Effects of fullerene C60 on the uptake of nitrogen and mineral elements in crops using synchrotron radiation micro-X-ray fluorescence spectrometry (SR-μXRF) and stable isotope labelling†
Received
10th April 2024
, Accepted 14th August 2024
First published on 1st October 2024
Abstract
The unique characteristics of fullerene (C60) have attracted great attention in the agricultural field. However, its potential effects on nitrogen sources and the uptake of various mineral nutrients required for plant growth remain unclear. In this study, we take advantage of the stable isotope 15N labeling technique combined with synchrotron radiation micro-X-ray fluorescence spectrometry (SR-μXRF) to investigate efficiently the effects of C60 (70–200 nm) on the uptake level of nitrogen and multiple mineral elements in three common crops (maize, wheat, and soybean). The results showed that C60 had different effects on the uptake of nitrogen and 15 mineral elements in different types of crops. C60 significantly decreased the uptake rate of nitrate nitrogen in maize and soybean by 52.4% and 66.1%, respectively, but it had no significant effects on the uptake of ammonium nitrogen. In contrast, C60 had no significant effect on the uptake of nitrate nitrogen in wheat, but it significantly increased the uptake rate of ammonium nitrogen by more than 3-fold. In addition, C60 tended to change the uptake of 15 mineral elements in wheat, maize and soybean, but significant differences were found only in the uptake of K, Ca and Fe in different tissues of three crops. Our results suggest that the joint analysis technology not only facilitates the simultaneous comparison of the uptake of total mineral nutrients (including organic and inorganic nutrients) in plants but also enables us to obtain the impact of nanomaterials on plant growth. C60 can improve the uptake of nitrogen and change mineral elements in crops, possibly avoiding damage to soils and the environment caused by the overuse of fertilizers and increasing the yield quantity and quality of crops.
Environmental significance
Fullerenes and their derivatives, as potential nutritional protectants or trace nutrient liquid additives, can affect the absorption and transport of nitrogen and mineral nutrients in plants and have great prospects for increasing crop yield. This study investigates the effects of C60 on the uptake of nitrogen and 15 mineral elements in typical crops such as maize, wheat, and soybean using the stable isotope 15N labelling technique and synchrotron radiation micro-X-ray fluorescence (SR-μXRF) technique. This innovative joint analysis technology can efficiently, non-destructively and simultaneously monitor and distinguish for changes in nitrogen sources and multiple mineral nutrients without any complex sample pretreatment, which will greatly accelerate the simultaneous fingerprints of trace or mineral nutrients in plants.
|
1. Introduction
Engineered nanomaterials, because of their unique chemical and physical properties such as high ratio of surface area to volume, extraordinary electronic and optical attributes, capability to engineer electron transfer, highly reactive surfaces, etc., have been used in biomedical, electrical and industrial fields.1–3 In particular, they have been also expanded to increase the quantity and quality of agricultural crop products.4–6 Nanomaterials include carbon nanomaterials (CNMs) and non-carbon nanomaterials (nCNMs). Some reports indicate that the utilization of nCNMs, such as metal and metal oxide nanoparticles, can have detrimental effects on plants. For instance, the application of silver nanoparticles (AgNPs) exhibits toxicity towards plant growth7,8 and leads to their release into the environment, causing ecological issues.9 Furthermore, a considerable amount of research has shown that different metal nanomaterials have a negative impact on plant growth.10–12 CNMs, composed of carbon atoms, exhibit excellent biocompatibility. They are not considered as environmental pollutants in aqueous solutions.13 Moreover, the small size allows carbon nanomaterials to easily cross cell walls and membranes to enter plant cells. Specific CNMs possess the ability to impede plant uptake of organic pollutants,14,15 enhance plant photosynthesis, and facilitate crop growth.16 However, the widespread applications of these nanomaterials have triggered some concerns about their potential adverse effects on both the environment and human health. Worldwide, there have been numerous reports to obtain insight on new interactions of carbon nanomaterials with plants.17–25 Published research reported positive or negative effects of CNMs on plant toxicity, biomass accumulation, development stages, nutritional and pharmaceutical compound accumulation and even some contradictory effects.18,19 For example, C60 (0.09 mg L−1, 98 nm) can reduce the contents of photosynthetic products and chlorophyll, eventually causing a sub-lethality in Scenedesmus obliquus and inhibiting the growth of Lemna gibba (1–10 mg L−1, 29–38 nm).20,21 Carboxylated C60 (0.01 mg mL−1, 146 nm) can reduce cell viability and the number of mitochondria, impair cellular function, and increase the levels of intracellular reactive oxygen species, thereby inhibiting the growth of tobacco BY-2 suspension cells and Arabidopsis seedlings.22 Multi-walled carbon nanotubes (MWCNTs) (0, 20, 200, 100, 2000 mg L−1 and 200 nm) can significantly inhibit the root and shoot lengths in lettuce, red spinach, cucumber and rice.23 However, some studies report that carbon nanomaterials can promote the growth of plants. For example, MWCNTs (10–40 μg mL−1) can significantly increase the germination rate and improve the growth of tomato seedlings.24 Fullerenol C60(OH)20 (0.943, 4.72, 9.43, 10.88, 47.2 nM, 1.5 ± 0.2 nm and 5.0 ± 0.7 nm) can increase the water content, plant biomass and fruit yield in bitter melon (Momordica charantia).25 Single-walled carbon nanotubes (28, 160, 900, 5000 mg L−1, 8 nm) have no effects on the growth of cabbage and carrots but significantly enhance the elongation of roots in onion and cucumber.26 Obviously, the small size of carbon nanomaterials is beneficial for their transmission into plant cells; however, the biological effects and potential impact of CNMs on crops is still challenging, which is not only related to the concentration of nanomaterials but also closely related to their shape, surface modification and the microenvironment of crops. A thorough understanding of the ecological effects of CNMs is required.
Fullerene (C60), as one of the most typical carbon nanomaterials, has unique physical and chemical properties based on its unique cage structure and surface modified properties and has been applied for crop growth, soil remediation and environmental pollution control, etc. in the agricultural field.15,27–30 The effects of C60 on plant growth have been reported. These studies focused on plant growth, physiological responses, and changes in biomolecular function. For example, Kumar et al. studied the effects of fullerenes on seed germination, and the results showed that fullerenes (<100 mg L−1) did not affect seed germination, but at higher concentrations (200 mg L−1) would cause toxicity to seedling growth.31 Our previous studies showed that 13C-labelled C60 (20 mg L−1, 100 mg L−1) could be absorbed by rice root and affected rice growth by reducing phytohormone levels in rice without significant concentration effect.19 Stable isotope 13C-labelled fullerenols (2.5, 5, 10 μg mL−1, and 95 nm) can enter the roots of wheat, promote root elongation and enhance the synthesis of chlorophyll.28 Avanasi et al. investigated the absorption and transport of 14C-C60 (1.01 μg g−1, ∼1500 nm) in different plants and its biodegradation in different soils, and studied the absorption of C60 by radish through a hydroponic system. The results showed that C60 can be absorbed by plant roots and transported to underground parts of plants.29 Although the particle size of C60 in the study was larger than 1500 nm, 14C-labeled C60 could be detected in plant roots. De La Torre-Roche et al. studied the effect of fullerenes (500–5000 mg L−1, 1450–1900 nm) on the immobilization of pesticide residues.15 They found that pesticide accumulation varies greatly with crop species and carbon nanomaterial type/concentration. Zucchini and tomato growth was unaffected by carbon nanomaterial co-exposure, while C60 at 500 mg kg−1 reduced corn and soybean biomass. Meanwhile, the effect of C60 on the absorption of organic pollutants in plants has also been reported.30,32 C60 nanoparticles (1670 mg kg−1) had no impact on the biomass of plants and had little impact on weathered dichlorodiphenyldichloroethylene bioaccumulation in plants.30 C60 nanoparticles (2–15 mg L−1, ∼50 nm) did not result in any acute toxicity to plants and increased plant uptake of trichloroethylene.32 Then, for the impact of fullerenes on plants, although there are complex concentration effects, even if the particle size is relatively large and the concentration is suitable, it will not damage the growth of crops. However, the effects of C60 on the uptake of different nitrogen and mineral nutrients in crops have been seldom reported. The simple, real-time and non-destructive detection or evaluation methods of nitrogen nutrient elements and a variety of mineral nutrient elements are also full of challenges.
To grow and develop, plants need a variety of nutrients; nutrients are mainly absorbed through the root system and then transported to other parts of the plant. Mineral elements play an important role in the electron transport chain, the synthesis of biological macromolecules (enzymes, hormones, vitamins, nucleic acids, etc.), and other physiological and biochemical functions. The changes in the contents of mineral elements can remarkably influence the growth, development or reproduction, physiological processes in plants. Nitrogen is one of the essential elements for plant growth and development, and it is the main component of proteins, nucleic acids, chlorophyll, vitamins, alkaloids, and hormones in plants.33–35 Nitrogen deficiency or excess has become an important factor limiting crop production and quality.36 Plants can absorb and utilize several forms of nitrogen in soils, including ammonia nitrogen (NH4+-N), nitrate nitrogen (NO3−-N), and some organic nitrogen with low molecular weights.37,38 However, many chemical products, such as pesticides, fertilizers and nutrient additives, have been widely used to increase crop yields. Their excessive use has disrupted the balance of soil minerals and reduced soil fertility, which substantially influences the contents of ammonia nitrogen, nitrate nitrogen and organic nitrogen in the soil and degraded the agricultural environment.39,40 Appropriate application of nitrogen fertilizers is a key measure to achieve high crop yields and reduce environmental pollution.41,42 Hao et al. found that carbon nanomaterials can increase the activities of some enzymes related to nitrogen metabolism in maize leaves and roots, thus promoting nitrogen utilization and plant growth.43 Zhao et al. showed that the application of nano-carbon had a great promotion effect on the soil urease activity of different soil types.44 Recently, studies have shown that nanomaterials play an important role in the absorption of mineral elements in plants. Abdel Latef et al. found that nTiO2 (0.01%, 0.02%, 0.03%, and 25 nm) supplements significantly increased the activity of enzymatic antioxidants and the levels of soluble sugars, amino acids and proline in salt-affected plants.45 Suriyaprabha et al. found that silica nanoparticles (15 g L−1, 20–40 nm) can be absorbed by the roots of maize (Zea mays L.) and then migrate and accumulate in the leaves, which increase the total leaf protein content, improve the absorption of trace elements (such as copper, iron, manganese, and zinc) and finally improve the growth performance of maize.46 Obviously, the uptake and transport (translocation, transformation) of nutrients in plants is of great significance for plant physiology and growth. In addition, fullerenes and their derivatives possess the potential ability to effectively permeate cell membranes and serve as carriers for both macro and trace elements.47 Recently, G. G. Panova et al. reported the positive effects of water-soluble fullerene derivatives (1 mg kg−1, 10 mg kg−1, and 100 mg kg−1) on the content of macro- and microelements in the soil and in plants (Chinese cabbage, tomato, and cucumber) physiological state, growth, and element content.48 Fullerene derivatives could activate nitrogen transformation in the soil, enhance the process of nitrification, and promote the migration of some macro- and microelements (such as selenium and zinc elements) from soil to cucumber leaves at appropriate concentrations and improve the physiological state and growth of plants. They believe that fullerene and its derivatives, as a nanopreparation for soil or vegetation, a nutrient protectant, or an additive for trace nutrient element liquids, have good perspectives for improving crop production.48,49 Therefore, it is necessary to study the effects of C60 on the acquisition and retention of nitrogen and mineral elements in typical crops. The study is expected to explore the potential agricultural application of C60 and to understand the role of C60 in regulating nutrient cycling in agroecosystems and improving crop yields.
Wheat, maize and soybean have different root systems; they are sensitive to changes in environmental conditions and pollutants, which enables them to be used as environmental and biological indicators for assessing the environmental safety of nanomaterials.50 Plant roots have a large surface area to absorb mineral nutrients (inorganic ions) from the soil. After absorption on the roots, mineral nutrients are transported to other parts of the plant, where they are utilized to perform various biological functions. In this study, we investigated the effects of C60 on the uptake of nitrogen and 15 mineral elements in three crops using the stable isotope 15N labelling technique and synchrotron radiation micro-X-ray fluorescence (SR-μXRF) technique and attempted to unravel the underlying mechanisms. The stable isotope labelling technique is a simple but powerful method with high accuracy, low detection limit, nonradioactive nature, high stability, and suitability in long-term tracing, which have been used to quantify and trace the biological behaviors of nanomaterials in complex biota and ecological environments.28,51–54 Mineral nutrient elements in plants are detected by conventional analysis technology, such as atomic absorption spectrometry, atomic fluorescence spectrometry, inductively coupled plasma mass spectrometry, etc., which often have the disadvantages of complex sample process, long time, large amounts of samples, poor reproducibility and so on. The SR-μXRF technology provides an important tool for monitoring the multi-elemental distribution in the microscopic tissue of organisms at low levels, which has the advantages of high sensitivity, real-time, non-destructive, and simultaneous analysis of multiple elements.55 We processed plants in a simple way and simultaneously obtained the distribution information of multiple mineral nutrients in three crops by the SR-μXRF technology. Combined with isotope labelling technology, we distinguished three forms of nitrogen sources and intuitively obtained the influence of C60 on the nitrogen absorption level of different types of crops. This combined analysis technique allows us to quickly and efficiently study the effects of CNMs on nitrogen and mineral element uptake in plants. The study is expected to provide new ideas for the agricultural application of C60 to regulate crop nutrients and promote crop productivity.
2. Materials and methods
2.1 Materials and reagents
Fullerene C60 (99%) was purchased from Suzhou Dade Carbon Nanotechnology Co., Ltd (Suzhou, China). Seeds of soybean, maize and wheat were purchased from the Institute of Crop Science, Chinese Academy of Agricultural Sciences (Beijing, China). Ammonium sulfate and potassium nitrate, with or without 15N labeling, were purchased from Shanghai Maclin Biochemical Technology Co., Ltd. (Shanghai, China). Soils were taken from a farmland in the suburb of Beijing (116.23′′E, 39.99′′N). XRF tape (TF-500) was purchased from DHJ Analysis Co., Ltd. (Beijing, China). The chemicals and reagents used in this study were of analytical grade or chromatographic grade.
2.2 Characterization of C60
The morphology and size of C60 in water were characterized using a scanning electron microscope (S-4800, Hitachi, Tokyo, Japan) and a nanosizer (Zetasizer Nano ZS90, Malvern, UK), respectively. To measure the size, C60 was dispersed in water at a concentration of 0.2 mg mL−1 and then sonicated for 5 min to make it disperse well in water. The size distribution was determined using the Zetasizer Nano ZS90, and a small amount of C60 aqueous solution was dropped on the silicon wafer and given surface spray gold treatment, and then observed by scanning electron microscopy. Furthermore, the cage-like carbon molecular structure of C60 was characterized using matrix-assisted laser desorption/ionization time-of-flight mass spectrometry (MALDI-TOF-MS, Autoflex III, Bruker, Germany). To avoid the interference of matrix, no matrix was used here. In addition, to increase the signal-to-noise ratio, C60 was directly dispersed in toluene, deposited on a grid and dried at room temperature. Toluene was used here as solvent because it can not only increase the solubility of C60 but also greatly reduce the diffusion of C60 on the smooth metal surface due to its high volatility, thereby ensuring the concentration and enrichment of C60 on the target and improving the signal-to-noise ratio.
2.3 Experimental
2.3.1 C60 treatment.
C60 (500 mg) was mixed with 50 g of air-dried soils and placed in a 50 mL large plastic centrifuge tube. The concentration of C60 was 10.0 mg g−1. Soil without C60 was used as the control. The seeds of maize, wheat, and soybean were germinated. Healthy seedlings were selected and planted in centrifuge tubes, with buds facing up and roots facing down, 4 replicates per group (a total of 48 tubes). Seedlings were irrigated with water at soil water-holding capacity of 70% and grown under a photoperiod of 12 h light/12 h dark at 25/20 °C day/night and 80% humidity for 30 days. After treatment, seedlings with their roots were collected and rinsed with water 3–5 times. The plants were labelled with 15N as described in section 2.3.2. After labelling, roots, stems and leaves were separated, and their fresh weights were measured. Then, they were lyophilized for 48 h to constant weight, and their dry weights were measured. Finally, the dried roots, stems and leaves were ground into powder, which was subject to synchrotron radiation micro-X-ray fluorescence analysis to observe the changes in the contents of mineral elements.
2.3.2 Stable isotope 15N labelling.
Two forms of nitrogen, including ammonia nitrogen and nitrate nitrogen, were labeled with stable isotope 15N. Solutions of ammonium sulfate and potassium nitrate, with or without 15N labelling, were separately prepared at a nitrogen content of 100 μmol N per L. A 100 μmol N per L concentration level for nitrogen is employed to simulate plant uptake under high-nitrogen conditions.56 The higher concentration level enables us to better observe and compare plants' nitrogen absorption levels when exposed to different nitrogen sources within a high-nitrogen background. To prevent potential transformation of nitrogen and maintain the membrane stability of root cells, the solution was supplemented with penicillin (10 mg L−1) and CaCl2·2H2O (100 μmol L−1). After 30 days of cultivation as described above, the plants with the roots were collected and washed. The roots were placed in 15 mL centrifuge tubes containing 100 μmol N per L ammonium sulfate and potassium nitrate, with or without 15N labeling, and incubated for 4 h. After incubation, roots, stems and leaves were separated and washed with 50 mmol L−1 KCl and distilled water. They were dried in an oven at 70 °C for 48 h, weighed, and ground to powder in a ball mill. The nitrogen content and the ratio of isotope (15N/14N) were determined using a MAT 253 stable ratio mass spectrometer (Thermo Fisher, USA) coupled with a Flash 2000 HT elemental analyzer (Thermo Fisher, USA). The uptake rate of nitrogen was calculated using the following equation (eqn (1)). |  | (1) |
where N (μg N per g d.w. root per h) is the nitrogen absorption rate, which indicates the contents of nitrogen absorbed per gram of dry weight tissue per hour, APEsample is the atom percent excess of 15N in plant tissues, which is calculated by subtracting that of 15N in control plant tissues from the percentage of 15N in labelled plant tissues, d.w. refers to the dry weight of plant tissue, and Ncontent refers to the percentage content of nitrogen in plant tissues. APEadded is the atom percent excess of 15N in the added nitrogen source, which is calculated by subtracting that of 15N in the atmosphere from the percentage of 15N in the added nitrogen source, and t refers to the labelling time.
2.3.3 Measurement of mineral elements using SR-μXRF.
The pressed pellets (6 mm in diameter and 30–100 μm in thickness) of dried tissue powders (about 20.0 mg) were prepared using a PP-20S automatic powder tablet press machine (Tianjin Jingtuo Instrument, Tianjin, China) under about 700 MPa for 30 s. The pellets were analyzed using SR-μXRF with an electron beam energy of 2.5 GeV, a current intensity of up to 120 mA and an incident beam slit of 50 μm × 50 μm. A silicon–lithium semiconductor detector (type Link-ISIS) was used to obtain the spectra, with the sample at 45° to the detector and at 90° to the incident beam. The distance between the beryllium window and the sample was 80 mm. The spectra were recorded and analyzed using PyMca, with a spectral collection time of 10 s. On each sample, a total of 70 points were randomly selected and measured to obtain the raw data of trace elements (Ar, K, Ca, Ti, Cr, Mn, Fe, Ni, Cu, Zn, Ga, As, Se, Kr, and Br) in plant tissues.
2.4 Data analysis
The data of samples were processed using PyMca 5.5.5. After selecting mineral elements of interest, the fit area values of the K energy layer were normalized due to the variations in measurement conditions (such as different incident light intensities, different measurement times, and different geometric structures). The experiment was repeated in triplicate. Data were processed in Excel, and figures were generated using Origin 2020 (OriginLab, Massachusetts, USA). Data are expressed as mean ± SD (n = 3).
3. Results and discussion
3.1 Characterization of C60
The absorption of nanomaterials by plants is affected by the particle size, shape, exposure conditions and concentration of nanomaterials, and the particle size is one of the main reasons affecting plant absorption. Slomberg et al. reported that SiO2 nanomaterials up to 200 nm could be absorbed by the roots of Arabidopsis thaliana.57 Larue et al. investigated in detail the accumulation of TiO2 with different particle sizes (36–140 nm) in wheat (Triticum aestivum). They found that the smaller the particle size (<36 nm), the easier to be accumulated in the root system and distributed throughout the plant tissue without dissolution or transformation, while nanoparticles of 36–140 nm accumulated in the root substance of wheat but did not transfer to the branches, and nanoparticles >140 nm did not accumulate in the root system of wheat.58 The size of nanoparticles influences their ability to enter plant cells, affecting their transport and crop growth.59 For carbon-based nanomaterials, due to their unique nano characteristics and exposure environment, from several nanometers to several micrometers, they may enter the plant roots and affect the physiological functions and growth of plants. Thus, mentioning particle size is essential for a comprehensive understanding of the effects of C60 nanomaterials on plants. Scanning electron microscopy (SEM) images (Fig. 1A) showed that C60 could form very small aggregates in water, with diameters ranging from 70 to 200 nm. As shown in Fig. 1B, the hydrated diameters of C60 in water were measured as around 131 nm by dynamic light scattering (DLS) measurement, which had a lognormal hydrated particle size distribution, mainly ranging from 68 to 220 nm. C60 nanoparticles in this size range may be absorbed by plant roots and transported to the aboveground part of the plant, affecting the potential internal physiology of the plant. Besides, most of the large size C60 nanoparticles may also remain outside the roots and enriched in the soil, influencing the availability of mineral elements in the soil,48 which in turn may affect the absorption and transport of these mineral elements in plants. Additionally, there was a single characteristic peak on the MALDI-TOF-MS spectrum of C60 at m/z = 719.59 (Fig. 1C), which is very close to the theoretical m/z of 720 for C60 and is consistent with other literature.60–62
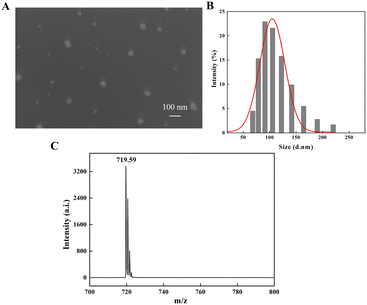 |
| Fig. 1 Characterization of C60. (A) SEM image, (B) DLS image, (C) time-of-flight mass spectrum. | |
3.2 Effects of C60 on the biomass of three crops
C60 affected the biomass of three crops (maize, soybean and wheat) to a certain extent, but had no significant change or toxic effects (Fig. 2 and S1 and Tables S1–S3 in the ESI†). In both control and C60 treatment groups, the crops were cultivated under the same conditions for 30 days, and to study the effects of C60 on the uptake of different forms of N, they were separated from the soils and incubated in water containing two forms of N (ammonia N and nitrate N) with or without 15N labelling for 4 h. The addition of nitrogen tended to promote the growth of maize compared with the water control group, but no significant changes in dry weights were found. Our results showed that C60 did not remarkably improve the growth of three crops, only with significant changes in dry weights found in individual groups. As for maize (Fig. 2A), the dry weight of the whole plant in control-H2O was significantly higher than that in C60-H2O, and there was no significant difference in dry weights of the whole plant, underground (roots), and aboveground (stem–leaves) parts of the plant between other groups. As for wheat (Fig. 2B), the dry weight of underground parts in C60-NO3− decreased significantly compared to that in control-NO3−, while there was no significant difference in other groups. As for soybean (Fig. 2C), the dry weights of aboveground parts (stem–leaves) of the plant and the whole plant in C60-NO3− increased significantly compared to that in control-NO3−, while there was no significant difference in other groups. Therefore, the inhibitory effects of high concentrations of C60 on crop growth could be ignored, and the effects of C60 on the uptake of N and mineral elements could be clearly determined.
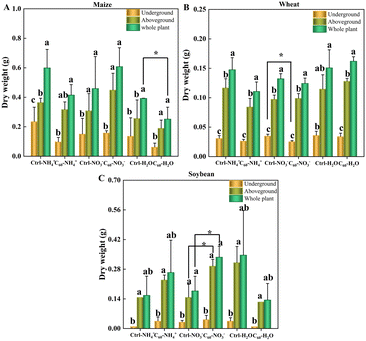 |
| Fig. 2 Effects of C60 on the biomass of three crops. (A) Maize, (B) wheat, and (C) soybean. Data are expressed as mean ± SD (n = 4). Differences were considered statistically significant at P < 0.05. Ctrl: control. The lowercase letters (a–c) represent statistically significant differences between variables. * indicates significant difference between the control and the C60 treatment. | |
3.3 Effects of C60 on the uptake of N element by three crops
Maize, wheat and soybean had different uptake rates of nitrogen: soybean (ammonia-N 150.41 ± 10.01, nitrate-N 71.19 ± 21.25 μg N per g d.w. tissue per h) > maize (ammonia-N 36.26 ± 9.7, nitrate-N 78.71 ± 22.11 μg N per g d.w. tissue per h) > wheat (ammonia-N 12.39 ± 4.02, nitrate-N 31.95 ± 4.42 μg N per g d.w. per tissue h). Soybean has an intrinsic nitrogen fixation ability, and its root cavities are similar in size to those in maize. In both soybean and maize, the nitrogen uptake capacity of the underground parts (roots) is about an order of magnitude higher than that of stems and the aboveground parts (stem–leaves). In contrast, the root cavities of wheat are relatively small, and the nitrogen uptake capacity of underground is lower than that of aboveground parts, indicating that wheat has the lowest nitrogen uptake capacity among these three crops. As shown in Fig. 3 and Tables S4–S6 in the ESI,† C60 had different effects on the uptake rates of two forms of N in these three crops. In untreated maize (Fig. 3A), the NO3−-N uptake rate was higher than the NH4+-N uptake rate, and the N uptake rate of underground was significantly higher than that of aboveground parts of maize. After C60 treatment, the NO3−-N uptake rate significantly decreased from 78.71 ± 22.11 μg N per g d.w. tissue per h to 37.49 ± 7.39 μg N per g d.w. tissue per h, but C60 had no significant effects on the NH4+-N uptake rates. As for the untreated wheat (Fig. 3B), the NO3−-N uptake rate was higher than the NH4+-N uptake rate, and the NO3−-N uptake rate of aboveground pats was also significantly higher than the NH4+-N uptake rate of aboveground parts of wheat. After C60 treatment, the NH4+-N uptake rate significantly increased from 12.39 ± 4.02 μg N per g d.w. tissue per h to 50.92 ± 16.38 μg N per g d.w. tissue per h, but there were no significant changes in the NO3−-N uptake rates. In untreated soybean (Fig. 3C), the NH4+-N uptake rate was higher than the NO3−-N uptake rate, and the N uptake rate of underground was significantly higher than that of aboveground parts. After C60 treatment, the NO3−-N uptake rate significantly decreased from 71.19 ± 21.25 μg N per g d.w. tissue per h to 24.13 ± 8 μg N per g d.w. tissue per h, but there were no significant changes in the NH4+-N uptake rates.
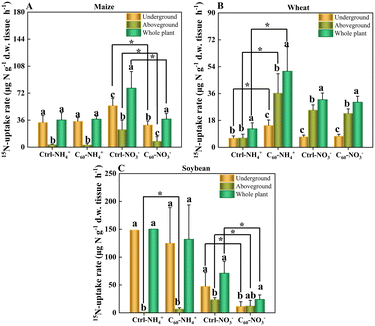 |
| Fig. 3 Effects of C60 on the nitrogen uptake rate of different crops. (A) Maize, (B) wheat, and (C) soybean. Data are expressed as mean ± SD (n = 4). Differences were considered statistically significant at P < 0.05. Ctrl: control. The lowercase letters (a–c) represent statistically significant differences between variables. * indicates significant difference between the control and the C60 treatment. | |
Here, our results showed that C60 could affect the uptake of nitrogen in three crops depending on crop types and nitrogen forms. In maize and soybean, C60 could significantly reduce the uptake of nitrate-N. In wheat, however, C60 could significantly increase the uptake of ammonia-N. This result may be because C60 interferes with the activity of nitrate reductase, glutamate dehydrogenase and glutamine synthetase,63 thus converting inorganic nitrogen into organic nitrogen, resulting in the conversion and utilization of nitrate nitrogen and ammonia nitrogen in plants. The investigation showed that C60 could regulate the ability of crops to absorb different forms of nitrogen, which was expected to optimize the application of nitrogen fertilizer in different crops.
3.4 Effects of C60 on the uptake of mineral elements by three crops
We measured the levels of 15 mineral elements in the tissues of three crops using SR-μXRF. As shown in Fig. 4, the SR-μXRF spectrum clearly showed the presence of 15 mineral elements in the underground and aboveground parts of plants. In three crops, the uptake levels of mineral elements in their underground were higher than the aboveground parts (Fig. 5 and 6). C60 treatment had different impacts on the uptake levels of mineral elements in different crops. In maize, C60 had little effects on the uptake levels of most of the mineral elements in the underground and aboveground parts of plant, but it caused a significant increase in the uptake level of Fe in the aboveground parts of plant (Fig. 6). In wheat, except for a significant decrease in the uptake level of Fe in the underground parts (Fig. 5) and an increase in the uptake level of K in the aboveground parts (Fig. 6), C60 had almost no effect on the uptake levels of the other mineral elements in the plants. Meanwhile, in soybean, C60 had no significant effects on 15 mineral elements in the underground parts of the plant. On the other hand, C60 significantly increased the level of Fe but significantly decreased the levels of Ca in the aboveground parts of soybean, with no effects on other mineral elements. The results suggested that C60 could change the uptake of mineral elements in the underground but exhibited differential effects on the uptake of mineral elements in aboveground parts. These results suggested that exposure to a certain concentration of fullerenes in soil can affect the absorption and transport of useful mineral elements (such as K, Ca, and Fe) by plants. Panova et al. had confirmed that the use of fullerenes and their derivatives will allow mineral elements to enter the plant, affecting the metabolic process and photosynthesis of the plant.49 Additionally, due to the increase of relevant mineral elements in the plant, the osmotic pressure in its cells may lead to more efficient water transport, thus affecting the plant.48 Moreover, C60 had more significant effects on the uptake of N and mineral elements in soybean than that of maize and wheat, possibly because soybean processes an intrinsic nitrogen fixation ability and has a deeper and more vertically oriented root system.64 It is reported that nanomaterials enter the plants mainly through the roots,65 so C60 had a more profound effect on the uptake of N and mineral elements in soybean.
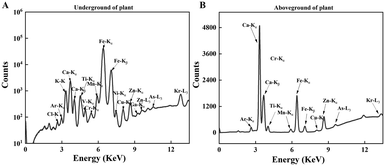 |
| Fig. 4 The SR-μXRF spectrum showing the presence of mineral elements in the (A) underground and (B) aboveground parts of plant. | |
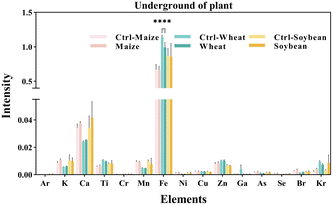 |
| Fig. 5 Effects of C60 on the levels of mineral elements in the underground parts of maize, wheat and soybean plants. * indicates significant difference between the control and the C60 treatment at P < 0.05. | |
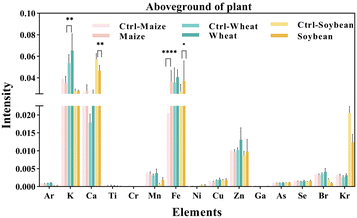 |
| Fig. 6 Effects of C60 on the levels of mineral elements in the aboveground parts of maize, wheat and soybean plants. * indicates significant difference between the control and the C60 treatment at P < 0.05. | |
Conclusions
For the uptake level of nitrogen and multiple mineral elements in plants, the advantage of the stable isotope labelling technique combined with synchrotron radiation micro-X-ray fluorescence spectrometry (SR-μXRF) can efficiently and non-destructively provide test data for almost all changes in element content without any complex sample pretreatment. Although C60 did not significantly improve the growth and invoke physiological responses in maize, wheat and soybean, it could regulate the uptake of nitrogen and mineral elements by these crops. The effects of C60 on the uptake of nitrogen and mineral elements differed in different crops, even in their tissues (roots and stems–leaves). In maize and soybean, C60 could significantly reduce the uptake of nitrate-N. In wheat, however, C60 could significantly increase the uptake of ammonia-N. In addition, C60 had little effect on the uptake of mineral elements in maize and wheat, but it could remarkably affect the uptake of some mineral elements in soybean. Our results demonstrated that C60 had great potential to be used as a fertilizer to improve the use efficiency of nitrogen fertilizers and mineral element fertilizers in agriculture. Simultaneously, we have also explored an analytical method that utilizes stable isotope labelling technology combined with synchrotron radiation micro-X-ray fluorescence spectrometry for comprehensive testing of the changes of nutrient elements in plants, which will greatly accelerate the fingerprint spectrum analysis of all of the microscale or mineral nutrient elements in plants. Accordingly, this research offers novel insights into utilizing fullerenes for regulating crop nutrients to improve productivity while also presenting prospects for applying nano-carbon materials in agricultural production.
Data availability
The data supporting this article have been included as part of the ESI.†
Conflicts of interest
The authors declare that they have no known competing financial interests or personal relationships that could have appeared to influence the work reported in this paper.
Acknowledgements
This work was supported by the National Basic Research Programs of China (2021YFA1200904), the National Natural Science Foundation (No. 11475194 and 11675189), and the Beijing Natural Science Foundation (No. 2202065 and 2152038). The directional institutionalized scientific research platform relies on the Beijing Synchrotron Radiation Facility of the Chinese Academy of Sciences. The SR-μXRF beam time was granted by the 4W1B beamline of Beijing Synchrotron Radiation Facility, Institute of High Energy Physics, Chinese Academy of Sciences. The staff members of 4W1B are acknowledged for their support in measurements and data reduction. We are grateful to Prof. Yongquan Cui at Huazhong University of Science and Prof. Xingliang Xu at the Institute of Geographic Sciences and Natural Resources Research, CAS, for their enthusiastic support and data discussion and analysis.
Notes and references
- V. L. Colvin, The Potential Environmental Impact Of Engineered Nanomaterials, Nat. Biotechnol., 2003, 21, 1166–1170 CrossRef CAS.
- R. Bakry, R. M. Vallant, M. Najam-ul-Haq, M. Rainer, Z. Szabo, C. W. Huck and G. K. Bonn, Medicinal applications of fullerenes, Int. J. Nanomed., 2007, 2, 639–649 CAS.
- P. Chaudhuri, A. Paraskar, S. Soni, R. A. Mashelkar and S. Sengupta, Fullerenol−cytotoxic conjugates for cancer chemotherapy, ACS Nano, 2009, 3, 2505–2514 CrossRef CAS.
- H. S. Abbas, A. M. Mahmoud, R. A. Wahed, M. A. A. Elsantawy, N. M. Hamdy, S. E. S. Ismail and M. A. Nabil, Prospects of using bioactive compounds in nanomaterials surface decoration and their biomedical purposes, Int. Nano Lett., 2022, 12, 125–138 CrossRef CAS.
- A. Saravanan, P. S. Kumar, S. Karishma, D.-V. N. Vo, S. Jeevanantham, P. R. Yaashikaa and C. S. George, A review on biosynthesis of metal nanoparticles and its environmental applications, Chemosphere, 2021, 264, 128580 CrossRef CAS PubMed.
- S. Sena, S. J. Ochatt and V. Kumar, Application of green synthesized nanoparticles in medicinal plant research: Revisiting an emerging eco-friendly approach, Plant Cell, Tissue Organ Cult., 2023, 155, 345–384 CrossRef.
- D. K. Tripathi, S. Singh, S. Singh, P. K. Srivastava, V. P. Singh, S. Singh, S. M. Prasad, P. K. Singh, N. K. Dubey, A. C. Pandey and D. K. Chauhan, Nitric oxide alleviates silver nanoparticles (AgNps)-induced phytotoxicity in Pisum sativum seedlings, Plant Physiol. Biochem., 2017, 110, 167–177 CrossRef CAS.
- A. Yan and Z. Chen, Impacts of Silver nanoparticles on plants: A focus on the phytotoxicity and underlying mechanism, Int. J. Mol. Sci., 2019, 20, 1003 CrossRef CAS PubMed.
- J. Dobias and R. Bernier-Latmani, Silver release from silver nanoparticles in natural waters, Environ. Sci. Technol., 2013, 47, 4140–4146 CrossRef CAS.
- M. Ruffini Castiglione, L. Giorgetti, C. Geri and R. Cremonini, The effects of nano-TiO2 on seed germination, development and mitosis of root tip cells of Vicia narbonensis L. and Zea mays L, J. Nanopart. Res., 2011, 13, 2443–2449 CrossRef CAS.
- Y. Tang, S. Wu, L. Huang, J. Head, D. Chen and I. Kong, Phytotoxicity of metal oxide nanoparticles is related to both dissolved metals ions and adsorption of particles on seed surfaces, J. Pet. Environ. Biotechnol., 2012, 03, 1–6 Search PubMed.
- P. Landa, T. Cyrusova, J. Jerabkova, O. Drabek, T. Vanek and R. Podlipna, Effect of metal oxides on plant germination: Phytotoxicity of nanoparticles, bulk materials, and metal ions, Water, Air, Soil Pollut., 2016, 227, 448 CrossRef.
- P. C. Ke and R. Qiao, Carbon nanomaterials in biological systems, J. Phys.: Condens. Matter, 2007, 19, 373101 CrossRef.
- E. J. Petersen, R. A. Pinto, P. F. Landrum and J. W. J. Weber, Influence of carbon nanotubes on pyrene bioaccumulation from contaminated soils by earthworms, Environ. Sci. Technol., 2009, 43, 4181–4187 CrossRef CAS PubMed.
- R. De La Torre-Roche, J. Hawthorne, Y. Deng, B. Xing, W. Cai, L. A. Newman, Q. Wang, X. Ma, H. Hamdi and J. C. White, Multiwalled carbon nanotubes and C60 fullerenes differentially impact the accumulation of weathered pesticides in four agricultural plants, Environ. Sci. Technol., 2013, 47, 12539–12547 CrossRef CAS.
- A. Mukherjee, S. Majumdar, A. D. Servin, L. Pagano, O. P. Dhankher and J. C. White, Carbon nanomaterials in agriculture: A critical review, Front. Plant Sci., 2016, 7, 172 Search PubMed.
- S. Samadi, B. Asgari Lajayer, E. Moghiseh and S. Rodríguez-Couto, Effect of carbon nanomaterials on cell toxicity, biomass production, nutritional and active compound accumulation in plants, Environ. Technol., 2021, 21, 101323 CAS.
- L. Chen, C. Wang, S. Yang, X. Guan, Q. Zhang, M. Shi, S.-T. Yang, C. Chen and X.-L. Chang, Chemical reduction of graphene enhances in vivo translocation and photosynthetic inhibition in pea plants, Environ. Sci.: Nano, 2019, 6, 1077–1088 RSC.
- K. R. Guo, M. Adeel, F. Hu, Z. Z. Xiao, K. X. Wang, Y. Hao, Y. K. Rui and X. L. Chang, Absorption of carbon-13 labelled fullerene (C60) on rice seedlings and effect of phytohormones on growth, J. Nanosci. Nanotechnol., 2021, 21, 3197–3202 CrossRef CAS.
- X. Tao, Y. Yu, J. D. Fortner, Y. He, Y. Chen and J. B. Hughes, Effects of aqueous stable fullerene nanocrystal (nC60) on Scenedesmus obliquus: Evaluation of the sub-lethal photosynthetic responses and inhibition mechanism, Chemosphere, 2015, 122, 162–167 CrossRef CAS.
- S. M. A. Santos, A. M. Dinis, D. M. F. Rodrigues, F. Peixoto, R. A. Videira and A. S. Jurado, Studies on the toxicity of an aqueous suspension of C60 nanoparticles using a bacterium (gen. Bacillus) and an aquatic plant (Lemna gibba) as in vitro model systems, Aquat. Toxicol., 2013, 142–143, 347–354 CrossRef CAS PubMed.
- S. Dang, Q. Liu, X. Zhang, K. He, C. Wang and X. Fang, Comparative cytotoxicity study of water-soluble carbon nanoparticles on plant cells, J. Nanosci. Nanotechnol., 2012, 12, 4478–4484 CrossRef CAS PubMed.
- P. Begum, R. Ikhtiari and B. Fugetsu, Potential impact of multi-walled carbon nanotubes exposure to the seedling stage of selected plant species, Nanomaterials, 2014, 4, 203–221 CrossRef PubMed.
- M. Khodakovskaya, E. Dervishi, M. Mahmood, Y. Xu, Z. Li, F. Watanabe and A. S. Biris, Carbon nanotubes are able to penetrate plant seed coat and dramatically affect seed germination and plant growth, ACS Nano, 2009, 3, 3221–3227 CrossRef CAS.
- C. Kole, P. Kole, K. M. Randunu, P. Choudhary, R. Podila, P. C. Ke, A. M. Rao and R. K. Marcus, Nanobiotechnology can boost crop production and quality: First evidence from increased plant biomass, fruit yield and phytomedicine content in bitter melon (Momordica charantia), BMC Biotechnol., 2013, 13, 37 CrossRef.
- J. E. Cañas, M. Long, S. Nations, R. Vadan, L. Dai, M. Luo, R. Ambikapathi, E. H. Lee and D. Olszyk, Effects of functionalized and nonfunctionalized single-walled carbon nanotubes on root elongation of select crop species, Environ. Toxicol. Chem., 2008, 27, 1922–1931 CrossRef.
- O. V. Yamskova, D. V. Kurilov, I. V. Zavarzin, M. S. Krasnov and T. V. Voronkova, Effects of the impact of water-soluble forms of fullerenes and their derivatives on metabolism of plants and yield of agricultural crops, Biol. Bull. Rev., 2023, 13, 357–370 CrossRef.
- C. Wang, H. Zhang, L. Ruan, L. Chen, H. Li, X.-L. Chang, X. Zhang and S.-T. Yang, Bioaccumulation of 13C-fullerenol nanomaterials in wheat, Environ. Sci.: Nano, 2016, 3, 799–805 RSC.
- R. Avanasi, W. A. Jackson, B. Sherwin, J. F. Mudge and T. A. Anderson, C60 fullerene soil sorption, biodegradation, and plant uptake, Environ. Sci. Technol., 2014, 48, 2792–2797 CrossRef CAS PubMed.
- J. W. Kelsey and J. C. White, Effect Of C60 fullerenes on the accumulation of weathered p,p′-DDE by plant and earthworm species under single and multispecies conditions, Environ. Toxicol. Chem., 2013, 32, 1117–1123 CrossRef CAS PubMed.
- S. Kumar, A. Patra, S. Datta, K. G. Rosin and T. Purakayastha, Phytotoxicity of nanoparticles to seed germination of plants, Int. J. Adv. Res., 2015, 3, 854–865 Search PubMed.
- X. Ma and C. Wang, Fullerene Nanoparticles Affect the fate and uptake of trichloroethylene in phytoremediation systems, Environ. Eng. Sci., 2010, 27, 989–992 CrossRef CAS.
- F. S. Chapin, P. M. Vitousek and K. Van Cleve, The nature of nutrient limitation in plant communities, Am. Nat., 1986, 127, 48–58 CrossRef.
- H. A. Mooney, P. M. Vitousek and P. A. Matson, Exchange of materials between terrestrial ecosystems and the atmosphere, Science, 1987, 238, 926–932 CrossRef CAS.
- P. M. Vitousek and R. W. Howarth, Nitrogen limitation on land and in the sea: How can it occur?, Biogeochemistry, 1991, 13, 87–115 CrossRef.
- R. F. Follett and J. L. Hatfield, Nitrogen in the environment: Sources, problems, and management, Sci. World J., 2001, 1, 640372 Search PubMed.
- T. Näsholm, K. Kielland and U. Ganeteg, Uptake of organic nitrogen by plants, New Phytol., 2009, 182, 31–48 CrossRef.
- A. I. Gärdenäs, G. I. Ågren, J. A. Bird, M. Clarholm, S. Hallin, P. Ineson, T. Kätterer, H. Knicker, S. I. Nilsson, T. Näsholm, S. Ogle, K. Paustian, T. Persson and J. Stendahl, Knowledge gaps in soil carbon and nitrogen interactions–From molecular to global scale, Soil Biol. Biochem., 2011, 43, 702–717 CrossRef.
- J. Kaushal, M. Khatri and S. K. Arya, A treatise on Organophosphate pesticide pollution: Current strategies and advancements in their environmental degradation and elimination, Ecotoxicol. Environ. Saf., 2021, 207, 111483 CrossRef CAS PubMed.
- S. Babu, S. Singh Rathore, R. Singh, S. Kumar, V. K. Singh, S. K. Yadav, V. Yadav, R. Raj, D. Yadav, K. Shekhawat and O. Ali Wani, Exploring agricultural waste biomass for energy, food and feed production and pollution mitigation: A review, Bioresour. Technol., 2022, 360, 127566 CrossRef CAS.
- X.-T. Ju, G.-X. Xing, X.-P. Chen, S.-L. Zhang, L.-J. Zhang, X.-J. Liu, Z.-L. Cui, B. Yin, P. Christie, Z.-L. Zhu and F.-S. Zhang, Reducing environmental risk by improving N management in intensive Chinese agricultural systems, Proc. Natl. Acad. Sci. U. S. A., 2009, 106, 3041–3046 CrossRef CAS PubMed.
- C. O. Dimkpa, J. Fugice, U. Singh and T. D. Lewis, Development of fertilizers for enhanced nitrogen use efficiency–Trends and perspectives, Sci. Total Environ., 2020, 731, 139113 CrossRef CAS PubMed.
- Y. Hao, Y. Yu, G. Sun, X. Gong, Y. Jiang, G. Lv, Y. Zhang, L. Li, Y. Zhao, D. Sun, W. Gu and C. Qian, Effects of multi-walled carbon nanotubes and nano-silica on root development, leaf photosynthesis, active oxygen and nitrogen metabolism in maize, Plants, 2023, 12, 1604 CrossRef CAS.
- F. Zhao, X. Xin, Y. Cao, D. Su, P. Ji, Z. Zhu and Z. He, Use of carbon nanoparticles to improve soil fertility, crop growth and nutrient uptake by corn (Zea mays L.), Nanomaterials, 2021, 11, 2717 CrossRef CAS PubMed.
- A. A. H. Abdel Latef, A. K. Srivastava, M. S. A. El-sadek, M. Kordrostami and L.-S. P. Tran, Titanium dioxide nanoparticles improve growth and enhance tolerance of broad bean plants under saline soil conditions, Land Degrad. Dev., 2018, 29, 1065–1073 CrossRef.
- R. Suriyaprabha, G. Karunakaran, R. Yuvakkumar, V. Rajendran and N. Kannan, Foliar application of silica nanoparticles on the phytochemical responses of maize (Zea mays L.) and its toxicological behavior, Synth. React. Inorg., Met.-Org., Nano-Met. Chem., 2014, 44, 1128–1131 CrossRef CAS.
- G. G. Panova, A. S. Zhuravleva, Y. V. Khomyakov, V. E. Vertebnyi, S. V. Ageev, A. V. Petrov, N. E. Podolsky, E. I. Morozova, V. V. Sharoyko and K. N. Semenov, Plant impact properties of carboxylated fullerene C60[C(COOH)2]3, J. Mol. Struct., 2021, 1235, 130163 CrossRef CAS.
- G. G. Panova, K. N. Semenov, A. S. Zhuravleva, Y. V. Khomyakov, E. N. Volkova, G. V. Mirskaya, A. M. Artemyeva, N. R. Iamalova, V. I. Dubovitskaya and O. R. Udalova, Obtaining vegetable production enriched with minor micronutrients using fullerene derivatives, Horticulturae, 2023, 9, 828 CrossRef.
- G. G. Panova, K. N. Semenov, A. M. Artemieva, E. A. Rogozhin, A. S. Barashkova, D. L. Kornyukhin, Y. V. Khomyakov, E. V. Balashov, A. S. Galushko, V. E. Vertebnyi, A. S. Zhuravleva, E. N. Volkova, A. M. Shpanev, O. R. Udalova and E. V. Kanash, Influence of nanocompositions based on light fullerene derivatives on cultural plants under favorable and stress conditions of their habitat, Tech. Phys., 2022, 92, 871 CrossRef.
- V. Demir, M. Ates, Z. Arslan, M. Camas, F. Celik, C. Bogatu and Ş. S. Can, Influence of alpha and gamma-iron oxide nanoparticles on marine microalgae species, Bull. Environ. Contam. Toxicol., 2015, 95, 752–757 CrossRef CAS.
- X.-L. Chang, L. Chen, B. Liu, S.-T. Yang, H. Wang, A. Cao and C. Chen, Stable isotope labeling of nanomaterials for biosafety evaluation and drug development, Chin. Chem. Lett., 2022, 33, 3303–3314 CrossRef CAS.
- L. Chen, C. Wang, H. Li, X. Qu, S.-T. Yang and X.-L. Chang, Bioaccumulation and toxicity of 13C-skeleton labeled graphene oxide in wheat, Environ. Sci. Technol., 2017, 51, 10146–10153 CrossRef CAS.
- C. Wang, X.-L. Chang, Q. Shi and X. Zhang, Uptake and transfer of 13C-fullerenols from Scenedesmus obliquus to Daphnia magna in an aquatic environment, Environ. Sci. Technol., 2018, 52, 12133–12141 CrossRef CAS PubMed.
- M. Du, H. Zhang, J. Li, C. Yan, X. Zhang and X. Chang, Bioaccumulation, Depuration, and transfer to offspring of 13C-labeled fullerenols by Daphnia magna, Environ. Sci. Technol., 2016, 50, 10421–10427 CrossRef CAS.
- C. Chen, Y.-F. Li, Y. Qu, Z. Chai and Y. Zhao, Advanced nuclear analytical and related techniques for the growing challenges in nanotoxicology, Chem. Soc. Rev., 2013, 42, 8266–8303 RSC.
- C. R. Warren and P. R. Adams, Uptake of nitrate, ammonium and glycine by plants of Tasmanian wet eucalypt forests, Tree Physiol., 2007, 27, 413–419 CrossRef CAS PubMed.
- D. L. Slomberg and M. H. Schoenfisch, Silica nanoparticle phytotoxicity to Arabidopsis thaliana, Environ. Sci. Technol., 2012, 46, 10247–10254 CrossRef CAS.
- C. Larue, J. Laurette, N. Herlin-Boime, H. Khodja, B. Fayard, A.-M. Flank, F. Brisset and M. Carriere, Accumulation, translocation and impact of TiO2 nanoparticles in wheat (Triticum aestivum spp.): Influence of diameter and crystal phase, Sci. Total Environ., 2012, 431, 197–208 CrossRef CAS.
- J. Lv, P. Christie and S. Zhang, Uptake, translocation, and transformation of metal-based nanoparticles in plants: Recent advances and methodological challenges, Environ. Sci.: Nano, 2019, 6, 41–59 RSC.
- G. Meijer and D. S. Bethune, Laser deposition of carbon clusters on surfaces: A new approach to the study of fullerenes, J. Chem. Phys., 1990, 93, 7800–7802 CrossRef CAS.
- C. C. Chen and C. M. Lieber, Synthesis of pure 13C60 and determination of the isotope effect for fullerene superconductors, J. Am. Chem. Soc., 1992, 114, 3141–3142 CrossRef CAS.
- T. W. Ebbesen, J. Tabuchi and K. Tanigaki, The mechanistics of fullerene formation, Chem. Phys. Lett., 1992, 191, 336–338 CrossRef CAS.
- C. Ozfidan-Konakci, F. N. Alp, B. Arikan, M. Balci, Z. Parmaksizoglu, E. Yildiztugay and H. Cavusoglu, The effects of fullerene on photosynthetic apparatus, chloroplast-encoded gene expression, and nitrogen assimilation in Zea mays under cobalt stress, Physiol. Plant., 2022, 174, e13720 CrossRef CAS PubMed.
- Q. Wang, L. Gao, Y. Li, N. Shakoor, Y. Sun, Y. Jiang, G. Zhu, F. Wang, Y. Shen, Y. Rui and P. Zhang, Nano-agriculture and nitrogen cycling: Opportunities and challenges for sustainable farming, J. Cleaner Prod., 2023, 421, 138489 CrossRef.
- T. Liu, L. Xiang, Z. X. Yu, C. H. Mo, Y. W. Li, H.-M. Zhao, Q. Y. Cai and H. Li, Responses of morphological and physiological characteristics in rice (Oryza sativa L.) seedling roots to its uptake of CuO nanopaticles, Zhongguo Huanjing Kexue, 2015, 35, 1480–1486 CAS.
|
This journal is © The Royal Society of Chemistry 2025 |
Click here to see how this site uses Cookies. View our privacy policy here.