Phosphorus release from sewage sludge and digestate driven by biological sulfate reduction: effect of feed sulfate concentration and thermal hydrolysis†
Received
20th April 2024
, Accepted 10th September 2024
First published on 19th September 2024
Abstract
Phosphorus recovery from waste streams stands out as a strategic practice to ensure phosphorus availability to future generations. The release of phosphate mediated by biological sulfate reduction is an interesting bioprocess for phosphorus recovery from sewage sludge in wastewater treatment plants in which chemical phosphorus recovery is foreseen. This study investigates the effect of biological sulfate reduction at different feed sulfate concentrations (up to 8000 mg L−1) on the anaerobic phosphate release from both sewage sludge and digestate as well as the impact of sulfate addition on energy recovery from the sludge via biomethane production. During anaerobic digestion, up to 62.3% of the phosphate initially present in the sludge as iron(III) phosphate was released with 8000 mg L−1 feed sulfate. However, biomethane production was significantly reduced (>40%) when sulfate was added at concentrations above 100 mg L−1. The use of thermal hydrolysis on the sludge digestate was found to be an effective strategy for phosphorus recovery from the sludge without compromising the biomethane production during anaerobic digestion. A phosphate release from iron(III) phosphate of up to 48.7% was obtained when adding 4000 mg L−1 sulfate to the digestate previously hydrolyzed for 2 hours. Finally, the implementation potential of the proposed strategy in full-scale wastewater treatment plants is discussed.
Water impact
This study holds crucial implications for water sustainability by introducing a novel approach to phosphorus release from sewage sludge. Biological sulfate reduction offers a pathway to mitigate phosphorus scarcity, ensuring its future availability. The findings underscore the potential for environmentally conscious wastewater treatment, with an optimized strategy balancing phosphorus recovery and energy production, contributing to more sustainable water management systems.
|
1 Introduction
In the modern paradigm of circular economy, the recovery of waste materials has become of utmost importance to reduce the depletion of natural resources and environmental pollution. In this context, the wastewater treatment plant (WWTP) can be considered as a source of valuable materials and energy to be recovered rather than discarded along with the effluent.1 Among the primary materials potentially recoverable within a WWTP, phosphorus (P) has been addressed as a scarce resource due to the finite availability and uneven geopolitical distribution of phosphate rock reserves.2 P is an essential element to secure food production to the ever-growing human population in the next future through the use of fertilizers in agriculture. The recovery and reuse of P from waste flows can help pursuing several sustainable development goals, including SDG2 (zero hunger), SDG6 (clean water and sanitation), SDG11 (sustainable cities and communities), and SDG13 (climate action). Moreover, improper P management and release in WWTPs can lead to serious environmental problems such as eutrophication. For this reason, P discharge in EU countries is regulated by European and national legislations, limiting the concentrations in treated water to 1–2 mg L−1 total P. Therefore, modern WWTPs are designed to remove P through chemical or biological methods, the first being the most widespread in Europe because of its high effectiveness, easy implementation, and lower capital expenditure.3,4
Chemical P removal in WWTPs is based on the addition of multivalent metal (e.g., iron, calcium, magnesium, and aluminium) salts, which act as precipitators of phosphate ions (PO43−) before or after the biological stage, resulting in P recovery typically ranging from 65% to 99%.5 As a result of chemical P precipitation, primary and/or secondary sludge is enriched in P and other metals and can be considered as a valuable pool of these elements. However, P separation from the complex sludge matrix is not an easy task and several chemical methods applicable to sewage sludge and/or sludge ashes have been proposed and applied in the past years, including wet leaching followed by struvite precipitation, magnetic vivianite separation, sludge melt gasification, white P recovery, and thermochemical sodium sulfate process.4 Alternatively, P could be retained in the sludge to be used as fertilizer after stabilization.6
Until now, biological methods for P recovery have been poorly explored. In this context, biological sulfate reduction (BSR) is a well-known bioprocess that may be exploited for P recovery from wastewater or sludge. BSR proceeds under anoxic conditions and consists in the reduction of sulfate ions (SO42−) to sulfide species by microbes known as sulfate-reducing bacteria (SRB), which typically use organic carbon as energy source, which also defines the process stoichiometry.7 Sulfide can exist in water as hydrogen sulfide (H2S), bisulfide (HS−), or monosulfide (S2−) depending on pH. Sulfide species have the capacity to form insoluble compounds with metals, which can be easily precipitated.8,9 In general, metal sulfides exhibit lower solubility than their phosphate and hydroxide counterparts, leading to a more efficient precipitation and higher stability over a wider pH range.10 For instance, ferric sulfide (Fe2S3, pKsp = 85.0) is much less soluble than ferric phosphate (Fe3(PO4)2, pKsp = 36.0). Previous experiences showed that sulfide addition was capable of mobilizing phosphate ions from ferric precipitates in sediment systems,11 chemical sludge from a drinking water plant,12 sewage sludge,13 and sludge digestate.14 Therefore, sulfide seems to act as an efficient PO43− extractor by reducing Fe(III) phosphates and forming highly insoluble Fe(II) sulfide precipitates.
Such studies have mainly focused on direct sulfide addition to the P-rich sludge matrix,12–14 while PO43− release from sludge via BSR co-occurring with anaerobic digestion (AD) remains poorly explored.15 Coupling BSR and AD in the same unit may simplify the sludge streamline of a WWTP where BSR-mediated P recovery is implemented, also considering that PO43− release from microbial biomass and organic matter (OM) is commonly observed in WWTP digesters.16,17 On the other hand, increasing sulfide levels in the anaerobic digester may cause the inhibition of fermentative and methanogenic microorganisms, thereby negatively affecting AD.18 In this case, recovering P from the sewage digestate might be a suitable solution, although a dedicated BSR-mediated P recovery stage involving digestate hydrolysis to release additional organic carbon should be foreseen. For a correct implementation of the BSR-mediated P recovery, it is therefore fundamental to investigate different scenarios, i.e., concomitant AD–BSR and post-digestion BSR, and identify the potential advantages and limitations of each process configuration.
In view of the above, the present study primarily investigates BSR-mediated P release from chemically pretreated waste activated sludge and thermally hydrolysed digestate in laboratory-scale digesters. At the same time, the effect of the feed SO42− concentration on the BSR and AD processes was also evaluated. To this end, experiments were run at different feed SO42− concentrations to investigate how these would affect the PO43− release and biomethane production from the sludge during concomitant AD–BSR as well as the PO43− release during post-digestion BSR operated on untreated or thermally hydrolysed sludge digestate. Finally, based on the obtained results, insights for future process implementation and upscaling are provided.
2 Materials and methods
2.1 Source and preparation of sewage sludge
The sewage sludge used for inoculum and experimental tests was collected from a municipal WWTP located in Nola (Campania region, Italy), characterized by a conventional pre-denitrification (Ludzack–Ettinger) system as biological phase. The sludge was collected from the pre-thickening stage, placed ahead of AD, at two different time points immediately before starting the experiments. The composition of the sludge substrates used during the experimental activity was quite homogeneous and characterized by the following values: pH 7.3 ± 0.1, total solids (TS) 33 ± 7 g L−1, volatile solids (VS) 22 ± 2 g L−1, total COD (tCOD) 29
953 ± 77 mg L−1, soluble COD (sCOD) 742 ± 235 mg L−1, sulfate (SO42−) 37 ± 23 mg L−1, and phosphate (PO43−) 214 ± 107 mg L−1. The sludge was spiked with an extra 1 g L−1 P, supplemented as iron phosphate (FePO4·2H2O, Sigma-Aldrich, USA), to simulate the occurrence of a chemical P precipitation in the wastewater streamline generating the sludge. Such a P concentration was chosen considering a PO43− concentration in the wastewater entering the WWTP of 10 mg P per L19 and considering that the sludge flow rate is approximately 1% of the influent wastewater flow.17 In the hypothesis that all influent PO43− precipitates with the added iron during chemical precipitation, the P concentration in the sludge would be 100 times higher (i.e., 1 g L−1) than in the influent wastewater.
2.2 Experimental design
All experiments were performed under batch conditions at laboratory scale in 500 mL SIMAX® borosilicate glass bottles (VWR, USA). Each bottle was filled with 300 mL of pre-thickened sludge and SO42− was dosed at different concentrations (as reported in the following sections) using sodium sulfate (Na2SO4, ITW Reagents, Italy). All bottles were sealed with caps allowing both gas and liquid sampling and placed in a thermostatic bath at 35 °C, as described by Morello et al.20 Before sealing, the bottle content was flushed with argon for 1 min to ensure anaerobic conditions. All experiments were performed in triplicate. Values reported without a standard deviation are average values. A comprehensive overview of all experiments performed is provided in Table 1. A maximum duration of 21 days was selected for the experiments, as the typical hydraulic retention time for AD of sewage sludge in municipal WWTPs is between 16 and 25 days.21
Table 1 List of the experiments (E) and preliminary tests (T) performed on sewage sludge and digestate
Experiment |
Objective |
Substrate |
Feed SO42− (mg L−1) |
Added PO43− (mg L−1) |
Duration |
Monitored parameters |
T1 |
Effect of initial SO42− concentration on CH4 production |
Sludge |
0, 100, 500, 2000, 4000 |
1000 |
21 d |
CH4, SO42−, PO43− |
E1 |
PO43− release during AD coupled to BSR |
Sludge |
0, 2000, 4000, 8000 |
1000 |
15 d |
SO42−, PO43− |
E2 |
PO43− release during BSR of digestate |
Digestate |
0, 2000, 4000, 6000 |
1000 |
21 d |
SO42−, PO43− |
T2 |
sCOD release from digestate after thermal hydrolysis at 90 °C |
Digestate |
0 |
0 |
0, 1, 2, 4 h |
sCOD, tCOD |
E3 |
PO43− release during BSR of hydrolyzed digestate |
Digestate |
0, 2000, 4000, 6000 |
1000 |
21 d |
SO42−, PO43−, sCOD, tCOD |
2.2.1 Phosphate release during anaerobic digestion.
Anaerobic tests were performed with FePO4-spiked sewage sludge at different concentrations of feed SO42−: 0, 2000, 4000, and 8000 mg L−1 (experiment 1). Monitoring of PO43− release and SO42− reduction was stopped after 15 days as no further variation was observed. A preliminary test (test 1) was performed to assess the impact of the feed SO42− concentration on biomethane production. For this test, SO42− was added to the bottles at concentrations of 0, 100, 500, 2000, and 4000 mg L−1 and the CH4 production was monitored for 21 days. The test allowed better evaluation of the temporal distribution of PO43− release and SO42− reduction and, thus, fine-tuning the design of experiment 1.
2.2.2 Phosphate release from sewage sludge digestate.
To obtain enough digestate to run the experiments, 5 L of sewage sludge were digested in 2 L glass bottles (similar to those described in section 2.2) for 30 days. The obtained digestate had the following composition: pH 7.7 ± 0.3, TS 29 ± 2 g L−1, VS 15 ± 2 g L−1, tCOD 20
658 ± 825 mg L−1, sCOD 197 ± 14 mg L−1, SO42− 95 ± 11 mg L−1, and PO43− 449 ± 21 mg L−1.
First, anaerobic tests were performed with a similar setup to the AD tests with sewage sludge (except that digestate was used instead of sludge) at feed SO42− concentrations of 0, 2000, 4000, and 6000 mg L−1 (experiment 2). In a following experiment (experiment 3), the digestate was first subjected to thermal hydrolysis (TH) with the aim to enhance COD bioavailability during the BSR stage. Anaerobic bottles were placed in an oven at a temperature of 90 °C for a limited duration. Afterwards, the experiment was run at 35 °C with the same feed SO42− concentrations as in experiment 2. A preliminary test (test 2) was performed with digestate to assess the best duration of the hydrolysis treatment. 500 mL glass bottles were filled with 40 mL of digestate, then closed without tightening the caps, and kept in the oven for different durations, i.e., 0, 1, 2, and 4 h. At the end of the treatment, both the tCOD and sCOD were measured to evaluate the effectiveness of hydrolysis on the release of OM from the digestate. Once the optimal duration was identified based on the amount of solubilized COD and process costs, the digestate was added to the test bottles, hydrolyzed, and supplemented with different SO42− concentrations (as for experiment 2) to evaluate PO43− release.
2.3 Calculations
The mass ratio of P-PO43− released to S-SO42− reduced (P/S) for each bottle during the experiments was calculated based on the linear trends of the PO43− release and SO42− reduction curves and was indicated as P/Slinear. The stoichiometric P/S ratio (P/Sst) was calculated hypothesizing that (1) all reduced SO42− was converted to S2−, and (2) all generated S2− reacted with FePO4, resulting in Fe3+ reduction to Fe2+ and release of PO43− (eqn (1)) followed by Fe2+ sequestration as FeS (eqn (2)):14 | 3Fe(III)PO4 + 1.5S2− ↔ (Fe(II))3(PO4)2 + 1.5S0 + PO43− | (1) |
| (Fe(II))3(PO4)2 + 3S2− ↔ 3FeS + 2PO43− | (2) |
Overall, PO43− release occurring from iron interactions with sulfide can be expressed as the sum of eqn (1) and (2): | 3Fe(III)PO4 + 4.5S2− ↔ 3Fe(II)S + 1.5S0 + 3PO43− | (3) |
The ratio of COD consumed to SO42− reduced (COD/SO42−) was calculated based on the linear trends of SO42− reduction curves based on the available data (days 8–13).
2.4 Analytical methods
TS, VS, and tCOD were measured on unfiltered sludge and digestate samples following the standard methods.22 sCOD and ionic concentrations were measured on the liquid fraction of the samples obtained after filtration through Minisart 0.45
μm syringe filters (Sartorius, Germany). sCOD in filtered liquid samples was measured as tCOD. Ionic concentrations, i.e., PO43−, SO42−, and thiosulfate (S2O32−), were measured by ion chromatography as described by Di Capua et al.23 The pH was measured in unfiltered samples using an InoLab level 2 pH meter (WTW, Germany) with a Polyplast pH electrode (Hamilton, USA). A TCN 115 laboratory oven (Argo Lab, Italy) used for TS and VS analysis was also used for the TH of digestate.
2.5 Statistical analysis
The statistical evaluation of the data was performed based on one-way analysis of variance (ANOVA) with the data analysis tool of Excel 365 (Microsoft, USA) with the aim to compare the PO43− concentrations released from the different anaerobic bottles. The significant difference was considered at 95% (p ≤ 0.05).
3 Results and discussion
3.1 Phosphate release during anaerobic digestion of sewage sludge
The first experiment confirmed that PO43− was effectively released from sewage sludge containing FePO4 during AD (Fig. 1). Test 1 revealed that PO43− release and SO42− reduction at feed SO42− concentrations up to 4000 mg L−1 mainly occurred within the first 7 days and that no considerable difference (p > 0.05) with the reference condition (i.e., 0 mg L−1 feed sulfate) in terms of PO43− released was observed up to 500 mg L−1 feed SO42− (Fig. S1†). Therefore, during experiment 1 the bottles were monitored for 15 days with a more frequent sampling and a higher range of feed SO42− concentrations (2000–8000 mg L−1) was investigated (Table 1). The experiment confirmed that PO43− was mostly released within the first 7–10 days at all tested feed SO42− concentrations. After 15 days, 1911 ± 199 mg L−1 PO43−, corresponding to 62.3% of the PO43− initially present as FePO4 in the sludge (i.e., 1 g P per L), was released at the highest feed SO42− concentration of 8000 mg L−1. As expected, less PO43− was released at lower initial SO42− concentrations. Specifically, 1590 ± 29 and 821 ± 15 mg L−1 PO43− were released at 4000 and 2000 mg L−1 feed SO42−, respectively. Only 452 ± 52 mg L−1 PO43− was released from the sludge with no feed SO42−. High PO43− release from sewage sludge during AD can be observed even in WWTPs where no P removal/recovery strategy is implemented. For instance, Ferraro et al.17 observed a PO43− concentration as high as 436 mg P per L in the digestate centrate produced within a conventional WWTP based on a modified Ludzack–Ettinger scheme. However, it is evident that the SO42− addition promoted PO43− release from the FePO4 used to mimic chemical P precipitation in the mainstream treatment.
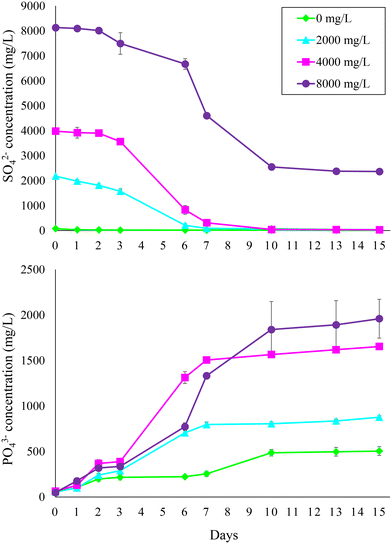 |
| Fig. 1 Sulfate reduction and phosphate release during anaerobic digestion of sewage sludge at different feed sulfate concentrations (i.e., 0, 2000, 4000, and 8000 mg L−1). | |
The link between PO43− release and SO42− reduction is evidenced by the trend of SO42− concentration during the experiment. Under all conditions, SO42− was consumed within the first 7–10 days, matching with the trends of PO43− release. No SO42− was present after 10 days except at 8000 mg L−1 feed SO42−. However, SO42− reduction stopped at day 10 even under this condition, likely indicating that the primary electron donor (i.e., rapidly biodegradable OM) fuelling SO42− reduction was consumed. The SO42− reduction rate was similar at 4000–8000 mg L−1 feed SO42− (683–718 mg L−1 d−1), while it was about half at 2000 mg L−1 (Table 2). Despite the increase of SO42− reduction rate at higher initial SO42− levels, the g of released PO43− per g of reduced SO42− did not increase accordingly but remained quite stable. The P/Slinear ratio calculated under the different operating conditions was in the range of 0.27–0.32 (Table 2). The P/Sst ratio calculated according to eqn (3) was 0.64, i.e., about twice the P/Slinear ratio.
Table 2 Ratios of P-PO43− released to S-SO42− reduced (P/Slinear) based on the linear trends of the PO43− release and SO42− reduction curves obtained with sewage sludge (E1) and hydrolyzed digestate (E3)
Experiment |
Feed SO42− concentration (mg L−1) |
Linear trend period (d) |
P/Slinear |
SO42−reduction rates (mg L−1 d−1) |
E1 |
2000 |
2–7 |
0.32 ± 0.04 |
345 ± 16 |
4000 |
2–7 |
0.31 ± 0.01 |
718 ± 11 |
8000 |
2–10 |
0.27 ± 0.06 |
683 ± 5 |
E3 |
2000 |
8–21 |
0.38 ± 0.01 |
146 ± 2 |
4000 |
8–21 |
0.32 ± 0.05 |
216 ± 56 |
6000 |
8–21 |
0.33 ± 0.04 |
266 ± 29 |
It should be noted that the calculation for P/Sst was based on a number of hypotheses, including the complete reaction of the generated S2− with iron phosphate to free PO43− in the liquid phase. However, the share of S2− that effectively reacts with iron is not 100%, as other sulfide precipitates can be formed, e.g., by sulfide reaction with other metals.24 This can explain the lower P/S ratios observed in comparison to the one calculated stoichiometrically.
Although the maximum P/Slinear value was obtained at the highest feed SO42− concentration of 8000 mg L−1, this would result in a too high residual SO42− level in the sludge at the end of AD due to the lack of available OM to complete the BSR process (Fig. 1). Moreover, as the supplementation of SO42− represents a cost for the proposed P recovery strategy, optimizing the SO42− dosage is necessary to make the process profitable. Besides, the main issue with coupling AD to BSR for P recovery is related to the impact of the generated sulfide on methane production, which is outlined in the following section.
3.2 Effect of the feed sulfate concentration on methane production from sewage sludge
Fig. 2 illustrates the specific methane production obtained during test 1 at the different feed SO42− concentrations. The baseline methane production represented by the reactor with no feed SO42− amounted to 160 ± 12 NL CH4 per kg VSin. The methane production decreased at increasing feed SO42− concentrations, reaching 145 ± 8, 94 ± 12, 64 ± 6, and 26 ± 15 NL CH4 per kg VSin at 100, 500, 2000, and 4000 mg L−1 feed SO42−, respectively. These results indicate that CH4 production was decreased by 9%, 41%, 60%, and 84% at 100, 500, 2000, and 4000 mg L−1 feed SO42− (Fig. 2). Therefore, due to inhibition of methanogenic activity, coupling BSR to AD imposes a trade-off between PO43− release and CH4 production from sewage sludge. A smarter approach might be to perform BSR on the sludge digestate in order to separate the CH4 production from the P recovery stage, as discussed in the next section.
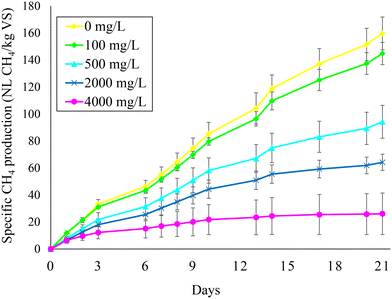 |
| Fig. 2 Methane production during anaerobic digestion at different feed sulfate concentrations (i.e., 0, 100, 500, 2000, and 4000 mg L−1). | |
3.3 Phosphate release from sludge digestate via biological sulfate reduction
Fig. 3 illustrates the PO43− release and SO42− reduction trends obtained with sludge digestate (experiment 2). SO42− concentrations only slightly decreased compared to the initial levels under all operating conditions, with the highest SO42− reduction of 40% achieved at 2000 mg L−1 feed sulfate. The lower SO42− consumption compared to that observed with undigested sludge led to little PO43− release. Indeed, the PO43− released from the digestate after 21 days was very similar (p > 0.05) under all tested feed SO42− concentrations (2000–6000 mg L−1), as it ranged from 420 to 519 mg L−1. This means that a maximum of 16.9% of the initial PO43− linked to Fe(III) was released in the aqueous phase. No significant variation of the PO43− concentration (579 ± 30 mg L−1) was observed in the experiment with no added SO42−, confirming that BSR was the main driver for PO43− release.
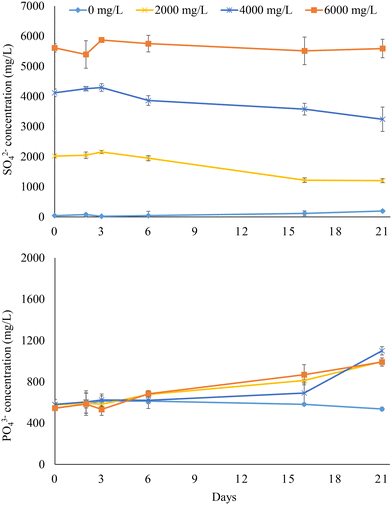 |
| Fig. 3 Sulfate reduction and phosphate release in the anaerobic bottles fed with sludge digestate at different feed sulfate concentrations (i.e., 0, 2000, 4000, and 6000 mg L−1). | |
The digestate was characterized by a tCOD of 20
658 ± 825 mg L−1 and an sCOD of 197 ± 14 mg L−1, being respectively 31% and 80% lower than those of the untreated sludge (Table 3). AD proceeds through the conversion of biodegradable OM into biogas, leaving the least biodegradable organic fraction as a residue. The lower sCOD, which was likely linked to the lower content of the more rapidly biodegradable OM fraction, compared to the untreated sludge explains the limited SO42− reduction in the bottles fed with sludge digestate, indicating that biodegradable OM is the primary energy source fuelling the BSR process. For successful P recovery via BSR from sludge digestate, strategies enhancing COD solubilization and bioavailability are thus necessary, as illustrated in the following section.
Table 3 Soluble and total chemical oxygen demand (sCOD and tCOD, respectively) as well as pH characteristics of sewage sludge, sludge digestate, and hydrolyzed sludge digestate prior to performing the biological sulfate reduction experiments (i.e., experiments 2 and 3)
|
Sewage sludge |
Sludge digestate |
Hydrolyzed digestate |
tCOD (mg L−1) |
30 030 ± 350 |
20 658 ± 825 |
21 013 ± 1563 |
sCOD (mg L−1) |
977 ± 11 |
197 ± 14 |
2077 ± 360 |
pH |
7.3 ± 0.1 |
7.7 ± 0.3 |
7.9 ± 0.1 |
3.4 Impact of thermal hydrolysis on phosphate release from digestate via biological sulfate reduction
TH can represent a solution to increase the amount of sCOD in the sludge digestate and enhance PO43− release through BSR. Table S1† shows how sCOD increased with time when applying a 90 °C TH to the sludge digestate (test 2). After 1 h of hydrolysis, sCOD increased by 6.4 times, reaching 1829 ± 152 mg L−1, with the ratio of sCOD to tCOD (sCOD/tCOD) increasing from 1.4% to 9.0%. Prolonging the hydrolysis time further promoted COD solubilization, although sCOD values observed at 2 and 4 h were quite similar. Indeed, sCOD was 4143 ± 40 mg L−1 at 2 h and 4910 ± 57 mg L−1 at 4 h, resulting in sCOD/tCOD ratios of 20.4% and 24.2%, respectively.
Although slightly better results were obtained after 4 h of hydrolysis in terms of sCOD increase, a duration of 2 h was selected to hydrolyze the sludge digestate in experiment 3 based on lower energy costs and shorter treatment times. Therefore, prior to experiment 3 the reactors used for the tests with different SO42− concentrations were hydrolyzed for 2 h, resulting in a sCOD concentration of 2077 ± 360 mg L−1. The COD concentrations of untreated, digested, and hydrolyzed sludge are listed in Table 3. The sCOD concentration obtained after 2 h of hydrolysis was lower than that observed in test 2 due to the higher amount of sludge in the bottles (300 mL) compared to that of the preliminary test (40 mL).
The SO42− reduction and PO43− release curves of experiment 3 are depicted in Fig. 4. Within the 21 days of the experiment, SO42− was completely reduced when a feed SO42− concentration of 2000 mg L−1 was used, while around 3500 mg L−1 SO42− were consumed at 4000 and 6000 mg L−1 feed sulfate. At these SO42− concentrations, PO43− concentration reached about 1800 mg L−1 at the end of the experiment, yielding between 1400 and 1500 mg L−1 PO43− released. This indicates that up to 48.7% of the PO43− initially associated with Fe(III) could be released.
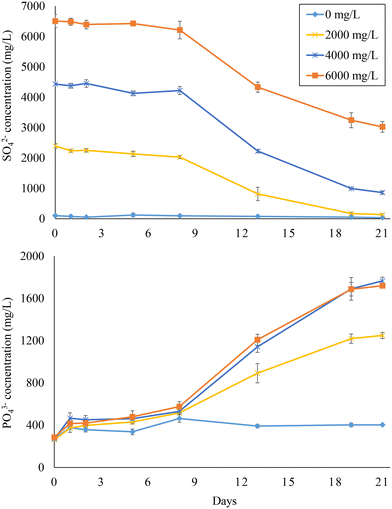 |
| Fig. 4 Sulfate reduction and phosphate release in bottles fed with hydrolyzed (2 h) sludge digestate at different feed sulfate concentrations (i.e., 0, 2000, 4000, and 6000 mg L−1). | |
P/Slinear values (days 8–21) were in the range of 0.32–0.38, close to the ratios observed in experiment 1, while SO42− reduction rates ranged from 146 ± 2 to 266 ± 29 mg L−1 d−1 (Table 2). Both SO42− reduction and PO43− release started after 8 days (lag phase) from the start of the experiment (Fig. 4), which contrasts with the trends observed with sewage sludge where no lag phase was observed (Fig. 1). Also, the SO42− reduction rates were lower than those observed during experiment 1 (Table 2). Most probably, SRB were heat-shocked during the thermal treatment, resulting in the initial lag phase and slower SO42− reduction. However, the thermal treatment worked as an efficient strategy for PO43− release based on the P/Slinear values and final yields. At the end of the BSR tests, no tCOD consumption was observed at 0 mg L−1 feed SO42−, while 40–53% of the initial tCOD was consumed at feed SO42− concentrations of 2000–4000 mg L−1 (Table S2†). The COD/SO42− ratios decreased from 2.4 ± 0.0 to 1.2 ± 0.4 when increasing the feed SO42− concentration from 2000 to 6000 mg L−1. This trend suggests that COD consumption was more effectively driven towards BSR at high SO42− concentrations when concomitant OM-consuming bioprocesses such as AD are inhibited.
3.5 Practical considerations
This study demonstrates that BSR can be an effective strategy to promote P recovery from sewage sludge in WWTPs performing chemical P precipitation. Based on the obtained results, the most suitable configuration of the sludge streamline would foresee the typical pre-thickening and AD stages followed by TH (e.g., at 90 °C for 2 h) and SO42− addition (e.g., 4000 mg L−1) prior to a second anaerobic stage for BSR (Fig. 5). In such a scheme, the formation of iron(II) sulfide and PO43− release would occur in the completely mixed BSR reactor, while the precipitate separation from the P-rich liquid phase would occur in the subsequent dewatering stage. The added SO42− could originate from chemicals such as Na2SO4, being widely produced worldwide also as a by-product of hydrochloric acid production and other chemical processes. As an alternative, SO42− might be generated on site through biogas desulfurization in anoxic biofilters fed with nitrified wastewater25,26 or in aerobic bioreactors fed with the sulfide-rich solution produced by chemical scrubbing. Both strategies would provide an inexpensive source for SO42− supply. When selecting the initial SO42− concentration, it is important to consider and limit the potential increase of free sulfide levels in the digestate. Free sulfide may cause corrosion to metallic installations of the plant.27 To prevent this, residual SO42− levels should be minimized and the sulfide-sink capacity of the digestate evaluated. pH plays an important role in the emission of gaseous H2S.28 Maintaining a slightly alkaline pH during BSR would prevent H2S emission while sustaining the biological process.
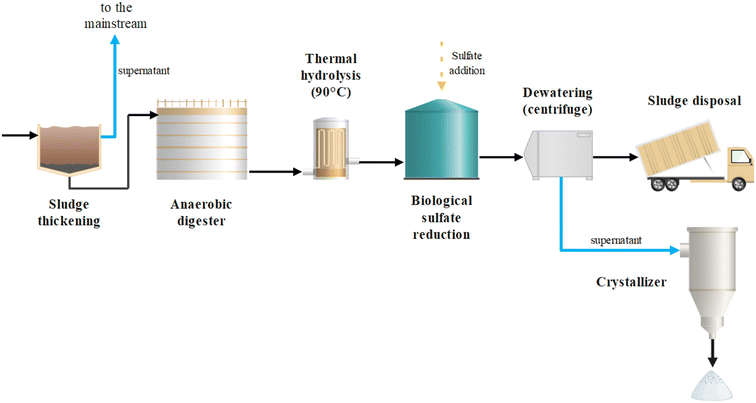 |
| Fig. 5 Layout of a WWTP sludge streamline integrating anaerobic digestion, thermal hydrolysis, and biological sulfate reduction combining energy and P recovery. | |
Dewatering can be performed afterwards to separate the solid fraction from the phosphate-rich supernatant to be used for P recovery. This last step could be performed by adopting one of the existing technologies, including crystallization and electrochemical precipitation systems, which mainly target the generation of P-rich precipitates (e.g., struvite and hydroxyapatite) to be used for fertilizing purposes and selected based on current market requirements.3 As an alternative to crystallization, the PO43− content of the liquid fraction of the digestate could be directly applied to soil in the context of treated water reuse in agriculture, which is being advocated also by the recent EU regulations (Regulation 2020/741). However, it should be noted that P speciation in liquid and solid phase influences its uptake by plants, as PO43− is more readily available than struvite-P, the latter being slowly released to the soil. The use of crystal P can be advantageous to avoid overfeeding phenomena and leaching of nutrients into the aquifers. Moreover, solid fertilizers are easier to handle and transport, which is an important factor to enhance their utilization considering the still limited acceptance of farmers towards the use of waste-derived fertilizers.
The techno-economic convenience of such a proposed P recovery strategy should be assessed through pilot- and full-scale trials, as especially the hydrolysis step seems to be affected by the applied scale and may significantly impact the overall costs of the process. Taboada et al.29 report an investment cost for the TH unit ranging from €1 to 2 million for WWTPs with size from 0.1 to 1 million population equivalent (PE). Considering an electricity cost of €0.12 per kW h and the reported electricity demand of 10 kW h m−3,29 the operational costs for the TH unit would be €1.2 per m3 of digestate (∼€0.01 per m3 of influent wastewater). This cost would be lower for the TH process considered in this work as it is performed at ambient pressure, and the required temperature of 90 °C is much lower than that typically applied for sludge solubilization prior to AD (>150 °C). The cost of Na2SO4 addition would be around €0.4 per m3 of digestate by considering a market price of €109 per ton and an initial SO42− concentration of 4000 mg L−1. The total cost for patented P crystallization technologies ranges between €6 and 10 per kg of P recovered,30 while the application of chemical and biological scrubbers for SO42− generation would cost €0.05–3.3 per kg S (corresponding to €0.7–4.3 per m3 of digestate at 4000 mg L−1 initial SO42−).31 The costs for implementing a P recovery strategy at a WWTP can be counteracted by savings, e.g., due to reduced P back-flow with the digester supernatant resulting in a lower demand of iron precipitants in the mainstream treatment, and revenues from sale of the produced P-rich products.
Implementing a TH stage after the AD process can also provide further solid reduction and enhance dewatering.32 Therefore, this approach will help to minimize the volumes of sewage sludge exiting the municipal WWTPs, which is highly topical considering the increasing amount of sewage sludge produced by wastewater treatment.33–35
4 Conclusions
P recovery from sewage sludge via BSR showed promising results when applied to sludge generated in WWTPs where chemical P precipitation is implemented. The obtained results indicate P recovery from sludge digestate as the most promising process configuration, as it enables keeping AD and BSR separate and avoids affecting the biomethane potential of the sewage sludge due to sulfide inhibition. However, since most of the biodegradable OM is consumed during AD, a 2 h TH at 90 °C was shown to increase the sCOD of the sludge digestate from 197 to 2077 mg L−1 and to ensure almost 50% of PO43− release from FePO4 when 4000 mg L−1 SO42− was fed prior to BSR. Based on these results, pilot- and full-scale trials are recommended for a more appropriate cost evaluation taking into account the costs for the implementation of the hydrolysis and BSR units, the savings in terms of reduced sludge disposal and improved energy recovery, and the revenues from the commercialization of solid P-rich fertilizer products.
Data availability
Data for this article, including tables and figures, are available in the open access repository at the link https://www.iris.unina.it or at the section “publications” of the webpage of the corresponding author: https://www.docenti.unina.it/stefano.papirio.
Conflicts of interest
There are no conflicts to declare.
References
- N. Di Costanzo, A. Cesaro, F. Di Capua and G. Esposito, Exploiting the Nutrient Potential of Anaerobically Digested Sewage Sludge: A Review, Energies, 2021, 14, 8149 CrossRef CAS.
- R. W. Scholz, A. E. Ulrich, M. Eilittä and A. Roy, Sustainable use of phosphorus: A finite resource, Sci. Total Environ., 2013, 461–462, 799–803 CrossRef CAS PubMed.
- F. Di Capua, S. de Sario, A. Ferraro, A. Petrella, M. Race, F. Pirozzi, U. Fratino and D. Spasiano, Phosphorous removal and recovery from urban wastewater, Sci. Total Environ., 2022, 823, 153750 CrossRef CAS PubMed.
- J. Uzkurt Kaljunen, R. A. Al-Juboori, W. Khunjar, A. Mikola and G. Wells, Phosphorus recovery alternatives for sludge from chemical phosphorus removal processes – Technology comparison and system limitations, Sustainable Mater. Technol., 2022, 34, e00514 CrossRef CAS.
- A. Magrí, M. Carreras-Sempere, C. Biel and J. Colprim, Recovery of Phosphorus from Waste Water Profiting from Biological Nitrogen Treatment: Upstream, Concomitant or Downstream Precipitation Alternatives, Agronomy, 2020, 10, 1039 CrossRef.
- F. Carraturo, A. Siciliano, A. Giordano, F. Di Capua, F. Barone, E. Casaletta, F. Cicotti, M. Guida and F. Adani, Ecotoxicological assessment of waste-derived organic fertilizers and long-term monitoring of fertilized soils using a multi-matrix and multi-species approach, Sci. Total Environ., 2024, 912, 169341 CrossRef CAS PubMed.
- S. Papirio, G. Esposito and F. Pirozzi, Biological inverse fluidized-bed reactors for the treatment of low pH- and sulphate-containing wastewaters under different COD/SO42− conditions, Environ. Technol., 2013, 34, 1141–1149 CrossRef CAS PubMed.
- G. Esposito, A. Veeken, J. Weijma and P. N. L. Lens, Use of biogenic sulfide for ZnS precipitation, Sep. Purif. Technol., 2006, 51, 31–39 CrossRef CAS.
- D. K. Villa-Gomez, S. Papirio, E. D. van Hullebusch, F. Farges, S. Nikitenko, H. Kramer and P. N. L. Lens, Influence of sulfide concentration and macronutrients on the characteristics of metal precipitates relevant to metal recovery in bioreactors, Bioresour. Technol., 2012, 110, 26–34 CrossRef CAS PubMed.
- J. F. Blais, Z. Djedidi, R. B. Cheikh, R. D. Tyagi and G. Mercier, Metals Precipitation from Effluents: Review, Pract. Period. Hazard., Toxic, Radioact. Waste Manage., 2008, 12, 135–149 CrossRef CAS.
- E. E. Roden and J. W. Edmonds, Phosphate mobilization in iron-rich anaerobic sediments: microbial Fe (III) oxide reduction versus iron-sulfide formation, Arch. Hydrobiol., 1997, 139, 347–378 CrossRef CAS.
- E. Mejia Likosova, J. Keller, R. A. Rozendal, Y. Poussade and S. Freguia, Understanding colloidal FeSx formation from iron phosphate precipitation sludge for optimal phosphorus recovery, J. Colloid Interface Sci., 2013, 403, 16–21 CrossRef CAS PubMed.
- F. Kato, H. Kitakoji, K. Oshita, M. Takaoka, N. Takeda and T. Matsumoto, Extraction efficiency of phosphate from pre-coagulated sludge with NaHS, Water Sci. Technol., 2006, 54, 119–129 CrossRef CAS PubMed.
- P. Wilfert, J. Meerdink, B. Degaga, H. Temmink, L. Korving, G. J. Witkamp, K. Goubitz and M. C. M. van Loosdrecht, Sulfide induced phosphate release from iron phosphates and its potential for phosphate recovery, Water Res., 2020, 171, 115389 CrossRef CAS PubMed.
- C. Lippens and J. De Vrieze, Exploiting the unwanted: Sulphate reduction enables phosphate recovery from energy-rich sludge during anaerobic digestion, Water Res., 2019, 163, 114859 CrossRef CAS PubMed.
- W. Yao, C.-X. Yang, Y. Lu, Y.-Y. Lu, S.-X. Wang, B.-C. Huang and R.-C. Jin, Enhancing phosphorus release from sewage sludge via anaerobic treatment: State-of-art progress and future challenges, Chem. Eng. J., 2024, 483, 149346 CrossRef CAS.
- A. Ferraro, S. de Sario, A. Attanasio, F. Di Capua, A. Gorgoglione, U. Fratino, M. C. Mascolo, F. Pirozzi, G. Trancone and D. Spasiano, Phosphorus recovery as struvite and hydroxyapatite from the liquid fraction of municipal sewage sludge with limited magnesium addition, J. Environ. Qual., 2023, 52, 584–595 CrossRef CAS PubMed.
- F. Di Capua, D. Spasiano, A. Giordano, F. Adani, U. Fratino, F. Pirozzi and G. Esposito, High-solid anaerobic digestion of sewage sludge: challenges and opportunities, Appl. Energy, 2020, 278, 115608 CrossRef CAS.
- N. Y. Mezenner and A. Bensmaili, Kinetics and thermodynamic study of phosphate adsorption on iron hydroxide-eggshell waste, Chem. Eng. J., 2009, 147, 87–96 CrossRef CAS.
- R. Morello, F. Di Capua, L. Pontoni, S. Papirio, D. Spasiano, U. Fratino, F. Pirozzi and G. Esposito, Microaerobic Digestion of Low-Biodegradable Sewage Sludge: Effect of Air Dosing in Batch Reactors, Sustainability, 2021, 13, 9869 CrossRef CAS.
-
N. Bachmann, Sustainable biogas production in municipal wastewater treatment plants, Technical report, IEA Bioenergy, 2015 Search PubMed.
-
APHA, Standard Methods for the Examination of Water & Wastewater, American Public Health Association (APHA), American Water Works Association (AWWA), Water Environment Federation (WEF), Washington, DC, 21st edn, 2005 Search PubMed.
- F. Di Capua, M. C. Mascolo, F. Pirozzi and G. Esposito, Simultaneous denitrification, phosphorus recovery and low sulfate production in a recirculated pyrite-packed biofilter (RPPB), Chemosphere, 2020, 255, 126977 CrossRef CAS PubMed.
- A. E. Lewis, Review of Metal Sulphide Precipitation, Hydrometallurgy, 2010, 104, 222–234 CrossRef CAS.
- R. Khanongnuch, F. Di Capua, A. M. Lakaniemi, E. R. Rene and P. N. L. Lens, H2S removal and microbial community composition in an anoxic biotrickling filter under autotrophic and mixotrophic conditions, J. Hazard. Mater., 2019, 367, 397–406 CrossRef CAS PubMed.
- R. Khanongnuch, F. Di Capua, A. Lakaniemi, E. R. Rene and P. N. L. Lens, Transient–state operation of an anoxic biotrickling filter for H2S removal, J. Hazard. Mater., 2019, 377, 42–51 CrossRef CAS PubMed.
- N. Di Costanzo, F. Di Capua, A. Cesaro, F. Carraturo, M. Salamone, M. Guida, G. Esposito and A. Giordano, Headspace micro-oxygenation as a strategy for efficient biogas desulfurization and biomethane generation in a centralized sewage sludge digestion plant, Biomass Bioenergy, 2024, 183, 107151 CrossRef CAS.
- D. Rathnayake, K. C. Bal Krishna, G. Kastl and A. Sathasivan, The role of pH on sewer corrosion processes and control methods: A review, Sci. Total Environ., 2021, 782, 146616 CrossRef CAS PubMed.
- A. Taboada-Santos, J. M. Lema and M. Carballa, Energetic and economic assessment of sludge thermal hydrolysis in novel wastewater treatment plant configurations, Waste Manage., 2019, 92, 30–38 CrossRef CAS PubMed.
- L. Egle, H. Rechberger, J. Krampe and M. Zessner, Phosphorus recovery from municipal wastewater: An integrated comparative technological, environmental and economic assessment of P recovery technologies, Sci. Total Environ., 2016, 571, 522–542 CrossRef CAS PubMed.
-
M. Arnold, Reduction and monitoring of biogas trace compounds, Espoo, 2009 Search PubMed.
- C. Cai, C. Hu, W. Yang, Y. Hua, L. Li, D. Yang and X. Dai, Sustainable disposal of excess sludge: Post-thermal hydrolysis for anaerobically digested sludge, J. Cleaner Prod., 2021, 321, 128893 CrossRef CAS.
- R. Morello, F. Di Capua, G. Esposito, F. Pirozzi, U. Fratino and D. Spasiano, Sludge minimization in mainstream wastewater treatment: Mechanisms, strategies, technologies, and current development, J. Environ. Manage., 2022, 319, 115756 CrossRef CAS PubMed.
- R. Morello, F. Di Capua, E. Sahinkaya, G. Esposito, F. Pirozzi, U. Fratino and D. Spasiano, Operational strategies enhancing sewage sludge minimization in a combined integrated fixed-film activated sludge – oxic settling anaerobic system, J. Environ. Manage., 2023, 345, 118808 CrossRef CAS PubMed.
- R. Morello, F. Di Capua, Ç. Kalkan Aktan, T. Yilmaz, G. Esposito, F. Pirozzi, U. Fratino, D. Spasiano and E. Sahinkaya, Unravelling the impact of oxic-settling-anaerobic cycle implementation and solid retention time on sludge generation, membrane operation, and contaminant removal in membrane bioreactors, Chem. Eng. J., 2024, 496, 153800 CrossRef CAS.
|
This journal is © The Royal Society of Chemistry 2024 |
Click here to see how this site uses Cookies. View our privacy policy here.