DOI:
10.1039/D3VA00296A
(Critical Review)
Environ. Sci.: Adv., 2024,
3, 522-542
Carbon farming: a circular framework to augment CO2 sinks and to combat climate change
Received
22nd September 2023
, Accepted 16th January 2024
First published on 21st February 2024
Abstract
Addressing the climate crisis is one of the most pressing issues of our time. Confronting climate change and meeting the 1.5 °C target set by the Conference of Parties (COP 28) requires the implementation of long-term carbon-sink measures. Carbon farming (CF) is a scalable, cost-effective, and efficient approach to achieving negative emissions that aligns with the larger goals of sustainability and climate resilience. CF is a carbon management system that facilitates the accumulation and storage of greenhouse gases within the Earth's systems. Notably, one-third of the Earth's land is used for crops and grazing, creating a significant opportunity to capture atmospheric CO2 and convert it into soil organic carbon (SOC). CF enables to establish a mechanism for sequestering carbon in long-term storage forms by improving soil health and agricultural output in the framework of nature-based solutions (NBS). In the midst of growing global efforts to combat climate change, the implementation of sustainable agriculture and soil conservation services (SCS) via ‘carbon farming’ is emerging as a critical approach to addressing environmental issues and promoting a resilient future. Voluntary participation in future carbon offset markets may provide incentives for this approach.
Environmental significance
The surge in economic activity and consumerism following industrialization multiplied the demand for materials, putting enormous stress on the Earth's system resilience. A fundamental shift is required to meet the Paris Agreement's 1.5 °C target in the context of net-zero emissions within the timeframe allotted. Given the interconnectedness and interdependence of ecosystem services, adopting a nexus approach is critically important to address climate change using natural carbon-sink resources like wetlands, oceans, and forests. Soil is an often overlooked and underutilized tool in the fight against global warming. Carbon farming strategically augments the natural carbon sinks and bears significant environmental importance through advancing sustainability. By incorporating nature-based solutions (NBS) and circular carbon economy (CCE), carbon farming can present a concrete and effective approach to addressing the critical issues of climate change and environmental degradation, benefiting both current and future generations. Practical viability and scalability are functional advantages of carbon farming that make climate change mitigation more affordable while also addressing sustainability.
|
1. Introduction
The swift transformations occurring within the Earth's system are eroding essential life-sustaining processes, resulting in noticeable repercussions for society, and have the potential to activate tipping points that could irreparably disrupt the Earth's stability.1 Planetary boundaries pertain to climate change, particularly focusing on carbon dioxide (CO2) emissions and other greenhouse gases (GHGs). Beyond a temperature increase of 1.5 °C or 2.0 °C, there is an increased risk of initiating tipping points that could endanger global carbon sinks and disrupt the boundaries of the Earth's systems (ESB).1 Soil contains a significant portion of the Earth's terrestrial carbon, with a total carbon content of approximately 3170 GT.2 Within the top 3 meters of soil are 2500 GT of carbon, comprising 1550 GT of soil organic carbon (SOC) and 950 GT of soil inorganic carbon.3 Notably, this soil carbon pool is 3.3 times larger than the atmospheric carbon pool, which contains 760 GT.4 The transformation of natural ecosystems into agricultural land has led to a reduction in SOC levels. This change resulted in the release of 50 to 100 GT of carbon from the soil into the atmosphere since the start of the industrial revolution in 1950.5
The Earth's climate is undergoing rapid transformations due to ongoing human-induced emissions of CO2 and other GHGs into the atmosphere.6 Among various GHGs (e.g., N2O and CH4), CO2 stands out as the primary driver of global climate change due to its substantial increase from preindustrial times to the present. Specifically, atmospheric CO2 concentrations have surged from approximately 280 ppm before 1850 to 417 ppm in 2023.7,8 Furthermore, there has been a consistent annual increase of 0.88 ppm (equivalent to 3.5 gigatons of carbon per year).9,10 Most of this overall rise in atmospheric CO2 is attributed to the burning of fossil fuels, accounting for about two-third of the increase. The remaining portion of the increase is linked to the loss of SOC due to changes in land use, such as deforestation and crop cultivation.11 According to the Food and Agriculture Organization,12 approximately 25% of global GHG emissions result from forestry, agriculture, and changes in land use (Fig. 1). Over the last 12
000 years, the expansion of farmland has resulted in the release of approximately 110 billion metric tons of carbon from the surface stratum of soil.13,14 This is roughly equivalent to the carbon emissions produced by the United States over 80 years. While agricultural lands typically contain lower levels of soil organic carbon compared to natural landscapes, agriculture possesses the potential to become a significant contributor to negative emissions.15,16 Adopting enhanced agricultural methods, it's projected that soils could capture approximately 20 petagrams of carbon (20 Pg C) over a span of 25 years. This amounts to more than 10% of the global anthropogenic emissions.17
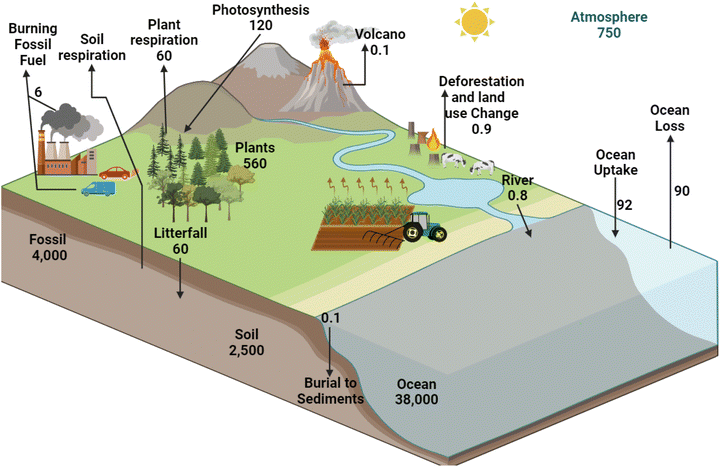 |
| Fig. 1 CO2 emission from land use and land cover change (LULCC; all numbers are in Pg C units). | |
Carbon farming (CF) is a system of carbon management that helps to accumulate and store more greenhouse gases in land instead of releasing those gases into the atmosphere.16 It encompasses a broader range of sustainable agricultural practices such as agroforestry, cover cropping, rotational grazing, minimizing chemical fertilizers, and reducing tillage.18–20 These practices aid in trapping CO2 from the atmosphere, storing it in either the soil or plants, while also offering additional advantages such as enhancing soil quality and disease resilience, reducing erosion, and boosting productivity.21,22 SOC serves a crucial role in responding to anticipated changes in land use and climate by acting as both a carbon sink and a means of reducing future CO2 emissions. Due to the significant potential of SOC in agricultural soils for generating negative emissions, there is a growing interest from both governments and the private sector in encouraging farmers to adopt cultivation practices that enhance carbon sequestration as part of broader climate change mitigation strategies.23,24 The new EU Soil Strategy 2030 aims to implement measures for increasing soil organic carbon (SOC) stock to achieve land-based climate neutrality in the EU by 2035 and to contribute to a climate-neutral Europe by 2050. Research has revealed that each land-based method for removing CO2 from the atmosphere contributes positively to various aspects of nature's role in supporting human well-being and the achievement of sustainable development goals.25 This review centers on the possibilities and obstacles associated with adopting carbon farming and regenerative agriculture to pursue the Paris climate target.
2. Soil carbon cycle
Soils rank among the Earth's most significant carbon reservoirs, second only to the hydrosphere,26 which accounts for almost two-thirds of the worldwide soil carbon content. SOC is produced by decaying plants, fungal and bacterial development, and the metabolic activities of living organisms (Fig. 2),27 while soil inorganic carbon (SIC) comprises carbon found in mineral forms such as calcium carbonate (CaCO3), magnesium carbonate (MgCO3), and calcium-magnesium carbonate (CaMg(CO3)2).28 Climate change has notable effects on SIC, with changes in soil pH being a prominent outcome. The rise in atmospheric CO2 results in the release of carbonates into the atmosphere, consequently lowering soil pH.11 Currently, 30% of CO2 emissions from fossil fuel combustion are transferred from the atmosphere to terrestrial ecosystems through a net carbon flow, corresponding to approximately 3.1 Pg per year.29 Increasing temperatures and heightened soil moisture levels can potentially enhance microbial activity, speeding up the breakdown of SOC and consequently resulting in increased soil respiration rates.30 Additionally, elevated temperatures may bring about changes in the chemical composition of soil organic matter, leading to the buildup of microbial carbon at the cost of plant lignin when microbial processes are stimulated.31–33 This constructive carbon-climate feedback mechanism could accelerate global warming by releasing more CO2 into the atmosphere. However, in regions experiencing prolonged heavy rainfall and high soil wetness, oxygen availability in the soil can become restricted, creating anaerobic conditions that slow down soil respiration.34 Droughts might add O2 to anoxic soils and encourage microbial carbon oxidation in these wet habitats.35
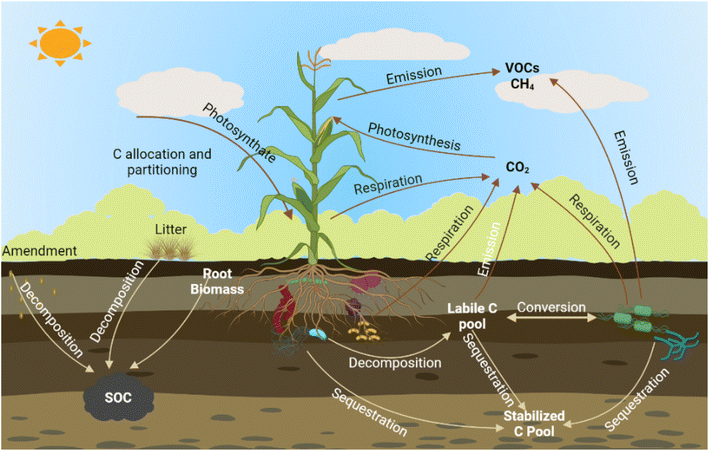 |
| Fig. 2 Carbon dissociation in the environment. | |
Peatlands and wetlands, characterized by their substantial soil carbon content, are particularly susceptible to drought stress.36,37 The uncertainty surrounding the influence of warming on soil carbon reservoirs stems from the delicate balance between climate-induced growth in carbon originating from plants and the breakdown of soil organic carbon.38,39 In warmed subarctic grasslands, for instance, carbon loss may be limited to the topsoil,39 but in a conifer forest, it may extend to the subsurface (with a loss of between 33% and 11% after 4.5 years of warming).40 Permafrost regions are particularly susceptible to warming and are expected to face more significant temperature increases due to climate change. These regions store substantial additional carbon, approximately 1460–1600 Pg C.41 Thawing permafrost can result in massive carbon emissions into the atmosphere, accelerating climate change. According to projections for the 21st century, warming may cause the top one meter of soil to lose 190 Pg of soil carbon and the overall soil profile to sustainably increase CO2 outflow by 30 to 40%.38
2.1 Soil nutrient cycle
Notably, the availability of nutrients, especially N and P, impacts how much carbon is lost due to increasing temperatures, creating intricate feedback loops. Denitrification is crucial in moist environments brought on by flooding or permafrost thawing because it can release N2O.42 It is a potent GHG with a global warming potential 300 times higher than that of CO2 over 100 years.43 The escalation of N2O emissions will exacerbate the adverse effects of climate change, worsening an already dire situation. Flooding-related fertilizer and soil runoff could worsen aquatic ecosystem eutrophication and impair drinking water quality. As extreme weather events occur more frequently, pollutants are also remobilized through runoff from agricultural fields with pesticide-contaminated topsoil and river sediments during flooding.44 Exposure to organisms containing these bioactive compounds will harm biodiversity and encourage pesticide resistance.45
2.2 Plant biomass – carbon stocks (green carbon)
Green carbon refers to the carbon sequestered through photosynthesis and stored in natural forests. Organic matter originating from plant residues is vital in sustaining soil health. It dictates the soil structure and porosity while facilitating the gradual release of water and nutrients to plants through the actions of soil biota.46 Around 450 gigatons (Gt) of carbon are sequestered within terrestrial biota, and mostly stored in plants (Table 1).47 The primary mechanism for carbon exchange between the atmosphere and terrestrial ecosystems revolves around plants absorbing roughly 123 GT of CO2 annually through photosynthesis. Out of this, around 3 GT annually can be attributed to human activities. Higher plants, algae, and cyanobacteria perform oxygenic photosynthesis by harnessing photon energy. This process not only helps to reduce atmospheric CO2, but also provides essential food and oxygen to sustain aerobic life on Earth. The carbon fixation abilities of terrestrial plants differ depending on factors such as the efficiency of their photosystem complexes, enzymatic processes, and their responsiveness to environmental cues.48,49 C4 plants demonstrate greater water efficiency, reduce photorespiration, and can sustain elevated rates of photosynthesis and CO2 assimilation compared to C3 plants, especially under intense light and high temperature conditions. For instance, Fagus sylvatica boasts the highest CO2 capture rate, achieving an annual carbon sequestration rate of 4.9 tons per hectare per year (t per Cha per year). By 2050, between 0.5 and 5 gigatonnes of CO2 could be absorbed, with an estimated cost ranging from USD 60 to USD 160 per ton.50 Regarding urban trees in India, they have shown a carbon sequestration rate ranging from 0.04 to 0.23 kilograms of carbon dioxide (CO2)/m2 per year, with an average rate of approximately 0.12 kilograms of CO2 m2 per year (eqn (1)). To calculate the weight of CO2 in trees, we use the ratio of CO2 to C, which is 44/12 = 3.67 (eqn (2)). Generally, approximately 50% of any plant species' biomass is considered carbon.51 | Carbon storage (kg) = total biomass (kg) × 50% or total biomass/2 | (1) |
| CO2 sequestered (kg) = carbon storage (kg) × 3.67 | (2) |
Table 1 Terrestrial and phytomass carbon pool by the IPCC climate region
IPCC climate region |
Total SOC (Pg C) |
Phytomass carbon (Pg C) |
Terrestrial carbon pool (Pg C) |
Reference |
Tropical wetland |
310–360 Mg h−1 at a soil depth of 0–50 cm |
140 |
268 |
56
|
Tropical montane |
149 Mg h−1 |
40 |
96 |
57
|
Tropical dry |
64 Mg h−1 at a soil depth of 0–50 cm |
42 |
178 |
58
|
Tropical moist |
150 |
151 |
302 |
59
|
Warm temperate dry |
78 |
24 |
102 |
60
|
Warm temperate moist |
63 |
28 |
91 |
60
|
Cool temperate dry |
102 |
9 |
111 |
61
|
Cool temperate moist |
210 |
28 |
238 |
61
|
Boreal dry |
69 |
5 |
74 |
62
|
Boreal moist |
356 |
23 |
380 |
62
|
Polar dry |
12 |
0.5 |
12 |
63
|
Polar moist |
52 |
2 |
54 |
63
|
Carbon is released back into the atmosphere through two major processes: (1) plant respiration, accounting for 60 GT per year, and (2) microbial respiration, also releasing 60 GT per year, from previously stored carbon (Fig. 2).52 Land use and land cover change (LULCC) emissions rank as the second-largest anthropogenic source of carbon released into the atmosphere, following emissions from fossil fuel combustion.10,53 The uncertainty in LULCC emissions arises from three main factors: the variability of carbon stocks in phytomass and soils, the spatial dispersal of carbon stocks, and the effect of land management practices on phytomass and SOC.23 The organic decomposition, nitrification, and denitrification processes in plant wastes (debris and lower-ground biomass) can cause C and N to be emitted into the atmosphere as CO2 and N2O.54 Bioenergy carbon capture and storage (BECCS) is another approach combining geological carbon capture and storage with energy production.55 This process involves using trees to capture and retain CO2, which can subsequently be utilized as a sustainable energy source for electricity generation through bioenergy.
2.3 Soil conservation services (SCS) – sustainable development goals (SDGs)
Soil conservation services (SCS) are pivotal in addressing the climate change by establishing a significant, though potentially reversible, sink for atmospheric CO2 and enhancing climate resilience, thereby improving soil fertility.64 SCS also contributes to providing clean water and sanitation (SDG 6) by mitigating soil erosion and reducing the entry of pollutants into water bodies. Addressing SDGs such as no poverty (SDG 1) and zero hunger (SDG 2) cannot be exclusively reliant on regular agriculture practices; sustainable soil management approaches are necessary for building resilient and productive agricultural systems that benefit both the environment and vulnerable populations.65 This, in turn, benefits life below water (SDG 14) by preserving water quality and preventing pollution. Additionally, SCS strengthens soil health, contributing to life on land (SDG 15) by promoting biodiversity and thriving ecosystems. Healthy soils act as effective barriers against various pollutants, thus positively influencing good health and well-being (SDG 3) through improved agricultural output, enhanced water and air quality, and the potential for soil organisms to produce medicinal resources. By supporting sustainable cities and communities, SCS fosters access to affordable and clean energy, particularly by cultivating energy crops. Overall, investing in SCS has multifaceted benefits, making it a crucial component of sustainable development.
3. Emissions from land-use and forestry
The net carbon dioxide flux associated with land use, land-use change, and forestry (ELULUCF) encompasses the release of CO2 stemming from actions such as deforestation, timber extraction, and forest deterioration. It also includes emissions from shifting cultivation, which involves clearing forests for agriculture and abandoning the land, and carbon uptake from forest regrowth following wood harvest or agriculture abandonment.
3.1 Agricultural practices
Agriculture occupies approximately 50% of the Earth's land surface and plays a substantial role in global GHG emissions, accounting for roughly one-third of the total emissions. Farming is a significant driver of global resource utilization, representing approximately 70% of freshwater withdrawals and accounting for roughly 31% of GHGs.66 This places agriculture as the third-largest contributor to environmental impact, following transportation and housing.67 India's Third Biennial Update Report, submitted to the United Nations Framework Convention on Climate Change (UNFCCC) in early 2021, indicates that the agriculture sector in India contributes to 14% of the nation's overall GHG emissions. Furthermore, considering the agriculture and food sectors together, it constitutes the second-largest material footprint, totaling 21.3 billion tons and a carbon footprint of 10 billion tons of CO2 equivalent. The primary sources of agricultural emissions are the livestock sector, responsible for 54.6% of emissions, and the application of nitrogenous fertilizers, contributing 19% to the total emissions. In India, agriculture is important, contributing almost 20% to the nation's gross domestic product (GDP) and offering employment to over half of its 1.4 billion inhabitants. For more than 70% of rural households, agriculture is the primary livelihood source. Rice cultivation is widespread across numerous countries and significantly impacts GHG emissions, contributing to approximately 30% and 11% of the world's agricultural CH4 and N2O emissions, respectively.68,69 Analysts estimate that the GHG emissions from rice production increased to 72.329 million tonnes “CO2 equivalent” in 2018–19 from 71.322 million tonnes “CO2 equivalent” in 2016. Most soils worldwide are typically unsaturated and oxygen-rich, resulting in carbon dioxide (CO2) being the most prevalent respiratory output. However, in waterlogged and oxygen-deprived soils such as rice paddies and peatlands, where methanogenesis occurs, hydrogenotrophic archaea play a crucial role in reducing CO2.70 The combined activity of methanogenic microorganisms, including those involved in acetate fermentation, as well as aerobic methane-oxidizing bacteria (methanotrophs) residing in the upper oxygen-rich layers of the soil, determines the overall methane flux under such conditions.71
3.1.1 Straw burning.
Open burning is one of the most commonly employed methods by farmers worldwide to manage leftover straw.72 However, when rice straw is burned, it releases greenhouse gases such as CO2, CH4, and N2O, along with other trace gases. These emissions contribute to global warming potential and harm ecosystems.73,74 Furthermore, burning leads to the loss of organic matter, resulting in decreased soil fertility.75 Hence, this practice not only poses environmental risks but also undermines the long-term productivity of the soil. Based on an Indian Agricultural Research Institute (IARI) report, approximately 63.6% (equivalent to 14 million tons) of the total 23 million tons of produced rice stubble is burned annually causing an estimated $30 billion in yearly health and economic costs.76 For e.g. burning 3.24 million tons of straw produced 3.84 million tons of CO2 and 29.5 Gg of CO while releasing 7.4 Gg of NOx and 31 Gg of CH4 pollutants.77 In the northern regions of India, the high silica, lignocellulose contents and low protein content (2% to 7%) of rice straw make it unsuitable for direct use as cattle feed. However, due to limited options, dairy operations still utilize the same.78,79
3.1.2 Use of fertilisers.
Nitric oxide emissions increase when nitrogen (N) fertilizers increase pasture or crop yields. Nitric oxide has a 300-fold potential to cause global warming than CO2.80 The utilization of chemical fertilizers has surged from approximately 12 million tons in 1961 to surpass 110 million tons by 2018. Notably, the use of nitrogen (N) and phosphorus (P) exceeds the limits set by planetary boundaries, surpassing them by a factor of two.54,81 Approximately 17.5 teragrams (Tg) of phosphorus (P) are being applied to crop lands through fertilizers each year. It's worth noting that P use is on the rise, and some studies have suggested that up to 32.5 Tg of P is reached per year. On the other hand, approximately 62 Tg of nitrogen (N) is fixed naturally. An additional 112 Tg of N is industrially fixed as fertilizers each year within the agricultural system.47 According to the Food and Agriculture Organization,12 anthropogenically fixed N into the agricultural system is estimated to be around 190 teragrams (Tg) annually. It's important to note that this threshold is also being transgressed globally. Since the green revolution, the soil has deteriorated due to the extensive use of fertilizers and pesticides. According to various estimates, up to 30% of India's entire geographical land area has been degraded; about 50% of this land is used for agriculture, especially unirrigated/rainfed agriculture. This impacts the viability of agricultural output and people who depend on it for their livelihoods.82 China, India, the United States, the EU28, and Brazil accounted for about 68% of this total nitrogen usage. Only some nitrogen fertilizers supplied to the soil are absorbed by plants; the remainder is used by soil microorganisms, who produce N2O as a byproduct of their metabolic activities. Moreover, some of the nitrogen applied may leach or volatilize away from the application site.83,84 The high soil N2O–N EFs (emission factors) contributed to elevated carbon intensities in certain European nations. On the other hand, regions like the Middle East and east Asia show high carbon intensities in their local manufacturing processes, which resulted in synthetic N fertilizers having carbon intensities higher than the global average.85
3.2 Livestock
The cattle industry accounts for 45% of the total agricultural emissions.86 Total emissions from the UK domestic agriculture industry were 55 MtCO2e in 1990 but had dropped to 44 MtCO2e by 2007. Livestock emissions constituted 36 MtCO2e, representing approximately 80% of the overall emission.87 Transitioning from producing and eating ruminant animal products (such as beef, lamb, mutton, and milk) to those of monogastric origin (such as pig, chicken, and eggs) is one option for lowering GHGs.88 Smart livestock farming (SLF) can also contribute to reducing GHGs by modifying potential factors such as temperature, feeding schedules, and quality. These adjustments can positively influence GHG emissions, helping mitigate their environmental impact.89 Grazing has a significant impact on plant production, which in turn affects soil carbon inputs. The majority of aboveground biomass is consumed by grazing animals, and the carbon from this biomass eventually returns to the soil as manure or enters the atmosphere via enteric fermentation. GHG emission per product unit can be reduced by improving livestock's genetic potential, reproduction, wellness, and liveweight gain rates.90
3.3 Soil carbon
Sustainable soil management (SSM) techniques are gaining considerable recognition as a crucial strategy for mitigating GHG emissions, primarily due to their potential to unlock a significant global capacity for carbon sequestration.91–93 Precisely measuring SOC stocks and alterations presents a challenging endeavor, primarily due to the multifaceted impact of a wide range of factors encompassing diverse land use practices, soil properties, vegetation cover, landscape features, climate conditions, and various soil-forming elements and processes.94,95 These variables significantly affect the amount and carbon sequestration in soils, adding complexity to the assessment and management process of carbon sinking. Inelastic neutron scattering, developed by the Brookhaven National Lab, has shown great promise.96 Laser induced breakdown spectroscopy (LIBS) technology is gaining popularity for its ability to quantitatively assess soil carbon, especially SOC, which makes it an attractive option.97 To improve the accuracy of assessing SOC stocks, particularly at different spatial scales, it is essential to establish proper sample procedures to reduce uncertainties associated with the measurements.98 Spectroscopic methods based on the spectral characteristics of soil have been extensively used in controlled laboratory settings.99,100 However, these techniques often only reflect the SOC content of the first few millimeters of the surface layer. Therefore, enhancing the application of traditional methodologies in field settings is a priority for further research. The amount of organic carbon at a soil depth of 10 cm, depth in soil with a carbon content of 2.14% (g C−1 kg−1 soil) and a bulk density of 1.3 g cm−3 (eqn (3)).101 | 10 000 m2 in one hectare × 0.1 m soil depth × 1.3 g cm−3 bulk density = 1300 t per ha soil | (3) |
4. Natural climate solution (NCS) – augmenting carbon sinks
Nature climate solutions (NCSs) encompass innovative strategies that harness and enhance the Earth's natural ecosystems to combat climate change.80 By leveraging the inherent capacity of forests, wetlands, soils, and other natural landscapes, NCS focuses on capturing and storing carbon dioxide (CO2) from the atmosphere (Fig. 3). Reforestation, sustainable forest management techniques, conservation agriculture, wetland restoration, and urban greening are some of the NCS intiatives contributing to lower GHG emissions while simultaneously supporting biodiversity, strengthening ecosystem resilience, and promoting sustainable development for a balanced planet.22,65
 |
| Fig. 3 Nature climate solutions for mitigating climate change through the carbon farming approach. | |
4.1 Afforestation and forest ecosystem restoration
Afforestation and reforestation are highly cost-effective and feasible nature-based climate solutions (NCSs) to combat climate change. By 2030, these approaches can make a remarkable contribution of up to 7 Pg CO2e (petagrams of carbon dioxide equivalent) annually to climate mitigation, particularly when considering a carbon price of $100 per Mg CO2e.102 Terrestrial ecosystems have been removing about 30% of annual human carbon emissions over the past ten years, with forests being the main source of this uptake.103 According to a computable general equilibrium model designed for China, it is forecasted that annual carbon removal through afforestation would reach 617 MtCO2 by the year 2060. The model further anticipated that the majority of biomass in 2060 would be composed of 43–47% cellulosic crops and 49–52% residues. Consequently, there would be a reduction of 6.9–8.3% in China's cropland due to land competition.20 Forest ecosystem restoration focuses on revitalizing degraded or damaged forest ecosystems to bring them back to their original or nearly original state. Through this process, forests can be empowered to sequester more carbon, contributing to climate change mitigation. Moreover, restoring degraded forests enhances ecosystem resilience to climate change effects, including extreme weather events.102 Restoration efforts typically involve carefully selecting native tree species well-adapted to the local environment. These species possess high carbon sequestration potential and provide long-term carbon storage as they grow and accumulate biomass over time. Restoring 15% of converted lands has the potential to prevent 60% of anticipated extinctions and sequester approximately 299 gigatonnes of CO2.104 This amount is equivalent to 30% of the total CO2 increase in the atmosphere since the industrial revolution, or about 14% of total emissions. However, it is crucial to approach these strategies with careful planning, considering expense calculations, evaluating risks, and acknowledging potential damage to ecosystems and human communities.105 Mangrove forests also play a crucial role in counteracting the impacts of climate change due to their capacity to sequester a substantial amount of carbon dioxide, totaling 14.07 gigatons, in the form of “blue carbon” within the coastal sediments.50 Adopting reforestation, sustainable forest management, and agroforestry practices can significantly enhance global carbon sequestration by harnessing the substantial capacity of trees to store carbon and augment soil carbon levels.
4.2 Agriculture as a climate-positive force
Land use, forestry, and agriculture together account for 24% of the world's GHG emissions, with livestock emissions accounting for 7.1 gigatons of CO2-equivalent annually, according to the Food and Agriculture Organization (Table 2) (Fig. 4).12 At COP26, India unveiled its comprehensive climate change strategy,106 which includes ambitious objectives such as reducing carbon emissions by one billion tonnes by 2030, decreasing the carbon intensity of its GDP by 45% by 2030, and achieving net-zero emissions by 2070.107 Farmlands can store up to 1.2 billion tons of carbon when properly managed with suitable techniques and tools (Carbon Engineering 1.0). This offers an opportunity to offset 4% of the world's average annual GHG emissions for the remainder of this century.108 Farmers can physically sequester about 3.6 metric tons of carbon per acre yearly, according to research commissioned by the Dutch bank, Rabo Bank. To do this, farmers may need to hire outside experts to help them conduct pricey soil analysis to effectively measure soil health and invest significant money in changing their agricultural operations. These actions are necessary to meet the required carbon sequestration targets and contribute substantially to the fight against climate change. In his 2017 paper, Australian microbiologist and climatologist Walter Jehne explained that the Earth's soil carbon sponge, comprising a blend of minerals, organic debris, and air, exhibits enhanced capabilities for absorbing and retaining rainfall. This sponge facilitates access to vital nutrients and sustains a diverse array of microbial processes. Consequently, when soil carbon levels decrease, the soil capacity to absorb and retain water diminishes. Beyond reducing the soil’s ability to manage rainfall, this also renders the topsoil more susceptible to runoff and erosion, exacerbating flooding and drought cycles.109 Techniques that boost biomass and lower carbon leakage from the soil must be used to promote soil health. Keyhole garden (KHG) is a a self-regenerating back-yard garden aligning closely with permaculture and beneficial especially in regions facing challenges such as water scarcity and limited fertile land due to consistent hot and arid climates.110,111 This sustainable approach aimed at enhancing food security, elevating nutrition levels, and advocating sustainable agriculture practices, that can be equally embedded into urban ecology.
Table 2 CO2 sequestration potential of different carbon farming practices with co-benefits
S. no |
Technique |
Reported rate of SOC sequestration potential |
Contribution to the carbon cycle |
Co-benefits for sustainable development |
Reference |
1 |
Biochar |
Biochar systems could reduce emissions by 3.4–6.3 PgCO2e, with CO2 removal accounting for half of the reductions |
Enhance the probable carbon sink of soil by adding carbon that is resistant to degradation, may additionally boost the amount of microbial biomass in surface soils |
Improve soil fertility |
118 and 119 |
1.8 Pg CO2–Ce per year |
2 |
Tillage |
Conservation tillage methods, particularly zero tillage, with a yield of 2.07 Mg ha−1 per year, and reduced tillage, yielding 1.98 Mg ha−1 per year, outperform conventional tillage systems in terms of crop productivity |
No till does not disturb soil and hence encourages the preservation of carbon in aggregates |
Reduces soil erosion caused by wind and water and preserves the natural environment of microorganisms |
120
|
Tilling the soil produces significant air pockets that fill up with oxygen and cause bacteria to convert carbon in the soil to CO2 |
3 |
Cover crop |
Sequester between 0.10 and 1 Mg ha−1 per year of SOC |
Cover crops are typically fast-growing plants that produce a significant amount of biomass. This refers to the above and below-ground plant material; hence, they increase above and below-ground plant carbon inputs. A habitat for beneficial microorganisms decomposing plant material and releasing carbon in the soil |
Act as natural mulch, protecting the soil from erosion and preventing the oxidation of SOC |
121
|
4 |
Rewetting grassland |
Drained peat soils save up to ≈20 t CO2eq ha−1 per year |
Support vegetation regrowth, stimulate root exudation, enhance microbial activity, and reduce carbon loss |
Permanent grasslands play a vital role in biological flood control and maintaining the ecosystem's water balance, ensuring a sustainable supply of water resources—crucial habitats for bird breeding and foraging activities |
122
|
5 |
Enhanced deep root |
1 Pg CO2(eq) per year |
Improve plant C inputs into deep soils with lower microbial densities and longer plant-derived C half-lives |
Prevent soil erosion and improve soil fertility |
123
|
6 |
Increased perennialization |
0.136 Mg C/ha/year (inclusion of perennials in rotation—0.5 t CO2/ha/year) |
Increase soil C inputs from above- and below-ground plants to assist the microbial metabolism of C |
Improve soil health, increase water infiltration, and provide habitats and resources for a wider range of plant and animal species than annual crops |
124
|
0.19 Mg C/ha/year (replacing annuals with perennials—0.7 t CO2/ha/year); typically, >10 years |
7 |
Restoring wetlands |
5–20 Mg C ha−1 per year |
Soils are rich in organic matter due to the high productivity of wetland vegetation and anaerobic conditions that slow down the decomposition of organic matter |
Holds significant economic value in water treatment. Offers advantages through cross-system nutrient transfer for coral reefs, coastal protection, and the maintenance of water quality |
125 and 126 |
8 |
Mangrooves |
654 tons of C per km2 |
Anaerobic (low-oxygen) conditions in mangrove soils slow down the decomposition of organic matter, enhancing long-term carbon sequestration |
Found in both terrestrial and marine systems. They act as a natural buffer against coastal erosion and storm surges, providing protection to coastal communities |
127
|
9 |
Crop residue management |
— |
Crop residues must be managed for improved C sequestration in order to maximise soil organic matter. Additionally, crop residue application quantities and locations must be carefully considered, along with the best usage of N fertilisers |
Management of crop residues improves soil physical properties, chemical properties, fertility, microbial activity and soil productivity |
128
|
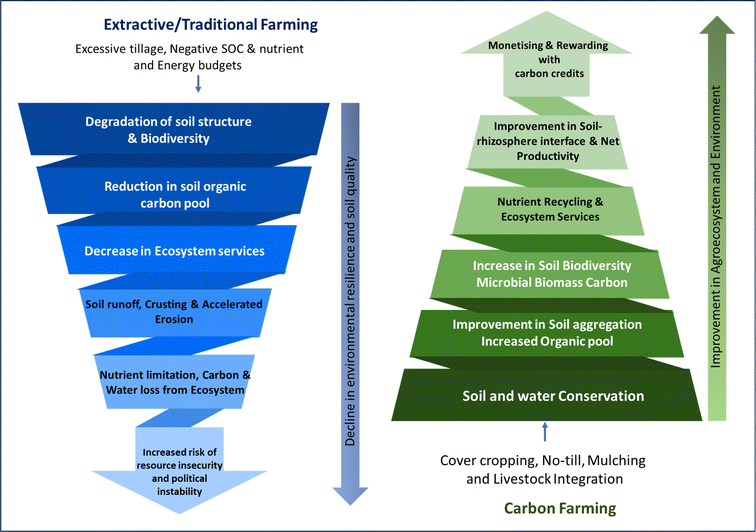 |
| Fig. 4 Scope of nature based solutions achieved through carbon farming over traditional farming practices. | |
4.2.1 No-till.
No-till farming is a practice that refrains from disturbing the soil and involves planting seeds in holes drilled directly into the ground.112 This approach has the competence to diminish GHGs from crop production by nearly a third while simultaneously increasing the capacity of soils to store carbon.113 In contrast to traditional farming techniques, which employ various tools for tilling, harrowing, sowing, and firming the ground, the soil creates air pockets filled with oxygen. However, with no-till farming, carbon accumulation during plant death and decomposition remains buried underground since it restricts the entry of excess oxygen into the soil, preventing contact with the bacteria.114 Research findings indicate that no-till farms have higher carbon storage in their soil over time, and the longer the soils remain undisturbed, the greater their capacity to hold carbon.115 Methane has more than 20 times greater heat-trapping capacity in the atmosphere, while nitrous oxide is roughly 300 times more efficient.116,117 Studies have shown that adopting no-till farming practices can significantly reduce overall emissions, with the most substantial decreases observed on farms that have implemented no-till for at least 15 years. By avoiding soil disturbance and minimizing the need for heavy machinery, farmers can significantly reduce diesel consumption, resulting in cost savings.129 For instance, in Haryana, India, adopting zero tillage was found to increase profits by nearly USD 97.5 per hectare due to lower labor, fuel, and tillage implement costs while reducing carbon emissions by 1.5 Mg per hectare per season.130 Comparatively, various tillage operations emit different amounts of carbon per hectare. Conventional practices such as mouldboard ploughing release 15 kg C/ha, heavy tandem disking and chisel ploughing release 8 kg C/ha, light tandem disking releases 6 kg C/ha, subsoiling releases 11 kg C/ha, cultivation releases 4 kg C/ha, and rotary hoeing releases 2 kg C/ha (11). By transitioning from conventional tillage to no-till farming, emissions can be reduced by approximately 30–35 kg C/ha per season.
4.2.2 Mulching.
Mulch plays a vital role in nutrient delivery as it actively participates in the carbon (C) and nitrogen (N) cycles, acting as a carbon sink.19 By using agricultural leftovers as mulch, crops can be benefitted from reduced cold stress.131 Furthermore, mulch significantly improves the physico-chemical characteristics of agricultural soils, leading to increased carbon sequestration levels ranging from 8 to 16 Mg per hectare per year.130 The application of mulch has proven effective in increasing soil organic matter (SOM) content. For instance, utilizing mulch has been shown to elevate the overall SOM from 1.26% to 1.50%.66 Particularly, mulch can substantially increase SOM and sequester CO2 in the top 0–5 cm layer of soil, providing multiple benefits for soil health and sustainability. For e.g. rice residue mulching improves long-term crop productivity by lowering weed pressure and root penetration resistance, increasing soil aeration, regulating temperature, reducing evapotranspiration and soil erosion, and enhancing the efficiency of water and nitrogen utilisation.72 In countries such as India, where there is a substantial production of agricultural wastes, these have the potential to serve as a significant biomass-based energy source.132 Despite the ideal prospect of generating electricity from biomass, Punjab has already established seven biomass power plants with six more in the process of construction.133 However, the overall capacity of these biomass power sources to utilize rice waste in the state is limited to approximately 10%.
4.2.3 Cover crops.
Cover crops are gaining popularity in the global effort to combat climate change, and they now occupy approximately 22 million acres of land, marking a 43% increase from previous years.134,135 It is estimated that 20 million acres of cover crops have the potential to sequester more than 66 million tons of CO2 equivalent annually, equivalent to the emissions of approximately 13 million vehicles. For example, cropland soils in the EU are estimated to lose about 7.4 million tons of carbon per year.23 Although certain crops are transformed into biofuel or utilized as animal fodder, the most ecologically advantageous approach is to let them decompose in the soil.136 Particularly, the cover cropping of olive orchards, vineyards, and almond orchards has been observed to exhibit the highest carbon sequestration rate, with values reaching up to 5.3 tons of carbon per hectare per year.137 There is growing acknowledgment among policymakers of the crucial role played by cover crops in mitigating GHG emissions by capturing and sequestering carbon dioxide (CO2) and nitrous oxide (N2O). The Biden Administration has put forth a significant budget of $28 billion for land conservation initiatives, with a dedicated $5 billion set aside for farmers and landowners who opt to implement cover crop planting.138 Considering that one carbon credit is equivalent to removing one ton of GHGs and the current maximum price for carbon credits is $20 per ton, this presents a substantial market opportunity ranging from approximately $2.6 billion to $3.3 billion. Projections indicate that by 2030, an estimated 40 to 50 million acres of land will be dedicated to cover crops, sequestering approximately 132 to 165 million tons of CO2.139
4.2.4 Peat/wet-lands.
Peatlands, comprising 50% of global wetlands, cover approximately 3% of the Earth's surface.140,141 These ecosystems contain an estimated 500–700 gigatons (Gt) of soil carbon, equivalent to 32% to 46% of all soil carbon and twice as much carbon found in all the global forests.142 Agricultural conversion, burning, and fuel mining has severely depleted and damaged these peatlands, leading to the release of significant volumes of GHGs, including CO2, N2O, and other trace gases.18,142 Peatlands absorb about 1% of all CO2 emissions produced by humans each year and store more carbon than all other vegetation types combined.143 Organic material, mainly from plants, accumulates under waterlogged conditions, creating an anaerobic environment and slowing down the decomposition process. As a result, the carbon remains trapped in the peat instead of being released into the atmosphere as CO2. Sundarbans, a vast mangrove forest covering approximately 10
000 square kilometers in India, serves as a crucial ecological entity by acting as a carbon sink, effectively sequestering 0.036 GT of CO2 from the atmosphere.144 In response to this environmental challenge, the Global Peatlands Initiative, led by UN Environment, aims to unite nations and partners to protect peatlands as the most significant terrestrial organic carbon resource worldwide, thereby mitigating emissions and revitalizing an essential ecosystem that provides numerous services for people, the planet, and climate.
4.2.5 Other factors.
The warming climate, elevated atmospheric CO2 levels, and nitrogen deposition have boosted gross primary productivity, encouraging vegetation growth and increasing nutrient demand.44 Though the effect of warming on phosphorus cycling remains a subject of debate, it is widely accepted that the current phosphorus supply may not be sufficient to meet the escalating demands of plants.145 Research showed that an acidogenic outlet rich in short-chain (volatile) fatty acids can be utilized as a soil amendment which functions as a phosphate solubilizing agent.146 Moreover, during vegetation succession, other rock-derived nutrients such as calcium become less abundant, as observed in the woodlands of central Africa.147 Consequently, it is essential to investigate whether the increased vegetation growth triggered by climate change exacerbates the scarcity of these nutrients. On the other hand, nitrogen (N) can be organically fixed, relieving the constraints associated with its availability. A higher nitrogen content in the 0–30 cm soil layer corresponds to increased CO2 sequestration.148 Plant organic carbon is introduced into the soil system primarily through two key processes: (i) the deposition of above-ground plant litter along with its leachates, including dissolved organic carbon carried into the soil via rainfall infiltration, and (ii) the decomposition of below-ground root litter and the release of organic compounds, commonly referred to as rhizodeposition.149,150 For instance, rotations involving dry peas produce significantly fewer GHG emissions than those involving oats and rapeseeds. This difference is because dry peas require less nitrogen fertilizer, especially in semi-arid farming environments.151,152 A reduction of only 5% in nitrogen (N) application rates in China's Shandong province could result in a 9% decrease in the region's contribution to global N2O emissions and a reduction of 0.35% in crop N2O emissions worldwide.153 Additionally, various agronomic strategies that collaborate with soil microbiota lessen the requirement for N application and decrease losses from soils, which helps to temper the overuse of synthetic N fertilizers.154 Self-induced in situ electrical stimuli at the rhizosphere of Vigna radiata and Cicer arietinum were shown to augment productivity as well as CO2 sequestration.155 Biochar, a carbon-enriched byproduct generated through the thermal degradation (pyrolysis) of biomass, contributes to climate change mitigation while concurrently offering energy benefits and improved soil fertility. Its climate-mitigation potential stems primarily from its nature which decelerates the release of photosynthetically captured carbon (C) into the atmosphere.119 On a global scale, the biochar application has the potential to offset a maximum reduction of 3.4–6.3 PgCO2e, with half of this amount being attributed to CO2 removal without jeopardizing food security, habitats, or soil conservation.118
4.3 Microalage
Microalgae are ubiquitous microorganisms that contribute to the production of approximately 50% of atmospheric oxygen, underscoring their crucial role in carbon capture and mitigation of global warming potential.20,156 It is approximated that within a cultivation area of 100
000 km2, microalgae have the capacity to sequester up to 2.35 gigatons of CO2.157 Eukaryotic algae and cyanobacteria have the ability to fix CO2 at rates ranging from 10 to 50 times faster than that of terrestrial plants. A notable characteristic is that approximately half of the dry weight of microalgal biomass consists of carbon.158 They are recognized as potential source for third-generation bioenergy/biofuels, offering a more efficient choice for carbon fixation compared to terrestrial plants due to their accelerated growth and quicker biomass production.127,159 A study model conducted in the USA validated that the pure CO2 generated from the corn ethanol production process could be employed in open pond algal cultivation. This approach has the potential to decrease GHG emissions, alleviate water stress, and eliminate the need for the energy-intensive carbon capture process in carbon capture and utilization (CCU).160 Azolla, a floating fern, has the potential to capture atmospheric nitrogen, sequester CO2 and support the bioeconomy when looped into a biorefinery.161
5. Crop management practices in India
India, with its vast agricultural land area of approximately 170 million hectares, holds a significant position in the global market and has immense potential for carbon storage and removal.162 The Energy Conservation (Amendment) Bill, passed into law on 8th August 2022 is to foster the expansion of the domestic carbon market, especially within the renewable energy sector. In response to nature's increasing unpredictability and variability, a paradigm shift was introduced, promoting the adoption of innovative agricultural practices. These practices include using climate-specific seeds, replacing chemical fertilizers with organic and natural manure, and incorporating leguminous plants to counteract the negative effects of chemicals while enhancing soil fertility with natural nutrients. Implementing these practices has significantly reduced water requirements, with nearly 40% less water needed for cultivation. Moreover, a crucial benefit has been observed in a considerable reduction in methane gas emissions, with a reduction of 22 kgs per hectare. The Indian state of Meghalaya is currently drafting a “carbon farming” act framework to promote sustainable agricultural techniques.162 The northeastern region of India has made remarkable strides in adopting organic and sustainable agricultural practices, with Sikkim becoming the world's first state to achieve full organic status in 2016.163 Cultyvate, a agri-tech startup, facilitates the shift of smallholder paddy/rice farmers in Punjab from conventional flood irrigation to the alternate wetting and drying (AWD) method. AWD not only slashes water usage by 40% but also mitigates methane emissions from submerged paddy fields by an impressive 50%.164 Implementing a system of rice intensification (SRI) practices substantially reduced CH4 emissions by more than four times compared to non-SRI system emissions.165,166 The procedures of intermittent flooding increase the oxygen flow to the roots, which reduces the production of aerenchyma, making the root system stronger and healthier and able to absorb more nutrients. For instance, employing green manure with N-fixing crops reduces the demand for N fertilizer and enhances soil structure and water retention, and prevents leaching and runoff.154
The burning of paddy stubble at the conclusion of the kharif season significantly exacerbates air pollution in north India. Startups that repurpose this paddy residue, such as those creating green bricks from paddy straw or Dharaksha, producing eco-friendly packaging materials from the stubble, present promising opportunities for carbon initiatives. Climate Sense is actively engaged in creating agro-forestry credits through collaboration with farmers in Maharashtra. They are involved in cultivating food forests on previously barren land, a practice that not only rejuvenates soil fertility but also effectively sequesters carbon. Despite these encouraging achievements, there are still issues, and Jharkhand is one of the most climate-vulnerable states in the nation, with a population of almost 32 million.167 Government initiatives such as the National Mission for Sustainable Agriculture (NMSA) and the Paramparagat Krishi Vikas Yojana (PKVY) play a pivotal role in advocating for regenerative agricultural practices. These programs offer incentives to farmers to adopt chemical-free farming techniques, thereby bolstering India's self-reliance.168
6. Augmenting carbon farming practices
6.1. Circular carbon economy (CCE)
The upsurge in economic activity and the rise in consumerism have increased the demand for raw materials, resulting in substantial GHG emissions released into the atmosphere. These emissions contribute to the risk of severe climate disruptions, threatening the environment and biodiversity. A transformative shift is imperative to steer the global trajectory toward achieving net-zero emissions by 2050, aligning with the 1.5 °C mark established during the Paris Agreement. Even if this ambitious goal is attained, the expenses incurred by the world economy due to climate change are forecasted to reach a staggering USD 54 trillion by the year 2100.169,170 On a global scale, the average per capita GHG emissions varied from 0.1 to 25 tons (t) of carbon dioxide equivalent (CO2e), with India and the Philippines registering the highest per capita emissions at approximately 1.7 tons (t per capita). From 1985 to 2015, India experienced a compound annual growth rate (CAGR) of 6% in its real GDP, while its resource consumption increased at a CAGR of 4%.171 To effectively curb carbon emissions using renewable energy sources, it is imperative to deploy techniques for low-carbon energy stowing and translation methods. The circular carbon economy (CCE) is a regenerative idea that depends on designing closed-loop systems and reusing materials and resources to reduce emissions, rely on renewable energy, and eliminate waste.172 The core tenet of CE is “reduce, reuse, and recycle,” which changes the conventional linear open paradigm of “resources/products/waste” into a cyclical one of “resources/products/wastes/resources”.50,173 Circular farming encompasses two distinct cycles, namely the biological and the technical cycles. The biological cycle is designed to extract value from waste materials, transforming them into novel and value-added products that contribute to crop cultivation, food processing, and energy generation.174,175 On the other hand, the technical cycle is applied to cropping technologies, emphasizing the adoption of techniques that encompass preservation, return, renewal, and reuse. These technological approaches enhance farming efficiency, concurrently curbing waste and lowering costs.81
Biomass carbon removal and storage (BiCRS) holds the promise of ushering the future where it facilitates the scope for producing an array of carbon-negative bioproducts, encompassing items such as wood products (Oriented Strand Board/OSB), bioplastics (e.g., polyethylene), biocarbon (biochar), and meticulously purified biogenic CO2 with subsequent geological sequestration.56,57,172 Over the past 5 to 10 years, the landscape of carbon-negative technologies in development has rapidly expanded and been streamlined into seven primary categories: bioenergy with carbon capture and storage (BECCS) (0.5–5 GtCO2), afforestation and reforestation (0.5–3.6 GtCO2), direct air carbon capture and storage (DACCS) (0.5–5 Gt CO2), enhanced weathering (2 to 4 GtCO2), biochar (0.5 to 2 GtCO2), and soil carbon sequestration (<5 Gt CO2).176 These technological avenues yield a cumulative annual CO2 removal of 9–24.6 Gt CO2, aligning with the targets established by the IPCC and the estimated cost ranges from $5 – $300 per tCO2.177 Utilizing regenerative cultivation techniques can curtail GHG emissions, sequester carbon within soils and plant material, and limit soil disruption.178 This approach also enhances soil structure, facilitating improved water retention and fostering biologically vibrant soils that independently generate fertility, thus diminishing the reliance on synthetic inputs.179 Circularizing agriculture revolves around three essential components that demand careful consideration. Firstly, it involves optimizing input utilization to prevent wastage. Secondly, it fosters sustainability across environmental, economic, and social domains. Thirdly, it emphasizes revitalizing structures that enable the efficient capture of nutrient cycles and the reduction of productivity losses.66 An essential element of a circular agriculture model involves integrating mixed crop-livestock methods, promoting organic farming, and adopting water recycling practices. This synergistic approach is vital in pursuing lower CO2 emissions and the sustainable use of natural resources.178 According to the European Commission (2018), CE principles could contribute to a 0.1% increase in GDP by 2030 and generate over 100
000 new job opportunities. Farmers stand to gain significantly from circularity, with potential profits of 3000 € per hectare. This approach enhances profitability and concurrently addresses pollution-related issues and the negative impacts associated with linear production processes.170 Due to lower carbon emissions, lesser costs, and decreased chain risk supply, the CE is predicted to save over $1 trillion annually globally.180 Additionally, the circular economy can safeguard about half of India's GDP value, worth about $600 billion. The practice of reusing and recycling agricultural waste holds the potential to invigorate local economies while concurrently mitigating environmental harm. Active involvement of stakeholders from both the public and private sectors within the bioeconomy is crucial in designing incentives that assign greater significance to the sequestration of biocarbon.181 In the United States, the bioeconomy is initially embracing the BiCRS concept, facilitated by various policy instruments such as California's low carbon fuel standard and the 45Q tax credit.172 Notably, adopting carbon-negative technology must extend beyond wealthy economies to meet the IPCC's 1.5 °C target, which probably calls for governments to be receptive to cross-cutting international agreements.182
6.2 Monetising carbon credits
Carbon credits serve as a means of exchange within the cap-and-trade regulations established by the Paris Agreement with “offset” CO2 emissions. The concept entails that companies responsible for CO2 emissions must either reduce their emissions (the “cap”) or financially support the efforts of entities such as farmers who can demonstrate their role in removing CO2 from the atmosphere (the “trade”).183 In conjunction with carbon taxes and subsidies, these carbon offsets and credits all fall under the umbrella of carbon pricing and are quantified in terms of carbon dioxide-equivalent (CO2e) units.184 The origin of the carbon credit concept can be traced back to the Kyoto Protocol, an international agreement designed to mitigate GHG emissions.183 A McKinsey analysis predicts that the demand for carbon credits will increase nearly 15-fold by 2030. This rise in demand offers a direct benefit to farmers as they can receive cash-based incentives for sequestering carbon on their lands.135 A farmer can earn around INR 780 for sequestering one carbon credit at prevailing market prices. However, larger corporations may offer even better rates, potentially up to INR 2000, when purchasing significant amounts of carbon credits directly from farmers.185 Farmers employing carbon farming practices can accumulate between one to four carbon credits per acre. This enhancement can be seen in traits like a larger capacity to hold water, a thinner layer of soil, better water infiltration, higher availability of nutrients, and a lower soil surface temperature.186 Nonetheless, the value of carbon credits can fluctuate significantly, contingent upon factors such as market dynamics, project nature, and geographical area. Technology breakthroughs are hastening the progression of detecting and corroborating carbon sequestration in the soil. To promote farmers' participation in carbon credit programs, governments at the state and federal levels might coordinate with already-existing cropping, regenerative, and organic farming practices.24 Within the European Union Common Agricultural Policy's agri-environmental and climate measures (AECM) framework, new private governance instruments, such as certification schemes for soil carbon sequestration, have recently emerged.23 Farmers now have the option to enroll some or all of their fields with commercial providers, which certify increases in soil organic carbon (SOC) achieved over a specified period. Subsequently, these farmers can sell these certificates to companies seeking to market their products as climate-neutral or to individuals aiming to offset their private GHG emissions. Certificates for carbon sequestration are already well established in the forestry sector187 where they are sold on the voluntary offset-market, and also contribute to public governance schemes, such as the Kyoto Protocol' s Clean Development Mechanism (CDM), or the REDD + (Reducing Emissions from Deforestation and Forest Degradation in Developing Countries) approach.188
6.3 Rewarding with carbon credits
Integrating technology and private sector involvement offers significant potential for enabling small-scale farmers to access carbon credit systems and other carbon sequestration opportunities.24 An international effort known as ‘4 per 1000,’ introduced during the 2015 Paris climate conference, underscored the significance of enhancing soil carbon.189 The initiative revealed that achieving a global annual increase of just 0.4% in soil carbon could effectively offset the new increase in CO2 emissions from fossil fuels for that particular year. In November 2021, the Nurture Farm, in partnership with the Indian Agricultural Research Institute, successfully implemented a crop residue management (CRM) initiative, convincing over 25
000 farmers across more than 420
000 acres of land to compost their rice chaff rather than resorting to burning it. This action resulted in preventing emissions equivalent to one million tonnes of CO2. The initiative involved the distribution of “PUSA,” a bio-enzyme that expedites crop residue decomposition and transforms it into compost, facilitating its implementation.190 As a result of this procedure, farmers can reduce reliance on commercial fertilizers since the soil quality is improved and its ability to store water is increased. Transforming agri-residues (straw, stubble and bagasse) into valuable biobased materials such as nanocellulose, lignin, and biohydrogen via biorefining techniques not only creates income opportunities for farmers but also reduces waste.191,192 Global business titans like McDonald's, Target, and Cargill are among those actively vowing to set aside money to encourage regenerative methods. Companies like CropIn leverage artificial intelligence to automate and offer guidance for farm-related decision-making.193 They achieved this by furnishing Indian farmers with mobile-based advisory dashboards that furnish valuable information on planting, soil conditions, seed treatment, and weather predictions. A recent report by the World Economic Forum highlights that by backing only one-fifth of EU farmers with environmentally sustainable agricultural methods, the European Union could potentially reduce GHG emissions by 6% annually and could lead to an annual boost in farmers' incomes ranging from €1.9 billion to €9.3 billion by 2030.
6.4 Startups
Startup incubators play a pivotal role in promoting awareness of carbon farming and assessing the carbon savings of products through scientific methods such as life cycle analysis (LCA). LCA allows startups to precisely quantify carbon negativity, ensuring transparency and preventing greenwashing. These incubators further facilitate the connection of outcomes to the carbon credit market, providing the patient capital essential for the development of carbon projects. Startups such as Dehaat and Bharat Rohan, in collaboration with FPOs and NGOs, routinely engage in activities like selling agricultural inputs or purchasing outputs, granting them direct connections to farmers engaged in or open to sustainable farming practices. Trace X in collaboration with Olam offers a blockchain-driven traceability solution to oversee their AWD and drip irrigation initiatives for Basmati rice. This comprehensive traceability enables Olam to qualify their rice project for carbon credits.194 Additionally, startups like Nurture Farms, Varaha, and Climes offer consultancy services, aiding both large and small entities in the agricultural sector. These startups assist in crafting carbon projects, accessing sustainable financing, and maneuvering through the intricate carbon markets.195 Beyond carbon farming efforts, startups such as CarbonCraft, which utilize CO2 in their building materials to sequester GHGs, and Zerund, which blends plastics into bricks, are fostering a more sustainable construction industry. Additionally, ventures like Krimanshi, focused on transforming wet waste into insect protein, and Hydrogreens, dedicated to generating low-emission cattle feed, are establishing compelling scenarios for reducing carbon emissions within the dairy sector as they expand.196 Two corporations – Nori and Indigo – are the two primary offset project producers for agricultural soil carbon offsets for the voluntary market currently in operation in the United States. Indigo began its carbon market program in 2019 and Nori in 2017.16
7. Conclusion
Carbon farming (CF) is emerging as a resilient method for offsetting carbon emissions by utilizing photosynthesis to sequester greenhouse gases from the atmosphere. It encompasses a wide array of sustainable agricultural practices, including agroforestry, cover cropping, rotational grazing, reduced chemical fertilizer usage, and minimal tillage. These practices not only enhance CO2 absorption but also provide additional soil conservation services such as improved fertility, disease resistance, erosion reduction, and increased productivity. Moreover, by integrating principles of the circular carbon economy (CCE), CF enables the monetization of carbon credits while encouraging startups to convert agricultural resources into bio-based products, thereby promoting sustainable development and contributing to a harmoniously balanced planet.
Author contributions
Dr S. Venkata Mohan: conceptualization, research methodology, supervision, funding acquisition, and original draft preparation; Shalini Singh and Boda Ravi Kiran: research methodology, data curation, and original draft preparation.
Conflicts of interest
The authors affirm that they have no known financial or interpersonal conflicts that would have appeared to impact the research presented in this study.
Acknowledgements
The authors duly acknowledge the Director, CSIR-IICT for support (Manuscript no. IICT/Pubs./2023/343).
References
- J. Rockström, J. Gupta, D. Qin, S. J. Lade, J. F. Abrams, L. S. Andersen, D. I. Armstrong McKay, X. Bai, G. Bala, S. E. Bunn and D. Ciobanu, Safe and just Earth system boundaries, Nature, 2023, 619, 1–10 CrossRef PubMed.
- C. Jansson, C. Faiola, A. Wingler, X. G. Zhu, A. Kravchenko, M. A. De Graaff, A. J. Ogden, P. P. Handakumbura, C. Werner and D. M. Beckles, Crops for carbon farming, Front. Plant Sci., 2021, 12, 636709 CrossRef PubMed.
- P. Friedlingstein, M. O'sullivan, M. W. Jones, R. M. Andrew, L. Gregor, J. Hauck, C. Le Quéré, I. T. Luijkx, A. Olsen, G. P. Peters and W. Peters, Global carbon budget 2022, Earth Syst. Sci. Data Discuss., 2022, 1–159 Search PubMed.
- R. Lal, Soil carbon sequestration impacts on global climate change and food security, Science, 2004, 304(5677), 1623–1627 CrossRef CAS PubMed.
-
IPCC, Summary for policymakers, in Global Warming of 1.5 °C. An IPCC Special Report on the Impacts of Global Warming of 1.5 °C above Pre- Industrial Levels and Related Global Greenhouse Gas Emission Pathways, in the Context of Strengthening the Global Response to the Threat of Climate Change, Sustainable Development, and Efforts to Eradicate Poverty, ed. V. Masson-Delmotte, P. Zhai, H.-O. Portner, D. Roberts, J. Skea, P. R. Shukla, A. Pirani, W. Moufouma-Okia, C. Pean, R. Pidcock, S. Connors, J. B. R. Matthews, Y. Chen, X. Zhou, M. I. Gomis, E. Lonnoy, T. Maycock, M. Tignor and T. Waterfield, World Meteorological Organization, Geneva, Switzerland, 2018, p. 32 Search PubMed.
-
IPCC Climate Change 2007: Synthesis Report. Contribution of Working Groups I, II and III to the Fourth Assessment Report of the Intergovernmental Panel on Climate Change, IPCC, Geneva, Switzerland, 2007 Search PubMed.
-
World Meteorological Organization [WMO], Greenhouse Gas Bulletin, World Meterological Organization, Geneva, Switzerland, 2006 Search PubMed.
- K. Richardson, W. Steffen, W. Lucht, J. Bendtsen, S. E. Cornell, J. F. Donges, M. Drüke, I. Fetzer, G. Bala, W. von Bloh and G. Feulner, Earth beyond six of nine planetary boundaries, Sci. Adv., 2023, 9(37), eadh2458 CrossRef PubMed.
-
Intergovernmental Panel on Climate Change [IPCC], Climate Change 2007: The Physical Science Basis, Cambridge University Press, Cambridge, UK, 2007 Search PubMed.
-
IPCC Climate Change 2007: Synthesis Report. Contribution of Working Groups I, II and III to the Fourth Assessment Report of the Intergovernmental Panel on Climate Change, IPCC, Geneva, Switzerland, 2007 Search PubMed.
- R. Lal, Soil carbon sequestration impacts on global climate change and food security, Science, 2004, 304(5677), 1623–1627 CrossRef CAS PubMed.
-
Compiled by MGSSI Based on FAO Data, (accessed on 19 January, 2023) Search PubMed.
- J. Sanderman, T. Hengl and G. J. Fiske, Soil carbon debt of 12,000 years of human land use, Proc. Natl. Acad. Sci. U. S. A., 2017, 114(36), 9575–9580, DOI:10.1073/pnas.1706103114.
-
U.S. Energy-Related Carbon Dioxide Fell by 2.8% in 2019, Slightly below 2017 Levels, Independent Statistics and Analysis – US Energy Information Administration (EIA), 5 May 2020 Search PubMed.
-
FAO, Global, regional and country trends 1990 – 2019. FAOSTAT analytical brief series no 28, in Land Use Statistics and Indicators Statistics, Rome, 2021 Search PubMed.
- C. T. Barbato and A. L. Strong, Farmer perspectives on carbon markets incentivizing agricultural soil carbon sequestration, npj Clim. Action, 2023, 2(1), 26 CrossRef.
-
FAO, Soils help to combat and adapt to climate change by playing a key role in the carbon cycle, in Fact Sheet 2015 International Year of Soils, 2015 Search PubMed.
- J. Leifeld and L. Menichetti, The underappreciated potential of peatlands in global climate change mitigation strategies, Nat. Commun., 2018, 9(1), 1071 CrossRef CAS PubMed.
- M. Sollen-Norrlin, B. B. Ghaley and N. L. J. Rintoul, Agroforestry benefits and challenges for adoption in Europe and beyond, Sustainability, 2020, 12(17), 7001 CrossRef CAS.
- P. Roy, A. K. Mohanty and M. Misra, Prospects of carbon capture, utilization
and storage for mitigating climate change, Environ. Sci.: Adv., 2023, 2(3), 409–423 CAS.
- M. Holka, J. Kowalska and M. Jakubowska, Reducing Carbon Footprint of Agriculture—Can Organic Farming Help to Mitigate Climate Change?, Agriculture, 2022, 12(9), 1383 CrossRef CAS.
- D. M. T. Denny, C. E. P. Cerri, M. R. Cherubin and H. L. Burnquist, Carbon Farming: Nature-Based Solutions in Brazil, Green Low-Carbon Econ., 2023, 1(3), 130–137 Search PubMed.
- C. Paul, B. Bartkowski, C. Dönmez, A. Don, S. Mayer, M. Steffens, S. Weigl, M. Wiesmeier, A. Wolf and K. Helming, Carbon farming: are soil carbon certificates a suitable tool for climate change mitigation?, J. Environ. Manage., 2023, 330, 117142 CrossRef PubMed.
- J. Leifeld, Carbon farming: climate change mitigation via non-permanent carbon sinks, J. Environ. Manage., 2023, 339, 117893 CrossRef PubMed.
- P. Smith, J. Adams, D. J. Beerling, T. Beringer, K. V. Calvin, S. Fuss, B. Griscom, N. Hagemann, C. Kammann, F. Kraxner and J. C. Minx, Land-management options for greenhouse gas removal and their impacts on ecosystem services and the sustainable development goals, Annu. Rev. Environ. Resour., 2019, 44, 255–286 CrossRef.
- S. Trumbore, Radiocarbon and soil carbon dynamics, Annu. Rev. Earth Planet. Sci., 2009, 37, 47–66 CrossRef CAS.
- N. H. Batjes, Total carbon and nitrogen in the soils of the world, Eurasian J. Soil Sci., 1996, 47, 151–163 CrossRef CAS.
- J. Lehmann, C. M. Hansel, C. Kaiser, M. Kleber, K. Maher, S. Manzoni, N. Nunan, M. Reichstein, J. P. Schimel, M. S. Torn and W. R. Wieder, Persistence of soil organic carbon caused by functional complexity, Nat. Geosci., 2020, 13(8), 529–534 CrossRef CAS.
- W. Amelung, D. Bossio, W. de Vries, I. Kögel-Knabner, J. Lehmann, R. Amundson, R. Bol, C. Collins, R. Lal, J. Leifeldand and B. Minasny, Towards a global-scale soil climate mitigation strategy, Nat. Commun., 2020, 11(1), 5427 CrossRef CAS PubMed.
- E. A. Davidson and I. A. Janssens, Temperature sensitivity of soil carbon decomposition and feedbacks to climate change, Nature, 2006, 440(7081), 165–173 CrossRef CAS PubMed.
- J. Jia, Z. Cao, C. Liu, Z. Zhang, L. I. Lin, Y. Wang, N. Haghipour, L. Wacker, H. Bao, T. Dittmar and M. J. Simpson, Climate warming alters subsoil but not topsoil carbon dynamics in alpine grassland, Global Change Biol., 2019, 25(12), 4383–4393 CrossRef PubMed.
- H. Wang, H. Liu, G. Cao, Z. Ma, Y. Li, F. Zhang, X. Zhao, X. Zhao, L. Jiang, N. J. Sanders and A. T. Classen, Alpine grassland plants grow earlier and faster but biomass remains unchanged over 35 years of climate change, Ecol. Lett., 2020, 23(4), 701–710 CrossRef PubMed.
- X. Feng, A. J. Simpson, K. P. Wilson, D. D. Williams and M. J. Simpson, Increased cuticular carbon sequestration and lignin oxidation in response to soil warming, Nat. Geosci., 2008, 1(12), 836–839 CrossRef CAS.
- N. Lu, H. Tian, B. Fu, H. Yu, S. Piao, S. Chen, Y. Li, X. Li, M. Wang, Z. Li and L. Zhang, Biophysical and economic constraints on China's natural climate solutions, Nat. Clim. Change, 2022, 12(9), 847–853 CrossRef.
- N. Fenner and C. Freeman, Drought-induced carbon loss in peatlands, Nat. Geosci., 2011, 4(12), 895–900 CrossRef CAS.
- N. Meyer, G. Welp and W. Amelung, The temperature sensitivity (Q10) of soil respiration: controlling factors and spatial prediction at regional scale based on environmental soil classes, Global Biogeochem. Cycles, 2018, 32(2), 306–323 CrossRef CAS.
- N. Fierer, B. P. Colman, J. P. Schimel and R. B. Jackson, Predicting the temperature dependence of microbial respiration in soil: a continental-scale analysis, Global Biogeochem. Cycles, 2006, 20(3), 1–10 CrossRef.
- J. M. Melillo, S. D. Frey, K. M. DeAngelis, W. J. Werner, M. J. Bernard, F. P. Bowles, G. Pold, M. A. Knorr and A. S. Grandy, Long-term pattern and magnitude of soil carbon feedback to the climate system in a warming world, Science, 2017, 358(6359), 101–105 CrossRef CAS PubMed.
- N. Verbrigghe, N. I. Leblans, B. D. Sigurdsson, S. Vicca, C. Fang, L. Fuchslueger, J. L. Soong, J. T. Weedon, C. Poeplau, C. Ariza-Carricondo and M. Bahn, Soil carbon loss in warmed subarctic grasslands is rapid and restricted to topsoil, Biogeosciences, 2022, 19(14), 3381–3393 CrossRef CAS.
- J. L. Soong, C. Castanha, C. E. Hicks Pries, N. Ofiti, R. C. Porras, W. J. Riley, M. W. Schmidt and M. S. Torn, Five years of whole-soil warming led to loss of subsoil carbon stocks and increased CO2 efflux, Sci. Adv., 2021, 7(21), eabd1343 CrossRef CAS PubMed.
- E. A. Schuur, A. D. McGuire, C. Schädel, G. Grosse, J. W. Harden, D. J. Hayes, G. Hugelius, C. D. Koven, P. Kuhry, D. M. Lawrence and S. M. Natali, Climate change and the permafrost carbon feedback, Nature, 2015, 520(7546), 171–179 CrossRef CAS PubMed.
- N. Wrage, G. L. Velthof, M. L. Van Beusichem and O. Oenema, Rol e of nitrifier denitrification in the production of nitrous oxide, Soil Biol. Biochem., 2001, 33(12–13), 1723–1732 CrossRef CAS.
- B. D. Stocker, R. Roth, F. Joos, R. Spahni, M. Steinacher, S. Zaehle, L. Bouwman and I. C. Prentice, Multiple greenhouse-gas feedbacks from the land biosphere under future climate change scenarios, Nat. Clim. Change, 2013, 3(7), 666–672 CrossRef CAS.
- K. G. Steinhäuser, A. Von Gleich, M. Große Ophoff and W. Körner, The necessity of a global binding framework for sustainable management of chemicals and materials—interactions with climate and biodiversity, Sustainable Chem., 2022, 3(2), 205–237 CrossRef.
- W. Huang, B. Z. Houlton, A. R. Marklein, J. Liu and G. Zhou, Plant stoichiometric responses to elevated CO2 vary with nitrogen and phosphorus inputs: evidence from a global-scale meta-analysis, Sci. Rep., 2015, 5(1), 18225 CrossRef CAS PubMed.
-
A. C. Abdullahi, C. Siwar, M. I. Shaharudin and I. Anizan, in Carbon Capture, Utilization and Sequestration, ed. R. K. Agarwal, IntechOpen, London, 2018 Search PubMed.
- K. Richardson, W. Steffen, W. Lucht, J. Bendtsen, S. E. Cornell, J. F. Donges, M. Drüke, I. Fetzer, G. Bala, W. von Bloh and G. Feulner, Earth beyond six of nine planetary boundaries, Sci. Adv., 2023, 9(37), 2458 CrossRef PubMed.
- M. L. Matthews, Engineering photosynthesis, nature's carbon capture machine, PLoS Biol., 2023, 21(7), e3002183 CrossRef CAS PubMed.
- R. V. Sreeharsha and S. Venkata Mohan, Symbiotic integration of bioprocesses to design a self-sustainable life supporting ecosystem in a circular economy framework, Bioresour. Technol., 2021, 326, 124712 CrossRef CAS PubMed.
- L. M. Alsarhan, A. S. Alayyar, N. B. Alqahtani and N. H. Khdary, Circular carbon economy (CCE): a way to invest CO2 and protect the environment, a review, Sustainability, 2021, 13(21), 11625 CrossRef CAS.
- A. A. Tak and U. B. Kakde, Analysis of carbon sequestration by dominant trees in urban areas of Thane city, Int. J. Glob. Warm., 2020, 20(1), 1–11 CrossRef.
- C. Jansson, C. Faiola, A. Wingler, X. G. Zhu, A. Kravchenko, M. A. De Graaff, A. J. Ogden, P. P. Handakumbura, C. Werner and D. M. Beckles, Crops for carbon farming, Front. Plant Sci., 2021, 12, 636709 CrossRef PubMed.
-
EEA: European Environment Agency, Annual European Union Greenhouse Gas Inventory 1990 – 2019 and Inventory Report 2021, 2021, https://tinyurl.com/baxw29er, (accessed 12 August 2022). accessed Search PubMed.
- T. G. Morais, R. F. M. Teixeira, C. Lauk, M. C. Theurl, W. Winiwarter, A. Mayer, L. Kaufmann, H. Haberl, T. Domingos and K. H. Erb, Agroecological measures and circular economy strategies to ensure sufficient nitrogen for sustainable farming, Glob. Environ. Change, 2021, 69, 102313 CrossRef.
- G. Santori, C. Charalambous, M. C. Ferrari and S. Brandani, Adsorption artificial tree for atmospheric carbon dioxide capture, purification and compression, Energy, 2018, 162, 1158–1168 CrossRef CAS.
- E. R. Mega, Apocalyptic’fires are ravaging a rare tropical wetland, Nature, 2020, 586(7827), 20–21 CrossRef PubMed.
- A. C. Encalada, A. S. Flecker, N. L. Poff, E. Suárez, G. A. Herrera-R, B. Ríos-Touma, S. Jumani, E. I. Larson and E. P. Anderson, A global perspective on tropical montane rivers, Science, 2019, 365(6458), 1124–1129 CrossRef CAS PubMed.
- D. Raha, J. A. Dar, P. K. Pandey, P. A. Lone, S. Verma, P. K. Khare and M. L. Khan, Variation in tree biomass and carbon stocks in three tropical dry deciduous forest types of Madhya Pradesh, India, Carbon Manage., 2020, 11(2), 109–120 CrossRef CAS.
- A. E. Russell, J. W. Raich, R. B. Arrieta, O. Valverde-Barrantes and E. González, Impacts of individual tree species on carbon dynamics in a moist tropical forest environment, Ecol. Appl., 2010, 20(4), 1087–1100 CrossRef PubMed.
- M. Teramoto, N. Liang, M. Takagi, J. Zeng and J. Grace, Sustained acceleration of soil carbon decomposition observed in a 6-year warming experiment in a warm-temperate forest in southern Japan, Sci. Rep., 2016, 6(1), 35563 CrossRef CAS PubMed.
- J. U. N. Koarashi, M. A. R. I. K. O. Atarashi-Andoh, S. Ishizuka, S. Miura, T. Saito and K. Hirai, Quantitative aspects of heterogeneity in soil organic matter dynamics in a cool-temperate Japanese beech forest: a radiocarbon-based approach, Global Change Biol., 2009, 15(3), 631–642 CrossRef.
- L. Heikkinen, M. Äijälä, M. Riva, K. Luoma, K. Dällenbach, J. Aalto, P. Aalto, D. Aliaga, M. Aurela, H. Keskinen and U. Makkonen, Long-term sub-micrometer aerosol chemical composition in the boreal forest: inter-and intra-annual variability, Atmos. Chem. Phys., 2020, 20(5), 3151–3180 CrossRef CAS.
- N. H. Batjes, Soil organic carbon stocks under native vegetation–Revised estimates for use with the simple assessment option of the Carbon Benefits Project system, Agric., Ecosyst. Environ., 2011, 142(3–4), 365–373 CrossRef.
- S. D. Keesstra, J. Bouma, J. Wallinga, P. Tittonell, P. Smith, A. Cerdà, L. Montanarella, J. N. Quinton, Y. Pachepsky, W. H. Van Der Putten and R. D. Bardgett, The significance of soils and
soil science towards realization of the United Nations Sustainable Development Goals, Soil, 2016, 2, 111–128 CrossRef.
-
B. R. Kiran, M. N. V. Prasad and S. Venkata Mohan, Farm to fork: sustainable agrifood systems, in Sustainable and Circular Management of Resources and Waste Towards a Green Deal, Elsevier Inc., USA. Paperback, 2023, pp. 25–38 Search PubMed.
- T. Rodríguez-Espinosa, I. Papamichael, I. Voukkali, A. P. Gimeno, M. B. A. Candel, J. Navarro-Pedreño, A. A. Zorpas and I. G. Lucas, Nitrogen management in farming systems under the use of agricultural wastes and circular economy, Sci. Total Environ., 2023, 876, 162666 CrossRef PubMed.
-
Circle Economy, The Circularity Gap Report 2019, 2019, available online, https://www.legacy.circularity-gap.world/2019, (accessed 11/3/23) Search PubMed.
- S. Hussain, S. Peng, S. Fahad, A. Khaliq, J. Huang, K. Cui and L. Nie, Rice management interventions to mitigate greenhouse gas emissions: a review, Environ. Sci. Pollut. Res., 2015, 22, 3342–3360 CrossRef PubMed.
-
FAO, FAO Rice Market Monitor, Food and Agriculture Organization of the United Nations, 2018, vol. 21, pp. 1–38 Search PubMed.
- Y. Lu and R. Conrad,
In situ stable isotope probing of methanogenic archaea in the rice rhizosphere, Science, 2005, 309, 1088–1090 CrossRef CAS PubMed.
- V. Gupta, K. A. Smemo, J. B. Yavitt, D. Fowle, B. Branfireun and N. Basiliko, Stable isotopes reveal widespread anaerobic methane oxidation across latitude and peatland type, Environ. Sci. Technol., 2013, 47, 8273–8279 CAS.
- G. Singh, M. K. Gupta, S. Chaurasiya, V. S. Sharma and D. Y. Pimenov, Rice straw burning: a review on its global prevalence and the sustainable alternatives for its effective mitigation, Environ. Sci. Pollut. Res., 2021, 28(25), 32125–32155 CrossRef CAS PubMed.
- N. K. Oanh, N. Upadhyay, Y. H. Zhuang, Z. P. Hao, D. V. S. Murthy, P. Lestari, J. T. Villarin, K. Chengchua, H. X. Co, N. T. Dung and E. S. Lindgren, Particulate air pollution in six Asian cities: spatial and temporal distributions, and associated sources, Atmos. Environ., 2006, 40(18), 3367–3380 CrossRef CAS.
- H. Arai, Y. Hosen, V. N. Pham Hong, N. T. Thi, C. N. Huu and K. Inubushi, Greenhouse gas emissions from rice straw burning and straw-mushroom cultivation in a triple rice cropping system in the Mekong Delta, Soil Sci. Plant Nutr., 2015, 61(4), 719–735 CrossRef CAS.
-
P. Kumar and L. Joshi, Pollution caused by agricultural waste burning and possible alternate uses of crop stubble: a case study of Punjab, in Knowledge Systems of Societies for Adaptation and Mitigation of Impacts of Climate Change, 2013, pp. 367–385 Search PubMed.
- M. I. Abdurrahman, S. Chaki and G. Saini, Stubble burning: effects on health & environment, regulations and management practices, Environ. Adv., 2020, 2, 100011 CrossRef.
- H. A. Le, Emission inventories of rice straw open burning in the Red River Delta of Vietnam: evaluation of the potential of satellite data, Environ. Pollut., 2020, 260, 113972 CrossRef CAS PubMed.
- Y. J. Na, I. H. Lee, S. S. Park and S. R. Lee, Effects of combination of rice straw with alfalfa pellet on milk productivity and chewing activity in lactating dairy cows, Asian-Australas. J. Anim. Sci., 2014, 27(7), 960 CrossRef CAS PubMed.
- S. Bhuvaneshwari, H. Hettiarachchi and J. M. Meegoda, Crop residue burning in India: policy challenges and potential solutions, Int. J. Environ. Res. Public Health, 2019, 16(5), 832 CrossRef CAS PubMed.
- B. W. Griscom, J. Adams, P. W. Ellis, R. A. Houghton, G. Lomax, D. A. Miteva, W. H. Schlesinger, D. Shoch, J. V. Siikamäki, P. Smith and P. Woodbury, Natural climate solutions, Proc. Natl. Acad. Sci. U. S. A., 2017, 114(44), 11645–11650 CrossRef CAS PubMed.
-
CEAT, Agriculture and the Circular Economy, 2021, available online, https://ceat.org.au/agriculture-and-the-circular-economy/, (accessed 11/3/23) Search PubMed.
- S. Menegat, A. Ledo and R. Tirado, Greenhouse gas emissions from global production and use of nitrogen synthetic fertilisers in agriculture, Sci. Rep., 2022, 12(1), 14490 CrossRef CAS PubMed.
- H. Tian, R. Xu, J. G. Canadell, R. L. Thompson, W. Winiwarter, P. Suntharalingam, E. A. Davidson, P. Ciais, R. B. Jackson, G. Janssens-Maenhout and M. J. Prather, A comprehensive quantification of global nitrous oxide sources and sinks, Nature, 2020, 586(7828), 248–256 CrossRef CAS PubMed.
- T. B. Sapkota, M. L. Jat, D. S. Rana, A. Khatri-Chhetri, H. S. Jat, D. Bijarniya, J. M. Sutaliya, M. Kumar, L. K. Singh, R. K. Jat and K. Kalvaniya, Crop nutrient management using Nutrient Expert improves yield, increases farmers' income and reduces greenhouse gas emissions, Sci. Rep., 2021, 11(1), 1564 CrossRef CAS PubMed.
-
F. Brentrup, J. Lammel, T. Stephani and B. Christensen, Updated carbon footprint values for mineral fertilizer from different world regions, in Global Food Challenges towards Sustainable Consumption and Production 17–19 Oct, Bangkok, 2018 Search PubMed.
- S. Friel, A. D. Dangour, T. Garnett, K. Lock, Z. Chalabi, I. Roberts, A. Butler, C. D. Butler, J. Waage, A. J. McMichael and A. Haines, Public health benefits of strategies to reduce greenhouse-gas emissions: food and agriculture, Lancet, 2009, 374(9706), 2016–2025 CrossRef PubMed.
-
J. Jackson, Y. Li, T. Murrells, N. assant, S. Sneddon, J. Thomas, G. Thistlethwaite, K. Dyson and L. Cardenas, Greenhouse Gas Inventories for England, Scotland, Wales and Northern Ireland: 2009, 1990–2007, AEA Search PubMed.
- A. J. McMichael, J. W. Powles, C. D. Butler and R. Uauy, Food, livestock production, energy, climate change, and health, Lancet, 2007, 370(9594), 1253–1263 CrossRef PubMed.
- P. Smith, D. Martino, Z. Cai, D. Gwary, H. Janzen, P. Kumar, B. McCarl, S. Ogle, F. O'Mara, C. Rice and B. Scholes, Greenhouse gas mitigation in agriculture, Philos. Trans. R. Soc., B, 2008, 363(1492), 789–813 CrossRef CAS PubMed.
-
T. Garnett, C. Godde, A. Muller, E. R. Smith, P. Smith, I. J. M. De Boer, E. zu Ermgassen, M. Herrero, C. E. Van Middelaar, C. Schader and H. H. E. Van Zanten, Grazed and confused?: ruminating on cattle, grazing systems, methane, nitrous oxide, the soil carbon sequestration question-and what it all means for greenhouse gas emissions, FCRN, University of Oxford, 2017, 1–127 Search PubMed.
- R. Lal, P. Smith, H. F. Jungkunst, W. J. Mitsch, J. Lehmann, P. R. Nair, A. B. McBratney, J. C. de Moraes Sá, J. Schneider, Y. L. Zinn and A. L. Skorupa, The carbon sequestration potential of terrestrial ecosystems, J. Soil Water Conserv., 2018, 73(6), 145A–152A CrossRef.
- P. Smith, J. F. Soussana, D. Angers, L. Schipper, C. Chenu, D. P. Rasse, N. H. Batjes, F. Van Egmond, S. McNeill, M. Kuhnert and C. Arias-Navarro, How to measure, report and verify soil carbon change to realize the potential of soil carbon sequestration for atmospheric greenhouse gas removal, Global Change Biol., 2020, 26(1), 219–241 CrossRef PubMed.
- K. Paustian, S. Collier, J. Baldock, R. Burgess, J. Creque, M. DeLonge, J. Dungait, B. Ellert, S. Frank, T. Goddard and B. Govaerts, Quantifying carbon for agricultural soil management: from the current status toward a global soil information system, Carbon Manage., 2019, 10(6), 567–587 CrossRef CAS.
- B. Minasny, B. P. Malone, A. B. McBratney, D. A. Angers, D. Arrouays, A. Chambers, V. Chaplot, Z. S. Chen, K. Cheng, B. S. Das and D. J. Field., Soil carbon 4 per mille, Geoderma, 2017, 292, 59–86 CrossRef.
- N. H. Batjes, Technologically achievable soil organic carbon sequestration in world croplands and grasslands, Land. Degrad. Dev, 2019, 30(1), 25–32 CrossRef.
- L. Wielopolski, A. Chatterjee, S. Mitra and R. Lal, In situ determination of soil carbon pool by inelastic neutron scattering: comparison with dry combustion, Geoderma, 2011, 160(3–4), 394–399 CrossRef CAS.
- G. S. Senesi and N. Senesi, Laser-induced breakdown spectroscopy (LIBS) to measure quantitatively soil carbon with emphasis on soil organic carbon. A review, Anal. Chim. Acta, 2016, 938, 7–17 CrossRef CAS PubMed.
- E. Maillard, B. G. McConkey and D. A. Angers, Increased uncertainty in soil carbon stock measurement with spatial scale and sampling profile depth in world grasslands: a systematic analysis, Agric., Ecosyst. Environ., 2017, 236, 268–276 CrossRef.
- A. Stevens, B. Van Wesemael, G. Vandenschrick, S. Touré and B. Tychon, Detection of carbon stock change in agricultural soils using spectroscopic techniques, Soil Sci. Soc. Am. J., 2006, 70(3), 844–850 CrossRef CAS.
- V. Bellon-Maurel and A. McBratney, Near-infrared (NIR) and mid-infrared (MIR) spectroscopic techniques for assessing the amount of carbon stock in soils–Critical review and research perspectives, Soil Biol. Biochem., 2011, 43(7), 1398–1410 CrossRef CAS.
-
A. E. Norton, Dynamic soil properties across a suburban landscape, MSc thesis, Iowa State University, Ankeny, Iowa, 2007.
- N. Lu, H. Tian, B. Fu, H. Yu, S. Piao, S. Chen, Y. Li, X. Li, M. Wang, Z. Li and L. Zhang, Biophysical and economic constraints on China's natural climate solutions, Nat. Clim. Change, 2022, 12(9), 847–853 CrossRef.
- G. Grassi, J. House, F. Dentener, S. Federici, M. den Elzen and J. Penman, The key role of forests in meeting climate targets requires science for credible mitigation, Nat. Clim. Change, 2017, 7(3), 220–226 CrossRef.
- B. B. Strassburg, A. Iribarrem, H. L. Beyer, C. L. Cordeiro, R. Crouzeilles, C. C. Jakovac., A. Braga Junqueira, E. Lacerda, A. E. Latawiec, A. Balmford and T. M. Brooks, Global priority areas for ecosystem restoration, Nature, 2020, 586(7831), 724–729 CrossRef CAS PubMed.
- F. Fleischman, S. Basant, A. Chhatre, E. A. Coleman, H. W. Fisc, D. Gupta, B. Güneralp, P. Kashwan, D. Khatri, R. Muscarella and J. S. Powers, Pitfalls of tree planting show why we need people-centered natural climate solutions, BioScience, 2020, 70(11), 947–950 Search PubMed.
- Y. K. Dwivedi, L. Hughes, A. K. Kar, A. M. Baabdullah, P. Grover, R. Abbas, D. Andreini, I. Abumoghli, Y. Barlette, D. Bunker and L. C. Kruse, Climate change and COP26: are digital technologies and information management part of the problem or the solution? An editorial reflection and call to action, Int. J. Inf. Manage, 2022, 63, 102456 CrossRef.
-
A. K. Padhee and A. M. Whitbread, Indian Agriculture: The Route Post-CoP 26, DownToEarth, 2022 Search PubMed.
- R. F. Follett, Soil management concepts and carbon sequestration in cropland soils, Soil Tillage Res., 2001, 61(1–2), 77–92 CrossRef.
- T. A. Ontl and L. A. Schulte, Soil carbon storage, Nature Education Knowledge, 2012, 3(10), 1–35 Search PubMed.
- S. Venkata Mohan, K. Amulya and J. A. Modestra, Urban biocycles–Closing metabolic loops for resilient and regenerative ecosystem: a perspective, Bioresour. Technol., 2020, 306, 123098 CrossRef CAS PubMed.
- S. Venkata Mohan, M. Hemalatha, K. Amulya, G. Velvizhi, P. Chiranjeevi, O. Sarkar, A. N. Kumar, K. V. Krishna, J. A. Modestra, S. Dahiya and D. K. Yeruva, Decentralized urban farming through keyhole garden: a case study with circular economy and regenerative perspective, Mater. Circ. Econ., 2020, 2, 1–12 Search PubMed.
-
B. O'Hara, No-Till Intensive Vegetable Culture: Pesticide-Free Methods for Restoring Soil and Growing Nutrient-Rich, High-Yielding Crops, Chelsea Green Publishing, 2020 Search PubMed.
-
No-Tillage Seeding in Conservation Agriculture, ed. C. J. Baker and K. E. Saxton, Published Jointly by Food and Agriculture Organization of the United Nations and Cabi Pub., 2007 Search PubMed.
-
D. R. Montgomery, Dirt: The Erosion of Civilizations, University of California Press, 2012 Search PubMed.
- D. S. Powlson, C. M. Stirling, M. L. Jat, B. G. Gerard, C. A. Palm, P. A. Sanchez and K. G. Cassman, Limited potential of no-till agriculture for climate change mitigation, Nat. Clim. Change, 2014, 4(8), 678–683 CrossRef.
- C. Li, S. Frolking and K. Butterbach-Bahl, Carbon sequestration in arable soils is likely to increase nitrous oxide emissions, offsetting reductions in climate radiative forcing, Clim. Change, 2005, 72(3), 321–338 CrossRef CAS.
-
T. R. Karl, J. M. Melillo and T. C. Peterson, Global Climate Change Impacts in the United States: A State of Knowledge Report from the US Global Change Research Program, Cambridge University Press, 2009 Search PubMed.
- J. Lehmann, A. Cowie, C. A. Masiello, C. Kammann, D. Woolf, J. E. Amonette, M. L. Cayuela, M. Camps-Arbestain and T. Whitman, biochar in climate change mitigation, Nat. Geosci., 2021, 14(12), 883–892 CrossRef CAS.
- D. Woolf, J. E. Amonette, F. A. Street-Perrott, J. Lehmann and S. Joseph, Sustainable biochar to mitigate global climate change, Nat. Commun., 2010, 1(1), 56 CrossRef PubMed.
- S. Rehman, S. S. Ijaz, M. A. Raza, A. M. U. Din, K. S. Khan, S. Fatima, T. Raza, S. Mehmood, A. Saeed and M. Ansar, Soil organic carbon sequestration and modeling under conservation tillage and cropping systems in a rainfed agriculture, Eur. J. Agron., 2023, 147, 126840 CrossRef CAS.
- H. Blanco-Canqui, Crop residue removal for bioenergy reduces soil carbon pools: how can we offset carbon losses?, BioEnergy Res., 2013, 6, 358–371 CrossRef CAS.
- F. Tanneberger, L. Appulo, S. Ewert, S. Lakner, N. Ó Brolcháin, J. Peters and W. Wichtmann, The power of nature-based solutions: how peatlands can help us to achieve key EU sustainability objectives, Adv. Sustainable Syst., 2021, 5(1), 2000146 CrossRef CAS.
- K. Paustian, J. Lehmann, S. Ogle, D. Reay, G. P. Robertson and P. Smith, Climate-smart soils, Nature, 2016, 532(7597), 49–57 CrossRef CAS PubMed.
-
A. J. Eagle, L. R. Henry, L. P. Olander, K. Haugen-Kozyra, N. Millar and G. P. Robertson, Greenhouse gas mitigation potential of agricultural land management in the United States, A Synthesis of the Literature. Technical Working Group on Agricultural Greenhouse Gases (T-AGG) Report, 2010 Search PubMed.
-
IPCC Intergovernmental Panel on Climate Change Guidelines for National Greenhouse Gas Inventories, in Agriculture, Forestry and Other Land Use (AFOLU), ed. Eggleston S., Buendia L., Miwa K., Ngara T. and Tanabe K., Institute for Global Environmental Strategies, 2006, vol. 4, https://www.ipcc-nggip.iges.or.jp/public/2006gl/vol4.html Search PubMed.
-
Ipcc, IPCC, Guidelines for National Greenhouse Gas Inventories. Prepared by the National Greenhouse Gas Inventories Programme, ed. Eggleston H. S., Buendia L., Miwa K., Ngara T. and Tanabe K., IGES, Japan, 2006 Search PubMed.
- I. Y. López-Pacheco, L. I. Rodas-Zuluaga, S. Fuentes-Tristan, C. Castillo-Zacarías, J. E. Sosa-Hernández, D. Barceló, H. M. Iqbal and R. Parra-Saldívar, Phycocapture of CO2 as an option to reduce greenhouse gases in cities: carbon sinks in urban spaces, J. CO2 Util., 2021, 53, 101704 CrossRef.
- S. Sarkar, M. Skalicky, A. Hossain, M. Brestic, S. Saha, S. Garai, K. Ray and K. Brahmachari, Management of crop residues for improving input use efficiency and agricultural sustainability, Sustainability, 2020, 12(23), 9808 CrossRef.
- C. S. Snyder, T. W. Bruulsema, T. L. Jensen and P. E. Fixen, Review of greenhouse gas emissions from crop production systems and fertilizer management effects, Agric., Ecosyst. Environ., 2009, 133(3–4), 247–266 CrossRef CAS.
- M. S. Kahlon, R. Lal and M. Ann-Varughese, Twenty two years of tillage and mulching impacts on soil physical characteristics and carbon sequestration in Central Ohio, Soil Tillage Res., 2013, 126, 151–158 CrossRef.
- D. P. Roberts and A. K. Mattoo, Sustainable agriculture—Enhancing environmental benefits, food nutritional quality and building crop resilience to abiotic and biotic stresses, Agriculture, 2018, 8(1), 8 CrossRef.
-
J. Allen, K. S. Pascual, R. R. Romasanta, M. Van Trinh, T. Van Thach, N. Van Hung, B. O. Sander and P. Chivenge, Rice straw management effects on greenhouse gas emissions and mitigation options, in Sustainable Rice Straw Management, 2020, pp. 145–159 Search PubMed.
-
N. I. Mohammed, N. Kabbashi and A. Alade, Significance of agricultural residues in sustainable biofuel development, in Agricultural Waste and Residues, 2018, pp. 71–88 Search PubMed.
- J. L. Vicente-Vicente, R. García-Ruiz, R. Francaviglia, E. Aguilera and P. Smith, Soil carbon sequestration rates under Mediterranean woody crops using recommended management practices: a meta-analysis, Agric., Ecosyst. Environ., 2016, 235, 204–214 CrossRef.
- J. Morizet-Davis, N. A. Marting Vidaurre, E. Reinmuth, E. Rezaei-Chiyaneh, V. Schlecht, S. Schmidt, K. Singh, R. Vargas-Carpintero, M. Wagner and M. von Cossel, Ecosystem Services at the Farm Level—Overview, Synergies, Trade-Offs, and Stakeholder Analysis, Glob. Chall., 2023, 2200225 CrossRef PubMed.
- M. Abdalla, A. Hastings, K. Cheng, Q. Yue, D. Chadwick, M. Espenberg, J. Truu, R. M. Rees and P. Smith, A critical review of the impacts of cover crops on nitrogen leaching, net greenhouse gas balance and crop productivity, Global Change Biol., 2019, 25(8), 2530–2543 CrossRef PubMed.
-
Z. U. R. Farooqi, M. Sabir, N. Zeeshan, K. Naveed and M. M. Hussain, Enhancing carbon sequestration using organic amendments and agricultural practices, in Carbon Capture, Utilization and Sequestration, 2018, vol. 17 Search PubMed.
-
R. Hooper, How to Spend a Trillion Dollars: The 10 Global Problems We Can Actually Fix, Profile Books, 2021 Search PubMed.
- N. E. Tautges, J. L. Chiartas, A. C. Gaudin, A. T. O'Geen, I. Herrera and K. M. Scow, Deep soil inventories reveal that impacts of cover crops and compost on soil carbon sequestration differ in surface and subsurface soils, Global Change Biol., 2019, 25(11), 3753–3766 CrossRef PubMed.
- D. J. Cooper, E. C. Wolf, C. Colson, W. Vering, A. Granda and M. Meyer, Alpine peatlands of the Andes, cajamarca, Peru, Arct. Antarct. Alp. Res., 2010, 42(1), 19–33 CrossRef.
-
H. Joosten, M. L. Tapio-Biström and S. Tol, Peatlands: Guidance for Climate Change Mitigation through Conservation, Rehabilitation and Sustainable Use, Food and Agriculture Organization of the United Nations, Rome, 2012 Search PubMed.
-
H. Joosten, Peatlands, Climate Change Mitigation and Biodiversity Conservation: An Issue Brief on the Importance of Peatlands for Carbon and Biodiversity Conservation and the Role of Drained Peatlands as Greenhouse Gas Emission Hotspots, Nordisk Ministerråd, Copenhagen, 2015, p. 14 Search PubMed.
- C. Rumpel, F. Amiraslani, L. S. Koutika, P. Smith, D. Whitehead and E. Wollenberg, Put more carbon in soils to meet paris climate pledges, Nature, 2018, 564(7734), 32–34 CrossRef CAS PubMed.
- A. Chanda and A. Akhand, Challenges towards the Sustainability and Enhancement of the Indian Sundarban Mangrove's Blue Carbon Stock, Life, 2023, 13(8), 1787 CrossRef PubMed.
- P. M. Vitousek, S. Porder, B. Z. Houlton and O. A. Chadwick, Terrestrial phosphorus limitation: mechanisms, implications, and nitrogen–phosphorus interactions, Ecol. Appl., 2010, 20(1), 5–15 CrossRef PubMed.
- O. Sarkar, S. Chatterjee and S. Venkata Mohan, Acidogenic outlet from biohydrogen reactor as phosphate solubilizing agent for integrated organic farming, J. Cleaner Prod., 2019, 208, 490–498 CrossRef CAS.
- M. Bauters, I. A. Janssens, D. Wasner, S. Doetterl, P. Vermeir, M. Griepentrog, T. W. Drake, J. Six, M. Barthel, S. Baumgartner and K. Van Oost, Increasing calcium scarcity along Afrotropical forest succession, Nat. Ecol. Evol., 2002, 6(8), 1122–1131 CrossRef PubMed.
- C. Bateni, M. Ventura, G. Tonon and A. Pisanelli, Soil carbon stock in olive groves agroforestry systems under different management and soil characteristics, Agrofor. Syst., 2021, 95, 951–961 CrossRef.
-
IPCC Intergovernmental Panel on Climate Change Guidelines for National Greenhouse Gas Inventories, in Agriculture, Forestry and Other Land Use (AFOLU), ed. Eggleston S., Buendia L., Miwa K., Ngara T. and Tanabe K., Institute for Global Environmental Strategies, 2006, vol. 4, https://www.ipcc-nggip.iges.or.jp/public/2006gl/vol4.html Search PubMed.
- Y. T. Gan, C. A. Campbell, H. H. Janzen, R. L. Lemke, P. Basnyat and C. L. McDonald, Carbon input to Soil from oilseed and pulse crops on the Canadian prairies, Agric., Ecosyst. Environ., 2009, 132(3–4), 290–297 CrossRef CAS.
- M. Rajaniemi, H. Mikkola and J. Ahokas, Greenhouse gas emissions from oats, barley, wheat and rye production, Agron. Res., 2011, 9, 189–195 Search PubMed.
- K. Tang, A. Hailu, M. E. Kragt and C. Ma, The response of broadacre mixed crop-livestock farmers to agricultural greenhouse gas abatement incentives, Agric. Syst., 2018, 160, 11–20 CrossRef.
- R. T. Venterea, A. D. Halvorson, N. Kitchen, M. A. Liebig, M. A. Cavigelli, S. J. D. Grosso, P. P. Motavalli, K. A. Nelson, K. A. Spokas, B. P. Singh and C. E. Stewart, Challenges and opportunities for mitigating nitrous oxide emissions from fertilized cropping systems, Front. Ecol. Environ., 2012, 10(10), 562–570 CrossRef PubMed.
- M. Lori,
et al., Organic farming enhances soil microbial abundance and activity—A meta-analysis and meta-regression, PLoS One, 2017, 12, e0180442 CrossRef PubMed.
- S. Venkata Mohan and D. K. Yeruva, In situ self-induced electrical stimulation to plants: modulates morphogenesis, photosynthesis and gene expression in Vigna radiata and Cicer arietinum, Bioelectrochemistry, 2023, 154, 108550 CrossRef CAS PubMed.
-
R. Williams, Microscopic Algae Produce Half the Oxygen We Breathe, ABC News, October 2013, available online, https://www.abc.Net.au/radionational/programs/scienceshow/microscopic-algae-produce-half-the-oxygen-we-breathe/5041338, (accessed on 19 May 2019) Search PubMed.
- B. Zhao and Y. Su, Macro assessment of microalgae-based CO2 sequestration: environmental and energy effects, Algal Res., 2020, 51, 102066 CrossRef.
- B. R. Kiran and S. Venkata Mohan, Microalgal cell biofactory—therapeutic, nutraceutical and functional food applications, Plants, 2021, 10(5), 836 CrossRef PubMed.
- J. S. Sravan, M. Hemalatha and S. Venkata Mohan, Cascading Integration of Electrofermentation and Photosynthesis—Low-Carbon Biorefinery in Closed Loop Approach, Adv. Sustainable Syst., 2023, 2300142 CrossRef CAS.
- L. Ou, S. Banerjee, H. Xu, A. M. Coleman, H. Cai, U. Lee, M. S. Wigmosta and T. R. Hawkins, Utilizing high-purity carbon dioxide sources for algae cultivation and biofuel production in the United States: opportunities and challenges, J. Cleaner Prod., 2021, 321, 128779 CrossRef CAS.
- M. Hemalatha, O. Sarkar and S. Venkata Mohan, Self-sustainable azolla-biorefinery platform for valorization of biobased products with circular-cascading design, Chem. Eng. J., 2019, 373, 1042–1053 CrossRef CAS.
- R. B. Jackson, E. G. Jobbaagy and R. Avissar,
et al., Trading water for carbon with biological carbon sequestration, Science, 2005, 310, 1944e1947 Search PubMed.
- M. Yadav, Towards a healthier nation: organic farming and government policies in India, Int. J. Adv. Res. Dev., 2017, 2(5), 153–159 Search PubMed.
-
T. Searchinger and T. K. Adhya, Wetting and Drying: Reducing Greenhouse Gas Emissions and Saving Water from Rice Production, 2015 Search PubMed.
- A. Dobermann, A critical assessment of the system of rice intensification (SRI), Agric. Syst., 2004, 79(3), 261–281 CrossRef.
-
N. Raut, B. K. Sitaula, R. M. Bajracharya and S. Karki, Methane emission from unsustainable crop production in Nepal, system of rice intensification as an option for mitigation, in Climate Change and Soil Interactions, Elsevier, 2020, pp. 37–49 Search PubMed.
- J. Busby, T. G. Smith, N. Krishnan, C. Wight and S. Vallejo-Gutierrez, In harm's way: climate security vulnerability in Asia, World Dev., 2018, 112, 88–118 CrossRef.
-
https://pib.gov.in
.
-
IPCC, Summary for policymakers, in Global Warming of 1.5 °C. An IPCC Special Report on the Impacts of Global Warming of 1.5 °C above Pre- Industrial Levels and Related Global Greenhouse Gas Emission Pathways, in the Context of Strengthening the Global Response to the Threat of Climate Change, Sustainable Development, and Efforts to Eradicate Poverty, ed. V. Masson-Delmotte, P. Zhai, H.-O. Portner, D. Roberts, J. Skea, P. R. Shukla, A. Pirani, W. Moufouma-Okia, C. Pean, R. Pidcock, S. Connors, J. B. R. Matthews, Y. Chen, X. Zhou, M. I. Gomis, E. Lonnoy, T. Maycock, M. Tignor and T. Waterfield, World Meteorological Organization, Geneva, Switzerland, 2018, p. 32 Search PubMed.
-
E. Macarthur and H. Heading, How the Circular Economy Tackles Climate Change, Ellen MacArthur Found, 2019, vol. 1, pp. 1–71 Search PubMed.
- D. Satterthwaite, The contribution of cities to global warming and their potential contributions to solutions, Environ. Urban. ASIA, 2010, 1(1), 1–12 CrossRef.
- J. Dees, K. Oke, H. Goldstein, S. T. McCoy, D. L. Sanchez, A. J. Simon and W. Li, Cost and Life Cycle Emissions of Ethanol Produced with an Oxyfuel Boiler and Carbon Capture and Storage, Environ. Sci. Technol., 2023, 57(13), 5391–5403 CrossRef CAS PubMed.
- R. Katakojwala, K. Advaitha, J. K. Patil and S. Venkata Mohan, Circular Economy Induced Resilience in Socio-Ecological Systems: an Ecolonomic Perspective, Mater. Circ. Econ., 2023, 5(1), 4 CrossRef.
- J. Hetland, P. Yowargana, S. Leduc and F. Kraxner, Carbon-negative emissions: systemic impacts of biomass conversion: a case study on CO2 capture and storage options, Int. J. Greenhouse Gas Control, 2016, 49, 330–342 CrossRef CAS.
- S. Dahiya, R. Katakojwala, S. Ramakrishna and S. Venkata Mohan, Biobased products and life cycle assessment in the context of circular economy and sustainability, Mater. Circ. Econ., 2020, 2, 1–28 Search PubMed.
- J. C. Minx, W. F. Lamb, M. W. Callaghan, S. Fuss, J. Hilaire, F. Creutzig, T. Amann, T. Beringer, W. de Oliveira Garcia, J. Hartmann and T. Khanna, Negative emissions—Part 1: research landscape and synthesis, Environ. Res. Lett., 2018, 13(6), 063001 CrossRef.
- J. C. Minx, W. F. Lamb, M. W. Callaghan, S. Fuss, J. Hilaire, F. Creutzig, T. Amann, T. Beringer, W. de Oliveira Garcia, J. Hartmann and T. Khanna, Negative emissions—Part 1: research landscape and synthesis, Environ. Res. Lett., 2018, 13(6), 063001 CrossRef.
- J. F. Velasco-Muñoz, J. A. Aznar-Sánchez, B. López-Felices and I. M. Román-Sánchez, Circular economy in agriculture. An analysis of the state of research based on the life cycle, Sustain. Prod. Consum., 2022, 34, 257–270 CrossRef.
-
W. R. Stahel, The Performance Economy, Palgrave Macmillan, Basingstoke, New York, 2nd edn, 2010 Search PubMed.
- J. Fiksel, P. Sanjay and K. Raman, Steps toward a resilient circular economy in India, Clean Technol. Environ. Policy, 2021, 23, 203–218 CrossRef PubMed.
- M. He, Z. Xu, D. Hou, B. Gao, X. Cao, Y. S. Ok, J. Rinklebe, N. S. Bolan and D. C. Tsang, Waste-derived biochar for water pollution control and sustainable development, Nat. Rev. Earth Environ., 2022, 3(7), 444–460 CrossRef CAS.
- M. Honegger and D. Reiner, The political economy of negative emissions technologies: consequences for international policy design, Clim. Policy, 2018, 18(3), 306–321 CrossRef.
- L. Xu, Y. A. Solangi and R. Wang, Evaluating and prioritizing the carbon credit financing risks and strategies for sustainable carbon markets in China, J. Cleaner Prod., 2023, 137677 CrossRef.
- T. Cadman and R. Hales, COP26 and a Framework for Future Global Agreements on Carbon Market Integrity, Int. J. Soc. Qual., 2022, 12(1), 76–99 Search PubMed.
-
C. Blaufelder, C. Levy, P. Mannion and D. Pinner, A Blueprint for Scaling Voluntary Carbon Markets to Meet the Climate Challenge, McKinsey, 2021, vol. 5, viewed Search PubMed.
- S. Babu, R. Singh, R. Avasthe, S. Kumar, S. S. Rathore, V. K. Singh, M. A. Ansari, D. Valente and I. Petrosillo, Soil carbon dynamics under organic farming: impact of tillage and cropping diversity, Ecol. Indic., 2023, 147, 109940 CrossRef CAS.
- K. R. M. Suiseeya and S. Caplow, In pursuit of procedural justice: lessons from an analysis of 56 forest carbon project designs, Glob. Environ. Change, 2013, 23(5), 968–979 CrossRef.
- J. Otto, Precarious participation: assessing inequality and risk in the carbon credit commodity chain, Ann. Assoc. Am. Geogr., 2019, 109(1), 187–201, DOI:10.1080/24694452.2018.1490167.
-
C. W. Nam, World Economic Outlook for 2022 and 2023, in CESifo Forum, ifo Institut – Leibniz-Institut für Wirtschaftsforschung an der Universität München, München, 2022, vol. 23, no. 3, pp. 50–51 Search PubMed.
- S. Korav, G. A. Rajanna, D. B. Yadav, V. Paramesha, C. M. Mehta, P. K. Jha, S. Singh and S. Singh, Impacts of Mechanized Crop Residue Management on Rice-Wheat Cropping System—A Review, Sustainability, 2022, 14(23), 15641 CrossRef.
- R. Katakojwala and S. Venkata Mohan, Microcrystalline cellulose production from sugarcane bagasse: sustainable process development and life cycle assessment, J. Cleaner Prod., 2020, 249, 119342 CrossRef CAS.
- R. Katakojwala and S. Venkata Mohan, Multi-product biorefinery with sugarcane bagasse: process development for nanocellulose, lignin and biohydrogen production and lifecycle analysis, Chem. Eng. J., 2022, 446, 137233 CrossRef CAS.
-
http://www.cropin.com
.
-
http://www.olamgroup.com
.
-
https://nurture.farm/endtheburn/
.
- S. Jagtap, G. Garcia-Garcia, L. Duong, M. Swainson and W. Martindale, Codesign of food system and circular economy
approaches for the development of livestock feeds from insect larvae, Foods, 2021, 10(8), 1701 CrossRef CAS PubMed.
|
This journal is © The Royal Society of Chemistry 2024 |
Click here to see how this site uses Cookies. View our privacy policy here.