DOI:
10.1039/D3MH00265A
(Review Article)
Mater. Horiz., 2023,
10, 4059-4082
Prospects of nanoparticle-based radioenhancement for radiotherapy
Received
21st February 2023
, Accepted 2nd August 2023
First published on 3rd August 2023
Abstract
Radiotherapy is a key pillar of solid cancer treatment. Despite a high level of conformal dose deposition, radiotherapy is limited due to co-irradiation of organs at risk and subsequent normal tissue toxicities. Nanotechnology offers an attractive opportunity for increasing the efficacy and safety of cancer radiotherapy. Leveraging the freedom of design and the growing synthetic capabilities of the nanomaterial-community, a variety of engineered nanomaterials have been designed and investigated as radiosensitizers or radioenhancers. While research so far has been primarily focused on gold nanoparticles and other high atomic number materials to increase the absorption cross section of tumor tissue, recent studies are challenging the traditional concept of high-Z nanoparticle radioenhancers and highlight the importance of catalytic activity. This review provides a concise overview on the knowledge of nanoparticle radioenhancement mechanisms and their quantification. It critically discusses potential radioenhancer candidate materials and general design criteria for different radiation therapy modalities, and concludes with research priorities in order to advance the development of nanomaterials, to enhance the efficacy of radiotherapy and to increase at the same time the therapeutic window.
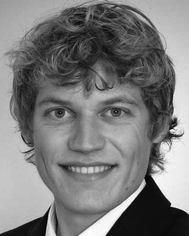
Lukas R. H. Gerken
| Dr Lukas Gerken graduated with a BSc in Physics at the University of Cologne (BSc) and received a Bachelor (BSc) in Sport Science from the German Sport University Cologne. He then pursued his MSc degree in Biomedical Engineering at the Technical University of Vienna with an exchange semester at the Swiss Laboratories for Materials Science and Technology (Empa) and ETH Zurich. Lukas then carried out his doctoral studies at ETH Zurich at the Nanoparticle Systems Engineering Laboratory chaired by Prof. Herrmann. He spearheaded the development of rationally-designed inorganic nanoparticle radioenhancers for precision radiation therapy with X-rays or protons. He won a prestigious Young Scientist Fellowship funded by the Ria&Arthur Dietschweiler Foundation and is currently a postdoctoral researcher at Empa. |
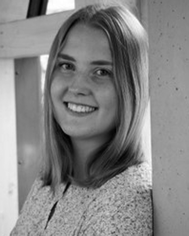
Maren E. Gerdes
| Maren Gerdes holds a BSc in Biomedicine and is currently a medical doctor in training at Karolinska Institute in Stockholm, Sweden. She completed her BSc thesis project at ETH Zurich and Empa focusing on materials design criteria for nanoparticles used in radiation therapy and has further gained research experience at Karolinska Institute and the German Center for Neurodegenerative diseases (DZNE). |
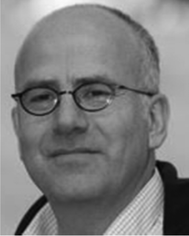
Martin Pruschy
| Prof. Martin Pruschy is a professor of radiobiology at the University of Zurich and Head of the Research Unit for Molecular Radiobiology at the Department of Radiation Oncology, University Hospital Zurich. He has also been visiting professor at the Rambam Medical Center and Technion, Haifa, Israel. His research focuses on different aspects of translational research in the field of radiobiology investigating the cellular and tumoral response on the molecular, cellular and in vivo level to ionizing radiation alone and in combination with classical chemo-therapeutical and novel pharmacological agents. |
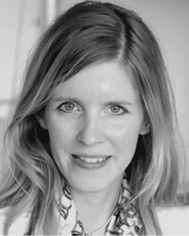
Inge K. Herrmann
| Prof. Inge K. Herrmann is a Swiss National Science Foundation (SNSF) Eccellenza professor of medical materials innovation at ETH Zurich and the Swiss Laboratories for Materials Science and Technology (Empa). She graduated from ETH Zurich with a PhD in biomedical engineering and underwent additional training in (pre)clinical research at the University Hospital Zurich, the University of Illinois, and Imperial College in London. Her research interests include the design and development of conceptionally novel materials and technologies for healthcare. |
1. Introduction
Approximately 50% of all cancer patients have an indication for radiotherapy at least once during the course of their disease,1–3 with an absolute number of patients steadily increasing assuming overall cancer rates remain unchanged.4 Radiotherapy can be administered to cure cancer or relieve cancer symptoms, either as monotherapy or in multimodal cancer treatment in combination with surgery, chemotherapy or immunotherapy (Fig. 1).5 It is either employed in a neoadjuvant setting to shrink the tumor before surgery, or in an adjuvant setting to destroy left-over cancer cells or both. Radiotherapy effects can be further enhanced by the addition of (nanomaterial-based) radioenhancers, extending the therapeutic window by increasing efficacy and reducing side effects.6 Advanced radiation treatments and radiotherapy techniques (e.g. particle- vs. photon-radiotherapy; intensity-modulated radiotherapy and volumetric arc therapy) are nowadays established in clinical practice and are well suited for planning and delivering of radiotherapy with conformity.7,8 Despite these technical advances in modern, image-guided, adaptive radiotherapy, radiation toxicity to co-irradiated adjacent normal tissue still determines and limits the maximal dose that can be applied to a tumor, and is a most critical limitation of contemporary radiotherapy.3 To reduce healthy tissue damage in organs at risk and to overcome radioresistance of tumors (e.g. due to a hypoxic tumor environment),9–12 new strategies rendering the tumor tissue more susceptible are sought after.
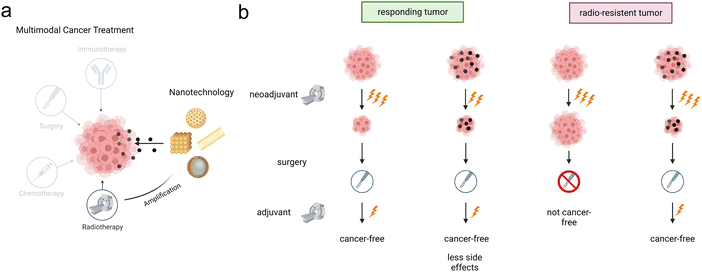 |
| Fig. 1 Schematic illustration of the potential integration of nanotechnology into cancer treatment (a), and a representation of beneficial treatment settings using radioenhancer nanoparticles (b). Figure created with BioRender.com. | |
Radiosensitizers and radioenhancers in the form of high-Z metal nanoparticles (NPs) deposited in the tumorous tissue have recently attracted considerable attention as an alternate therapeutic route that can overcome these limitations and widen the therapeutic window. A pioneering study in 2004 demonstrated control of a malignant tumor in vivo by the administration of gold nanoparticles prior to radiotherapy.13 Presently, the radioenhancing effect of NPs is widely accepted, with two candidate materials, AGuIX® (NH TherAguix, Lyon, France) and CE-certified NBTXR3/Hensify® (Nanobiotix, Paris, France), in clinical evaluation.14–16 In addition to widely studied metal and metal oxide radioenhancer nanoparticles, new material design strategies offer the possibility of (multimodal) combination treatments, paving the way to even more effective cancer treatments in the future. For example, drug-loadable metal–organic frameworks (MOFs) can generate toxic reactive oxygen species (ROS) during irradiation, offering new prospects for combination treatments.17,18 Although a vast number of different nanomaterials have been explored as radioenhancers, the translation to clinical application is slow.19 Current major translational barriers include the scalability of high-quality nanoparticle manufacturing and the significant knowledge gaps in nanoparticle design, effectiveness and biological activity.19,20 In particular, the limited understanding of nanoparticle-based radioenhancement precludes rational material designs and limits the full exploitation of the available materials design space. The scarcity of comparative studies and benchmarking prevents relative comparison of candidate materials and subsequent rational selection of the best performing candidate.21–23 The deficient understanding of radiotherapy enhancement mechanisms and nanomaterials toxicity additionally inhibits the design of optimal candidate materials.19 This gap in fundamental mechanistic understanding implies that potentially considerable gains in performance can be achieved through rationally designed materials and appropriate material selection based on irradiation conditions (external vs. internal radiotherapy, photons vs. protons, beam energy).
In the following, we provide a concise summary of the current knowledge on radioenhancement mechanisms. Key aspects of physical, chemical and biological mechanisms and their quantifications during radiation therapy with and without nanoparticles are discussed. Nanoparticle radioenhancer material candidates and their radioenhancing properties from a preclinical perspective and their clinical progress are presented. From this, we deduce key materials design criteria and corresponding considerations for future research in this emerging field.
2. Principles of radiotherapy: physical, chemical and biological responses to ionizing radiation
To delineate the complex processes in between initial energy deposition and biological responses to irradiation, four stages can be defined: (i) the physical, (ii) the physico-chemical, (iii) the chemical, and (iv) the biological stage.9 At the physical stage, ionizing radiation travels in the form of particles or electromagnetic waves through the target medium and excites or ionizes the molecules in its path. Initial physical processes in local track regions happen within the first ∼10−15 s.10 Dissociative decay, auto-ionization, thermalization, or solvation characterize the physico-chemical stage (time scale ∼10−15–10−12 s). The following chemical stage (∼10−12–10−6 s) is associated with the diffusion and production of initial or new chemical species, resulting in a homogenous distribution of radiolysis products. Since the water content in tumors, tissues, and organs typically is around 70–85%,11 water radiolysis takes on an important role in the cellular response especially to ionizing radiation at the low LET (linear energy transfer) level of clinically relevant photon and proton irradiation. The physical and chemical processes related to water radiolysis are very well studied: irradiated water after the initial physical stage contains excited H2O* and the ionized species H2O+ and e−. During the physico-chemical stage, H2O* molecules can dissociate to produce ˙H and ˙OH, H2 and ˙O, and H2O+ and e− species or radicals. Further processes such as ion–molecule reactions (H2O+ + H2O) and/or electron and ion hydration lead to the production of (H3O+)aq, (e−)aq, H3O+, and ˙OH species. During the chemical stage, the initially formed radiolysis products diffuse and interact with each other to create OH−, H2O2, and additional H2 while consuming some of the initially generated ˙OH, ˙H, H3O+, and (e−)aq species.9,12 Yields of the different water radiolysis products can be found in several simulation reports and are dependent on the ionizing source used.12–14
At the biological stage, the responses can be determined at different spatial and temporal resolution with some biological processes manifested only months, years or decades after irradiation (e.g. chronic normal tissue toxicities and secondary malignancies), phenomena which are beyond the focus of this review. The immediate biological damage can be induced by direct or indirect irradiation effects. Direct damage, caused by incident photons, charged particles and their subsequently generated electron splashes, is only detrimental when vital elements of the cell are directly affected. Critically important cellular components for ionizing radiation-induced damage include the DNA, the cellular membrane, and essential cell organelles (mitochondria,15 endoplasmic reticulum, ribosomes, and lysosomes).16 Low LET photon and proton irradiation only induce a small proportion of (water radiolysis-independent) direct biological damage, while its relative importance increases with increasing LET radiation.17 The dominant indirect effect of low LET irradiation is mainly induced through water radiolysis products, which can cause DNA breaks and complex chromosomal aberrations, protein denaturation, cell membrane disruption, enzyme inactivation, and DNA or RNA mutations.9 An important and dominating role driving indirect damage is attributed to ˙OH radicals, which are considered the most powerful oxidant among the water derivatives.8 In fact, it has been shown through the use of hydroxyl scavengers that 60–90% of cellular radiation damage can be attributed to ˙OH radicals.17–20 This hydroxyl-mediated indirect damage action is strongly dependent on the oxygen partial pressure (pO2) in the tumor microenvironment.19 A commonly used explanation for the observed oxygen enhancement effect is that free-radical-induced DNA damage is “fixed” in the presence of molecular oxygen (“oxygen fixation theory”), thereby making it permanent.16 Additionally, highly deleterious oxidizing radicals (superoxide, ˙O2−, and hydroperoxyl radicals, ˙OOH) are created during the chemical stage of radiolysis in the presence of molecular oxygen.14 Thus, the presence of oxygen in the target medium has a crucial impact on the success of the radiotherapy and tumor hypoxia represents a major radioresistance mechanism. Overall, the complex radiation response on the cellular and (patho-) physiological level have been described as the 6 Rs of radiobiology, where a change in any one of the Rs can increase or decrease the net therapeutic effect of fractionated radiotherapy (see below).22,23
3. Nanoparticle radioenhancement mechanisms
Nanoparticles that can increase the effect of radiotherapy by altering one or more of the aforementioned response stages can be classified as radioenhancers or radiosensitizers (Fig. 2a). While “radiosensitizer” defines any substance that sensitizes cells to radiation therapy, and has thus a more general meaning, the term “radioenhancer” implies an amplification role, such as delivering a higher dose to the tumor24 or increasing the chemical ROS species. Therefore, in this review we use the term radioenhancer for nanoparticles that are able to increase ionizing dose or ROS inside the tumor, while radiosensitizers are nanoparticles that biologically sensitize cells to ionizing radiation.
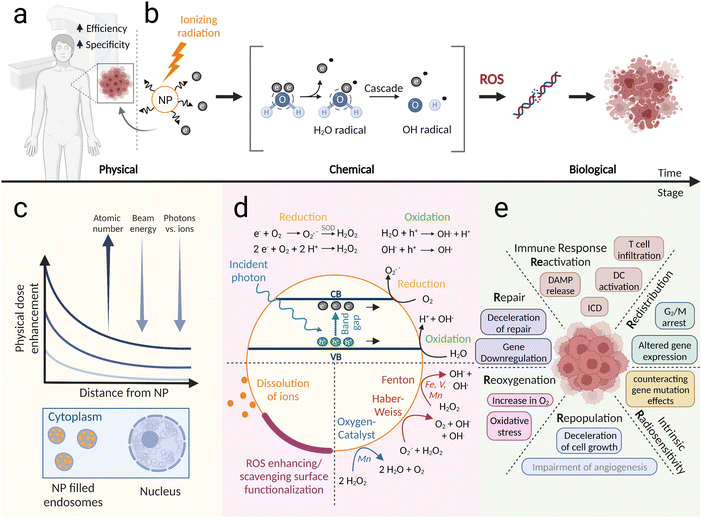 |
| Fig. 2 Mechanisms of nanoparticle radioenhancement. (a) Metal or metal oxide nanoparticles may be inserted into tumorous tissue, e.g. via injection or intravenously via the EPR effect, and act to enhance the efficiency and specificity of the applied radiation (e.g. X-rays, protons). (b) The mechanism of action of nanoparticle radioenhancers can be divided into three stages: a physical, a chemical and a biological stage. In the physical stage, ionizing radiation leads to the ejection of secondary electrons which can then, in the chemical stage, interact with other molecules, e.g. water, causing the creation of ROS. In the biological stage, the created ROS interacts with the components of the cell (e.g. DNA), eventually triggering cell death. (c) and (d) Detailed view of the three stages: (c) physical dose enhancement by NPs located in endosomes decreases with increasing distance from the NP, as well as beam energy and when photons instead of ions are used as the modality. The higher the atomic number of the particles, the stronger the physical dose enhancement. (d) Chemically, NPs can contribute to the creation of ROS through photocatalytic activity (top), surface effects (bottom left) or the action of oxygen catalysts (e.g. Mn) or Fenton and Haber–Weiss reactions (e.g. Fe) (bottom right). (e) Biological nanoparticle enhancement mechanism can be viewed in context of the 6 Rs of radiobiology. Figure created with BioRender.com. | |
The processes underlying nanoparticle radioenhancement can be divided into different stages (Fig. 2b): excitation and ionization of the NPs (physical stage), generation of reactive oxygen species (ROS) in the surrounding medium (chemical stage), and biological implications of increased damage on a cytoplasmic or nuclear level (biological stage). Furthermore, NPs can modulate biological processes, thereby sensitizing cells to ionizing radiation and increasing the effects of radiotherapy. The exact mechanisms through which NPs enhance ionizing radiation stage-dependent processes on the physical, chemical, and biological level are influenced by the nanoparticle composition and are the subject of ongoing research.
3.1 Physical enhancement
The physical interaction between NPs and ionizing radiation depends significantly on the modality of radiation used and its energy (Fig. 2c). Low energy kiloelectronvolt photons (<500 keV) interact with NPs via photoelectric absorption, resulting in the ejection of a photoelectron from the K, L, or M shell and the subsequent emission of fluorescence and Auger electrons.25 Regarding secondary electron emission after ionization of a NP, Monte Carlo simulations have shown that the NP dose enhancement decreases exponentially with increasing distance from the NP surface.26 Auger electrons contribute to enormous low-range (∼10 nm) dose enhancement, while electrons of higher energy (photo- or Compton electrons) contribute to micrometer-range (up to 30–40 μm) enhancement, which can lead to direct DNA damage.26,27 The photoelectric effect probability scales roughly with Z4/E3, rendering high-Z materials attractive candidates for radioenhancement using low-energy photon beams.28 However, clinically relevant applications typically use higher energies, and megaelectronvolt (MeV) photon interactions with NPs predominantly result in Compton scattering or pair production with interaction probabilities only linearly proportional to Z.25 Physical dose enhancement for MV photons was simulated to be negligible in cellular scenarios even for high-Z NPs,29 although very localized nanoscopic dose enhancement around gold nanoparticles remains a topic of discussion.30–32 For a highly localized dose to grant a therapeutic advantage from secondary species emission, NPs can be functionalized in order to target sensitive subcellular localizations. It has been shown that targeting the cells’ nucleus33,34 or mitochondria35–37 can indeed lead to enhanced X-ray treatment efficiency.
The major difference between photon- and particular proton-based radiotherapy at the macrolevel is their differential spatial distribution of energy deposition. Photon beams have the highest dose deposition close to the entrance surface and continuously deposit dose along the entire path throughout the tissue. Generally, this involves healthy tissue being co-irradiated proximally as well as distally of the target volume. In contrast, proton beams commonly deposit a lower dose in the entry field, and maximum dose deposition occurs within the so-called Bragg peak at a depth defined by the velocity of the applied protons. Behind this Bragg peak region – or Spread-Out Bragg Peak (SOBP) in clinical applications – no significant dose is deposited. The reduced volume of healthy tissue exposed to intermediate and low doses of proton radiotherapy results in reduced co-irradiation of dose-limiting organs at risk (OAR) such as brain stem, spinal cord, oral cavity, or the optic nerve and subsequently also a reduced risk of secondary malignancies in these co-irradiated organs. A physical characteristics of proton radiotherapy is the increase in LET towards the distal end of the spread-out Bragg Peak (SOBP).38,39 Irradiation with elevated LET induces more complex respectively clustered DNA lesions than yielded by conventional low LET photon irradiation. Utilizing proton-based instead of photon-based external beam irradiation alters the beam's interactions with NPs.40 These positively charged subatomic particles deposit energy through ionization and excitation of matter via Coulombic interactions.41 While the beneficial interaction of protons with NP radioenhancers has been demonstrated,40,42–45 a mechanistic understanding at the nanoscale level is very much under debate. Kim et al. first attributed the observed dose enhancement effects to particle-induced X-ray emission (PIXE).42 This explanation, along with enhancement by particle-induced gamma-ray emission (PIGE), was later rejected. Other mechanisms were considered as being more relevant, such as a very local (<100 nm range) secondary electron emission through Coulombic interactions of the protons with the NP.43,46 Indeed, Monte Carlo simulations have indicated a potential proton dose enhancement from small nanoparticles within <10 nm distance stemming from excess Auger electrons.47 A simulation with 1.3 MeV protons showed, that the yield of such secondary electrons from titanium surfaces could even be higher than from high-Z nanoparticles.48 Auger electrons have the ability to form ROS by radiolysis of water molecules.49 Other reports, using a model that underestimates the low-energy electron emission spectra, have concluded that physical dose enhancement from proton irradiated nanoparticles is negligible, especially when compared to kV photons.29,50 The accurate reproduction of the low-energy electron emission spectra remains a key challenge in simulations.51,52 It remains to be elucidated, if the nanoscale physical effects contribute to enhanced ROS creation observed during nanoparticle irradiation with protons, or whether they are solely created by catalytic effects.40,46,53–55
Quantifying physical dose enhancement.
To assess and gain insights into physical dose enhancement by various NPs, macroscopic calculations56 and Monte Carlo simulations27,29,30,57 can be employed to predict the physical NP dose enhancement effect at the nanoscopic to macroscopic scale. These can be complemented by experimental observations of physical dose enhancements in acellular (cell-free) systems, although such observations are generally more complicated to perform and interpret. A few studies are available and generally in line with simulations. For instance, nanoparticle-impregnated DL-Alanin wax pellets have demonstrated dose enhancements of approximately 60% (for kV X-rays), 10% (for 10 MV X-rays), and ≤5% (for proton and electron beams) for gold nanoparticles.58 Nanoparticle-loaded water-equivalent PRESAGE dosimeters have been used to show physical dose enhancement of Au, Bi2S3, and Bi NPs, with a higher dose enhancement from kV X-rays (12–32%) than from a clinical 6 MV X-ray beam (2–5%).59 The use of PRESAGE dosimeters is limited, however, due to the optical readout method, which is susceptible to NP interference, especially at higher NP concentrations. Alternative methods using MRI or CT as readout methods are found in other polymer dosimeters,60 such as MAGIC (Methacrylic and Ascorbic acid in Gelatin Initiated by Copper),61,62 MAGAT (methacrylic acid gelatine and tetrakis (hydroxymethyl) phosphonium chloride),63 or nPAG (normoxic polyacrylamide).64 Most of the studies using gel dosimeters, or similar methods, focus on dose enhancement with Au NPs. Thus, a systematic comparison of different NPs in such systems is missing. A more detailed knowledge of the system, coupled with Monte Carlo simulations, could provide useful understanding of the physical mechanisms of nanoparticle dose enhancement.
3.2 Chemical enhancement
Generally, in vitro and in vivo dose enhancement findings have greatly exceeded predictions made by simulations of physical enhancement under clinically relevant photon or particle beam irradiation conditions, suggesting that chemical and biological effects play crucial roles.51,65–67 Increased ROS generation has been suggested as a major driving force of NP-enhanced radiation damage.68 Several in vitro studies have shown that ROS quenchers decrease the nanoparticle-induced enhancement effect.55,69,70 The mechanisms of NP-based ROS formation in cells, however, are complex and occur on different levels. NPs can enhance ROS formation through secondary electron emission, which is the chemical follow-up stage to the aforementioned physical dose enhancement processes. Furthermore, ROS formation can be increased by catalytic nanoparticle surface processes, such as lowering the ionizing potential of surrounding molecules71 or acting as an electron or hole donor (Fig. 2d).72,73 The latter process is commonly found in semiconductor nanoparticles and is most effective when the potentials of the valence and charge bands are suitable for water splitting.74,75 In a comparison of different semiconductor nanomaterials, our group has shown that TiO2 and WO3 nanoparticles have higher ROS generation ability compared to HfO2, TiN and SiO2 during the irradiation with X-rays and protons.55 The nanoparticles also generated more ROS under X-ray compared to proton irradiation. Other chemical enhancement mechanisms include upregulation of the local oxygen concentration via exogenous oxygen delivery or catalytic decomposition of H2O2 (to overcome hypoxia) or turning H2O2 into more toxic ˙OH radicals via Fenton or Haber–Weiss reactions (Fig. 2d).68,76,77 Successful modulation of the hypoxic tumor microenvironment has been demonstrated using a variety of particles (such as perfluorocarbon-, hemoglobin-, metal–organic framework-, Mn-, Pt-, or Fe-based nanoparticles), and a comparison of their oxygenation efficiency can be found in a review by Li et al. (2021).78 Fenton agents for cancer therapy include Fe-based nanomaterials or redox-active transition metals (such as Cu, Mn, Ag, V, Co, and W),76 and the reader is referred to reviews from Cao et al. (2021) and Zhang et al. (2021) for a perspective on Fenton/Fenton-like agents or ROS elevating nanomedicines.77,79 Interestingly, nanoparticle surface bound ions as well as released ions can contribute to Fenton reactions. For example, the controlled release of Fe ions from FePt NPs in lysosomal conditions (acidic pH), led to more increased ROS formation by the catalytic decomposition of H2O2.80 In a study with iron oxide NPs and iron ions, ROS concentrations were increased by ions and by the NP surface after X-ray exposure.81 In another study with CuO NPs and dissolved Cu ions, it was found that via Fenton-like and Haber–Weiss reactions the NP surface contributed to a significant portion of the observed amount of ROS and plasmid DNA damage.82 Such reactions with copper ions have also been shown to enhance ROS and cell death after X-ray exposure.83,84 The functionalization of NP surfaces can provide an additional strategy to modulate ROS generation (Fig. 2d). However, since surface modifications can decrease the surface reactivity inhibiting ROS generation,85 they have to be chosen carefully. For example, it was shown for different PEG and human serum albumin functionalizations of Au NPs, that the amount of ˙OH radical production and plasmid DNA damage during X-ray irradiation dramatically decreased with the number of atoms in the coating.86 On the contrary, surface functionalization can be used to enhance charge separation and transfer and improve catalytic reactions,87 or allow the creation of other highly reactive species such as singlet oxygen (1O2) which can be produced by e.g. porphyrins.88 Ideally, a nanoparticle coating has several beneficial therapeutic effects. For instance, surface coatings of mixed-phase iron oxide NPs resulted in more reactive surfaces during X-ray exposure while also improving their biocompatibility.89
Quantifying chemical enhancement.
Several methods are available to detect ROS such as electron spin resonance (ESR/EPR), fluorescent/chemiluminescent probes or proteins, chromatography/spectrophotometry methods, and electrochemical biosensors, each having its advantages and disadvantages.90 Fluorophores or chemiluminescent probes provide an easy means of measuring irradiation-induced ROS in cells or in acellular nanoparticle solutions using a microplate reader or fluorescence microscope. To measure ROS in acellular nanoparticle solutions, assays need to be optimized and nanoparticle–fluorophore interferences need to be understood and minimized.91–93 Frequently used ROS fluorophores include 2,7-dichlorofluorescein diacetate (DCFDA; unspecific ROS or nitrogen species),55,91,94 coumarin-3-carboxylic acid (3-CCA)/7-hydroxycoumarin (˙OH specificity),95–97 Amplex® Red (H2O2),98 dihydrorhodamine (DHR; ˙O2−, ONOO−, ˙OH specificity),99,100 3-(p-aminophenyl) fluorescein (APF; ˙OH, ONOO−, OCl− specificity),101,102 dihydroethidium (DHE; ˙OH, ˙O2− specificity),96,102 singlet oxygen sensor green (SOSG; 1O2 specificity),96 CellROX (unspecific ROS),103 and MitoSOX (mitochondrial ˙O2− specificity).68,104,105 Indirect harvesting methods can also show the importance of certain radicals during nanoparticle-enhanced radiation therapy. Dimethyl sulfoxide (DMSO), for instance, has been applied as a hydroxyl radical scavenger during the irradiation of cells with and without nanoparticles to show the importance of those radicals in successful radiotherapy and nanoparticle enhancement mechanisms.55,69,70,106
3.3 Biological sensitization mechanisms
In radiotherapy, tumor control and normal tissue toxicity are determined by the total dose, fraction size, number of fractions, time between fractions and the overall treatment time. Increasing the fraction size and decreasing the time between fractions and the overall treatment time improve tumor control, but at the same time, decrease the normal tissue protection effect of fractionation. Mechanistically, the differential tumor and normal tissue responses could be linked to the so-called 5 Rs of radiotherapy, namely repair, redistribution, repopulation, reoxygenation and intrinsic radiosensitivity. These “hallmarks of radiotherapy” group the plethora of molecular processes induced by fractionated radiotherapy into biologically relevant concepts. Lately and based on the advancement of immunotherapy and single high dose radiotherapy, an additional 6th R has been proposed, which is the reactivation of anti-tumor immune response. These Rs of radiotherapy have often been used as guidelines for the development of novel combined treatment modalities, to understand differential response patterns to low dose fractionated versus hypofractionated radiotherapy and to different radiation modalities. Nanoparticle radioenhancers will sensitize both tumor and normal cells to ionizing radiation and it will be important to identify which R of radiotherapy and related biological process is most affected or could be exploited to further increase a therapeutic window and to even design a personalized regimen with NPs (see Fig. 2e).
Repair.
Human cells have developed sophisticated DNA damage repair machineries to guarantee genomic integrity after an insult. NPs have been shown to interfere with a cell's DNA repair system, reducing the capability of cancer cells to respond to ionizing radiation-induced DNA damage and thereby increasing treatment efficacy. Au NPs may be capable of slowing down the repair machinery107–109 (as opposed to inducing more DNA double-strand breaks) and downregulating DNA repair genes such as BRCA1,110 MSH3,111 and MRE11A111 [see also review by Penninckx et al. (2020)112 for details]. How exactly Au NPs exert this influence is unclear and requires further investigation. Effects on DNA repair have also been observed with other materials. For instance, Wojewodzka et al. (2011) reported that Ag NPs delayed repair of DNA damage inflicted by kV X-rays in human HepG2 cells, while TiO2 NPs were less effective.113 However, a prolonged, 2 month exposure to TiO2 has been shown to impair DNA repair processes in A549 cells, suggesting that chronic exposure to TiO2 sensitizes cells towards genotoxic agents.114,115 In another study, Ti nanotubes were shown to decrease DNA repair efficacy in human glioblastoma cells.116 A study by Štefančíková et al. (2016) indicated that Gd-based NPs (AguIX), currently in phase 2 clinical trials, had no impact on the creation of DNA lesions or DNA repair in glioblastoma cells under irradiation with gamma rays.117 Nanomaterials can be categorized into 4 main groups in an attempt to forecast the modes of action not only towards apical toxicity effects, but also towards impacting DNA repair processes: (i) soluble nanomaterials that would release metal ions in their surrounding environment (e.g. Ag, ZnO, CuO, or CdSe NPs); (ii) biopersistent high aspect ratio nanomaterials which show fibre-like effects (e.g. carbon nanotubes); (iii) passive nanomaterials that carry a non-reactive surface; and (iv) active nanomaterials with reactive surface properties that may activate or inversely inactivate biological molecules and reactions (e.g. TiO2 or CeO2).118,119 One common mechanism influencing DNA repair, that can apply to nanomaterials of all 4 groups, is the sequestration of DNA repair proteins in the nanomaterial protein corona.118
Redistribution.
A classic rationale for the use of fractionated radiotherapy is the differential radiosensitivity of cancer cells in different phases of the cell cycle, which may be exploited to synchronize cancer cells into radiosensitive phases.120 Interestingly, nanoparticles can also lead to cell cycle synchronization. However, this might be highly cell specific, and might also be influenced by nanoparticle size or surface. For instance, some groups could not show that Au NPs have significant cell cycle effects,109,121–123 while others could demonstrate an NP-induced shift of the cellular cell cycle distribution into a more radiosensitive cell cycle phase thereby increasing the response to irradiation.124–126 Altered gene expression, for instance of cyclins and checkpoint inhibitors, might be one of the underlying mechanisms,124,125,127 but a detailed understanding is currently lacking. In addition to Au NPs, Gd NPs128 and Ag NPs129 were also reported to display G2/M phase arrest in F98 rat glioma and U251 human glioblastoma cells, respectively.
Last but not least, a NP-dependent increase of ROS-generation in response to irradiation might also lead to enhanced levels of DNA damage per se, with subsequently more cytotoxic chromosomal aberrations and differential cell cycle checkpoint activation. Corrupted DNA repair machineries and cell cycle checkpoints, which are often abundant in tumor cells but not in untransformed cells, might thereby contribute to an increased therapeutic window, and could be further enhanced on a personalized level. For example, DNA damage in response to high-energy proton irradiation requires different DNA repair machineries than in response to photon irradiation,130,131 which could be exacerbated in combination with NPs.
Repopulation.
Tumor control in response to fractionated radiotherapy might not be reached in highly proliferating tumors due to (accelerated) repopulation, an event in which tumor cells (possibly tumor stem cells) rapidly proliferate after receiving sublethal irradiation.132 NPs may be employed to decelerate cell growth and to hamper repopulation. Au NPs have been shown to negatively affect proliferation of certain cell lines, for instance by cell cycle arrest, and revascularization, which could be mechanistically linked to direct binding to heparin-binding growth factors thereby impeding VEGF-dependent angiogenesis,133–135 and limiting the secretion of inflammatory cytokines (IL-6, IL-1β).136 In contrast, repopulation could also lead to the diffusion of administered NPs in the tumor, as shown for Au NPs,137,138 which in turn can limit NP-mediated radiosensitization. Furthermore, it will be important to investigate to which extent NPs will interfere with the proliferation of normal tissue to manage acute normal tissue toxicities.
Reoxygenation.
As discussed above and independent of the cellular genotype, hypoxic cells are up to three-fold more radioresistant than normoxic cells, which is explained by the oxygenation fixation theory. This theory implies that radiation-induced free radical sites in the DNA are chemically derivatized (“fixed”) in the presence of oxygen so that they cannot be repaired and accumulate, leading to an enhanced rate of cell death. Furthermore, normoxic conditions favor the generation of reactive oxygen species, in particular superoxide and hydroperoxyl radicals, in response to ionizing radiation which eventually results in a higher amount of DNA damage.14,139 Fractionated irradiation exploits the phenomenon of (iterative) reoxygenation in which hypoxic cells become reoxygenated and subsequently more radiosensitive, i.e. a dose of ionizing radiation will preferentially kill the normoxic cell population and the remaining cell population with a higher relative proportion of hypoxic cells will become reoxygenated (and more radiosensitive) by the microenvironment thereafter to be killed by the next dose of a fractionated treatment schedule. The presence of oxygen and the generation of ROS are also important for NP radioenhancement. For instance, results from Au NP radioenhancement studies have shown that enhancement is greater under normoxia than hypoxia,108,140 and dampened in the presence of radical scavengers.69,141 Though Au NPs alone cannot reoxygenate tissue, they can be coupled to an oxygen reservoir such as liquid perfluorooctyl bromide (PFOB), which rapidly releases O2 upon ultrasound (US) treatment, in order to relieve hypoxia, generate more ROS and prevent DNA repair, leading to a higher radiotherapeutic effect.142 In contrast, other inorganic nanomaterials can generate O2 by dissolution (e.g. CaO2)143 or via an enzyme-like decomposition of H2O2. In the latter case, MnFe2O4 nanoparticles have successfully been used to increase the local pO2via the decomposition of H2O2 in in vivo tumor tissues to render radiotherapy more efficient.144 Similar, catalase-like, effects were observed with a variety of materials, e.g. CeO2145 and V2O5146 (for details see review by Ruan et al. (2021)147). Targeting hypoxic cancer cells and inducing reoxygenation are important steps in overcoming radioresistance, and more emphasis should be placed on developing appropriate materials and unraveling their underlying mechanisms.
Intrinsic radiosensitivity.
On the preclinical level, it is very well established that the genetic make-up of tumor cells and normal tissue influence the treatment response to irradiation. However, insights on the genetic background and its impact to a differential radiation response – have not yet developed into clinical radiotherapy strategies on the personalized level.148 Irradiation may induce different modes of cell death in different tumor entities in dependence of the genetic and normal tissue background they derive of. A widely accepted hypothesis suggests that the presence of acquired mutations can render cells more or less prone to programmed cell death, with a prominent example being p53.149 Therefore, nanoparticles that are able to modify the cell intrinsic radiosensitivity through inhibition of specific targets responsible for treatment resistance of the cancer cell would be interesting for therapy. Au NPs have been implicated in inducing apoptosis, for instance by activation of caspases and, as a result, triggering the rupture of the mitochondrial membrane and the release of cytochrome c,150 thereby aiding in counteracting intrinsic radiation resistance effects (e.g. from p53 mutations).8 Similar effects have been observed with, e.g., silver151,152 and titania NPs.153 However, none of these studies were specifically conducted with a focus on intrinsic radiosensitivity. There are only few studies available correlating the nanoparticle radioenhancement efficiency to the intrinsic radiosensitivity of cell lines. Marill et al. (2014) found a positive correlation between dose enhancement of HfO2 (NBTXR3) NPs and the intrinsic radiosensitivity of different cancer cell lines in vitro.24 The underlying mechanisms remain unknown. At this stage and taking our lack of mechanistic understanding into consideration, it will be more important to identify optimal NPs with a selective advantage for general tumor cell versus normal tissue radiosensitization than the design of NPs directed for specific genetic tumor cell make-ups.
Reactivation of an antitumor immune response.
On the clinical level, the recent achievements in imaging technologies and highly conform radiotherapy have resulted in a shift from classic low-dose fractionated radiotherapy regimens to hypofractionated and stereotactic body radiotherapy applying single or only a few high dose radiotherapy fractions to individual tumor sites. While previously thought to be immunosuppressive, recent studies have clearly demonstrated an important relationship between hypofractionated radiotherapy and the immune system. Its exploitation can achieve impressive responses, which have not been observed by conventional fractionated radiotherapy. Indeed, high doses of irradiation has been shown to instigate potent immune responses, and has been classified as an ‘in situ vaccine’, whereby the induction of immunogenic cell death (ICD) sets off an inflammatory cascade. This results in the release of antigens, damage-associated molecular pattern (DAMP) molecules and type 1 interferons, activating antigen presenting cells such as dendritic cells (DCs) in the tumor. In turn, DCs upregulate co-stimulatory molecules, which facilitate successful priming of CD8+ T cells, ultimately resulting in the recruitment of antigen specific immune cells to the tumor. While in some cases this leads to an effective and efficient anti-tumor response,154 radiotherapy alone is often not sufficient enough to overcome the immunosuppressive tumor microenvironment (TME), and as such the potency of infiltrating immune cells is often hampered. A combined treatment modality of NPs and radiotherapy does result in enhanced direct cytotoxicity and tumor control mediated via the multiple mechanisms related to the first Rs of radiotherapy. Eventually increased amounts of small DNA fragments, antigen presentation, and release of DAMPs may also stimulate immunogenic cell death as demonstrated for Au155 and HfO2 NPs156 and might even lead to a systemic immune response towards abscopal effects alone and in combination with immune response stimulatory agents.157,158 In a study with anti-PD1–resistant tumor model mice, HfO2 nanoparticles in combination with radiotherapy and anti-PD1 produced abscopal effects.157 In another study, the combination of HfO2 nanoparticles with radiotherapy and NF-αCTLA4 and αPD1 check point inhibitors improved the control of local tumors and metastases.159 However, at this stage, we have insufficient insights how to rationally direct these processes with NPs towards immunogenic cell death and an increased therapeutic window.
The emerging understanding of the underlying biological mechanisms for nanoparticle-based radioenhancement is important for the design of safe and effective nanomedicines. However, studies focusing on materials other than gold are scarce. Further (comparative) investigations into relevant materials including hafnium, titanium, iron, gadolinium, and silver metals are required to understand how these particles interact with biological matter in the context of radiation biology. As has been pointed out previously, the nanotoxicology field might be an underutilized resource in this regard, and collaborative efforts with this field should be established and reinforced.
Quantifying biological mechanisms contributing to damage.
A wide range of methods has been established to study the biological effects of nanoparticles. Specific, often well-established, assays linked to the 6 Rs may be used. Examples include the comet160 and γ-H2AX117,161 assay to address DNA damage and repair, the TUNEL162,163 assay and micronuclei counting to assess apoptosis and quantify mitotic catastrophe, respectively, or fluorophores such as H2DCF-DA to assess in vitro ROS production. In addition, gene and protein expression studies may provide a powerful tool to identify treatment-induced signaling processes and to improve our mechanistic understanding. A common and effective approach to investigating the overall radiation response in vitro is the clonogenic cell survival (or colony formation) assay, established over 60 years ago and is considered to be the gold standard to quantify164 the cells’ reproductive abilities in response to irradiation. However, the clonogenic cell survival assay is tedious and time-consuming and offers very low sample throughput.165,166 As such, metabolic-based assays (e.g. MTT or CellTiter-Glo®) can in part overcome these limitations and are often used as a first screening approach to identify reasonable NP concentration and ionizing radiation dose ranges to probe novel combined treatment modalities on the in vitro level, followed by the more exact clonogenic cell survival assay.94,165,167 See, for example, Subiel et al. (2016)168 for a detailed review on radiobiological techniques and their quantification in vitro. Eventually, the combined treatment modality will have to be probed in respective in vivo tumor models (subcutaneous vs. orthotopic tumors; immuno-compromised versus immuno-competent hosts) in order to evaluate NP-mediated radioenhancement towards an enlarged therapeutic window.
4. Nanoparticles candidate materials with radioenhancing properties
Early findings of using substances to enhance radiation damage by means of the photoelectric effect go back to the 1980s, where iodine contrast medium was found to sensitize cells to X-rays.169,170 Before that, it was already well known, that the dose absorbed by tissue at the boundary to a higher-Z material (such as bone) is greatly enhanced.171,172 Around two decades ago, gold metal foil or microspheres have been shown to enhance the cytotoxic effect of ionizing radiation due to the release of secondary radiation using kV X-rays.173,174 Using platinum-DNA complexes, Kobayashi et al. were able to conclude from their experiments, that platinum atoms acted as enhancers of X-ray-induced DNA breaks by increasing the production of hydroxyl radicals due to photoelectric and Auger effects.175 Until now, several metals and metal oxides have been investigated as NP radioenhancers, either in silico, in vitro, or in vivo, with Au NPs being the most extensively studied formulation in literature.1,67 They appear to be an intuitive choice as they possess seemingly good biocompatibility, passive accumulation in tumors, low toxicity, and easy synthesis methods.25,38 Most importantly, their atomic number is very high (Z = 79), which is particularly relevant for kV photon interactions. Nevertheless several studies indicated a decreased, yet appreciable gold nanoparticle radioenhancement effect at MV vs. kV X-ray treatment.109,176 Hafnium-based NPs have already completed clinical trials, and gadolinium-based NPs have entered trials; both display good dose enhancement effects under X-ray irradiation.4,24,177,178 In addition, various other metal-related materials have proven to be effective enhancers of X-rays, such as materials with silver, platinum, titanium, tungsten, iron (SPIONS), zinc, or bismuth metals (Table 1 and Fig. 3). All materials listed in Table 1 show promising therapeutic efficiency in vitro and in vivo for kV or clinically relevant MV irradiation. Radioenhancement success is mostly described by increased DNA damage, ROS levels, and apoptosis levels. While chemical and physical interaction of nanoparticles and irradiations are easily accessed and well described, in-depth biological response mechanisms are investigated only in some cases. While the therapeutic performance of single nanoparticles can be tailored by size or surface functionalization, hybrid materials offer the integration of multimodal imaging and/or multimodal therapy options.
Table 1 Studies on single or hybrid nanoparticle (NP) formulations
NP type |
Model |
Beam sources |
Important outcomes |
Ref. |
CT: computer tomography; MR: magnet resonance; MSOT: multi-spectral optoacoustic tomography; PDT: photodynamic therapy; PTT: photothermal therapy; RT: radiotherapy |
Single metal and metal oxide nanoparticles
|
TiO2 (un-/doped) |
PRESAGE phantom |
kV Photons |
• X-ray energy differential therapeutic effects observed in PRESAGE |
94 and 182–184
|
In vitro
|
MV photons |
• NP radioenhancement effect during MV X-ray irradiation explained by increased ROS generation |
In vivo
|
|
• Rare earth dopants (e.g. Sm, Gd, Nd, Eu, Er, Tb) increased X-ray induced ROS, and apoptosis markers |
|
(PAA-) TiO2/H2O2 |
In vitro
|
kV Photons |
• NPs increased intracellular H2O2 concentrations by gradual release thereof leading to increased radiotherapy efficiency |
163, 185 and 186 |
In vivo
|
|
• Increased ˙OH radical and H2O2 concentration, DNA damage and apoptosis during X-ray irradiation |
|
MnFe2O4 |
In vitro
|
kV Photons |
• Oxygen delivery via catalytic H2O2 decomposition |
144 and 187
|
In vivo (normoxia and hypoxia) |
MV photons |
• Hypoxic conditions were alleviated in vitro and in vivo |
|
|
• Decreased HIF-1a levels, and increased apoptosis and DNA damage under irradiation during hypoxic conditions |
|
|
• Immune-modulating effect: suppression of PD-L1 expression and increased infiltration of T cells even after irradiation |
|
|
• PEGylated NPs showed good cytocompatibility, passive tumor accumulation, O2 generation via H2O2 decomposition, GSH consumption via glutathione-peroxidase-like activity, enhanced ROS and double-strand break levels in hypoxic conditions, and hypoxia attenuation and good radioenhancement efficiency in vivo. |
|
SPIONS (γFe2O3, Fe3O4) |
In vitro
|
kV Photons |
• Excellent biocompatibility |
81
|
|
|
• Increased ROS production via Fenton and Haber–Weiss reactions from released iron ions and catalytically active nanoparticle surface |
|
|
• X-ray irradiation led to additional oxidative stress from increased catalytically active nanoparticle surfaces |
|
CuO |
In vitro
|
MV photons |
• Increased ROS levels with X-ray treatment and CuO NPs |
83
|
In vivo
|
|
• Increased radiosensitivity by the NP-induced modulation of the cell cycle distribution towards increased G2/M phase |
|
|
• Increased level of self-destructive autophagy observed with the combination of CuO NPs and X-rays |
|
ZnO |
In vitro
|
kV Photons |
• Radioenhancing effects explained by increased apoptosis and DNA damage; oxidative stress as possible driver identified |
188–191
|
In vivo
|
MV photons |
• Discussed cytotoxic effects of ZnO: dissolution of Zn++ in acidic conditions; e−h+ pair production even in the dark leading to surface ROS production |
|
|
• Discussed genotoxic effects of ZnO: oxidative stress |
|
|
• Gd-doped ZnO NPs increased cells in G1 phase and decreased DNA repair efficiency |
|
|
• ZnO-CaffeicAcid NPs showed radioenhancement via ROS/oxidative stress generation, DNA damage, DNA repair, and mitochondrial dysfunction, suppression of cell cycle checkpoint machinery and cell death promotion (via apoptosis, necrosis, and disregulations of gene and protein expressions) |
|
Y2O3 |
In vitro
|
kV Photons |
• Increased ROS and DNA double-strand breaks with NPs alone or in combination with X-rays |
103
|
|
|
• NPs affected irradiation induced DNA damage and repair response |
|
|
• Synergistic effects of NP treatment and irradiation proposed by clonogenic assay |
|
Ag |
In vitro
|
MV photons |
• Increased apoptosis levels after irradiation with Ag NPs |
192–197
|
In vivo
|
|
• Antiproliferative activity in combination with radiotherapy |
|
|
• Decrease of mitochondria membrane potential, promotion of apoptosis and enhanced destructive autophagy under irradiation in hypoxic conditions with Ag NPs |
|
|
• Increased ROS and protective autophagy during irradiation with Ag NPs |
|
|
• Radiosensitization might be Ag+ cation release dependent |
|
Gd chelate (AGuIX) |
In vitro
|
kV Photons, |
• Good safety profile |
2, 178 and 198–202 |
In vivo
|
In human |
• Rapid and safe renal elimination |
|
MV photons |
• Preferential accumulation in tumor due to EPR effect after intravenous injection |
|
MeV hadrons |
• Possible emission of low-energy photoelectrons and Auger electrons leading to higher ROS |
|
|
• Dose enhancement higher for kV than MV photon, and C6+ than He2+ ion irradiation |
|
|
• Photon radiation-induced ROS and DNA double-strand break increase and DNA repair reduction observed |
|
|
• Ion irradiation increased DNA damage mediated by ROS |
|
Gd2O3 |
In vitro
|
kV Photons |
• Compared to a solution of separate Gd-atom species, Gd2O3 NPs showed higher ROS generation, suggesting a Gd–Gd interatomic de-excitation-driven nanoradiator effect |
203 and 204
|
|
MeV protons |
• Increased hydroxyl radical and ROS production, increased DNA double-strand breaks and cell cycle arrest at G2/M phase |
|
C6+ ions |
• Increased apoptosis and cytostatic autophagy identified as radiosensitization mechanism |
|
HfO2 (NBTXR3) |
In vitro
|
kV Photons |
• NBTXR3 has a good safety profile and improves radiotherapy efficiency via physical mechanisms |
4, 24, 156–159 and 205–208 |
In vivo
|
In human |
• Increased necrosis, DNA double-strand breaks, micronuclei formation and an activation of the cGAS-STING pathway detected after irradiation |
|
MV photons |
• In combination with radiotherapy: enhancement of early apoptosis, early necrosis and late apoptosis/necrosis; abscopal effects driven by an increased CD8+ cell infiltration |
|
|
• Modulation of the immunopeptidome observed in vivo leading to an anti-tumor immune response |
|
WO3−x |
In vitro
|
kV Photons |
• Increased DNA double-strand breaks and apoptosis after irradiation with NPs |
209 and 210
|
In vivo
|
|
• Remarkable synergistic effect of radiotherapy and phototherapy (PTT/PDT) |
|
Pt |
In vitro
|
kV Photons |
• ROS scavenging capabilities of Pt NPs during irradiation detected, which might counteract nanoparticle radioenhancement |
70 and 211–213
|
|
MV photons |
• Pt NPs amplified radiation therapy by confined production of ROS in nano-volumes around nanoparticles |
|
C6+ ions |
• Amplified DNA damage detected during hadron therapy |
|
Au |
In vitro
|
kV Photons, |
• Physical, chemical and biological mechanisms described |
1, 45, 104, 109, 121, 124, 176 and 214–220 |
In vivo
|
MV photons, |
• Less radioenhancement observed at MV compared to kV irradiations |
|
Protons |
• Observed enhancement effects at MV energies cannot be explained by physical effects |
|
|
• Enhancement effects are cell-line specific |
|
|
• Cell cycle arrest in G2/M phase has been observed for some cell lines |
|
|
• Cytoplasmic damage can drive DNA damage; mitochondrial function identified as possible driver |
|
|
• Increased DNA dmage and/or mitochondrial and/or ER stress with and/or without irradiation lead to increased apoptosis and/or necrosis |
|
|
• Au NPs have radiosensitization effect via various biological mechanisms, such as weakening the detoxification system |
|
Bi2O3 |
In vitro
|
kV Photons |
• In vitro enhancement ratio for kV irradiation higher compared to MV irradiation |
62, 66 and 221 |
|
MV photons |
• Monte Carlo studies predicted dose enhancement for kV irradiation but not for MV irradiation |
|
|
• Radiocatalytic surface of NPs hypothesized to lead to water splitting |
|
BiFeO3 |
Gel dosimetry |
kV Photons |
• Promising results in radiotherapy amplification, magnetic hyperthermia and imaging |
222
|
In vitro
|
|
|
|
BiGdO3 |
MAGIC gel dosimeter |
kV Photons |
• Multifunctional pegylated nanoparticles showed radiation enhancement in gel dosimetry, in vitro and in vivo. |
223
|
In vitro
|
|
|
In vivo
|
|
|
|
Bi2WO6 |
In vitro
|
kV Photons |
• Bi2WO6 generated radiocatalytic ROS in an acellular system, with ˙OH as main oxidative species identified |
224
|
In vivo
|
MV photons |
• Radiosensitization in vitro was found in a clonogenic assay together with increased ROS and DNA double strand break levels |
|
|
• Efficient radiotherapy enhancement shown in vivo without toxicity effects in the time span of 30 days. |
|
Hybrid nanoparticle systems
|
Au–TiO2 hybrid |
In vitro
|
kV Photons |
• Production of large amount of ROS during irradiation |
225
|
In vivo
|
|
• Synergistic X-ray therapeutic effect of Au and TiO2 due to their interfacial contact |
|
Au@MnO2-PEG |
In vitro
|
kV Photons |
• O2 generation from H2O2 decomposition via MnO2 shell and relieve of cellular hypoxia |
226
|
In vivo
|
|
• H2O2 decomposition led to Mn2+ release and enhanced T1-weighted MR imaging |
|
|
• Compared to the individual components, Au@MnO2 core@shell hybrid NPs led to synergistic radioenhancement effects in vitro and in vivo and to more increased double-strand breaks and apoptosis levels |
|
Au@Pt nano-dendrites |
In vitro
|
Photons (energy N/A) |
• Integration of CT imaging, PTT & RT with synergistic therapeutic effects |
227
|
|
Au:Pt-PEG |
Plasmid DNA
|
kV Photons |
• NPs enhanced the MV X-ray induced double-strand breaks in plasmid DNA mainly by influencing the chemical stage that takes place after the physical interaction |
228 and 229
|
In vitro
|
MV photons |
• NP radioenhancing effect with kV X-rays observed in vitro and in vivo by enhancing double-strand breaks, apoptosis and relieving of hypoxia via H2O2 decomposition |
In vivo
|
|
|
|
WS2:Gd3+-PEG 2D-nanoflakes |
In vitro
|
Photons (energy N/A) |
• Triple-modal imaging: CT/MR/photo acoustic |
230
|
In vivo
|
|
• Synergistic PTT/RT therapeutic effects observed |
|
|
• X-ray enhanced DNA double-strand breaks observed |
|
Gd2(WO4)3:10%Tb@PEG/MC540 |
In vitro
|
kV Photons |
• X-ray induced generation of 1O2 observed from photo excitation of surface coupled merocyanine (MC) 540 |
231
|
In vivo
|
|
• Synergistic radioenhancement and PDT effects observed in vitro and in vivo |
|
|
• Dual-modal imaging properties (CT/MR) |
|
Fe3O4@Ag |
In vitro
|
MV photons |
• Synergistic radioenhancement effects of the Fe3O4 core and Ag shell as compared to the individual components alone |
232
|
|
|
• Radiosensitivity enhancement through decrease of the cytoprotective autophagy at an early stage, followed by an increase of calcium-dependent apoptosis at a later stage |
|
Gd2O3/BSA@MoS2-HA |
In vitro
|
kV Photons |
• Enhanced DNA double-strand breaks in combination with X-rays in vitro |
233
|
In vivo
|
|
• In vivo tumor growth inhibition in combination with X-rays |
|
|
• Best therapeutic effects in combination with X-ray and PTT treatments |
|
|
• In vivo multimodal imaging properties (MSOT/CT/MR) |
|
BiNPs@SiO2@BamCS/PCM |
In vitro
|
kV Photons |
• Acellular detection of elevated ROS (˙OH, 1O2 and ˙O2−) under X-ray irradiation |
234
|
In vivo
|
|
• Elevated cell death, ROS and DNA double-strand breaks detected in combination with X-rays in vitro |
|
|
• Depleted GSH levels due to hyperthermia-released and H2O2-activated proalkylation agent BamCS |
|
|
• Excessive ROS and irreversible depletion of endocellular GSH led to cell death via mitochondria-mediated apoptosis pathway |
|
|
• Tumor growth inhibition in combination with X-rays |
|
|
• Synergistic PTT/RT effects observed |
|
Nanoscale metal–organic frameworks (nMOFs)
|
MnTCPP–Hf–FA |
In vitro
|
kV Photons |
• O2 generation via catalytic H2O2 decomposition |
235
|
In vivo
|
|
• Decreased HIF-1a expression suggested hypoxia alleviation due to intracellular H2O2 decomposition and O2 generation |
|
|
• Increased ROS levels and DNA double-strand breaks after irradiation even in hypoxic conditions |
|
UiO-66-NH2(Hf) |
In vitro
|
Photons (energy N/A) |
• Radioenhancement due to increased ROS levels, DNA double-strand breaks and cell apoptosis |
236
|
In vivo
|
|
|
|
Hf6-DBB-Ru |
In vitro
|
kV Photons |
• MOFs accumulated in mitochondria through cationic nature of Ru-based photosensitizer |
237
|
In vivo
|
|
• Irradiation induced ˙OH radical generation from Hf6 units and 1O2 generation from the DBB-Ru photosensitizer |
|
|
• Clonogenic radioenhancement effects observed, along with increased double-strand break, 1O2, lipid peroxidation (COX-2 upregulation) and apoptosis/necrosis levels after irradiation |
|
|
• Irradiation induced depolarization of the mitochondrial membrane leading to cytochrome c release and caspase-3 activation (apoptosis pathway) |
|
|
• Effective in vivo tumor regression after intratumoral or intravenous nMOF administration and low dose (6 or 8 Gy) X-ray therapy, with increased levels of necrosis and apoptosis. |
|
W18@Hf12-DBB-Ir |
In vitro
|
kV Photons |
• Hierarchical assembly of Hf12, DBB-Ir, and W18 generated 3 distinct ROSs: ˙OH, 1O2 and ˙O2−, respectively |
238
|
In vivo
|
|
• Irradiation in vitro lead to enhanced ROS, DNA double-strand breaks and apoptosis/necrosis |
|
|
• Superb anticancer efficacy (>99% tumor growth inhibition) on two in vivo tumor models with irradiation |
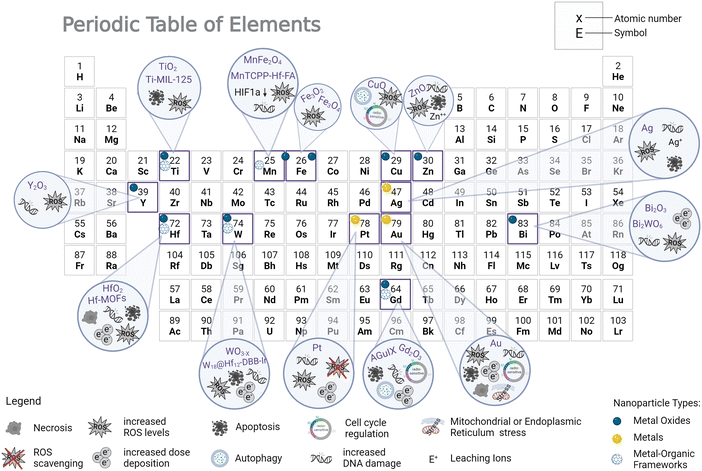 |
| Fig. 3 Overview of major elements applied as radioenhancer candidate materials and suggested mechanisms of action. Elements may be applied in nanoparticulate form as metal, metal oxide or metal organic framework, or in ionic form. | |
To understand which materials are most efficient in radiotherapeutic settings, material comparison studies (in clinically relevant settings) are highly valuable. Table 2 lists a selection of such studies. While in some studies the radio- and ROS-enhancing efficiency scaled with the atomic number,53 other comparison studies showed less atomic number dependency. For example, Ag NPs have outperformed Au NPs due to higher biological radiosensitization mechanisms.179 A recent investigation by Guerreiro et al. found that out of 22 metal oxide NPs examined, TiO2 and V2O5 showed the most significant damage to plasmid DNA probes and increase in ROS generation under 6 MV X-ray irradiation. This draws attention to the importance of physico-chemical surface effects and ROS generation at clinically relevant MeV X-ray energies and highlights the role of photocatalysts (especially TiO2) in radioenhancement.96 These findings also direct attention away from high-Z nanoparticles as clinically relevant radioenhancers and direct it towards photocatalysts, which can also be low-Z nanoparticles. Recent comparisons of low- to high-Z nanomaterials under kV and MV X-rays and under MeV proton irradiation also demonstrated these trends.55 Additionally, metal organic frameworks (MOFs) with highly accessible surface area have been shown to outperform their metal oxide nanoparticle counterpart in ROS generation and radiotherapy efficiency and can even be loaded with therapeutic drugs.101
Table 2 Studies comparing multiple types of nanoparticle (NP) radioenhancers
NP type |
Model |
Beam sources |
Important outcomes |
Ref. |
Nanoparticle comparisons |
Ag and Au NPs |
In vitro
|
MV photons |
• Ag NPs showed higher radiosensitizing ability compared to Au NPs, combined with increased apoptosis and authophagy levels |
179
|
In vivo
|
|
|
|
Pt and Gd-based (AGuIX) NPs |
Plasmid DNA |
MeV protons |
• Pronounced nanosize damage (>2 nm) at the end of proton track (Bragg peak) |
40, 163, 185 and 186 |
|
|
• Gd-based NPs less capable of producing complex lesions than Pt NPs |
|
|
• DMSO (radical scavenger) reduced plasmid damage, hydroxyl radicals identified as important mediators for both NPs |
|
HfO2 NPs, Hf6- & Hf12-DBA MOFs |
APF acellular assay |
kV Photons |
• MOFs generated more ˙OH radicals compared to HfO2 at same Hf molar concentration |
101
|
In vitro
|
Co60 photons |
• Better local radiotherapy outcomes for MOFs than HfO2 |
In vivo
|
|
• MOFs in combination with PD-L1 checkpoint blockade induced systemic antitumor immunity |
|
Au NPs, SPIONS, PtNDs (Pt nano dendrites), BiNRs (Bi2O3 nano rods) |
In vitro
|
MeV protons |
• Proton beam irradiation with nanoparticles enhance ROS creation |
53
|
|
|
• ROS generation and in vitro radioenhancement biggest for BiNRs > PtNDs > Au NPs > SPIONS |
|
Comparison of 22 metal oxides |
Plasmid DNA |
MV photons |
• Surface chemistry of NP important criterion for success |
96
|
Aequous ROS (˙OH, ˙O2−, 1O2) probes |
|
• Only TiO2 and V2O5 showed ROS and DNA damage enhancement and were identified as good radiosensitizers, with V2O5 having a too high toxicity profile |
|
PAA-TiOx NPs and Au NPs |
Aequous ROS probe |
kV Photons |
• H2O2 identified as important mediator of the more effective and generally safe PAA-TiOx NP radioenhancer (Au NPs were tested at significantly lower mass concentrations) |
239
|
In vitro
|
|
|
In vivo
|
|
|
|
SiO2, TiO2, TiN, ZrO2, WO3, HfO2, Au |
Aequous ROS (H2DCF-DA) probe |
kV Photons |
• Dose enhancement efficiency for kV photons follows physical high-Z rationale |
55
|
In vitro
|
MV photons |
• Surface catalytic properties/ROS enhancement important for MV photons and protons |
|
MeV protons |
• TiO2, WO3 performed best in ROS production under all radiation sources |
|
|
• Dissolution of WO3 led to limited radioenhancment |
|
Au and SPION-DX (Dextran coated iron oxide) NPs |
In vitro
|
MV photons |
• Enhancement effects were cell-line differential |
240
|
|
|
• At similar metal-mass uptake Au NPs showed higher enhancement than SPION-DX |
|
MOFs: Hf-DBA, Hf-TCPP, Ti/Zr-PCN-415, Ti-MIL-125 |
In vitro
|
kV Photons |
• Greatest in vitro radio-enhancement effects were found for Ti-MIL-125, followed by Ti/Zr-PCN-415 nanoMOFs |
241
|
Oxide NPs: HfO2, ZrO2, TiO2 |
|
|
• nanoMOFs outperformed corresponding equi-molar metal oxide nanoparticles in in vitro X-ray radio-enhancement |
Nanoparticle radioenhancement studies using proton therapy are less widely available. While the effects of gold NP size, coating, and beam energies under proton irradiation have been investigated extensively through Monte Carlo simulations,38,180 only few materials have been tested in vitro or in vivo with proton irradiation, with the main focus so far on gold, platinum, gadolinium and iron-related materials.40,45 It is perceived that for proton therapy, chemical and biological mechanisms are more relevant than physical mechanisms.181 In view of the pivotal role proton therapy plays for certain patient groups and the ongoing global expansion and advancement of this treatment modality, efforts should be made to enhance the understanding of proton-NP interactions.
5. Nanoparticle-aided augmentation of other radiation therapy techniques
In addition to a combined treatment modality of NPs with external beam radiotherapy, NPs can also be used to enhance the efficacy of other radiation-based cancer treatment modalities, such as brachytherapy or phototherapy. In brachytherapy, radioactive seeds are locally implanted into the tumor tissue and it is therefore also called internal radiation therapy. In combination with kV photon-emitting radioseeds, high-Z nanoparticles can be applied to increase the dose deposition, leveraging the photoelectric effect. Another way of performing brachytherapy is by the incorporation of radioactive elements (such as α, β, or Auger electron emitters) into nanosized agents (such as Au or iron oxide NPs). This technique is called nanobrachytherapy or nanoseed brachytherapy and has made advancements in preclinical research to overcome limitations of traditional millimeter-sized radioactive seeds, such as inhomogeneous dose deposition and technical applications.242 For instance, intratumoral injections of Au NPs labeled with 177Lu (β emitter), 225Ac, or 211At (α emitters) have shown good growth inhibition of breast, glioma, or pancreatic tumors in rodents.243–245 The choice of the radioactive element depends on the therapeutic suitability and involves considering several properties such as decay type, half-life, tissue penetration depth, LET, and toxicity of the parent and daughter element.242 The choice of the radiolabeled nanoparticle also depends on therapeutic and practical factors, such as labeling technique (chelator-based or chelator-free), its ability for surface functionalization, biocompatibility/toxicity, and imaging or multifunctional capability.242 Since most of the radionuclide-emitted particles are in the keV energy range, it is still a matter of debate whether or not an additional amplification via the radioenhancement effects of high-Z nanoparticles can be expected from internal brachytherapy sources. An in silico investigation estimated a radioenhancement effect of up to 20% from iron oxide nanoparticles and different clinically used radionuclides depending on the isotope proximity and nanoparticle clustering distance.246 Similarly, it has been shown via Monte Carlo simulation,247in vitro,248 and in vivo249 experiments that the radioenhancement properties of Au nanoparticles can be leveraged for internal brachytherapy.
Nanoparticles can also be used for (solid) cancer treatment via phototherapy, which uses photon sources of much lower energy (UV to NIR) compared to X-rays. The two most important modalities are photothermal therapy (PTT), in which photons are converted into toxic heat, and photodynamic therapy (PDT), in which photons are converted into the production of ROS.250 For NP-based PTT, nanoparticles ideally should have a high absorption and photothermal conversion efficiency in the NIR-II range (1000–1350 nm) paired with a high biocompatibility and low toxicity.251 Most inorganic NPs create heat via surface plasmon oscillations (also called LSPR, or localized surface plasmon resonance), while organic PTT agents transfer heat via vibrational relaxation upon photoexcitation.251 Nanoparticles turning NIR light into heat that have been used as synergistic PTT and radioenhancing agents, include WO3−x, Au@Pt dendrites, WS2:Gd3+-PEG 2D-nanoflakes, Gd2O3/BSA@MoS2-HA and BiNPs@SiO2@BamCS/PCM (Table 1). For NP-based PDT, fluorescent dye photosensitizers (PSs) are supplied via nanoparticles to the region of interest, where they transfer energy from incoming photons to surrounding oxygen molecules to generate toxic ROS, typically 1O2.252 Most PS molecules alone, such as porphyrins or chlorins, are hydrophobic and prone to aggregate intracellularly, which imposes a quenched fluorescence emission as well as reduced singlet oxygen generation.253,254 While one strategy to overcome this challenge is by developing fluorogens with aggregation induced emission (AIE) characteristics, such as TPECM-2TPP,254 such molecules rely on low-energy excitation wavelengths from laser light sources for efficient excitation. Therefore, nanoparticles for PDT play roles either as a carrier or as an absorber of incoming light that activates the surrounding PS molecule, which has an intrinsic absorbance range. Since UV, visible, and NIR light have low tissue penetration depth, X-ray-triggered PDT has been used to treat deep-seated tumors. X-rays can be converted into a form of light that can excite PSs via light-scintillating nanoparticles (e.g. rare-earth based nanoparticles).255 X-ray-triggered PDT has been proven to be successful in vivo, using, for example, radioenhancing Gd2(WO4)3:Tb nanoparticles as scintillator and merocyanine 540 as PS (Table 1),231 or a metal doped silicate nanoscintillator with rose bengal as PS.256 Photosensitizers can also be integrated into MOFs to additionally produce 1O2 next to ˙OH upon X-ray exposure, as shown for Hf6-DBB-Ru or W18@Hf12DBB-Ir (Table 1).237,238 A rather new type of PDT agent is copper-cysteamine (Cu-Cy) nanoparticles, which can produce ROS, including 1O2, directly under UV light, X-rays, microwaves, or ultrasound, and has shown in vitro and in vivo therapeutic efficacy.257–259
6. Clinical progress
Gold nanoparticles remain the best investigated nanomaterial for the enhancement of radiotherapy, with first preclinical success already achieved in 2004.1 To our knowledge, no gold formulation is being evaluated in combination with radiotherapy in clinical studies. The main challenge of gold nanoparticles that prohibits their translation into clinical usage is the incomplete understanding of their biological fate, safety, and long-term biocompatibility in vivo with respect to its physico-chemical properties.8,260,261 Although not developed as radiosensitizers, a few gold nanoformulations have overcome the toxicity problem and transitioned into clinical studies, including materials such as CYT-6091 (Cytimmune Sciences, USA) or AuroShells® of the AuroLase™ therapy (Nanospectra Biosciences, USA).8 The latter therapy aims to ablate solid tumors using near infrared light, while CYT-6091 is a drug delivery gold nanoparticle with bound tumor necrosis factor-α on its surface. The successful clinical transition of these particles raises the hope for gold nanoformulations also entering clinics as radiosensitizers.
Hafnium dioxide-based radioenhancer nanoparticles (NBTXR3) developed by Nanobiotix are currently under evaluation in several clinical trials, both as a single agent added to radiotherapy or in combination with chemo- or immunotherapeutic agents. Studies with NBTXR3 as a single agent involve the radio-treatment of lung (Phase I, NCT04505267), pancreatic (Phase I: NCT04484909), liver (completed Phase I: NCT02721056), head and neck (Phase III: NCT04892173, Phase I: NCT01946867), and soft tissue (completed Phase III: NCT02379845) cancers. Studies with NBTXR3 that include radiation and immunotherapy are under investigation for the treatment of recurrent head and neck, lung, or liver metastasis (Phase I: NCT03589339, drugs: Nivolumab, Pembrolizumab), head and neck cancers (Phase II: NCT04834349, drug: Pembrolizumab; Phase III: NCT04892173, drug: Cetuximab), or solid tumors (Phase I/II: NCT05039632, drugs: Ipililumab, Nivolumab). Combinational studies of NBTXR3 with radiation and chemoterapeutic drugs are clinically investigated for the treatment of esophageal (Phase I: NCT04615013, chemotherapeutic drugs: capecitabine, carboplatin, docetaxel, fluorouracil, leucovorin, oxaliplatin, paclitaxel) and head and neck (Phase II: NCT04862455, chemotherapeutic agent: pembrolizumab) cancers. Positive completion of phase III clinical trials for the treatment of locally advanced soft-tissue sarcoma, and the subsequent CE Mark approval of NBTXR3 (under the name of Hensify®) in 2019, represents the first demonstration of a radioenhancer to provide therapeutic benefits in synergy with standard radiotherapy treatment methods.207,262
A second radiotherapy-enhancing formulation being evaluated in clinical trials in combination with radiotherapy is a polysiloxane Gd-chelate-based nanoparticle called AGuIX. Current studies investigating the use of AGuIX as a radiosensitizer with radiation alone are focusing on the treatment for brain metastasis (completed Phase I: NCT02820454, Phase II: NCT03818386, NCT04899908) and for lung tumors and pancreatic cancer (Phase I/II: NCT04789486). AGuIX is also evaluated in combination with a chemotherapy drug (Temozolomide) for the radiotreatment of glioblastoma (Phase I/II: NCT04881032) and in combination with chemotherapy (cisplatin) and brachytherapy for the radiotherapy treatment of gynecologic cancers (Phase I: NCT03308604). As a first radiosensitizer, AGuIX is also enrolled for a phase II study for proton therapy of recurrent tumors (NCT04784221). Attempts have recently been made to further optimize AGuIX particles by adding the feature of copper chelation (CuPRiX) with the aim of reestablishing copper homeostasis.263
Another emerging radioenhancement formulation is rare-earth-doped TiO2 nanoparticles (Oxilia), which increase ROS during radiotherapy through water splitting. The company Xerion Healthcare, based in the UK, has shown promising results in the pre-clinical stage in in vitro and in vivo mouse xenograft models, claiming effectiveness of their nanoparticle formulation in pancreatic cancer models.264,265 In 2019, further funding was secured to support clinical investigations, none of which have been initiated to date. It will be interesting to follow the further development and clinical translation of these known photocatalysts as radioenhancers and how they perform in clinical trials in comparison to, e.g. hafnia-based particles.
A few iron formulations are in clinical trials for various cancer therapies.266 Although developed and FDA-approved for the treatment of iron deficiency anemia, Ferumoxytol (iron oxide NPs) may serve as a radiotherapy adjuvant, releasing iron during irradiation.267 Ferumoxytol is now in clinical trials for the therapy of primary and metastatic hepatic cancers (NCT04682847). Though not developed as a radioenhancer, another iron oxide nanoparticle formulation, NanoTherm® (developed by MagForce) is FDA approved and currently in clinical trials for the focal ablation of prostate cancers using a magnetic field (Phase IIb: NCT05010759). Thus, iron oxide might in the future combine and enhance two cancer treatment strategies, hyperthermia and radiotherapy, that possess synergistic therapy potential.268
From the new classes of materials, a MOF formulation, RiMO-301, is now in clinical trials for the radiotherapy of advanced tumors (Phase I: NCT03444714).
The few nanoparticle formulations which are being evaluated in clinical trials are not reflecting the plethora of nanoparticles which have been tested preclinically (see e.g.Tables 1 and 2). The translation of a nanoparticulate material from bench to bedside is a long process with the safety being a key priority. Relevant barriers for the clinical translation of nanoparticle radioenhancers have been identified by a dedicated multi-disciplinary cooperative in 2018.7 Amongst them were (i) the discovery of radioenhancement mechanisms, including the standardization of experimental methods to allow meaningful comparison of nanoparticle systems, (ii) the in vivo fate of nanoparticles, (iii) understanding patient priorities, as well as (iv) the nanoparticle manufacturing and its scalability, which should ideally be integrated into the early nanoparticle design phases. While the knowledge on topics (i)–(iii) is constantly updated by ongoing research activities, it is interesting to note that most, if not all, of the above-mentioned clinical formulations are synthesized by wet-chemical, batch synthesis methods, for which upscaling can pose a big challenge, especially when high nanoparticle quantities become necessary for clinical evaluations or after regulatory product acceptance. Nanoparticle production via continuous flow processing methods, including flame spray pyrolysis, can offer production rates on lab and industrial scales (mg to kg per day), while offering significant versatility in nanoparticle design in a one-step process.269–271 Testing those materials already in pre-clinical stages could accelerate nanoparticles entering clinical translation.
7. Delivery of radioenhancers
The targeted delivery of nanoparticles to tumors remains a major focus of research. Based on the recent meta-analysis by Wilhelm et al.,272 an average of less than 1% of the injected dose of nanoparticles typically accumulates in the tumor tissue, irrespective of active or passive delivery approaches. Interestingly, the tumor accumulation can be increased to around 10% by kinetically saturating the uptake of the liver by high dose injections of inactive nanoparticles, however, off-target accumulation is still considerable.273 For radioenhancement, therapeutically effective nanomaterial concentrations in the tumor are typically rather high, and are therefore especially challenging to achieve by conventional intravenous administration. Therefore, current clinical studies (both for HfO2 (Hensify)274 and for nanoparticle tumor hyperthermia)275 employ intratumoral injection as a preferred route of delivery. While clinically successful (improved patient outcome), this delivery route limits the applicability of the therapy to a small subset of patients with only locally advanced and well-accessible tumors. Alternative delivery strategies to tumors, and their effect on outcome, are therefore a major priority in the field for increasing the impact by making nanoparticle radioenhancement accessible to a larger cancer patient population.
8. Conclusions and future directions
Overall, a plethora of radioenhancer material candidates have been tested and evaluated in numerous experimental studies, showing excellent therapeutic anticancer efficacies in preclinical stages. While simple oxide nanoparticles can be tailored to create ROS bursts during radiotherapy, emerging 2D or porous 3D nanomaterials hold additional potential due to their favorable surface-to-volume ratio facilitating the maximization of catalytic ROS generation. Due to the high freedom in design and the modularity of the synthesis of materials, such as metal organic frameworks, additional functions can be introduced, including drug loading as well as the functionalization with targeting moieties, provided those modifications do not hamper the radioenhancing properties of the materials. Using radioenhancers to reverse a patient's resistance to immune checkpoint inhibitors, is a promising emerging area of research. Immune modulating properties should therefore be understood and leveraged to further maximize cancer treatment efficacy, for example as part of combination therapy settings. However, the efficient translation of nanomaterial-based radioenhancers to the clinical stage is hampered by, among other factors, a lack of standardization of experimental designs and methodologies as well as the absence of direct performance benchmarking. These factors largely preclude data-driven material design. Additionally, the scalable and cost-effective synthesis of high-quality nanomaterials remains challenging for materials other than metal oxides. Comparative studies of different materials using different types of radiation are imperative for a better understanding of the radioenhancing properties of nanomaterials hence enabling the design of performance-optimized radioenhancers for best possible therapy results. This then opens the path to evaluations of the cost-effectiveness of (nanomaterial) radio-enhancement and a careful evaluation of the risk/benefit ratio.
Importantly, the radiation therapy settings, including the type of irradiation and its energy, should also be taken into consideration. At a preclinical stage, low-Z NP development can be evaluated with kV X-ray sources, while high-Z NPs should always be evaluated with clinical irradiation sources due to differential physical effects. Harnessing advancements from the catalysis community for the nanoparticle design, engineering and analytics in order to achieve performance-optimized generation of X-ray-induced hydroxyl radicals or other ROS species holds promise. While physical and chemical mechanisms are readily accessible via simulations or the use of ROS-reactive fluorophores, the understanding of biological radiosensitization mechanisms of NPs in cellular environments requires evaluation in biologically relevant, more complex systems. In addition to the radioenhancement properties, in-depth toxicity evaluations are imperative. Since comparison studies in vivo pose ethical concerns, easily accessible preclinical platforms allowing for high-throughput high-content measurements, such as 3D cell models,276 should be further integrated into radioenhancer development to gain relevant physical, chemical, and biological data on different nanoparticle candidate materials. Importantly, such advanced in vitro models are sufficient for direct performance benchmarking of novel candidate materials against the current clinical gold standard (HfO2 nanoparticles), and may hence accelerate clinical translation and reduce animal use.
All in all, recent preclinical and clinical data provide direct evidence for the significant potential of nanomaterials for enhancing clinically established (photon or particle) as well as emerging (e.g. FLASH) radiation therapy modalities in an additional way, complementary to developments of advanced instrumentation and treatment planning. This field offers ample opportunities for the material science community to directly contribute to the improvement of radiation therapy by designing performance-optimized radioenhancer nanomaterials.
Conflicts of interest
There are no conflicts to declare.
Acknowledgements
We acknowledge funding from the Swiss National Science Foundation (Eccellenza grant no. 181290, I. K. H.), the Swiss Cancer Research Foundation (Grant number KFS-4868-08-2019, I. K. H.), and from ETH Zurich (grant no. ETH-07 21-2, I. K. H.). L. R. H. G. is supported by the Empa Young Scientist Fellowship sponsored by the Ria&Arthur Dietschweiler Foundation.
References
- J. F. Hainfeld, D. N. Slatkin and H. M. Smilowitz, Phys. Med. Biol., 2004, 49, N309 CrossRef CAS PubMed.
- C. Verry, S. Dufort, J. Villa, M. Gavard, C. Iriart, S. Grand, J. Charles, B. Chovelon, J.-L. Cracowski, J.-L. Quesada, C. Mendoza, L. Sancey, A. Lehmann, F. Jover, J.-Y. Giraud, F. Lux, Y. Crémillieux, S. McMahon, P. J. Pauwels, D. Cagney, R. Berbeco, A. Aizer, E. Deutsch, M. Loeffler, G. Le Duc, O. Tillement and J. Balosso, Radiother. Oncol., 2021, 160, 159 CrossRef CAS PubMed.
- A. C. Anselmo and S. Mitragotri, Bioeng. Transl. Med., 2019, 4, e10143, DOI:10.1002/btm2.10143.
- S. Bonvalot, C. Le Pechoux, T. De Baere, G. Kantor, X. Buy, E. Stoeckle, P. Terrier, P. Sargos, J. M. Coindre, N. Lassau, R. Ait Sarkouh, M. Dimitriu, E. Borghi, L. Levy, E. Deutsch and J.-C. Soria, Clin. Cancer Res., 2017, 23, 908 CrossRef CAS PubMed.
- K. Lu, C. He, N. Guo, C. Chan, K. Ni, G. Lan, H. Tang, C. Pelizzari, Y.-X. Fu, M. T. Spiotto, R. R. Weichselbaum and W. Lin, Nat. Biomed. Eng., 2018, 2, 600 CrossRef CAS PubMed.
- M. J. Neufeld, A. Lutzke, G. Pratx and C. Sun, Chem. – Eur. J., 2021, 27, 3229 CrossRef CAS PubMed.
- K. Ricketts, R. Ahmad, L. Beaton, B. Cousins, K. Critchley, M. Davies, S. Evans, I. Fenuyi, A. Gavriilidis, Q. J. Harmer, D. Jayne, M. Jefford, M. Loizidou, A. Macrobert, S. Moorcroft, I. Naasani, Z. Y. Ong, K. M. Prise, S. Rannard, T. Richards, G. Schettino, R. A. Sharma, O. Tillement, G. Wakefield, N. R. Williams, E. Yaghini and G. Royle, Br. J. Radiol., 2018, 91, 20180325 CrossRef PubMed.
- S. Penninckx, A.-C. Heuskin, C. Michiels and S. Lucas, Cancers, 2020, 12, 2021, DOI:10.3390/cancers12082021.
-
I. Obodovskiy, in Fundamentals of Radiation and Chemical Safety, ed. I. Obodovskiy, Elsevier, Amsterdam, 2015, pp. 87–131 Search PubMed.
-
J. E. Turner, Atoms, Radiation, and Radiation Protection, Wiley-VCH, Weinheim, 2007 Search PubMed.
- M.-F. Penet, S. Kakkad, F. Wildes and Z. M. Bhujwalla, Front. Oncol., 2021, 10, 599204 CrossRef PubMed.
- J. Ramos-Méndez, J. Perl, J. Schuemann, A. McNamara, H. Paganetti and B. Faddegon, Phys. Med. Biol., 2018, 63, 105014 CrossRef PubMed.
- M. S. Kreipl, W. Friedland and H. G. Paretzke, Radiat. Environ. Biophys., 2008, 48, 11 CrossRef PubMed.
- Y. Lai, X. Jia and Y. Chi, Phys. Med. Biol., 2021, 66, 025004 CrossRef CAS PubMed.
- N. Aykin-Burns, B. G. Slane, A. T. Y. Liu, K. M. Owens, M. S. O’Malley, B. J. Smith, F. E. Domann and D. R. Spitz, Rare, 2010, 175, 150 CrossRef PubMed.
- J. Wang, H. Wang and H. Qian, Mil. Med. Res., 2018, 5, 20 Search PubMed.
- R. Hirayama, A. Ito, M. Tomita, T. Tsukada, F. Yatagai, M. Noguchi, Y. Matsumoto, Y. Kase, K. Ando, R. Okayasu and Y. Furusawa, Rare, 2009, 171, 212 CrossRef CAS PubMed.
- A. Ito, H. Nakano, Y. Kusano, R. Hirayama, Y. Furusawa, C. Murayama, T. Mori, Y. Katsumura and K. Shinohara, Rare, 2006, 165, 703 CrossRef CAS PubMed.
- R. Hirayama, A. Ito, M. Noguchi, Y. Matsumoto, A. Uzawa, G. Kobashi, R. Okayasu and Y. Furusawa, Radiat. Res., 2013, 180, 514 CrossRef CAS PubMed.
- K. Shinohara, H. Nakano and H. Ohara, Acta Oncol., 1996, 35, 869 CrossRef CAS PubMed.
- D. R. Grimes and M. Partridge, Biomed. Phys. Eng. Express, 2015, 1, 045209 CrossRef PubMed.
- K. Harrington, P. Jankowska and M. Hingorani, Clin. Oncol., 2007, 19, 561 CrossRef CAS PubMed.
- J. Boustani, M. Grapin, P.-A. Laurent, L. Apetoh and C. Mirjolet, Cancers, 2019, 11, 860 CrossRef CAS PubMed.
- J. Marill, N. M. Anesary, P. Zhang, S. Vivet, E. Borghi, L. Levy and A. Pottier, Radiat. Oncol., 2014, 9, 150 CrossRef PubMed.
- Z. Kuncic and S. Lacombe, Phys. Med. Biol., 2018, 63, 02TR01 CrossRef PubMed.
- W. B. Li, A. Belchior, M. Beuve, Y. Z. Chen, S. Di Maria, W. Friedland, B. Gervais, B. Heide, N. Hocine, A. Ipatov, A. P. Klapproth, C. Y. Li, J. L. Li, G. Multhoff, F. Poignant, R. Qiu, H. Rabus, B. Rudek, J. Schuemann, S. Stangl, E. Testa, C. Villagrasa, W. Z. Xie and Y. B. Zhang, Phys. Med., 2020, 69, 147 CrossRef CAS PubMed.
- S. Incerti, B. Suerfu, J. Xu, V. Ivantchenko, A. Mantero, J. M. C. Brown, M. A. Bernal, Z. Francis, M. Karamitros and H. N. Tran, Nucl. Instrum. Methods Phys. Res., Sect. B, 2016, 372, 91 CrossRef CAS.
- K. T. Butterworth, S. J. McMahon, F. J. Currell and K. M. Prise, Nanoscale, 2012, 4, 4830 RSC.
- B. Rudek, A. McNamara, J. Ramos-Méndez, H. Byrne, Z. Kuncic and J. Schuemann, Phys. Med. Biol., 2019, 64, 175005 CrossRef CAS PubMed.
- S. J. McMahon, W. B. Hyland, M. F. Muir, J. A. Coulter, S. Jain, K. T. Butterworth, G. Schettino, G. R. Dickson, A. R. Hounsell, J. M. O’Sullivan, K. M. Prise, D. G. Hirst and F. J. Currell, Radiother. Oncol., 2011, 100, 412 CrossRef CAS PubMed.
- M. C. Fuss, D. Boscolo, M. Durante, E. Scifoni and M. Krämer, Phys. Med. Biol., 2020, 65, 075008 CrossRef CAS PubMed.
- Y. Lin, S. J. McMahon, M. Scarpelli, H. Paganetti and J. Schuemann, Phys. Med. Biol., 2014, 59, 7675 CrossRef PubMed.
- M. L. Aliru, K. Aziz, M. Bodd, K. Sanders, L. S. K. Mahadevan, N. Sahoo, R. C. Tailor and S. Krishnan, Int. J. Radiat. Oncol., Biol., Phys., 2017, 99, E574 CrossRef.
- S. Özçelik and G. Pratx, Nanotechnology, 2020, 31, 415102 CrossRef PubMed.
- N. Li, L. Yu, J. Wang, X. Gao, Y. Chen, W. Pan and B. Tang, Chem. Sci., 2018, 9, 3159 RSC.
- W. Deng, K. J. McKelvey, A. Guller, A. Fayzullin, J. M. Campbell, S. Clement, A. Habibalahi, Z. Wargocka, L. Liang, C. Shen, V. M. Howell, A. F. Engel and E. M. Goldys, ACS Cent. Sci., 2020, 6, 715 CrossRef CAS PubMed.
- S. J. McMahon, A. L. McNamara, J. Schuemann, K. M. Prise and H. Paganetti, J. Phys.: Conf. Ser., 2017, 777, 012008 CrossRef.
- D. Peukert, I. Kempson, M. Douglass and E. Bezak, Med. Phys., 2020, 47, 651 CrossRef CAS PubMed.
- B. Jones, S. J. McMahon and K. M. Prise, Clin. Oncol., 2018, 30, 285 CrossRef CAS PubMed.
- T. Schlathölter, P. Eustache, E. Porcel, D. Salado, L. Stefancikova, O. Tillement, F. Lux, P. Mowat, A. K. Biegun, M.-J. van Goethem, H. Remita and S. Lacombe, Int. J. Nanomed., 2016, 11, 1549 CrossRef PubMed.
- S. H. Park and J. O. Kang, Radiather. Oncol. J., 2011, 29, 135 CrossRef PubMed.
- J.-K. Kim, S.-J. Seo, K.-H. Kim, T.-J. Kim, M.-H. Chung, K.-R. Kim and T.-K. Yang, Nanotechnology, 2010, 21, 425102 CrossRef PubMed.
- J.-K. Kim, S.-J. Seo, H.-T. Kim, K.-H. Kim, M.-H. Chung, K.-R. Kim and S.-J. Ye, Phys. Med. Biol., 2012, 57, 8309 CrossRef PubMed.
- J. C. Polf, L. F. Bronk, W. H. P. Driessen, W. Arap, R. Pasqualini and M. Gillin, Appl. Phys. Lett., 2011, 98, DOI:10.1063/1.3589914.
- C. Cunningham, M. de Kock, M. Engelbrecht, X. Miles, J. Slabbert and C. Vandevoorde, Front. Public Health, 2021, 9, 1065 Search PubMed.
- J. Cho, C. Gonzalez-Lepera, N. Manohar, M. Kerr, S. Krishnan and S. H. Cho, Phys. Med. Biol., 2016, 61, 2562 CrossRef CAS PubMed.
- C. Wälzlein, E. Scifoni, M. Krämer and M. Durante, Phys. Med. Biol., 2014, 59, 1441 CrossRef PubMed.
- A.-C. Heuskin, B. Gallez, O. Feron, P. Martinive, C. Michiels and S. Lucas, Med. Phys., 2017, 44, 4299 CrossRef CAS PubMed.
- A. Ku, V. J. Facca, Z. Cai and R. M. Reilly, EJNMMI Radiopharm. Chem., 2019, 4, 27 CrossRef PubMed.
- M. Sotiropoulos, N. T. Henthorn, J. W. Warmenhoven, R. I. Mackay, K. J. Kirkby and M. J. Merchant, Nanoscale, 2017, 9, 18413 RSC.
- J. Schuemann, A. F. Bagley, R. Berbeco, K. Bromma, K. T. Butterworth, H. L. Byrne, B. D. Chithrani, S. H. Cho, J. R. Cook, V. Favaudon, Y. H. Gholami, E. Gargioni, J. F. Hainfeld, F. Hespeels, A.-C. Heuskin, U. M. Ibeh, Z. Kuncic, S. Kunjachan, S. Lacombe, S. Lucas, F. Lux, S. McMahon, D. Nevozhay, W. Ngwa, J. D. Payne, S. Penninckx, E. Porcel, K. M. Prise, H. Rabus, S. M. Ridwan, B. Rudek, L. Sanche, B. Singh, H. M. Smilowitz, K. V. Sokolov, S. Sridhar, Y. Stanishevskiy, W. Sung, O. Tillement, N. Virani, W. Yantasee and S. Krishnan, Phys. Med. Biol., 2020, 65, 21RM02 CrossRef CAS PubMed.
- F. Hespeels, A. C. Heuskin, E. Scifoni, M. Kraemer and S. Lucas, Nucl. Instrum. Methods Phys. Res., Sect. B, 2017, 401, 8 CrossRef CAS.
- R. Abdul Rashid, S. Zainal Abidin, M. A. Khairil Anuar, T. Tominaga, H. Akasaka, R. Sasaki, K. Kie, K. Abdul Razak, B. T. T. Pham, B. S. Hawkett, M.-A. Carmichael, M. Geso and W. N. Rahman, OpenNano, 2019, 4, 100027 CrossRef.
- C. Behrends, C. M. Bäcker, I. Schilling, S. Zwiehoff, J. Weingarten, K. Kröninger, C. Rehbock, S. Barcikowski, J. Wulff, C. Bäumer and B. Timmermann, Phys. Med. Biol., 2022, 67, 155023 CrossRef PubMed.
- L. R. H. Gerken, A. Gogos, F. H. L. Starsich, H. David, M. E. Gerdes, H. Schiefer, S. Psoroulas, D. Meer, L. Plasswilm, D. C. Weber and I. K. Herrmann, Nat. Commun., 2022, 13, 3248 CrossRef CAS PubMed.
- J. C. Roeske, L. Nuñez, M. Hoggarth, E. Labay and R. R. Weichselbaum, Technol. Cancer Res. Treat., 2007, 6, 395 CrossRef PubMed.
- M. K. K. Leung, J. C. L. Chow, B. D. Chithrani, M. J. G. Lee, B. Oms and D. A. Jaffray, Med. Phys., 2011, 38, 624 CrossRef CAS PubMed.
- C. L. Smith, T. Ackerly, S. P. Best, F. Gagliardi, K. Kie, P. J. Little, G. McCorkell, C. A. Sale, Y. Tsunei, T. Tominaga, S. S. Volaric and M. Geso, Radiat. Meas., 2015, 82, 122 CrossRef CAS.
- F. M. Gagliardi, R. D. Franich and M. Geso, J. Synchrotron Radiat., 2020, 27, 1590 CrossRef CAS PubMed.
- P. Zhang, L. Jiang, H. Chen and L. Hu, Gels, 2022, 8, 238 CrossRef CAS PubMed.
- Z. Behrouzkia, R. Zohdiaghdam, H. R. Khalkhali and F. Mousavi, J. Biomed. Phys. Eng., 2019, 9, 89–96, DOI:10.31661/jbpe.v0i0.1019.
- A. Rajaee, S. Wang, L. Zhao and Y. Liu, J. Phys.: Conf. Ser., 2019, 1305, 012046 CrossRef CAS.
- S. Farahani, N. Riyahi Alam, S. Haghgoo, M. Khoobi, G. Geraily and E. Gorji, J. Biomed. Phys. Eng., 2019, 9, 199 CAS.
- W. N. Rahman, C. J. Wong, T. Ackerly, N. Yagi and M. Geso, Australas. Phys. Eng. Sci. Med., 2012, 35, 301 CrossRef PubMed.
- K. T. Butterworth, S. J. McMahon, L. E. Taggart and K. M. Prise, Transl. Cancer Res., 2013, 2, 269 CAS.
- C. Stewart, K. Konstantinov, S. McKinnon, S. Guatelli, M. Lerch, A. Rosenfeld, M. Tehei and S. Corde, Phys. Med., 2016, 32, 1444 CrossRef PubMed.
- T. Wolfe, D. Chatterjee, J. Lee, J. D. Grant, S. Bhattarai, R. Tailor, G. Goodrich, P. Nicolucci and S. Krishnan, Nanomedicine, 2015, 11, 1277 CrossRef CAS PubMed.
- D. Howard, S. Sebastian, Q. V.-C. Le, B. Thierry and I. Kempson, Int. J. Mol. Sci., 2020, 21, 579 CrossRef CAS PubMed.
- J. C. G. Jeynes, M. J. Merchant, A. Spindler, A.-C. Wera and K. J. Kirkby, Phys. Med. Biol., 2014, 59, 6431 CrossRef CAS PubMed.
- S. Li, E. Porcel, H. Remita, S. Marco, M. Réfrégiers, M. Dutertre, F. Confalonieri and S. Lacombe, Cancer Nanotechnol., 2017, 8, 4 CrossRef PubMed.
- M. Gilles, E. Brun and C. Sicard-Roselli, J. Colloid Interface Sci., 2018, 525, 31 CrossRef CAS PubMed.
- Y. Nosaka and A. Y. Nosaka, Chem. Rev., 2017, 117, 11302 CrossRef CAS PubMed.
- J. Bogdan, J. Pławińska-Czarnak and J. Zarzyńska, Nanoscale Res. Lett., 2017, 12, 225 CrossRef PubMed.
- K. Takanabe, ACS Catal., 2017, 7, 8006 CrossRef CAS.
- X. Li, J. Yu and M. Jaroniec, Chem. Soc. Rev., 2016, 45, 2603 RSC.
- L. Zhang, C. Zhu, R. Huang, Y. Ding, C. Ruan and X.-C. Shen, Front. Chem., 2021, 9, 630969 CrossRef CAS PubMed.
- C. Zhang, X. Wang, J. Du, Z. Gu and Y. Zhao, Adv. Sci., 2021, 8, 2002797 CrossRef CAS PubMed.
- X. Li, Y. Wu, R. Zhang, W. Bai, T. Ye and S. Wang, Front. Mol. Biosci., 2021, 8, 683519 CrossRef CAS PubMed.
- C. Cao, X. Wang, N. Yang, X. Song and X. Dong, Chem. Sci., 2022, 13, 863 RSC.
- C. Xu, Z. Yuan, N. Kohler, J. Kim, M. A. Chung and S. Sun, J. Am. Chem. Soc., 2009, 131, 15346 CrossRef CAS PubMed.
- S. Klein, A. Sommer, L. V. R. Distel, W. Neuhuber and C. Kryschi, Biochem. Biophys. Res. Commun., 2012, 425, 393 CrossRef CAS PubMed.
- C. Angelé-Martínez, K. V. T. Nguyen, F. S. Ameer, J. N. Anker and J. L. Brumaghim, Nanotoxicology, 2017, 11, 278 CrossRef PubMed.
- Y.-W. Jiang, G. Gao, H.-R. Jia, X. Zhang, J. Zhao, N. Ma, J.-B. Liu, P. Liu and F.-G. Wu, ACS Biomater. Sci. Eng., 2019, 5, 1569 CrossRef CAS PubMed.
- J. Du, X. Zheng, Y. Yong, J. Yu, X. Dong, C. Zhang, R. Zhou, B. Li, L. Yan, C. Chen, Z. Gu and Y. Zhao, Nanoscale, 2017, 9, 8229 RSC.
- T. Mai and J. Z. Hilt, Colloids Surf., A, 2019, 576, 9 CrossRef CAS.
- M. Gilles, E. Brun and C. Sicard-Roselli, Colloids Surf., B, 2014, 123, 770 CrossRef CAS PubMed.
- Y. Cho, T. A. Le and H. Lee, Molecules, 2020, 25, 1965 CrossRef CAS PubMed.
- H. Montaseri, C. A. Kruger and H. Abrahamse, Int. J. Mol. Sci., 2020, 21, 3358 CrossRef CAS PubMed.
- S. Klein, A. Sommer, L. V. R. Distel, J.-L. Hazemann, W. Kröner, W. Neuhuber, P. Müller, O. Proux and C. Kryschi, J. Phys. Chem. B, 2014, 118, 6159 CrossRef CAS PubMed.
- Y. Zhang, M. Dai and Z. Yuan, Anal. Methods, 2018, 10, 4625 RSC.
- J. Zhao and M. Riediker, J. Nanopart. Res., 2014, 16, 2493 CrossRef PubMed.
- W. Huang, Y. Zhang, Y. Zhang, D. Fang and J. J. Schauer, Water, Air, Soil Pollut., 2016, 227, 164 CrossRef.
- Y. Nosaka and A. Y. Nosaka, J. Phys. Chem. C, 2019, 123, 20682 CrossRef CAS.
- E. Q. Youkhana, B. Feltis, A. Blencowe and M. Geso, Int. J. Med. Sci., 2017, 14, 602 CrossRef CAS PubMed.
- C. Sicard-Roselli, E. Brun, M. Gilles, G. Baldacchino, C. Kelsey, H. McQuaid, C. Polin, N. Wardlow and F. Currell, Small, 2014, 10, 3338 CrossRef CAS PubMed.
- A. Guerreiro, N. Chatterton, E. M. Crabb and J. P. Golding, Cancer Nanotechnol., 2019, 10, 10 CrossRef CAS.
- Y. Manevich, K. D. Held and J. E. Biaglow, Radiat. Res., 1997, 148, 580 CrossRef CAS PubMed.
- D. Dębski, R. Smulik, J. Zielonka, B. Michałowski, M. Jakubowska, K. Dębowska, J. Adamus, A. Marcinek, B. Kalyanaraman and A. Sikora, Free Radical Biol. Med., 2016, 95, 323 CrossRef PubMed.
- A. Detappe, S. Kunjachan, P. Drané, S. Kotb, M. Myronakis, D. E. Biancur, T. Ireland, M. Wagar, F. Lux, O. Tillement and R. Berbeco, Sci. Rep., 2016, 6, 34040 CrossRef CAS PubMed.
- B. J. Choi, K. O. Jung, E. E. Graves and G. Pratx, Nanotechnology, 2018, 29, 504001 CrossRef PubMed.
- K. Ni, G. Lan, C. Chan, B. Quigley, K. Lu, T. Aung, N. Guo, P. La Riviere, R. R. Weichselbaum and W. Lin, Nat. Commun., 2018, 9, 2351, DOI:10.1038/s41467-018-04703-w.
- M. Misawa and J. Takahashi, Nanomedicine, 2011, 7, 604 CrossRef CAS PubMed.
- I. Porosnicu, C. M. Butnaru, I. Tiseanu, E. Stancu, C. V. A. Munteanu, B. I. Bita, O. G. Duliu and F. Sima, Molecules, 2021, 26, 3403 CrossRef CAS PubMed.
- F. Tabatabaie, R. Franich, B. Feltis and M. Geso, Int. J. Mol. Sci., 2022, 23, 6887 CrossRef CAS PubMed.
- G. T. Huynh, V. Kesarwani, J. A. Walker, J. E. Frith, L. Meagher and S. R. Corrie, Front. Chem., 2021, 9, 728717 CrossRef CAS PubMed.
- T. T. Khalil, R. Bazzi, S. Roux and M. Fromm, Colloids Surf., B, 2019, 175, 606 CrossRef CAS PubMed.
- S. Penninckx, A.-C. Heuskin, C. Michiels and S. Lucas, Nanomedicine, 2018, 13, 2917 CrossRef CAS PubMed.
- L. Cui, K. Tse, P. Zahedi, S. M. Harding, G. Zafarana, D. A. Jaffray, R. G. Bristow and C. Allen, Radiat. Res., 2014, 182, 475 CrossRef PubMed.
- S. Jain, J. A. Coulter, A. R. Hounsell, K. T. Butterworth, S. J. McMahon, W. B. Hyland, M. F. Muir, G. R. Dickson, K. M. Prise, F. J. Currell, J. M. O’Sullivan and D. G. Hirst, Int. J. Radiat. Oncol., Biol., Phys., 2011, 79, 531 CrossRef CAS PubMed.
- J. J. Li, L. Zou, D. Hartono, C.-N. Ong, B.-H. Bay and L.-Y. Lanry Yung, Adv. Mater., 2008, 20, 138 CrossRef CAS.
- N. M. Schaeublin, L. K. Braydich-Stolle, A. M. Schrand, J. M. Miller, J. Hutchison, J. J. Schlager and S. M. Hussain, Nanoscale, 2011, 3, 410 RSC.
- S. Penninckx, A.-C. Heuskin, C. Michiels and S. Lucas, Cancers, 2020, 12, 2021 CrossRef CAS PubMed.
- M. Wojewódzka, A. Lankoff, M. Dusińska, G. Brunborg, J. Czerwińska, T. Iwaneńko, T. Stepkowski, I. Szumiel and M. Kruszewski, Nukleonika, 2011, 56, 29 Search PubMed.
- L. Armand, A. Tarantini, D. Beal, M. Biola-Clier, L. Bobyk, S. Sorieul, K. Pernet-Gallay, C. Marie-Desvergne, I. Lynch, N. Herlin-Boime and M. Carriere, Nanotoxicology, 2016, 10, 913 CrossRef CAS PubMed.
- L. Armand, M. Biola-Clier, L. Bobyk, V. Collin-Faure, H. Diemer, J.-M. Strub, S. Cianferani, A. Van Dorsselaer, N. Herlin-Boime, T. Rabilloud and M. Carriere, J. Proteomics, 2016, 134, 163 CrossRef CAS PubMed.
- C. Mirjolet, A. L. Papa, G. Créhange, O. Raguin, C. Seignez, C. Paul, G. Truc, P. Maingon and N. Millot, Radiother. Oncol., 2013, 108, 136 CrossRef CAS PubMed.
- L. Štefančíková, S. Lacombe, D. Salado, E. Porcel, E. Pagáčová, O. Tillement, F. Lux, D. Depeš, S. Kozubek and M. Falk, J. Nanobiotechnol., 2016, 14, 63 CrossRef PubMed.
- M. Carriere, S. Sauvaigo, T. Douki and J.-L. Ravanat, Mutagenesis, 2017, 32, 203 CrossRef CAS PubMed.
- J. H. E. Arts, M. Hadi, M.-A. Irfan, A. M. Keene, R. Kreiling, D. Lyon, M. Maier, K. Michel, T. Petry, U. G. Sauer, D. Warheit, K. Wiench, W. Wohlleben and R. Landsiedel, Regul. Toxicol. Pharmacol., 2015, 71, S1 CrossRef CAS PubMed.
- T. M. Pawlik and K. Keyomarsi, Int. J. Radiat. Oncol., Biol., Phys., 2004, 59, 928 CrossRef PubMed.
- Y. Pan, A. Leifert, D. Ruau, S. Neuss, J. Bornemann, G. Schmid, W. Brandau, U. Simon and W. Jahnen-Dechent, Small, 2009, 5, 2067 CrossRef CAS PubMed.
- L. Cui, K. Tse, P. Zahedi, S. M. Harding, G. Zafarana, D. A. Jaffray, R. G. Bristow and C. Allen, Rare, 2014, 182, 475 CrossRef PubMed.
- K. T. Butterworth, J. A. Coulter, S. Jain, J. Forker, S. J. McMahon, G. Schettino, K. M. Prise, F. J. Currell and D. G. Hirst, Nanotechnology, 2010, 21, 295101 CrossRef CAS PubMed.
- W. Roa, X. Zhang, L. Guo, A. Shaw, X. Hu, Y. Xiong, S. Gulavita, S. Patel, X. Sun, J. Chen, R. Moore and J. Z. Xing, Nanotechnology, 2009, 20, 375101 CrossRef PubMed.
- Q. Li, C. Huang, L. Liu, R. Hu and J. Qu, Nanomaterials, 2018, 8, 1063 CrossRef PubMed.
- S. Abdel-Ghany, M. Mahfouz, N. Ashraf, H. Sabit, E. Cevik and M. El-Zawahri, Inorg. Nano-Met. Chem., 2020, 50, 926 CrossRef CAS.
- R. Bhattacharya, C. R. Patra, R. Verma, S. Kumar, P. R. Greipp and P. Mukherjee, Adv. Mater., 2007, 19, 711 CrossRef CAS.
- F. Taupin, M. Flaender, R. Delorme, T. Brochard, J.-F. Mayol, J. Arnaud, P. Perriat, L. Sancey, F. Lux, R. F. Barth, M. Carrière, J.-L. Ravanat and H. Elleaume, Phys. Med. Biol., 2015, 60, 4449 CrossRef PubMed.
- P. V. AshaRani, G. Low Kah Mun, M. P. Hande and S. Valiyaveettil, ACS Nano, 2009, 3, 279 CrossRef CAS PubMed.
- S. Deycmar, E. Faccin, T. Kazimova, P. A. Knobel, I. Telarovic, F. Tschanz, V. Waller, R. Winkler, C. Yong, D. Zingariello and M. Pruschy, BJR, 2020, 93, 20190494 CrossRef PubMed.
- Z. Nikitaki, A. Velalopoulou, V. Zanni, I. Tremi, S. Havaki, M. Kokkoris, V. G. Gorgoulis, C. Koumenis and A. G. Georgakilas, Expert Rev. Mol. Med., 2022, 24, e15 CrossRef CAS PubMed.
-
M. Gladstone and T. T. Su, International Review of Cell and Molecular Biology, Elsevier, 2012, pp. 235–253 Search PubMed.
- J. H. Kim, M. H. Kim, D. H. Jo, Y. S. Yu, T. G. Lee and J. H. Kim, Biomaterials, 2011, 32, 1865 CrossRef CAS PubMed.
- R. Bhattacharya, P. Mukherjee, Z. Xiong, A. Atala, S. Soker and D. Mukhopadhyay, Nano Lett., 2004, 4, 2479 CrossRef CAS.
- P. Mukherjee, R. Bhattacharya, P. Wang, L. Wang, S. Basu, J. A. Nagy, A. Atala, D. Mukhopadhyay and S. Soker, Clin. Cancer Res., 2005, 11, 3530 CrossRef CAS PubMed.
- S. R. Satapathy, A. Nayak, S. Siddharth, S. Das, D. Nayak and C. N. Kundu, Nanomedicine, 2018, 14, 883 CrossRef CAS PubMed.
- L. Cui, P. Zahedi, J. Saraceno, R. Bristow, D. Jaffray and C. Allen, Nanomedicine, 2013, 9, 264 CrossRef CAS PubMed.
- J. A. Kim, C. Åberg, A. Salvati and K. A. Dawson, Nat. Nanotechnol., 2012, 7, 62 CrossRef CAS PubMed.
- K. Graham and E. Unger, IJN, 2018, 13, 6049 CrossRef CAS PubMed.
- S. Jain, J. A. Coulter, K. T. Butterworth, A. R. Hounsell, S. J. McMahon, W. B. Hyland, M. F. Muir, G. R. Dickson, K. M. Prise, F. J. Currell, D. G. Hirst and J. M. O’Sullivan, Radiother. Oncol., 2014, 110, 342 CrossRef CAS PubMed.
- S. Li, S. Penninckx, L. Karmani, A.-C. Heuskin, K. Watillon, R. Marega, J. Zola, V. Corvaglia, G. Genard, B. Gallez, O. Feron, P. Martinive, D. Bonifazi, C. Michiels and S. Lucas, Nanotechnology, 2016, 27, 455101 CrossRef PubMed.
- W. Jiang, Q. Li, L. Xiao, J. Dou, Y. Liu, W. Yu, Y. Ma, X. Li, Y.-Z. You, Z. Tong, H. Liu, H. Liang, L. Lu, X. Xu, Y. Yao, G. Zhang, Y. Wang and J. Wang, ACS Nano, 2018, 12, 5684 CrossRef CAS PubMed.
- A. Rastinfard, M. H. Nazarpak and F. Moztarzadeh, RSC Adv., 2017, 8, 91 RSC.
- S.-W. Shin, K. Yang, M. Lee, J. Moon, A. Son, Y. Kim, S. Choi, D. Kim, C. Choi, N. Lee and H. C. Park, Int. J. Mol. Sci., 2021, 22, 2637 CrossRef CAS PubMed.
- S. Dong, Y. Dong, T. Jia, S. Liu, J. Liu, D. Yang, F. He, S. Gai, P. Yang and J. Lin, Adv. Mater., 2020, 32, 2002439 CrossRef CAS PubMed.
- C. Li, X. Zheng, W. Chen, S. Ji, Y. Yuan and X. Jiang, Nano Lett., 2020, 20, 6526 CrossRef CAS PubMed.
- C. Ruan, K. Su, D. Zhao, A. Lu and C. Zhong, Front. Chem., 2021, 9, 649158 CrossRef CAS PubMed.
- G. G. Steel, T. J. McMillan and J. H. Peacock, Int. J. Radiat. Biol., 1989, 56, 1045 CrossRef CAS PubMed.
- J. R. Williams, Y. Zhang, H. Zhou, D. S. Gridley, C. J. Koch, J. Russell, J. S. Slater and J. B. Little, Int. J. Radiat. Biol., 2008, 84, 253 CrossRef CAS PubMed.
- R. Wahab, S. Dwivedi, F. Khan, Y. K. Mishra, I. H. Hwang, H.-S. Shin, J. Musarrat and A. A. Al-Khedhairy, Colloids Surf., B, 2014, 123, 664 CrossRef CAS PubMed.
- F. Wang, Z. Chen, Y. Wang, C. Ma, L. Bi, M. Song and G. Jiang, Environ. Sci. Technol., 2022, 56, 5706 CrossRef CAS PubMed.
- J.-C. Simard, I. Durocher and D. Girard, Apoptosis, 2016, 21, 1279 CrossRef CAS PubMed.
- Z. Xia, J. He, B. Li, K. He, W. Yang, X. Chen, J. Zhang and G. Xiang, RSC Adv., 2018, 8, 31764 RSC.
- S. Demaria and S. C. Formenti, Br. J. Radiol., 2020, 93, 20200042 CrossRef PubMed.
- B. Janic, S. L. Brown, R. Neff, F. Liu, G. Mao, Y. Chen, L. Jackson, I. J. Chetty, B. Movsas and N. Wen, Cancer Biol. Ther., 2021, 22, 124 CrossRef CAS PubMed.
- A. Darmon, P. Zhang, J. Marill, N. Mohamed Anesary, J. Da Silva and S. Paris, Cancer Cell Int., 2022, 22, 208 CrossRef CAS PubMed.
- Y. Hu, S. Paris, H. Barsoumian, C. O. Abana, K. He, M. Wasley, A. I. Younes, F. Masrorpour, D. Chen, L. Yang, J. D. Dunn, J. Zhang, S. Gandhi, Q.-N. Nguyen, M. A. Cortez and J. Welsh, Int. J. Radiat. Oncol., Biol., Phys., 2021, 111, 647 CrossRef PubMed.
- P. Zhang, A. Darmon, J. Marill, N. Mohamed Anesary and S. Paris, Int. J. Nanomed., 2020, 15, 3843 CrossRef CAS PubMed.
- Y. Hu, S. Paris, G. Bertolet, H. B. Barsoumian, Q. Wang, J. Da Silva, N. B. Patel, N. Nguyen, D. J. Doss, A. Huang, E. Hsu, C. S. K. Leyton, T. A. Voss, F. Masrorpour, C. Leuschner, J. T. Pietz, N. Puebla-Osorio, S. Gandhi, Q.-N. Nguyen, J. Wang, M. A. Cortez and J. W. Welsh, Front. Immunol., 2022, 13, 1022011 CrossRef CAS PubMed.
- A. Pandey, V. Vighetto, N. Di Marzio, F. Ferraro, M. Hirsch, N. Ferrante, S. Mitra, A. Grattoni and C. S. Filgueira, Nanomaterials, 2020, 10, 1717 CrossRef CAS PubMed.
- M. Á. Pérez-Amor, L. Barrios, G. Armengol and J. F. Barquinero, Biology, 2022, 11, 1193 CrossRef PubMed.
- S. Teraoka, Y. Kakei, M. Akashi, E. Iwata, T. Hasegawa, D. Miyawaki, R. Sasaki and T. Komori, Biomed. Rep., 2018, 9, 415 CAS.
- M. Nakayama, R. Sasaki, C. Ogino, T. Tanaka, K. Morita, M. Umetsu, S. Ohara, Z. Tan, Y. Nishimura, H. Akasaka, K. Sato, C. Numako, S. Takami and A. Kondo, Radiat. Oncol., 2016, 11, 91 CrossRef PubMed.
- T. T. Puck and P. I. Marcus, J. Exp. Med., 1956, 103, 653 CrossRef CAS PubMed.
- K. Buch, T. Peters, T. Nawroth, M. Sänger, H. Schmidberger and P. Langguth, Radiat. Oncol., 2012, 7, 1 CrossRef PubMed.
- D. Kumar Maurya, J. Clin. Toxicol., 2017, 07, 1000358, DOI:10.4172/2161-0495.1000358.
- K. Khoshgard, B. Hashemi, A. Arbabi, M. J. Rasaee and M. Soleimani, Phys. Med. Biol., 2014, 59, 2249 CrossRef PubMed.
- A. Subiel, R. Ashmore and G. Schettino, Theranostics, 2016, 6, 1651 CrossRef CAS PubMed.
- H. Matsudaira, A. M. Ueno and I. Furuno, Radiat. Res., 1980, 84, 144 CrossRef CAS PubMed.
- R. S. Mello, H. Callisen, J. Winter, A. R. Kagan and A. Norman, Med. Phys., 1983, 10, 75 CrossRef CAS PubMed.
- F. W. Spiers, Br. J. Radiol., 1949, 22, 521 CrossRef CAS PubMed.
- M. Hosoe, J. Radiat. Res., 1961, 2, 68 CrossRef.
- D. F. Regulla, L. B. Hieber and M. Seidenbusch, Radiat. Res., 1998, 150, 92 CrossRef CAS.
- D. M. Herold, I. J. Das, C. C. Stobbe, R. V. Iyer and J. D. Chapman, Int. J. Radiat. Biol., 2000, 76, 1357 CrossRef CAS PubMed.
- K. Kobayashi, H. Frohlich, N. Usami, K. Takakura and C. L. Sech, Rare, 2002, 157, 32 CrossRef CAS.
- D. B. Chithrani, S. Jelveh, F. Jalali, M. van Prooijen, C. Allen, R. G. Bristow, R. P. Hill and D. A. Jaffray, Rare, 2010, 173, 719 CAS.
- A. Detappe, S. Kunjachan, J. Rottmann, J. Robar, P. Tsiamas, H. Korideck, O. Tillement and R. Berbeco, Cancer Nanotechnol., 2015, 6, 4 CrossRef PubMed.
- F. Lux, V. L. Tran, E. Thomas, S. Dufort, F. Rossetti, M. Martini, C. Truillet, T. Doussineau, G. Bort, F. Denat, F. Boschetti, G. Angelovski, A. Detappe, Y. Crémillieux, N. Mignet, B.-T. Doan, B. Larrat, S. Meriaux, E. Barbier, S. Roux, P. Fries, A. Müller, M.-C. Abadjian, C. Anderson, E. Canet-Soulas, P. Bouziotis, M. Barberi-Heyob, C. Frochot, C. Verry, J. Balosso, M. Evans, J. Sidi-Boumedine, M. Janier, K. Butterworth, S. McMahon, K. Prise, M.-T. Aloy, D. Ardail, C. Rodriguez-Lafrasse, E. Porcel, S. Lacombe, R. Berbeco, A. Allouch, J.-L. Perfettini, C. Chargari, E. Deutsch, G. Le Duc and O. Tillement, Br. J. Radiol., 2019, 92, 20180365 Search PubMed.
- P. D. Liu, H. Jin, Z. Guo, J. Ma, J. Zhao, D. Li, H. Wu and N. Gu, IJN, 2016, 11, 5003 CrossRef CAS PubMed.
- H. N. Tran, M. Karamitros, V. N. Ivanchenko, S. Guatelli, S. McKinnon, K. Murakami, T. Sasaki, S. Okada, M. C. Bordage, Z. Francis, Z. El Bitar, M. A. Bernal, J. I. Shin, S. B. Lee, P. Barberet, T. T. Tran, J. M. C. Brown, T. V. Nhan Hao and S. Incerti, Nucl. Instrum. Methods Phys. Res., Sect. B, 2016, 373, 126 CrossRef CAS.
- I. Martínez-Rovira and Y. Prezado, Med. Phys., 2015, 42, 6703 CrossRef PubMed.
- H. E. Townley and G. Wakefield, BioNanoSci, 2014, 4, 307 CrossRef.
- H. E. Townley, J. Kim and P. J. Dobson, Nanoscale, 2012, 4, 5043 RSC.
- M. Nakayama, C. L. Smith, B. N. Feltis, T. J. Piva, F. Tabatabaie, P. D. Harty, F. M. Gagliardi, K. Platts, S. Otto, A. Blencowe, K. Morita and M. Geso, Phys. Med., 2020, 75, 69 CrossRef PubMed.
- K. Morita, Y. Nishimura, S. Nakamura, Y. Arai, C. Numako, K. Sato, M. Nakayama, H. Akasaka, R. Sasaki, C. Ogino and A. Kondo, Colloids Surf., B, 2021, 198, 111451 CrossRef CAS PubMed.
- K. Morita, S. Miyazaki, C. Numako, S. Ikeno, R. Sasaki, Y. Nishimura, C. Ogino and A. Kondo, Free Radical Res., 2016, 50, 1319 CrossRef CAS PubMed.
- Z. He, H. Yan, W. Zeng, K. Yang and P. Rong, J. Mater. Chem. B, 2021, 9, 1625 RSC.
- M. Zangeneh, H. A. Nedaei, H. Mozdarani, A. Mahmoudzadeh, S. Kharrazi and M. Salimi, Nanomed. J., 2019, 6, 276–290, DOI:10.22038/nmj.2019.06.000006.
- M. Zangeneh, H. A. Nedaei, H. Mozdarani, A. Mahmoudzadeh and M. Salimi, Mater. Sci. Eng., C, 2019, 103, 109739 CrossRef CAS PubMed.
- T. J. Meyer, A. Scherzad, H. Moratin, T. E. Gehrke, J. Killisperger, R. Hagen, G. Wohlleben, B. Polat, S. Dembski, N. Kleinsasser and S. Hackenberg, Materials, 2019, 12, 4062 CrossRef CAS PubMed.
- R. Generalov, W. B. Kuan, W. Chen, S. Kristensen and P. Juzenas, Colloids Surf., B, 2015, 129, 79 CrossRef CAS PubMed.
- P. Liu, Z. Huang, Z. Chen, R. Xu, H. Wu, F. Zang, C. Wang and N. Gu, Nanoscale, 2013, 5, 11829 RSC.
- R. Xu, J. Ma, X. Sun, Z. Chen, X. Jiang, Z. Guo, L. Huang, Y. Li, M. Wang, C. Wang, J. Liu, X. Fan, J. Gu, X. Chen, Y. Zhang and N. Gu, Cell Res., 2009, 19, 1031 CrossRef CAS PubMed.
- J. Ma, R. Xu, J. Sun, D. Zhao, J. Tong and X. Sun, J. Nanosci. Nanotechnol., 2013, 13, 1472 CrossRef CAS PubMed.
- J. Zhao, P. Liu, J. Ma, D. Li, H. Yang, W. Chen and Y. Jiang, IJN, 2019, 14, 9483 CrossRef CAS PubMed.
- Z. Liu, H. Tan, X. Zhang, F. Chen, Z. Zhou, X. Hu, S. Chang, P. Liu and H. Zhang, Artif. Cells, Nanomed., Biotechnol., 2018, 46, S922 CrossRef PubMed.
- H. Wu, J. Lin, P. Liu, Z. Huang, P. Zhao, H. Jin, J. Ma, L. Wen and N. Gu, Biomaterials, 2016, 101, 1 CrossRef CAS PubMed.
- Y. Du, H. Sun, F. Lux, Y. Xie, L. Du, C. Xu, H. Zhang, N. He, J. Wang, Y. Liu, G. Leduc, T. Doussineau, K. Ji, Q. Wang, Z. Lin, Y. Wang, Q. Liu and O. Tillement, ACS Appl. Mater. Interfaces, 2020, 12, 56874 CrossRef CAS PubMed.
- G. Bort, F. Lux, S. Dufort, Y. Crémillieux, C. Verry and O. Tillement, Theranostics, 2020, 10, 1319 CrossRef CAS PubMed.
- C. Verry, S. Dufort, E. L. Barbier, O. Montigon, M. Peoc’h, P. Chartier, F. Lux, J. Balosso, O. Tillement, L. Sancey and G. Le Duc, Nanomedicine, 2016, 11, 2405 CrossRef CAS PubMed.
- M. Luchette, H. Korideck, M. Makrigiorgos, O. Tillement and R. Berbeco, Nanomedicine, 2014, 10, 1751 CrossRef CAS PubMed.
- A.-S. Wozny, M.-T. Aloy, G. Alphonse, N. Magné, M. Janier, O. Tillement, F. Lux, M. Beuve and C. Rodriguez-Lafrasse, Nanomedicine, 2017, 13, 2655 CrossRef CAS PubMed.
- S.-J. Seo, S.-M. Han, J.-H. Cho, K. Hyodo, A. Zaboronok, H. You, K. Peach, M. A. Hill and J.-K. Kim, Radiat. Environ. Biophys., 2015, 54, 423 CrossRef CAS PubMed.
- F. Li, Z. Li, X. Jin, Y. Liu, P. Li, Z. Shen, A. Wu, X. Zheng, W. Chen and Q. Li, Nanoscale Res. Lett., 2019, 14, 328 CrossRef PubMed.
- P. Zhang, J. Marill, A. Darmon, N. Mohamed Anesary, B. Lu and S. Paris, Int. J. Nanomed., 2021, 16, 2761 CrossRef CAS PubMed.
- L. Maggiorella, G. Barouch, C. Devaux, A. Pottier, E. Deutsch, J. Bourhis, E. Borghi and L. Levy, Future Oncol., 2012, 8, 1167 CrossRef CAS PubMed.
- S. Bonvalot, P. L. Rutkowski, J. Thariat, S. Carrère, A. Ducassou, M.-P. Sunyach, P. Agoston, A. Hong, A. Mervoyer, M. Rastrelli, V. Moreno, R. K. Li, B. Tiangco, A. C. Herraez, A. Gronchi, L. Mangel, T. Sy-Ortin, P. Hohenberger, T. de Baère, A. Le Cesne, S. Helfre, E. Saada-Bouzid, A. Borkowska, R. Anghel, A. Co, M. Gebhart, G. Kantor, A. Montero, H. H. Loong, R. Vergés, L. Lapeire, S. Dema, G. Kacso, L. Austen, L. Moureau-Zabotto, V. Servois, E. Wardelmann, P. Terrier, A. J. Lazar, J. V. M. G. Bovée, C. Le Péchoux and Z. Papai, Lancet Oncol., 2019, 20, 1148 CrossRef CAS PubMed.
- J. Marill, N. M. Anesary and S. Paris, Radiother. Oncol., 2019, 141, 262 CrossRef CAS PubMed.
- J. Qiu, Q. Xiao, X. Zheng, L. Zhang, H. Xing, D. Ni, Y. Liu, S. Zhang, Q. Ren, Y. Hua, K. Zhao and W. Bu, Nano Res., 2015, 8, 3580 CrossRef CAS.
- L. Wen, L. Chen, S. Zheng, J. Zeng, G. Duan, Y. Wang, G. Wang, Z. Chai, Z. Li and M. Gao, Adv. Mater., 2016, 28, 5072 CrossRef CAS PubMed.
- M. Hullo, R. Grall, Y. Perrot, C. Mathé, V. Ménard, X. Yang, S. Lacombe, E. Porcel, C. Villagrasa, S. Chevillard and E. Bourneuf, Int. J. Mol. Sci., 2021, 22, 4436 CrossRef CAS PubMed.
- E. Porcel, S. Liehn, H. Remita, N. Usami, K. Kobayashi, Y. Furusawa, C. L. Sech and S. Lacombe, Nanotechnology, 2010, 21, 085103 CrossRef PubMed.
- D. Salado-Leza, E. Porcel, X. Yang, L. Štefančíková, M. Bolsa-Ferruz, F. Savina, D. Dragoe, J.-L. Guerquin-Kern, T.-D. Wu, R. Hirayama, H. Remita and S. Lacombe, NSA, 2020, 13, 61 CrossRef CAS PubMed.
- A. Tudda, E. Donzelli, G. Nicolini, S. Semperboni, M. Bossi, G. Cavaletti, R. Castriconi, P. Mangili, A. del Vecchio, A. Sarno, G. Mettivier and P. Russo, Med. Phys., 2022, 49, 568 CrossRef CAS PubMed.
- Y. Chen, J. Yang, S. Fu and J. Wu, IJN, 2020, 15, 9407 CrossRef CAS PubMed.
- M. Ghita, S. J. McMahon, L. E. Taggart, K. T. Butterworth, G. Schettino and K. M. Prise, Sci. Rep., 2017, 7, 44752 CrossRef PubMed.
- S. Penninckx, A.-C. Heuskin, C. Michiels and S. Lucas, Nanomaterials, 2019, 9, 295 CrossRef CAS PubMed.
- S. Penninckx, A.-C. Heuskin, C. Michiels and S. Lucas, Nanomedicine, 2018, 13, 2917 CrossRef CAS PubMed.
- N. Daems, S. Penninckx, I. Nelissen, K. Van Hoecke, T. Cardinaels, S. Baatout, C. Michiels, S. Lucas and A. Aerts, Int. J. Nanomed., 2019, 14, 4991 CrossRef CAS PubMed.
- S. Rosa, C. Connolly, G. Schettino, K. T. Butterworth and K. M. Prise, Cancer Nanotechnol., 2017, 8, 2 CrossRef PubMed.
- N. N. Talik Sisin, S. Zainal Abidin, Y. Muhammad Amir, M. Z. Hafiz, K. Abdul Razak and W. N. Rahman, Int. J. Adv. Sci., Eng. Inf. Technol., 2019, 9, 1434 CrossRef.
- A. Rajaee, X. Wensheng, L. Zhao, S. Wang, Y. Liu, Z. Wu, J. Wang and F. Si-Shen, J. Biomed. Nanotechnol., 2018, 14, 1159 CrossRef CAS PubMed.
- A. Rajaee, S. Wang, L. Zhao, D. Wang, Y. Liu, J. Wang and K. Ying, Phys. Med. Biol., 2019, 64, 195007 CrossRef CAS PubMed.
- Y. Zang, L. Gong, L. Mei, Z. Gu and Q. Wang, ACS Appl. Mater. Interfaces, 2019, 11, 18942 CrossRef CAS PubMed.
- K. Cheng, M. Sano, C. H. Jenkins, G. Zhang, D. Vernekohl, W. Zhao, C. Wei, Y. Zhang, Z. Zhang, Y. Liu, Z. Cheng and L. Xing, ACS Nano, 2018, 12, 4946 CrossRef CAS PubMed.
- X. Yi, L. Chen, X. Zhong, R. Gao, Y. Qian, F. Wu, G. Song, Z. Chai, Z. Liu and K. Yang, Nano Res., 2016, 9, 3267 CrossRef CAS.
- X. Liu, X. Zhang, M. Zhu, G. Lin, J. Liu, Z. Zhou, X. Tian and Y. Pan, ACS Appl. Mater. Interfaces, 2017, 9, 279 CrossRef CAS PubMed.
- S. Yang, G. Han, Q. Chen, L. Yu, P. Wang, Q. Zhang, J. Dong, W. Zhang and J. Huang, IJN, 2021, 16, 239 CrossRef PubMed.
- D. Salado-Leza, A. Traore, E. Porcel, D. Dragoe, A. Muñoz, H. Remita, G. García and S. Lacombe, Int. J. Mol. Sci., 2019, 20, E5648 CrossRef PubMed.
- L. Cheng, C. Yuan, S. Shen, X. Yi, H. Gong, K. Yang and Z. Liu, ACS Nano, 2015, 9, 11090 CrossRef CAS PubMed.
- X. Yu, X. Liu, W. Wu, K. Yang, R. Mao, F. Ahmad, X. Chen and W. Li, Angew. Chem., Int. Ed., 2019, 58, 2017 CrossRef CAS PubMed.
- X. Zhang, Z. Liu, Z. Lou, F. Chen, S. Chang, Y. Miao, Z. Zhou, X. Hu, J. Feng, Q. Ding, P. Liu, N. Gu and H. Zhang, Artif. Cells, Nanomed., Biotechnol., 2018, 46, 975 CrossRef CAS PubMed.
- Y. Cheng, T. Lu, Y. Wang, Y. Song, S. Wang, Q. Lu, L. Yang, F. Tan, J. Li and N. Li, Mol. Pharm., 2019, 16, 3489 CrossRef CAS PubMed.
- H. Xiang, Y. Wu, X. Zhu, M. She, Q. An, R. Zhou, P. Xu, F. Zhao, L. Yan and Y. Zhao, J. Am. Chem. Soc., 2021, 143, 11449 CrossRef CAS PubMed.
- Y. Chen, H. Zhong, J. Wang, X. Wan, Y. Li, W. Pan, N. Li and B. Tang, Chem. Sci., 2019, 10, 5773 RSC.
- W. Zhou, Z. Liu, N. Wang, X. Chen, X. Sun and Y. Cheng, ACS Omega, 2022, 7, 12021 CrossRef CAS PubMed.
- K. Ni, G. Lan, S. S. Veroneau, X. Duan, Y. Song and W. Lin, Nat. Commun., 2018, 9, 4321 CrossRef PubMed.
- G. Lan, K. Ni, S. S. Veroneau, T. Luo, E. You and W. Lin, J. Am. Chem. Soc., 2019, 141, 6859 CrossRef CAS PubMed.
- M. Hassan, M. Nakayama, M. Salah, H. Akasaka, H. Kubota, M. Nakahana, T. Tagawa, K. Morita, A. Nakaoka, T. Ishihara, D. Miyawaki, K. Yoshida, Y. Nishimura, C. Ogino and R. Sasaki, Nanomaterials, 2020, 10, 1125 CrossRef CAS PubMed.
- D. B. Guerra, E. M. N. Oliveira, A. R. Sonntag, P. Sbaraine, A. P. Fay, F. B. Morrone and R. M. Papaléo, Sci. Rep., 2022, 12, 9602 CrossRef CAS PubMed.
- A. L. Neuer, A. Jessernig, L. R. H. Gerken, A. Gogos, F. Starsich, A. H. C. Anthis and I. K. Herrmann, Biomater. Sci., 2022, 10, 6558–6569 RSC.
- B. Seniwal, V. C. Thipe, S. Singh, T. C. F. Fonseca and L. Freitas de Freitas, Front. Oncol., 2021, 11, 766407 CrossRef CAS PubMed.
- H. Kato, X. Huang, Y. Kadonaga, D. Katayama, K. Ooe, A. Shimoyama, K. Kabayama, A. Toyoshima, A. Shinohara, J. Hatazawa and K. Fukase, J. Nanobiotechnol., 2021, 19, 223 CrossRef CAS PubMed.
- S. Yook, Z. Cai, Y. Lu, M. A. Winnik, J.-P. Pignol and R. M. Reilly, J. Nucl. Med., 2016, 57, 936 CrossRef CAS PubMed.
- E.-A. Salvanou, D. Stellas, C. Tsoukalas, B. Mavroidi, M. Paravatou-Petsotas, N. Kalogeropoulos, S. Xanthopoulos, F. Denat, G. Laurent, R. Bazzi, S. Roux and P. Bouziotis, Pharmaceutics, 2020, 12, 188 CrossRef CAS PubMed.
- Y. H. Gholami, R. Maschmeyer and Z. Kuncic, Sci. Rep., 2019, 9, 14346 CrossRef PubMed.
- S. H. Cho, B. L. Jones and S. Krishnan, Phys. Med. Biol., 2009, 54, 4889 CrossRef CAS PubMed.
- W. Ngwa, H. Korideck, A. I. Kassis, R. Kumar, S. Sridhar, G. M. Makrigiorgos and R. A. Cormack, Nanomedicine, 2013, 9, 25 CrossRef CAS PubMed.
- S. Moeendarbari, R. Tekade, A. Mulgaonkar, P. Christensen, S. Ramezani, G. Hassan, R. Jiang, O. K. Öz, Y. Hao and X. Sun, Sci. Rep., 2016, 6, 20614 CrossRef CAS PubMed.
- B. Nasseri, E. Alizadeh, F. Bani, S. Davaran, A. Akbarzadeh, N. Rabiee, A. Bahadori, M. Ziaei, M. Bagherzadeh, M. R. Saeb, M. Mozafari and M. R. Hamblin, Appl. Phys. Rev., 2022, 9, 011317 CAS.
- X. Dai, X. Li, Y. Liu and F. Yan, Mater. Des., 2022, 217, 110656 CrossRef CAS.
- D. Lee, S. Kwon, S. Jang, E. Park, Y. Lee and H. Koo, Bioactive Mater., 2022, 8, 20 CrossRef CAS PubMed.
- J. Mei, Y. Huang and H. Tian, ACS Appl. Mater. Interfaces, 2018, 10, 12217 CrossRef CAS PubMed.
- C.-J. Zhang, Q. Hu, G. Feng, R. Zhang, Y. Yuan, X. Lu and B. Liu, Chem. Sci., 2015, 6, 4580 RSC.
- A. Kamkaew, F. Chen, Y. Zhan, R. L. Majewski and W. Cai, ACS Nano, 2016, 10, 3918 CrossRef CAS PubMed.
- W. Sun, T. Shi, L. Luo, X. Chen, P. Lv, Y. Lv, Y. Zhuang, J. Zhu, G. Liu, X. Chen and H. Chen, Adv. Mater., 2019, 31, 1808024 CrossRef PubMed.
- X. Huang, F. Wan, L. Ma, J. B. Phan, R. X. Lim, C. Li, J. Chen, J. Deng, Y. Li, W. Chen and M. He, Cancer Biol. Ther., 2019, 20, 812 CrossRef CAS PubMed.
- L. Huang, L. Ma, W. Xuan, X. Zhen, H. Zheng, W. Chen and M. R. Hamblin, J. Biomed. Nanotechnol., 2019, 15, 2142 CrossRef CAS PubMed.
- X. Chen, J. Liu, Y. Li, N. K. Pandey, T. Chen, L. Wang, E. H. Amador, W. Chen, F. Liu, E. Xiao and W. Chen, Bioactive Mater., 2022, 7, 504 CrossRef CAS PubMed.
- L. Cui, S. Her, G. R. Borst, R. G. Bristow, D. A. Jaffray and C. Allen, Radiother. Oncol., 2017, 124, 344 CrossRef CAS PubMed.
- A. C. Anselmo and S. Mitragotri, Bioeng. Transl. Med., 2016, 1, 10 CrossRef PubMed.
- N. Scher, S. Bonvalot, C. Le Tourneau, E. Chajon, C. Verry, J. Thariat and V. Calugaru, Biotechnol. Rep., 2020, 28, e00548 CrossRef CAS PubMed.
- P. Rocchi, D. Brichart-Vernos, F. Lux, I. Morfin, L. David, C. Rodriguez-Lafrasse and O. Tillement, Pharmaceutics, 2022, 14, 814 CrossRef CAS PubMed.
- G. Wakefield, M. Gardener, M. Stock and M. Adair, J. Nanomater. Mol. Nanotechnol., 2018, s6, 002, DOI:10.4172/2324-8777.S6-002.
- H. E. Townley, E. Rapa, G. Wakefield and P. J. Dobson, Nanomedicine, 2012, 8, 526 CrossRef CAS PubMed.
- X. Liu, H. Zhang, T. Zhang, Y. Wang, W. Jiao, X. Lu, X. Gao, M. Xie, Q. Shan, N. Wen, C. Liu, W. S. V. Lee and H. Fan, Prog. Biomed. Eng., 2021, 4, 012005 CrossRef.
- M. S. Petronek, D. R. Spitz, G. R. Buettner and B. G. Allen, J. Radiat. Res., 2022, 63, 378 CrossRef CAS PubMed.
- J. Le Guevelou, M. E. Chirila, V. Achard, P. C. Guillemin, O. Lorton, J. W. E. Uiterwijk, G. Dipasquale, R. Salomir and T. Zilli, Int. J. Hyperthermia, 2022, 39, 547 CrossRef CAS PubMed.
- W. Y. Teoh, R. Amal and L. Mädler, Nanoscale, 2010, 2, 1324 RSC.
- R. Mueller, L. Mädler and S. E. Pratsinis, Chem. Eng. Sci., 2003, 58, 1969 CrossRef CAS.
- R. Koirala, S. E. Pratsinis and A. Baiker, Chem. Soc. Rev., 2016, 45, 3053 RSC.
- S. Wilhelm, A. J. Tavares, Q. Dai, S. Ohta, J. Audet, H. F. Dvorak and W. C. W. Chan, Nat. Rev. Mater., 2016, 1, 16014 CrossRef CAS.
- B. Ouyang, W. Poon, Y.-N. Zhang, Z. P. Lin, B. R. Kingston, A. J. Tavares, Y. Zhang, J. Chen, M. S. Valic, A. M. Syed, P. MacMillan, J. Couture-Senécal, G. Zheng and W. C. W. Chan, Nat. Mater., 2020, 19, 1362 CrossRef CAS PubMed.
- S. Bonvalot, P. L. Rutkowski, J. Thariat, S. Carrere, M.-P. Sunyach, E. Saada, P. Agoston, A. Hong, A. Mervoyer, M. Rastrelli, C. Le Pechoux, V. Moreno, R. Li, B. Tiangco, A. Casado Herraez, A. Gronchi, L. Mangel, P. Hohenberger, M. Delannes and Z. Papai, Ann. Oncol., 2018, 29, viii753 CrossRef.
- B. Thiesen and A. Jordan, Int. J. Hyperthermia, 2008, 24, 467 CrossRef CAS PubMed.
- A. L. Neuer, L. R. H. Gerken, K. Keevend, A. Gogos and I. K. Herrmann, Nanoscale Adv., 2020, 2, 2992 RSC.
|
This journal is © The Royal Society of Chemistry 2023 |
Click here to see how this site uses Cookies. View our privacy policy here.