Zero-dimensional luminescent carbon dots as fascinating analytical tools for the treatment of pharmaceutical based contaminants in aqueous media
Received
30th March 2023
, Accepted 18th October 2023
First published on 25th October 2023
Abstract
Carbon dots (CDs), a fascinating category of nanomaterials, have sparked into an intense area of research among researchers, particularly in the diverse applications of environmental remediation ascribed to their inimitable features, including excellent biocompatibility, photostability, physicochemical attributes, and low toxicity. Herein, we have reviewed the most recent research on the environmental implications of pharmaceutical contaminants, conventional treatment techniques, and the application of CDs for the extraction and degradation of pharmaceutics in wastewater. Beyond presenting these features, the photocatalytic applications of CDs for the decomposition of pharmaceutical pollutants, along with pertinent structures and their probable effects on photocatalytic activity, have also been the topic of discussion in this review. Finally, challenges, future prospects, and typical limitations of CD technologies are addressed along with potential future directions for environmental remediation. Consequently, this study offers a succinct overview of the most recent advancements and difficulties in the field of CDs, particularly for researchers working on the degradation of pharmaceutical pollutants.
Water impact
One of the most important resources on Earth is water. Even though it is precious and in great demand, the contamination of the water that humans need to survive is a growing concern since it could lead to a shortage of water that is fit for human use. Industrial waste, organic contaminants, and heavy metals are prominent sources of water contamination. Extended exposure to pharmaceuticals poses a serious risk to human health because it could result in the unintentional development of resistance to the therapeutic effect of a particular molecule. For example, in the case of antibiotics, following ingestion, while some of the substance is processed by the human body, the majority is expelled through urine and feces, going mostly intact into home sewage systems. Antibiotics are now almost invariably found in aquatic habitats as a result of their frequent high-water solubility and inadequate regulatory standards. This is regarded as one of the primary channels through which antibiotic resistance spreads in European wastewater treatment facilities. Therefore, studies related to creating trustworthy techniques for monitoring pharmaceutics are hotspots across the globe. It has been suggested that carbon dots (C-dots), which have outstanding biocompatibility, strong photostability, and low toxicity, could replace other materials in remediating the pharmaceutics from water bodies.
|
1. Introduction
One of the most important natural resources on earth is water. Even though it is precious and in great demand, the contamination of water that humans need to survive is a growing concern since it could lead to a shortage of water that is fit for human use.1–3 Industrial waste, organic contaminants, and heavy metals are prominent sources of water contamination. Extended exposure to pharmaceuticals leads to a serious risk to human health as it could result in the unintentional development of resistance to the therapeutic effect of a particular molecule.4–6 For instance, in the case of antibiotics, following ingestion, while some of the substance is processed by the human body, the majority is expelled through urine and feces, going mostly intact into home sewage systems.7,8 Antibiotics are now almost invariably found in aquatic habitats as a result of their frequent high-water solubility9 and inadequate regulatory standards. This is regarded as one of the primary channels through which antibiotic resistance spreads in European wastewater treatment facilities.10,11
Activated sludge and adsorption are two examples of conventional treatment methods used for customary contaminant cleaning.12,13 However, current methods are insufficient to eliminate pharmacological compounds entirely.14 As a result, new methods and materials have to be created in order to properly degrade these growing contaminants. There is a variety of materials that can be employed for the aforesaid issue. For example, metal oxide semiconductors (e.g., ZnO, TiO2), metal–organic frameworks (e.g., MIL-100, MIL-125), and noble metals (e.g., Pt, Au, Ag) have been reported.15 Additionally, carbon nanoparticles have been the subject of study.16 The most commonly used carbon-based materials for water purification are CNTs and graphene derivatives. For instance, Li and his team documented a review article summarizing the graphene-based materials for pharmaceutical waste treatment. CDs are a novel form of carbon nanomaterials that have recently attracted considerable attention. They are being preferred over conventional options owing to their excellent up-conversion efficiencies, biocompatibility, chemical inertness, less toxicity, enhanced photoluminescence tunability, water dispersibility, facile synthesis, outstanding ability to separate holes and electrons, production from sustainable raw materials, and improved photoinduced electron capabilities. As compared to other options, research on CDs is in a nascent stage. Scalable and systematic production with tailorable structures is the need of the hour. Also, there is a lack of understanding of nucleation and reaction mechanisms. Purification schemes should have been introduced. Moreover, in situ techniques can help in the characterization of synthesis mechanisms. At the same time, the poor stability also poses a challenge, i.e., CDs are unable to maintain the attributes for extended periods.17 Against this backdrop, CDs are modified through doping, surface modification, and creating CD-based hybrid structures. For instance, surface passivation is utilized to enhance the absorption properties of CDs. It is achieved by treating the CDs with acids and interacting with the insulating layers. Likewise, doping is another approach to enhance the performance of the CDs. In addition to that, composites of CDs with metal–organic frameworks and metal oxides (e.g., MnO2) have also been reported.18 A few reports are available on CD-based materials for applications as a photocatalyst for pharmaceutical wastewater treatment. There is need to document the related literature in this regard. In addition to the aforementioned candidates, nanocomposites comprising combination of above materials are also being used for the decontamination of the pharmaceutical based waste water.19–23 Schematic illustration of the classification and properties of different useful decontaminates are shown in Fig. 1.
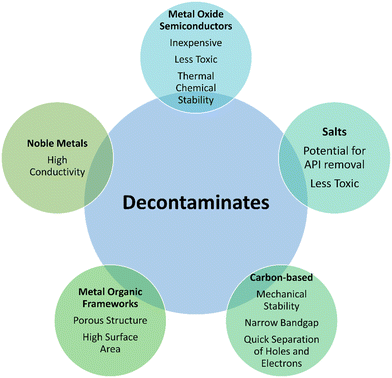 |
| Fig. 1 Schematic representation of different decontaminates and their properties. | |
The main objective of this study is to pinpoint the entry points by which pharmaceuticals end up in the natural world. Next, we will look at how CDs are being used now and in the future to clean pharmaceuticals. CD-made materials are reviewed, with an emphasis on their main characteristics and how they affect remedial measures of pollutant removal capacity. CDs are examined for their potential use in cleaning up pharmaceutical pollution, and modern problems with drug storage and distribution are discussed. Fig. 2 represents the overall scope of the present study.
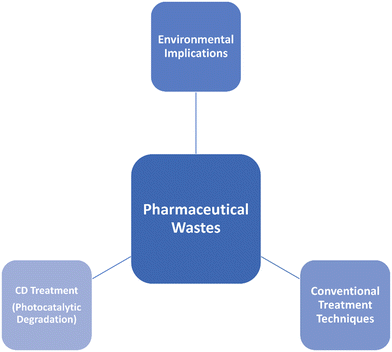 |
| Fig. 2 Pharmaceutical wastes, their environmental implications, and probable solutions. | |
2. Environmental implications of pharmaceutical contaminants
2.1. Pharmaceutical contaminants
Pharmaceutics is a class of chemical substances that have been developed for use as a therapeutic treatment for ailments and have improved human lifespan and health. The huge increase in life expectancy after 1940 can be linked to pharmaceutical advances such as streptomycin, penicillin, and vaccines.24 Access to advanced, inexpensive, effective, and safe medications for the worldwide population is also important; as a result, pharmaceutical corporations and governments are working on measures to make medicines more accessible and affordable to everyone.25 The pharmaceutical business is one of the world's highest-income industries due to its relevance and undeniable advantages.26 The worldwide pharmaceutical production market accounted for US$ 324.42 billion in 2019, accompanied by a compound annual growth rate (CAGR) of 13.74% projected up to 2027.27 Likewise, since 2014, worldwide pharmaceutical consumption has a CAGR of 3%.28 Consequently, the rising pharmaceutical business has become a global public health issue, as increased sales and usage of medicines imply more chemicals are added to the environment.29–32 Pharmaceutical compounds have been discovered in nearly every aquatic environment on the planet, including groundwater and surface water.33–35 Around 4000 distinct pharmaceutical active ingredients (PAIs) are manufactured globally and used in thousands or even millions of pharmaceutical formulations.36,37 Moreover, not just the final product would generate waste during the production process of PAIs; other liquids and materials can also become contaminated by this increasing amount of waste.9 Biological or chemical pharmaceutical materials for veterinary and human medical purposes, either excreted or unused by the animal body or humans; wastes or residues from production processes introduced in either of those of the commonly occurring forms such as liquids, solids, or gases, as well as solvents used for purification or a chemical reaction and the unused medicinal supplies that come in interactions with pharmacological stuff, such as vials, bottles, and bags, are regarded as pharmaceutical pollutants.31 Antibiotics, among a wide range of medicinal drugs, pose a significant environmental threat to terrestrial and aquatic ecosystems.38 Antibiotics have proved to be effective therapeutics for treating diseases in animals and humans for many years.34 Antibiotics have garnered more consideration recently as a result of their ecological consequences once they enter the environment. Antimicrobial resistance (AMR) is an evolutionary pressure that favors the aesthetic of resistant pathogens due to the gross negligence and inappropriate discretion of antibiotics employed for therapeutic tenacities, as well as their exorbitant use on livestock to improve feed and growth efficacy.34,38 In this perspective, AMR is presently cited as a significant worldwide health hazard by the WHO.39 Antibiotics are perhaps the most commonly prescribed medications worldwide, and their use is on the rise as a result of increased availability and affordability.40 Antibiotic usage climbed by 91% between 2000 and 2015 in the underdeveloped nations.40 Antimicrobial intake is higher in livestock than in humans.38,40,41 Antibiotics are utilized on livestock in various developed countries, accounting for 50 to 80% of global antibiotic production. Animals alone utilized 63
151 tons of antimicrobials globally in 2010, with that number expected to rise to 105
596 tons by 2030.42
2.2. Pathways and sources of pharmaceutical contaminants
Pharmaceutical contaminants can enter the environment through four main routes: (i) disposal of wastewater from production plants of pharmaceutical industries; (ii) hospital and domestic run-offs comprising pharmaceutics defecated from the feces and urine of humans; (iii) agronomic pollutants encompassing pharmaceutics found in the feces and urine of animals; (iv) improperly disposed of expired or unused pharmaceutics via the sewage system.38,43–46 Other routes have been documented, including the direct administration of aquatic medicinal compounds for agricultural and aquaculture wastewater irrigation.43,45,47 The illustration of how pharmaceutically active compounds enter the environment is portrayed in Fig. 3.
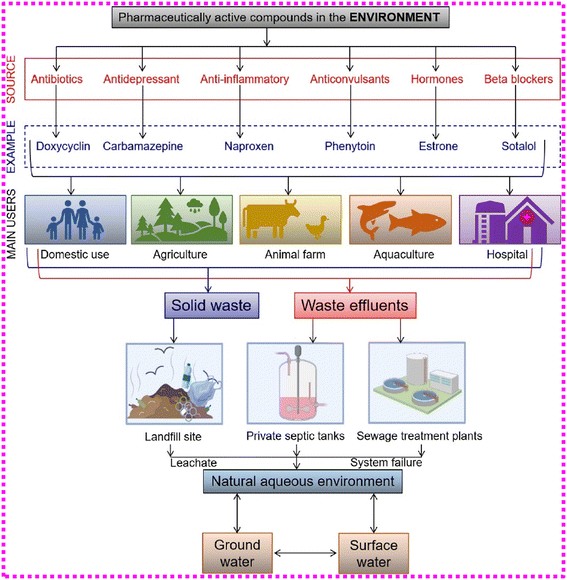 |
| Fig. 3 Pharmaceutically active entities and their transformation routes to our water supplies and environment. Reproduced with permission from ref. 47. Copyright 2020, Elsevier Inc. | |
2.3. Pharmaceutical contamination throughout a pharmaceutical product's lifecycle
Production, consumption, and sewage disposal are the three life cycle stages of pharmaceutical-based pollutants. Each phase of the process is a critical pathway for pharmaceutical contaminants to enter the environment.48 There are three basic processes in the production of medicinal products: 1) PAI manufacture, which can be accomplished through natural product extraction or chemical synthesis; R&D; 2) research and developments; and 3) composition of the finalized medicinal products, which involves combining the PAI with active ingredients and additives.49 Microbiological, chemical, and pharmacological research are all part of the pharmaceutical industry's research and development. As a result, biological and chemical wastes are created, with oxidizers, halogenated and non-halogenated solvents, and bases being the most frequent producers.49 The amount of waste produced during the chemical production of PAIs is determined by the pharmaceutical procedure utilized and the variety of phases concerning reactions of chemical species. The synthesis of different chemical products involves a variety of chemical reactions, washing and separation of the final product by using filtration and extraction, etc. All this processing needs extra solvents, which are finally disposed of in the form of waste liquid. Furthermore, sometimes recrystallization and centrifugation are also carried out for the purification of the final products. These subsequent purification steps also require solvents for their completion and ultimately become the participants in the disposal of more and more hazardous solvents in the environment.50,51 Therefore, we can conclude that all the processes employed for the manufacture of PAIs are the sources of releasing vapor solvents, harmful liquids in the form of organic or inorganic substances, and catalysts.49,51 Natural product extraction techniques are used to make pharmaceutical medicines from natural materials. Extractive medications are expensive and complex to manufacture commercially. Further, the synthesis of natural products is less common in comparison to their extraction.52 Therefore, the waste products from natural extraction procedures include raw materials, such as cleaning and detergent antiseptics, solvent vapors, and water-soluble solvents,.49,52 Because extracted PAIs are often available at extremely small concentrations in raw materials, the wastes' mass is nearly equivalent to the original weight of the raw materials.52 The final medicine production process, on the other hand, is less harmful to the environment.51 The PAIs are transformed into a dosage form for easy customer use throughout this procedure, which commonly includes liquid formulations (syrups), uncompressed solids (capsules), and compressed solids (tablets), among others.51 In general, only minor volumes of polluted wastewater are utilized for equipment washing and cooling activities created during the final medication manufacture.52 For several years, pharmaceutical processing plants have dismissed environmental pollution as insignificant, based on the idea that the large values of pharmaceuticals preclude their discharge into the environment for economic purposes.53,54 Pharmaceutical pollution from manufacturing plants, on the other hand, has been seen and recognized as a substantial local source.55 Drug manufacturers in several regions of the world have improperly discharged production effluents, harming rivers and lakes;54,56–59 for example, high antibiotic percentages have been discovered in all samples taken from 28 distinct sampling areas in the region of drug production, as well as in the proximity of two rivers and treatment plants of wastewater. Correspondingly, two investigations56 found unusually high levels of pharmaceutical pollutants in samples taken from the plant operating in Patancheru, India, whose wastewater importing capacity is around 1500 m3 per day.54,56 These research studies show that drug manufacturing plants are the most significant emitters of pharmaceutical pollutants in specific geographic locations.60 Pharmaceuticals used in veterinary and human medicine are physiologically active substances that can be metabolically converted by enzyme systems in the body.61 These molecules have an intended impact on the body; nevertheless, the unmetabolized products' elimination and environmental implications are generally overlooked during production. Metabolic transformations are divided into two stages. Phase I consists of reduction, hydrolysis, and oxidation processes, which convert the medicinal merchandise into polar and water-soluble compounds. If the pharmaceutical substance is already moderately water-soluble, it can be excreted following this phase. Throughout phase II, conjugation processes are used to modify the phase I outputs or the unmodified medicinal product, making metabolites more readily excreted or destroyed enzymatically.45,62 The physicochemical features of the substance greatly affect the metabolism processes of a pharmaceutical, such as lipophilicity and molecular size, as well as biological aspects, including pharmacodynamics and ethnic differences.63 Generally, 30 to 90% of an orally administered dosage is not completely digested and excreted as an active agent, whether in people or animals.31,44 The excreted dose varies depending on the PAI: just a few pharmaceutical items have a balanced metabolism of 30 to 70%, whereas, the vast majority are either extensively metabolized or excreted unaltered.37 Pharmaceutical compounds are primarily excreted by urine, accounting for 55 to 80% of total excretion (on average 70%) and partially through feces (30% of total excretion).64 The inclusion of antibiotics in human urine65,66 and feces66 has been established in studies indicating partial metabolization of pharmaceutical drugs.67 Pharmaceuticals administered by topical or transdermal routes, on the other hand, can enter the environment through skin release, such as after bathing53 Animals, like people, do not entirely digest the medications they are given. Antibiotics were found in the excrement of animal samples taken from various species in China, according to Zhao and coworkers.68 Previous reports have widely presented the occurrence of a number of antibiotics in swine manure, animals, and poultry samples,69–73 the most widely cited being tetracycline, sulfonamides, and fluoroquinolones.74 Each phase of the manufacturing process of a medicine should be studied to minimize pharmaceutical pollution; nonetheless, the disposal procedure plays one of the most essential part in contamination of the environment.48 Domestic effluents, whether from garbage, or sink/toilet disposal, are the leading source of pharmaceutical pollution.43 A variety of studies in many countries have confirmed the findings. For example, Ahmed and coworkers75 studied the proper disposal for expired and unused pharmaceutics in Pakistan and discovered that only 2% of people are involved in properly disposing of their medicinal products by providing them to the community pharmacies, with the most communal disposal exercise (83%) being trying to discard in a routine garbage bin with other trash without any further handling. Other studies, such as those conducted in Ireland,76 Afghanistan,77 Bangladesh,78 and Brazil76 have shown that dumping leftover medications with regular waste is the most typical disposal technique.79 When the medicine composition is offered in a liquid form, however, consumers choose to dispose of it in the sink or toilet.80 Furthermore, Alnahas and coworkers81 reviewed the medicinal product disposing techniques in 34 realms and find that 72.7 ± 20.5% relates to environmentally harmful moieties as sewer and garbage removal. Although it is not recommended to dispose of medications in landfills, they have long been the main means of dumping for both industrial and municipal trash.82 If pharmaceuticals are disposed of in landfills, they may degrade, adsorb, or eventually seep into the groundwater.83 This was confirmed in investigations that identified pharmaceuticals in landfill effluent82 and on municipal solid waste dumps.57,82 As a result, several waste products are formed throughout the lifespan of a pharmacological product, including PAIs, oxidizers, solvents, leftover materials, and unrestricted dermatological products, which finally end up in the
atmosphere.
3. Conventional treatment techniques for medicinal wastes
The main sources to dispose of the medicinal wastes are: (i) incineration following “take-back” programs; (ii) landfilling following disposal in a standard rubbish can; and (iii) wastewater treatment plants (WWTPs) following draining into the sewage system.84 Attributes of these techniques are depicted in Fig. 4.
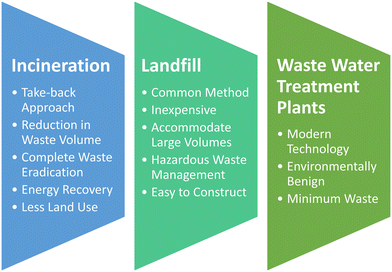 |
| Fig. 4 Attributes of conventional treatment techniques. | |
3.1. Incineration
Pharmaceutical substances must be carefully collected, classified, sorted, and disposed of to minimize their influence on the environment. In this way, effective pharmaceutical waste disposal starts with collection at “take-back” facilities, which are mostly carried out by pharmacies.85 These “take-back” outlets provide a link between customers and companies with the necessary capabilities for disposing of medications.85 Despite the fact that “take-back” tactics are unpopular when compared to unsustainable disposal procedures,75,78 considerable proportions of returned pharmaceuticals have been documented.86 Returned pharmaceuticals and pharmaceutical waste from production plants are ideally incinerated at high temperatures to reduce environmental contamination.85 Incineration is a thermal process that converts garbage into mineral and gaseous leftovers under controlled circumstances.87 Pharmaceuticals leftovers are necessarily incinerated at elevated temperatures, usually in two chambers with an arrangement having continuous passage of the air. The waste is received in the primary chamber, which operates with less air than needed to optimize combustion effectiveness and decrease emissions into the environment. Excess air is then fed to the chamber positioned at number 2, which operates at an elevated temperature, to accomplish the burning of the effluent gases from the first chamber.88 To effectively destroy pharmaceuticals, operational variables such as pretreatments, temperature and residence time as well as management of waste ashes must satisfy stringent emission control criteria. The incinerator should preferably operate at 1200 °C with a gas residence period of greater than 2 s.87 Furthermore, pollution control technologies are the most important gadgets. The incinerator operating at moderate temperature can be an option where the high temperature incinerators are not possible. To reduce emissions, pharmaceutical waste processed with this approach should be combined 1
:
1000 with municipal garbage, and halogenated chemicals might not surpass 1% of the total, to circumvent halogen in the combustion gases.31 In this way, compared to other approaches such as landfills, incinerator treatment offers several benefits such as reduction of large volume waste, complete waste eradication, energy recovery, and less land use.89 However, there are drawbacks as well. Incinerators can discharge acid gases, particle matter, and polynuclear organic matter, among other hazards, if they are not correctly constructed and operated. This was demonstrated in a Colombian study that assessed the quality of incinerator emissions and discovered total dioxin emission and suspended particle concentrations.90 Incinerators not only emit harmful fumes into the environment, but they also generate solid ash with high heavy metal concentrations.31 In this connection, Tufail and coworkers91 studied Zn, Pb, Cd, Cu, and Cr concentrations in ash samples collected from Pakistani incinerators. The greater costs connected with incinerator infrastructure transportation or operating costs were due to being located primarily in industrialized nations.92 As a result, incineration potential is insufficient, and many countries lack access to appropriate designs and operations. Incineration at higher temperatures with suitable emission control after “take-back” initiatives is the greatest choice for disposing of pharmaceutical waste from an environmental standpoint; unfortunately, many countries lack such incinerator infrastructure or have not established “take-back” programs.92,93 In addition, some pharmacies around the globe have banned or condemned the practice of discarding unwanted medications.94 These conditions encourage the use of environmentally unfriendly practices, such as dumping in a conventional waste bin with other ordinary garbage,94 which results in MSW that is then disposed of in landfills.
3.2. Landfilling
Landfilling is a process in which a piece of land is used for the dumping of garbage in order to prevent the interaction of hazardous pollutants to the environment, specifically water resources.95 Landfilling is categorized into three groups: 1) methodical landfilling is commonly used in unindustrialized nations, and their procedure focusses entirely on discarding the leftovers in open land; 2) there are some nominated locations known as semi-controlled landfills, wherever the discarded waste is compressed and a soil surface cover is decided to add on a regular basis; and 3) managed and well-constructed landfills termed as sanitary landfills with the ability to prevent and treat effluents, and they are primarily utilized in developed nations.31,95 Landfills are the primary destinations for waste from several countries,96–98 including China,99 Taiwan, and Poland. These countries dump their 90% of urban wastes into landfills.96,97 In addition, the landfill technique is utilized after other waste remedies are completed in order to reduce waste volume;100 for example, the remaining ash after incineration might be landfilled. The widespread usage of landfills, coupled with higher pharmaceutical usage and a strong tendency to discard them with routine compost, has resulted in high pharmaceutical levels in MSW. Pharmaceuticals have been found in MSW in amounts as high as 8.1 mg kg−1.83 When medications are abandoned in landfills, they may degrade, adsorb, or leak out through the leachate.31,83 Masoner and coworkers101 examined fresher leachate models taken from 19 landfilled sites, finding a diverse combination of pollutants such as animal/plant sterols and pharmaceuticals in amounts from 100 to 1
000
000 ng L−1. Correspondingly, several investigations have shown substantial pharmaceutical concentrations in various waste leachates.102,103 Despite the advent of the contemporary landfill process of acquiring and treating leachates,31,95 pharmaceutical removal rates following leachate treatments are restricted.104 Yu and coworkers98 have looked at the ecological consequences of a well-built and maintained landfill with sophisticated seepage protection and leachate assortment (Fig. 5). Their findings revealed a significant influence on neighboring fresh water, additionally, a pronounced influence of pharmaceutical leftovers in nearby surface water was also observed.98
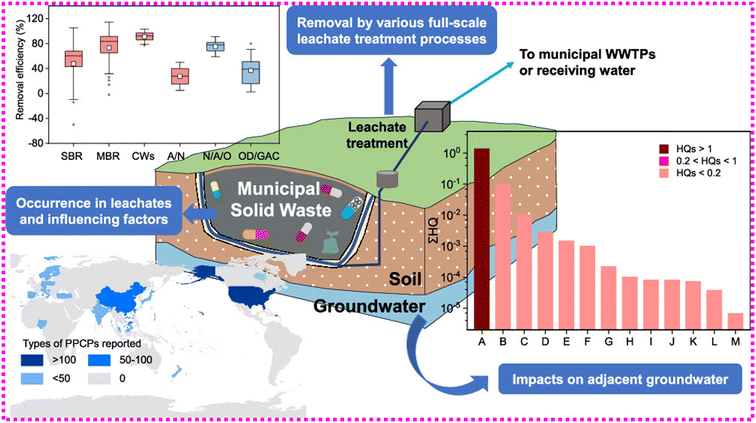 |
| Fig. 5 Illustration of a well-built and maintained municipal solid waste landfill with sophisticated seepage protection and leachate collection. Reproduced with permission from ref. 98. Copyright 2020, ACS Publications. | |
3.3. Wastewater treatment plants
Pharmaceutical leftover is typically discharged into the sewage system using the sink or toilet rather than being dumped into MSWs. Furthermore, pharmaceutical substances and their metabolites excreted by humans reach wastewater treatment plants (WWTPs), resulting in significant levels of pharmaceutical contaminants in their effluents. WWTPs are typically made up of a combination of physicochemical and biological treatments administered in three stages, such as, sedimentation, treatment, and final sedimentation.105,106 Adsorption, membrane filtration, biodegradation, chlorination, filtration, coagulation/flocculation and sedimentation, using a range of carbon-based materials, and UV-irradiation are some of the traditional wastewater treatment processes.107,108 These procedures are mostly successful in removing traditional pollutants such as nutrients and biodegradable organic materials; but even at lower concentrations, they are insufficient to remove pharmaceutical compounds.9,106 Some of the documented reports demonstrated that pharmaceutical products have been found in WWTP effluents and influents,105,106,109 as well as wastewater sludge,109 demonstrating that conventional WWTPs are unable to totally remediate the pollutants. This is important because pharmaceutical wastes are inappropriately dumped at practically every stage of a pharmaceutical product's lifespan and are not entirely eliminated. Moreover, overcapacity, overflows, and system failures on ageing infrastructures may lead sewage transportation and treatment systems to seep into the aquatic environment.37,110 The likelihood of subsurface leaks dramatically increases as infrastructure ages and the population grows.37 Additionally, the most common pharmaceutical wastage managed by each conventional organization has been documented. In conclusion, while incineration appears to be a successful approach for treating pharmaceutical wastage, landfill is common, and WWTPs are modern technology; none of the traditional approaches totally eradicate the environmental danger of pharmaceutical wastage.90 In this context, in addition to direct emissions, failure/inefficiency of these traditional procedures leads to pharmacological pollutants in water bodies. Therefore, innovative materials for water decontamination and remediation are necessary to mitigate environmental and health risks.
4. CDs assisted degradation of pharmaceutics in wastewater
4.1. Photocatalytic degradation
CDs, a unique class of carbon-based nanostructures with a quasi-spherical shape and size of below 10 nm, have been the topic of a growing number of scientific investigations owing to their intriguing attributes such as photoluminescence, water solubility, ease of surface modification, biocompatibility, optical stability, and their inexpensive and simple nature.111,112Fig. 6 gives a complete overview of the synthetic approaches, raw materials, and photocatalytic activities of CDs.
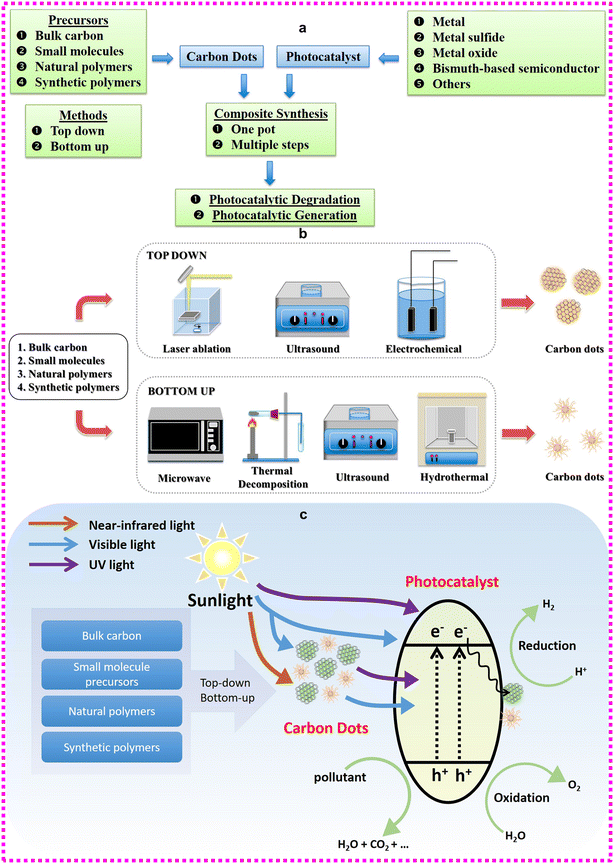 |
| Fig. 6 Illustration of the (a and b) raw materials and synthetic approaches for CDs. (c) Photocatalytic activity of CDs. Reproduced from ref. 112 an open access Creative Common CC BY license. | |
CDs have a carbon core that can be nanocrystalline, amorphous, or both and are divided into carbon quantum dots (CQDs), carbon nanodots (CNDs), and graphene quantum dots (GQDs), depending on their inherent structure. GQDs are single or multiple-layered crystalline nanomaterials accompanied by conjugated π-electron and a 0.24 nm crystal lattice. The GQDs also exhibit quantum confinement.113 The quantum confinement is also portrayed by the CQDs, though their crystal lattice has an interlayer difference of 0.34 nm, which is the same as graphite.114 On the other hand, the CNDs are a class of nanoparticles comprising crosslinked or aggregated linear polymers or monomers, which do not show any quantum internment.113 More classes can be identified because CDs have a complicated architecture with a variety of variations based on their synthetic methods and precursor.115
The properties of CDs can be tailored by varying the intrinsic structure. Quantum size, which is an important feature, depends upon the precursor ratio, temperature, nature of the solvent, and reaction time. The surface of CDs can be modified by employing a number of approaches, such as doping, functionalization, and surface passivation. The surface modification and size are of prime importance as they directly influence the energy gaps. Another feature that has a significant effect on the physiochemical attributes of CDs is chirality. It is worth mentioning that chirality can be transferred when CDs are combined with other molecules. Recently, computational models and the use of artificial intelligence have also proven helpful while designing the CDs. CDs are also being employed for the construction of nanocomposites. The tailorable bandgap helps in the fabrication of heterojunction through Z-scheme.116,117
It has been revealed by DFT calculations that the properties of CDs depend upon the preparation methods. Moreover, graphitic character plays a pivotal role in overall performance. Interestingly, the smaller size of CDs leads to outstanding properties, which cannot be realized through other materials. It is of great concern how efficiently DFT calculations are used to describe the mechanisms, study materials attributes, and provide proper interpretations. It took three stages for the development of DFT calculations for CDs, i.e., highlighting the experimental phenomenon, estimating the results of the experiment, and paving the path for experimental research. Though such studies have guided the interpretation of the experimental results, they lack guidance regarding the experimental phase. So, there is more reliance on the experiments rather than theoretical studies.118,119
CDs are promising prospects for photocatalysis because of their attractive characteristics, such as efficient electron transfer, photoluminescence, up-conversion photoluminescence capabilities, and a high capacity for sunlight absorption. Heterogeneous photocatalysis120 is defined as a method of conversion of energy that takes advantage of the synergistic effect of light and solid photocatalyst to boost reactions with the intention of engaging with reactants on a liquid surface (Fig. 7). Valence band (VB) electrons jump to higher energy state by the absorption of light energy in the photocatalytic process, which results in their movement to the conduction band (CB). This movement of electrons creates a positive hole. Further, the photogeneration creates free radicals in these charged bodies. These free radicals are used in the degradation of pharmaceutical contaminants, which converts them into H2O, CO2, and other degradation products.121 The light should be absorbed by these photocatalysts over a broad spectral range, and the generated charge carriers should effectively be separated during the process.
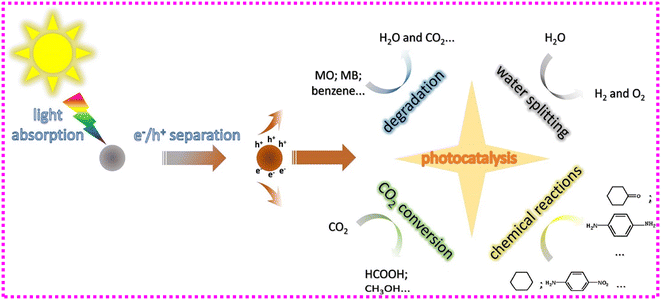 |
| Fig. 7 The photocatalytic process of CDs-based photocatalysts (the solid circles represent CDs-based photocatalysts). Reproduced with permission from ref. 120. Copyright 2018, Elsevier Inc. | |
Moreover, most of those commonly utilized conventional photocatalysts have yet to surmount their low photocatalytic accuracy and improved recombination probabilities from photo-generated charge carriers.120,122 Although CDs do not have inherent photocatalytic properties, their distinct optical and electronic characteristics, such as tunable bandgap, excellent light absorption, photoluminescence, up-conversion photoexcited electron transfer ability, and restriction of e/h+ recombination, play a key role in the formation of highly efficient CDs-based photocatalytic systems.123,124 In comparison to their corresponding pristine photocatalyst, the addition of CDs significantly enhances the degradation rate. The improved photocatalytic activity that leads to the accumulation of carbon dots is primarily ascribed to different pathways: 1) effective separation of e/h+ and 2) up-conversion character of CDs. Additionally, increased hydrophilicity and their photosensitizing ability by lowering the bandgap have been linked to the enhancement caused by CDs. In the case of the latter, graphitic carbon nitride (g-C3N4) (Fig. 8a) has a bandgap of 2.7 eV;125 however, the introduction of CDs narrowed the bandgap to 2.25 from 2.62 eV.125–129 Another extensively used semiconductor, titanium dioxide (TiO2), exhibits a greater bandgap of 3.2 eV;130 although, with the introduction of CDs, a bandgap of 2.75 to 2.87 eV has been recorded.131,132Fig. 8b shows a schematic illustration of the photocatalytic system and the involvement of CDs in the process. To summarize, the activated electrons from the semiconductor photocatalyst's VB to CB can be transported to the CDs, resulting in effective isolation of e/h+, preventing recombination and therefore enhancing photocatalytic performance. Furthermore, CDs' up-conversion capabilities suggest that near-infrared and visible light can be transformed to greater energy wavelengths, expanding the spectral response of the semiconductor photocatalyst and so boosting pharmaceutical pollutant degradation. The photocatalysts made from CDs alleviate some of the problems that plagued earlier generations of materials such as g-C3N4 and TiO2, but there are still some tricky issues related to CD manufacturing. Some CD production processes are harmful to the environment, and obtaining mono-dispersed and pure CDs often necessitates additional purification or separation procedures.
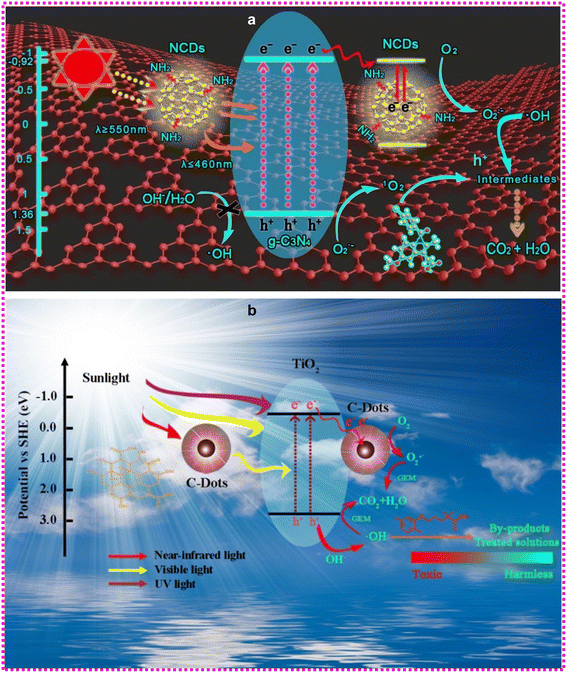 |
| Fig. 8 (a) Illustration of N-doped carbon dots/g-C3N4 photocatalyst with enhanced visible-light photocatalytic activity for the degradation of indomethacin. Reproduced with permission from ref. 125. Copyright 2017, Elsevier Inc. (b) Schematic photocatalytic mechanism of gemfibrozil (a drug for regulating blood lipid content) for the TiO2/C-dots composites under simulated sunlight irradiation. Reproduced with permission from ref. 131. Copyright 2017, Elsevier Inc. | |
Understanding the role and effect of CDs in photodegradation processes requires studying their properties. Here, the structure of the carbon material, and thus their characteristics, are profoundly affected by the precursor employed and the synthesis route. A large number of studies have relied on CDs produced using hydrothermal processes.126,133–136 This technique is part of the bottom-up strategy, which involves employing chemical or physical forces to organize molecules or atoms until they achieve the required nanostructure. The hydrothermal approach, in particular, is frequently utilized because it is inexpensive and easy to control parameters like time and temperature. Because of its biocompatibility and reasonable cost, citric acid is commonly used as a carbon precursor in hydrothermal carbon dots.123 CDs doped with heteroatoms are commonly used in catalytic applications because they have a lower surface activity, which allows for more comfortable electron transfer between reactions.124 In the reviewed investigations, nitrogen125,127,137,138 is a more prominent doping heteroatom.127,137 Photoluminescence and charge delocalization are both enhanced by N-CDs.137 Furthermore, N-doping causes surface flaws in CDs, resulting in increased electronic conductivity and better adsorption due to the increased surface area.139 CDs derived from citric acid and other materials are routinely doped with N2 to improve photocatalytic performance.140 Furthermore, it has been observed that the high blue fluorescence emitted by nitrogen-doped CDs from citric acid is caused by the existence of citrazinic acid-based compounds141 (Fig. 9a). Likewise, S-doping can boost CD photoexcitation and electron concentration considerably.137 Surprisingly, CDs produced from top-down methodologies were barely used in wastewater remediation. Chen and coworkers142 used a chemical oxidation approach to create carbon dots from starch with the goal of eliminating cefradine. Calcined starch was dissolved in deionized water and refluxed for 2 h at 120 °C with hydrogen peroxide and acetic acid. Chemical oxidation is beneficial for mass production due to its low cost of equipment; nonetheless, it necessitates the use of strong acids or bases.143 Various methodologies have been used to synthesize CDs, each of which has a profound impact on their performance and properties; moreover, the impacts of these methodologies are difficult to assess due to the numerous variables involved, such as reaction time, process temperature, concentrations, and reagent types among the few. CDs are commonly inserted in semiconductors such as g-C3N4 (ref. 125, 126, 133 and 135) and titanium dioxide (TiO2).126,127,132,133,136,137,142 Most of the other reasons that g-C3N4 is commonly employed in photocatalysis reactions include its great thermal and chemical stability accompanied with acceptable bandgap (2.7 eV).111 However, due to its small surface area, poor visible-light absorption, and low quantum efficacy, its photocatalytic efficiency is severely limited.111 Furthermore, amidst its high efficiency and inexpensive cost, TiO2's photocatalytic efficiency is restricted to a UV irradiation range of 3.2 eV due to a bandgap of 3.2 eV.130 Whenever these nanomaterials are treated with CDs, considerable gains for widening light adsorption wavelengths, prevention of e/h+ recombination, and increased number of available active sites happen,130 resulting in better photocatalytic efficiency. CDs have also been coupled with other semiconductors, such as bismuth molybdenum oxide (Bi2MoO6), cerium dioxide (CeO2), bismuth oxybromide (BiOBr), and goethite (–FeOOH), for the same purpose. Various methods for preparing CD-based composites have been tried. Zeng and coworkers136 used the calcination approach to create CDs/TiO2 photocatalysts for degrading ciprofloxacin. CDs were introduced to the mixture of ethanol and titania, which was then vaporized at 75 °C followed by calcination at 300 °C for 3 h in a muffle furnace. For photocatalytic efficiency, the preparation technique is crucial. Wang and coworkers125 investigated the photocatalytic activity of CDs/g-C3N4 synthesized using two methods: an amalgamated photocatalyst created using a polymerization process and a corporal combination of two compounds. Authors determined that the combined catalytic action occurs only with the aforementioned compound.125 The relationship between the methodologies and the features of the generated CDs-derived composites needs further investigation. In this context, research has been conducted to determine the impact of various fabrication processes on carbon-derived photocatalysts.144 However, as far as we know, no work has been done on the methods of synthesis of CDs-derived photocatalysts, which gives useful insights given that some of the features of CDs are still being debated.145 CQDs were the most commonly employed form of CDs to improve photocatalytic capability (Fig. 9b);133,134,146 nevertheless, GQDs have demonstrated the same capability,130 while research utilizing CNDs are rare. Furthermore, several research studies have not disclosed the sort of CDs that were utilized to make their CD-derived photocatalysts. The present study supports (i) the necessity to assess the impact of CD architecture on their protagonist in promoting photocatalytic degradation, and (ii) requirement to completely illustrate CDs when utilized for applications in order to determine the kind of CD and any structural changes.
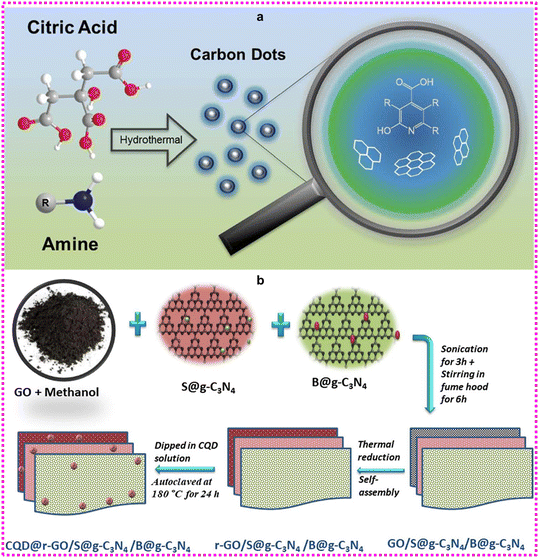 |
| Fig. 9 (a) Molecular fluorescence in citric acid-based carbon dots. Reproduced with permission from ref. 141. Copyright 2016, ACS Publications. (b) Synthesis scheme for CQDs catalyst. Reproduced with permission from ref. 146. Copyright 2017, Elsevier Inc. | |
According to our research, CD-derived photocatalysts for the eradication of pharmaceutical contaminants have been used more extensively in the breakdown of antibiotics like tetracycline and ciprofloxacin. This could be owing to the widespread use of these types of pharmaceuticals, as well as widespread awareness about the health and environmental consequences of their presence in aquatic environments. A number of pharmaceutical compounds, on the other hand, are found in wastewater, resulting in complicated combinations of contaminants. Fewer studies assessed the effectiveness of CD-derived photocatalysts in the removal/remediation of various medicinal goods, according to our review. In this context, Wu and coworkers135 prepared CDs/g-C3N4 photocatalyst and found its un-selected oxidative ability to photo-catalytically degrade a variety of pharmacological compounds, including diclofenac, norfloxacin, naproxen, ciprofloxacin, and indomethacin. However, the effectiveness of degradation differed contingent on the pharmacological molecule; ciprofloxacin, naproxen, and indomethacin were virtually entirely destroyed, but diclofenac and norfloxacin had less than 80% degradation efficiency.135 Using a CDs/g-C3N4 photocatalyst, Jin and coworkers133 found degradation rates of 71.7%, 83.7%, and 93.8% for triclosan, naproxen, and diclofenac, respectively.
4.2. Parameters affecting the photocatalytic performance of CDs
Various variables have been linked to the photocatalytic performance of CD-based photocatalysts in earlier research. Liu and coworkers147 studied the influence of the concentration of CDs introduced to the photocatalyst. The researchers used a polymerization approach to add various amounts of CQDs to g-C3N4. Photocatalytic studies were performed on composite formulations employing diclofenac as a reference pharmaceutical contaminant (Fig. 10).
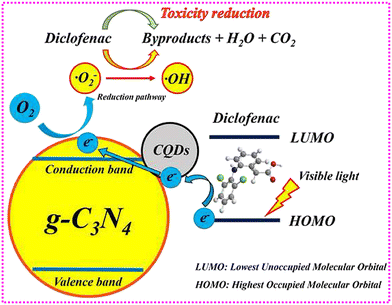 |
| Fig. 10 Illustration of carbon quantum dots modified porous g-C3N4: mechanisms, degradation pathway, and DFT calculation. Reproduced with permission from ref. 147. Copyright 2019, Elsevier Inc. | |
Benefiting from earlier research findings, similar approaches were applied.133–137,142 Surprisingly, the research evaluated here agrees that the number of CDs required for the proper functioning of photocatalysts is crucial. Generally, the degradation of the pharmaceutical contaminant is enhanced by the introduction of CDs regardless of the nature of the semiconductor used. Because an excess of CDs has the opposite effect, this augmentation is only seen until a maximum CD concentration is achieved. In this regard, findings reported that an excessive amount of CDs inhibits the production of reactive intermediates by reducing the number of active sites that serve as interaction centers; additionally, absorption of light might be hindered by an additional amount of CDs.147,148 Generally, trace amounts of CDs (from 0.5 to 4.0 wt%) applied to a standard semiconductor (TiO2 or g-C3N4) achieve the best pharmaceutical contaminant degradation rate.127,135 Only a few research works have documented the best results outside of the aforesaid range. For instance, Jin and coworkers133 observed the best degradation efficacy of diclofenac by CDs/g-C3N4-based material with CDs level of 0.2 wt%, while Zheng and coworkers128 disclosed the best degradation of ciprofloxacin by using CDs/TiO2 with a trace amount of CDs (6 wt%). These discrepancies could be ascribed to variables related to pharmaceutical contaminants or variances in photodegradation test settings. The impact of the photocatalyst concentration followed a similar trend. For example, Jin and coworkers133 evaluated the diclofenac removal rate of CDs/g-C3N4 photocatalyst in the 0.4–1.5 g L−1 range. They discovered that raising the CDs-derived photocatalyst dose improved efficiency; raising further reflected a minor improvement. As a result, 1.0 g L−1 was chosen as the best dose, owing to the photocatalyst's low cost and high degradation rate. Liang and coworkers138 studied the impact of photocatalyst quantity across a larger range (from 0.2 to 3.0 g L−1), indicating that 0.8 g L−1 was the ideal amount of their CDs-derived photocatalyst for the greatest pharmaceutical degradation since higher dosages resulted in slower degradation rates. With less photocatalyst, less e−/h+ is generated, and hence, a lesser degradation proportion is projected. Though, an overabundance of photocatalyst can prevent photons from propagating, and this, along with the scattering phenomenon, can result in a reduction in photocatalytic degradation.139 Other research studies coincided with these findings.133,135,138 The photocatalyst compound should normally be applied in smaller quantities (from 0.5 to 2.0 g L−1) to obtain optimal performance.133,135,138,142 The optimum pharmaceutical contaminant decomposition by titania has been documented to be around 1.0–1.5 g L−1,149,150 whereas 1.0–1.67 g-C3N4 materials are expected to decompose pharmaceutical contaminants effectively.149–152 Likewise, other researchers have assessed the influence of the irradiation method.131,135,137,138 Pharmaceutical concentrations in waterbodies and effluents/influents from WWTPs are in different ranges. Pharmaceutical wastes were found in surface water at amounts ranging from μg L−1 to ng L−1,44 whereas pharmaceutical waste concentrations in influents from numerous WWTPs were in the range of 11.5 to 30.2 g L−1. As a result, Sharma and coworkers132 investigated how the starting quantities of the pharmaceutical contaminant affected photocatalytic performance. They examined the decomposition rate of prepared photocatalysts at varied levofloxacin initial doses, determining that the composite performed better at 5 mg L−1. Increasing the concentration resulted in a considerable decrease (2%) in photocatalytic activity; however, a starting dose of 15 mg L−1 resulted in a large reduction to 84 percent. In the degradation of diclofenac with the help of CDs/g-C3N4 by Jin and coworkers,133 the loss in performance was significantly evident. Enhancing the starting amount resulted in a drop-in degradation efficiency from 100% to 64%, according to their findings. Other researchers have studied this parameter extensively.132,133,135,138,142 Overall, these investigations show that high pollution levels result in inadequate photon absorption due to a photon competition impact by contaminant molecules, resulting in a reduction of degradation rate. Similarly, pH, is another critical factor in photocatalysis for multiple reasons: (i) it influences the relationship between the pollutant and photocatalyst; (ii) the chemical reaction of pharmaceutical compounds is extremely susceptible to pH changes; (iii) photocatalyst surface characteristics are based on it; and (iv) the creation of radical intermediates such as hydroxyl radicals can be influenced by it.127,146 For instance, porous-g-C3N4/CDs synthesized by Liu and coworkers147 demonstrated a significantly higher diclofenac degradation rate under an alkaline environment, which was due to the increased OH adsorption influencing the photocatalyst's electronegativity; as a result, a reduction in the conduction band potential occurred, which increased ˙O2− generation. Huang and coworkers134 produced CQDs/–FeOOH with a greater tetracycline degradation rate at pH 7. Kumar and coworkers146 developed a CDs-derived photocatalyst that demonstrated a greater performance due to the electrostatic relationship between different species. The same charge between the photocatalyst and the pollutant induced electrostatic repulsion, resulting in reduced performance at severely basic and acidic environments.146 Other investigations, such as those conducted by Sharma and coworkers, have found that the pH has no effect on photocatalytic activity; the greatest removal of levofloxacin was observed at pH 6, while at higher values, performance was slightly reduced (88%). The researchers observed that a somewhat weak alkaline environment created the optimal quantity of hydroxyl radicals, which improved levofloxacin breakdown efficiency.132 To be regarded as acceptable options for treating wastewater, photocatalysts must have outstanding catalytic efficiency throughout a wide pH range. Water samples with inorganic ions contain pharmaceutical contaminants. These ions can interfere with the formation of active species or influence the behavior of the pollutant in photocatalytic processes. As a result, inorganic ions have a strong influence on the photocatalytic system. Using a CQDs/TiO2 nanocomposite, for example, NO3 had essentially negligible influence on the photodegradation of cefradine. However, when chloramphenicol was photodegraded utilizing the composite of rGO, CDs and g-C3N4, NO3 ions enhanced the degradability due to the production of ˙OH radicals with visible light. In this way, the effect of CDs-based photocatalytic activity on photodegradation performance must be identified in order to improve their ability for a number of compounds at ambient settings.
5. Recommendations and challenges
Inadequate monitoring of pharmaceutical items is the root cause of the issues related to pharmaceutical commodities contaminating wastewater. So, as a first step, preventative measures must be taken into consideration. Although pollution control is frequently underutilized to reduce the ecological effects of the pharmacological sector, it is crucial to prevent the preliminary discharge of pharmaceutical waste into the atmosphere. We examined the pharmaceutical-based wastage produced at every point of production of a pharmaceutical product in this review. Further research should be done on each stage to determine the best ways to stop the emission of pharmaceutical waste. For instance, some preventive steps during the manufacturing stage should be taken, such as process optimization to reduce waste output and solvent waste recycling. Addressing the consuming stage, it is vital to include a cultural viewpoint to comprehend how environmental issues are connected to the misuse of pharmaceutical goods because excretion is among the most significant paths for the proclamation of pharmaceutics-based waste into the environment. Additionally, proper consuming habits would greatly aid in the prevention of pharmaceutical contamination. To promote sensible drug usage, pharmacist involvement is very important. The pharmacist can also participate in precautionary procedures during the discarding stage by researching the most effective ways to get rid of pharmaceutical waste and offering fresher approaches.
However, the environmental contamination caused by pharmaceutical residues necessitates remedial measures as well. In this regard, CDs have demonstrated remarkable photodegradation performance on pharmaceutical contaminants; consequently, CDs play a major role in environmental cleanup. As a result, it is still difficult and urgently needed to synthesize them using simple, efficient, and economical methods. As a result, additional research into the top-down synthetic approach should be done. Waste products with greater carbonaceous content may make ideal contenders for precursors. Only a few of the research we have reviewed had CDs-derived photocatalysts with un-selective degradation ability. This needs to be well thought out because wastewater often contains a variety of pollutants. The use of such CDs-derived photocatalysts in actual, multifaceted water bodies still has to be investigated.
The comprehension of the kinetics of the chemical reaction with various pharmaceutical based contaminants, investigation of the properties of various structures to adjust photocatalytic ability, and a thorough comprehension of the impact of the arrangement of CDs upon their photocatalytic activity are additional areas of research.
6. Conclusions
Pharmaceutical waste can enter the environment more easily, thanks to how pharmaceutical items are currently manufactured, used, and disposed off. When dealing with pharmaceutical items, these issues, combined with the procedures to remove traditional pollutants, are inadequate efforts and result in the contamination of aquatic ecosystems, negatively impacting the ecosystem and human health. Alternative remediation methods, including photocatalysis with photocatalysts based on CDs, are becoming more common for water purification.
During photocatalytic reactions, the distinctive electrical and optical characteristics of CDs have a synergistic effect. In this way, given the environmental conditions, extremely effective photocatalytic devices based on CDs can be simply employed to totally decompose water-based contaminants into carbon dioxide and inorganic species. The approaches to obtain optimal performance were examined in this review, considering pertinent factors, including the fluctuating pH, inorganic ions' presence, initial amounts of pollutants, and the necessary number of CDs-based hybrids that can act as photocatalysts. To conclude, novel techniques using cutting-edge nanomaterials for cleaning up contaminated water should not be viewed as the final step but rather as a component of a comprehensive approach that starts with proper handling of these issues throughout the course of the pharmaceuticals' entire lifecycle. For the widespread application of CD-based photocatalysts, there is a need to introduce rational designs for the facile synthesis of such materials with higher yields along with different functionalities and employing green carbon precursors. Moreover, theoretical interpretations may also prove helpful, along with experimental studies.
Conflicts of interest
There is no conflict of interest to disclose by the authors in any capacity, either financial or competing.
Acknowledgements
M. I. appreciates the support of the Research Center for Advanced Materials Science (RCAMS) at King Khalid University Abha, Saudi Arabia, through grant RCAMS/KKU/024/23.
References
- M. M. Mekonnen and A. Y. Hoekstra, Sci. Adv., 2016, 2, e1500323 CrossRef PubMed
.
- M. Rodell, I. Velicogna and J. S. Famiglietti, Nature, 2009, 460, 999–1002 CrossRef CAS PubMed
.
- J. Tollefson, Nature, 2011, 473, 134–136 CrossRef CAS PubMed
.
- Q. Bu, B. Wang, J. Huang, S. Deng and G. Yu, J. Hazard. Mater., 2013, 262, 189–211 CrossRef CAS PubMed
.
- R. Prosser and P. Sibley, Environ. Int., 2015, 75, 223–233 CrossRef CAS
.
- S. Webb, T. Ternes, M. Gibert and K. Olejniczak, Toxicol. Lett., 2003, 142, 157–167 CrossRef CAS PubMed
.
- R. Kafaei, F. Papari, M. Seyedabadi, S. Sahebi, R. Tahmasebi, M. Ahmadi, G. A. Sorial, G. Asgari and B. Ramavandi, Sci. Total Environ., 2018, 627, 703–712 CrossRef CAS PubMed
.
- H. Zhang, P. Liu, Y. Feng and F. Yang, Mar. Pollut. Bull., 2013, 73, 282–290 CrossRef CAS PubMed
.
-
K. Kümmerer, in Pharmaceuticals in the Environment, Springer, 2008, pp. 3–21 Search PubMed
.
- T. U. Berendonk, C. M. Manaia, C. Merlin, D. Fatta-Kassinos, E. Cytryn, F. Walsh, H. Bürgmann, H. Sørum, M. Norström and M.-N. Pons, Nat. Rev. Microbiol., 2015, 13, 310–317 CrossRef CAS PubMed
.
- R. M. Klevens, J. R. Edwards, C. L. Richards Jr, T. C. Horan, R. P. Gaynes, D. A. Pollock and D. M. Cardo, Public Health Rep., 2007, 122, 160–166 CrossRef
.
- X. Jin, C. Yu, Y. Li, Y. Qi, L. Yang, G. Zhao and H. Hu, J. Hazard. Mater., 2011, 186, 1672–1680 CrossRef CAS PubMed
.
- X. Su, J. Yuan, W. Dong, F. Sun, M. Wang, Z. Dong, W. Li and C. Du, Bioresour. Technol., 2021, 330, 124959 CrossRef CAS
.
- J. Rivera-Utrilla, M. Sánchez-Polo, M. Á. Ferro-García, G. Prados-Joya and R. Ocampo-Pérez, Chemosphere, 2013, 93, 1268–1287 CrossRef CAS PubMed
.
- M.-T. Valdivia, M. A. Taggart, S. Pap, A. Kean, S. Pfleger and I. L. Megson, J. Water Process. Eng., 2023, 52, 103553 CrossRef
.
- L. Y. Jun, N. Mubarak, M. J. Yee, L. S. Yon, C. H. Bing, M. Khalid and E. Abdullah, J. Ind. Eng. Chem., 2018, 67, 175–186 CrossRef CAS
.
- J. Liu, R. Li and B. Yang, ACS Cent. Sci., 2020, 6, 2179–2195 CrossRef CAS PubMed
.
- F.-T. Wang, L.-N. Wang, J. Xu, K.-J. Huang and X. Wu, Analyst, 2021, 146, 4418–4435 RSC
.
- H. S. Abbo, K. C. Gupta, N. G. Khaligh and S. J. Titinchi, ChemBioEng Rev., 2021, 8, 463–489 CrossRef CAS
.
- U. Abd Rani, L. Y. Ng, C. Y. Ng and E. Mahmoudi, Adv. Colloid Interface Sci., 2020, 278, 102124 CrossRef CAS PubMed
.
- C. Li, X. Wu, J. Hu, J. Shan, Z. Zhang, X. Huang and H. Liu, Environ. Sci. Pollut. Res., 2022, 29, 35657–35681 CrossRef CAS
.
- V. Mishra, A. Patil, S. Thakur and P. Kesharwani, Drug Discovery Today, 2018, 23, 1219–1232 CrossRef CAS PubMed
.
- R. M. Sendão, J. C. E. da Silva and L. P. da Silva, Chemosphere, 2022, 301, 134731 CrossRef PubMed
.
- S. Mishra, Indian Heart J., 2016, 68, 19–27 CrossRef PubMed
.
- S. H. Woolf, JAMA, J. Am. Med. Assoc., 2008, 299, 211–213 CAS
.
- R. B. González-González, A. Sharma, R. Parra-Saldívar, R. A. Ramirez-Mendoza, M. Bilal and H. M. Iqbal, J. Hazard. Mater., 2022, 423, 127145 CrossRef
.
-
P. M. M. Size, Grand View Research. URL: https://www.grandviewresearch.com/industryanalysis/pharmaceutical-manufacturing-market.
- D. M. Cutler, JAMA, J. Am. Med. Assoc., 2020, 323, 109–110 CrossRef PubMed
.
- L. D. A. A. Freitas and G. Radis-Baptista, J. Xenobiot., 2021, 11, 61–76 CrossRef PubMed
.
- M. R. Hamblin, BBA Clin., 2016, 6, 113–124 CrossRef PubMed
.
- M. N. Nyaga, D. M. Nyagah and A. Njagi, Journal of PeerScientist, 2020 DOI:10.5281/zenodo.4088224
.
- N. Taoufik, W. Boumya, F. Janani, A. Elhalil and F. Mahjoubi, J. Environ. Chem. Eng., 2020, 8, 104251 CrossRef CAS
.
- L. M. Bexfield, P. L. Toccalino, K. Belitz, W. T. Foreman and E. T. Furlong, Environ. Sci. Technol., 2019, 53, 2950–2960 CrossRef CAS PubMed
.
- S.-C. Kim and K. Carlson, Water Res., 2006, 40, 2549–2560 CrossRef CAS PubMed
.
- A. Watkinson, E. Murby, D. W. Kolpin and S. Costanzo, Sci. Total Environ., 2009, 407, 2711–2723 CrossRef CAS PubMed
.
- C. G. Daughton, Compr. Anal. Chem., 2007, 50, 1–58 CAS
.
- C. G. Daughton, Sci. Total Environ., 2014, 493, 392–404 CrossRef CAS PubMed
.
- S. Bagheri, A. TermehYousefi and T.-O. Do, Catal. Sci. Technol., 2017, 7, 4548–4569 RSC
.
- S. McGuire, Adv. Nutr., 2016, 7(2), 418–419 CrossRef PubMed
.
- S. Bekiros and D. Kouloumpou, Chaos, Solitons Fractals, 2020, 136, 109828 CrossRef PubMed
.
- T. T. H. Van, Z. Yidana, P. M. Smooker and P. J. Coloe, J. Global Antimicrob. Resist., 2020, 20, 170–177 CrossRef PubMed
.
- T. P. Van Boeckel, C. Brower, M. Gilbert, B. T. Grenfell, S. A. Levin, T. P. Robinson, A. Teillant and R. Laxminarayan, Proc. Natl. Acad. Sci., 2015, 112, 5649–5654 CrossRef CAS PubMed
.
- T. A. der Beek, F. A. Weber, A. Bergmann, S. Hickmann, I. Ebert, A. Hein and A. Küster, Environ. Toxicol. Chem., 2016, 35, 823–835 CrossRef PubMed
.
- D. O'Flynn, J. Lawler, A. Yusuf, A. Parle-McDermott, D. Harold, T. Mc Cloughlin, L. Holland, F. Regan and B. White, Anal. Methods, 2021, 13, 575–594 RSC
.
- M. Patel, R. Kumar, K. Kishor, T. Mlsna, C. U. Pittman Jr and D. Mohan, Chem. Rev., 2019, 119, 3510–3673 CrossRef CAS PubMed
.
- M. Veiga-Gómez, C. Nebot, C. M. Franco, J. M. Miranda, B. Vázquez and A. Cepeda, J. Dairy Sci., 2017, 100, 3373–3383 CrossRef PubMed
.
- T. Rasheed, M. Bilal, A. A. Hassan, F. Nabeel, R. N. Bharagava, L. F. Romanholo Ferreira, H. N. Tran and H. M. N. Iqbal, Environ. Res., 2020, 185, 109436 CrossRef CAS PubMed
.
- M. P. Kusturica, S. Golocorbin-Kon, T. Ostojic, M. Kresoja, M. Milovic, O. Horvat, T. Dugandzija, N. Davidovac, A. Vasic and A. Tomas, Waste Manage., 2020, 104, 246–253 CrossRef PubMed
.
- K. Shalini, Z. Anwer, P. K. Sharma, V. K. Garg and N. Kumar, Int. J. PharmTech Res., 2010, 2, 2265–2270 Search PubMed
.
-
U. S. E. P. A. Enforcement and C. Assurance, Identification of Pollution Prevention (P2) Technologies for Possible Inclusion in Enforcement Agreements Using Supplemental Environmental Projects (SEPs) and Injunctive Relief, United States Environmental Protection Agency, Enforcement and Compliance…, 1997.
- P. J. Dunn, A. S. Wells and M. T. Williams, Green Chem. Pharm. Ind., 2010, 333–355 CAS
.
-
C. M. Browner, J. C. Fox, S. Frace, M. B. Rubin and F. Hund, Development Document for Final Effluent Limitations Guidelines and Standards for the Pharmaceutical Manufacturing Point Source Category, Engineering and Analysis Division Office Science and Technology, US Environmental Protection Agency, Washington, DC, 1998 Search PubMed.
-
R. E. Hester and R. M. Harrison, Pharmaceuticals in the Environment, Royal Society of Chemistry, 2015 Search PubMed
.
- D. J. Larsson, Philos. Trans. R. Soc., B, 2014, 369, 20130571 CrossRef PubMed
.
- D. Taylor and T. Senac, Chemosphere, 2014, 115, 95–99 CrossRef CAS PubMed
.
- J. Fick, H. Söderström, R. H. Lindberg, C. Phan, M. Tysklind and D. J. Larsson, Environ. Toxicol. Chem., 2009, 28, 2522–2527 CrossRef CAS PubMed
.
- J. V. Holm, K. Ruegge, P. L. Bjerg and T. H. Christensen, Environ. Sci. Technol., 1995, 29, 1415–1420 CrossRef CAS PubMed
.
- C. Lübbert, C. Baars, A. Dayakar, N. Lippmann, A. C. Rodloff, M. Kinzig and F. Sörgel, Infection, 2017, 45, 479–491 CrossRef
.
- C. Rutgersson, J. Fick, N. Marathe, E. Kristiansson, A. Janzon, M. Angelin, A. Johansson, Y. Shouche, C.-F. Flach and D. J. Larsson, Environ. Sci. Technol., 2014, 48, 7825–7832 CrossRef CAS
.
- D. J. Larsson, C. de Pedro and N. Paxeus, J. Hazard. Mater., 2007, 148, 751–755 CrossRef CAS PubMed
.
- H. I. Abdel-Shafy and M. S. Mohamed-Mansour, Egypt. J. Chem., 2013, 56, 449–471 CrossRef
.
- V. Burkina, V. Zlabek and G. Zamaratskaia, Environ. Toxicol. Pharmacol., 2015, 40, 430–444 CrossRef CAS PubMed
.
- N. Taxak and P. V. Bharatam, Resonance, 2014, 19, 259–282 CrossRef CAS
.
- S. S. Hassan, H. I. Abdel-Shafy and M. S. Mansour, Arabian J. Chem., 2019, 12, 4074–4083 CrossRef CAS
.
- H. Jin, A. P. Kumar, D.-H. Paik, K.-C. Ha, Y.-J. Yoo and Y.-I. Lee, Microchem. J., 2010, 94, 139–147 CrossRef CAS
.
- A. M. Lerbech, J. A. Opintan, S. O. Bekoe, M.-A. Ahiabu, B. P. Tersbøl, M. Hansen, K. T. Brightson, S. Ametepeh, N. Frimodt-Møller and B. Styrishave, PLoS One, 2014, 9, e113055 CrossRef PubMed
.
- M. Steinbakk, E. Lingaas, B. Carlstedt-Duke, T. Høverstad, A.-C. Midtvedt, K. Norin and T. Midtvedt, Microb. Ecol. Health Dis., 1992, 5, 269–276 CrossRef
.
- L. Zhao, Y. H. Dong and H. Wang, Sci. Total Environ., 2010, 408, 1069–1075 CrossRef CAS PubMed
.
- R. M. P. Leal, R. F. Figueira, V. L. Tornisielo and J. B. Regitano, Sci. Total Environ., 2012, 432, 344–349 CrossRef CAS PubMed
.
- Y.-X. Li, X.-L. Zhang, W. Li, X.-F. Lu, B. Liu and J. Wang, Environ. Monit. Assess., 2013, 185, 2211–2220 CrossRef CAS PubMed
.
- X. Pan, Z. Qiang, W. Ben and M. Chen, Chemosphere, 2011, 84, 695–700 CrossRef CAS PubMed
.
- M. Qiao, W. Chen, J. Su, B. Zhang and C. Zhang, J. Environ. Sci., 2012, 24, 1047–1052 CrossRef CAS PubMed
.
- H. Zhang, M. Zhang and G. Gu, Shengtai Yu Nongcun Huanjing Xuebao, 2008, 24, 69–73 CAS
.
- A. Van Epps and L. Blaney, Curr. Pollut. Rep., 2016, 2, 135–155 CrossRef CAS
.
- A. Ahmed and N. Mushtaq, J. Univ. Med. Dent. Coll., 2013, 4, 42–48 Search PubMed
.
- G. R. Quadra, P. S. Silva, J. R. Paranaíba, I. I. Josué, H. Souza, R. Costa, M. Fernandez, J. Vilas-Boas and F. Roland, Sci. Total Environ., 2019, 671, 505–509 CrossRef CAS PubMed
.
- M. Bashaar, V. Thawani, M. A. Hassali and F. Saleem, BMC Public Health, 2017, 17, 1–8 CrossRef
.
- M. M. Begum, S. F. Rivu, M. M. A. Hasan, T. T. Nova, M. M. Rahman, M. A. Alim, M. S. Uddin, A. Islam, N. Tabassum and M. M. R. Moni, Pharmacy, 2021, 9, 103 CrossRef PubMed
.
- A. Vellinga, S. Cormican, J. Driscoll, M. Furey, M. O'Sullivan and M. Cormican, Sci. Total Environ., 2014, 478, 98–102 CrossRef CAS PubMed
.
- K. A. I. Albaroodi, Pharmacy, 2019, 7, 57 CrossRef PubMed
.
- F. Alnahas, P. Yeboah, L. Fliedel, A. Y. Abdin and K. Alhareth, Int. J. Environ. Res. Public Health, 2020, 17, 787 CrossRef PubMed
.
- S. Heim, J. Schwarzbauer and R. Littke, Environ. Chem. Lett., 2004, 2, 21–25 CrossRef CAS
.
- S. E. Musson and T. G. Townsend, J. Hazard. Mater., 2009, 162, 730–735 CrossRef CAS PubMed
.
- Q. Bu, H. Cao, X. He, H. Zhang and G. Yu, Bull. Environ. Contam. Toxicol., 2020, 105, 784–789 CrossRef CAS
.
-
B. M. Peake, R. Braund, A. Tong and L. Tremblay, The Life-cycle of Pharmaceuticals in the Environment, Elsevier, 2015 Search PubMed
.
- A. J. Mackridge and J. F. Marriott, J. Public Health, 2007, 29, 258–262 CrossRef PubMed
.
- A. Zakaria and O. Labib, J. Egypt. Public Health Assoc., 2003, 78, 225–244 Search PubMed
.
- Y. Kerchich, Y. Moussaoui, G. Scholl and G. Eppe, Atmos. Pollut. Res., 2018, 968–975 Search PubMed
.
- H. T. Van, Q. H. Pham, M. V. Trinh and H. M. Bui, Pol. J. Chem. Technol., 2020, 22, 29–34 Search PubMed
.
- A. Hoyos, M. Cobo, B. Aristizábal, F. Córdoba and C. M. de Correa, Chemosphere, 2008, 73, S137–S142 CrossRef CAS
.
- M. Tufail and S. Khalid, Microchem. J., 2008, 90, 77–81 CrossRef
.
-
W. H. Organization, WHO monographs on selected medicinal plants, World Health Organization, 1999 Search PubMed
.
- T. G. Bean, E. Bergstrom, J. Thomas-Oates, A. Wolff, P. Bartl, B. Eaton and A. Boxall, Environ. Manage., 2016, 58, 707–720 CrossRef PubMed
.
- A. Y. Tong, B. M. Peake and R. Braund, Environ. Int., 2011, 37, 292–298 CrossRef PubMed
.
- T. Narayana, Waste Manage., 2009, 29, 1163–1166 CrossRef CAS PubMed
.
- T. Eggen, M. Moeder and A. Arukwe, Sci. Total Environ., 2010, 408, 5147–5157 CrossRef CAS PubMed
.
- M. R. Sabour, E. Alam and A. M. Hatami, J. Mater. Cycles Waste Manage., 2020, 22, 711–723 CrossRef
.
- X. Yu, Q. Sui, S. Lyu, W. Zhao, J. Liu, Z. Cai, G. Yu and D. Barcelo, Environ. Sci. Technol., 2020, 54, 9757–9768 CrossRef CAS PubMed
.
- B. Zhou, C. Sun and H. Yi, Sustainability, 2017, 9, 2234 CrossRef
.
- M. K. Ghasemi and R. B. Yusuff, Pol. J. Environ. Stud., 2016, 25, 25–30 Search PubMed
.
- J. R. Masoner, D. W. Kolpin, E. T. Furlong, I. M. Cozzarelli, J. L. Gray and E. A. Schwab, Environ. Sci.: Processes Impacts, 2014, 16, 2335–2354 RSC
.
- X. Peng, W. Ou, C. Wang, Z. Wang, Q. Huang, J. Jin and J. Tan, Sci. Total Environ., 2014, 490, 889–898 CrossRef CAS PubMed
.
- Q. Sui, W. Zhao, X. Cao, S. Lu, Z. Qiu, X. Gu and G. Yu, J. Hazard. Mater., 2017, 323, 99–108 CrossRef CAS PubMed
.
- M.-C. Lu, Y. Y. Chen, M.-R. Chiou, M. Y. Chen and H.-J. Fan, Waste Manage., 2016, 55, 257–264 CrossRef CAS PubMed
.
- E. Gracia-Lor, J. V. Sancho, R. Serrano and F. Hernández, Chemosphere, 2012, 87, 453–462 CrossRef CAS PubMed
.
- W.-J. Sim, J.-W. Lee and J.-E. Oh, Environ. Pollut., 2010, 158, 1938–1947 CrossRef CAS PubMed
.
- G. Crini and E. Lichtfouse, Environ. Chem. Lett., 2019, 17, 145–155 CrossRef CAS
.
- A. O. Oluwole, E. O. Omotola and O. S. Olatunji, BMC Chem., 2020, 14, 1–29 CrossRef PubMed
.
- K. I. Ekpeghere, J.-W. Lee, H.-Y. Kim, S.-K. Shin and J.-E. Oh, Chemosphere, 2017, 168, 1211–1221 CrossRef CAS PubMed
.
- S. Rajbongshi, Y. D. Shah and A. U. Sajib, Eur. J. Biomed. Pharm. Sci., 2016, 3, 192–206 CAS
.
- Y. Chen and X. Bai, Catalysts, 2020, 10, 142 CrossRef CAS
.
- K.-W. Chu, S. L. Lee, C.-J. Chang and L. Liu, Polymer, 2019, 11, 689 CAS
.
- B. B. Chen, M. L. Liu, C. M. Li and C. Z. Huang, Adv. Colloid Interface Sci., 2019, 270, 165–190 CrossRef CAS PubMed
.
- M. Semeniuk, Z. Yi, V. Poursorkhabi, J. Tjong, S. Jaffer, Z.-H. Lu and M. Sain, ACS Nano, 2019, 13, 6224–6255 CrossRef CAS PubMed
.
- C. Xia, S. Zhu, T. Feng, M. Yang and B. Yang, Adv. Sci., 2019, 6, 1901316 CrossRef CAS PubMed
.
- S. Li, L. Li, H. Tu, H. Zhang, D. S. Silvester, C. E. Banks, G. Zou, H. Hou and X. Ji, Mater. Today, 2021, 51, 188–207 CrossRef CAS
.
- W. Liu, C. Li, Y. Ren, X. Sun, W. Pan, Y. Li, J. Wang and W. Wang, J. Mater. Chem. B, 2016, 4, 5772–5788 RSC
.
- J. Yu, H. Song, X. Li, L. Tang, Z. Tang, B. Yang and S. Lu, Adv. Funct. Mater., 2021, 31, 2107196 CrossRef CAS
.
- J. Yu, X. Yong, Z. Tang, B. Yang and S. Lu, J. Phys. Chem. Lett., 2021, 12, 7671–7687 CrossRef CAS PubMed
.
- M. Han, S. Zhu, S. Lu, Y. Song, T. Feng, S. Tao, J. Liu and B. Yang, Nano Today, 2018, 19, 201–218 CrossRef CAS
.
- P. Kar, K. Shukla, P. Jain, G. Sathiyan and R. K. Gupta, Nano Mater. Sci., 2021, 3, 25–46 CrossRef CAS
.
- A. G. Variar, M. Ramyashree, V. U. Ail, K. Sudhakar and M. Tahir, J. Ind. Eng. Chem., 2021, 99, 19–47 CrossRef CAS
.
- J. Ren, L. Malfatti and P. Innocenzi, C, 2020, 7(1), 2 Search PubMed
.
- Z. Zhang, G. Yi, P. Li, X. Zhang, H. Fan, Y. Zhang, X. Wang and C. Zhang, Nanoscale, 2020, 12, 13899–13906 RSC
.
- F. Wang, P. Chen, Y. Feng, Z. Xie, Y. Liu, Y. Su, Q. Zhang, Y. Wang, K. Yao and W. Lv, Appl. Catal., B, 2017, 207, 103–113 CrossRef CAS
.
- B. Song, Q. Wang, L. Wang, J. Lin, X. Wei, V. Murugadoss, S. Wu, Z. Guo, T. Ding and S. Wei, J. Colloid Interface Sci., 2020, 559, 124–133 CrossRef CAS PubMed
.
- F. Wang, Y. Wu, Y. Wang, J. Li, X. Jin, Q. Zhang, R. Li, S. Yan, H. Liu and Y. Feng, Chem. Eng. J., 2019, 356, 857–868 CrossRef CAS
.
- X. Zheng, Q. Zhang, T. Chen, Y. Wu, J. Hao, C. Tan, P. Chen, F. Wang, H. Liu and W. Lv, J. Hazard. Mater., 2020, 386, 121961 CrossRef CAS PubMed
.
- C. Zhou, Z. Zeng, G. Zeng, D. Huang, R. Xiao, M. Cheng, C. Zhang, W. Xiong, C. Lai and Y. Yang, J. Hazard. Mater., 2019, 380, 120815 CrossRef CAS PubMed
.
- A. Bokare, S. Chinnusamy and F. Erogbogbo, Catalysts, 2021, 11, 319 CrossRef CAS
.
- P. Chen, F. Wang, Z.-F. Chen, Q. Zhang, Y. Su, L. Shen, K. Yao, Y. Liu, Z. Cai and W. Lv, Appl. Catal., B, 2017, 204, 250–259 CrossRef CAS
.
- S. Sharma, A. Umar, S. K. Mehta, A. O. Ibhadon and S. K. Kansal, New J. Chem., 2018, 42, 7445–7456 RSC
.
- X. Jin, Y. Wu, Y. Wang, Z. Lin, D. Liang, X. Zheng, D. Wei, H. Liu, W. Lv and G. Liu, Chem. Eng. J., 2021, 420, 129935 CrossRef CAS
.
- F. Wang, Y. Huang, P. Wen and Q. Li, Chemosphere, 2021, 263, 128198 CrossRef CAS PubMed
.
- Y. Wu, F. Wang, X. Jin, X. Zheng, Y. Wang, D. Wei, Q. Zhang, Y. Feng, Z. Xie and P. Chen, Water Res., 2020, 172, 115492 CrossRef CAS PubMed
.
- Y. Zeng, D. Chen, T. Chen, M. Cai, Q. Zhang, Z. Xie, R. Li, Z. Xiao, G. Liu and W. Lv, Chemosphere, 2019, 227, 198–206 CrossRef CAS PubMed
.
-
W. S. Koe, BS, UTAR, 2019 Search PubMed
.
- L. Liang, S. Gao, J. Zhu, L. Wang, Y. Xiong, X. Xia and L. Yang, Chem. Eng. J., 2020, 391, 123599 CrossRef CAS
.
- Z. Li, Y. Zhang, Q. Niu, M. Mou, Y. Wu, X. Liu, Z. Yan and S. Liao, J. Lumin., 2017, 187, 274–280 CrossRef CAS
.
- W. Shi, F. Guo, M. Li, Y. Shi, M. Wu and Y. Tang, J. Alloys Compd., 2019, 775, 511–517 CrossRef CAS
.
- J. Schneider, C. J. Reckmeier, Y. Xiong, M. von Seckendorff, A. S. Susha, P. Kasák and A. L. Rogach, J. Phys. Chem. C, 2017, 121, 2014–2022 CrossRef CAS
.
- J. Chen, J. Shu, Z. Anqi, H. Juyuan, Z. Yan and J. Chen, Diamond Relat. Mater., 2016, 70, 137–144 CrossRef CAS
.
- K. K. Chan, S. H. K. Yap and K.-T. Yong, Nano-Micro Lett., 2018, 10, 1–46 CrossRef PubMed
.
- Y. Hendrix, A. Lazaro, Q. Yu and H. Brouwers, J. Photochem. Photobiol., A, 2019, 371, 25–32 CrossRef CAS
.
- Z. Gan, H. Xu and Y. Hao, Nanoscale, 2016, 8, 7794–7807 RSC
.
- A. Kumar, A. Kumari, G. Sharma, B. Du, M. Naushad and F. J. Stadler, J. Mol. Liq., 2020, 300, 112356 CrossRef CAS
.
- W. Liu, Y. Li, F. Liu, W. Jiang, D. Zhang and J. Liang, Water Res., 2019, 151, 8–19 CrossRef CAS PubMed
.
- L. Guo, Y. Zhang and R.-X. Zhang, Asian J. Chem., 2014, 26, 1077 CrossRef
.
- R. A. Palominos, M. A. Mondaca, A. Giraldo, G. Peñuela, M. Pérez-Moya and H. D. Mansilla, Catal. Today, 2009, 144, 100–105 CrossRef CAS
.
- G. Safari, M. Hoseini, M. Seyedsalehi, H. Kamani, J. Jaafari and A. Mahvi, Int. J. Environ. Sci. Technol., 2015, 12, 603–616 CrossRef CAS
.
- Y. Deng, L. Tang, C. Feng, G. Zeng, J. Wang, Y. Zhou, Y. Liu, B. Peng and H. Feng, J. Hazard. Mater., 2018, 344, 758–769 CrossRef CAS PubMed
.
- W. Xu, S. Lai, S. C. Pillai, W. Chu, Y. Hu, X. Jiang, M. Fu, X. Wu, F. Li and H. Wang, J. Colloid Interface Sci., 2020, 574, 110–121 CrossRef CAS PubMed
.
|
This journal is © The Royal Society of Chemistry 2024 |
Click here to see how this site uses Cookies. View our privacy policy here.