A novel covalent organic framework for efficient photocatalytic reduction of Cr(VI) and synergistic removal of organic pollutants under visible light irradiation†
Received
16th October 2023
, Accepted 21st November 2023
First published on 23rd November 2023
Abstract
Polluted wastewater containing organic pollutants and heavy metals is increasing and harms environmental ecosystems and human health. Designing and producing an efficient photocatalyst is therefore crucial for treating polluted wastewater. In this study, electron-deficient benzothiadiazole was used as the electron acceptor, and electron-rich thiophene as the electron donor using a solvothermal method. The successful preparation of a novel visible-light responsive donor–acceptor type covalent organic framework (HDU-26) has been demonstrated using various characterization techniques. The conjugated structure of the electron-donating and electron-accepting units promoted the charge transfer of the HDU-26 photocatalyst, effectively reduced the band gap, and suppressed the electron–hole (e−–h+) complexation. The photocatalytic performance was investigated under the influence of different parameters. Under optimal conditions, HDU-26 achieved a photocatalytic reduction efficiency of up to 99.5% for Cr(VI). Over five reaction cycles, HDU-26 was still able to maintain a high level of photocatalytic activity, with the Cr(VI) removal rate reaching 97.4%. The major active species for the photocatalytic reduction of Cr(VI) were e− and ·O2− radicals. In addition, the oxidation of organic matter results in an increase in photogenerated e−–h+ separation. Moreover, the photocatalytic rate of HDU-26 was significantly accelerated due to the synergistic effect in the mixed system of Cr(VI)/organic pollutants (RhB, MB, MO). In conclusion, the high availability of active sites, excellent stability, and recyclability of HDU-26 indicate its potential applications for removing organic pollutants and heavy metal ions from contaminated wastewater.
Environmental significance
With the rapid industrialization, water quality is deteriorating significantly. Pollution in wastewater, consisting of organic dyes and heavy metals, is on an upward trend, harming ecosystems and survival of plants and animals. As an advanced oxidation technology, photocatalytic technology offers straightforward operation, prompt reaction times, and environmentally friendly qualities. This technology possesses unparalleled benefits in purifying contaminated water. Covalent organic frameworks possessing high specific surface area, intrinsic porosity, π-conjugated structures, excellent light absorption, and adequate forbidden bandwidth are optimal for photocatalysts. These materials show promising potential for removing organic pollutants and heavy metal ions from contaminated wastewater.
|
1. Introduction
As a vital component for human sustenance and progress, water bodies have encountered diverse material contamination to differing extents owing to the swift development of industrialization. In particular, polluted wastewater comprising organic and heavy metal elements is increasing, causing harmful effects on the ecological system and jeopardizing human well-being.1 Organic dyes in wastewater are generally chemically stable and highly colored. Their persistence in nature challenges the growth of aquatic flora and fauna and poses significant risks to human health and the sustainable development of ecosystems.2,3 Wastewater containing organic dyes is commonly accompanied by Cr(VI), a heavy metal pollutant. Cr(VI) is a highly toxic pollutant known for its carcinogenic and mutagenic properties, which is typically used in the tanning, electroplating, textile dyeing, petroleum refining, and paint manufacturing industries.4,5 By contrast with Cr(VI), Cr(III) is less hazardous and is a trace element essential for normal human life activities.6,7 Consequently, reducing Cr(VI) to Cr(III) has become a hot research topic in the environmental field.
Contaminated wastewater must be rigorously treated and purified before it can be discharged, which improves the quality of the water environment and provides significant economic advantages. Standard treatment methods, for instance, membrane separation,8 chemical precipitation,9 adsorption,10 biodegradation,11 and photocatalytic techniques,12 have been widely used to remove contaminated wastewater containing organic dyes and Cr(VI). In particular, photocatalytic technology is an advanced oxidation technology that uses green and renewable light to photodegrade harmful substances, converting solar energy into chemical energy. Compared to other technologies, it has unrivaled advantages in treating organic pollutants and is an easy-to-use, rapid response, and environmentally friendly means of treating pollutants.13
As a type of organic porous material, covalent organic frameworks (COFs), formed by covalent bonds interconnected in a mesh-like structure, have become the focus of attention in recent years.14 The unique topology, multiple cell configurations, and adjustable apertures have greatly expanded the range of applications for COFs.15 Furthermore, they exhibit excellent photocatalytic performance with high carrier mobility, broad light harvesting, and charge separation efficiency.16 The π-conjugated structural units in donor–acceptor (D–A) type COFs play a crucial role in light trapping. Herein, the conjugated structure of the electron-donating and electron-absorbing units can effectively promote the charge transfer on the framework of COFs, thereby narrowing the bandgap, broadening the absorption, and modulating the energy levels.17 The simultaneous construction of D–A framework materials with uniformly arranged units facilitates the formation of independent hole and singlet transport channels, which promotes exciton segregation and suppresses electron–hole (e−–h+) complexation.18,19 After a long exploration period, COFs have shown significant value and potential in gas separation,20 multiphase catalysis,21 energy storage,22,23 drug delivery,24,25 and other fields.
Herein, we have successfully prepared a visible light-responsive novel D–A type structural unit covalent organic framework denoted as HDU-26 by a solvothermal method, using electron-deficient benzothiadiazole as the electron acceptor and electron-rich thiophene as the electron donor. The optical properties, surface properties, and crystal structure of HDU-26 were characterized using different techniques, indicating that it is an efficient photocatalyst for Cr(VI) reduction and synergistic removal of organic pollutants. The study investigated the impact of various factors, including initial pH, catalyst dosage, and Cr(VI) concentration, on the photocatalytic reduction of Cr(VI). The stability of HDU-26 was also examined under different conditions. In addition, a potential mechanism for the effective photocatalytic reduction of Cr(VI) by the HDU-26 photocatalyst has been put forth, building upon free radical trapping experiments and electron spin resonance spectroscopy (ESR) signal monitoring.
2. Experimental
2.1. Materials
All materials, reagents, and solvents were obtained from commercial sources. They were used as received without further purification, including benzo[1,2-b:3,4-b′:5,6-b′′]trithiophene-2,5,8-tricarbaldehyde (BTT), 4,4′-(benzo[c][1,2,5]thiadiazole-4,7-diyl)dianiline (BT), acetic acid (AcOH), 1,4-dioxane, mesitylene, ethanol (EtOH), tetrahydrofuran (THF), sulfuric acid (H2SO4), sodium hydroxide (NaOH), nitric acid (HNO3), N,N-dimethylformamide (DMF), ethylenediaminetetraacetic acid disodium salt (EDTA-2Na), isopropyl alcohol (IPA), acetone, diphenylcarbazide (DPC), barium sulfate (BaSO4), potassium dichromate (K2Cr2O7), rhodamine B (RhB), methylene blue (MB) and methyl orange (MO), which were purchased from Macklin's Reagent Co., Ltd, Jilin Chinese Academy of Sciences-Yanshen Technology Co., Ltd, and Shanghai Aladdin Co., Ltd, China. In addition, ultrapure water was used throughout the experiment. Ultrapure water (18.25 MΩ cm) purified using an ultrapure water system was used in all experiments.
2.2. Synthesis of HDU-26
The synthesis method of HDU-26 is shown in Fig. 1a. In the present study, HDU-26 was successfully prepared based on a solvothermal method by combining the acceptor unit BT (0.075 mmol, 23.9 mg) and the donor unit BTT (0.05 mmol, 16.5 mg) in a 10 mL glass tube with 1,4-dioxane (0.625 mL), mesitylene (0.208 mL) and AcOH (12 M, 0.166 mL). Ultrasonic treatment was conducted for 20 min using an ultrasonic cleaner to achieve homogeneous mixing of the reactants. The glass tube was rapidly frozen in liquid nitrogen, sealed after three cycles of degassing in the thawing mode of the freezing pump, and then subjected to a 72 h condensation reaction at 120 °C. Following cooling to room temperature, the product was separated by centrifugation and washed alternately with THF and EtOH until the supernatant was clarified. Eventually, after drying in a vacuum oven at 60 °C for several hours, the corresponding orange-colored HDU-26 was obtained in 90% yield.
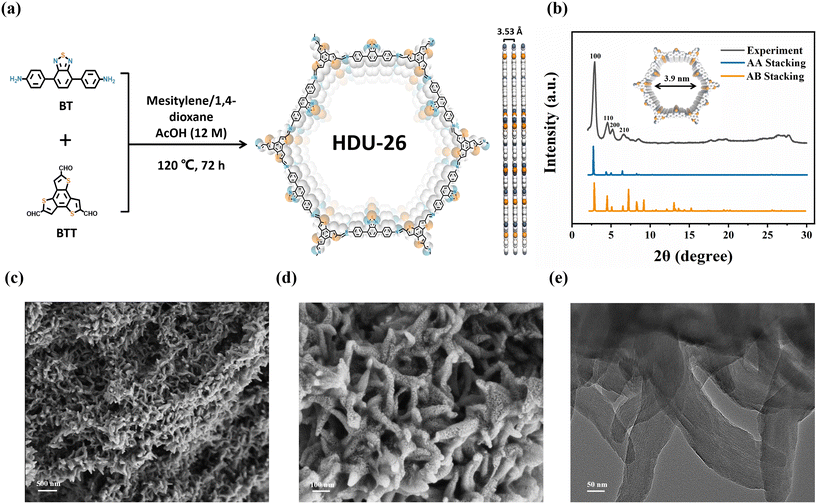 |
| Fig. 1 (a) Synthetic scheme of HDU-26. (b) Experimental and simulated XRD patterns of HDU-26. (c and d) SEM and (e) TEM images of HDU-26. | |
2.3. Characterization
The details of HDU-26 are shown in the ESI.†
2.4. Photodegradation experiment
In the photocatalytic experiments, the reduction of Cr(VI) was performed by exposing K2Cr2O7 solution to simulated visible light (λ > 420 nm, 300 W xenon lamp with an optical power density of 397 mW cm−2) to assess the efficiency of the prepared HDU-26 catalyst. The photocatalytic reaction temperatures were kept at 25 °C by passing circulating water to cool the reaction system to eliminate the interference of the photocatalytic reaction temperature. Initially, 8 mg of HDU-26 was dispersed in K2Cr2O7 solution (50 mL 10 mg L−1), and the initial pH of the solution was adjusted to 3.0 with H2SO4/NaOH solution without adding any sacrificial agent. The reaction was carried out in the dark for 60 min to reach the adsorption–desorption equilibrium and exclude the adsorption effect on the photocatalytic reduction efficiency. After the dark reaction, the xenon lamp was turned on, and 1 mL of the suspension was taken and filtered through a 0.22 μm filter membrane after a period of illumination. Finally, the DPC method measured the absorbance at the maximum absorption wavelength of 540 nm to evaluate the performance of the prepared photocatalyst HDU-26 for the reduction of Cr(VI).
To further investigate the photocatalytic performance of HDU-26, 8 mg of HDU-26 was added into a 50 mL mixed solution containing 10 mg L−1 organic pollutants (RhB, MB, MO) and 10 mg L−1 K2Cr2O7, respectively. The initial pH of the solution was adjusted to 3.0, and the absorbance of RhB, MB, MO, and Cr(VI) at 554, 664, 464, and 540 nm was determined by UV-vis spectrophotometry. These absorbances were used to calculate the change in concentration and removal of organic pollutants and Cr(VI).
3. Results and discussion
3.1. Characterization of HDU-26
In order to analyze the crystal structure of HDU-26 with the novel D–A structure, it was characterized using X-ray diffraction (XRD). The strong diffraction peak of HDU-26 at 2.51° (2θ) corresponds to the (100) facet reflection. Three other weak diffraction peaks appeared at 4.11°, 4.71°, and 6.17°, which corresponded to the (110), (200), and (210) facet reflections, respectively (Fig. 1b). The crystal structure was simulated employing Materials Studio (MS) software. The data from the AA stacking simulation and the sample powder diffraction peaks were in good agreement, with a layer spacing of 3.53 Å. Therefore, it is concluded that HDU-26 has been successfully synthesized and had a high degree of crystallinity. The surface morphology of HDU-26 was analyzed by transmission electron microscopy (TEM) and scanning electron microscopy (SEM), and we found that HDU-26 was mainly stacked in nanoscale rod-like structures (Fig. 1c–e).
Besides, Fourier-transform infrared (FTIR), X-ray photoelectron spectroscopy (XPS), and solid-state 13C nuclear magnetic resonance (NMR) spectroscopy characterization methods were used further to confirm the chemical composition and structure of HDU-26. In the FTIR spectrum of HDU-26 (Fig. 2a), the vibrational peaks of –NH2 at positions 3200–3500 cm−1 in the BT monomer disappeared, and the C
O vibrational peak at position 1664 cm−1 in the BTT monomer disappeared, while it was observed in HDU-26 the appearance of a new imine (C
N) stretching vibrational peak at position 1614 cm−1 with positions 1587 and 1253 cm−1 corresponding to C
C and C–N stretching vibrational peaks, respectively.26,27 The 13C NMR spectrum of HDU-26 (Fig. 2b) demonstrated that the characteristic signal peak of the enamine carbon (C
N) was at about 153 ppm, further confirming the conversion of the monomer to highly crystalline HDU-26 by condensation reaction.28 The presence of C 1s, S 2p, and N 1s peaks in the full XPS spectrum (Fig. 2c) further validated the presence of carbon, nitrogen, and sulfur in the HDU-26 framework. The high-resolution C 1s region (Fig. 2d) displayed three peaks at 284.04, 284.8, and 288.17 eV, corresponding to C
C, C–N, and C
N–C carbons, respectively.29 The high-resolution N 1s region (Fig. 2e) displayed two peaks at 398.26 and 399.18 eV, corresponding to C
N and C–NH, respectively.28 The combined results of these analyses indicate that the novel D–A structure of HDU-26 has been successfully synthesized.
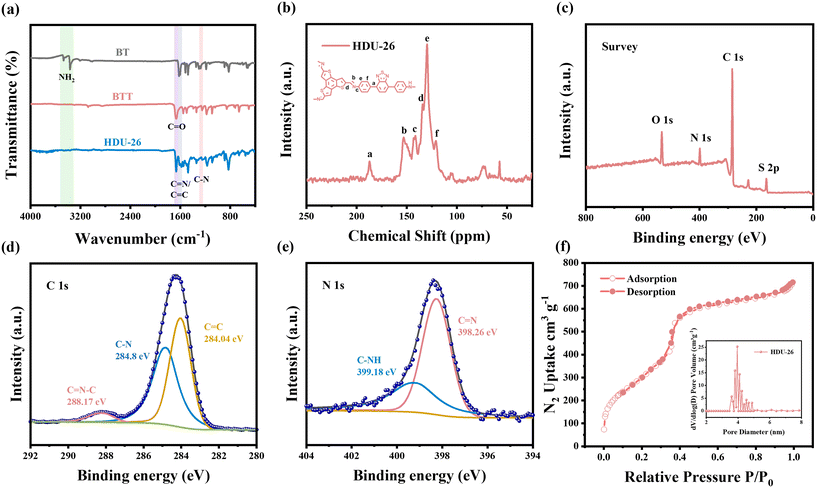 |
| Fig. 2 (a) FT-IR spectra of HDU-26. (b) 13C NMR spectrum of HDU-26. (c) XPS survey scan of HDU-26 and high-resolution XPS spectra of the C 1s (d) and N 1s (e) regions. (f) N2 adsorption–desorption isotherms of HDU-26. | |
The N2 adsorption–desorption isotherm characterization was used to obtain an understanding of the specific surface area and porosity of HDU-26 (Fig. 2f). The adsorption started to rise slowly near a P/P0 of 0.2, which indicated that a large number of mesopores existed within the material, a type-IV mesopore isotherm. The Brunauer–Emmett–Teller (BET) specific surface area of HDU-26 was determined to be 1176.91 m2 g−1, and the pore size of HDU-26 was calculated to be 4.0 nm by using the nonlocal density flooding theory (NLDFT) model. Consequently, the high specific surface area and nanoscale empty structure provide more active sites, which favor the rapid migration of photogenerated charges to the surface to participate in the photocatalytic oxidation–reduction reaction.30
The chemical and thermal stability of HDU-26 was explored. Thermogravimetric (TG) analysis showed that HDU-26 had excellent thermal stability (Fig. 3a), with a weight loss of 5.23% from 25–300 °C due to the volatilization of the solvent on the surface of the material or some of the residual solvent in the pores.31 As the temperature continued to increase, the weight of HDU-26 decreased by 21.79% at approximately 300–600 °C. When the temperature exceeded 600 °C, the weight of HDU-26 entered a rapid decrease phase due to the decomposition of the skeleton.32 In addition, HDU-26 was immersed in different solutions for 12 h, including DMF, HNO3 (3 M), and NaOH (3 M). The thermal stability of HDU-26 was analyzed from XRD patterns and FT-IR spectra (Fig. 3b and c). The diffraction peaks of HDU-26 had the same positions in the XRD patterns and favorable crystallinity was retained. The functional groups such as imine (C
N), C
C, and C–N were still clearly observed in the FT-IR spectra, which indicated that HDU-26 had excellent chemical stability. Consequently, the potential of HDU-26 applications in various fields can be tapped.
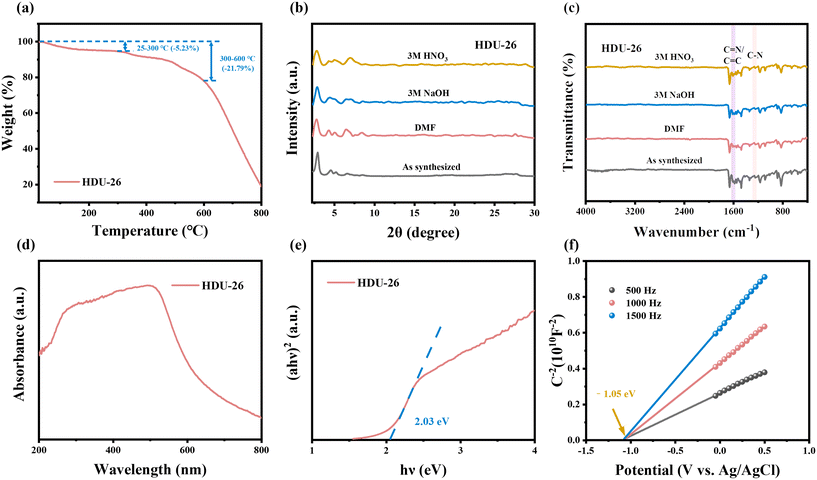 |
| Fig. 3 (a) TG curve of HDU-26. XRD patterns (b) and FT-IR spectra (c) of HDU-26 before and after treatment under different harsh conditions. (d) UV-vis DRS spectrum. (e) (αhν)n/2vs. photo energy curve. (f) MS profile of HDU-26. | |
The light absorption properties of photocatalysts are closely related to the catalytic redox ability of the materials, which led to the evaluation of the visible light absorption properties of HDU-26 by UV-vis diffuse reflectance spectroscopy (DRS). Due to the π–π conjugation effect, a broad light absorption range of 400–600 nm was observed for HDU-26 in the DRS spectrum (Fig. 3d), which was able to capture more visible light. The optical band gap of HDU-26 was calculated from the following equation:33
| (αhν)n/2 = A(hν − Eg) | (3.1) |
where
α denotes the absorbance index,
h denotes Planck's constant,
ν denotes the frequency of light,
A is a constant,
Eg is the energy value of the bandgap, and
n is a power index determined by the type of optical jump, with
n = 1 denoting a direct jump and
n = 4 denoting an indirect jump. Since the hopping type of HDU-26 was direct, the bandgap energy was calculated to be
n = 1 by extrapolating a straight line on the
X-axis intersection, which resulted in a direct bandgap of 2.03 eV for HDU-26 (
Fig. 3e). The narrower band gap absorbed the visible light more efficiently, thus exhibiting superior photocatalytic redox activity, an ideal photocatalyst for photocatalytic research applications.
The Mott Schottky (MS) profile (Fig. 3f) was taken in order to determine further the type of the two pure samples and the associated flat-band potential (EFB). The positive correlation between the C2 value and the potential value at 500, 1000, 1500 Hz implied that HDU-26 was a typical n-type semiconductor. The MS spectrogram can be roughly deduced from the fact that the EFB of HDU-26 was −1.05 V concerning the Ag/AgCl electrode. In addition, the conduction band (CB) potential of n-type semiconductors is approximately 0.1 V negative than their EFB.34 Therefore, the ECB value of HDU-26 can be estimated to be −1.15 V concerning NHE, corresponding to a valence band value (VB) of 0.88 V.
3.2. Photocatalytic performance
In order to differentiate the photocatalytic and adsorption properties of the HDU-26 catalyst, the photocatalytic Cr(VI) (10 mg L−1, 50 mL) reduction efficiency of HDU-26 at pH = 3.0 was investigated (Fig. S1a†), where C is the Cr(VI) concentration at the irradiation time t (min), and C0 is the initial Cr(VI) concentration. When no catalyst was added, just under visible light, Cr(VI) could not be reduced, indicating that the Cr(VI) solution was stable and not easily reduced by visible light, and the photocatalytic effect of the Cr(VI) solution itself was negligible. With the addition of the catalyst, there was almost no Cr(VI) adsorption under dark conditions, indicating that light is an essential condition in the HDU-26 photocatalytic reduction of the Cr(VI) reaction system. Surprisingly, Cr(VI) was not adsorbed after adding 8 mg of HDU-26 to the solution for 60 min under dark conditions, and the reduction efficiency of Cr(VI) was as high as 99% after 60 min of visible light irradiation. Fig. S1b† demonstrates that Cr(VI) could hardly be removed under dark conditions with and without the addition of HDU-26. Fig. S2a† shows the gradual evolution of the UV-vis absorption spectra of the photocatalytic reduction of Cr(VI) by HDU-26 at certain time intervals, and the intensity of the Cr(VI) absorption peaks decreased rapidly until they disappeared within 60 min with the prolongation of the photocatalytic reaction time. In summary, the novel D–A structure of HDU-26 with an extended π-conjugated backbone obtained by the Schiff base reaction exhibited a wide range of light-harvesting ability and ultra-high photocatalytic activity in the visible region. Previous studies have shown that the π-conjugated structural units in D–A type COFs play a crucial role in light trapping.18,35,36 Our conjugated structure had thiophene in BTT as the electron-donor unit and benzothiadiazole in BT as the electron-absorbing unit, and this structure promoted the spatial segregation of charge carriers, thus attenuating charge complexation and giving HDU-26 excellent photocatalytic performance. Consequently, the photocatalytic active sites are assumed to be mainly located on the thiophene and benzothiadiazole units. This work investigated the effects of varying the initial pH, catalyst dosage and Cr(VI) concentration and synergistic removal of organic pollutants on the photocatalytic reduction of Cr(VI) by HDU-26.
3.3. Effect of different conditions on the photocatalytic reduction of Cr(VI)
3.3.1. Effect of varying initial pH.
The basic process of the photocatalytic reaction is the adsorption of pollutants on the surface of the catalyst. Then, under irradiation of visible light, the catalyst absorbs the light energy, and the active sites are excited to degrade the pollutant and complete the purification of the water body. Consequently, the initial pH of the solution is a crucial factor in the photocatalytic reaction, affecting the form of the pollutant present and the catalyst's active sites. To investigate the effect of pH on the photocatalytic reduction of Cr, H2SO4 and NaOH were chosen to adjust the system's pH in this test. As shown in Fig. 4a and d, HDU-26 showed significant differences in the photocatalytic reduction of Cr under different pH conditions. At pH = 4.0–8.0, the photocatalytic efficiency of HDU-26 gradually decreased with increasing pH, and when pH = 8.0, the photocatalytic efficiency was merely 17.5%, and the proposed primary photoreduction reaction rate k was 0.00281 min−1. The photocatalysts adsorbed more Cr(VI) in the form of Cr2O72−, HCrO4− and CrO42− anions under acidic conditions at low pH, and the abundant H+ also promoted the transition from Cr(VI) to Cr(III), which enhanced the efficiency and rate of photocatalytic reduction.37 Particularly at pH = 3.0, the photocatalytic reduction efficiency reached 99.5% within 60 min, and the photoreduction rate constant was 0.0849 min−1, which was 30.2 times higher than that at pH = 8.0. The efficiency of the photocatalytic reduction of Cr by HDU-26 was slightly decreased when pH = 2.0 was affected by the catalyst's tendency to aggregate in strongly acidic environments.38 From the zeta potential plot of HDU-26 (Fig. S2b†), its equipotential point is 4.5. Hence, under acidic conditions, its photocatalytic reduction efficiency was higher owing to the substantially positively charged surface and electrostatic attraction by HDU-26. Under neutral or alkaline conditions, Cr(VI) mainly exists as CrO42−.39 Due to the substantial negative charge on the surface of HDU-26, which generated electrostatic repulsion to CrO42−, and the presence of Cr(OH)3 precipitates on its surface, the photocatalytic active sites were reduced, which led to a decrease in its photocatalytic effect (eqn (3.2)–(3.4)).40,41 | Cr2O72– + 14H+ + 6e− → 2Cr3+ + 7H2O | (3.2) |
| HCrO4− + 7H+ + 3e− → Cr3+ + 4H2O | (3.3) |
| CrO42− + 4H2O + 3e− → Cr(OH)3↓ + 5OH− | (3.4) |
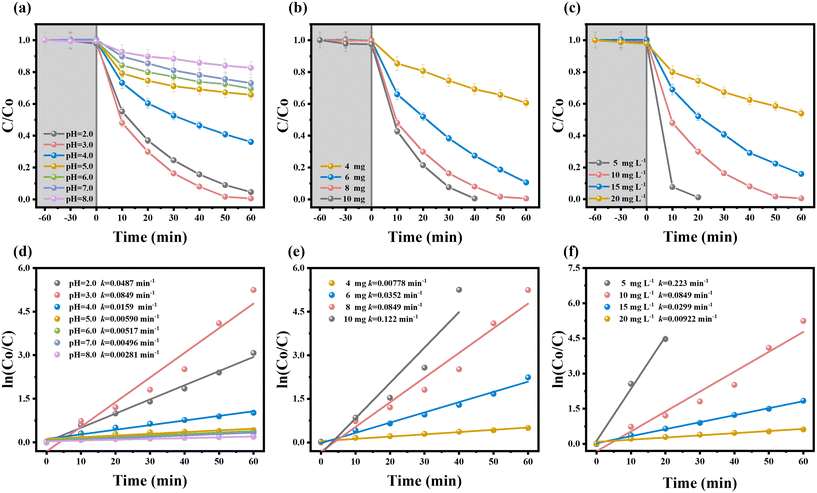 |
| Fig. 4 Impact of (a) initial pH, (b) catalyst dosage, and (c) Cr(VI) concentration on Cr(VI) photoreduction. (d) Primary photoreduction reaction rate k for different initial pH values. (e) Primary photoreduction reaction rate k for different catalyst dosages. (f) Primary photoreduction reaction rate k for different Cr(VI) concentrations. | |
3.3.2. Effect of varying catalyst dosage.
The catalyst dosage in the system significantly affects the reaction activity in the photocatalytic process. The photocatalytic reduction efficiency increased from 39.4% to 99.5% with a corresponding gradual increase in k when the catalyst dosage was increased from 4 mg to 10 mg in the system at pH = 3.0 (Fig. 4b and e). In particular, when the catalyst dosage was 10 mg, the Cr(VI) reduction reached 99.5% in only 40 min, with a maximum photoreduction rate constant of 0.122 min−1. This improvement may be attributed to the increased catalyst concentration leading to enhanced photocatalytically active sites and light absorption.1 Notably, the photocatalytic reduction effectiveness was also at the peak of 99.5% in 60 min when the catalyst concentration was 8 mg. Considering the economic aspect, 8 mg was chosen as the most favorable HDU-26 concentration in the system.
3.3.3. Effect of varying Cr(VI) concentration.
The effect of varying Cr(VI) concentration in the system on the photocatalytic performance was investigated and is shown in Fig. 4c and f. The photocatalytic reduction of Cr(VI) solutions with different concentrations by HDU-26 showed that the photocatalytic reduction efficiency was weakened with increased Cr(VI) concentration, and the corresponding k decreased gradually. For 5 mg L−1 Cr(VI) solution, the photocatalytic reduction efficiency reached 99.2% in merely 20 min of visible light irradiation, where k was 0.223 min−1 maximum. For solutions containing 10, 15, and 20 mg L−1 Cr(VI) after 60 min of reaction, the photocatalytic reduction efficiencies were 99.5%, 84.2%, and 46.1%, respectively. The corresponding values of k were 0.0849, 0.0229, and 0.00922 min−1, respectively. When the concentration of Cr(VI) increased, the amount adsorbed on the surface of HDU-26 increased, occupying the adsorption sites on the surface of the catalyst, hindering the transmission of visible light, underutilizing light, and producing less active substances, leading to the decrease of the photocatalytic reduction rate.42,43
3.3.4. Synergistic photocatalysis of Cr(VI) reduction and degradation of organic pollutants.
The potential application of simultaneously removing Cr(VI)/organic dyes from industrial wastewater is notable due to heavy metal pollutants and other organic contaminants. The photocatalytic activity of HDU-26 for the synergistic removal of Cr(VI)/organic pollutants (RhB, MB, MO) was investigated (Fig. 5a–d). Due to the high specific surface area and suitable pore size of HDU-26, it has strong adsorption and photocatalytic ability for organic dyes (50 mL 10 mg L−1) in a single system, and the removal rates of RhB, MB, and MO were 82.6, 56.4, and 99.1%, respectively. The photocatalytic experiments were carried out at pH = 3.0, where the surface of HDU-26 was positively charged and had the most substantial adsorption effect on the anionic dye MO, with an adsorption efficiency of 73.7% in 60 min. When RhB, MB, and MO were mixed with Cr(VI), their removal rates were significantly increased to 97.2, 62.3, and 99.2%, respectively. The Cr(VI) reduction rate in the RhB and MB mixed system was also slightly increased. The improved photocatalytic activity of the system was primarily caused by the enhanced separation of photogenerated e−–h+ due to organic matter oxidation, leading to a synergistic effect of Cr(VI) reduction and organic matter degradation.5
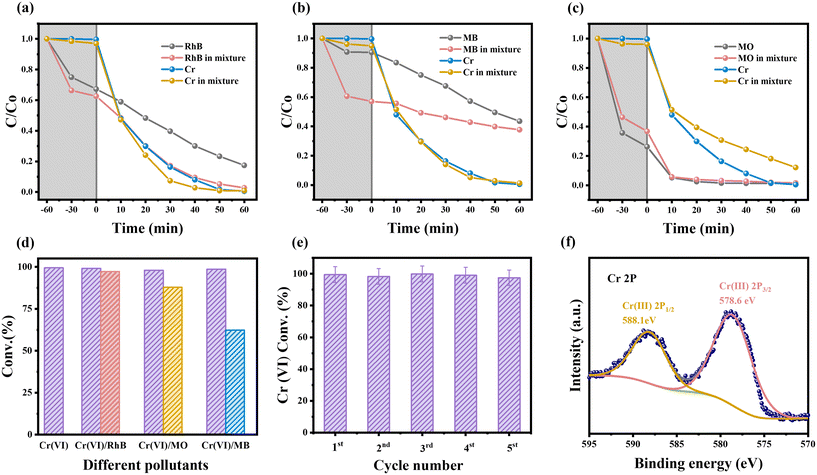 |
| Fig. 5 (a–d) Photocatalytic reduction of Cr(VI) and synergistic removal of organic pollutants by HDU-26. (e) The reusability of HDU-26 in the photocatalytic reduction of Cr(VI). (f) XPS spectrum of the Cr element on the surface of HDU-26 after the photocatalytic reduction. | |
3.4. Examining the stability and reusability of the HDU-26 photocatalyst
The stability and reusability of the catalyst in practical applications are precious. The study tested the stability of HDU-26 through multiple cycling experiments in the photocatalytic reduction of Cr(VI) under optimal conditions. After each cycle, HDU-26 was separated from the dispersion by centrifugal separation. It was washed several times with 1 M HNO3 and then with ultrapure water and EtOH. After drying the treatments at 60 °C, the photocatalytic experiments were continued under the same conditions. The test results are shown in Fig. 5e, which showed that after five cycles, HDU-26 still achieved 97.4% Cr(VI) removal. Furthermore, the XRD pattern (Fig. S3a†) after five cycles showed a well-structured crystal structure, the positions of the characteristic peaks matched with those of the pristine HDU-26, and the FT-IR spectra showed that the functional groups on the surface were similar to those of the fresh material (Fig. S3b†). The XPS spectra revealed that the elemental composition of HDU-26 remained unchanged before and after the photocatalytic reaction, except for Cr(VI), and the C, N, and S binding energies of HDU-26 were consistent with those measured before the photocatalytic reaction (Fig. S3c–f†). As shown in Fig. 5f, two prominent characteristic peaks of Cr(III) appeared at positions of 588.1 eV (Cr 2p1/2) and 578.6 eV (Cr 2p3/2), which indicated that Cr(VI) on the surface of the material was reduced to Cr(III).44 The optimum results of the photocatalytic reduction of Cr(VI) by HDU-26 were compared with other porous catalysts, as shown in Table 1. In general, HDU-26 demonstrates excellent stability and reusability in photocatalytic reduction of Cr(VI), proving an effective solution for treating polluted water.
Table 1 Comparison of photocatalytic performance of typical porous materials for the reduction of Cr(VI)
Photocatalysts |
Volume (ml) |
pH |
Dosage (mg) |
Concentration (mg L−1) |
Hole scavenger |
Irradiation time (min) |
Reduction efficiency (%) |
Ref. |
JOU-7 |
30 |
2.3 |
10 |
9 |
IPA |
100 |
95.2 |
18
|
TPB-TP-COF |
10 |
7 |
10 |
10 |
NA |
105 |
80 |
35
|
Au@C2 |
30 |
2.3 |
10 |
9 |
IPA |
120 |
98.2 |
36
|
Py–SO1 |
50 |
4 |
5 |
10 |
NA |
90 |
93 |
44
|
20%COF-CNNS |
20 |
3 |
20 |
10 |
NA |
30 |
99 |
45
|
DTB-BT-COF |
20 |
3 |
10 |
10 |
C2H5OH |
15 |
99.6 |
46
|
COF-PDZ |
60 |
7 |
15 |
10 |
NA |
20 |
95 |
47
|
TzDa-COF |
50 |
2 |
10 |
10 |
NA |
100 |
40.5 |
48
|
TACTF |
30 |
— |
30 |
50 |
BnOH |
120 |
75 |
49
|
dDAAQ-TFP |
30 |
3 |
10 |
30 |
NA |
90 |
96 |
50
|
UiO-66-NH2 |
40 |
2 |
20 |
10 |
NA |
80 |
97 |
51
|
JOU-22(Fe) |
20 |
3 |
10 |
4 |
NA |
20 |
100 |
52
|
MIL-68(In)–NH2 |
40 |
2 |
40 |
20 |
NA |
180 |
97 |
53
|
HDU-26 |
50 |
3 |
8 |
10 |
NA |
60 |
99.5 |
This work |
3.5. Photocatalytic reduction mechanism analyses
To analyze the role of reactive substances in the Cr(VI) reduction reaction, IPA, EDTA-2Na, and Na2S2O8 were added as ·OH, h+, and e− radical scavengers, respectively. As shown in Fig. 6a, the introduction of IPA and EDTA-2Na into the system significantly increased the reduction rate of Cr(VI) due to the enhanced e−–h+ separation. Furthermore, IPA acted as an ·OH scavenger and blocked the oxidation of Cr(III) on the HDU-26 surface, preventing its conversion to Cr(VI).36,54 In contrast, the reduction rate of Cr(VI) decreased significantly after adding Na2S2O8, and it was only 29% after 60 min of reaction. The reduction rate of Cr(VI) was decreased by the addition of BQ. To prevent the formation of ·O2−, the photocatalytic reaction was carried out under N2 conditions, and the reduction rate of Cr(VI) was also slightly inhibited. It was demonstrated that e− and ·O2− were important active substances, among which e− played the critical role. The generation of ·O2− during the photocatalytic reduction of Cr(VI) was further verified using ESR with 5,5-dimethyl-1-pyrroline N-oxide (DMPO) as a spin-trapping reagent. No characteristic signal was detected under dark conditions, and the characteristic signal of ·O2− appeared after visible light irradiation, which demonstrated that light exposure was crucial for the production of the active species (Fig. 6b). Moreover, to analyze the role of active substances in the photocatalytic degradation of organic pollutants by HDU-26, IPA, EDTA-2Na, and BQ were added as ·OH, h+, and ·O2− radical scavengers, respectively (Fig. S4a–c†). In three different organic pollutant solutions, RhB, MB, and MO, the addition of radical scavengers showed different degrees of inhibition, indicating that the active substances involved in the photocatalytic degradation of organic dyes are ·OH, h+, and ·O2−. The production of ·OH during photocatalytic degradation of organic pollutants was further verified using ESR characterization (Fig. S4d†).
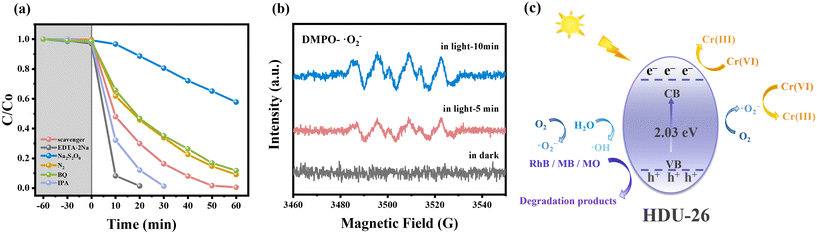 |
| Fig. 6 (a) Effects of different free radical scavengers on the photocatalytic reduction of Cr(VI). (b) ESR spectrum by DMPO spin-trapping for ·O2−. (c) The possible mechanisms of photocatalytic reduction of Cr(VI) and synergistic removal of organic pollutants by HDU-26. | |
Based on the above experimental analyses, Fig. 6(c) showed the possible mechanisms of photocatalytic reduction of Cr(VI) and synergistic removal of organic pollutants by HDU-26. The CB potential of HDU-26 was −1.15 V, which was more negative than the reduction potential of the Cr2O72−/Cr3+ couple (Eθ(Cr2O72−/Cr3+) = +1.33 V).39 From a thermodynamic point of view, the photoreduction of Cr(VI) to Cr(III) was possible due to the reducing properties of e−. On the one hand, the separation of e−–h+ occurred after visible light irradiation, and the e− attached to the surface of HDU-26 reduced Cr(VI) to Cr(III). On the other hand, e− can reduce O2 in the system to ·O2− (Eθ(O2/·O2−) = −0.33 V), which further accelerated the photocatalytic reduction of Cr(VI).45,55,56 Besides, h+ was presumably consumed by organic pollutants, which promoted the effective separation of e−–h+ and inhibited the reoxidation of Cr(III). Consequently, the photocatalytic reduction of Cr(VI) by HDU-26 exhibited excellent performance with the addition of RhB, MB or MO.
4. Conclusion
The successful preparation of a novel visible-light responsive D–A type covalent organic framework (HDU-26) was demonstrated by analysis using various characterization techniques. Electron-deficient benzothiadiazole acted as an electron acceptor, and electron-rich thiophene acted as an electron donor, effectively narrowing the band gap and suppressing e−–h+ complexation. It was characterized in various forms, showing excellent crystallinity, favorable stability, and high specific surface area. The optimum conditions for the photocatalytic reduction of Cr(VI) were tested by varying the initial pH, catalyst dosage, and Cr(VI) concentration. After 60 min of exposure to visible light at pH = 3.0, the reduction efficiency of Cr(VI) (50 mL 10 mg L−1) by 8 mg HDU-26 was 99.5%. The Cr(VI) removal rate was still up to 97.4%, with the crystal structure and morphology of HDU-26 undamaged after five reaction cycles. The major active species for the photocatalytic reduction of Cr(VI) were e− and ·O2− radicals. Besides, the photocatalytic rate of HDU-26 was significantly increased due to the synergistic effect in the mixed system of Cr(VI)/organic pollutants (RhB, MB, MO). Consequently, HDU-26 exhibits significant potential for eliminating organic pollutants and heavy metal ions from contaminated wastewater, owing to its abundant active sites and broad visible light absorption capacity.
Author contributions
Wen Lu: conceptualization, investigation, formal analysis, writing – original draft. Chen Wang: resources, project administration. Yuxuan Bai: software. Chengde Xie, Zhixiong Zhang, Wenhui Song: data curation. Jianjun Wang: supervision, funding acquisition, writing – review and editing.
Conflicts of interest
There are no conflicts to declare.
Acknowledgements
This work was financially supported by the National Natural Science Foundation of China (22376058).
References
- F. Yang, Y. D. Guo, C. X. Li, D. D. Tang, H. Jiang, G. H. Wang and K. Xuan, Facile fabrication of AgFe1-xCuxO2 composite with abundant oxygen vacancies for boosted photocatalytic Cr (VI) reduction and organic pollutants degradation under visible light, Colloids Surf., A, 2021, 628, 127305 CrossRef CAS
.
- Y. C. Chen, Y. M. Zhang, Y. H. Yuan, H. X. Li, K. Dong and X. M. Li, Progress Research on Treatment Methods of Printing and Dyeing Wastewater, IOP Conf. Ser. Earth Environ. Sci., 2019, 330, 032073 CrossRef
.
- Z. C. Liu, T. A. Khan, M. A. Islam and U. Tabrez, A review on the treatment of dyes in printing and dyeing wastewater by plant biomass carbon, Bioresour. Technol., 2022, 354, 127168 CrossRef CAS
.
- F. Mushtaq, X. Z. Chen, A. Veciana, M. Hoop, B. J. Nelson and S. Pane, Magnetoelectric reduction of chromium(VI) to chromium(III), Appl. Mater. Today, 2022, 26, 101339 CrossRef
.
- J. C. Wang, J. Ren, H. C. Yao, L. Zhang, J. S. Wang, S. Q. Zang, L. F. Han and Z. J. Li, Synergistic photocatalysis of Cr(VI) reduction and 4-Chlorophenol degradation over hydroxylated alpha-Fe2O3 under visible light irradiation, J. Hazard. Mater., 2016, 311, 11–19 CrossRef CAS
.
- X. D. Xie, J. X. Li, J. Liu, Z. Chen, X. Q. Yuan, T. Chen and L. Luo, Efficient degradation of chromium picolinate with simultaneous chromium removal from aqueous solutions using the Fenton process, Sep. Purif. Technol., 2023, 317, 123864 CrossRef CAS
.
- S. Luo, F. Qin, Y. Ming, H. P. Zhao, Y. L. Liu and R. Chen, Fabrication uniform hollow Bi2S3 nanospheres via Kirkendall effect for photocatalytic reduction of Cr(VI) in electroplating industry wastewater, J. Hazard. Mater., 2017, 340, 253–262 CrossRef CAS PubMed
.
- A. Bakshi, H. Bustamante, X. Sui and R. Joshi, Structure Dependent Water Transport in Membranes Based on Two-Dimensional Materials, Ind. Eng. Chem. Res., 2021, 60, 10917–10959 CrossRef CAS
.
- B. Verma and C. Balomajumder, Hexavalent Chromium Reduction from Real Electroplating Wastewater by Chemical Precipitation, Bull. Chem. Soc. Ethiop., 2020, 34, 67–74 CrossRef CAS
.
- S. Senguttuvan, P. Senthilkumar, V. Janaki and S. Kamala-Kannan, Significance of conducting polyaniline based composites for the removal of dyes and heavy metals from aqueous solution and wastewaters - A review, Chemosphere, 2021, 267, 129201 CrossRef CAS PubMed
.
- P. Singh and A. Borthakur, A review on biodegradation and photocatalytic degradation of organic pollutants: A bibliometric and comparative analysis, J. Cleaner Prod., 2018, 196, 1669–1680 CrossRef CAS
.
- K. Kaur and R. Jindal, Synergistic effect of organic-inorganic hybrid nanocomposite ion exchanger on photocatalytic degradation of Rhodamine-B dye and heavy metal ion removal from industrial effluents, J. Environ. Chem. Eng., 2018, 6, 7091–7101 CrossRef CAS
.
- S. Y. Hu, Y. N. Sun, Z. W. Feng, F. O. Wang and Y. K. Lv, Design and construction strategies to improve covalent organic frameworks photocatalyst's performance for degradation of organic pollutants, Chemosphere, 2022, 286, 131646 CrossRef CAS PubMed
.
- J. Yang, F. Y. Kang, X. Wang and Q. C. Zhang, Design strategies for improving the crystallinity of covalent organic frameworks and conjugated polymers: a review, Mater. Horiz., 2022, 9, 121–146 RSC
.
- J. L. Shi, R. F. Chen, H. M. Hao, C. Wang and X. J. Lang, 2D sp(2) Carbon-Conjugated Porphyrin Covalent Organic Framework for Cooperative Photocatalysis with TEMPO, Angew. Chem., Int. Ed., 2020, 59, 9088–9093 CrossRef CAS PubMed
.
- C. S. Mo, M. J. Yang, F. S. Sun, J. H. Jian, L. F. Zhong, Z. S. Fang, J. S. Feng and D. S. Yu, Alkene-Linked Covalent Organic Frameworks Boosting Photocatalytic Hydrogen Evolution by Efficient Charge Separation and Transfer in the Presence of Sacrificial Electron Donors, Adv. Sci., 2020, 7, 1902988 CrossRef CAS PubMed
.
- T. Sick, A. G. Hufnagel, J. Kampmann, I. Kondofersky, M. Calik, J. M. Rotter, A. Evans, M. Doblinger, S. Herbert, K. Peters, D. Bohm, P. Knochel, D. D. Medina, D. Fattakhova-Rohlfing and T. Bein, Oriented Films of Conjugated 2D Covalent Organic Frameworks as Photocathodes for Water Splitting, J. Am. Chem. Soc., 2018, 140, 2085–2092 CrossRef CAS PubMed
.
- W. Y. Geng, X. X. Lu, H. Zhang, Y. H. Luo, Z. X. Wang, S. F. Guo, Z. Y. Zhou and D. E. Zhang, Effective design and synthesis of donor-acceptor covalent triazine polymers with boosted photocatalytic performance for Cr(VI) reduction, Sep. Purif. Technol., 2022, 290, 120829 CrossRef CAS
.
- Z. P. Li, T. Q. Deng, S. Ma, Z. W. Zhang, G. Wu, J. A. Wang, Q. Z. Li, H. Xia, S. W. Yang and X. M. Liu, Three-Component Donor-π-Acceptor Covalent-Organic Frameworks for Boosting Photocatalytic Hydrogen Evolution, J. Am. Chem. Soc., 2023, 145, 8364–8374 CAS
.
- S. Kumar, M. A. Abdulhamid, A. D. D. Wonanke, M. A. Addicoat and G. Szekely, Norbornane-based covalent organic frameworks for gas separation, Nanoscale, 2022, 14, 2475–2481 RSC
.
- S. X. Li, R. Ma, S. Q. Xu, T. Y. Zheng, H. P. Wang, G. E. Fu, H. Y. Yang, Y. Hou, Z. Q. Liao, B. Z. Wu, X. L. Feng, L. Z. Wu, X. B. Li and T. Zhang, Two-Dimensional Benzobisthiazole-Vinylene-Linked Covalent Organic Frameworks Outperform One-Dimensional Counterparts in Photocatalysis, ACS Catal., 2023, 13, 1089–1096 CrossRef CAS
.
- D. G. Wang, T. J. Qiu, W. H. Guo, Z. B. Liang, H. Tabassum, D. G. Xia and R. Q. Zou, Covalent organic framework-based materials for energy applications, Energy Environ. Sci., 2021, 14, 688–728 RSC
.
- X. Y. Yang, Y. C. Jin, B. Q. Yu, L. Gong, W. B. Liu, X. L. Liu, X. Chen, K. Wang and J. Z. Jiang, Two-dimensional conjugated N-rich covalent organic frameworks for superior sodium storage, Sci. China: Chem., 2022, 65, 1291–1298 CrossRef CAS
.
- S. K. Das, S. Roy, A. Das, A. Chowdhury, N. Chatterjee and A. Bhaumik, A conjugated 2D covalent organic framework as a drug delivery vehicle towards triple negative breast cancer malignancy, Nanoscale Adv., 2022, 4, 2313–2320 RSC
.
- B. W. Ma, Y. M. Xu, F. J. Hu, L. P. Zhai, Y. Y. Huang, H. J. Qiao, J. B. Xiong, D. H. Yang, Z. H. Ni, X. F. Zheng and L. W. Mi, Fluorinated covalent organic frameworks for efficient drug delivery, RSC Adv., 2022, 12, 31276–31281 RSC
.
- H. Z. Lv, X. L. Zhao, H. Y. Niu, S. J. He, Z. Tang, F. C. Wu and J. P. Giesy, Ball milling synthesis of covalent organic framework as a highly active photocatalyst for degradation of organic contaminants, J. Hazard. Mater., 2019, 369, 494–502 CrossRef CAS PubMed
.
- B. C. Luo, Y. Chen, Y. B. Zhang and J. Q. Huo, Benzotrithiophene and triphenylamine based covalent organic frameworks as heterogeneous photocatalysts for benzimidazole synthesis, J. Catal., 2021, 402, 52–60 CrossRef CAS
.
- S. J. He, B. Yin, H. Y. Niu and Y. Q. Cai, Targeted synthesis of visible-light-driven covalent organic framework photocatalyst via molecular design and precise construction, Appl. Catal., B, 2018, 239, 147–153 CrossRef CAS
.
- C. X. Lin, X. L. Liu, B. Q. Yu, C. Z. Han, L. Gong, C. M. Wang, Y. Gao, Y. Z. Bian and J. Z. Jiang, Rational Modification of Two-Dimensional Donor-Acceptor Covalent Organic Frameworks for Enhanced Visible Light Photocatalytic Activity, ACS Appl. Mater. Interfaces, 2021, 13, 27041–27048 CrossRef CAS PubMed
.
- J. Q. Pan, L. P. Guo, S. Q. Zhang, N. Wang, S. B. Jin and B. Tan, Embedding Carbon Nitride into a Covalent Organic Framework with Enhanced Photocatalysis Performance, Chem. – Asian J., 2018, 13, 1674–1677 CrossRef CAS PubMed
.
- Y. Tao, R. Krishna, L. X. Yang, Y. L. Fan, L. Wang, Z. Gao, J. B. Xiong, L. J. Sun and F. Luo, Enhancing C2H2/C2H4 separation by incorporating low-content sodium in covalent organic frameworks, Inorg. Chem. Front., 2019, 6, 2921–2926 RSC
.
- B. Q. Liu, H. Guo, L. Sun, Z. L. Pan, L. P. Peng, M. Y. Wang, N. Wu, Y. Chen, X. Q. Wei and W. Yang, Electrochemical sensor based on covalent organic frameworks/MWCNT for simultaneous detection of catechol and hydroquinone, Colloids Surf., A, 2022, 639, 128335 CrossRef CAS
.
- Y. F. Zhang, M. Y. Zhu, S. Zhang, Y. W. Cai, Z. M. Lv, M. Fang, X. L. Tan and X. K. Wang, Highly efficient removal of U(VI) by the photoreduction of SnO2/CdCO3/CdS nanocomposite under visible light irradiation, Appl. Catal., B, 2020, 279, 119390 CrossRef CAS
.
- Y. W. Cai, Y. F. Zhang, Z. M. Lv, S. Zhang, F. X. Gao, M. Fang, M. G. Kong, P. S. Liu, X. L. Tan, B. W. Hu and X. K. Wang, Highly efficient uranium extraction by a piezo catalytic reduction-oxidation process, Appl. Catal., B, 2022, 310, 121343 CrossRef CAS
.
- W. B. Chen, Z. F. Yang, Z. Xie, Y. S. Li, X. Yu, F. L. Lu and L. Chen, Benzothiadiazole functionalized D-A type covalent organic frameworks for effective photocatalytic reduction of aqueous chromium(VI), J. Mater. Chem. A, 2019, 7, 998–1004 RSC
.
- W. Y. Geng, F. Y. Chen, Y. H. Luo, Z. Y. Liu, S. F. Guo, Y. Y. Zhang, D. E. Zhang and X. Y. Yu, Boosting photocatalytic Cr(VI) reduction activities of layered COF through regulating donor-acceptor units and the orientation of imine bonds, Microporous Mesoporous Mater., 2023, 351, 112479 CrossRef CAS
.
- T. Ge, L. Shen, J. Li, Y. C. Zhang and Y. Zhang, Morphology-controlled hydrothermal synthesis and photocatalytic Cr(VI) reduction properties of α-Fe2O3, Colloids Surf., A, 2022, 635, 128069 CrossRef CAS
.
- M. Chen, C. S. Guo, S. Hou, J. P. Lv, Y. Zhang, H. Zhang and J. Xu, A novel Z-scheme AgBr/P-g-C3N4 heterojunction photocatalyst: Excellent photocatalytic performance and photocatalytic mechanism for ephedrine degradation, Appl. Catal., B, 2020, 266, 118614 CrossRef CAS
.
- F. Zhang, Y. C. Zhang, Y. Y. Wang, A. P. Zhu and Y. Zhang, Efficient photocatalytic reduction of aqueous Cr (VI) by Zr4+ doped and polyaniline coupled SnS2 nanoflakes, Sep. Purif. Technol., 2022, 283, 12016 Search PubMed
.
- L. H. Sun, S. Y. Dong, S. L. Chen, H. Chen, M. S. Luan and T. L. Huang, Efficient reduction of hexavalent chromium by nubbly mesoporous NH2-MIL-125(Ti)/SnS2 Z-scheme heterostructures with enhanced visible photocatalytic activity, J. Environ. Chem. Eng., 2023, 11, 110192 CrossRef CAS
.
- Y. X. Liu, D. Z. Yang, T. Xu, Y. Z. Shi, L. N. Song and Z. Z. Yu, Continuous photocatalytic removal of chromium (VI) with structurally stable and porous Ag/Ag3PO4/reduced graphene oxide microspheres, Chem. Eng. J., 2020, 379, 122200 CrossRef CAS
.
- W. Lu, C. Wang, Y. A. Han, Y. X. Bai, S. Y. Wang, W. K. Xi and J. J. Wang, An active two-dimensional covalent organic framework for persulfate-assisted high efficiency photocatalytic degradation of rhodamine B, Appl. Catal., A, 2022, 647, 118907 CrossRef CAS
.
- R. Lakra, M. S. Kiran and P. S. Korrapati, Furfural mediated synthesis of silver nanoparticles for photocatalytic reduction of hexavalent chromium, Environ. Technol. Innovation, 2021, 21, 101348 CrossRef CAS
.
- Q. Zhuang, H. Chen, C. F. Zhang, S. Y. Cheng, W. Dong and A. Xie, Rapid chromium reduction by metal-free organic polymer photocatalysis via molecular engineering, J. Hazard. Mater., 2022, 434, 128938 CrossRef CAS PubMed
.
- S. C. Zhong, Y. Wang, S. X. Li, S. Wang, X. Y. Que, L. Sheng, J. Peng, L. Zhao, L. Y. Yuan and M. L. Zhai, Enhanced photo-reduction of chromium(VI) from aqueous solution by nanosheet hybrids of covalent organic framework and graphene-phase carbon nitride, Sep. Purif. Technol., 2022, 294, 121204 CrossRef CAS
.
- N. Qin, A. J. Mao, L. Q. Li, X. B. Yang, J. Liu, K. Y. Chen, L. P. Zhai, R. W. Liang and L. W. Mi, Construction of benzothiadiazole-based D-A covalent organic frameworks for photocatalytic reduction of Cr (VI) and synergistic elimination of organic pollutants, Polymer, 2022, 262, 125483 CrossRef CAS
.
- F. Y. Liu, Z. Y. Ma, Y. C. Deng, M. Wang, P. Zhou, W. Liu, S. J. Guo, M. P. Tong and D. Ma, Tunable Covalent Organic Frameworks with Different Heterocyclic Nitrogen Locations for Efficient Cr(VI) Reduction, Escherichia coli Disinfection, and Paracetamol Degradation under Visible-Light Irradiation, Environ. Sci. Technol., 2021, 55, 5371–5381 CrossRef CAS PubMed
.
- Y. Zhang, Z. Chen, Z. X. Shi, T. T. Lu, D. L. Chen, Q. Wang and Z. L. Zhan, A direct Z-scheme BiOBr/TzDa COF heterojunction photocatalyst with enhanced performance on visible-light driven removal of organic dye and Cr(VI), Sep. Purif. Technol., 2021, 275, 119216 CrossRef CAS
.
- X. H. Xu, W. Huang, X. D. Li, Y. Sui, W. T. Chen, Y. T. Li, H. X. Ye, C. W. Pan, H. Zhong and M. C. Wen, Triphenylamine-based covalent triazine framework @ carbon nanotube complex: efficient photogenerated charges migration for metal free photocatalytic Cr(VI) reduction, J. Environ. Chem. Eng., 2023, 11, 109331 CrossRef CAS
.
- S. Kumar and D. M. Parks, Strain Shielding from Mechanically Activated Covalent Bond Formation during Nanoindentation of Graphene Delays the Onset of Failure, Nano Lett., 2015, 15, 1503–1510 CrossRef CAS PubMed
.
- L. J. Shen, S. J. Liang, W. M. Wu, R. W. Liang and L. Wu, Multifunctional NH2-mediated zirconium metal-organic framework as an efficient visible-light-driven photocatalyst for selective oxidation of alcohols and reduction of aqueous Cr(VI), Dalton Trans., 2013, 42, 13649–13657 RSC
.
- A. D. Xie, M. G. Hu, Y. H. Luo, X. G. Zhu, Z. H. Wang, W. Y. Geng, H. Zhang, D. E. Zhang and H. Zhang, Synthesis of a stable iron(III)-organic framework for a visible light induced simultaneous photocatalytic reduction of Cr(VI) and the degradation of organic dyes in water, New J. Chem., 2021, 45, 13406–13414 RSC
.
- R. W. Liang, L. J. Shen, F. F. Jing, W. M. Wu, N. Qin, R. Lin and L. Wu, NH2-mediated indium metal-organic framework as a novel visible-light-driven photocatalyst for reduction of the aqueous Cr(VI), Appl. Catal., B, 2015, 162, 245–251 CrossRef CAS
.
- X. Wang, Y. X. Li, X. H. Yi, C. Zhao, P. Wang, J. G. Deng and C. C. Wang, Photocatalytic Cr(VI) elimination over BUC-21/N-K2Ti4O9 composites: Big differences in performance resulting from small differences in composition, Chin. J. Catal., 2021, 42, 259–270 CrossRef CAS
.
- F. Zhang, Y. C. Zhang, G. S. Zhang, Z. J. Yang, D. D. Dionysiou and A. P. Zhu, Exceptional synergistic enhancement of the photocatalytic activity of SnS2 by coupling with polyaniline and N-doped reduced graphene oxide, Appl. Catal., B, 2018, 236, 53–63 CrossRef CAS
.
- C. Wang, W. Lu, W. H. Song, Z. X. Zhang, C. D. Xie, Z. Y. Ji, Y. Li and J. J. Wang, Dual application of a cyano-containing covalent organic framework: Photocatalytic degradation of dyes with fluorescence detection studies, Appl. Catal., A, 2023, 666, 119433 CrossRef CAS
.
|
This journal is © The Royal Society of Chemistry 2024 |
Click here to see how this site uses Cookies. View our privacy policy here.