yang, N. W. Lamb, S. Cai, T. Yu, E. Freire and J. Hanes, Impact of Surface Polyethylene Glycol (PEG) Density on Biodegradable Nanoparticle Transport in Mucus ex Vivo and Distribution in Vivo, ACS Nano, 2015, 9, 9217–9227 CrossRef CAS PubMed.
- Z. Xie, Z. Ji, Z. Zhang, T. Gong and X. Sun, Adenoviral vectors coated with cationic PEG derivatives for intravaginal vaccination against HIV-1, Biomaterials, 2014, 35, 7896–7908 CrossRef CAS PubMed.
- H. Freire Haddad, J. A. Burke, E. A. Scott and G. A. Ameer, Clinical Relevance of Pre-Existing and Treatment-Induced Anti-Poly(Ethylene Glycol) Antibodies, Regener. Eng. Transl. Med., 2022, 8(1), 32–42 CrossRef CAS PubMed.
- E. D. H. Mansfield, V. R. de la Rosa, R. M. Kowalczyk, I. Grillo, R. Hoogenboom, K. Sillence, P. Hole, A. C. Williams and V. V. Khutoryanskiy, Side chain variations radically alter the diffusion of poly(2-alkyl-2-oxazoline) functionalised nanoparticles through a mucosal barrier, Biomater. Sci., 2016, 4, 1318–1327 RSC.
- X. Shan, S. Aspinall, D. B. Kaldybekov, F. Buang, A. C. Williams and V. V. Khutoryanskiy, Synthesis and Evaluation of Methacrylated Poly(2-ethyl-2-oxazoline) as a Mucoadhesive
Polymer for Nasal Drug Delivery, ACS Appl. Polym. Mater., 2021, 3, 5882–5892 CrossRef CAS.
- E. A. Krogstad, R. Ramanathan, C. Nhan, J. C. Kraft, A. K. Blakney, S. Cao, R. J. Y. Ho and K. A. Woodrow, Nanoparticle-releasing nanofiber composites for enhanced in vivo vaginal retention, Biomaterials, 2017, 144, 1–16 CrossRef CAS PubMed.
- Y. Cu, C. J. Booth and W. M. Saltzman, In vivo distribution of surface-modified PLGA nanoparticles following intravaginal delivery, J. Control. Release, 2011, 156, 258–264 CrossRef CAS PubMed.
- R. L. Creighton and K. A. Woodrow, Microneedle-Mediated Vaccine Delivery to the Oral Mucosa, Adv. Healthcare Mater., 2019, 8, 1801180 Search PubMed.
- D. G. Koutsonanos, M. del P. Martin, V. G. Zarnitsyn, S. P. Sullivan, R. W. Compans, M. R. Prausnitz and I. Skountzou, Transdermal Influenza Immunization with Vaccine-Coated Microneedle Arrays, PLoS One, 2009, 4, e4773 CrossRef PubMed.
- C. L. McNeilly, M. L. Crichton, C. A. Primiero, I. H. Frazer, M. S. Roberts and M. A. F. Kendall, Microprojection arrays to immunise at mucosal surfaces, J. Controlled Release, 2014, 196, 252–260 CrossRef CAS PubMed.
- Y. Ma, W. Tao, S. J. Krebs, W. F. Sutton, N. L. Haigwood and H. S. Gill, Vaccine delivery to the oral cavity using coated microneedles induces systemic and mucosal immunity, Pharm. Res., 2014, 31, 2393–2403 CrossRef CAS PubMed.
- Y.-J. Oh, H.-R. Cha, S. J. Hwang, D.-S. Kim, Y.-J. Choi, Y.-S. Kim, Y.-R. Shin, T. T. Nguyen, S.-O. Choi, J. M. Lee and J.-H. Park, Ovalbumin and cholera toxin delivery to buccal mucus for immunization using microneedles and comparison of immunological response to transmucosal delivery, Drug Delivery Transl. Res., 2021, 11, 1390–1400 CrossRef CAS PubMed.
- X. Hong, Z. Wu, L. Chen, F. Wu, L. Wei and W. Yuan, Hydrogel Microneedle Arrays for Transdermal Drug Delivery, Nano-Micro Lett., 2014, 6, 191–199 CrossRef CAS.
- N. Wang, Y. Zhen, Y. Jin, X. Wang, N. Li, S. Jiang and T. Wang, Combining different types of multifunctional liposomes loaded with ammonium bicarbonate to fabricate microneedle arrays as a vaginal mucosal vaccine adjuvant-dual delivery system (VADDS), J. Controlled Release, 2017, 246, 12–29 CrossRef CAS PubMed.
- T. Wang, Y. Zhen, X. Ma, B. Wei, S. Li and N. Wang, Mannosylated and lipid A-incorporating cationic liposomes constituting microneedle arrays as an effective oral mucosal HBV vaccine applicable in the controlled temperature chain, Colloids Surf., B, 2015, 126, 520–530 CrossRef CAS PubMed.
- R. S. J. Ingrole, E. Azizoglu, M. Dul, J. C. Birchall, H. S. Gill and M. R. Prausnitz, Trends of microneedle technology in the scientific literature, patents, clinical trials and internet activity, Biomaterials, 2021, 267, 120491 CrossRef CAS PubMed.
- Y. Shen and L. Qiu, Effective oral delivery of gp100 plasmid vaccine against metastatic melanoma through multi-faceted blending-by-blending nanogels, Nanomedicine, 2019, 22, 102114 CrossRef CAS PubMed.
- Y. Zhang, H. Li, Q. Wang, X. Hao, H. Li, H. Sun, L. Han, Z. Zhang, Q. Zou and X. Sun, Rationally Designed Self-Assembling Nanoparticles to Overcome Mucus and Epithelium Transport Barriers for Oral Vaccines against Helicobacter pylori, Adv. Funct. Mater., 2018, 28, 1802675 CrossRef.
- M. K. Amin and J. S. Boateng, Enhancing Stability and Mucoadhesive Properties of Chitosan Nanoparticles by Surface Modification with Sodium Alginate and Polyethylene Glycol for Potential Oral Mucosa Vaccine Delivery, Mar. Drugs, 2022, 20, 156 CrossRef CAS PubMed.
- M. K. Amin and J. S. Boateng, Comparison and process optimization of PLGA, chitosan and silica nanoparticles for potential oral vaccine delivery, Ther. Delivery, 2019, 10, 8 Search PubMed.
- H. Jeong, C.-S. Lee, J. Lee, J. Lee, H. S. Hwang, M. Lee and K. Na, Hemagglutinin Nanoparticulate Vaccine with Controlled Photochemical Immunomodulation for Pathogenic Influenza-Specific Immunity, Adv. Sci., 2021, 8, 2100118 CrossRef CAS PubMed.
- X. Wei, M. Beltrán-Gastélum, E. Karshalev, B. Esteban-Fernández de Ávila, J. Zhou, D. Ran, P. Angsantikul, R. H. Fang, J. Wang and L. Zhang, Biomimetic Micromotor Enables Active Delivery of Antigens for Oral Vaccination, Nano Lett., 2019, 19, 1914–1921 CrossRef CAS PubMed.
- I. Pereira de Sousa, B. Cattoz, M. D. Wilcox, P. C. Griffiths, R. Dalgliesh, S. Rogers and A. Bernkop-Schnürch, Nanoparticles decorated with proteolytic enzymes, a promising strategy to overcome the mucus barrier, Eur. J. Pharm. Biopharm., 2015, 97, 257–264 CrossRef CAS PubMed.
- T. Jiang, B. Singh, H.-S. Li, Y.-K. Kim, S.-K. Kang, J.-W. Nah, Y.-J. Choi and C.-S. Cho, Targeted oral delivery of BmpB vaccine using porous PLGA microparticles coated with M cell homing peptide-coupled chitosan, Biomaterials, 2014, 35, 2365–2373 CrossRef CAS PubMed.
- D. Walker, B. T. Käsdorf, H.-H. Jeong, O. Lieleg and P. Fischer, Enzymatically active biomimetic micropropellers for the penetration of mucin gels, Sci. Adv., 2015, 1, e1500501 CrossRef PubMed.
- J. Lv, Y. Xing, T. Xu, X. Zhang and X. Du, Advanced micro/nanomotors for enhanced bioadhesion and tissue penetration, Appl. Mater. Today, 2021, 23, 101034 CrossRef.
- D. Fu, Z. Wang, Y. Tu and F. Peng, Interactions between Biomedical Micro-/Nano-Motors and the Immune Molecules, Immune Cells, and the Immune System: Challenges and Opportunities, Adv. Healthcare Mater., 2021, 10, 2001788 CrossRef CAS PubMed.
- W. Gao, R. Dong, S. Thamphiwatana, J. Li, W. Gao, L. Zhang and J. Wang, Artificial Micromotors in the Mouse's Stomach: A Step toward in Vivo Use of Synthetic Motors, ACS Nano, 2015, 9, 117–123 CrossRef CAS PubMed.
- J. Li, S. Thamphiwatana, W. Liu, B. Esteban-Fernández de Ávila, P. Angsantikul, E. Sandraz, J. Wang, T. Xu, F. Soto, V. Ramez, X. Wang, W. Gao, L. Zhang and J. Wang, Enteric Micromotor Can Selectively Position and Spontaneously Propel in the Gastrointestinal Tract, ACS Nano, 2016, 10, 9536–9542 CrossRef CAS PubMed.
- E. Karshalev, B. Esteban-Fernández de Ávila, M. Beltrán-Gastélum, P. Angsantikul, S. Tang, R. Mundaca-Uribe, F. Zhang, J. Zhao, L. Zhang and J. Wang, Micromotor Pills as a Dynamic Oral Delivery Platform, ACS Nano, 2018, 12, 8397–8405 CrossRef CAS PubMed.
- S. U. Christl, P. R. Murgatroyd, G. R. Gibson and J. H. Cummings, Production, metabolism, and excretion of hydrogen in the large intestine, Gastroenterology, 1992, 102, 1269–1277 CrossRef CAS.
- N. Roohani, R. Hurrell, R. Kelishadi and R. Schulin, Zinc and its importance for human health: An integrative review, J. Res. Med. Sci., 2013, 18, 144–157 Search PubMed.
- A. Abramson, E. Caffarel-Salvador, M. Khang, D. Dellal, D. Silverstein, Y. Gao, M. R. Frederiksen, A. Vegge, F. Hubálek, J. J. Water, A. V. Friderichsen, J. Fels, R. K. Kirk, C. Cleveland, J. Collins, S. Tamang, A. Hayward, T. Landh, S. T. Buckley, N. Roxhed, U. Rahbek, R. Langer and G. Traverso, An ingestible self-orienting system for oral delivery of macromolecules, Science, 2019, 363, 611–615 CrossRef CAS PubMed.
- A. Abramson, A. R. Kirtane, Y. Shi, G. Zhong, J. E. Collins, S. Tamang, K. Ishida, A. Hayward, J. Wainer, N. U. Rajesh, X. Lu, Y. Gao, P. Karandikar, C. Tang, A. Lopes, A. Wahane, D. Reker, M. R. Frederiksen, B. Jensen, R. Langer and G. Traverso, Oral mRNA delivery using capsule-mediated gastrointestinal tissue injections, Matter, 2022, 5(3), 975–987 CrossRef CAS.
- A. Abramson, M. R. Frederiksen, A. Vegge, B. Jensen, M. Poulsen, B. Mouridsen, M. O. Jespersen, R. K. Kirk, J. Windum, F. Hubálek, J. J. Water, J. Fels, S. B. Gunnarsson, A. Bohr, E. M. Straarup, M. W. H. Ley, X. Lu, J. Wainer, J. Collins, S. Tamang, K. Ishida, A. Hayward, P. Herskind, S. T. Buckley, N. Roxhed, R. Langer, U. Rahbek and G. Traverso, Oral delivery of systemic monoclonal antibodies, peptides and small molecules using gastric auto-injectors, Nat. Biotechnol., 2022, 40, 103–109 CrossRef CAS PubMed.
- C. Müller, K. Leithner, S. Hauptstein, F. Hintzen, W. Salvenmoser and A. Bernkop-Schnürch, Preparation and characterization of mucus-penetrating papain/poly(acrylic acid) nanoparticles for oral drug delivery applications, J. Nanopart. Res., 2012, 15, 1353 CrossRef.
- C. Müller, G. Perera, V. König and A. Bernkop-Schnürch, Development and in vivo evaluation of papain-functionalized nanoparticles, Eur. J. Pharm. Biopharm., 2014, 87, 125–131 CrossRef PubMed.
- R. Pavan, S. Jain, Shraddha and A. Kumar, Properties and Therapeutic Application of Bromelain: A Review, Biotechnol. Res. Int., 2012, 2012, 976203 Search PubMed.
-
C. R. Engwerda, D. Andrew, A. Ladhams and T. L. Mynott, Bromelain Modulates T Cell and B Cell Immune Responses in Vitro and in Vivo 10.
- R. Bottega, I. Persico, F. De Seta, F. Romano and G. Di Lorenzo, Anti-inflammatory properties of a proprietary bromelain extract (BromeyalTM) after in vitro simulated gastrointestinal digestion, Int. J. Immunopathol. Pharmacol., 2021, 35, 1–9 Search PubMed.
- E. Samaridou, K. Karidi, I. P. de Sousa, B. Cattoz, P. Griffiths, O. Kammona, A. Bernkop-Schnürch and C. Kiparissides, Enzyme-Functionalized PLGA Nanoparticles with Enhanced Mucus Permeation Rate, Nano LIFE, 2014, 04, 1441013 CrossRef CAS.
- C. Leichner, C. Menzel, F. Laffleur and A. Bernkop-Schnürch, Development and in vitro characterization of a papain loaded mucolytic self-emulsifying drug delivery system (SEDDS), Int. J. Pharm., 2017, 530, 346–353 CrossRef CAS PubMed.
- M. Kristensen and H. M. Nielsen, Cell-penetrating peptides as tools to enhance non-injectable delivery of biopharmaceuticals, Tissue Barriers, 2016, 4, e1178369 CrossRef PubMed.
- S.-H. Kim and Y.-S. Jang, Antigen targeting to M cells for enhancing the efficacy of mucosal vaccines, Exp. Mol. Med., 2014, 46, e85–e85 CrossRef CAS PubMed.
- M.-K. Yoo, S.-K. Kang, J.-H. Choi, I.-K. Park, H.-S. Na, H.-C. Lee, E.-B. Kim, N.-K. Lee, J.-W. Nah, Y.-J. Choi and C.-S. Cho, Targeted delivery of chitosan nanoparticles to Peyer's patch using M cell-homing peptide selected by phage display technique, Biomaterials, 2010, 31, 7738–7747 CrossRef CAS PubMed.
- S.-H. Kim, K.-W. Seo, J. Kim, K.-Y. Lee and Y.-S. Jang, The M cell-targeting ligand promotes antigen delivery and induces antigen-specific immune responses in mucosal vaccination, J. Immunol., 2010, 185, 5787–5795 CrossRef CAS PubMed.
- P. Long, Q. Zhang, M. Xue, G. Cao, C. Li, W. Chen, F. Jin, Z. Li, R. Li, X. Wang and W. Ge, Tomato lectin-modified nanoemulsion-encapsulated MAGE1-HSP70/SEA complex protein vaccine: Targeting intestinal M cells following peroral administration, Biomed. Pharmacother., 2019, 115, 108886 CrossRef CAS PubMed.
- B. Singh, S. Maharjan, T. Jiang, S.-K. Kang, Y.-J. Choi and C.-S. Cho, Combinatorial Approach of Antigen Delivery Using M Cell-Homing Peptide and Mucoadhesive Vehicle to Enhance the Efficacy of Oral Vaccine, Mol. Pharm., 2015, 12, 3816–3828 CrossRef CAS PubMed.
- D. Liu, S. Zhang, E. Poteet, C. Marin-Muller, C. Chen and Q. Yao, Sublingual Immunization with Chimeric C1q/CD40 Ligand/HIV Virus-like Particles Induces Strong Mucosal Immune Responses against HIV, Vaccines, 2021, 9, 1236 CrossRef CAS PubMed.
- Z. Wang, K. D. Popowski, D. Zhu, B. L. de Juan Abad, X. Wang, M. Liu, H. Lutz, N. De Naeyer, C. T. DeMarco, T. N. Denny, P.-U. C. Dinh, Z. Li and K. Cheng, Exosomes decorated with a recombinant SARS-CoV-2 receptor-binding domain as an inhalable COVID-19 vaccine, Nat. Biomed. Eng., 2022, 6, 791–805 CrossRef CAS PubMed.
- Q. Bao, X. Li, G. Han, Y. Zhu, C. Mao and M. Yang, Phage-based vaccines, Adv. Drug Delivery Rev., 2019, 145, 40–56 CrossRef CAS PubMed.
- R. Sausset, M. A. Petit, V. Gaboriau-Routhiau and M. De Paepe, New insights into intestinal phages, Mucosal Immunol., 2020, 13, 205–215 CrossRef CAS PubMed.
- J. S. Fraser, Z. Yu, K. L. Maxwell and A. R. Davidson, Ig-like domains on bacteriophages: a tale of promiscuity and deceit, J. Mol. Biol., 2006, 359, 496–507 CrossRef CAS PubMed.
- J. J. Barr, R. Auro, M. Furlan, K. L. Whiteson, M. L. Erb, J. Pogliano, A. Stotland, R. Wolkowicz, A. S. Cutting, K. S. Doran, P. Salamon, M. Youle and F. Rohwer, Bacteriophage adhering to mucus provide a non–host-derived immunity, Proc. Natl. Acad. Sci. U. S. A., 2013, 110, 10771–10776 CrossRef CAS PubMed.
- P. González-Cano, L. N. A. Gamage, K. Marciniuk, C. Hayes, S. Napper, S. Hayes and P. J. Griebel, Lambda display phage as a mucosal vaccine delivery vehicle for peptide antigens, Vaccine, 2017, 35, 7256–7263 CrossRef PubMed.
- J. Bal, H.-Y. Jung, L. N. Nguyen, J. Park, Y.-S. Jang and D.-H. Kim, Evaluation of cell-surface displayed synthetic consensus dengue EDIII cells as a potent oral vaccine candidate, Microb. Cell Fact., 2018, 17, 146 CrossRef CAS PubMed.
- F. Yang, L. Meng, S. Lin, F. Wu and J. Liu, Polyethyleneimine-complexed charge-reversed yeast cell walls for the enhanced oral delivery of pseudovirus-based antigens, Chem. Commun., 2021, 57, 12768–12771 RSC.
- D. Hirayama, T. Iida and H. Nakase, The Phagocytic Function of Macrophage-Enforcing Innate Immunity and Tissue Homeostasis, Int. J. Mol. Sci., 2018, 19, 92 CrossRef PubMed.
- B. Zheng, W. Peng, M. Guo, M. Huang, Y. Gu, T. Wang, G. Ni and D. Ming, Inhalable nanovaccine with biomimetic coronavirus structure to trigger mucosal immunity of respiratory tract against COVID-19, Chem. Eng. J., 2021, 418, 129392 CrossRef CAS PubMed.
- X. Jiang, S. Xia, X. He, H. Ma, Y. Feng, Z. Liu, W. Wang, M. Tian, H. Chen, F. Peng, L. Wang, P. Zhao, J. Ge and D. Liu, Targeting peptide-enhanced antibody and CD11c + dendritic cells to inclusion bodies expressing protective antigen against ETEC in mice, FASEB J., 2019, 33, 2836–2847 CrossRef CAS PubMed.
- H.-B. Lee, S.-Y. Yoon, B. Singh, S.-H. Oh, L. Cui, C. Yan, S.-K. Kang, Y.-J. Choi and C.-S. Cho, Oral Immunization of FMDV Vaccine Using pH-Sensitive and Mucoadhesive Thiolated Cellulose Acetate Phthalate Microparticles, Tissue Eng. Regener. Med., 2018, 15, 1–11 CrossRef CAS PubMed.
- S. Bonengel, F. Prüfert, G. Perera, J. Schauer and A. Bernkop-Schnürch, Polyethylene imine-6-phosphogluconic acid nanoparticles–a novel zeta potential changing system, Int. J. Pharm., 2015, 483, 19–25 CrossRef CAS PubMed.
- J. Wu, Y. Zheng, M. Liu, W. Shan, Z. Zhang and Y. Huang, Biomimetic Viruslike and Charge Reversible Nanoparticles to Sequentially Overcome Mucus and Epithelial Barriers for Oral Insulin Delivery, ACS Appl. Mater. Interfaces, 2018, 10, 9916–9928 CrossRef CAS PubMed.
- F. Sharifi, M. Jahangiri, I. Nazir, M. H. Asim, P. Ebrahimnejad, A. Hupfauf, R. Gust and A. Bernkop-Schnürch, Zeta potential changing nanoemulsions based on a simple zwitterion, J. Colloid Interface Sci., 2021, 585, 126–137 CrossRef CAS PubMed.
- L. E. Mansfield, S. Ting, R. W. Haverly and T. J. Yoo, The incidence and clinical implications of hypersensitivity to papain in an allergic population, confirmed by blinded oral challenge, Ann. Allergy, 1985, 55, 541–543 CAS.
Footnote |
† Authors contributed equally to this work. |
|
This journal is © The Royal Society of Chemistry 2023 |
Click here to see how this site uses Cookies. View our privacy policy here.