DOI:
10.1039/D3SU00471F
(Tutorial Review)
RSC Sustain., 2024,
2, 832-851
Nanomaterial-based electrochemical chemo(bio)sensors for the detection of nanoplastic residues: trends and future prospects
Received
18th December 2023
, Accepted 8th February 2024
First published on 8th February 2024
Abstract
Nanoplastic (NPL) residues, minute plastic particles measuring less than 1 micron (μm), have become pervasive in aquatic ecosystems, infiltrating through various sources such as wastewater treatment, industrial discharges, and plastic degradation. The escalating global concern over the extensive pollution caused by NPL residues underscores the critical need for effective detection and monitoring methods to comprehensively understand its prevalence, impacts, and potential mitigation strategies. In this context, electrochemical chemo(bio)sensors emerge as a promising solution for detecting NPL residues. Offering a unique combination of specificity, sensitivity, rapid response times, and the ability to detect trace contaminants in complex matrices, these chemo(bio)sensors are poised to play a pivotal role in addressing the challenges posed by NPL residue pollution. This review exclusively delves into electrochemical methods and materials, encompassing nanomaterials such as stable carbon, noble metals, metal oxides, and polymer films. Furthermore, it explores chemo(bio)sensors utilizing enzymes, antibodies, and aptamers for NPL residue detection. The review critically examines the challenges inherent in developing electrochemical chemo(bio)sensors, including considerations related to recognition elements and their practical applications. Additionally, it offers insights into future prospects, paving the way for advancements in this field. By addressing these challenges and exploring innovative solutions, electrochemical chemo(bio)sensors hold the promise of safeguarding aquatic environments from the detrimental effects of NPL residues. The overarching goal of this review is to raise awareness regarding NPL residues and to underscore the potential of electrochemical chemo(bio)sensors in protecting global water and environmental resources. The specific focus on electrochemical approaches aims to provide a comprehensive understanding of these techniques' role in tackling the pressing issue of NPL residue pollution.
Sustainability spotlight
Nanoplastic (NPL) residues are infiltrating our aquatic ecosystems and are now a serious global concern, demanding effective detection and monitoring methods to implement the actions and control measures. Electrochemical chemo(bio)sensors are very promising for NPL residues detection and in safeguarding our aquatic environments for future sustainable developments.
|
1 Introduction
The escalating global concern surrounding nanoplastic (NPL) residues pollution in aquatic ecosystems necessitates a comprehensive understanding of the associated environmental and health risks. These minute plastic particles infiltrate water bodies through diverse sources, including urban sewage, industrial effluents, polluted rainwater, and the fragmentation of larger plastic debris (Fig. 1).1–7 According to estimates from various datasets, the quantity of plastics in aquatic ecosystems has witnessed a substantial increase. Reports indicate an escalation of 7000 tonnes to as much as 250
000 tonnes in recent years.8,9 Projections suggest that this volume could surge to a staggering 270 million tonnes by 2060.10 NPL residues, recognized as an emerging anthropogenic contaminant, have been detected across various aquatic environments, posing significant threats to marine organisms and potentially impacting human health through the consumption of contaminated seafood.11–14 Once in aquatic ecosystems, they can persist for long periods of time and accumulate in various organisms and environmental compartments. NPL residues pose serious threats to our natural resources.15–22 The taxonomy of NPL residues encompasses a diverse array of compounds, prominently featuring various phenolic substances such as bisphenol A, hydroquinone, catechol, and resorcinol,23,24 alongside diverse polymers including polystyrene, polyamide, and polyethylene.25 These compounds, integral to industrial processes and consumer goods production, infiltrate aquatic ecosystems through multifaceted pathways, significantly contributing to their pervasive presence. The direct impact of NPL residues on aquatic organisms manifests in physical damage, reduced feeding efficiency, and altered behavior. Moreover, these residues have the capacity to adsorb and transport other contaminants, potentially causing harm to organisms and disrupting ecosystems.26–30 Effectively addressing the challenges posed by NPL residue pollution necessitates the deployment of quantification methods capable of assessing distribution, contamination extent, and ecological and human health impacts.31–33 Although traditional analytical techniques like gas chromatography,34 high-performance liquid chromatography,35 and mass spectrometry36 are accurate, they present limitations, as outlined in Table 1. In response, our research ventures into the forefront of NPL residue detection by ingeniously incorporating nanomaterial-based electrochemical techniques, thereby augmenting the capabilities of established methodologies. This synergistic approach not only complements traditional detection techniques but also introduces cost-effectiveness, mobility, and the potential for real-time, on-site monitoring.37 The strategic focus on electrochemical chemo(bio)sensors is pivotal, given their advantages over conventional methods, including cost-effective operation, simplicity in transportation, quick response times, and continuous monitoring capabilities.38,39
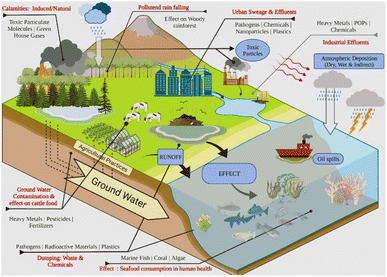 |
| Fig. 1 Schematic illustration depicting the origin and release of mixed pollutants (NPL residues) in the ecosystem. Reprinted from ref. 40, copyright (2022), with permission from Elsevier. | |
Table 1 Advantages and disadvantages of conventional methods and sensors
Aspect |
Conventional methods |
Sensors |
Advantages
|
Real-time monitoring |
Limited real-time data |
Provide real-time data |
Portability |
Lab-based |
Portable and versatile |
Cost-effectiveness |
Resource-intensive |
Generally cost-effective |
Reduced reagent usage |
High reagent use |
Lower reagent consumption |
Continuous monitoring |
Batch processing |
Continuous data streams |
![[thin space (1/6-em)]](https://www.rsc.org/images/entities/char_2009.gif) |
Disadvantages
|
Calibration |
Calibration is often necessary for precision |
Require regular calibration for accuracy |
Limited sensitivity |
Conventional methods may not be effective to detect the lower levels |
Some sensors have limited sensitivity |
Limited lifespan |
Instruments may have a limited operational lifespan |
Operational lifespan may be limited |
Energy |
Some methods may be energy-intensive |
Energy consumption can be a concern |
Size and form factor |
Conventional equipment may be bulky |
Size and form factor may limit applications |
Mitigating NPL pollution necessitates a comprehensive strategy that entails stringent waste management, the promotion of recycling, and the utilization of biodegradable materials. Regulatory efforts must be directed towards enforcing rules on plastic production and limiting microplastics, while public awareness campaigns play a crucial role in educating individuals on responsible plastic use. Essential components of this strategy encompass innovative technologies, efficient removal method research, encouragement of alternative materials, and sustainable packaging. Collaborative efforts with industries to reduce plastic release and advancements in water treatment technologies for NPL removal are critical. Supporting research, fostering collaboration, and instituting continuous monitoring contribute to a collective effort for a more sustainable environment.
This review meticulously narrows its focus to electrochemical chemo(bio)sensors, leveraging their advantages over optical methods, especially in inspecting turbid specimens. Various nanomaterials, including graphene, carbon nanotubes, metal nanoparticles, metal oxides, and hybrid nanocomposites, take center stage in NPL residue detection, offering innovative solutions due to their exceptional properties.40–47 Furthermore, the ability of electrochemical NPL residue sensors to function across a wide range of potentials holds promise for thorough water quality monitoring.48–51 Our review systematically explores the contributions of diverse nanomaterials to the field, shedding light on their roles in enhancing sensor performance and expanding the horizons of environmental monitoring.
Given the significance of electrochemical NPL residue sensors, our recent publication provides a comprehensive summary of the latest developments in sensors and their applications for water monitoring.23,52–55 While numerous review papers exist on NPL residue (bio)sensors, a noticeable gap persists regarding simultaneous electrochemical monitoring of different NPL residues. This review aims to bridge this knowledge gap, offering a comprehensive overview of recent developments in nanomaterial-based electrochemical chemo(bio)sensors. By systematically addressing challenges, summarizing advancements, and amalgamating essential ideas, our study seeks to elevate the awareness of NPL residues concerns. Furthermore, it aims to facilitate understanding for researchers without an extensive background in electroanalytical chemistry and sensor technology, thereby fostering future sustainable developments in this critical area.
2 Electrochemical sensing methods for NPL residues detection
Electrochemical techniques have been used to identify waterborne contaminants, proving their usefulness. These methods are favoured over other analytical techniques because of their price, portability, user-friendliness, and speed.56 The sensors and bio-sensors described in this study include voltammetric/amperometric and impedimetric, which are divided into groups according to the particular signal to be quantified – current, potential, or impedance. These approaches have made it easier to develop (bio)sensors that can quickly and continuously analyse NPL residues in complex sample matrices such as tap and river water.57
2.1 Voltammetric and amperometric methods
Voltammetry encompasses a range of electrochemical methods, including cyclic voltammetry, differential pulse voltammetry, and square wave voltammetry. These techniques are based on measuring the current response of an electrochemical cell while applying a potential sweep or pulse to the working electrode. The variations in the current can provide valuable information about the presence and concentration of NPL residues. We briefly discuss some of the most commonly used electroanalytical techniques here, as we have recently reported detailed information on electroanalytical techniques in another publication.58
2.1.1 Cyclic voltammetry (CV).
One of the most popular electrochemical techniques, CV allows for flexibility in the analysis of many electrochemical systems. It measures the amount of electric current produced inside an electrochemical cell when the voltage rises above what the Nernst equation predicts. A CV experiment involves a linear time-sweep of the voltage applied to the working electrode. It starts at a given initial potential and is then ramped in one direction to a given potential (scan direction) and is finally ramped back to the initial potential in the opposite direction (reverse direction). The potential range over which this sweep occurs is called the scan range. The resulting current is recorded during the voltage sweep and a plot of current versus applied voltage is obtained. This plot is called a cyclic voltammogram or CV scan. CV offers qualitative insights into the electrochemical phenomena occurring within the system. It has the capacity to uncover the existence of intermediate compounds in redox reactions and assess the reversibility of a given chemical reaction. Reversible reactions show symmetrical peaks in the CV scan, while irreversible reactions show asymmetrical peaks. Through the examination of the voltammogram's peak characteristics and their positions, scientists have the capability to ascertain the electron stoichiometry of the system and calculate the diffusion coefficient of an analyte (based on the peak width), and the formal potential (the midpoint potential at which the redox reaction is half complete).56
2.1.2 Differential pulse voltammetry (DPV).
DPV is derived from linear sweep voltammetry (LSV), a common electrochemical technique in which the potential is linearly and consistently varied over time. In DPV, the linear sweep is superimposed with periodic voltage pulses. Following every pulse, there is a short interval where the current is quantified before any alterations in potential occur. Just prior to each forthcoming potential adjustment, an instantaneous current measurement is taken, and the resulting current difference is graphically represented relative to the applied potential.59 Due to the proximity of current sampling to potential transitions in DPV, the influence of the charging current is reduced. This adaptable electrochemical technique is useful for exploring reaction processes, kinetics, and the thermodynamics inherent in chemical reactions and for quantitative chemical assessment. Due to its unique approach and short pulse duration, DPV has a remarkable sensitivity that allows it to successfully identify analytes even at extremely low concentrations, frequently in the parts per billion (ppb) range. Furthermore, given that there is no overlap in their individual redox potentials, DPV can distinguish a variety of analytes in a single trial (sweep). This characteristic makes it easier to recognize and distinguish between various analytes simultaneously. However, DPV has several drawbacks, just like other voltammetric techniques. The likelihood of interference caused by electroactive chemicals found in complex matrices is the main cause of these worries. Accurately differentiating and quantifying the intended analytes can be challenging.60
2.1.3 Square wave voltammetry (SWV).
In SWV, a balanced square wave is superimposed over a staircase waveform to represent the stimulation waveform. A recurrent pattern known as a square wave is defined by equally spaced positive and negative voltage excursions.61 The current is frequently measured near the end of each potential pulse, a time of stability when the potential is constant to reduce the impact of the charging current. The advancing and receding regions of the SWV voltammogram, respectively, show the currents accumulated during the progressive and regressive potential pulses. This makes it easier to compare and evaluate the redox processes taking place in the advancing and receding images. The net current is determined by computing the disparity between the forward and backward currents associated with each potential pulse. This differential nature increases sensitivity and provides a clear and distinguishable signal from non-faradaic or charging currents. This technique is a versatile electrochemical technique that has applications in various fields, including electrokinetic measurements (e.g., electrochemical kinetics) and analytical measurements (e.g., quantitative analysis of analytes).62 This voltammetry method has several advantages, including remarkable sensitivity, a quick turnaround for analyses, and efficient separation from non-faradaic or charging currents. Additionally, the use of differential measurement helps to reduce background noise and improve the signal-to-noise ratio.
2.1.4 Amperometry.
The amperometric method is an electrochemical technique used to quantitatively analyse a substance by measuring the electric current generated in an electrochemical cell.63–67 An electrochemical cell with working, reference, and auxiliary electrodes is submerged in an electrolyte solution in the setup. At the surface of the working electrode, the analyte of interest experiences a particular electrochemical reaction. Depending on the analyte, this reaction may be an oxidation or reduction. An electric current is created by the reaction, which releases electrons that move through the circuit. A steady potential (voltage) is provided between the working and reference electrodes to power the electro-chemical process. The desired redox reaction of the analyte is promoted by setting this potential at a certain value. The electrochemical reaction's current is measured using an ammeter. Following Faraday's law of electrolysis, the current's size is directly proportional to the analyte's concentration. Normally, a calibration curve is created to determine the analyte concentration in a sample. This involves measuring the current at various known concentrations of the analyte and plotting a current concentration graph. The method's sensitivity is indicated by the calibration curve's slope, which also enables the quantification of unidentified amounts. The high sensitivity, relatively straightforward apparatus, and applicability to a broad range of analytes are the benefits of the amperometric approach. It is widely employed in many industries, including medicines, food analysis, environmental monitoring, and clinical diagnostics. The amperometric approach does have certain drawbacks though, such as electrode fouling, outside interference, and meticulous electrode preparation and maintenance.
2.2 Electrochemical impedance spectroscopy (EIS)
EIS is an electrochemical method for measuring an interface's electrical impedance under steady-state alternating current conditions with constant direct current bias. The system is subjected to a tiny sinusoidal voltage at various frequencies, and the current response as a result is monitored.68 EIS is particularly effective at probing the electrode/electrolyte interface and providing valuable information about the properties and processes occurring at this interface. It can detect changes in impedance at the electrode/electrolyte interface as target biomolecules bind to surface-immobilized biorecognition elements. This makes it a powerful tool for detecting complex formations such as antibody–antigen or aptamer–target interactions. In a broader context, when compared to DC techniques or single-frequency measurements, EIS offers more detailed data on the sensor system. EIS can identify diffusion-limited events, separate several electrochemical processes occurring simultaneously, and provide label-free detection. It does require the presence of a dissolved redox species, typically added to the electrolyte, to facilitate impedance measurements.69 However, aside from the redox probe, no other specific reagents are needed. EIS is therefore one of the most crucial detection methods for (bio)sensors because of a number of well-known benefits, including a broad measurable range and strong detection stability.70
3 Nanomaterial based electrochemical sensors for NPL residues detection
Nanomaterials, with their unique properties arising from their nanoscale dimensions, offer distinct advantages for enhancing the sensitivity and efficiency of electro-chemical sensors.71,72 These materials can be made to have particular qualities, such as a large surface area, superior conductivity, and increased catalytic activity, which are essential for creating platforms that effectively detect NPL residues.73 This section provides an overview of recent advancements in nanoplastic detection using electrochemical sensors, highlighting the particularly inventive features made possible by cutting-edge nano-materials. Based on the substrate surface modifications, we classify and briefly provide electrochemical sensors, as shown in Table 2. We also examine the current state and potential uses of electrochemical nanoplastic sensors, taking into account both their analytical capabilities and novel applications. The creation of a small electrochemical framework with the goal of monitoring and controlling water and environmental contamination through quick NPL residue detection has been the focus of academic research for the last two decades. The monitoring of NPL residues in water sources and its consequences for human well-being emerges as a key aspect of the fusion of nanotechnology, employing sophisticated nanomaterials combined with electrochemical techniques.
Table 2 Performance summary of various nanomaterial-based modified electrodes towards the detection of distinct types of NPL residuesa
NPL residues |
Modified electrode |
Technique |
LOD (μM) |
LDR (μM) |
Sample |
Ref. |
CoFe2Se4: cobalt–iron selenides; PCF: porous carbon nanofibers; COFs: covalent-organic frameworks; TpBD: 1,3,5-triformylphloroglucinol and benzidine; UiO-66-NDC: metal–organic framework; CPE: carbon paste electrode; CaCu2O3: nanorod-shaped; MCPE: modified carbon paste electrode; ZnO/Co3O4: zinc oxide/cobalt oxide; P(GN)MGPPE: glutamine-activated graphite paste electrode; MnO2NRs/GO: manganese dioxide nanorods/graphene oxide nanocomposite; Ni/N-GO: Ni/N-doped graphene oxide; NS-MC: N and S dual doped mesoporous carbon; CTpPa-2: porous crystalline covalent organic framework; La2O3: lanthanum oxide; SDS: sodium dodecyl sulfate; CT: catechol; 2-AP: 2-aminophenol; 2-CP: 2-chlorophenol; 2-NP: 2-nitrophenol.
|
CC, HQ |
P4VPBA/PPy/GO/GCE |
CV/DPV |
0.96 |
7.0–16 |
Tap water |
74
|
0.53 |
4.0–22 |
CC, HQ, BPA, phenol |
AgNPs/MWCNT/GCE |
SWV |
0.20, 0.16, 2.40, 3.00 |
20–260, 2.5–260, 5.0–152, 2.4–152 |
Tap water |
75
|
HQ, CC, RS |
CoFe2Se4/PCF/GCE |
DPV |
0.13 |
0.5–200 |
Lake water |
76
|
0.15 |
0.5–190 |
1.36 |
5.0–350 |
CC, HQ, RC |
CB/AuSNPs/SNGCE |
DPV |
1.70 |
5–350 |
Tap, dam, and swamp water |
23
|
5.10 |
5–350 |
4.50 |
5–350 |
HQ, RS |
ZnO/Co3O4-MCPE |
CV/DPV |
3.22 |
1–100 |
Tap water |
77
|
2.92 |
10–60 |
CC |
CS/MWCNTs/PDA/AuNPs/GCE |
DPV |
0.95 |
0.1–10 |
Tap and lake water |
78
|
CC |
P(GN)MGPPE |
CV |
0.045 |
2.0–200 |
Water samples |
79
|
BPA |
AuNPs@TpBD-COFs/GCE |
DPV |
1 |
5–1000 |
Lake water |
80
|
BPA |
UiO-66-NDC/GO/CPE |
DPV |
0.025 |
10–70 |
Tap and drinking water and fresh liquid milk |
81
|
HQ |
MnO2NRs/GO/GCE |
DPV |
0.012 |
0.5–300 |
River water and tap water |
82
|
HQ, CC |
Ni/N-GO/GCE |
DPV |
0.16 |
1.0–800 |
Tap water |
83
|
0.06 |
1.4–800 |
2-AP |
CaCu2O3/GCE |
CV |
0.00574 |
0.175–68 |
Tap water and agricultural water |
84
|
2-CP |
0.00138 |
0.050–90 |
2-NP |
0.00103 |
0.025–32 |
4-(Methylamino)phenol |
LSO@f-HNT/GCE |
DPV |
0.0021 |
0.01–480 |
River and tap water |
85
|
HQ, CC, BPA, BPS |
Mesoporous nickel/GCE |
DPV |
0.0053, 0.0057, 0.0056, 0.0615 nM |
0.03–3.0, 2.0–18.2, 0.05–0.7, 1.0–35.0 |
Tap water |
86
|
HQ, CC |
N and S dual doped mesoporous carbon (NS-MC)/GCE |
DPV |
0.69 |
2–40 |
River stream water |
87
|
0.39 |
2–40 |
BPA |
MWCNTs/GCE |
DPV |
2–30 |
0.5 |
River water |
88
|
BPA |
Covalent organic framework CTpPa-2/GCE |
DPV |
0.1–50 |
0.02 |
Mineral water |
89
|
HQ, CT, RS |
La2O3 nanoparticles@snowflake-like Cu2S |
DPV |
0.66–266.6 |
0.056, 0.058, 0.059 |
Tap water |
90
|
CC, RS |
SDS modified graphene paste electrode |
CV |
0.106 |
2–10 |
Tap water |
91
|
15–150 |
3.1 Carbon based nanomaterials
Carbon-based electrochemical sensors exhibit significant potential in identifying NPL residues within water systems. These sensors achieve accurate and sophisticated nano-plastic particle identification by taking advantage of the specific properties of carbon-based materials. Nevertheless, recent investigations into the advancement of electro-chemical sensors based on nanostructured carbon materials, including carbon nanotubes, carbon dots, and graphene/graphene oxide (GO), have been relatively limited for the detection and localization of NPL residues. This section conducts a comprehensive examination of the current progress in nanostructured carbon-based electrochemical sensors, encompassing their fabrication techniques and their electrochemical efficacy in discerning a di-verse array of nanoplastic variations.
3.1.1 Carbon nanotubes (CNTs).
CNTs are divided into single-walled carbon nanotubes (SWCNTs) and multi-walled carbon nanotubes (MWCNTs), and are one of the most commonly employed carbon nanomaterials for electrochemical sensing applications.92,93 They are suitable for detecting low concentrations of NPL residues due to their high surface-to-volume ratio and strong electrical conductivity. Numerous sensors have been devised using carbon nanotubes for the purpose of detecting and quantifying NPL residues. In their work utilizing DPV, Aragon et al.94 designed an electrochemical sensor that incorporated AuNPs, electrochemically reduced graphene oxide (ErGO), and carboxylated multi-walled carbon nanotubes (cMWCNT) to enable the concurrent detection of NPL residues like hydroquinone (HQ), catechol (CC), and resorcinol (RS). The specifics of the GCE modification processes are shown in Fig. 2a. Under ideal conditions, the LODs of the GCE/ErGO-cMWCNT/AuNP sensor were 0.39 μM, 0.54 μM, and 0.61 μM, respectively, for the linear concentration ranges of HQ, CC, and RS of 1.2–170 μM and 2.4–400 μM, respectively (Fig. 2b). The detection of NPL residues in tap water resulted in an average recovery rate of 107.11%. The enhanced electrocatalytic properties of cMWCNT, attributed to their larger number of active edge surfaces and carboxyl groups that facilitate nanoplastic binding, contributed to this improved performance. The proposed sensor demonstrated rapid, precise, and user-friendly NPL residue monitoring within complex samples, exhibiting remarkable sensitivity. Additionally, Ali et al.95 devised a cost-effective and practical electrochemical sensor for the detection of BPA in water by employing chemically modified MWCNTs with cyclodextrin (CD) on a screen-printed carbon electrode (SPCE) (see Fig. 2c).
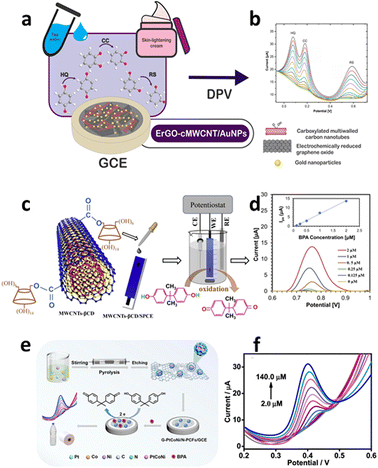 |
| Fig. 2 Examples of CNT-based sensors used to detect NPL residues – graphical abstract: (a) step-by-step approach used to create the GCE/ErGO-cMWCNT/AuNPs, and (b) DPV voltammogram showing the effect of increasing the concentrations of HQ, CC, and RS in PBS. Reproduced under the terms of the CC-BY license from ref. 94 [Biosensors], Copyright 2021, The Authors, published by MDPI. Graphical abstract: (c) a modified MWCNT-based electrochemical sensor for BPA detection with a CD on a SPCE, and (d) DPV voltammogram recorded at MWCNTs-CD/SPCE for increasing BPA concentrations. Reprinted from ref. 95, copyright (2020), with permission from Elsevier. (e) Schematic showing the G-PtCoNi/N-PCF synthetic route and application as an electrode material for BPA detection and (f) DPV voltammograms recorded at G-PtCoNi/N-PCFs/GEC for increasing BPA concentrations (2–140 μM). “Reprinted (adapted) with permission from {ref. 96}. Copyright {2023} American Chemical Society”. | |
The sensor exhibited a two-phase linear response within the concentration range of 0.125 μM to 2 μM (see Fig. 2d) and 2 μM to 30 μM under optimal conditions, accompanied by correlation coefficients of 0.997 and 0.995, respectively. The LOD for BPA was estimated to be 13.76 nM (SNR = 3).95
The increased detection effectiveness was related to MWCNT CDs' capacity to interact with BPA both as a guest and host as a result of interactions between their high surface area and hydrophilic nature. The sensors demonstrated great repeatability (RSD = 4.7%) and constant response over a period of five weeks, and they were very sensitive to normal chemical entities in water. The sensor's recovery of BPA in tap and lake water varied from 96.05% to 108.70% over the course of reliability testing.95 Zhu et al.96 introduced another electrochemical sensor designed for the detection of BPA. This sensor utilized a graphitic carbon-coated PtCoNi alloy supported on N-doped porous carbon nanoflakes (G-PtCoNi/N-PCFs), as shown in Fig. 2e. The incorporation of a graphitic carbon shell notably enhanced the catalytic stability of BPA. Under optimal conditions, the resultant sensor effectively detected BPA in thermal paper and plastic bottles, as illustrated in Fig. 2f, showcasing exceptional catalytic activity and analytical performance. It exhibited a wide linear detection range spanning from 2.0 to 140.0 μM and achieved a LOD of 0.19 μM (S/N = 3).
3.1.2 Carbon dots (CDs).
CDs represent a category of nanomaterials derived from carbon and exhibit distinctive optical and electrochemical characteristics. They are small, zero-dimensional carbon nanoparticles, typically less than 10 nm in diameter.97 CDs have garnered substantial interest in recent times owing to their exceptional biocompatibility, minimal toxicity, chemical resilience, and adjustable characteristics.98 Electrochemical sensing using CD-based materials has shown promise for the detection of NPL residues in water due to its sensitivity, selectivity, and cost effectiveness. Some papers use CDs for the electrochemical sensing of NPL residues in water. Conversely, Wei et al.99 proposed an electrochemical sensor based on a modified GCE incorporating Nafion/multi-walled carbon nanotubes/carbon dots/multi-walled carbon nanotubes (Nafion/MWCNTs/CDs/MWCNTs) for the simultaneous determination of three NPL residues: HQ, CC, and resorcinol (RS). The simultaneous analysis of these compounds was conducted via DPV. The resulting peak current exhibited a linear relationship with the concentrations of HQ, CC, and RS within the ranges of 1.0 to 200.0 μM, 4.0 to 200.0 μM, and 3.0 to 400.0 μM, respectively. The LODs for HQ, CC, and RS were found to be 0.07 μM, 0.06 μM, and 0.15 μM, respectively (S/N = 3). This modified electrode was effectively employed for the detection of these NPL residues in tap water, well water, and river water, with recoveries ranging from 100.3% to 109.8% for HQ, 96.0% to 105.8% for CC, and 83.4% to 101.6% for RS. Similarly, Zhan et al.100 developed a novel electrochemical sensor for the detection of BPA utilizing a GCE modified with metal–organic frameworks (MOFs), AuNPs, and electro-reduced carbon (ErCD) dots (AuNPs-ErCDs-MOF/GCE) (see Fig. 3a). The electrochemical behaviour of this proposed sensor was thoroughly investigated through CV, EIS, and DPV. The results revealed that the AuNPs-ErCDs-MOFs/GCE sensor, featuring a LOD of 32 nmol L−1 (S/N = 3), exhibited an extensive linear range spanning from 7.0 × 10−8 mol L−1 to 5.0 × 10−7 mol L−1 and 5.0 × 10−7 mol L−1 to 1.3 × 10−6 mol L−1 for BPA (refer to Fig. 3b and c). Moreover, this proposed sensor was successfully applied to the analysis of BPA in plastic products, yielding satisfactory recovery results. Additionally, Rajesh et al.101 developed an electrochemical sensor based on a carbon dot-vanadium pentoxide (CDs-V2O5) nanoporous material-modified GCE (CDs-V2O5/GCE) for the detection of BPA (as depicted in Fig. 3d). The electrochemical behaviour of the CDs-V2O5/GCE sensor for BPA detection in the concentration range of 5.0 × 10−9 M to 9.2 × 10−3 M was systematically examined using CV and EIS techniques (see Fig. 3e). The electrochemical assessment confirmed that the CDs-V2O5/GCE sensor possessed a substantial electrochemical surface area, featuring a maximum sensitivity of 0.0681 μA μM−1, enabling the detection of BPA at concentrations as low as 8.0 × 10−10 μM (see Fig. 3f). This proposed sensor was also applied to the analysis of BPA in milk and drinking water samples.
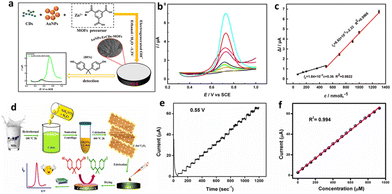 |
| Fig. 3 Nanoplastic electrochemical sensors based on carbon dots: (a) preparation (one-pot) of the AuNPs-ErCDs-MOF composite and construction of the BPA electrochemical sensor, (b) DPV voltammogram recorded at AuNPs-ErCDs-MOFs/GCE for increasing BPA concentrations in PBS (pH = 7), and (c) corresponding calibration plot. Reprinted from ref. 100, copyright (2021), with permission from Elsevier. (d) Graphical abstract – synthesis route of the C-dot-V2O5 nanoporous material and electrochemical sensing approach, (e) amperometric i–t curve of C-dot-V2O5/GCE at BPA concentration from 5 × 10−9 M to 9.2 × 10−3 M, and (f) calibration curve of current versus BPA concentration. Reprinted from ref. 101, copyright (2022), with permission from Elsevier. | |
3.1.3 Graphene (GR)/graphene oxide.
Graphene and graphene oxide are two-dimensional carbon-based nanomaterials that have attracted considerable attention in various fields, including electrochemical sensing.102 The distinctive characteristics of GR and GO, such as their great chemical stability, wide specific surface area, and superior electrical conductivity, make them ideal for electrochemical sensing applications. Several electrochemical sensors using GR and/or GO as key components have been created. For example, Zhou et al.103 devised a sensor by modifying a GCE with a GR-GO nanocomposite to detect HQ and CC in water simultaneously. The utilization of GR-GO in the electrode's modification resulted in a notable enhancement in the peak currents of HQ and CC due to improved electrochemical process kinetics. Within the concentration range of 0.5–300 μM, the oxidation peak currents for both HQ and CC exhibited linearity, and their respective detection limits were determined to be 0.16 μM (S/N = 3) for HQ and 0.2 μM (S/N = 3) for CC. Furthermore, the GR-GO-modified electrode demonstrated exceptional stability, repeatability, and resistance to interference. Additionally, the GR-GO-modified electrode showed outstanding stability, repeatability, and interference-resistance. Palanisamy et al.104 developed an electrochemical sensor for the detection of CC based on a modification of a GCE with a composite consisting of graphene oxide-polydopamine (GO@PDA) modified with AuNPs. This sensor achieved a LOD of 0.015 μM, and the current response at the GO@PDA-AuNP composite-modified electrode displayed linearity across the concentration range of 0.3–67.55 μM. It was effectively applied for the determination of CC in tap water samples, exhibiting a sensitivity of 4.66 ± 0.15 μA μM−1 cm−2. Additionally, Chen et al.105 created an electrochemical sensor based on a GCE coated with a graphene/polymer layer for the measurement of phenols in water. With a correlation coefficient of 0.9963, the decrease in peak current exhibited a direct correlation with the quantity of phenols. The LOD was 2.00 × 10−4 mol L−1 (S/N = 3). For the quick, easy, and quantitative detection of phenols in actual samples, the sensor showed promise. In a similar vein, Zheng et al.106 introduced an electrochemical sensor employing a metal–organic framework (CoNi-MOF) combined with a modified GCE (CoNi-MOF/GO/GCE) for the simultaneous detection of CC and HQ (see Fig. 4a). The observed peak-to-peak separation between CC (0.223 V) and HQ (0.120 V), as depicted in Fig. 4b, was 103 mV, highlighting the sensor's capability to simultaneously and selectively identify these two nanoplastic compounds. Under optimal conditions, including a wide linear range (0.1–100 μM) (Fig. 4c), low LOD values (0.04 μM for HQ and 0.03 μM for CC), and high resistance to interference, the CoNi-MOF/GO/GCE sensor exhibited excellent analytical performance for the simultaneous determination of CC and HQ. Moreover, the sensor was successfully applied to the detection of CC and HQ in river water samples. Additionally, to enhance the analytical capabilities for BPA detection, Zha et al.107 proposed an electrochemical sensor for BPA quantification based on AuNPs supported on zinc oxide/reduced graphene oxide (Au/ZnO/rGO) nanosheets, with the inclusion of the surfactant cetyltrimethylammonium bromide (CTAB). The electrochemical transfer characteristics of the CTAB/Au/ZnO/rGO sensor are discussed and the LOD was assessed using DPV for various BPA concentrations. The results revealed that the proposed sensor featured two linear ranges with a LOD of 4.95 nmol L−1, spanning from 10 to 1340 nmol L−1 and from 1340 to 10
000 nmol L−1. Furthermore, the CTAB/Au/ZnO/rGO sensor demonstrated excellent re-producibility, selectivity, and potential practical applicability for BPA detection in real samples.
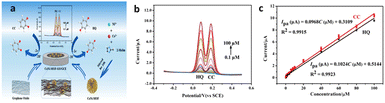 |
| Fig. 4 Graphene-based nanoplastic electrochemical sensors: (a) the production of the CoNi-MOF/GO/GCE sensor and its use in the electrochemical determination of CC and HQ. The oxidation process at the electrode interface is indicated by arrows. (b) DPV curves of CoNi-MOF/GO/GCE reveal simultaneous determination ranges of 0.1–100 μM for both CC and HQ, and (c) corresponding calibration plots illustrate the oxidation peak currents as functions of CC and HQ concentrations, respectively. Reproduced under the terms of the CC-BY license from ref. 106 [Sensors], copyright 2023, The Authors, published by MDPI. | |
3.2 Noble metal based nanomaterials
Noble metal nanoparticles, such as AuNPs, AgNPs, and copper nanoparticles (CuNPs), are commonly employed in electrochemical sensors for NPL residue detection due to their unique properties, high surface area, and excellent catalytic activity.108 There have been several reports of electrochemical sensors based on noble metal nanoparticles that can be used to detect various NPL residues.52,53,109,110 Recently, these nanoparticles are often used individually or in combination with other composites, such as MWCNTs or CBs, for detecting various NPL residues.
With regard to the use of AuNPs, Jebril et al.23 developed an environmentally friendly Sonogel carbon (SCGC) electrode modified with a carbon black/gold sononanoparticle nanocomposite (CB/AuSNPs) for the electrochemical simultaneous detection of three NPL residues, namely HQ, CC, and resorcinol (RC) using the DPV method. When CB and AuSNPs are combined, a nanocomposite with a high surface area and good electro-conductivity is formed, which inhibits the transport of electrons and lowers the response current of SNGCEs. Therefore, based on the response current differential before and after the reaction, the concentration of NPL residues may be determined. These findings demonstrated that the suggested sensor has good sensitivity for HQ, CC, and RC detection, which was examined in both solo and triple-component solutions, leading to low LODs and a broad linear range. The simultaneous detection of HQ, CC, and RC had LODs of 1.7, 5.1, and 4.5 μM (S/N = 3), respectively. The created sensor also demonstrated excellent reproducibility, repeatability, and selectivity. Additionally, the amperometric sensor was effectively used to find these NPL residues in samples of tap, dam, and swamp water. This method is used in additional research by Jebril et al.52 to identify NPL residues like BPA, a frequent component in plastic items that is known to seep into water and may have negative health effects. Benefiting from the advantageous interaction between green gold AuSNPs and CB as cost-effective nano-materials, a sensor was developed based on a SNGCE modified with the CB/AuSNP nano-composite (CB/AuSNPs/SNGCE). This sensor exhibited heightened electroactivity, characterized by superior sensitivity and a relatively low LOD of 60 nM (n = 3) within a BPA concentration range of 0.5–15 μM. The newly developed sensor also demonstrated exceptional selectivity against various interfering substances. Furthermore, this analytical sensor was effectively employed for BPA detection in samples of both tap and mineral water. Incorporating AgNPs, Ben Jaballah et al.54 designed a nanosensor based on a GCE modified with functionalized multi-walled carbon nanotubes (f-MWCNT) and AgNPs for the detection of BPA in drinking water. This sensor boasted a LOD of 40 nM and exhibited a broad linear response range for BPA (70–6000 nM), successfully detecting BPA in drinking water using the AgNPs/f-MWCNT/GCE sensor. Another notable example is the electrochemical sensor developed by Goulart et al.75 for the simultaneous measurement of four NPL residues, including HQ, CC, BPA, and phenol. This sensor utilized modified glassy carbon electrodes that were further enhanced with silver nanoparticles and carbon nanotubes. The study's findings showcased a linear rise in oxidation current with increasing concentrations of HQ, CC, BPA, and phenol. The LODs for all four species were 0.16 μM, 0.20 μM, 2.40 μM and 3.00 μM respectively, and the sensor exhibited remarkable stability and repeatability. This sensor was effectively employed for the simultaneous determination of HQ, CC, BPA, and phenol in samples of contaminated tap water. Additionally, Eftekhari et al.111 introduced an electrochemical sensor for BPA detection based on a GCE modified with an organosilicaionic liquid periodic mesoporous material and functionalised with copper nanoparticles. This sensor demonstrated a LOD of 1.5 nM and a linear detection range for BPA spanning from 5.0 nM to 2.0 μM and 4.0 to 500 μM.
Furthermore, the detection of NPL residues has been explored using hybrid nanomaterials or composites, incorporating various noble metals such as bimetallic nanoparticles (e.g., Au–Ag and Pt–Ag), metal oxide/noble metal hybrids, or metal nanoparticles. These hybrid nanomaterials can enhance sensing performance, sensitivity, and selectivity of electrochemical sensors for NPL residue detection by leveraging the unique properties of different metals.
3.3 Metal oxide based nanomaterials
Metal oxide nanoparticles, such as zinc oxide (ZnO), zinc cobaltite (ZnCo2O4), and iron oxide (Fe3O4), offer a substantial surface area and distinctive electrochemical properties that contribute to the detection of NPL residues. Below are examples of electrochemical sensors employing metal oxide-based nanomaterials for NPL residue detection. In their research, Amiri et al.112 introduced an innovative electrochemical sensor tailored for detecting BPA in water, utilizing modified screen-printed electrodes (SPEs) composed of zinc cobaltite and a zinc cobaltite nanocomposite (ZnO/ZnCo2O4). This sensor demonstrated a commendable sensitivity and selectivity for BPA, with a LOD of approximately 0.01 μM. The correlation coefficient reached 0.9985, and the linear detection range spanned from 0.06 to 200.0 μM. Additionally, the sensor exhibited robust stability and reproducibility, making it a promising tool for BPA detection in water samples. Similarly, Mohammadzadeh Jahani et al.113 proposed the use of a SPE modified with Fe3O4 nanoparticles as an electrochemical sensor for BPA detection in water. The Fe3O4-modified electrode exhibited an enhanced oxidation peak current for BPA compared to the unmodified electrode. A linear relationship between BPA oxidation peak current and concentration within the range of 0.03 to 700.0 μM was observed using DPV. The sensor achieved a LOD of less than 0.01 μM. These studies underscore the potential of metal oxide nanoparticles, specifically ZnO, ZnCo2O4, and Fe3O4, in enhancing the electrochemical detection of NPL residues, particularly BPA in water. The developed sensors exhibited remarkable sensitivity, selectivity, and low LODs, making them effective tools for NPL residue monitoring and analysis.
In another study, Movahed et al.114 designed a sensing layer with ZnO@MnO2-rGO nanocomposite modification on a GCE for the simultaneous detection of HQ, Mono-tert-butyl hydroquinone (MTBHQ), and CC (Fig. 5a). The DPV results displayed the significant electrocatalytic activities of the ZnO@MnO2-rGO/GCE toward the electro-oxidation of MTBHQ, HQ, and CC within linear ranges of 0.008 to 10 μM and 10 to 350 μM for MTBHQ, 0.008 to 10 μM and 10 to 320 μM for HQ, and 0.008 to 8 μM and 8 to 330 μM for CC (Fig. 5b), with LODs of 0.0011, 0.0012, and 0.001 μM, respectively (Fig. 5c). Furthermore, this sensor has demonstrated successful simultaneous detection of HQ, MTBHQ, and CC in various real samples. Shen et al.115 developed a ratiometric electrochemical sensor for BPA detection using an Ag@Fe3O4-rGO composite-modified GCE (Fig. 5d). The two-dimensional structure and chemical activity of rGO, combined with Ag@Fe3O4 core–shell nanoparticles, provided an effective platform for BPA detection (Fig. 5e). The proposed ratiometric electrochemical BPA sensor exhibited excellent selectivity, repeatability, and stability, with a linear detection range of 0.1–10.0 μM (R2 = 0.997) and a LOD of 0.028 μM (S/N = 3) (Fig. 5f). This ratiometric sensor has also been successfully applied to detect BPA in real water samples.
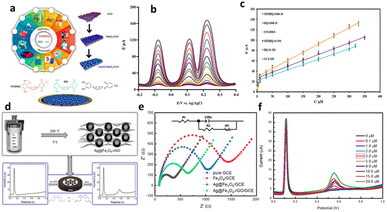 |
| Fig. 5 Nanoplastic electrochemical sensors based on metal oxides: (a) application, drawbacks, and proposed electro-oxidation of MTBHQ, HQ, and CC, (b) DPVs of simultaneous detection of MTBHQ, HQ, and CC at ZnO@MnO2-rGO/GCE, and (c) calibration plots of simultaneous detection. Reprinted from ref. 114, copyright (2023), with permission from Elsevier. (d) Schematic of the BPA electrochemical sensor preparation process, (e) EIS of various electrodes in 5.0 mM [Fe(CN)6]3−/4− containing 0.1 M KCl, in the frequency range of 105 to 0.1 Hz, with an amplitude of 5 mV at open circuit voltage, and (f) DPVs of Ag@Fe3O4-rGO/GCE in various BPA concentrations, ranging from 0.1 μM to 20.0 μM. Reprinted from ref. 115, copyright (2023), with permission from Elsevier. | |
3.4 Polymer film based nanomaterials
The distinctive physicochemical attributes of polymers have garnered significant attention in advancing the field of electrochemical sensors and biosensors. These properties play a pivotal role in elevating sensor performance through augmentation of electrode conductivity and provision of an expanded specific surface area, thereby facilitating enhanced interactions. Polymers have demonstrated their versatility as prime materials for fabricating a wide array of sensing devices, encompassing temperature, gas, ion-selective, pH, and biological sensors.116 Notably, they enable the precise detection of NPL residues through tailored selection. Polymers can be deliberately designed or chosen based on their chemical composition and functional groups, enabling them to exhibit robust affinity towards NPL residues. These polymers consist of recurrent monomer units, wherein the arrangement of positive or negative charges within these monomers can be strategically manipulated to amplify electrostatic interactions. This repetitive charge distribution markedly heightens the attractive forces between the polymer matrix and NPL residues. It's important to note that both natural and artificial polymers have potential in the field of detecting NPL residues.
For instance, Park et al.117 presented the innovative use of poly-L-lysine (PLL) in conjunction with a terahertz (THz) metamaterial sensor for the detection of microplastic (MP) particles. This approach exploits the unique properties of PLL and the capabilities of THz metamaterials to achieve sensitive and specific detection. Metamaterials are artificially engineered materials that are designed to have properties not found in naturally occurring substances. In this case, a THz metamaterial sensor is specifically designed to interact with terahertz frequencies. This involved depositing layers of Cr (chromium) and Au (gold) in a specific pattern on a highly resistive Si (silicon) substrate. The patterned deposition is critical to achieving the desired interactions with the target particles. To avoid non-specific interactions, the remaining portions of the metamaterial were covered with an anti-adhesive hexamethyldisilazane layer. In a microfluidic chip, the functionalized THz metamaterial was housed. This chip allowed controlled flow of the aqueous sample (containing microplastic particles) over the surface of the metamaterial. As the PLL-functionalized metamaterial trapped negatively charged microplastic particles from the flowing sample, the resonant frequency of the metamaterial changed. This change in resonant frequency was detected and analysed using spectroscopy. Overall, this approach demonstrates how PLL-functionalized THz metamaterials can be used to selectively detect microplastic particles based on their negative charge. The change in resonant frequency upon particle capture provides a measurable signal that can be correlated to the presence and concentration of MPLs. This innovative combination of polymer chemistry, metamaterial design, and microfluidics contributes to the development of advanced sensor technologies for environmental monitoring and microplastic detection.
Besides, Tan and colleagues introduced an electrochemical sensor that relied on a composite material of molecularly imprinted polypyrrole and graphene quantum dots (MIPPy/GQD) for the purpose of detecting BPA within water samples.118 They developed the MIPPy/GQD composite layer by means of electro-polymerizing pyrrole on a GCE with BPA serving as a template. Their findings indicated the successful and selective identification of BPA within aqueous solutions using the MIPPy/GQD composite. This recognition led to a notable reduction in the peak currents of K3[Fe(CN)6] at the electrode modified with MIPPy/GQD in both DPV and CV. Furthermore, the research revealed a linear relationship between BPA concentrations within the range of 0.1 to 50 μM. The LOD in DPV was determined to be 0.04 μM. Finally, they applied their sensor to quantify BPA levels in samples of both tap and seawater, achieving a recovery rate ranging from 94.5% to 93.7%. Consequently, this method offers a robust tool for the swift and highly sensitive detection of BPA in environmental samples. While the concept is promising, there are challenges to overcome, including the development of polymers with high selectivity and sensitivity for different types of NPL residues. In addition, the potential impact of the polymers themselves on the environment or the sample matrix must be carefully evaluated.
4 Biosensors for electrochemical NPL residues detection
Biosensors have emerged as promising tools for the detection of NPL residues in aquatic ecosystems. Their high sensitivity, specificity, real-time monitoring capabilities, and compatibility with complex environmental matrices make them valuable tools for understanding the presence and distribution of NPL residues. These devices combine biological recognition elements with transducers to convert binding events into measurable signals, allowing specific and sensitive detection of NPL residues (Fig. 6).119 These recognition elements can be enzymes, antibodies or aptamers designed to have high affinity and specificity for NPL residues. These elements are selected based on their ability to selectively bind to the target NPL residues, thereby increasing the sensitivity and selectivity of the biosensor. In this section, we discuss the types of biosensors used in the electrochemical detection of NPL residues and highlight their advantages and limitations.
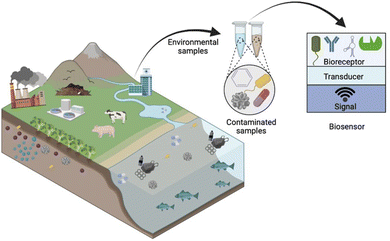 |
| Fig. 6 Schematic of the typical components of a biosensor for application in environmental samples and main sources of emerging pollutants. “Reprinted (adapted) with permission from {ref. 119}. Copyright {2023} American Chemical Society”. | |
4.1 Enzyme based biosensors
Enzymatic sensors have gained widespread recognition for their exceptional precision and sensitivity, making them invaluable for detecting a broad spectrum of analytes.58 In the realm of environmental applications, these biosensors have progressively supplanted traditional analytical techniques due to their scalability, affordability in terms of instrumentation, and user-friendliness, which includes minimal sample preparation, and potential for multiple applications. These attributes become especially pronounced when addressing the detection of contaminants such as NPL residues in water. Enzymatic sensors have come a long way since their initial development by Clark and Lyons in 1962.119,120 The biosensor they designed used glucose oxidase as the enzyme and an amperometric electrode for glucose monitoring. Today, enzymes such as glucose oxidase remain one of the most common biorecognition elements used in biosensors. Enzymatic sensors are potent analytical tools that use digital signal processors, transducers, and biorecognition components (enzymes) to quickly, precisely, and constantly identify specified compounds. The enzyme (1), which serves as the biorecognition component, is the primary component. It selectively interacts with the target analyte, resulting in a specific reaction; (2) the transducer, which plays a crucial role in converting the physical, biological, or chemical changes that arise from the interaction between the enzyme and the target analyte into signals that can be detected and measured. Among the commonly used transducers are electrodes, specifically designed for amperometric or potentiometric measurements. These electrodes are instrumental in capturing and quantifying the desired signals, making them essential components of biosensors designed for a wide range of applications; (3) the digital signal processor, which receives and processes the signals generated by the transducer. It digitizes the information, performs signal conditioning, noise reduction, and amplification, and prepares the signal for interpretation and analysis.121,122
The matrix, which can take the form of polyelectrolytes, conductive polymers, or pyroelectric polymers, plays an important part in the development of enzymatic sensors by ensuring stability and durability over time and enabling optimal immobilization of the enzyme.123 One of the critical phases in developing enzymatic sensors involves the enzyme's assembly or immobilization, and there exists a variety of techniques for achieving this, including adsorption, encapsulation, cross-linking, covalent bonding, and entrapment. The technique is determined by the particular demands of the biosensor. Enzyme immobilization on the electrode's or transducer's surface enhances stability, repeatability, and reusability. It also provides an accessible active site that mimics the natural state of the enzyme and enhances the catalytic activity of the enzymatic sensor.124 Electrochemical transducers are commonly used elements in EBB development due to their high sensitivity and small sample volume requirements. Various types of electro-chemical sensors are available, depending on the fabrication method. They include carbon paste-based electrodes, screen-printed electrodes on rigid or flexible substrates, and microfabricated electrodes. Microfabricated electrodes offer several advantages, such as easy miniaturization, improved time stability, and integration into portable devices.125 The selection of enzymes in enzymatic sensors is determined by the specific application and the target analyte. Enzymes commonly used in enzymatic sensors encompass hydrolases such as lipases, phosphatases, glycosidases, peptidases, and nucleosidases; oxidoreductases (including tyrosinase and nitrate reductase); oxidases (like glucose oxidase, monoamine oxidases, and laccases); peroxidases (e.g., horseradish peroxidase); aminooxidases. These enzymes, either through activation or activity inhibition, enable the detection of various hazards.126,127 This specifically refers to NPL residues, which are the most dangerous pollutants introduced into the environment. They have grown to be a significant issue as a result of human activities, when they are abundant in water.128,129
Several enzymatic sensors have been developed for the detection and quantification of NPL residues. One instance is the creation of a nanocapsule-based biosensor by Wu et al.130 that uses tyrosinase (nTyr) in water for the ultrasensitive and quick detection of BPA. In this context, we chose tyrosinase as the model enzyme, known for its catalytic activity towards BPA. To enhance enzyme stability and efficiency, we developed tyrosinase nanocapsules (nTyr), encapsulating individual tyrosinase molecules within a thin network polymer shell. The encapsulation was achieved through an aqueous solution and the in situ polymerization technique. Subsequently, we constructed an electrochemical (bio)sensor for BPA detection utilizing these nTyr nanocapsules. This biosensor exhibited exceptional performance, featuring a low LOD of 12 nmol L−1 and an extensive linear range for BPA concentration from 5 × 10−8 to 2 × 10−6 mol L−1. Additionally, as shown in Fig. 7a, Liu et al.131 introduced a novel 3D aloe-like Au–ZnO nanocomposite on an ITO substrate for CC detection in water. The combination of highly conductive AuNPs and the large specific surface area of aloe-like ZnO resulted in excellent electrocatalysis and rapid electron transfer during enzymatic processes. This biosensor offered selective detection of CC, even in the presence of various dihydroxybenzenes and phenols, with an impressive sensitivity of 131 μA mM−1 (Fig. 7b) across a broad linear range from 75 nM to 1100 μM (Fig. 7c), and a notably low LOD of approximately 25 nM. Moreover, the biosensor demonstrated reliable performance in assessing real lake samples and exhibited satisfactory anti-interference capabilities.131
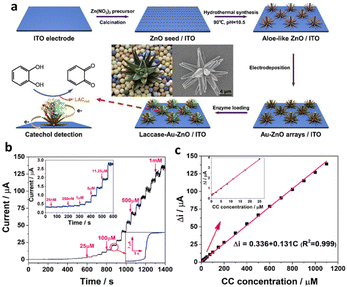 |
| Fig. 7 (a) Schematic preparation of aloe-like Au–ZnO arrays on an ITO electrode. (b) Amperometric responses amplified upon repeated CC addition occurring every 50 s, inset: the curve magnified from 0 to 600 s, (c) linear correlation between peak current and CC concentration; the curve is magnified at low CC concentrations in the inset. Reprinted from ref. 131, copyright (2020), with permission from Elsevier. | |
According to Shan et al.,132 they developed an electrochemical (bio)sensor for HQ detection based on oriented Prussian blue/polyaniline (PB/PANI) nanoarrays, as depicted in Fig. 8a. Fig. 8b illustrates the unique nanostructure of PB/PANI, which combines excellent electrocatalysis and conductivity from PB and PANI, along with a remarkably high surface area for abundant active sites required for laccase immobilisation, resulting in strong synergistic effects. The biosensor exhibited reliability in evaluating materials from real lakes, boasting a high sensitivity of 931.39 μA mM−1 cm−2 and an LOD of 250 nM (0.027 ppm) after laccase immobilization (Fig. 8c–e).
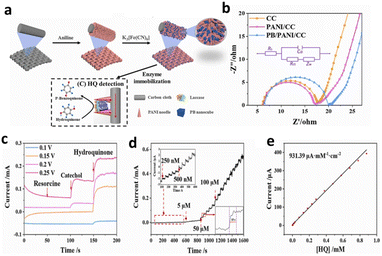 |
| Fig. 8 (a) Synthesis process of the HQ biosensor using PB/PANI, (b) EIS diagram, which involves the three mentioned electrodes, spanning a frequency range from 0.1 Hz to 1 MHz, (c) amperometric responses at different operating potentials, (d) the signals' reactions, and (e) linear calibration of the Lac-PB/PANI/CC biosensor at 0.15 V in PBS, with insets displaying the detection duration and a magnification of the curve from 100 to 600 s. Reprinted from ref. 132, copyright (2023), with permission from Elsevier. | |
Additionally, Zhao et al.133 proposed an economical electrochemical (bio)sensor that overcomes the application challenges of BLac and MXenes/PEI-MWCNT nanohybrids on a GCE for the detection of common NPL residues like CC and HQ. Their approach involved co-immobilizing cell surface displayed bacterial laccase (CSDBLac), as depicted in Fig. 9a. The biosensor exhibited remarkable sensitivity with a low LOD (0.15 μM for CC and 0.09 μM for HQ), excellent repeatability, and stability attributed to the synergistic effect of nanohybrids and CSDBLac (Fig. 9b and 9c). It was successfully employed for CC and HQ detection in real water samples. The capacity to detect NPL residues in complicated samples and high specificity and sensitivity are only a few benefits of enzyme-based biosensors. However, they also face challenges such as enzyme stability, reproducibility, and potential interference from other components in the sample matrix.
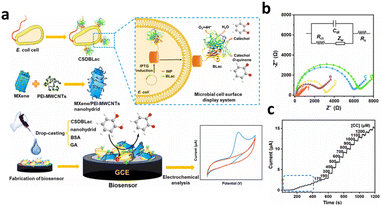 |
| Fig. 9 (a) The CSDBLac and biosensor application and construction schematics. (b) GCE's impedance spectrum (b(a)), CSDBLac/GCE (b(b)), GA-CSDBLac/GCE (b(c)), GA-BSA-CSDBLac/GCE (b(d)), GA-BSA-MXene-CSDBLac/GCE (b(e)) and GA-BSA-MXene-PEI-MWCNTs-CSDBLac/GCE (b(f)). (c) CC's magnification curves. Reprinted from ref. 133, copyright (2023), with permission from Elsevier. | |
Furthermore, Liu et al.134 introduced a novel variety of carbon dots known as F,N-CDs, which are co-doped with nitrogen and fluorine through the utilization of p-phenylenediamine (p-PD) and 5-fluorouracil (5-Fu). Leveraging F-, N-CDs in conjunction with laccase (Lac), they developed an electrochemical sensor tailored for the detection of CC. In contrast to traditional catechol sensing methods, the sensor Lac-F, N-CDs/GCE as synthesised has a significantly stronger sensitivity of 219.17 μA cm−2 mM−1 and a relatively lower LOD of roughly 0.014 μM. In practice, the detection of CC in both tap water and lake water has been effectively accomplished using the developed Lac-F, N-CDs/GCE sensor. This study introduces an innovative approach to stabilize laccase, enabling the creation of efficient electrochemical sensors for the measurement of catechol and similar analytes using CDs and various carbon nanostructures.
Overcoming these challenges through appropriate enzyme selection, immobilization techniques, and optimization of assay conditions is critical to the successful implementation of enzyme-based biosensors for NPL residue detection.
4.2 Antibody based biosensors
Antibody-based electrochemical biosensors stand as exemplars of exceptional selectivity and specificity, making them indispensable across a wide spectrum of fields, including the food industry, biomedicine, and environmental monitoring. What sets them apart is their unique ability to deliver real-time, on-site detection, a feature that greatly amplifies their utility in diverse applications. Within the domain of antibody-based electrochemical biosensors, various antibody types play pivotal roles. Polyclonal antibodies (pAbs), stemming from plasma cells in serum, possess a remarkable versatility. They can recognize multiple epitopes on the target analyte's surface, expanding their specificity to encompass various forms of the analyte. On the other hand, monoclonal antibodies (mAbs), originating from the fusion of B cells with immortal myeloma cells, exhibit exceptional precision. They zero in on a single epitope on the target analyte's surface, making them invaluable in diagnostics and analytical applications where consistent specificity is paramount.
An increasingly relevant alternative is the utilization of antibody fragments or recombinant antibodies. These fragments, including single-chain variable fragments (scFv) or antigen-binding domain fragments, can be crafted through proteolytic digestion or genetic engineering. They offer numerous advantages over full-length antibodies, such as heightened stability and solubility. Furthermore, producing antibody fragments or recombinant antibodies allows for the customization of specific characteristics, like enhanced affinity and amplified specificity for the target analyte.
The detection of interactions between the analyte (antigen) and the antibody within antibody-based electrochemical biosensors hinges on the electrochemical transducer, an essential component. This critical element enables the precise determination of both the analyte's presence and its concentration. In essence, antibody-based electrochemical bio-sensors, fortified by these diverse antibodies and advanced transduction methods, form an indispensable toolkit for accurate, sensitive, and quantitative analysis across a wide array of applications.135,136
Numerous antibody-based biosensors have been developed for detecting and quantifying NPL residues. In a notable example, Marchesini et al.137 developed a biosensor for the detection of BPA. To enable this biosensor's detection capabilities, they raised antibodies, including both pAbs and mAbs antibodies, against a structural analogue of BPA known as 4,4-bis-(4-hydroxyphenyl) valeric acid (BVA). The researchers conducted a comprehensive evaluation of the kinetics of the MAb–BPA interaction, identifying MAb 12 as the antibody with the highest affinity. This antibody was subsequently directly immobilized onto the sensor chip surface to create a direct assay. In their experiments, MAb 12 exhibited the highest sensitivity, achieving a remarkable LOD of 0.4 μg L−1 in the direct assay. However, the researchers found that the inhibition assay demonstrated superior robustness. In this assay, the PAbs exhibited the highest sensitivity, with an LOD ranging from 0.5 to 1 μg L−1. Importantly, these antibodies displayed specificity for both BVA and BPA, with minimal cross-reactivity observed towards structurally related compounds or other endocrine disruptors. In the inhibition assay, which had a rapid run time of 6 minutes, water samples spiked with BPA at different concentrations (ranging from 0.5 to 50 μg L−1) yielded recoveries between 68% and 121%. Notably, the sensitivity of the inhibition assay could be significantly enhanced (achieving a LOD of 0.03 μg L−1 with the MAb 12-based assay) through the incorporation of solid-phase extraction (SPE). This improvement underscores the biosensor's potential for highly sensitive BPA detection, particularly when coupled with SPE techniques.
In conclusion, antibody-based biosensors serve as invaluable tools with wide-ranging applications in fields such as the food industry, biomedicine, and environmental monitoring, thanks to their unmatched selectivity and specificity. The ability to provide real-time and in situ detection makes them a preferred choice for various applications. Within this realm, polyclonal and monoclonal antibodies, as well as innovative antibody fragments, offer versatile solutions for precise analyte detection. These biosensors, coupled with diverse transducers, facilitate the detection and quantification of target analytes.
4.3 Aptamer based biosensors
Aptamers, short single-stranded oligonucleotides composed of either DNA or RNA, exhibit exceptional affinity and selectivity toward specific target molecules. These aptamers are crafted through a systematic process known as the Systematic Evolution of Ligands by Exponential Enrichment (SELEX). In the initial stage of SELEX, a vast library of random oligonucleotide sequences, sometimes comprising as many as 1015 distinct sequences, is generated.138 These sequences contain a central region with randomly positioned bases, flanked by two conserved regions designed for primer binding during PCR amplification at the 5′ and 3′ ends. Under regulated binding circumstances, the target molecule is exposed to the random oligonucleotide library. Sequences that exhibit target binding are next amplified by PCR and subjected to a thorough analysis.139 Aptamers provide a number of benefits over conventional antibodies, including high selectivity, quick synthesis, cost effectiveness, and environmental tolerance. Due to their various structural topologies, including loops, multi-branched loops, and G-quadruplexes, aptamers have consequently emerged as excellent candidates for environmental sample monitoring.140,141 By taking advantage of these benefits, aptamers have the potential to completely change environmental monitoring by delivering more effective, affordable, and trustworthy instruments for identifying environmental pollutants and toxins. Aptamers, characterized by their exceptional selectivity, diverse structural motifs, and resilience under harsh conditions, present themselves as appealing alternatives for a wide spectrum of environmental sensing applications. In this context, a variety of aptasensors have been devised for NPL residue detection. For example, in a study by Cui et al.142 a label-free, single-step approach was introduced for BPA detection in aqueous samples. This method employed an aptamer-based molecular probe immobilized on a commercially available array of interdigitated aluminum microelectrodes. The quantification of BPA levels relied on monitoring changes in the interfacial capacitance rate due to specific binding events between the analyte and the immobilized aptamer. Impressively, this sensor achieved a detection limit as low as 10 fM and demonstrated a rapid response time of just 20 seconds. Such an economical, highly sensitive, and rapid technique holds significant promise for on-site BPA detection in water samples.
In contrast, Xue et al.143 developed an electrochemical aptasensor designed for swift and ultra-high sensitivity detection of BPA, as well as for screening the presence of BPA in drinking water. This aptasensor employed a specific aptamer targeting BPA and its complementary DNA probe, which were immobilized on a gold electrode through self-assembly and hybridization, respectively. The detection of BPA relied on competitive binding interactions between BPA and the immobilized aptamer on the electrode surface. This electrochemical aptasensor exhibited the capability to detect BPA in drinking water with an exceptional detection limit of 0.284 pg mL−1 in less than 30 minutes. Such remarkable sensitivity establishes this method as a potent tool for on-site monitoring of water quality and food safety.
It is noteworthy that aptamer-based biosensors offer distinct advantages over their antibody-based counterparts. Aptamers can be specifically engineered to target a wide array of molecules, even those challenging to address with antibodies. Furthermore, aptamers can be readily customized and modified to enhance their stability and selectivity.
5 Challenges, future prospects and avenues
The detection and monitoring of NPL residues in aquatic ecosystems are crucial for addressing global concerns about NPL pollution. Electrochemical chemo(bio)sensors offer advantages such as heightened specificity, sensitivity, rapid response times, and the ability to discern trace contaminants. However, several challenges must be addressed for successful development and implementation. A primary challenge is the judicious selection of recognition elements, such as enzymes, antibodies, or aptamers, to ensure biosensor specificity. Developing elements capable of distinguishing NPL residues from other contaminants is crucial. Aquatic environments, rich in interfering substances, pose difficulties, demanding robust and selective electrochemical (bio)sensors for real-world conditions. Accurately quantifying NPL residue concentrations in water samples presents another challenge due to limited calibration standards. Portable, miniaturized electrochemical chemo(bio)sensors are needed for effective on-site, real-time monitoring. Understanding ecological and human health impacts requires integrating NPL residue detection with impact assessments. The advancement of electrochemical chemo(bio)sensors for NPL residue detection offers substantial potential. The resolution of NPL pollution requires collaboration among researchers, policymakers, industries, and environmental agencies. Future prospects involve multi-recognition element biosensors and machine learning for data analysis. Our review emphasizes the need for cohesive efforts across scientific communities, regulatory bodies, and industrial sectors, fostering collaboration and inspiring confidence in NPL pollution's tangible impact and effective resolution. To enhance quantification accuracy, standardization and reference materials are crucial. Integrating electrochemical chemo(bio)sensors with remote sensing and IoT enables continuous, real-time monitoring. Global collaboration is encouraged to share data and insights, fostering a better understanding of the global distribution and impact of NPL residues. Developing regulatory frameworks and guidelines is essential for integrating electrochemical chemo(bio)sensors into environmental monitoring programs, contributing to a more sustainable and healthier planet.
6 Conclusions
This comprehensive review delves deep into the realm of NPL residue detection, recognizing the increasing environmental concerns and the pressing need for reliable monitoring techniques. Within this context, nanomaterials play a pivotal role in advancing sensing and biosensing capabilities. Nanomaterials, ranging from graphene and carbon nano-tubes to metal nanoparticles and hybrid nanocomposites, serve as the foundational building blocks for the development of advanced sensors dedicated to NPL residue detection. Their unique properties, such as high surface area, excellent conductivity, and tailored electrochemical characteristics, endow these chemo(bio)sensors with the sensitivity and specificity needed to detect and monitor NPL residues in complex environmental settings effectively. Throughout the review, nanomaterials play a central role in enhancing sensor/biosensor performance, enabling real-time monitoring, and expanding the horizons of environmental assessment. The review also focuses on electrochemical biosensors covering enzyme, antibody and aptamer-based approaches, elucidating their mechanisms, advantages, and limitations. It provides valuable insights into the practicality and performance of diverse sensors and biosensors for NPL residue detection, underscoring their respective strengths and weaknesses. In addition to the rich insights provided in this comprehensive review, it is imperative to highlight the exciting prospects presented by nanopore technology. As a pioneering method, nanopore technology has the potential to revolutionise NPL residue detection through its unique capacity for single-molecule analysis and real-time monitoring. The integration of nanopore technology with established nanomaterial-based sensors promises to further enhance sensitivity and specificity, shedding light on NPL residue behaviour and environmental impact.
Looking forward, the collaboration of traditional, nanomaterial-based, and cutting-edge electrochemical techniques, including nanopore technology, is poised to underpin robust environmental monitoring. This collective approach empowers scientists, decision-makers, and stakeholders to protect global water resources and public health in the face of escalating environmental concerns. The dynamic interplay of these methodologies, informed by the insights presented in this review, sets the stage for a promising future in addressing the complex challenges of NPL residues in our environment and for further sustainable developments.
Conflicts of interest
There are no conflicts to declare.
Abbreviations
NPL | Nanoplastic |
CC | Catechol |
HQ | Hydroquinone |
MTBHQ | Mono-tert-butyl hydroquinone |
BPA | Bisphenol A |
BVA | 4,4-Bis-(4-hydroxyphenyl) valeric acid |
RS/RC | Resorcinol |
o-AP |
ortho-Aminophenol or 2-aminophenol |
o-CP |
ortho-Chlorophenol or 2-chlorophenol |
o-NP |
ortho-Nitrophenol or 2-nitrophenol |
PS | Polystyrene |
PA | Polyamide |
PMA | Polymethyl acrylate |
LDPE | Low-density polyethylene |
GCE | Glassy carbon electrode |
SPE | Screen-printed electrode |
CV | Cyclic voltammetry |
LSV | Linear sweep voltammetry |
DPV | Differential pulse voltammetry |
SWV | Square-wave voltammetry |
EIS | Electrochemical impedance spectroscopy |
MIPs | Molecularly imprinted polymers |
FLISA | Fluorescence-linked immunoassays |
SELEX | Systematic evolution of ligands by exponential enrichment |
CD | Cyclodextrin |
PLL | Poly-L-lysine |
nTyr | Tyrosinase nanocapsules |
PB | Prussian blue |
PANI | Polyaniline |
GR | Graphene |
GO | Graphene oxide |
MWCNTs | Multi-walled carbon nanotubes |
CDs | Carbon dots |
SCGC | Sonogel carbon |
CB | Carbon black |
AuSNPs | Gold sono-nanoparticles |
AuNPs | Gold nanoparticles |
MOFs | Metal–organic frameworks |
LOD | Limit of detection |
LDR | Low dynamic range |
RSD | Relative standard deviation |
THz | Terahertz |
Acknowledgements
Dr Baljit Singh would like to thank and acknowledge the Enterprise Ireland Technology Gateways Programme, which is co-funded by the Government of Ireland and the European Union under ERDF (European Regional Development Fund) Regional Programmes. MiCRA Biodiagnostics Technology Gateway at Technological University Dublin (TU Dublin) is funded and supported under the Enterprise Ireland Technology Gateways Programme.
Notes and references
- S. Galafassi, L. Nizzetto and P. Volta, Plastic Sources: A Survey across Scientific and Grey Literature for Their Inventory and Relative Contribution to Microplastics Pollution in Natural Environments, with an Emphasis on Surface Water, Sci. Total Environ., 2019, 693, 133499, DOI:10.1016/j.scitotenv.2019.07.305.
- L. Peng, D. Fu, H. Qi, C. Q. Lan, H. Yu and C. Ge, Micro- and Nano-Plastics in Marine Environment: Source, Distribution and Threats — A Review, Sci. Total Environ., 2020, 698, 134254, DOI:10.1016/j.scitotenv.2019.134254.
- K. Yin, Y. Wang, H. Zhao, D. Wang, M. Guo, M. Mu, Y. Liu, X. Nie, B. Li and J. Li,
et al., A Comparative Review of Microplastics and Nanoplastics: Toxicity Hazards on Digestive, Reproductive and Nervous System, Sci. Total Environ., 2021, 774, 145758, DOI:10.1016/j.scitotenv.2021.145758.
- A. L. Andrady, Microplastics in the Marine Environment, Mar. Pollut. Bull., 2011, 62, 1596–1605, DOI:10.1016/j.marpolbul.2011.05.030.
-
J. Gigault, Current Opinion: what Is a Nanoplastic? Environmental Pollution, 2018 Search PubMed.
- A. A. Horton and D. K. A. Barnes, Microplastic Pollution in a Rapidly Changing World: Implications for Remote and Vulnerable Marine Ecosystems, Sci. Total Environ., 2020, 738, 140349, DOI:10.1016/j.scitotenv.2020.140349.
- C. F. Araujo, M. M. Nolasco, A. M. P. Ribeiro and P. J. A. Ribeiro-Claro, Identification of Microplastics Using Raman Spectroscopy: Latest Developments and Future Prospects, Water Res., 2018, 142, 426–440, DOI:10.1016/j.watres.2018.05.060.
- A. Cózar, F. Echevarría, J. I. González-Gordillo, X. Irigoien, B. Úbeda, S. Hernández-León, Á. T. Palma, S. Navarro, J. García-de-Lomas and A. Ruiz,
et al., Plastic Debris in the Open Ocean, Proc. Natl. Acad. Sci. U. S. A., 2014, 111, 10239–10244, DOI:10.1073/pnas.1314705111.
- M. Eriksen, L. C. M. Lebreton, H. S. Carson, M. Thiel, C. J. Moore, J. C. Borerro, F. Galgani, P. G. Ryan and J. Reisser, Plastic Pollution in the World's Oceans: More than 5 Trillion Plastic Pieces Weighing over 250,000 Tons Afloat at Sea, PLoS One, 2014, 9, e111913, DOI:10.1371/journal.pone.0111913.
- L. Lebreton and A. Andrady, Future Scenarios of Global Plastic Waste Generation and Disposal, Palgrave Commun., 2019, 5, 1–11, DOI:10.1057/s41599-018-0212-7.
- D. Eerkes-Medrano, R. C. Thompson and D. C. Aldridge, Microplastics in Freshwater Systems: A Review of the Emerging Threats, Identification of Knowledge Gaps and Prioritisation of Research Needs, Water Res., 2015, 75, 63–82, DOI:10.1016/j.watres.2015.02.012.
- N. U. Benson, O. D. Agboola, O. H. Fred-Ahmadu, G. E. De-la-Torre, A. Oluwalana and A. B. Williams, Micro (Nano) Plastics Prevalence, Food Web Interactions, and Toxicity Assessment in Aquatic Organisms: A Review, Front. Mar. Sci., 2022, 9, 851281 CrossRef.
- D. d. A. Miranda and G. F. de Carvalho-Souza, Are We Eating Plastic-Ingesting Fish?, Mar. Pollut. Bull., 2016, 103, 109–114, DOI:10.1016/j.marpolbul.2015.12.035.
-
C. M. Rochman, The Complex Mixture, Fate and Toxicity of Chemicals Associated with Plastic Debris in the Marine Environment, Marine Anthropogenic Litter, 2015, pp. 117–140 Search PubMed.
- X. Lim, Microplastics Are Everywhere — but Are They Harmful?, Nature, 2021, 593, 22–25, DOI:10.1038/d41586-021-01143-3.
- C. Campanale, C. Massarelli, I. Savino, V. Locaputo and V. F. Uricchio, A Detailed Review Study on Potential Effects of Microplastics and Additives of Concern on Human Health, Int. J. Environ. Res. Public Health, 2020, 17, 1212, DOI:10.3390/ijerph17041212.
- J. C. Prata, J. P. da Costa, I. Lopes, A. L. Andrady, A. C. Duarte and T. Rocha-Santos, A One Health Perspective of the Impacts of Microplastics on Animal, Human and Environmental Health, Sci. Total Environ., 2021, 777, 146094, DOI:10.1016/j.scitotenv.2021.146094.
- A. Rahman, A. Sarkar, O. P. Yadav, G. Achari and J. Slobodnik, Potential Human Health Risks Due to Environmental Exposure to Nano- and Microplastics and Knowledge Gaps: A Scoping Review, Sci. Total Environ., 2021, 757, 143872, DOI:10.1016/j.scitotenv.2020.143872.
- J. Gasperi, S. L. Wright, R. Dris, F. Collard, C. Mandin, M. Guerrouache, V. Langlois, F. J. Kelly and B. Tassin, Microplastics in Air: Are We Breathing It In?, Curr. Opin. Environ. Sci .Health, 2018, 1, 1–5, DOI:10.1016/j.coesh.2017.10.002.
- D. Schymanski, B. E. Oßmann, N. Benismail, K. Boukerma, G. Dallmann, E. von der Esch, D. Fischer, F. Fischer, D. Gilliland and K. Glas,
et al., Analysis of Microplastics in Drinking Water and Other Clean Water Samples with Micro-Raman and Micro-Infrared Spectroscopy: Minimum Requirements and Best Practice Guidelines, Anal. Bioanal. Chem., 2021, 413, 5969–5994, DOI:10.1007/s00216-021-03498-y.
- E. Danopoulos, M. Twiddy and J. M. Rotchell, Microplastic Contamination of Drinking Water: A Systematic Review, PLoS One, 2020, 15, e0236838, DOI:10.1371/journal.pone.0236838.
- Y. Cai, C. Li and Y. Zhao, A Review of the Migration and Transformation of Microplastics in Inland Water Systems, Int. J. Environ. Res. Public Health, 2022, 19, 148, DOI:10.3390/ijerph19010148.
- S. Jebril, M. de Valme García-Moreno, J. M. Palacios-Santander, C. Dridi and L. Cubillana-Aguilera, Development of a Cost-Effective and Sustainable Nanoplatform Based on a Green Gold Sononanoparticles/Carbon Black Nanocomposite for High-Performance Simultaneous Determination of Nanoplastics, Environ. Sci.: Nano, 2022, 9, 3126–3138 RSC.
- A. Fdhila, S. Jebril and C. Dridi, Development Cost-Effective Sensor for Simultaneous Determination of Nanoplastics Using Artificial Neural Network, IEEE Sens. J., 2023, 1, DOI:10.1109/JSEN.2023.3321995.
-
Z. Smith and K. R. Zodrow, Nanoplastic Settling Potential in Saline Environments, 2017 Search PubMed.
- M. A. Bhat, K. Gedik and E. O. Gaga, Atmospheric Micro (Nano) Plastics: Future Growing Concerns for Human Health, Air Qual., Atmos. Health, 2023, 16, 233–262, DOI:10.1007/s11869-022-01272-2.
- B. Toussaint, B. Raffael, A. Angers-Loustau, D. Gilliland, V. Kestens, M. Petrillo, I. M. Rio-Echevarria and G. Van Den Eede, Review of Micro- and Nanoplastic Contamination in the Food Chain, Food Addit. Contam.: Part A, 2019, 36, 639–673, DOI:10.1080/19440049.2019.1583381.
- A. Behera, S. R. Mahapatra, S. Majhi, N. Misra, R. Sharma, J. Singh, R. P. Singh, S. S. Pandey, K. R. Singh and R. G. Kerry, Gold Nanoparticle Assisted Colorimetric Biosensors for Rapid Polyethylene Terephthalate (PET) Sensing for Sustainable Environment to Monitor Microplastics, Environ. Res., 2023, 234, 116556, DOI:10.1016/j.envres.2023.116556.
- J. Wang, Y. Li, L. Lu, M. Zheng, X. Zhang, H. Tian, W. Wang and S. Ru, Polystyrene Microplastics Cause Tissue Damages, Sex-Specific Reproductive Disruption and Transgenerational Effects in Marine Medaka (Oryzias Melastigma), Environ. Pollut., 2019, 254, 113024, DOI:10.1016/j.envpol.2019.113024.
- J. Wang, X. Li, M. Gao, X. Li, L. Zhao and S. Ru, Polystyrene Microplastics Increase Estrogenic Effects of 17α-Ethynylestradiol on Male Marine Medaka (Oryzias Melastigma), Chemosphere, 2022, 287, 132312, DOI:10.1016/j.chemosphere.2021.132312.
- J. C. Prata, Airborne Microplastics: Consequences to Human Health?, Environ. Pollut., 2018, 234, 115–126, DOI:10.1016/j.envpol.2017.11.043.
- S. Martic, M. Tabobondung, S. Gao and T. Lewis, Emerging Electrochemical Tools for Microplastics Remediation
and Sensing, Front. Sens., 2022, 3, 958633 CrossRef.
- Z. Sobhani, Y. Lei, Y. Tang, L. Wu, X. Zhang, R. Naidu, M. Megharaj and C. Fang, Microplastics Generated When Opening Plastic Packaging, Sci. Rep., 2020, 10, 4841 CrossRef CAS PubMed.
- G. Jiménez-Skrzypek, C. Ortega-Zamora, J. González-Sálamo, C. Hernández-Sánchez and J. Hernández-Borges, The Current Role of Chromatography in Microplastic Research: Plastics Chemical Characterization and Sorption of Contaminants, J. Chromatogr. Open, 2021, 1, 100001 CrossRef.
- J. Huang, H. Chen, Y. Zheng, Y. Yang, Y. Zhang and B. Gao, Microplastic Pollution in Soils and Groundwater: Characteristics, Analytical Methods and Impacts, Chem. Eng. J., 2021, 425, 131870 CrossRef CAS.
- S. Mariano, S. Tacconi, M. Fidaleo, M. Rossi and L. Dini, Micro and Nanoplastics Identification: Classic Methods and Innovative Detection Techniques, Front. Toxicol., 2021, 3, 636640 CrossRef PubMed.
- S. Aralekallu, M. Palanna, S. Hadimani, K. Prabhu C. P., V. A. Sajjan, M. O. Thotiyl and L. K. Sannegowda, Biologically Inspired Catalyst for Electrochemical Reduction of Hazardous Hexavalent Chromium, Dalton Trans., 2020, 49, 15061, 10.1039/D0DT02752A.
- C. I. Justino, A. C. Duarte and T. A. Rocha-Santos, Recent Progress in Biosensors for Environmental Monitoring: A Review, Sensors, 2017, 17, 2918 CrossRef PubMed.
- A. Hashem, M. M. Hossain, M. Al Mamun, K. Simarani and M. R. Johan, Nanomaterials Based Electrochemical Nucleic Acid Biosensors for Environmental Monitoring: A Review, Appl. Surf. Sci. Adv., 2021, 4, 100064 CrossRef.
- K. Saravanakumar, S. SivaSantosh, A. Sathiyaseelan, K. V. Naveen, M. A. AfaanAhamed, X. Zhang, V. V. Priya, D. MubarakAli and M.-H. Wang, Unraveling the Hazardous Impact of Diverse Contaminants in the Marine Environment: Detection and Remedial Approach through Nanomaterials and Nano-Biosensors, J. Hazard. Mater., 2022, 433, 128720 CrossRef CAS PubMed.
- T. M. B. F. Oliveira, F. W. P. Ribeiro, S. Morais, P. de Lima-Neto and A. N. Correia, Removal and Sensing of Emerging Pollutants Released from (Micro)Plastic Degradation: Strategies Based on Boron-Doped Diamond Electrodes, Curr. Opin. Electrochem., 2022, 31, 100866, DOI:10.1016/j.coelec.2021.100866.
- N. P. Ivleva, Chemical Analysis of Microplastics and Nanoplastics: Challenges, Advanced Methods, and Perspectives, Chem. Rev., 2021, 121, 11886–11936, DOI:10.1021/acs.chemrev.1c00178.
- N. Belkhamssa, J. P. da Costa, C. I. L. Justino, P. S. M. Santos, S. Cardoso, A. C. Duarte, T. Rocha-Santos and M. Ksibi, Development of an Electrochemical Biosensor for Alkylphenol Detection, Talanta, 2016, 158, 30–34, DOI:10.1016/j.talanta.2016.05.044.
- T. O. Hara and B. Singh, Electrochemical biosensors for detection of pesticides and heavy metal toxicants in water: Recent trends and progress, ACS EST Water, 2021, 1, 462–478, DOI:10.1021/acsestwater.0c00125.
- A. Adekunle, V. Raghavan and B. Tartakovsky, A Comparison of Microbial Fuel Cell and Microbial Electrolysis Cell Biosensors for Real-Time Environmental Monitoring, Bioelectrochemistry, 2019, 126, 105–112, DOI:10.1016/j.bioelechem.2018.11.007.
- H. Zheng, Z. Yan, M. Wang, J. Chen and X. Zhang, Biosensor Based on Polyaniline-Polyacrylonitrile-Graphene Hybrid Assemblies for the Determination of Phenolic Compounds in Water Samples, J. Hazard. Mater., 2019, 378, 120714, DOI:10.1016/j.jhazmat.2019.05.107.
- A. Nag, M. E. E. Alahi, S. Feng and S. C. Mukhopadhyay, IoT-Based Sensing System for Phosphate Detection Using Graphite/PDMS Sensors, Sens. Actuators, A, 2019, 286, 43–50, DOI:10.1016/j.sna.2018.12.020.
- A. Khanmohammadi, A. Jalili Ghazizadeh, P. Hashemi, A. Afkhami, F. Arduini and H. Bagheri, An Overview to Electrochemical Biosensors and Sensors for the Detection of Environmental Contaminants, J. Iran. Chem. Soc., 2020, 17, 2429–2447, DOI:10.1007/s13738-020-01940-z.
- J. Guo, Uric Acid Monitoring with a Smartphone as the Electrochemical Analyzer, Anal. Chem., 2016, 88, 11986–11989, DOI:10.1021/acs.analchem.6b04345.
- J. Guo and X. Ma, Simultaneous Monitoring of Glucose and Uric Acid on a Single Test Strip with Dual Channels, Biosens. Bioelectron., 2017, 94, 415–419, DOI:10.1016/j.bios.2017.03.026.
- A. Tsopela, A. Laborde, L. Salvagnac, V. Ventalon, E. Bedel-Pereira, I. Séguy, P. Temple-Boyer, P. Juneau, R. Izquierdo and J. Launay, Development of a Lab-on-Chip Electrochemical Biosensor for Water Quality Analysis Based on Microalgal Photosynthesis, Biosens. Bioelectron., 2016, 79, 568–573, DOI:10.1016/j.bios.2015.12.050.
- S. Jebril, L. Cubillana-Aguilera, J. M. Palacios-Santander and C. Dridi, A Novel Electrochemical Sensor Modified with Green Gold Sononanoparticles and Carbon Black Nanocomposite for Bisphenol A Detection, Mater. Sci. Eng., B, 2021, 264, 114951, DOI:10.1016/j.mseb.2020.114951.
- S. Jebril, A. Fdhila and C. Dridi, Nanoengineering of Eco-Friendly Silver Nanoparticles Using Five Different Plant Extracts and Development of Cost-Effective Phenol Nanosensor, Sci. Rep., 2021, 11, 22060, DOI:10.1038/s41598-021-01609-4.
- M. Ben Jaballah, N. Ben Messaoud and C. Dridi, Nanoengineering of New Cost-Effective Nanosensor Based on Functionalized MWCNT and Ag Nanoparticles for Sensitive Detection of BPA in Drinking Water, Appl. Phys. A, 2021, 127, 713, DOI:10.1007/s00339-021-04857-3.
- N. Ben Messaoud, A. Ait Lahcen, C. Dridi and A. Amine, Ultrasound Assisted Magnetic Imprinted Polymer Combined Sensor Based on Carbon Black and Gold Nanoparticles for Selective and Sensitive Electrochemical Detection of Bisphenol A, Sens. Actuators, B, 2018, 276, 304–312, DOI:10.1016/j.snb.2018.08.092.
- F. Li, Z. Yu, X. Han and R. Y. Lai, Electrochemical Aptamer-Based Sensors for Food and Water Analysis: A Review, Anal. Chim. Acta, 2019, 1051, 1–23, DOI:10.1016/j.aca.2018.10.058.
- Z. Fredj and M. Sawan, Advanced Nanomaterials-Based Electrochemical Biosensors for Catecholamines Detection: Challenges and Trends, Biosensors, 2023, 13, 211, DOI:10.3390/bios13020211.
- Z. Fredj, B. Singh, M. Bahri, P. Qin and M. Sawan, Enzymatic Electrochemical Biosensors for Neurotransmitters Detection: Recent Achievements and Trends, Chemosensors, 2023, 11, 388, DOI:10.3390/chemosensors11070388.
- Y. Zheng, X. Song, Z. Fredj, S. Bian and M. Sawan, Challenges and Perspectives of Multi-Virus Biosensing Techniques: A Review, Anal. Chim. Acta, 2023, 1244, 340860 CrossRef CAS PubMed.
- L. Su, W. Jia, C. Hou and Y. Lei, Microbial Biosensors: A Review, Biosens. Bioelectron., 2011, 26, 1788–1799, DOI:10.1016/j.bios.2010.09.005.
- V. Mirceski, R. Gulaboski, M. Lovric, I. Bogeski, R. Kappl and M. Hoth, Square-Wave Voltammetry: A Review on the Recent Progress, Electroanalysis, 2013, 25, 2411–2422 CrossRef CAS.
- Z. Fredj, M. B. Ali, M. N. Abbas and E. Dempsey, Simultaneous Determination of Ascorbic Acid, Uric Acid and Dopamine Using Silver Nanoparticles and Copper Monoamino-Phthalocyanine Functionalised Acrylate Polymer, Anal. Methods, 2020, 12, 3883–3891 RSC.
- S. Palanisamy, B. Thirumalraj, S.-M. Chen, Y.-T. Wang, V. Velusamy and S. K. Ramaraj, A Facile Electrochemical Preparation of Reduced Graphene Oxide@Polydopamine Composite: A Novel Electrochemical Sensing Platform for Amperometric Detection of Chlorpromazine, Sci. Rep., 2016, 6, 33599, DOI:10.1038/srep33599.
- X. Xiao, Z. Zhang, F. Nan, Y. Zhao, P. Wang, F. He and Y. Wang, Mesoporous CuCo2O4 Rods Modified Glassy Carbon Electrode as a Novel Non-Enzymatic Amperometric Electrochemical Sensors with High-Sensitive Ascorbic Acid Recognition, J. Alloys Compd., 2021, 852, 157045, DOI:10.1016/j.jallcom.2020.157045.
- W. Qin, X. Liu, H. Chen and J. Yang, Amperometric Sensors for Detection of Phenol in Oilfield Wastewater Using Electrochemical Polymerization of Zincon Film, Anal. Methods, 2014, 6, 5734–5740, 10.1039/C3AY41855C.
- M. N. Karim and H. J. Lee, Amperometric Phenol Biosensor Based on Covalent Immobilization of Tyrosinase on Au Nanoparticle Modified Screen Printed Carbon Electrodes, Talanta, 2013, 116, 991–996 CrossRef PubMed.
- T. G. Satheesh Babu, D. Varadarajan, G. Murugan, T. Ramachandran and B. G. Nair, Gold Nanoparticle–Polypyrrole Composite Modified TiO2 Nanotube Array Electrode for the Amperometric Sensing of Ascorbic Acid, J. Appl. Electrochem., 2012, 42, 427–434, DOI:10.1007/s10800-012-0416-2.
- Y. Wang, Z. Ye and Y. Ying, New Trends in Impedimetric Biosensors for the Detection of Foodborne Pathogenic Bacteria, Sensors, 2012, 12, 3449–3471, DOI:10.3390/s120303449.
- F. Zina, N. M. Nooredeen, S. Azzouzi, M. B. Ali, M. N. Abbas and A. Errachid, Novel Sensitive Impedimetric Microsensor for Phosphate Detection Based on a Novel Copper Phthalocyanine Derivative, Anal. Lett., 2018, 51, 371–386 CrossRef CAS.
- A. Hayat, L. Barthelmebs and J.-L. Marty, Electrochemical Impedimetric Immunosensor for the Detection of Okadaic Acid in Mussel Sample, Sens. Actuators, B, 2012, 171–172, 810–815, DOI:10.1016/j.snb.2012.05.075.
- A. Chen and S. Chatterjee, Nanomaterials Based Electrochemical Sensors for Biomedical Applications, Chem. Soc. Rev., 2013, 42, 5425–5438 RSC.
- Z. Zhang, Y. Cong, Y. Huang and X. Du, Nanomaterials-Based Electrochemical Immunosensors, Micromachines, 2019, 10, 397 CrossRef PubMed.
- Z. Fredj, P. Wang, F. Ullah and M. Sawan, A Nanoplatform-Based Aptasensor to Electrochemically Detect Epinephrine Produced by Living Cells, Microchim. Acta, 2023, 190, 343 CrossRef CAS PubMed.
- H. Mao, M. Liu, Z. Cao, C. Ji, Y. Sun, D. Liu, S. Wu, Y. Zhang and X.-M. Song, Poly(4-Vinylphenylboronic Acid) Functionalized Polypyrrole/Graphene Oxide Nanosheets for Simultaneous Electrochemical Determination of Catechol and Hydroquinone, Appl. Surf. Sci., 2017, 420, 594–605, DOI:10.1016/j.apsusc.2017.05.188.
- L. A. Goulart, R. Gonçalves, A. A. Correa, E. C. Pereira and L. H. Mascaro, Synergic Effect of Silver Nanoparticles and Carbon Nanotubes on the Simultaneous Voltammetric Determination of Hydroquinone, Catechol, Bisphenol A and Phenol, Microchim. Acta, 2018, 185, 1–9 CrossRef CAS PubMed.
- D. Yin, J. Liu, X. Bo and L. Guo, Cobalt-Iron Selenides Embedded in Porous Carbon Nanofibers for Simultaneous Electrochemical Detection of Trace of Hydroquinone, Catechol and Resorcinol, Anal. Chim. Acta, 2020, 1093, 35–42, DOI:10.1016/j.aca.2019.09.057.
- S. B. Arpitha, B. E. Kumara Swamy and J. K. Shashikumara, An Efficient Electrochemical Sensor Based on ZnO/Co3O4 Nanocomposite Modified Carbon Paste Electrode for the Sensitive Detection of Hydroquinone and Resorcinol, Inorg. Chem. Commun., 2023, 152, 110656, DOI:10.1016/j.inoche.2023.110656.
- I. C. Lekshmi, I. Rudra, R. Pillai, C. Sarika, M. S. Shivakumar, C. Shivakumara, S. B. Konwar and B. Narasimhamurthy, Enhanced Catechol Biosensing on Metal Oxide Nanocrystal Sensitized Graphite Nanoelectrodes through Preferential Molecular Adsorption, J. Electroanal. Chem., 2020, 867, 114190, DOI:10.1016/j.jelechem.2020.114190.
- N. Hareesha, J. G. Manjunatha, C. Raril, A. M. Tighezza, M. D. Albaqami and M. Sillanpää, Electrochemically Polymerized Glutamine-Activated Graphite Paste Surface as a Green Biosensor for Sensitive Catechol Detection in Water Samples, J. Mater. Sci.: Mater. Electron., 2023, 34, 533, DOI:10.1007/s10854-023-09951-1.
- X. Zhang, J. Zhu, Z. Wu, W. Wen, X. Zhang and S. Wang, Electrochemical Sensor Based on Confined Synthesis of Gold Nanoparticles @ Covalent Organic Frameworks for the Detection of Bisphenol A, Anal. Chim. Acta, 2023, 1239, 340743, DOI:10.1016/j.aca.2022.340743.
- T. S. Sunil Kumar Naik, S. Singh, P. N, R. Varshney, B. Uppara, J. Singh, N. A. Khan, L. Singh, M. Zulqarnain Arshad and C. P. Ramamurthy, Advanced Experimental Techniques for the Sensitive Detection of a Toxic Bisphenol A Using UiO-66-NDC/GO-Based Electrochemical Sensor, Chemosphere, 2023, 311, 137104, DOI:10.1016/j.chemosphere.2022.137104.
- P. Karami-Kolmoti, H. Beitollahi and S. Modiri, Electrochemical Sensor for Simple and Sensitive Determination of Hydroquinone in Water Samples Using Modified Glassy Carbon Electrode, Biomedicines, 2023, 11, 1869, DOI:10.3390/biomedicines11071869.
- L. Liao, P. Zhou, F. Xiao, W. Tang, M. Zhao, R. Su, P. He, D. Yang, L. Bian and B. Tang, Electrochemical Sensor Based on Ni/N-Doped Graphene Oxide for the Determination of Hydroquinone and Catechol, Ionics, 2023, 29, 1605–1615, DOI:10.1007/s11581-023-04892-5.
- G. Veerapandi, R. Govindan and C. Sekar, Quick and Accurate Determination of Hazardous Phenolic Compounds Using CaCu2O3 Nanorods Based Electrochemical Sensor, Chemosphere, 2023, 313, 137370, DOI:10.1016/j.chemosphere.2022.137370.
- A. Radha and S.-F. Wang, Designing Hybrid Lanthanum Stannate/Functionalized Halloysite Nanotubes as Electrode Material for Electrochemical Detection of 4-(Methylamino)Phenol (Metol) in Environmental Samples, ACS Sustainable Chem. Eng., 2023, 11, 5072–5081, DOI:10.1021/acssuschemeng.2c06924.
- Y. Xue, M. Noroozifar, R. M. A. Sullan and K. Kerman, Electrochemical Simultaneous Determination of Hydroquinone, Catechol, Bisphenol A, and Bisphenol S Using a Novel Mesoporous Nickel-Modified Carbon Sensor, Chemosphere, 2023, 342, 140003 CrossRef CAS PubMed.
- N. Sivaraman, V. Duraisamy, S. M. S. Kumar and R. Thangamuthu, N, S Dual Doped Mesoporous Carbon Assisted Simultaneous Electrochemical Assay of Emerging Water Contaminant Hydroquinone and Catechol, Chemosphere, 2022, 307, 135771 CrossRef CAS PubMed.
- X. Wang, M. Li, M. Wu, Y. Shi, J. Yang, J. Shan and L. Liu, Simultaneous Determination of Bisphenol A and Bisphenol S Using Multi-Walled Carbon Nanotubes Modified Electrode, Int. J. Electrochem. Sci., 2018, 13, e11922 Search PubMed.
- Y.-H. Pang, Y.-Y. Huang, L. Wang, X.-F. Shen and Y.-Y. Wang, Determination of Bisphenol A and Bisphenol S by a Covalent Organic Framework Electrochemical Sensor, Environ. Pollut., 2020, 263, 114616 CrossRef CAS.
- S. Jahani, A. Sedighi, A. Toolabi and M. M. Foroughi, Development and Characterization of La2O3 Nanoparticles@ Snowflake-like Cu2S Nanostructure Composite Modified Electrode and Application for Simultaneous Detection of Catechol, Hydroquinone and Resorcinol as an Electrochemical Sensor, Electrochim. Acta, 2022, 416, 140261 CrossRef CAS.
- J. G. A. Manjunatha, Surfactant Enhanced Graphene Paste Electrode as an Effective Electrochemical Sensor for the Sensitive and Simultaneous Determination of Catechol and Resorcinol, Chem. Data Collect., 2020, 25, 100331 CrossRef CAS.
- M. E. Napier, D. O. Hull and H. H. Thorp, Electrocatalytic Oxidation of DNA-Wrapped Carbon Nanotubes, J. Am. Chem. Soc., 2005, 127, 11952–11953 CrossRef CAS PubMed.
- S. Aralekallu, I. Mohammed, N. Manjunatha, M. Palanna, Dhanjai and L. K. Sannegowda, Synthesis of Novel Azo Group Substituted Polymeric Phthalocyanine for Amperometric Sensing of Nitrite, Sens. Actuators, B, 2019, 282, 417, DOI:10.1016/j.snb.2018.11.093.
- A. Domínguez-Aragón, R. B. Dominguez and E. A. Zaragoza-Contreras, Simultaneous Detection of Dihydroxybenzene Isomers Using Electrochemically Reduced Graphene Oxide-Carboxylated Carbon Nanotubes/Gold Nanoparticles Nanocomposite, Biosensors, 2021, 11, 321 CrossRef PubMed.
- M. Y. Ali, A. U. Alam and M. M. Howlader, Fabrication of Highly Sensitive Bisphenol A Electrochemical Sensor Amplified with Chemically Modified Multiwall Carbon Nanotubes and β-Cyclodextrin, Sens. Actuators, B, 2020, 320, 128319 CrossRef CAS.
- Y.-P. Zhu, L. Yang, A.-J. Wang and J.-J. Feng, Graphitic Carbon-Coated PtCoNi Alloys Supported on N-Doped Porous Carbon Nanoflakes for Sensitive Detection of Bisphenol A, ACS Appl. Nano Mater., 2023, 6, 8726–8734, DOI:10.1021/acsanm.3c01113.
- Y. Dong, J. Lin, Y. Chen, F. Fu, Y. Chi and G. Chen, Graphene Quantum Dots, Graphene Oxide, Carbon Quantum Dots and Graphite Nanocrystals in Coals, Nanoscale, 2014, 6, 7410–7415 RSC.
- H. Li, Z. Kang, Y. Liu and S.-T. Lee, Carbon Nanodots: Synthesis, Properties and Applications, J. Mater. Chem., 2012, 22, 24230–24253 RSC.
- C. Wei, Q. Huang, S. Hu, H. Zhang, W. Zhang, Z. Wang, M. Zhu, P. Dai and L. Huang, Simultaneous Electrochemical Determination of Hydroquinone, Catechol and Resorcinol at Nafion/Multi-Walled Carbon Nanotubes/Carbon Dots/Multi-Walled Carbon Nanotubes Modified Glassy Carbon Electrode, Electrochim. Acta, 2014, 149, 237–244 CrossRef CAS.
- X. Zhan, S. Hu, J. Wang, H. Chen, X. Chen, J. Yang, H. Yang and Z. Su, One-Pot Electrodeposition of Metal Organic Frameworks Composite Accelerated by Gold Nanoparticles and Electroreduced Carbon Dots for Electroanalysis of Bisphenol A in Real Plastic Samples, Sens. Actuators, B, 2021, 346, 130499, DOI:10.1016/j.snb.2021.130499.
- K. Rajesh, D. R. Kumar, P. Balaji Bhargav, R. Manigandan, N. Ahmed, C. Balaji and J. Shim, Carbon Dot–V2O5 Layered Nanoporous Architectures for Electrochemical Detection of Bisphenol A: An Analytical Approach, J. Environ. Chem. Eng., 2022, 10, 108206, DOI:10.1016/j.jece.2022.108206.
- A. Martin and A. Escarpa, Graphene: The Cutting–Edge Interaction between Chemistry and Electrochemistry, TrAC, Trends Anal. Chem., 2014, 56, 13–26 CrossRef CAS.
- X. Zhou, Z. He, Q. Lian, Z. Li, H. Jiang and X. Lu, Simultaneous Determination of Dihydroxybenzene Isomers Based on Graphene-Graphene Oxide Nanocomposite Modified Glassy Carbon Electrode, Sens. Actuators, B, 2014, 193, 198–204, DOI:10.1016/j.snb.2013.11.085.
- S. Palanisamy, K. Thangavelu, S.-M. Chen, B. Thirumalraj and X.-H. Liu, Preparation and Characterization of Gold Nanoparticles Decorated on Graphene Oxide@ Polydopamine Composite: Application for Sensitive and Low Potential Detection of Catechol, Sens. Actuators, B, 2016, 233, 298–306 CrossRef CAS.
- K. Chen, Z.-L. Zhang, Y.-M. Liang and W. Liu, A Graphene-Based Electrochemical Sensor for Rapid Determination of Phenols in Water, Sensors, 2013, 13, 6204–6216, DOI:10.3390/s130506204.
- S. Zheng, N. Zhang, L. Li, T. Liu, Y. Zhang, J. Tang, J. Guo and S. Su, Synthesis of Graphene Oxide-Coupled CoNi Bimetallic MOF Nanocomposites for the Simultaneous Analysis of Catechol and Hydroquinone, Sensors, 2023, 23, 6957, DOI:10.3390/s23156957.
- A.-Y. Zha, Q.-B. Zha, Z. Li, H.-M. Zhang, X.-F. Ma, W. Xie and M.-S. Zhu, Surfactant-Enhanced Electrochemical Detection of Bisphenol A Based on Au on ZnO/Reduced Graphene Oxide Sensor, Rare Met., 2023, 42, 1274–1282, DOI:10.1007/s12598-022-02172-1.
-
S. Aralekallu and L. K. Sannegowda, Chapter 25 - Metal Nanoparticles for Electrochemical Sensing Applications, in Micro and Nano Technologies: Handbook of Nanomaterials for Sensing Applications, ed. Hussain, C. M. and Kailasa, S. K., Elsevier, 2021, pp. 589–629, 978-0-12-820783-3 Search PubMed.
- J. Huang, X. Zhang, S. Liu, Q. Lin, X. He, X. Xing and W. Lian, Electrochemical Sensor for Bisphenol A Detection Based on Molecularly Imprinted Polymers and Gold Nanoparticles, J. Appl. Electrochem., 2011, 41, 1323 CrossRef CAS.
- M. Baccarin, M. A. Ciciliati, O. N. Oliveira Jr, E. T. Cavalheiro and P. A. Raymundo-Pereira, Pen Sensor Made with Silver Nanoparticles Decorating Graphite-Polyurethane Electrodes to Detect Bisphenol-A in Tap and River Water Samples, Mater. Sci. Eng., C, 2020, 114, 110989 CrossRef CAS PubMed.
- A. Eftekhari, M. Dalili, Z. Karimi, S. Rouhani, A. Hasanzadeh, S. Rostamnia, S. Khaksar, A. O. Idris, H. Karimi-Maleh and M. L. Yola,
et al., Sensitive and Selective Electrochemical Detection of Bisphenol A Based on SBA-15 like Cu-PMO Modified Glassy Carbon Electrode, Food Chem., 2021, 358, 129763, DOI:10.1016/j.foodchem.2021.129763.
- M. Amiri and H. Mahmoudi-Moghaddam, Green Synthesis of ZnO/ZnCo2O4 and Its Application for Electrochemical Determination of Bisphenol A, Microchem. J., 2021, 160, 105663, DOI:10.1016/j.microc.2020.105663.
- P. Mohammadzadeh Jahani, H. Beitollahi, S. Tajik and H. Tashakkorian, Selective Electrochemical Determination of Bisphenol A via a Fe3O4 NPs Derivative-Modified Graphite Screen-Printed Electrode, Int. J. Environ. Anal. Chem., 2020, 100, 1209–1225, DOI:10.1080/03067319.2019.1651299.
- V. Movahed, L. Arshadi, M. Ghanavati, E. M. Nejad, Z. Mohagheghzadeh and M. Rezaei, Simultaneous Electrochemical Detection of Antioxidants Hydroquinone, Mono-Tert-Butyl Hydroquinone and Catechol in Food and Polymer Samples Using ZnO@MnO2-rGO Nanocomposite as Sensing Layer, Food Chem., 2023, 403, 134286, DOI:10.1016/j.foodchem.2022.134286.
- M. Shen, W. Li, F. Chen, L. Chen, Y. Chen, S. Chen, S. Ren and D. Han, A Ratiometric Electrochemical Sensor for Bisphenol A Detection Based on Ag@Fe3O4-rGO Composite, Microchem. J., 2023, 186, 108315, DOI:10.1016/j.microc.2022.108315.
- S. Cichosz, A. Masek and M. Zaborski, Polymer-Based Sensors: A Review, Polym. Test., 2018, 67, 342–348, DOI:10.1016/j.polymertesting.2018.03.024.
- S. J. Park and Y. H. Ahn, Detection of Polystyrene Microplastic Particles in Water Using Surface-Functionalized Terahertz Microfluidic Metamaterials, Appl. Sci., 2022, 12, 7102, DOI:10.3390/app12147102.
- F. Tan, L. Cong, X. Li, Q. Zhao, H. Zhao, X. Quan and J. Chen, An Electrochemical Sensor Based on Molecularly Imprinted Polypyrrole/Graphene Quantum Dots Composite for Detection of Bisphenol A in Water Samples, Sens. Actuators, B, 2016, 233, 599–606 CrossRef CAS.
- R. B. González-González, E. A. Flores-Contreras, E. González-González, N. E. T. Castillo, R. Parra-Saldívar and H. M. N. Iqbal, Biosensor Constructs for the Monitoring of Persistent Emerging Pollutants in Environmental Matrices, Ind. Eng. Chem. Res., 2023, 62(11), 4503–4520, DOI:10.1021/acs.iecr.2c00421.
- L. Alvarado-Ramírez, M. Rostro-Alanis, J. Rodríguez-Rodríguez, J. E. Sosa-Hernández, E. M. Melchor-Martínez, H. M. N. Iqbal and R. Parra-Saldívar, Enzyme (Single and Multiple) and Nanozyme Biosensors: Recent Developments and Their Novel Applications in the Water-Food-Health Nexus, Biosensors, 2021, 11, 410, DOI:10.3390/bios11110410.
-
Y.-C. Zhu, L.-P. Mei, Y.-F. Ruan, N. Zhang, W.-W. Zhao, J.-J. Xu and H.-Y. Chen, Chapter 8 - Enzyme-Based Biosensors and Their Applications, in Biomass, Biofuels, Biochemicals: Advances in Enzyme Technology, ed. Singh, R. S., Singhania, R. R., Pandey, A. and Larroche, C., Elsevier, 2019, pp. 201–223, ISBN 978-0-444-64114-4 Search PubMed.
-
A. Sarkar, K. D. Sarkar, V. Amrutha and K. Dutta, Chapter 15 - An Overview of Enzyme-Based Biosensors for Environmental Monitoring, in Tools, Techniques and Protocols for Monitoring Environmental Contaminants, ed. Kaur Brar, S., Hegde, K. and Pachapur, V. L., Elsevier, 2019, pp. 307–329, ISBN 978-0-12-814679-8 Search PubMed.
-
J. R. Aggas and A. Guiseppi-Elie, 2.5.13 - Responsive Polymers in the Fabrication of Enzyme-Based Biosensors, in Biomaterials Science, ed. Wagner, W. R., Sakiyama-Elbert, S. E., Zhang, G. and Yaszemski, M. J., Academic Press, 4th edn, 2020, pp. 1267–1286, ISBN 978-0-12-816137-1 Search PubMed.
- A. Othman, A. Karimi and S. Andreescu, Functional Nanostructures for Enzyme Based Biosensors: Properties, Fabrication and Applications, J. Mater. Chem. B, 2016, 4, 7178–7203, 10.1039/C6TB02009G.
- A. M. Baracu and L. A. D. Gugoasa, Review—Recent Advances in Microfabrication, Design and Applications of Amperometric Sensors and Biosensors, J. Electrochem. Soc., 2021, 168, 037503, DOI:10.1149/1945-7111/abe8b6.
- M. Pohanka, Biosensors and Bioassays Based on Lipases, Principles and Applications, a Review, Molecules, 2019, 24, 616, DOI:10.3390/molecules24030616.
-
M. Nazal and H. Zhao, Heavy Metals: Their Environmental Impacts and Mitigation, BoD – Books on Demand, 2021, ISBN 978-1-83968-121-9 Search PubMed.
- P. Mehrotra, Biosensors and Their Applications – A Review, J Oral Biol. Craniofac. Res., 2016, 6, 153–159, DOI:10.1016/j.jobcr.2015.12.002.
-
S. Ahmed, N. Shaikh, N. Pathak, A. Sonawane, V. Pandey and S. Maratkar, Chapter 3 - An Overview of Sensitivity and Selectivity of Biosensors for Environmental Applications, in Tools, Techniques and Protocols for Monitoring Environmental Contaminants, ed. Kaur Brar, S., Hegde, K. and Pachapur, V. L., Elsevier, 2019, pp. 53–73, ISBN 978-0-12-814679-8 Search PubMed.
- L. Wu, X. Lu, K. Niu, Dhanjai and J. Chen, Tyrosinase Nanocapsule Based Nano-Biosensor for Ultrasensitive and Rapid Detection of Bisphenol A with Excellent Stability in Different Application Scenarios, Biosens. Bioelectron., 2020, 165, 112407, DOI:10.1016/j.bios.2020.112407.
- T. Liu, Q. Zhao, Y. Xie, D. Jiang, Z. Chu and W. Jin, In Situ Fabrication of Aloe-like Au–ZnO Micro/Nanoarrays for Ultrasensitive Biosensing of Catechol, Biosens. Bioelectron., 2020, 156, 112145, DOI:10.1016/j.bios.2020.112145.
- Y. Shan, Y. Han, X. Yao, T. Liu, Y. Liu, Z. Chu and W. Jin, A Novel Prussian Blue/PANI Nanostructure-Based Biosensor for Ultrasensitive Determination of Trace Hydroquinone, Sens. Actuators, B, 2023, 393, 134137, DOI:10.1016/j.snb.2023.134137.
- Y. Zhao, J. Yang, Y. Wu, B. Huang, L. Xu, J. Yang, B. Liang and L. Han, Construction of Bacterial Laccase Displayed on the Microbial Surface for Ultrasensitive Biosensing of Phenolic Pollutants with Nanohybrids-Enhanced Performance, J. Hazard. Mater., 2023, 452, 131265, DOI:10.1016/j.jhazmat.2023.131265.
- L. Liu, S. Anwar, H. Ding, M. Xu, Q. Yin, Y. Xiao, X. Yang, M. Yan and H. Bi, Electrochemical Sensor Based on F, N-Doped Carbon Dots Decorated Laccase for Detection of Catechol, J. Electroanal. Chem., 2019, 840, 84–92 CrossRef CAS.
-
E. B. Aydin, M. Aydin and M. K. Sezgintürk, Chapter One - Advances in Electrochemical Immunosensors, in Advances in Clinical Chemistry, ed. Makowski, G. S., Elsevier, 2019, vol. 92, pp. 1–57 Search PubMed.
-
M. Zourob, S. Elwary and A. Khademhosseini, Recognition Receptors in Biosensors, Springer, 2010 Search PubMed.
- G. R. Marchesini, E. Meulenberg, W. Haasnoot and H. Irth, Biosensor Immunoassays for the Detection of Bisphenol A, Anal. Chim. Acta, 2005, 528, 37–45 CrossRef CAS.
- C. Tuerk and L. Gold, Systematic Evolution of Ligands by Exponential Enrichment: RNA Ligands to Bacteriophage T4 DNA Polymerase, Science, 1990, 249, 505–510 CrossRef CAS PubMed.
- B. Kudlak and M. Wieczerzak, Aptamer Based Tools for Environmental and Therapeutic Monitoring: A Review of Developments, Applications, Future Perspectives, Crit. Rev. Environ. Sci. Technol., 2020, 50, 816–867 CrossRef.
- R. Sullivan, M. C. Adams, R. R. Naik and V. T. Milam, Analyzing Secondary Structure Patterns in DNA Aptamers Identified via CompELS, Molecules, 2019, 24, 1572 CrossRef CAS PubMed.
- C. Roxo, W. Kotkowiak and A. Pasternak, G-Quadruplex-Forming Aptamers—Characteristics, Applications, and Perspectives, Molecules, 2019, 24, 3781 CrossRef CAS PubMed.
- H. Cui, J. Wu, S. Eda, J. Chen, W. Chen and L. Zheng, Rapid Capacitive Detection of Femtomolar Levels of Bisphenol A Using an Aptamer-Modified Disposable Microelectrode Array, Microchim. Acta, 2015, 182, 2361–2367, DOI:10.1007/s00604-015-1556-y.
- F. Xue, J. Wu, H. Chu, Z. Mei, Y. Ye, J. Liu, R. Zhang, C. Peng, L. Zheng and W. Chen, Electrochemical Aptasensor for the Determination of Bisphenol A in Drinking Water, Microchim. Acta, 2013, 180, 109–115, DOI:10.1007/s00604-012-0909-z.
|
This journal is © The Royal Society of Chemistry 2024 |
Click here to see how this site uses Cookies. View our privacy policy here.