DOI:
10.1039/D4TB01216J
(Paper)
J. Mater. Chem. B, 2024,
12, 12207-12219
A bioinspired porous and electroactive reduced graphene oxide hydrogel based biosensing platform for efficient detection of tumor necrosis factor-α†
Received
5th June 2024
, Accepted 7th October 2024
First published on 11th October 2024
Abstract
Oral cancer is one of the leading cancer types, which is frequently diagnosed at an advanced stage, giving patients a poor prognosis and fewer therapeutic choices. To address this gap, exploiting biosensors utilizing anti-biofouling hydrogels for early-stage oral cancer detection in non-invasive body fluids is gaining utter importance. Herein, we have demonstrated the fabrication of an innovative electrochemical immunosensor for the rapid, label-free, non-invasive, and affordable detection of tumor necrosis factor-α (TNF-α), a biomarker associated with oral cancer progression in artificial saliva samples. The gold screen-printed electrodes (gSPEs) are modified with a green synthesized porous and electroactive reduced graphene oxide (rGO) hydrogel utilizing L-cystine (L-cys) as both in situ reducing and surface functionalization agent, followed by covalent immobilization of anti-TNF-α and blocking of residual sites with bovine serum albumin (BSA) to fabricate the BSA/anti-TNF-α/L-cys_rGO hydrogel/gSPE immunosensing platform. The fabricated platform demonstrates excellent performance, with a low limit of detection of 1.20 pg mL−1, a broad linear range from 1 to 200 pg mL−1, and a high sensitivity of 2.10 μA pg−1 mL cm−2 carried out with differential pulse voltammetry (DPV) technique. Moreover, it exhibits specificity towards TNF-α, even in the presence of potential interferents and other cancer biomarkers. Besides, the biosensor showed good reproducibility and repeatability with a relative standard deviation (%RSD) of 5.11% and 1.85%, respectively. Thus, integrating the L-cys_rGO hydrogel in the immunosensor design offers enhanced performance, paving the way for its application in early-stage oral cancer diagnosis.
1. Introduction
Cancer poses a significant global health challenge, being a primary contributor to mortality rates and a significant obstacle to the global endeavour of prolonging the human lifespan.1 Among the various types of cancer, oral cancer ranks as the sixth most prevalent form of cancer,2 with approximately 378
000 cases recorded worldwide in 2020.3 It has a strong foothold in countries undergoing significant economic changes.4 Oral cancer usually develops in the buccal cavity and oropharynx, with oral squamous cell carcinoma (OSCC) constituting over 90% of cases of cancers within the head and neck region.5 Factors that contribute to the development of oral cancer encompass various etiological agents, with smoking and alcohol consumption being the foremost risk factors.6 Despite the advancements in diagnostic technologies and easily accessible buccal cavity, a substantial number of oral cancer cases are diagnosed in advanced stages, which leads to a reduction in the chances of effective treatment. Furthermore, the 5-year survival rates for oral cancer cases detected in later stages have been observed at below 30%, while for those detected at an early stage are above 80%, underscoring the critical importance and urgent need for early detection and intervention of oral cancer.7
Although currently there are many diagnostic approaches for oral cancer, such as tumor imaging, biopsy analysis, proteomics analysis, and enzyme-linked immunosorbent assay (ELISA), due to their invasive, time-consuming, painful procedures and highly trained personnel requirements, their usage is restricted.8–10 Moreover, these are often applicable only in later stages of cancer progression, where substantial tumor growth has already occurred. To address this diagnostic gap, detecting biomarker levels indicative of cancer development using biosensors could be a promising alternative.11 Among various oral cancer biomarkers like Interleukin 6 (IL-6),12 IL-8,13 MMP-9,14 CYFRA-21-1,15 and tumor necrosis factor-alpha (TNF-α),16 which play crucial roles in oral cancer progression, TNF-α has gained significant attention in recent times. Furthermore, it is also a considerable biomarker in stress diagnosis, whose increased levels make it a valuable indicator for stress-related diseases. TNF-α is a 17 kDa, trimeric, pleiotropic, pro-inflammatory cytokine with significant involvement in various inflammatory, metabolic, infectious, and neoplastic diseases. TNF-α serves as a critical mediator and plays a central role in initiating immune response.17 It is primarily secreted by activated macrophages but can also be synthesized by other cells, including muscle, fibroblast, and endothelial cells.18 Numerous research studies have highlighted an association between elevated TNF-α levels in body fluids and the advancement of oral cancer.19,20 TNF-α is implicated in promoting the survival and proliferation of cancer cells, facilitating angiogenesis and metastasis.21 The salivary concentration of TNF-α typically measures around 30 pg mL−1 in individuals with oral cancer, compared to approximately 3 pg mL−1 in healthy subjects.22
Nowadays, biosensors have demonstrated an effective route for fast and effective biomarker quantification, facilitating early detection as well as assessment of cancer treatment efficacy.23,24 Among the biosensors, electrochemical biosensors offer an efficient way of biomarker level detection with improved speed, sensitivity, specificity, and economics of the diagnosis system.25,26 One key advantage of the electrochemical biosensor platform is its adaptability, allowing modifications with different transducer materials to improve the characteristics of the sensor.27,28 Over the last decade, extensive research has been directed towards developing novel and innovative transducer materials for electrochemical biosensors, which can uphold good catalytic performance along with enhanced stability.25,27,29,30
Nanostructured graphene-based hydrogels are garnering considerable attention as transducer materials for electrochemical biosensors.31 However, the utility of graphene and its derivatives in hydrogels is hindered by their limited water dispersibility.32 Nevertheless, the reduction of graphene oxide (GO) has been shown to improve this dispersion within a polymeric matrix and enhance performance by providing exciting electrochemical properties and a larger surface area with good functionalization capability. Reduced graphene oxide (rGO) that possesses a hexagonal carbon structure with inherent crystallinity along with various functional groups and defects in the structure has proved to influence the electrical properties, including mass transfer of electrons and electrochemical reactivity, potentially enhancing the biosensor's sensitivity and assay time.33,34 Though, for synthesizing the rGO hydrogel, chemical functionalization of rGO could be done by utilizing widely used compounds like (3-aminopropyl)triethoxysilane (APTES),35 oleic acid, polyethylene glycol, and 3-mercaptopropyltriethoxysilane.36 However, such chemicals require an additional step for functionalization and may affect human health adversely.37,38 To address these issues, biomolecules, such as amino acids that are non-toxic in nature, are emerging for functionalization of nanomaterials and immobilization of preferred biomolecules. L-Cysteine (L-cys) is a natural and easily available proteinogenic amino acid having amino (–NH2), carboxyl (–COOH), and sulfur (–S) groups, which make it a suitable linker for the functionalization of nanomaterials.39,40 Functionalizing the rGO hydrogel with L-cys can provide not only increased loading of the desired antibody molecules but also covalent attachment of the hydrogel to the gold surface, owing to functional groups such as amine (–NH2) and thiol (–SH), which further increase the stability of the biosensing platform.41
In this study, we have demonstrated the green synthesis of an L-cys functionalized rGO hydrogel using a one pot method and further used it for the fabrication of an electrochemical immunosensor to quantify TNF-α biomarker in artificial saliva sample. The porous L-cys functionalized rGO hydrogel provided excellent conductivity along with an enhanced surface-to-volume ratio, improving the overall electrochemical performance. Besides, the bifunctional L-cys molecules not only reduce GO to rGO but simultaneously act as the bridging as well as functionalization agent to facilitate the assembly of rGO sheets into porous nanostructures. A series of techniques, including field electron scanning electron microscopy (FESEM), high-resolution transmission electron microscopy (HRTEM), Fourier transform infrared spectroscopy (FTIR), Brunauer–Emmett Teller (BET) analysis, and X-ray diffraction (XRD), have been utilized to study the morphology, structure, and functionalization of the rGO hydrogels. Leveraging the porosity, anti-biofouling nature, high electrical conductivity, and expansive surface area of the L-cys_rGO hydrogel, the fabricated biosensor demonstrated good sensitivity, high selectivity, broad linearity, and a very low detection limit for TNF-α detection.
2. Experimental section
The sections describing the “Chemicals and reagents” and “Instrumentation and characterization” are fully explained in the ESI† under subsections S1 and S2, respectively.
2.1 Synthesis of the L-cysteine functionalized rGO (L-cys_rGO) hydrogel
The L-cys functionalized rGO hydrogel was synthesized by a simple coprecipitation method utilizing L-cys as both in situ reducing and surface functionalization agent.42 Different ratios of GO
:
L-cys (5
:
1, 1
:
1, and 1
:
5) were used to optimize the process of reduction and synthesis of hydrogel. For preparing the L-cys_rGO hydrogel, a 3 mg mL−1 dispersion of GO was firstly prepared by adding 30 mg of GO to 10 mL of deionized water, followed by bath sonication for around 3 h and transfer to a 15 mL vial. For the reduction of GO along with the simultaneous formation of the hydrogel, L-cys in different amounts (6, 30, and 150 mg in ratios 5
:
1, 1
:
1, and 1
:
5, respectively) was added dropwise to the GO dispersion. The resulting solutions were then kept overnight at 80 °C for hydrogel formation, followed by washing with deionized water and ethanol. Following the completion of hydrogel formation, the hydrogels were washed thrice by centrifugation. Initially, 5 mL of deionized water was added to the hydrogels. Then, they were centrifuged at 4000 rpm for 10 min at room temperature. The supernatant was discarded after centrifugation. The previous step was repeated two more times, once with deionized water and then finally with ethanol. The resultant hydrogels were then lyophilized at −80 °C for 8 h and crushed to obtain the L-cys_rGO powder.
2.2 Fabrication of the BSA/anti-TNF-α/L-cys_rGO hydrogel/gSPE immunosensor
The immunosensing platform was fabricated using the freeze-dried L-cys_rGO hydrogel powder with a 1
:
5 (GO
:
L-cys) ratio. A 5 mg mL−1 suspension of L-cys_rGO hydrogel powder was prepared using deionized water, followed by probe sonication for 15 min. After sonication, 5 μL of this suspension was drop cast onto the working area of the gSPE and left to dry overnight at 37 °C. The TNF-α antibody was immobilized onto the working electrode using EDC-NHS chemistry for covalent linking of the antibodies to the hydrogel-modified gSPE. For this, a 50 μg mL−1 stock solution of anti-TNF-α antibody was prepared using PBS (pH 7.0). Later, this anti-TNF-α antibody solution was mixed with NHS (0.1 M) and EDC (0.4 M) in a ratio of 2
:
1
:
1, respectively, and the mixture was kept under humid conditions for 45 min to activate the solution. Then, 5 μL of the activated antibody solution was dropped onto the working area of the L-cys_rGO hydrogel/gSPE. It was then kept at 4 °C for 6 h in a humid chamber. During this incubation, a covalent amide bond formed between the amine group in the L-cys_rGO hydrogel and the carboxyl group of anti-TNF-α antibodies, resulting in the covalent immobilization of antibodies. The immunoelectrodes were then washed with PBS. Furthermore, to avoid non-specific attachment of the antigen to the hydrogel surface, free sites devoid of anti-TNF-α antibody were blocked using 2% BSA solution, fabricating the BSA/anti-TNF-α/L-cys_rGO hydrogel/gSPE immunosensing platform (Scheme 1). After blocking, the working electrode surface was again washed with PBS to remove the excess BSA.
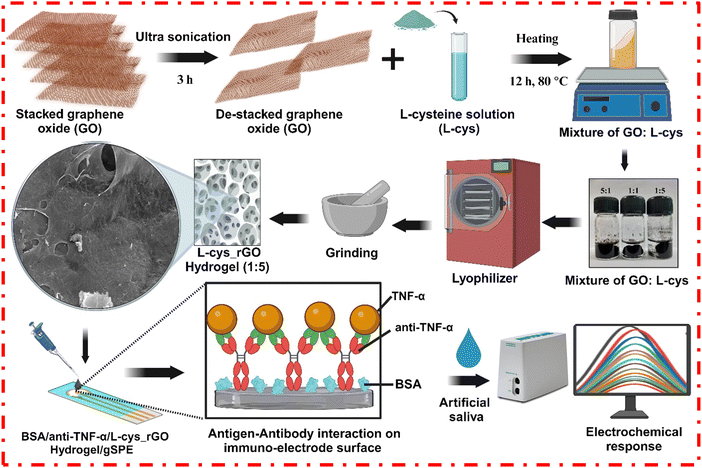 |
| Scheme 1 Synthesis of the L-cys_rGO hydrogel and fabrication of the BSA/anti-TNF-α/L-cys_rGO hydrogel/gSPE immunosensor. | |
3. Results and discussion
The synthesized L-cys_rGO hydrogels are depicted in Fig. 1. The suspensions of GO, upon reduction by L-cys, became black and viscous. This black hydrogel mass formation could be due to the bonding between L-cys molecules and the GO surface with bonds such as C–S, S
O, and amido bonds, which led to the bridging of the nanosheets.42 Initially, in reaction with a 5
:
1 ratio of GO
:
L-cys, a non-fluidic hydrogel with weak crosslinking can be observed. With an increase in the L-cys concentration, typical rGO hydrogel formation and precipitation from the solution could be observed, suggesting increased crosslinking of rGO by L-cys. For further analysis and immunosensor fabrication, the L-cys_rGO hydrogel with a 1
:
5 (GO
:
L-cys) ratio was used, as it showed a well-formed hydrogel structure, which is confirmed using different analytical techniques including XRD, FTIR, FESEM, BET and HRTEM.
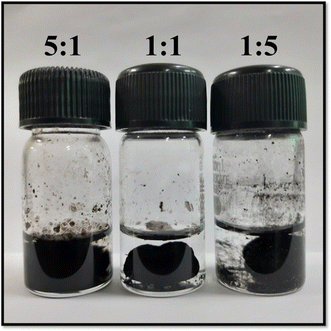 |
| Fig. 1 Synthesis of the L-cys_rGO hydrogel using three different ratios of GO : L-cys (5 : 1, 1 : 1, and 1 : 5). | |
3.1 Structural and morphological characterization
The XRD diffraction plots (Fig. 2) illustrate the phase and the crystallographic structure of the materials within the 2θ range from 10° to 80° at a scan rate of 5° per min including (i) GO, (ii) L-cys, (iii) L-cys_rGO hydrogel (5
:
1), (iv) L-cys_rGO hydrogel (1
:
1), and (v) L-cys_rGO hydrogel (1
:
5). In Fig. 2(i), the XRD plot of GO shows a characteristic peak at 2θ = 10.8° corresponding to the (001) plane due to the graphitic nature,43 while in the case of Fig. 2(ii), the XRD plot of L-cys is shown depicting peaks at 2θ = 12.9°, 18.1°, 19.5°, 20.9°, 21.7°, 24.4°, 28.3°, 31.2°, 32.9° and 33.8° that correspond to the (110), (120), (101), (111), (210), (121), (211), (221), (002) and (310) planes, respectively, that were consistent with the monoclinic system.44 Moreover, XRD patterns of the freeze-dried hydrogels [Fig. 2(iii)–(v)] show the disappearance of the characteristic peak at 2θ = 10.8° that revealed the successful reduction of GO into rGO by L-cys. Also, the appearance of new peaks in the curve (iii) of the L-cys_rGO hydrogel (5
:
1) showed broad peaks at 2θ = 22.1 and 43 that belong to the (002) and (001) planes, respectively.45 This is because the content of GO in this hydrogel is more as compared to L-cys, showing no extra peaks of L-cys; however, in the case of Fig. 2(iv) and (v), the appearance of additional peaks of L-cys occurred due to equal and more content of L-cys, respectively as compared to GO. Furthermore, in the L-cys_rGO hydrogel (1
:
5) [Fig. 2(v)], the incorporation of L-cys has led to the diminishing of the broad peak of rGO, forming a cross-link to form a fully 3D porous network structure.
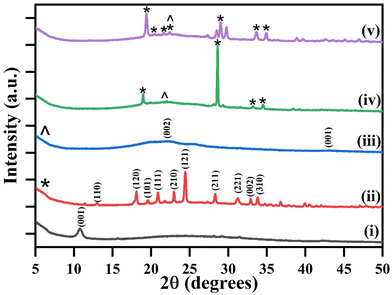 |
| Fig. 2 XRD spectrum of (i) GO, (ii) L-cys, and L-cys_rGO hydrogels with varying GO : L-cys ratios: (iii) 5 : 1, (iv) 1 : 1, and (v) 1 : 5. | |
The surface modifications of the L-cys_rGO hydrogels, GO, L-cys, and the fabricated bioelectrodes were studied using FTIR analysis. Fig. 3(i) demonstrates the characteristic peaks of GO at 1036 cm−1, 1220 cm−1, 1399 cm−1, 1617 cm−1, 1720 cm−1, and 3160 cm−1 that are consistent with the C–O, C–O–C bending, C–OH, C
C, C
O, and O–H stretching respectively.46 Also, a broad peak from 3160–3595 cm−1 corresponds to the stretching of the O–H bond in the C–OH group and water in the material.47 The 2996 cm−1 and 2584 cm−1 peaks correspond to the –NH2 and –SH groups of cysteine attached to the L-cys_rGO hydrogel.42 Furthermore, the peak at 1724 cm−1 belongs to the carbonyl group and is characteristic of GO. As the L-cys concentration used in the production of hydrogel was increased, the peak for the carbonyl group weakened, suggesting that a reaction occurred between L-cys and GO. Next, Fig. 3(ii) presents the FTIR spectrum of L-cys, showcasing the mercapto (S–H) group's peaks at 2549 cm−1 and 866 cm−1, which are characteristic of L-cys. The other peaks at 1295–1390 cm−1, 2965 cm−1, and 1578 cm−1 are related to the symmetric C–O stretching of the carboxyl group, amino groups, and the deformation of the NH2 group of L-cys, respectively. While in the case of the L-cys_rGO hydrogel [Fig. 3(iii)], the absence of peaks at 1720 cm−1 and 2549 cm−1 confirms the successful reduction of GO to rGO and the formation of S–Au bond between the mercapto of the L-cys_rGO hydrogel and gold SPE, suggesting that thiol-terminated L-cys_rGO molecules are likely attached to the gold surface of the electrode. Along with this, a reduction of peak intensity occurred with a slight shift to a low wavenumber. Post antibody immobilization on the L-cys_rGO hydrogel/gSPE surface [Fig. 3(iv)], minor peak shifts occurred, with some additional peaks emerging at 1575 cm−1 and 1716 cm−1 which were likely due to the formation of amide I and II bonds between the antibodies and the L-cys_rGO hydrogel, indicating successful antibody attachment on the L-cys_rGO/gSPE surface. Subsequent BSA treatment [Fig. 3(v)] maintained the same peaks with reduced intensity and peak broadening at 1716 cm−1 and 1575 cm−1 confirming the blocking of the non-specific vacant sites on the surface of immunoelectrodes due to BSA.48
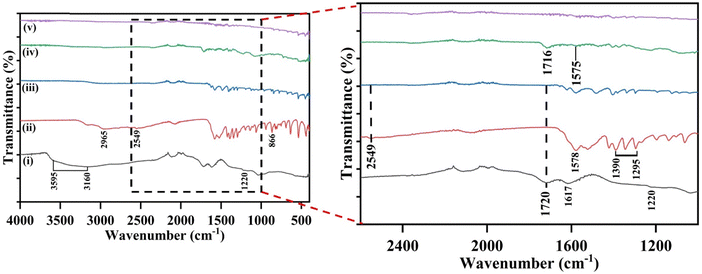 |
| Fig. 3 FTIR spectra of (i) GO, (ii) L-cys, (iii) L-cys_rGO hydrogel (1 : 5), (iv) anti-TNF-α/L-cys_rGO hydrogel/gSPE, and (v) BSA/anti-TNF-α/L-cys_rGO hydrogel/gSPE. | |
The morphological characterization of the L-cys_rGO hydrogel was performed using FESEM-EDX, revealing that L-cys_rGO hydrogels exhibit porous structures. Fig. 4(a)–(c) show the FESEM images of the surface of the L-cys_rGO hydrogels having different GO
:
L-cys ratios. In the hydrogel with a low concentration of L-cys (5
:
1 and 1
:
1) [Fig. 4(a) and (b)], small pores could be observed on the surface of the rGO nanosheet. As the GO to L-cys ratio decreased from 5
:
1 to 1
:
5, an increase in the pore size of the hydrogel was observed. This change in pore size across the hydrogels can be attributed to the phase separation occurring between water molecules and the hydrogel network. While in the case of Fig. 4(c), increasing L-cys content leads to more interconnected GO nanosheets in the polymeric network. A higher L-cys content indicates a denser network. When the concentration of L-cys significantly surpasses the quantity of GO nanosheets, the excess L-cys might have been adsorbed onto the nanosheets, enhancing the network's hydrophilicity. Consequently, the hydrogels exhibited swelling [Fig. 4(c)] and a further increase in pore size. Moreover, element composition was confirmed utilizing EDX [Fig. 4(d)] showing the presence of elements like carbon (C, 70.72%) and oxygen (O, 29.28%). Furthermore, surface changes could be observed in the case of anti-TNF-α/L-cys_rGO hydrogel/gSPE [Fig. 4(e)] and BSA/anti-TNF-α/L-cys_rGO hydrogel/gSPE [Fig. 4(f)], thus confirming the successful fabrication of electrodes.
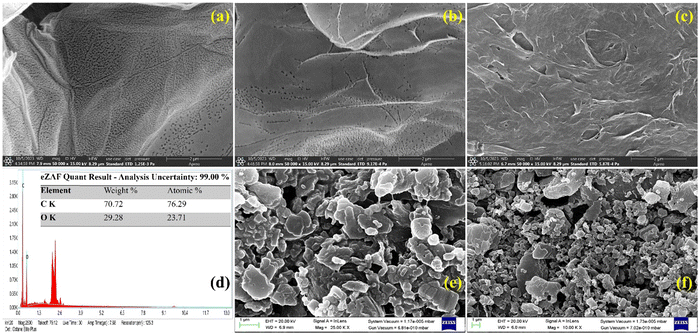 |
| Fig. 4 FESEM images of freeze-dried L-cys_rGO hydrogels of various ratios of GO : L-cys: (a) 5 : 1, (b) 1 : 1, and (c) 1 : 5, (d) EDX results of the L-cys_rGO hydrogel (1 : 5), (e) anti-TNF-α/L-cys_rGO hydrogel/gSPE, and (f) BSA/anti-TNF-α/L-cys_rGO hydrogel/gSPE. | |
Furthermore, transmission electron microscopy (TEM) was used to characterize the crystalline nature and surface structures of the L-cys_rGO hydrogel with GO
:
L-cys (1
:
5). The TEM images of the hydrogel [Fig. 5(a)–(c)] show a thin layer of exfoliated rGO along with the folding of nanosheets. The wrinkled and porous structure of the L-cys_rGO hydrogel could be observed, confirming the crosslinking between the nanosheets through L-cys. Furthermore, the SAED pattern [Fig. 5(d)] confirms the crystalline structure of rGO ascribed to the (002) reflection plane.49 The FESEM and TEM characterization signifies the successful reduction and formation of the L-cys_rGO hydrogel using the one-step coprecipitation method.
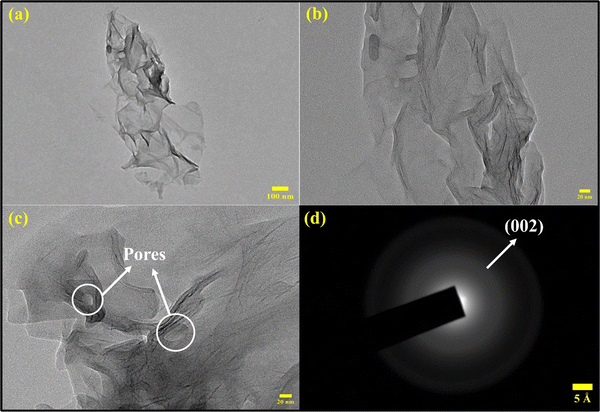 |
| Fig. 5 (a)–(c) TEM images of freeze-dried L-cys_rGO hydrogels with GO : L-cys (1 : 5) and (d) SAED pattern of the L-cys_rGO hydrogel (1 : 5). | |
The nitrogen (N2) adsorption–desorption isotherm recorded at 77.35 K was used to analyze the pore configuration of the L-cys_rGO hydrogel, including the specific BET surface area, average pore radius, and total pore volume. Fig. 6(a) and (b) illustrate the pore size distribution and the N2 adsorption–desorption isotherm, respectively. The average pore diameter and total BET surface area were determined using the Barrett–Joyner–Halenda (BJH) approach, yielding values of 3.92 nm and 36.87 m2 g−1, respectively. Additionally, the pore volume was calculated to be 0.052 cc g−1. The isotherm illustrated in Fig. 6(b) displays an H3-type hysteresis loop which is characteristic of type-4 behavior. The characteristics indicate that the nanomaterial possesses a mesoporous surface, aligning with morphology studies such as FESEM and TEM, which reveal numerous pores within the material.50,51
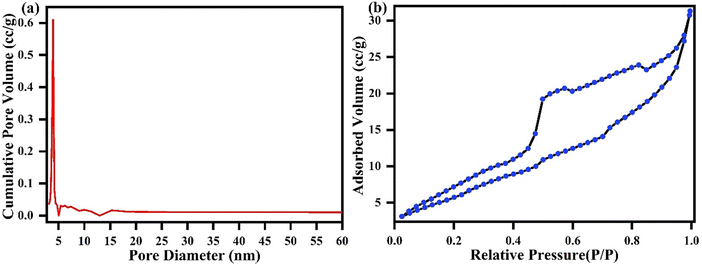 |
| Fig. 6 (a) Pore size distribution and (b) N2 adsorption–desorption isotherm of the L-cys_rGO hydrogel with GO : L-cys (1 : 5). | |
3.2 Electrochemical studies
3.2.1 Electrode and electrokinetic studies.
All the electrochemical studies of fabricated electrodes were done using phosphate-buffered saline (PBS) (0.2 M) (pH 7.0) solution infused with 5 mM [Fe(CN)6]3−/4− as the redox coupler. PBS of pH 7.0 was used for the whole study because biomolecules like antibodies have the highest activity in the pH range of 7–7.4. If pH varies too much, then antibodies can get denatured, which could lead to a loss in the specificity of the antibody towards the antigen. Differential pulse voltammetry (DPV) and cyclic voltammetry (CV) were employed to study the electrochemical behavior of the fabricated immunoelectrodes. Fig. 7a shows the CV curves of (i) unmodified bare gSPE, (ii) L-cys_rGO hydrogel/gSPE, (iii) anti-TNF-α/L-cys_rGO hydrogel/gSPE, and (iv) BSA/anti-TNF-α/L-cys_rGO hydrogel/gSPE across a potential range of −0.8 to +0.8 V. The anodic peak current observed for the bare gSPE was 78.61 μA [Fig. 7a(i)]. However, this peak current increased to 91.34 μA for the L-cys_rGO hydrogel/gSPE [Fig. 7a(ii)], indicating enhanced electron transfer at the L-cys_rGO hydrogel/gSPE-electrolyte interface.25 It shows the good electrocatalytic abilities of the rGO hydrogel. The anodic peak current decreased further with the immobilization of antibodies and BSA onto the electrode, which might be because of the non-conductive nature of the respective biomolecules.30 The peak anodic current in the case of anti-TNF-α/L-cys_rGO hydrogel/gSPE [Fig. 7a(iii)] and BSA/anti-TNF-α/L-cys_rGO hydrogel/gSPE [Fig. 7a(iv)] was 87.68 μA and 77.88 μA, respectively. A similar trend was monitored in DPV measurements [Fig. 7(b)] for (i) unmodified bare gSPE, (ii) L-cys_rGO hydrogel/gSPE, (iii) anti-TNF-α/L-cys_rGO hydrogel/gSPE, and (iv) BSA/anti-TNF-α/L-cys_rGO hydrogel/gSPE wherein the obtained peak current values were 92.13 μA, 110.06 μA, 83.63 μA, and 65.10 μA, respectively, in the potential range of −0.4 to +0.8 V. Table S1 of the ESI† depicts the current values of various electrodes obtained from CV and DPV.
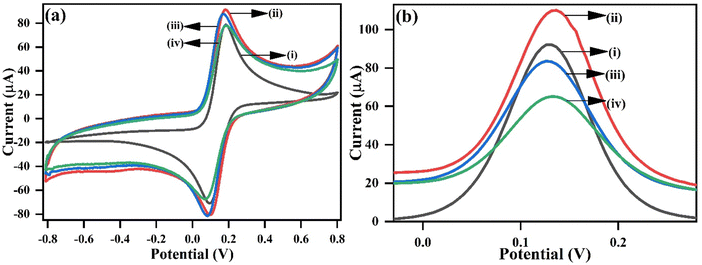 |
| Fig. 7 (a) CV and (b) DPV responses of (i) unmodified bare gSPE, (ii) L-cys_rGO hydrogel/gSPE, (iii) anti-TNF-α/L-cys_rGO hydrogel/gSPE, and (iv) BSA/anti-TNF-α/L-cys_rGO hydrogel/gSPE. | |
Furthermore, electro-kinetic analysis was performed for L-cys_rGO hydrogel/gSPE and BSA/anti-TNF-α/L-cys_rGO hydrogel/gSPE immunoplatforms, with different scan rates ranging across 10 to 100 mV s−1 to examine the various electrochemical parameters as depicted in Fig. S1(a) and (d) of the ESI,† respectively. The resulting peak current values are displayed versus the square root of the scan rate. The anodic and cathodic peak current values were observed to exhibit a linearly increasing and decreasing behavior, respectively, with the square root of the scan rate [Fig. S1(b) and (e) of the ESI†], indicating that the electrochemical reaction adheres to a diffusion-controlled process.52 As modifications were incrementally introduced, the peak separation increased [Fig. S1(c) and (f) of the ESI†], reflecting the occupation of the defective rapid kinetic vacancies, while the residual slow ones accounted for the higher separation values, which reflect slower charge-transfer rates. The functionalization of L-cys_rGO hydrogel/gSPE led to an enhancement in the separation values of peak potential (ΔEp = Epanodic − Epcathodic) as shown in Table 1 (L-cys_rGO hydrogel/gSPE = 0.196 V; BSA/anti-TNF-α/L-cys_rGO hydrogel/gSPE = 0.203 V), suggesting slow electron transmission at the electrolyte and immunoelectrode interface.
Table 1 Scan rate derived parameters of fabricated electrodes
Electrode |
K
s (s−1) |
A (cm2) |
I* (mol cm−2) |
D (cm2 s−1) |
I
pa (A) |
I
pc (A) |
E
a (V) |
L-cys_rGO hydrogel/gSPE |
0.161 |
0.07 |
2.89 × 10−8 |
2.02 × 10−11 |
94.54 × 10−6 |
−83.16 × 10−6 |
0.196 |
BSA/anti-TNF-α/L-cys_rGO hydrogel/gSPE |
0.246 |
0.07 |
2.27 × 10−8 |
1.24 × 10−11 |
74.15 × 10−6 |
−62.86 × 10−6 |
0.203 |
The Brown-Anson equation53 (eqn (1)) was used for calculating the iconic species concentration (I* in mol cm−2) at the surface.
|
 | (1) |
wherein F represents the Faraday constant, T denotes room temperature in Kelvin, and R represents the universal gas constant (8.314 J mol−1 K−1). The surface ionic concentration (I*) values for various immunoelectrodes were determined as follows: L-cys_rGO hydrogel/gSPE = 2.89 × 10−8 mol cm−2 and BSA/anti-TNF-α/L-cys_rGO hydrogel/gSPE = 2.27 × 10−8 mol cm−2. The lower I* value for BSA/anti-TNF-α/L-cys_rGO hydrogel/gSPE signifies the BSA functionalization on the immunoelectrode surface.
The standard heterogeneous electron transfer rate constant (Ks) plays a pivotal role in determining the electron transfer rates at the electrolyte/electrode interface. To calculate the rate constant (Ks) for each immunoelectrode, the Laviron model (eqn (2)) was utilized.54
|
 | (2) |
where symbols retain their previous definitions. The Ks values calculated for L-cys_rGO hydrogel/gSPE and BSA/anti-TNF-α/L-cys_rGO hydrogel/gSPE platforms were found to be 0.161 s−1 and 0.246 s−1, respectively.
The Randles–Sevcik formula55 was also applied to determine the coefficient of diffusion (D) for the [Fe(CN)6]3−/4− redox coupler, as described in eqn (3).
|
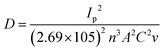 | (3) |
Here, ‘v’ represents the scan rate (0.05 V s−1), ‘D’ denotes the diffusion coefficient, ‘C’ represents the concentration of the redox coupler (5 × 10−3 M), ‘n’ indicates the number of electrons transferred (i.e., 1), ‘A’ represents the surface area of the electrodes (0.007 cm2), and Ip denotes the peak current of immunoelectrodes [L-cys_rGOhydrogel/gSPE = 95.54 μA; BSA/anti-TNF-α/L-cys_rGO hydrogel/gSPE = 74.15 μA]. Using this formula, the diffusion coefficient values were calculated for L-cys_rGO hydrogel/gSPE and BSA/anti-TNF-α/L-cys_rGO hydrogel/gSPE to be 2.02 × 10−11 and 1.24 × 10−11 respectively. The higher D-value for the former electrode suggests enhanced electron transfer at the electrolyte/electrode interface, indicating the superior analytical capability of L-cys_rGO hydrogel/gSPE.
3.2.2 Electrochemical response study.
The electrochemical performance of the immunosensor was evaluated through DPV assessment across a range of TNF-α concentrations from 0.01 to 200 pg mL−1 (0.01, 0.05, 1.0, 20, 40, 60, 80, 100, 120, 140, 160, 180, and 200 pg mL−1) using PBS buffer containing 5 mM [Fe(CN)]3−/4− as depicted in Fig. 8(a). With each increment in TNF-α concentration, a reduction was observed in the peak current. This decreasing trend in the electrochemical behavior of immunosensors is ascribed to the formation of an immunocomplex (antigen–antibody) on the electrode surface between anti-TNF-α and TNF-α concentrations, resulting in a reduction in the electron transfer rate.56Fig. 8(b) shows the linear relationship between the peak currents and TNF-α concentrations obtained by DPV. The regression coefficient (R2) for this correlation is 0.979. The linear range extended from 1 to 200 pg mL−1. From the curve, the following electrochemical parameters were calculated: a limit of quantification (LOQ) of 56.75 pg mL−1, a sensitivity of 2.10 μA pg−1 mL cm−2, and a detection limit of 1.20 pg mL−1. The detection limit, LOQ, and sensitivity of the fabricated immunosensing platform were calculated using standard eqn (4)–(6), respectively. |
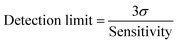 | (4) |
|
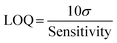 | (5) |
where σ denotes the standard deviation of intercept for the BSA/anti-TNF-α/L-cys_rGO hydrogel/gSPE immunoplatform estimated from the linear plot. |
 | (6) |
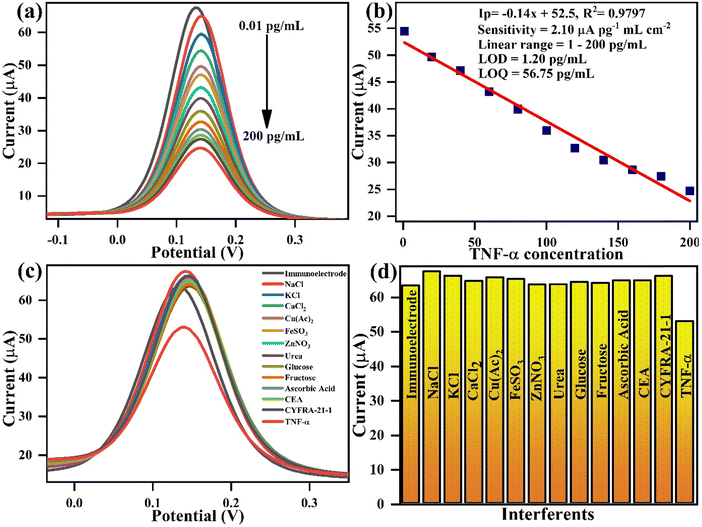 |
| Fig. 8 The electrochemical response study of the BSA/anti-TNF-α/L-cys_rGO hydrogel/gSPE immunosensor against TNF-α. (b) A linear correlation plot of the BSA/anti-TNF-α/L-cys_rGO hydrogel/gSPE immunosensor demonstrates the relationship between peak currents and TNF-α concentrations. The interferent study of the BSA/anti-TNF-α/L-cys_rGO hydrogel/gSPE immunosensor in PBS containing the redox species showing (c) a DPV response curve and (d) a bar graph showing peak currents of the BSA/anti-TNF-α/L-cys_rGO hydrogel/gSPE immunosensor with different interferents. | |
Table 2 summarizes the details of various attempts made toward electrochemical detection of TNF-α in serum and saliva samples. Most of these developed biosensors possess a very narrow detection range and high assay time. For instance, Vozgirdaite et al.57 showed a sensing range of 5–20 pg mL−1. Aydin et al.58 fabricated a biosensor based on polythiophene acetic acid with a 3.7 fg mL−1 LOD. However, the assay time was high (45 min), and the linear range was only 0.01–2 pg mL−1. Compared to existing biosensors, our fabricated immunosensor represents a good combination of a broad linear range, a low LOD, a fast response time, and good sensitivity. Furthermore, Qi et al. developed a biosensor for TNF-α detection with a linear range of 0.1–150 pg mL−1, and our biosensor demonstrates improved performance with a wider linear range of 1–200 pg mL−1. Although LOD was slightly lower in their case, however, our approach offers several key advantages as it achieves a significantly faster response time of just 5 s, compared to 20 min in their study, making it faster and more efficient. Additionally, our synthesis method is green and simple, utilizing a precipitation technique, while the other approach involves complex and potentially toxic chemicals. Both sensors perform similarly, but our method is more sustainable, easier to fabricate, and holds greater potential for commercialization due to its simplicity.59
Table 2 The various fabricated biosensors for the quantification of TNF-α
Electrodes |
Technique |
Linear range (pg mL−1) |
LOD (pg mL−1) |
Sensitivity |
Assay time |
Sample |
Ref. |
Au WE/CMA/Ab-TNF-α/antigen-Ag-TNF-α/Ab-conjugate-HRP |
Chronoamperometry |
1–30 |
1 |
0.075 pg mL−1 |
30 min |
Saliva |
60
|
TNFa-anti-TNF-Ag@Pt/MWCNTs/Chit/SPCE |
Amperometry |
6–60 |
1.6 |
— |
>8 h |
Spiked serum |
61
|
Si3N4/SiO2/Si[P]/Al |
Capacitance |
1–30 |
1 |
4.4 mV pM−1 |
— |
Artificial saliva |
62
|
Si3N4 ISFET/TESUD/Ab-TNF-α |
Electrochemical |
5–20 |
5 |
— |
30 min |
Artificial saliva |
57
|
Poly(3-thiophene acetic acid) (P3) |
Impedance spectroscopy |
0.01–2 |
3.7 × 103 |
— |
45 min |
Serum and saliva |
58
|
Polymer-coated magnetic microparticles onto gold SPE |
Amperometry |
1–15 |
0.3 |
−0.116 mA per ppt |
— |
Artificial saliva |
63
|
SPCE/DWCNTs/Phe-Ab-TNF/TNFα/Biotin-Ab-TNF/poly-HRP |
Amperometry |
1–200 |
0.85 |
— |
2 h 30 min |
Serum and saliva |
64
|
Aryldiazonium and AuNP decorated rGO nanosheets |
Amperometry |
1–150 |
0.01 |
0.065 μA pg−1 mL−1 |
20 min |
Live cells |
59
|
BSA/anti-TNF-α/L-cys_rGO hydrogel/gSPE
|
Amperometry
|
1–200
|
1.20
|
2.1
|
5 s
|
Artificial saliva
|
This work
|
3.2.3 Interferent study.
Since any saliva sample contains numerous molecules, specificity becomes a significant characteristic of any immunosensor, which ensures that the immunosensor could detect a specific analyte from a mixture of analytes. So, to analyze the specificity of the immunoelectrode towards TNF-α, an interferent study was carried out using the DPV technique. 0.1 mM solutions of interfering agents like NaCl, KCl, CaCl2, Cu(Ac)2, FeSO3, ZnNO3, urea, glucose, fructose, and ascorbic acid, were prepared in PBS. Dilutions of other cancer biomarkers like CEA (100 ng mL−1) and CYFRA-21-1 (30 ng mL−1) were also prepared in PBS. The abovementioned compounds were then utilized as analytes to assess their interference. DPV studies [Fig. 8(c)] showed that the addition of interferent analytes initially caused only a slight decrease in the peak current of the immunoelectrode, which did not change with further interferents, while the addition of TNF-α (1 pg mL−1) caused a sharp decline in peak current, confirming the specificity of the fabricated BSA/anti-TNF-α/L-cys_rGO hydrogel/gSPE immunosensor. The same has been depicted using the bar graph [Fig. 8(d)].
3.2.4 Reproducibility, repeatability, and control studies.
An important factor in the development of an electrochemical immunosensor is its reproducibility, which determines the reliability of the data obtained. The reproducibility studies [Fig. 9(a) and (b)] were performed on 6 electrodes separately. The electrodes were fabricated under the same conditions and had the same dimensions. DPV spectra for different electrodes were noted, and the current values were plotted, with estimated relative standard deviation (%RSD) values. The current values were found to be almost similar, with slight variation. The calculated %RSD values for the electrodes are as low as 5.11%. The electrode performance showed stability for up to 3 weeks, with the maximum current decreasing marginally after the 4th week.
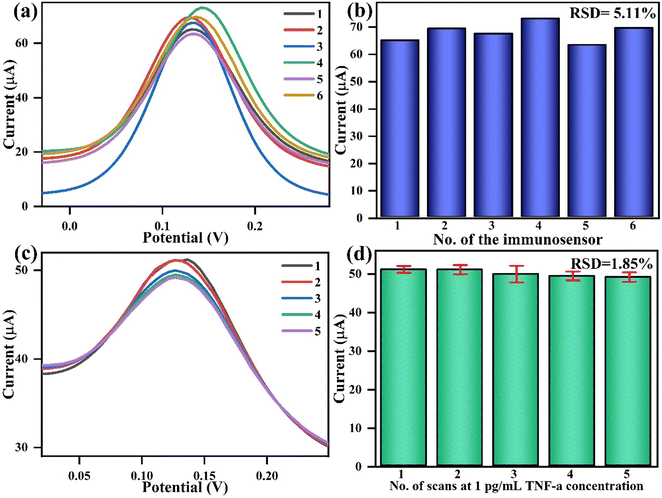 |
| Fig. 9 Reproducibility studies of the BSA/anti-TNF-α/L-cys_rGO hydrogel/gSPE immunosensor showing (a) DPV curves and (b) bar diagram showing peak current values of 6 different immunoelectrodes. Repeatability studies of the BSA/anti-TNF-α/L-cys_rGO hydrogel/gSPE immunosensor showing (c) DPV curves and (d) bar diagram showing peak current values of 5 consecutive measurements at a specific TNF-α concentration of 20 pg mL−1 in PBS. | |
Similarly, a repeatability study is vital for ensuring the stability of the immunoelectrode performance during successive measurements. Fig. 9(c) and (d) show the repeatability studies with BSA/anti-TNF-α/L-cys_rGO hydrogel/gSPE in which 5 successive DPV measurements were taken for the TNF-α concentration of 20 pg mL−1 in PBS containing redox species. Measurements show that the peak current values were similar for all the readings, with a %RSD of 1.85%, which confirms the reliability of the fabricated electrodes.
The control study was performed using rGO/gSPE as the fabricated electrode to check and compare the biosensor performance. For this, non-functionalized rGO (5 μL) was drop cast onto the working surface of gold SPE. The rGO/gSPE was then utilized to determine the electrochemical response by DPV against various TNF-α concentrations from 0.01 to 200 pg mL−1 using PBS buffer comprising 5 mM [Fe(CN)]3−/4−. We noticed a downward trend in current values till 140 pg mL−1 concentration of TNF-α; however, on addition of further higher concentrations, the current values started to increase. This shows an unusual trend in the biosensing performance of the platform [Fig. S2, ESI†]. Thus, a lack of sensitivity towards TNF-α and too much variability in the readings in the control study confirm that the non-functionalized rGO/gSPE is not able to determine such a wide range of concentration of TNF-α alone with lower sensitivity. However, modification of the biosensor with the BSA/anti-TNF-α/L-cys_rGO hydrogel/gSPE immunosensor provides better electrochemical parameters along with good specificity towards TNF-α.
3.2.5 Spiked-sample study.
To show the practical use of the fabricated BSA/anti-TNF-α/L-cys_rGO hydrogel/gSPE immunosensor platform for detection of salivary TNF-α, artificial saliva was employed as it mimics the composition of saliva. At first, addition of artificial saliva was done to observe the change in current. As no current change was seen, a spiked sample study was carried out, in which artificial saliva was spiked with known concentrations of TNF-α (0.01, 0.05, 1.0, 20, 40, 60, 80, 100, 120, 140, 160, 180, and 200 pg mL−1). The peak current responses of these spiked samples were compared with those of standard samples with known concentrations of TNF-α in PBS, using the DPV method. Fig. 10 shows the peak current responses of spiked artificial saliva samples and standard TNF-α concentrations. Table S2 (ESI†) presents the percent recoveries (%) for spiked artificial saliva samples for different TNF-α concentrations and their average %RSD values. The %RSD values were below 5%, and %recoveries were within the 94.15–103.55% range. The obtained data affirm that BSA/anti-TNF-α/L-cys_rGO hydrogel/gSPE is useful for practical applications in determining TNF-α concentrations.
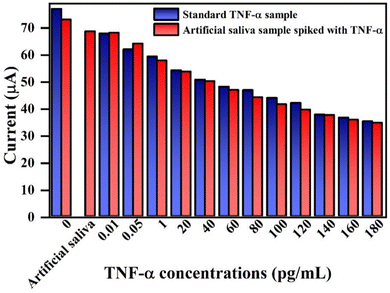 |
| Fig. 10 Comparative bar graph showing a spiked artificial saliva sample study with standard sample utilizing BSA/anti-TNF-α/L-cys_rGO hydrogel/gSPE for TNF-α biomarker detection. | |
4. Conclusion
In summary, we have developed an electrochemical immunosensor platform for the easy and rapid detection of TNF-α, a salivary oral cancer biomarker. The immunosensor platform employed a porous, electroactive L-cys_rGO hydrogel integrated with a screen-printed chip for TNF-α detection. The combination of the L-cys_rGO hydrogel and gold electrode facilitated the rapid and efficient electron transfer, as well as the improved electrochemical surface area on the electrode, thereby enabling the covalent immobilization of anti-TNF-α antibodies at multiple sites. The increased immobilization of the antibody imparted better sensitivity to the immunosensor platform. The developed sensor demonstrated a broad detection range of 1 pg mL−1 to 200 pg mL−1, depicting a strong linear relationship towards TNF-α concentrations and a low detection limit of 1.20 pg mL−1. Our study highlights that the L-cys_rGO hydrogel is a promising nanostructured transducer material due to its high surface area and porous 3D structure. The fabricated biosensor platform showed excellent experimental performance for TNF-α quantification with improved sensitivity, good reproducibility, and specificity as compared to other previous reports on TNF-α determination. Consequently, this fabricated sensor holds promise for point-of-care diagnostic (POC) applications and multiplexed biosensing by integrating with portable electronic devices and microfluidics.
Author contributions
Parth Kapil: methodology, investigation, data curation, validation, and writing – original draft. Damini Verma: conceptualization, methodology, investigation, data curation, validation, writing – original draft, formal analysis, and writing – review & editing. Rangadhar Pradhan: validation, formal analysis, and writing – review & editing. Ashish Kalkal: conceptualization, methodology, formal analysis, writing – review & editing, and supervision. Gopinath Packirisamy: conceptualization, validation, writing – review & editing, resources, supervision, and funding acquisition.
Data availability
The data supporting this article have been included as part of the ESI.†
Conflicts of interest
The authors declare no conflict of interest.
Acknowledgements
The present work has been supported by the Indian Council of Medical Research (ICMR)-ICRC Task Force project (No. 5/13/34/GP/ICRC/2022/NCD-III) and the Anusandhan National Research Foundation (ANRF) funded project (CRG/2023/003549). Parth Kapil is grateful to the Department of Biotechnology (DBT), for the fellowship. Prof. Gopinath Packirisamy is thankful to the Department of Biotechnology, Government of India for the TATA Innovation Fellowship. The authors extend their gratitude to the Institute Instrumentation Centre, IIT Roorkee, for providing various characterization facilities and AIRF, Jawaharlal Nehru University, New Delhi for providing TEM facility. The schematic was created using BioRender.com.
References
- F. Bray, M. Laversanne, E. Weiderpass and I. Soerjomataram, Cancer, 2021, 127, 3029–3030 CrossRef
.
- S. Warnakulasuriya, Oral Oncol., 2010, 46, 407–410 CrossRef
.
- Z. W. Chan, Y. F. Phuan, P. Y. Ooi, N. Nor Azmi, D. G. S. Pateel, H. Y. Y. Yap and S. Gunjal, BMC Oral Health, 2023, 23, 1–7 CrossRef PubMed
.
- D. M. Parkin, P. Pisani and J. Ferlay, J. Cancer, 1999, 80, 827–841 CrossRef
.
- P. Tandon, A. Dadhich, H. Saluja, S. Bawane and S. Sachdeva, Wspolczesna Onkol., 2017, 21, 178–183 CrossRef
.
- S. Graham, H. Dayal, T. Rohrer, M. Swanson, H. Sultz, D. Shedd and S. Fischman, J. Natl. Cancer Inst., 1977, 59, 1611–1618 CrossRef
.
- C. C. R. Ragin, F. Modugno and S. M. Gollin, J. Dent. Res., 2007, 86, 104–114 CrossRef
.
-
L. Acker-man and J. Del Regato, Cancer diagnosis, treatment and prognosis, 1955, p. 1001 Search PubMed.
- S. A. Soper, K. Brown, A. Ellington, B. Frazier, G. Garcia-Manero, V. Gau, S. I. Gutman and D. F. Hayes, Biosens. Bioelectron., 2006, 21, 1932–1942 CrossRef PubMed
.
- R. Goldoni, M. Farronato, S. T. Connelly, G. M. Tartaglia and W. H. Yeo, Biosens. Bioelectron., 2021, 171, 112723 CrossRef PubMed
.
- S. N. Topkaya, M. Azimzadeh and M. Ozsoz, Electroanalysis, 2016, 28, 1402–1419 CrossRef
.
- G. Yang, Z. Xiao, C. Tang, Y. Deng, H. Huang and Z. He, Biosens. Bioelectron., 2019, 141, 111416 CrossRef PubMed
.
- N. Pachauri, G. B. V. S. Lakshmi, S. Sri, P. K. Gupta and P. R. Solanki, Mater. Sci. Eng., C, 2020, 113, 110911 CrossRef PubMed
.
- A. Peisker, G. F. Raschke, M. D. Fahmy, A. Guentsch, K. Roshanghias, J. Hennings and S. Schultze-Mosgau, Med. Oral Patol. Oral Cir. Bucal, 2017, 22, e270 CAS
.
- R. Malhotra, A. B. Urs, A. Chakravarti, S. Kumar, V. K. Gupta and B. Mahajan, Tumor Biol., 2016, 37, 9263–9271 CrossRef PubMed
.
- D. Tang, D. Tao, Y. Fang, C. Deng, Q. Xu and J. Zhou, Med. Sci. Monit. Basic Res., 2017, 23, 141 CrossRef
.
- T. Horiuchi, H. Mitoma, S. I. Harashima, H. Tsukamoto and T. Shimoda, Rheumatology, 2010, 49, 1215–1228 CrossRef
.
- G. Epstein Shochet, E. Brook, L. Israeli-Shani, E. Edelstein and D. Shitrit, Respir. Res., 2017, 18, 1–12 CrossRef
.
- C. Goertzen, H. Mahdi, C. Laliberte, T. Meirson, D. Eymael, H. Gil-Henn and M. Magalhaes, Oncotarget, 2018, 9, 29047 CrossRef
.
- G. Deepthi, S. R. K. Nandan and P. G. Kulkarni, Asian Pac. J. Cancer Prev., 2019, 20, 2087 CrossRef PubMed
.
- Y. Nakano, W. Kobayashi, S. Sugai, H. Kimura and S. Yagihashi, Jpn. J. Cancer Res., 1999, 90, 858–866 CrossRef
.
- S. Sri, D. Chauhan, G. B. V. S. Lakshmi, A. Thakar and P. R. Solanki, Electrochim. Acta, 2022, 405, 139736 CrossRef CAS
.
- D. Verma, N. Dubey, A. K. Yadav, A. Saraya, R. Sharma and P. R. Solanki, Mater. Adv., 2024, 5, 2153–2168 RSC
.
- A. Kalkal, S. Kadian, S. Kumar, G. Manik, P. Sen, S. Kumar and G. Packirisamy, Biosens. Bioelectron., 2022, 195, 113620 CrossRef CAS PubMed
.
- S. Kumar, A. S. Kumar, S. Augustine, S. Yadav, B. K. Yadav, R. P. Chauhan, A. K. Dewan and B. D. Malhotra, Biosens. Bioelectron., 2018, 102, 247–255 CrossRef CAS PubMed
.
- R. Gupta, N. Salaris, A. Kalkal, P. Mandal, S. Balabani, R. Motallebzadeh and M. K. Tiwari, IEEE Sens. Lett., 2024, 8, 1–4 Search PubMed
.
-
S. Kumar and A. Kalkal, Nanotechnology in Cancer Management: Precise Diagnostics toward Personalized Health Care, 2021, pp. 43–71 Search PubMed
.
- A. Kalkal, A. Tiwari, D. Sharma, M. K. Baghel, P. Kumar, R. Pradhan and G. Packirisamy, Int. J. Biol. Macromol., 2023, 253, 127260 CrossRef PubMed
.
- R. Gupta, N. Salaris, A. Kalkal, P. Mandal, S. Balabani, R. Motallebzadeh and M. K. Tiwari, IEEE Sens. Lett., 2024, 8, 1–4 Search PubMed
.
- D. Verma, S. K. Yadav, A. Kalkal, R. Pradhan and G. Packirisamy, IEEE Sens. Lett., 2023, 7, 1–4 Search PubMed
.
- H. S. Song, O. S. Kwon, J. H. Kim, J. Conde and N. Artzi, Biosens. Bioelectron., 2017, 89, 187–200 CrossRef PubMed
.
- X. Huang, X. Qi, F. Boey and H. Zhang, Chem. Soc. Rev., 2012, 41, 666–686 RSC
.
- S. Cui, H. Pu, E. C. Mattson, Z. Wen, J. Chang, Y. Hou, C. J. Hirschmugl and J. Chen, Anal. Chem., 2014, 86, 7516–7522 CrossRef PubMed
.
- A. Kalkal, R. Pradhan and G. Packirisamy, Int. J. Biol. Macromol., 2023, 242, 125157 CrossRef PubMed
.
- V. K. S. Hsiao, J. R. Waldeisen, Y. Zheng, P. F. Lloyd, T. J. Bunning and T. J. Huang, J. Mater. Chem., 2007, 17, 4896–4901 RSC
.
- A. Chen and S. Chatterjee, Chem. Soc. Rev., 2013, 42, 5425–5438 RSC
.
- S. K. Vashist, E. Lam, S. Hrapovic, K. B. Male and J. H. T. Luong, Chem. Rev., 2014, 114, 11083–11130 CrossRef PubMed
.
- S. Tiwari, P. K. Gupta, Y. Bagbi, T. Sarkar and P. R. Solanki, Biosens. Bioelectron., 2017, 89, 1042–1052 CrossRef PubMed
.
-
R. Levine, in Principles of Biochemistry, ed. A. White, P. Handler, E. L. Smith and D. Stetten, Jr., Perspectives in Biology and Medicine, 1960, vol. 3, pp. 439–440 Search PubMed
.
- K. J. Huang, J. Li, Y. M. Liu, X. Cao, S. Yu and M. Yu, Microchim. Acta, 2012, 177, 419–426 CrossRef
.
- G. Yao and Q. Huang, J. Phys. Chem. C, 2018, 122, 15241–15251 CrossRef
.
- Y. Wang, Y. Xiao, G. Gao, J. Chen, R. Hou, Q. Wang, L. Liu and J. Fu, J. Mater. Chem. B, 2017, 5, 511–516 RSC
.
- C. M. Chen, Q. H. Yang, Y. G. Yang, W. Lv, Y. F. Wen, P. X. Hou, M. Z. Wang and H. M. Cheng, Adv. Mater., 2009, 21, 3007–3011 CrossRef CAS
.
- Y. Su, E. P. Hessou, E. Colombo, G. Belletti, A. Moussadik, I. T. Lucas, V. Frochot, M. Daudon, S. Rouzière, D. Bazin, K. Li, P. Quaino and F. Tielens, Amino Acids, 2022, 54, 1123–1133 CrossRef CAS
.
- H. S. Maharana, P. K. Rai and A. Basu, J. Mater. Sci., 2017, 52, 1089–1105 CrossRef CAS
.
- R. A. Rochman, S. Wahyuningsih, A. H. Ramelan and Q. A. Hanif, IOP Conf. Ser.: Mater. Sci. Eng., 2019, 509(1), 012119 CAS
.
- E. Andrijanto, S. Shoelarta, G. Subiyanto and S. Rifki, AIP Conf. Proc., 2016, 1725, 20003 CrossRef
.
- S. Tiwari, P. K. Gupta, Y. Bagbi, T. Sarkar and P. R. Solanki, Biosens. Bioelectron., 2017, 89, 1042–1052 CrossRef CAS PubMed
.
- D. Panda, A. Nandi, S. K. Datta, H. Saha and S. Majumdar, RSC Adv., 2016, 6, 47337–47348 RSC
.
- M. Thommes, K. Kaneko, A. V. Neimark, J. P. Olivier, F. Rodriguez-Reinoso, J. Rouquerol and K. S. W. Sing, Pure Appl. Chem., 2015, 87, 1051–1069 CrossRef CAS
.
- Y. Lu, Q. Yang, S. Wang, M. Liu, Y. Chen, Z. Zhao, A. R. Akbar, C. Xiong and G. H. Hu, Ionics, 2021, 27, 3615–3626 CrossRef CAS
.
- D. Verma, T. K. Dhiman, M. Das Mukherjee and P. R. Solanki, J. Electrochem. Soc., 2021, 168, 97504 CrossRef CAS
.
- D. Verma, R. Sajwan, G. B. V. S. Lakshmi, A. Kumar, R. K. Sajwan and P. R. Solanki, Environ. Sci.: Nano, 2022, 9, 3992–4006 RSC
.
- D. Chauhan, A. K. Yadav and P. R. Solanki, Microchim. Acta, 2021, 188, 1–11 CrossRef
.
- D. Verma, D. Chauhan, M. Das Mukherjee, K. R. Ranjan, A. K. Yadav and P. R. Solanki, J. Appl. Electrochem., 2021, 51, 447–462 CrossRef CAS
.
- A. K. Yadav, P. Gulati, R. Sharma, A. Thakkar and P. R. Solanki, Talanta, 2022, 243, 123376 CrossRef CAS
.
- D. Vozgirdaite, H. Ben Halima, F. G. Bellagambi, A. Alcacer, F. Palacio, N. Jaffrezic-Renault, N. Zine, J. Bausells, A. Elaissari and A. Errachid, Chemosensors, 2021, 9, 26 CrossRef CAS
.
- E. B. Aydın, M. Aydın and M. K. Sezgintürk, Biosens. Bioelectron., 2017, 97, 169–176 CrossRef PubMed
.
- M. Qi, Y. Zhang, C. Cao, M. Zhang, S. Liu and G. Liu, Anal. Chem., 2016, 88, 9614–9621 CrossRef CAS
.
- L. Barhoumi, A. Baraket, F. G. Bellagambi, G. S. Karanasiou, M. Ben Ali, D. I. Fotiadis, J. Bausells, N. Zine, M. Sigaud and A. Errachid, Sens. Actuators, B, 2018, 266, 477–484 CrossRef CAS
.
- M. Mazloum-Ardakani and L. Hosseinzadeh, RSC Adv., 2015, 5, 70781–70786 RSC
.
- M. Bahri, A. Baraket, N. Zine, M. Ben Ali, J. Bausells and A. Errachid, Talanta, 2020, 209, 120501 CrossRef CAS
.
- L. Barhoumi, F. G. Bellagambi, F. M. Vivaldi, A. Baraket, Y. Clément, N. Zine, M. Ben Ali, A. Elaissari and A. Errachid, Sensors, 2019, 19, 692 CrossRef
.
- E. Sánchez-Tirado, C. Salvo, A. González-Cortés, P. Yáñez-Sedeño, F. Langa and J. M. Pingarrón, Anal. Chim. Acta, 2017, 959, 66–73 CrossRef
.
|
This journal is © The Royal Society of Chemistry 2024 |
Click here to see how this site uses Cookies. View our privacy policy here.