DOI:
10.1039/D4CC02837F
(Feature Article)
Chem. Commun., 2024,
60, 9340-9351
Design of hollow structured nanoreactors for liquid-phase hydrogenations
Received
12th June 2024
, Accepted 29th July 2024
First published on 30th July 2024
Abstract
Inspired by the attractive structures and functions of natural matter (such as cells, organelles and enzymes), chemists are constantly exploring innovative material platforms to mimic natural catalytic systems, particularly liquid-phase hydrogenations, which are of great significance for chemical upgrading and synthesis. Hollow structured nanoreactors (HSNRs), featuring unique nanoarchitectures and advantageous properties, offer new opportunities for achieving excellent catalytic activity, selectivity, stability and sustainability. Notwithstanding the great progress made in HSNRs, there still remain the challenges of precise synthetic chemistry, and mesoscale catalytic kinetic investigation, and smart catalysis. To this extent, we provide an overview of recent developments in the synthetic chemistry of HSNRs, the unique characteristics of these materials and catalytic mechanisms in HSNRs. Finally, a brief outlook, challenges and further opportunities for their synthetic methodologies and catalytic application are discussed. This review might promote the creation of further HSNRs, realize the sustainable production of fine chemicals and pharmaceuticals, and contribute to the development of materials science.
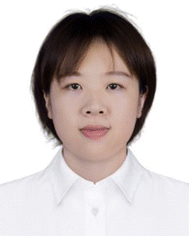
Yutong Pi
| Yutong Pi received her PhD degree in physical chemistry from the Dalian Institute of Chemical Physics, Chinese Academy of Sciences, in 2023. Now she is a researcher at the College of Chemistry and Chemical Engineering, Inner Mongolia University. Her research interests focus on the design and synthesis of nanoreactors for heterogeneous catalysis. |
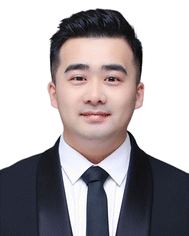
Haitao Li
| Haitao Li received his PhD degree in materials science and engineering from China University of Geosciences (Wuhan, China) in 2021, and then became a postdoctoral fellow in the Micro–Nano Reactor & Reaction Engineering Science Group led by Prof. Jian Liu in the Dalian Institute of Chemical Physics, Chinese Academy of Sciences, China. Subsequently, he joined the College of Chemistry and Chemical Engineering of Inner Mongolia University. His research interests primarily focus on the rational design of functional transition-metal-/carbon-based nanoreactors for application of heterogeneous catalysis including artificial production of H2O2 and advanced oxidation processes. |
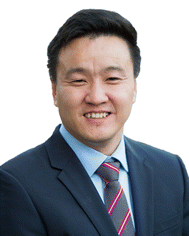
Jian Liu
| Jian Liu received his PhD degree in physical chemistry from the Dalian Institute of Chemical Physics, Chinese Academy of Sciences, China, in 2008. Now he is a chaired professor and head of the College of Chemistry and Chemical Engineering, Inner Mongolia University, China. He also held an adjunct Reader position in the Department of Chemical and Process Engineering, University of Surrey, UK. His research focuses on the design of micro–nanoreactors, porous carbon spheres, and the industrialization of homogeneous catalysis in heterogeneous systems. His h-index is 71 (Web of Science). |
1. Introduction
Hollow nanoreactors (HSNRs) are small reaction vessels designed and synthesized artificially, which can allow chemical reactions to occur within their nanospace, and be distinguished from other materials by their unique structures and customizable functions.1,2 HSNRs can either alter the basic chemical properties of molecules or change their behavior in chemical transformations, thus accelerating the reaction, acquiring new understanding of chemical systems, and accordingly being the subject of versatile research areas, such as catalysis, biomedicine, and energy storage and conversion.3–7
Nowadays, HSNRs have emerged as exceptional alternatives to traditional catalytic materials, especially in liquid-phase hydrogenation reactions, because the isolated space offers nanoscale environments partitioned from the surrounding bulk space.8,9 The morphology and chemical contents of this nanospace dictate the reaction chemical/spatial microenvironment, reactant enrichment, compartmentalization of active sites, and diffusion processes, improving its activity, long-term stability and regulating product selectivity, making HSNRs ideal catalysts. To control and boost their catalytic performance, and even generate distinct and intriguing properties, the morphological and compositional control of HSNRs is particularly interesting. Several recent reviews have discussed advances in synthetic strategies for HSNRs.10–12 Wan and coworkers outlined new synthetic strategies manipulating particle chemistry for the creation of hollow carbon-based nanospheres.11 The fabrication of other hollow nanostructured materials, such as hollow metal–organic frameworks (MOFs) and covalent organic frameworks (COFs), has also been a focus.13–15 Most reviews and perspectives focus mainly on the construction of specific materials, and a systematic summary of the engineering of HSNRs with various materials is still lacking. Additionally, when considering the application of research, a number of comprehensive reviews have made thorough summaries. For example, an updated and critical investigation of hollow nanostructures in all catalytic branches (including, bio-, electro, and photocatalysis) was presented by Schüth et al., and promising applications for sensing, separation and storage were reported by Zou and coworkers.5 Despite many general and potential applications of HSNRs being summarized in previous literature, there have been limited reviews covering HSNRs for liquid-phase hydrogenation with an emphasis on structure–performance correlation.
In this review, we systematically summarize the controllable and precise synthetic chemistry of HSNRs, discussing in detail their unique properties and how these properties affect liquid-phase hydrogenation performance. Finally, we further put forward a brief outlook for the future developments and challenges of these synthetic principles and applications of HSNRs. These are systematically reviewed and in-depth understandings are expected to open up new avenues for the rational design of advanced HSNRs, and inspire further development for broad application fields.
2. Synthetic strategies for hollow structured nanoreactors
To date, tremendous research work has been devoted to constructing various hollow nanostructures in pursuit of their appealing physical–chemical properties. Even so, the development of preparation processes can be categorized according to two general paradigms: bottom-up and top-down (Fig. 1). Similar to assembling building blocks, the bottom-up strategy starts from each small part, finally assembling them into an ideal nanostructure. By contrast, the process of a top-down strategy is to extract unnecessary parts from a built monolith, leaving the desired hollow nanostructure.
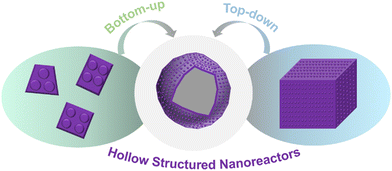 |
| Fig. 1 Schematic illustration of the two main strategies for the synthesis of HSNRs. | |
2.1. Bottom-up strategy
Very recently, bottom-up strategies, typically soft-template directed protocols have been widely used to fabricate various hollow materials with unique structures and pore configurations as well as fascinating physical–chemical properties.16,17 Synthetic methods can be categorized into micelle-directed assembly, emulsion-guided assembly, and interfacial assembly methods.
The micelle-directed assembly method relies on the ordered arrangement and assembly of amphiphilic surfactants and block copolymers: for example, cationic cetyltrimethyl ammonium bromide (CTAB), Pluronic F127 (EO106PO70EO106) and poly(styreneblock4vinylpyridine) (PSbP4VP).18 After assembly with precursors, like polymer oligomers, metal ions or silanes, micelle–oligomer composites can be formed.19 Finally, these composites proceed through crosslinking to synthesize desirable hollow structures. The detailed morphology and mesostructure of hollow materials are highly dependent on the structure of the micelle, the type of surfactants and other reaction parameters. Zhao et al. reported a lamellar micelle assembled from Pluronic P123 block copolymer and dopamine (Fig. 2a).20 After continuous growth and self-assembly, hollow multi-shelled nanospheres can be created. Meanwhile, by changing P123 to F108, F127, or P105, nanospheres with a single cavity, ordered mesochannels, and dendritic mesopores can be formed. The micelle-directed assembly method enables the library of hollow materials to be extended, such as hollow CaP,21 WO3,22 and Au23 nanospheres.
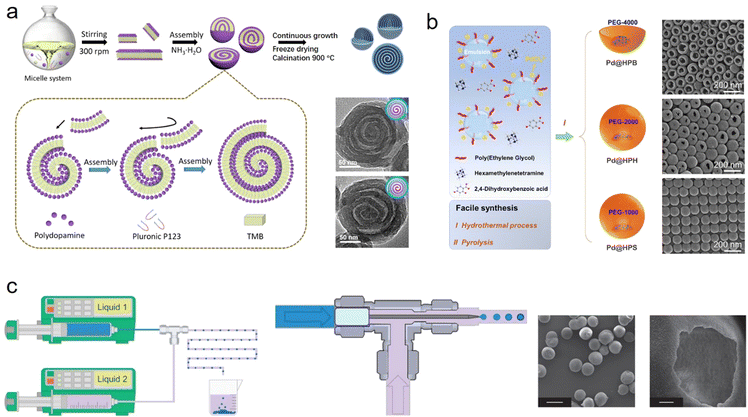 |
| Fig. 2 Schematic illustration of various bottom-up methods and transmission electron microscopy images of corresponding hollow nanomaterials: (a) micelle-directed assembly,20 Copyright 2021, Science; (b) emulsion-guided assembly,24 Copyright 2023, American Chemical Society; and (c) interfacial assembly methods,25 Copyright 2011, Nature Publishing Group. | |
Another typical and facile synthetic method is emulsion-guided assembly, which is one of the strategies with the most potential for synthesizing nanomaterials with various nanostructures, especially hollow nanoarchitectures.26,27 An emulsion involves the mixing of two immiscible liquids, generally water and oil, in the presence of surfactants.13 An oil/water or water/oil emulsion system provides the template for tailoring the multiple architectures of the materials.5,28 Recently, Ma et al. reported a nanodroplet remodeling method in a water/oil emulsion system and synthesized multi-chambered (from single to tri-chambered) silica nanoparticles.29 In this method, tetrahydrofuran (THF), a new solvent, was introduced to trigger the expansion of water nanodroplets in n-pentanol, causing the formation of multi-chambered silica nanoparticles with the continuous hydrolysis of silicate precursor adsorbed on the nanodroplet surface. Wang and coworkers adopted a similar emulsion-guided strategy and synthesized various hollow carbon nanoparticles, where P123/sodium oleate served as an emulsion precursor, polyethylene glycol as a reverse demulsifier, and 2,4-dihydroxybenzoic acid/hexamethylenetetramine as a polymer precursor (Fig. 2b).24 This strategy is applicable to building other types of hollow material with multilevel structures, shapes, and functions.
Interfacial assembly, micelles and framework precursors self-assembled on the two-phase interface, allow the controllable synthesis of unique hollow architectures, greatly enriching those structures and functionality. Usually, three types of interface, liquid–liquid, solid–liquid and liquid–gas interfaces, are utilized to manipulate the assembly. In liquid–liquid interfacial assembly, two immiscible liquids provide the interface as the reaction space and strongly confine the construction of various hollow nanomaterials. As a typical example, De Vos's group developed a microfluidic environment that enables the formation of a spherical liquid–liquid interface and a spherical MOF shell (Fig. 2c).25 Through a capillary, the generated monodispersed droplets containing aqueous cupric solution can react with the ligand molecules at the interface, and then form a Cu3(BTC)2 hollow capsule. As another useful interface, a solid–liquid interface can effectively build hollow nanomaterials. Yu et al. reported a novel anisotropic hollow carbon material with this solid–liquid interface assembly method.30 The approach allows regioselective surface assembly of a carbon precursor, dopamine, on the different positions of slicalite-1. Upon carbonization and removal of these hard substrates, carbon with anisotropic hollows was successfully achieved. Zhao et al. proposed a monomicelle interface-confined assembly approach to synthesize a 3D hierarchical mesoporous superstructure, with colloidal silica nanospheres as the hard substrate, PS–PVP–PEO as monomicelles and dopamine as the carbon precursor.31 Furthermore, a gas–liquid interface also shows huge potential for the fabrication of hollow nanomaterials. For instance, Zhang et al. added CO2 bubbles into continuous ionic liquid, allowing the assembly of organic linkers and metal ions on the solid–liquid interface, resulting in the generation of hollow Zn-BTC.32
On the basis of the above-mentioned synthetic methods, numerous hollow materials with diverse morphologies, shapes, compositions and functionality have been prepared. But, in pursuit of enhanced properties and functionality, and meeting the requirements for broad applications, the design and synthesis of hollow nanostructures are still of great significance.
2.2. Top-down strategy
The top-down strategy underscores the control over particles themselves with a labile chemical structure and the removal process: (1) by delicately regulating reaction conditions, solid nanomaterials with unstable inner parts and stable outer parts are constructed, in which the unstable parts are easier to remove; (2) removing the labile interior with the assistance of extra solvent (such as acetone, ethanol, H2O, or metal salt solution), and transforming these solid nanoparticles into hollow nanostructured materials. Generally, the labile chemical structure can be constructed by building inner constitutional inhomogeneities, surface protecting methods, and building core–shell materials.
To manipulate particle chemistry, Chen and coworkers first revisited the Stöber method of silica synthesis and demonstrated the inhomogeneous nature of the silica shell.33 Due to the presence of a more robust outer layer, the weakened inner layer is easier to etch with hot water, forming hollow nanostructures. Recently, the focus has been on the synthesis of hollow nanostructured phenolic resins by controlling their compositional chemistry.11 Because of the tailorable growth kinetics of the polymerization process, different degrees of polymerization or the distribution of different polymeric components within a single phenolic particle can be prepared. Wan's group demonstrated that through tuning the time and temperature of the polymerization process, synthesized 3-aminophenol formaldehyde (APF) resin nanospheres have different location-specific molecular weights, those of the exterior part being higher than those of the interior part (Fig. 3a).34 Therefore, after acetone treatment, the core of these nanospheres could be selectively dissolved, forming hollow particles. Very recently, we reported another method to control growth kinetics by molecular-level design to build phenolic resin nanospheres with periodic variation in polymerization.35 By simply changing the mole ratio of different phenol precursors, 3-aminophenol (3-AP) and resorcinol (R), solid phenolic resins with a wave-like degree of polymerization can be successfully obtained, enabling the construction of a hollow multishelled structure in combination with a subsequent ethanol scissoring process. In addition to managing the degree of polymerization, the following removal process is equally important in expanding the diversity and complexity of phenolic resins. In our previous work, a nanoscale chemical scissoring strategy was developed, which relies on the utilization of ethanol as a chemical scalpel to tailor the nanostructure and chemical composition of APF.36 Due to this facile and reliable strategy, multilevel hollow structured APF nanoparticles with accurate positioning of organic functional groups were successfully prepared. Similarly, Yu et al. successfully constructed attractive concave hollow APF spheres via an acetone-guided deflation–inflation asymmetric growth process.37 The synthetic concept of constructing a single particle with inhomogeneous chemistry for hollow nanostructures is suitable for other material systems. So far, increasing attention and efforts have been paid to the nanoengineering of hollow MOFs.38,39 Usually, due to incomplete coordination between metal nodes and grain boundaries, more defects are present in the inner region of MOFs, resulting in their inhomogeneity. The presence of defects allows the preferential etching of core parts and the formation of hollow MOFs. In addition, incorporating an extra agent during synthesis makes the internal core with weak coordination less stable than the external surface, which helps to build hollow MOFs. Furthermore, by means of control over the degree of crystallization and chemical reactivity, hollow organic–inorganic composites (such as hollow TiO2–C, ZrO2–C, SnO2–C, and CeO2–C), metal oxides and COFs can also be successfully achieved.11,40–42
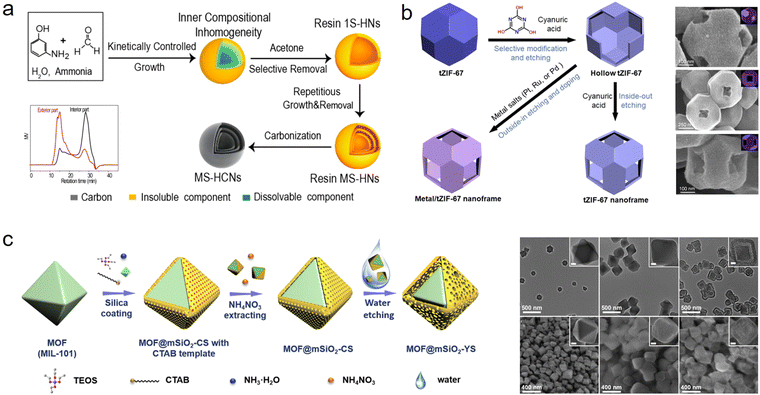 |
| Fig. 3 Schematic illustration of the formation of hollow nanomaterials based on various top-down methods and corresponding transmission electron microscopy: (a) building inner constitutional inhomogeneity,34 Copyright 2017, American Chemical Society; (b) surface protecting method,43 Copyright 2021, Wiley-VCH; and (c) building of core–shell materials,44 Copyright 2020, Elsevier. | |
In addition to controlling the inhomogeneous nature of nanoparticles, the surface protecting method is commonly used to prepare hollow nanomaterials. By utilizing stabilizing agents, generally polymers, to shield the coordination bonds of the surface, a more stable and anticorrosive surface can be created, which could be left after treatment with extra etchants. In early research, Yin and coworkers proposed a “surface-protected etching” strategy to prepare hollow silica spheres, in which poly(vinyl pyrrolidone) (PVP) was used as a protecting agent because of the formation of strong hydrogen bonds with a silica surface.45 Therefore, after treatment with NaOH, it can selectively etch the internal core of silica and form hollow nanostructures. Apart from PVP, other ligands including polyacrylic acid,46 polyethylene glycol,47 and polyethyleneimine,33 have also been chosen to strengthen the surface of specific materials. Furthermore, the success of this strategy has encouraged researchers to explore the possibility of applying it to other material systems. For instance, Yamauchi et al. demonstrated that cyanuric acid can anisotropically modify and protect the exposed crystal facets (111) of ZIF-67 from etching, thus constructing hollow nanoframes (Fig. 3b).43 Hollow TiO2, CeO2, and ZnO can also be obtained by applying this synthetic concept.48
Currently, building core–shell structured solid precursors with differentiable stability between the inner part and the outer part for creating hollow nanomaterials has been extensively investigated, since it is easy to manipulate the selective removal process. Tsung et al. first fabricated core–shell MOFs (UiO-66-(OH)2@UiO-66) with controllable oxidizing capacity, and then introduced 2,5-dihydroxyterephthalic acid (DOBDC) as a sacrificial linker to selectively engrave these MOFs.49 Finally, depending on the degradation of DOBDC into small molecules to guide the controllable collapse of the inner core, a series of hollow and yolk–shell MOFs were produced. By applying this facile top-down strategy, various hollow structured MOFs have been successfully prepared, such as hollow ZIF-67 nanocages, hollow Zn-BTC, hollow MIL-88A, and yolk–shell ZIF-67@ZIF-8.50–52 Apart from MOFs, Yu and coworkers chose core–shell MOF@SiO2 with the structure of a water-stable MOF core and a water-unstable SiO2 shell to synthesize yolk–shell MOF@mesoporous SiO2 nanoparticles through a green water-etching approach (Fig. 3c).44 This method provides an idea for precisely changing the unstable core–shell to simple hollow structures, and even complex hollow structures such as muti-shell bowl-like or multi-cavity.
In order to design more attractive and functional hollow MOFs, the family of post-treatment agents is gradually increasing and diversifying, from liquid- to gas-phase agents (including tannic acid, gallic acid, amino acids, NaOH, ethanol, acetone, NH3, and CO2). Noticeably, the kind and dosage of the above agents should be rationally considered to avoid the destruction of the whole structure. In brief, based on these different synthetic strategies, numerous hollow nanostructured materials with fascinating morphologies and compositions have been successfully constructed. A comparative list summarizing the key synthetic methods and the advantages of the representative HSNRs is given in Table 1, which could provide guidance for rationally designing desired nanoreactors.
Table 1 Summary of various synthesis methods of HSNRs
Strategies |
Synthetic methods |
Compositions |
Advantages |
|
Micelle-directed assembly |
PDAs, COFs, phenolic resins, MOFs, CaP, WO3, Au, etc. |
High complexity of designable structures |
Emulsion-guided assembly |
PDAs, PS, COFs, phenolic resins, MOFs (ZIF-8, UiO-66), TiO2, SiO2, etc. |
Controllable morphology, internal and external composition |
Interfacial assembly methods |
PDAs, PS, COFs, phenolic resins, composites (TiO2-PDA, SiO2-PDA), etc. |
High operability and structural diversity |
|
Building inner constitutional inhomogeneity |
Phenolic resins, MOFs, SiO2, COFs organic–inorganic composites, etc. |
Precisely manipulating the nanostructure and composition |
Surface protecting method |
SiO2, ZIF-67, metal oxides (TiO2, CeO2, ZnO), etc. |
Convenient operation, high repeatability |
Architecting of core shell materials |
MOF@mesoporous SiO2, MOF-1@MOF-2, etc. |
High expansibility, and well-defined nanostructures |
3. Unique properties of directed liquid-phase hydrogenation
Liquid-phase hydrogenation, one of the most challenging catalytic reactions, plays a significant role in petroleum refining, and the production of both bulk and fine chemicals.53 Although great enhancements have been made in designing catalysts and regulating catalytic performance, the requirements for catalysts still remain highly stringent, such as accurately controlling specific reaction steps to achieve the target product, preventing the leaching and sintering of active metal nanoparticles to improve long-term stability, and improving mechanical strength.54,55 The successful construction of HSNRs endows them with particular properties and greatly enhances the catalytic performance thereafter.56 In this section, we attempt to illustrate in detail the unique properties of HSNRs and how they affect the liquid–phase hydrogenation performance (Fig. 4).
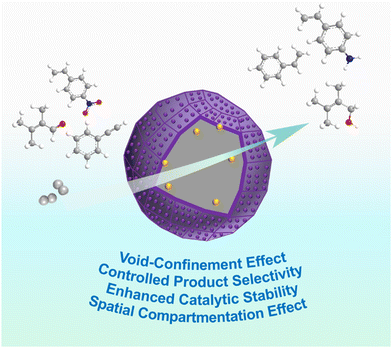 |
| Fig. 4 Schematic illustration of the properties of HSNRs applied in liquid-phase hydrogenation reactions. | |
3.1. Void-confinement effect
One of the main characteristics of HSNRs is the presence of nanospaces, which allow the storage and catalytic reaction of reactants within them. It is exactly because of the existence of nanosize vessels that the unique cavity confinement effect appears, which can be specifically divided into a reactant enrichment effect and controllable molecular activation processes. Recently, Li et al. studied in detail the reactant enrichment behavior in a hollow nanoreactor, Pt NPs@MnOx (Fig. 5a–d).57 Compared to crushed nanomaterials (Pt NPs&MnOx) without hollow cavities, the as-obtained Pt NPs@MnOx showed better catalytic performance in the selective hydrogenation of α,β-unsaturated aldehydes, highlighting the structural superiority of hollow nanospaces in boosting hydrogenation performance. Besides, by conducting adsorption experiments, the authors demonstrated that the increased amount of reactant adsorbed within the confined space derived from its directional diffusion via a local concentration gradient. In addition, the enhanced reaction rate occurring in HSNRs can be illustrated by the rate equation. Obviously, in comparison with chemical reactions proceeding in bulk solution, the boosted reaction rate of HSNRs is attributed to the increased reactant concentration: (NR⊃A·B) > ([A][B]), which indicates that these reactants are well preorganized and enriched in HSNRs.8,58,59
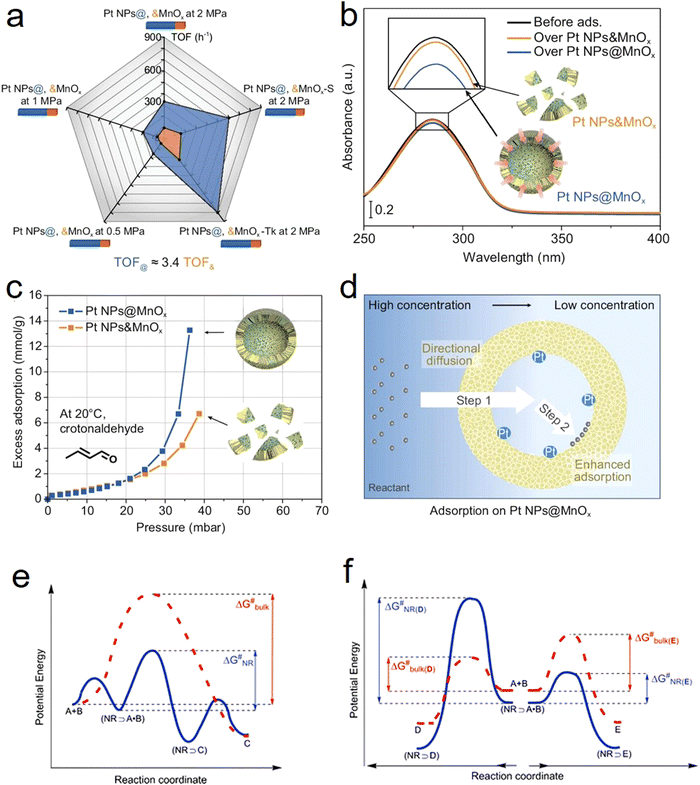 |
| Fig. 5 Reactant enrichment effect of HSNRs: (a) comparison of catalytic hydrogenation activity between a series of Pt NPs@MnOx HSNRs with crushed samples; (b) UV-vis spectrum of cinnamaldehyde adsorption experiment; (c) in situ gravimetric adsorption analysis profiles; (d) schematic illustration of reactant enrichment effect on a Pt NPs@MnOx nanoreactor.57 Copyright 2023. The controlled molecular activation processes: Comparison of reaction energy profiles over HSNRs and bulk phase in (e) A and B as reactants to produce C; and (f) A and B as reactants to produce D and E.59 Copyright 2008, Royal Society of Chemistry. | |
Reaction rate equation in bulk solution:
Reaction rate equation in a hollow nanoreactor:
Aside from the reactant enrichment effect, the hollow nanostructure can also affect molecular activation processes, providing a relatively low Gibbs free energy (ΔG#) (Fig. 5e and f).59 For parallel reactions, by adjusting the interaction between HSNRs and specific molecules, HSNRs allow regulation of the ΔG# of different products, thus adjusting the catalytic selectivity. The void-confinement effect on boosting catalytic behavior can also be observed in Ru@HCS hollow spheres for the hydrogenation of levulinic acid,60 PdCu@HCS for alkene and alkyne hydrogenation,61 a Pt@CeO2 nanoreactor for selective cinnamaldehyde hydrogenation,62 and so forth. Furthermore, the void-confinement effect is highly dependent on the nanostructures of HSNRs, such as shell curvature, particle size, shell composition, and interior structure.
3.2. Controlled product selectivity
Owning to the adjustable pore size/length, composition, and spatial position of their active sites, HSNRs are ideal catalysts to achieve the goal of regulating catalytic selectivity.63,64 For example, the channels or porous shell of HSNRs can strongly determine mass transfer behavior by the steric hindrance and diffusion rate, thus endowing them with a molecular sieving effect. Fang et al. prepared various hollow COFs, and encapsulated Pd nanoparticles within their hollow cavities, forming efficient size-selective catalysis in nitroarene hydrogenation (Fig. 6a and b).65 Because of its narrow pore diameter (1 nm), Pd@AB-HCONs showed better activity in the hydrogenation of nitrobenzene with small molecule size (0.46 × 0.66 nm) than that of 2,4,6-triphenylnitrobenzene with a large molecule size substrate (1.01 × 1.16 nm). Otherwise, Zheng and coworkers demonstrated that reactants are very sensitive to the shell thickness of HSNRs in p-nitrophenol hydrogenation, where the reaction rates decreased dramatically from 0.21 to 0.08 min−1 when the size of the carbon shell increased from 3 to 13 nm.66 Besides size selectivity, HSNRs can also achieve chemoselectivity. Jiang et al. implanted PdAg nanocages into hollow ZIF-8, forming a PdAg@ZIF yolk–shell nanoreactor.67 The ZIF-8 outer shell not only allows efficient mass transfer and sufficient exposure of active metal, but also enhances substrate enrichment. As a result, owing to the nanostructure and plasmonic metal nanoparticles, the as-made nanoreactor displayed excellent chemoselectivity (97.5%) in the hydrogenation of nitrostyrene to aminostyrene (Fig. 6c and d). In addition to the nanostructure, the precise position of active sites is equally important for controlling the reaction pathway and even cascade reactions.68,69 Xu et al. implanted Pt nanoparticles into MFI zeolites and demonstrated their efficient tandem aldol condensation and hydrogenation reaction of furfural and acetone with a high combined yield of 87% in C8 products.70 It should be noted that it is important to maintain both the excellent catalytic activity and mechanical strength of HSNRs, because the ultra-thin shell in HSNRs is easily broken, leading to the leaching of metal nanoparticles. The outer shell of HSNRs is vital for sieving substrates and molecules, enabling highly size- and shape-selective catalysis.
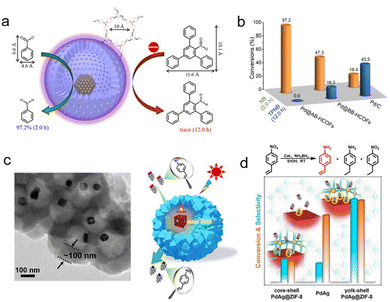 |
| Fig. 6 (a) Schematic illustration for the size-selectivity of a Pd@AB-HCOFs nanoreactor in nitroarene hydrogenation; (b) catalytic activity comparisons of various catalysts.65 Copyright 2022, American Chemical Society. (c) TEM image and structural model of a core–shell PdAg@ZIF-8 nanoreactor; (d) chemoselective hydrogenation of nitrostyrene with different catalysts.67 Copyright 2022, American Chemical Society. | |
3.3. Enhanced catalytic stability
Persistent catalytic stability for catalysts, especially for metal-loaded catalysts, is a key scientific issue and necessary prerequisite in research into and practice of nano-catalysts. Because metal nanoparticles are easily aggerated at harsh temperatures and leached under recycling processes, a rapid decline is caused in catalytic activity.71 The unique hollow architecture is ideal for confining metal nanoparticles and preventing them from pernicious aggregation and leaching, thus maintaining a long catalyst lifetime. Tsung et al. confined solitary Pd nanoparticles within a MOF with unique architecture, a multi-yolk–shell UiO-66-(OH)2, and investigated its stability for the catalytic conversion of allylbenzene (Fig. 7a and b).49 No obvious nanoparticle agglomeration was observed and marked hydrogenation reactivity was maintained after high-temperature treatment. Apart from the multi-chamber structure, enhanced catalytic stability can also be observed in multi-shelled HSNRs. Liu et al. encapsulated ultrafine Pd nanoparticles between the narrow spaces of inner shell reduced graphene oxide and an outer shell carbon layer (Fig. 7c and d).72 The unique nanostructure of the as-obtained RGO@Pd@C can effectively prevent the aggregation and leaching of metal nanoparticles, and maintain excellent 4-nitrophenol hydrogenation performance after 10 cycles. Another example of catalytic stability controlled by HSNRs was demonstrated for a Pt@hollow carbon shell (Pt@hmC) system. Compared to pure Pt nanoparticles and commercial Pt/C, Pt@hmC showed remarkable reusability in nitrobenzene hydrogenation.
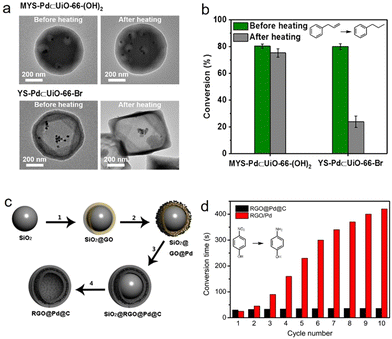 |
| Fig. 7 (a) TEM images of HSNRs with different nanostructures before and after heating; (b) catalytic conversion of allylbenzene on HSNRs.49 Copyright 2019, American Chemical Society. (c) Schematics of the synthesis of RGO@Pd@C hollow spheres; (d) the stability testing of these nanocatalysts.72 Copyright 2014, Nature Publishing Group. | |
3.4. Spatial compartmentation effect
Spatially positioning single or multiple active components into one support is the cutting edge of designing supported catalysts, which can promote the rapid conversion of catalytic reactions, and realize selective catalysis and synergistic catalysis between different active sites.73–76 As the nanostructure parameters of HSNRs can be ingeniously controlled, it is possible to precisely locate metal nanoparticles in these nanostructures. Recently, we designed and synthesized a hollow multi-shelled carbon carrier, which provides an ideal platform to precisely locate Pd nanoparticles, internally encapsulated (Pd@HoMS-C) or externally loaded (Pd/HoMS-C) (Fig. 8a).35 Combining unique nanostructures and spatially compartmented active sites, these HSNRs showed size- and shape-selective catalytic hydrogenation performance; that is, Pd@HoMS-C tends to catalyze small aliphatic substrates while Pd/HoMS-C shows excellent catalytic performance toward large aromatic substrates (Fig. 8b). Theoretical calculations demonstrated that the differential catalytic behaviors derive from the different adsorption energy barriers of the substrates (Fig. 8c). In localizing multiple metal nanoparticles, Zhao et al. spatially isolated Au and Pd nanoparticles at the bottom and upper chambers of a dual-chambered silica nanoreactor (Fig. 8d).29 Due to the efficient transformation of reactants and intermediates, this nanoreactor can effectively convert 1-nitro-2-(phenylethynyl)benzene to 2-phenylindole with a selectivity of up to 76.5% (Fig. 8e). Similarly, a multi-compartmentalized organosilica with co-localization of Pd and Ru nanoparticles was reported by Yang and coworkers.68 Such a unique catalyst exhibited excellent sequential hydrogenation behavior in converting nitroarenes into cyclohexylamines. In addition to active nanoparticles, HSNRs can also realize the spatial compartmentation of nonmetallic active sites for efficient catalytic conversion. For instance, Ma et al. spatially separated QDNH2/–SO3H (or QNNH2/–SO3H) and ProTMS/–CO2H in the inner and outer shells of hollow nanospheres for catalyzing an asymmetric organocascade.77
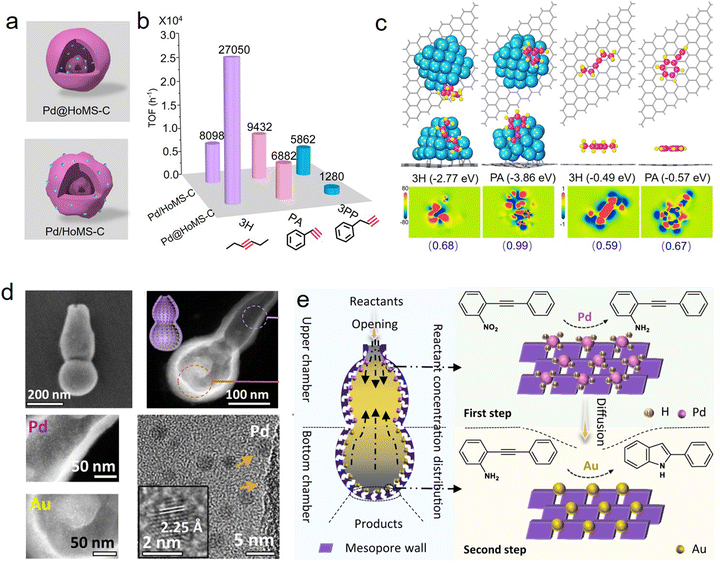 |
| Fig. 8 (a) Structural models for multi-shelled HSNRs with different Pd locations; (b) the corresponding TOF values of these nanoreactors in hydrogenation reactions; (c) theoretical calculations of the adsorption energies of different substrates on carbon carriers.35 Copyright 2023, Wiley-VCH. (d) Structural characterization of dual-chambered silica nanoreactor with separated position of Au and Pd nanoparticles; and (e) concentration distribution of reactants as well as cascade reactions.29 Copyright 2022, Nature Publishing Group. | |
4. Conclusions and perspectives
As a class of cell-mimicking materials that is attracting great attention, HSNRs are promising candidates with enhanced efficiency for numerous scientific disciplines. The advantageous nanostructures endow them with many unique properties, such as faster mass transport, highly dispersed metal nanoparticles, adjustable geometric and electronic characteristics, and more exposed active sites. So far, great progress has been made in the preparation of novel HSNRs with distinct compositions/structures, as well as their advanced applications. However, the precise engineering of HSNRs with required functionality and properties is still at a relatively basic level. More universal synthetic methods and synthetic mechanisms have not yet been systematically studied. Moreover, it should also be noted that the fundamental theory of HSNRs in driving catalysis requires increasing dedicated efforts and improvements.
In this review, the main concern was the significant progress of the construction of HSNRs and their unique properties in liquid-phase hydrogenation. Two general synthetic strategies, bottom-up and top-down, were introduced, and their underlying formation mechanism, merits and limitations were emphatically discussed. Furthermore, a distinctive viewpoint on the unique effects and properties of HSNRs in hydrogenation reactions of alkenes, alkynes, aromatics and biomass was illustrated and highlighted. Meanwhile, we also discussed the target of developing HSNRs to overcome the difficulties of hydrogenation.
With respect to the research passion of designing cell-mimicking systems, the increased level of controllable synthesis and mechanism exploration in both the structure and application of HSNRs creates many opportunities for the development of the next generation of multifunctional heterogeneous catalysts. Although many remarkable strides have been made, there is still much to do (Fig. 9). (1) Precise synthetic chemistry, based on the specific reactions to construct HSNRs, is highly desirable. The cavity and shell geometric parameters of HSNRs, e.g. the size and structure of internal cavities, pore structure, and pore orientation, offer ideal models for modulating mass transfer, substrate enrichment and reaction efficiency/pathways. (2) Developing multi-functional HSNRs is urgent for intricate catalysis, such as cascade/chiral/molecular-sieve reactions. For effective cascade multistep reactions (such as the sequential hydrogenation of biomass/nitroaromatic compounds, or the tandem oxidation of cinnamyl alcohol), HSNRs with multiple cavities and compartmentalizations would be an ideal platform for modifying multiple localized functional groups, spatially positioned reactive sites, and different compositions within one single nanoreactor. These HSNRs enable the efficient diffusion of reaction intermediates, effective synergistic catalysis between active sites, and finally boosted catalytic performance. (3) There is still limited understanding of the synthesis mechanism of HSNRs, especially for complex hollow nanostructures. It will be necessary to develop advanced techniques to visualize and track the structural evolution process, reveal the synthesis mechanism in kinetic/thermodynamics, and finally guide and inspire researchers to construct the next generation of HSNRs. (4) Advanced characterization techniques, such as density functional theory (DFT) calculation, finite element analyses (FDTD), and semi-in situ or ex situ approaches are highly desirable to study nanoreactor effects and hydrogenation reaction mechanisms, which will be beneficial for the precise design of high-performance HSNRs.
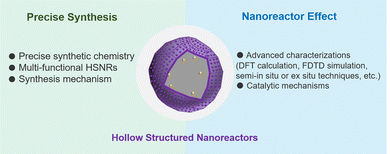 |
| Fig. 9 Schematic illustration of future research on HSNRs. | |
In short, the insights afforded by this Review will contribute to a deep understanding of HSNRs in the design, fabrication, catalytic behavior, and further development of multifunctional catalysts, and rational bridging of homogeneous and heterogeneous catalysis.
Author contributions
Yutong Pi: writing – original draft. Haitao Li: visualization. Jian Liu: writing – review & editing, supervision.
Data availability
No primary research results, software or code have been included and no new data were generated or analysed as part of this review.
Conflicts of interest
There are no conflicts to declare.
Acknowledgements
This work was supported by the National Natural Science Foundation of China (Grant 22279139), and the Program of Higher Level Talents of Inner Mongolia University (10000-23112101/119).
Notes and references
- J. H. Swisher, L. Jibril, S. H. Petrosko and C. A. Mirkin, Nanoreactors for particle synthesis, Nat. Rev. Mater., 2022, 7(6), 428 CrossRef
.
- S. H. Petrosko, R. Johnson, H. White and C. A. Mirkin, Nanoreactors: Small Spaces, Big Implications in Chemistry, J. Am. Chem. Soc., 2016, 138(24), 7443 CrossRef PubMed
.
- S. Roy, D. Skoff, D. V. Perroni, J. Mondal, A. Yethiraj, M. K. Mahanthappa, M. T. Zanni and J. L. Skinner, Water Dynamics in Gyroid Phases of Self-Assembled Gemini Surfactants, J. Am. Chem. Soc., 2016, 138(8), 2472 CrossRef PubMed
.
- D. E. Williams, E. A. Dolgopolova, P. J. Pellechia, A. Palukoshka, T. J. Wilson, R. Tan, J. M. Maier, A. B. Greytak, M. D. Smith and J. A. Krause,
et al., Mimic of the Green Fluorescent Protein β-Barrel: Photophysics and Dynamics of Confined Chromophores Defined by a Rigid Porous Scaffold, J. Am. Chem. Soc., 2015, 137(6), 2223 CrossRef PubMed
.
- G. Prieto, H. Tüysüz, N. Duyckaerts, J. Knossalla, G.-H. Wang and F. Schüth, Hollow Nano- and Microstructures as Catalysts, Chem. Rev., 2016, 116(22), 14056 CrossRef CAS PubMed
.
- Y. Boyjoo, H. Shi, Q. Tian, S. Liu, J. Liang, Z.-S. Wu, M. Jaroniec and J. Liu, Engineering nanoreactors for metal–chalcogen batteries, Energy Environ. Sci., 2021, 14(2), 540 RSC
.
- L.-P. Wang, A. Titov, R. McGibbon, F. Liu, V. S. Pande and T. J. Martínez, Discovering chemistry with an ab initio nanoreactor, Nat. Chem., 2014, 6(12), 1044 CrossRef CAS PubMed
.
- Z. Yu, N. Ji, X. Li, R. Zhang, Y. Qiao, J. Xiong, J. Liu and X. Lu, Kinetics Driven by Hollow Nanoreactors: An Opportunity for Controllable Catalysis, Angew. Chem., Int. Ed., 2023, 62(3), e202213612 CrossRef CAS
.
- R.-P. Ye, X. Wang, C.-A. H. Price, X. Liu, Q. Yang, M. Jaroniec and J. Liu, Engineering of Yolk/Core–Shell Structured Nanoreactors for Thermal Hydrogenations, Small, 2021, 17(9), 1906250 CrossRef CAS
.
- W. Zhu, Z. Chen, Y. Pan, R. Dai, Y. Wu, Z. Zhuang, D. Wang, Q. Peng, C. Chen and Y. Li, Functionalization of Hollow Nanomaterials for Catalytic Applications: Nanoreactor Construction, Adv. Mater., 2019, 31(38), 1800426 CrossRef PubMed
.
- D.-S. Bin, Y.-S. Xu, S.-J. Guo, Y.-G. Sun, A.-M. Cao and L.-J. Wan, Manipulating Particle Chemistry for Hollow Carbon-based Nanospheres: Synthesis Strategies, Mechanistic Insights, and Electrochemical Applications, Acc. Chem. Res., 2021, 54(1), 221 CrossRef CAS
.
- J. Wang, J. Wan and D. Wang, Hollow Multishelled Structures for Promising Applications: Understanding the Structure–Performance Correlation, Acc. Chem. Res., 2019, 52(8), 2169 CrossRef CAS PubMed
.
- T. Qiu, S. Gao, Z. Liang, D.-G. Wang, H. Tabassum, R. Zhong and R. Zou, Pristine Hollow Metal–Organic Frameworks: Design, Synthesis and Application, Angew. Chem., Int. Ed., 2021, 60(32), 17314 CrossRef CAS PubMed
.
- L. Huang, J. Yang, Y. Asakura, Q. Shuai and Y. Yamauchi, Nanoarchitectonics of Hollow Covalent Organic Frameworks: Synthesis and Applications, ACS Nano, 2023, 17(10), 8918 CrossRef CAS PubMed
.
- S. Liu, K. Dou, B. Liu, M. Pang, P. A. Ma and J. Lin, Construction of Multiform Hollow-Structured Covalent Organic Frameworks via a Facile and Universal Strategy for Enhanced Sonodynamic Cancer Therapy, Angew. Chem., Int. Ed., 2023, 62(18), e202301831 CrossRef CAS PubMed
.
- J. Liu, T. Yang, D.-W. Wang, G. Q. Lu, D. Zhao and S. Z. Qiao, A facile soft-template synthesis of mesoporous polymeric and carbonaceous nanospheres, Nat. Commun., 2013, 4(1), 2798 CrossRef
.
- Y. Zhao and L. Jiang, Hollow Micro/Nanomaterials with Multilevel Interior Structures, Adv. Mater., 2009, 21(36), 3621 CrossRef
.
- T. Zhao, A. Elzatahry, X. Li and D. Zhao, Single-micelle-directed synthesis of mesoporous materials, Nat. Rev. Mater., 2019, 4(12), 775 CrossRef
.
- P. Qiu, B. Ma, C.-T. Hung, W. Li and D. Zhao, Spherical Mesoporous Materials from Single to Multilevel Architectures, Acc. Chem. Res., 2019, 52(10), 2928 CrossRef PubMed
.
- L. Peng, H. Peng, Y. Liu, X. Wang, C.-T. Hung, Z. Zhao, G. Chen, W. Li, L. Mai and D. Zhao, Spiral self-assembly of lamellar micelles into multi-shelled hollow nanospheres with unique chiral architecture, Sci. Adv., 2021, 7(45), eabi7403 CrossRef CAS PubMed
.
- B. P. Bastakoti, M. Inuoe, S. Yusa, S. H. Liao, K. C. W. Wu, K. Nakashima and Y. Yamauchi, A block copolymer micelle template for synthesis of hollow calcium phosphate nanospheres with excellent biocompatibility, Chem. Commun., 2012, 48(52), 6532 RSC
.
- M. Sasidharan, N. Gunawardhana, M. Yoshio and K. Nakashima, WO3 hollow nanospheres for high-lithium storage capacity and good cyclability, Nano Energy, 2012, 1(3), 503 CrossRef CAS
.
- Y. Chen, D. Yang, Y. J. Yoon, X. Pang, Z. Wang, J. Jung, Y. He, Y. W. Harn, M. He and S. Zhang,
et al., Hairy Uniform Permanently Ligated Hollow Nanoparticles with Precise Dimension Control and Tunable Optical Properties, J. Am. Chem. Soc., 2017, 139(37), 12956 CrossRef CAS PubMed
.
- Q. Yu, J. Zhou, W. Wang, D.-C. Li, X. Sun and G.-H. Wang, Space-Confined Carbon-Doped Pd Nanoparticles as a Highly Efficient Catalyst for Selective Phenol Hydrogenation, ACS Catal., 2023, 13(6), 3925 CrossRef CAS
.
- R. Ameloot, F. Vermoortele, W. Vanhove, M. B. J. Roeffaers, B. F. Sels and D. E. De Vos, Interfacial synthesis of hollow metal-organic framework capsules demonstrating selective permeability, Nat. Chem., 2011, 3(5), 382 CrossRef CAS PubMed
.
- B. Y. Guan, L. Yu and X. W. Lou, Formation of Asymmetric Bowl-Like Mesoporous Particles via Emulsion-Induced Interface Anisotropic Assembly, J. Am. Chem. Soc., 2016, 138(35), 11306 CrossRef CAS PubMed
.
- B. Y. Guan, S. L. Zhang and X. W. Lou, Realization of Walnut-Shaped Particles with Macro-/Mesoporous Open Channels through Pore Architecture Manipulation and Their Use in Electrocatalytic Oxygen Reduction, Angew. Chem., Int. Ed., 2018, 57(21), 6176 CrossRef CAS PubMed
.
- G.-H. Wang, J. Hilgert, F. H. Richter, F. Wang, H.-J. Bongard, B. Spliethoff, C. Weidenthaler and F. Schüth, Platinum–cobalt bimetallic nanoparticles in hollow carbon nanospheres for hydrogenolysis of 5-hydroxymethylfurfural, Nat. Mater., 2014, 13(3), 293 CrossRef CAS PubMed
.
- Y. Ma, H. Zhang, R. Lin, Y. Ai, K. Lan, L. Duan, W. Chen, X. Duan, B. Ma and C. Wang,
et al., Remodeling nanodroplets into hierarchical mesoporous silica nanoreactors with multiple chambers, Nat. Commun., 2022, 13(1), 6136 CrossRef CAS PubMed
.
- G. Chen, J. Han, Z. Niu, P. She, L. Li, B. Guan and J. Yu, Regioselective Surface Assembly of Mesoporous Carbon on Zeolites Creating Anisotropic Wettability for Biphasic Interface Catalysis, J. Am. Chem. Soc., 2023, 145(16), 9021 CrossRef CAS PubMed
.
- Z. Zhao, L. Duan, Y. Zhao, L. Wang, J. Zhang, F. Bu, Z. Sun, T. Zhang, M. Liu and H. Chen,
et al., Constructing Unique Mesoporous Carbon Superstructures via Monomicelle Interface Confined Assembly, J. Am. Chem. Soc., 2022, 144(26), 11767 CrossRef CAS PubMed
.
- L. Peng, J. L. Zhang, J. S. Li, B. X. Han, Z. M. Xue, B. B. Zhang, J. H. Shi and G. Y. Yang, Hollow metal-organic framework polyhedra synthesized by a CO2-ionic liquid interfacial templating route, J. Colloid Interface Sci., 2014, 416, 198 CrossRef CAS PubMed
.
- Y. J. Wong, L. Zhu, W. S. Teo, Y. W. Tan, Y. Yang, C. Wang and H. Chen, Revisiting the Stöber Method: Inhomogeneity in Silica Shells, J. Am. Chem. Soc., 2011, 133(30), 11422 CrossRef CAS PubMed
.
- D.-S. Bin, Z.-X. Chi, Y. Li, K. Zhang, X. Yang, Y.-G. Sun, J.-Y. Piao, A.-M. Cao and L.-J. Wan, Controlling the Compositional Chemistry in Single Nanoparticles for Functional Hollow Carbon Nanospheres, J. Am. Chem. Soc., 2017, 139(38), 13492 CrossRef CAS PubMed
.
- Y. Pi, L. Cui, W. Luo, H. Li, Y. Ma, N. Ta, X. Wang, R. Gao, D. Wang and Q. Yang,
et al., Design of Hollow Nanoreactors for Size- and Shape-Selective Catalytic Semihydrogenation Driven by Molecular Recognition, Angew. Chem., Int. Ed., 2023, 62(43), e202307096 CrossRef CAS PubMed
.
- Y. Pi, Y. Ma, X. Wang, C.-A. H. Price, H. Li, Q. Liu, L. Wang, H. Chen, G. Hou and B.-L. Su,
et al., Multilevel Hollow Phenolic Resin Nanoreactors with Precise Metal Nanoparticles Spatial Location toward Promising Heterogeneous Hydrogenations, Adv. Mater., 2022, 34(43), 2205153 CrossRef CAS PubMed
.
- R. Yu, X. Huang, Y. Liu, Y. Kong, Z. Gu, Y. Yang, Y. Wang, W. Ban, H. Song and C. Yu, Shaping Nanoparticles for Interface Catalysis: Concave Hollow Spheres via Deflation–Inflation Asymmetric Growth, Adv. Sci., 2020, 7(13), 2000393 CrossRef CAS PubMed
.
- T. He, X. Xu, B. Ni, H. Lin, C. Li, W. Hu and X. Wang, Metal–Organic Framework Based Microcapsules, Angew. Chem., Int. Ed., 2018, 57(32), 10148 CrossRef CAS PubMed
.
- W. X. Liu, J. J. Huang, Q. Yang, S. J. Wang, X. M. Sun, W. N. Zhang, J. F. Liu and F. W. Huo, Multi-shelled Hollow Metal-Organic Frameworks, Angew. Chem., Int. Ed., 2017, 56(20), 5512 CrossRef CAS PubMed
.
- L.-L. Hu, L.-P. Yang, D. Zhang, X.-S. Tao, C. Zeng, A.-M. Cao and L.-J. Wan, Designed synthesis of SnO2–C hollow microspheres as an anode material for lithium-ion batteries, Chem. Commun., 2017, 53(81), 11189 RSC
.
- L.-P. Yang, X.-J. Lin, X. Zhang, W. Zhang, A.-M. Cao and L.-J. Wan, General Synthetic Strategy for Hollow Hybrid Microspheres through a Progressive Inward Crystallization Process, J. Am. Chem. Soc., 2016, 138(18), 5916 CrossRef PubMed
.
- T.-Q. Zhang, J. Liu, L.-B. Huang, X.-D. Zhang, Y.-G. Sun, X.-C. Liu, D.-S. Bin, X. Chen, A.-M. Cao and J.-S. Hu,
et al., Microbial-Phosphorus-Enabled
Synthesis of Phosphide Nanocomposites for Efficient Electrocatalysts, J. Am. Chem. Soc., 2017, 139(32), 11248 CrossRef PubMed
.
- Z.-X. Cai, Z.-L. Wang, Y.-J. Xia, H. Lim, W. Zhou, A. Taniguchi, M. Ohtani, K. Kobiro, T. Fujita and Y. Yamauchi, Tailored Catalytic Nanoframes from Metal–Organic Frameworks by Anisotropic Surface Modification and Etching for the Hydrogen Evolution Reaction, Angew. Chem., Int. Ed., 2021, 60(9), 4747 CrossRef PubMed
.
- S. Bao, J. Li, B. Guan, M. Jia, O. Terasaki and J. Yu, A Green Selective Water-Etching Approach to MOF@Mesoporous SiO2 Yolk-Shell Nanoreactors with Enhanced Catalytic Stabilities, Matter, 2020, 3(2), 498 CrossRef
.
- Q. Zhang, T. R. Zhang, J. P. Ge and Y. D. Yin, Permeable silica shell through surface-protected etching, Nano Lett., 2008, 8(9), 2867 CrossRef CAS PubMed
.
- Y. Hu, J. Ge, Y. Sun, T. Zhang and Y. Yin, A Self-Templated Approach to TiO2 Microcapsules, Nano Lett., 2007, 7(6), 1832 CrossRef CAS PubMed
.
- F. H. Chen, L. M. Zhang, Q. T. Chen, Y. Zhang and Z. J. Zhang, Synthesis of a novel magnetic drug delivery system composed of doxorubicin-conjugated Fe3O4 nanoparticle cores and a PEG-functionalized porous silica shell, Chem. Commun., 2010, 46(45), 8633 RSC
.
- N. T. Yang, F. Pang and J. P. Ge, One-pot and general synthesis of crystalline mesoporous metal oxides nanoparticles by protective etching: potential materials for catalytic applications, J. Mater. Chem. A, 2015, 3(3), 1133 RSC
.
- L. Luo, W.-S. Lo, X. Si, H. Li, Y. Wu, Y. An, Q. Zhu, L.-Y. Chou, T. Li and C.-K. Tsung, Directional Engraving within Single Crystalline Metal–Organic Framework Particles via Oxidative Linker Cleaving, J. Am. Chem. Soc., 2019, 141(51), 20365 CrossRef CAS PubMed
.
- W. Wang, H. Yan, U. Anand and U. Mirsaidov, Visualizing the Conversion of Metal–Organic Framework Nanoparticles into Hollow Layered Double Hydroxide Nanocages, J. Am. Chem. Soc., 2021, 143(4), 1854 CrossRef CAS PubMed
.
- G.-Y. Jeong, R. Ricco, K. Liang, J. Ludwig, J.-O. Kim, P. Falcaro and D.-P. Kim, Bioactive MIL-88A Framework Hollow Spheres via Interfacial Reaction In-Droplet Microfluidics for Enzyme and Nanoparticle Encapsulation, Chem. Mater., 2015, 27(23), 7903 CrossRef CAS
.
- Y. C. Tan and H. C. Zeng, Self-templating synthesis of hollow spheres of MOFs and their derived nanostructures, Chem. Commun., 2016, 52(77), 11591 RSC
.
- X. Han, T. Zhang, X. Wang, Z. Zhang, Y. Li, Y. Qin, B. Wang, A. Han and J. Liu, Hollow mesoporous atomically dispersed metal-nitrogen-carbon catalysts with enhanced diffusion for catalysis involving larger molecules, Nat. Commun., 2022, 13(1), 2900 CrossRef CAS PubMed
.
- M. Guo, S. Jayakumar, M. Luo, X. Kong, C. Li, H. Li, J. Chen and Q. Yang, The promotion effect of π–π interactions in Pd NPs catalysed selective hydrogenation, Nat. Commun., 2022, 13(1), 1770 CrossRef CAS PubMed
.
- J. Xiao, K. Cheng, X. Xie, M. Wang, S. Xing, Y. Liu, T. Hartman, D. Fu, K. Bossers and M. A. van Huis,
et al., Tandem catalysis with double-shelled hollow spheres, Nat. Mater., 2022, 21(5), 572 CrossRef CAS PubMed
.
- H. Chen, K. Shen, Y. Tan and Y. Li, Multishell Hollow Metal/Nitrogen/Carbon Dodecahedrons with Precisely Controlled Architectures and Synergistically Enhanced Catalytic Properties, ACS Nano, 2019, 13(7), 7800 CrossRef CAS PubMed
.
- Y. Ma, L. Wang, W. Zhao, T. Liu, H. Li, W. Luo, Q. Jiang, W. Liu, Q. Yang and J. Huang,
et al., Reactant enrichment in hollow void of Pt NPs@MnOx nanoreactors for boosting hydrogenation performance, Nat. Sci. Rev., 2023, 10(10), nwad201 CrossRef CAS PubMed
.
- Z. Yu, X. Lu, L. Sun, J. Xiong, L. Ye, X. Li, R. Zhang and N. Ji, Metal-Loaded Hollow Carbon Nanostructures as Nanoreactors: Microenvironment Effects and Prospects for Biomass Hydrogenation Applications, ACS Sustainable Chem. Eng., 2021, 9(8), 2990 CrossRef CAS
.
- T. S. Koblenz, J. Wassenaar and J. N. H. Reek, Reactivity within a confined self-assembled nanospace, Chem. Soc. Rev., 2008, 37, 237 RSC
.
- Z. Yu, N. Ji, J. Xiong, X. Li, R. Zhang, L. Zhang and X. Lu, Ruthenium-Nanoparticle-Loaded Hollow Carbon Spheres as Nanoreactors for Hydrogenation of Levulinic Acid: Explicitly Recognizing the Void-Confinement Effect, Angew. Chem., Int. Ed., 2021, 60(38), 20786 CrossRef PubMed
.
- C. Dong, Q. Yu, R.-P. Ye, P. Su, J. Liu and G.-H. Wang, Hollow Carbon Sphere Nanoreactors Loaded with PdCu Nanoparticles: Void-Confinement Effects in Liquid-Phase Hydrogenations, Angew. Chem., Int. Ed., 2020, 59(42), 18374 CrossRef
.
- L. Wang, R. Han, Y. Ma, M. S. Duyar, W. Liu and J. Liu, Spatial Location and Microenvironment Engineering of Pt-CeO2 Nanoreactors for Selective Hydrogenation of Cinnamaldehyde to Cinnamyl Alcohol, J. Phys. Chem. C, 2021, 125(41), 22603 CrossRef
.
- J. Kou, W. D. Wang, J. Fang, F. Li, H. Zhao, J. Li, H. Zhu, B. Li and Z. Dong, Precisely controlled Pd nanoclusters confined in porous organic cages for size-dependent catalytic hydrogenation, Appl. Catal., B, 2022, 315, 121487 CrossRef
.
- M. Zhao, K. Yuan, Y. Wang, G. Li, J. Guo, L. Gu, W. Hu, H. Zhao and Z. Tang, Metal–organic frameworks as selectivity regulators for hydrogenation reactions, Nature, 2016, 539(7627), 76 CrossRef CAS PubMed
.
- Z. Xiong, B. Sun, H. Zou, R. Wang, Q. Fang, Z. Zhang and S. Qiu, Amorphous-to-Crystalline Transformation: General Synthesis of Hollow Structured Covalent Organic Frameworks with High Crystallinity, J. Am. Chem. Soc., 2022, 144(14), 6583 CrossRef CAS PubMed
.
- X. Fang, S. Liu, J. Zang, C. Xu, M.-S. Zheng, Q.-F. Dong, D. Sun and N. Zheng, Precisely controlled resorcinol–formaldehyde resin coating for fabricating core–shell, hollow, and yolk–shell carbon nanostructures, Nanoscale, 2013, 5(15), 6908 RSC
.
- L. Li, Y. Li, L. Jiao, X. Liu, Z. Ma, Y.-J. Zeng, X. Zheng and H.-L. Jiang, Light-Induced Selective Hydrogenation over PdAg Nanocages in Hollow MOF Microenvironment, J. Am. Chem. Soc., 2022, 144(37), 17075 CrossRef CAS PubMed
.
- H. Zou, J. Dai, J. Suo, R. Ettelaie, Y. Li, N. Xue, R. Wang and H. Yang, Dual metal nanoparticles within multicompartmentalized mesoporous organosilicas for efficient sequential hydrogenation, Nat. Commun., 2021, 12(1), 4968 CrossRef CAS PubMed
.
- J. Lim, N. Kumari, T. B. Mete, A. Kumar and I. S. Lee, Magnetic–Plasmonic Multimodular Hollow Nanoreactors for Compartmentalized Orthogonal Tandem Catalysis, Nano Lett., 2022, 22(15), 6428 CrossRef CAS PubMed
.
- H. J. Cho, D. Kim, J. Li, D. Su and B. Xu, Zeolite-Encapsulated Pt Nanoparticles for Tandem Catalysis, J. Am. Chem. Soc., 2018, 140(41), 13514 CrossRef CAS PubMed
.
- L. Wang, L. Wang, X. Meng and F.-S. Xiao, New Strategies for the Preparation of Sinter-Resistant Metal-Nanoparticle-Based Catalysts, Adv. Mater., 2019, 31(50), 1901905 CrossRef CAS PubMed
.
- Z. Y. Zhang, F. Xiao, J. B. Xi, T. Sun, S. Xiao, H. R. Wang, S. Wang and Y. Q. Liu, Encapsulating Pd Nanoparticles in Double-Shelled Graphene@Carbon Hollow Spheres for Excellent Chemical Catalytic Property, Sci. Rep., 2014, 4, 4053 CrossRef PubMed
.
- L. Schoonen and J. C. M. van Hest, Compartmentalization Approaches in Soft Matter Science: From Nanoreactor Development to Organelle Mimics, Adv. Mater., 2016, 28(6), 1109 CrossRef CAS PubMed
.
- Y. Ma, Y.-L. Zhu, R. Lin, Y. Ai, L. Duan, K. Lan, B. Ma, J. Jia, W. Zhang and C. Wang,
et al., Synthesis of branched silica nanotrees using a nanodroplet sequential fusion strategy, Nat. Synth., 2024, 3, 236 CrossRef
.
- Y. Wei, J. Li, D. Zhao, Y. Zhao, Q. Zhang, L. Gu, J. Wan and D. Wang, ZnO HoMS@ZIF-8 Nanoreactors for Efficient Enrichment and Photoreduction of Atmospheric CO2, CCS Chem., 2024, 1 Search PubMed
.
- Y. Koizumi, K. Yonesato, S. Kikkawa, S. Yamazoe, K. Yamaguchi and K. Suzuki, Small Copper Nanoclusters Synthesized through Solid-State Reduction inside a Ring-Shaped Polyoxometalate Nanoreactor, J. Am. Chem. Soc., 2024, 146(21), 14610 CrossRef CAS PubMed
.
- G. Xie, J. Zhang and X. Ma, Compartmentalization of Multiple Catalysts into Outer and Inner Shells of Hollow Mesoporous Nanospheres for Heterogeneous Multi-Catalyzed/Multi-Component Asymmetric Organocascade, ACS Catal., 2019, 9(10), 9081 CrossRef CAS
.
|
This journal is © The Royal Society of Chemistry 2024 |
Click here to see how this site uses Cookies. View our privacy policy here.