DOI:
10.1039/D4BM00941J
(Review Article)
Biomater. Sci., 2025,
13, 1114-1130
Biomaterial-based strategies for primary human corneal endothelial cells for therapeutic applications: from cell expansion to transplantable carrier
Received
17th July 2024
, Accepted 12th January 2025
First published on 13th January 2025
Abstract
The treatment of corneal blindness due to corneal diseases and injuries often requires the transplantation of healthy cadaveric corneal endothelial graft tissue to restore corneal clarity and visual function. However, the limited availability of donor corneas poses a significant challenge in meeting the demand for corneal transplantation. As a result, there is a growing interest in developing strategies alleviate this unmet need, and one of the postulated approaches is to isolate and expand primary human corneal endothelial cells (HCECs) in vitro for use in cell therapy. This review summarizes the recent advancements in the expansion of HCECs using biomaterials. Two principal biomaterial-based approaches, including extracellular matrix (ECM) coating and functionalized synthetic polymers, have been investigated to create an optimal microenvironment for the expansion and maintenance of corneal endothelial cells (CECs). This review highlights the challenges and opportunities in expanding primary HCECs using biomaterials. It emphasizes the importance of optimizing biomaterial properties, cell culture conditions, and the roles of biophysical cues to achieve efficient expansion and functional maintenance of CECs. Biomaterial-based strategies hold significant promise for expanding primary HCECs and improving the outcomes of CEC transplantation. The integration of biomaterials as cell culture substrates and transplantable carriers offers a comprehensive approach to address the limitations associated with current corneal tissue engineering techniques.
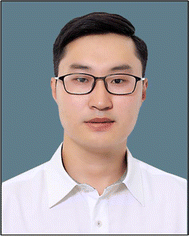 Myagmartsend Enkhbat | Mr Myagmartsend Enkhbat is currently pursuing a Ph.D. in Biomedical Engineering at Shanghai Jiao Tong University, China. He earned a Bachelor of Science in Biomedicine from the Mongolian National University of Medical Sciences. Subsequently, he completed a Master's degree in Biomedical Engineering at Taipei Medical University and a second Master's degree in Chemical Engineering at the University of Waterloo. His research interests include in vitro disease modeling, cell apoptosis, biomaterials, stem cells, and organoid technology. |
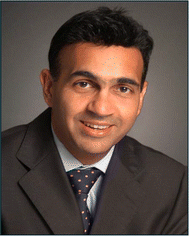 Jodhbir S. Mehta | Prof Jodhbir Mehta is Executive Director of the Singapore Eye Research Institute (SERI), Distinguished Professor, Corneal & External Eye Disease and Refractive Department at Singapore National Eye Centre (SNEC). He has won 74 prestigious awards nationally and internationally for his clinical and research work. His research work has generated 18 patents, 5 of which have been commercialized and licensed to companies. He has published >520 peer-reviewed publications and 24 book chapters, with a H-index 76. He has been invited to speak at >300 international conferences and given 25 named/keynote lectures. His work has been sited >19 250 times. He has trained 28 international fellows and 8 PhD students, 5 Masters students. |
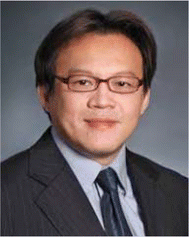 Gary S. L. Peh | Assistant Professor Gary Peh is Junior Principal Investigator at the Singapore Eye Research Institute (SERI). He earned his Ph.D. from Monash University, Australia, where his work using small molecules. After returning to Singapore, he transitioned his research focus towards ophthalmology, specifically in the field of corneal regenerative cell therapies. His current work centers on the process development and clinical translation of using primary corneal endothelial cells for the treatment of corneal endothelial dysfunction. His academic contributions include over 75 peer-reviewed publications, with a H-index 31. |
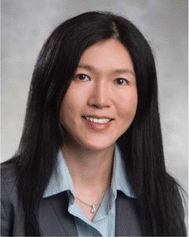 Evelyn K. F. Yim | Professor Evelyn Yim is Professor and University Research Chair in the Department of Chemical Engineering at the University of Waterloo in Canada. Professor Yim and her group are interested to study cell-biomaterial interactions, and apply the knowledge to direct stem cell growth and tissue engineering for vascular and corneal regeneration applications. She received her PhD at the Johns Hopkins University. She started her academic career at the National University of Singapore, and she joined University of Waterloo in 2016. She has published >100 peer-reviewed publications, and she has trained 13 PhD students, 17 master students and 12 postdoctoral fellows. |
Introduction
The human corneal endothelium (CE) is essential for the regulation of corneal hydration, maintaining the appropriate thickness of the cornea, and keeping it transparent. Substantial loss of the human corneal endothelial cell (HCECs) will affect corneal deturgescence, resulting in increased stromal thickness, loss of visual acuity, and when left untreated, will lead to corneal blindness. Currently, corneal transplantation using a cadaveric donor cornea is the gold standard treatment for corneal blindness.1 Within the human eye, HCECs are principally non-proliferative, mitotically inactive and arrested at the G1 phase of cell cycle,2,3 but retain the capacity to proliferate in vitro.4,5 In the field of corneal transplantation, the shortage of suitable donor corneas poses a significant challenge on a global scale. Particularly, considering that corneal transplantation is a one-to-one surgery where one donor cornea is required for each recipient.6 The demand for corneal transplants far exceeds the availability of donor corneas, leading to long waiting lists and delayed treatment for patients with corneal blindness. Various factors contribute to this shortage, including limited donor pool, logistical constraints in corneal procurement and transportation, and the need for meticulous screening to ensure the safety and suitability of donor corneas. As a result, alternative approaches to address this unmet need have gained increasing attention. One such promising avenue is the utilization of HCECs as an alternative source for cell therapy.7–9
By harnessing the proliferative potential of these cells, researchers are exploring innovative strategies to expand and transplant HCECs, offering a potential solution to alleviate the global shortage of donor corneas since it provides a high number of CECs from a single donor. Several approaches have been developed in the last few decades, but effective approaches remain limited for HCECs expansion with less variation and high yields. Two principal substrates with defined expansion medium have been recognized for in vitro proliferation of the primary HCECs. Tissue culture polystyrene (TCPS) dishes coated with extracellular matrix (ECM), by simple physical adsorption,10,11 represent one approach to create an optimal microenvironment for the expansion and maintenance of primary HCECs. The ECM coating on the dish provides a biomimetic substrate that mimics the natural ECM, promoting cell adhesion, and its proliferation. Functionalized polymeric substrates12,13 offer an alternative strategy for supporting the growth and expansion of primary HCECs. These synthetic materials provide a customizable platform that can be tailored to specific cell culture requirements, allowing for fine-tuning of cell behavior, and optimizing cell expansion protocols. Moreover, these surface functionalization strategies can also be combined with micro- and nanostructured patterns, allowing for the integration of tailored surface features that further enhance the functionality and behavior of primary HCECs.14–16 In this review, we first discuss the basic anatomy and physiology of the human cornea and the development of CE, and following with some of the corneal diseases that require donor cornea transplantation. This is followed by the current advancement of substrate materials including ECM proteins used for coating, and functionalization of polymeric materials for primary HCECs expansion in vitro (Fig. 1).
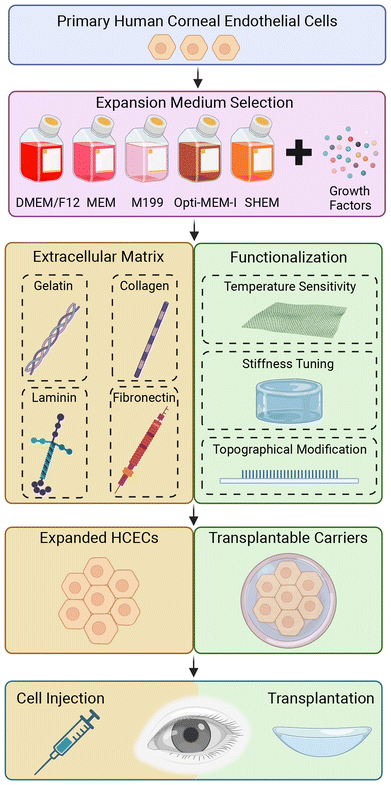 |
| Fig. 1 Schematic overview of biomaterial-based approaches for the expansion and transplantation of primary HCECs. ECM coatings and functionalized synthetic polymers as strategies to create an optimal microenvironment incorporating with current medium-dependent approach, that enhance HCEC proliferation and functionality for therapeutic applications. Created with BioRender.com. | |
Structure and function of cornea
The cornea is a transparent and avascular tissue located at the most outer layer of the eye. It consists of five distinct layers including (I) epithelium; (II) Bowman's layer; (III) stroma; (IV) Descemet's membrane (DM); (V) endothelium (Fig. 2).17 Thickness of the human cornea in the central region is approximately 500 μm thick in healthy adults.18 In the cornea, the nonkeratinized and stratified epithelium layer constitutes approximately 10% of tissue which has 4 to 6 cell layers 40–50 μm thick. The function of corneal epithelial cells is to ensure the integrity of the tear film-cornea interface by creating a barrier to the outside environment. It is vital to the refractive power of the eye and the prevention of toxins and microbes from entering the inner layers of the cornea. Bowman's layer lies just under the epithelium and this smooth layer is approximately 15 μm thick and assists the cornea to sustain its shape.19 The bulk of the cornea is the corneal stroma, that roughly comprises 80–85% of the corneal and is approximately 450 μm thick. Keratocytes are the main cell type of the stroma, and it maintains the ECM environment of stroma and corneal transparency. The DM is located at the posterior boundary of the stroma, and secretion from the CECs after birth can contribute up to an additional 10 μm in thickness with age.20 The corneal endothelium (CE), located at the posterior of the cornea, forms a monolayer of hexagonal cells. This layer serves as the key regulator of corneal deturgescence and acts as a barrier facilitated by gap junctions and tight junctions throughout the monolayer. These CECs are known to be highly metabolically active, that function continuously to actively transport fluid from the stroma to the anterior chamber of the eye.21,22 This continuous fluid movement, coupled with the dynamic balance between a “leaky” barrier and active pumping, plays a crucial role in the regulation of corneal hydration and the maintenance of visual transparency.23,24
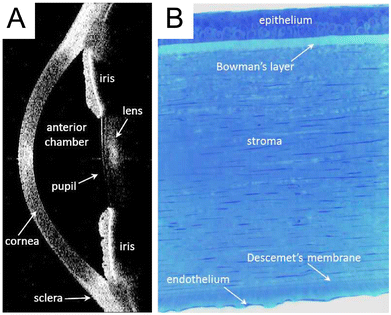 |
| Fig. 2 The human corneal anatomy. (A) In vivo cross-section of the cornea and anterior eye by anterior segment optical coherence tomography. The bright areas indicate light scatter while the dark areas are transparent. The bright area in the central cornea is an artifact. (B) Histologic view of the human cornea with toluidine blue staining, indicating the five principal layers of the normal cornea. Adapted from Neil Lagali et al.25 under the terms of the Creative Commons Attribution 3.0 License (CC BY 3.0). | |
Human CECs development and stem-like cell population
The development of the human cornea is a multifaceted process influenced by various signaling pathways and growth factors. The corneal endothelium (CE) begins to form around the fifth to sixth weeks of human gestation,26 deriving primarily from the neural crest during embryonic development.27 Neural crest cells contribute significantly to the development of the periocular mesenchyme, which serves as a crucial source for multiple mature cell lineages essential for normal ocular development. These include the corneal endothelium, stroma, trabecular meshwork (TM), ciliary body muscles, iris stroma, extraocular muscles, and ocular blood vessels (Fig. 3).
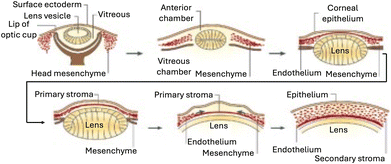 |
| Fig. 3 Formation of the human cornea. The cornea begins to develop when the surface ectoderm closes after the formation of the lens vesicle and its detachment from the surface ectoderm. Mesenchymal cells (neural crest cells) invade the cornea and form the corneal stroma after condensation. Adapted with permission.28 Copyright 2010, Elsevier. | |
The human corneal endothelium consists of cells of mostly hexagonal morphology that form a uniform monolayer structure. An average of 3000 cells per mm2 in adults, of which 75% of the cells are hexagonal.29 The density and topography of the CECs continuously change throughout age. Cell density decreases every year by 0.6%3 and down to about 2600 cells per mm2 in the eight decades.30 Since CECs are non-proliferative, adjacent normal CECs undergo cellular migration and expansion resulting in polymegathism (cell enlargement) and pleomorphism (loss of cell hexagonality)31 to compensate for damaged or non-functional cells. This process helps maintain the overall functional integrity of the corneal endothelium, ensuring proper regulation of corneal hydration. However, in cases of disease or acute loss of corneal endothelial cells, leading to a significant impact on their regulatory function, it may result in corneal blindness. In those cases, corneal transplantation will be required to restore vision and an optimal corneal health.
Corneal endothelial cells express phenotypical and function-associated markers such as Na+/K+-ATPase pumps, focal tight junctions (zonula occludens-1), N-cadherin and CD166.32 However, Na+/K+-ATPase pumps and focal tight junctions (ZO-1) could be found in many other non-corneal cells. Additional markers such as sodium bicarbonate transporter-like protein 11 (SLC4A11), cadherin-2/N-cadherin (CDH2), COL8A2 and sPRDX-6 have been identified as healthy corneal endothelial cell marker in recent studies.33–37 These markers are crucial for identifying and studying HCECs and can provide insights into their functional properties in health and disease.
A growing body of evidence suggests the existence of endothelial progenitor cells with stem-like characteristics beyond the peripheral endothelium (PE), within the transition zone beyond the periphery of the CE.38,39 These cells express markers such as Sox2, Lgr5, CD34, Pitx2, and telomerase, indicating their potential for proliferation–induction approaches.38 These stem-like progenitor cells are believed to be sequestered in the niche at the transitional zone where the corneal endothelial cells meet the TM.40
Furthermore, studies have identified cells with stem cell markers such as nestin, alkaline phosphatase, and telomerase in the transition region between the peripheral endothelium and the TM.39 After corneal injury, Oct 3/4, Pax6, Sox2, and Wnt-1 expression in the corneal endothelium suggests the presence of stem cells in the posterior limbus, initiating endothelial regeneration.39 Additionally, TM stem cells expressing neural crest markers (N-cadherin, ABCG2, myc, nestin, p75NTR) have been found beneath the Schwalbe's line, capable of differentiating into corneal endothelial-like cells.41 These findings collectively demonstrate the presence of stem-like progenitor cells in the transitional zone between the peripheral endothelium and the TM fibers. Future studies on the development of these cells should include a robust approach to isolate and propagate these unique stem/progenitor cells.
Corneal endothelial diseases and current treatments
Corneal diseases are the third leading cause of blindness.42 The causes of corneal blindness include congenital or acquired through injury, trauma, infection, or surgical operation in the eye, resulting in the loss of transparency or visual dysfunctions.43,44 The inability for the HCECs to replace damaged or lost cells in vivo is a significant factor in the development of human corneal endothelial diseases.45 Fuchs endothelial corneal dystrophy (FECD) is one of the most frequent cause of primary corneal endothelial dysfunction, and is characterized by the progressive decrease in endothelial cell density and morphological alterations of CECs due to abnormal secretion of ECM forming guttate.46,47 Some of the other causes of corneal endothelial disorder includes: posterior polymorphous corneal dystrophy (PPCD), congenital hereditary endothelial dystrophy (CHED), iridocorneal endothelial (ICE) syndrome, viral infections, posttraumatic fibrous downgrowth, glaucoma and diabetes mellitus.48
Currently, there are several non-surgical treatment options including topical administration of antibiotics, steroids, nonsteroidal anti-inflammatory drugs to relieve symptoms,49–53 but only a corneal transplantation is the definitive treatment to reverse corneal blindness for corneal endothelium diseases such as end-stage FECD. Full-thickness corneal transplantation or penetrating keratoplasty (PK) where the whole corneal button is transplanted is being gradually replaced with endothelial keratoplasty (EK), which has gained widespread acceptance as the established standard treatment approach.54,55 With the global shortage of suitable donor graft material, alternative therapeutic approaches such as cell injection therapy and tissue-engineered (TE) corneal endothelial grafts derived from propagated HCECs are emerging as promising solutions for the treatment of corneal endothelial dystrophy.56–60
Corneal endothelial cell replacement therapy has been shown to produce outcomes similar to those of traditional endothelial keratoplasty.61 Specifically, the injection of expanded CECs for treating corneal endothelial dysfunction has been reported in a first-in-man study, shows significant potential.62 Delivery of the expanded CECs on TE scaffold has also been shown to reverse blindness in a pre-clinical rabbit model of bullous keratopathy, but it should be noted that in comparison, CEC injection therapy potentially offers a faster functional recovery profile, as it eliminates the need to remove DM.63 More importantly, it is clear that the maintenance of a Good Manufacturing Practice (GMP)-compliant cell production process within a regulatory-compliant environment remains to be a significant challenge, which is required to produce consistent yield of high-quality primary CECs with their preserved phenotypical and functional characteristics.64 Currently, we stand at a pivotal juncture marked by promising advancements in medical technology, poised to reshape the management of corneal endothelial diseases in the coming decades.
Current approaches of in vitro HCECs expansion
The expansion of HCECs in vitro heavily relies on the use of serums, soluble factors, and growth factors with the basal medium. Various culture media have been established from different basal media and containing growth factors for HCECs proliferation, as shown in Table 1.22 However, some medium formulations, such as SHEM, was not as successful as others in terms of its capacity to support cellular proliferation rate of CECs, with Opti-MEM I or F99 being more effective. To facilitate better attachment of HCECs and its formation of a continuous monolayer on TCPS, a combination of growth factors and ECM coating is commonly used. Lastly, co-culture with other cell types,65 induced pluripotent stem cells (iPSCs),66,67 and gene editing technologies (e.g., CRISPR-Cas9)68 have also been developed to enhance the proliferation and functions of HCECs, as well as to introduce genetic modifications for therapeutic purposes.
Table 1 Summary of HCECs expansion medium complement
Basal medium |
Serum |
Growth factors and supplements |
Substrate |
Cell morphology |
Ref. |
MEM: minimum essential medium; DMEM: Dulbecco's Modified Eagle Medium; SHEM: supplemented hormonal epithelial medium. ECGS, endothelial cell growth supplement; bFGF, basic fibroblast growth factor; NGF, nerve growth factor; EGF, epidermal growth factor; DMSO, dimethyl sulfoxide; VEGF, vascular endothelial growth factor; IGF, insulin-like growth factor. |
MEM |
10% |
5 μg mL−1 insulin |
FN or BCE-derived ECM-coated tissue culture plastic |
Hexagonal |
69
|
|
5 μg mL−1 transferrin |
|
|
|
|
5 ng mL−1 sodium selenite |
|
|
|
|
150 μg mL−1 ECGS |
|
|
|
|
50 μg mL−1 gentamicin |
|
|
|
|
100 U mL−1 penicillin |
|
|
|
|
100 μg mL−1 streptomycin |
|
|
|
|
0.25 μg mL−1 amphotericin B |
|
|
|
MEM |
15% |
200 mM glutamine |
Tissue culture plastic |
Polygonal |
70
|
|
2% essential amino acids |
|
|
|
|
1% nonessential amino acids |
|
|
|
|
10 μg mL−1 gentamicin |
|
|
|
|
1.2 μg mL−1 amphotericin B |
|
|
|
DMEM/F12 |
20% |
120 μg mL−1 penicillin |
FN coated Tissue culture plastic |
Polygonal/hexagonal |
71
|
|
220 μg mL−1 penicillin |
|
|
|
DMEM |
15% |
30 mg L−1L-glutamine |
BCE-derived ECM coated tissue culture plastic |
Varying |
72 and 73 |
|
2.5 mg L−1 fungizone |
|
|
|
|
2.5 mg L−1 doxycycline |
|
|
|
|
2 ng mL−1 bFGF |
|
|
|
M199 |
20% |
4 mM glutamine |
Fibronectin- or gelatin-coated tissue culture plastic |
Polygonal |
74
|
|
200 μg mL−1 ECGS |
|
|
|
|
100 μg mL−1 penicillin |
|
|
|
|
100 μg mL−1 streptomycin |
|
|
|
Opti-MEM-I |
8% |
20 ng mL−1 NGF |
FNC Coating Mix® coated tissue culture plastic |
Polygonal |
5
|
|
5 ng mL−1 EGF |
|
|
|
|
20 μg mL−1 ascorbic acid |
|
|
|
|
200 mg L−1 calcium chloride |
|
|
|
|
100 μg mL−1 bovine pituitary extract |
|
|
|
|
50 μg mL−1 gentamicin |
|
|
|
|
1× antibiotic/antimycotic |
|
|
|
|
0.08% chondroitin sulfate |
|
|
|
F99 Ham's F12 and M199 (1 : 1 ratio) |
2–5% |
20 μg mL−1 ascorbic acid |
BCE-derived ECM coated tissue culture plastic |
Cobblestone-like |
75 and 76 |
|
20 μg mL−1 bovine insulin |
|
|
|
|
2.5 μg mL−1 transferrin |
|
|
|
|
0.6 ng mL−1 sodium selenite |
|
|
|
|
10 ng mL−1 bFGF |
|
|
|
SHEM Ham's F12 and DMEM (1 : 1 ratio) |
5% |
0.5% DMSO |
Tissue culture plastic |
Hexagonal |
77
|
|
2 ng mL−1 EGF |
|
|
|
|
5 μg mL−1 insulin |
|
|
|
|
5 μg mL−1 transferrin |
|
|
|
|
5 ng mL−1 selenium |
|
|
|
|
0.5 μg mL−1 hydrocortisone |
|
|
|
|
1 nM cholera toxin |
|
|
|
|
50 μg mL−1 gentamicin |
|
|
|
|
1.25 μg mL−1 amphotericin B |
|
|
|
DMEM |
10% |
2 ng mL−1 bFGF |
Collagen IV–coated tissue culture plastic |
Polygonal |
78
|
|
50 U mL−1 penicillin |
|
|
|
|
50 μg mL−1 streptomycin |
|
|
|
EGM-2 endothelial growth medium |
10% |
VEGF |
FN and collagen IV–coated tissue culture plastic |
Typical CECs (hexagonal or polygonal) |
10
|
|
EGF |
|
|
|
|
bFGF |
|
|
|
|
IGF |
|
|
|
|
Ascorbic acid |
|
|
|
|
Hydrocortisone |
|
|
|
|
Gentamicin |
|
|
|
|
Amphotericin B |
|
|
|
In vitro HCECs expansion
Expansion of HCECs in vitro is very challenging as it requires a conducive condition for (1) cellular propagation to increase cell numbers; and (2) to retain its functional capacity. Without the appropriate culture conditions, expansion of HCECs may result in cells that do not proliferate, or cells that undergo endothelial–mesenchymal-transition (EndMT).22,32 EndMT is a process wherein an endothelial cell undergoes a sequence of molecular events,79 leading to a transformation of its phenotype into that of a mesenchymal cell, accompanied by the loss of HCEC monolayer integrity and functionality.80 Furthermore, a monolayer of cultivated corneal endothelium is fragile and hard to handle it. Hence, developing suitable substrates that not only provide mechanical support but also mimic the native microenvironment of the corneal endothelium could greatly enhance the expansion and transplantation of engineered human corneal endothelial sheets.
In recent years, scientists have been actively exploring a variety of strategies to optimize the expansion of HCECs in vitro, achieving to identify the best conditions that not only promote cell proliferation but also preserve endothelial functionality.81 Research efforts focus on fine-tuning culture conditions, such as growth factors, media compositions, and ECM components, in order to better mimic the in vivo environment of the corneal endothelium.82 This includes the identification of optimal substrates and biomaterials that can enhance cell attachment, maintain monolayer integrity, and prevent undesirable transitions like EndMT. By overcoming these challenges, researchers hope to establish more reliable protocols for expanding HCECs, which could significantly advance the development of cell-based therapies for corneal diseases.
To further explore the use of biomaterials for HCECs culture, the following section discuss specific examples of ECM protein for physical adsorption and functionalization strategy of synthetic polymers commonly used for HCECs expansion in vitro (Table 2).
Table 2 Summary of substrate materials for HCECs expansion and tissue engineering
Materials |
Advantages |
Limitations |
Functional markers expression |
Applications |
Ref. |
ECM proteins
|
Collagens |
Replicate main components of the DM, enhance cell attachment and cell growth, and maintain cell phenotype |
Xenogeneic and not well defined |
Na+/K+-ATPase; ZO-1 |
Tissue engineering, cell expansion |
11 and 99 |
Fibronectin |
Simple and reproducible method, easily manipulated, enhance cell adhesion and compact cellular morphology |
Batch variability, risks of xeno-contamination |
Na+/K+-ATPase; ZO-1 |
Cell expansion |
5, 10 and 11 |
Laminin |
Enhances cell adhesion and proliferation, potentially xeno-free |
Challenges in the production of recombinant laminin poses |
Na+/K+-ATPase; ZO-1 |
Tissue engineering |
90 and 91 |
Gelatin |
Natural biomaterial with suitable biodegradability, transparent, ability to maintain permeability and to support cell attachment and pumping function |
Xenogeneic, temperature sensitivity, low mechanical strength |
Na+/K+-ATPase; ZO-1; N-cadherin |
Tissue engineering |
102 and 103 |
Functionalization of polymeric materials
|
Thermal responsive polymers |
Easy detachment of confluent HCEC sheets without the need for proteolytic enzymes or other contaminants |
Detached cell monolayers fragility, requires cell-carrier for transplantation |
Na+/K+-ATPase; ZO-1 |
Cell expansion |
12, 104 and 105 |
Hydrogel polymers |
Promote cell attachment and growth, adjustable thickness, transparency, porosity and robustness and allow fluids and nutrients penetration |
Biocompatibility of hydrogels, poor mechanical strength and stiffness |
Na+/K+-ATPase; ZO-1 |
Tissue engineering |
106 and 107 |
Topographical modification |
Support cell attachment, enhance cell proliferation, ability to replicate surface topography onto wide range of polymers, topographic memory in cell phenotype maintenance |
Complex fabrication processes, long-term stability of topographical features, materials durability after topographical modification |
Na+/K+-ATPase; ZO-1 |
Tissue engineering, cell expansion |
16 and 107 |
ECM proteins for HCECs expansion
The extracellular microenvironment plays a vital role in cell proliferation and cell behavior by modifying cell contractility and the cytoskeleton83 Initial cell attachment and functional markers could be harnessed in adherent cells with cell–substrate and integrin–matrix interactions.14,84 In particular, ECM components are crucial to promoting cell attachment to the TCPS.85 For instance, commercially available collagen and fibronectin mixture (FNC Coating Mix®) coated TCPS could improve the HCECs attachment and expansion rate when used with an appropriate media composition.22 Naturally derived ECM proteins are always preferred due to their biocompatibility. Collagen,10,11,86,87 fibronectin,69,88 laminin,89–91 and gelatin92–94 are well-established ECM proteins for primary HCECs in vitro culture. These ECM proteins can be used to coat cell culture dishes to provide a favorable microenvironment, and mechanical support, and to promote initial cell adhesion.
Collagen.
Collagen, particularly collagen types IV and VIII, constitutes a significant portion of the ECM present in various tissues.95 Its primary function is to facilitate cell adhesion and promote growth on the substrate.77 In DM, collagen types IV and VIII are the predominant ECM proteins, establishing a foundation for crucial cellular interactions.96,97 Due to its enhanced ability to promote cell adhesion and proliferation, collagen type IV emerges as the most promising ECM constituent for attachment and proliferation of HCECs, compared to other ECM components such as collagen type I, fibronectins, and laminins.11,98
Collagen type I is another main protein that has been used as a coating or even as a free-standing substrate for HCECs expansion.11,99 Previous studies have shown promising results with free-standing collagen type I sheets, as they were found to promote the formation of confluent monolayers and enhance the expression of ZO-1 and Na+K+-ATPase in primate CECs.100 Furthermore, the TE corneal endothelial graft remained transparent and maintained high cell density (1992–2475 cells per mm2) with hexagonal morphology after six months when transplanted into monkeys. This study was conducted using monkey CECs and not human CECs; however, it still provided valuable preliminary data for HCECs expansion on collagen. Mimura et al., grew HCECs on cross-linked sheets of type I collagen and reported that the cultivated HCECs were able to maintain 76%–95% of pump function of human donor corneas when assessed with a Ussing chamber and ouabain, a Na+K+-ATPase inhibitor.99 However, achieving reproducibility in Ussing chamber experiments and ouabain assays can be challenging due to the intricate experimental design, requiring careful validation. Plastic compressed collagen called RAFT was developed as a novel carrier for cultured human corneal endothelial cells for transplantation.101 After being cultured on collagen constructs, both a human corneal endothelial cell line and primary HCECs maintained their distinctive cobblestone morphology and continued to express tight junction protein ZO-1 and pump protein Na+/K+-ATPase α1. Also, its ease of handling is crucial when considering that these sheets may undergo transplantation and need to be handled by surgeons. These studies demonstrated the potential of collagen-based materials for corneal tissue engineering, with xeno-free polymers being particularly advantageous for use as substrate, thereby facilitating the clinical application of expanded HCECs.
Fibronectin.
Another ECM protein that is used for HCECs expansion is fibronectin (FN) due to its ability to promote cell proliferation, aid the maintenance of cellular phenotype, as well as retaining the expression of function-associated markers.10,11 FN is an adhesive glycoprotein that plays a pivotal role during embryogenesis, and also important in tissue regeneration, regulating HCECs attachment and proliferation.108 Choi et al. expanded primary HCECs on both FN and collagen IV coated dish before testing on human decellularized stroma.10 The growth rate of the HCECs on each ECM substrate was similar and HCECs grown on both substrates show the same level of functional marker expressions with typical compact cellular morphology. Their findings revealed the importance of the FN for the growth of HCECs. Thus, using chemical grade, which has been purified and processed to remove contaminants and impurities, FN could be advantageous as it simplifies the process and reduces the possibility of an immune response to the cells. Moreover, FN also has the potential to improve in vitro HCECs proliferation, resulting in higher cell density with compact cellular morphology when compared to the uncoated dish.11 The α3β1 integrin receptor for laminin-5 and fibronectin is expressed by HCECs, and the use of recombinant laminin-5 and human FN has been shown to promote HCECs adhesion, migration, and moderate proliferation in culture.88
The FNC Coating Mix®, consisting of fibronectin, collagen, and albumin, is commonly employed as an ECM coating to improve the attachment of adherent cells. This mixture is widely utilized for promoting primary HCECs attachment and proliferation. Since the initial cell attachment is the key for further cell proliferation and final expansion yields. Zhu et al. demonstrated that HCECs attachment could be improved by coating tissue culture plates with FNC Coating Mix®.5 Consequently, HCECs from both young and older donors could proliferate in vitro in response to growth factors after cell attachment. Moreover, FNC also could use to coat plastic compressed collagen as a supporting material for transfer of cells into recipients.101 Primary HCECs maintained their distinctive cobblestone morphology and exhibited expression of tight junction protein ZO-1 and pump protein Na+/K+-ATPase α1 even after being cultured on collagen constructs for a duration of 14 days. This finding suggests that FN may play a crucial role in HCECs culture and could be an important factor in the development of cell therapy for clinical use.
Laminin.
Laminins play a pivotal role as the primary constituents of the basement membrane, influencing various cellular processes, including migration, survival, proliferation, and differentiation.109,110 There have been reports of the presence of laminin-411 and -511 expression within the Descemet's membrane, which constitutes the basement membrane of the corneal endothelium.111,112 Nonetheless, a complete comprehension of the laminin composition and its biological significance remains unclear.113
Okumura et al. demonstrated the effectiveness of utilizing laminins (recombinant human laminin-511 or human laminin-521) as coatings, revealing superior results in terms of HCEC attachment.91 When HCECs were cultured on laminin-511 or -521, they reached a cell density ranging from 2200 to 2400 cells per mm2, whereas the cell density on an uncoated control was only 1100 cells per mm2. In addition, recombinant laminin E8 fragments (E8s) supported HCEC growth with a similar yield to that achieved with laminin. Moreover, Peh et al. affirmed that both human laminin-511 and laminin-521 yielded superior results in terms of HCEC attachment.90 However, for their subsequent study, they opted for human collagen IV due to its cost-effectiveness. Hence, a plausible explanation could be that the improvement in HCEC adhesion facilitated by laminin-511 and -521 sustained their capacity for proliferation, subsequently resulting in a greater saturation density. Finally, incorporating laminin E8s might create an optimal xeno-free and well-defined surface for cultivating CECs in clinical settings.
Gelatin.
Gelatin is a natural material derived via collagen hydrolysis and one of the most commonly studied compatible and biodegradable materials for corneal tissue engineering. To use gelatin for corneal engineering application, researchers mainly constructed free-standing membranes by crosslinking the gelatin to support corneal cells. Several studies have investigated gelatin as a substrate for HCECs expansion and as a transplantable carrier.102,103,107,114 Primary HCECs seeded on gelatin, expressed normal levels of functional markers, zona occludens-1 (ZO-1), Na+/K+-ATPase and N-cadherin with continuous endothelial monolayer.103 Researchers have also extensively used gelatin as a base material, for example, Lai et al. modified the gelatin with chondroitin sulfate (CS) to improve the cell growth.115 Moreover, the addition of heparin during crosslinking was able to enhance the absorption of bFGF and release kinetics.102 This scaffold had sufficient flexibility to be folded to facilitate surgical implantation and HCECs were successfully grown on the scaffold, while maintaining morphological and functional integrity.
Functionalization of polymeric materials for HCEC tissue engineering
Polymer substrates have been commonly used for cell culture such as TCPS dishes, or to functionalize materials to module cell–materials interactions. Synthetic polymeric substrates can also be easily functionalized to provide and fine tune various surface and materials properties such as surface energy, stiffness, roughness, and topography; thereby, facilitating the expansion of HCECs in vitro and enabling the generation of transplantable cells or grafts.16,104,107 Surface energy12,105 and topography16,107 parameters are crucial for initial cell attachment and growth of HCECs. Polymer substrates are often used in combination with ECM to provide a suitable microenvironment for HCECs proliferation.
Temperature-responsive polymers, hydrogel polymers and topographically modified polymers (i.e., tissue culture polystyrene) have been explored as a substrate of HCECs expansion in vitro. These materials offer promising potential in maintaining cell functionality and promoting strong cell attachments. Additionally, some of the functionalization strategies can be extended to generating transplantable carrier film or cell monolayer intended for transplantation, enabling the creation of functional corneal endothelial monolayers that express functional markers. Consequently, these advancements hold the key to preserving cell functionality and preventing cell detachment, which are paramount considerations that directly impact the quality of subsequent cell–cell and cell-carrier construct transplantations.107,116
Functionalization with thermal responsive polymer.
Thermo-sensitive polymeric materials have been extensively studied for the generation of cell sheets for cell transplantation. Temperature-responsive polymer poly(N-isopropylacrylamide) (PNIPAAm) has been utilized as a substratum for HCECs sheet generation12,104,105 due to temperature-dependent hydrophobicity and hydrophilicity alteration with a lower critical solution temperature at 32 °C,117 which is essential for detachment of cells sheets. HCEC sheets can generate on PNIPAAm and harvest as intact transplantable sheets by decreasing the culture temperature (Fig. 4A–C).93 For instance, Nishida and colleagues obtained a confluent monolayer of primary HCECs with polygonal morphology and sustained makers expression105 on PNIPAAm-grafted surfaces after four weeks (Fig. 4D). The structure and function of HCECs sheets detached from pure PNIPAAm were able to restore corneal clarity in rabbit models12,94 (Fig. 4G and H).
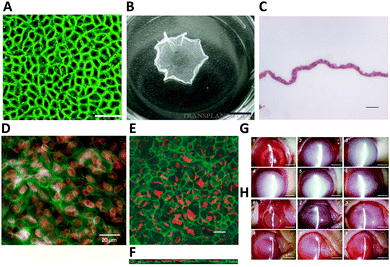 |
| Fig. 4 HCEC morphology and functional markers expression on poly(N-isopropylacrylamide) (PNIPAAm) and HCEC sheet transplantation in a rabbit model. (A) ZO-1 (green) localization in HCEC monolayers was observed after 3 weeks in culture at 37 °C on the PNIPAAm-grafted surfaces. Scale bar = 50 μm. (B) PNIPAAm-grafted surfaces after incubation at 20 °C for 45 min. The size of an intact cell sheet is around 0.75 cm2. Scale bar = 5 mm. Adapted with permission.94 Copyright 2007, Wolters Kluwer Health, Inc. (C) Example of harvested HCEC sheets were composed of a continuous, single cell layer with hematoxylin and eosin staining. Scale bar = 20 μm. Adapted with permission.12 Copyright 2005, John Wiley & Sons. (D) Following four weeks in culture, HCEC sheets were harvested and underwent immunostaining. The tight junction component ZO-1 (green) was observed to be localized at cell boundaries. Nuclei were co-stained with propidium iodide (red). Scale bar = 20 μm. Adapted with permission.105 Copyright 2005, Elsevier. Harvested human corneal endothelial cell sheets were affixed to slide glasses and treated with an anti-Na+/K+-ATPase antibody (green) for staining. Propidium iodide was utilized to highlight the position of cell nuclei (red). Confocal laser scanning microscopy was employed to capture (E) x–y and (F) x–z projection images of the cell sheet. Scale bar = 20 μm. Adapted with permission.12 Copyright 2005, John Wiley & Sons. Clinical observations following transplantation of HCECs sheets in a rabbit model were recorded. Representative slit-lamp biomicroscopic images depict the time-course changes in the surgical corneas of both the wound groups (G) and the HCEC sheet groups (H). Images were captured immediately post-operation (1), and at 1 day (2), 1 week (3), 2 weeks (4), 2 months (5), and 6 months (6) post-surgery. Scale bar = 5 mm. Adapted with permission.94 Copyright 2007, Wolters Kluwer Health, Inc. | |
By incorporating glycidylmethacrylate (GMA) through copolymerization with PNIPAAm, N-isopropylacrylamide-co-glycidylmethacrylate (NGMA), it allows to adjustment of the critical temperature and introduces epoxy groups into the resulting polymer, enabling ECM deposition. When a copolymer of NIPAAm and (diethyleneglycol methacrylate) DEGMA is used, it facilitates quicker detachment of cells, and the critical temperature is closer to the physiological range.118,119 Teichmann and colleagues presented the development of a temperature-responsive carrier through the simultaneous electron beam immobilization and cross-linking, it becomes possible to control layer thickness, stiffness, switching amplitude, and functionalization of poly(vinyl methyl ether) (PVME) on polymeric surfaces.120 HCEC sustained metabolic activity on the PVME-blend-PVMEMA comparable with that of HCEC grown on the TCPS. This effectiveness of approach with HCEC, which demand precise cell culture conditions and exhibit strong adhesion to the substrate, has been demonstrated. This innovative approach has the potential to open new avenues in corneal endothelium tissue engineering and substantially enhance therapeutic possibilities.
In spite of thermally detached cell monolayers offering numerous benefits, they are typically fragile, and it is crucial to use appropriate delivery methods when manipulating sheet grafts during the surgery. Therefore, different carriers have been studied for delivering created endothelial layer on implantable carriers.
Functionalization with hydrogel polymer.
Numerous polymer hydrogels have been applied for the HCECs expansion13,121 and corneal bioengineering.122,123 However, despite their controllable mechanical and topographical properties, these hydrogel materials typically exhibit poor attachment of corneal cells on their surfaces without appropriate modifications.124 To improve cell attachment and growth of rabbit and human primary corneal cells, hydrogels modified with collagen I, phosphate groups, and arginylglycylaspartic acid (RGD).125–127 Moreover, peptide hydrogel using poly-ε-lysine (pεK) was developed for HCECs and porcine CECs (pCECs) culture and corneal endothelial grafts106 (Fig. 5A). Poly-ε-lysine hydrogel was functionalized with synthetic cell-binding peptide RGD or DGEA, α2β1 integrin recognition sequence resulted in enhanced pCEC adhesion on the hydrogel with the RGD peptide only (Fig. 5B). This study demonstrated the potential of poly-ε-lysine hydrogel for CEC attachment and growth.
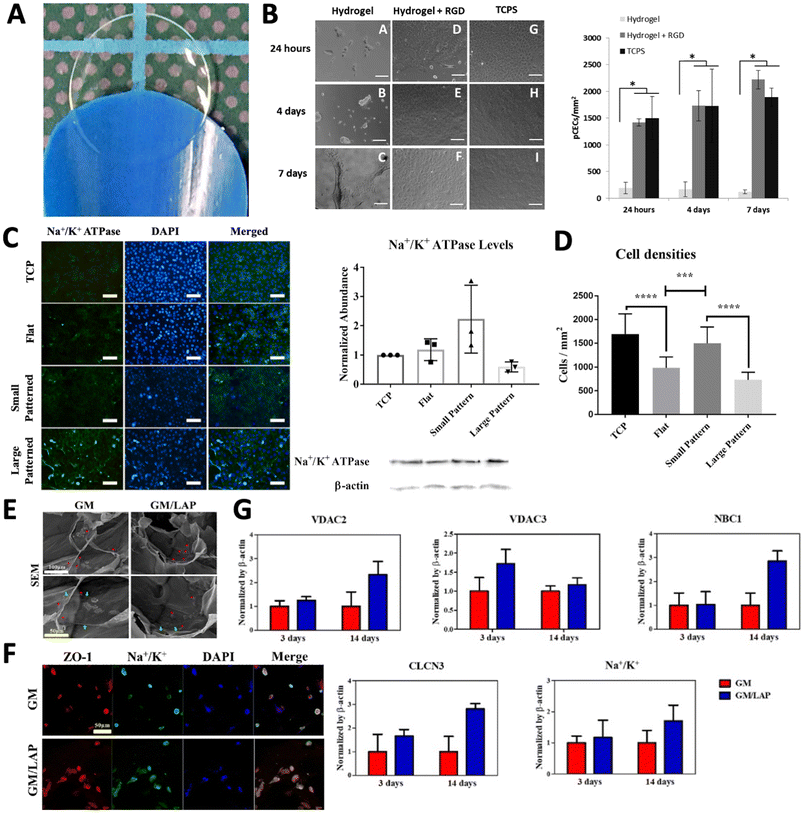 |
| Fig. 5 Hydrogel substrates for CEC culture. (A) Thin and transparent poly-ε-lysine (pεK) hydrogel. (B) Primary porcine CECs expanded on pεK hydrogels functionalized with/without RGD up to 7 days. Scale bar = 100 μm. Quantification of cell density (mm2) on pεK hydrogels at specified time points. Adapted with permission.106 Copyright 2019, Springer Nature. (C) Fluorescence microscopy images of bovine corneal endothelial cells (BCECs) immuno-stained for Na+/K+-ATPase 7 days after seeding on PA hydrogels with different patterns. Scale bar = 100 μm. Total Na+/K+-ATPase levels of these groups as probed by western blot assays and sample bands. (D) Cell densities of these groups as calculated from these images. Adapted with permission.121 Copyright 2021, John Wiley and Sons. (E) SEM images of rabbit CECs encapsulated hydrogels (cells are represented in pink *, green arrows represent secretion of ECM around cells). (F) Immunofluorescence staining of ZO-1, and Na+/K+ of encapsulated CECs cultured for 14 days. Scale bar = 100 μm (white) and 50 μm (yellow). (G) mRNA expression of CECs cultured on GM and GM/LAP hydrogels analysed on 3 and 14 days normalized by β-actin. Adapted with permission.136 Copyright 2023, Elsevier. | |
Another study presented that polyacrylamide (PA) hydrogel can be used as a biomaterial to study the CEC response to in vivo-like microenvironment and DM rigidity. PA is an established purely elastic material with a wide range of rigidity within the Young's moduli of physiological tissues from 0.1 kPA to 100 kPA.128–130 The study involved modifying the PA hydrogel with hexagonal microtopography (∼2000 hexagons per mm2). This modification has shown to be able to support more densely populated bovine corneal endothelial cells (BCECs) monolayer compared to the flat after one week.121 The PA hydrogel was designed to mimic native DM by harnessing the hydrogel stiffness and functionalizing with collagen IV to have mechanical and biochemical support, respectively. Furthermore, essential functional markers such as Na+/K+-ATPase and N-cadherin were consistently observed in BCECs monolayer on the PA hydrogel (Fig. 5C). Cell density and the formation of CE monolayers were controlled by positioning hexagons with optimal distances from each other through surface topography (Fig. 5D). These bioinspired patterned hydrogels and innovate concepts of engineered cell monolayers have the potential to advance in vitro ocular drug testing and clinical applications. Consequently, the provision of chemical and mechanical cues in vitro is crucial for retaining functional cell markers and establishing a continuous CEC monolayer.
Another example is hydrogel of gellan gum (GG), which is a natural, anionic polysaccharide. GG, derived from the bacterium Sphingomonas elodea, consisting of repeating units of two rhamnose, one glucuronic acid, and one glucose residue. GG has several advantages such as non-toxicity, biocompatibility, biodegradability, and viscoelastic properties.131 These characteristics make it particularly suitable for applications in biomedical fields and tissue engineering.132,133 However, its biomaterial application is limited by challenges such as low mechanical strength and a high gelation temperature, for example 50 °C for low acyl gellan.134 To address these issues, chemical modifications, adjusting crosslinking methods, and blending with other biomaterials or fillers have been utilized, transforming GG into a semi-synthetic polymer.135 The stability of GG hydrogels was enhanced by incorporating methacrylate anhydride (MA) into the GG backbone, resulting in methacrylated gellan gum (GM). This was further photo-crosslinked using lithium phenyl-2,4,6-trimethylbenzoylphosphinate (LAP) to create GM/LAP, which had an enhanced mechanical property with the modulus range of 250 ± 6 kPa compared to GM, 90 ± 5 kPa. The GM/LAP was tested as a corneal endothelial cell (CEC) carrier for corneal tissue engineering (Fig. 5E).136 Immunofluorescence staining of membrane proteins, including ZO-1 and Na+/K+-ATPase, demonstrated successful adhesion and morphology of rabbit CECs cultured on GM and GM/LAP substrates (Fig. 5F). Notably, CEC-specific gene expression was observed in both GM and GM/LAP, with enhanced expression on the GM/LAP substrate after 14 days (Fig. 5G). However, this hydrogel have not yet been evaluated with primary HCECs. Further studies could explore the potential of this hydrogel for supporting the growth and functionality of human primary HCECs. Such investigations would help establish their suitability for clinical applications, advancing the development of engineered corneal tissues for therapeutic use.
Functionalization with topographical modification.
The use of nano- and micro-topography as a signaling mechanism for regulating cell proliferation and function is a significant factor in modulating the interaction between mammalian cells and their environment. Micro or nanofabricated topography can influence cell morphology, alignment, adhesion, proliferation, and function.137,138 Recently our group has developed several micro- and nanostructured surfaces for the expansion of HCECs. We first fabricated and screened several polydimethylsiloxane (PDMS) patterns including channels, gratings, concentric circles, pillars, and wells on bovine corneal endothelial cells (BCEC) to modulation of cell behavior.14 The monolayer formation, corneal cell endothelial phenotype and functional markers expression (barrier pump functions) of BCEs were enhanced on both 1 μm and 250 nm pillars. Further, we extended our study to on HCECs expansion with TCPS with micropatterned pillars and wells.16 To enhance cell adhesion, TCPS patterns were coated with FNC Coating Mix® and laminin–chondroitin sulfate (LC). FNC Coating Mix® coated 1 μm TCPS pillar showed a nearly three-fold increase in the proliferation rate of HCECs with elevated expression of ZO-1 functional marker, compared with the unpatterned control. However, the underlying mechanism needs to be explored in further study. Moreover, another functional marker, Na+/K+-ATPase, indicative of cell phenotype, was steadily expressed and evenly distributed in the cell cytoplasm. While morphometry of HCECs such as cell area and coefficient of variation (CoV) of cell area varied between the patterns. For example, the cell areas and CoV were lesser on FNC-coated 250 nm TCPS pillars. The CoV was also reduced on LC coated 1 μm TCPS wells; however, the cell area was larger, and cell circularity was similar for all surface topographies. Moreover, we illustrated that the impact of surface topography on the expression of tight junctions and the morphology of cells remained consistent across multiple passages, and the cells were able to retain this effect adequately (Fig. 6A–F). These findings carry two significant implications. First, the platform has the potential to serve as a valuable tool for cell culture and serial passaging, particularly in the context of HCEC expansion. It can be utilized akin to FNC coating or specialized media, facilitating HCEC growth and maintenance. Secondly, the observed enhancement persists even when the cells are transplanted onto an unpatterned surface. This suggests its potential utility in generating HCECs for cell therapy and transplantation. This development could play a pivotal role in cell-based approaches by enabling the formation of in situ endothelial cell monolayers upon delivery. Researchers focused on human corneal endothelial cell cultivation stand to benefit significantly from the advancement of patterned TCPS culture platforms.
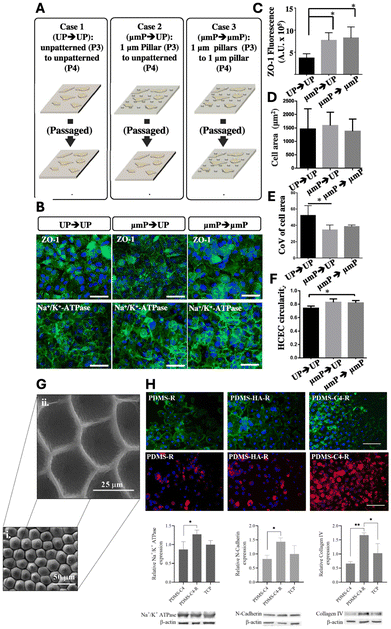 |
| Fig. 6 Impact of biophysical cues on HCEC functional marker expressions and cellular phenotype. (A) Experiment overview depicting the investigation into the impact of altering biophysical cues on ZO-1 expression levels. (B) ZO-1 and Na+/K+-ATPase expression in HCECs under three conditions: Case 1 – passage from unpatterned TCPS at P3 to unpatterned TCPS at P4; Case 2 – passage from 1 μm TCPS pillars to unpatterned TCPS; Case 3 – passage from 1 μm TCPS pillars to 1 μm TCPS pillars. (C) ZO-1 fluorescence at cell boundaries for all three cases. Notably, HCECs exhibit enhanced ZO-1 expression when cultured on patterned TCPS compared to those on unpatterned TCPS, suggesting the potential retention of cellular memory of prior conditions. (D) Effect of topographic cues on cell area. (E) Coefficient of variation of cell area as influenced by topographic cues. (F) Changes in cell circularity due to variations in topographic cues. Scale bar = 100 μm. Adapted with permission.16 Copyright 2015, Elsevier. (G) SEM images of rose petal patterns on PDMS surfaces, rose patterned (i) mold and (ii) cell substrate. (H) Actin cytoskeleton (green) and nuclei (blue) staining of BCECs on PDMS substrates (upper panel). Scale bar = 100 μm. Immunofluorescent staining of BCECs for Na+/K+-ATPase (red) and DAPI (blue) (middle panel). Scale bar = 100 μm. Western blot analysis of BCECs on PDMS-C4, PDMS-C4-R and TCP. Quantification of Na+/K+-ATPase, N-cadherin and collagen IV markers relative to β-actin (lower panel). R = replica. Adapted with permission.139 Copyright 2021, Elsevier. | |
Recently, Özgen Öztürk-Öncel et al. demonstrated that a biomimetic PDMS substrate featuring rose petals (negative mouldings with array of concave microstructure) enhanced bovine CECs (BCECs) response, resulting in higher cell density monolayers and increased expression of phenotypic markers.139 These topographical features closely mimic those of the native corneal endothelium, particularly the hexagonal cell shapes and cell density (∼2000 cells per mm2) (Fig. 6G). Additionally, the patterned PDMS substrate was replicated onto a TCPS substrate and functionalized with collagen IV and hyaluronic acid (HA), two essential components of ECM. The replicated TCPS substrate coated with collagen IV significantly improved BCEC numbers and functional marker expression (Fig. 6H).
Surface morphology plays a critical role in tissue engineering, as it is well established that CECs, like all cells, are sensitive to their microenvironment. The topographical features of the ECM play key role in supporting cell adhesion, arrangement, and organization while preserving their phenotype and function. Taken together, substratum with optimized geometry and proper ECM coating can effectively maintain the HCEC phenotype and promote cell growth.
Surface functionalization with topography can also applied to ECM-derived implantable carrier. Crosslinked gelatin such as gelatin methacrylate (GelMA) has been increasingly used in corneal tissue engineering due to its biocompatibility and tunable physical properties. We have previously developed a sequential hybrid crosslinked GelMA hydrogel called “GelMA+” by incubating prepolymer solution at 4 °C for 1 h before the UV crosslinking.107 GelMA+ films showed stronger mechanical properties, up to 5 time stronger, compared to GelMA films without the sequential crosslinking. When combined with topographical features such as 1 μm pillars, the micropatterned GelMA films provide relevant cues to generate HCEC monolayers (Fig. 6A and B). In particular, HCECs monolayers formed on GelMA+ with 1 μm pillars of square array presented enhanced ZO-1 expression compared to unpatterned control (Fig. 7C–E). Meanwhile, the monolayers on 1 μm pillar of hexagonal-array GelMA+ showed higher cell density (Fig. 7F) and homogeneous cell size (Fig. 7G and H), which are characteristic of a functionally superior monolayer. In vivo biodegradation studies demonstrated that kinetics could be effectively modified by integrating hybrid crosslinking via pre-cooling of the gelatin base material prior to UV crosslinking. The capacity to adjust GelMA+ degradation rates hold promise for tissue engineering scaffolds with precisely controlled degradation during tissue regeneration.140 By enhancing their properties and integrating topographical patterns, GelMA+ thin films can serve as mechanical support while offering pertinent guidance, making them suitable for the development of transplantable monolayers to address the needs of patients with corneal endothelial dysfunction.
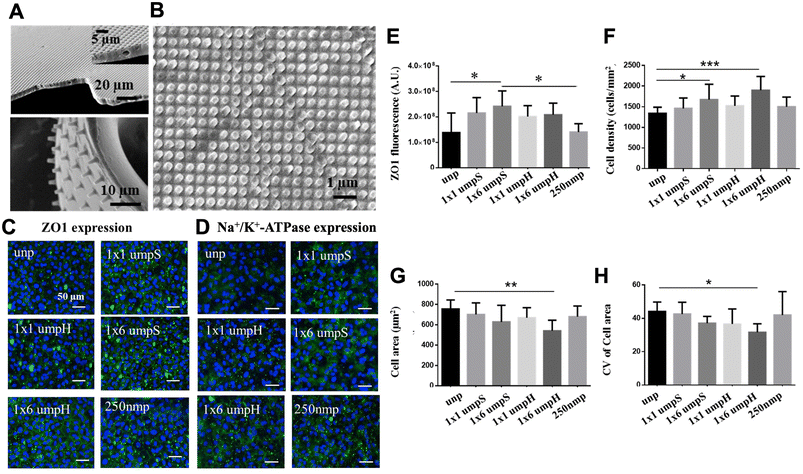 |
| Fig. 7 Effect of GelMA+ topographical cues on HCECs. (A) High aspect ratio pillars and wells on GelMA+ thin films and (B) nano patterns (250 nm pillars). (C) Expression of the tight junctional protein ZO-1 under the influence of GelMA+ topographical cues. (D) Expression of Na+/K+-ATPase pumps in response to the same topographical cues. Scale bar = 50 μm. (E) Quantification of ZO-1 expression across various GelMA+ topographies. (F) Effect of patterned GelMA+ topographical cues on cell density. (G) Changes in cell area resulting from the influence of topographical cues. (H) Coefficient of variation of cell area (CoV) within the HCEC monolayer in the presence of patterned GelMA+ topographical cues. unp = unpatterned; 1 × 1 μmpS = square-array of 1 μm pillars with 1 μm spacing; 1 × 1 μmpH = hexagonal-array of 1 μm pillars with 1 μm spacing; 250 nmp = 250 nm pillars. Adapted with permission.107 Copyright 2017, Elsevier. | |
Conclusions and future perspectives
Corneal blindness due to corneal endothelial dysfunction are primarily treated with corneal transplants, but the shortage of donor corneas presents a challenge.54,141 Cellular therapeutics using stem cells, such as induced pluripotent stem cells (iPSCs) or embryonic stem cells (ESC), are being explored, though research is in the early stages.142–146 Limitations exist regarding purity of differentiation and regulatory issues. Expanding primary HCECs in vitro while preserving their cellular characteristics is a significant challenge, but it could revolutionize the treatment of corneal endothelial diseases, benefiting more patients through engineered corneal grafts or cell injection therapy.61 Researchers have explored various media compositions,22,147 and these media approaches combined with ECM coated dishes22 and topographical patterns16 to enhance primary HCECs’ proliferation and behavior. A synergistic approach combining patterned substrates with appropriate biophysical and biochemical cues is crucial for promoting HCECs’ growth and maintaining functional markers.116 Understanding the mechanisms behind HCECs’ proliferation at the cell–substrate interaction is vital for developing efficient substrates that enable high-yield expansion.148 This approach not only improves HCECs’ growth and sustains marker expression but also has the potential to reduce reliance on costly soluble factors in cell culture media.
The focus on enhancing cell delivery methods is a crucial component of ongoing efforts to revolutionize the treatment landscape for corneal endothelial diseases. Two prominent strategies for cell delivery in the treatment of corneal endothelial diseases are cell injection and TE grafts.63 The use of TE grafts represents a translational concept in corneal therapeutics.90,149 Cell injection, supported by evidence from animal studies demonstrating its efficacy,150–153 is currently ongoing in human trials, and have shown promising outcomes.62,154,155 In comparison, TE grafts provide a more controlled method of delivering cells, and ongoing clinical trials are underway to validate their potential.90 However, the development of a suitable tissue-engineering material that meets regulatory standards remains a significant challenge.
Author contributions
Myagmartsend Enkhbat: conceptualization, writing – original draft, writing – review & editing; Evelyn K. F. Yim: conceptualization, writing – review & editing; Gary S. L. Peh: writing – review & editing; Jodhbir S. Mehta: writing – review & editing.
Data availability
No primary research results, software or code have been included and no new data were generated or analysed as part of this review.
Conflicts of interest
There are no conflicts to declare.
Acknowledgements
This work was supported by the Natural Sciences and Engineering Research Council (NSERC) Discovery (RGPIN-2021-03200), and NSERC-CREATE Training in Global Biomedical Technology Research and Innovation at the University of Waterloo [CREATE-509950-2018]. M. E. was partially supported by NSERC Discovery (RGPIN-2021-03200) and NSERC CREATE (401207296). This work was also supported in part by the National Medical Research Council (Singapore) Clinician Scientist-Individual Research Grant (CS-IRG) Award (MOH-001232-00).
References
- P. M. Mathews, K. Lindsley, A. J. Aldave and E. K. Akpek, Cornea, 2018, 37, 1198–1203 CrossRef PubMed.
- N. C. Joyce, Prog. Retinal Eye Res., 2003, 22, 359–389 CrossRef CAS PubMed.
- W. M. Bourne, L. R. Nelson and D. O. Hodge, Invest. Ophthalmol. Visual Sci., 1997, 38, 779–782 CAS.
- K. Engelmann, M. Bohnke and P. Friedl, Invest. Ophthalmol. Visual Sci., 1988, 29, 1656–1662 CAS.
- C. Zhu and N. C. Joyce, Invest. Ophthalmol. Visual Sci., 2004, 45, 1743–1751 CrossRef PubMed.
- M. Fuest, G. H. Yam, G. S. Peh and J. S. Mehta, Regener. Med., 2016, 11, 601–615 CrossRef CAS PubMed.
- G. S. Peh, R. W. Beuerman, A. Colman, D. T. Tan and J. S. Mehta, Transplantation, 2011, 91, 811–819 CrossRef PubMed.
- K. Kitazawa, C. Sotozono and S. Kinoshita, Asia-Pac. J. Ophthalmol., 2022, 11, 335–345 CrossRef PubMed.
- M. Ueno, M. Toda, K. Numa, H. Tanaka, K. Imai, J. Bush, S. Teramukai, N. Okumura, N. Koizumi, A. Yamamoto, M. Tanaka, C. Sotozono, J. Hamuro and S. Kinoshita, Am. J. Ophthalmol., 2022, 237, 267–277 CrossRef CAS PubMed.
- J. S. Choi, J. K. Williams, M. Greven, K. A. Walter, P. W. Laber, G. Khang and S. Soker, Biomaterials, 2010, 31, 6738–6745 CrossRef CAS PubMed.
- J. S. Choi, E. Y. Kim, M. J. Kim, M. Giegengack, F. A. Khan, G. Khang and S. Soker, Biomed. Mater., 2013, 8, 014108 CrossRef CAS PubMed.
- T. Sumide, K. Nishida, M. Yamato, T. Ide, Y. Hayashida, K. Watanabe, J. Yang, C. Kohno, A. Kikuchi, N. Maeda, H. Watanabe, T. Okano and Y. Tano, FASEB J., 2006, 20, 392–394 CrossRef CAS PubMed.
- E. Y. Kim, N. Tripathy, S. A. Cho, D. Lee and G. Khang, J. Tissue Eng. Regener. Med., 2017, 11, 2471–2478 CrossRef CAS.
- B. K. Teo, K. J. Goh, Z. J. Ng, S. Koo and E. K. Yim, Acta Biomater., 2012, 8, 2941–2952 CrossRef CAS PubMed.
- M. Kruse, P. Walter, B. Bauer, S. Rutten, K. Schaefer, N. Plange, T. Gries, S. Jockenhoevel and M. Fuest, Curr. Eye Res., 2018, 43, 1–11 CrossRef CAS PubMed.
- R. Muhammad, G. S. Peh, K. Adnan, J. B. Law, J. S. Mehta and E. K. Yim, Acta Biomater., 2015, 19, 138–148 CrossRef CAS PubMed.
- D. W. DelMonte and T. Kim, J. Cataract Refractive Surg., 2011, 37, 588–598 CrossRef PubMed.
- M. J. Doughty and M. L. Zaman, Surv. Ophthalmol., 2000, 44, 367–408 CrossRef CAS PubMed.
- I. K. Gipson, S. J. Spurr-Michaud and A. S. Tisdale, Invest. Ophthalmol. Visual Sci., 1987, 28, 212–220 CAS.
- D. H. Johnson, W. M. Bourne and R. J. Campbell, Arch. Ophthalmol., 1982, 100, 1942–1947 CrossRef CAS PubMed.
- D. M. Maurice, J. Physiol., 1972, 221, 43–54 CrossRef CAS PubMed.
- G. S. Peh, K. P. Toh, F. Y. Wu, D. T. Tan and J. S. Mehta, PLoS One, 2011, 6, e28310 CrossRef CAS PubMed.
- W. M. Bourne, Trans. Am. Ophthalmol. Soc., 1998, 96, 229–239 CAS ; discussion 239–242.
- M. V. Riley, B. S. Winkler, C. A. Starnes, M. I. Peters and L. Dang, Invest. Ophthalmol. Visual Sci., 1998, 39, 2076–2084 CAS.
-
N. Lagali, B. B. Peebo, J. Germundsson, U. Edén, R. Danyali, M. Rinaldo and P. Fagerholm, Confocal Laser Microscopy—Principles and Applications in Medicine, Biology, and the Food Sciences, 2013 Search PubMed.
- P. Y. Lwigale, Prog. Mol. Biol. Transl. Sci., 2015, 134, 43–59 Search PubMed.
- P. J. Gage, W. Rhoades, S. K. Prucka and T. Hjalt, Invest. Ophthalmol. Visual Sci., 2005, 46, 4200–4208 CrossRef PubMed.
- J. Graw, Curr. Top. Dev. Biol., 2010, 90, 343–386 Search PubMed.
- C. H. Worner, A. Olguin, J. L. Ruiz-Garcia and N. Garzon-Jimenez, PLoS One, 2011, 6, e19483 CrossRef CAS PubMed.
- R. W. Yee, M. Matsuda, R. O. Schultz and H. F. Edelhauser, Curr. Eye Res., 1985, 4, 671–678 CrossRef CAS PubMed.
- G. O. Waring 3rd, W. M. Bourne, H. F. Edelhauser and K. R. Kenyon, Ophthalmology, 1982, 89, 531–590 CrossRef CAS PubMed.
- R. F. Frausto, V. S. Swamy, G. S. L. Peh, P. M. Boere, E. M. Hanser, D. D. Chung, B. L. George, M. Morselli, L. Kao, R. Azimov, J. Wu, M. Pellegrini, I. Kurtz, J. S. Mehta and A. J. Aldave, Sci. Rep., 2020, 10, 7402 CrossRef CAS PubMed.
- M. Toda, M. Ueno, A. Hiraga, K. Asada, M. Montoya, C. Sotozono, S. Kinoshita and J. Hamuro, Invest. Ophthalmol. Visual Sci., 2017, 58, 2011–2020 CrossRef CAS PubMed.
- Z. Chng, G. S. Peh, W. B. Herath, T. Y. Cheng, H. P. Ang, K. P. Toh, P. Robson, J. S. Mehta and A. Colman, PLoS One, 2013, 8, e67546 CrossRef CAS PubMed.
- V. Ding, A. Chin, G. Peh, J. S. Mehta and A. Choo, mAbs, 2014, 6, 1439–1452 CrossRef PubMed.
- P. Catala, N. Groen, V. L. S. LaPointe and M. M. Dickman, Sci. Rep., 2023, 13, 9361 CrossRef CAS PubMed.
- A. Swarup, R. Phansalkar, M. Morri, A. Agarwal, V. Subramaniam, B. Li and A. Y. Wu, Stem Cell Rep., 2023, 18, 2482–2497 CrossRef CAS PubMed.
- G. H. Yam, X. Seah, N. Yusoff, M. Setiawan, S. Wahlig, H. M. Htoon, G. S. L. Peh, V. Kocaba and J. S. Mehta, Cells, 2019, 8, 1244 CrossRef CAS PubMed.
- S. L. McGowan, H. F. Edelhauser, R. R. Pfister and D. R. Whikehart, Mol. Vision, 2007, 13, 1984–2000 CAS.
- W. Y. Yu, I. Grierson, C. Sheridan, A. C. Lo and D. S. Wong, Stem Cells Dev., 2015, 24, 624–639 CrossRef PubMed.
- H. Yun, Y. Zhou, A. Wills and Y. Du, J. Ocul. Pharmacol. Ther., 2016, 32, 253–260 CrossRef CAS PubMed.
- J. P. Whitcher, M. Srinivasan and M. P. Upadhyay, Bull. W. H. O., 2001, 79, 214–221 CAS.
- A. Shoham, M. Hadziahmetovic, J. L. Dunaief, M. B. Mydlarski and H. M. Schipper, Free Radicals Biol. Med., 2008, 45, 1047–1055 CrossRef CAS PubMed.
- P. McCluskey and R. J. Powell, Lancet, 2004, 364, 2125–2133 CrossRef PubMed.
- A. Bartakova, N. J. Kunzevitzky and J. L. Goldberg, Curr. Ophthalmol. Rep., 2014, 2, 81–90 CrossRef PubMed.
- J. K. Darlington, S. D. Adrean and I. R. Schwab, Ophthalmology, 2006, 113, 2171–2175 CrossRef PubMed.
- F. Altamirano, G. Ortiz-Morales, M. A. O'Connor-Cordova, J. P. Sancen-Herrera, J. Zavala and J. E. Valdez-Garcia, Int. Ophthalmol., 2024, 44, 61 CrossRef PubMed.
- L. J. Jeang, C. E. Margo and E. M. Espana, Exp. Eye Res., 2021, 205, 108495 CrossRef CAS PubMed.
- N. Okumura, S. Kinoshita and N. Koizumi, J. Ophthalmol., 2017, 2017, 2646904 Search PubMed.
- M. Srinivasan, J. Mascarenhas, R. Rajaraman, M. Ravindran, P. Lalitha, D. V. Glidden, K. J. Ray, K. C. Hong, C. E. Oldenburg, S. M. Lee, M. E. Zegans, S. D. McLeod, T. M. Lietman, N. R. Acharya and G. Steroids for Corneal Ulcers Trial, Arch. Ophthalmol., 2012, 130, 143–150 CrossRef CAS PubMed.
- M. Camellin, J. Refractive Surg., 2004, 20, S693–S698 Search PubMed.
- S. Futterknecht, E. Chatzimichail, K. Gugleta, G. D. Panos and Z. Gatzioufas, Drug Des., Dev. Ther., 2024, 18, 97–108 CrossRef CAS PubMed.
- H. Fujimoto and J. Kiryu, J. Ophthalmol. Res., 2021, 4, 231–243 Search PubMed.
- D. T. Tan, J. K. Dart, E. J. Holland and S. Kinoshita, Lancet, 2012, 379, 1749–1761 CrossRef PubMed.
- Y. Q. Soh, G. S. Peh and J. S. Mehta, Regener. Med., 2018, 13, 97–115 CrossRef CAS PubMed.
- P. Catala, G. Thuret, H. Skottman, J. S. Mehta, M. Parekh, S. Ni Dhubhghaill, R. W. J. Collin, R. Nuijts, S. Ferrari, V. L. S. LaPointe and M. M. Dickman, Prog. Retinal Eye Res., 2022, 87, 100987 CrossRef PubMed.
- M. Chi, B. Yuan, Z. Xie and J. Hong, Bioengineering, 2023, 10, 1284 CrossRef CAS PubMed.
- P. A. Faye, F. Poumeaud, P. Chazelas, M. Duchesne, M. Rassat, F. Miressi, A. S. Lia, F. Sturtz, P. Y. Robert, F. Favreau and Y. Benayoun, Exp. Eye Res., 2021, 204, 108462 CrossRef CAS PubMed.
- X. Luo, X. He, H. Zhao, J. Ma, J. Tao, S. Zhao, Y. Yan, Y. Li and S. Zhu, Nanomaterials, 2023, 13, 1976 CrossRef CAS PubMed.
- P. Gronroos, A. Moro, P. Puistola, K. Hopia, M. Huuskonen, T. Viheriala, T. Ilmarinen and H. Skottman, Stem Cell Res. Ther., 2024, 15, 81 CrossRef CAS PubMed.
- Y. Q. Soh, S. S. J. Poh, G. S. L. Peh and J. S. Mehta, Cornea, 2021, 40, 1365–1373 CrossRef PubMed.
- S. Kinoshita, N. Koizumi, M. Ueno, N. Okumura, K. Imai, H. Tanaka, Y. Yamamoto, T. Nakamura, T. Inatomi, J. Bush, M. Toda, M. Hagiya, I. Yokota, S. Teramukai, C. Sotozono and J. Hamuro, N. Engl. J. Med., 2018, 378, 995–1003 CrossRef CAS PubMed.
- G. S. L. Peh, H. S. Ong, K. Adnan, H. P. Ang, C. N. Lwin, X. Y. Seah, S. J. Lin and J. S. Mehta, Sci. Rep., 2019, 9, 6087 CrossRef PubMed.
- D. S. J. Ting, G. S. L. Peh, K. Adnan and J. S. Mehta, Tissue Eng., Part B, 2022, 28, 52–62 CrossRef CAS PubMed.
- A. E. K. Hutcheon, J. D. Zieske and X. Guo, Exp. Eye Res., 2019, 184, 183–191 CrossRef CAS PubMed.
- M. Ali, S. Y. Khan, S. Vasanth, M. R. Ahmed, R. Chen, C. H. Na, J. J. Thomson, C. Qiu, J. D. Gottsch and S. A. Riazuddin, Invest. Ophthalmol. Visual Sci., 2018, 59, 2437–2444 CrossRef CAS PubMed.
- S. Hatou, T. Sayano, K. Higa, E. Inagaki, Y. Okano, Y. Sato, H. Okano, K. Tsubota and S. Shimmura, Stem Cell Res., 2021, 55, 102497 CrossRef CAS PubMed.
- Y. K. Chang, J. S. Hwang, T. Y. Chung and Y. J. Shin, Stem Cells, 2018, 36, 1851–1862 CrossRef CAS PubMed.
- D. A. Blake, H. Yu, D. L. Young and D. R. Caldwell, Invest. Ophthalmol. Visual Sci., 1997, 38, 1119–1129 CAS.
- B. Y. Yue, J. Sugar, J. E. Gilboy and J. L. Elvart, Invest. Ophthalmol. Visual Sci., 1989, 30, 248–253 CAS.
- M. A. Mishan, S. Balagholi, T. Chamani, S. Feizi, Z. S. Soheili and M. R. Kanavi, J. Ophthalmic Vision Res., 2021, 16, 349–356 Search PubMed.
- K. Miyata, J. Drake, Y. Osakabe, Y. Hosokawa, D. Hwang, K. Soya, T. Oshika and S. Amano, Cornea, 2001, 20, 59–63 CrossRef CAS PubMed.
- S. Amano, Cornea, 2003, 22, S66–S74 CrossRef PubMed.
- M. Y. Pistsov, E. Sadovnikova and S. M. Danilov, Exp. Eye Res., 1988, 47, 403–414 CrossRef CAS PubMed.
- K. Engelmann and P. Friedl, In Vitro Cell. Dev. Biol., 1989, 25, 1065–1072 CrossRef CAS PubMed.
- K. Engelmann and P. Friedl, Cornea, 1995, 14, 62–70 CrossRef CAS PubMed.
- W. Li, A. L. Sabater, Y. T. Chen, Y. Hayashida, S. Y. Chen, H. He and S. C. Tseng, Invest. Ophthalmol. Visual Sci., 2007, 48, 614–620 CrossRef PubMed.
- Y. Ishino, Y. Sano, T. Nakamura, C. J. Connon, H. Rigby, N. J. Fullwood and S. Kinoshita, Invest. Ophthalmol. Visual Sci., 2004, 45, 800–806 CrossRef PubMed.
- J. Xiong, H. Kawagishi, Y. Yan, J. Liu, Q. S. Wells, L. R. Edmunds, M. M. Fergusson, Z. X. Yu, I. I. Rovira, E. L. Brittain, M. J. Wolfgang, M. J. Jurczak, J. P. Fessel and T. Finkel, Mol. Cell, 2018, 69, 689–698 CrossRef CAS PubMed.
- U. V. Jurkunas, Cornea, 2018, 37(Suppl 1), S50–S54 CrossRef PubMed.
- M. Beena, J. M. Ameer and N. Kasoju, ACS Omega, 2022, 7, 29634–29646 CrossRef CAS PubMed.
- C. Petsoglou, L. Wen, M. Hoque, M. Zhu, M. Valtink, G. Sutton and J. You, Exp. Eye Res., 2021, 208, 108613 CrossRef CAS PubMed.
- K. Burridge and M. Chrzanowska-Wodnicka, Annu. Rev. Cell Dev. Biol., 1996, 12, 463–518 CrossRef CAS PubMed.
- M. Enkhbat, B. Zhong, R. Chang, J. Geng, L. S. Lu, Y. J. Chen and P. Y. Wang, ACS Appl. Bio Mater., 2022, 5, 322–333 CrossRef CAS PubMed.
- Y. Zhang, Y. He, S. Bharadwaj, N. Hammam, K. Carnagey, R. Myers, A. Atala and M. Van Dyke, Biomaterials, 2009, 30, 4021–4028 CrossRef CAS PubMed.
- R. Numata, N. Okumura, M. Nakahara, M. Ueno, S. Kinoshita, D. Kanematsu, Y. Kanemura, Y. Sasai and N. Koizumi, PLoS One, 2014, 9, e88169 CrossRef PubMed.
- Y. T. Zhu, S. Tighe, S. L. Chen, Y. Zhang, S. Y. Chen, W. W. Y. Kao and S. C. G. Tseng, Ocul. Surf., 2023, 29, 301–310 CrossRef PubMed.
- M. Yamaguchi, N. Ebihara, N. Shima, M. Kimoto, T. Funaki, S. Yokoo, A. Murakami and S. Yamagami, Invest. Ophthalmol. Visual Sci., 2011, 52, 679–684 CrossRef CAS PubMed.
- C. Zhao, Q. Zhou, H. Duan, X. Wang, Y. Jia, Y. Gong, W. Li, C. Dong, Z. Li and W. Shi, Tissue Eng., Part A, 2020, 26, 1158–1168 CrossRef CAS PubMed.
- G. S. L. Peh, H. P. Ang, C. N. Lwin, K. Adnan, B. L. George, X. Y. Seah, S. J. Lin, M. Bhogal, Y. C. Liu, D. T. Tan and J. S. Mehta, Sci. Rep., 2017, 7, 14149 CrossRef PubMed.
- N. Okumura, K. Kakutani, R. Numata, M. Nakahara, U. Schlotzer-Schrehardt, F. Kruse, S. Kinoshita and N. Koizumi, Invest. Ophthalmol. Visual Sci., 2015, 56, 2933–2942 CrossRef CAS PubMed.
- S. K. Nayak and P. S. Binder, Invest. Ophthalmol. Visual Sci., 1984, 25, 1213–1216 CAS.
- G. H. Hsiue, J. Y. Lai, K. H. Chen and W. M. Hsu, Transplantation, 2006, 81, 473–476 CrossRef CAS PubMed.
- J. Y. Lai, K. H. Chen and G. H. Hsiue, Transplantation, 2007, 84, 1222–1232 CrossRef PubMed.
- G. Abbenante and D. P. Fairlie, Med. Chem., 2005, 1, 71–104 CrossRef CAS PubMed.
- R. C. de Oliveira and S. E. Wilson, Exp. Eye Res., 2020, 197, 108090 CrossRef CAS PubMed.
- Y. Tamura, H. Konomi, H. Sawada, S. Takashima and A. Nakajima, Invest. Ophthalmol. Visual Sci., 1991, 32, 2636–2644 CAS.
- M. C. Tsai and J. T. Daniels, Exp. Eye Res., 2021, 209, 108690 CrossRef CAS PubMed.
- T. Mimura, S. Yamagami, S. Yokoo, T. Usui, K. Tanaka, S. Hattori, S. Irie, K. Miyata, M. Araie and S. Amano, Invest. Ophthalmol. Visual Sci., 2004, 45, 2992–2997 CrossRef PubMed.
- N. Koizumi, Y. Sakamoto, N. Okumura, N. Okahara, H. Tsuchiya, R. Torii, L. J. Cooper, Y. Ban, H. Tanioka and S. Kinoshita, Invest. Ophthalmol. Visual Sci., 2007, 48, 4519–4526 CrossRef PubMed.
- H. J. Levis, G. S. Peh, K. P. Toh, R. Poh, A. J. Shortt, R. A. Drake, J. S. Mehta and J. T. Daniels, PLoS One, 2012, 7, e50993 CrossRef CAS PubMed.
- G. Niu, J. S. Choi, Z. Wang, A. Skardal, M. Giegengack and S. Soker, Biomaterials, 2014, 35, 4005–4014 CrossRef CAS PubMed.
- R. Watanabe, R. Hayashi, Y. Kimura, Y. Tanaka, T. Kageyama, S. Hara, Y. Tabata and K. Nishida, Tissue Eng., Part A, 2011, 17, 2213–2219 CrossRef CAS PubMed.
- J. Y. Lai, K. H. Chen, W. M. Hsu, G. H. Hsiue and Y. H. Lee, Arch. Ophthalmol., 2006, 124, 1441–1448 CrossRef CAS PubMed.
- T. Ide, K. Nishida, M. Yamato, T. Sumide, M. Utsumi, T. Nozaki, A. Kikuchi, T. Okano and Y. Tano, Biomaterials, 2006, 27, 607–614 CrossRef CAS PubMed.
- S. Kennedy, R. Lace, C. Carserides, A. G. Gallagher, D. A. Wellings, R. L. Williams and H. J. Levis, J. Mater. Sci. Mater. Med., 2019, 30, 102 CrossRef PubMed.
- M. Rizwan, G. S. L. Peh, H. P. Ang, N. C. Lwin, K. Adnan, J. S. Mehta, W. S. Tan and E. K. F. Yim, Biomaterials, 2017, 120, 139–154 CrossRef CAS PubMed.
- J. Zhao, M. Tian, Y. Li, W. Su and T. Fan, Acta Biomater., 2022, 147, 185–197 CrossRef CAS PubMed.
- J. H. Miner and P. D. Yurchenco, Annu. Rev. Cell Dev. Biol., 2004, 20, 255–284 CrossRef CAS PubMed.
- S. Scheele, A. Nystrom, M. Durbeej, J. F. Talts, M. Ekblom and P. Ekblom, J. Mol. Med., 2007, 85, 825–836 CrossRef CAS PubMed.
- A. V. Ljubimov, R. E. Burgeson, R. J. Butkowski, A. F. Michael, T. T. Sun and M. C. Kenney, Lab. Invest., 1995, 72, 461–473 CAS.
- A. Kabosova, D. T. Azar, G. A. Bannikov, K. P. Campbell, M. Durbeej, R. F. Ghohestani, J. C. Jones, M. C. Kenney, M. Koch, Y. Ninomiya, B. L. Patton, M. Paulsson, Y. Sado, E. H. Sage, T. Sasaki, L. M. Sorokin, M. F. Steiner-Champliaud, T. T. Sun, N. Sundarraj, R. Timpl, I. Virtanen and A. V. Ljubimov, Invest. Ophthalmol. Visual Sci., 2007, 48, 4989–4999 CrossRef PubMed.
- B. Bystrom, I. Virtanen, P. Rousselle, D. Gullberg and F. Pedrosa-Domellof, Invest. Ophthalmol. Visual Sci., 2006, 47, 777–785 CrossRef PubMed.
- J. Y. Lai, D. H. Ma, M. H. Lai, Y. T. Li, R. J. Chang and L. M. Chen, PLoS One, 2013, 8, e54058 CrossRef CAS PubMed.
- J. Y. Lai, Int. J. Mol. Sci., 2013, 14, 2036–2055 CrossRef CAS PubMed.
- S. Koo, R. Muhammad, G. S. Peh, J. S. Mehta and E. K. Yim, Acta Biomater., 2014, 10, 1975–1984 CrossRef CAS PubMed.
- K. Jain, R. Vedarajan, M. Watanabe, M. Ishikiriyama and N. Matsumi, Polym. Chem., 2015, 6, 6819–6825 RSC.
- B. K. Madathil, P. R. Kumar and T. V. Kumary, BioMed Res. Int., 2014, 2014, 450672 Search PubMed.
- M. Nitschke, S. Gramm, T. Gotze, M. Valtink, J. Drichel, B. Voit, K. Engelmann and C. Werner, J. Biomed. Mater. Res., Part A, 2007, 80, 1003–1010 CrossRef PubMed.
- J. Teichmann, M. Valtink, S. Gramm, M. Nitschke, C. Werner, R. H. Funk and K. Engelmann, Acta Biomater., 2013, 9, 5031–5039 CrossRef CAS PubMed.
- F. Z. Erkoc-Biradli, A. Ozgun, M. O. Ozturk-Oncel, M. Marcali, C. Elbuken, O. Bulut, R. Rasier and B. Garipcan, J. Tissue Eng. Regener. Med., 2021, 15, 244–255 CrossRef CAS PubMed.
- Q. Zhang, Z. Fang, Y. Cao, H. Du, H. Wu, R. Beuerman, M. B. Chan-Park, H. Duan and R. Xu, ACS Macro Lett., 2012, 1, 876–881 CrossRef CAS PubMed.
- L. Hartmann, K. Watanabe, L. L. Zheng, C. Y. Kim, S. E. Beck, P. Huie, J. Noolandi, J. R. Cochran, C. N. Ta and C. W. Frank, J. Biomed. Mater. Res., Part B, 2011, 98, 8–17 CrossRef PubMed.
- S. Rimmer, C. Johnson, B. Zhao, J. Collier, L. Gilmore, S. Sabnis, P. Wyman, C. Sammon, N. J. Fullwood and S. MacNeil, Biomaterials, 2007, 28, 5319–5331 CrossRef CAS PubMed.
- H. Kobayashi, Key Eng. Mater., 2007, 342–343, 209–212 CAS.
- Zainuddin, Z. Barnard, I. Keen, D. J. Hill, T. V. Chirila and D. G. Harkin, J. Biomater. Appl., 2008, 23, 147–168 CrossRef CAS PubMed.
- B. Yanez-Soto, S. J. Liliensiek, C. J. Murphy and P. F. Nealey, J. Biomed. Mater. Res., Part A, 2013, 101, 1184–1194 CrossRef CAS PubMed.
- A. J. Engler, L. Richert, J. Y. Wong, C. Picart and D. E. Discher, Surf. Sci., 2004, 570, 142–154 CrossRef CAS.
- F. Brandl, F. Sommer and A. Goepferich, Biomaterials, 2007, 28, 134–146 CrossRef CAS PubMed.
- H. N. Kim and N. Choi, BioChip J., 2019, 13, 8–19 CrossRef CAS.
- L. R. Stevens, K. J. Gilmore, G. G. Wallace and M. In Het Panhuis, Biomater. Sci., 2016, 4, 1276–1290 RSC.
- X. Qi, T. Su, M. Zhang, X. Tong, W. Pan, Q. Zeng, Z. Zhou, L. Shen, X. He and J. Shen, ACS Appl. Mater. Interfaces, 2020, 12, 13256–13264 CrossRef CAS PubMed.
- X. Qi, M. Zhang, T. Su, W. Pan, X. Tong, Q. Zeng, W. Xiong, N. Jiang, Y. Qian, Z. Li, X. He, L. Shen, Z. Zhou and J. Shen, J. Agric. Food Chem., 2020, 68, 3770–3778 CrossRef CAS PubMed.
- G. Lorenzo, N. Zaritzky and A. Califano, Food Hydrocolloids, 2013, 30, 672–680 CrossRef CAS.
- Z. Xu, Z. Li, S. Jiang and K. M. Bratlie, ACS Omega, 2018, 3, 6998–7007 CrossRef CAS PubMed.
- J. S. Seo, N. E. Tumursukh, J. H. Choi, Y. Song, G. Jeon, N. E. Kim, S. J. Kim, N. Kim, J. E. Song and G. Khang, Int. J. Biol. Macromol., 2023, 236, 123878 CrossRef CAS PubMed.
- C. J. Bettinger, R. Langer and J. T. Borenstein, Angew. Chem., Int. Ed., 2009, 48, 5406–5415 CrossRef CAS PubMed.
- M. Enkhbat, Y. C. Liu, J. Kim, Y. S. Xu, Z. Y. Yin, T. M. Liu, C. X. Deng, C. Zou, X. Xie, X. W. Li and P. Y. Wang, Adv. Ther., 2021, 4, 2100017 CrossRef.
- M. Ö. Öztürk-Öncel, F. Z. Erkoc-Biradli, R. Rasier, M. Marcali, C. Elbuken and B. Garipcan, Mater. Sci. Eng., C, 2021, 126, 112147 CrossRef PubMed.
- H. J. Sung, C. Meredith, C. Johnson and Z. S. Galis, Biomaterials, 2004, 25, 5735–5742 CrossRef CAS PubMed.
- P. Gain, R. Jullienne, Z. He, M. Aldossary, S. Acquart, F. Cognasse and G. Thuret, JAMA Ophthalmol., 2016, 134, 167–173 CrossRef PubMed.
- X. Y. Ng, G. S. L. Peh, G. H. Yam, H. G. Tay and J. S. Mehta, Int. J. Mol. Sci., 2023, 24, 12433 CrossRef CAS PubMed.
- S. Hatou and S. Shimmura, Jpn. J. Ophthalmol., 2023, 67, 541–545 CrossRef PubMed.
- Q. Zhou, Z. Li and H. Duan, Handb. Exp. Pharmacol., 2023, 281, 257–276 Search PubMed.
- S. So, Y. Park, S. S. Kang, J. Han, J. H. Sunwoo, W. Lee, J. Kim, E. A. Ye, J. Y. Kim, H. Tchah, E. Kang and H. Lee, Int. J. Mol. Sci., 2022, 24, 701 CrossRef PubMed.
- D. Zou, T. Wang, W. Li, X. Wang, B. Ma, X. Hu, Q. Zhou, Z. Li, W. Shi and H. Duan, Exp. Eye Res., 2024, 242, 109883 CrossRef CAS PubMed.
- G. S. Peh, Z. Chng, H. P. Ang, T. Y. Cheng, K. Adnan, X. Y. Seah, B. L. George, K. P. Toh, D. T. Tan, G. H. Yam, A. Colman and J. S. Mehta, Cell Transplant., 2015, 24, 287–304 Search PubMed.
- S. M. Thomasy, B. C. Leonard, M. A. Greiner, J. M. Skeie and V. K. Raghunathan, Prog. Retinal Eye Res., 2024, 99, 101234 CrossRef PubMed.
- E. Crouzet, Z. He, O. Ben Moussa, M. Mentek, P. F. Isard, B. Peyret, F. Forest, P. Gain, N. Koizumi, N. Okumura and G. Thuret, Acta Ophthalmol., 2022, 100, 690–699 CrossRef CAS PubMed.
- P. Sun, L. Shen, Y. B. Li, L. Q. Du and X. Y. Wu, Stem Cell Res. Ther., 2022, 13, 228 CrossRef CAS PubMed.
- F. Bandeira, G. T. Grottone, J. L. Covre, P. C. Cristovam, R. R. Loureiro, F. I. Pinheiro, R. P. Casaroli-Marano, W. Donato and J. A. P. Gomes, Int. J. Mol. Sci., 2023, 24, 2982 CrossRef CAS PubMed.
- E. N. Wong, V. H. X. Foo, G. S. L. Peh, H. M. Htoon, H. P. Ang, B. Y. L. Tan, H. S. Ong and J. S. Mehta, Cells, 2023, 12, 1167 CrossRef PubMed.
- C. Dong, D. Zou, H. Duan, X. Hu, Q. Zhou, W. Shi and Z. Li, Eye Vis., 2023, 10, 34 CrossRef PubMed.
- K. Numa, K. Imai, M. Ueno, K. Kitazawa, H. Tanaka, J. D. Bush, S. Teramukai, N. Okumura, N. Koizumi, J. Hamuro, C. Sotozono and S. Kinoshita, Ophthalmology, 2021, 128, 504–514 CrossRef PubMed.
- F. Filev, M. Stein, M. Schultheiss, A. D. E. Fitzek, J. Feuerstake, O. Engel and O. J. C. Hellwinkel, Graefes Arch. Clin. Exp. Ophthalmol., 2023, 261, 2593–2602 CrossRef PubMed.
|
This journal is © The Royal Society of Chemistry 2025 |
Click here to see how this site uses Cookies. View our privacy policy here.