DOI:
10.1039/D4SU00297K
(Critical Review)
RSC Sustain., 2024,
2, 2768-2780
Ionic liquid-based extraction of metal ions via polymer inclusion membranes: a critical review
Received
11th June 2024
, Accepted 9th September 2024
First published on 10th September 2024
Abstract
Polymer Inclusion Membranes (PIMs) have significantly advanced the field of membrane-based separation technologies introducing an innovative method for the selective transport and extraction of metal ions. The incorporation of ionic liquids (ILs) into PIMs leverages the exceptional characteristics of ILs to boost both selectivity and efficiency in the separation of metal ions. This synergy advances metal separation processes towards greener and more sophisticated solutions perfectly aligning with green chemistry and environmental sustainability. This review presents an overview of current knowledge on PIMs including the distinct roles of their different components. It critically assesses the different strategies essential for achieving optimal membrane performance and ensuring stability and selectivity of PIMs. Future research directions are discussed particularly focusing on the understanding of transport dynamics within PIMs and refining membrane compositions to reduce the risk of carrier leakage. These investigations promise to enhance the efficiency and environmental friendliness of metal ion separation propelling the field towards more effective and sustainable practices. This review can serve as a roadmap for ongoing research, promoting the advancement of IL-based extraction of metal ions via PIMs for sustainable and efficient metal separation processes.
Sustainability spotlight
Metals are essential to modern technology, including electric vehicle batteries and renewable energy systems. The increasing demand for critical metals such as cobalt, platinum group metals, and rare earth elements has intensified the need for sustainable metal recovery and recycling methods. Traditional extraction and separation techniques often involve hazardous chemicals and energy-intensive processes, leading to significant environmental impact. The development of ionic liquid-based polymer inclusion membranes (PIMs) presents an advancement in sustainable metal recovery. These PIMs selectively extract and recycle metal ions using less energy and fewer harmful reagents, reducing the ecological footprint of metal extraction. This innovative approach aligns with multiple United Nations Sustainable Development Goals (SDGs), including SDG 12 (Responsible Consumption and Production) and SDG 13 (Climate Action). By enhancing the efficiency and environmental sustainability of metal recovery processes, ionic liquid-based PIMs contribute to a circular economy and help mitigate the environmental impact of metal extraction and waste, supporting the transition to more sustainable industrial practices.
|
1 Introduction
The modern world relies heavily on metals for a wide range of applications, from basic infrastructure and transportation to advanced technologies and renewable energy systems. Metals such as copper, nickel, cobalt, and rare earth elements are critical to the production of electronic devices, batteries, and other high-tech equipment. Efficient use of these metals has become increasingly important as the surge in global demand for metals.1,2 Metals predominantly occur in ores and complex blends with other metals, minerals, and impurities posing considerable challenges in their extraction.
Efficient metal extraction and separation methods are crucial for maintaining the sustainable metal supply chain, as ongoing technological advancement relies heavily on a stable supply of metals. However, despite the importance of metal extraction for economic and technological growth, it presents substantial environmental challenges which include habitat destruction and soil and water contamination. These impacts underscore the need for more sustainable extraction methods that minimize ecological damage and reduce carbon footprints. Furthermore, the uneven geographical distribution of metal resources poses supply chain risks. Diversifying sources and advancing extraction technologies are vital for mitigating these risks. Sustainable practices in metal extraction not only conserve natural resources but also address the urgent issues of environmental degradation and climate change.3–6
As shown in Table 1 conventional methods such as solvent extraction, precipitation, ion exchange, and adsorption have been utilized for metal separation. However, each method has its advantages and disadvantages concerning selectivity, environmental footprint, and energy efficiency. For example, solvent extraction often employs large amount of volatile organic solvents posing disposal and health risks.7–10 Precipitation can result in less pure outcomes and significant waste sludge; while adsorption processes, though effective, face limitations in capacity and selectivity, making them unsuitable for certain separation tasks.11 These challenges have led to the pursuit of novel separation techniques aimed at enhancing process efficiency, product purity, and eco-friendliness, culminating in the development of liquid membrane-based technologies.
Table 1 Comparison of different conventional methods for metal ion separations
Methods |
Advantages |
Disadvantages |
Solvent extraction |
• High efficiency for specific extractions |
• Less flexible; may require multiple extraction steps for mixed ions |
• Widely used in industrial processes |
• Large volumes of extractant needed |
|
• Environmental concerns, solvent disposal issues |
Ion exchange |
• Well established and simple process for metal separation |
• Matrix degradation over time |
•Regeneration of resins |
• Resin fouling and saturation can limit continuous operation |
Adsorption |
• Tunable with different adsorbents |
• Lower selectivity, variability in performance |
• Effective and simple process |
• Disposal of spent adsorbents can pose environmental concerns |
Precipitation |
• Simple and easy process |
• Sludge generation |
• Effective for separating metal ions at high concentration |
• Impurities may co-precipitate with the desired product |
Polymer inclusion membranes |
• High selectivity due to tunable IL properties |
• Stability of membrane |
• Lower environmental footprint |
• Scalability to industrial process |
• One step process of extraction and stripping |
|
Liquid membrane technologies represent a significant leap in separation science and offer innovative solutions for the selective and efficient separation of metal species across various sectors including chemical processing, environmental remediation, and resource recovery. These technologies operate by transporting ions or molecules selectively through a liquid phase that acts as a barrier between two different phases. Over time, various types of liquid membranes have been developed, each with their own unique properties and applications. These types include bulk liquid membranes (BLMs), emulsion liquid membranes (ELMs), and supported liquid membranes (SLMs). Each membrane type has been specifically designed to address specific separation requirements and challenges.12–16
Despite the advancements in liquid membrane technologies, challenges such as inadequate mechanical stability and the risk of carrier leakage persist. To address these limitations, polymer inclusion membranes (PIMs) were introduced. PIMs are designed by embedding the liquid phase within a solid matrix, which enhances the membrane's stability and extends its operational lifespan, making it more viable for large-scale industrial applications.17 These membranes mark a significant advancement in membrane-based separation technologies, particularly for the extraction and transport of metal ions. PIMs have revolutionized traditional separation techniques by offering superior selectivity, versatility, cost-effectiveness and ability to perform simultaneous extraction and stripping in a single step. Notably, PIMs are distinguished by their selectivity, ease of use, recyclability, and lower energy requirements, positioning them as a sustainable and environmentally friendly option for metal resource recovery.
Yoshida et al., investigated the separation of Sc(III) from other metal ions using both solvent extraction (SX) and through PIMs with D2EHAF as the extractant/carrier. Their results indicated that SX effectively separated Sc(III) and Fe(III) from other metal ions. However, separating Sc(III) from Fe(III) proved challenging due to their same charge and similar hydration enthalpies. In contrast, the use of a PIM system, comprising 30 wt% cellulose triacetate (CTA), 40 wt% N-[N,N-di(2-ethylhexyl)aminocarbonylmethyl] phenylalanine (D2EHAF), and 30 wt% 2-nitrophenyl octyl ether (2NPOE), enabled quantitative transport of Sc(III) and partial separation from Fe(III) through kinetic differences in extraction and stripping processes. Minimal transport of other metal ions was observed through the PIM, with transport efficiencies calculated at 94% for Sc(III) and 32% for Fe(III).18 Similarly, Pospiech et al. investigated the separation of Fe(III) from chloride solutions containing Mn(II), Ni(II), Co(II), and Cu(II) using both SX and PIM techniques. In their study, TBP served as the extractant/ion carrier in both methods. SX results showed effective extraction of Fe(III) with minimal co-extraction of other metal ions. However, in the PIM system – featuring TBP as the ion carrier, o-nitrophenyl octyl ether (ONPOE) as the plasticizer, and CTA as the polymer matrix – Fe(III) was selectively transported from acidic chloride solutions,19 underscoring the high selectivity of PIMs. These membranes offer advantages such as minimal carrier usage, lower environmental footprint, and a simplified one-step extraction and stripping process compared to traditional solvent extraction methods used in industrial applications.
In the pursuit of enhancing environmental safety and achieving metal sustainability, ionic liquids (ILs) have emerged as promising green solvents or extractants for the separation of metal ions. Their distinctive characteristics, such as negligible vapor pressure, exceptional thermal stability, and the ability to dissolve a diverse range of substances, distinguish them from conventional organic solvents. The minimal volatility and reduced flammability of ILs compared to traditional organic solvents diminish the potential for atmospheric pollution, making them more eco-friendly option for specific applications. However, it is crucial to recognize that the environmental and safety profiles of ILs can vary considerably based on their specific chemical structures. While some ILs present minimal environmental risks, others, such as those based on long alkyl chain imidazolium, may have more significant environmental impacts.20–24 The integration of ILs in extraction processes via PIMs represents an innovative approach in metal ion separation. By leveraging on the distinctive properties of ILs, such as their selectivity, non-volatility, and thermal stability, this method enhances separation efficiency while minimizing environmental impact. This advancement underscores the potential of IL-based PIMs in providing effective and sustainable solutions for metal ion separation.
This review presents a thorough overview of IL-based PIMs for metal ion separation. It delves into the fundamental insights governing metal ion transport within PIMs, explores strategies for enhancing membrane performance, and discusses methods for detailed membrane characterization, stability assessment. Additionally, it examines the potential application of IL-based PIMs in metal ion separation. The review concludes by outlining future research directions aimed at advancing PIM technology to promote metal sustainability. This review will serve as a valuable resource for researchers working with IL-based PIMs for metal ion separation, fostering innovations that contribute to more sustainable metal production and recycling practices.
2 Polymer inclusion membranes
PIMs are advanced materials comprising a polymer matrix, carriers, and plasticizers enabling selective transport of target species across the membrane. The composition of these membranes imparts durability, flexibility, and chemical resistance. PIMs offer a more sustainable and streamlined approach compared to traditional solvent extraction methods, reducing the need for extractants. The fabrication of PIMs is pivotal, as it directly influences their efficacy in metal ion separation processes. The primary fabrication technique involves solution casting followed by solvent evaporation. This process entails dissolving a polymer with desirable mechanical strength and chemical stability in a suitable solvent, along with incorporating carriers that selectively bind to target metal ions, and plasticizers to enhance membrane flexibility. The resulting mixture is spread onto a flat surface and allowed to dry, enabling the solvent to evaporate and the membrane to form. This method is preferred for its simplicity and scalability, as it allows for precise control over membrane thickness.
The efficacy of PIMs has been demonstrated through various studies. For instance, Schow et al., demonstrated that a PIM incorporating CTA, and a crown ether achieved a transport rate for K+ ions that was three orders of magnitude higher than that of a SLM. This PIM also exhibited remarkable durability, maintaining consistent ion flux without degradation over three months.25 Similarly, Aguilar et al. reported that a PIM utilizing CTA and Kelex 100 as carriers showed exceptional selectivity in separating Pb(II) from Cd(II), outperforming traditional solvent extraction methods.26 The superior selectivity, mechanical robustness, and longevity of PIMs make them a versatile and effective solution for various separation tasks, illustrating their potential across numerous applications. The combination of polymer matrix, carriers, and plasticizers is crucial to the membrane's performance in metal ion separation, underscoring the importance of each component in optimizing PIM effectiveness.
2.1 Polymer matrix
The polymer matrix acts as the structural backbone of PIMs, offering mechanical support, chemical stability, and a house for the carrier and plasticizer. Essential attributes of polymer matrix hosts include robust mechanical properties, high chemical resistance, thermoplastic behaviour, and compatibility with the carriers and plasticizers.27,28 Common polymer matrix used in PIMs are polyvinyl chloride (PVC), CTA, polyvinylidene fluoride (PVDF), and poly(vinylidene fluoride-co-hexafluoropropylene) (PVDF-HFP). These polymers are selected for their unique properties like polarity, crystallinity, and chemical stability, which are crucial for PIM's performance in different applications.29–36 The selection of the polymer matrix is pivotal and influences the membrane's stability, durability, and efficiency in transporting ions. For instance, CTA is known for its polar nature and strong mechanical properties, derived from its hydrogen bonding and crystalline structure. However, it can be susceptible to hydrolysis in extreme pH conditions.27,37 PVC offers excellent mechanical strength and flexibility due to its amorphous nature and stability across a range of pH values.38–40 PVDF and PVDF-HFP are notable for their hydrophobicity, chemical resistance, and thermoplastic characteristics, making them suitable candidates for PIM applications.41–44 A thorough understanding of the interplay between the physicochemical properties of polymer matrix, carriers, and plasticizers is crucial for optimizing membrane performance, and achieving effective and selective metal ion transport and recovery (Table 2).
Table 2 Common polymer matrix used in PIMs
Polymer matrix |
Structure |
Metal ion |
Ref. |
PVC |
|
Vanadium |
45
|
Bismuth |
46
|
CTA |
|
Bismuth |
47
|
Cadmium |
48
|
PVDF |
|
Chromium |
49
|
Lutetium |
50
|
PVDF-HFP |
|
Chromium |
51
|
Cobalt |
52
|
2.2 Carriers
Carriers are integral to the functionality of PIMs, playing a pivotal role in the selective transport and separation of metal ions. These substances operate by specifically binding to metal ions in the source solution, thus facilitating their transfer across the membrane. The effectiveness of carriers is determined by several factors: their selectivity and affinity for certain metal ions, solubility in the chosen solvents, and compatibility with both the polymer matrix and the plasticizer within the PIM. The selection of carrier depends on several criteria, including the specific metal ion targeted for transport, the pH levels of both the source and receiving solutions, and the concentration of metal ions in the source solution. Carriers are categorized based on their chemical properties into acidic and chelating, basic, neutral or solvating, and macrocyclic or macromolecular types as illustrated in Fig. 1.28,53
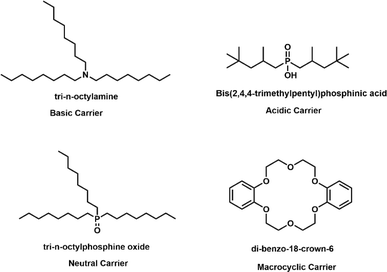 |
| Fig. 1 Chemical structures of basic, acidic, neutral and macrocyclic carriers. | |
2.2.1 Basic carriers.
Basic carriers, which are positively charged, interact selectively with anions in the source solution to form ion pairs. This interaction, driven by the carrier's positively charged groups, facilitates the transport of these anions across the membrane, which is crucial for the selective separation and recovery of target ions. These carriers often contain nitrogen and phosphorus atoms, including quaternary amines, phosphonium compounds, tertiary amines, and pyridine derivatives.54–59
2.2.2 Acidic carriers.
Acidic or chelating carriers comprise of organophosphoric acids, sulfonic acids, and carboxylic acids. These carriers operate through complexation and ion exchange, where the carrier exchanges a proton with the aqueous phase while simultaneously binding to a metal ion to form a neutral complex. This mechanism allows for the selective transport of metal ions, with the process's efficiency being tunable through pH adjustment.28,60 Examples of such carriers include di-2-ethylhexyl phosphoric acid (D2EHPA), di(2,4,4-trimethylpentyl)dithiophosphinic acid (Cyanex 301), and bis(2,4,4-trimethylpentyl)phosphinic acid (Cyanex 272) (Fig. 2).61–66
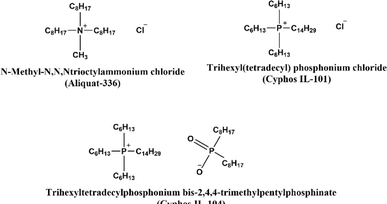 |
| Fig. 2 Examples of commercial ionic liquids used in polymer inclusion membranes. | |
2.2.3 Neutral or solvating carriers.
Neutral or solvating carriers, primarily phosphorus-based, exhibit a particular affinity for actinides and lanthanides. These carriers, including tri-n-butylphosphate (TBP), trioctylphosphine oxide (TOPO), and dibutyl butyl phosphonate (DBBP), function through solvation and complexation rather than ion exchange.27,28,47,67,68 Their high coordinating abilities allow them to form stable complexes with metal ions, while maintaining the non-ionic nature of the organic phase because of their low dielectric constants.69
2.2.4 Macrocyclic or macromolecular carriers.
This category includes carriers with significant crown ether structures, chosen for their exceptional selectivity in complexing with metal ions. These carriers can be structurally adjusted to target specific metal ions and typically exhibit low solubility in aqueous solutions. Despite their promising attributes, it is important to note that many of these carriers are not yet available for commercial application.28,70
2.3 Plasticizers
Plasticizers, when incorporated into PIMs along with the polymer matrix and the carrier, significantly enhance the membranes' flexibility, metal ion permeability, and overall performance. These additives are key to increasing the softness and pliability of the membrane, by diminishing the intermolecular forces within the polymer chains. The primary objective of plasticizer integration into PIMs is to elevate membrane flexibility, reduce brittleness, and thereby improve the membrane's operational efficacy. This improvement allows more efficient diffusion of metal ions across the membrane by minimizing physical hindrances. Furthermore, plasticizers improve the solubility of the carrier or the metal-carrier complex within the membrane matrix.27,28,70 Notable plasticizers used in PIMs include 2-nitrophenyl octyl ether (2-NPOE) and 2-nitrophenyl pentyl ether (2-NPPE).29,71–73 In certain instances, the carrier itself can act as a plasticizer, eliminating the need for additional plasticizers.
3 Ionic liquids
ILs are salts that exist in a liquid state at or close to room temperature, distinct from traditional salts which are typically solid due to their high melting points. ILs have drawn considerable interest for their wide-ranging applications in various scientific domains due to their unique properties.74 These properties include negligible vapor pressure, broad liquidus range, superior thermal stability, and the capability to create tailored solvents via the combinations of different cations and anions. Their inherent viscosity further aids in their retention within membrane structures, enhancing their operational stability.75–78 Consequently, ILs have emerged as promising alternatives to conventional organic solvents in processes such as organic synthesis and catalysis, primarily due to their negligible vapor pressure compared to conventional organic solvents.79–81 The tunable nature of ILs, achieved by varying their cation and anion combinations, allows for the design of ILs with specific solubility and interaction properties, making them highly valuable in separation science and technology, including the liquid–liquid extraction of metal ions.77 Beyond extraction, ILs have shown promise as selective carriers in membrane-based separation, exploring new avenues in metal ion extraction methodologies. This innovative use of ILs in both extraction and membrane separation is set to revolutionize traditional techniques, offering sustainable solutions for metal recovery from industrial waste, electronic waste recycling, and hydrometallurgy, thereby positioning ILs at the forefront of advancements in extraction and separation technologies.
3.1 Ionic liquid-based PIM for extraction of metals
The exploration of ILs as carriers within PIMs has shown significant promise. ILs are characterized by their negligible vapor pressure, ensuring stable retention within the membrane pores. Coupled with their chemical and thermal stability, as well as the adaptability to tailor ILs for specific separation tasks, they offer significant advantages for diverse separation processes. When integrated into PIMs, ILs not only improve the membrane's selectivity and permeability but also enhance its overall stability and longevity. This synergy between ILs and PIMs paves the way for developing efficient and environmentally friendly advanced separation processes. The selection of an appropriate IL is crucial, influencing the membrane's affinity for specific ions. ILs can serve dual roles as solvent and carrier within the PIM, enabling the transport of targeted species across the membrane. Their capacity to dissolve various substances combined with their low volatility minimizes the risk of solvent loss, thereby contributing to the safety and sustainability of the process. The following section explores successful applications of IL-based PIMs in metal ion extraction.
3.1.1 Separation of transition metals.
IL-based PIMs are particularly effective in the selective separation of metal ions from aqueous solutions. In the battery industry, cobalt recovery is crucial due to its limited and uneven global distribution. Research has shown that IL-PIMs can efficiently recover cobalt. Pospiech's study focused on the selective transport of Co(II) ions from mixed aqueous chloride solutions containing Ni(II) and Li(I). They optimized a PIM consisting of 25% CTA as the polymer matrix, 40% NPOE as the plasticizing agent, and 35% trihexyl(tetradecyl)phosphonium thiosalicylate ([PR4][TS]) as the IL carrier. This PIM achieved over 90% recovery of Co(II) ions, transferring from a hydrochloric acid solution (HCl) to a sulfuric acid solution (H2SO4). The efficiency of this PIM system in terms of transport and separation was significantly affected by both the concentration of the IL carrier within the membrane and the acidity levels in both the feed and receiving solutions. Increasing the carrier concentration and the acidity of the feed solution improved separation performance, while higher acidity in the receiving phase reduced its efficiency. The system's stability was tested over three transport cycles, showing a gradual decline in Co(II) separation efficiency due to the leaching of the IL carrier.82
In another study by Baczyńska et al., PIMs incorporating phosphonium-based ILs (Cyphos IL 101 or Cyphos IL 104) as carriers, NPOE as the plasticizer, and CTA as the polymer matrix were used to separate Zn(II) from Fe(III) in chloride solutions. The study highlighted that the choice of the receiving phase significantly influences the transport efficiency and selectivity of metal ions. A 1 M H2SO4 solution effectively received both Zn(II) and Fe(III), whereas a 1 M HCl solution preferentially transported Fe(III), leaving most Zn(II) in the feed source. The highest selectivity coefficient for Fe(III) over Zn(II) was achieved with 1 M HCl as the receiving solution.83
The recovery of chromium from industrial and end-of-life products is critical due to its environmental and health hazards, including toxicity and carcinogenicity. Lin Guo et al., developed a PIM system with PVDF as the polymer matrix and various ILs as both carrier and plasticizer. The system utilized trihexyl(tetradecyl)phosphonium bis(2,4,4-trimethylpentyl)phosphinate as the carrier, alongside imidazolium-based ILs ([Cnmim][PF6] or [BF4], where n = 4, 8) as plasticizers. Imidazolium ILs enhanced the effective contact area of the membrane, thereby quadrupling the permeability compared to membranes without IL plasticizers. This improvement highlights ILs as promising alternatives to traditional molecular plasticizers for developing PIM systems with improved reusability and flux capabilities, especially for Cr(VI) ion transport, influenced by the specific cation and anion combination of the imidazolium ILs.84
Qin et al. demonstrated the high selectivity of PIMs employing electrodialysis-assisted PIM system (PIMED) for the separation of V(V) and Mo(VI) from polymetallic solutions containing Co(II), Ni(II), Mn(II), and Al(III). The PIMED process, which applies an electric field to enhance ion transport, utilized a membrane consisting of 40% PVDF-HFP and 60% Aliquat 336, with sulfuric acid as the stripping solution and a current density of 20 mA cm−2. The results showed no transport of Co(II), Ni(II), Mn(II), and Al(III), indicating highly selectivity. Mo(VI) transported more rapidly than V(V), with extraction and stripping efficiencies of 96% and 37%, respectively. V(V), had extraction and stripping efficiencies of 40% and 33%, respectively. Increased sulfuric acid concentrations improved Mo(VI) transport but hindered V(V) transport. With 1.0 M sulfuric acid stripping solution, while maintaining other conditions, Mo(VI) extraction efficiency remained above 95% and stripping efficiency improved to 78%, while V(V) efficiencies fell below 20%. These results demonstrate that electrodialysis can enhance PIM selectivity and permeability, achieving high separation efficiency for metal ions with similar physicochemical properties.35
3.1.2 Rare earth and critical metal-ion separation.
The chemical and physical similarities among rare earth elements (REEs) make their separation challenging. These elements are vital for various high-tech applications, and their recovery from waste materials has become increasingly important due to potential supply risks. ILs are instrumental in developing new and efficient carriers for the separation of REEs.85–87 In their study on the selective separation of ytterbium (Yb) and lutetium (Lu) ions, Wang et al., employed tetradecyl-(trihexyl)phosphonium bis-(2,4,4-trimethylpentyl)phosphinate, as the carrier within a PIM and ethylenediamine tetraacetic acid (EDTA) as the stripping phase. The PIM showed preferential permeability for Yb(III) over Lu(III), with permeability coefficients of 156 and 114.82 μm s−1, respectively. This differential permeation resulted in a separation factor of 1.37, favoring Yb(III) over Lu(III).88
In another study focused on the extraction and recovery of technologically critical metals, specifically bismuth (Bi), Meziani et al. employed a PIM incorporating 30% CTA for the polymer matrix, 25% IL (a combination of tricaprylmethylammonium chloride (Aliquat 336) and tetradecyl(trihexyl)phosphonium chloride (THTDPCl)) as the carrier, and 45% NPOE as the plasticizer. This PIM enabled the complete transfer of Bi(III) ions from a 1 M HCl solution to a 0.5 M HNO3 solution within 24 hours. The comparative efficacy of Aliquat 336 and THTDPCl in mediating Bi(III) transport was attributed to the shorter alkyl chain of Aliquat 336, which induces a stronger steric hindrance effect compared to the longer alkyl chain of THTDPCl. Stability assessments of the membranes indicated that THTDPCl-incorporated PIMs offered enhanced durability, with potential for up to seven cycles of reuse, owing to its longer hydrophobic alkyl chain that effectively minimizes carrier leakage.89
3.1.3 PGM and precious metal separation.
Platinum Group Metals (PGMs), comprising platinum (Pt), palladium (Pd), rhodium (Rh), iridium (Ir), osmium (Os), and ruthenium (Ru), are precious metals known for their exceptional chemical stability, high melting points, and excellent catalytic properties. These attributes make PGMs indispensable in various industrial, technological, and environmental applications. Efficient recovery of PGMs is essential for resource conservation. Fajar et al., explored the selective separation of Pd(II) and Rh(III) ions in chloride solutions utilizing a PIM composed of 50 wt% PVDF-co-HFP, 40 wt% trioctyl(dodecyl)phosphonium chloride (P88812Cl), and 10 wt% 2NPOE. Their findings demonstrated that Pd(II) could be selectively transported over Rh(III) from solutions with concentrations exceeding 3 M HCl. The effect of the thiourea as the receiving solution was examined, revealing that an optimal 1
:
4 molar ratio of Pd(II) thiourea is necessary for efficient Pd(II) recovery. Insufficient thiourea resulted in suboptimal Pd(II) recovery, while excess thiourea risked contaminating the feed solution.90
In a further investigation on the sequential extraction of Pt(IV), Pd(II), and Rh(III) from spent automotive catalyst leachates, Fajar et al. employed two types of PIMs: isotropic dense (ID-PIM) and anisotropic porous (AP-PIM), both incorporating P88812Cl. The transport of each metal ion was optimized using tailored receiving solutions: 0.1 M NaClO4 in 1 M HCl for Pt(IV), a mixture of 10 mM thiourea and 0.1 M potassium thiocyanate (KSCN) in 1 M HCl for Pd(II), and 4.9 M ammonium chloride (NH4Cl) in 0.1 M HCl for Rh(III). The study demonstrated that both PIMs facilitated over 90% recovery of Pt and Pd with high purity. Although ID-PIM was less effective in transporting Rh(III), AP-PIM exhibited superior efficiency, particularly at elevated temperatures, showcasing a higher recovery rate for Rh(III). Additionally, the membranes demonstrated exceptional durability, retaining their structural integrity and functionality without degradation or loss of carrier molecules after 10 days in harsh chemical environments.91
In a separate study, Pospiech employed a PIM with the thiol-containing task-specific IL tricaprylmethylammonium thiosalicylate ([A336][TS]). The PIM, comprising 40 wt% CTA, 38 wt% ONPOE, and 22 wt% [A336][TS] as the carrier, was used to recover Pd(II) from hydrochloric acid solutions into 0.3 M thiourea in 0.1 M HCl as the receiving solution. The study identified this solution as the most effective stripping phase for Pd(II) transport from the [A336][TS]-containing membrane. The separation from mixed solutions containing Pt(IV), Fe(III), Ni(II), and Mn(II) demonstrated that preferential transport of Pd(II) over these ions. The PIM showed good stability with only a slight decline in initial flux over four cycles. These findings illustrate the potential of task-specific ILs as effective carriers for the separation and recovery of metal ions.92 Hoque et al. investigated the recovery of gold from e-waste using cross-linked PIMs (CL-PIMs). The suitability of polymer matrixes such as CTA, PVC, and PVDF-HFP with cross-linking agents like poly(ethylene glycol) dimethylacrylate (PEGDMA), poly(ethylene glycol) divinyl ether (PEGDVE), triarylsulfonium hexafluorophosphate (TASHFP), and N-ethylmaleimide (NEM) was examined. The study demonstrated that CL-PIMs composed of Cyphos® IL 104 transported Au(III) from 2.5 M HCl solutions twice as fast as their non-cross-linked counterparts, showing excellent stability over five successive transport experiments. The CL-PIM that exhibited good transport efficiency in aqua regia achieved 92% Au(III) transport. The CL-PIM was employed for the recovery of Au(III) from gold-coated e-waste digested in aqua regia. The resulting e-waste digest was used as the feed solution in a transport experiment with 0.5 M Na2SO3 as the receiving solution. ICP-OES analysis revealed that the digest contained Al, Cr, Cu, Fe, Ni, and Zn at concentrations 12 to 825 times higher than that of Au. The recovery experiment results indicated that 94% of Au(III) was transported to the receiving solution in less than 24 hours, while recoveries for other metals were below 4%, despite their higher concentrations.93
4 Advancement in PIMs
4.1 Optimization of PIM performance
The optimization of PIMs for selective separation of metals hinges on a thorough understanding and precise control of several key operational factors. These factors are pivotal in efficiency, selectivity, and stability of PIMs in metal ion separation. Below, we delve into the primary operational variables crucial for the optimal transport of metal ions.
4.1.1 Carrier concentration.
The efficacy of metal ion transport across PIMs is intrinsically linked to the concentration of carrier molecules embedded within the membrane. Increasing carrier concentration generally enhances the interaction between the carriers and the metal ions, thereby improving membrane permeability and overall flux. This relationship is underscored by various studies, such as those by Wang et al., who investigated Zn(II) ion transport through a membrane composed of 30 wt% D2EHPA, 30 wt% NPOE, and 40 wt% PVC, which exhibited a 20% efficiency over a 24 hours period. By adjusting the membrane composition 55 wt% D2EHPA and eliminating NPOE, the Zn(II) transport efficiency significantly enhances to 91.5%.94 Further investigation into the optimal concentration of [A336][SCN] using PVDF as the polymer matrix with 10 wt% NPOE revealed that increasing carrier concentration from 10 wt% to 60 wt% optimized Au(I) ion transport, with peak extraction and recovery rate observed at 40 wt%. However, concentrations above 40 wt% diminished efficiency due to increased membrane viscosity and fragility.34 Therefore, while higher carrier concentration can elevate transport efficiency, they may compromise membrane durability, necessitating a balance between carrier concentration and polymer matrix integrity to maintain stability and minimize fragility.
4.1.2 Membrane thickness.
The thickness of the membrane affects transport kinetics by balancing efficiency and mechanical robustness. Thicker membranes offer enhanced strength but can reduce transport flux and permeability due to the extended diffusion path required for metal ions. Conversely, thinner membranes shorten the diffusion path, improving transport flux and recovery rates but potentially compromising physical durability. Optimal membrane thickness is crucial for maximizing PIM performance while ensuring membrane longevity. Experimental studies, such as those by Yoshida et al. on transport rate of Sc(III), have demonstrated that PIMS composed of 40 wt% D2EHAF, 30 wt% CTA, and 30 wt% 2NPOE (with thickness ranging from 23 ± 5 to 54 ± 4 μm) exhibit an inverse relationship between membrane thickness and initial flux, indicating reduced ion transport efficiency with increase thickness.95 Similarly, Adigun et al. confirmed these findings with membranes of thickness ranging from 45 ± 8 to 104 ± 2 μm, showing a decline in transport efficiency as membrane thickness increased.96 Therefore, careful optimization of membrane thickness is necessary, considering the specific application and operational conditions for PIM use.
4.1.3 Acidity.
The pH level significantly influences metal ion transport through PIMs by affecting the ion concentration gradient and the complexation process with carriers. Tailoring the pH can enhance transport efficiency, as certain pH levels favor the selective the transport of specific metal ions. The acidity of the feed phase, especially with the addition of HCl, plays a vital role in facilitating ion transport by altering the ions' charge and solubility, aiding their complexation within the PIM. Research by Pospiech demonstrated that increasing HCl concentration in the feed solution can enhance permeability for Cd(II) and Co(II) ions, while Ni(II) ion transport remains minimal. The selectivity between Cd(II) and Co(II) decreases at higher HCl concentrations due to the formation of stable anionic chlorocomplexes.97 Yıldız et al. also explored the influence of pH on cobalt(II) ion transport in PIMs, finding that Co(II) ions, which convert into anionic species at pH 4, are more readily absorbed by the membrane, showing a higher affinity for Co(II) than Ni(II). This is attributed to the ion exchange characteristics of the quaternary alkyl ammonium salt in the membrane. Additionally, the study noted a decrease in cobalt ion transport speed with reduced pH differences, emphasizing the critical role of pH in driving the extraction and release of cobalt ions.29 Optimizing the feed phase's acidity and pH are essential for improving the selectivity and efficiency of PIMs in metal ion separation processes.
In PIM research, optimizing various parameters is crucial for efficient transport of metal ions. Therefore, balancing these factors is important for achieving optimal PIM performance.
4.2 Transport mechanism
Understanding the transport mechanisms and models for PIMs is crucial for evaluating their efficiency in selective separation processes (Fig. 3). The primary transport mechanism for metal ions across PIMs is similar to that observed in SLMs, is known as the carrier-mediated diffusion mechanism. In this process, metal ions transfer from the feed phase to the permeate phase through the membrane. Initially, metal ions in the source solution bind with the carrier molecules at the source solution-membrane interface, forming a carrier-metal ion complex. This complex then diffuses across the membrane and, upon reaching the permeate phase-receiving solution interface, releases the metal ions into the receiving solution through ion exchange process.28,98 The transport of metal ions through a membrane can occur via two main mechanisms: co-transport and counter-transport. In co-transport, metal ions and their associated anions form complexes with neutral carriers, forming metal-carrier complexes that diffuse together through the membrane. This mechanism can be represented by eqn (1). | Mn+ + nY− + L MYnL(membrane) | (1) |
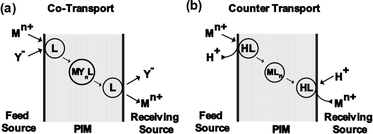 |
| Fig. 3 Transport mechanism of metal ions across membranes (a) co-transport mechanism (b) counter transport mechanism. | |
In the counter-transport mechanism, metal ions form complexes with organic carriers, and these complexes diffuse through the membrane by exchanging cations or anions with the carrier. For example, with acidic carriers, protons are exchanged with metal ions, as shown in eqn (2).99,100
| Mn+ + nHL MLn(membrane) + nH+ | (2) |
The solution-diffusion model, which follows Fick's law of diffusion, is frequently applied to model the transport process within PIMs. According to this model, diffusion of metal ions across the membrane is driven by the concentration gradient between the feed and receiving sides. The transport behaviors are described by Fick's law of diffusion, represented as:
|  | (3) |
where
D is the membrane diffusion coefficient of the metal ion,

is the concentration gradient and
J is the flux. Despite the widespread application of this model, it does not adequately account for the role of carriers in facilitating transport across PIMs. Various studies have shown that increasing the concentration of the carrier significantly enhances the permeability and the transport efficiency.
94,101
4.3 Characterization of PIM
A detailed characterization of PIMs is essential for comprehending their physicochemical properties, transport behaviours, and overall efficacy. Utilizing a comprehensive suite of analytical techniques such as Fourier Transform Infrared (FTIR) spectroscopy, X-ray Diffraction (XRD), Energy-Dispersive X-ray Spectroscopy (EDX), Scanning Electron Microscopy (SEM), Atomic Force Microscopy (AFM), Differential Scanning Calorimetry (DSC), and contact angle measurements enables researchers to thoroughly analyze the structural integrity, chemical composition, and functional capabilities of PIMs. Techniques like SEM and AFM are instrumental in providing intricate details on membrane morphology, highlighting the distribution and homogeneity of carriers and plasticizers within the membrane. For instance, Kazemi et al. employed SEM and AFM to investigate the surface morphology of PIMs compared to pure PVC films, noting that PVC films exhibited smooth surfaces while PIMs displayed wrinkled textures (Fig. 4 and 5). Cross-sectional views identified small droplets and channel-like formations within the PIMs. AFM data confirmed these observations, with the PVC films showing a surface roughness of 0.7 nm and the PIM exhibiting a roughness of 1.6 nm, attributed to the presence of carriers.46
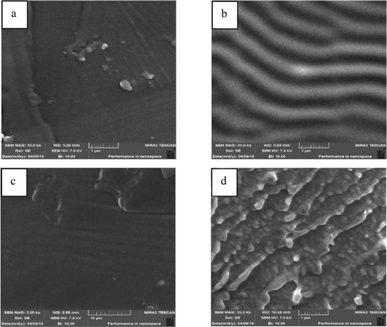 |
| Fig. 4 SEM images of the surface and the cross section of the PVC film (a and c), and the 50% D2EHPA PIM (b and d). Reprinted with permission from Kazemi et al. (2021).46 Copyright 2021 Elsevier. | |
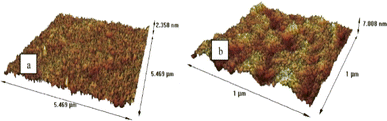 |
| Fig. 5 AFM images of (a) pure PVC film and (b) PIM. Reused with permission from Kazemi et al. (2021).46 Copyright 2021 Elsevier. | |
The mechanical robustness and thermal stability of PIMs are critical for their application across diverse conditions, influencing their operational lifespan and effectiveness. Nasser et al. demonstrated that both CTA blank and CTA-PIM membranes possess enhanced mechanical properties over PVC-based PIMs by measuring the Young's Modulus (Fig. 6). The superior tensile strength of the blank CTA membrane, compared to the CTA-PIM, is ascribed to the Aliquat 336 inclusion, which diminishes tensile strength while augmenting elasticity. This inclusion also leads to a reduced Young's modulus. TGA (Fig. 7) revealed that PIMs containing Aliquat 336 begin to degrade at lower temperatures due to the volatilization of Aliquat 336 and BEHS, leading to notable weight loss.102
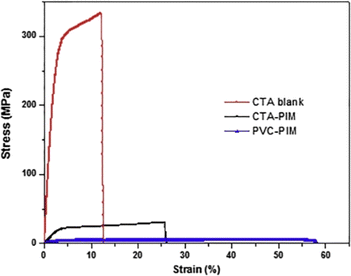 |
| Fig. 6 Stress strain-curve of blank CTA, CTA based PIM, and PVC based PIM. Reused with permission from Nasser et al. (2016).102 Copyright 2016 Elsevier. | |
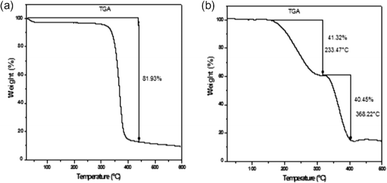 |
| Fig. 7 Thermograms of: (a) CTA and (b) PIM. Nasser et al. (2016).102 Copyright 2016 Elsevier. | |
FTIR spectroscopy has been pivotal in identifying the chemical composition, functional groups, and interaction within PIMs. Cai et al. utilized FTIR spectroscopy to confirm the presence of distinct components in PIMs (Fig. 8). Their study analyzed FT-IR spectra for a CTA blank membrane, thenoyltrifluoroacetone (TTA), TOPO, and a composite PIM containing CTA, TTA, and TOPO, focusing on identifying specific vibrational bands corresponding to functional groups. The persistence of characteristic bands of CTA, TTA, and TOPO within the PIM, without any observed band displacement, indicates that these carrier molecules are effectively encapsulated within the polymer matrix without undergoing chemical modifications, allowing them to interact freely with ions in the solution.68
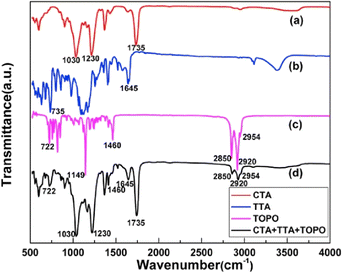 |
| Fig. 8 FT-IR spectra of the (a) CTA blank membrane, (b) TTA, (c) TOPO, and (d) PIM respectively. Cai et al. (2019).68 Copyright 2019 Elsevier. | |
4.4 Stability of PIM
The stability of PIMs is crucial for their efficacy in various separation processes, particularly for long-term use and industrial-scale operations. PIMs must exhibit both chemical and physical stability for effective application. Chemical stability refers to the membrane's ability to retain the carrier within the polymer matrix and minimize leaching. It is essential to carefully assess the physical and chemical characteristics of each component during the design of PIMs. While plasticizers can enhance flexibility and processability, they may also reduce the membrane's tensile strength, leading to increased susceptibility to physical damage during handling and operation.
Chemical degradation of the polymer matrix can occur due to interactions with the feed solution, particularly under harsh chemical environments. Exposure to acidic or basic conditions, oxidative agents, and certain solvents can degrade the polymer structure, leading to membrane deterioration and reduced performance. Keskin et al., investigated the stability of PIMs by evaluating weight loss under acidic (1 M HNO3) and basic (0.05 M NaOH) conditions. They recorded the average mass percentage lost after exposing PIMs to these solutions, finding that degradation was more pronounced in basic conditions. This was attributed to the interaction between hydroxide ions and the positively charged carrier, Aliquat 336, which leads to carrier leaching and membrane degradation. PIMs showed greater stability in acidic environments due to reduced interaction between the cationic carrier and hydronium ions, thereby minimizing carrier leaching. Among the polymers tested, membranes with CTA demonstrated the highest stability, while PVDF membrane showed the least stability, owing to differences in chemical bonding and susceptibility to hydrolysis and degradation.103
Leaching of the carrier and plasticizer into the aqueous phase is a significant concern as it can diminish membrane performance over time. This leaching often results from the interaction between membrane components and the feed or receiving solutions, particularly when there is a strong affinity between the carrier/plasticizer and the solute. Addressing leachability involves optimizing the membrane's composition and structural integrity. Yoshida et al. examined how the structural attributes of carriers affect the resilience of PIMs during the extraction of Sc(III) from sulfuric acid/ammonium sulfate buffer solutions. They compared membranes utilizing two different carriers: aminocarbonyl[methyl]glycine (D2EHAG) and D2EHAF, the latter incorporating a phenyl group. Their findings demonstrated that PIMs featuring D2EHAF exhibited superior stability and maintained higher extraction efficiency over five cycles compared to D2EHAG containing membranes. This enhanced stability of D2EHAF-containing PIMs was attributed to the hydrophobic and steric effects of the phenyl group, which favored better carrier retention within the membrane.95 To mitigate leachability, improvements in membrane composition and structure are essential for long-term functionality and minimize adverse impacts. More chemically resistant polymers, such as PVDF-HFP, have been employed to enhance chemical stability in harsh chemical environments and reduce degradation of membranes.49,51 Additionally, cross-linking the polymer matrix or using polymer blends can improve mechanical and thermal stability.104–106 Recent research on PIMs has focused primarily on their applications and stability, with limited attention to their regeneration. Huang et al. developed a method for regenerating used PIMs, specifically those made with P227 and PVDF. Their process involves weighing and cutting the used membranes into small pieces, soaking them in a 3 M HCl solution to eliminate any P227-Lu(III) complexes, and then dissolving the fragments in DMAC. The regenerated casting solution is analyzed using TGA instrument to determine the proportions of P227 and PVDF, which are then adjected to restore the original composition. The regenerated PIMs are then prepared following the standard method. The success of this regeneration process relies on the non-overlapping weight loss temperature ranges of the components in P227-PVDF PIMs. SEM imaging and ATR-FTIR spectroscopy were used to compare the surface morphology and chemical composition of the regenerated vs. standard PIMs, showing minimal differences. Additionally, the transport efficiency of Lu(III) across regenerated and standard PIMs was comparable.50
5 Conclusions and future outlook
This review provides a comprehensive overview of the evolution of PIM membrane technology, with a particular focus on IL-PIMs for metal ion separation presenting a significant advancement in membrane-based separation technology. It explores the unique properties of ILs, such as selectivity, non-volatility, thermal stability, and tunability, which enhances selectivity and efficiency of PIMs in metal ion separation. Additionally, it provides insights into PIM components, optimization parameters, and applications in metal recycling, emphasizing role of IL-PIM in promoting sustainable and efficient metal separation processes. IL-PIMs exhibit significant potential across various industrial applications due to their high selectivity, efficiency, and minimal environmental impact, making them a transformative technology for sustainable industrial processes. The following sections highlight the potential challenges that PIM technology can address and the possible industrial applications of IL-based PIMs for metal separation:
(1) Battery recycling: IL-PIMs are gaining attention for their role in the recycling of lithium-ion batteries (LIBs). These batteries contain valuable metals such as cobalt, nickel, and lithium, which are essential for manufacturing new batteries and electronic devices. Recovering these metals from spent batteries is crucial for metal sustainability and mitigating environmental concerns associated with the increasing demand for batteries related product.52,96,107
(2) Wastewater treatment: IL-PIMs provide an innovative solution for treating industrial wastewater, particularly in removing heavy metals such as lead, cadmium, and mercury. This application is vital for industries such as mining, metal plating, and electronics manufacturing, where wastewater often contains high concentrations of toxic metals.36,43,108
(3) Environmental remediation: IL-PIMs are also being explored for environmental remediation, specifically for removing dyes, heavy metal pollution, and pharmaceutical contaminants, which pose significant environmental and health risks.109–112
While the applicability of IL-PIMs for metal ion extraction and recovery has seen considerable advancements, there remains a vast potential for further exploration and innovation. Key areas for future research include:
(1) Enhanced selectivity: developing ILs with higher specificity for certain metal ions like rare earth metals, which are critical and economically valuable or environmentally hazardous. This could involve synthesizing novel ILs or modifying existing ones to improve their selectivity and binding affinity for target ions.
(2) Eco-friendly ILs: given the increasing concern over environmental safety, the development of eco-friendly ILs that maintain high efficiency in metal ion extraction is crucial. Research could explore naturally derived ILs or those that can be easily broken down into non-toxic components.
(3) Modelling and mechanistic studies: existing diffusion models, based on Fick's law, inadequately capture the carrier's role in metal ion transport within IL-PIM systems. Enhancing these models to reflect the carrier's impact more accurately could improve predictions of the transport process. Future research should aim at creating detailed models to anticipate membrane behaviours under various conditions, leveraging advanced simulations to understand the interactions between ILs, polymers, and metal ions.
(4) Minimization of leachability: efforts to minimize leachability in PIMs hinge on optimizing their composition and architecture. This involves employing chemically resistant polymers, synthesizing novel hydrophobic carriers, and designing membranes with superior entrapment mechanisms. Surface modification techniques that establish barrier layers or alter surface properties can also effectively reduce leachability.
(5) Scalability and process optimization: many IL-based PIM processes are still at the laboratory or pilot scale. The long-term performance and durability of IL-based PIMs under industrial conditions are critical for their adoption. Ensuring the mechanical and chemical stability of the ILs and the polymer matrix is crucial to improving the durability of IL-based PIMs in continuous operation and their application for industrial use. Future research should address scalability, including the design of scalable membrane fabrication techniques for the integration of IL-based PIMs into industrial setups. The scalability of IL-based PIMs from laboratory to industrial applications presents several significant challenges. Addressing these issues is critical for the successful industrial deployment of this promising technology. Continued research, innovation, and collaboration between academia and industry will be essential to overcome these hurdles and unlock the full potential of IL-PIMs for sustainable industrial processes.
Abbreviations
SLMs | Supported liquid membranes |
BLM | Bulk liquid membranes |
ELM | Emulsion liquid membranes |
PIMs | Polymer inclusion membranes |
SX | Solvent extraction |
ILs | Ionic liquids |
PVC | Poly(vinyl chloride) |
CTA | Cellulose triacetate |
PVDF-HFP | Poly(vinylidene fluoride-co-hexafluoropropylene) |
PVDF | Poly(vinylidene fluoride) |
Cyphos IL 101 | Trihexyl(tetradecyl)phosphonium chloride |
Cyanex 301 | Di(2,4,4-trimethylpentyl)dithiophosphinic acid |
Cyanex 272 | Bis(2,4,4-trimethylpentyl)phosphinic acid |
2-NPPE | 2-Nitrophenyl pentyl ether |
2-NPOE | 2-Nitrophenyl octyl ether ONPOE o-nitrophenyl octyl ether |
TBP | Tributylphosphate |
TOPO | Trioctylphosphine oxide |
DBBP | Dibutyl butyl phosphonate |
D2EHPA | Bis(2-ethylhexyl) phosphate |
[PR4][TS] | Trihexyl(tetradecyl)phosphonium thiosalicylate |
EDTA | Ethylenediamine tetraacetic acid |
Cyphos IL 104 | Trihexyl(tetradecyl)phosphonium bis(2,4,4-trimethylpentyl)phosphinate |
Aliquat 336 | Tricaprylmethylammonium chloride |
P66614Cl | Tetradecyl(trihexyl)phosphonium chloride |
P88812Cl | Trioctyl(dodecyl)phosphonium chloride |
[A336][SCN] | Methyl trioctyl ammonium thiocyanate |
D2EHAG | Aminocarbonyl[methyl]glycine |
D2EHAF |
N-[N,N-Di(2-ethylhexyl)aminocarbonylmethyl]phenylalanine |
BEHS | Bis(2-ethylhexyl)sebacate |
P227 | Di(2-ethylhexyl)phosphinic acid |
[A336][TS] | Tricaprylmethylammonium thiosalicylate |
Data availability
No primary research results, software or code have been included and no new data were generated or analysed as part of this review.
Conflicts of interest
There are no conflicts to declare.
Acknowledgements
This research was performed through the Re-Cell Center, which gratefully acknowledges support from the U. S. Department of Energy (DOE), Office of Energy Efficiency and Renewable Energy, and the Vehicle Technologies Office. This manuscript has been authored in part by UT-Battelle, LLC, under contract DE-AC05-00OR22725 with the US Department of Energy (DOE). The US government retains and the publisher, by accepting the article for publication, acknowledges that the US government retains a nonexclusive, paid-up, irrevocable, worldwide license to publish or reproduce the published form of this manuscript, or allow others to do so, for US government purposes. DOE will provide public access to these results of federally sponsored research in accordance with the DOE Public Access Plan (http://energy.gov/downloads/doe-public-access-plan).
References
- S. Zhang, Y. Ding, B. Liu and C.-c. Chang, Waste Manage., 2017, 65, 113–127 CrossRef.
- M. Henckens, P. Driessen and E. Worrell, Resour., Conserv. Recycl., 2014, 93, 1–8 CrossRef.
- R. M. Izatt, S. R. Izatt, R. L. Bruening, N. E. Izatt and B. A. Moyer, Chem. Soc. Rev., 2014, 43, 2451–2475 RSC.
- S. H. Ali, D. Giurco, N. Arndt, E. Nickless, G. Brown, A. Demetriades, R. Durrheim, M. A. Enriquez, J. Kinnaird, A. Littleboy, L. D. Meinert, R. Oberhänsli, J. Salem, R. Schodde, G. Schneider, O. Vidal and N. Yakovleva, Nature, 2017, 543, 367–372 CrossRef CAS.
- B. K. Reck and T. E. Graedel, Science, 2012, 337, 690–695 CrossRef CAS PubMed.
- R. U. Ayres, Resour., Conserv. Recycl., 1997, 21, 145–173 CrossRef.
- X. Q. Sun, H. M. Luo and S. Dai, Talanta, 2012, 90, 132–137 CrossRef CAS PubMed.
-
S. Hussaini and A. M. Tita, in Recycling Technologies for Secondary Zn-Pb Resources, Springer, 2023, pp. 403–459 Search PubMed.
- S. Gupta and B. Babu, Chem. Eng. J., 2009, 150, 352–365 CrossRef CAS.
- A. B. Botelho Junior, D. B. Dreisinger and D. C. Espinosa, Min., Metall., Explor., 2019, 36, 199–213 Search PubMed.
- G. Crini and E. Lichtfouse, Environ. Chem. Lett., 2019, 17, 145–155 CrossRef CAS.
- A. Yιlmaz, A. Kaya, H. Alpoguz, M. Ersoz and M. Yilmaz, Sep. Purif. Technol., 2008, 59, 1–8 CrossRef.
- H. Ma, K. E. Waters and H. Wang, Min., Metall., Explor., 2023, 40, 13–39 Search PubMed.
- A. Kumar, A. Thakur and P. S. Panesar, Rev. Environ. Sci. Bio/Technol., 2019, 18, 153–182 CrossRef.
- N. Kocherginsky, Q. Yang and L. Seelam, Sep. Purif. Technol., 2007, 53, 171–177 CrossRef CAS.
- M. Amini, A. Rahbar-Kelishami, M. Alipour and O. Vahidi, J. Membr. Sci. Res., 2018, 4, 121–135 CAS.
- A. J. B. Kemperman, D. Bargeman, T. Van Den Boomgaard and H. Strathmann, Sep. Sci. Technol., 1996, 31, 2733–2762 CrossRef CAS.
- W. Yoshida, F. Kubota, Y. Baba, S. D. Kolev and M. Goto, ACS Omega, 2019, 4, 21122–21130 CrossRef CAS PubMed.
- B. Pospiech, W. Walkowiak and M. Wozniak, Physicochem. Probl. Miner. Process., 2005, 39, 89–98 CAS.
- K. D. Clark, M. N. Emaus, M. Varona, A. N. Bowers and J. L. Anderson, J. Sep. Sci., 2018, 41, 209–235 CrossRef CAS PubMed.
- H. Zhao, S. Xia and P. Ma, J. Chem. Technol. Biotechnol., 2005, 80, 1089–1096 CrossRef CAS.
- K. Kuroda, New J. Chem., 2022, 46, 20047–20052 RSC.
- Z. Li, Q. Han, K. Wang, S. Song, Y. Xue, X. Ji, J. Zhai, Y. Huang and S. Zhang, Catal. Rev., 2024, 66, 484–530 CrossRef CAS.
- Z. D. Lei, C. N. Dai, J. Hallett and M. Shiflett, Chem. Rev., 2024, 124, 7533–7535 CrossRef CAS PubMed.
- A. J. Schow, R. T. Peterson and J. D. Lamb, J. Membr. Sci., 1996, 111, 291–295 CrossRef CAS.
- J. Aguilar, M. Sanchez-Castellanos, E. R. de San Miguel and J. De Gyves, J. Membr. Sci., 2001, 190, 107–118 CrossRef CAS.
- M. I. G. Almeida, R. W. Cattrall and S. D. Kolev, J. Membr. Sci., 2012, 415, 9–23 CrossRef.
- L. D. Nghiem, P. Mornane, I. D. Potter, J. M. Perera, R. W. Cattrall and S. D. Kolev, J. Membr. Sci., 2006, 281, 7–41 CrossRef CAS.
- Y. Yıldız, A. Manzak and O. Tutkun, Desalin. Water Treat., 2016, 57, 4616–4623 Search PubMed.
- K. Annane, A. Sahmoune, P. Montels and S. Tingry, Chem. Eng. Res. Des., 2015, 94, 605–610 CrossRef CAS.
- C. Specht, R. W. Cattrall, T. G. Spassov, M. I. Spassova and S. D. Kolev, React. Funct. Polym., 2018, 130, 81–89 CrossRef CAS.
- N. S. Abdul-Halim, P. G. Whitten and L. D. Nghiem, Sep. Sci. Technol., 2016, 51, 1515–1522 CAS.
- Y. Sun, Z. Wang, Y. Wang, M. Liu, S. Li, L. Tang, S. Wang, X. Yang and S. Ji, Chem. Eng. Res. Des., 2020, 153, 136–145 CrossRef CAS.
- Z. Wang, Y. Sun, N. Tang, C. Miao, Y. Wang, L. Tang, S. Wang and X. Yang, Sep. Purif. Technol., 2019, 222, 136–144 CrossRef CAS.
- Z. H. Qin, Y. Z. Wang, L. Sun, Y. X. Gu, Y. Zhao, L. Xia, Y. Liu, B. Van der Bruggen and Y. Zhang, J. Hazard. Mater., 2022, 436, 129315 CrossRef CAS PubMed.
- F. Sellami, O. Kebiche-Senhadji, S. Marais, L. Colasse and K. Fatyeyeva, Sep. Purif. Technol., 2020, 248, 117038 CrossRef CAS.
- N. S. W. Zulkefeli, S. K. Weng and N. S. Abdul Halim, Curr. Pollut. Rep., 2018, 4, 84–92 CrossRef CAS.
- E. I. Oluwasola, A. L. Ahmad and N. F. Shoparwe, J. Water Process Eng., 2023, 52, 103508 CrossRef.
- B. Wang, Y. Wang and T. Xu, Adv. Mater. Technol., 2023, 8, 2300829 CrossRef CAS.
- K. Witt, E. Radzyminska-Lenarcik, A. Kosciuszko, M. Gierszewska and K. Ziuziakowski, Polymers, 2018, 10, 134 CrossRef PubMed.
- H. Cascon and S. Choudhary, Sep. Sci. Technol., 2013, 48, 1616–1626 CrossRef CAS.
- A. Nath and A. Kumar, J. Membr. Sci., 2014, 453, 192–201 CrossRef CAS.
- H. I. Turgut, V. Eyupoglu, R. A. Kumbasar and I. Sisman, Sep. Purif. Technol., 2017, 175, 406–417 CrossRef CAS.
- D. Wang, R. W. Cattrall, J. Li, M. I. G. Almeida, G. W. Stevens and S. D. Kolev, Sep. Purif. Technol., 2018, 202, 59–66 CrossRef CAS.
- S. Bahrami, L. Dolatyari, H. Shayani-Jam and M. R. Yaftian, J. Appl. Polym. Sci., 2022, 139, e52434 CrossRef CAS.
- D. Kazemi, M. R. Yaftian and S. D. Kolev, React. Funct. Polym., 2021, 164, 104935 CrossRef CAS.
- N. Ghaderi, L. Dolatyari, D. Kazemi, H. R. Sharafi, H. Shayani-Jam and M. R. Yaftian, J. Appl. Polym. Sci., 2022, 139, 51480 CrossRef CAS.
- P. Szczepański, H. P. Guo, K. Dzieszkowski, Z. Rafiński, A. Wolan, K. Fatyeyeva, J. Kujawa and W. Kujawski, J. Membr. Sci., 2021, 638, 119674 CrossRef.
- D. Wang, R. W. Cattrall, J. Li, M. I. G. Almeida, G. W. Stevens and S. D. Kolev, J. Membr. Sci., 2017, 542, 272–279 CrossRef CAS.
- S. Huang, J. Chen and D. Zou, J. Rare Earths, 2021, 39, 1256–1263 CrossRef CAS.
- S. Bahrami, M. R. Yaftian, P. Najvak, L. Dolatyari, H. Shayani-Jam and S. D. Kolev, Sep. Purif. Technol., 2020, 250, 117251 CrossRef CAS.
- V. Eyupoglu, A. Unal, E. Polat, B. Eren and R. A. Kumbasar, Sep. Purif. Technol., 2022, 303, 122201 CrossRef CAS.
- B. Keskin, B. Zeytuncu-Gokoglu and I. Koyuncu, Chemosphere, 2021, 279, 130604 CrossRef CAS PubMed.
- A. Nowik-Zajac, C. Kozłowski and W. Walkowiak, Physicochem. Probl. Miner. Process., 2010, 44, 179–186 CAS.
- R. Kumar, A. K. Pandey, A. Tyagi, G. Dey, S. V. Ramagiri, J. R. Bellare and A. Goswami, J. Colloid Interface Sci., 2009, 337, 523–530 CrossRef CAS PubMed.
- B. Wionczyk, W. A. Apostoluk and K. Prochaska, Anal. Chim. Acta, 2001, 428, 89–101 CrossRef CAS.
- J. D. Lamb, A. Y. Nazarenko and H. Tsukube, Anal. Chim. Acta, 1998, 373, 167–173 CrossRef CAS.
- H. Dahdah, F. Sellami, S. Dekkouche, M. Benamor and O. Senhadji-Kebiche, Polym. Bull., 2023, 80, 6495–6525 CrossRef CAS.
- M. Matsumoto, N. Kita and Y. Tahara, Solvent Extr. Res. Dev., Jpn., 2023, 30, 177–181 CrossRef CAS.
- A. Kaya, C. Onac, H. K. Alpoguz, A. Yilmaz and N. Atar, Chem. Eng. J., 2016, 283, 141–149 CrossRef CAS.
- S. P. Best, S. D. Kolev, J. R. P. Gabriel and R. W. Cattrall, J. Membr. Sci., 2016, 497, 377–386 CrossRef CAS.
- A. Yilmaz, G. Arslan, A. Tor and I. Akin, Desalination, 2011, 277, 301–307 CrossRef CAS.
- B. Pospiech, Physicochem. Probl. Miner. Process., 2013, 49, 641–649 CAS.
- B. Pośpiech, Sep. Sci. Technol., 2012, 47, 1413–1419 CrossRef.
- X. Meng, Y. Long, Y. Tian, W. Li, T. Liu and S. Huo, Hydrometallurgy, 2021, 202, 105615 CrossRef CAS.
- H. Arabi, S. Milani, H. Abolghasemi and F. Zahakifar, J. Radioanal. Nucl. Chem., 2021, 327, 653–665 CrossRef CAS.
- M. d. L. Ballinas, E. Rodríguez de San Miguel, M. T. d. J. Rodríguez, O. Silva, M. Muñoz and J. De Gyves, Environ. Sci. Technol., 2004, 38, 886–891 CrossRef PubMed.
- C. Cai, F. Yang, Z. Zhao, Q. Liao, R. Bai, W. Guo, P. Chen, Y. Zhang and H. Zhang, J. Membr. Sci., 2019, 579, 1–10 CrossRef CAS.
- L. Burger, Nucl. Sci. Eng., 1963, 16, 428–439 CrossRef CAS.
- B. Keskin, B. Zeytuncu-Gökoğlu and I. Koyuncu, Chemosphere, 2021, 279, 130604 CrossRef CAS PubMed.
- O. Tutkun and K. Kaparova, Korean J. Chem. Eng., 2022, 39, 1011–1019 CrossRef CAS.
- O. Kebiche-Senhadji, S. Tingry, P. Seta and M. Benamor, Desalination, 2010, 258, 59–65 CrossRef CAS.
- D. Wang, J. Hu, Y. Li, M. Fu, D. Liu and Q. Chen, J. Membr. Sci., 2016, 501, 228–235 CrossRef CAS.
- J. F. Brennecke and E. J. Maginn, AlChE J., 2001, 47, 2384 CrossRef CAS.
- M. L. Dietz, Sep. Sci. Technol., 2006, 41, 2047–2063 CrossRef CAS.
- X. Han and D. W. Armstrong, Acc. Chem. Res., 2007, 40, 1079–1086 CrossRef CAS PubMed.
- X. Q. Sun, H. M. Luo and S. Dai, Chem. Rev., 2012, 112, 2100–2128 CrossRef CAS PubMed.
-
O. Pius Dore and M. Michiaki, in Ionic Liquids, ed. H. Scott, IntechOpen, Rijeka, 2017, ch. 23, DOI:10.5772/65754.
- E. Rynkowska, K. Fatyeyeva and W. Kujawski, Rev. Chem. Eng., 2018, 34, 341–363 CrossRef CAS.
- B. Pospiech and W. Kujawski, Rev. Chem. Eng., 2015, 31, 179–191 CAS.
-
M. Regel-Rosocka and K. Materna, in Ionic Liquids in Separation Technology, Elsevier, 2014, pp. 153–188 Search PubMed.
- B. Pospiech, Physicochem. Probl. Miner. Process., 2022, 58, 152997 CAS.
- M. Baczyńska, Ż. Słomka, M. Rzelewska, M. Waszak, M. Nowicki and M. Regel-Rosocka, J. Chem. Technol. Biotechnol., 2018, 93, 1767–1777 CrossRef.
- L. Guo, Y. H. Liu, C. Zhang and J. Chen, J. Membr. Sci., 2011, 372, 314–321 CrossRef CAS.
- Y. Chen, H. Wang, Y. Pei, J. Ren and J. Wang, ACS Sustain. Chem. Eng., 2015, 3, 3167–3174 CrossRef CAS.
- C.-L. Do-Thanh, H. M. Luo and S. Dai, RSC Sustainability, 2023, 1168–1176 RSC.
- Y. Baba, F. Kubota, N. Kamiya and M. Goto, J. Chem. Eng. Jpn., 2011, 44, 679–685 CrossRef CAS.
- Y. Wang, L. Chen, Y. Yan, J. Chen, J. Dai and X. Dai, J. Membr. Sci., 2020, 610, 118263 CrossRef CAS.
- R. Meziani, L. Mitiche, C. Fontàs and A. Sahmoune, Chem. Eng. Process., 2022, 175, 108911 CrossRef CAS.
- A. T. Fajar, F. Kubota, M. L. Firmansyah and M. Goto, Ind. Eng. Chem. Res., 2019, 58, 22334–22342 CrossRef CAS.
- A. T. Fajar, T. Hanada and M. Goto, J. Membr. Sci., 2021, 629, 119296 CrossRef CAS.
- B. Pospiech, Chem. Pap., 2018, 72, 301–308 CrossRef CAS.
- B. Hoque, S. D. Kolev, R. W. Cattrall, T. G. Gopakumar and M. I. G. Almeida, Waste Manage., 2021, 124, 54–62 CrossRef CAS PubMed.
- D. Wang, J. Hu, D. Liu, Q. Chen and J. Li, J. Membr. Sci., 2017, 524, 205–213 CrossRef CAS.
- W. Yoshida, Y. Baba, F. Kubota, S. D. Kolev and M. Goto, J. Membr. Sci., 2019, 572, 291–299 CrossRef CAS.
- B. Adigun, B. P. Thapaliya, H. M. Luo and S. Dai, RSC Sustainability, 2024, 2, 1859–1867 RSC.
- B. Pospiech, Arch. Metall. Mater., 2015, 2933–2938 CAS.
- M. O'Rourke, R. W. Cattrall, S. D. Kolev and I. D. Potter, Solvent Extr. Res. Dev., Jpn., 2009, 16, 1–12 Search PubMed.
- L. Chen, Y. Wu, H. Dong, M. Meng, C. Li, Y. Yan and J. Chen, Sep. Purif. Technol., 2018, 197, 70–85 CrossRef CAS.
- P. R. Danesi, Sep. Sci. Technol., 1984, 19, 857–894 CrossRef CAS.
- T. Hanada, M. L. Firmansyah, W. Yoshida, F. Kubota, S. D. Kolev and M. Goto, ACS Omega, 2020, 5, 12989–12995 CrossRef CAS PubMed.
- I. I. Nasser, F. I. E. H. Amor, L. Donato, C. Algieri, A. Garofalo, E. Drioli and C. Ahmed, Chem. Eng. J., 2016, 295, 207–217 CrossRef.
- B. Keskin, A. Yuksekdag, B. Zeytuncu and I. Koyuncu, J. Water Process Eng., 2023, 52, 103576 CrossRef.
- F. Nitti, O. T. E. Selan, B. Hoque, D. Tambaru and M. C. Djunaidi, Indones. J. Chem., 2021, 22, 284–302 CrossRef.
- B. Wang, Q. Lang, M. Tan, H. Jiang, L. Wang, Y. Liu and Y. Zhang, AIChE J., 2022, 68, e17397 CrossRef CAS.
- B. Hoque, M. I. G. Almeida, R. W. Cattrall, T. G. Gopakumar and S. D. Kolev, J. Membr. Sci., 2019, 589, 117256 CrossRef.
- B. Wang, F. Liu, F. Zhang, M. Tan, H. Jiang, Y. Liu and Y. Zhang, Chem. Eng. J., 2022, 430, 132924 CrossRef CAS.
- G. Elias, E. Marguí, S. Diez and C. u. Fontàs, Anal. Chem., 2018, 90, 4756–4763 CrossRef CAS PubMed.
- A. C. Sadiq, A. Olasupo, N. Y. Rahim, W. S. W. Ngah, M. A. K. M. Hanafiah and F. B. M. Suah, Int. J. Biol. Macromol., 2023, 244, 125400 CrossRef CAS.
- K. Maiphetlho, K. Netshiongolwe, H. Tutu, L. Chimuka and H. Richards, J. Water Process Eng., 2022, 49, 103061 CrossRef.
- A. Olasupo and F. B. M. Suah, J. Hazard. Mater., 2021, 406, 124317 CrossRef CAS PubMed.
- A. Manzak, Ö. Demirbaş, Y. Yıldız and M. Teker, Water, Air, Soil Pollut., 2024, 235, 501 CrossRef CAS.
|
This journal is © The Royal Society of Chemistry 2024 |
Click here to see how this site uses Cookies. View our privacy policy here.