DOI:
10.1039/D3GC05187K
(Perspective)
Green Chem., 2025,
27, 403-412
Green chemistry startups: some lessons learned
Received
29th December 2023
, Accepted 4th November 2024
First published on 12th November 2024
Abstract
There has been an explosive growth in environmentally driven startups in recent years. Much of this has included university spin-outs aiming to translate academic research into commercial practice. This activity dovetails with the principle aim of green chemistry research – improving the sustainability of the chemical and materials industries. However, academics are not always fully aware of the activities, needs, timelines and considerations of startup companies and how these differ from academic research. This can lead to a misunderstanding of whether, when or how to start a cleantech company and what to do once one is formed. Through a careful consideration of the founders, their motivations, the industry, scale and market forces behind an innovation, a business roadmap can be drawn which will help determine whether the technology is appropriate for deployment in the commercial sector. Considerations such as scale-up, cost, capital fundraising needs and teambuilding must all come together as part of the translation journey. Based on lessons learned through my group forming 9 cleantech startups since 2016, I have tried to blend facts, perspective and anecdotes to demonstrate how the green chemistry translation can help us achieve the ultimate goals of the field: sustainable chemical production and a fully decarbonized chemical industry.
Introduction
By its very nature, the aims of green chemistry and sustainability research lend themselves well to technology transfer and commercialization. The primary goal of sustainability research and, one suspects, of Anastas and Warner in 1998,1 is to effect a positive change on how we impact the environment. A high level of impact derived from green chemistry can only be achieved if the primary research is eventually exploited. Exploitation of chemical technologies is currently the province of the commercial chemical industry, who are therefore also responsible for the environmental damage these technologies either cause or prevent. As such, it is impossible to realise a meaningful positive impact from a chemical technology on the environment without engaging with the chemical industry in a very direct manner. The petrochemical industry is responsible for 6% of global CO2 emissions, making it the third largest industrial emitter, trailing only iron/steel and cement/concrete production.2 The wider chemical industry is also considered the largest source of (non-CO2) industrial air pollution.3 Combining air, water and land pollution, the petrochemical industry is considered the world's most polluting industry (and the intricately related textile industry is second).4 This yields clear targets for green chemistry and sustainable engineering research: develop new technologies for sustainable chemical and material production. These goals are underpinned by a recent global focus on decarbonization and net-zero production, including within the chemical industry.5 However, the practice of sustainability, based firmly on the principles of green chemistry, can only lead us to a greener future if academics engage with industry on their turf: the commercial sector. But how does one translate academic research into commercial industrial practice?
Translating academic research
Routes to commercialization
There are many different routes into the commercial sector for an academic research technology (I am not qualified to comment on best practice in industrial R&D). While many of us do not think much beyond the research article or possible patent, a patent is the beginning of the exploitation journey, not the end. The easiest route to commercial translation is by direct licencing of a technology to an existing commercial partner, hopefully one capable of exploiting the technology directly. This puts the onus of further development squarely on the commercial partner, but if this is a large, established company then likely they have a wealth of experience in commercializing new chemical technologies. Unfortunately, this route is amongst the most difficult for an academic to instantly traverse, as primary, fundamental chemical research rarely develops a technology sufficiently for an existing partner to evaluate its potential for scale-up and deployment. To address this issue, a specialised vehicle (startup company) is often created to raise the inherent readiness of the technology through further research and development not suited for an academic environment. Such startup companies come in many varieties, but the two primary ones are a startup without intellectual property (IP) and one with protected IP (normally a patent). The latter, when coming out of a university, is normally referred to as a university spin-out company. Whether protected IP exists or not, the activities of tech startups have common objectives regardless of the underlying technology.
Typical startup company activities
Before embarking on my first startup journey, I envisioned that a cleantech (green chemistry or renewable energy or similar) startup was sort of like an academic research group – researchers working in the lab, writing proposals for fundraising and speaking at conferences. This image was quickly dispelled. While research and development is an important activity in a startup, it is neither the most important task, nor the task to which the most important individuals dedicate their time. It is said that the purpose of a startup company is to discover a viable business plan.6 There are a plethora of activities required to develop a business plan, and R&D is quite low on this list, at least initially. The earlier activities are mostly centred around understanding the industry (market research) and the business (customer discovery). One of the most common questions asked of a startup company is “who are you going to sell to?” Customer discovery and understanding market dynamics – who buys what and what they want, is extremely important early in a company's lifespan. There is an adage in business that new technologies need to satisfy either “pain or gain” criteria7 – either take away a customer's “pain” by solving one of their problems or offer them “gain” in the form of new products (more market share) or higher margins. In my experience, the conservative nature of business tends to lead to “pain” being valued much higher than potential “gain”. This is a golden opportunity for green chemistry, as one of the largest current sources of commercial “pain” in the chemical industry is the sustainability of products – including carbon footprint, water pollution and more recently the use of renewables or waste in place of fossil feedstocks.8 While this presents an opportunity to solve emerging problems, the disruptive nature of new technologies tends to work against their adoption. The main reason industry seems to downplay “gain” is the high risk of a new technology failing to deliver. This tends to lead to the adoption of more conservative options, such as drop-in replacements, over new products. As such, a low disruption “disruptive” new technology wins the race to market. It is imperative to pay attention to these forces – potential economic success alone is not enough to overcome the tyranny of “steel in the ground” – fully depreciated commercial assets that can safely deliver and can often drop price points below the profit line to drive out competition (and for good reason – these plants are by definition sunk costs). It is clearly best to have a disruptive technology, so long as it isn't too disruptive.
As an example of different approaches to replacing existing products, biobased plastics offers many examples. Origin Materials is a US technology company that produced bio-derived polyethylene terephthalate (PET) as a direct replacement of fossil PET. The key technology is based on the production of 5-chloromethylfurfural from biomass9 and subsequent conversion to 1,4-xylene and then use of the traditional process to convert this feedstock to polyester. This “drop-in” replacement plastic offers the same plastic as today's bottles and textile fibres but with enhanced sustainability credentials. Market pull has been tremendous, with the company reporting a market capitalization in excess of $1.5 billion and pre-orders exceeding their first plant's capacity in 2023.10 Meanwhile, Avantium has pioneered production of the new plastic polyethylene furoate (PEF) from sugar.11 This offers a 100% biobased alternative to PET with superior physical properties in key areas for packaging such as gas transport resistance.12 However, adoption has been slower and setbacks with scale-up partners13 have led to a higher priced option which has not yet reached full scale. In both cases, a biobased alternative to a petrochemical product is offered, with superior sustainability credentials, similar or enhanced recyclability and performance but at a higher price. The direct replacement has led on early market demand, presumably as the more familiar product offering is easier for customers to accept.
Only after there is a clear indication of who the customer is and what they will buy (and preferably what they would pay and for how much volume) does the more intensive development work begin. It can be an irresistible temptation to try and scale up too quickly – until there is sufficient demand for sample testing of an end product, there is very little point in producing more than small laboratory samples. The larger scale production (pilot, demonstration scale) ideally serves a specific purpose beyond engineering practice. For example, some process aspects (solvent recycling, especially of a novel solvent) are close to impossible to achieve in a laboratory. One of the first research projects we undertook to demonstrate the commercial potential of ionoSolv biomass fractionation was solvent recycling.14 In that publication, we recycled the solvent 4 times and later 6 times at laboratory scale.15 These endeavours took 4–6 months of time in the laboratory to control. Given that the pilot scale ionoSolv fractionation time is ca. 20 minutes, those 6 months equated to 2 hours of plant operation, or 25% of one worker shift. The pilot plant of our startup Lixea16 was built primarily to demonstrate that the ionic liquid could be recycled for at least a year. At our previous rate of laboratory work, this would have required 2000 years of student effort (or 500 entire PhD projects). This was a very good reason to build a larger pilot plant; exploring the effects of scale-up on product quality were decidedly secondary. Other good reasons to scale-up are to provide larger (multi-kg) samples to customers for pilot testing and to determine the options for key pieces of process equipment (i.e. filter and evaporator design) or to determine what impurities may build up in a solvent over long-term recycling. Running a pilot plant is extremely expensive; as such it needs convincing justification beyond increasing the technology readiness level (TRL).17
Tracking progress with TRLs
At some point, the development of a new scientific idea will cease being an academic research project and become a commercial project. The investment community generally has relied on the TRL scale to act as guidance, with the move above TRL4 (laboratory validation) acting as a natural move out of the academic domain. The main aim of early-stage investment is to help bridge the so-called valley death between TRL4-6.18 This is the key de-risking stage for a new technology, as it has been proven in the lab already but is not yet in a position to be deployed at any relevant (pilot) scale. While some startups tend to overstate their TRL, the cut-off at TRL4 is quite a useful indicator – any new technology can only be developed to TRL4 in the laboratory where it was invented, as achieving TRL5 requires validation in an external facility.17 Hence, this represents an ideal point for a startup company to form, as the academic work has mostly finished.
Scaling a technology is a seemingly attractive means of proving that “it works at scale”; however, simply running a larger volume version of a laboratory experiment is not useful as a commercial scaling exercise. Scale-up is normally associated with key advances in the technology, and usually linked to the business development rather than the technical development. As an example, my spin-out company Bioataraxis manufactures biobased detergents.19 Key questions of scale did not arise when establishing that the synthesis can be performed at larger scale (those engineering questions are solved) but rather to create commercially relevant samples. For example, switching from lab-scale sulfonation with chlorosulfonic acid to a commercial falling film SO3 reactor requires 200 kg hr−1 of material to be produced. This enabled us to produce surfactants for customer testing with the likely commercial impurities inside the formulation – the lab-scale product is unreasonably pure for testing. These sorts of commercial-technical considerations are far more important to development than the raw amounts produced. In fact, our 200 kg hr−1 “pilot run” would be enough to produce the annual supply of COVID-19 vaccine in around 6 hours.20 Ultimately, having a grasp of your core technology and its actual stage of development is a better indicator of “readiness” than somewhat arbitrary indicators of scale, and running larger volumes to prove “scale-up” often serves little useful purpose in either the development of the technology or the business. Understanding the scale of an industry is vital to understanding what does and does not need to be demonstrated by a startup company.
The purpose of startup companies
There are lots of reasons why people start cleantech companies.21,22 Academics often state a desire to see their research reach the commercial space, validating the time and effort they dedicated to the science. Scale of potential solutions are of utmost importance in business, as certain products (i.e. fuels, commodity chemicals) are only traded or sold at massive scale. New technologies often come at higher cost and meeting the cost point of a commodity chemical or fuel is challenging. While economies of scale do provide some relief (provided one scales up rather than scaling out), unfortunately, the scales required for different technologies often are dictated by market demands. Commodity chemicals and materials usually cannot be produced under commercially relevant process conditions without advance to a pilot plant (TRL7), which may cost tens of millions of dollars to build. This creates an enormous roadblock in development, as relevant customer testing cannot proceed during the TRL4-6 valley of death. While this has often led startups to pivot to “higher value products”, the switch to higher value, lower volume targets can be self-defeating – for example, if the initial aim was to combat climate change (40 GTpa CO2e) there are only a small number of commercial activities (cement and steel manufacture and primary petroleum refining, fertilizer production) or products (transport fuels and heat and particularly electricity, some bulk chemicals such as plastics, ammonia or methanol; one can also substitute hydrogen to represent the latter two – see Fig. 1) which are capable of achieving any meaningful impact. Furthermore, the high value products often create extreme scale mismatches. For example, even if one could make 1 ton of a high-value product from waste plastic, it would not impact the 400
000
000 tons of plastic waste generated annually;23 conversely, making one ton of plastic from any source is an irrelevant volume in the industry regardless of how green that ton turns out. I also once had a grand plan for making 20 million litres of nearly zero-cost bioethanol from wastewood24 only to be informed by a commercial partner that this volume was too insignificant to even be traded. This is especially true with co-products, as selling two products at vastly different scales produced from the same feedstock is logistically challenging. Petrochemical refineries have built up product portfolios over more than a century; to duplicate this achievement from other sources will take decades. For the time being, it is important to ensure that feedstock supply and product demand match up, as failure to do so will render the business aspects of the technology impossible to translate.
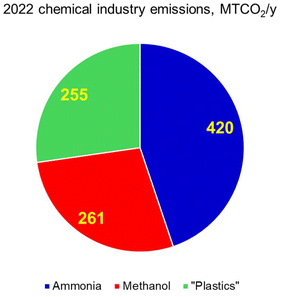 |
| Fig. 1 Breakdown of CO2 emissions for the global chemical industry sector, 2022. “Plastics” is the sum of ethylene, propylene, benzene, toluene and mixed xylenes, which are predominantly used to create polymers. Reproduced from ref. 5 with permission from The International Energy Agency, copyright 2023. | |
Key ingredients in a startup company
There is nothing more deterministic of the success of a startup than the team. Not only are the roles and responsibilities concentrated, but investors invest in people first, not technology. The CEO of any startup needs to devote their full attention to the success of the company – it does not make for a good moonlighting job. Choosing the right steward for the company is paramount – someone who understands the technology and is capable of bringing it to market while building a sustainable team throughout the business. When my first startup had only the 3 founders working for it (and only one full time), the same person was in charge of fundraising (CEO), technology development (CTO), finances (COO) filing taxes, handling payroll (to make sure she got paid) and more. This multitasking certainly lends itself to what in my opinion is the most valuable output of a startup – a highly skilled, highly motivated founder CEO.25
Once the team is secure, securing funding becomes the next challenge. Fundraising for startups (grants and investment) requires a balance of development in the team, business and market forces beyond the technology. While the technology is supposed to drive the innovation, it is the business plan that determines the success or failure of a tech startup. Recent research at KTH has acknowledged this growing awareness, substituting a set of “innovation readiness levels”26 or IRLs to supplement the TRLs, formally acknowledging the technology as only one ingredient in a tech startup. The innovation readiness categories they identify are: technology, business model, intellectual property rights, team, funding and customer. This more holistic view highlights that technology readiness is not the only aspect of a startup business. The investment part of this exercise focusses on how to raise money – the strategic importance of different types of financing. Grants are attractive as they are non-dilutive but bring little business value beyond some partnership building. Angels (especially green angels) are vital sources of very early stage investment in cleantech, and often work closely with the team and even sometimes the technology itself. Venture capital, especially from corporate sources, can provide a stable backdrop to company financing. Making choices about where to seek investment is one of the hardest aspects of early stage company life, and dominates the more rewarding activity of improving the performance of the technology.
One final aspect of setting up the tech startup business model is determining the optimum route to exit. This is essential as it is intricately linked to the deployment of the technology after development. There are many different options, but the main ones are licencing, trade sale (exit) or initial public offering (IPO, stock market listing). The distribution between these in the cleantech sector is shown in Fig. 2. Licencing is a common mechanism of technology transfer in the chemical industry, where manufacturers licences key pieces of technology to use in their process. It is relatively uncommon for entire processes to be licenced, unless a dedicated vehicle (such as a joint venture) between the startup and customer/partner is employed. However, this option does lend a more strategic focus to the technology development, as the target customers, scale-up partners and product offerings are identified from the start. This route is therefore best chosen when the route to market can be eased through the partner company and especially for larger scale products (commodities or bulk chemicals) which have complicated distribution chains. A more common route for early-stage startups is to envision a trade sale (where a large company acquires a smaller one). This can lead to a lot of tension and nervousness amongst the founding team, trying to determine the right time to sell the company. The acquiring company will take over the development, which is ideal for complex, multi-stage manufacturing processes, large scale production or systems with complex supply chains. An IPO (publicly traded company) stage is usually the largest exit available. However, this would also require the longest development time. An IPO results in a fully independent chemical company, which is usually only accessible for startups with small scale or no manufacturing (specialty chemicals or service products) where large profit margins are anticipated. While typical early-stage investors anticipate a founder exit in 3–5 years (which only the trade sale can accomplish) the other routes can take decades to reach fruition. Unfortunately, chemistry, as a manufacturing exercise, is not as rapid a business prospect as more electronic platforms; a new sustainable chemical process does not develop as rapidly as a new social media platform. This can test the patience of investors and founders alike.
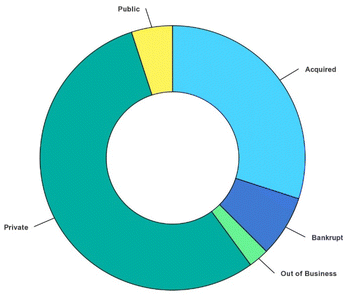 |
| Fig. 2 Status of 40 cleantech companies tracked from 2010–2021. Reproduced from ref. 27 with permission from The International Energy Agency, copyright 2021. | |
Where does green chemistry fit in?
The link between green chemistry and entrepreneurship is very strong. One of the quickest means of realising impact from research is through commercial adoption of the developed technology. One of the primary aims of green chemistry is to reduce the negative impacts of manufacturing on the environment, and it is clear that such impacts can only be realised through large-scale commercial practice. This is also reflected in the translation space, as the rise of cleantech entrepreneurship in the past decade has been driven by an increased focus by the commercial sector on the need to combat climate change and pollution. There is currently a strong push to decarbonize the chemical industry. In a recent report McKinsey28 reveal how the chemical industry is being transformed by sustainability efforts. While many chemical end users (customers) have committed to reducing greenhouse gas emissions, these actions may not be enough to shift the industry away from fossil or high-emission chemicals, in part due to an initial focus on scope 1 and scope 2 emissions only. For example, brand owners in the fashion industry often want recycled materials in their products but the supply chain for such feedstocks remains underdeveloped. However, McKinsey do note that companies can take advantage of the shift to sustainability by selling materials and solutions to end markets that are prioritizing sustainability and that companies can “greenify” their products and sell them at a premium to markets that value sustainable solutions. Some of this underlying trend is consumer driven. There have been many studies on the so-called “green premium” to identify whether consumers will pay more for goods that are considered more environmentally friendly. Recent consumer surveys29 indicate that 82% of consumers claim to be willing to pay more for products with green packaging, including 88% of consumers under 45. This sort of trend would have been unthinkable in past decades, but growing awareness of climate change and the environmental impacts of plastic waste are driving rapid shifts in consumer outlook. This trend is no doubt present in many sectors today. This shift in consumer attitude is being reflected both in industry (in particular through fast moving consumer goods, packaging and other materials that are directly bought by consumers) and investors. Trends in investment suggest that the share of venture capital investment in cleantech companies tripled between 2000 and a 2010 bubble30 and has been on the rise again since 2015.31 While these trends can be fickle, the underlying consumer, corporate and investor push towards more sustainable, green technologies is clear. In light of the decarbonization pledges made by many countries after COP-26 and the follow-on, aggressive policy pushes that have backed up these pledges, it is increasingly clear that consumer pushes and evolving government policy will make future reliance on green subsidies unnecessary as the market will soon only support products that can clearly demonstrate sustainable credentials.
It should be noted that some sustainability improvements will come from innovations in logistics, e.g. more personalised waste collection, but the role of green chemistry in developing new technologies that improve the products themselves is prevalent. Society will eventually need to reconcile its dependence on existing, familiar items (almost entirely derived from fossil fuels) and transition to more sustainable feedstocks and resources. Whether this transitions through mimicry (bio-derived direct replacements, e.g. polyethylene derived from bioethanol) or eventually leads to new products (replacing function with new materials), the role of green chemistry in entrepreneurship, technology translation and the future of manufacturing will be front and centre.
Startups frequently fail
It is a well-accepted statistic that 90% of startups fail.32 A recent IEA report indicated that 81% of clean energy startups who completed seed funding failed to reach the growth phase.27 This is attributed to a number of factors, such as time to market, policy shifts, perceived high risk and failure to deliver on (often exaggerated) promises by the company. It is noteworthy that 80% of those companies failed to meet their investors’ expectations, which likely correlates with this failure rate. The success rate was not even across the sector, with very high success rates in energy storage and energy efficiency startups higher than solar, wind and bioenergy.27 While this is certainly attributable to many factors beyond the sector or technology (the cohort sizes varied greatly between these areas), it is the type of information investors rely on when choosing where to place their bets and indicates what preconceptions a startup may need to overcome during fundraising. As a rule, technologies in more established markets tend to have a harder time raising money but a much higher rate of survival. The trends toward sustainability in industry are certainly providing a boost to investment in this space, and green chemistry is becoming more prevalent in startup companies. Investors are gaining more confidence with the approach and outlook of green chemistry, policy actions continue promote these principles,32 de-risking these investments, and market drivers and consumer pressure are bringing sustainable products to the forefront of innovation. On the other hand, companies and investors that are more “myopic” in their continued investment in fossil-based products are clearly facing a long-term threat to their viability.33 The intersection of government and corporate policy in this area is reflected in the corporate sustainability strategy for carbon neutrality recommended by ISO 14068:202334 which requires a commitment from top management to provide the necessary resources and commit to a company strategy and business model aligned with its carbon neutrality pledges to ensure effective delivery. This approach necessitates defining the timeline, system boundaries, baseline, targets and methodology for assessment and then assigning the responsibilities, financial and human resources required to commit to delivery.35 These trends all suggest a very bright future for the translation of academic research in the sustainability space into commercial practice, provided we observe not only the principles of green chemistry, but also the economic viability and overall practicality of our inventions.
Despite its clear sustainability credentials,36 cellulosic ethanol has had many false starts due to changing market dynamics, particularly centring on policy uncertainty. Recently, Clariant closed their 50 ktpa bioethanol plant in Romania after roughly one year of production.37 The company cited struggles with an undersized feedstock processing unit and issues with enzyme production and wastewater as key reasons for lower production than anticipated.
The question of market timing extends to other products as well. Renewcell launched a process for recycling cotton textiles in 2023.38 The process is highly efficient and met every technical target during a rapid scale-up process, establishing a potentially transformative recycling process with a 120 ktpa recycling plant. Unfortunately, the company filed for bankruptcy after less than a year of operation, not because of failure to meet production or technical development targets but they cited sluggish customer orders, despite an apparent market pull for recycled fibres. A misunderstanding of the supply chain and product verification between technology stages appears to have been the root cause of failure.39
A great research idea is not enough
There is a significant difference between a great research idea and a great business idea – in my own research, the two have rarely aligned. Most of the makings of a translatable research programme lie in the end product itself – whether a process or a product or a technology – and how much market pull it is experiencing. Too often great scientific advances result in a “research push” which could at best translate to a market push; this is a much more challenging road to commercialization compared to a technology or process or (especially) product that the market already wants – a market pull. Henry Ford's (possibly apocryphal40) quote “if I had asked people what they wanted, they would have said faster horses” might apply to the development of new software applications and even new consumer products, but is less appropriate for the bulk chemical industry, where the product selection has long been fixed. In order to translate a chemical process technology into practice it is imperative that the process delivers something that the market already wants to buy. To determine this, market research is illustrative and can help shape the desired product, as discussed above. After establishing the product offering of choice, a business plan needs to be developed, which will illustrate what steps will be taken in what order (with timings and costs) and this ties heavily into fundraising.41 Underpinning the fundraising process it can be useful to have some familiar staples of sustainable chemistry – technoeconomic analysis and life cycle assessment. These have different aims and are useful in different parts of the fundraising community. Technoeconomic analysis, long a cornerstone of systems engineering,42 is an excellent tool for assessing the potential economic viability of a proposed process concept, estimate price points and determine the amount of capital required at various stages of technology demonstration. This not only gives investors comfort that the team has a pathway to a profitable return (or if one is even possible), but also ensures that the business capital needs are laid out at an early stage. While the economic aspects of a technology are important at any stage, they are particularly important when projecting out to commercial scale operation. Most products are not expected to be economical below TRL7 (as there is no process in place) but demonstration of economic viability is essential as progress is made during progression to TRL7 – pilot operation, where products are expected to be representative but potentially saleable to early adopters at a premium – and TRL8 – demonstration scale, where the price point is often set at the “break even” point. At TRL8 the product and process are both expected to be representative of commercial production, and the price set near market value so large-scale order can be met without operating at a significant loss. At the final, commercial scale (TRL9), the market price is expected to turn a profit with margins commensurate to the size of production (i.e. low for commodities through to high for specialty products). Meanwhile, a life cycle assessment (LCA) underpins more advanced green chemistry thinking but has only recently begun to turn up in investor due diligence procedures. An LCA is essential for a business whose primary market drive is sustainability, though recently carbon footprints seem to dominate the commercial space.43 Unfortunately, the drive to back up sustainability claims with LCA has led to instances of startups making unrealistic claims (or even publishing on their websites LCA studies which violate multiple laws of thermodynamics). This may erode trust in the procedure unless the LCA is backed up by a validated academic expert or an established commercial partner – many large companies have internal LCA heuristics which provide excellent benchmarking, and as the academic space evolves in this regard the opportunities for hiring consultants to aid in assessing the sustainability features and limitations of nascent technologies are increasing rapidly. Regardless, the use of impact assessment and establishment of sustainability credentials, particularly regarding carbon intensity, are clearly a topic of increasing need for industry. Recent policy shifts, such as the EU's Safe and Sustainable by Design (SSdB)44 will only deepen this requirement in the future. SSdB is anticipated to have a much earlier and more positive impact than the EU's current REACH45 approach, which can take more than 20 years to phase out dangerous chemicals.46 As policy and public awareness of sustainability grows, more intricate sustainability and impact assessments will become requirements. This should encourage new processes and products and push the chemical industry to quantify resource consumption and emissions.47 The overall effect should be a more sustainable chemical industry and a better understanding of diverse aspects of impact on our planet, the topic of recent and exciting efforts to quantify planetary boundaries.48
Timing is everything
Market forces are not static. What looks to be an attractive investment today might not be so one year from now and vice versa. Shifting market forces can be attributed to macro-level economic forces, such as shifts in policy or technology, but also transient fluctuations, such as consumer attitude and priorities including awareness of climate change. The most obvious example of these transient forces in the price of energy. Our entire energy and chemical sectors are linked indelibly to the current trading price of natural gas and petroleum. Even bioresources (food and first-generation chemical feedstocks) feel this directly as ammonia-based, and therefore natural gas derived, fertilizer is one of the main costs of commercial crop production. This translates directly to first generation bioethanol prices through sugarcane and corn prices. Second generation cellulosic sugars are often considered an attractive alternative to first generation sugars because they are divorced from food production, require less intensive land use and carry a greatly reduced carbon footprint.36 However, it is noteworthy that the lack of fertilizer inputs, which is the main agent responsible for the difference in carbon footprint, also combines with the 10–20 years growth cycle to largely divorce cellulosic sugar prices from energy prices. As a result, while both energy prices and sugar prices are extremely volatile, the price of wood chips is remarkably stable. This can be seen in Fig. 3, which plots the fluctuations in prices of natural gas, sugar and wood chips over time. The ratio of the highest price to the lowest price is approximately 9 for natural gas, 7 for sugar and 1.7 for wood chips.49 This price stability should be an immense advantage for cellulosic sugars (and hence, cellulosic ethanol) in the marketplace, where 20–30 years contracts can be pre-purchased by customers.
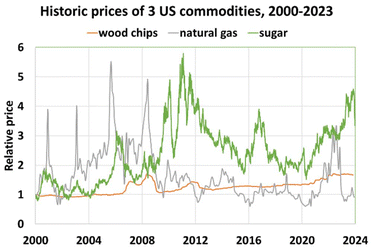 |
| Fig. 3 US trading prices of wood chips (orange), natural gas (grey) and sugar (green) from 2000–2023, relative to closing the price on 1 January 2000. All data from the US Federal Reserve Economic Data database.49 | |
As a further illustration of this, my group published a study in 2017 that included the estimated cost (via technoeconomic modelling) of ionoSolv-derived cellulosic glucose and compared it to the trading price of sugar at that point in time.14 In early 2016, sugar prices were relatively low, at $0.26 per kg. At that time, we estimated the cellulosic sugars produced by our process would cost $0.19 per kg, or 30% below the sugar trading price. However, the sugar price is immensely volatile compared with the price of lignocellulosic feedstocks, as noted in Fig. 3. Additionally, the ionoSolv lignocellulosic biorefinery cost is dominated by capital costs, which are more closely linked to raw inflation than energy prices. Fig. 4 demonstrates how the cost of ionoSolv cellulosic sugar has evolved since 2016, plotted against the sugar trading price at the same point in time. It is clear that the CAPEX- and wood-dominated ionoSolv costs suffer much less variance than the energy-dominated sugar price. Since the absolute values are prone to error in the estimates, I have also included a 50% increase in the CAPEX estimate for ionoSolv in Fig. 4. In this pessimistic scenario, an ionoSolv plant is sometimes a tremendous investment (2016–2017, 2020-present) and sometimes a poor one (sugar prices crashed in 2018–2019). Unfortunately, the former leads to great profits while the latter would quickly lead to bankruptcy, even if persisting for only a few months. This highlights that a great business idea is not always a great business idea right now. Additionally, the inconsistent nature of fossil-derived energy prices provides a burden of uncertainty but also a golden opportunity for green chemistry.
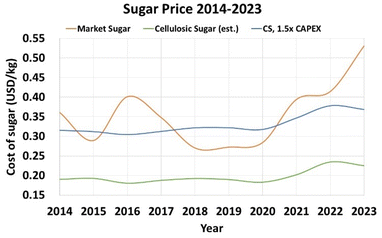 |
| Fig. 4 Comparison of sugar prices for glucose derived from corn stover and US trading price for sugar from 2014–2023. US sugar (brown) price data taken from the US Federal Reserve Economic Data database.49 Cellulosic sugar price adapted from the technoeconomic model for ionoSolv corn stover14 corrected for CEPCI cost factors and energy prices (green) and also with a 1.5× CAPEX increase to reflect uncertainty in new technology prices (blue). | |
Scale-up ahead of market demand has also proven detrimental to companies, notably in the bioplastics arena. Both BioAmber50 and TMO Renewables51 attempted to bring bio-based succinic acid (and plastics derived from succinate polymers) to market at scale. In both cases the large-scale production came ahead of sufficient market demand and the production plants proved too costly to sustain operation. This issue can sometimes be addressed when startups partner with a more established partner in their supply chain. A recent successful example of this came when Holiferm partnered with Sasol to bring a biotechnologically produced rhamnolipid surfactant to market as a detergent. The product is due for 15 ktpa commercial production,52 no doubt in part due to Sasol's experience in scaling up new chemical products.
Keep your motivation in mind
While there are a plethora of reasons why individuals may start a green chemistry cleantech company, there is always some environmental benefit at the heart of it. Nevertheless, it is a natural inclination of researchers (especially academic researchers) to fall back into their comfort zone. The temptation to commercialize one's research as a validation of its quality can be very strong, but the application needs to always be considered, and an objective view of whether the technology is appropriate for a particular market segment is crucial. In an inspiring perspective in Green Chemistry in 2011, Jessop53 analysed the proportion of research papers on the topic of green solvents by application area. He observed that the applications which green solvents researchers were focussing on (synthesis, biomass processing, see Fig. 5) did not match the application areas where solvents are most used or advances most needed to effect a change in industry. This is similar to an observation by Clark et al.54 on the mismatch between solvents research and industrial solvent usage. As they demonstrated in Fig. 6, industrial solvent usage is dominated by bulk, high volatility applications such as paints and coatings. When developing a novel green solvent, it is clear that targeting such applications would have the greatest positive impact on the environment but often researchers find more niche, less impactful applications to be more suitable. One possible exception is the clear utility of academics exploring emerging applications as these will need solvents but have not yet experienced market maturity. Taken together, these solvent analyses highlight the need to monitor motivation – if one desires the greening of the solvent industry, targeting applications where solvents are used will have greater and more immediate impact than those where they are not. I contrast two personal experiences here – during my PhD study, I spent several months developing a solvent system capable of catalysing the alkylation of isobutane to produce reformulated gasoline. Eventually, my supervisor pointed out that the reaction works perfectly well in the gas phase, which would make solvent adoption impractical. On the opposite end, I have taken great inspiration from a former student of mine who founded Oorja, a company which brings renewable energy to off-grid rural farming communities in India.55 The company uses solar PV mini-arrays, because they are inexpensive and easy to deploy, to replace diesel generators. This is a tremendous success story for green energy, as diesel electricity has a high carbon footprint, and a highly successful business opportunity, as diesel electricity is more expensive than solar PV. The student's PhD project was actually on scale-up of biorefining,56 and as she was considering the use of rice straw gasification for electricity co-generation, we performed a technoeconomic feasibility study which ultimately determined that a hybrid solar PV/biomass gasification arrangement was economically superior.57 The company did not deploy this however, not because the environmental or economic justification was lacking, but rather because the greatly increased complexity of operation outweighed the rather marginal benefits. It was therefore a quality academic study but an impractical business idea. In the end, the company chose impact over the more self-satisfying use of their founder's PhD research. The success of that particular startup came in part because the founder saw a problem and deployed the best technological solution (which had no IP involved) rather than attempting to force her own research into the marketplace.
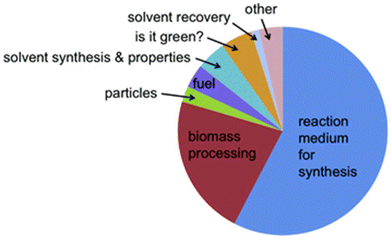 |
| Fig. 5 Distribution of publications on green solvents by application area, published in the journal Green Chemistry in 2010. Reproduced from ref. 53 with permission from the Royal Society of Chemistry, copyright 2011. | |
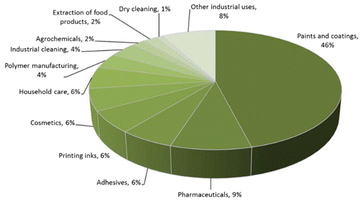 |
| Fig. 6 Proportion of solvent use segmented by industrial sector. Reproduced from ref. 54 with permission from MDPI, copyright 2015. | |
Conclusions
Startup companies can be a powerful vehicle for deriving impact from academic research through translation of ideas into commercial practice. Despite the recent growth in the number of academic startups, the translation path has not become straightforward and many decisions and activities unfamiliar to academic researchers are required during this journey.
It is vital to ensure that the translation activities are consistent with the aims and motivations of the founders. Key tools such as market research and business model development should take precedence over primary research or technology development during the early stages in order to ensure a viable commercial vehicle emerges. Misunderstanding market dynamics, investor outlook, timing and one's own motivation are essential to success. Reading the market is essential as some products can be profitable or unprofitable depending on external forces, such as energy prices. Temptations to scale-up a technology too early, to misunderstand the context of TRLs or too focus too much on technology rather than business are common mistakes and scaling ahead of a market pull can lead to an immediate failure. It should be recognised that in some instances a tremendous research paper is the best means of achieving impact for an idea.
Green chemistry has played a leading role within the cleantech startups space, as the aims of the field match perfectly with the goals of translation, achieving a positive change in industrial practice and thereby a positive impact on the environment. Increasing commercial and government attention on decarbonization and net-zero chemical production provide an ideal opportunity to bring new, sustainable chemical technologies to market. The tools of sustainable engineering and green chemistry, including technoeconomic analysis and life cycle assessment, can play a vital role in inspiring investor confidence and market acceptance. LCA is a clearly emerging necessity in establishing the carbon impact credentials of new products. It is no accident that these two metrics work closely together. To paraphrase Roger Sheldon,58 under the right circumstances cost is a reasonably good green metric. Lessons like these abound in the translation space and I can attest that even a seasoned academic can learn a great deal about their research from the commercialization journey, provided they keep their eyes open.
Data availability
The data supporting the findings of this study is available within the article.
Conflicts of interest
The author declares that he is a co-founder of several of the startup companies mentioned in the anecdotes, where noted.
Acknowledgements
I am grateful to all of his startup co-founders for the lessons included in this review, and especially Dr Agi Brandt-Talbot for helpful suggestions on what to include. I also wish to acknowledge the United Kingdom Department for Science, Innovation and Technology (DSIT) for funding his Royal Academy of Engineering Chair in Emerging Technologies, specifically so I would have time to write articles like this one.
References
-
P. T. Anastas and J. C. Warner, Green chemistry: Theory and practice, Oxford University Press, New York, 1998 Search PubMed.
-
https://www.iea.org/energy-system/industry/chemicals
.
-
https://www.epa.gov/trinationalanalysis/air-releases-chemical-industry
.
- K. Bailey, A. Basu and S. Sharma, Water, 2022, 14, 1073 CrossRef CAS.
- IEA: Chemicals 2023; https://www.iea.org/energy-system/industry/chemicals.
-
S. Blank and B. Dorf, The startup owner's manual: The step-by-step guide for building a great company, John Wiley & Sons, New Jersey, 2020 Search PubMed.
-
T. Eisenmann, Why Startups Fail: A New Roadmap for Entrepreneurial Success, Currency, New York, 2021 Search PubMed.
- S. V. Mohan and R. Katakojwala, Curr. Opin. Green Sustainable Chem., 2021, 28, 100434 CrossRef CAS.
- M. Mascal, ACS Sustainable Chem. Eng., 2019, 7, 5588–5601 CrossRef CAS.
-
https://www.innovationintextiles.com/origin-secures-15-billion-in-funding/
.
- E. de Jong, H. R. A. Visser, A. S. Dias, C. Harvey and G. J. M. Gruter, Polymers, 2022, 14, 943 CrossRef CAS PubMed.
- L. Sun, J. Wang, S. Mahmud, Y. Jiang, J. Zhu and X. Liu, Eur. Polym. J., 2019, 118, 642–650 CrossRef CAS.
-
https://avantium.com/basf-notifies-avantium-of-its-exit-from-synvina/
.
- A. Brandt-Talbot, F. J. V. Gschwend, P. S. Fennell, T. M. Lammens, B. Tan, J. Weale and J. P. Hallett, Green Chem., 2017, 19, 3078–3102 RSC.
- A. R. Abouelela, A. Al Ghatta, P. Verdía, M. Shan Koo, J. Lemus and J. P. Hallett, ACS Sustainable Chem. Eng., 2021, 9, 10524–10536 CrossRef CAS.
-
https://www.lixea.co
.
- NASA: https://www.nasa.gov/wp-content/uploads/2017/12/458490main_trl_definitions.pdf; EU: https://www.ec.europa.eu/research/participants/data/ref/h2020/wp/2014_2015/annexes/h2020-wp1415-annex-g-trl_en.pdf.
- C. Frank, C. Sink, L. Mynatt, R. Rogers and A. Rappazzo, J. Technol. Transfer, 1996, 21, 61–69 CrossRef.
- A. Al Ghatta, R. C. Aravenas, Y. Wu, J. M. Perry, J. Lemus and J. P. Hallett, ACS Sustainable Chem. Eng., 2022, 10, 8846–8855 CrossRef CAS PubMed.
-
https://ourworldindata.org/covid-vaccinations
.
- E. S. Bjornali and A. Ellingsen, Energy Procedia, 2014, 58, 43–50 CrossRef.
- P. Hegeman and R. Sorheim, J. Cleaner Prod., 2021, 294, 126315 CrossRef.
- P. G. C. Nayanathara Thathsarani Pilapitiya and A. Sandaruwan Ratnayake, Clean. Mater., 2024, 11, 100220 CrossRef.
- F. J. V. Gschwend, L. M. Hennequin, A. Brandt-Talbot, F. Bedoya-Lora, G. H. Kelsall, K. Polizzi, P. S. Fennell and J. P. Hallett, Green Chem., 2020, 22, 5032–5041 RSC.
- J. Hallett, TCE – The Chem. Eng., 2023, 981, 27–29 Search PubMed.
-
https://www.kthinnovationreadinesslevel.com
.
- IEA 2021; Ten years of clean energy start-ups, https://www.iea.org/articles/ten-years-of-clean-energy-start-ups.
-
T. Brennan, W. Chyan, M. Göbel, P. Klevnäs, T. Melgin, C. Pakari, M. Pley, A. Spamann and C. Witte, https://www.mckinsey.com/industries/chemicals/our-insights/sustainable-feedstocks-accelerating-recarbonization-in-chemicals#/.
- Trivium Packaging, https://www.triviumpackaging.com/media/pe5hfxsp/2023buyinggreenreport.pdf.
- A. Croce and R. Bianchini, Rev. Corp. Fin., 2022, 2, 587–616 Search PubMed.
- M. Van den Heuvel and D. Popp, Energy Econ., 2023, 124, 106877 CrossRef.
- Forbes Magazine, 2015, https://www.forbes.com/sites/neilpatel/2015/01/16/90-of-startups-will-fail-heres-what-you-need-to-know-about-the-10/?sh=1b8450856679.
- C. Zibunas, R. Meys, A. Kätelhön and A. Bardow, Comput. Chem. Eng., 2024, 187, 108721 CrossRef CAS.
- ISO 14068-1:2023, Climate Change Management—Transition to Net Zero—Part 1: Carbon Neutrality, Vernier, Geneva, 2023.
-
W.-P. Schmidt, Solutions For Sustainability Challenges: Technical Sustainability Management and Life Cycle Thinking, Springer Nature Switzerland, 2024, pp. 97–113 Search PubMed.
- A. J. Ragauskas, C. K. Williams, B. H. Davison, G. Britovsek, J. Cairney, C. A. Eckert, W. J. Frederick, J. P. Hallett, D. J. Leak, C. L. Liotta, J. R. Mielenz, R. Murphy, R. Templer and T. Tschaplinski, Science, 2006, 311, 484–489 CrossRef CAS PubMed.
- C. Bettenhausen, Chem. Eng. News, 2023, 101(41) Search PubMed , https://cen.acs.org/business/biobased-chemicals/Clariant-latest-firm-pull-cellulosic/101/web/2023/12.
-
S. M. T. Rahman, Exploring feedstock supply chain for large scale manufacturing of recycled cellulosic textile fiber: A collaborative business model analysis, Uppsala University, 2022 Search PubMed.
-
B. Roberts-Islam, https://www.forbes.com/sites/brookerobertsislam/2024/02/27/what-we-can-learn-from-renewcells-financial-struggles/.
- P. Vlaskovits, Harv. Bus. Rev., 2011, 29, 2011 Search PubMed.
-
A. Osterwalder and Y. Pigneur, Business model generation: A handbook for visionaries, game changers, and challengers, John Wiley & Sons, New Jersey, 2010 Search PubMed.
- J. Wunderlich, K. Armstrong, G. A. Buchner, P. Styring and R. Schomäcker, J. Cleaner Prod., 2021, 287, 125021 CrossRef CAS.
- E. J. Abakah, A. K. Tiwari, J. A. Oliyide and K. O. Appiah, Sustainability, 2023, 15(20), 14897 CrossRef.
-
C. Patinha Caldeira, R. Farcal, C. Moretti, L. Mancini, H. Rauscher, K. Rasmussen, J. Riego Sintes and S. Sala, Safe and Sustainable by Design chemicals and materials Review of safety and sustainability dimensions, aspects, methods, indicators, and tools, EUR 30991 EN, Publications Office of the European Union, Luxembourg, 2022, JRC127109. ISBN 978-92-76-47560-6, DOI:10.2760/879069.
- European Commission, 2006. Regulation (EC) No 1907/2006 of the European Parliament and of the Council of 18 December 2006 concerning the Registration, Evaluation, Authorisation and Restriction of Chemicals (REACH), establishing a European Chemicals Agency, amending Directive 1999/45/EC and repealing Council Regulation (EEC) No 793/93 and Commission Regulation (EC) No 1488/94 as well as Council Directive 76/769/EEC and Commission Directives 91/155/EEC, 93/67/EEC, 93/105/EC and 2000/21/EC. Official Journal of the European Union, 396, 1–849.
- E. Berggren and A. P. Worth, Regul. Toxicol. Pharmacol., 2023, 142, 105431 CrossRef CAS PubMed.
- J. Kleinekorte, J. Kleppich, L. Fleitmann, V. Beckert, L. Blodau and A. Bardow, ACS Sustainable Chem. Eng., 2023, 11, 9303–9319 CrossRef CAS.
- F. Meng, A. Wagner, A. B. Kremer, D. Kanazawa, J. J. Leung, P. Goult, M. Guan, S. Herrmann, E. Speelman, P. Sauter and S. Lingeswaran, Proc. Natl. Acad. Sci. U. S. A., 2023, 120, e2218294120 CrossRef CAS.
-
https://fred.stlouisfed.org/
.
-
https://bioplasticsnews.com/2018/05/07/bioamber-is-bankrupt/
.
-
A. Chudasama, https://www.spglobal.com/commodityinsights/en/ci/research-analysis/the-faltering-bioeconomy.html.
- C. Bettenhausen, Chem. Eng. News, 2024, 102(3) Search PubMed , https://cen.acs.org/magazine/102/10203.html.
- P. G. Jessop, Green Chem., 2011, 13, 1391–1398 RSC.
- J. H. Clark, T. J. Farmer, A. J. Hunt and J. Sherwood, Int. J. Mol. Sci., 2015, 16, 17101–17159 CrossRef CAS PubMed.
-
https://www.oorjasolutions.org
.
- C. L. Chambon, P. Verdía, P. S. Fennell and J. P. Hallett, Sci. Rep., 2021, 11, 15383 CrossRef CAS PubMed.
- C. L. Chambon, T. Karia, P. Sandwell and J. P. Hallett, Renewable Energy, 2020, 154, 432–444 CrossRef.
- R. A. Sheldon, ACS Sustainable Chem. Eng., 2018, 6, 32–48 CrossRef CAS.
|
This journal is © The Royal Society of Chemistry 2025 |
Click here to see how this site uses Cookies. View our privacy policy here.