DOI:
10.1039/D4EN00943F
(Critical Review)
Environ. Sci.: Nano, 2025,
12, 2128-2153
Nanotechnology-enabled soil management for sustainable agriculture: interactions, challenges, and prospects
Received
7th October 2024
, Accepted 27th January 2025
First published on 24th February 2025
Abstract
Nanotechnology has significant potential to improve the quality of life through its applications in numerous fields, including the agricultural sector. Recently, many applications of nanotechnology in agriculture have emerged, focusing on crop production and protection, with an emphasis on nano-fertilizers, nano-irrigation, and nano-enabled remediation strategies for contaminated soils. The functionalization of nanomaterials is a growing solution to solve many agricultural problems due to the unique physical and chemical properties of nanomaterials, such as their superior performance and benefits in different fields compared to traditional materials. Recently, numerous studies have reported the promising effects of nanotechnologies on the agricultural sector. This review aims to provide an overview of state-of-the-art sustainable nanotechnologies in soil management. The production routes and features of nanomaterials applicable in agricultural areas will be briefly described. The application of nanotechnology in soil management will be discussed in detail, including interaction mechanisms, nanomaterial classification, and various implementations related to soil remediation, fertility and nano-irrigation systems. The challenges associated with the application of nanotechnology in soil will also be presented. Finally, prospective trends will be suggested for maximizing nanotechnology performance and enhancing soil quality.
Environmental significance
Nanotechnology has recently had significant impacts across various sectors, including soil and agriculture. With increasing environmental and sustainability awareness, numerous studies have reported the role of nanotechnology in achieving food security and preserving the soil matrix. In this review, we highlight state-of-the-art sustainable nanotechnologies applicable to soil management. The mechanisms of nanomaterials' interactions with soil for nutrition and remediation are also discussed. The role of nano-irrigation, as an innovative irrigation system, is presented. Finally, the challenges and prospects of nanotechnology applications in soil management are summarized.
|
1. Introduction
The land area covers 29% of the Earth's surface. Of this, only 11% is arable land, which is insufficient to meet the growing demands of population and urbanization worldwide.1 This not only threatens food security and increases poverty rates but may also result in severe economic ramifications, hindering sustainable development goals.2 The quality of food and plant production relies on the quality of terrestrial ecosystems. However, issues associated with soil deterioration raise serious concerns, including loss of fertility, high salinity, loss of useful microorganisms, loss of moisture, desertification, lack of nutrients and minerals, pH changes, high toxicity, and land degradation.3–5 This situation substantially calls for the development and innovation of new strategies to protect and restore damaged areas and reclaim new lands.
Recently, nanotechnology has sparked tremendous applications in nearly every aspect of life, from fundamental sciences to multidisciplinary engineering.6,7 These formidable interests are evident in the increasing number of publications using the term “nanotechnology” on the Web of Science since 2000 (Fig. 1a). In recent years, there has been a surge in publications focusing on the application of nanotechnology in the fields of environment and agriculture, leveraging the unique properties of nanomaterials. Nanomaterials have seen significant growth in soil amendment and food production due to their unique physical and chemical properties, high efficacy, cost-effectiveness, and environmentally friendly features. The number of publications using the terms “nanoparticles” and “soil” increased dramatically in 2008 (117 publications) and reached its peak in the last three years, with over “1650” publications in 2022 (Fig. 1a). This rapid growth of nanoparticles in soil science is evident from the data extracted from the Web of Science, which shows over 3400 articles published in the past two decades (Fig. 1b). The co-occurrence of the extracted keywords was analyzed using VOSviewer software, reflecting the correlation between the terms “nanoparticles”, “soil”, and “agriculture” (Fig. 1b), where the larger nodes represent a higher frequency of occurrence, while the connected lines illustrate the relevance of the keywords.8 The most relevant nanoparticles applicable in soil and agriculture are silver nanoparticles, iron nanoparticles, gold nanoparticles, CeO2, nZVI, ZnO, TiO2, CuO, carbon nanotubes, and nano-biochar. Regarding application, the adsorption of heavy metals and degradation of organic pollutants are the most frequent keywords, followed by nanobioremediation and nanofertilizers.
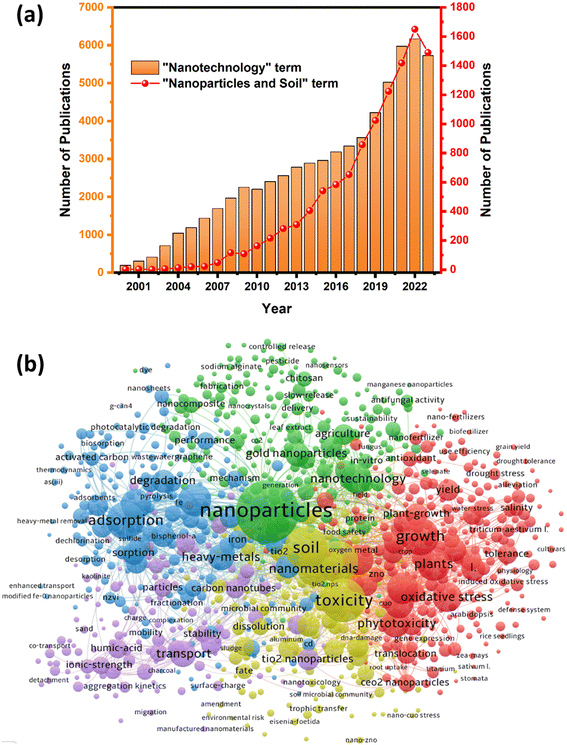 |
| Fig. 1 (a) Number of publications using “nanotechnology” as a topic keyword since 2000 (columns) and number of publications using “nanoparticles and soil” as topic keywords since 2000 (red plots). Adapted from Web of Science, February 2024. (b) Co-occurrence network extracted from the Web of Science database over the last 2 decades with keywords “nanoparticles”, “soil”, and “agriculture”, analyzed using VOSviewer software. | |
With this rapidly accelerating number of literature reports, many comprehensive reviews have been recently published regarding the application of nanotechnology in soil and agricultural systems. As proposed by Usman et al., nanomaterials affect the nutrient release in soil, soil biota, soil organic matter, and plant morphology and physiology, and the mechanisms involved in nanomaterial uptake and translocation within plants and their associated defence mechanisms were also explored.9 Saritha et al. summarized the potential of nanotechnology in agriculture, the environment and the food industry including seed nano-priming, smart delivery systems, and nano-food packaging.10 The limitations of nanotechnology were discussed focusing on the harmful impacts of nanotechnology on the soil microbiota, plant growth, underground water, and human health. Hofmann et al. explored the barriers in nanotechnology-enabled plant agriculture systems including the limitation of information about delivery methods on the field scale, regulation and safety concerns and consumer acceptance of foods produced using nano-enabled technologies.11 The authors further proposed pathways to overcome these barriers including nanotechnology-based genetic engineering, fertilizers, seed coatings, plant protection, and enhanced soil microbiome. Many other reviews have described nanotechnologies as potential solutions for soil remediation,12–15 crop protection and growth enhancement,16 biotic and abiotic stress tolerance,17,18 plant quality monitoring,19 and safe food processing and packaging.20,21
This review article identifies state-of-the-art of sustainable nanotechnology-based soil applications. The first section briefly describes the synthesis and properties of nanomaterials applied in soil amendment. Next, we shed light on the cutting-edge and advanced sustainable nanotechnology implemented for soil preservation and reclamation. In this part, the main remediation processes supported by interaction mechanisms with soil content include nano-based adsorption, catalysis, and bioremediation. Nano-based adsorbents are divided into four dominant classifications based on the most nanomaterials reported for soil remediation including iron, carbon, natural minerals and polymers, and metal(loid)s-derived nano-adsorbents. In addition, the advances in catalysis for the degradation of pollutants in the soil are also discussed involving advanced oxidation processes, heterogeneous catalysis, and photocatalysis. The potential of nano-induced microorganisms and phytoremediation is also highlighted as sustainable nano-bioremediation processes. We also underline the advanced application of nano-fertilizers as green alternatives to traditional commercialized fertilizers. The emerging nanotechnology named nano-irrigation is also highlighted as a new irrigation strategy. Regarding this part, we discuss two key nano-irrigation systems, nano-pores tubes and micro/nano bubble aerated irrigation. Finally, the barriers obstructing the application of nanotechnology and the future outlook are both highlighted.
2. Synthesis of nanomaterials
The two primary strategies for synthesizing nanoparticles are bottom-up and top-down processes.22–24 The top-down method starts with the standard technique for nanoparticle production, and then reduces the particle size using erosion and various approaches such as mechanical alloying, reactive milling, and high-energy ball milling.25,26 The bottom-up approach is the most recent method, which is based on the atom-by-atom or molecule-by-molecule construction of a substance, such as molecular self-assembly, sol–gel, and chemical/physical vapor deposition.27,28 Each method has certain benefits and drawbacks. Crystallographic and surface structure damage during particle size reduction is the fundamental issue with the top-down method. Regardless of the flaws generated by the top-down process, it will continue to play an important role in the synthesis of nanoparticles. The two main issues with the bottom-up method are the need for chemical purification and the difficulty in large-scale production. Regardless, the bottom-up approach is the preferred technology given that it can be employed to manufacture materials with precise qualities customized to the remediation need based on the fabrication pathway chosen.29Fig. 2 shows some instances of several applicable nanomaterials manufacturing methodologies including physical, biological, and chemical methods.19,30–33
 |
| Fig. 2 Applicable nanomaterials and their manufacturing methods (physical, chemical, and biological). | |
Furthermore, chemical and physical methods for the synthesis of nanomaterials frequently produce nanomaterials with a defined shape and size, but these methods have been proven to be hazardous to the environment due to the use of poisonous chemical agents and high temperatures during the synthesis processes.34 Given that the biological synthesis of nanomaterials is performed via biological entities such as bacteria,35 fungi,36 algae,37 and plant extracts, biological methods for the synthesis of nanomaterials with a defined shape and size require further investigation.38 Biological entities have been proven to be high secretors of proteins and enzymes, which are primarily responsible for reducing metal ions and controlling the produced nanomaterial. As illustrated in the microbial synthesis of nanomaterials, it can be performed via extracellular and intracellular mechanisms. The biogenic synthesis of nanomaterials is green and environmentally friendly technology because it does not require toxic chemicals. Furthermore, the synthesis procedure is often carried out under standard pressure and temperature conditions. As a result, rather than employing chemical or physical approaches, many researchers have directed their efforts to the creation of biological nanomaterials.34
3. Properties of nanomaterials
Nanomaterials have abundant desirable features, and thus they are recognized as highly efficient materials and ideal candidates for implementation in the agriculture sector. These features include enormous surface area, abundant sorption sites, low-temperature modification, short intraparticle diffusion distance, variable pore size, and surface chemistry.39 The characteristics and efficacy of nanomaterials are also influenced by their form and morphology. The delicate balance among the energy from polar charges, surface area, and elastic deformation results in a variety of morphologies. According to Gatoo et al., the shape, surface area and chemical composition of nanomaterials influence their behavior and activity.40
Also, the innate surface properties, intrinsic compositions, apparent sizes, and external functionalization of nanomaterials have a significant impact on their physical, material, and chemical properties.41 The nature and distribution of active sites on the surface and the types and number of functional groups in nanomaterials contribute to explaining their behavior and properties, such as the location of the domain atoms on their surface, high surface area, high chemical activity, high adsorptive capacity, and high surface binding energy. These distinguishable chemical and physical characteristics have led to an increase in their application compared to traditional commercial compounds.42
4. Application of nanotechnology in soil management
4.1. Soil remediation
The rapid pace of industrialization and urbanization growth has resulted in severe environmental issues and poses a great threat to terrestrial and aquatic resources, as well as human health. Soil contamination is a global issue that is of concern and a menace to food security. The remediation of a wide variety of soil pollutants is a challenging task, requiring highly efficient removal techniques. The major pollutants in soil can be divided into organic pollutants such as total petroleum hydrocarbons (TPHs), pesticides, herbicides, microplastic contaminants, and emerging micropollutants, and inorganic pollutants such as heavy metals (Cu, Cd, Zn, Pb, and Hg), metalloids (Sb, and As), and radioactive elements, resulting from different resources including industrial, domestic, agricultural and mining activities.1,43–47 The deleterious effects of these pollutants can be evaluated by their mobility and bioavailability in contaminated soil. Bioavailability is determined by the total amount of chemicals that can be transferred to living organisms, posing a great threat to food security.45 Thus, reducing the mobility and bioavailability of contaminants is crucial in the sustainable remediation of contaminated soil. Nanotechnology offers efficient emerging techniques that can work to immobilize and convert pollutant molecules to harmless by-products in the soil matrix.9,48 In this section, we discuss the efficiency of recently advanced nanomaterials in the remediation of contaminated soil via adsorption, catalysis, and bioremediation.
4.1.1. Nano-adsorbents.
The adsorption or immobilization of pollutants by nanoparticles has attracted increasing attention in recent years as an efficient strategy to reduce the bioavailability of pollutant molecules and/or ions. The investigation of the interaction kinetics between nano-adsorbents and pollutant compounds is crucial for estimating their adsorption mechanism and feasibility. The domain mechanisms for the adsorption process can be summarized as follows (Fig. 3):
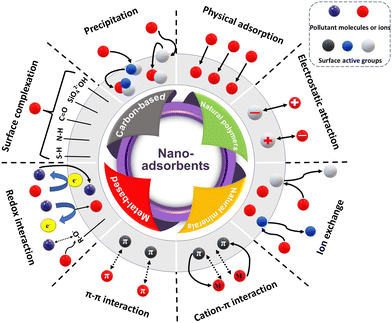 |
| Fig. 3 Possible adsorption mechanisms of contaminants in soil by nano-adsorbents. | |
1 – Physical adsorption and pore-filling: this adsorption mechanism does not rely on chemical interactions. The adsorption process depends on the diffusion and desorption of the guest molecules/ions in the pores of nano-adsorbents until adsorption equilibrium is reached.49 The main factor influencing physical adsorption is the conditions for the synthesis of nanoparticles. For instance, pre-treatment activation, high-temperature pyrolysis, and ball-milling utilization towards the synthesis of carbon-based nanomaterials such as nano-biochar promote the formation of mesopores and micropores and enhance the pore diameter, volume, and specific surface area.50 Thus, the diffusion and filling of metal ions or organic molecules in the pores of the nano-adsorbent will be facilitated and the physical adsorption kinetics will be accelerated.
2 – Electrostatic interaction: the interaction in this process takes place between the opposite ions, between the cation ions of the pollutant and the anionic surface of the nano-adsorbent and vice versa. This process is mostly facilitated on the surface enriched with oxygenated functional groups such as hydroxyl (–OH), carboxylic (–COOH), and other functional groups; besides, it can be influenced by the solution pH and ionic strength.51 The heavy metals and organic pollutants present in soil solutions, such as pesticides and other agrochemicals, remain in the ionic state, resulting in bonding with groups with different charges.
3 – Ion exchange: ion exchange, indicated by cation exchange capacity (CEC), occurs between cations such as K+, Na+, Ca2+, and Mg2+ or the protons of oxygen-contained functional groups (–OH, –COOH) on the surface of nano-adsorbents and divalent or trivalent metal cations (M2+ or M3+).52 A lower soil pH is crucial for boosting the desorption and diffusion of metal cations in the soil solution, and thus increases the possibility of the ion exchange process.53
4 – Surface complexation: surface complexation occurs between the enriched functional groups on the surface of nano-adsorbents, such as –O–, –OH, –C
O, –COOH, –P
O, –C–NH–, –SH, SiO42−, and phenols, and heavy metals ions to form more stable multiatom structures. The mechanism of surface complexation can be mainly deduced from some characterization techniques such as X-ray diffraction (XRD), Fourier transform infrared spectroscopy (FTIR), and X-ray photoelectron spectroscopy (XPS) analyses of contaminated soil before and after treatment.54
5 – Precipitation/coprecipitation: when heavy metal ions come to contact with the minerals on the surface of nano-adsorbents, such as K+, Mg2+, Ca2+, SO42−, CO32−, and PO43−, their mobility is reduced and they dissociate from the soil solution to form solid precipitates. For instance, Pb and Cd can form precipitates with mineral anions, i.e. PbCO3, Pb5(PO4)3OH, and Cd3(PO4)2. XRD and XPS analyses can confirm the precipitation mechanism.55
6 – Cation–pi (C–π) and pi–pi (π–π) interactions: these interactions are considered new binding forces compared to the classical electrostatic interactions. C–π interaction is the intermolecular interaction between metal cations and the aromatic systems in nano-adsorbents.55 Alternatively, π–π interactions occur between the aromatic moieties of organic pollutants (e.g. PAHs, pesticides, and antibiotics) and aromatic natures of nano-adsorbents such as graphitic structure of carbon-based nanomaterials, where π-electron interactions play the leading role. During the polarization of aromatic compounds, the residues of π-electrons in the π-bonds of the aromatic moiety groups are shared to form an aromatic π-cloud. Besides, the unique geometry positions of the aromatic moieties (face-to-face or edge-to-face), owing to their flat feature, facilitate π–π stacking.49 The strength of both C–π and π–π interactions on the surface aromaticity of nano-adsorbents varies, where more π-conjugated aromatic structures have stronger electron-donating potential. The π stacking mechanism can be investigated using XPS spectroscopy.50,55
Iron-based nanoparticles especially nano zero-valent iron (nZVI) have been widely applied to immobilize pollutants in contaminated soil.51,56 For instance, Baragaño et al. assessed the efficiency of nZVI and goethite nanospheres (nGoethite) for the immobilization of arsenic (As) in polluted soil (sandy loam).57 The results showed that nZVI and nGoethite nanoparticles could reduce the As concentration based on the toxicity characteristic leaching procedure test (TCLP) by 89.5% and 82.5%, respectively. In another study, Zialame et al. studied the influence of manganese ferrite (MnFe2O4) nanoparticles on the stabilization of As in contaminated sandy soil.58 Their study demonstrated that magnetite nanoparticles have significant potential in immobilizing As in contaminated soil by reducing the amount of arsenic leaching from the solid phase of the soil in the TCLP test by 90.2%. Furthermore, the presence of MnFe2O4 nanoparticles in contaminated soil could oxidize and convert some of the arsenic to the less toxic arsenate, which facilitated and enhanced its adsorption on the surface of Fe–Mn oxides in the structure of manganese ferrite. Sulfidation of nZVI (S-nZVI) is another approach that provides better conductivity, higher electron transfer efficiency, selectivity, and less toxicity compared to bare nZVI.59 Both nZVI and S-nZVI with a concentration of 5% w/w (nanoparticles/soil) showed efficient immobilization of As in a sandy loam soil matrix by decreasing the arsenic concentration leaching by 100% and 99.6%, respectively, after 7 days.60 However, S-nZVI exhibited considerable suppression of As bioaccumulation by earthworms (Eisenia fetida) more than nZVI, where the survival of the earthworms increased from 10% for nZVI to 73% for S-nZVI when the soil was treated with 0.3% adsorbent. This was ascribed to the strong stability of S-nZVI, which led to less change in pH and slower transformation, resulting in a lower impact on E. fetida. In another study, S-nZVI exhibited an excellent performance towards the immobilization of Cd in contaminated soil. After 30 days, the exchangeable Cd was diminished to less than 1% with an immobilization efficiency of 97.6–100% compared with the untreated soil. The proposed remediation mechanism relied on the adsorption of Cd particles onto the S-nZVI nanoparticles, followed by the formation of iron-manganese oxide bonds and the conversion of Cd to CdO and CdS, indicating that S-nZVI is a promising material for the remediation of Cd-contaminated soil.59 The use of natural minerals and clays as a support for nZVI is also another approach to promote its immobilization. Li et al. investigated nZVI supported by zeolite (Z-nZVI) to immobilize Cd, Pb, and As in soil for 180 days.61 The results showed that Z-nZVI amendment with a loading of 30 g kg−1 was the most effective in reducing the bioavailability of Cd, Pb, and As in acidic soil by 10.2%, 73.8%, and 17.1% and in alkaline soil by 44.3%, 96.8%, and 31.0%, respectively. The divergent immobilization efficiency of metalloids on the surface of Z-nZVI could be explained by the unique encapsulation mechanism, where the pollutants adsorbed rapidly on the surface of Z-nZVI by iron oxyhydroxides and aluminosilicates, and then a specific redox reaction of As(V), As(III) and Pb(II) occurred, which depended on soil pH and standard electrode potential (SEP). In this concurrent redox reaction, Fe0 can reduce As(V) to As(III), and then to As0 and Pb(II) is reduced to Pb0. Finally, the secondary mineralization of Z-nZVI provides a catalytic interface to form more stable B-type ternary complexes and heterogeneous coprecipitates. Alternatively, montmorillonite-supported nano-zero-valent iron (nZVI@TP-Mont) is an efficient amendment to remove chromium Cr(VI) from acidic and neutral soils. nZVI@TP-Mont showed almost complete Cr(VI) removal in acidic soil and about 84% removal in neutral soil.62 Its excellent Cr(VI) removal performance in acidic soil is attributed to the high H+ concentration in soil, which facilitates the dissolution of iron oxide on the surface of nZVI@TP-Mont, releasing more Fe2+ and providing more active sites. The enriched active sides enhance the reduction of Cr(VI) into Cr(III) through electron loss, and then foster Cr(III) immobilization by the nZVI@TP-Mont composites in soil. Alternatively, at high pH, OH− competes with the active sites, which hinders the reduction of Cr(VI).
Recently, nano-biochar and biochar-supported nanoparticles have been investigated as novel and promising adsorbents and amendments for the highly efficient removal of organic and inorganic pollutants in soil.63–68 Among the various biochar-supported nanocomposites, nZVI-doped biochar has been widely used for the immobilization of contaminants in soil.51 Biochar-supported nZVI has numerous advantages including improving the distribution of nZVI on the porous surface of biochar as discrete nanoparticles and enabling the manipulation of its particle size. This can hinder the steric and magnetic attraction between the nZVI particles and increase the surface area, thus enhancing the adsorption of pollutants.69–71 Thus far, Qian et al. studied the impact of biochar-supported nZVI (BC-nZVI) on the simultaneous stabilization of heavy metals (Cd and Pb) in clay soil.72 This study concluded that BC-nZVI exhibited better immobilization of Cd and Pb (high, reaching 86.49% and 80.14%, respectively) than pristine biochar and nZVI and could significantly reduce the bioavailability of these metals in contaminated soil. Furthermore, at a low concentration of 2,4-dichlorophenol (2,4-DCP) (2.65 mg kg−1), BC-nZVI could effectively remove 2,4-DCP in soil by nearly 90% via adsorption and reduction reaction with Cd or Pb immobilization. These recent results indicate the applicability of nanoparticle-modified biochar and some recent nanocomposites include biochar-supported nZVI,73 graphene oxide (GO),74 magnetic iron oxide nanoparticles,75 nano-hydroxyapatite,76 Fe–Mn nanoparticle-modified biochar,77 biochar-supported iron phosphate nanoparticles,78 and biochar-Ni/Fe bimetallic nanoparticles79 for the immobilization and removal of pollutants from contaminated soil. Other carbon-based nanomaterials such as carbon nanotubes (CNTs), fullerenes (nC60), graphene oxide, and carbon black nanoparticles have been also applied for the removal of contaminants in polluted soil environments.80,81 Due to the unique properties of carbon nanotubes such as high surface area, abundant adsorption sites along their cylinder structure, and tunable surface chemistry, CNTs have been applied in water and soil remediation.82,83 Li et al. explored the leaching behaviors of polyaromatic hydrocarbons (PAHs) in sandy loam soil in the presence of CNTs.84 The results showed that CNTs had significant potential to retain PAHs and reduce their bioavailability in the soil due to strong the PAH adsorption capacities of CNTs and their low mobilities. In addition to organic pollutants, CNTs showed the ability to immobilize inorganic pollutants such as phosphorus with a maximum adsorption capacity of P on sediments increasing from 0.646 to 0.996 mg g−1 with an increase in the CNT content in contaminated sediment.85
Natural minerals and polymers also play a considerable role in the adsorption of contaminants.86–89 For instance, halloysite nanotubes coated with carboxylic groups (HNTs@CRC) showed high efficiency in stabilizing four heavy metals, Co, Ni, Cu, and Zn, in river sediments. The stabilization of HNTs@CRC was further enhanced with the addition of Ca(OH)2 and reached high ratios of 98.82% for Co, 83.58% for Ni, 90.71% for Cu and 73.99% for Zn.90 These results can be attributed to the ability of carboxylic groups to adsorb heavy metals. Furthermore, the addition of Ca(OH)2 increased the pH, leading to stronger electrostatic affinity toward positively charged metal ions. In contaminated sandy loam soil amended with sewage sludge, Feizi and Jalali recommended the use of zeolite, ZnO and MgO nanoparticles as efficient heavy metal adsorbents, which reduced the leaching of Cd, Cu, Ni, and Zn from the contaminated soil.91 In addition, hydroxyapatite nanoparticles (nHAP) also exhibited high immobilization efficiency for reducing Pb uptake by plants in Pb-contaminated soils.92 A field assessment was also performed to investigate the immobilization of Pb in soil. Li et al. monitored long-term (1 year) Pb immobilization over a new type of carboxymethyl cellulose (CMC)-bridged chlorapatite nano/microparticles (CMC-CAP) at an abandoned chemical plant site.93 The pilot-scale results showed that CMC-CAP at a dosage of 0.5 wt% resulted in the highest immobilization of Pb and decreased the acid-leachable Pb in the sandy loam soil up to 99.4% after only 1 day of reaction and fixed at 99.6% after 365 days. Furthermore, the extraction data confirmed that the CMC-CAP amendment converted the more leachable Pb fractions into the less available Pb species. The use of natural polymers was also investigated as a green immobilization strategy for pollutants in the soil matrix. Tao et al. studied the role of three natural polymers, lignin, carboxymethyl cellulose, and sodium alginate, for soil amendment to stabilize Pb and Cd.94 The TCLP test showed that the leaching concentrations of Pb and Cd were reduced from 5.46% and 4.25% to 71.1% and 49.6%, respectively. The abundant oxygen groups on the surface of the natural polymers endowed them with the ability to chelate heavy metals. Compared to carboxymethyl cellulose and sodium alginate, lignin exhibited a higher immobilization performance due to its phenolic nature as a three-dimensional amorphous aromatic polymer. Embedding nanomaterials with these natural polymers paves a way toward expanding their adsorption potential with manifold interaction mechanisms. Novel metal–organic frameworks incorporated with TEMPO-oxidized nano-fibrillated cellulose/lignin/acrylamide-based hydrogel adsorbents revealed excellent adsorption capacity for Pb and Cu in contaminated soil with a decline in Pb and Cu bioavailability up to 85.9% and 74.5% within 45 days, respectively.95 The adsorption mechanisms could be ascribed to various interactions including physical sorption by swelling, ion exchange, electrical attraction, and π-acceptor (amide-cations) interactions, and complexation through unsaturated Fe(III) sites and hydroxyl/carboxyl/carbonyl groups.
Silicon (Si), the second most abundant element in the Earth's crust, was reported to be beneficial for alleviating heavy metal bioavailability and improving plant growth.96 Si nanoparticle amendment could enhance the immobilization of heavy metals and reduce their bioavailability in the soil, their translocation from the roots to aboveground parts, and their accumulation in plant tissues.96 Alternatively, the application of SiO2 nanoparticle-supported-lipid-bilayers was shown to be an effective method for the remediation of hydrophobic organic contaminants in soils using benzo-a-pyrene (BaP) as an example, where the lipids provided both an extraction method and stability for transport to the contaminant site.97 Thiol-functionalized nano-silica (SiO2-SH) was also adopted to remediate contaminated silt loam soil and decrease the biotoxicity of Pb, Cd, and Cu.98 Although mercapto is an effective functional group for immobilizing heavy metals when grafted to various materials, it is easily oxidized, limiting its practical application to a great extent. Thus, to improve the stability of amendments, Qiu et al. prepared mercapto iron complex-functionalized nanosilica (RNS-SF), which had high immobilization efficiencies in Pb/Cd-contaminated soil.99 The results showed that RNS-SF exhibited unique immobilization efficiencies in contaminated soil with immobilizing percentages of 98.7% for Pb and 97.9% for Cd, which remained unchanged from −20 °C to 50 °C, and even after 360 days storage at room temperature, the immobilization efficiencies of Pb and Cd were all about 90%.
Other nanoparticles, such as copper oxide (nCuO), can effectively reduce the mobility of As in soil and inhibit its accumulation in rice grains.100 Also, selenium nanoparticles (SeNPs) can efficiently remediate Hg0-contaminated soils to form more stable HgSe; however, the high aggregation of SeNPs hinders their applicability.101 Thus, to address this issue, it was found that extracellular polymeric substances (EPS) with SeNPs were an excellent dispersant for SeNPs in soil solution and could significantly enhance the remediation performance for Hg0 contamination under natural conditions. The reason for the stabilization effects was the strong interaction between EPS and SeNPs, making SeNPs more negatively charged.101 Another novel nanoparticle is a flower-like magnetic MoS2 nanohybrid (MoS2/Fe3O4). This nanohybrid material showed high adsorption capacity for Hg(II) (428.9 mg g−1) and Pb(II) (263.6 mg g−1) at the optimized operating parameters.102 For efficient radioactive pollutant removal from contaminated soil, Kim et al. proposed a novel two-step strategy for the remediation of Cs-contaminated soil assisted by magnetic polyethyleneimine-grafted Fe3O4 nanoparticles.103 The magnetic composites were added to dispersion-contaminated soil (a mass of Cs-sorbed soil was dispersed in DI water using an ultrasonic bath), and then selectively bonded with clay particles through electrostatic attraction, enabling the clay particles to be easily separated from sand under an external magnetic field. After treating the residue-contaminated sand with acid, the addition of a magnetic adsorbent (Fe3O4@SiO2@KTiFC, where KTiFC is potassium titanium(IV) hexacyanoferrate) to the acid treatment substantially improved the desorption of Cs+ (95.2%) by preventing the re-adsorption of Cs+ onto the sand. Practically, this approach demonstrated high efficiency in the remediation of Cs-contaminated soil with a high radioactivity reduction of 96.7%. The performances of the above-mentioned nano-adsorbents are summarized in Table 1. However, despite the great role of nano-adsorbents in immobilizing and removing toxic heavy metals from contaminated soil, the remediation of other soil pollutants such as recalcitrant and emerging organic contaminants requires more efficient removal techniques.
Table 1 Comparison of different nano-adsorbents for the removal of pollutants from soil
Adsorbent class |
Adsorbent |
Pollutant |
Adsorption efficiency/removal rate |
Interaction mechanism |
Ref. |
Iron-based nano-adsorbents |
nZVI + goethite (nGoethite) |
As |
89.5% reduction of TLCP test |
Arsenate adsorption occurs in the outer layer of the nanoparticles |
57
|
MnFe2O4 |
As |
90.2% of TLCP test |
Oxidation of As(III) to As(V) followed by adsorption of arsenate on the surface of binary Fe–Mn oxides |
58
|
S-nZVI |
Cd |
97.6–100% |
Precipitation of Cd(II) to form CdS by SH− and complexation with iron oxides |
59
|
S-nZVI |
As |
99.6% reduction after 7 days |
Co-precipitation of arsenic with iron and sulfur species; the sulfhydryl (–SH) group in S-nZVI increases the binding sites for inorganic species, including arsenic |
60
|
nZVI + zeolite (Z-nZVI) |
Cd, Pb, As |
44.3%, 96.8%, and 31.0%, in alkaline soil |
Ternary complexation by iron oxyhydroxides and aluminosilicates, along with concurrent redox reactions |
61
|
nZVI@TP-Mont |
Cr |
Complete removal in acidic soil and about 84% removal in neutral soil |
Cr(VI) reduction to Cr(III) and co-precipitation on the nZVI@TP-Mont surface as combined Cr(III)/Fe(III) hydroxides |
62
|
Carbon-based nano-adsorbents |
Biochar-supporting GO/nZVI |
Cu |
75.16% after 14 days at soil-neutral pH |
Cu complexation with the nZVI oxyhydroxide layer (Fe–OOH), electrostatic interaction and co-precipitation with biochar-GO/nZVI. |
74
|
Nano-biochar |
Cd |
∼30% after 90 days |
Surface complexation and electrostatic actions through core–shell structures of nano-biochar |
104
|
BC@MnO2 |
Pb, Cd, Zn, Ni |
96.98%, 95.29%, 95.08%, 94.35% reduction in bio-concentration factor (BCF) |
Formation of insoluble inorganic compounds via ion exchange, complexation, and precipitation in the presence of a greater number of exchangeable divalent cations such as Mg(II) in biochar |
105
|
CNTs |
PAHs |
28.8% retention in soil |
Hydrophobic interactions between PAHs and CNTs, pore-filling, and π–π interactions |
84
|
Natural mineral and polymers-based nano-adsorbents |
HNTs@CRC |
Co, Ni, Cu, Zn |
98.82%, 83.58%, 90.71%, 73.99% stabilization ratio |
Adsorption and precipitation on the surface of HNTs@CRC, along with complexation and interaction binding with Ca(OH)2 groups |
90
|
Zeolite |
Cu, Cd |
99.9%, 99.9% immobilization rate, and 0.005% and 0.05% leaching rate |
After replacing Na+ ions, immobilization of Cu and Cd via zeolitization, where Cu2+ strongly bonds to hydroxyl (forming Cu2+–OH) in the small cages, and Cd2+ coordinates with the oxygen (Cd2+–O) in large channels of zeolite |
106
|
Carboxymethyl cellulose bridged chlorapatite nano/microparticles (CMC-CAP) |
Pb |
99.6% stable immobilization rate from 1 day to 365 days |
Surface complexation/cation exchange between Ca2+ in chlorapatite and Pb2+, where metastable precipitates (Pb(OH)2 or PbCO3) transform into the most stable lead precipitate (pyromorphite) |
93
|
Metal(loid)-based nano-adsorbents |
SiO2-SH |
Cd, Pb, Cu |
92.02%, 68.03%, 76.34% immobilization rate |
Formation of metal complexes via thiol groups, transforming the active fraction of heavy metals into stable ones |
98
|
Mercapto iron/nanosilica complex |
Pb, Cd |
98.7%, 97.9% immobilization rate for 360 days |
Formation of insoluble and stable complexes with enriched –SFe and –OH functional groups |
90
|
ZnO nanoparticles |
Carbamazepine (CBZ) |
90% retention rate in the first leachate to ∼99% after the fifth leaching |
Adsorption of CBZ over ZnO by intermolecular forces, including hydrogen bonds, π–π, electron, electrostatic, hydrophobic interactions, and the pore-filling effect |
107
|
δ-MnO2 |
Cd |
65.8% reducible Cd |
Cd immobilization via adsorption and antagonistic effects of the released Mn ions with Cd in the wheat rhizosphere |
108
|
4.1.2. Nano-catalysts and -photocatalysts.
To overcome the drawbacks associated with the prolongation of the remaining residues and the difficulty of cleaning up pollutants, particularly organic substances, in the soil body during the adsorption process, the degradation and removal of contaminants in the soil to harmless by-products have been widely studied in the last few decades (Fig. 4). The catalytic degradation of organic pollutants in the soil by advanced oxidation processes (AOPs), a branch of chemical oxidation, has been proven to have the capability and efficiency to mineralize recalcitrant and highly toxic organic molecules such as PAHs to non-harmless substances such as CO2 and water. Using AOPs, oxidation occurs more quickly and more efficiently in soil, resulting in greater adaptability and performance. Typically, the effects of AOPs are primarily caused by free radical generation using oxidants such as hydrogen peroxide, persulfate, ozone (O3), permanganate, peracetic acid, and other Fenton reagents. Several factors play a role in AOPs, particularly temperature, pH, and concentration of oxidants and catalysts involved.
 |
| Fig. 4 Dominant green catalytic processes for soil remediation. | |
Besides being broadly used as adsorbents, iron-based nanoparticles show a superior performance in advanced oxidation catalysis for the cleanup of contaminated soil. Amongst them, the effectiveness of nZVI in Fenton and persulfate oxidation and removal of organic pollutants such as PAHs from soil has been proven in several previous studies.109–113 Bajagain and Jeong investigated the role of nZVI in activating peroxymonosulfate (PMS) for the degradation of TPHs in aged diesel-contaminated soil.114 The results showed that the nZVI/PMS system (optimal concentrations were 0.2% nZVI and 3% PMS) produced more reactive oxygen species (hydroxyl and sulfate free radicals) compared to other systems, such as Co(II)/PMS, providing the best degradation efficiency of TPHs (61.2% in 2 h), respectively. Alternatively, Yuan et al. reported the degradation of tetrabromobisphenol A (TBBPA) through the activation of persulfate (PS) by nZVI.115 The degradation rate of TBBPA was 78.32% within 12 h in a low pH soil medium at 25 °C, where the degradation behavior of TBBPA effectively occurred at lower pH and higher temperature. The oxidative degradation of TBBPA was ascribed to the generation of active species of SO4˙−, ·OH, and O2˙−, resulting in attack of the C–Br bond, and then debromination of TBBPA, and the cleavage between the isopropyl group and one of the benzene rings completed the decomposition. Recently, honeycomb iron nanoparticles supported on N-doped carbon foam microstructure (Fe@CF-N) were fabricated and used as an activator of potassium peroxymonosulfate (PMS) to degrade fluoranthene (FLT) in soil.116 The reactive oxygen species such as hydroxyl radicals (·OH), sulfate radical (SO4˙−) and singlet oxygen (1O2) were the major species responsible for the degradation of FLT into low molecular weight species of 1,2,3-benzenetriol and 1,3-propanediol, and finally degraded into water and carbon dioxide. The as-fabricated composite exhibited excellent degradation of FLT in soil with a degradation rate of 77.90% after 180 min. Besides, Fe@CF-N/PMS not only improved the physicochemical properties of the contaminated soil but also decreased the bioavailable toxicity of FLT. In the case of integrated processing with Fenton oxidation, Barzegar et al. studied the role of solo Fenton-like oxidation on the degradation of pyrene in simulated contaminated soil by Fe3O4 nanoparticles.112 The observed pyrene removal was about 98.37% and this value was verified with a variation in H2O2, nanoparticles dosage, pH, and ultrasonic power. Furthermore, the sono-Fenton oxidation was also applied for real PAH-contaminated soil. The removal rate ranged between 37.7% and 85.19% for different PAHs, which was less than that of the simulated soil, which could be attributed to the high pollution load with various aromatic and aliphatic hydrocarbons, requiring a longer reaction time for remediation. Alternatively, pyrene-contaminated soil was remediated by a microwave/magnetite-activated persulfate oxidation (Fe3O4/MW/PS) hybrid system.117 The presence of Fe3O4 nanoparticles remarkably enhanced the degradation of pyrene by 91.7% within 45 min treatment, which was three-times higher than that in the absence of Fe3O4. Moreover, the pyrene removal rate in the Fe3O4/MW/PS system was 5.18- and 3.00-times higher than that in the Fe3O4/PS and MW/PS systems, respectively. The pyrene degradation depended on the PS concentration, Fe3O4 dosage, MW temperature, and soil moisture content. According to the analysis, radical species such as SO4˙−, ·OH, O2˙−, and 1O2 were responsible for the degradation of pyrene. Bimetallic nanoparticles supporting biochar were also applied for the remediation of contaminated soil. Biochar-supported Ni/Fe bimetallic nanoparticles (BC@Ni/Fe) had an excellent efficiency for removal of decabromodiphenyl ether from contaminated soil with a removal efficiency of 87.7% after 72 h, which was 30.2% and 69% higher than that by neat Ni/Fe and biochar, respectively. Essentially, BC@Ni/Fe could reduce the in situ bioavailability of metals and adsorb the degradation byproducts after decabromodiphenyl ether degradation.118
Besides the high performance of catalysts for the in situ degradation of pollutants in soil, the use of photocatalysts provides a promising and sustainable strategy for the degradation of contaminants in polluted soil under sunlight. The mechanism of photocatalysis relies on the absorption of photons by electrons, and then the electrons are excited from the valence band to the conduction band, initiating a series of reactive radical species and redox reactions.119 Since the pioneering work on the photocatalysis process for hydrogen production from water splitting in 1972,120 the semiconductor titanium dioxide (TiO2) has been broadly used for environmental remediation by removal of organic contaminants in wastewater and air. Recent years have witnessed the increasing application of TiO2 nanoparticles in contaminated soil remediation. Wang et al. studied the role of TiO2 nanoparticles in the degradation of diphenyl arsenic acid (DPAA) in contaminated soil under UV irradiation.121 With 5% of TiO2 in soil and 3 h irradiation time, the removal efficiency of DPAA increased to 82.7%, and arsenate was identified as the final product, resulting from the generation of ·OH by TiO2. In an alkaline medium, the TiO2 and montmorillonite composite photocatalyst showed a higher degradation of γ-hexachlorocyclohexane (γ-HCH) in soil with a degradation efficiency of 76% within 2 h, and the photocatalytic performance was varied with the content of TiO2.122 The reason for the higher photocatalytic activity in alkaline soil was that more ·OH radicals could be generated at higher pH via the photooxidation of OH− by holes. Compared with the degradation of PAHs in water, Rachna et al. reported that the photocatalytic degradation rate of toxic three-membered PAHs, namely acenaphthene (ACN), phenanthrene (PHN), and fluorene (FLU), over a TiO2-based zinc hexacyanoferrate framework (TiO2@ZnHCF) in contaminated soil (82–86%) and river sediment (81.63–85.43%) was slower than that in contaminated water (93–96%).123 This was attributed to the reduced diffusion due to the interaction between PAH molecules and the organic content of soil and sediment. Shanker et al. studied the performance of potassium zinc hexacyanoferrate (KZnHCF) nanocubes as another photocatalyst in the degradation of different toxic PAHs (BaP, chrysene, fluorene, phenanthrene and anthracene) in soil under UV and sunlight.124 This study concluded that the KZnHCF nanocubes have high potential for the degradation of PAHs by 70–93%. The order of degradation of PAHs depended on their molecular size, weight, structure, and aromaticity. Furthermore, small and non-toxic byproducts were investigated such as malealdehyde, 4-oxobut-2-enoic acid and ando-xylene.
Another promising and green photocatalyst is graphitic carbon nitride (g-C3N4), which has been widely applied in environmental and energy applications during the last decade.125–127 The unique properties of g-C3N4, such as its activity under visible light, ease of preparation, high surface area, enriched active sites, non-toxicity, and low cost, make it tremendously attractive with enormous applications for water and air purification.128 However, there are limited reports that studied the role of g-C3N4 in the remediation of contaminated soil under visible light. Recently, Luo et al. studied the role of g-C3N4 synthesized using three different precursors including urea, melamine, and dicyandiamide in the photodegradation of phenanthrene in contaminated soil under visible light and natural sunlight in circumneutral pH.129 The results showed that g-C3N4 synthesized using urea had the highest surface area (67.10 m2 g−1) and degradation activity (81.25%) for phenanthrene in soil compared with g-C3N4 synthesized using dicyandiamide (40.46 m2 g−1 and 72.87%, respectively) and melamine (28.19 m2 g−1 and 75.39%, respectively) under visible light. Furthermore, g-C3N4 synthesized using three different precursors could remarkably reduce the toxicity of phenanthrene-contaminated soil, which was demonstrated by promoting lettuce growth indexes and reducing the mortality and weight loss of earthworms (Eisenia fetida). The same research group also investigated the remediation of phenanthrene-contaminated soil over the g-C3N4/Fe3O4 nano-photocatalyst.130 The hybrid composite exhibited excellent phenanthrene degradation with a photodegradation efficiency of 92.26% after 120 min of visible light irradiation. It was clear that the hybrid composite showed a better photocatalytic performance compared with the pure g-C3N4, which was attributed to the enhanced separation and transfer of electrons and inhibition of electron–hole recombination in the interface between g-C3N4 and Fe3O4. This was proven by UV-visible diffuse reflection spectra (DRS) and photoluminescence (PL) spectra. Yang et al. reported the application of Z-scheme g-C3N4/α-Fe2O3 in the photocatalytic degradation of fluoranthene in soil.131 The optimal fluoranthene photodegradation efficiency after simulated sunlight irradiation for 12 h at neutral pH was 88.7%, where the mass of contaminated soil was 2 g, initial fluoranthene concentration was 36 mg kg−1, and catalyst dosage was 5%. Compared with P25, g-C3N4/α-Fe2O3 displayed a higher fluoranthene photocatalytic degradation with an increase in removal rate by 50.2%. The enhanced photocatalytic performance could rely on the unique interfacial charge transfer route via the Z-scheme between g-C3N4 and α-Fe2O3, which enhanced the separation and transfer of photogenerated electrons and holes and suppressed their recombination. The degradation mechanism analysis demonstrated that superoxide and hole radicals were the dominant active groups for fluoranthene decontamination. The performances of the above-mentioned nano-catalysts are summarized in Table 2. Lastly, the photocatalysis process paves a clean and cost-effective way for the sustainable remediation of recalcitrant contaminants in polluted soil, but the difficulty in its large-scale use and daylight dependence limit its application.
Table 2 Comparison of different nano-catalysts for the removal of pollutants from soil
Nanocatalyst |
Pollutant |
Process |
Remediation condition |
Nanocatalyst dosage |
Pollutant concentration |
Degradation % |
Interaction mechanism |
Ref. |
Fe@CF–N |
Fluoranthene |
AOPs, PMS activation |
Adding 6.08 mg PMS, reaction time within 180 min |
25 mg catalyst per 5 g soil |
20 mg kg−1 |
77.90% |
PMS and FLT were rapidly adsorbed onto its surface. PMS generates ROS, such as SO4˙−, ·OH and 1O2via electron transfer. The 1, 2 carbon of FLT was attacked by free radicals through cycloaddition of the double bond, electrophilic attack and nucleophilic attack, which resulted in the oxidation of FLT to the intermediate 9H-fluorene-1-carboxylicacid. This intermediate was further decarboxylated to 9H-fluoren-9-ol |
116
|
Fe3O4 nanoparticles |
Pyrene |
Sono-AOPs |
H2O2 activation (concentration of 78 mM) associated with ultrasonic power of 313 W, within 60 min |
18 mM |
100 mg kg−1 soil |
98.37% |
Continuous activation of the Fe3O4 surface produces ·OH radicals via the turbulence generated by cavitation bubble collapse and related microstreaming at the surface of the sonocatalyst |
112
|
Fe3O4 nanoparticles |
Pyrene |
Microwave/AOPs |
Persulfate (PS, 1.5 M) oxidation activated by microwave (MW with temperature of 60 °C) and magnetite (Fe3O4) within 445 min |
40 mg catalyst per 5 g soil |
50 mg kg−1 |
91.7% |
PS was activated by the Fe2+/Fe3+ system. Fe(II) was oxidized to Fe(III) and catalyzed the activation of PS to produce SO4˙−. SO4˙− then reacted with water molecules to produce ·OH. Moreover, Fe(II) could activate O2 to form O2˙−, promoting the degradation of pyrene |
117
|
BC@Ni/Fe |
Decabromodiphenyl ether (BDE209) |
Heterogeneous catalysis |
Adding nanocatalysts to spiked soil within 72 h |
0.12 g catalyst per 2 g soil |
8.7 ± 0.9 mg kg−1 |
87.7% |
Active hydrogen is generated by the corrosion of Fe with water. The active hydrogen species then dissolved into the Ni lattice to form Ni·H2. Finally, a hydrogenation–debromination reaction occurs between Ni·H2 and BDE209 on the surface of Ni/Fe |
118
|
TiO2 nanoparticles |
Diphenyl arsenic acid |
Photocatalysis |
UV irradiation with a 500 W high-pressure mercury lamp (40 mW cm−2 light intensity) for 3 h |
5% w/w TiO2 in soil |
20 mg kg−1 |
82.7% |
Degradation of DPAA by ·OH radicals results in arsenate as the final product |
121
|
TiO2/montmorillonite composite |
γ-Hexachlorocyclohexane |
Photocatalysis |
UV-light irradiation with a 300 W high-pressure mercury lamp for 2 h under alkaline soil |
2% w/w catalyst in soil |
1.23 mg g−1 |
76% |
More ·OH radicals can be formed via the photooxidation of OH− by holes on the composite photocatalyst surfaces, which may contribute to the relatively high photodegradation rate |
122
|
TiO2@ZnHCF |
Acenaphthene, phenanthrene, and fluorene |
Photocatalysis |
Under sunlight for 24 h |
25 mg per 0.5 g soil |
0.5 g of soil spiked with 2.5 mL of the PAH |
82–86% |
The encapsulated nanocomposite with a high surface area and negative charge was able to absorb PAHs. Its superior interaction with H2O or O2 and charge carriers produced ·OH radicals, which degraded and eventually mineralized the PAHs under sunlight |
123
|
KZnHCF nanocubes |
PAHs |
Photocatalysis |
UV light (λmax = 254 nm) and sunlight for 48 h |
50 mg nanoparticles per g soil |
50–200 mg L−1 |
70–93% |
PAHs are adsorbed onto the surface of KZnHCF, where electron–hole pairs produce ·HO active species under sunlight or UV, breaking down the large, harmful PAHs |
124
|
g-C3N4 |
Phenanthrene |
Photocatalysis |
Under visible light (a 250 W xenon lamp with a 420 nm cutoff filter) for 3 h and natural sunlight for 10 h |
60 mg g−1 |
200 mg kg−1 |
81.25% under visible light and 97.07% undue sunlight |
O2˙− and ·OH are the main active radicals for the degradation of phenanthrene in soil |
129
|
g-C3N4/Fe3O4 |
Phenanthrene |
Photocatalysis |
Under visible light (a 250 W xenon lamp with a 420 nm cutoff filter) for 120 min |
30 mg g−1 |
200 mg kg−1 |
92.26% |
Fe3O4 extends the adsorption region of the nanocomposite and suppresses the recombination rate. Phenanthrene can be oxidized by O2˙− and ·OH radicals, as well as photo-induced holes in g-C3N4 |
130
|
g-C3N4/α-Fe2O3 |
Fluoranthene |
Photocatalysis |
Under simulated sunlight irradiation (xenon lamp, 35 W) for 12 h |
5% w/w |
36 mg kg−1 |
88.7% |
Z-scheme charge transfer route was proposed to explain the separation and transfer mechanism of photogenerated charge carriers in the g-C3N4/α-Fe2O3 heterojunction, with O2˙− and h+ being the dominant reactive species for the photocatalytic degradation of fluoranthene |
131
|
4.1.3. Nanobioremediation.
Bioremediation is one of the eco-friendly and cost-effective strategies for cleaning up contaminated soil in a large area.132,133 The combination of nanomaterials with bioremediation can offer a viable approach for stimulating the remediation process by enhancing the growth of microorganisms or immobilizing pollutants through the production of microbial enzymes and biosurfactants in microorganisms.134 Besides its application as an adsorbent, as mentioned above, nZVI has been investigated for initiating the nanobioremediation process, followed by the addition of bacterial strains in soil contaminated with polychlorinated biphenyls (PCB).135 The results showed that the integrated remediation system has the potential for the removal of PCB up to 99%. The merit of using nZVI is its ability for the dechlorination of highly substituted PCBs, which are barely degradable by bacterial strains. Zhang et al. studied the combination of chemical oxidation and biochar-supported nZVI in the presence of PS for the bioremediation of TPH-contaminated soil.136 nZVI was responsible for the oxidation process of TPHs in the soil via the activation of PS. Alternatively, biochar played a crucial role in enhancing the bioremediation by inducing microbial metabolism activities and increasing the TPH-degradation bacterium. This was ascribed to the abundant and external carbon sources in biochar, which could both encourage the growth of carbon-specialized metabolism microbes and enhance the microbial metabolic efficiency.
Phytoremediation is also a green approach to achieve the bioremediation of contaminated soil.137,138 In this process, tolerant plants have the potential to remove pollutants from the soil either by phytostabilization, phytoextraction, rhizodegradation, or phytovolatilization.139 The interaction between nanoparticles and plants for soil cleanup has also been widely investigated to assess the performance of hybrid systems towards biological and environmental systems. For instance, Zand and Heir studied the impact of TiO2 NPs on the growth of Sorghum bicolor and their potential in Sb-contaminated soil remediation.140 According to the data, it was concluded that TiO2 NPs enhanced both the biomass of Sorghum bicolor and bioaccumulation of Sb in the plant parts, especially in the shoots. Based on this evidence, Chen et al. investigated the role of multi-walled carbon nanotubes (MWCNTs) in the growth of Sorghum bicolor and the alleviation of the phytotoxicity and bioavailability of Cd and As in contaminated soil.141 The application of MWCNTs significantly enhanced the growth of Sorghum bicolor, where carbon nanotubes agglomerated on the surface of the plant roots and restrained the bioavailability of Cd and As by MWCNTs in the rhizosphere area. The reduction of the bioavailability of Cd and As reinforced the plant growth and biomass, leading to an enhancement in the phytoextraction efficiency. Besides heavy metals, MWCNTs also played an important role in alleviating the phytotoxicity of herbicides compared with biochar by inhibiting their bioavailable concentration.142 In addition, MWCNTs also promoted the growth of tobacco, and even prolonged MWCNT exposure had no significant effect on the soil microbial diversity. In another study, Romeh and Saber reported that coupling of nanoparticles (Fe0 and Ag0) with P-major plants boosted the removal of chlorfenapyr from the polluted soil.143
4.2. Nano-fertilizers
Two billion people or more around the world suffer from various forms of nutrient deficiencies, causing intense diseases such as anemia for women and blindness for children according to the FAO announcement.1 Considering the limited agricultural arable land (40%) and growing food demand, agricultural production should enormously increase.144 Thus, providing innovative and sustainable ways to improve the productivity of crops is critical for eliminating hunger and malnutrition.145 In this context, fertilization plays a remarkable role in crop productivity given that the crop yield (30–50%) is highly dependent on the input of macronutrients (N, P, K, S, Ca, and Mg) and micronutrients (B, Fe, Mn, Cu, Zn, Mo, and Cl) in the agricultural field.146,147 However, despite the benefits gained from fertilization, the nutrient use efficiency of plants is still less than 50%; this reduction is due to the physical and chemical properties of soil, leaching, gaseous losses, and fertilizer characteristics.148 Utilizing nanomaterials in fertilization for developing novel types of fertilizers (nano-fertilizers) offers a promising trajectory for sustainable agriculture.149,150 Nano-fertilizers are nutrients encapsulated by nanomaterials, enabling slow release for the controlled delivery of essential plant nutrients.150 Recently, significant effort has been devoted to developing smart nano-fertilizers that offer distinct advantages over conventional ones. Nano-fertilizers provide the following advantages: i) sustainable and slow release of nutrients, together with higher nutrient use efficiency, ii) significantly higher uptake due to their ability to freely transport to nano-sized pores, plasmodesmata, and root exudates, iii) economic benefits because they require smaller amounts and result in fewer losses compared to synthetic fertilizers, and iv) eco-friendly with less environmental impact by reducing nutrient leakage and emissions.151,152 The application of nano-fertilizers typically resembles traditional fertilizers and involves foliar spraying, seed impregnation, and soil fertilization. Despite the widespread use of soil fertilization, most applied fertilizers are not readily available to plants.153,154 For instance, 40–70% of nitrogen (N), 80–90% of phosphorus (P), and 50–90% of potassium (K) are lost or fixed in soils, leading to economic losses.153 Therefore, utilizing nano-fertilizers is a favourable avenue for efficient plant nutrition, particularly when applied through soil irrigation, which improves the soil fertility and enhances the nutrient absorption by the roots.153,155 The roots of plants serve as the site of interaction between plants and the soil, facilitating the absorption of nutrients, water, and essential physiological substances.155 Due to their unique physicochemical properties, TiO2-NPs are one of the most widely used nanomaterials worldwide. In the agricultural sector, TiO2-NPs offer a promising agrochemical for enhancing agricultural production.156 The utilization of TiO2 NPs as nano-fertilizer improved the performance and adhesion of plant growth-promoting rhizobacteria (PGPR) to the plant roots, promoting reproducible field usage and sustainable agriculture productivity.157,158 A zinc aluminosilicate (ZnAl2Si10O24) mesoporous nanocomposite, as a new nano-fertilizer carrier, was synthesized, and then loaded with urea.159 This nanocomposite could hold urea longer than bulk aluminosilicates, meeting the demand for the slow release of both zinc and urea. When used as a nano-fertilizer on Oryza sativa L., the urea-loaded ZnAl2Si10O24 nanocomposite resulted in a significantly higher yield compared to commercial urea.
For preferable sustainable agricultural practices, it is essential to adopt innovative economic and environmental appraisal models using multi-criteria evaluation analysis to estimate the benefits of nanofertilizers. These criteria include irrigation water and nitrogen use efficiency, nitrate leaching, greenhouse gas change, energy input, crop yield, etc.160 Heiba et al. developed a novel sustainable irrigation matrix (SIM)-based model in tomato cultivation using four irrigation scenarios, furrow and drip techniques and chemical and nanomaterial fertilizers.161 The analysis results demonstrated that the combination of drip irrigation and the use of nano-fertilizers was the best irrigation technique and was highly influenced by the environmental and economic dimensions, followed by the social pillar of sustainability. Moreover, this study concluded that the SIM-related model can be employed to ensure the sustainable performance of crop irrigation treatments globally. Thus, it has been found that utilizing nano-fertilizers in combination with other techniques can effectively and sustainably increase plant productivity.
4.3. Micro/nano-irrigation technology
The water body is essential for both soil maintenance and agriculture security. Over the ages, flood irrigation has been employed for irrigating cultivated areas, consuming excess capacity and wasting clean water sources. Thus, the last century has witnessed tremendous efforts to develop more water-economical technology to save water and provide a minimum water capacity required for cultivation. In this case, drip irrigation technology is the most efficient among modern irrigation systems and ideal for delivering nutrients to the root zone, optimizing nutrient use efficiency.4 During the last decade, nanotechnology drip irrigation has sparked novel concepts in the field of agricultural water management.162,163 This strategy can reduce the water consumption by up to 80% compared with traditional irrigation systems. Nano-irrigation systems can be divided into two main categories, nano-pores pipes or tubes and micro/nano bubble aerated irrigation. An early nano-pore irrigation system was developed from moulded clay mixed with sawdust for an emitter diaphragm.164 A clay diaphragm mixed with sawdust at a ratio of 7
:
3 (clay to sawdust) was quite suitable for meeting the discharge of water quantity (2 L per day) equivalent of the water requirement of okra and pumpkin vegetables. Furthermore, it was found that the nano-emitter was 100 times cost effective than that of other pottery wares around the study area. Al-Issawi et al. compared two trickle irrigation systems, a mobile system and a subsurface nano-irrigation system, using root guard drippers to evaluate the water use efficiency and yield of beans.165 The results showed that the water use efficiency of subsurface nano-irrigation (1.73) was higher than that of the mobile system (1.14). Moreover, the bean productivity significantly increased under the subsurface nano-irrigation system, producing 7.71 tons per ha compared to 6.62 tons per ha obtained from mobile trickle irrigation.
Recently, nano irrigation has been applied and developed on a large scale such as the Moistube irrigation system.166 In this system, subsurface water flows out of the Moistube nanopores as a function of soil water potential and operating pressure and it consists of two semipermeable layers, as shown in Fig. 5a. The inner semipermeable layer has approximately 100
000 nanopores per square centimeter with a pore diameter range of 10–900 nm.167 When the soil is dry, water oozes out from the Moistube nanopores until potential equilibrium between the two sides of the membrane is achieved. At this point, the water seepage will reduce or stop. The Moistube flow due to soil water potential is shown in Fig. 5b.167,168 As illustrated in Fig. 5c, Moistube shows superior water consumption efficiency and does not need mechanical power to operate the system, which reduces the consumed energy to 95% compared to traditional drip irrigation systems.168
 |
| Fig. 5 (a) Internal structure of Moistube,169 (b) water flow from Moistube to the surrounding soil, depending on soil water potential, and (c) irrigation water use efficiency of Moistube compared to the conventional surface drip irrigation system. | |
Instead of nano-pores pipes, tubes, or pottery, micro/nano bubble (MNB) water oxygenation or aerated irrigation has been also reported to investigate its effects on the irrigation water use efficiency and crop yield and quality.170,171 In this technique, MNB can be mostly generated by a Venturi oxygenation generator, where air bubbles are broken to narrower sizes, i.e. micro and nanoscale, under low pressure and high velocity, and transmitted through tapered output flow.172 Moreover, MNB can be also generated by ultrasonication or by chemical reactions such as electrolysis.173,174 MNB irrigation can foster soil fertility through enhanced nutrient utilization efficiency, save water and fertilizer quantity, improve hypoxia stress, and promote plant production and quality.172,175,176 Besides, MNB irrigation can reinforce the potential bacterial functions related to nitrification and nitrogen fixation and enhance the dynamic mutual feedback between soil fertility and microorganisms.177,178 Liu et al. investigated the role of integrating MNB water and groundwater with different oxygenation frequencies on the yield and quality of cucumber and tomato cultivation.162 The results indicated that the integrated micro-nano bubble water oxygenation system could significantly increase the quantity and quality of cucumber and tomato yield, as well as the irrigation water use efficiency. This is due to the longer retention time, stronger mass transfer ability of micro-nano bubbles, and the increment in soil oxygen content. Recently, Khan et al. treated water with CO2 and air to obtain MNB, using tap water as a control.179 According to the results, it was concluded that MNB + CO2 enhanced both the physical and chemical parameters of the amaranth plant compared to MNB + air and the control (tap water). The chemical parameters of amaranth promoted by the MNB + CO2 treatment included amino acid content, soluble sugar content, protein content, plant height, and significant enhancement in leaf area. MNB not only directly enhanced the quality and yield of the plant, but also improved the quality of the drip irrigation emitter. For example, Li et al. reported that micro/nano aeration efficaciously hindered the formation of clogging materials connected to the emitter flow channel.180 The influential effect of MNB is attributed to the significant depression of the abundance and diversity of the microbial communities in the clogging material attached to the emitter and reduction in the number of core flora affecting the clogging of the emitter. In conclusion, nano-irrigation systems open a sustainable way toward achieving optimal water consumption and high crop quality and yield.
5. Challenges in the application of nanotechnology in soil management
Despite the promising application of nanotechnology in enhancing soil quality and ensuring food security, critical barriers should be addressed before its practical enforcement, including a lack of mechanistic understanding of nano-bio (plants and microorganisms) interactions, the actual impact of the fate and transportation of applied nanotechnology on the surrounding environment, and insufficient grasping of human health risks from the chain food impacted by nanomaterials. In addition, the prolonged stability and performance of nanoparticles in the soil matrix and how long they can maintain their performance are also crucial.
5.1. Environmental toxicity
The application of nanomaterials in the agricultural sector has become popular for solving agricultural problems and achieving sustainability.181 However, employing nanomaterials in agriculture (i.e., soil) has drawbacks related to their ecological and toxicological impacts that are not observed with bulk particles.182 Nanomaterial-based agrochemicals can be prepared from several types of nanoparticles with extraordinary physical and chemical properties (specific surface structures and reactivity), and these nanoparticles can generate reactive oxygen species (ROS).183,184 As a result, the formation of ROS leads to non-targeted cell death/apoptosis, besides the premature cell death caused by the undesirable involvement of nanoparticles.184 The toxicity of nanoparticles depends on distinct factors including particle size, applied dose, composition of the fabricated material, functionality, and crystallinity.185 Recent studies have shown that exposure to higher concentrations of nanomaterials has negative effects on plants, whereas utilizing a lower dose under specific conditions has beneficial effects.186 However, the poor understanding of how nanomaterials transform and behave in the environment is an obstacle in entirely assessing their potential risk in the long term.11 Moreover, there is uncertainty regarding how nanofertilizers may impact the overall greenhouse gas emissions from their production and use.160 This is why the Food and Drug Administration (FDA) considered the potential harmful impacts of nanomaterials, not regarding them as completely safe or dangerous for use.151 Thus, future research should focus on expanding the knowledge about nano-bio interactions and increasing the information on nanomaterial-associated environmental toxicity.
5.2. Performance stability
The long-term stability of the performance of nanoparticles in soil bodies is also important for their lasting benefits. For instance, soil organic matter may exhibit a paradoxical effect on the stability and mobility of nanomaterials in the soil depending upon their nature. Organic matter tends to accelerate the aggregation of nanomaterials by bridging flocculation, which impacts the surface area, and thus the feature and potential of nano-based substances such as nano-adsorbents and catalysts.9 Regarding the bioremediation process, its prolonged remediation periods and its limitation to high pollutant concentration may obstruct its applicability, particularly in contaminated areas requiring an urgent call.187,188
Alternatively, controlling the delivery behaviour of nano-fertilizers and nanocarriers of nutrients into plants, either through soil irrigation application or foliar spray, at the appropriate dose, place, and time remains a formidable challenge. In nutrient delivery to roots in soil irrigation application, the untargeted transportation of nano-fertilizers into the soil, even at low concentrations, is likely to be energy inefficient, owing to the sheer amount of soil to be treated and the relatively high embodied energy of many nanomaterials. The applicability of the foliar spray technique is restrained by climate-related stressors such as weather (rain or wind can remove the applied nanomaterials) and ultraviolet degradation of nano-fertilizers.18 Thus, developing smart nanomaterials with surface functionality or coating that are not suppressed by biomacromolecules, such as proteins in plant cells and organic matter in the soil, and tolerance to climate and environmental triggers, such as pH and salinity, is urgently demanded to achieve the utmost benefits and long-lasting food security.
Clogging or fouling issues of nanopores in Moistube, as another example, may hinder its performance stability. The clogging of Moistube is mainly affected by the presence of dissolved solids, suspended solids and microbiological impurities such as bacteria, algae, and vegetative matter in water sources. In addition, the existence of soil particles in the irrigation water causes the clogging of its pores, where the soil particle concentration and size are positively correlated with the clogging degree of nanopores.189 In contrast, some factors positively influence the performance of Moistube such as the temperature and pressure, owing to the elastic and polymeric semi-permeable nature of Moistube. Nevertheless, the evidence of how the Moistube discharge dynamics is affected by other water sources such as wastewater and saline water is limited and needs further research to reduce the pressure on the freshwater resources.190
5.3. Scalability and cost-effectiveness
The commercialization rate of nanotechnology in the agricultural sector has vastly accelerated during the last few years. However, several parameters may suppress the pace of its growth including the high-cost manufacturing of nano-based materials and the extravagant scaling up and implementation of nanotechnology with a sheer area of real soils. Besides, given that most promising nanotechnology applications in soil science are still on the lab scale, their scalability without compromising their function and quality remains a great hurdle.
In the case of nano-fertilizers, for example, considering the total cost of N, P, and K nano-fertilizers, utilizing lower dosages is estimated to be about $350 per ha compared to $200 per ha for conventional fertilizers in wheat cultivation.160 Su et al. performed a preliminary cost–benefit analysis of employing nano-fertilizers compared to traditional fertilizers.160 The cost–benefit analysis showed that current nano-fertilizers, partially replacing their conventional counterparts, can potentially increase net revenues from crop production while reducing the environmental impact. Moreover, their study concluded that further innovation is needed to improve the efficiency of nano-fertilizers for their widescale adoption.
Alternatively, the scalability of nano-irrigation technology faces some obstacles. One of them is the abovementioned clogging effects on Moistube nanopores, limiting its scalability. In this context, the maintenance of pores fouling requires additional chemical treatment or a pre-handling process, which increases the costs and environment impact. To minimize or prevent the fouling impact, chlorine and phosphoric acid will be added to prohibit bacteria film growth inside the lines. Besides, acid injections are required to dissolve the chemical precipitates such as magnesium and calcium carbonates. Filtration systems may be also demanded for the pretreatment elimination of the suspended solids, which need high energy for occasionally flushing these particles.189 In addition, the large-scale application of Moistube is unsuitable for all crops requiring high irrigation water amounts such as rice, sugarcane, and cotton. On the contrary, it will be more promising and appropriate for nanotechnology in arid or semi-arid regions.
6. Prospective trends
6.1. Life cycle assessment
Performing a life cycle assessment (LCA), which is based on the concept of life cycle thinking, of nanotechnology-based soil applications is considered a valuable tool for evaluating the potential of their environmental footprints.191 LCA can furnish pervasive insights in assessing the ecological impacts of applied nanotechnologies from the inception to the conclusion, by considering all stages from nanomaterials synthesis to transportation, field applications, and final pathways. The application of the nano-soil–plant nexus can benefit from LCA to identify potential zones for improvement, identify potential “environmental hotspots”, and enhance their environmental sustainability.16 Therefore, it is essential to conduct specific nanotechnological LCAs to optimize green synthesis methods, maximize cost-effectiveness, and address potential environmental risks.
It is necessary to develop an accurate step-by-step LCA evaluation system for agricultural nanomaterials to address the lack of knowledge regarding the availability, variability, and uncertainty associated with elevating emerging nanotechnologies between laboratory-scale and full-scale applications.16,192 In this respect, field-scale studies are required to track nanotechnology flows and allow a comprehensive and reliable data inventory. LCA systems should adopt and incorporate complete literature data on nano-specific properties to enhance their fate, behavior, and persistence in agricultural soil. Besides, developing nano-specific fate models to understand transformations and interaction mechanisms, such as aggregations, stability, dissolution and nano-eco-bio interactions, with different types of soil invertebrates and plants should be integrated with full toxicological assessments and analyses on the entire life cycle.193 By applying an anticipatory approach, alternatives are ranked probabilistically based on their environmental preferences, allowing researchers and practitioners to prioritize research opportunities to advance the methodology. These pathways are identified and suggested as potential trials towards incorporating nano-specific behaviors, reducing the data uncertainty and facilitating decision-making.192
6.2. Machine learning
Owing to the global population growth, diminishing number of farmers, and labour shortage associated with the complexity of available heterogeneous data of smart nanotechnology applications, new automated approaches are essential to meet the requirements of agriculture sustainability and facilitate the performance and decision-making by farmers. Researchers have been working on “smart farming” by using nanotechnologies to improve the quality of the soil microbial diversity and quantity of agricultural products, making them intelligent, cost-effective, and sustainable.194 In recent years, artificial intelligence (AI) has become more accessible to a wider range of parts of the world economy, with inroads into almost all industries due to advances in computational capabilities and increased cloud penetration. The agricultural sector is one of the main fields that has started to reap the benefits of AI.194 Similar to other computer-based techniques, machine learning (ML) models can reveal complex relationships and project future trends with great effectiveness. The use of machine learning and integrating lab and field data will boost the precision and sustainable nano-enhanced soil quality and agricultural production by analyzing variable covariance and making predictions at the local and global scales.195
As a baseline for using eco-friendly nanoparticles, ML can provide a profile of nanoparticle properties, which can maximize their performance. In addition, ML can be used for environmental risk assessment (ERA) of nanomaterials to ensure sustainability.196 One of the main ML tools relevant to ERA is automated image analysis, which is mainly performed using classification techniques. It can improve nanoparticle identification in transmission electron microscopy (TEM)/scanning electron microscopy (SEM) images and speed up the semi-quantification of particle size and number with convolutional neural networks. Additionally, automated image analysis has also been used for environmental monitoring by identifying species in digital images of soil, sediment, and water using ML algorithms.196 ML can combine information from different molecular levels within organisms and from population data, together with nanomaterial descriptors. Using this approach, it is possible to discover mechanisms of toxicity and develop safe and sustainable nanomaterials by design and for grouping or read-across. Alternatively, a spatial–temporal analysis (for example, fate over time in a diverse landscape) and omics analysis (e.g., determining transcript-, proteome-, and metabolomics over time) are particularly useful for ERA, which involves highly complex multidimensional datasets.196,197 In modern agriculture, machine learning integrating laboratory and field studies will promote the development of nanomaterials and enhance agriculture and soil quality standards.195
6.3. Guidelines and regulation policies
The use of nanotechnologies in soil applications requires regulatory guidelines to ensure their safety. Governments and legislative bodies should work together with scientists, industries, and other collaborators to develop appropriate guidelines for the green and cost-effective synthesis, spacious and productive application, and safe fate and disposal of nanomaterials. In this way, the potential negative environmental impact of nanomaterials will be minimized, and the sustainable use of nanotechnology will be enhanced. Moreover, adopting dynamics and reliable guidelines and regulations will rely on the research results obtained under real-field conditions on a large scale.16 Using green and sustainable nanotechnologies should be marketed more effectively to the public and farmers, making them aware of their benefits and safety protocols.
7. Conclusion
This review shed light on the advancements of sustainable nanotechnology applications in enhancing soil quality via nano-enabled soil reclamation, nutrition and efficient irrigation water use. Owing to their abundant features and unique physical and chemical characteristics, nanomaterials are recognized as highly efficient materials and ideal candidates for implementation in all sectors including the agricultural sector compared to traditional compounds. Nanomaterials can be prepared using two primary strategies, “bottom-up and top-down processes”. The bottom-up approach is the preferred technology given that it can be employed to manufacture materials with precise qualities customized to the task requirement based on the fabrication pathway chosen. Nanomaterials play an important role in the sustainability of soil management including the remediation and recovery of contaminated soil, providing nano-fertilizers, and enhancement of micro/nano-irrigation technology in agriculture production. Reducing the mobility and bioavailability of contaminants is crucial in the sustainable remediation of contaminated soil. Nanomaterials have sparked tremendous attraction in the immobilization and conversion of pollutant molecules to harmless byproducts in the soil matrix. Iron-based nanoparticles especially nano zero-valent iron (nZVI) and other nanomaterials, e.g., nano-metal oxides, nano-carbon, nano-biochar and biochar-supported nanoparticles, have been widely applied to immobilize the pollutants in contaminated soil. In addition, natural polymers and minerals demonstrate promising and green adsorption performances. As sustainable nano-enhanced catalytic processes, TiO2 and g-C3N4 nanocomposites show propitious pollutant removal, particularly for recalcitrant organic pollutants. Alternatively, nano-enabled bioremediation and phytoremediation are considered promising strategies for green and cost-effective field-scale cleaning up.
Fertilization is vital in crop production given that their yield depends on macronutrients and micronutrient inputs. Herein, we summarized the development of green and smart nano-fertilizers, offering innovative and sustainable methods to improve soil nutrition and enhance crop productivity. Alternatively, developing more water-efficient technology to minimize water wastage and ensure a sufficient water supply for cultivation has been achieved by utilizing nano-irrigation technology. Nanotechnology drip irrigation also introduces innovative concepts in agricultural water management, reducing water consumption by 80% compared to traditional irrigation systems. Despite the tremendous advantages of nanotechnology for enforcing soil quality and promoting crop production yields, there are still gaps that should be carefully managed including the unclear mechanistic fate and behaviour of nanomaterials and their stability in the environment. Besides, the scalability and cost of the manufacturing and application of nanotechnology compared to the sheer area of the existing land may be a real challenge for upscaling this promising technology. Thus, integrating life cycle assessment and machine learning coupled with embracing active and reliable guidelines and regulations will create new opportunities towards achieving sustainable nanotechnologies in soil and agricultural applications.
Data availability
The data presented in Fig. 1a were obtained from the Web of Science (https://access.clarivate.com/login?app=wosalternative=trueshibShireURL=https:%2F%2Fwww.webofknowledge.com%2F%3Fauth%3DShibbolethshibReturnURL=https:%2F%2Fwww.webofknowledge.com%2Froaming=true) and designed by OriginLab (https://www.originlab.com/).
The co-occurrence network in Fig. 2b was extracted from the Web of Science database and analyzed using VOSviewer software (https://www.vosviewer.com/).
Fig. 5a was redesigned from https://doi.org/10.1016/j.jhydrol.2024.131083.
Fig. 2–5b and c were generated using Microsoft PowerPoint (https://www.microsoft.com/en-us/microsoft-365/powerpoint).
Conflicts of interest
There are no conflicts to declare.
Acknowledgements
The authors would like to express their gratitude to the National Committee of Soil Science in Egypt for its scientific support.
References
-
J. Bruinsma, World agriculture: Towards 2015/2030: An FAO Study, Food and Agriculture Organization of the United Nations (FAO), 2017, 1–431 Search PubMed.
-
United Nations, The Sustainable Development Goals Report 2020, 2020 Search PubMed.
- G. Liu, C. Zhang, M. Zhao, W. Guo and Q. Luo, Comparison of nanomaterials with other unconventional materials used as additives for soil improvement in the context of sustainable development: A review, Nanomaterials, 2021, 11, 1–26 CAS.
-
M. Zaman, S. A. Shahid and L. Heng, Guideline for Salinity Assessment, Mitigation and Adaptation Using Nuclear and Related Techniques, SpringerOpen, 2018 Search PubMed.
- Á. Horel, G. Gelybó, I. Potyó, K. Pokovai and Z. Bakacsi, Soil nutrient dynamics and nitrogen fixation rate changes over plant growth in temperate soil, Agronomy, 2019, 9, 179 CrossRef.
- S. Mobasser and A. A. Firoozi, Review of Nanotechnology Applications in Science and Engineering, Journal of Civil Engineering and Urbanism, 2016, 6, 84–93 Search PubMed.
- S. A. Ashraf, A. J. Siddiqui, A. E. O. Elkhalifa, M. I. Khan, M. Patel, M. Alreshidi, A. Moin, R. Singh, M. Snoussi and M. Adnan, Innovations in nanoscience for the sustainable development of food and agriculture with implications on health and environment, Sci. Total Environ., 2021, 768, 144990 CrossRef CAS PubMed.
- J. Zhu, G. Dong, F. Feng, J. Ye, C.-H. Liao, C.-H. Wu and S.-C. Chen, Microplastics in the soil environment: Focusing on the sources, its transformation and change in morphology, Sci. Total Environ., 2023, 896, 165291 CrossRef CAS PubMed.
- M. Usman, M. Farooq, A. Wakeel, A. Nawaz, S. A. Cheema, H. ur Rehman, I. Ashraf and M. Sanaullah, Nanotechnology in agriculture: Current status, challenges and future opportunities, Sci. Total Environ., 2020, 721, 137778 CrossRef CAS PubMed.
- G. N. G. Saritha, T. Anju and A. Kumar, Nanotechnology - Big impact: How nanotechnology is changing the future of agriculture?, J. Agric. Food Res., 2022, 10, 1 Search PubMed.
- T. Hofmann, G. V. Lowry, S. Ghoshal, N. Tufenkji, D. Brambilla, J. R. Dutcher, L. M. Gilbertson, J. P. Giraldo, J. M. Kinsella, M. P. Landry, W. Lovell, R. Naccache, M. Paret, J. A. Pedersen, J. M. Unrine, J. C. White and K. J. Wilkinson, Technology readiness and overcoming barriers to sustainably implement nanotechnology-enabled plant agriculture, Nat. Food, 2020, 1, 416–425 CrossRef CAS.
- J. Liu, J. Jiang, Y. Meng, A. Aihemaiti, Y. Xu, H. Xiang, Y. Gao and X. Chen, Preparation, environmental application and prospect of biochar-supported metal nanoparticles: A review, J. Hazard. Mater., 2020, 388, 122026 CrossRef CAS PubMed.
- I. Corsi, I. Venditti, F. Trotta and C. Punta, Environmental safety of nanotechnologies: The eco-design of manufactured nanomaterials for environmental remediation, Sci. Total Environ., 2023, 864, 161181 CrossRef PubMed.
- Y. Qian, C. Qin, M. Chen and S. Lin, Nanotechnology in soil remediation − applications vs. implications, Ecotoxicol. Environ. Saf., 2020, 201, 110815 CrossRef CAS PubMed.
- T. Sathish, N. Ahalya, M. Thirunavukkarasu, T. S. Senthil, Z. Hussain, M. I. Haque Siddiqui, H. Panchal and K. Kumar Sadasivuni, A comprehensive review on the novel approaches using nanomaterials for the remediation of soil and water pollution, Alexandria Eng. J., 2024, 86, 373–385 Search PubMed.
- S. A. Younis, K. H. Kim, S. M. Shaheen, V. Antoniadis, Y. F. Tsang, J. Rinklebe, A. Deep and R. J. C. Brown, Advancements of nanotechnologies in crop promotion and soil fertility: Benefits, life cycle assessment, and legislation policies, Renewable Sustainable Energy Rev., 2021, 152, 111686 CrossRef CAS.
- S. Mariyam, S. K. Upadhyay, K. Chakraborty, K. K. Verma, J. S. Duhan, S. Muneer, M. Meena, R. K. Sharma, G. Ghodake and C. S. Seth, Nanotechnology, a frontier in agricultural science, a novel approach in abiotic stress management and convergence with new age medicine-A review, Sci. Total Environ., 2024, 912, 169097 CrossRef CAS PubMed.
- G. V. Lowry, A. Avellan and L. M. Gilbertson, Opportunities and challenges for nanotechnology in the agri-tech revolution, Nat. Nanotechnol., 2019, 14, 517–522 Search PubMed.
- S. Sharma, B. K. Sahu, P. Bindra, K. Kaur and S. Vijayakumar, Porous nanomaterials: main vein of agriculture nanotechnology, Prog. Mater. Sci., 2021, 121, 100812 Search PubMed.
- A. Wahab, M. Muhammad, S. Ullah, G. Abdi, G. M. Shah, W. Zaman and A. Ayaz, Agriculture and environmental management through nanotechnology: Eco-friendly nanomaterial synthesis for soil-plant systems, food safety, and sustainability, Sci. Total Environ., 2024, 926, 171862 CrossRef CAS PubMed.
- N. Dasgupta, S. Ranjan and C. Ramalingam, Applications of nanotechnology in agriculture and water quality management, Environ. Chem. Lett., 2017, 15, 591–605 CrossRef CAS.
- N. A. Fathy, Carbon nanotubes synthesis using carbonization of pretreated rice straw through chemical vapor deposition of camphor, RSC Adv., 2017, 7, 28535–28541 RSC.
-
J. Virkutyte and R. S. Varma, Sustainable Nanotechnology and the Environment: Advances and Achievements, in Green Synthesis of Nanomaterials: Environmental Aspects, American Chemical Society, 2013, vol. 1124, pp. 11–39 Search PubMed.
-
C. Han, M. Pelaez, M. N. Nadagouda, S. O. Obare, P. Falaras, P. S. M. Dunlop, J. A. Byrne, H. Choi and D. D. Dionysiou, The Green Synthesis and Environmental Applications of Nanomaterials, in Sustainable Preparation of Metal Nanoparticles: Methods and Applications, Royal Society of Chemistry, 2013, pp. 106–143 Search PubMed.
- J. Tong, L. Zhang, F. Li, M. Li and S. Cao, An efficient top-down approach for the fabrication of large-aspect-ratio g-C3N4 nanosheets with enhanced photocatalytic activities, Phys. Chem. Chem. Phys., 2015, 17, 23532–23537 RSC.
- A. Chen, H. Wang, X. Zhan, K. Gong, W. Xie, W. Liang, W. Zhang and C. Peng, Applications and synergistic degradation mechanisms of nZVI-modified biochar for the remediation of organic polluted soil and water: A review, Sci. Total Environ., 2024, 911, 168548 CrossRef CAS PubMed.
- Y. Jiang, G. H. Ryu, S. H. Joo, X. Chen, S. H. Lee, X. Chen, M. Huang, X. Wu, D. Luo, Y. Huang, J. H. Lee, B. Wang, X. Zhang, S. K. Kwak, Z. Lee and R. S. Ruoff, Porous Two-Dimensional Monolayer Metal-Organic Framework Material and Its Use for the Size-Selective Separation of Nanoparticles, ACS Appl. Mater. Interfaces, 2017, 9, 28107–28116 CrossRef CAS PubMed.
- Y. Zeng, X. Liu, C. Liu, L. Wang, Y. Xia, S. Zhang, S. Luo and Y. Pei, Scalable one-step production of porous oxygen-doped g-C3N4 nanorods with effective electron separation for excellent visible-light photocatalytic activity, Appl. Catal., B, 2018, 224, 1–9 CrossRef CAS.
-
M. Tulinski and M. Jurczyk, Nanomaterials Synthesis Methods, in Metrology and Standardization of Nanotechnology, Wiley-VCH Verlag GmbH & Co. KGaA, 2017, pp. 75–98 Search PubMed.
- P. Wang, H. Ye, Y.-X. Yin, H. Chen, Y.-B. Bian, Z.-R. Wang, F.-F. Cao and Y.-G. Guo, Fungi-Enabled Synthesis of Ultrahigh-Surface-Area Porous Carbon, Adv. Mater., 2018, 1805134, 1–7 Search PubMed.
- L. Huang, M. He, B. Chen, Q. Cheng and B. Hu, Facile Green Synthesis of Magnetic Porous Organic Polymers for Rapid Removal and Separation of Methylene Blue, ACS Sustainable Chem. Eng., 2017, 5, 4050–4055 CrossRef CAS.
- M. S. McKee and J. Filser, Impacts of metal-based engineered nanomaterials on soil communities, Environ. Sci.:Nano, 2016, 3, 506–533 RSC.
- Y. Lu and S. Ozcan, Green nanomaterials: On track for a sustainable future, Nano Today, 2015, 10, 417–420 CrossRef CAS.
-
N. Sh. El-Gendy and B. A. Omran, in Green Synthesis of Nanoparticles for Water Treatment, Scrivener Publishing LLC, 2019, pp. 205–264 Search PubMed.
- C. Pandit, A. Roy, S. Ghotekar, A. Khusro, M. N. Islam, T. Bin Emran, S. E. Lam, M. U. Khandaker and D. A. Bradley, Biological agents for synthesis of nanoparticles and their applications, J. King Saud Univ., Sci., 2022, 34, 101869 CrossRef.
- D. Ballottin, S. Fulaz, F. Cabrini, J. Tsukamoto, N. Durán, O. L. Alves and L. Tasic, Antimicrobial textiles: Biogenic silver nanoparticles against Candida and Xanthomonas, Mater. Sci. Eng., C, 2017, 75, 582–589 CrossRef CAS PubMed.
- A. Pugazhendhi, D. Prabakar, J. M. Jacob, I. Karuppusamy and R. G. Saratale, Synthesis and characterization of silver nanoparticles using Gelidium amansii and its antimicrobial property against various pathogenic bacteria, Microb. Pathog., 2018, 114, 41–45 CrossRef CAS PubMed.
- S. Thanganadar Appapalam and R. Panchamoorthy, Aerva lanata mediated phytofabrication of silver nanoparticles and evaluation of their antibacterial activity against wound associated bacteria, J. Taiwan Inst. Chem. Eng., 2017, 78, 539–551 CrossRef CAS.
- M. M. Khin, A. S. Nair, V. J. Babu, R. Murugan and S. Ramakrishna, A review on nanomaterials for environmental remediation, Energy Environ. Sci., 2012, 5, 8075–8109 RSC.
- M. A. Gatoo, S. Naseem, M. Y. Arfat, A. Mahmood Dar, K. Qasim and S. Zubair, Physicochemical properties of nanomaterials: Implication in associated toxic manifestations, BioMed Res. Int., 2014, 2014, 498420 Search PubMed.
- M. Khajeh, S. Laurent and K. Dastafkan, Nanoadsorbents: Classification, preparation, and applications (with emphasis on aqueous media), Chem. Rev., 2013, 113, 7728–7768 CrossRef CAS PubMed.
- M. E. A. El-sayed, Assess the influence of using treated wastewater by nano hydroxyapatite and its modification on some soil and faba bean plant properties, N. Y. Sci. J., 2019, 12, 1–7 Search PubMed.
- J. O. Tijani, O. O. Fatoba, O. O. Babajide and L. F. Petrik, Pharmaceuticals, endocrine disruptors, personal care products, nanomaterials and perfluorinated pollutants: a review, Environ. Chem. Lett., 2016, 14, 27–49 CrossRef CAS.
- R. Qi, D. L. Jones, Z. Li, Q. Liu and C. Yan, Behavior of microplastics and plastic film residues in the soil environment: A critical review, Sci. Total Environ., 2020, 703, 134722 CrossRef CAS PubMed.
- A. S. Ganie, S. Bano, N. Khan, S. Sultana, Z. Rehman, M. M. Rahman, S. Sabir, F. Coulon and M. Z. Khan, Nanoremediation technologies for sustainable remediation of contaminated environments: Recent advances and challenges, Chemosphere, 2021, 275, 130065 CrossRef CAS PubMed.
- Z. Li, L. Wang, J. Meng, X. Liu, J. Xu, F. Wang and P. Brookes, Zeolite-supported nanoscale zero-valent iron: New findings on simultaneous adsorption of Cd(II), Pb(II), and As(III) in aqueous solution and soil, J. Hazard. Mater., 2018, 344, 1–11 CrossRef CAS PubMed.
- F. O. Sefiloglu, U. Tezel and I. A. Balcloǧlu, Validation of an Analytical Workflow for the Analysis of Pesticide and Emerging Organic Contaminant Residues in Paddy Soil and Rice, J. Agric. Food Chem., 2021, 69, 3298–3306 CrossRef CAS PubMed.
- Y. Li, H. P. Zhao and L. Zhu, Remediation of soil contaminated with organic compounds by nanoscale zero-valent iron: A review, Sci. Total Environ., 2021, 760, 143413 CrossRef CAS PubMed.
- M. N. H. Sani, M. Amin, A. B. Siddique, S. O. Nasif, B. B. Ghaley, L. Ge, F. Wang and J. W. H. Yong, Waste-derived nanobiochar: A new avenue towards sustainable agriculture, environment, and circular bioeconomy, Sci. Total Environ., 2023, 905, 166881 CrossRef CAS PubMed.
- H. K. Pathak, C. S. Seth, P. K. Chauhan, G. Dubey, G. Singh, D. Jain, S. K. Upadhyay, P. Dwivedi and K. S. Khoo, Recent advancement of nano-biochar for the remediation of heavy metals and emerging contaminants: Mechanism, adsorption kinetic model, plant growth and development, Environ. Res., 2024, 255, 119136 CrossRef CAS PubMed.
- A. Chen, H. Wang, X. Zhan, K. Gong, W. Xie, W. Liang, W. Zhang and C. Peng, Applications and synergistic degradation mechanisms of nZVI-modified biochar for the remediation of organic polluted soil and water: A review, Sci. Total Environ., 2024, 911, 168548 CrossRef CAS PubMed.
-
M. E. A. El-Sayed, H. Ayoub and I. Abdelhafeez, Agricultural Applications of Activated Carbon,in Activated Carbon: Progress and Applications, Royal Society of Chemistry, 2023, pp. 134–151 Search PubMed.
- Z. Sun, M. Zhao, L. Chen, Z. Gong, J. Hu and D. Ma, Electrokinetic remediation for the removal of heavy metals in soil: Limitations, solutions and prospection, Sci. Total Environ., 2023, 903, 165970 CrossRef CAS PubMed.
- D. Lin, G. Hu, H. Li, F. Wu, L. Li, G. Yang, L. Zhuang and Y. Gong, Green remediation of mercury-contaminated soil using iron sulfide nanoparticles: Immobilization performance and mechanisms, effects on soil properties, and life cycle assessment, Sci. Total Environ., 2024, 944, 173928 CrossRef CAS PubMed.
- J. Z. Su, M. Y. Zhang, W. H. Xu, W. M. Xu, C. Liu, S. Rui, Y. F. Tuo, X. H. He and P. Xiang, Preparation and applications of iron/biochar composites in remediation of heavy metal contaminated soils: Current status and further perspectives, Environ. Technol. Innovation, 2024, 35, 103671 CrossRef CAS.
- T. Zheng, Q. Zhou, Z. Tao and S. Ouyang, Magnetic iron-based nanoparticles biogeochemical behavior in soil-plant system: A critical review, Sci. Total Environ., 2023, 904, 166643 CrossRef CAS PubMed.
- D. Baragaño, J. Alonso, J. R. Gallego, M. C. Lobo and M. Gil-Díaz, Zero valent iron and goethite nanoparticles as new promising remediation techniques for As-polluted soils, Chemosphere, 2020, 238, 1246424 CrossRef PubMed.
- A. Zialame, A. Jamshidi-Zanjani and A. K. Darban, Stabilized magnetite nanoparticles for the remediation of arsenic contaminated soil, J. Environ. Chem. Eng., 2021, 9, 104821 CrossRef CAS.
- Y. Guo, X. Li, L. Liang, Z. Lin, X. Su and W. Zhang, Immobilization of cadmium in contaminated soils using sulfidated nanoscale zero-valent iron: Effectiveness and remediation mechanism, J. Hazard. Mater., 2021, 420, 126605 CrossRef CAS PubMed.
- Z. Han, O. A. Salawu, J. E. Zenobio, Y. Zhao and A. S. Adeleye, Emerging investigator series: immobilization of arsenic in soil by nanoscale zerovalent iron: role of sulfidation and application of machine learning, Environ. Sci.:Nano, 2021, 8, 619–633 RSC.
- Z. Li, L. Wang, J. Wu, Y. Xu, F. Wang, X. Tang, J. Xu, Y. S. Ok, J. Meng and X. Liu, Zeolite-supported nanoscale zero-valent iron for immobilization of cadmium, lead, and arsenic in farmland soils: Encapsulation mechanisms and indigenous microbial responses, Environ. Pollut., 2020, 260, 114098 CrossRef CAS PubMed.
- J. Yang, S. Wang, N. Xu, Z. Ye, H. Yang and X. Huangfu, Synthesis of montmorillonite-supported nano-zero-valent iron via green tea extract: Enhanced transport and application for hexavalent chromium removal from water and soil, J. Hazard. Mater., 2021, 419, 126461 CrossRef CAS PubMed.
- J. Liu, J. Jiang, Y. Meng, A. Aihemaiti, Y. Xu, H. Xiang, Y. Gao and X. Chen, Preparation, environmental application and prospect of biochar-supported metal nanoparticles : A review, J. Hazard. Mater., 2020, 388, 122026 CrossRef CAS PubMed.
- H. Lyu, J. Tang, M. Cui, B. Gao and B. Shen, Biochar/iron (BC/Fe) composites for soil and groundwater remediation: Synthesis, applications, and mechanisms, Chemosphere, 2020, 246, 125609 CrossRef CAS PubMed.
- A. El-Naggar, N. Ahmed, A. Mosa, N. K. Niazi, B. Yousaf, A. Sharma, B. Sarkar, Y. Cai and S. X. Chang, Nickel in soil and water: Sources, biogeochemistry, and remediation using biochar, J. Hazard. Mater., 2021, 419, 126421 CrossRef CAS PubMed.
- J. Anae, N. Ahmad, V. Kumar, V. K. Thakur, T. Gutierrez, X. J. Yang, C. Cai, Z. Yang and F. Coulon, Recent advances in biochar engineering for soil contaminated with complex chemical mixtures: Remediation strategies and future perspectives, Sci. Total Environ., 2021, 767, 144351 CrossRef CAS PubMed.
- Y. Hu, Y. Cao, C. Ma and W. Yan, Nano-biochar as a potential amendment for metal(loid) remediation: Implications for soil quality improvement and stress alleviation, J. Environ. Manage., 2024, 351, 119658 CrossRef CAS PubMed.
- K. Nishshankage, A. B. Fernandez, S. Pallewatta, P. K. C. Buddhinie and M. Vithanage, Current trends in antimicrobial activities of carbon nanostructures: potentiality and status of nanobiochar in comparison to carbon dots, Biochar, 2024, 6, 2 CrossRef.
- C. Xue, J. Wu, K. Wang, Y. Yi, Z. Fang, W. Cheng and J. Fang, Effects of different types of biochar on the properties and reactivity of nano zero-valent iron in soil remediation, Front. Environ. Sci. Eng., 2021, 15, 101 CrossRef CAS.
- S. Wang, M. Zhao, M. Zhou, Y. C. Li, J. Wang, B. Gao, S. Sato, K. Feng, W. Yin, A. D. Igalavithana, P. Oleszczuk, X. Wang and Y. S. Ok, Biochar-supported nZVI (nZVI/BC) for contaminant removal from soil and water: A critical review, J. Hazard. Mater., 2019, 373, 820–834 CrossRef CAS PubMed.
- X. Chen, X. Li, D. Xu, W. Yang and S. Bai, Application of nanoscale zero-valent iron in hexavalent chromium-contaminated soil: A review, Nanotechnol. Rev., 2020, 9, 736–750 CrossRef CAS.
- W. Qian, J.-Y. Liang, W.-X. Zhang, S.-T. Huang and Z.-H. Diao, A porous biochar supported nanoscale zero-valent iron material highly efficient for the simultaneous remediation of cadmium and lead contaminated soil, J. Environ. Sci., 2022, 113, 231–241 CrossRef CAS PubMed.
- S. Mandal, S. Pu, L. Shangguan, S. Liu, H. Ma, S. Adhikari and D. Hou, Synergistic construction of green tea biochar supported nZVI for immobilization of lead in soil: A mechanistic investigation, Environ. Int., 2020, 135, 105374 CrossRef CAS PubMed.
- S. Mandal, S. Pu, L. He, H. Ma and D. Hou, Biochar induced modification of graphene oxide & nZVI and its impact on immobilization of toxic copper in soil, Environ. Pollut., 2020, 259, 113851 CrossRef CAS PubMed.
- Y. Liu, J. Huang, H. Xu, Y. Zhang, T. Hu, W. Chen, H. Hu, J. Wu, Y. Li and G. Jiang, A magnetic macro-porous biochar sphere as vehicle for the activation and removal of heavy metals from contaminated agricultural soil, Chem. Eng. J., 2020, 390, 124638 CrossRef CAS.
- Z. Yang, Z. Fang, L. Zheng, W. Cheng, P. E. Tsang, J. Fang and D. Zhao, Remediation of lead contaminated soil by biochar-supported nano-hydroxyapatite, Ecotoxicol. Environ. Saf., 2016, 132, 224–230 CrossRef CAS PubMed.
- L. Lin, Z. Song, X. Liu, Z. H. Khan and W. Qiu, Arsenic volatilization in flooded paddy soil by the addition of Fe-Mn-modified biochar composites, Sci. Total Environ., 2019, 674, 327–335 CrossRef CAS PubMed.
- L. Lin, M. Gao, W. Qiu, D. Wang, Q. Huang and Z. Song, Reduced arsenic accumulation in indica rice (Oryza sativa L.) cultivar with ferromanganese oxide impregnated biochar composites amendments, Environ. Pollut., 2017, 231, 479–486 CrossRef CAS PubMed.
- J. Wu, Y. Yi, Z. Fang and E. P. Tsang, Effects of biochar on phytotoxicity and translocation of polybrominated diphenyl ethers in Ni/Fe bimetallic nanoparticle-treated soil, Environ. Sci. Pollut. Res., 2018, 25, 2570–2579 CrossRef CAS PubMed.
- G. Sigmund, C. Jiang, T. Hofmann and W. Chen, Environmental transformation of natural and engineered carbon nanoparticles and implications for the fate of organic contaminants, Environ. Sci.:Nano, 2018, 5, 2500–2518 RSC.
- Y. Zhang, Y. Zhang, O. U. Akakuru, X. Xu and A. Wu, Research progress and mechanism of nanomaterials-mediated in-situ remediation of cadmium-contaminated soil: A critical review, J. Environ. Sci., 2021, 104, 351–364 CrossRef CAS PubMed.
- M. E. A. El-sayed, Nanoadsorbents for water and wastewater remediation, Sci. Total Environ., 2020, 739, 139903 CrossRef CAS PubMed.
- J. Trujillo-Reyes, J. R. Peralta-Videa and J. L. Gardea-Torresdey, Supported and unsupported nanomaterials for water and soil remediation: Are they a useful solution for worldwide pollution?, J. Hazard. Mater., 2014, 280, 487–503 CrossRef CAS PubMed.
- S. Li, U. Turaga, B. Shrestha, T. A. Anderson, S. S. Ramkumar, M. J. Green, S. Das and J. E. Cañas-Carrell, Mobility of polyaromatic hydrocarbons (PAHs) in soil in the presence of carbon nanotubes, Ecotoxicol. Environ. Saf., 2013, 96, 168–174 CrossRef CAS PubMed.
- J. Qian, K. Li, P. Wang, C. Wang, M. Shen, J. Liu, X. Tian and B. Lu, Effects of carbon nanotubes on phosphorus adsorption behaviors on aquatic sediments, Ecotoxicol. Environ. Saf., 2017, 142, 230–236 CrossRef CAS PubMed.
- J. Dhiman, S. O. Prasher, E. ElSayed, R. M. Patel, C. Nzediegwu and A. Mawof, Effect of hydrogel based soil amendments on heavy metal uptake by spinach grown with wastewater irrigation, J. Cleaner Prod., 2021, 311, 127644 CrossRef CAS.
- H. Shaghaleh, Y. Alhaj Hamoud and Q. Sun, Functionalized nanocellulose nanocomposite hydrogels for soil and water pollution prevention, remediation, and monitoring: A critical review on fabrication, application properties, and potential mechanisms, J. Environ. Chem. Eng., 2024, 12, 111892 CrossRef CAS.
- H. Shaghaleh, Y. Alhaj Hamoud, Q. Sun, M. S. Sheteiwy and H. AbdElgawad, Soil flushing coupled with aminated-nanocellulose/MOF hydrogel nanocomposites adsorbents: A novel sustainable remediation strategy for Cr(VI)-contaminated agricultural soils, Sep. Purif. Technol., 2025, 353, 128440 CrossRef CAS.
- H. Lyu, H. Zhao, J. Tang, Y. Gong, Y. Huang, Q. Wu and B. Gao, Immobilization of hexavalent chromium in contaminated soils using biochar supported nanoscale iron sulfide composite, Chemosphere, 2018, 194, 360–369 CrossRef CAS PubMed.
- Y. Liu, Y. Tang, P. Wang and H. Zeng, Carbonaceous halloysite nanotubes for the stabilization of Co, Ni, Cu and Zn in river sediments, Environ. Sci.:Nano, 2019, 6, 2420–2428 RSC.
- M. Feizi and M. Jalali, Leaching of Cd, Cu, Ni and Zn in a sewage sludge-amended soil in presence of geo- and nano-materials, J. Cleaner Prod., 2021, 297, 126506 CrossRef CAS.
- X. X. Ye, G. Z. Wang, Y. X. Zhang and H. J. Zhao, Hydroxyapatite nanoparticles in root cells: Reducing the mobility and toxicity of Pb in rice, Environ.
Sci.:Nano, 2018, 5, 398–407 RSC.
- Z. Li, Y. Gong, D. Zhao, H. Deng, Z. Dang and Z. Lin, Field assessment of carboxymethyl cellulose bridged chlorapatite microparticles for immobilization of lead in soil: Effectiveness, long-term stability, and mechanism, Sci. Total Environ., 2021, 781, 146757 CrossRef CAS.
- X. Tao, A. Li and H. Yang, Immobilization of metals in contaminated soils using natural polymer-based stabilizers, Environ. Pollut., 2017, 222, 348–355 CrossRef CAS PubMed.
- H. Shaghaleh, Y. Alhaj Hamoud and Q. Sun, Effective and green in-situ remediation strategies based on TEMPO-nanocellulose/lignin/MIL-100(Fe) hydrogel nanocomposite adsorbent for lead and copper in agricultural soils, Environ. Pollut., 2024, 360, 124623 CrossRef CAS PubMed.
- Y. Li, Y. Li, N. Zhu, X. Liang, X. Bai, L. Zheng, J. Zhao, Y. F. Li, Z. Zhang and Y. Gao, Silica nanoparticles alleviate mercury toxicity: via immobilization and inactivation of Hg(ii) in soybean (Glycine max), Environ. Sci.:Nano, 2020, 7, 1807–1817 RSC.
- H. Wang, B. Kim and S. L. Wunder, Nanoparticle-supported lipid bilayers as an in situ remediation strategy for hydrophobic organic contaminants in soils, Environ. Sci. Technol., 2015, 49, 529–536 CrossRef PubMed.
- M. Lian, L. Wang, Q. Feng, L. Niu, Z. Zhao, P. Wang, C. Song, X. Li and Z. Zhang, Thiol-functionalized nano-silica for in-situ remediation of Pb, Cd, Cu contaminated soils and improving soil environment, Environ. Pollut., 2021, 280, 116879 CrossRef CAS PubMed.
- K. Qiu, L. Zhao, Y. An, X. Li and Z. Zhang, Stable and efficient immobilization of lead and cadmium in contaminated soil by mercapto iron functionalized nanosilica, Chem. Eng. J., 2021, 426, 128483 CrossRef CAS.
- J. Liu, J. Li, K. Wolfe, B. Perrotta and G. P. Cobb, Mobility of arsenic in the growth media of rice plants (Oryza sativa subsp. japonica. ‘Koshihikari’) with exposure to copper oxide nanoparticles in a life-cycle greenhouse study, Sci. Total Environ., 2021, 774, 145620 CrossRef CAS PubMed.
- X. Wang, W. Song, H. Qian, D. Zhang, X. Pan and G. M. Gadd, Stabilizing interaction of exopolymers with nano-Se and impact on mercury immobilization in soil and groundwater, Environ. Sci.:Nano, 2018, 5, 456–466 RSC.
- Z. Wang, J. Zhang, T. Wen, X. Liu, Y. Wang, H. Yang, J. Sun, J. Feng, S. Dong and J. Sun, Highly effective remediation of Pb(II) and Hg(II) contaminated wastewater and soil by flower-like magnetic MoS2 nanohybrid, Sci. Total Environ., 2020, 699, 134341 CrossRef CAS PubMed.
- J. Kim, S. Kim, I. Yoon, H. Yang and I. Kim, Novel two-step process for remediation of Cs-contaminated soil assisted by magnetic composites, Chem. Eng. J., 2021, 424, 130554 CrossRef CAS.
- W. Liu, Y. Li, Y. Feng, J. Qiao, H. Zhao, J. Xie, Y. Fang, S. Shen and S. Liang, The effectiveness of nanobiochar for reducing phytotoxicity and improving soil remediation in cadmium-contaminated soil, Sci. Rep., 2020, 10, 858 CrossRef CAS PubMed.
- M. V. Ghandali, S. Safarzadeh, R. Ghasemi-Fasaei and S. Zeinali, Heavy metals immobilization and bioavailability in multi-metal contaminated soil under ryegrass cultivation as affected by ZnO and MnO2 nanoparticle-modified biochar, Sci. Rep., 2024, 14, 10684 CrossRef CAS PubMed.
- Y. Zhang, J. Ma, J. Miao, L. Yue, M. Cheng, Y. Li and Z. Jing, Self-regulated immobilization behavior of multiple heavy metals via zeolitization towards a novel hydrothermal technology for soil remediation, Environ. Res., 2023, 216, 114726 CrossRef CAS PubMed.
- S. Mehmood, W. Ahmed, M. Rizwan, J. Bundschuh, A. S. M. Elnahal and W. Li, Green synthesized zinc oxide nanoparticles for removal of carbamazepine in water and soil systems, Sep. Purif. Technol., 2024, 334, 125988 CrossRef CAS.
- T. Lu, W. Wang, L. Liu, L. Wang, J. Hu, X. Li and G. Qiu, Remediation of cadmium-polluted weakly alkaline dryland soils using iron and manganese oxides for immobilized wheat uptake, J. Cleaner Prod., 2022, 365, 132794 CrossRef CAS.
- M. Mazarji, T. Minkina, S. Sushkova, S. Mandzhieva, G. N. Bidhendi, A. Barakhov and A. Bhatnagar, Effect of nanomaterials on remediation of polycyclic aromatic hydrocarbons-contaminated soils: A review, J. Environ. Manage., 2021, 284, 112023 CrossRef CAS PubMed.
- M. Usman, P. Faure, C. Ruby and K. Hanna, Remediation of PAH-contaminated soils by magnetite catalyzed Fenton-like oxidation, Appl. Catal., B, 2012, 117–118, 10–17 CrossRef CAS.
- F. Pardo, A. Santos and A. Romero, Fate of iron and polycyclic aromatic hydrocarbons during the remediation of a contaminated soil using iron-activated persulfate: A column study, Sci. Total Environ., 2016, 566–567, 480–488 CrossRef CAS PubMed.
- G. Barzegar, S. Jorfi, R. D. C. Soltani, M. Ahmadi, R. Saeedi, M. Abtahi, B. Ramavandi and Z. Baboli, Enhanced Sono-Fenton-Like Oxidation of PAH-Contaminated Soil Using Nano-Sized Magnetite as Catalyst: Optimization with Response Surface Methodology, Soil Sediment Contam., 2017, 26, 538–557 CrossRef CAS.
- M. Usman, K. Hanna and S. Haderlein, Fenton oxidation to remediate PAHs in contaminated soils: A critical review of major limitations and counter-strategies, Sci. Total Environ., 2016, 569–570, 179–190 CrossRef CAS PubMed.
- R. Bajagain and S. W. Jeong, Degradation of petroleum hydrocarbons in soil via advanced oxidation process using peroxymonosulfate activated by nanoscale zero-valent iron, Chemosphere, 2021, 270, 128627 CrossRef CAS PubMed.
- X. Yuan, T. Li, Y. He and N. Xue, Degradation of TBBPA by nZVI activated persulfate in soil systems, Chemosphere, 2021, 284, 131166 CrossRef CAS PubMed.
- C. Li, Y. Zhu, T. Zhang, Y. Nie, W. Shi and S. Ai, Iron nanoparticles supported on N-doped carbon foam with honeycomb microstructure: An efficient potassium peroxymonosulfate activator for the degradation of fluoranthene in water and soil, Chemosphere, 2022, 286, 131603 CrossRef CAS PubMed.
- D. Wu, H. Kan, Y. Zhang, T. Wang, G. Qu, P. Zhang, H. Jia and H. Sun, Pyrene contaminated soil remediation using microwave/magnetite activated persulfate oxidation, Chemosphere, 2021, 286, 131787 CrossRef PubMed.
- J. Wu, Y. Q. Yi, Y. Q. Li, Z. Fang and E. P. Tsang, Excellently reactive Ni/Fe bimetallic catalyst supported by biochar for the remediation of decabromodiphenyl contaminated soil: Reactivity, mechanism, pathways and reducing secondary risks, J. Hazard. Mater., 2016, 320, 341–349 CrossRef CAS PubMed.
- I. A. Abdelhafeez, J. Chen and X. Zhou, Scalable one-step template-free synthesis of ultralight edge-functionalized g-C3N4 nanosheets with enhanced visible light photocatalytic performance, Sep. Purif. Technol., 2020, 250, 117085 CrossRef CAS.
- A. Fujishima and K. Honda, Electrochemical Photolysis of Water at a Semiconductor Electrode, Nature, 1972, 238, 37–38 CrossRef CAS PubMed.
- A. n. Wang, Y. Teng, X. f. Hu, L. h. Wu, Y. j. Huang, Y. m. Luo and P. Christie, Diphenylarsinic acid contaminated soil remediation by titanium dioxide (P25) photocatalysis: Degradation pathway, optimization of operating parameters and effects of soil properties, Sci. Total Environ., 2016, 541, 348–355 CrossRef CAS PubMed.
- X. Zhao, X. Quan, S. Chen, H. m. Zhao and Y. Liu, Photocatalytic remediation of γ-hexachlorocyclohexane contaminated soils using TiO2 and montmorillonite composite photocatalyst, J. Environ. Sci., 2007, 19, 358–361 CrossRef CAS PubMed.
- M. Rani Rachna and U. Shanker, Degradation of tricyclic polyaromatic hydrocarbons in water, soil and river sediment with a novel TiO2 based heterogeneous nanocomposite, J. Environ.
Manage., 2019, 248, 109340 CrossRef CAS PubMed.
- U. Shanker, V. Jassal and M. Rani, Degradation of toxic PAHs in water and soil using potassium zinc hexacyanoferrate nanocubes, J. Environ. Manage., 2017, 204, 337–348 CrossRef CAS PubMed.
- I. A. Abdelhafeez, Q. Yao, C. Wang, Y. Su, X. Zhou and Y. Zhang, Green synthesis of ultrathin edge-activated foam-like carbon nitride nanosheets for enhanced photocatalytic performance under visible light irradiation, Sustainable Energy Fuels, 2019, 3, 1764–1775 RSC.
- Q. Hao, G. Jia, W. Wei, A. Vinu, Y. Wang, H. Arandiyan and B. J. Ni, Graphitic carbon nitride with different dimensionalities for energy and environmental applications, Nano Res., 2020, 13, 18–37 CrossRef CAS.
- D. Wang, N. B. Saleh, W. Sun, C. M. Park, C. Shen, N. Aich, W. J. G. M. Peijnenburg, W. Zhang, Y. Jin and C. Su, Next-generation multifunctional carbon-metal nanohybrids for energy and environmental applications, Environ. Sci. Technol., 2019, 53, 7265–7287 CrossRef CAS PubMed.
- W. J. Ong, L. L. Tan, Y. H. Ng, S. T. Yong and S. P. Chai, Graphitic Carbon Nitride (g-C3N4)-Based Photocatalysts for Artificial Photosynthesis and Environmental Remediation: Are We a Step Closer to Achieving Sustainability?, Chem. Rev., 2016, 116, 7159–7329 CrossRef CAS PubMed.
- Z. Luo, Y. Song, M. Wang, X. Zheng, L. Qu, J. Wang, X. Wu and Z. Wu, Comparison of g-C3N4 synthesized by different precursors in remediation of phenanthrene contaminated soil and ecotoxicity, J. Photochem. Photobiol., A, 2020, 389, 112241 CrossRef CAS.
- J. Wang, Z. Luo, Y. Song, X. Zheng, L. Qu, J. Qian, Y. Wu, X. Wu and Z. Wu, Remediation of phenanthrene contaminated soil by g-C3N4/Fe3O4 composites and its phytotoxicity evaluation, Chemosphere, 2019, 221, 554–562 CrossRef CAS PubMed.
- G. Yang, Y. Jiang, B. Yin, G. Liu, D. Ma, G. Zhang, G. Zhang, Y. Xin and Q. Chen, Efficiency and mechanism on photocatalytic degradation of fluoranthene in soil by Z-scheme g-C3N4/α-Fe2O3 photocatalyst under simulated sunlight, Environ. Sci. Pollut. Res., 2023, 30, 70260–70276 CrossRef CAS PubMed.
- G. Vasilyeva, V. Kondrashina, E. Strijakova and J. J. Ortega-Calvo, Adsorptive bioremediation of soil highly contaminated with crude oil, Sci. Total Environ., 2020, 706, 135739 CrossRef CAS PubMed.
- G. O. Adams, P. T. Fufeyin, S. E. Okoro and I. Ehinomen, Bioremediation, Biostimulation and Bioaugmention: A Review, Int. J. Environ. Biorem. Biodegrad., 2015, 3, 28–39 CAS.
- B. Kumari and D. P. Singh, A review on multifaceted application of nanoparticles in the field of bioremediation of petroleum hydrocarbons, Ecol. Eng., 2016, 97, 98–105 CrossRef.
- H. Horváthová, K. Lászlová and K. Dercová, Bioremediation vs. Nanoremediation: Degradation of Polychlorinated Biphenyls (PCBS) Using Integrated Remediation Approaches, Water, Air, Soil Pollut., 2019, 230, 204 CrossRef.
- B. Zhang, Y. Guo, J. Huo, H. Xie, C. Xu and S. Liang, Combining chemical oxidation and bioremediation for petroleum polluted soil remediation by BC-nZVI activated persulfate, Chem. Eng. J., 2020, 382, 123055 CrossRef CAS.
- I. A. Abdelhafeez, S. A. El-Tohamy and F. M. S. El-Dars, Enhanced phytoaccumulation dynamics of chromium and nickel from spent engine oil-contaminated soil amended with biomass-derived bulking agent, Sci. Radices, 2024, 3, 142–156 Search PubMed.
- L. Han, H. Gu, W. Lu, H. Li, W. x. Peng, N. L. Ma, S. S. Lam and C. Sonne, Progress in phytoremediation of chromium from the environment, Chemosphere, 2023, 344, 140307 CrossRef CAS PubMed.
- I. A. Abdelhafeez, S. A. El-tohamy, M. A. Abd and S. A. A. Abdel-raheem, A review on green remediation techniques for hydrocarbons and heavy metals contaminated soil, Curr. Chem. Lett., 2022, 11, 43–62 CrossRef.
- A. D. Zand and A. V. Heir, Phytoremediation: Data on effects of titanium dioxide nanoparticles on phytoremediation of antimony polluted soil, Data Brief, 2020, 31, 105959 CrossRef PubMed.
- X. Chen, J. Wang, K. Hayat, D. Zhang and P. Zhou, Small structures with big impact: Multi-walled carbon nanotubes enhanced remediation efficiency in hyperaccumulator Solanum nigrum L. under cadmium and arsenic stress, Chemosphere, 2021, 276, 130130 CrossRef CAS PubMed.
- T. Yao, L. Liu, S. Tan, H. Li, X. Liu, A. Zeng, L. Pan, X. Li, L. Bai, K. Liu and B. Xing, Can the multi-walled carbon nanotubes be used to alleviate the phytotoxicity of herbicides in soils?, Chemosphere, 2021, 283, 131304 CrossRef CAS PubMed.
- A. A. Romeh and R. A. Ibrahim Saber, Green nano-phytoremediation and solubility improving agents for the remediation of chlorfenapyr contaminated soil and water, J. Environ. Manage., 2020, 260, 110104 CrossRef CAS PubMed.
- E.-M. Meemken and M. Qaim, Organic Agriculture, Food Security, and the Environment, Annu. Rev. Resour. Economics, 2018, 10, 39–63 CrossRef.
- D. Tilman, C. Balzer, J. Hill and B. L. Befort, Global food demand and the sustainable intensification of agriculture, Proc. Natl. Acad. Sci. U. S. A., 2011, 108, 20260–20264 CrossRef CAS PubMed.
-
M. Weih, A. Westerbergh and P. O. Lundquist, Role of nutrient-efficient plants for improving crop yields: bridging plant ecology, physiology, and molecular biology, in Plant Macronutrient Use Efficiency: Molecular and Genomic Perspectives in Crop Plants, Elsevier, 2017, pp. 31–44 Search PubMed.
- W. M. Stewart, D. W. Dibb, A. E. Johnston and T. J. Smyth, The contribution of commercial fertilizer nutrients to food production, Agron. J., 2005, 97, 1–6 CrossRef.
-
V. C. Baligar and N. K. Fageria, Nutrient Use Efficiency in Plants: An Overview,in Nutrient Use Efficiency: From Basics to Advance, Springer India, 2015, pp. 1–14 Search PubMed.
- R. Prasad, A. Bhattacharyya and Q. D. Nguyen, Nanotechnology in sustainable agriculture: Recent developments, challenges, and perspectives, Front. Microbiol., 2017, 8, 1014 CrossRef PubMed.
- R. Raliya, V. Saharan, C. Dimkpa and P. Biswas, Nanofertilizer for Precision and Sustainable Agriculture: Current State and Future Perspectives, J. Agric. Food Chem., 2018, 66, 6487–6503 CrossRef CAS PubMed.
- R. Yaseen, A. I. S. Ahmed, A. Mohamed, M. K. M. Agha and T. M. Emam, Nano-fertilizers: Bio-fabrication, application and biosafety, Novel Res. Microbiol. J., 2020, 4, 884–900 CrossRef.
-
M. A. Iqbal, Nano-Fertilizers for Sustainable Crop Production under Changing Climate: A Global Perspective,Nano-Fertilizers for Sustainable Crop Production under Changing Climate: A Global Perspective, IntechOpen, 2016 Search PubMed.
- M. T. El-Saadony, A. S. ALmoshadak, M. E. Shafi, N. M. Albaqami, A. M. Saad, A. M. El-Tahan, E. S. M. Desoky, A. S. M. Elnahal, A. Almakas, T. A. Abd El-Mageed, A. E. Taha, A. S. Elrys and A. M. Helmy, Vital roles of sustainable nano-fertilizers in improving plant quality and quantity-an updated review, Saudi J. Biol. Sci., 2021, 28, 7349–7359 CrossRef CAS PubMed.
- D. M. Mahapatra, K. C. Satapathy and B. Panda, Biofertilizers and nanofertilizers for sustainable agriculture: Phycoprospects and challenges, Sci. Total Environ., 2022, 803, 149990 CrossRef CAS PubMed.
- G. Fellet, L. Pilotto, L. Marchiol and E. Braidot, Tools for nano-enabled agriculture: Fertilizers based on calcium phosphate, silicon, and chitosan nanostructures, Agronomy, 2021, 11, 1239 CrossRef CAS.
- M. A. Irshad, R. Nawaz, M. Zia ur Rehman, M. Imran, J. Ahmad, S. Ahmad, A. Inam, A. Razzaq, M. Rizwan and S. Ali, Synthesis and characterization of titanium dioxide nanoparticles by chemical and green methods and their antifungal activities against wheat rust, Chemosphere, 2020, 258, 127352 CrossRef CAS PubMed.
- S. Timmusk, G. Seisenbaeva and L. Behers, Titania (TiO2) nanoparticles enhance the performance of growth-promoting rhizobacteria, Sci. Rep., 2018, 8, 617 CrossRef PubMed.
- M. Javan, Y. Selahvarzi, P. Sayyad-Amin and S. Rastegar, Potential application of TiO2 nanoparticles to improve the nutritional quality of strawberry cv. Camarosa under drought stress, Sci. Hortic., 2024, 330, 113055 CrossRef CAS.
- F. Naseem, Y. Zhi, M. A. Farrukh, F. Hussain and Z. Yin, Mesoporous ZnAl2Si10O24 nanofertilizers enable high yield of Oryza sativa L., Sci. Rep., 2020, 10, 10841 CrossRef CAS PubMed.
- Y. Su, X. Zhou, H. Meng, T. Xia, H. Liu, P. Rolshausen, C. Roper, J. E. McLean, Y. Zhang, A. A. Keller and D. Jassby, Cost–benefit analysis of nanofertilizers and nanopesticides emphasizes the need to improve the efficiency of nanoformulations for widescale adoption, Nat. Food, 2022, 3, 1020–1030 CrossRef PubMed.
- Y. Heiba, M. G. Ibrahim, A. E. Mohamed, M. Fujii and M. Nasr, Developing smart sustainable irrigation matrix (SIM)-based model for selection of best irrigation techniques: A framework to achieve SDGs, J. Cleaner Prod., 2023, 420, 138404 CrossRef CAS.
- Y. Liu, Y. Zhou, T. Wang, J. Pan, B. Zhou, T. Muhammad, C. Zhou and Y. Li, Micro-nano bubble water oxygation: Synergistically improving irrigation water use efficiency, crop yield and quality, J. Cleaner Prod., 2019, 222, 835–843 CrossRef CAS.
- Z. Ouyang, J. Tian, X. Yan and Z. Yang, Micro-nano oxygenated irrigation improves the yield and quality of greenhouse cucumbers under-film drip irrigation, Sci. Rep., 2023, 13, 19453 CrossRef CAS PubMed.
-
O. I. Olaposi and A. I. Joshua, Development of nano irrigation system with clay diaphragm for reduced water loss in vegetable production in low income countries, Revista Espinhaço, 2017, 6, 21–28 Search PubMed.
- H. H. Edna Al-Issawi, A. A. W. I. Al-Abaied and I. M. Abdul Hameed, Evaluation of mobile trickle irrigation and sub-surface nanotechnology trickle irrigation by irrigating bean (cv. Veto), IOP Conf. Ser. Earth Environ. Sci., 2021, 779, 012016 CrossRef.
- T. L. Dirwai, T. Mabhaudhi, E. K. Kanda and A. Senzanje, Moistube irrigation technology development, adoption and future prospects: A systematic scoping review, Heliyon, 2021, 7, e06213 CrossRef CAS PubMed.
- E. K. Kanda, W. Niu, T. Mabhaudhi and A. Senzanje, Moistube Irrigation Technology: A Review, Agric. Res., 2020, 9, 139–147 CrossRef.
-
GET, Nano Irrigation, https://ghirass.innugate.com/green-technology/nano-irrigation/ Search PubMed.
- J. Ye, C. Wang, X. Chen, W. Kurexi, M. Huang, Z. Duan, R. Xu, Y. Li and Z. Zhang, Experimental and numerical investigations on soil–water dynamics in Moistube irrigation under dynamically regulated working pressure heads: scenario simulations under maize cultivation, J. Hydrol., 2024, 634, 131083 CrossRef.
- Y. Zhou, B. Zhou, F. Xu, T. Muhammad and Y. Li, Appropriate dissolved oxygen concentration and application stage of micro-nano bubble water oxygation in greenhouse crop plantation, Agric. Water Manag., 2019, 223, 105713 CrossRef.
- H. Lei, W. Wang, Y. Liang, Z. Xiao, H. Pan, L. Wang and M. Du, Effect of Nano-Bubble Irrigation on the Yield and Greenhouse Gas Warming Potential of Greenhouse Tomatoes, Agronomy, 2023, 13, 2917 CrossRef CAS.
- H. H. Sang, X. Y. Jiao, S. F. Wang, W. H. Guo, M. K. Salahou and K. H. Liu, Effects of micro-nano bubble aerated irrigation and nitrogen fertilizer level on tillering, nitrogen uptake and utilization of early rice, Plant, Soil Environ., 2018, 64, 297–302 CrossRef CAS.
- P. Khan, W. Zhu, F. Huang, W. Gao and N. A. Khan, Micro-nanobubble technology and water-related application, Water Sci. Technol.: Water Supply, 2020, 20, 2021–2035 CAS.
- Y. Zhang, Z. Wei, J. Xu, H. Wang, H. Zhu and H. Lv, Numerical simulation and application of micro-nano bubble releaser for irrigation, Mater. Express, 2021, 11, 1007–1015 CrossRef CAS.
- Y. Qian, X. Guan, C. Shao, C. Qiu, X. Chen, J. Chen and C. Peng, Effects of Different Concentrations of Micro-Nano Bubbles on Grain Yield and Nitrogen Absorption and Utilization of Double Cropping Rice in South China, Agronomy, 2022, 12, 2196 CrossRef CAS.
- E. J. Yafuso and P. R. Fisher, Oxygenation of irrigation water during propagation and container production of bedding plants, Hortscience, 2017, 52, 1608–1614 CAS.
- Y. Zhou, F. Bastida, B. Zhou, Y. Sun, T. Gu, S. Li and Y. Li, Soil fertility and crop production are fostered by micro-nano bubble irrigation with associated changes in soil bacterial community, Soil Biol. Biochem., 2020, 141, 107663 CrossRef CAS.
- J. Wang, Q. He, K. Cao, B. Zhou, X. Niu, D. Wang, R. Chen and Z. Zheng, Micro-nano bubble water with potassium fertigation improves strawberry yield and quality by changing soil bacterial community, Rhizosphere, 2023, 28, 100783 CrossRef.
- P. Khan, H. Wang, W. Gao, F. Huang, N. A. Khan and N. Shakoor, Effects of micro-nano bubble with CO2 treated water on the growth of Amaranth green (Amaranthus viridis), Environ. Sci. Pollut. Res., 2022, 29, 72033–72044 CrossRef PubMed.
- H. Li, P. Li, J. Li, Y. Jiang and X. Huang, Influence of micro/nano aeration on the diversity of the microbial community in drip irrigation to reduce emitter clogging, Biosyst. Eng., 2023, 235, 116–130 CrossRef CAS.
- M. Kalwani, H. Chakdar, A. Srivastava, S. Pabbi and P. Shukla, Effects of nanofertilizers on soil and plant-associated microbial communities: Emerging trends and perspectives, Chemosphere, 2022, 287, 132107 CrossRef CAS PubMed.
- A. A. Yaqoob, H. Ahmad, T. Parveen, A. Ahmad, M. Oves, I. M. I. Ismail, H. A. Qari, K. Umar and M. N. Mohamad Ibrahim, Recent Advances in Metal Decorated Nanomaterials and Their Various Biological Applications: A Review, Front. Chem., 2020, 8, 341 CrossRef CAS PubMed.
-
S. Sachdev and S. Ahmad, Role of Nanomaterials in Regulating Oxidative Stress in Plants, in Nanobiotechnology, Springer International Publishing, 2021, pp. 305–326 Search PubMed.
- A. A. Dayem, M. K. Hossain, S. Bin Lee, K. Kim, S. K. Saha, G. M. Yang, H. Y. Choi and S. G. Cho, The role of reactive oxygen species (ROS) in the biological activities of metallic nanoparticles, Int. J. Mol. Sci., 2017, 18, 120 CrossRef PubMed.
- Y. Liu, S. Zhu, Z. Gu, C. Chen and Y. Zhao, Toxicity of manufactured nanomaterials, Particuology, 2022, 69, 31–48 CrossRef CAS.
- V. L. Reddy Pullagurala, I. O. Adisa, S. Rawat, B. Kim, A. C. Barrios, I. A. Medina-Velo, J. A. Hernandez-Viezcas, J. R. Peralta-Videa and J. L. Gardea-Torresdey, Finding the conditions for the beneficial use of ZnO nanoparticles towards plants-A review, Environ. Pollut., 2018, 241, 1175–1181 CrossRef CAS PubMed.
- P. Bhatt, S. C. Pandey, S. Joshi, P. Chaudhary, V. M. Pathak, Y. Huang, X. Wu, Z. Zhou and S. Chen, Nanobioremediation: A sustainable approach for the removal of toxic pollutants from the environment, J. Hazard. Mater., 2022, 427, 128033 CrossRef CAS PubMed.
- I. Cecchin, K. R. Reddy, A. Thomé, E. F. Tessaro and F. Schnaid, Nanobioremediation: Integration of nanoparticles and bioremediation for sustainable remediation of chlorinated organic contaminants in soils, Int. Biodeterior. Biodegrad., 2017, 119, 419–428 CrossRef CAS.
- E. K. Kanda, W. Niu, T. Mabhaudhi and A. Senzanje, Moistube Irrigation Technology: A Review, Agric. Res., 2020, 9, 139–147 CrossRef.
- T. L. Dirwai, T. Mabhaudhi, E. K. Kanda and A. Senzanje, Moistube irrigation technology development, adoption and future prospects: A systematic scoping review, Heliyon, 2021, 7, e06213 CrossRef CAS PubMed.
- B. Salieri, D. A. Turner, B. Nowack and R. Hischier, Life cycle assessment of manufactured nanomaterials: Where are we?, NanoImpact, 2018, 10, 108–120 CrossRef.
- L. Pourzahedi, M. Pandorf, D. Ravikumar, J. B. Zimmerman, T. P. Seager, T. L. Theis, P. Westerhoff, L. M. Gilbertson and G. V. Lowry, Life cycle considerations of nano-enabled agrochemicals: Are today's tools up to the task?, Environ. Sci.:Nano, 2018, 5, 1057–1069 RSC.
- N. U. M. Nizam, M. M. Hanafiah and K. S. Woon, A content review of life cycle assessment of nanomaterials: Current practices, challenges, and future prospects, Nanomaterials, 2021, 11, 3324 CrossRef CAS PubMed.
- M. Javaid, A. Haleem, I. H. Khan and R. Suman, Understanding the potential applications of Artificial Intelligence in Agriculture Sector, Adv. Agrochem, 2023, 2, 15–30 CrossRef.
- P. Deng, Y. Gao, L. Mu, X. Hu, F. Yu, Y. Jia, Z. Wang and B. Xing, Development potential of nanoenabled agriculture projected using machine learning, Proc. Natl. Acad. Sci. U. S. A., 2023, 120, e2301885120 CrossRef CAS PubMed.
- J. J. Scott-Fordsmand and M. J. B. Amorim, Using Machine Learning to make nanomaterials sustainable, Sci. Total Environ., 2023, 859, 160303 CrossRef CAS PubMed.
- N. Xu, J. Kang, Y. Ye, Q. Zhang, M. Ke, Y. Wang, Z. Zhang, T. Lu, W. J. G. M. Peijnenburg, J. Penuelas, G. Bao and H. Qian, Machine learning predicts ecological risks of nanoparticles to soil microbial communities, Environ. Pollut., 2022, 307, 119528 CrossRef CAS PubMed.
|
This journal is © The Royal Society of Chemistry 2025 |
Click here to see how this site uses Cookies. View our privacy policy here.