DOI:
10.1039/D2BM01414A
(Review Article)
Biomater. Sci., 2024,
12, 252-269
Research progress and perspective of metallic implant biomaterials for craniomaxillofacial surgeries
Received
31st August 2022
, Accepted 4th October 2023
First published on 3rd January 2024
Abstract
Craniomaxillofacial bone serves a variety of functions. However, the increasing number of cases of craniomaxillofacial bone injury and the use of selective rare implants make the treatment difficult, and the cure rate is low. If such a bone injury is not properly treated, it can lead to a slew of complications that can seriously disrupt a patient's daily life. For example, premature closure of cranial sutures or skull fractures can lead to increased intracranial pressure, which can lead to headaches, vomiting, and even brain hernia. At present, implant placement is one of the most common approaches to repair craniomaxillofacial bone injury or abnormal closure, especially with biomedical metallic implants. This review analyzes the research progress in the design and development of degradable and non-degradable metallic implants in craniomaxillofacial surgery. The mechanical properties, corrosion behaviours, as well as in vitro and in vivo performances of these materials are summarized. The challenges and future research directions of metallic biomaterials used in craniomaxillofacial surgery are also identified.
1. Introduction
For human beings, in addition to maintaining the facial contour and shape, the maxillofacial bone is also closely related to the functions of chewing, pronunciation and speech. Injury or diseases (such as tumors, functional atrophy, congenital diseases, periodontitis, iatrogenic injuries, etc., which can lead to the loss of bone tissues in the oral cavity) will affect the normal life and mental state of patients.1 Cranial problems are also very complex, such as craniosynostosis, traumatic skull defects and deformity. These diseases commonly need the intervention of implant materials to cure, so it promotes the development of implant materials to a certain extent. Currently, bone substitutions including autografts, allografts, xenografts, and artificial materials are used to reconstruct cranio-maxillofacial regions. Autologous bone grafts are considered the gold standard of implants because they contain growth factors for osteoinduction, cells for osteogenesis and the framework for osteoconduction,2 which are thought to favor bone formation. The disadvantages of autologous bone grafts, allografts and xenografts, however, are limited sources, high surgical risks, and high psychological stress.3
Aiming at natural bone grafted defects, researchers have gradually developed various artificial materials. Since hard tissues are responsible for the mechanical stability of the body, materials required to repair, replace and/or restore hard tissues must possess strength, an anti-corrosion/degradation ability, good biocompatibility and good wear resistance, while biomedical metallic materials happen to possess high yield strength (YS), high ultimate tensile strength (UTS), and high fatigue resistance and fracture toughness, and thus have become one of the most widely used materials in craniomaxillofacial surgery;4 particularly, biomedical nondegradable metals such as titanium (Ti) have been widely used in the treatment of calvarial defects, reducing the source of infection after craniotomy5 and repairing the maxillofacial defects of patients with common diseases in craniomaxillofacial surgery.6 But biomedical nondegradable metals also have limitations. Taking Ti plates as an example, due to their excellent corrosion resistance, they will not disappear with the patient's recovery after implantation in the human body, which means that they need to be removed by secondary surgery after healing, and due to the complex craniomaxillofacial structure, reoperation means a greater risk; in addition, Ti plates will cause an obstacle to child growth and produce hypersensitivity to cold stimuli and stress shielding.7,8 Therefore, while continuing to optimize the performance of nondegradable metal materials for biomedical applications, researchers are actively developing degradable metal materials, such as iron (Fe), magnesium (Mg), zinc (Zn), and their alloys which are representative of the current situation at a certain scale, but still have various deficiencies, such as the mechanical properties being relatively poor, the degradation rate not matching the rate of wound recovery and so on. Furthermore, there is a lack of up-to-date and systematic summary of the current research progress of biomedical metal materials in craniomaxillofacial surgery, which may hinder the continuous optimization design of the performance of biomedical metal materials. Therefore, this article summarizes the recent research progress in conventional biomedical metal materials including Ti and its alloys, stainless steel (SS), tantalum (Ta) and biomedical degradable metal materials including iron (Fe), magnesium (Mg), and zinc (Zn), and their alloys in craniomaxillofacial surgery including the skull, maxilla, and mandible. In addition, the challenges and future research directions on metallic biomaterials that can be used in craniomaxillofacial surgery are discussed.
2. Conventional nondegradable metallic biomaterials in craniomaxillofacial reconstruction
As one of the first artificial materials to be implanted in craniomaxillofacial bone, biomedical non-degradable metals have been extensively investigated and are widely used in clinical practice due to their excellent mechanical properties, biocompatibility. and corrosion resistance.4,9 In particular, Ti and its alloys have been developed in various forms for use in craniomaxillofacial surgery such as titanium–nickel (Ti–Ni) springs for the treatment of sagittal cranial suture premature closure,10 Ti mesh for the treatment of skull defects,5 and Ti plates and screws for the treatment of mandibular fractures.6 This section will focus on the progress of research on the use of Ti-based alloys in craniomaxillofacial surgery. Other commonly used conventional nondegradable biomedical metal materials are also presented, including SS, cobalt–chromium (Co–Cr) alloys, and Ta and their main mechanical and biological performances in craniomaxillofacial reconstruction applications are summarized and compared in Table 1.
Table 1 Conventional nondegradable metallic biomaterials and their mechanical and biological performances in craniomaxillofacial reconstruction applications
Type |
Alloy |
Implant site |
Results |
Ref. |
Mechanical properties |
Biocompatibility |
ε: elongation; CP-Ta: commercially pure Ta; UCS: ultimate compression strength; CYS: compression yield strength; and BS: Bending strength. The minimum inhibitory concentration of Ag ions for bacteria and yeast is 30 to 1000 ppb (μg L−1), depending on the type of bacterium and the initial concentration. The cytotoxic concentration of Ag ions to mouse fibroblasts (L929) and human fibroblasts during a short incubation was 500 and 2000 ppb, respectively. |
SS |
SS |
Cranial bone |
In vitro
|
In vivo
|
11
|
-SS springs exerted about 1.7 times more force than Ti-Mo springs. |
-No infections and postoperative complications |
In vivo
|
- There is osteogenic activity, but osteogenesis is slow. |
- Force (SD) provided at variant sites: |
|
F
2 mm = 2.42 N, F9 mm = 2.18 N |
|
In vitro
|
In vivo
|
-SS springs exerted about 1.7 times more force than the memory metal (Ti-based). |
|
In vivo
|
|
12
|
- Force (SD) provided at 2 mm arm spread: |
-No short- or long-term technical complications |
F
SS = 2.3 N, FTi-based = 1.3 N |
-Have bony ingrowth |
—
|
In vivo
|
13
|
|
-The patient returns to normal. |
Ti |
Pure Ti |
Cranial bone |
70% deformation
|
In vivo
|
14
|
YS = 750 MPa, ε = 8% |
-New bone formation was found beside and above the miniplates and screws. |
TorgueTi = 0.53 Nm ≈ TorgueXZCrNiMo = 0.52 Nm |
-No connective tissue |
Pure Ti |
Cranial bone |
— |
In vivo
|
5
|
-No further postoperative complications and recurrent infection (over a follow-up period of greater than 3 years) |
Pure Ti |
Oral and Maxillofacial |
— |
In vivo
|
15
|
-No wound infections and exposures |
-Excellent long-term stability of the reconstructions |
- Sinus walls were reconstructed. |
Ti–6Al–4V |
Cranial bone |
-Construct E (MPa):
|
— |
16 and 17 |
146 ± 9 to E-PBF, 156 ± 10 to L-PBF |
-Energy absorbed at peak load (J):
|
3.1 ± 0.1 to E-PBF, 6.0 ± 0.4 to L-PBF |
Ti–6Al–4V |
Mandibular bone |
— |
In vitro
|
18
|
-Hydrophilic |
-Sample extracts from plasma polishing have serious cytotoxicity. |
-V significantly inhibited the proliferation of human gingival cells (human parotid gland epithelial cells and human prostatic growth factor). |
Ti–6Al–4V (porous) |
Frontal skull |
Parallel:
|
In vivo
|
19
|
E = 12.9(±0.9) GPa, CYS = 107.5(±3.6) MPa, UCS = 148.4(±3.5) MPa |
-The percentage of bone volume over tissue volume inside the pores steadily increased. |
Perpendicular:
|
- After 60 days the implants were filled completely with bony tissue. |
E = 3.9(±2.1) GPa, CYS = 49.6(±20.6) MPa, UCS = 127.1(±29.2) MPa |
- The histological analysis revealed only scarce bone–implant contact. |
Ti–2Ag |
Mandible |
|
In vitro
|
20
|
- Ag ion release: ∼200 ppb (4 h), ∼150 ppb (24 h)a |
- Lower cytotoxicity than CP-Ti |
In vivo
|
- Mild inflammation and no infection |
- The mandible was completely repaired. |
Ti–Mo |
Cranial bone |
In vitro
|
In vivo
|
11
|
SS springs exerted about 1.7 times more force than the Ti–Mo springs. |
- No infections and postoperative complications |
|
In vivo
|
- There is osteogenic activity, but osteogenesis is slow. |
|
- Ti–Mo expander elements gave 1.39 (0.25) N at 2 mm arm spread and 1.09 (0.22) N at 9 mm arm spread. |
|
|
Ti–Ni |
Skull and mandible |
In vitro
|
In vivo
|
10 and 21 |
- The force varied with the shape and the number of deformations cycling. |
Clinical research confirmed the possibility of applying superelastic rings and springs in cranioplasty. |
- Have a permanent plastic strain of 4% |
In vivo
|
-Implant movement |
Ti–Ni |
Cranial bone |
Microhardness values of nitinol averaged 350 kg mm−2 |
In vivo
|
22
|
- No macrophage adhesion |
- New bone was similar in hardness to old bone. |
- Bone contact: BCHA > BCNiTi |
- Bone ingrowth: BIHA < BINiTi |
Ti–Ni |
Cranial bone |
— |
In vivo
|
23
|
New bone formation |
No apparent inflammatory response |
Others |
CP-Ta |
Cranial bone |
— |
The rate of infection is alarmingly high in these large constructs |
24
|
CP-Ta (porous) |
Cranial bone |
UCS = 50–70 MPa |
- There was a complete closure of the cranial defect by the new bone formation. |
25 and 26 |
UTS = 63 MPa, BS = 110 MPa |
- No inflammatory reaction |
|
- The connective tissue might form on the Ta surface in the early stage. |
CoCr |
Mandibular bone |
Prostheses were well tolerated by surrounding tissues and no evidence of wear of the metal implants causing osteolysis and formation of granulomatous. |
- Normal organ function |
27
|
|
- New tissue and bone formation |
Vitallium |
Skull |
— |
- Produced no evidence of tissue reaction |
28
|
2.1. SS for craniomaxillofacial reconstruction
The use of SS for craniofacial repair can be traced back to 1886, but the effect was unsatisfactory until 1967, when Luhr29 performed a maxillofacial surgery using a compression plate, and then evolved the experience of craniofacial reconstruction. Examples include the use of dynamic compression plates,30 self-healing plates for mandibular splits,31,32 and the use of monocortical microplates for mandibular and midface fractures without axial compression.33
SS springs are also used in the field of craniofacial repair. Gewalli et al.11 implanted a dynamic spring made of SS into the rabbit's skull to verify the treatment of premature fusion of the cranial sutures by the dynamic spring (in this experiment, the titanium–molybdenum (Ti–Mo) alloy was used as the control group). The results showed that the force exerted by the SS spring on the skull was stronger than that of the Ti-based shape memory alloy, both in vivo and in vitro. In addition, relatively long-term (24 weeks) in vivo experiments using rabbits also showed that this spring had good biocompatibility, which was manifested in the formation of tissue in the sutures. These results were later confirmed by David et al.12 Lauritzen et al.13 were more concerned about the role of SS springs in children's skull remodeling. Through the implantation of SS springs, the shape of the patient's skull was restored to the normal range within three months. At the same time, the removed spring remains in its original shape. These experiences all brought revolutionary changes to the high corrosion-resistant metallic implants in the field of maxillofacial correction. Since then, many researchers have indirectly tested the relevant properties of non-spring SS by choosing stainless steel as the experimental reference. The results showed that SS exhibited lower biocompatibility and corrosion resistance than Ti and its alloys and lower wear resistance than Co–Cr alloys.4
2.2. Ti and Ti-based biomedical metals for craniomaxillofacial reconstruction
Ti is one of the few materials that naturally meet the requirements of human implantation due to its light weight, high specific strength, low toxicity, and high corrosion resistance.34 Despite the significant developments in complete tissue-engineered cranial and maxillofacial structures, Ti is still the preferred artificial material for the repair of defects in load-bearing areas, including the mandible, maxilla and craniofacial.35,36
2.2.1. Pure Ti.
Brem et al.14 found that commercially pure Ti (CP-Ti; Grade 2) could combine hardness and maximum strength with sufficient malleability to avoid brittle fracture, so suitable plates and screws were prepared by this method. Eichner37 and Schwickerath38 made a mechanical comparison of the Ti plate formed in this way, and found that the bearable force of the plate depends on the YS and geometric shape of the plate-screw system (the size and notch effect caused by screws and screw holes), in which maxillary plate < mandibular plate < SS (590 N < 1250 N < 1250 N). These forces are much greater than the force required for physical chewing (100 N), which can meet the requirements of daily chewing. Animal experiments showed that the abovementioned materials accelerated new bone formation, while restrained connective tissue appearance in mini-pigs. Kuttenberger et al.15 conducted a long-term follow-up study of Ti implants in the oral and maxillofacial bones. The results showed that no wound infections, exposures or loss of the mesh were observed, which suggested that the long-term stability of the reconstructions was excellent. Researchers then conducted the study of the walls of paranasal sinus reconstruction and found that over time, complete re-pneumatization took place. Wind et al.5 then used Ti to perform immediate cranioplasty on three patients and found that the patients had no further postoperative complications and no evidence of recurrent infection over a follow-up period of greater than 3 years. However, the lack of mechanical properties and wear resistance of commercially pure titanium limited its development to some extent.
2.2.2. Ti alloys.
Lewin et al.16,17 investigated the effect of different pore shapes and different printing techniques on the mechanical properties of the additively manufactured Ti–6Al–4V meshes implanted in cranial bone. On the one hand, they compared the mechanical properties of two Ti meshes (D1 and D2) with different pore shapes and showed that the quasi-static applied pressure causes the D2 structure to fracture after 20 mm of deformation, while the D1 structure is only partially deformed. In the impact test, there was no significant difference between the two designs. In general, the deformation of D1 was better controlled and it was less dependent on the loading rate. Furthermore, Ti–6Al–4V samples were prepared by the hot isostatic laser powder bed melting technique (HIPed PBF-L) and the electron beam powder bed melting technique (PBF-EB), respectively, using the D2 model, and the mechanical testing showed that HIPed PBF-L had better mechanical properties.
The main reason for this discrepancy is the large geometric deviation of the samples prepared by PBF-EB. In addition, the large surface roughness of the samples prepared by the PBF-EB technique is also a critical issue, because the surface roughness concentrates stress and reduces the mechanical strength of the printed parts. In addition, larger roughness has a greater effect on cell activity. Bernhardt et al.18 studied the influence of the surface roughness of additively manufactured Ti–6Al–4V implants on their material properties and in vitro biocompatibility. According to the rule that the effective roughness spectrum Ra = 0.2–2 μm which is suitable for short-term oral and maxillofacial implants, the researchers subjected the samples prepared by laser melting deposition to different surface treatment procedures. The results showed that plasma electrolytic polishing would lead to excessive release of vanadium(V) ions, thus reducing cell activity. However, the biocompatibility of samples formed by vibration polishing and electropolishing basically met the requirements of implantation. In addition, the combination of different polishing methods also affected cell activity.
Aluminum (Al) enrichment of the α-phase in Ti was found to be detrimental to its passivity and corrosion resistance.39 Moreover, Al may cause Alzheimer's disease,40,41 while V is toxic to cells and can cause severe allergic reactions,42 and hence, researchers focused their attention on Al-free and/or V-free Ti alloys, such as titanium–silver (Ti–Ag)20 and nickel–titanium (NiTi).21–23 As shown in Table 1, several papers about NiTi were published in recent decades focusing on cranial bone repair,21–23 particularly for children,21 because of its superelasticity. For instance, Lekston et al.10 fabricated U- and Ω-shaped springs using NiTi wires with different diameters and performed mechanical assessments both in vivo and in vitro, and the results indicated that the smaller the diameter of the ring, the larger the pre-deformation during formation and the higher the induced stress. Moreover, U-shaped springs increased the force and the increase in the deformation cycling decreased the force. Morawiec et al.21 suggested that small formation favorably affected induced superelasticity. Simske and Sachdeva22 proved that porous NiTi alloy had good biocompatibility and osteogenic properties through in vivo assessments. For osteogenic properties, they found that a relationship existed between the pore size and both bone apposition and ingrowth. Ayers et al.,23 however, thought if implants were pressed into bone, bone growth might be independent of pore size. Meanwhile, above 150 μm pore size, increased porosity was unnecessary to enhance early cartilaginous ingrowth.
However, the allergy, toxicity, and potential carcinogenicity associated with Ni ion release in NiTi alloys limit their use.43 In order to overcome this problem, surface modifications such as oxidation treatment of NiTi to obtain a Ni-free surface44 and several alternative Ni-free shape memory alloys, such as Nb-based, have been developed.45
There are also studies on the corrosion resistance of Ti in the craniomaxillofacial region, for example, in order to investigate the effect of the surface modification on the corrosion behavior of Ti, long-term open circuit potential (OCP) measurement, cyclic voltammetry, and electrochemical impedance spectroscopy (EIS) were carried out by Suba et al.46 Researchers have found that Ti-oxide layers produced by thermal and electrochemical processes prevent corrosion of pure Ti substrates, both in vivo and in vitro. However, the non-density of oxide coating and mechanical damage can make Cl− in the body fluid directly in contact with the substrate, thus accelerating corrosion. However, the spontaneous formation of phosphate and hydro-phosphate (apatite)47,48 in the human body may close these pores, and thus realize the continuous re-passivation and repair of defects in the layer. González and Mirza-Rosca39 and Marino and Mascaro49 studied the corrosion of Ti in the oral environment. It was found that Mo, V and Fe improved the passivation of Ti and inhibited the active corrosion of the β-phase.39 The EIS of grade 2 Ti was tested by immersion in artificial saliva, and the results showed that with the extension of immersion time, different structures of TiO2 (the anodically grown oxide is generally amorphous whereas thermal oxidation produced mainly the anatase phase) would be formed on the surface of Ti, which has long-term and stable passivation performance; however, the porous structure in grade 2 Ti reduced the corrosion resistance compared to solid pure Ti.49
2.3. Other nondegradable metallic biomaterials for craniomaxillofacial reconstruction
Ta implants in the cranio-maxillofacial area have a longer history than Ti because of its unique combination of properties such as high corrosion resistance25 and biological inertia, as demonstrated experimentally by Ta cranioplasties in 11 cats.50 One important finding in this animal study was the closure of the cranial defect by new bone formation.25 The first Ta biomaterial implanted in a human skull can be traced back to 1941 in the US Naval Hospital in Washington, DC by Fulcher.51 Afterwards, especially in World War II, more than 1000 cases of Ta cranioplasty were performed in the subsequent 5 years. However, the high thermal conductivity of Ta leading to cold or heat sensitivity was a shortcoming and there has been no definitive study on whether Ta implantation in human cranial and maxillofacial regions would have adverse effects on patients or the therapeutic effect on diseases, and Ta use in cranioplasty declined precipitously in the early 1950s.52 However, with the advancement of manufacturing technology, the porosity and average pore size of Ta-based alloys can reach 75%∼85% and 400–600 μm, respectively. However, studies have shown that the average pore size of 400 μm and the porosity of more than 70% can promote osteogenic differentiation and the formation of blood vessels and bone tissues.53–56 In addition, Ta has a low bacterial adhesion rate but poor bactericidal effect.57 Porous Ta also has excellent mechanical properties such as a large coefficient of friction (i.e., high stability at the initial stage of implantation)58 and elastic modulus (E) and compressive strength close to those of cortical bone (i.e., load-bearing capacity).53,59 These superior properties of Ta-based alloys enabled them to find widespread use in orthopedics and dentistry. However, the excellent biological properties of porous Ta are obtained at the expense of some excellent mechanical properties.60 Therefore, balancing the biological and mechanical properties of porous Ta becomes a new challenge for Ta alloys.61
In addition, there have been a small number of cases of cranial and maxillofacial implants made of Co–Cr or vitallium alloys. For example, several Vitallium plates have been fabricated and tested,58 which showed excellent in vivo biocompatibility with no adverse effects to the patients' skulls.62,63 Kummoona et al.27 concluded that Co-Cr implants could satisfy the patients’ physiological needs for mandibular implants and muscle fiber attachments, based on long-term health tracking of patients with 2-part Co–Cr prosthesis.
3. Biodegradable metallic materials for craniomaxillofacial reconstruction
It is estimated that 10–12% of craniofacial implants are removed due to infection, exposure, pain and discomfort.64 Therefore, the development of biodegradable materials continues to receive increasing attention. A variety of biodegradable metallic materials for biomedical use with potential for implantation into the craniomaxillofacial surface have been initially developed in recent years, such as splints and screws for fixation of maxillofacial fractures,65 metallic membranes for guided craniomaxillofacial bone regeneration66,67 and devices for anti-cranial osteolysis and/or antibacterial purposes.68,69 However, most experiments remain in in vitro studies and are particularly underdeveloped for Fe and its alloys. In this section, we focus on the progress of research in craniomaxillofacial bone with three most typical biodegradable metals for biomedical use, namely Fe, Mg and Zn. The biodegradable metallic biomaterials and their main mechanical and biological performances in craniomaxillofacial reconstruction applications are summarized in Table 2.
Table 2 Biodegradable metallic biomaterials for craniomaxillofacial reconstruction and their main mechanical and biological performances in craniomaxillofacial reconstruction applications
Alloy |
Implant site |
Results |
Ref. |
Mechanical properties |
Degradability |
Biological properties |
HV – Vickers hardness. Blocks fibrous connective tissue cells and epithelial cells from surrounding soft tissue, but allows osteoblasts to enter the bone defect area. |
Fe–30Mn |
Cranial bone |
YS = 106.07 ± 8.13 MPa, |
- Corrosion rate: |
In vitro
|
70
|
UTS = 115.53 ± 1.05 MPa |
v = 2.81 ± 0.88 mm per year (nominal surface area); |
- At the initial stage, it was toxic to MC3T3 cells. |
ε = 0.73 ± 0.15% |
v = 0.73 ± 0.22 mm per year (estimated true surface area) |
- At the later stage, it showed good cell compatibility. |
Fe–35Mn |
Cranial and maxillofacial bones |
UTS = 228.1 MPa |
- Corrosion unevenness |
In vitro
|
71
|
Fe–34Mn–1Ca |
|
YS = 189.7 MPa, |
|
|
E = 39 GPa, ε = 1.5% |
- Corrosion rate: |
- Numerous cell-to-cell junctions and cytoplasmic expansion |
UTS = 296.6 MPa, E = 163 GPa, ε = 0.1% (Brittle fracture occurred) |
|
- Fe–Mn–Ca exhibited more live cells than Fe–Mn. |
|
|
- Good cell viability |
Pure Mg |
Mandible |
UTS = 86 MPa, YS = 20 MPa, |
V
cortical bone = 71.5% > Vbone marrow = 9.6% |
In vivo
|
72
|
ε = 13% |
→vcortical bone < vbone marrow |
- Bone remodeling occurred in the area surrounding the screws. |
|
|
- New bone was seen growing up next to and in contact with the metal. |
AZ31 |
|
The pull force of AZ31 was similar to SS |
V
cortical bone = 44.2% > Vbone marrow = 20% |
In vivo
|
→vcortical bone < vbone marrow |
- New bone was seen growing up next to and in contact with the metal. |
V
screw head = 61.5% < Vscrew shaft ∼ 39% |
- Significant bone overgrowth was also observed around the AZ31 screws. |
→vscrew head > vscrew shafvMg > vZK31 |
|
ZK60 + PLLA |
Le Fort I |
UTS = 372.94 ± 20.10 MPa |
- Gas formation |
In vivo
|
7
|
YS = 251.16 ± 33.43 MPa |
- Uneven corrosion: |
- The implant moved, the wound opened and became infected. |
|
ε = 15.12 ± 1.95% |
→vcortical bone < vbone marrow |
|
|
|
WE43 |
Frontal bone |
— |
Volume change (V
12–V24): |
In vivo
|
73
|
|
ΔVWE43 = 70 ± 8.2%−43.0 ± 20.9% |
- Bone density (BV/TV): |
>ΔVcoated WE43 = 80 ± 6.3%−76.3 ± 19.2% |
ρ
Ti > ρcoated WE43 > ρWE43 |
Production of gas:
|
- Bone contact area: |
|
BICTi > BICcoated WE43 > BICWE43 |
Gas occurrence decreased with time. |
- No disturbances in wound healing or signs of foreign body reaction. |
WE43 |
Le Fort I |
- Extruded WE43: |
V
60 d = 60.07 ± 15.40 = 60%Vinitial |
In vivo
|
74
|
UTS = 303 MPa, YS = 195 MPa, ε = 6% |
V
24 weeks = 19.37%Vinitial |
- Producing slight inflammation and gas in the initial stage (12 weeks). |
- WE43: |
|
- New bone was well formed near the screw head and covered the screw body. |
UTS = 260 MPa, YS = 160 MPa, ε = 6% |
|
|
WE43 |
Cranial bone |
- As-cast: |
- Implant degradation was more pronounced for the WE43 group relative to the WE43-T5 group. |
In vivo
|
75
|
YS = 157.5 ± 11.1 MPa |
- Absence of increased inflammatory content at both bone and soft tissues interface; |
UTS = 384.9 ± 10.4 MPa |
- New woven bone formation; |
- As-cast + heat treatment |
- Lymph node Mg content quantification: |
(WE43-T5):
|
No implant = 1126 ± 430 ppb |
YS = 244.1 ± 10.3 MPa |
WE43 = 2125 ± 411ppb ≈ WE43 − T5(p > 0.38) |
UTS = 427.7 ± 17.1 MPa |
|
Mg–4Y–3RE |
Cranium |
HV = 114, YS = 280 MPa, UTS = 316 MPa, ε = 5.3%, CYS = 239 MPa, UCS = 402 MPa |
In vitro:
|
In vivo
|
76
|
- vcorrosion rate = 1.51 mm per year |
The biodegradable Mg-based implants have no adverse effects on the behavior or physical condition of rats |
- Local corrosion |
In vivo:
|
- vcorrosion rate = 0.90 mm per year |
- Corrosion products were thick and irregular. |
Mg–6Zn–RE (Y, Gd, La and Ce) |
Cranial bone |
— |
In vivo
|
In vivo
|
77
|
Rapid degradation, 2 weeks to fragmentation and cavitation |
- Swelling at the wound area at the beginning of implantation, which subsides within 2 weeks |
- Excellent osteogenic properties |
- Milder inflammation |
- Rare earth elements accumulate in many organs and are metabolized through lymphatic vessels. |
Pure Zn |
Maxillofacial bone |
YS = 92.16 ± 8.76 MPa, |
In vitro
|
In vitro
|
66
|
UTS = 108 ± 4.87 MPa, |
A relatively uniform corrosion mode |
- Favorable biocompatibility |
ε = 42.82 ± 2.69% |
In vivo
|
- Well attached to the cranial defect area |
- New bones formed in the edge of implants. |
- No obstruction, histopathological changes and accumulation of degradation products |
CP–Zn |
Maxillofacial bone |
A significant reduction in UTS |
In vitro
|
In vitro
|
|
- Local corrosion (pore) |
- Favorable biocompatibility to 300 μm |
- The bigger the hole, the faster the corrosion. |
- Noticeable toxicity to 1000 μm |
In vivo
|
In vivo
|
- The pure Zn membrane with 1000 μm pores almost broke down at week 10. |
- Well attached to the cranial defect area |
- No obstruction, histopathological changes and accumulation of degradation products |
- New bones formed in the edge of implants. |
Zn–xAg (x = 0.5, 1, 2%) |
Cranial bone |
- Increased tensile strength |
In vitro
|
In vitro
|
69
|
- Significant reduction in compressive strength |
- The addition of Ag accelerated the corrosion rate, with the highest corrosion rate for Zn–0.5Ag |
- Antibacterial properties: |
- ε > 30% |
Zn < Zn–0.5Ag ≪ Zn–1Ag < Zn–2Ag |
- Excellent cytocompatibility |
- Zn–2Ag significantly inhibited osteoblast differentiation. |
In vivo (only Zn–2Ag)
|
- Good antibacterial properties and osseointegration in the femur. |
- Effective inhibition of titanium particle-induced cranial osteolysis. |
Zn–xCu (x = 1, 2, 4 wt%) |
Cranio-maxillofacial bone |
Zn–1Cu:
|
- RfZn−Cu was either maintained or increased over 40 days of immersion. |
In vitro
|
68
|
YS = 25.8 MPa, UTS = 32.5 MPa |
- The rolling process appears not to enhance the corrosion resistance of the Zn–Cu alloys. |
- Good cell-to-cell connections |
Zn–2Cu:
|
- The degradation rate of the Zn–Cu alloy may be reduced, while Zn–4Cu has the highest corrosion resistance. |
- No apparent cytotoxicity (reduction of cell viability <30%) |
YS = 50.1 MPa, UTS = 59.5 MPa |
- Promotes cell proliferation to as-rolled Zn–4Cu |
Zn–4Cu:
|
- Poor resistance to adherent mixed oral bacteria |
YS = 73.0 MPa, UTS = 105.4 MPa, ε = 42.2% |
|
Zn–0.5Cu–xFe (x = 0, 0.1, 0.2 and 0.4 wt%) |
Cranial and maxillofacial bones |
- YS and UTS: |
In vitro
|
In vitro
|
67
|
Zn < ZnCu < ZnCuFe |
- Min: ZnCu, Max: ZnCu-0.4Fe |
- ZnCu–0.2Fe initially meets the requirements of the GBR membrane for cell selectiona. |
Max: ZnCu–0.4Fe |
- Compared to α-MEM, ZnCuFe corrodes more rapidly in artificial saliva and increases significantly with increasing Fe content. |
- ZnCu–0.2Fe has a good bacteriostatic and not bactericidal effect. |
- ε (%): |
20.5 = ZnCu−0.4Fe < Zn |
Zn < ZnCu < 40 < ZnCuFe |
Max: ZnCu–0.1Fe |
Zn–2Mg |
Cranial bone |
HV = 97, YS = 235 MPa, |
In vitro:
|
In vivo
|
76
|
UTS = 365 MPa, El. = 4.9%, |
- vcorrosion rate = 0.09 mm per year |
- Favorable biocompatibility to rats |
CYS = 231 MPa, UCS = 426 MPa |
In vivo:
|
|
- vcorrosion rate = 0.10 mm per year |
|
- Corrosion was relatively slow and uniform. |
|
Zn–Mg–Fe |
Mandibular bone |
In a relatively long time (24 weeks), the mechanical properties of Zn–Mg–Fe did not decrease with the extension of time. |
In vivo:
|
— |
65
|
v
4 weeks = 0.033 ± 0.015 mm per year, v12 weeks = 0.079 ± 0.009 mm per year, v24 weeks = 0.095 ± 0.009 mm per year |
|
3.1. Fe-based biodegradable metals for craniomaxillofacial reconstruction
Fe exists in almost all organisms and is essential for their development and survival.78 It is a vital part of various enzymes involved in many biological processes, including DNA biosynthesis, oxygen transport, and cellular energy generation.79
Ferroalloy therefore became one of the earliest metals implanted in the human body. But in the field of metal implants, Fe is different from other metals in that it contains not only SS, a traditional implant with excellent corrosion resistance in the human body, but also new degradable metals that degrade less quickly than Zn and Mg (1–4 months), which researchers have begun to explore the way to increase their degradation rate. For instance, porous Fe-30Mn samples were prepared using 3D printing technology (Fe-30Mn 3D-P).70 Mechanical testing showed that the YS of the porous samples was inferior to that of pure Fe and Fe-30Mn made by sintering and rolling, but it was the closest to that of natural bone, especially the Young's modulus (32.47 ± 5.05 GPa for Fe-30Mn 3D-P compared to 14.1–17.3 GPa for natural cortical bone80). Corrosion testing and cytotoxicity assessment showed that Fe-30Mn 3D-P exhibited a significantly higher corrosion rate than pure Fe with localized corrosion and good biocompatibility.70 Hong et al.71 reported that the addition of Ca/Mg to Fe–Mn increased the corrosion rate of the alloy, especially the addition of Mg had a stronger effect on the corrosion rate (Fig. 1a and b); and the microporous Fe–Mn and Fe–Mn–1Ca metal sheets created by binder-jet 3D printing demonstrated good biocompatibility (Fig. 1c).
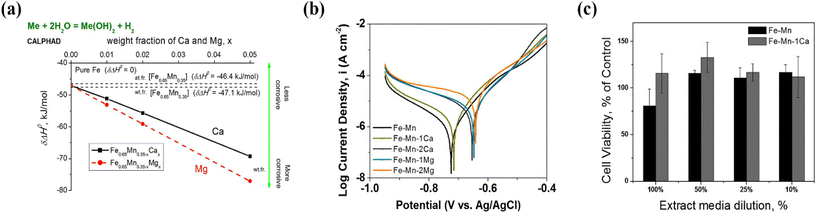 |
| Fig. 1 (a) CALPHAD simulation showing that addition of Ca or Mg to a binary Fe–Mn alloy can accelerate the corrosion of the alloy; (b) Tafel curves measured by potentiodynamic polarization of Fe–Mn, Fe–Mn–Ca, and Fe–Mn–Mg pressed pellets; (c) cell viability of MC3T3-E1 cells incubated with extracts of 3D printing Fe–Mn and Fe–Mn–1Ca for 3 d (n = 3)71 (Copyright 2016 by Elsevier). | |
As Fe presents different valence states under physiological conditions, when it exists in the form of ferrous -Fe(II), it produces reactive oxygen species (ROS) through the Fenton reaction, which damage the functions of cells and organs. In addition, Fe-induced damage is aggravated by the fact that there is no physiological way of Fe excretion, apart from blood loss and (to a lesser extent) shedding of cells.81 The precipitation form and content of Fe limit the implantation of Fe in cranial and maxillofacial areas.
3.2. Mg-based biodegradable metals for craniomaxillofacial reconstruction
The suggested daily intake of Mg lies in the range of 375–500 mg, and 0.75–1.15 mmol L−1 within the blood plasma.82,83 Mg ions are involved in many reactions in the body, such as DNA synthesis, RNA transcription,84 ion diffusion, and formation of cellular signals.85 Excess Mg also collects in the kidneys and is excreted in the urine, which provides for Mg implantation in the body without disrupting the body Mg balance. In addition, the good osteogenic properties72,77 and biomechanical stability of Mg in the craniomaxillofacial region have been demonstrated.86,87 For example, three Mg screws on top plus two Mg screws at the bottom can maintain the mechanical stability of mandibular propulsion, while two Mg screws on top combined with one Mg screw at the bottom can meet the implantation requirements for sagittal split osteotomy of the mandibular branch.86
The excellent biological properties and good mechanical properties have led to a faster progress of research on Mg alloys in craniomaxillofacial surgery than Fe and Zn, and the Mg-based MAGNEZIX®CS screws developed by Syntellix AG are currently in clinical use, which are used to accomplish sturdy internal fixation of mandibular condylar fractures by incision and reduction.88 In addition, sufficient mechanical properties and degradability have led to the use of Mg-based alloys for the preparation of metal membranes to guide cranial regeneration, such as Mg–Al–Zn alloy,89 Mg–Zn–RE(Y, Gd, La and Ce),77 WE43, and Mg3Gd alloys.90
However, the corrosion behavior of Mg, such as excessive degradation and the production of hydrogen gas,91 has led to particular interest in improving the composition and surface properties of Mg alloys for craniomaxillofacial bone applications. The corrosion reactions of Mg in a physiological environment can be expressed as follows:
Mg → Mg2+ + 2e− #(anodic reaction) |
2H2O + 2e− → H2↑ + 2OH− #(cathodic reaction) |
Mg2+ + 2OH− → Mg(OH)2↓ #(overall reaction) |
Mg(OH)2 + Cl− → Mg2+ + Cl− + 2OH− |
Mg(OH)2 + HCO3− → Mg5(CO3)4(OH)2·5H2O |
Henderson et al.72 studied the in vivo degradation behavior of Mg and AZ31 and showed that the degradation rate of Mg-based materials varied depending on the surrounding tissue, exhibiting a significantly lower degradation in the cortical bone than in the medullary cavity, besides, the degradation rate varied between different parts of the same screw.
Based on the fact that alloying has an important influence on the properties of Mg alloys, especially on corrosion behavior, Kubásek et al.76 studied the in vitro and in vitro mechanical properties and corrosion behaviors of Mg-4Y-3RE and reported that rare earth element (REE) suppressed the formation of strong basal texture so that resulted in a decrease in the anisotropy of the mechanical properties of the Mg alloy, and its corrosion rate was still faster than that of the Zn–2Mg alloy (corrosion rate = ∼0.1 mm per year) with local corrosion. In contrast, Zhao et al.77 investigated the degradation behavior of Mg–6Zn–RE (REE = yttrium (Y), gadolinium (Gd), lanthanum (La) and cerium (Ce)) in critical-sized rat calvarial defects and showed that the large surface area accelerated the corrosion of the Mg alloy and that the corrosion channels formed inside the metal film decomposed the membrane into fragments within two weeks. However, these fragments continued to remain stable in the skull defect and formed gas bubbles due to the presence of surface deposits, and these fragments continued to decompose in the gas bubbles.77 In addition, a rapid increase in the content of REEs was observed in the lymph nodes at 4 weeks of implantation due to the dissolution of deposits on the implant surface, which may have adverse effects on human health,92 and it has also suggested that REEs may be phagocytosed by macrophages and enter the lymphatic system to complete metabolism. shows micro-CT images showing the new bone mass at the skull defect after implantation for 2 weeks, 5 weeks, 8 weeks, and a blank defect at 8 weeks.77 It can be seen that an increase in bone mass was observed with increasing implantation time and the defect was completely covered at eight weeks.
Byun et al.7 attempted coating the ZK60 Mg alloy with a poly(L-lactic)-acid (PLLA) polymer and investigated its degradation behavior in a LeFort I osteotomy canine model for maxillofacial applications; the μ-CT images indicated that all the PLLA-coated plates were completely dissolved in the five beagles and the wound dehiscence did not heal after 12 weeks of implantation. Rapid biodegradation of PLLA-coated ZK60 was observed due to the formation of microcracks during the bending process while fitting on the bone, which allowed the bodily fluid to penetrate into the coating and directly contact with ZK60. To improve the corrosion behavior and torsional strength of Mg alloys on the frontal bone, Schaller et al.73 used a proprietary Magoxid electrolyte to form an electrocoating layer on WE43 and reported that this coating caused the degradation rate of WE43 to be slowed and stable. In addition, the presence of the coating also delayed the release of hydrogen gas, and the occurrence of soft tissue cavities caused by gas release decreased with increasing implantation time. Although the formation of a coating enhanced the bone-forming ability of WE43, it was still insufficient compared with Ti.
Byun et al.74 and Torroni et al.75 improved the properties of the WE43 Mg alloy by improving the preparation conditions to satisfy the conditions of cranial and maxillofacial implantation. In the former study,74 the mechanical properties of WE43 were improved by tempering after casting, with 55% and 28% improvement in the YS and UTS for WE43-T5 specimens over the as-cast counterparts, respectively. Extrusion before heat treatment also improved the YS of WE43.75 WE43 was biocompatible (e.g., showing new bone formation and without long-term inflammation), except that the WE43-T5 produced hydrogen gas at the beginning of implantation.74
Overall, the Mg-based alloys in a physiological environment not only exhibit a rapid corrosion rate but also lead to the excessive evolution of hydrogen gas. Due to the special craniomaxillofacial anatomical characteristics, subcutaneous gas cavities may lead to wound healing disorders. These issues impede the widespread use of Mg-based alloys in craniomaxillofacial surgery.68
3.3. Zn-based biodegradable metals for cranio-maxillofacial reconstruction
Zn is the second most abundant micronutrient in living organisms93 with normal plasma levels of 70–120 mg dL−1.94 Humans consume an average of 4–15 mg day−1 of Zn94 to regulate various essential biological functions, including nucleic acid metabolism, signal transduction, apoptosis regulation, and gene expression in the human body,95 especially for bone formation processes, such as activation of amino acid-tRNA synthetase in osteoblasts96 and inhibition of differentiation of bone marrow cells to osteoclasts.97 Furthermore, studies have shown that Zn has an important role in the prevention and treatment of skeletal and neurological disorders,98–100 which provides strong support for the use of Zn alloys in craniomaxillofacial surgery. On the other hand, the moderate degradation behavior of Zn does not leave large amounts of corrosion products in the body that are difficult to be eliminated and its corrosion products,77 such as Zn phosphate, may also improve the biocompatibility of Zn-based implants.101,102
3.3.1. Pure Zn.
The critical role of Zn in the human body and its corrosive nature has promoted some research in craniomaxillofacial surgery. Guo et al.66 prepared pure Zn membranes with different pore sizes (0, 300 μm, and 1000 μm) for guided bone regeneration (GBR) using the degradability of Zn and its effect on bone marrow cell differentiation in order to investigate the feasibility of using pure Zn membranes for GBR in maxillofacial applications. This study demonstrated that the degradation rate increased with increasing pore size and the Zn membrane with 1000 μm pore size corroded most rapidly, and even showed severe cracking after immersion for 28 d in Hanks’ solution. The degradation rates of the Zn membranes with different pore sizes were consistent with the Zn2+ ion concentrations of the immersion solutions with C(0 μm) = 14.4 ± 1.5 μg ml−1 for membranes without pores, C(300 μm) = 19.1 ± 0.9 μg ml−1 for membranes with 300 μm pore size, and C(1000 μm) = 21.3 ± 0.8 μg ml−1 for membranes with 1000 μm pore size. The 1000 μm Zn membrane showed poor biocompatibility, insufficient mechanical strength, low bone regeneration ability, and even new bone collapse. In contrast, the 300 μm Zn membrane showed sufficient mechanical properties (even stronger than pure Ti), excellent biocompatibility, and good osteogenic properties. Therefore, reducing the E by increasing the pore size is not a one-time-for-all solution, and the E, degradation rate and biocompatibility of the implant materials need to be balanced to meet the requirements of craniomaxillofacial implants.103
3.3.2. Zn alloys.
Deficiencies in the properties of Zn and its alloys for craniomaxillofacial bone implants are usually improved by adding other elements in addition to increasing the porosity. The antimicrobial properties and osteolysis resistance of Zn alloys were improved by adding different concentrations of silver (0.5, 1, 2 wt%) and 2 wt% Ag addition was proved most effective.69 The good antimicrobial properties were significant not only against coagulase-positive and negative staphylococci, the common types of bacteria tested, but also against drug-resistant strains (methicillin resistant Staphylococcus aureus and methicillin-resistant Staphylococcus epidermidis). The mechanical and degradation properties of these alloys are listed in Table 2. Cu2+ plays a role in improving the antimicrobial properties of Zn–Cu alloys, but previous research has shown that neither too high nor too low copper content can make the mechanical properties of Zn–Cu alloys meet the minimum requirements for biodegradable craniomaxillofacial bone implants (i.e., YS > 230 MPa, UTS > 300 MPa, and ε > 15–18%),104–106 and that a lower copper content also makes Zn–Cu alloys insufficiently resistant to osteolysis.106 Li et al.68 reported the tensile properties of the as-cast and hot-rolled Zn–xCu alloys (x = 0, 1, 2, and 4 wt%) (Fig. 2). It can be seen that for both the as-cast and as-rolled Zn–xCu alloys, the YS and UTS increased with increasing Cu addition, and the increase for the as-rolled Zn–Cu alloys was more significant, but ε did not show a significant change with the Cu addition, and it was concluded that Zn–4Cu appeared to be the most suitable material for craniomaxillofacial bone implant applications based on its highest YS and UTS values among this series of Zn–xCu alloys. The corrosion of the Cu-containing alloys was more uniform due to the increase in the content or refinement of the second phase CuZn5. Furthermore, Zn–xCu alloys showed good biocompatibility with a promotion effect on cell proliferation. However, it is not realistic to solely rely on the copper ions released by the metal matrix degradation to achieve a rapid and complete sterilization effect.
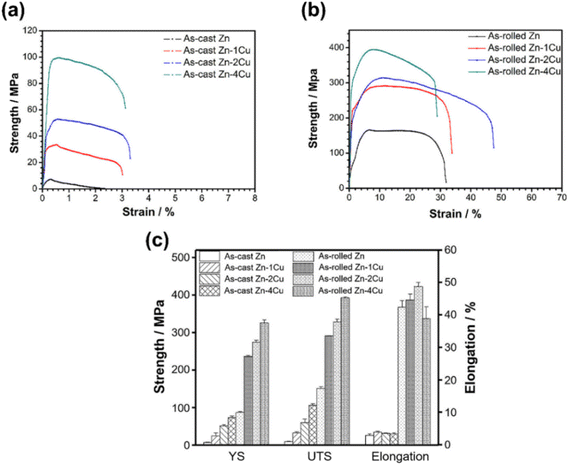 |
| Fig. 2 Tensile properties of as-cast and as-rolled Zn–xCu (x = 0, 1, 2, and 4 wt%) alloys: (a) stress–strain curves of as-cast alloys; (b) stress–strain curves of as-rolled alloys; (c) YS, UTS, and ε of as-cast and as-rolled alloys68 (Copyright 2019 by Elsevier). | |
On the other hand, the high solubility of Cu in Zn makes the microstructure of the Zn–Cu alloys similar to that of pure Zn and it is difficult to form a second phase which would provide a reinforcing effect. Zhang et al.67 added Fe to Zn to form an FeZn13 second phase and fabricated a non-porous Zn–0.5Cu–xFe (x = 0.1, 0.2, 0.4 wt%) membranes with 1.5 mm thickness by hot extrusion for guided bone regeneration applications. It was demonstrated that the mechanical properties of the films were significantly improved, except for a significant decrease in the ε of Zn–0.5Cu–0.4Fe. In vitro antimicrobial and cytocompatibility tests also showed good bacterial inhibition against S. gordonii media and mixed oral bacteria and appropriate cytocompatibility in Zn–0.5Cu–0.2Fe due to the release of Zn ions. Notably, the degradation rates of Zn–0.5Cu and Zn–0.5Cu–xFe in α-MEM and artificial saliva simulating the craniomaxillofacial environment differed considerably. For example, Zn–0.5Cu–0.2Fe did not degrade as fast as Zn–0.1Cu–0.1Fe in α-MEM, but in artificial saliva, the former was significantly faster than the latter; overall, a significant increase in the degradation rate was observed with increasing the contents of Fe. This could be due to the organic components in α-MEM decomposing with pH and adsorbing onto the Zn alloy, which in turn affects the corrosion behavior of the Zn alloys. Therefore, for biodegradable Zn-based alloys, the transient electrochemical corrosion results cannot directly translate into a prediction of their long-term degradation.
Fig. 3 shows a comparison of the tensile properties of hot-extruded biodegradable Zn–xMg (x = 0, 0.02, 0.08, 0.5, 0.8, 1, 2, and 3 wt%) alloys. It can be seen that the addition of Mg significantly strengthened pure Zn,107 while Kubásek et al.76 showed that the Zn–2Mg alloy showed a more uniform and low degradation rate after implanted in rat cranium compared to WE43.
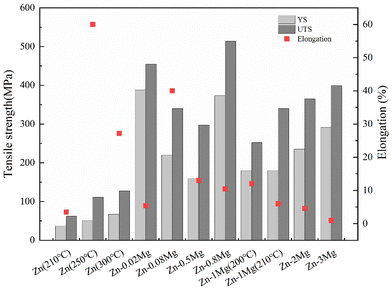 |
| Fig. 3 Comparison of tensile properties of hot-extruded biodegradable Zn–xMg alloys (x = 0, 0.02, 0.08, 0.5, 0.8, 1, 2, and 3 wt%)107 (Copyright 2021 by Elsevier). | |
Based on this, Wang et al.65 added Fe to Zn–Mg alloys (Zn ≥ 95, 0.001 ≤ Mg ≤ 2.5, and 0.01 ≤ Fe ≤ 2.5, wt%) and carried out a comprehensive study using a canine mandibular fracture model. The results showed that the Zn–Mg–Fe alloys corroded uniformly and slowly in vivo at a degradation rate of 0.095 ± 0.009 mm per year with good biosafety and biocompatibility. At the same time, the Zn ions played a significant role in the bone formation process, which imparted the Zn–Mg–Fe alloy with superior osteogenic properties compared to Ti and PLLA. Fig. 4 shows histological and micro-CT cross-sectional images of the Zn–Mg–Fe alloy, PLLA, and Ti–6Al–4V bone plates and screws after implantation in beagles for 0, 4, and 12 weeks. It can be seen that at 4 weeks post-operation, more external callus appeared in the PLLA implant area than in the other two groups. At 12 weeks post-operation, large areas of wide and thick woven bone were formed around the Zn–Mg–Fe and Ti–6Al–4V alloys, whereas there were small and discontinuous areas of woven bone observed in the PLLA group.65
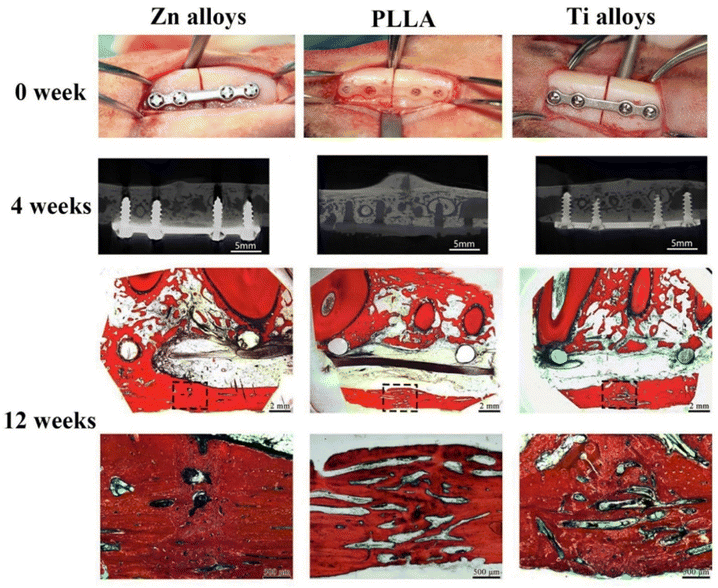 |
| Fig. 4 Histological and micro-CT cross-sectional images of Zn–Mg–Fe alloy, PLLA, and Ti–6Al–4V bone plates and screws after implantation in beagles for 0, 4, and 12 weeks65 (Copyright 2019 by Elsevier). | |
4. Summary and outlook
In summary, as illustrated in Fig. 5, biomedical metals have great research value in the field of craniofacial repair and plastic surgery. However, the research on large animal experiments and clinical trials is still limited. The design requirements for artificial implant materials include: (1) facilitating clinical manipulation, (2) being structurally compatible with the defect, (3) good biocompatibility (meaning that the implant can promote the development of tissues and cells in the environment of bone defects, including the proliferation and adhesion of osteogenic-related cells, the differentiation of bone progenitor cells, the integration of bone tissue with the implant material, and osteogenic-related angiogenesis108), (4) sufficient mechanical properties to provide a stable space for bone regeneration, and (5) minimizing complications.109,110 These requirements make most of the currently developed biomedical metal materials still inadequate for implantation, including in the craniomaxillofacial area.111,112 In addition, minimizing the impact of implant infection on the implantation is also an urgent issue that must be addressed in the field of craniofacial repair and plastic surgery. Therefore, appropriate materials and processing techniques must be continuously explored in the future. Some possible research directions on these aspects are proposed below.
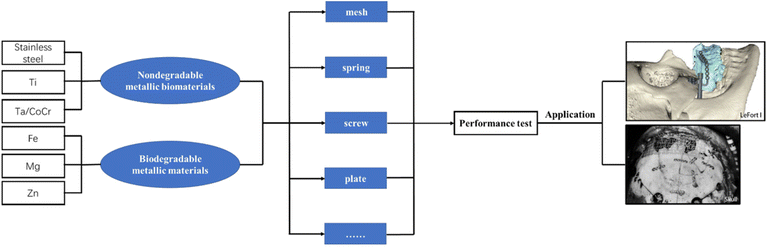 |
| Fig. 5 Illustration of biomedical metals in the field of craniofacial repair and plastic surgery. | |
4.1. Conventional nondegradable metallic biomaterials
4.1.1. Improving antibacterial activity.
Infection with implant materials is detrimental to patient recovery and, once infected, it would lead to implant failure and is often associated with chronic and/or recurrent disease. On the other hand, infection is often difficult to treat because of antibiotic resistance, tolerance, and/or persistence.113–115 Yavari et al.116 applied a multilayer gelatin and chitosan-based coating containing bone morphogenetic protein (BMP)-2 and/or vancomycin to the surface of a porous Ti fabricated by selective laser melting. This structure with different types and concentrations of active substances in each layer allowed the continuous release of antibiotics to kill any bacteria that enter the body during the perioperative period and help host cells win the “surface competition”.117 The results also verified the bactericidal effect and bone formation promoting effect of the multi-layer structure. Of course, how the coating on the surface of the drug or material is firmly combined with the base metal is also a problem that cannot be ignored in design. In this regard, bionics is a strategy worth learning. For example, Wang et al.118 learned from the molecular adhesion mechanism of the marine mussel foot protein, and used this protein to firmly “lock” metal ions (Zn2+) and bone induction growth factor (BMP-2 peptide) on the titanium group, which greatly improved the mechanical and osteogenic properties of the Ti group. In addition, the fluidity and buffering of body fluids significantly diminished the antimicrobial effect of the material in vivo67 and therefore placed a greater demand on in vitro antimicrobial testing.
4.1.2. Modulation of inflammatory response to promote osteogenic performance.
In the treatment of diseases by implantation of implants, it is inevitable to evoke a response of immune cells dominated by macrophages, which trigger inflammation, while inflammation is involved in tissue regeneration and the development of fibrosis,119 in which fibrous tissue formed through early inflammation can provide a barrier to osseointegration, but earlier studies have shown that organisms cannot select by themselves the inflammatory response for the course of tissue regeneration and fibrosis, such as in amputated salamanders. Either an antifibrotic process or both a profibrotic and antifibrotic process mediated by immunity (this process is balanced) is generated,120 and thus promoting wound healing with the aid of inflammatory response and achieving osseointegration need to be artificially regulated. Zhao et al.121 developed a type I collagen (COL1) modified nano-porous network on Ti substrates via alkali treatment, polydopamine coating, and layer-by-layer (LBL) self-assembly, and this coating could regulate the differentiation of macrophages and inhibit the expression of inflammation and osteoclast related genes, thereby promoting subsequent vasculature/bone formation. Huang et al.122 studied the effects of M1 type cells and M2 type cells, respectively, on human gingival fibroblasts attached to Ti substrates and similarly showed that in the M1 immune microenvironment, fibroblasts seem to aggravate inflammation in a positive cyclic manner, whereas in the M2 immune microenvironment, fibroblasts produce better soft tissue regeneration, adhesion to and contraction of metallic materials, and proposed the concept of an “inflammation-fibrous” complex. Yu et al.123 promoted the repair of infectious (bacterial) fractures by directly regulating the release of exosomal mir-708-5p.
4.2. Biodegradable metallic materials
Although it is difficult to obtain craniomaxillofacial bones at all ages of the human body, and the research on the mechanics and biology of craniomaxillofacial bones is very limited, there are still some data for reference. The data for 6 to 10 months are more representative as the samples are fresh, when they correspond to an E of 2.3 GPa to 6.4 GPa, an HV of 4.0 GPa to 7.2 GPa and a bending failure strain of 6.7%.124 In addition, cranial tissue hardens with age, and previous studies have shown that elastic modulus (determined by assuming a solid section) increases from approximately 0.5 GPa at birth125 to as high as 10 GPa by the age of 6 years (at which point the elastic modulus of the cortical bone of the skull is 9.8 ± 71.24 GPa and the elastic modulus of the sutures is 1.10 ± 0.53 GPa. The triple-layered bone has an effective modulus of elasticity is 3.69 ± 0.92 GPa).126 By adulthood, the hardness of skull tissue will be three times that of children. The bending strength decreases correspondingly, and in adulthood, the bending strength is only one-fifth of that of young children.124,127 Since maxillofacial fractures are the most common disease among craniomaxillofacial fractures, especially mandibular fractures, which can account for 70% of facial fractures,128 researchers have done more research on the mechanical properties and stress distribution of maxillofacial bone, among which the elastic modulus is 1500 MPa.87
According to the above data of craniomaxillofacial bone, there is still a large gap between the mechanical properties of the degradable metal materials developed at present and those of natural bone, which may cause the movement of the degradable metal materials implanted into craniomaxillofacial bone, especially in the skull (in addition to the high hardness, there is also the presence of intracranial pressure). This unmet clinical need will continue to prompt the development of new alloys. Specifically, it is a great challenge to manufacture efficient tissue-engineered craniomaxillofacial bone grafts. It can reproduce the complex structure of craniomaxillofacial bone, which is the key factor in achieving natural and appropriate mechanical properties, so as to achieve the desired curative effect. Ceramic materials have high hardness, but their toughness is poor, while biomedical degradable materials have relatively high elongation. The combination of the two may further improve the strength of the metal matrix to meet the requirements of mechanical properties of implanted materials in the early stage of bone repair. For example, Bagherifard et al.129 also sandblasted the surface of AZ331, and compared its mechanical properties with those of untreated Mg alloy. It is found that the microhardness of Mg alloy after sandblasting is 133% higher than that of untreated Mg alloy. Jamalian et al.130 also sandblasted the surface of AZ31 and found that the surface grain of AZ31 is refined under high pressure. The microhardness of AZ31 after treatment was increased from 50 Hv to 92 Hv. In addition, ceramics can also protect metal substrates to a certain extent. For example, the protection of Ti substrates by Ca–P under impact force is described in section 2.
Conflicts of interest
There are no conflicts to declare.
Acknowledgements
The authors are thankful for the support by the National Natural Science Foundation of China (Grant No. 31700819), the Young Elite Scientists Sponsorship Program by CAST (YESS, Grant No. 2018QNRC001), and the Fundamental Research Funds for the Central Universities and the Youth Teacher International Exchange & Growth Program (No. QNXM20220020).
References
- J. Rubio-Palau, A. Prieto-Gundin, A. Cazalla, M. Serrano, G. Fructuoso and F. Ferrandis, Bar, #243, A., Three-dimensional planning in craniomaxillofacial surgery, Ann. Maxillofac. Surg., 2016, 6(2), 281–286 CrossRef PubMed
.
- V. Martin and A. Bettencourt, Bone regeneration: Biomaterials as local delivery systems with improved osteoinductive properties, Mater. Sci. Eng., C, 2018, 82, 363–371 CrossRef CAS PubMed
.
-
R. Mazzonetto, H. D. Netto and F. F. Nascimento, Enxertos ósseos em implantodontia, Napoleão, Nova Odessa, 2012 Search PubMed
.
-
A. Nouri and C. Wen, Chapter 3 - Stainless steels in orthopedics, in Structural Biomaterials, ed. C. Wen, Woodhead Publishing, 2021, pp. 67–101 Search PubMed
.
- J. J. Wind, C. Ohaegbulam, F. M. Iwamoto, P. M. Black and J. K. Park, Immediate titanium mesh cranioplasty for treatment of postcraniotomy infections, World Neurosurg., 2013, 79(1), 207e11–207e13 CrossRef PubMed
.
- H. K. Lim, Y. J. Choi, W. C. Choi, I. S. Song and U. L. Lee, Reconstruction of maxillofacial bone defects using patient-specific long-lasting titanium implants, Sci. Rep., 2022, 12(1), 7538 CrossRef CAS PubMed
.
- S.-H. Byun, H.-K. Lim, S.-M. Lee, H.-E. Kim, S.-M. Kim and J.-H. Lee, Biodegradable Magnesium Alloy (ZK60) with a Poly(l-lactic)-Acid Polymer Coating for Maxillofacial Surgery, Metals, 2020, 10(6), 724 CrossRef
.
- J. Nagels, M. Stokdijk and P. M. Rozing, Stress shielding and bone resorption in shoulder arthroplasty, J. Shoulder Elbow Surg., 2003, 12(1), 35–39 CrossRef PubMed
.
- H. Pereira, I. Cengiz, F. Maia, F. Bartolomeu, J. M. Oliveira, R. Reis and F. S. Silva, Physicochemical properties and cytocompatibility assessment of non-degradable scaffolds for bone tissue engineering applications, J. Mech. Behav. Biomed. Mater., 2020, 112, 103997 CrossRef CAS PubMed
.
- Z. Lekston, J. Drugacz and H. Morawiec, Application of superelastic NiTi wires for mandibular distraction, Mater. Sci. Eng., A, 2004, 378(1–2), 537–541 CrossRef
.
- F. Gewalli, J. P. da Silva Guimarães-Ferreira, G. Maltese, U. Örtengren and C. Lauritzen, Expander Elements In Craniofacial Surgery: An Experimental Study In Rabbits, J. Oral Surg., 2001, 35(2), 149–156 CAS
.
- L. R. David, F. Gewalli, J. Guimãraes-Ferreira, C. Sanger, S. Glazier and L. C. Argenta, Dynamic Spring-Mediated Cranioplasty in a Rabbit Model, J. Craniofac. Surg., 2002, 13(6), 794–801 CrossRef PubMed
.
- C. Lauritzen, Y. Sugawara, O. Kocabalkan and R. Olsson, Spring mediated dynamic craniofacial reshaping: Case report, Scand. J. Plast. Reconstr. Surg. Hand Surg., 1998, 32(3), 331–338 CrossRef CAS PubMed
.
- E. S. J. Breme and G. Paulus, Commercially pure titanium Steinhäuser plate-screw system for maxillofacial surgery, Biomaterials, 1988, 9(4), 310–313 CrossRef PubMed
.
- J. J. Kuttenberger and N. Hardt, Long-term results following reconstruction of craniofacial defects with titanium micro-mesh systems, J. Cranio-Maxillofac. Surg., 2001, 29(2), 75–81 CrossRef CAS PubMed
.
- S. Lewin, J. Åberg, D. Neuhaus, H. Engqvist, S. J. Ferguson, C. Öhman-Mägi, B. Helgason and C. Persson, Mechanical behaviour of composite calcium phosphate–titanium cranial implants: Effects of loading rate and design, J. Mech. Behav. Biomed. Mater., 2020, 104, 103701 CrossRef CAS PubMed
.
- S. Lewin, I. Fleps, J. Åberg, S. J. Ferguson, H. Engqvist, C. Öhman-Mägi, B. Helgason and C. Persson, Additively manufactured mesh-type titanium structures for cranial implants: E-PBF vs. L-PBF, Mater. Des., 2021, 197, 109207 CrossRef CAS
.
- A. Bernhardt, J. Schneider, A. Schroeder, K. Papadopoulous, E. Lopez, F. Bruckner and U. Botzenhart, Surface conditioning of additively manufactured titanium implants and its influence on materials properties and in vitro biocompatibility, Mater. Sci. Eng., C, 2021, 119, 111631 CrossRef CAS PubMed
.
- S. Ponader, C. von Wilmowsky, M. Widenmayer, R. Lutz, P. Heinl, C. Korner, R. F. Singer, E. Nkenke, F. W. Neukam and K. A. Schlegel, In vivo performance of selective electron beam-melted Ti-6Al-4 V structures, J. Biomed. Mater. Res., Part A, 2010, 92(1), 56–62 CrossRef PubMed
.
- J.-S. K. Jung-Hwan Lee, S.-K. Moon, S.-H. Uhm, B.-H. Choi, U.-H. Joo, K.-M. Kim and K.-N. Kim, Titanium-Silver Alloy Miniplates for Mandibular Fixation: In Vitro and In Vivo Study, J. Oral Maxillofac. Surg., 2016, 74(8), 1622e1–1622.e12 CrossRef PubMed
.
- H. Z. Morawiec, Z. H. Lekston, K. F. Kobus, M. C. Wegrzyn and J. T. Drugacz, Superelastic NiTi springs for corrective skull operations in children with craniosynostosis, J. Mater. Sci. Mater. Med., 2007, 18(9), 1791–1798 CrossRef CAS PubMed
.
- S. Simske and R. Sachdeva, Cranial bone apposition and ingrowth in a porous nickel–titanium implant, J. Biomed. Mater. Res., 1995, 29(4), 527–533 CrossRef CAS PubMed
.
- R. A. Ayers, S. J. Simske, T. Bateman, A. Petkus, R. Sachdeva and V. Gyunter, Effect of nitinol implant porosity on cranial bone ingrowth and apposition after 6 weeks, J. Biomed. Mater. Res., 1999, 45(1), 42–47 CrossRef CAS PubMed
.
- S. R. Durham, J. G. McComb and M. L. Levy, Correction of large (> 25 cm2) cranial defects with “reinforced” hydroxyapatite cement: technique and complications, Neurosurgery, 2003, 52(4), 842–845 CrossRef PubMed
.
- R. H. Pudenz, The repair of cranial defects with tantalum: an experimental study, J. Am. Med. Assoc., 1943, 121, 478–481 CrossRef
.
- L. D. Zardiackas, D. E. Parsell, L. D. Dillon, D. W. Mitchell, L. A. Nunnery and R. Poggie, Structure, metallurgy, and mechanical properties of a porous tantalum foam, J. Biomed. Mater. Res., 2001, 58(2), 180–187 CrossRef CAS PubMed
.
- R. Kummoona, Temporomandibular joint reconstruction with a 2-part chrome-cobalt prosthesis, chondro-osseous graft, and silastic: clinical and experimental studies, J. Craniofac. Surg., 2009, 20(6), 2125–2135 CrossRef PubMed
.
- C. S. Beck, Repair of defects in skull by ready made vitallium plates, J. Am. Med. Assoc., 1942, 118(10), 798–799 CrossRef
.
- H. G. Luhr, Entwicklung der modernen Osteosynthese, Mund-, Kiefer- und Gesichtschirurgie, 2000, 4(1), S084–S090 Search PubMed
.
- B. Spiessl, G. Schargus and K. Schroll, Die stabile Osteosynthese bei Frakturen des unbezahnten Unterkiefers, Schweiz Mschr Zahnheilk, 1971, 81, 39 CAS
.
-
R. Becker and E. Machtens, Druckplattenosteosynthese zur Frakturbehandlung und bei orthopädischchirurgischen Maßnahmen am Gesichtsschädel, Osteo News, 1973, 19 Search PubMed.
- H. Niederdellmann and W. Schilli, Zur Plattenosteosynthese bei unterkieferfrakturen, Dtsch zahnarztl Z, 1973, 28, 638 CAS
.
- F. Michelet and A. Moll, Traitements chirurgicaux des fractures du corps mandibulaire sans blocage, par plaques vissées miniatures insérées par voie endobuccale, Rev. Odontostomatol., 1971, 29(2), 87–105 CAS
.
- Q. Zhang, W. Wu, C. Qian, W. Xiao, H. Zhu, J. Guo, Z. Meng, J. Zhu, Z. Ge and W. Cui, Advanced biomaterials for repairing and reconstruction of mandibular defects, Mater. Sci. Eng., C, 2019, 103, 109858 CrossRef CAS PubMed
.
- S. W. On, S. W. Cho, S. H. Byun and B. E. Yang, Bioabsorbable Osteofixation Materials for Maxillofacial Bone Surgery: A Review on Polymers and Magnesium-Based Materials, Biomedicines, 2020, 8(9), 300 CrossRef CAS PubMed
.
- Q. Zhang, W. Wu, C. Qian, W. Xiao, H. Zhu, J. Guo, Z. Meng, J. Zhu, Z. Ge and W. Cui, Advanced biomaterials for repairing and reconstruction of mandibular defects, Mater. Sci. Eng., C, 2019, 103, 109858 CrossRef CAS PubMed
.
- K. Eichner, Messung der Krafte bei Kauvorgangen, Dtsch, 1963, 18, 915–924 Search PubMed
.
- H. Schwickerath, Kaukraft. Kaudruck. Belastbarkeit, Dtsch, 1976, 31, 870–873 CAS
.
- J. E. G. González and J. C. Mirza-Rosca, Study of the corrosion behavior of titanium and some of its alloys for biomedical and dental implant applications, J. Electroanal. Chem., 1999, 471(2), 109–115 CrossRef
.
- M. Crapper and D. Boni, Aluminium in human brain disease: an overview, Neurotoxicology, 1980, 1(4), 3–16 Search PubMed
.
- D. P. Perl and A. R. Brody, Alzheimer's disease: X-ray spectrometric evidence of aluminum accumulation in neurofibrillary tangle-bearing neurons, Science, 1980, 208(4441), 297–299 CrossRef CAS PubMed
.
- P. G. Laing, A. B. Ferguson Jr. and E. S. Hodge, Tissue reaction in rabbit muscle exposed to metallic implants, J. Biomed. Mater. Res., 1967, 1(1), 135–149 CrossRef CAS PubMed
.
- J. Black, Biologic performance of tantalum, Clin. Mater., 1994, 16(3), 167–173 CrossRef CAS PubMed
.
- E. P. Strecker, D. Liermann, K. H. Barth, H. Wolf, N. Freudenberg, G. Berg, M. Westphal, P. Tsikuras, M. Savin and B. Schneider, Expandable tubular stents for treatment of arterial occlusive diseases: experimental and clinical results. Work in progress, Radiology, 1990, 175(1), 97–102 CrossRef CAS PubMed
.
- P. A. Ribeiro, R. Gallo, J. Antonius, L. Mimish, R. Sriram, S. Bianchi and C. G. Duran, A new expandable intracoronary tantalum (Strecker) stent: early experimental results and follow-up to twelve months, Am. Heart J., 1993, 125(2), 501–510 CrossRef CAS PubMed
.
- C. Suba, M. Lakatos-Varsányi, A. Mikó, L. Kovács, N. Velich, B. Kádár and G. Szabó, Study of the electrochemical behaviour of Ti osteosynthesis plates used in maxillofacial surgery, Mater. Sci. Eng., A, 2007, 447(1–2), 347–354 CrossRef
.
- D. Krupa, J. Baszkiewicz, B. Rajchel, A. Barcz, J. W. Sobczak and A. Biliński, Effect of sodium-ion implantation on the corrosion resistance and bioactivity of titanium, Vacuum, 2005, 78(2), 161–166 CrossRef CAS
.
- M. Pankuch, R. Bell and C. A. Melendres, Composition and structure of the anodic films on titanium in aqueous solutions, Electrochim. Acta, 1993, 38(18), 2777–2779 CrossRef CAS
.
- C. E. B. Marino and L. H. Mascaro, EIS characterization of a Ti-dental implant in artificial saliva media: dissolution process of the oxide barrier, J. Electroanal. Chem., 2004, 568, 115–120 CrossRef CAS
.
- G. L. Burke, The corrosion of metals in tissues; and an introduction to tantalum, Can. Med. Assoc. J., 1940, 43(2), 125 CAS
.
- O. H. Fulcher, Tantalum as a metallic implant to repair cranial defects: a preliminary report, J. Am. Med. Assoc., 1943, 121(12), 931–933 CrossRef
.
- P. Flanigan, V. R. Kshettry and E. C. Benzel, World War II, tantalum, and the evolution of modern cranioplasty technique, Neurosurg Focus, 2014, 36(4), E22 Search PubMed
.
- S. Arabnejad, R. Burnett Johnston, J. A. Pura, B. Singh, M. Tanzer and D. Pasini, High-strength porous biomaterials for bone replacement: A strategy to assess the interplay between cell morphology, mechanical properties, bone ingrowth and manufacturing constraints, Acta Biomater., 2016, 30, 345–356 CrossRef CAS PubMed
.
- F. S. L. Bobbert and A. A. Zadpoor, Effects of bone substitute architecture and surface properties on cell response, angiogenesis, and structure of new bone, J. Mater. Chem. B, 2017, 5(31), 6175–6192 RSC
.
- S. Van Bael, Y. C. Chai, S. Truscello, M. Moesen, G. Kerckhofs, H. Van Oosterwyck, J. P. Kruth and J. Schrooten, The effect of pore geometry on the in vitro biological behavior of human periosteum-derived cells seeded on selective laser-melted Ti6Al4 V bone scaffolds, Acta Biomater., 2012, 8(7), 2824–2834 CrossRef CAS PubMed
.
- J. Van der Stok, O. P. Van der Jagt, S. Amin Yavari, M. F. P. De Haas, J. H. Waarsing, H. Jahr, E. M. M. Van Lieshout, P. Patka, J. A. N. Verhaar, A. A. Zadpoor and H. Weinans, Selective laser melting-produced porous titanium scaffolds regenerate bone in critical size cortical bone defects, J. Orthop. Res., 2013, 31(5), 792–799 CrossRef CAS PubMed
.
- T. Miyazaki, H.-M. Kim, T. Kokubo, C. Ohtsuki, H. Kato and T. Nakamura, Mechanism of bonelike apatite formation on bioactive tantalum metal in a simulated body fluid, Biomaterials, 2002, 23(3), 827–832 CrossRef CAS PubMed
.
- Y. Zhang, P. B. Ahn, D. C. Fitzpatrick, A. D. Heiner, R. A. Poggie and T. D. Brown, Interfacial frictional behavior: cancellous bone, cortical bone, and a novel porous tantalum biomaterial, J. Musculoskeletal Res., 1999, 03(04), 245–251 CrossRef
.
- B. R. Levine, S. Sporer, R. A. Poggie, C. J. Della Valle and J. J. Jacobs, Experimental and clinical performance of porous tantalum in orthopedic surgery, Biomaterials, 2006, 27(27), 4671–4681 CrossRef CAS PubMed
.
- H. P. Tang, K. Yang, L. Jia, W. W. He, L. Yang and X. Z. Zhang, Tantalum Bone Implants Printed by Selective Electron Beam Manufacturing (SEBM) and Their Clinical Applications, JOM, 2020, 72(3), 1016–1021 CrossRef CAS
.
- G. Huang, S. T. Pan and J. X. Qiu, The Clinical Application of Porous Tantalum and Its New Development for Bone Tissue Engineering, Materials, 2021, 14(10), 2647 CrossRef CAS PubMed
.
- F. W. Geib, Vitallium skull plates, J. Am. Med. Assoc., 1941, 117(1), 8–12 CrossRef
.
- C. S. Venable, W. G. Stuck and A. Beach, The effects on bone of the presence of metaIs, based on eIectroIysis, Ann. Surg., 1937, 103, 917 CrossRef PubMed
.
- D. Meslemani and R. M. Kellman, Recent advances in fixation of the craniomaxillofacial skeleton, Curr. Opin. Otolaryngol. Head Neck Surg., 2012, 20(4), 304–309 CrossRef PubMed
.
- X. Wang, X. Shao, T. Dai, F. Xu, J. G. Zhou, G. Qu, L. Tian, B. Liu and Y. Liu, In vivo study of the efficacy, biosafety, and degradation of a zinc alloy osteosynthesis system, Acta Biomater., 2019, 92, 351–361 CrossRef CAS PubMed
.
- H. Guo, D. Xia, Y. Zheng, Y. Zhu, Y. Liu and Y. Zhou, A pure zinc membrane with degradability and osteogenesis promotion for guided bone regeneration: In vitro and in vivo studies, Acta Biomater., 2020, 106, 396–409 CrossRef CAS PubMed
.
- W. Zhang, P. Li, G. Shen, X. Mo, C. Zhou, D. Alexander, F. Rupp, J. Geis-Gerstorfer, H. Zhang and G. Wan, Appropriately adapted properties of hot-extruded Zn-0.5Cu-xFe alloys aimed for biodegradable guided bone regeneration membrane application, Bioact. Mater., 2021, 6(4), 975–989 CAS
.
- P. Li, W. Zhang, J. Dai, A. B. Xepapadeas, E. Schweizer, D. Alexander, L. Scheideler, C. Zhou, H. Zhang, G. Wan and J. Geis-Gerstorfer, Investigation of zinccopper alloys as potential materials for craniomaxillofacial osteosynthesis implants, Mater. Sci. Eng., C, 2019, 103, 109826 CrossRef CAS PubMed
.
- X. Qu, H. Yang, B. Jia, M. Wang, B. Yue, Y. Zheng and K. Dai, Zinc alloy-based bone internal fixation screw with antibacterial and anti-osteolytic properties, Bioact. Mater., 2021, 6(12), 4607–4624 CAS
.
- D. T. Chou, D. Wells, D. Hong, B. Lee, H. Kuhn and P. N. Kumta, Novel processing of iron-manganese alloy-based biomaterials by inkjet 3-D printing, Acta Biomater., 2013, 9(10), 8593–8603 CrossRef CAS PubMed
.
- D. Hong, D. T. Chou, O. I. Velikokhatnyi, A. Roy, B. Lee, I. Swink, I. Issaev, H. A. Kuhn and P. N. Kumta, Binder-jetting 3D printing and alloy development of new biodegradable Fe-Mn-Ca/Mg alloys, Acta Biomater., 2016, 45, 375–386 CrossRef CAS PubMed
.
- S. E. Henderson, K. Verdelis, S. Maiti, S. Pal, W. L. Chung, D. T. Chou, P. N. Kumta and A. J. Almarza, Magnesium alloys as a biomaterial for degradable craniofacial screws, Acta Biomater., 2014, 10(5), 2323–2332 CrossRef CAS PubMed
.
- B. Schaller, N. Saulacic, T. Imwinkelried, S. Beck, E. W. Liu, J. Gralla, K. Nakahara, W. Hofstetter and T. Iizuka, In vivo degradation of magnesium plate/screw osteosynthesis implant systems: Soft and hard tissue response in a calvarial model in miniature pigs, J. Craniomaxillofac. Surg., 2016, 44(3), 309–317 CrossRef PubMed
.
- S. H. Byun, H. K. Lim, K. H. Cheon, S. M. Lee, H. E. Kim and J. H. Lee, Biodegradable magnesium alloy (WE43) in bone-fixation plate and screw, J. Biomed. Mater. Res., Part B, 2020, 108(6), 2505–2512 CrossRef CAS PubMed
.
- A. Torroni, C. Xiang, L. Witek, E. D. Rodriguez, P. G. Coelho and N. Gupta, Biocompatibility and degradation properties of WE43 Mg alloys with and without heat treatment: In vivo evaluation and comparison in a cranial bone sheep model, J. Craniomaxillofac. Surg., 2017, 45(12), 2075–2083 CrossRef PubMed
.
- J. Kubásek, D. Dvorský, J. Šedý, Š. Msallamová, J. Levorová, R. Foltán and D. Vojtěch, The Fundamental Comparison of Zn-2Mg and Mg-4Y-3RE Alloys as a Perspective Biodegradable Materials, Materials, 2019, 12(22), 3745 CrossRef PubMed
.
- M. Zhao, G. Liu, Y. Li, X. Yu, S. Yuan, Z. Nie, J. Wang, J. Han, C. Tan, C. Guo and D. Behavior, Transport Mechanism and Osteogenic Activity of Mg–Zn–RE Alloy Membranes in Critical-Sized Rat Calvarial Defects, Coatings, 2020, 10(5), 496 CrossRef CAS
.
- P. Aisen, C. Enns and M. Wessling-Resnick, Chemistry and biology of eukaryotic iron metabolism, Int. J. Biochem. Cell Biol., 2001, 33(10), 940–959 CrossRef CAS PubMed
.
- B. J. Crielaard, T. Lammers and S. Rivella, Targeting iron metabolism in drug discovery and delivery, Nat. Rev. Drug Discovery, 2017, 16(6), 400–423 CrossRef CAS PubMed
.
- D. T. Reilly and A. H. Burstein, The Mechanical Properties of Cortical Bone, JBJS J., 1974, 56(5), 1001–1022 CrossRef CAS
.
- K. Roemhild, F. von Maltzahn, R. Weiskirchen, R. Knuchel, S. von Stillfried and T. Lammers, Iron metabolism: pathophysiology and pharmacology, Trends Pharmacol. Sci., 2021, 42(8), 640–656 CrossRef CAS PubMed
.
-
F.-J. Kretz and J. Schäffer, Anästhesie, Intensivmedizin, Notfallmedizin, Schmerztherapie, Springer-Verlag, 2008 Search PubMed
.
-
M. J. Müller, J. Westenhöfer, A. Bosy-Westphal, C. Löser and O. Selberg, Ernährungsmedizinische Untersuchungen, Ernährungsmedizinische Praxis, Springer, 2007, pp. 1–196 Search PubMed
.
- D. Fiorentini, C. Cappadone, G. Farruggia and C. Prata, Magnesium: Biochemistry, Nutrition, Detection, and Social Impact of Diseases Linked to Its Deficiency, Nutrients, 2021, 13(4), 1136 CrossRef CAS PubMed
.
- W. Qiao, K. H. M. Wong, J. Shen, W. Wang, J. Wu, J. Li, Z. Lin, Z. Chen, J. P. Matinlinna, Y. Zheng, S. Wu, X. Liu, K. P. Lai, Z. Chen, Y. W. Lam, K. M. C. Cheung and K. W. K. Yeung, TRPM7 kinase-mediated immunomodulation in macrophage plays a central role in magnesium ion-induced bone regeneration, Nat. Commun., 2021, 12(1), 2885 CrossRef CAS PubMed
.
- J.-Y. Lee, J.-W. Lee, K.-M. Pang, H.-E. Kim, S.-M. Kim and J.-H. Lee, Biomechanical Evaluation of Magnesium-Based Resorbable Metallic Screw System in a Bilateral Sagittal Split Ramus Osteotomy Model Using Three-Dimensional Finite Element Analysis, J. Oral Maxillofac. Surg., 2014, 72(2), 402 CrossRef PubMed
.
- J. H. Lee, H. S. Han, Y. C. Kim, J. Y. Lee and B. K. Lee, Stability of biodegradable metal (Mg-Ca-Zn alloy) screws compared with absorbable polymer and titanium screws for sagittal split ramus osteotomy of the mandible using the finite element analysis model, J. Craniomaxillofac. Surg., 2017, 45(10), 1639–1646 CrossRef PubMed
.
- H. Leonhardt, A. Franke, N. M. H. McLeod, G. Lauer and A. Nowak, Fixation of fractures of the condylar head of the mandible with a new magnesium-alloy biodegradable cannulated headless bone screw, Br. J. Oral Maxillofac. Surg., 2017, 55(6), 623–625 CrossRef CAS PubMed
.
- Y. Chen, S.-H. Ye, H. Sato, Y. Zhu, V. Shanov, T. Tiasha, A. D'Amore, S. Luketich, G. Wan and W. R. Wagner, Hybrid scaffolds of Mg alloy mesh reinforced polymer/extracellular matrix composite for critical-sized calvarial defect reconstruction, J. Tissue Eng. Regener. Med., 2018, 12(6), 1374–1388 CrossRef CAS PubMed
.
- Y. Guo, W. Liu, S. Ma, J. Wang, J. Zou, Z. Liu, J. Zhao and Y. Zhou, A preliminary study for novel use of two Mg alloys (WE43 and Mg3Gd), J. Mater. Sci.: Mater. Med., 2016, 27(5), 82 CrossRef PubMed
.
- Z. Zhen, T.-f. Xi and Y.-f. Zheng, A review on in vitro corrosion performance test of biodegradable metallic materials, Trans. Nonferrous Met. Soc. China, 2013, 23(8), 2283–2293 CrossRef CAS
.
- J. Zhang, H. Li, W. Wang, H. Huang, J. Pei, H. Qu, G. Yuan and Y. Li, The degradation and transport mechanism of a Mg-Nd-Zn-Zr stent in rabbit common carotid artery: A 20-month study, Acta Biomater., 2018, 69, 372–384 CrossRef CAS PubMed
.
- T. Kambe, T. Tsuji, A. Hashimoto and N. Itsumura, The Physiological, Biochemical, and Molecular Roles of Zinc Transporters in Zinc Homeostasis and Metabolism, Physiol. Rev., 2015, 95(3), 749–784 CrossRef CAS PubMed
.
- P. J. Little, R. Bhattacharya, A. E. Moreyra and I. L. Korichneva, Zinc and cardiovascular disease, Nutrition, 2010, 26(11), 1050–1057 CrossRef CAS PubMed
.
- M. Dermience, G. Lognay, F. Mathieu and P. Goyens, Effects of thirty elements on bone metabolism, J. Trace Elem. Med. Biol., 2015, 32, 86–106 CrossRef CAS PubMed
.
- H.-J. Seo, Y.-E. Cho, T. Kim, H.-I. Shin and I.-S. Kwun, Zinc may increase bone formation through stimulating cell proliferation, alkaline phosphatase activity and collagen synthesis in osteoblastic MC3T3-E1 cells, Nutr. Res. Pract., 2010, 4(5), 356–361 CrossRef CAS PubMed
.
- C. J. Frederickson, J.-Y. Koh and A. I. Bush, The neurobiology of zinc in health and disease, Nat. Rev. Neurosci., 2005, 6(6), 449–462 CrossRef CAS PubMed
.
- Y. Qiao, W. Zhang, P. Tian, F. Meng, H. Zhu, X. Jiang, X. Liu and P. K. Chu, Stimulation of bone growth following zinc incorporation into biomaterials, Biomaterials, 2014, 35(25), 6882–6897 CrossRef CAS PubMed
.
- Z. Xu, S. Kim and J. Huh, Zinc plays a critical role in the cardioprotective effect of postconditioning by enhancing the activation of the RISK pathway in rat hearts, J. Mol. Cell. Cardiol., 2014, 66, 12–17 CrossRef CAS PubMed
.
- D. Zhu, Y. Su, Y. Zheng, B. Fu, L. Tang and Y. X. Qin, Zinc regulates vascular endothelial cell activity through zinc-sensing receptor ZnR/GPR39, Am. J. Physiol.: Cell Physiol., 2018, 314(4), C404–C414 CrossRef CAS PubMed
.
- Y. Su, K. Wang, J. Gao, Y. Yang, Y.-X. Qin, Y. Zheng and D. Zhu, Enhanced cytocompatibility and antibacterial property of zinc phosphate coating on biodegradable zinc materials, Acta Biomater., 2019, 98, 174–185 CrossRef CAS PubMed
.
- Y. Su, H. Yang, J. Gao, Y.-X. Qin, Y. Zheng and D. Zhu, Interfacial Zinc Phosphate is the Key to Controlling Biocompatibility of Metallic Zinc Implants, Adv. Sci., 2019, 6(14), 1900112 CrossRef PubMed
.
- H. Guo, D. Xia, Y. Zheng, Y. Zhu, Y. Liu and Y. Zhou, A pure zinc membrane with degradability and osteogenesis promotion for guided bone regeneration: In vitro and in vivo studies, Acta Biomater., 2020, 106, 396–409 CrossRef CAS PubMed
.
- L. Heiden, More Predictive Cell-Based Assays, Genet. Eng. Biotechnol. News, 2015, 35(2), 1 Search PubMed
, 10, 12–13.
- J. Venezuela and M. S. Dargusch, The influence of alloying and fabrication techniques on the mechanical properties, biodegradability and biocompatibility of zinc: A comprehensive review, Acta Biomater., 2019, 87, 1–40 CrossRef CAS PubMed
.
- H. Yang, B. Jia, Z. Zhang, X. Qu, G. Li, W. Lin, D. Zhu, K. Dai and Y. Zheng, Alloying design of biodegradable zinc as promising bone implants for load-bearing applications, Nat. Commun., 2020, 11(1), 401 CrossRef PubMed
.
- W. Pachla, S. Przybysz, A. Jarzebska, M. Bieda, K. Sztwiertnia, M. Kulczyk and J. Skiba, Structural and mechanical aspects of hypoeutectic Zn-Mg binary alloys for biodegradable vascular stent applications, Bioact. Mater., 2021, 6(1), 26–44 CAS
.
- X. Wei, W. Zhou, Z. Tang, H. Wu, Y. Liu, H. Dong, N. Wang, H. Huang, S. Bao, L. Shi, X. Li, Y. Zheng and Z. Guo, Magnesium surface-activated 3D printed porous PEEK scaffolds for in vivo osseointegration by promoting angiogenesis and osteogenesis, Bioact. Mater., 2023, 20, 16–28 CAS
.
- G. I. Benic and C. H. F. Hämmerle, Horizontal bone augmentation by means of guided bone regeneration, Periodontology, 2014, 66(1), 13–40 CrossRef PubMed
.
- H. Saijo, U.-i. Chung, K. Igawa, Y. Mori, D. Chikazu, M. Iino and T. Takato, Clinical application of artificial bone in the maxillofacial region, J. Artif. Organs, 2008, 11(4), 171 CrossRef PubMed
.
- S. Aydin, B. Kucukyuruk, B. Abuzayed, S. Aydin and G. Z. Sanus, Cranioplasty: Review of materials and techniques, J. Neurosci. Rural Pract., 2011, 2(2), 162–167 CrossRef PubMed
.
- D. P. Blake, The Use of Synthetics in Cranioplasty: A Clinical Review, Mil. Med., 1994, 159(6), 466–469 CrossRef CAS PubMed
.
-
C. R. Arciola, D. Campoccia, G. D. Ehrlich and L. Montanaro, Biofilm-Based Implant Infections in Orthopaedics, in Biofilm-based Healthcare-associated Infections: Volume I, ed. G. Donelli, Springer International Publishing, Cham, 2015, pp. 29–46 Search PubMed
.
- C. R. Arciola, D. Campoccia and L. Montanaro, Implant infections: adhesion, biofilm formation and immune evasion, Nat. Rev. Microbiol., 2018, 16(7), 397–409 CrossRef CAS PubMed
.
- L. Montanaro, P. Speziale, D. Campoccia, S. Ravaioli, I. Cangini, G. Pietrocola, S. Giannini and C. R. Arciola, Scenery of Staphylococcus implant infections in orthopedics, Future Microbiol., 2011, 6(11), 1329–1349 CrossRef CAS PubMed
.
- S. Amin Yavari, M. Croes, B. Akhavan, F. Jahanmard, C. C. Eigenhuis, S. Dadbakhsh, H. C. Vogely, M. M. Bilek, A. C. Fluit, C. H. E. Boel, B. C. H. van der Wal, T. Vermonden, H. Weinans and A. A. Zadpoor, Layer by layer coating for bio-functionalization of additively manufactured meta-biomaterials, Addit. Manuf., 2020, 32, 100991 CAS
.
- H. J. Busscher, H. C. v. d. Mei, G. Subbiahdoss, P. C. Jutte, J. J. A. M. v. d. Dungen, S. A. J. Zaat, M. J. Schultz and D. W. Grainger, Biomaterial-Associated Infection: Locating the Finish Line in the Race for the Surface, Sci. Transl. Med., 2012, 4(153), 153rv10–153rv10 Search PubMed
.
- T. Wang, J. Bai, M. Lu, C. Huang, D. Geng, G. Chen, L. Wang, J. Qi, W. Cui and L. Deng, Engineering immunomodulatory and osteoinductive implant surfaces via mussel adhesion-mediated ion coordination and molecular clicking, Nat. Commun., 2022, 13(1), 160 CrossRef CAS PubMed
.
- M. Mack, Inflammation and fibrosis, Matrix Biol., 2018, 68–69, 106–121 CrossRef CAS PubMed
.
- J. W. Godwin, A. R. Pinto and N. A. Rosenthal, Macrophages are required for adult salamander limb regeneration, Proc. Natl. Acad. Sci. U. S. A., 2013, 110(23), 9415–9420 CrossRef CAS PubMed
.
- Y. Zhao, L. Bai, Y. Zhang, R. Yao, Y. Sun, R. Hang, X. Chen, H. Wang, X. Yao, Y. Xiao and R. Hang, Type I collagen decorated nanoporous network on titanium implant surface promotes osseointegration through mediating immunomodulation, angiogenesis, and osteogenesis, Biomaterials, 2022, 288, 121684 CrossRef CAS PubMed
.
- P. Huang, J. Xu, L. Xie, G. Gao, S. Chen, Z. Gong, X. Lao, Z. Shan, J. Shi, Z. Zhou, Z. Chen, Y. Cao, Y. Wang and Z. Chen, Improving hard metal implant and soft tissue integration by modulating the “inflammatory-fibrous complex” response, Bioact. Mater., 2023, 20, 42–52 CAS
.
- C. Yu, L. Chen, W. Zhou, L. Hu, X. Xie, Z. Lin, A. C. Panayi, X. Zhan, R. Tao, B. Mi and G. Liu, Injectable Bacteria-Sensitive Hydrogel Promotes Repair of Infected Fractures via Sustained Release of miRNA Antagonist, ACS Appl. Mater. Interfaces, 2022, 14, 34427–34442 CrossRef CAS PubMed
.
- B. J. Igo, P. S. Cottler, J. S. Black and M. B. Panzer, The mechanical and microstructural properties of the pediatric skull, J. Mech. Behav. Biomed. Mater., 2021, 120, 104578 CrossRef PubMed
.
- B. C. a. S. S. Margulies, Material Properties of Human Infant Skull and Suture at High Rates, J. Neurotrauma, 2006, 23(8), 1222–1232 CrossRef PubMed
.
- M. T. Davis, A. M. Loyd, H. Y. Shen, M. H. Mulroy, R. W. Nightingale, B. S. Myers and C. D. Bass, The mechanical and morphological properties of 6 years-old cranial bone, J. Biomech., 2012, 45(15), 2493–2498 CrossRef PubMed
.
- J. H. C. Lee, B. Ondruschka, L. Falland-Cheung, M. Scholze, N. Hammer, D. C. Tong and J.
N. Waddell, An Investigation on the Correlation between the Mechanical Properties of Human Skull Bone, Its Geometry, Microarchitectural Properties, and Water Content, J. Healthcare Eng., 2019, 2019, 6515797 Search PubMed
.
- S. Kanala, S. Gudipalli, P. Perumalla, K. Jagalanki, P. V. Polamarasetty, S. Guntaka, A. Gudala and R. P. Boyapati, Aetiology, prevalence, fracture site and management of maxillofacial trauma, Ann. R. Coll. Surg. Engl., 2021, 103(1), 18–22 CrossRef CAS PubMed
.
- S. Bagherifard, D. J. Hickey, S. Fintová, F. Pastorek, I. Fernandez-Pariente, M. Bandini, T. J. Webster and M. Guagliano, Effects of nanofeatures induced by severe shot peening (SSP) on mechanical, corrosion and cytocompatibility properties of magnesium alloy AZ31, Acta Biomater., 2018, 66, 93–108 CrossRef CAS PubMed
.
- M. Jamalian and D. P. Field, Effects of shot peening parameters on gradient microstructure and mechanical properties of TRC AZ31, Mater. Charact., 2019, 148, 9–16 CrossRef CAS
.
|
This journal is © The Royal Society of Chemistry 2024 |
Click here to see how this site uses Cookies. View our privacy policy here.